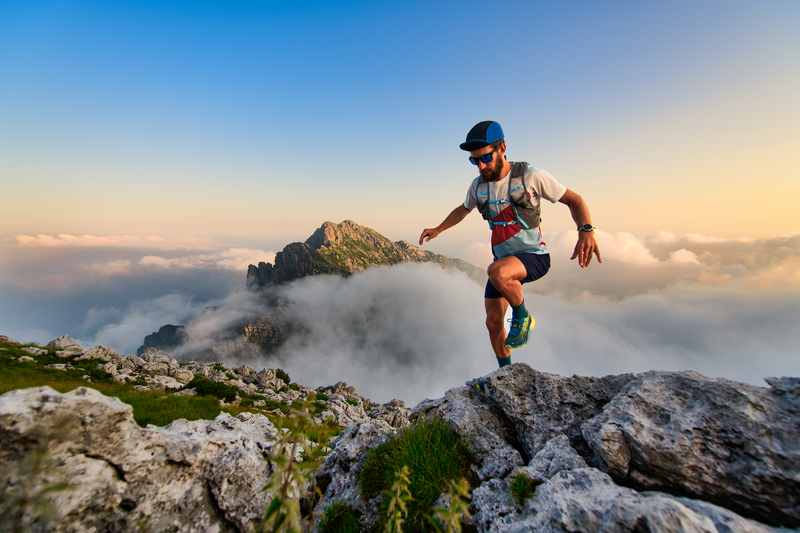
95% of researchers rate our articles as excellent or good
Learn more about the work of our research integrity team to safeguard the quality of each article we publish.
Find out more
BRIEF RESEARCH REPORT article
Front. Behav. Neurosci. , 07 July 2022
Sec. Emotion Regulation and Processing
Volume 16 - 2022 | https://doi.org/10.3389/fnbeh.2022.919831
This article is part of the Research Topic Noradrenergic Modulation of (Mal)Adaptive Behavior View all 4 articles
Altered fear learning is a strong behavioral component of anxiety disorders such as post-traumatic stress disorder (PTSD). Recent efforts have attempted to combine exposure therapies with drugs that target fear memory retrieval and memory reconsolidation, in order to improve treatment efficacy. The noradrenergic (NA) signaling system is of particular interest, due to its role in regulating the stress response and its involvement in fear and learning processes. Importantly, propranolol (P), a non-selective β-adrenergic antagonist, has shown the potential in decreasing exaggerated fear in both humans and animal models. In a previous study, we utilized an activity-dependent tagging murine model to determine the neural mechanisms by which propranolol attenuates learned fear. We found that propranolol acutely decreased memory trace reactivation specifically in the dorsal dentate gyrus (dDG), but not in CA3 or CA1. Here, we extended our previous study by investigating whether propranolol additionally altered activity in the hilus, a polymorphic layer that consists of neurons, mossy cells, and GABAergic interneurons. We found that propranolol acutely reduced overall hilar activity in both the dorsal and ventral hilus. Moreover, we report that propranolol significantly altered the activity of parvalbumin (PV)+ cells in the ventral (vDG), but not dorsal DG (dDG). Together, these results suggest that a β-adrenergic blockade may affect the activity of excitatory and inhibitory cell types in the hilar layer of the DG, and that these alterations may contribute to manipulating fear memory traces.
Post-traumatic stress disorder (PTSD) and other anxiety disorders such as specific phobias, often include abnormally strong fear responses that are resistant to therapeutic approaches with exposure therapies (Rauch et al., 2012; Botella et al., 2017). Numerous efforts have been made to couple exposure therapies for PTSD with pharmacological manipulations to successfully manage exaggerated fear responses (Taylor et al., 2008; Belkin and Schwartz, 2015; Brunet et al., 2018). While research is still ongoing, some results suggest that this combinatorial approach may result in effective, positive clinical outcomes for managing fear and anxiety disorders.
One of the targets of this approach is the noradrenergic system, which is known to be involved in vigilance, attention, threat response, and in learning and memory (Berridge and Waterhouse, 2003; Berridge, 2005; Avery and Krichmar, 2017). The main source of noradrenergic innervation in the brain is the locus coeruleus (LC), a brain nucleus in the pons of the brain stem, and part of the reticular ascending system (Berridge and Waterhouse, 2003; Schwarz and Luo, 2015; Aston-Jones and Waterhouse, 2016). Drugs that manipulate adrenergic signaling have been used to manipulate either the reactivation, the reconsolidation, and/or the extinction processes involved in the weakening of a fearful memory (Taylor et al., 2008; Lonergan et al., 2013; McGuire et al., 2014; Belkin and Schwartz, 2015). The non-selective β-adrenergic antagonist propranolol (P), in particular, has been used for several decades in anxiety disorders including performance anxiety (Tyrer, 1988; Liu et al., 1991; Soeter and Kindt, 2015) and generalized anxiety disorder (GAD) (Fagerström et al., 1985; Tyrer, 1988; Grillon et al., 2004). Propranolol has also been used to aid in the treatment of phobias and in exposure therapies for PTSD (Grillon et al., 2004; Soeter and Kindt, 2015; Kroes et al., 2016).
To better understand the mechanism of action of propranolol, it is critical to test the drug’s effects in different behavioral situations, with varied conditioned stimuli and administration timelines, while analyzing the underlying brain activity. Of particular interest are several regions that are involved in the learning of fearful associative memories, and that also receive abundant noradrenergic projections from the LC. These include the hippocampal formation (HPC), the medial prefrontal cortical areas prelimbic (PL) and infralimbic areas (ILA), and several amygdalar nuclei, the most studied of which are the basolateral (BLA) and the lateral (LA) amygdalar nuclei, all of which have been shown to be affected by propranolol administration in humans (Hurlemann et al., 2010; Schwabe et al., 2012; Kroes et al., 2016) and in animal models (Ji et al., 2003; Chen and Sara, 2007; Do Monte et al., 2008; Qi et al., 2008; Ehrlich et al., 2009; Rodriguez-Romaguera et al., 2009; Vetere et al., 2013; Fitzgerald et al., 2015; Hagena et al., 2016; Giustino et al., 2017).
Engrams or memory traces were conceptualized by Richard Semon in the early twentieth century (Semon, 1921) and refer to the change in biological matter that occurs with learning, that is maintained, and whose reactivation leads to retrieval of the memory. In the past decade, numerous studies have utilized the expression of immediate early genes (IEGs), which undergo an increase in transcription when a neuron has increased activity, as a proxy for engrams (Tonegawa et al., 2015). By utilizing IEG promoters in inducible, transgenic systems, it became possible to tag a population of neurons active during an individual memory. These recent genetic techniques, paired with optogenetics, have allowed scientists to identify and manipulate engrams (Liu et al., 2012; Denny et al., 2014; Redondo et al., 2014; Ramirez et al., 2015; Roy et al., 2016; Perusini et al., 2017; Khalaf et al., 2018; Chen et al., 2019; Lacagnina et al., 2019), and even may be modified to improve mood and cognition (Reijmers et al., 2007; Denny et al., 2017).
One of these IEGs Arc/Arg3.1 has been widely implicated in synaptic plasticity (Link et al., 1995; Lyford et al., 1995; Pastuzyn et al., 2018). We previously created the ArcCreERT2 x enhanced yellow fluorescent protein (eYFP) mice, which allow for the permanent labeling of activated Arc+ neurons (Denny et al., 2014). Using this transgenic murine line, we have shown that the HPC subregions dentate gyrus (DG) and cornus ammonis 3 (CA3) encode memory traces of contextual fear memories (Denny et al., 2014). This work showed that, for both the DG and CA3: (1) the number of tagged cells was greater in mice following CFC compared to mice that underwent exposure to the context with no shock; (2) that the percentage of reactivated cells was higher when mice were re-exposed to the CFC context, compared to mice exposed to a different context; and (3) that the neuronal ensembles active upon encoding of contextual fear conditioning (CFC) memories are necessary for memory retrieval (Denny et al., 2014; Cazzulino et al., 2016; Mastrodonato et al., 2018; Lacagnina et al., 2019). In a more recent study (Leal Santos et al., 2021), we utilized the ArcCreERT2 mouse model to study the effects of propranolol in the DG, CA3, and CA1, in prefrontal cortical areas, and in several amygdalar nuclei. We found that propranolol administered immediately before fear retrieval decreased fear expression, which was paralleled by a decreased reactivation of the fearful memory trace specifically in the dorsal DG (dDG).
Here, we sought to further our prior study and to investigate how propranolol administration impacts activity in the hilus. The hilus is the layer of the DG that receives the highest density of afferents of cholinergic, serotoninergic, and, importantly, noradrenergic fibers (Pickel et al., 1974; Swanson and Hartman, 1975), suggesting an important role in mediating how these stimuli affect the activity of the granule cells. Using the ArcCreERT2 x eYFP mice to tag fear memory traces, we report that propranolol administration significantly reduced c-Fos expression in both the dorsal and ventral hilus. Propranolol did not alter the expression of either somatostatin (SST) or parvalbumin (PV), markers of inhibitory interneurons. Propranolol administration increased the activity of PV-expressing cells in the ventral hilus. Together, these results suggest that a β-adrenergic blockade may affect the activity of excitatory and inhibitory cell types in the hilar layer of the DG and that these alterations may contribute to reducing fear behavior.
ArcCreERT2(+) (Denny et al., 2014) × R26R-STOP-floxed-enhanced yellow fluorescent protein (eYFP) (Srinivas et al., 2001) homozygous female mice were bred, reared, and tested as previously described (Leal Santos et al., 2021). Food and water were provided ad libitum. All experiments were approved by the Institutional Animal Care and Use Committee (IACUC) at the New York State Psychiatric Institute (NYSPI).
4-Hydroxytamoxifen (4-OHT) (Sigma, St. Louis, MO, H7904) was used to induce recombination, as previously described (Cazzulino et al., 2016). 4-OHT was dissolved by sonication in 10% EtOH/90% corn oil at a concentration of 10 mg/ml. One injection of 200 μl (2 mg) was administered intraperitoneally (i.p.) into each adult mouse.
(+)-Propranolol hydrochloride was dissolved in 0.9% NaCl at a concentration of 1 mg/ml. A single injection of saline (Sal) (0.9% NaCl) or propranolol hydrochloride (P) [(+)-Propranolol hydrochloride, Sigma Aldrich, #PHR1308] (10 mg/kg) was administered i.p. once immediately before re-exposure to the CFC context. Dosing was based on previous studies, indicating that 10 mg/kg of propranolol was effective at decreasing fear expression acutely or in subsequent re-exposures in mice (Do Monte et al., 2008; Ehrlich et al., 2009; Do-Monte et al., 2010; Muravieva and Alberini, 2010; Fitzgerald et al., 2015; Giustino et al., 2017).
A 4-shock CFC paradigm and context re-exposure (RE) was administered as previously described (Leal Santos et al., 2021).
Mice were deeply anesthetized, and perfusions with 4% paraformaldehyde (PFA) and brain processing were performed as previously described in Denny et al. (2014), Cazzulino et al. (2016), Pavlova et al. (2018), and Leal Santos et al. (2021).
An iDISCO-based immunohistochemistry protocol was performed as previously described (Pavlova et al., 2018; Leal Santos et al., 2021). All antibodies and corresponding information are listed in Supplementary Table 1.
All samples were imaged on a confocal scanning microscope (Leica TCS SP8, Leica Microsystems Inc., Wetzlar, Germany) with 2 PMT detectors, as previously described (Leal Santos et al., 2021). Sections were imaged with a dry Leica 20 × objective (NA 0.70, working distance 0.5 mm), with a pixel size of 1.08 × 1.08 μm2, a z step of 3 μm, and z-stack of 27 μm. Fields of view were stitched together to form tiled images by using an automated stage and the tiling function and algorithm of the LAS X software.
An investigator blind to treatment counted eYFP+, c-Fos+, SST, or PV immunoreactive cells bilaterally in the hilus (also called polymorphous layer of the DG) of the hippocampus. Cells were counted bilaterally using Fiji (Schindelin et al., 2012). A minimum of 3 hemi-sections were analyzed per mouse per dorsal or ventral hilus. The limits of the hilus were defined using the coronal Allen Brain Atlas as a reference. Cell counts were normalized to the volume of hilus for each mouse. Normalized levels per mm3 and the reactivation levels (percentage of co-labeled cells) are presented throughout.
All data were analyzed using Prism 9.0.0. Alpha was set to 0.05 for all analyses. Normality was tested using the D’Agostino–Pearson test. As normality was present, the effect of drug on average levels of immunolabeled cells was analyzed using t-tests. Pearson correlations between levels of activity in the hilus and freezing levels were calculated.
In our previous study, we reported differences in dDG memory trace reactivation following propranolol administration (Leal Santos et al., 2021). Importantly, we quantified cells active during fear encoding and fear memory retrieval in the granule cell layer (GCL) of the dDG, but we did not analyze other layers of the DG, such as the polymorphous layer (PML), which has also been referred to as CA4 or the hilus (De Nó, 1934). Although most excitatory cells in the DG are localized in the GCL, the hilus harbors important excitatory cells (Scharfman and Myers, 2012; Scharfman, 2016) and several populations of inhibitory interneurons (Amaral et al., 2007; Scharfman, 2016). Most notably, the hilus receives the highest density of afferents of cholinergic, serotoninergic, and, importantly, noradrenergic fibers (Pickel et al., 1974; Swanson and Hartman, 1975) and is the layer with the highest expression of β-adrenergic receptors (Cox et al., 2008).
To determine how propranolol alters neural activity in the hilus, ArcCreERT2 x eYFP mice (Figure 1A) were injected with 4-OHT 5 h prior to 4-shock CFC (Figure 1B) to tag cells with eYFP. Five days later, mice were administered an injection of saline or propranolol prior to context re-exposure (RE) and euthanized 1 h following context RE to capture cells expressing c-Fos or Arc protein. As previously reported, propranolol administration significantly decreased fear expression during context RE when compared with saline administration (Leal Santos et al., 2021). The transgenic model allowed for brain-wide labeling of eYFP+ cells active during fear encoding (Figures 1C–F).
Figure 1. Propranolol administration decreases hilar neural activity in the dorsal hippocampus. (A) Genetic design. (B) Experimental design. 4-OHT was administered 5 h before CFC for neural tagging. Five days later, mice were given an injection of saline or propranolol. Immediately following the injection, mice were re-exposed the fear context and euthanized 1 h later. (C) Representative section showing eYFP (green) and c-Fos (red) expression throughout the brain in the ArcCreERT2 × eYFP mice. (D) eYFP+, (E) c-Fos+, and (F) co-labeled cells in the dorsal hilus. Representative hilar sections are outlined in white. (G) eYFP+, (H) c-Fos+, (I) co-labeled/eYFP (%), and (J) co-labeled/c-Fos+ (%) hilar cell counts. Correlation plots of average freezing vs. (K) c-Fos+ cells, (L) co-labeled/eYFP+ (%), and (M) co-labeled/c-Fos+ (%). Error bars indicate ± SEM. *p < 0.05, **p < 0.01, ***p < 0.0001. eYFP, enhanced yellow fluorescent protein; 4-OHT, 4-hydroxytamoxifen; CFC, contextual fear conditioning; RE, context re-exposure; sac, sacrifice; Sal, saline; P, propranolol.
We first sought to determine whether administration of propranolol alters activity in the dorsal hilus. We found that expression of hilar eYFP+ cells did not differ between the saline and propranolol mice [t(16) = 0.06094, p = 0.9522] (Figure 1G), as expected since eYFP+ cells were tagged during CFC and before drug administration. Interestingly, the number of hilar c-Fos+ cells was significantly less in propranolol-injected mice when compared with saline-injected mice [t(16) = 2.311, p = 0.0345] (Figure 1H). Notably, this difference was not previously observed in the GCL of the dDG (Leal Santos et al., 2021).
When analyzing hilar memory trace reactivation, propranolol-injected mice administered had a trending, but not significant decrease in co-labeled/eYFP+ (%) of cells when compared with saline-injected mice [t(15) = 2.076, p = 0.0555] (Figure 1I). No difference was observed between the groups in the percentage of co-labeled/c-Fos+ cells [t(16) = 1.654, p = 0.1175] (Figure 1J). The lack of an effect in this metric may be due to the c-Fos+ cells being more variable than the eYFP+ population, encompassing not only excitatory cells but also inhibitory interneurons (Staiger et al., 2002; Tyssowski et al., 2018).
Next, we performed correlation analyses between the levels of hilar c-Fos expression and freezing levels, and between the percentage of hilar memory trace reactivation and freezing (Figures 1K–M). No correlation was found between the numbers of c-Fos+ cells and freezing levels for both groups (Figure 1K). When analyzing the correlation between hilar memory trace reactivation and freezing, we found no correlation between the percentage of co-labeled cells and freezing for both groups (Figures 1L,M). These data indicate that hilar memory trace activity does not directly reflect the behavioral levels of fear expression.
We next sought to determine whether administration of propranolol alters activity in the ventral hilus (Figures 2A–D). We found that expression of hilar eYFP+ cells did not differ between the groups [t(16) = 1.965, p = 0.067] (Figure 2E). Interestingly, the number of hilar c-Fos+ cells was significantly lower in propranolol-injected mice when compared with saline-injected mice [t(16) = 4.336, p = 0.0005] (Figure 2F), as was seen in the dorsal hilus.
Figure 2. Propranolol administration decreases hilar neural activity in the ventral hippocampus. (A) Representative section showing eYFP (green) and c-Fos (red) expression throughout the brain in the ArcCreERT2 × eYFP mice. (B) eYFP+, (C) c-Fos+, and (D) co-labeled cells in the ventral hilus. Representative hilar sections are outlined in white. (E) eYFP+, (F) c-Fos+, (G) co-labeled/eYFP (%), and (H) co-labeled/c-Fos+ (%) hilar cell counts in the ventral hilus. Correlation plots of average freezing versus (I) c-Fos+ cells, (J) co-labeled/eYFP+ (%), and (K) co-labeled/c-Fos+ (%). Error bars indicate ± SEM. *p < 0.05, **p < 0.01, ***p < 0.0001. eYFP, enhanced yellow fluorescent protein; Sal, saline; P, propranolol; ns, non significant.
When analyzing hilar memory trace reactivation, propranolol-injected mice administered had comparable percentages of co-labeled/eYFP+ [t(16) = 1.911, p = 0.0742] (Figure 2G) and co-labeled/c-Fos+ [t(16) = 0.1253, p = 0.9019] (Figure 2H) when compared with saline-injected mice. Overall, these data suggest that ventral memory trace reactivation in the hilus is not altered following propranolol administration.
Finally, we performed correlation analyses between the levels of hilar c-Fos expression and freezing levels, and between the percentage of hilar memory trace reactivation and freezing (Figures 2I–K). No correlation was found between the numbers of c-Fos+ cells and freezing levels for mice administered either saline or propranolol (Figure 2I). When analyzing the correlation between hilar memory trace reactivation and freezing, we found no correlation between the percentage of co-labeled cells and freezing, for either the saline- or propranolol-injected mice (Figures 2J,K). These data indicate that hilar activity does not directly reflect the behavioral levels of fear expression, and this is not altered by propranolol administration.
We then hypothesized that propranolol could reduce dorsal hilar activity by enhancing the activity or expression of inhibitory interneurons. We next quantified the expression of somatostatin (SST) in the dorsal and ventral hilus (Supplementary Figures 1A,B). SST was chosen due to its demonstrated role in regulating long-term plasticity and hippocampal-dependent learning and memory, as well as its high co-expression with β-adrenergic receptors (Cox et al., 2008; Honoré et al., 2021). SST density was comparable between both groups in both the dorsal [t(16) = 1.223, p = 0.2389] (Supplementary Figure 1C) and ventral [t(16) = 0.0189, p = 0.9852] (Supplementary Figure 1E) hilus, indicating that propranolol administration does not significantly affect hilar SST expression.
We next performed correlation analyses between the levels of hilar SST expression and freezing levels (Supplementary Figures 1D,F). In the dorsal hilus, there was a positive correlation between freezing during RE and SST expression in propranolol-, but not saline-administered mice (Supplementary Figure 1C). Similarly, in the ventral hilus, there was a positive correlation between freezing during RE and SST in propranolol-, but not saline-injected mice (Supplementary Figure 1F). Together, these results suggest that although propranolol does not alter hilar SST expression, levels of SST+ interneurons may positively reflect the behavioral levels of fear expression in propranolol-administered mice.
Next, we quantified hilar expression of parvalbumin (PV), another marker of inhibitory interneurons (Figures 3A–H). PV-expressing interneurons are GABAergic cells that mediate synchronous neural activity to promote memory consolidation, and their expression and activity can be significantly altered by stress or mood disorders (Ognjanovski et al., 2017; Chen et al., 2018; Perlman et al., 2021). PV is also co-expressed with β-adrenergic receptors in the hilus (Cox et al., 2008). As we previously demonstrated, in the dorsal hilus, propranolol significantly reduced c-Fos expression [t(16) = 3.179, p = 0.0058], but did not alter the expression of eYFP [t(16) = 0.3359, p = 0.7413] (Figures 3I,J). PV expression was comparable between both groups [t(16) = 0.0587, p = 0.9539] (Figure 3K). There was no significant difference in eYFP+PV+/PV+ (%) [t(16) = 0.597, p = 0.5589] or c-Fos+PV+/PV+ (%) [t(16) = 0.045, p = 0.9647] of cells between both groups (Figures 3L,M). These data suggest that propranolol reduces cellular activity in the dorsal hilus during re-exposure in a PV-independent manner.
Figure 3. Propranolol administration increases the activity of parvalbumin-expressing cells in the ventral, but not dorsal hilus. (A) Representative section showing eYFP (green), c-Fos (red), and PV (blue) expression in the dorsal hippocampus. A representative hilar section is outlined in white. (B) eYFP+, (C) c-Fos+, and (D) PV+ cells in the dorsal hilus. (E) Representative section showing eYFP (green), c-Fos (red), and PV (blue) expression in the ventral hippocampus. A representative hilar section is outlined in white. (F) eYFP+, (G) c-Fos+, (H) PV+ cells in the ventral hilus. (I) eYFP+, (J) c-Fos+, (K) PV+, (L) eYFP+PV+/PV+ (%), and (M) c-Fos+PV+/PV+ (%) cells in the dorsal hilus. (N) eYFP+, (O) c-Fos+, (P) PV+, (Q) eYFP+PV+/PV+ (%), and (R) c-Fos+PV+/PV+ (%) cells in the ventral hilus. Correlation plots of average freezing vs. (S) c-Fos+ cells in the dorsal hilus, (T) c-Fos+ cells in the ventral hilus, (U) c-Fos+PV+/PV+ cells (%) in the dorsal hilus, and (V) c-Fos+PV+/PV+ cells (%) in the ventral hilus. Error bars indicate ± SEM. *p < 0.05, **p < 0.01, ***p < 0.0001. eYFP, enhanced yellow fluorescent protein; PV, parvalbumin; Sal, saline; P, propranolol.
In the ventral hilus, propranolol administration did not significantly alter eYFP expression [t(16) = 0.5123, p = 0.6154] (Figure 3N). As we previously demonstrated, propranolol significantly decreased c-Fos expression [t(16) = 0.3.247, p = 0.0051], but not PV expression [t(16) = 0.9995, p = 0.3324] (Figures 3O,P). eYFP+PV+/PV+ (%) [t(16) = 0.835, p = 0.416] of cells was comparable between saline- and propranolol-injected mice (Figure 3Q). Surprisingly, propranolol significantly increased the percentage of c-Fos+PV+/PV+ cells in the ventral hilus [t(16) = 3.418, p = 0.0035] (Figure 3R). Together, these data suggest that in the ventral hilus, propranolol increases the proportion of PV-expressing cells that are active during re-exposure. These findings are in line with previous data suggesting that increased inhibitory activity in vDG reduces stress-induced anxiety-like behaviors (Anacker et al., 2018).
Finally, we performed correlation analyses as described above (Figures 3S–V). c-Fos expression was not significantly correlated with freezing during RE in both the dorsal and ventral hilus, as previously demonstrated (Figures 3S,T). In the dorsal hilus, fear behavior was not correlated with c-Fos+PV+/PV+ (%) of cells (Figure 3U). In the ventral hilus, freezing was negatively correlated with the percentage of activated PV+ cells in saline-, but not propranolol-administered mice (Figure 3V). Together, these results suggest that the percentage of activated PV+ cells in the ventral hilus is negatively correlated with freezing behavior in controls, but that propranolol alters this behavioral correlation.
Here, we investigated how propranolol impacts activity in the hilus when administered prior to re-exposure to an aversive CFC context. We utilized the ArcCreERT2 x eYFP mice to allow tagging of the CFC encoding cells and to quantify reactivated cells following fear retrieval. We then examined the effect of acute administration of propranolol on SST and PV, two markers of inhibitory interneurons. We show that propranolol acutely: (1) decreases neural activity in the dorsal and ventral hilus, (2) alters the correlation between the activity of PV-expressing interneurons and freezing behavior, and (3) increases the activity of PV-expressing interneurons in the ventral, but not dorsal hilus.
We previously showed that propranolol decreased fear expression and decreased memory trace reactivation specifically in the dDG (Leal Santos et al., 2021). Studies of hippocampal connectivity have shown that information in the hippocampus flows mostly unidirectionally (Amaral et al., 2007; Bienkowski et al., 2018), with the DG being the entryway, and the hilus is the layer of the DG that receives the highest noradrenergic input (Pickel et al., 1974; Swanson and Hartman, 1975) and has discrete populations of cells expressing β-adrenergic receptors (Cox et al., 2008). These characteristics place the hilus as the layer most likely to be directly altered by propranolol, possibly relaying these effects to the GCL. Both β1- and β2-adrenoreceptors in the HPC have been extensively implicated in the modulation of memory formation (Qi et al., 2008). In rodents, β1- and β2-adrenoreceptors are expressed throughout the HPC, with higher levels of expression in the DG and lower levels of both receptor subtypes in CA3. These receptors are also expressed in interneurons and, to a lesser extent, in glial cells (Booze et al., 1993; Milner et al., 2000; Guo and Li, 2007; Cox et al., 2008). Here, we provide another layer of evidence of preferential noradrenergic modulation of the dDG upon retrieval of a contextual fear memory and show that β-adrenergic inhibition can decrease overall cellular activity in the hilus.
We explored the relation between either hilar activity and freezing during re-exposure, and found no correlation between these measures, suggesting that hilar activity alone cannot explain the behavioral effect of propranolol. In our paradigm, we previously reported a correlation between freezing and memory trace reactivation in the anterior cingulate area and in the lateral amygdala, but not in the GCL of the DG (Leal Santos et al., 2021). Memory trace reactivation in the DG has been shown to correlate with fear expression (Khalaf et al., 2018); however, the tagging systems and timelines differed between these studies, which might explain differences in engagement of the DG memory trace. Future analyses that encompass alterations across several regions and timepoints may yield a better understanding of network wide changes and of nodes that can more crucially impact the behavioral outcome (Vetere et al., 2017; Khalaf et al., 2018; Khalaf and Gräff, 2019; Leal Santos et al., 2021).
Here, we also combined our activity-based analysis with labeling for interneuron types (Houser, 2007; Cox et al., 2008), which can also be components of the memory trace (Trouche et al., 2013), in order to determine: (1) whether the change in overall activity was due to the excitatory or the inhibitory cell populations, or both, and (2) whether there is an inhibitory component of the memory trace in the hilus. Indeed, previous data indicate that modulation of noradrenergic inputs to the DG, resulting in altered contextual fear discrimination, can modulate the signaling of local interneuron populations (Seo et al., 2021). Here, we report that in propranolol-, but not saline-administered mice, there was a significant positive correlation between SST expression and freezing behavior, suggesting that the expression of SST interneurons could reflect behavioral output during fear memory retrieval. It has previously been demonstrated that SST is an important neuromodulator of hippocampal function at the behavioral level and that local hippocampal injections of SST can exert anxiolytic effects (Honoré et al., 2021). Additionally, we showed that propranolol administration can alter PV-expressing interneuron activity in the ventral, but not dorsal hilus. PV+ interneurons, particularly those localized in the hilar layer of the DG, exert fast-spiking inhibitory connections that are speculated to provide rapid suppression and to enable efficient higher-order network functions (Espinoza et al., 2018). Thus, by increasing the activity of inhibitory PV+ cellular input in the ventral hilus, propranolol may enable more efficient and powerful suppression of neuronal activity in the DG, thereby reducing the negative valence of the fear memory engram and suppressing freezing behavior. However, further study is necessary to fully characterize how propranolol alters the inhibitory component of a memory engram during memory retrieval.
Because PV+ interneurons express β-adrenergic receptors, it was unexpected that administration of propranolol, a β-adrenergic antagonist, would increase the percentage of cFos+PV+/PV+ cells during fear retrieval. It is known that within the hilus, different interneuron subtypes are interconnected and regulate each other’s activity (Sik et al., 1997). Therefore, whichever subpopulation of interneurons is more susceptible to propranolol may have a decrease in firing and subsequently disinhibit downstream interneurons, and this effect may predominate over the direct effect of the β-adrenergic antagonist to the latter interneurons. As quantified in Cox et al. (2008), PV+ interneurons are third to SOM+ neurons and then to NYP neurons in expression of β-adrenergic receptors. It is possible, therefore, that other populations of hilar interneurons undergo stronger inhibition by propranolol and disinhibit PV+ interneurons. Alternatively, although propranolol is a potent non-selective β-adrenergic antagonist, previous studies have shown that it may have partial agonist activity, leading to the activation of ERK pathways in β-adrenergic receptor-expressing cells (Azzi et al., 2003; Baker et al., 2003; Galandrin and Bouvier, 2006). Thus, although propranolol broadly acts to suppress the activity of β-adrenergic cells, in some cases, it may lead to cellular excitation due to activation of distinct GPCR signaling effectors. We speculate that this paradoxical upregulation of an intracellular signaling cascade may lead to increased activity of β-adrenergic receptor-expressing PV+ interneurons in the ventral hilus.
Another major cell type in the hilus is the mossy cell, which has unique structural and physiological properties. Mossy cells are glutamatergic neurons that have intrinsic and circuit properties that are thought to make them modulators of the activity of granule cells. Deletion of mossy cells leads to a transient increase in excitability and impaired contextual discrimination, and optogenetic activation of mossy cells primarily inhibits granule cells, suggesting that mossy cells primarily activate GABAergic interneurons that inhibit granule cells (as reviewed in Scharfman and Myers, 2012; Scharfman, 2016). c-Fos expression has been shown in mossy cells at baseline and under acute restraint stress (Duffy et al., 2013; Moretto et al., 2017). Although here we have initially focused on dissecting the activity of interneuron subtypes in the hilus, for a more complete understanding of how DG activity is affected by β-adrenergic modulation during fear retrieval, further studies will address how mossy cell activity is affected during β-adrenergic blockade and how the balance of inhibition and excitation between mossy cells and hilar interneurons modulate granule cell activity.
The hippocampal subfields display heterogeneity along their dorso-ventral axis in terms of gene expression, connectivity, and function (Moser and Moser, 1998; Bannerman et al., 2004; Fanselow and Dong, 2010; Kheirbek et al., 2013; Bienkowski et al., 2018). The dorsal HPC is necessary for learning and memory associated with spatial navigation (Moser et al., 1995; Bannerman et al., 1999; Leal Santos et al., 2021), whereas the ventral HPC is associated with innate fear and anxiety (Richmond et al., 1999; Kjelstrup et al., 2002). The dorsal HPC receives projections from sensory cortical areas and projects to associational cortical areas, while the vHPC is bidirectionally connected to regions such as the prefrontal cortex, amygdala and hypothalamus, implicated in the processing of emotional stimuli (Fanselow and Dong, 2010; Bienkowski et al., 2018). Interestingly, earlier we only reported an effect of propranolol in the dDG. However, here we report similar effects observed in the dorsal and ventral hilus for c-Fos, suggesting that propranolol may differentially impact hippocampal subregions and ultimately, fear behavior. However, in the ventral hilus, increased activity of a PV-expressing inhibitory population of interneurons could suppress the emotional valence of fear, perhaps by suppressing downstream excitatory projections to regions such as the basolateral amygdala (BLA) and prefrontal cortex (PFC). These combined effects could contribute to a significant overall reduction in freezing behavior in propranolol-administered mice at the time of memory retrieval.
In both human and animal studies that investigate how to improve propranolol’s efficacy and the neural mechanisms that mediate it (Fitzgerald et al., 2015; Giustino et al., 2016; Giustino and Maren, 2018), there has been difficulty establishing the boundary conditions in terms of timing, dosing, and stimulation that lead to a successful therapeutic outcome (Schroyens et al., 2017). Here, we show that, under certain conditions, propranolol’s effect on fear behavior is correlated with changes not only in the dDG, but now throughout the hilus. These data favor the use of propranolol for acute symptomatic relief. Where reconsolidation therapies are concerned, a greater understanding of the stimulation and dose parameters that suit this purpose is warranted. In this study, we have expanded the knowledge of the neurobiology underlying propranolol’s effect on fear behavior and identified the hilus as a target of noradrenergic modulation that may alter the recruitment of DG fear memory traces.
The raw data supporting the conclusions of this article will be made available by the authors, without undue reservation.
All experiments were approved by the Institutional Animal Care and Use Committee (IACUC) at the New York Psychiatric Institute (NYSPI).
SL, BC, and CD contributed to the conception and design of the work, the analysis and interpretation of data for the work, drafting the work, and revising it critically for important intellectual content. SL, BC, GP, and VP contributed to the acquisition of data. SL, BC, GP, VP, and CD approved the version of the manuscript to be published and agreed to be accountable for all aspects of the work in ensuring that questions related to the accuracy and integrity of any part of the work are appropriately investigated and resolved. All authors contributed to the article and approved the submitted version.
SL was supported by a fellowship from Fundação para a Ciência e a Tecnologia (FCT) (Grant No. PD/BD/128076/2016) and a Whitehall Foundation grant. BC was supported by an F31 MH121023-01A1. VP was supported by an NICHD R01HD101402. CD was supported by a retention package from the NYSPI, a Whitehall Foundation grant, an NICHD R01HD101402, an NIA R21AG064774, an NINDS R21NS114870 and a gift from the For the Love of Travis Foundation.
SL, VP, and CD were employed by Research Foundation for Mental Hygiene, Inc.
The remaining authors declare that the research was conducted in the absence of any commercial or financial relationships that could be construed as a potential conflict of interest.
All claims expressed in this article are solely those of the authors and do not necessarily represent those of their affiliated organizations, or those of the publisher, the editors and the reviewers. Any product that may be evaluated in this article, or claim that may be made by its manufacturer, is not guaranteed or endorsed by the publisher.
We thank members of the Denny laboratory for their insightful comments on this project and manuscript.
The Supplementary Material for this article can be found online at: https://www.frontiersin.org/articles/10.3389/fnbeh.2022.919831/full#supplementary-material
Amaral, D. G., Scharfman, H. E., and Lavenex, P. (2007). The dentate gyrus: fundamental neuroanatomical organization (dentate gyrus for dummies). Prog. Brain Res. 163, 3–22. doi: 10.1016/S0079-6123(07)63001-5
Anacker, C., Luna, V. M., Stevens, G. S., Millette, A., Shores, R., Jimenez, J. C., et al. (2018). Hippocampal neurogenesis confers stress resilience by inhibiting the ventral dentate gyrus. Nature 559, 98–102. doi: 10.1038/s41586-018-0262-4
Aston-Jones, G., and Waterhouse, B. (2016). Locus coeruleus: From global projection system to adaptive regulation of behavior. Brain Res. 1645, 75–78.
Avery, M. C., and Krichmar, J. L. (2017). Neuromodulatory Systems and Their Interactions: A Review of Models, Theories, and Experiments. Front. Neural. Circuits 11:108. doi: 10.3389/fncir.2017.00108
Azzi, M., Charest, P. G., Angers, S., Rousseau, G., Kohout, T., Bouvier, M., et al. (2003). Beta-arrestin-mediated activation of MAPK by inverse agonists reveals distinct active conformations for G protein-coupled receptors. Proc. Natl. Acad. Sci. U.S.A. 100, 11406–11411. doi: 10.1073/pnas.1936664100
Baker, J. G., Hall, I. P., and Hill, S. J. (2003). Agonist actions of “beta-blockers” provide evidence for two agonist activation sites or conformations of the human beta1-adrenoceptor. Mol. Pharmacol. 63, 1312–1321.
Bannerman, D. M., Rawlins, J. N., Mchugh, S. B., Deacon, R. M., Yee, B. K., Bast, T., et al. (2004). Regional dissociations within the hippocampus–memory and anxiety. Neurosci. Biobehav. Rev. 28, 273–283. doi: 10.1016/j.neubiorev.2004.03.004
Bannerman, D. M., Yee, B. K., Good, M. A., Heupel, M. J., Iversen, S. D., and Rawlins, J. N. (1999). Double dissociation of function within the hippocampus: a comparison of dorsal, ventral, and complete hippocampal cytotoxic lesions. Behav. Neurosci. 113, 1170–1188. doi: 10.1037//0735-7044.113.6.1170
Belkin, M. R., and Schwartz, T. L. (2015). Alpha-2 receptor agonists for the treatment of posttraumatic stress disorder. Drugs Context 4:212286.
Berridge, C. (2005). Chapter 4.3 The locus coeruleus-noradrenergic system and stress: modulation of arousal state and state-dependent behavioral processes. Tech. Behav. Neural Sci. 15, 437–464.
Berridge, C. W., and Waterhouse, B. D. (2003). The locus coeruleus-noradrenergic system: modulation of behavioral state and state-dependent cognitive processes. Brain Res. Brain Res. Rev. 42, 33–84. doi: 10.1016/s0165-0173(03)00143-7
Bienkowski, M. S., Bowman, I., Song, M. Y., Gou, L., Ard, T., Cotter, K., et al. (2018). Integration of gene expression and brain-wide connectivity reveals the multiscale organization of mouse hippocampal networks. Nat. Neurosci. 21, 1628–1643. doi: 10.1038/s41593-018-0241-y
Booze, R. M., Crisostomo, E. A., and Davis, J. N. (1993). Beta-adrenergic receptors in the hippocampal and retrohippocampal regions of rats and guinea pigs: autoradiographic and immunohistochemical studies. Synapse 13, 206–214. doi: 10.1002/syn.890130303
Botella, C., Fernández-Álvarez, J., Guillén, V., García-Palacios, A., and Baños, R. (2017). Recent Progress in Virtual Reality Exposure Therapy for Phobias: A Systematic Review. Curr. Psychiatry Rep. 19:42. doi: 10.1007/s11920-017-0788-4
Brunet, A., Saumier, D., Liu, A., Streiner, D. L., Tremblay, J., and Pitman, R. K. (2018). Reduction of PTSD Symptoms With Pre-Reactivation Propranolol Therapy: A Randomized Controlled Trial. Am. J. Psychiatry 175, 427–433. doi: 10.1176/appi.ajp.2017.17050481
Cazzulino, A. S., Martinez, R., Tomm, N. K., and Denny, C. A. (2016). Improved specificity of hippocampal memory trace labeling. Hippocampus 26, 752–762.
Chen, B. K., Murawski, N. J., Cincotta, C., Mckissick, O., Finkelstein, A., Hamidi, A. B., et al. (2019). Artificially Enhancing and Suppressing Hippocampus-Mediated Memories. Curr. Biol. 29, 1885.e–1894.e. doi: 10.1016/j.cub.2019.04.065
Chen, C. C., Lu, J., Yang, R., Ding, J. B., and Zuo, Y. (2018). Selective activation of parvalbumin interneurons prevents stress-induced synapse loss and perceptual defects. Mol. Psychiatry 23, 1614–1625. doi: 10.1038/mp.2017.159
Chen, F. J., and Sara, S. J. (2007). Locus coeruleus activation by foot shock or electrical stimulation inhibits amygdala neurons. Neuroscience 144, 472–481.
Cox, D. J., Racca, C., and Lebeau, F. E. (2008). Beta-adrenergic receptors are differentially expressed in distinct interneuron subtypes in the rat hippocampus. J. Comp. Neurol. 509, 551–565. doi: 10.1002/cne.21758
De Nó, R. L. (1934). Studies on the Structure of the Cerebral Cortex: continuation of the Study of the Ammonic System. Leipzig :Johann Ambrosius Barth.
Denny, C. A., Kheirbek, M. A., Alba, E. L., Tanaka, K. F., Brachman, R. A., Laughman, K. B., et al. (2014). Hippocampal memory traces are differentially modulated by experience, time, and adult neurogenesis. Neuron 83, 189–201. doi: 10.1016/j.neuron.2014.05.018
Denny, C. A., Lebois, E., and Ramirez, S. (2017). From Engrams to Pathologies of the Brain. Front. Neural. Circuits 11:23. doi: 10.3389/fncir.2017.00023
Do Monte, F. H., Canteras, N. S., Fernandes, D., Assreuy, J., and Carobrez, A. P. (2008). New perspectives on beta-adrenergic mediation of innate and learned fear responses to predator odor. J. Neurosci. 28, 13296–13302. doi: 10.1523/JNEUROSCI.2843-08.2008
Do-Monte, F. H., Kincheski, G. C., Pavesi, E., Sordi, R., Assreuy, J., and Carobrez, A. P. (2010). Role of beta-adrenergic receptors in the ventromedial prefrontal cortex during contextual fear extinction in rats. Neurobiol. Learn. Mem. 94, 318–328. doi: 10.1016/j.nlm.2010.07.004
Duffy, A. M., Schaner, M. J., Chin, J., and Scharfman, H. E. (2013). Expression of c-fos in hilar mossy cells of the dentate gyrus in vivo. Hippocampus 23, 649–655.
Ehrlich, I., Humeau, Y., Grenier, F., Ciocchi, S., Herry, C., and Lüthi, A. (2009). Amygdala inhibitory circuits and the control of fear memory. Neuron 62, 757–771.
Espinoza, C., Guzman, S. J., Zhang, X., and Jonas, P. (2018). Parvalbumin(+) interneurons obey unique connectivity rules and establish a powerful lateral-inhibition microcircuit in dentate gyrus. Nat. Commun. 9:4605. doi: 10.1038/s41467-018-06899-3
Fagerström, K. O., Hugdahl, K., and Lundström, N. (1985). Effect of beta-receptor blockade on anxiety with reference to the three-systems model of phobic behavior. Neuropsychobiology 13, 187–193. doi: 10.1159/000118186
Fanselow, M. S., and Dong, H. W. (2010). Are the dorsal and ventral hippocampus functionally distinct structures? Neuron 65, 7–19.
Fitzgerald, P. J., Giustino, T. F., Seemann, J. R., and Maren, S. (2015). Noradrenergic blockade stabilizes prefrontal activity and enables fear extinction under stress. Proc. Natl. Acad. Sci. U.S.A. 112, E3729–E3737. doi: 10.1073/pnas.1500682112
Galandrin, S., and Bouvier, M. (2006). Distinct signaling profiles of beta1 and beta2 adrenergic receptor ligands toward adenylyl cyclase and mitogen-activated protein kinase reveals the pluridimensionality of efficacy. Mol. Pharmacol. 70, 1575–1584. doi: 10.1124/mol.106.026716
Giustino, T. F., and Maren, S. (2018). Noradrenergic Modulation of Fear Conditioning and Extinction. Front. Behav. Neurosci. 12:43. doi: 10.3389/fnbeh.2018.00043
Giustino, T. F., Fitzgerald, P. J., and Maren, S. (2016). Revisiting propranolol and PTSD: Memory erasure or extinction enhancement? Neurobiol. Learn. Mem. 130, 26–33.
Giustino, T. F., Seemann, J. R., Acca, G. M., Goode, T. D., Fitzgerald, P. J., and Maren, S. (2017). β-Adrenoceptor Blockade in the Basolateral Amygdala, But Not the Medial Prefrontal Cortex, Rescues the Immediate Extinction Deficit. Neuropsychopharmacology 42, 2537–2544. doi: 10.1038/npp.2017.89
Grillon, C., Cordova, J., Morgan, C. A., Charney, D. S., and Davis, M. (2004). Effects of the beta-blocker propranolol on cued and contextual fear conditioning in humans. Psychopharmacology 175, 342–352. doi: 10.1007/s00213-004-1819-5
Guo, N. N., and Li, B. M. (2007). Cellular and subcellular distributions of beta1- and beta2-adrenoceptors in the CA1 and CA3 regions of the rat hippocampus. Neuroscience 146, 298–305. doi: 10.1016/j.neuroscience.2007.01.013
Hagena, H., Hansen, N., and Manahan-Vaughan, D. (2016). β-Adrenergic Control of Hippocampal Function: Subserving the Choreography of Synaptic Information Storage and Memory. Cereb Cortex 26, 1349–1364. doi: 10.1093/cercor/bhv330
Honoré, E., Khlaifia, A., Bosson, A., and Lacaille, J.-C. (2021). Hippocampal Somatostatin Interneurons, Long-Term Synaptic Plasticity and Memory. Front. Neural. Circ. 15:687558. doi: 10.3389/fncir.2021.687558
Houser, C. R. (2007). Interneurons of the dentate gyrus: an overview of cell types, terminal fields and neurochemical identity. Prog. Brain Res. 163, 217–232. doi: 10.1016/S0079-6123(07)63013-1
Hurlemann, R., Walter, H., Rehme, A. K., Kukolja, J., Santoro, S. C., Schmidt, C., et al. (2010). Human amygdala reactivity is diminished by the β-noradrenergic antagonist propranolol. Psychol. Med. 40, 1839–1848. doi: 10.1017/S0033291709992376
Ji, J. Z., Zhang, X. H., and Li, B. M. (2003). Deficient spatial memory induced by blockade of beta-adrenoceptors in the hippocampal CA1 region. Behav. Neurosci. 117, 1378–1384. doi: 10.1037/0735-7044.117.6.1378
Khalaf, O., and Gräff, J. (2019). Reactivation of Recall-Induced Neurons in the Infralimbic Cortex and the Basolateral Amygdala After Remote Fear Memory Attenuation. Front. Mol. Neurosci. 12:70. doi: 10.3389/fnmol.2019.00070
Khalaf, O., Resch, S., Dixsaut, L., Gorden, V., Glauser, L., and Gräff, J. (2018). Reactivation of recall-induced neurons contributes to remote fear memory attenuation. Science 360, 1239–1242. doi: 10.1126/science.aas9875
Kheirbek, M. A., Drew, L. J., Burghardt, N. S., Costantini, D. O., Tannenholz, L., Ahmari, S. E., et al. (2013). Differential control of learning and anxiety along the dorsoventral axis of the dentate gyrus. Neuron 77, 955–968. doi: 10.1016/j.neuron.2012.12.038
Kjelstrup, K. G., Tuvnes, F. A., Steffenach, H. A., Murison, R., Moser, E. I., and Moser, M. B. (2002). Reduced fear expression after lesions of the ventral hippocampus. Proc. Natl. Acad. Sci. U.S.A. 99, 10825–10830.
Kroes, M. C., Tona, K. D., Den Ouden, H. E., Vogel, S., Van Wingen, G. A., and Fernández, G. (2016). How Administration of the Beta-Blocker Propranolol Before Extinction can Prevent the Return of Fear. Neuropsychopharmacology 41, 1569–1578.
Lacagnina, A. F., Brockway, E. T., Crovetti, C. R., Shue, F., Mccarty, M. J., Sattler, K. P., et al. (2019). Distinct hippocampal engrams control extinction and relapse of fear memory. Nat. Neurosci. 22, 753–761. doi: 10.1038/s41593-019-0361-z
Leal Santos, S., Stackmann, M., Muñoz Zamora, A., Mastrodonato, A., De Landri, A. V., Vaughan, N., et al. (2021). Propranolol Decreases Fear Expression by Modulating Fear Memory Traces. Biol. Psychiatry 89, 1150–1161. doi: 10.1016/j.biopsych.2021.01.005
Link, W., Konietzko, U., Kauselmann, G., Krug, M., Schwanke, B., Frey, U., et al. (1995). Somatodendritic expression of an immediate early gene is regulated by synaptic activity. Proc. Natl. Acad. Sci. U.S.A. 92, 5734–5738.
Liu, H. H., Milgrom, P., and Fiset, L. (1991). Effect of a beta-adrenergic blocking agent on dental anxiety. J. Dent. Res. 70, 1306–1308.
Liu, X., Ramirez, S., Pang, P. T., Puryear, C. B., Govindarajan, A., Deisseroth, K., et al. (2012). Optogenetic stimulation of a hippocampal engram activates fear memory recall. Nature 484, 381–385.
Lonergan, M. H., Olivera-Figueroa, L. A., Pitman, R. K., and Brunet, A. (2013). Propranolol’s effects on the consolidation and reconsolidation of long-term emotional memory in healthy participants: a meta-analysis. J. Psychiatry Neurosci. 38, 222–231. doi: 10.1503/jpn.120111
Lyford, G. L., Yamagata, K., Kaufmann, W. E., Barnes, C. A., Sanders, L. K., Copeland, N. G., et al. (1995). Arc, a growth factor and activity-regulated gene, encodes a novel cytoskeleton-associated protein that is enriched in neuronal dendrites. Neuron 14, 433–445. doi: 10.1016/0896-6273(95)90299-6
Mastrodonato, A., Martinez, R., Pavlova, I. P., Lagamma, C. T., Brachman, R. A., Robison, A. J., et al. (2018). Ventral CA3 Activation Mediates Prophylactic Ketamine Efficacy Against Stress-Induced Depressive-like Behavior. Biol. Psychiatry 84, 846–856. doi: 10.1016/j.biopsych.2018.02.011
McGuire, J. F., Lewin, A. B., and Storch, E. A. (2014). Enhancing exposure therapy for anxiety disorders, obsessive-compulsive disorder and post-traumatic stress disorder. Expert Rev. Neurother. 14, 893–910.
Milner, T. A., Shah, P., and Pierce, J. P. (2000). beta-adrenergic receptors primarily are located on the dendrites of granule cells and interneurons but also are found on astrocytes and a few presynaptic profiles in the rat dentate gyrus. Synapse 36, 178–193. doi: 10.1002/(SICI)1098-2396(20000601)36:3<178::AID-SYN3>3.0.CO;2-6
Moretto, J. N., Duffy, ÁM., and Scharfman, H. E. (2017). Acute restraint stress decreases c-fos immunoreactivity in hilar mossy cells of the adult dentate gyrus. Brain Struct. Funct. 222, 2405–2419. doi: 10.1007/s00429-016-1349-z
Moser, M. B., and Moser, E. I. (1998). Functional differentiation in the hippocampus. Hippocampus 8, 608–619.
Moser, M. B., Moser, E. I., Forrest, E., Andersen, P., and Morris, R. G. (1995). Spatial learning with a minislab in the dorsal hippocampus. Proc. Natl. Acad. Sci. U.S.A. 92, 9697–9701. doi: 10.1073/pnas.92.21.9697
Muravieva, E. V., and Alberini, C. M. (2010). Limited efficacy of propranolol on the reconsolidation of fear memories. Learn. Mem. 17, 306–313.
Ognjanovski, N., Schaeffer, S., Wu, J., Mofakham, S., Maruyama, D., Zochowski, M., et al. (2017). Parvalbumin-expressing interneurons coordinate hippocampal network dynamics required for memory consolidation. Nat. Communicat. 8:15039.
Pastuzyn, E. D., Day, C. E., Kearns, R. B., Kyrke-Smith, M., Taibi, A. V., Mccormick, J., et al. (2018). The Neuronal Gene Arc Encodes a Repurposed Retrotransposon Gag Protein that Mediates Intercellular RNA Transfer. Cell 172, 275.e–288.e.
Pavlova, I. P., Shipley, S. C., Lanio, M., Hen, R., and Denny, C. A. (2018). Optimization of immunolabeling and clearing techniques for indelibly labeled memory traces. Hippocampus 28, 523–535. doi: 10.1002/hipo.22951
Perlman, G., Tanti, A., and Mechawar, N. (2021). Parvalbumin interneuron alterations in stress-related mood disorders: A systematic review. Neurobiol. Stress 15:100380. doi: 10.1016/j.ynstr.2021.100380
Perusini, J. N., Cajigas, S. A., Cohensedgh, O., Lim, S. C., Pavlova, I. P., Donaldson, Z. R., et al. (2017). Optogenetic stimulation of dentate gyrus engrams restores memory in Alzheimer’s disease mice. Hippocampus 27, 1110–1122. doi: 10.1002/hipo.22756
Pickel, V. M., Segal, M., and Bloom, F. E. (1974). A radioautographic study of the efferent pathways of the nucleus locus coeruleus. J. Comp. Neurol. 155, 15–42. doi: 10.1002/cne.901550103
Qi, X. L., Zhu, B., Zhang, X. H., and Li, B. M. (2008). Are beta-adrenergic receptors in the hippocampal CA1 region required for retrieval of contextual fear memory? Biochem. Biophys. Res. Commun. 368, 186–191.
Ramirez, S., Liu, X., Macdonald, C. J., Moffa, A., Zhou, J., Redondo, R. L., et al. (2015). Activating positive memory engrams suppresses depression-like behaviour. Nature 522, 335–339. doi: 10.1038/nature14514
Rauch, S. A., Eftekhari, A., and Ruzek, J. I. (2012). Review of exposure therapy: a gold standard for PTSD treatment. J. Rehabil. Res. Dev. 49, 679–687. doi: 10.1682/jrrd.2011.08.0152
Redondo, R. L., Kim, J., Arons, A. L., Ramirez, S., Liu, X., and Tonegawa, S. (2014). Bidirectional switch of the valence associated with a hippocampal contextual memory engram. Nature 513, 426–430. doi: 10.1038/nature13725
Reijmers, L. G., Perkins, B. L., Matsuo, N., and Mayford, M. (2007). Localization of a stable neural correlate of associative memory. Science 317, 1230–1233.
Richmond, M. A., Yee, B. K., Pouzet, B., Veenman, L., Rawlins, J. N., Feldon, J., et al. (1999). Dissociating context and space within the hippocampus: effects of complete, dorsal, and ventral excitotoxic hippocampal lesions on conditioned freezing and spatial learning. Behav. Neurosci. 113, 1189–1203. doi: 10.1037/0735-7044.113.6.1189
Rodriguez-Romaguera, J., Sotres-Bayon, F., Mueller, D., and Quirk, G. J. (2009). Systemic propranolol acts centrally to reduce conditioned fear in rats without impairing extinction. Biol. Psychiatry 65, 887–892. doi: 10.1016/j.biopsych.2009.01.009
Roy, D. S., Arons, A., Mitchell, T. I., Pignatelli, M., Ryan, T. J., and Tonegawa, S. (2016). Memory retrieval by activating engram cells in mouse models of early Alzheimer’s disease. Nature 531, 508–512. doi: 10.1038/nature17172
Scharfman, H. E. (2016). The enigmatic mossy cell of the dentate gyrus. Nat. Rev. Neurosci. 17, 562–575. doi: 10.1038/nrn.2016.87
Scharfman, H. E., and Myers, C. E. (2012). Hilar mossy cells of the dentate gyrus: a historical perspective. Front. Neural. Circuits 6:106. doi: 10.3389/fncir.2012.00106
Schindelin, J., Arganda-Carreras, I., Frise, E., Kaynig, V., Longair, M., Pietzsch, T., et al. (2012). Fiji: an open-source platform for biological-image analysis. Nat. Methods 9, 676–682. doi: 10.1038/nmeth.2019
Schroyens, N., Beckers, T., and Kindt, M. (2017). In Search for Boundary Conditions of Reconsolidation: A Failure of Fear Memory Interference. Front. Behav. Neurosci. 11:65. doi: 10.3389/fnbeh.2017.00065
Schwabe, L., Nader, K., Wolf, O. T., Beaudry, T., and Pruessner, J. C. (2012). Neural signature of reconsolidation impairments by propranolol in humans. Biol. Psychiatry 71, 380–386. doi: 10.1016/j.biopsych.2011.10.028
Schwarz, L. A., and Luo, L. (2015). Organization of the locus coeruleus-norepinephrine system. Curr. Biol. 25, R1051–R1056.
Seo, D.-O., Zhang, E. T., Piantadosi, S. C., Marcus, D. J., Motard, L. E., Kan, B. K., et al. (2021). A locus coeruleus to dentate gyrus noradrenergic circuit modulates aversive contextual processing. Neuron 109, 2116.e–2130.e. doi: 10.1016/j.neuron.2021.05.006
Sik, A., Penttonen, M., and Buzsáki, G. (1997). Interneurons in the hippocampal dentate gyrus: an in vivo intracellular study. Eur. J. Neurosci. 9, 573–588. doi: 10.1111/j.1460-9568.1997.tb01634.x
Soeter, M., and Kindt, M. (2015). An Abrupt Transformation of Phobic Behavior After a Post-Retrieval Amnesic Agent. Biol. Psychiatry 78, 880–886. doi: 10.1016/j.biopsych.2015.04.006
Srinivas, S., Watanabe, T., Lin, C. S., William, C. M., Tanabe, Y., Jessell, T. M., et al. (2001). Cre reporter strains produced by targeted insertion of EYFP and ECFP into the ROSA26 locus. BMC Dev. Biol. 1:4. doi: 10.1186/1471-213x-1-4
Staiger, J. F., Masanneck, C., Bisler, S., Schleicher, A., Zuschratter, W., and Zilles, K. (2002). Excitatory and inhibitory neurons express c-Fos in barrel-related columns after exploration of a novel environment. Neuroscience 109, 687–699. doi: 10.1016/s0306-4522(01)00501-2
Swanson, L. W., and Hartman, B. K. (1975). The central adrenergic system. An immunofluorescence study of the location of cell bodies and their efferent connections in the rat utilizing dopamine-beta-hydroxylase as a marker. J. Comp. Neurol. 163, 467–505. doi: 10.1002/cne.901630406
Taylor, H. R., Freeman, M. K., and Cates, M. E. (2008). Prazosin for treatment of nightmares related to posttraumatic stress disorder. Am. J. Health Syst. Pharm. 65, 716–722.
Tonegawa, S., Liu, X., Ramirez, S., and Redondo, R. (2015). Memory Engram Cells Have Come of Age. Neuron 87, 918–931. doi: 10.1016/j.neuron.2015.08.002
Trouche, S., Sasaki, J. M., Tu, T., and Reijmers, L. G. (2013). Fear extinction causes target-specific remodeling of perisomatic inhibitory synapses. Neuron 80, 1054–1065. doi: 10.1016/j.neuron.2013.07.047
Tyrer, P. (1988). Current status of beta-blocking drugs in the treatment of anxiety disorders. Drugs 36, 773–783. doi: 10.2165/00003495-198836060-00006
Tyssowski, K. M., Destefino, N. R., Cho, J.-H., Dunn, C. J., Poston, R. G., Carty, C. E., et al. (2018). Different Neuronal Activity Patterns Induce Different Gene Expression Programs. Neuron 98, 530.e–546.e.
Vetere, G., Kenney, J. W., Tran, L. M., Xia, F., Steadman, P. E., Parkinson, J., et al. (2017). Chemogenetic Interrogation of a Brain-wide Fear Memory Network in Mice. Neuron 94, 363.e–374.e. doi: 10.1016/j.neuron.2017.03.037
Vetere, G., Piserchia, V., Borreca, A., Novembre, G., Aceti, M., and Ammassari-Teule, M. (2013). Reactivating fear memory under propranolol resets pre-trauma levels of dendritic spines in basolateral amygdala but not dorsal hippocampus neurons. Front. Behav. Neurosci. 7:211. doi: 10.3389/fnbeh.2013.00211
Keywords: hippocampus, hilus, Arc, Fos, somatostatin, parvalbumin
Citation: Leal Santos S, Chen BK, Pereira GR, Pham V and Denny CA (2022) Propranolol Administration Modulates Neural Activity in the Hippocampal Hilus During Fear Retrieval. Front. Behav. Neurosci. 16:919831. doi: 10.3389/fnbeh.2022.919831
Received: 14 April 2022; Accepted: 13 June 2022;
Published: 07 July 2022.
Edited by:
Priyanka Rao-Ruiz, VU Amsterdam, NetherlandsReviewed by:
Andressa Radiske, Edmond and Lily Safra International Institute of Neuroscience (IIN-ELS/ISD), BrazilCopyright © 2022 Leal Santos, Chen, Pereira, Pham and Denny. This is an open-access article distributed under the terms of the Creative Commons Attribution License (CC BY). The use, distribution or reproduction in other forums is permitted, provided the original author(s) and the copyright owner(s) are credited and that the original publication in this journal is cited, in accordance with accepted academic practice. No use, distribution or reproduction is permitted which does not comply with these terms.
*Correspondence: Christine A. Denny, Y2FkMjEyNUBjdW1jLmNvbHVtYmlhLmVkdQ==
†These authors have contributed equally to this work and share first authorship
Disclaimer: All claims expressed in this article are solely those of the authors and do not necessarily represent those of their affiliated organizations, or those of the publisher, the editors and the reviewers. Any product that may be evaluated in this article or claim that may be made by its manufacturer is not guaranteed or endorsed by the publisher.
Research integrity at Frontiers
Learn more about the work of our research integrity team to safeguard the quality of each article we publish.