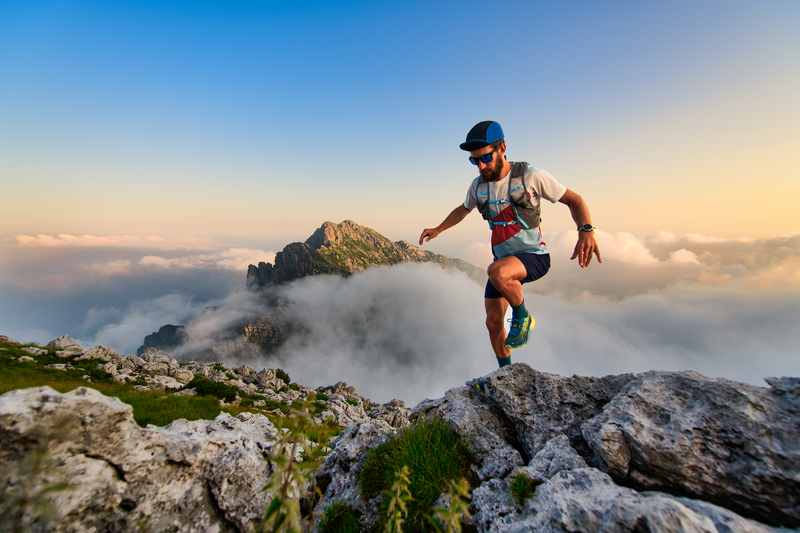
95% of researchers rate our articles as excellent or good
Learn more about the work of our research integrity team to safeguard the quality of each article we publish.
Find out more
ORIGINAL RESEARCH article
Front. Behav. Neurosci. , 18 September 2024
Sec. Individual and Social Behaviors
Volume 18 - 2024 | https://doi.org/10.3389/fnbeh.2024.1450540
This article is part of the Research Topic Home Cage-based Phenotyping in Rodents: Innovation, Standardization, Reproducibility and Translational Improvement – Volume II View all 8 articles
Aggression is an adaptive social behavior crucial for the stability and prosperity of social groups. When uncontrolled, aggression leads to pathological violence that disrupts group structure and individual wellbeing. The comorbidity of uncontrolled aggression across different psychopathologies makes it a potential endophenotype of mental disorders with the same neurobiological substrates. Serotonin plays a critical role in regulating impulsive and aggressive behaviors. Mice lacking in brain serotonin, due to the ablation of tryptophan hydroxylase 2 (TPH2), the rate-limiting enzyme in serotonin synthesis, could serve as a potential model for studying pathological aggression. Home cage monitoring allows for the continuous observation and quantification of social and non-social behaviors in group-housed, freely-moving mice. Using an ethological approach, we investigated the impact of central serotonin ablation on the everyday expression of social and non-social behaviors and their correlations in undisturbed, group-living Tph2-deficient and wildtype mice. By training a machine learning algorithm on behavioral time series, “allogrooming”, “struggling at feeder”, and “eating” emerged as key behaviors dissociating one genotype from the other. Although Tph2-deficient mice exhibited characteristics of pathological aggression and reduced communication compared to wildtype animals, they still demonstrated affiliative huddle behaviors to normal levels. Altogether, such a distinct and dynamic phenotype of Tph2-deficient mice influenced the group's structure and the subsequent development of its hierarchical organization. These aspects were analyzed using social network analysis and the Glicko rating methods. This study demonstrates the importance of the ethological approach for understanding the global impact of pathological aggression on various aspects of life, both at the individual and group levels. Home cage monitoring allows the observation of the natural behaviors of mice in a semi-natural habitat, providing an accurate representation of real-world phenomena and pathological mechanisms. The results of this study provide insights into the neurobiological substrate of pathological aggression and its potential role in complex brain disorders.
Aggression is an adaptive behavior, which is often the result of competition (Nelson and Trainor, 2007). It is an important social behavior that ensures the stability and prosperity of a social group (Van Loo et al., 2003; Sapolsky, 2005; Wang et al., 2011). When it cannot be avoided, aggression is typically short and directed to acquire and keep resources including territory, mating partners, and food (Kiser et al., 2012). On the pathological side, escalated aggression or violence, excessively and repeatedly hurting others or the perpetrator itself, happens in all contexts and is devoid of a communication purpose (Natarajan et al., 2009). This extreme behavior is detrimental to the individual as it can result in death or invalidity, and it severely disrupts the group's structure, security, and comfort (WHO, 2004). Interpersonal violence has a high economic cost and a better understanding of how aggression impacts group structure is critical for “diagnosis, prevention, and treatment, but also for guidance of public and judicial policies” (WHO, 2004; Miczek et al., 2007).
Uncontrolled aggression and violence are diagnostic criteria for different psychiatric disorders, such as schizophrenia, alcoholism, intermittent explosive disorder, autism, or dementia (Lesch et al., 2012; Volavka, 1999). Considering a dimensional and trans-diagnostic view of mental disorders, the comorbidity of uncontrolled aggression across different psychopathologies makes it a good potential endophenotype of mental disorders (Niederkofler et al., 2016; Nestler and Hyman, 2010) with the neurobiological (and heritable) substrates of pathological aggression being the same across different psychiatric disorders (American Psychiatric Association, Committee on Nomenclature and Statistics, 2018; Gottesman and Gould, 2003; Gould and Gottesman, 2006; Robbins et al., 2012). Research into neurobiological substrates underlying aggression is thus essential to our insight into the etiology of complex brain disorders (Kalueff et al., 2015) and its potential global impact on society.
Serotonin (5-hydroxytryptamine, 5-HT) is a monoamine that plays a critical role in the regulation of impulsive and aggressive behaviors in humans and animals. Mice with a congenital lack of serotonin in the brain due to the lack of a rate-limiting serotonin-producing enzyme Tryptophan hydroxylase 2, TPH2 (Tph2-deficient mice, Alenina et al., 2009), show higher levels of aggression toward strangers, poor social recognition abilities, and an impulsive-like phenotype (Angoa-Pérez et al., 2012). Tph2-deficient mice also present behavioral abnormalities similar to human symptoms of autism spectrum disorder (ASD) and impulsive related disorders (Mosienko et al., 2012, 2015a,b; Kane et al., 2012; Beis et al., 2015; Angoa-Pérez et al., 2012; Kästner et al., 2019). In line with the search for trans-nosological symptoms of mental and neurological disorders, Tph2-deficient mice represent a potentially valuable model for studying pathological aggression.
The visible burrow system (VBS) is a semi-natural habitat first developed in rats (Blanchard and Blanchard, 1989) and more recently in mice (Arakawa et al., 2007; Pobbe et al., 2012; Bove et al., 2018), where ethological aspects (e.g., day/night fluctuation of activity spatial distribution, place preference) and social and non-social behaviors of group-housed, freely-moving individuals can be continuously observed and quantified (Alonso et al., 2020, 2023).
In an effort to model real-world phenomena and pathological mechanisms reminiscent of the everyday life of human patients in mice (McCloskey et al., 2011), we chose to apprehend the individuals' behaviors and the group dynamic of Tph2-deficient (Tph2−/−) and wildtype (Tph2+/+) mice directly in their housing environment. To this end, a new version of the VBS was designed and built. This study aimed to investigate the impact of central serotonin loss on the everyday expression of social and non-social behaviors and their correlations over days in undisturbed, group-living mice of the same-genotype. Training a machine learning algorithm (Random Forest classifier, Breiman, 2001) on this extended behavioral data allowed us to identify key variables dissociating one genotype from the other when living in such an ethologically-relevant environment. Because excessive aggression does not only drastically affect the life of the perpetrator but simultaneously affects the dynamics of the group and its structural organization, we evaluated if and how a lack of central serotonin influenced the group's structure and development of its hierarchical organization using social network analysis (SNA, Krause et al., 2010) and the Glicko rating methods (Glickman, 1999), respectively.
Mice were maintained at the Max Delbrück Center (MDC) animal facility in individually ventilated cages (Tecniplast, Italy) under specific pathogen-free, standardized conditions in accordance with the German Animal Protection Law. Mice were group-housed at a constant temperature of 21 ± 2°C with a humidity of 65 ± 5%, under 12/12 h light/dark cycle (light off at 18:00), and had ad libitum access to food and water throughout the project. In total, 10 groups of four Tph2-deficient (Tph2−/−) or wildtype (Tph2+/+) male mice (ntotal = 40) were used in this project: five groups of Tph2+/+ and five groups of Tph2−/− mice on C57BL/6N genetic background (Alenina et al., 2009; Mosienko et al., 2012). An independent cohort of C57BL/6N male mice (n = 6) was used as unfamiliar mice in the three-chamber test.
Tph2−/− and Tph2+/+ mice were born from heterozygous parents and genotyped at weaning as previously described (Alenina et al., 2009). After weaning, four males of the same genotype from different litters were group housed in a regular home cage. At 6 weeks of age, four mice of the same genotype were transferred to the same cage and were individually marked with unique radio frequency identification tags (RFID: 12 × 2.1 mm, 124 kHz, Sokymat, Germany, subcutaneous implantation in the scruff of the neck and under short isoflurane anesthesia). Afterward, the animals' activity and health were regularly monitored (1 and 3 h after marking and every day on the following days). At 7 weeks of age, the four mice from the same home cage were transferred for six consecutive days (120 h in total) to a new version of the VBS, which was designed and built for this project. The three-chamber test was scheduled 1–2 days after the mice left the semi-automated VBS, which did not allow sufficient time to change to an inverted light cycle, as adapting to an inverted light cycle usually requires 2 weeks. Thus, conducting the test during the light phase ensured consistency with the standard operating conditions of the animal facility, maintaining the wellbeing and normal circadian rhythms of the animals while reducing stress associated with changing the light cycle. The three-chamber test was performed during the light phase.
All procedures followed the national regulations in accordance with the European Union Directive 2010/63/EU. The protocols were approved by the responsible governmental authorities [Landesamt für Gesundheit und Soziales (LaGeSo), Berlin, Germany]. The experimental procedures were designed to allow for maximal animal welfare. Animals lived undisturbed as a group within their home cage. Briefly, data collection was performed using automated observational methods applied to undisturbed group-housed animals. The health of the animals was monitored daily. Due to the observational nature of the study, the experimental procedure did not cause any damage, pain, or suffering to the animals.
The semi-automated VBS was designed and built following the description of the VBS used in Arakawa et al. (2007), as shown in Figure 1. It consisted of a large regular home-cage (P2000, Tecniplast, Italy) into which the different compartments were integrated. The cage was separated into two (open and burrow) areas by a dark wall (PVC, 35 × 9 × 0.7 cm). The open area was a square (39 × 40 x 72 cm) delimited by the dark PVC wall and three transparent walls of the cage (22 cm high) on top of which extra white walls (particle wood board with smooth white finish, 50 cm high) were added. The extra white walls kept the area bright, prevented escapes, and blocked most of the outside view of the cage. On top of the open area, a transparent Plexiglas lid was set, which kept the illusion of the openness of the area. The lid was tilted to avoid light reflections on the videos and had ventilation holes on the side (Plexiglas 44 × 38 × 14.5 cm). In the open area, regular food chow and water were available at two apertures (12 × 4 cm for food) on opposite sides (Figures 1A, B). The bottom of the open area was covered with bedding (0.5 cm thickness). The other area, called the “burrow area” consisted of two separated dark chambers (PVC, 8 × 13 × 6.5 cm), connected to the open surface by transparent tunnels (Plexiglas). Chamber 1 had one straight tunnel (4 × 5 × 3 cm) connected to the open area while chamber 2 had two tunnels (straight: 4 × 5 × 3 cm and L shaped: 8 + 13 cm long × 5 × 3 cm) leading to the open area. A black plate covered the entire burrow area (burrows and tunnels; infrared (IR) transparent acrylic glass, 18 × 38 × 0.8 cm) and the three transparent walls of this side of the large home cage were taped with black vinyl film so that the chambers and the tunnels were in near to complete darkness (Figure 1). A grid of 24 RFID transponder readers (ID grid; Phenosys, Germany) placed under the VBS cage provided automated, continuous, and simultaneous spatio-temporal information on each RFID tagged-animal present above. An infrared black and white video camera and two infrared lights were placed above the VBS cage. In the videos, all animals were visible from all places in the VBS and in both light and dark phases. The ID grid and the video camera were connected to the same computer, and data were saved on an external hard drive for later manual analysis of the animal's behaviors. The VBS cage could be easily disassembled/reassembled to clean the parts in contact with animals.
Figure 1. Illustrations of the semi-automated VBS. (A) A large rat cage was placed on the top of a grid of 24 RFID readers and its walls were topped by extra high white walls. A camera was placed on the top of the cage and aligned with the edge of the vertical partition (IR transparent, dark) of the burrow/open areas (drawn on SketchUp.com). (B) A schematic top view of the VBS cage with B1: one-tunnel burrow, B2: two-tunnel burrows, w: external water bottle with spout inside the cage, *food access zone with outside food chow reservoir. (C) Still frame of a synchronized video with RFID tag detection for each mouse (superimposed blue, white, yellow, and pink triangle symbols).
Each mouse was weighed before entering and after leaving the VBS cage. Each group of four mice spent five experimental days (five dark and five light phases) spanning over six calendar days in the VBS cage. They entered the VBS at the onset (or a maximum of 30 min before) of the dark phase on experimental day 1 (at 18:00) and were removed from the system after the end of the dark phase of experimental day 5 (during the light phase after 13:00). In the VBS, the mice were left undisturbed (e.g., no bedding change) and water and food were available ad libitum. The wellbeing of the animals was checked daily by inspection of the animals' fur, posture, and locomotion through the clear walls of the cage and by evaluation, on video, of their level of activity during the previous dark phase. After the VBS, mice were placed back together into the same (empty) regular home cage.
An RFID event was automatically recorded each time the RFID transponder of a given animal was detected by an RFID reader. For each event, the control program (PhenoSoft Control program, PhenoSys GmbH) specified the date and time, the duration of the event, the identity of the detected mouse, and the activated RFID reader. Events were continuously collected and saved for the entire duration of the experiment. A total of 30 s long videos were recorded every 10 min during the five experimental days (CamUniversal software, Crazypixel). On each video, colored dots (one color per animal) were superimposed to the images of each mouse to allow visual identification of each individual of the group (Figure 1C; Kolonikaefig software, PhenoSys). Marking the animals on the videos instead of color-marking their fur or ears was a more accurate, less invasive, and also simpler method for the long-term identification of individuals within a group (Lewejohann et al., 2010; Arakawa et al., 2007).
Similar to previous studies (Pobbe et al., 2012), only the videos of the first 4 h of each phase (dark and light) of all experimental days were analyzed (25 videos per phase, two phases per experimental day, and five experimental days = 250 videos were analyzed per group). Each time a mouse expressed one of the behaviors listed in Table 1, (1) the type, (2) its duration, (3) where it took place in the cage, and (4) the identity of any other mouse the focal mouse was interacting with during this behavior (e.g., the mouse “m1” is “chasing” for “5 s” in the “open area” the mouse “m4”) was reported in a behavioral ethogram. One focal animal was observed at a time, and all four animals were observed per video. The videos were scored by two observers (MR and AH) trained to specifically and similarly recognize the behaviors described in Table 1. The same observer scored all videos of a given group of mice. During video-scoring, the observer was blind to the genotype of the group. Consistency between observers was evaluated as follows: one observer would randomly select 10–20 videos of a group she had not yet annotated, score these videos, and compare her results with the other observers' results. Before all the other videos were scored, if the results differed, the two observers discussed discrepancies and adjusted their scorings' strategies accordingly. This was repeated until scorings were similar between observers.
An experimental day consisted of 12h of dark and 12 h of light phases starting at the onset of the dark phase. An experimental day spans over 2 calendar days with the dark phase lasting from 18:00 of the first day to 05:59 of the next day.
The distance traveled per hour for each mouse was calculated from the event-based data generated by the ID-grid software (Phenosoft and Phenosoft Analytics, PhenoSys GmbH, Berlin). Distance traveled per hour is an indicator of the animal's spontaneous activity over time. Due to a technical problem, data from four Tph2+/+ animals are missing from the dark phase on experimental day 4.
Place preference in the VBS was calculated using the data generated by the ID-grid software. The relative frequency (%) of activation of each RFID-reader of the 24-RFID-reader-grid located under the floor of the VBS indicated the relative preference of the animals (averaged per phase and per genotype over all experimental days) for each of the 24 zones of the VBS. In the open area of the VBS, four zones can be distinguished: a zone with access to the feeder, a zone with access to the water spout, a “safer” zone close to the separating wall and entries to the burrow area, and a more “risky”, central zone (Figures 1, 3). In the burrow area, half of the readers were located under one burrow and its two tunnels and the other half were located under the other burrow and its one tunnel (Figure 3). A 24-tiles heatmap represents the spatial disposition of the 24-RFID reader. The darker the color of a tile, the more the corresponding RFID reader was activated [relative to the activation of all the other readers of the grid (%)] by the presence of animals above it and thus the greater the animals (on average) preferred this location in the VBS.
For each behavior described in Table 1, the total number of occurrences per animal, per genotype, and per phase were analyzed (Figure 4). Potential body mass changes during the VBS housing were calculated as the difference of weight before/after VBS. Per genotype, relationships between social and non-social behaviors were evaluated using a correlation table (Supplementary Tables 1, 2).
Machine learning was used to identify which variables, from all of the variables extracted during VBS housing (behaviors and activity, Figure 4) were key to differentiating the Tph2−/− from the Tph2+/+ mice. To this end, we trained a Random Forest (RF) classifier (Breiman, 2001) using the R package “randomForest” (Liaw and Wiener, 2002) and extracted the implemented feature importance (Gini index) for further analysis. We evaluated classification performance using a simple accuracy metric based on leave-one-out cross validation to ensure feasible features with regard to the differentiability of genotypes. The input variables for the RF classifier were the total number of occurrences of each behavior (illustrated in Figure 4) during the dark or light phase separately, and the total distance traveled during VBS housing, for each animal of each genotype.
The estimated labels (i.e., genotype) of the test dataset were compared to the true labels of the animals, and the overall accuracy of each classification, i.e., the ratio of correctly classified animals, was computed. The Gini index was automatically assigned to each variable for its contribution to differentiating the genotypes in each classification step. For robust results of the Gini index and RF, we ran this procedure 100 times and reported the average (±SD) classification accuracy and Gini index. We considered behaviors with a Gini index of at least 1 as the main contributors to differentiate between the genotypes.
For each Tph2−/− and Tph2+/+ social network, a node represents an animal (four nodes per network), a line between two nodes (a dyad) indicates the occurrence of at least one interaction between them, and the thickness of the line (the total number of interactions between a dyad) represents the strength (weight) of this relationship (weighted directed network). The higher the number of interactions, the thicker the edge between the respective animals. For each selected behavior, the development of its social network was evaluated during the dark phase of each day as more occurrences of behaviors happened during this phase. With such day-by-day network representation, we can visually illustrate the dynamics and quality of interactions between pairs of individuals within their social network. The social network analysis of this study focused on the daily dynamics of overall interaction strength of both “struggle at feeder” and “allogrooming” networks in the VBS. This parameter (the overall interaction strength) reflects the role of a single animal or its “relationship strength” within a network. The overall interaction strength was assessed as a node's (a single animal) total number of interactions within the directed network (in and out). We focused the analysis on overall interaction strength instead of incorporating the directed versions of in-strength and out-strength due to the high similarity between those parameters in the observed data. For the choice of these variables and of this parameter, see the Supplementary material.
Individuals' social rankings established by the Glicko rating system (Glickman, 1999) have been found to highly correlate with other methods for dominance ranking [i.e., David's scores and Inconsistencies and Strength of Inconsistencies (I&SI) ranking for instance in So et al., 2015]. The clear advantage of the Glicko rating metric is to report on the dynamic changes in individual dominance ratings for each of the dyadic interactions within a group (So et al., 2015; Williamson et al., 2016). Briefly, the Glicko analysis calculates individual ratings based on the evaluation of the direction of the attack of each agonistic interaction between two animals. If an animal initiated a directed “struggle at feeder” behavior, its rating increased while the rating for the losing animal decreased, and all other ratings were updated accordingly to these changes in rating. The Glicko rating model (PlayerRating R-Package) is an extension of the Elo dynamic paired comparison model (Neumann et al., 2011) that did not only iteratively compute the animals' rank but also the standard deviation of its ranking history to get an estimation of the rating certainty, which is further used to update an animal's ranking. Additionally, this model updated an animal's ranking when dyadic interactions occurred between remaining animals, recognizing the group as a network being more than a sum of separated pairs of individuals. Following Williamson et al. (2016) we set the initial ranking and certainty values equal for all animals. Differing from Williamson et al. (2016) we set the ranking and certainty equal to zero to enable negative ratings to improve the visualization of the development of social hierarchy. We further set the ranking update constant equal to 1, which creates little impact on the final results and still represents an accepted value for mouse agonistic interactions (So et al., 2015). The Glicko rating and power distribution analysis were performed using the data “struggle at feeder” after the social network behavior analysis of this study.
We used the Glicko rating system to appraise (1) if similar group stratification or hierarchy was observed in the Tph2−/− groups as in Tph2+/+ groups with the final ranking of individuals spreading above and below the initial rank mark and the most dominant animals being defined as having the highest overall ranks, (2) if one distinct dominant animal could be identified at the end of the test, (3) how individual hierarchical ratings dynamically developed over time, and (4) how rapidly, in terms of the number of scored interactions, the finally dominant animal continuously received the highest rank until the end of VBS housing. Finally, we evaluated how inequitable the distribution of power could be within same-genotype groups of mice. Here, the power of the dominant male was evaluated as a ratio (relative proportion) of power, defined as the difference in Glicko rating the dominant male is imposing on the first subordinate male (the second highest Glicko rating score) compared to the power projected from the dominant male to the most subordinate animal (the lowest Glicko rating score). A high value represents a more strongly despotic dominant male imposing relatively similar amounts of power toward all other animals.
These analyses were performed on the results from the video scoring. These data were not continuously available due to intermittent video recording and scoring. This may have introduced minor inaccuracies in the history of dyadic interactions, however, such effect may have been partially mitigated by the inclusion of rank certainty in the Glicko rating algorithm.
For each individual, we used from the behavioral ethogram the three following quantitative parameters: (1) the latency to the first attack (filtering for “struggling” or “struggling at feeder” or “chasing”), (2) the frequency, and (3) the mean duration of attacks (Miczek et al., 2003; Takahashi et al., 2010). A short latency to attack associated with increased frequency and duration of attacks would suggest escalated aggression in mice (Table 2). Other qualitative aspects of abnormal aggression were more difficult to extract from our data. The body location of bites (especially vulnerable body parts) could not be assessed in our study as very few instances of biting were observed or skin wounds were found. The lack of ritualistic behaviors (Haller et al., 2005) could only be indirectly and tentatively measured as the (4) ratio of fight/threat behaviors [Fight: struggle + struggle at feeder and Threats: chasing + following + approach to back (atb)]. The theory is that at a lower fight/threat ratio, the more the threats stop escalated aggression. Any lack of responses to appeasing signals could not be evaluated from the angle (top view) of our videos. The conditions in the VBS did not allow us to evaluate if attacks were context independent, such as “aimed at the opponent regardless of its sex or state (free-living/anesthetized/dead) or the environment (home/neutral cage)” (Natarajan and Caramaschi, 2010). All the same, we could spatially locate where aggressions happened the most and if these places were appropriate places for such behavior (Haller et al., 2005; Table 2).
Table 2. Pathological aggression in mice based on Takahashi et al. (2010) and Haller et al. (2005).
The apparatus consisted of a rectangular white box (60 × 40 × 22 cm) divided into three equal-sized chambers (20 × 40 × 22 cm). Dividing walls were made from clear Plexiglas, with rectangular openings (9 × 0.8 × 12 cm) allowing free access to each chamber. Two clear Plexiglas doors were used to block the openings when needed. Two round metal-wire grid cages with gray PVC covers at the bottom and top (Ø10 × 21 cm) were used. In each metal-wire cage could be placed one unfamiliar (stranger) mouse. Once the metal-wire cage was placed in the center of a lateral chamber, the opening in the wire mesh allowed the subject and the stranger mice to see, hear, smell, and touch each other but prevented fighting. A video camera above the apparatus recorded the position and behaviors shown by the subject mouse at any time and in the entire apparatus. All videos were saved on a computer for later analysis.
The subject mouse was weighed before entering the three-chamber apparatus. The stranger mice were kept in a separate experimental room and only transferred to the testing room when needed. The stranger mice had been previously habituated to the metal-wire cage (10 min daily, for at least three consecutive days before the testing day). The three-chamber test consisted of three phases (habituation, social preference, and social recognition). In the habituation phase, the test subject was first placed in the middle of the chamber and allowed to explore this chamber for 5 min while access to the lateral chambers was blocked by transparent doors. Then, the lateral doors were removed, and the mouse could explore the entire apparatus for 10 more min. At the end of this habituation phase, the mouse was gently pushed back into the center of the apparatus, and accesses to the lateral chambers were blocked. In the social preference phase, an empty metal-wire cage was placed in one lateral chamber, and a metal-wire cage with an unfamiliar mouse that had no prior contact with the subject mouse (stranger 1) in the other lateral chamber. The location of the unfamiliar mouse in the left vs. right side chamber was systematically alternated between test animals. After the lateral doors were removed, the subject mouse could explore the entire apparatus for 10 min (the social preference test). The subject mouse was then gently guided back into the center of the apparatus, accesses to the lateral chambers were blocked, and the two metal-wire cages were removed from the apparatus. After an inter-test interval of 5 min, the lateral doors were opened, and the subject mouse was allowed to explore the entire apparatus for 10 more min (Social Recognition test). During the social recognition test, an unfamiliar mouse (stranger 2) was placed in the previously empty metal-wired grid. The cage with stranger 1 was placed back into the same lateral chamber as before. The mouse had a choice between the first, already-investigated mouse (now-familiar mouse), and the novel unfamiliar mouse. At the end of the social recognition test, the subject mouse and the metal-wired grids were removed. The three chambers and doors were cleaned with ethanol (70%), and the metal-wired grids (emptied from the stranger mice) were wiped, cleaned with water, and dried.
Videos of the tests were recorded and saved for offline analysis by the video-tracking system Viewer 3 (Viewer, Biobserve). In the three-chamber test, both Social Preference and Social Recognition were measured as the total time spent in each chamber (%) and in close proximity with the grid-cage per 5 min bins.
We performed two types of statistical analyses. We analyzed the continuously-collected-RFID-data with regards to the influence of experimental (genotype) and random (animals, batch) factors using Markov chain Monte Carlo simulations of general linear mixed models (MCMCglmm R-package, Hadfield, 2010) and checked for a significant difference of the posterior distribution of the simulations with zero to assess the influence of the random variables. Other statistical tests were genotype based comparisons on a variety of experimental (distance traveled overall, per phase, per day; place preference; total behavior occurrences; parameters of pathological aggression and weight) and analytical (social network parameters) variables. To this end, we computed the exact Wilcoxon-Mann-Whitney test with multiple comparison corrections. The code base is written in the R and Python programming languages and will be made available via GitHub for the published version of the article.
Animals of both genotypes showed a similar pattern of activity across days. Their activity level drastically rose and fell at the onset of each dark (high-activity) and light (low-activity) phase across the five experimental days, respectively (Figure 2A). Although the activity level was higher during dark phases than during light phases, the animals were also more active during the first and the last 4 h of each dark phase with a 2-fold decrease of activity during the 4 h in between. Their activity levels were low and constant throughout light phases (Figure 2A). Despite this similar pattern of activity, Tph2−/− mice covered longer averaged distances over days than Tph2+/+ animals [Figure 2A, MCMCglmm random factors as animal and group: pMCMC = 0.011, post.mean = 9.865, (l-95% CI = 2.375, u-95% CI= 16.857)] during both dark and light phases (Figure 2B, Exact Wilcoxon-Mann-Whitney test: all dark phases, Z = −2.4616, p-value = 0.01319; all light phases Z = −2.5157, p-value = 0.01121). More specifically, the Tph2−/− mice were found consistently more active in all, except the first and last, dark phases in the VBS (Figure 2C., Exact Wilcoxon-Mann-Whitney test: day1, Z = −0.9197, p-value = 0.3689; day2, Z = −2.2722, p-value = 0.02272; day3, Z = −3.0296, p-value = 0.00196; day4, Z = −2.164, p-value = 0.03041; and day5, Z = −1.5148, p-value = 0.1344). Tph2−/− mice were also more active than Tph2+/+ mice during the first and third light phase (Figure 2C, Exact Wilcoxon-Mann-Whitney test: day1, Z = −3.5165, p-value = 0.00026; day2, Z = −0.5951, p-value = 0.5648; day3, Z = −2.0558, p-value = 0.04018, and day4, Z = −1.8935, p-value = 0.05956). Surprisingly, after entering the VBS for the first time, Tph2−/− mice appeared unusually less active than Tph2+/+ mice (Figure 2D, Exact Wilcoxon-Mann-Whitney test: first 4 h of the dark phase, Z = 1.4337, p-value = 0.1572), which was not the case during the later peak of activity of the same dark phase (Figure 2D, Exact Wilcoxon-Mann-Whitney test: last 4 h of the dark phase, Z = −2.2993, p-value = 0.02108) and on the following days in the VBS.
Figure 2. Distance traveled in the automated VBS. (A) Mean distance traveled per hour (+SD) across 5 days by Tph2+/+ (solid symbols) and Tph2−/− (open symbols) mice. Each experimental day starts (dashed line) at the onset of the dark phase (18:00–05:59; gray box) and finishes with the end of the following light phase (06:00–17:59; white box). (B, C) The boxplot of mean distance traveled per hour during light and dark phases, averaged over 5 days (B) or for each of the five consecutive days (C). (D) The mean distance traveled per hour during the first and last 4 h of the dark phase in experimental day 1. Boxplots show median, quartiles, 5th/95th percentiles, and outlying points ({ggplot2}, R) for Tph2+/+ (gray bars) and Tph2−/− (open bars). Exact Wilcoxon-Mann-Whitney test, *p < 0.05 between the genotypes. m, meter.
The heat maps in Figure 3 revealed distinct diurnal and nocturnal spatial preferences for different zones within the VBS and between genotypes. During the light phase, animals of both genotypes were mostly detected in the burrow area with a clear preference for the two-tunnel burrow (top half of the burrow area; Figure 3A). Although the pattern of occupation of the different zones of the VBS seemed equivalent between the genotypes (similar locations with similar shades of colors per genotype), the difference between both heat maps (Δ = [Tph2−/−] – [Tph2+/+]) indicated that during the inactive (light) phase Tph2−/− mice spent less time in the two-tunnel burrow and more time in the open zone than the Tph2+/+ mice (Figure 3B, Exact Wilcoxon-Mann-Whitney test: Open area, Z = −3.821, p-value = 9.294e–5). During the dark phase, mice of both genotypes were mostly detected at the feeder and close to the separating wall on the open side of the cage and more often in the burrow with two tunnels than in the burrow with a single tunnel (Figure 3C). During this active (dark) phase of the day, the difference in occupation of these zones between the genotype was even more pronounced than during the light phase (stronger variations of colors on the Δ heatmap), with the Tph2−/− mice significantly more often detected in the open area, especially at the feeder than the Tph2+/+ mice and less often in the two-tunnel burrow (Figure 3D, Exact Wilcoxon-Mann-Whitney test: Open area, Z = −5.3018, p-value = 1.741e−10).
Figure 3. Place preference in the VBS during light (A, B) and dark (C, D) phases. (A) Averaged place preference (%) of Tph2−/− (top) and Tph2+/+ (bottom) animals during the light phase (all days). Each tile of the heat map represents the position of one RFID reader as it was located under the VBS (24-RFID-grid). Food (orange rectangle) and water (blue square) were available from two distinct zones in the open area. The vertical black dashed line indicates the separation between the open and the burrow areas. The brighter the color of a tile is, the more often the RFID reader was activated [relative to the activation of all the other readers of the grid (%)] and thus the greater the animals preferred on average this location in the VBS. (B-top) Differences in place preference (%) between Tph2−/− and Tph2+/+ [subtraction of heat-maps in (A)] during the light phase. (B-bottom) The boxplot representation of averaged place preference (%) in Δ, by zone (open vs. burrow). (C) Averaged place preference (%) of Tph2−/− (top) and Tph2+/+ (bottom) animals during the dark phase (all days). (D-top) Differences in place preference (%) between Tph2−/− and Tph2+/+ [subtraction of heat maps in (C)] and (D-bottom) The boxplot representation of averaged place preference (%) in Δ, by zone (open vs. burrow). Boxplots show median, quartiles, 5th/95th percentiles, and outlying points ({ggplot2}, R). Exact Wilcoxon-Mann-Whitney test, *p < 0.05 between the genotypes.
Despite Tph2−/− spent more time at feeder, after 5 days in the VBS, Tph2−/− mice gained less weight than Tph2+/+ mice (Exact Wilcoxon-Mann-Whitney test: Z = −2.6436, p-value = 0.0072; Tph2−/− weight: before, mean = 18.8 ± SD: 2.0 g, after, mean = 18.9 ± SD: 3.4 g; Tph2+/+ weight: before, mean = 19.8 ± SD: 5.1 g; after, mean = 20.4 ± SD: 4.7 g).
In the semi-automated VBS, all behaviors listed in Table 1 are seen in both genotypes. Only “biting” (occurred five times in total: three times in Tph2+/+ and two times in Tph2−/− mice) and “mounting” (occurred six times: three times in each genotype) were very rarely seen. All behaviors, except for “huddle” which happens while sleeping, were most expressed during the active (dark) phase of the day (see light phase in Supplementary Figure S1). Tph2−/− mice performed significantly more offensive aggression, such as “approach to back,” “chasing,” “contact,” and “struggle at feeder” than Tph2+/+ mice and in both phases (except for “approach to back” and “chasing” during the light phase, for which the occurrences of behaviors are too rare to be meaningfully quantified; Figure 4A, Exact Wilcoxon-Mann-Whitney test: Dark phase, approach to back Z = −2.7593, p-value = 0.0048, chasing Z = −2.3208, p-value = 0.0196; contact: Z = −2.3702, p-value = 0.0169; struggle Z = −1.4001, p-value = 0.1652; struggle at feeder: Z = −4.5191, p-value = 7.158e−7; Supplementary Figure S1, Exact Wilcoxon-Mann-Whitney test: Light phase, contact: Z = −2.0371, p-value = 0.0414; struggle Z = −4.3624, p-value = 2.657e−6). In Tph2+/+ mice, “chasing” (both phases) and “approach to back” (in light phase only), “struggle” (in light phase only) and “struggle at feeder” (in light phase only) were rare behaviors (Figure 4A; Supplementary Figure S1). Tph2−/− mice were more defensive (“flight”) than the Tph2+/+ mice during the light phase (Supplementary Figure S1, Z = −2.1969, p-value = 0.0304) but not during the dark phase (Figure 4B, Z = −1.8483, p-value = 0.0652). Regarding social non-aggressive approaches, during the dark phase, Tph2−/− mice exhibited significantly less “sniffing” behaviors (Z = 2.4759, p-value = 0.0124) than Tph2+/+ mice but did not differ in total number of “approach to front” or “following” behavior during this same phase (Figure 4C, APF Z = −1.0565, p-value = 0.2979; following Z = −0.58745, p-value = 0.5655). These latter behaviors were rarely observed during the light phase in both genotypes and thus were not compared statistically (Supplementary Figure S1). In both phases, Tph2−/− mice were found eating and drinking (although “drinking” was more rarely observed, probably because of the shortness of the behavior) significantly more often than Tph2+/+ mice (Figure 4D: Dark phase, drinking Z = −3.1292, p-value = 0.0012; eating Z = −3.5444, p-value = 0.0002; Supplementary Figure S1: Light phase, drinking Z = −3.1837, p-value = 0.0013; eating Z = −4.2074, p-value = 6.191e−6) and grooming during the dark phase was less often witnessed in Tph2−/− mice than in Tph2+/+ mice (Figure 4D: Dark phase, Z = 2.5875, p-value = 0.0087; Supplementary Figure S1: Light phase Z = −0.7856, p-value = 0.4403). Finally considering affiliative behaviors, Tph2−/− mice showed less “allogrooming” behavior than Tph2+/+ mice, in both phases (Figure 4E, Dark phase, Z = 4.3102, p-value = 3.562e−6; Light phase, Z = 2.6884, p-value = 0.0064) while “huddled” was shown as many times as in Tph2+/+ mice and during both phases (Figure 4E, Supplementary Figure S1).
Figure 4. The total number of occurrences of social and non-social behaviors in the home cage. (A–E) The total number of behaviors (grouped by domains) per genotype and over all experimental days during dark phases. ATB, approach to back; ATF, approach to front. (F) From the RF classifier, plots of 100 Gini values, for each VBS variable, during the dark and light phases separately. Gini index >1 (dotted line) indicates behaviors highly different between genotypes. SAF: struggle at feeder. Boxplots show median, quartiles, 5th/95th percentiles, and outlying points ({ggplot2}, R). Exact Wilcoxon-Mann-Whitney test, *p < 0.05. (G) Social networks for “struggle at feeder” (top) and “allogrooming” (bottom) of an exemplary group of Tph2−/− and of Tph2+/+ mice across dark phases of successive days. A node represents an individual and the width of a line of the overall strength of that behavior between the pair of animals for the given day.
The relationships between social and non-social behaviors were then explored per genotype with Spearman correlations (Supplementary Tables S1, S2). After 5 days in the VBS, Tph2−/− mice did not show a weight gain different from Tph2+/+ mice that slightly increased in weight (Exact Wilcoxon-Mann-Whitney test: Z = −2.6436, p-value = 0.0072; Tph2−/− weight: before, mean = 18.8 ± SD: 2 g, after, mean = 18.9 ± 3.4 g; Tph2+/+ weight: before, mean = 19.8 ± 5.1 g; after, mean = 20.4 ± 4.7 g).
The training of the Random Forest (RF) classifier, on the social and non-social behaviors, and distance traveled in the VBS of the Tph2−/− and Tph2+/+ mice, led to a high precision in genotype prediction with an averaged accuracy of 81.4% (±1.2) over 100 runs. For each LOOCV run of the classifier, we obtained the Gini index for each input variable. These values provided a robust estimate for the importance of each of the given behaviors to differentiate Tph2−/− from Tph2+/+ mice (Figure 4F). With the Gini index ≥1, the behaviors with the greatest potential for differentiation of the two genotypes were “allogrooming”, “struggling at feeder” and “eating” during the dark phase and “eating” and “struggle” during the light phase (Figure 4F).
The network of two of the most discriminative variables between genotypes, “struggle at feeder” (offensive) and “allogrooming” (affiliative), showed clear differences in their topologies across days (Exact Wilcoxon-Mann-Whitney test, overall interaction strength, struggling at feeder, Z = −4.2158, p-value = 1.2446e−5; allogrooming, Z = −4.6798, p-value = 1.4358e−6; Figure 4G showing two representative groups of mice). On a daily basis, Tph2−/− mice compared to Tph2+/+ mice struggled at feeder with a higher interaction strength, from days 1 to 5, (day 1: Z = −3.6607, p-value = 0.0001; day 2: Z = −3.7961, p-value = 7.3489e−5; day 3: Z = −2.8738, p-value = 0.0020; day 4: Z = −5.1147, p-value = 1.5709e−7; day 5: Z = −4.277, p-value = 9.4703e−6; Figure 4G) but performed “allogrooming” with a lower interaction strength from days 2 to 5 (day 2: Z = −5.1973, p-value = 1.0111e−7; day 3: Z = −3.3003, p-value = 0.0005; day 4: Z = −2.3549, p-value = 0.0093; day 5: Z = −3.8115, p-value = 6.907e−5; Figure 4G). This showed that each mouse would struggle at feeder with all the other mice of the group (between all pairs), repeatedly (edges are thick) and consistently over days, in contrast to the Tph2+/+ networks where struggling at feeder was observed between fewer and varying pairs of mice over days.
In both genotypes changes in individual Glicko ratings over time indicated the emergence of dominance within each group (Figure 5A for two groups and all groups in Supplementary Figure S3). In all groups, one (in two groups) or two animals' rankings were found above their initial Glicko rating (y = 0; Figure 5B) indicating in both genotypes, stratification of the individuals at the end of the VBS, into higher and lower ranked individuals (Figure 5B). In the Tph2+/+ groups, the highest ranked individual already emerged as the most dominant animal after a median number of 61 agonistic interactions (mean = 85.8 ± SD: 54.13; group scores: 16, 60, 61, 171, 121; Figure 5C). In the Tph2−/− groups, dominant males emerged as dominant individuals of their groups after a median number of 243 agonistic interactions (mean = 269.2 ± SD: 122.53; group scores: 125, 243, 158, 382, 438; Figure 5C). Taken together, dominance emerged more readily, after less interactions, in the Tph2+/+ groups compared to the Tph2−/− groups. The results were consistent after controlling for the overall higher number of interactions in the Tph2−/− groups by dividing the count at emergence by the overall count of interactions within the groups (Supplementary Figure S3).
Figure 5. Temporal and social properties of individual Glicko ratings for Tph2+/+ groups (batches 1–5) and Tph2−/− groups (batches 6-10). (A) Change in individual Glicko ratings over time of two selected groups [batch 3 (Tph2+/+) and batch 10 (Tph2−/−)] illustrating early and late establishment of dominance depending on genotype. Each line represents the ratings of one individual in the group, while the solid black line represents the final dominant male. Ratings are recalculated for every individual after each agonistic interaction. Rating values are normalized as the ratio of absolute maximum rating within a given group history ({matplotlib}, Python). (B) The final individual ranking of each group normalized to the absolute maximum rating within a given genotype. The initial rating value is shown as a vertical dotted line at zero. (C) The minimum number of agonistic interactions, each dominant animal engaged in before they reached the top Glicko rating that remained their rank until the end of the rating period. (D) The power of the dominant male is defined as the ratio of absolute power exhibited by the dominant toward the second-ranked male over the total power expressed toward the lowest ranking animal. Despotic dominance is above 0.33 (dotted line).
Finally, we examined the power exerted by the dominant male on subordinate animals by evaluating how much each dominant male monopolized agonistic interactions within their social group or said otherwise, how (un)equally distributed the power was within groups. Considering the individual's final Glicko rating, a dominant male is considered “despotic” when it imposes toward the second-highest-ranked male power of one-third or more of the total imposed power on the lowest-ranked animal. Here we found that three out of the six most despotic males (above alpha = 0.33) were Tph2+/+ animals, indicating no difference in (despotic) style between genotypes (Figure 5D, Supplementary Table S3).
The first attack in Tph2−/− mice occurred sooner than for the Tph2+/+ mice. They attacked (struggle and struggle at feeder together) with a higher frequency and used significantly fewer warning signals (e.g., threats: chasing, following, ATB) than Tph2+/+ mice. However, the duration of a given attack was not longer for the Tph2−/− mice than Tph2+/+ mice and fights occurred mainly at the feeder (Table 2).
In the first 5 min of the social preference test, both groups of mice preferred interacting with the “unfamiliar” individual (Figure 6A, Mann-Whitney test, Tph2−/− mice: W = 96, p-value = 3.544e−6; Tph2+/+ mice: W = 61.5, p-value = 0.001556). In the next 5 min while Tph2+/+ mice lost interest in the now “familiar” animal, the Tph2−/− mice kept interacting more with the mouse than with the empty cage (Figure 6B, Tph2−/− mice: W = 185, p-value = 0.001575). Tph2−/− mice did not habituate as fast as the Tph2+/+ mice to a new individual. They kept investigating the novel individual over the 10 min of the test while the preference for the novel individual was reduced in Tph2+/+ after 5 min of the test. In the social recognition test, for both genotypes, the duration spent in the chamber with the “familiar” individual was not different from the time spent in the chamber with the “unfamiliar” individual, precluding further interpretation of this second phase of the test (Supplementary Figure S4).
Figure 6. Social preference and habituation in the three-chamber test. The total duration of interaction with the empty grid or the grid with enclosed unfamiliar mouse during 1–5 min (A) and 6–10 min (B) of test ({ggplot2}, R). Wilcoxon-Mann-Whitney test, *p < 0.05 between empty and unfamiliar for the same genotype. s, seconds.
In this study, we performed an in-depth analysis of home cage behavior of group living mice to investigate the role of central serotonin in the expression of everyday-life aggression, social and non-social activities and the dynamics of group organization. In this semi-natural environment, Tph2−/− animals did show some well-conserved mouse behavioral characteristics. However, the lack of brain serotonin also resulted in significant behavioral anomalies in mice and led to altered social network characteristics and dynamics of group formation, indicative of broader cognitive impairments.
In the undisturbed ethological-like conditions of the VBS housing, Tph2−/− mice showed typical day-night fluctuations of activity and a similar pattern of home-cage zone use throughout the day. Similar to wild-type controls, they visited more frequently the food and open areas during active phases and the safe and sheltered areas during inactive phases. Serotonin ablation did not impact the typical day/night fluctuations in mouse activity observed as a response to changes in environmental light. Despite known interactions between serotonin and circadian control systems (regulation of the sleep-wake cycle) and their respective roles in the expression of seasonal mood disorders for instance (Gallardo et al., 2020), this result confirms the role of a larger neurobiological network for the regulation of these homeostatic processes. Moreover, the lack of congenital serotonin did not abolish the expression of any specific behavior in mice, however, it drastically affected their relative frequencies. Only the life-essential affiliative behavior of “huddling” (i.e. “sleeping in direct contact with at least one other mouse”), in Tph2−/− mice was unaffected. The expression of “huddling”, which is essential for the maintenance of group cohesion (Gilbert et al., 2010; Arakawa et al., 2007), was well-preserved and negatively correlated with offensive behaviors in both Tph2−/− and Tph2+/+ groups. Finally, despite the altered behavior patterns of Tph2−/− mice, their groups are still organized hierarchically with time. The conservation of typical daily life characteristics along with the simultaneous expression of deficits give this model comprehensive face validity. Indeed, in their everyday life, violent patients are not socially maladapted in all contexts and all endeavors, they can also adapt to some social contexts.
Nevertheless, the lack of brain serotonin had a strong impact on most other individual and group characteristics. Pathological aggression has been described as inappropriate, frequent, and prolonged bouts of aggression, which leads to an increased burden for the individual (Haller et al., 2005). On the five criteria of pathological aggression that we could analyze (Table 2), Tph2−/− mice attacked sooner, more often, and displayed fewer “warning” signs than Tph2+/+ animals. However, fights were of similar duration between genotypes and rarely occurred in inappropriate zones of the cage, such as in the burrows, but mostly occurred at the feeder, a typical area for fights. Moreover, Tph2−/− mice might visit the feeder more often due to their increased metabolism (van Lingen et al., 2019), increasing their chances to meet and potentially fight as food is a resource that naturally triggers aggression (Blanchard and Blanchard, 2003). While these observations could indicate more adaptive aggression, the analysis of the day-to-day network of their fights revealed an atypical lack of de-escalation of aggression over time. In the absence of de-escalation of aggression and in addition to the other markers of pathological aggression, this home cage analysis suggests Tph2−/− mice as a potential model to study pathological aggression.
On a complementary note, the lack of de-escalation of aggression in these mice could indicate poor behavioral control and cognitive flexibility. Indeed, serotonin and its multiple receptors are essential players in the control of behavior, behavioral flexibility, and the extinction of context-dependent conditioned behaviors (Bacqué-Cazenave et al., 2020; Dellu-Hagedorn et al., 2018; Alvarez et al., 2021).
Moreover, our study highlights impairments of the Tph2−/− mice to form familiar social memories and to show typical communication behavior. Since exhibiting adaptable and appropriate social behaviors, in particular, inhibiting aggression and preventing conflict, is crucial to establishing social memories and effectively communicating this knowledge to others, a lack of appropriate social knowledge could prevent Tph2−/− mice from flexibly adjusting their behavior and controlling aggression.
While Tph2−/− mice do not have olfactory deficits, which allows them to use olfactory cues to form social knowledge (Carlson et al., 2016; Mosienko, 2013), in the three-chamber test, Tph2−/− mice kept investigating the unfamiliar mouse for twice as long as a Tph2+/+ mouse indicating a deficit in their ability to build familiar memories. The role of serotonin in forming social memories is consistent with serotonin being a new pharmacological target to counter memory alteration through lack of synaptic plasticity (González-Burgos and Feria-Velasco, 2008). It further plays an essential role in memory formation, especially short and working memory (Hritcu et al., 2007; Coray and Quednow, 2022) and the encoding of familiarity and phenomena of déjà vu (Kalra et al., 2007). In regards to these studies and following a “for better for worse model” (Kiser et al., 2012), one hypothesis is the lack of serotonin in the Tph2−/− mice could dampen their sensitivity to social and non-social cues present in the environment, potentially delaying the formation of new social memories.
Another key result is the lack of expression of typical communication skills. Tph2−/− mice showed low “sniffing” and “allogrooming” counts (here and Beis et al., 2015; Kane et al., 2012), which are also among the four most discriminating behaviors between genotypes (along with “aggression” and “feeding behavior”) identified by the Random Forest classifier. These two behaviors are essential for social communication (Berg et al., 2018) in animals to build social knowledge (Lee et al., 2019) and for the maintenance of group cohesion in mice (Wu et al., 2021; Schweinfurth et al., 2017). In mice, through their tactile sensitivity, sniffing (i.e., air movement on face and fur between animals) and allogrooming are important modalities for sharing an understanding of each individual's leadership position (Wesson, 2013; Lee et al., 2019). In the undisturbed environment of their home cage, these animals did not display the typical behaviors that allow them to gather, communicate, and use important social cues from their conspecifics. In the absence of such social information, the Tph2−/− mice could not have typical social knowledge of the different individuals in the cage which would lead to an inability to behavioral adjustment.
Here we also showed that very aggressive genetically-similar mice did dynamically organize their groups into individually stratified and stable hierarchies (dynamic and final Glicko ratings). This was although hierarchies emerged later, and the power of the alpha male was more diffuse in Tph2−/− mice than in Tph2+/+ groups. These results highlight the non-essential role of serotonin in building up a social hierarchy but at the same time serotonin's absence impacts the structuring and dynamic aspects of group formation (SNA, diffused power, late emergence of leader). The emergence of a dominant individual is a dynamic process relying in part on communication, social knowledge, and behavioral flexibility of the individuals of the group (Wesson, 2013), which are social competences for which Tph2−/− mice are highly impaired.
Our findings confirm the increased aggression, delayed social memory formation, and impulsivity previously reported in male and female Tph2-deficient mice and rats, which resemble aspects of ASD in humans (Angoa-Pérez et al., 2012; Mosienko et al., 2012, 2015a,b; Kane et al., 2012; Beis et al., 2015; Kästner et al., 2019; Maddaloni et al., 2018; Meng et al., 2022). These behavioral abnormalities are probably not caused by developmental alterations in a serotonin-deficient brain, since the silencing of serotonergic neurons by overexpression of the inhibiting autoreceptor 5-HT1A in adulthood also increased aggressive behavior (Audero et al., 2013).
Finally, while most of the social and everyday life impairments in human patients are not easily measurable in preclinical or clinical settings, in pre-clinical research, the use of ethological-like testing systems offers a novel avenue to catch everyday-life complexity. With this new semi-natural cage and the corresponding analytical tools, we developed for this project, we could study the everyday-life longitudinal symptomatology of our animal models, in different cage contexts, at both individual and group levels. This methodology provided a unique set-up for evaluating complex behaviors and further expanded our knowledge of the role of brain serotonin in pathological aggression.
This study has several limitations. We aimed to investigate the effect of the congenital lack of brain serotonin on group behavior in mice. Only males, but not females were included in the experimental design. Although females do not typically engage in aggressive behaviors as males do, they can engage in affiliative types of behaviors that could have been disturbed by a lack of serotonin. Therefore, it is of high importance to perform similar studies in cohorts of female mice in the future. Another limitation of our study is the potential confounding effect introduced by the use of unfamiliar mice from different litters. Due to the constraints of our breeding scheme, it was necessary to group animals of the same genotype from different litters, resulting in the introduction of mice that were not previously co-housed. However, these animals were mixed together between 5 and 6 weeks of age and kept in the same cage for at least 1 week before experimentation, this period may not have been sufficient for full acclimatization and establishment of social hierarchies. Consequently, the developing familiarity among the mice could have influenced their behavior and physiological responses, potentially impacting the study's outcomes. This limitation should be taken into account when interpreting the findings, and future studies may consider extending the acclimatization period or employing alternative strategies to mitigate this confounding factor. In addition, the use of a congenital model lacking the enzyme responsible for the central serotonin synthesis did not allow us to distinguish whether the differences in the behavioral profile are a result of developmental alterations caused by the absence of brain serotonin or serotonergic transmission itself. Although studies in 5-HT1A animal models (Audero et al., 2013) argue for the direct involvement of serotonergic neurotransmission in the manifestation of observed phenotypes, the reestablishment of other systems due to the congenital lack of serotonin (Maddaloni et al., 2024) cannot be excluded. To overcome this limitation and to study more precisely the mechanisms and downstream pathways resulting in the behavioral phenotypes observed in Tph2-deficeint mice, future studies should involve more sophisticated methods using conditional knockouts or chemogenetic/optogenetic approaches A combination of innovative methods developed in frames of this study in combination with such animal models in a follow up study may shed light on the nature of observed phenotypes.
In this study, we show that Tph2−/− mice present several characteristics of pathological aggression. However, beyond aggression, in their undisturbed housing conditions, Tph2−/− mice have a more subtle, complex, and dynamically maladaptive phenotype. Mice lacking serotonin had poorer communication skills (i.e., sniffing and allogrooming), possibly poorer sensitivity to environmental cues (social and non-social), and altered short term memory formation of social knowledge, as well as more slowly developing hierarchical ranking and different social network dynamics. In this study, we highlighted the great advantages of using home cage monitoring systems for the integrated analysis of the several layers, temporality, and relationships of social and non-social behaviors in mice, from individual to group levels.
We consider Tph2−/− mice to be a great potential tool to further investigate the role of serotonin in the expression of food related aggression, short term social memory formation, and aspects of social competence. Finally, in this study, by integrating home cage environments with specialized analytical methods tailored for assessing complex, spontaneously occurring home cage behaviors, we enabled the study of the dynamic and temporal aspects of mice living in self-organized groups.
The original contributions presented in the study are included in the article/Supplementary material, further inquiries can be directed to the corresponding authors.
The animal study was approved by Landesamt für Gesundheit und Soziales (LaGeSo), Berlin, Germany. The study was conducted in accordance with the local legislation and institutional requirements.
MR: Conceptualization, Formal analysis, Funding acquisition, Methodology, Supervision, Validation, Writing – original draft, Writing – review & editing, Data curation, Investigation, Visualization, Project administration. LA: Writing – review & editing, Formal analysis, Investigation, Project administration, Visualization. VM: Writing – review & editing, Conceptualization, Investigation. PB: Writing – review & editing, Software, Formal analysis, Visualization. AH: Writing – review & editing, Investigation. MB: Funding acquisition, Supervision, Writing – review & editing, Resources. YW: Funding acquisition, Supervision, Writing – review & editing, Conceptualization, Methodology, Software. NA: Conceptualization, Funding acquisition, Methodology, Supervision, Writing – review & editing, Project administration.
The author(s) declare that financial support was received for the research, authorship, and/or publication of this article. This study was supported by grants from the German Research Foundation to MR (DFG RI 2474/2-1 and DFG NeuroCure) and to NA (AL1197/5-1). It was also supported by the EU H2020 MSCA ITN projects “Serotonin and Beyond” (N 953327) to NA and MB and by the AMS Springboard award SBF005/1102 and the MRC Career Development Award MR/T031115/1 to VM.
We thank Melissa Long, Susanne da Costa Goncalves, Alexej Schatz, Mihail Todiras, Felix Brun, Nina Soto, and Milena Brunet for their support through technical assistance and knowledgeable discussion. We thank Vivien Rabke, for the work with the animals.
YW owns equity in PhenoSys.
The remaining authors declare that the research was conducted in the absence of any commercial or financial relationships that could be construed as a potential conflict of interest.
The author(s) declared that they were an editorial board member of Frontiers, at the time of submission. This had no impact on the peer review process and the final decision.
All claims expressed in this article are solely those of the authors and do not necessarily represent those of their affiliated organizations, or those of the publisher, the editors and the reviewers. Any product that may be evaluated in this article, or claim that may be made by its manufacturer, is not guaranteed or endorsed by the publisher.
The Supplementary Material for this article can be found online at: https://www.frontiersin.org/articles/10.3389/fnbeh.2024.1450540/full#supplementary-material
Alenina, N., Kikic, D., Todiras, M., Mosienko, V., Qadri, F., Plehm, R., et al. (2009). Growth retardation and altered autonomic control in mice lacking brain serotonin. Proc. Natl. Acad. Sci. U. S. A. 106, 10332–10337. doi: 10.1073/pnas.0810793106
Alonso, L., Peeva, P., Ramos-Prats, A., Alenina, N., Winter, Y., and Rivalan, M. (2020). Inter-individual and inter-strain differences in cognitive and social abilities of Dark Agouti and Wistar Han rats. Behav. Brain Res. 377:112188. doi: 10.1016/j.bbr.2019.112188
Alonso, L., Peeva, P., Stasko, S., Bader, M., Alenina, N., Winter, Y., et al. (2023). Constitutive depletion of brain serotonin differentially affects rats' social and cognitive abilities. iScience 26:105998. doi: 10.1016/j.isci.2023.105998
Alvarez, B. D., Morales, C. A., and Amodeo, D. A. (2021). Impact of specific serotonin receptor modulation on behavioural flexibility. Pharmacol. Biochem. Behav. 209, 173243. doi: 10.1016/j.pbb.2021.173243
American Psychiatric Association Committee on Nomenclature and Statistics. (2018). Diagnostic and Statistical Manual of Mental Disorders (DSM-5), 5th Edn. Washington, DC: American Psychiatric Press.
Angoa-Pérez, M., Kane, M. J., Briggs, D. I., Sykes, C. E., Shah, M. M., Francescutti, D. M., et al. (2012). Genetic depletion of brain 5HT reveals a common molecular pathway mediating compulsivity and impulsivity. J. Neurochem. 121, 974–984. doi: 10.1111/j.1471-4159.2012.07739.x
Arakawa, H., Blanchard, D. C., and Blanchard, R. J. (2007). Colony formation of C57BL/6J mice in visible burrow system: identification of eusocial behaviours in a background strain for genetic animal models of autism. Behav. Brain Res. 176, 27–39. doi: 10.1016/j.bbr.2006.07.027
Audero, E., Mlinar, B., Baccini, G., Skachokova, Z. K., Corradetti, R., and Gross, C. (2013). Suppression of serotonin neuron firing increases aggression in mice. J. Neurosci. 33, 8678–8688. doi: 10.1523/JNEUROSCI.2067-12.2013
Bacqué-Cazenave, J., Bharatiya, R., Barrière, G., Delbecque, J.-P., Bouguiyoud, N., Di Giovanni, G., et al. (2020). Serotonin in animal cognition and behaviour. Int. J. Mol. Sci. 21:51649. doi: 10.3390/ijms21051649
Beis, D., Holzwarth, K., Flinders, M., Bader, M., Wöhr, M., and Alenina, N. (2015). Brain serotonin deficiency leads to social communication deficits in mice. Biol. Lett. 11:57. doi: 10.1098/rsbl.2015.0057
Berg, E.L., Copping, N.A., Rivera, J.K., Pride, M.C., Careaga, M., Bauman, M.D., et al. (2018). Developmental social communication deficits in the Shank3 rat model of phelan-mcdermid syndrome and autism spectrum disorder. Autism Res. 11, 587–601. doi: 10.1002/aur.1925
Blanchard, D. C., and Blanchard, R. J. (2003). What can animal aggression research tell us about human aggression? Horm. Behav. 44, 171–177. doi: 10.1016/S0018-506X(03)00133-8
Blanchard, R. J., and Blanchard, D. C. (1989). Antipredator defensive behaviours in a visible burrow system. J. Comp. Psychol. 103, 70–82. doi: 10.1037/0735-7036.103.1.70
Bove, M., Ike, K., Eldering, A., Buwalda, B., de Boer, S. F., Morgese, M. G., et al. (2018). The Visible Burrow System: a behavioural paradigm to assess sociability and social withdrawal in BTBR and C57BL/6J mice strains. Behav. Brain Res. 344, 9–19. doi: 10.1016/j.bbr.2018.02.003
Carlson, K. S., Whitney, M. S., Gadziola, M. A., Deneris, E. S., and Wesson, D. W. (2016). Preservation of essential odor-guided behaviours and odor-based reversal learning after targeting adult brain serotonin synthesis. eNeuro 3, ENEURO.0257-16.2016. doi: 10.1523/ENEURO.0257-16.2016
Coray, R., and Quednow, B. B. (2022). The role of serotonin in declarative memory: a systematic review of animal and human research. Neurosci. Biobehav. Rev. 139:104729. doi: 10.1016/j.neubiorev.2022.104729
Dellu-Hagedorn, F., Rivalan, M., Fitoussi, A., and De Deurwaerdère, P. (2018). Inter-individual differences in the impulsive/compulsive dimension: deciphering related dopaminergic and serotonergic metabolisms at rest. Philos. Transact. R. Soc. B Biol. Sci. 373:20170154. doi: 10.1098/rstb.2017.0154
Gallardo, C. M., Martin, C. S., and Steele, A. D. (2020). Food anticipatory activity on circadian time scales is not dependent on central serotonin: evidence from tryptophan hydroxylase-2 and serotonin transporter knockout mice. Front. Mol. Neurosci. 13:534238. doi: 10.3389/fnmol.2020.534238
Gilbert, C., McCafferty, D., Maho, Y. L., Martrette, J.-M., Giroud, S., Blanc, S., et al. (2010). One for all and all for one: the energetic benefits of huddling in endotherms. Biol. Rev. 85, 545–569. doi: 10.1111/j.1469-185X.2009.00115.x
Glickman, M. E. (1999). Parameter estimation in large dynamic paired comparison experiments. J. R. Stat. Soc. Ser. C 48, 377–394. doi: 10.1111/1467-9876.00159
González-Burgos, I., and Feria-Velasco, A. (2008). Serotonin/dopamine interaction in memory formation. Prog. Brain Res. 172, 603–623. doi: 10.1016/S0079-6123(08)00928-X
Gottesman, I. I., and Gould, T. D. (2003). The endophenotype concept in psychiatry: etymology and strategic intentions. Am. J. Psychiatry 160, 636–645. doi: 10.1176/appi.ajp.160.4.636
Gould, T. D., and Gottesman, I. I. (2006). Psychiatric endophenotypes and the development of valid animal models. Genes Brain Behav. 5, 113–119. doi: 10.1111/j.1601-183X.2005.00186.x
Hadfield, J. D. (2010). MCMC methods for multi-response generalized linear mixed models: the MCMCglmm R package. J. Stat. Softw. 33, 1–22. doi: 10.18637/jss.v033.i02
Haller, J., Mikics, E., Halász, J., and Tóth, M. (2005). Mechanisms differentiating normal from abnormal aggression: glucocorticoids and serotonin. Eur. J. Pharmacol. 526, 89–100. doi: 10.1016/j.ejphar.2005.09.064
Hritcu, L., Clicinschi, M., and Nabeshima, T. (2007). Brain serotonin depletion impairs short-term memory, but not long-term memory in rats. Physiol. Behav. 91, 652–657. doi: 10.1016/j.physbeh.2007.03.028
Kalra, S., Chancellor, A., and Zeman, A. (2007). Recurring déjà vu associated with 5-hydroxytryptophan. Acta Neuropsychiatr. 19, 311–313. doi: 10.1111/j.1601-5215.2007.00245.x
Kalueff, A. V., Stewart, A. M., Song, C., and Gottesman, I. I. (2015). Targeting dynamic interplay among disordered domains or endophenotypes to understand complex neuropsychiatric disorders: Translational lessons from preclinical models. Neurosci. Biobehav. Rev. 53, 25–36. doi: 10.1016/j.neubiorev.2015.03.007
Kane, M. J., Angoa-Peréz, M., Briggs, D. I., Sykes, C. E., Francescutti, D. M., Rosenberg, D. R., et al. (2012). Mice genetically depleted of brain serotonin display social impairments, communication deficits and repetitive behaviours: possible relevance to autism. PLoS ONE 7:e48975. doi: 10.1371/journal.pone.0048975
Karigo, T., Kennedy, A., Yang, B., Liu, M., Tai, D., Wahle, I. A., et al. (2020). Distinct hypothalmic control of same- and opposite-sex mounting behaviour in mice. Nature 589, 258–263. doi: 10.1038/s41586-020-2995-0
Kästner, N., Richter, S. H., Urbanik, S., Kunert, J., Waider, J., Lesch, K.-P., et al. (2019). Brain serotonin deficiency affects female aggression. Sci. Rep. 9:1366. doi: 10.1038/s41598-018-37613-4
Kiser, D., Steemers, B., Branchi, I., and Homberg, J. R. (2012). The reciprocal interaction between serotonin and social behaviour. Neurosci. Biobehav. Rev. 36, 786–798. doi: 10.1016/j.neubiorev.2011.12.009
Krause, J., James, R., and Croft, D. P. (2010). Personality in the context of social networks. Phil. Trans. R. Soc. B 365, 4099–4106. doi: 10.1098/rstb.2010.0216
Lee, W., Fu, J., Bouwman, N., Farago, P., and Curley, J. P. (2019). Temporal microstructure of dyadic social behaviour during relationship formation in mice. PLoS ONE 14:e0220596. doi: 10.1371/journal.pone.0220596
Lesch, K.-P., Araragi, N., Waider, J., van den Hove, D., and Gutknecht, L. (2012). Targeting brain serotonin synthesis: insights into neurodevelopmental disorders with long-term outcomes related to negative emotionality, aggression and antisocial behaviour. Philos. Trans. R. Soc. Lond. B Biol. Sci. 367, 2426–2443. doi: 10.1098/rstb.2012.0039
Lewejohann, L., Kloke, V., Heiming, R. S., Jansen, F., Kaiser, S., Schmitt, A., et al. (2010). Social status and day-to-day behaviour of male serotonin transporter knockout mice. Behav. Brain Res. 211, 220–228. doi: 10.1016/j.bbr.2010.03.035
Liaw, A., and Wiener, M. (2002). The R journal: classification and regression by randomForest. R News 2, 18–22.
Maddaloni, G., Barsotti, N., Migliarini, S., Giordano, M., Nazzi, S., Picchi, M., et al. (2024). Impact of serotonin deficiency on circadian dopaminergic rhythms. Int. J. Mol. Sci. 25:6475. doi: 10.3390/ijms25126475
Maddaloni, G., Migliarini, S., Napolitano, F., Giorgi, A., Nazzi, S., Biasci, D., et al. (2018). Serotonin depletion causes valproate-responsive manic-like condition and increased hippocampal neuroplasticity that are reversed by stress. Sci. Rep. 8:11847. doi: 10.1038/s41598-018-30291-2
McCloskey, D. P., Kress, M. E., Imberman, S. P., Kushnir, I., and Briffa-Mirabella, S. (2011). “From market baskets to mole rats: using data mining techniques to analyze RFID data describing laboratory animal behaviour,” in Proceedings of the 17th ACM SIGKDD International Conference on Knowledge Discovery and Data Mining, KDD '11 (New York, NY: ACM), 301–306.
Meng, X., Grandjean, J., Sbrini, G., Schipper, P., Hofwijks, N., Stoop, J., et al. (2022). Tryptophan hydroxylase 2 knockout male rats exhibit a strengthened oxytocin system, are aggressive, and are less anxious. ACS Chem. Neurosci. 13, 2974–2981 doi: 10.1021/acschemneuro.2c00448
Miczek, K. A., de Almeida, R. M. M., Kravitz, E. A., Rissman, E. F., de Boer, S. F., and Raine, A. (2007). Neurobiology of escalated aggression and violence. J. Neurosci. 27, 11803–11806. doi: 10.1523/JNEUROSCI.3500-07.2007
Miczek, K. A., Fish, E. W., and De Bold, J. F. (2003). Neurosteroids, GABAA receptors, and escalated aggressive behaviour. Horm. Behav. 44, 242–257. doi: 10.1016/j.yhbeh.2003.04.002
Mosienko, V. (2013). Behavioural, Neuronal and Developmental Consequences of Genetically Decreased Tryptophan Hydroxylase 2 Activity. Berlin: Humboldt-Universität zu Berlin.
Mosienko, V., Beis, D., Alenina, N., and Wöhr, M. (2015a). Reduced isolation-induced pup ultrasonic communication in mouse pups lacking brain serotonin. Mol. Autism 6:13. doi: 10.1186/s13229-015-0003-6
Mosienko, V., Beis, D., Pasqualetti, M., Waider, J., Matthes, S., Qadri, F., et al. (2015b). Life without brain serotonin: reevaluation of serotonin function with mice deficient in brain serotonin synthesis. Behav. Brain Res. 277, 78–88. doi: 10.1016/j.bbr.2014.06.005
Mosienko, V., Bert, B., Beis, D., Matthes, S., Fink, H., Bader, M., et al. (2012). Exaggerated aggression and decreased anxiety in mice deficient in brain serotonin. Transl. Psychiatry 2 :e122. doi: 10.1038/tp.2012.44
Natarajan, D., and Caramaschi, D. (2010). Animal violence demystified. Front. Behav. Neurosci. 4:9. doi: 10.3389/fnbeh.2010.00009
Natarajan, D., de Vries, H., Saaltink, D.-J., Boer, S. F., and de Koolhaas, J. M. (2009). Delineation of violence from functional aggression in mice: an ethological approach. Behav. Genet. 39, 73–90. doi: 10.1007/s10519-008-9230-3
Nelson, R. J., and Trainor, B. C. (2007). Neural mechanisms of aggression. Nat. Rev. Neurosci. 8, 536–546. doi: 10.1038/nrn2174
Nestler, E. J., and Hyman, S. E. (2010). Animal models of neuropsychiatric disorders. Nat. Neurosci. 13, 1161–1169. doi: 10.1038/nn.2647
Neumann, C., Duboscq, J., Dubuc, C., Ginting, A., Irwan, A. M., Agil, M., et al. (2011). Assessing dominance hierarchies: validation and advantages of progressive evaluation with Elo-rating. Anim. Behav. 82, 911–921. doi: 10.1016/j.anbehav.2011.07.016
Niederkofler, V., Asher, T. E., Okaty, B. W., Rood, B. D., Narayan, A., Hwa, L. S., et al. (2016). Identification of serotonergic neuronal modules that affect aggressive behaviour. Cell Rep. 17, 1934–1949. doi: 10.1016/j.celrep.2016.10.063
Pobbe, R. L. H., Pearson, B. L., Defensor, E. B., Bolivar, V. J., Young, W. S. III., Lee, H.-J., et al. (2012). Oxytocin receptor knockout mice display deficits in the expression of autism-related behaviours. Horm. Behav. 61, 436–444. doi: 10.1016/j.yhbeh.2011.10.010
Robbins, T. W., Gillan, C. M., Smith, D. G., de Wit, S., and Ersche, K. D. (2012). Neurocognitive endophenotypes of impulsivity and compulsivity: towards dimensional psychiatry. Trends Cogn. Sci. 16, 81–91. doi: 10.1016/j.tics.2011.11.009
Sapolsky, R. M. (2005). The influence of social hierarchy on primate health. Science. 08, 648–652. doi: 10.1126/science.1106477
Schweinfurth, M. K., Stieger, B., and Taborsky, M. (2017). Experimental evidence for reciprocity in allogrooming among wild-type Norway rats. Sci. Rep. 7:4010. doi: 10.1038/s41598-017-03841-3
So, N., Franks, B., Lim, S., and Curley, J. P. (2015). A social network approach reveals associations between mouse social dominance and brain gene expression. PLoS ONE 10:e0134509. doi: 10.1371/journal.pone.0134509
Takahashi, A., Quadros, I. M., Almeida, R. M. M., and Miczek, K. A. (2010). Brain serotonin receptors and transporters: initiation vs. termination of escalated aggression. Psychopharmacology 213, 183–212. doi: 10.1007/s00213-010-2000-y
van Lingen, M., Sidorova, M., Alenina, N., and Klempin, F. (2019). Lack of brain serotonin affects feeding and differentiation of newborn cells in the adult hypothalamus. Front. Cell Dev. Biol. 7:65. doi: 10.3389/fcell.2019.00065
Van Loo, P. L. P., Van Zutphen, L. F. M., and Baumans, V. (2003). Male management: coping with aggression problems in male laboratory mice. Lab. Anim. 37, 300–313. doi: 10.1258/002367703322389870
Volavka, J. (1999). The neurobiology of violence: an update. J. Neuropsychiatry Clin. Neurosci. 11, 307–314. doi: 10.1176/jnp.11.3.307
Wang, F., Zhu, J., Zhu, H., Zhang, Q., Lin, Z., and Hu, H. (2011). Bidirectional control of social hierarchy by synaptic efficacy in medial prefrontal cortex. Science 334, 693–697. doi: 10.1126/science.1209951
Wesson, D. W. (2013). Sniffing behaviour communicates social hierarchy. Curr. Biol. 23, 575–580. doi: 10.1016/j.cub.2013.02.012
WHO (2004). The Global Burden of Disease: 2004 Update. WHO. Available at: http://www.who.int/healthinfo/global_burden_disease/2004_report_update/en/
Williamson, C. M., Lee, W., and Curley, J. P. (2016). Temporal dynamics of social hierarchy formation and maintenance in male mice. Anim. Behav. 115, 259–272. doi: 10.1016/j.anbehav.2016.03.004
Keywords: social, serotonin, visible burrow system, aggression, machine learning
Citation: Rivalan M, Alonso L, Mosienko V, Bey P, Hyde A, Bader M, Winter Y and Alenina N (2024) Serotonin drives aggression and social behaviors of laboratory male mice in a semi-natural environment. Front. Behav. Neurosci. 18:1450540. doi: 10.3389/fnbeh.2024.1450540
Received: 17 June 2024; Accepted: 29 August 2024;
Published: 18 September 2024.
Edited by:
Stefano Gaburro, Tecniplast, ItalyReviewed by:
Matthew Wanat, University of Texas at San Antonio, United StatesCopyright © 2024 Rivalan, Alonso, Mosienko, Bey, Hyde, Bader, Winter and Alenina. This is an open-access article distributed under the terms of the Creative Commons Attribution License (CC BY). The use, distribution or reproduction in other forums is permitted, provided the original author(s) and the copyright owner(s) are credited and that the original publication in this journal is cited, in accordance with accepted academic practice. No use, distribution or reproduction is permitted which does not comply with these terms.
*Correspondence: Marion Rivalan, bWFyaW9uLnJpdmFsYW5AY25ycy5mcg==; Natalia Alenina, YWxlbmluYUBtZGMtYmVybGluLmRl
†These authors have contributed equally to this work
‡ORCID: Marion Rivalan orcid.org/0000-0002-1642-7522
Natalia Alenina orcid.org/0000-0002-6071-5433
Disclaimer: All claims expressed in this article are solely those of the authors and do not necessarily represent those of their affiliated organizations, or those of the publisher, the editors and the reviewers. Any product that may be evaluated in this article or claim that may be made by its manufacturer is not guaranteed or endorsed by the publisher.
Research integrity at Frontiers
Learn more about the work of our research integrity team to safeguard the quality of each article we publish.