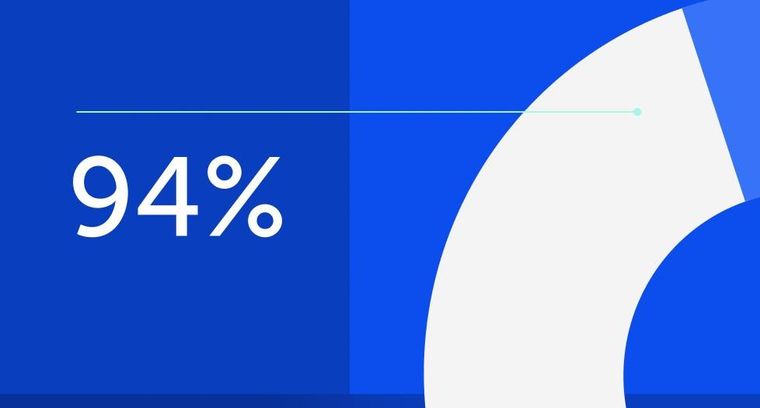
94% of researchers rate our articles as excellent or good
Learn more about the work of our research integrity team to safeguard the quality of each article we publish.
Find out more
EDITORIAL article
Front. Behav. Neurosci., 04 May 2023
Sec. Learning and Memory
Volume 17 - 2023 | https://doi.org/10.3389/fnbeh.2023.1205371
This article is part of the Research TopicUpdates on Memory Modulation in Health and DiseaseView all 5 articles
Editorial on the Research Topic
Updates on memory modulation in health and disease
One of the most intriguing questions in the memory field is how memory systems can be modulated in their nature and strength. By sculpting the contribution of different neuronal populations and target structures in the brain, neurotransmitters and neuromodulators are key players of high-order cognitive functions. So, which factors can modulate the memory process? The main objective of this issue is to approach this multifactorial question from a multidisciplinary perspective. Articles in this issue highlight general properties that make neuromodulatory systems crucial players in the behavioral neuroscience field and explain how different neuropathological conditions can alter these systems.
The long-term stabilization of information in the brain requires the reorganization of pre-existing networks. Hippocampal interneurons play a pivotal role in this matter, by controlling the size of the neural ensemble encoding new memories (Stefanelli et al., 2016). Indeed, it has been shown that inhibition of parvalbumin (Karunakaran et al., 2016; Xia et al., 2017), and somatostatin (Adler et al., 2019; Morales et al., 2021) interneurons in the hippocampus alters encoding of contextual memories. It is thought that neuromodulation shapes memory strength by configuring microcircuits and target structures that are recruited by an encoded event. Critically, hippocampal interneurons highly express acetylcholine (ACh) receptors (Morales et al., 2008; Son and Winzer-Serhan, 2008), suggesting a role of this neurotransmission system in the modulation of the hippocampal inhibitory activity. However, how Ach signaling mediates memory formation by modulating the excitatory/inhibitory balance in the hippocampus remains unclear. In this Issue, Goral et al. have provided evidence suggesting that the loss of GABA co-transmission from Ach-activatable interneurons alters spatial and contextual fear memories.
Spatial memory deficits are one of the most common cognitive symptoms in neurodegenerative disorders that selectively affect the medial-temporal lobe (MTL), such as Alzheimer's disease (AD) (Visser et al., 2002; Berron et al., 2020). Interestingly, AD disease leads to a bulk of neuromodulatory changes that impact over several neurotransmitter systems (Fahnestock et al., 2002; Rissman et al., 2007; Dinamarca et al., 2012; Revett et al., 2013; Kandimalla and Reddy, 2017; Wang et al., 2019; Chen et al., 2022). The hippocampal formation (HPC), one of the most affected MTL structures in AD, presents neurons tuned to fire at particular places in the environment (i.e., place cells) that are crucial for encoding spatial information (O'Keefe and Dostrovsky, 1971). Decoding accuracy and stability of hippocampal spatial representations is modulated by Ach (Sun et al., 2021) and NMDAR activity (Tonegawa et al., 1996; Cabral et al., 2014). Remapping, the process by which spatial hippocampal information stored in place cells is modified (Muller and Kubie, 1987; O'Keefe and Burgess, 1996; Leutgeb et al., 2005; Colgin et al., 2010), is thought to be an essential mechanism by which hippocampal formation maintains updated internal representations of changing environments. Hippocampal alteration in AD might lead to remapping impairments and potentially to the typical symptomatology of this disease. In this regard, the article by Silva and Martinez reviewed evidence pointing out the mapping and remapping disruption in the HPC as a possible circuit mechanism involved in deficits observed in AD. Moreover, they discuss how tau mediated changes in NMDA and AMPA receptors function in hippocampus-entorhinal cortex (HP-EC) region could contribute to these deficits.
Memories are susceptible to near-learning experiences that can change the internal state of an individual and influence memory strength (Moncada et al., 2011; Tyng et al., 2017; Tarder-Stoll et al., 2020). Neuromodulators are key players for experience-dependent changes in memory strength. For example, exercise and environmental enrichment, are interventions that are known to increase several neuromodulatory systems such as BDNF levels and lead to an increase in memory performance (Grech et al., 2018; Xu et al., 2021). Brain-Derived Neurotrophic Factor (BDNF) has been implicated in the formation and stabilization of the synapses (Cohen-Cory et al., 2010), and postulated as a marker of the occurrence/progression of many mnemonic symptoms that are common to different neuropathological conditions (Miranda et al., 2019). In addition, BDNF is also a key neuromodulator of the nociceptive response (Thompson et al., 1999; Pezet et al., 2002). This evidence suggests possible connections between nociceptive and memory systems. Indeed, a recent study elucidates the interaction between the concentration of this neurotrophic factor and tDCS-dependent alleviation of cognitive impairment observed in fibromyalgia (Dos Santos et al., 2018), a disease characterized by chronic neuropathic pain (Wood, 2007). In this issue, Caumo et al. describe a positive relationship between levels of BDNF and severity of cognitive impairment in subjects that responded to the conditioned pain modulation test, which is not seen in non-responders. These results open the possibility of the involvement of BDNF in moderating the effect of chronic pain on cognitive functions.
One of the most important questions in the study of memory is how individuals learn to avoid real or perceived dangers. Memories resulting from these experiences are of clinical interest as maladaptive memories are thought to be at the core of anxiety-related disorders observed in humans (Gazarini et al., 2023). To tackle this challenge, several animal models that mimic aspects of human aversive memories have been developed over the years. In this regard, fear conditioning has been the most widely used procedure to study the processing of emotional memories in rodents (LeDoux, 2000). However, due to the great complexity of rodent models, it is vitally important to study these processes using other animal models. Here, Pribadi and Chalasani review studies in invertebrates like Aplysia californica, Drosophila melanogaster, and Caenorhabditis elegans showing mechanisms underlying learning and memory processes conserved across these species. This review pays particular attention to predator-induced fear in these three organisms opening potential applications to more naturalistic trials.
In sum, this issue addresses the role of neuromodulatory transmission in shaping microcircuit memory encoding and explains how pathological conditions can impact memory function influencing several neurotransmitter systems, and crucially, how different animal models can help to understand these processes. Also, it discusses the development of naturalistic invertebrate animal models for studying learning and memory processes in maladaptive-memory formation.
JM and MM contributed substantially to the concept and design of the article, as well as making the original draft of the article. MG and NW revised it critically for important intellectual content. All authors contributed to the article and approved the submitted version.
This work was supported by the Fyssen Foundation (MM and JM), HFSP (MM), EMBO Postdoctoral Fellowship (JM), PICT 2018-1062, 2019-2580 (NW), and PICT-2021-I-INVI 00562 (MG).
The authors declare that the research was conducted in the absence of any commercial or financial relationships that could be construed as a potential conflict of interest.
All claims expressed in this article are solely those of the authors and do not necessarily represent those of their affiliated organizations, or those of the publisher, the editors and the reviewers. Any product that may be evaluated in this article, or claim that may be made by its manufacturer, is not guaranteed or endorsed by the publisher.
Adler, A., Zhao, R., Shin, M. E., Yasuda, R., and Gan, W. B. (2019). Somatostatin-Expressing Interneurons Enable and Maintain Learning-Dependent Sequential Activation of Pyramidal Neurons. Neuron. 102, 202–216.e7. doi: 10.1016/j.neuron.2019.01.036
Berron, D., van Westen, D., Ossenkoppele, R., Strandberg, O., and Hansson, O. (2020). Medial temporal lobe connectivity and its associations with cognition in early Alzheimer's disease. Brain 143, 1233–1248. doi: 10.1093/brain/awaa068
Cabral, H. O., Fouquet, C., Rondi-Reig, L., Pennartz, C. M. A., and Battaglia, F. P. (2014). Single-trial properties of place cells in control and CA1 NMDA receptor subunit 1-KO mice. J. Neurosci. 34, 15861–15869. doi: 10.1523/JNEUROSCI.5320-13.2014
Chen, Z.-. R, Huang, J-, B., Yang, S.-. L, and Hong, F-, F. (2022). Role of Cholinergic Signaling in Alzheimer's Disease. Molecules 27, 1816. doi: 10.3390/molecules27061816
Cohen-Cory, S., Kidane, A. H., Shirkey, N. J., and Marshak, S. (2010). Brain-derived neurotrophic factor and the development of structural neuronal connectivity. Dev. Neurobiol. 70, 271–288. doi: 10.1002/dneu.20774
Colgin, L. L., Leutgeb, S., Jezek, K., Leutgeb, J. K., Moser, E. I., McNaughton, B. L., et al. (2010). Attractor-map versus autoassociation based attractor dynamics in the hippocampal network. J. Neurophysiol. 104, 35–50. doi: 10.1152/jn.00202.2010
Dinamarca, M. C., Rios, J. A., and Inestrosa, N. C. (2012). Postsynaptic receptors for amyloid-beta oligomers as mediators of neuronal damage in Alzheimer's disease. Front. Physiol. 3, 464. doi: 10.3389/fphys.2012.00464
Dos Santos, V. S. D., Zortea, M., Alves, R. L., Naziazeno, C. C. D., Saldanha, J. S., Carvalho, d. e., et al. (2018). SdR, et al. Cognitive effects of transcranial direct current stimulation combined with working memory training in fibromyalgia: a randomized clinical trial. Sci. Rep. 8, 12477. doi: 10.1038/s41598-018-30127-z
Fahnestock, M., Garzon, D., Holsinger, R. M. D., and Michalski, B. (2002). Neurotrophic Factors and Alzheimer's Disease: Are We Focusing on the Wrong Molecule? Vienna: Springer. 241–52. doi: 10.1007/978-3-7091-6139-5_22
Gazarini, L., Stern, C. A., and Bertoglio, L. J. (2023). On making (and turning adaptive to) maladaptive aversive memories in laboratory rodents. Neurosci. Biobehav. Rev. 147, 105101. doi: 10.1016/j.neubiorev.2023.105101
Grech, A. M., Ratnayake, U., Hannan, A. J., van den Buuse, M., and Hill, R. A. (2018). Sex-Dependent Effects of Environmental Enrichment on Spatial Memory and Brain-Derived Neurotrophic Factor (BDNF) Signaling in a Developmental “Two-Hit” Mouse Model Combining BDNF Haploinsufficiency and Chronic Glucocorticoid Stimulation. Front. Behav. Neurosci. 12, 227. doi: 10.3389/fnbeh.2018.00227
Kandimalla, R., and Reddy, P. H. (2017). Therapeutics of Neurotransmitters in Alzheimer's Disease. J. Alzheimers Dis. 57, 1049–1069. doi: 10.3233/JAD-161118
Karunakaran, S., Chowdhury, A., Donato, F., Quairiaux, C., Michel, C. M., Caroni, P., et al. (2016). PV plasticity sustained through D1/5 dopamine signaling required for long-term memory consolidation. Nat. Neurosci. 19, 454–464. doi: 10.1038/nn.4231
LeDoux, J. E. (2000). Emotion circuits in the brain. Annu. Rev. Neurosci. 23, 155–184. doi: 10.1146/annurev.neuro.23.1.155
Leutgeb, S., Leutgeb, J. K., Barnes, C. A., Moser, E. I., McNaughton, B. L., Moser, M.-. B., et al. (2005). Independent codes for spatial and episodic memory in hippocampal neuronal ensembles. Science 309, 619–623. doi: 10.1126/science.1114037
Miranda, M., Morici, J. F., Zanoni, M. B., and Bekinschtein, P. (2019). Brain-Derived Neurotrophic Factor: A Key Molecule for Memory in the Healthy and the Pathological Brain. Front. Cell Neurosci. 13, 363. doi: 10.3389/fncel.2019.00363
Moncada, D., Ballarini, F., Martinez, M. C., Frey, J. U., and Viola, H. (2011). Identification of transmitter systems and learning tag molecules involved in behavioral tagging during memory formation. Proc. Natl. Acad. Sci. U S A. 108, 12931–12936. doi: 10.1073/pnas.1104495108
Morales, C., Morici, J. F., Espinosa, N., Sacson, A., Lara-Vasquez, A., García-Pérez, M. A., et al. (2021). Dentate Gyrus Somatostatin Cells are Required for Contextual Discrimination During Episodic Memory Encoding. Cereb Cortex 31, 1046–1059. doi: 10.1093/cercor/bhaa273
Morales, M., Hein, K., and Vogel, Z. (2008). Hippocampal interneurons co-express transcripts encoding the alpha7 nicotinic receptor subunit and the cannabinoid receptor 1. Neuroscience 152, 70–81. doi: 10.1016/j.neuroscience.2007.12.019
Muller, R. U., and Kubie, J. L. (1987). The effects of changes in the environment on the spatial firing of hippocampal complex-spike cells. J. Neurosci. 7, 1951–1968. doi: 10.1523/JNEUROSCI.07-07-01951.1987
O'Keefe, J., and Burgess, N. (1996). Geometric determinants of the place fields of hippocampal neurons. Nature 381, 425–428. doi: 10.1038/381425a0
O'Keefe, J., and Dostrovsky, J. (1971). The hippocampus as a spatial map. Preliminary evidence from unit activity in the freely-moving rat. Brain Res. 34, 171–175. doi: 10.1016/0006-8993(71)90358-1
Pezet, S., Malcangio, M., and McMahon, S. B. (2002). BDNF: a neuromodulator in nociceptive pathways? Brain Res. Rev. 40, 240–249. doi: 10.1016/S0165-0173(02)00206-0
Revett, T. J., Baker, G. B., Jhamandas, J., and Kar, S. (2013). Glutamate system, amyloid ss peptides and tau protein: functional interrelationships and relevance to Alzheimer disease pathology. J. Psychiatry Neurosci. 38, 6–23. doi: 10.1503/jpn.110190
Rissman, R. A., de Blas, A. L., and Armstrong, D. M. (2007). GABA(A) receptors in aging and Alzheimer's disease. J. Neurochem. 103, 1285–1292. doi: 10.1111/j.1471-4159.2007.04832.x
Son, J. H., and Winzer-Serhan, U. H. (2008). Expression of neuronal nicotinic acetylcholine receptor subunit mRNAs in rat hippocampal GABAergic interneurons. J. Comp. Neurol. 511, 286–299. doi: 10.1002/cne.21828
Stefanelli, T., Bertollini, C., Lüscher, C., Muller, D., and Mendez, P. (2016). Hippocampal somatostatin interneurons control the size of neuronal memory ensembles. Neuron 89, 1074–1085. doi: 10.1016/j.neuron.2016.01.024
Sun, D., Unnithan, R. R., and French, C. (2021). Scopolamine impairs spatial information recorded with “miniscope” calcium imaging in hippocampal place cells. Front. Neurosci. 15, 640350. doi: 10.3389/fnins.2021.640350
Tarder-Stoll, H., Jayakumar, M., Dimsdale-Zucker, H. R., Günseli, E., and Aly, M. (2020). Dynamic internal states shape memory retrieval. Neuropsychologia 138, 107328. doi: 10.1016/j.neuropsychologia.2019.107328
Thompson, S. W., Bennett, D. L., Kerr, B. J., Bradbury, E. J., and McMahon, S. B. (1999). Brain-derived neurotrophic factor is an endogenous modulator of nociceptive responses in the spinal cord. Proc. Natl. Acad. Sci. U S A. 96, 7714–7718. doi: 10.1073/pnas.96.14.7714
Tonegawa, S., Tsien, J. Z., McHugh, T. J., Huerta, P., Blum, K. I., Wilson, M. A., et al. (1996). Hippocampal CA1-region-restricted knockout of NMDAR1 gene disrupts synaptic plasticity, place fields, and spatial learning. Cold Spring Harb. Symp. Quant. Biol. 61, 225–238. doi: 10.1101/SQB.1996.061.01.025
Tyng, C. M., Amin, H. U., Saad, M. N. M., and Malik, A. S. (2017). The influences of emotion on learning and memory. Front. Psychol. 8, 1454. doi: 10.3389/fpsyg.2017.01454
Visser, P. J., Verhey, F. R. J., Hofman, P. A. M., Scheltens, P., and Jolles, J. (2002). Medial temporal lobe atrophy predicts Alzheimer's disease in patients with minor cognitive impairment. J. Neurol. Neurosurg. Psychiat. 72, 491–497.
Wang, K.-. W, Ye, X-, L., Huang, T., Yang, X.-. F, and Zou, L-, Y. (2019). Optogenetics-induced activation of glutamate receptors improves memory function in mice with Alzheimer's disease. Neural Regen. Res. 14, 2147–2155. doi: 10.4103/1673-5374.262593
Wood, P. B. (2007). Fibromyalgia. Encyclopedia of Stress. Second Edition. 56–62. doi: 10.1016/B978-012373947-6.00494-3
Xia, F., Richards, B. A., Tran, M. M., Josselyn, S. A., Takehara-Nishiuchi, K., Frankland, P. W., et al. (2017). Parvalbumin-positive interneurons mediate neocortical-hippocampal interactions that are necessary for memory consolidation. Elife 6, 20. doi: 10.7554/eLife.27868.020
Keywords: memory, neuromodulation, neurodegeneration, neurotransmission dysfunction, memory impairment
Citation: Miranda M, Giachero M, Weisstaub NV and Morici JF (2023) Editorial: Updates on memory modulation in health and disease. Front. Behav. Neurosci. 17:1205371. doi: 10.3389/fnbeh.2023.1205371
Received: 13 April 2023; Accepted: 17 April 2023;
Published: 04 May 2023.
Edited and reviewed by: Denise Manahan-Vaughan, Ruhr University Bochum, Germany
Copyright © 2023 Miranda, Giachero, Weisstaub and Morici. This is an open-access article distributed under the terms of the Creative Commons Attribution License (CC BY). The use, distribution or reproduction in other forums is permitted, provided the original author(s) and the copyright owner(s) are credited and that the original publication in this journal is cited, in accordance with accepted academic practice. No use, distribution or reproduction is permitted which does not comply with these terms.
*Correspondence: Magdalena Miranda, bWlyYW5kYS5tYWdkYWxlbmEubGJAZ21haWwuY29t; Juan Facundo Morici, ZmFxLm1vcmljaUBnbWFpbC5jb20=
Disclaimer: All claims expressed in this article are solely those of the authors and do not necessarily represent those of their affiliated organizations, or those of the publisher, the editors and the reviewers. Any product that may be evaluated in this article or claim that may be made by its manufacturer is not guaranteed or endorsed by the publisher.
Research integrity at Frontiers
Learn more about the work of our research integrity team to safeguard the quality of each article we publish.