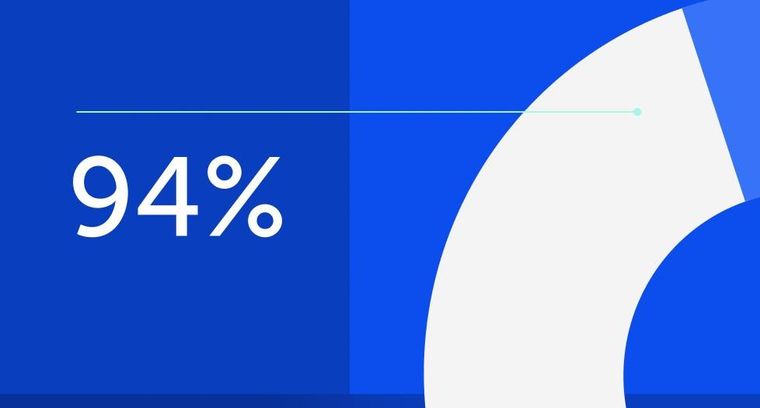
94% of researchers rate our articles as excellent or good
Learn more about the work of our research integrity team to safeguard the quality of each article we publish.
Find out more
ORIGINAL RESEARCH article
Front. Behav. Neurosci., 17 May 2022
Sec. Learning and Memory
Volume 16 - 2022 | https://doi.org/10.3389/fnbeh.2022.903100
This article is part of the Research TopicBasic and Translation Research in Learning and MemoryView all 13 articles
Trauma, as well as chronic stress that characterizes a modern fast-paced lifestyle, contributes to numerous psychopathologies and psychological problems. Psychiatric patients with traumas, as well as healthy individuals who experienced traumas in the past, are often characterized by diminished cognitive abilities. In our protocol, we used an animal model to explore the influence of chronic trauma on cognitive abilities and behavior in the group of 20 rats (Rattus norvegicus). The experimental group was introduced to chronic (12 consecutive days) exposure to predator odor (bobcat urine). We measured the reinforcement learning of each individual before and after the exposition via the Probabilistic Selection Task (PST) and we used Social Interaction Test (SIT) to assess the behavioral changes of each individual before and after the trauma. In the experimental group, there was a significant decrease in reinforcement learning after exposure to a single trauma (Wilcoxon Test, p = 0.034) as well as after 11 days of chronic trauma (Wilcoxon-test, p = 0.01) in comparison to pre-trauma performance. The control group, which was not exposed to predator odor but underwent the same testing protocol, did not present significant deterioration in reinforcement learning. In cross-group comparisons, there was no difference between the experimental and control group in PST before odor protocol (U Mann-Whitney two-sided, p = 0.909). After exposure to chronic trauma, the experimental group deteriorated in PST performance compared to control (U Mann-Whitney Two-sided, p = 0.0005). In SIT, the experimental group spent less time in an Interaction Zone with an unfamiliar rat after trauma protocol (Wilcoxon two-sided test, p = 0.019). Major strengths of our models are: (1) protocol allows investigating reinforcement learning before and after exposition to chronic trauma, with the same group of rats, (2) translational scope, as the PST is displayed on touchscreen, similarly to human studies, (3) protocol delivers chronic trauma that impairs reward learning, but behaviorally does not induce full-blown anhedonia, thus rats performed voluntarily throughout all the procedures.
Throughout life, the environment puts numerous stressors on every living organism. In humans, extreme stress (trauma) captures a range of severe adverse experiences, such as physical, sexual, or emotional abuse, neglect, parental death, bullying, or omission by caregiver during childhood. Trauma contributes to the development of numerous mental disorders such as posttraumatic stress disorder (PTSD), anxiety disorders, schizophrenia, personality disorders, mood disorders (Jansen et al., 2016; Misiak et al., 2017). It is estimated that prevalence of PTSD reaches 7% in general population (McLaughlin et al., 2015), while in subgroups exposed to severe psychological trauma numbers are even more prominent, for example, 10% of US veterans meet criteria of PTSD (Mota et al., 2016) as well as 60% minor refugees in Germany that sought general medical treatment (Veeser et al., 2021). In the general population, only a small proportion of individuals with a positive history of traumatic events develop full-blown PTSD (Breslau, 2009). Trauma affects cognitive abilities (Petkus et al., 2018; Aas et al., 2019), disrupts the immune system (Mehta et al., 2020), causes structural changes in the brain (Assogna et al., 2020), affects the severity of symptoms among those with mental disorders (Duhig et al., 2015; Ay and Erbay, 2018; Bailey et al., 2018). Chronic stress, defined as an exposition to a series of stressful or potentially traumatic events, characterizes a modern, fast-paced western lifestyle (Matosin et al., 2017). Chronic stress turns out to be closely related to numerous health issues: obesity, diabetes, mental disorders, psychological deficits, substance dependence (Sinha, 2008; Farag and Gaballa, 2011; Misiak et al., 2017; Bielawski et al., 2019). All are major epidemiological health concerns that generate enormous public cost (Simon et al., 2006; Farag and Gaballa, 2011; Laramée et al., 2013; Masodkar et al., 2016). The purpose of this study is to present a novel protocol to examine cognitive impairment in reinforcement learning as chronic trauma progresses. We use a simplified Probabilistic Selection Task (PST) to approximate our model to human studies. In humans, experimental studies of PTSD, chronic stress, and trauma are limited. Therefore, our research is to explore the translational scope of PTSD studies in rodents. We want to test whether rats will perform voluntarily while exposed to chronic trauma. If so, our aim is to study rats’ ability to learn the PST protocol, as well as their ability to adapt to a system, where interaction with a touchscreen is related to reward collection. Our procedure examines reward learning before and after exposure to chronic trauma, with the same group of rats. This approach allows us to measure cognitive disruptions as the trauma progresses. We hypothesize that rats exposed to trauma will perform poorer in PST, in comparison to their performance before exposure to chronic trauma. Moreover, we want to explore whether a single exposure to trauma will affect cognitive functioning. Furthermore, we hypothesize that traumatized individuals will be less socially oriented during Social Interaction Test (SIT), compared to the control.
Medically oriented understanding of psychological trauma is strictly related with PTSD diagnosis (Yehuda, 1998), while in psychoanalytic approach trauma is a powerful stimulus, that breaches one’s psychological defense mechanisms, and induces experience of helplessness (Rothgeb, 1971). In both definitions trauma is an extreme stress, that is beyond one’s ability to cope with. An abundant literature presents negative impact of trauma on cognitive functions in patients with psychosis (Lysaker et al., 2001; Schenkel et al., 2005; Shannon et al., 2011) and among healthy individuals who experienced trauma in the past (Majer et al., 2010; Vasilevski and Tucker, 2016; Petkus et al., 2018). Trauma and prolonged (chronic) stress activate the hypothalamic-pituitary-adrenal (HPA) axis via the rise of corticosteroids, activate the endocannabinoid system, and indirectly affect dopamine bursts in the striatum and medial prefrontal cortex (Joëls et al., 2012; Bielawski et al., 2019). Different regions of the brain (for example hippocampus, amygdala, medial prefrontal cortex, hypothalamus) involved in stimulus recognition, memory, and learning are affected by increased detrimental corticosteroids rise during chronic or acute stress (Pruessner et al., 2017; Bielawski et al., 2019). The neurobiology of trauma and its impact on cognitive abilities is complex, and studies in human subjects have certain limitations. Thus, several animal models have been developed to assess symptoms associated with exposure to trauma and the development of PTSD (Whitaker et al., 2014; Harro, 2018; Planchez et al., 2019). The Diagnostic and Statistical Manual of Mental Disorders version 5 (DSM-V) delivered by the American Psychiatric Association (APA) presents four clusters of symptoms of PTSD: intrusive recollection of the original traumatic event, avoidance of trauma-related reminders, negative changes in cognition and mood, and alterations in arousal or reactivity, each of which must start or be significantly exacerbated after exposure to the traumatic event (Roehr, 2013). The variety of animal models put its focus on different aspects of PTSD symptomatology, such as contextual avoidance (Albrechet-Souza et al., 2020), changes in arousal and reactivity (Knox et al., 2012), and behavior alterations (Krishnan et al., 2007). These models measure different parameters after the exposition to stress. Our approach is to measure cognitive and behavioral parameters as chronic trauma progresses. That way, an animal model gives us an opportunity to expose rats to chronic stress, as we measure their cognitive functions simultaneously. Chronic stress lacks a clear definition, but most authors agree that it is an exposition to a series of intense, potentially traumatic experiences or involvement in prolonged stress situations that leads to psychopathologies and/or adverse medical conditions (Matosin et al., 2017). Chronic stress is widely used in animal models of anxiety disorders, depression, and PTSD (Saavedra-Rodríguez and Feig, 2013; Reber et al., 2016; Wang et al., 2021). In humans, prolonged stress is an important factor in etiopathology of different mental disorders (Matosin et al., 2017; McEwen, 2017; Ross et al., 2017), for example chronic stress can induce mild PTSD symptoms in humans (Davidson and Baum, 1986). Stress influences the ability to learn from rewards among those with a familial predisposition to psychosis and individuals with major depressive disorder (Reinen et al., 2021). Furthermore, chronic stress induces hyper inflammation, thus being discussed to enhance susceptibility to infectious diseases such as COVID-19 (Lamontagne et al., 2021), or mental diseases linked to immune system dysregulations (Dennison et al., 2012). Chronic exposure to trauma is particularly harmful; many individuals repeatedly exposed to traumatic events carry a heavy burden of psychopathologies (Sharhabani-Arzy et al., 2003; Éthier et al., 2004; Salcioglu et al., 2017). In our experiment, we expose male Wistar Rats to chronic trauma for 12 consecutive days. In the literature, there are animal models of PTSD that reveal alteration in cognitive performance, although they often apply a single prolonged stress procedure (George et al., 2015). Indeed, single exposure to predator odor is sufficient to induce trauma (Albrechet-Souza and Gilpin, 2019), but our goal is to mimic chronic stress, thus our protocol’s prolonged exposure to stressful stimulus with parallel cognitive examination.
In humans, the Probabilistic Selection Task (PST) was shown to be associated with dopaminergic effects on learning (Frank et al., 2007). Positron emission tomography and functional magnetic resonance imaging studies showed that reinforcement-based decisions are associated with signaling in the striatum and prefrontal cortex (Jocham et al., 2011; Kasanova et al., 2018). Furthermore, PST was used to assess learning deficits among those with PTSD (Myers et al., 2013). During PST, participants are presented stimulus pairs and learn to choose one of them. After each choice, probabilistic feedback follows the choice to indicate whether it was correct or incorrect. PST (and its different variants) are widely used in animal studies—in rodents stimulus selection is most often recorded via nose poke in aperture (Amitai et al., 2014) or by pressing the lever (George et al., 2015; Seib et al., 2020), while in humans selection is usually done via tap on a touchscreen or pressing a button on a keyboard (Frank et al., 2004).
The Social Interaction Test (SIT) is a popular method to assess levels of anxiety, social interaction, locomotor activity, and arousal in rodents (File and Seth, 2003). In our experiment, an examined rat is introduced into the test box with a tunnel, open field arena, and Interaction Zone with unfamiliar rat. Examined rat behavior is monitored; time spent in different parts of the test arena, number of droppings, or freezing behavior. Our model explores cognitive changes among Wistar Rats through the PST, as well as anxiety level and social interaction through the Social Interaction Test. We used SIT procedure similar to the one in social defeat experiments (Golden et al., 2011; Toyoda, 2017).
In our study, we use an animal model with predator odor exposure that produces behavioral, physiological, and molecular alterations that recapitulate many of the same alterations observed in PTSD patients (Cohen et al., 2012). We use bobcat urine as a stressor, it is a well-established model used in a series of studies done by Gilpin and colleagues (Albrechet-Souza and Gilpin, 2019). Bobcat urine contains the biogenic amine 2-phenylethylamine, which activates specific receptors within the rodent olfactory cortex, the trace amine-associated receptor 4 (TAART4), and can induce avoidance behavior in rats and mice (Ferrero et al., 2011). Furthermore, bobcat urine activates the amygdala-piriform transition area, which is responsible for increases in circulating stress hormones (Kondoh et al., 2016). In 1993, Yehuda and Antelman developed 5 criteria that animal models must meet, to parallel PTSD-related phenotypes: (1) Even a brief stressor should be able to induce biological and behavioral sequelae of PTSD, (2) The stressor should be able to produce PTSD-like sequelae in a dose-dependent manner, (3) Stressors should produce biological alterations that persist over time or become more pronounced with passage of time, (4) The stressor should induce biobehavioral alterations that have the potential for bidirectional expression, (5) Interindividual variability in response to a stressor should be present either as a function of experience, genetics, or an interaction of the two (Yehuda and Antelman, 1993). Studies done with bobcat urine meet most of those criteria (Albrechet-Souza and Gilpin, 2019), and are well discussed in the context of animal PTSD model (Albrechet-Souza et al., 2020, 2021). Taking the literature mentioned above, we feel confident using this type of traumatizing stimulus in our protocol.
In our procedure, we used male Wistar Rats (Animal Research Center, Wrocław Medical University, PL) in a total number of 26 individuals (n = 26), although 20 individuals were included in our experiment (n = 20). Rats arrived at the age of 39–42 days, weighing 210–245 g at the day of arrival, were submitted to a handling period (7 days), and then entered P0. Six individuals did not meet the criteria to enter the P1, and were excluded during P0. Excluded animals either: (1) did not learn the tapping procedure throughout phase 0 or (2) presented freezing behavior during 3 consecutive days. Due to housing conditions and experimental procedure, the exclusion of a rat resulted in the exclusion of its cotenant. Therefore, even though n = 3 rats met the exclusion criteria, the total sum of n = 6 individuals was excluded.
A random group of rats (n = 10) participated as a control group, the second group (n = 10) participated as an experimental group (n = 10). Rats were pair housed on a non-reversed 12 h/12 h light/dark cycle (lights off at 7 p.m.). All behavioral tests were constructed during the light period. Rats had ad libitum access to food (dry pellets) and water.
The experiment was conducted in accordance with the NIH Guide for the Care and Use of Laboratory Animals. All procedures were approved by the Local Ethics Committee for Animal Experiments, Hirszfeld Institute of Immunology and Experimental Therapy, Polish Academy of Sciences, Wrocław, Poland.
The PST chamber was part of the device built by our team to measure PST in rats. It had a perforated metal floor that allowed animals to move freely and comfortably. Under the perforated floor there was a compartment where a sponge with odor could have been placed. The walls and floor of the chamber were easy to sanitize and safe for the animals to explore. The front wall had a hole, where a touchscreen apparatus displayed stimuli. Opposite the front wall, there was a feeder and a diode. Feeder was the place where rewards was delivered, a diode signaled when reward was about to be delivered (see Figure 1A).
Figure 1. (A) Probabilistic Selection Task testing chamber. (B) Social Interaction Test testing chamber.
SIT chamber was constructed from polyvinyl chloride (PCV) and Plexiglas. The main structure was a square 90 × 90 × 40 cm (length × width × height). Inside, there was a PCV wall 70 × 30 cm (length × height) that formed a tunnel. Furthermore, two additional transparent Plexiglas walls (20 × 30 cm) formed a closed space in one of the corners, where a new and unfamiliar rat was trapped (see Figure 1B). The 25 cm from plexiglas walls was marked as an “interaction Zone.”
PST- one pair of stimuli is presented in random order arrangement (left of right side of the screen) (see Figure 2B). Rats learned to choose one pair. Feedback was probabilistic; it means that in BC trials, a choice of stimulus B results in 90% positive feedback (10% negative feedback), while choice of stimulus C results in 90% negative feedback (10% positive feedback). Feedback follows the choice to indicate if it was correct (reward) or incorrect (punishment). The correct choice resulted in reward—a drop of sweet protein shake (Strawberry Nutridrink Protein, NUTRICIA, Poland). Incorrect choice resulted in punishment—lack of reward. The touchscreen was 26.5 cm width × 17 cm height and “tappable”—nose poke, strike, or touch with paw resulted with stimulus selection. When the stimulus was selected, the touchscreen went black for 8 s and a reward was delivered to the feeder, simultaneously with a light signal. After 8 s, the touchscreen displayed a randomly arranged pair of stimuli again.
Figure 2. (A) Experiment time schedule. (B) Probabilistic Selection Task procedure. P0, Phase 0; P1, Phase 1; P2, Phase 2.
SIT was performed twice throughout the experiment, P1 Day 6 and P2 Day 6. Examined individual was placed at the beginning of the tunnel. The session lasted 10 min and was videotaped.
The rats were subjected to 1 week of handling before the phase 0. During handling sessions, rats were exposed to a sweet liquid, to adapt with sweet reward and feeder mouthpiece. An experiment consisted of 3 phases: phase 0 (P0), phase 1 (P1), and phase 2 (P2) (see Figure 2A). Each rat was examined via PST in the testing chamber once every day. Our protocol is a variation of the autoshaping task described by Horner et al. (2013).
P0 lasted 10 days and was designed to teach each animal the experimental procedure. During Day 1–3, the paired rats (according to the pair housing) were placed in the testing chamber to accommodate. The rats were able to explore the chamber for 20 min and collect rewards. During the first 6 days, the touchscreen displayed one visual stimulus on the left or right side (see Figure 2B). During the first 6 days, the rest of the touchscreen was “untappable”—there was no selection when tap occurred outside the stimulus sector. From 4 to Day 10, the rats were placed in the testing chamber separately, 10 min each.
Throughout Day 7–10 the stimulus was randomly displayed on the left or right side of the touchscreen, although the whole surface of the touchscreen was tappable. Tap delivered within the sector outside of the stimulus resulted in punishment—touchscreen went black for 8 s, no reward was delivered into the feeder. After 8 s, the touchscreen displayed the stimulus again randomly (left or right).
P1 lasted 12 days. Throughout P1, a pair of stimuli (B and C) was used in PST (see Figure 2B). Each animal was placed in the testing chamber for 20 min or until the session was completed. After each session, the testing chamber was thoroughly cleaned with disinfectant. The last day (P1 day 12) animals were exposed to predator odor, a sponge soaked with 3 ml of bobcat urine (Lynx rufus; Maine Outdoor Solutions, Hermon, ME, United States) was placed on the testing chamber floor. In the control group, sponges were not soaked with bobcat urine.
P2 lasted 12 days. Throughout P2, a pair of stimuli (D and E) was used in PST (see Figure 2B). Each animal was placed in the testing chamber for 20 min or until the session was completed. After each session, the testing chamber was thoroughly cleaned with disinfectant. Throughout P2, a sponge soaked with bobcat urine (Lynx rufus; Maine Outdoor Solutions, Hermon, ME, United States) was placed under the testing chamber floor. The Last day (P2 Day 12) the animals were not exposed to predator odor. Control rats are treated identically to rats exposed to odors, but the sponges were not soaked with bobcat urine.
During the experiment, the rats performed PST once a day. Each session had 20 trials, the sessions ended when the last trial was completed or when 20 min passed. P1 and P2 lasted 12 days; we measured performance of each rat during 1, 11, and Day 12 (see Figure 2A). During those days, we recorded the number of wins (rewards delivered) and loses (punishment received).
In the experimental group, Day 1 was the day when a novel pair of stimuli was presented for the first time. Day 12 was the last day with a pair of known stimuli, but with changed environmental factors (odor or no odor exposure). Thus, P1 Day 1 was the first day when stimuli BC were displayed during PST, without exposure to odor. Day 11 of P1 was the day when stimuli BC were displayed without odor for the last time. P1 Day 12 was the day when BC stimuli were displayed for the last time, but this time with odor exposure. Accordingly, Day 1 ofP2 was the first day when stimuli DE were displayed during PST sessions, with odor exposure. P2 Day 11 was the day when DE stimuli were displayed with odor for the last time. P2 Day 12 was the day when stimuli DE were displayed for the last time, but this time without exposure to odor (see Figure 2A).
Video records were scored by the independent observer, who used stopwatch to measure the time spent in the Interaction Zone of each rat. Interaction Zone was outlined on the SIT floor. Crossing the line with hind limbs was considered as entry into the Interaction Zone.
Analysis and interpretation of behavioral data acquired via PST is commonly aided by different variants of theoretical Q learning models (Frank et al., 2004; Frank, 2006; Frank and Claus, 2006; Brown et al., 2018; Kane et al., 2019; Metha et al., 2020). In this way, the research hypothesis is expressed as a set of mathematical equations that govern the analysis of the data. However, the theoretical model introduces its own assumptions and requires advanced routines to adjust the model to the dataset, which may bias the results in an unpredictable manner. Since our study involves a small amount of data, we decided to rely only on directly measurable variables, making the analysis model independent; thus, we present our data without a computational framework.
The test score of each individual was calculated during Days 1, 11, and 12—ratio of the gained rewards to all trials taken that day
where Nrewards Dx is the total number of rewards received during day X (Dx) and N losses Dx is the total number of punishment received during day X (Dx).
Then, we calculated the WinRatio of each individual for P1 and P2. WinRatio was a difference between Test score Day 11 and Test Score Day 1:
Day 1 and Day 11 test scores (used to calculate individual WinRatios) are presented in Figure 3A. Each rat’s P1 WinRatio and P2 WinRatio is presented numerically in Figure 3B. Days 11 and 12 test scores are presented in Figure 4.
Figure 3. (A) Test scores of each individual (obtained in Days 1 and 11) used to calculate P1 and P2 WinRatios. (B) Numerical representation of the overall WinRatio of each individual in P1 and P2.
Figure 4. Test scores gained during PST in the last 2 days of each phase. For the experimental group, P1 Day 11 was the day with known stimuli in PST and no odor, but the P1 Day 12 was the first exposure to odor, with stimuli known from previous days. Inversely, P2 Day 11 was the day with known stimuli in PST with odor, while P2 Day 12 was the day with known stimuli in PST, but without odor exposure.
Due to a low number of rats and possibly non-normal distribution of variables, we used non-parametric statistical tests. To compare the performance of PST during P1 and P2 of the same rat, we used the Wilcoxon two-sided test. In cross-group comparisons, the U-Mann-Whitney two-sided test was used. Behavioral results were analyzed using the Wilcoxon two-sided test to compare times each rat spent in an Interaction Zone before and after the trauma, U-Mann-Whitney two-sided test was applied for cross-group comparisons. The statistical significance level was established at p < 0.05.
Statistical analysis was performed using the scipy.stats library belonging to the Python programming language ecosystem.1
With each individual’s WinRatio for P1 (no odor) and P2 (with odor), we compared reinforcement learning before (P1) and after (P2) exposure to trauma in the experimental group, as well as reinforcement learning in the control group (see Figure 3B). In the experimental group, WinRatio during P1 was significantly greater than during P2 (Wilcoxon test, p = 0.01). In the control group, there was no significant difference in WinRatio between P1 and P2 (Wilcoxon Two-Sided Test, p = 0.73). In cross-group comparisons, the control group had a higher P2 WinRatio than experimental group P2 WinRatio (U Mann-Whitney Two-sided, p = 0.0005). There was no significant difference between the experimental P1 WinRatio and the control P1 WinRatio (two-sided Mann-Whitney U, p = 0.909). In general, both groups WinRatios are presented in Figure 5A.
Figure 5. (A) Rewards collected in PST throughout the entire experiment. (B) Time spent in an Interaction Zone in SIT, comparison between two groups of rats. (C) Time spent in an Interaction Zone during SIT (experimental group). Raw measurements data are drawn as diamonds. For easy visual groups comparison we provided kernel density estimates of Probability Density Functions (Silverman, 2018). The experimental group spent significantly more time in an Interaction Zone before trauma exposure (P1). Furthermore, exposure to trauma (P2) induced a bimodal data distribution that has not occurred in P1. (D) The experimental group P2 times compared to the control group P2 times. There is no significant differences in group comparisons (p = 0.91), but this may be due to bimodality that characterizes post stress-performance of the experimental group.
The test score was calculated for Day 12 in P1 and P2 (see Figure 4). In the experimental group, the P1 Day 12 Test score was significantly worse than the P1 Day 11 test score (Wilcoxon Test, p = 0.034). In the control group, the P1 Day 12 Test score was similar to the P1 Day 11 test score (Wilcoxon two-sided, p = 0.0557). In the experimental group, day 12 P1 and day 12 P2 Day 12 did not differ (Wilcoxon, two sides, p = 1.0). In the control group, Day 12 P1 and Day 12 P2 Day 12 did not differ significantly (Wilcoxon two-sided, p = 0.314). In cross-group comparisons, the experimental group P1 Day 12 test score was significantly lower than in the control group (U Mann-Whitney, p = 0.003).
Figure 5B present differences in the time spent in an Interaction Zone of SIT in P1 and P2. The experimental group spent significantly more time in the Interaction Zone before trauma (P1) compared to time spent in Interaction Zone after predator odor (P2) (Wilcoxon two-sided test, p = 0.019). In the control group, there were no significant differences in the time spent in an Interaction Zone during P1 and P2 (Wilcoxon two-sided test, p = 0.43). During P1, the experimental group spent similar time in an Interaction Zone to the control group (Wilcoxon two-sided test, p = 0.038). Similarly, cross-group comparisons did not reveal differences between both groups in time spent in an Interaction Zone during P2 (Wilcoxon two-sided test, p = 0.91) (see Figure 5B).
In our study, we examined reinforcement learning (through PST) before and after trauma and compared obtained results with the untraumatized control group. In the experimental group, exposure to chronic trauma (which occurred every day for 12 consecutive days) significantly reduced the ability to perform on PST. The decline in cognitive ability was significant immediately after the first exposure to trauma, although this result is not surprising. Previous findings indicate that single exposure to predator odor is sufficient to induce a behavioral and physiological response such as avoidance (Albrechet-Souza and Gilpin, 2019) or an increase in alcohol intake (Edwards et al., 2013). To our knowledge, we are the first to report a decline in reinforcement learning immediately after exposure to predator odor. We did not find a significant improvement in PST performance 1 day after the odor removal. This result stays in line with studies reporting that the consequences of odor exposure persist weeks after initial exposure (Albrechet-Souza and Gilpin, 2019; Schreiber et al., 2019). To the best of our knowledge, our study is the first to examine rodent cognitive abilities via PST before and after exposure to predator odor. Moreover, our study confirmed bobcat urine utility as a traumatizing factor, as it significantly affected cognitive abilities, and influenced social behavior among rats exposed to odor.
Overall, the control group performed significantly better in P2 of the experiment. During that period of time, the experimental group was chronically exposed to predator odor. This enforced vigilance and anxiety among rats, which resulted in significant deterioration in PST performance, even though neither punishment nor the physical threat was ever delivered. There are numerous animal models with severe physical punishments, for example foot shock, underwater trauma, restrained stress (Whitaker et al., 2014). Our model is not one of them; the punishment was the lack of the reward. In humans, there are protocols that expose subjects to the possibility of punishment that is never delivered. These studies confirm that anticipation stress reduces reward sensitivity, reward responsiveness (Bogdan and Pizzagalli, 2006; Berghorst et al., 2013) and generally impairs reinforcement learning (Cavanagh et al., 2011). Interestingly, it is hypothesized that stress-susceptible individuals may be more vulnerable to punishment than reward collection (Berghorst et al., 2013). In that case, our protocol (which did not present tangible punishment) may have been less perceptive to those subjects. On the other hand, literature implies that individuals who are less stress-susceptible may be more vulnerable to reward collection than to punishment deliverance (Cavanagh et al., 2011), an observation that validates our approach. This distinction in susceptibility is discussed to be related to striatal dopamine levels, which are known to guide decision making in relation to learning from positive and negative stimuli. Patients with pharmacologically elevated dopamine levels learn better from rewards in PST, compared to those with reduced dopamine levels, who learn better to avoid punishment in PST (Frank et al., 2004). Thus, we hypothesize that the experimental group performed in PST poorer in P2, due to disrupted dopamine levels in the striatum. This implies decline in PST was related to the disruption in reward learning circuits. In humans, exposure to chronic stressors results in blunted ventral striatal (VS) neural activity during reward processing in healthy individuals (Nikolova et al., 2012), as well as in those with PTSD (Mehta et al., 2020). The prominent function of dopaminergic VS neurotransmission in reinforcement learning was confirmed in human positron emission tomography studies that mark right caudate and VS as motivational centers of engagement in activity that brings profit (Kasanova et al., 2017). Stress-related blunted dopaminergic neurotransmission results in overall worse performance in PST, a phenomenon that was observed among individuals with a familial risk of psychosis. Thus, disruption in VS is often symptomatically related to anhedonia, depression, and motivation deficits in both humans and animals (Malone et al., 2009; Roesch et al., 2009; Corral-Frías et al., 2015). We hypothesize that chronic trauma, induced in the experimental group, reduced dopamine level in VS that decreased the performance of experimental rats in PST P2. Our protocol delivered chronic trauma that compromised reward learning, but behaviorally did not induce full-blown anhedonia. We believe that is an important advantage of our model—rats perform voluntarily, which facilitates measurement of cognitive and behavioral deficits in rodents.
Our results are in agreement with studies that indicate deterioration in cognitive abilities among those exposed to trauma. Schizophrenia patients with a history of trauma exhibit poorer cognitive functioning in terms of memory, executive functions, attention, concentration, and mental speed (Misiak et al., 2017). Computational studies present altered reinforcement learning in veterans with diagnosed PTSD, indicating alteration in reward and punishment perception and valuation (Myers et al., 2013; Brown et al., 2018). Moreover, individuals with PTSD have increased sensitivity to an unexpected outcome during PST (Brown et al., 2018). To our knowledge, this phenomenon has not been validated in animal models, although we believe our protocol may be in use in further research of this topic. If this mechanism of overreaction to an unexpected outcome occurs in the rodent model of trauma, it could have explained the deterioration in learning during P2. We hypothesize that our traumatized subjects were more susceptible to unexpected punishment in P2—as feedback was probabilistic, rewarding stimulus rarely delivered punishment. To test this hypothesis in the future, our protocol needs to be recreated using a computational model.
In SIT, the experimental group proved to be less socially oriented in P2, in comparison to P1—after trauma, rats spent less time in an Interaction Zone with an unfamiliar rat. In humans, chronic trauma influences social interactions, especially in children. Youngsters exposed to chronic traumatic stress present substantial difficulties in constructing relationships. They have troubles in interactions with other children as they often display avoidant symptoms, present inadequately sensitive flight/fight responses, respond to minor stressors by freezing (Streeck-Fischer and van der Kolk, 2000). In another study, adults with PTSD after 2-years of military deployments presented avoidance behavior, social withdrawal, had less positive engagement in relation with their families during post-deployment reengagement (Brockman et al., 2016). In rodent, chronic social defeat model reveals significant decreases in interpersonal interactions after exposure to trauma (Venzala et al., 2012). We believe results obtained during our experiment stays in line with these reports. We hypothesize that it may be related to dopamine disruption, since social behavior in rodents has been shown to be strongly dependent on neural activity in the ventral tegmental area (VTA) of the brain (Chaudhury et al., 2013). Dopamine neurons in VTA project signals to different structures in the striatum (for example, nucleus accumbens) as the well as amygdala or medial prefrontal cortex. Manipulation in neural projection dynamics of VTA influences social interactions in rodents (Gunaydin et al., 2014); therefore, we hypothesize that our trauma protocol disrupts dopamine levels in the midbrain, which results in reduced social behavior after exposition. In the control group, there was no significant difference in SIT performance in P1 and P2, as rats were not exposed to trauma. Similarly, there was no significant difference in the performance of the experimental group P1 and the control group P1 in SIT, as none of the subjects was exposed to predator odor. Although the experimental group spent significantly more time in an Interaction Zone during P1 in comparison with P2, statistical analysis does not reveal differences in time spent in an Interaction Zone between experimental group and the control during P2. This result is inconclusive—two factors have to be taken into consideration. First, a performance difference was observed (see Figure 5B), but we cannot support this with statistical verification, probably due to the small number of rats tested. Second, the distribution of the time spent in an Interaction Zone among rats exposed to trauma was bimodal (see Figure 5C). This makes the verification of this particular result ambiguous, as a control group did not present this tendency (see Figure 5D). This may be a random result, as the group was small in number, but it may also be hypothesized that exposition to trauma divided the experimental group into two subgroups; individuals more susceptible to chronic trauma (less time in an Interaction Zone) and those more resilient (more time in an Interaction Zone). This requires further verification with a larger group, but if confirmed, that would imply that SIT shows individual variability in reactivity to stress induced by predator odor.
There are components of our research that should be expanded. As discussed earlier, a categorization is often applied in human studies of the subject, where individuals are characterized as stress-susceptible or resilient. We believe that our protocol could benefit if such a distinction was applied. A viable possibility may be the Avoiders/Non-Avoiders distinction proposed by Albrechet-Souza and Gilpin (2019) in their animal model of PTSD, or a hypothesized distinction delivered by SIT, as we discussed in paragraph above. While rats were in PST chambers, we did not videotape their activity. This is why we could not provide behavioral data from that time-period, that might have been interesting. Our conclusions regarding dopamine-related VTA and VS activity need further verification by molecular studies in animal models. Furthermore, there are interesting reports on striatal activity heavily influenced by increased inflammatory biomarkers, in the context of trauma (Mehta et al., 2020). We believe that our protocol could be of use in further exploration of these topics.
We believe further studies with our protocol should apply an additional group of rats exposed to non-predator odor. This could validate our approach with bobcat urine as a stressor, and deliver much needed comparative context. Changes in rodent behavior could be explored in exposure to different odors, for example alpha-pinene or green leaf odor that are known to have stress-alleviating effects (Akutsu et al., 2003). Studies that use different odors to examine behavioral and cognitive changes are sparse, thus we hypothesize our protocol could be of use to study this subject. We believe this comparative context would deliver interesting results in the wide issue of rodents behavioral and cognitive performance analysis.
We present our protocol that may be useful in assessing cognitive abilities in rodents. Rats performed PST voluntarily, when exposed to chronic trauma induced by predator odor. Performance in PST was measured before and after trauma in the same group of rats. Subjects obtained better results in PST before exposure to predator odor. Overall, the experimental group scored lower in PST compared to not-traumatized control. After exposure to chronic trauma, rats were less socially oriented in SIT, compared to the results obtained before the trauma protocol. Moreover, traumatized rats presented a bimodal tendency in time spent in an Interaction Zone with unknown rat, but due to a small number of animals tested, this result needs further verification.
The original contributions presented in the study are included in the article/supplementary material, further inquiries can be directed to the corresponding author/s.
The animal study was reviewed and approved by the Local Ethics Committee for Animal Experiments, Hirszfeld Institute of Immunology and Experimental Therapy, Polish Academy of Sciences, Wrocław, Poland.
TB designed the research, carried out laboratory experiments, and wrote the manuscript with input from all authors. PK designed and constructed Testing Chambers. JD performed mathematical calculations and analyzed obtained data. BS delivered theoretical framework. DF provided critical feedback and helped shape the research.
This study was sponsored by the Wrocław Medical University (Grant no. STM.c230.18.037).
The authors declare that the research was conducted in the absence of any commercial or financial relationships that could be construed as a potential conflict of interest.
All claims expressed in this article are solely those of the authors and do not necessarily represent those of their affiliated organizations, or those of the publisher, the editors and the reviewers. Any product that may be evaluated in this article, or claim that may be made by its manufacturer, is not guaranteed or endorsed by the publisher.
Aas, M., Pizzagalli, D. A., Laskemoen, J. F., Reponen, E. J., Ueland, T., Melle, I., et al. (2019). Elevated hair cortisol is associated with childhood maltreatment and cognitive impairment in schizophrenia and in bipolar disorders. Schizophrenia Res. 213, 65–71. doi: 10.1016/j.schres.2019.01.011
Akutsu, H., Kikusui, T., Takeuchi, Y., and Mori, Y. (2003). Effects of alpha-pinene odor in different concentrations on stress-induced hyperthermia in rats. J. Vet. Med. Sci. 65, 1023–1025. doi: 10.1292/jvms.65.1023
Albrechet-Souza, L., and Gilpin, N. W. (2019). The predator odor avoidance model of post-traumatic stress disorder in rats. Behav. Pharmacol. 30, 105–114. doi: 10.1097/FBP.0000000000000460
Albrechet-Souza, L., Nastase, A. S., Hill, M. N., and Gilpin, N. W. (2021). Amygdalar endocannabinoids are affected by predator odor stress in a sex-specific manner and modulate acoustic startle reactivity in female rats. Neurobiol. Stress 15:100387. doi: 10.1016/j.ynstr.2021.100387
Albrechet-Souza, L., Schratz, C. L., and Gilpin, N. W. (2020). Sex differences in traumatic stress reactivity in rats with and without a history of alcohol drinking. Biol. Sex Differ. 11:27. doi: 10.1186/s13293-020-00303-w
Amitai, N., Young, J. W., Higa, K., Sharp, R. F., Geyer, M. A., and Powell, S. B. (2014). Isolation rearing effects on probabilistic learning and cognitive flexibility in rats. Cogn. Affect. Behav. Neurosci. 14, 388–406. doi: 10.3758/s13415-013-0204-4
Assogna, F., Piras, F., and Spalletta, G. (2020). “Neurobiological Basis of Childhood Trauma and the Risk for Neurological Deficits Later in Life,” in Childhood Trauma in Mental Disorders: A Comprehensive Approach, eds G. Spalletta, D. Janiri, F. Piras, and G. Sani (Cham: Springer International Publishing), 385–410. doi: 10.1007/978-3-030-49414-8_18
Ay, R., and Erbay, L. G. (2018). Relationship between childhood trauma and suicide probability in obsessive-compulsive disorder. Psychiatr. Res. 261, 132–136. doi: 10.1016/j.psychres.2017.12.054
Bailey, T., Alvarez-Jimenez, M., Garcia-Sanchez, A. M., Hulbert, C., Barlow, E., and Bendall, S. (2018). Childhood Trauma Is Associated With Severity of Hallucinations and Delusions in Psychotic Disorders: A Systematic Review and Meta-Analysis. Schizophrenia Bull. 44, 1111–1122. doi: 10.1093/schbul/sbx161
Berghorst, L., Bogdan, R., Frank, M., and Pizzagalli, D. (2013). Acute stress selectively reduces reward sensitivity. Front. Hum. Neurosci. 7:133 doi: 10.3389/fnhum.2013.00133
Bielawski, T., Misiak, B., Moustafa, A., and Frydecka, D. (2019). Epigenetic mechanisms, trauma, and psychopathology: targeting chromatin remodeling complexes. Rev.Neurosci. 30, 595–604. doi: 10.1515/revneuro-2018-0055
Bogdan, R., and Pizzagalli, D. A. (2006). Acute Stress Reduces Reward Responsiveness: Implications for Depression. Biol. Psychiatr. 60, 1147–1154. doi: 10.1016/j.biopsych.2006.03.037
Breslau, N. (2009). The Epidemiology of Trauma. PTSD, and Other Posttrauma Disorders. Trauma Violence Abuse 10, 198–210. doi: 10.1177/1524838009334448
Brockman, C., Snyder, J., Gewirtz, A., Gird, S. R., Quattlebaum, J., Schmidt, N., et al. (2016). Relationship of Service Members’ Deployment Trauma, PTSD Symptoms and Experiential Avoidance to Postdeployment Family Reengagement. J. Fam. Psychol. 30, 52–62. doi: 10.1037/fam0000152
Brown, V. M., Zhu, L., Wang, J. M., Frueh, B. C., King-Casas, B., and Chiu, P. H. (2018). Associability-modulated loss learning is increased in posttraumatic stress disorder. eLife 7:e30150. doi: 10.7554/eLife.30150
Cavanagh, J. F., Frank, M. J., and Allen, J. J. B. (2011). Social stress reactivity alters reward and punishment learning. Soc. Cogn. Affect. Neurosci. 6, 311–320. doi: 10.1093/scan/nsq041
Chaudhury, D., Walsh, J. J., Friedman, A. K., Juarez, B., Ku, S. M., Koo, J. W., et al. (2013). Rapid regulation of depression-related behaviours by control of midbrain dopamine neurons. Nature 493, 532–536. doi: 10.1038/nature11713
Cohen, H., Kozlovsky, N., Alona, C., Matar, M. A., and Joseph, Z. (2012). Animal model for PTSD: From clinical concept to translational research. Neuropharmacology 62, 715–724. doi: 10.1016/j.neuropharm.2011.04.023
Corral-Frías, N. S., Nikolova, Y. S., Michalski, L. J., Baranger, D. A. A., Hariri, A. R., and Bogdan, R. (2015). Stress-related anhedonia is associated with ventral striatum reactivity to reward and transdiagnostic psychiatric symptomatology. Psychol. Med. 45, 2605–2617. doi: 10.1017/S0033291715000525
Davidson, L. M., and Baum, A. (1986). Chronic stress and posttraumatic stress disorders. J. Consult. Clin. Psychol. 54, 303–308. doi: 10.1037/0022-006X.54.3.303
Dennison, U., McKernan, D., Cryan, J., and Dinan, T. (2012). Schizophrenia patients with a history of childhood trauma have a pro-inflammatory phenotype. Psychol. Med. 42, 1865–1871. doi: 10.1017/S0033291712000074
Duhig, M., Patterson, S., Connell, M., Foley, S., Capra, C., Dark, F., et al. (2015). The prevalence and correlates of childhood trauma in patients with early psychosis. Aust. N.Z.J. Psychiatr. 49, 651–659. doi: 10.1177/0004867415575379
Edwards, S., Baynes, B. B., Carmichael, C. Y., Zamora-Martinez, E. R., Barrus, M., Koob, G. F., et al. (2013). Traumatic stress reactivity promotes excessive alcohol drinking and alters the balance of prefrontal cortex-amygdala activity. Transl. Psychiatr. 3, e296–e296. doi: 10.1038/tp.2013.70
Éthier, L. S., Lemelin, J.-P., and Lacharité, C. (2004). A longitudinal study of the effects of chronic maltreatment on children’s behavioral and emotional problems. Child Abuse Neglect 28, 1265–1278. doi: 10.1016/j.chiabu.2004.07.006
Farag, Y. M. K., and Gaballa, M. R. (2011). Diabesity: an overview of a rising epidemic. Nephrol. Dialysis Transplant. 26, 28–35. doi: 10.1093/ndt/gfq576
Ferrero, D. M., Lemon, J. K., Fluegge, D., Pashkovski, S. L., Korzan, W. J., Datta, S. R., et al. (2011). Detection and avoidance of a carnivore odor by prey. Proc.Natl. Acad. Sci. 108, 11235–11240. doi: 10.1073/pnas.1103317108
File, S. E., and Seth, P. (2003). A review of 25 years of the social interaction test. Eur. J. Pharmacol. 463, 35–53. doi: 10.1016/S0014-2999(03)01273-1
Frank, M. J. (2006). Hold your horses: A dynamic computational role for the subthalamic nucleus in decision making. Neural. Networks 19, 1120–1136. doi: 10.1016/j.neunet.2006.03.006
Frank, M. J., and Claus, E. D. (2006). Anatomy of a decision: Striato-orbitofrontal interactions in reinforcement learning, decision making, and reversal. Psychol. Rev. 113, 300–326. doi: 10.1037/0033-295X.113.2.300
Frank, M. J., Moustafa, A. A., Haughey, H. M., Curran, T., and Hutchison, K. E. (2007). Genetic triple dissociation reveals multiple roles for dopamine in reinforcement learning. Proc.Natl. Acad. Sci. 104, 16311–16316. doi: 10.1073/pnas.0706111104
Frank, M. J., Seeberger, L. C., and O’reilly, R. C. (2004). By carrot or by stick: cognitive reinforcement learning in parkinsonism. Science 306, 1940–1943. doi: 10.1126/science.1102941
George, S. A., Rodriguez-Santiago, M., Riley, J., Abelson, J. L., Floresco, S. B., and Liberzon, I. (2015). Alterations in cognitive flexibility in a rat model of post-traumatic stress disorder. Behav. Brain Res. 286, 256–264. doi: 10.1016/j.bbr.2015.02.051
Golden, S. A., Covington, H. E., Berton, O., and Russo, S. J. (2011). A standardized protocol for repeated social defeat stress in mice. Nat. Protoc. 6, 1183–1191. doi: 10.1038/nprot.2011.361
Gunaydin, L. A., Grosenick, L., Finkelstein, J. C., Kauvar, I. V., Fenno, L. E., Adhikari, A., et al. (2014). Natural Neural Projection Dynamics Underlying Social Behavior. Cell 157, 1535–1551. doi: 10.1016/j.cell.2014.05.017
Harro, J. (2018). Animals, anxiety, and anxiety disorders: How to measure anxiety in rodents and why. Behav. Brain Res. 352, 81–93. doi: 10.1016/j.bbr.2017.10.016
Horner, A. E., Heath, C. J., Hvoslef-Eide, M., Kent, B. A., Kim, C. H., Nilsson, S. R., et al. (2013). The touchscreen operant platform for testing learning and memory in rats and mice. Nat. Protoc. 8, 1961–1984.
Jansen, K., Cardoso, T. A., Fries, G. R., Branco, J. C., Silva, R. A., Kauer-Sant’Anna, M., et al. (2016). Childhood trauma, family history, and their association with mood disorders in early adulthood. Acta Psychiatr. Scand. 134, 281–286. doi: 10.1111/a.12551
Jocham, G., Klein, T. A., and Ullsperger, M. (2011). Dopamine-Mediated Reinforcement Learning Signals in the Striatum and Ventromedial Prefrontal Cortex Underlie Value-Based Choices. J. Neurosci. 31, 1606–1613. doi: 10.1523/JNEUROSCI.3904-10.2011
Joëls, M., Sarabdjitsingh, R. A., and Karst, H. (2012). Unraveling the Time Domains of Corticosteroid Hormone Influences on Brain Activity: Rapid. Slow, and Chronic Modes. Pharmacol. Rev. 64, 901–938. doi: 10.1124/pr.112.005892
Kane, G. A., Bornstein, A. M., Shenhav, A., Wilson, R. C., Daw, N. D., and Cohen, J. D. (2019). Rats exhibit similar biases in foraging and intertemporal choice tasks. eLife 8:e48429. doi: 10.7554/eLife.48429
Kasanova, Z., Ceccarini, J., Frank, M. J., Amelsvoort, T., van, Booij, J., et al. (2017). Striatal dopaminergic modulation of reinforcement learning predicts reward—oriented behavior in daily life. Biol. Psychol. 127, 1–9. doi: 10.1016/j.biopsycho.2017.04.014
Kasanova, Z., Ceccarini, J., Frank, M. J., van Amelsvoort, T., Booij, J., Heinzel, A., et al. (2018). Daily-life stress differentially impacts ventral striatal dopaminergic modulation of reward processing in first-degree relatives of individuals with psychosis. Eur. Neuropsychopharmacol. 28, 1314–1324. doi: 10.1016/j.euroneuro.2018.10.002
Knox, D., George, S. A., Fitzpatrick, C. J., Rabinak, C. A., Maren, S., and Liberzon, I. (2012). Single prolonged stress disrupts retention of extinguished fear in rats. Learn. Mem. 19, 43–49. doi: 10.1101/lm.024356.111
Kondoh, K., Lu, Z., Ye, X., Olson, D. P., Lowell, B. B., and Buck, L. B. (2016). A specific area of olfactory cortex involved in stress hormone responses to predator odours. Nature 532, 103–106. doi: 10.1038/nature17156
Krishnan, V., Han, M.-H., Graham, D. L., Berton, O., Renthal, W., Russo, S. J., et al. (2007). Molecular Adaptations Underlying Susceptibility and Resistance to Social Defeat in Brain Reward Regions. Cell 131, 391–404. doi: 10.1016/j.cell.2007.09.018
Lamontagne, S. J., Pizzagalli, D. A., and Olmstead, M. C. (2021). Does inflammation link stress to poor COVID-19 outcome? Stress Health 37, 401–414. doi: 10.1002/smi.3017
Laramée, P., Kusel, J., Leonard, S., Aubin, H.-J., François, C., and Daeppen, J.-B. (2013). The Economic Burden of Alcohol Dependence in Europe. Alcohol Alcohol. 48, 259–269. doi: 10.1093/alcalc/agt004
Lysaker, P., Meyer, P., Evans, J., and Marks, K. (2001). Neurocognitive and Symptom Correlates of Self-Reported Childhood Sexual Abuse in Schizophrenia Spectrum Disorders. Ann. Clin. Psychiatr. 13, 89–92. doi: 10.3109/10401230109148953
Majer, M., Nater, U. M., Lin, J.-M. S., Capuron, L., and Reeves, W. C. (2010). Association of childhood trauma with cognitive function in healthy adults: a pilot study. BMC Neurol. 10:61. doi: 10.1186/1471-2377-10-61
Malone, D. A., Dougherty, D. D., Rezai, A. R., Carpenter, L. L., Friehs, G. M., Eskandar, E. N., et al. (2009). Deep Brain Stimulation of the Ventral Capsule/Ventral Striatum for Treatment-Resistant Depression. Biol. Psychiatr. 65, 267–275. doi: 10.1016/j.biopsych.2008.08.029
Masodkar, K., Johnson, J., and Peterson, M. J. (2016). A Review of Posttraumatic Stress Disorder and Obesity: Exploring the Link. Prim. Care Companion CNS Dis. 18:10.4088/PCC.15r01848 doi: 10.4088/PCC.15r01848
Matosin, N., Cruceanu, C., and Binder, E. B. (2017). Preclinical and Clinical Evidence of DNA Methylation Changes in Response to Trauma and Chronic Stress. Chronic. Stress 1:2470547017710764. doi: 10.1177/2470547017710764
McEwen, B. S. (2017). Neurobiological and Systemic Effects of Chronic Stress. Chronic Stress 1:2470547017692328. doi: 10.1177/2470547017692328
McLaughlin, K. A., Koenen, K. C., Friedman, M. J., Ruscio, A. M., Karam, E. G., Shahly, V., et al. (2015). Sub-threshold Post Traumatic Stress Disorder in the WHO World Mental Health Surveys. Biol. Psychiatr. 77, 375–384. doi: 10.1016/j.biopsych.2014.03.028
Mehta, N. D., Stevens, J. S., Li, Z., Gillespie, C. F., Fani, N., Michopoulos, V., et al. (2020). Inflammation, reward circuitry and symptoms of anhedonia and PTSD in trauma-exposed women. Soc. Cogn. Affect. Neurosci. 15, 1046–1055. doi: 10.1093/scan/nsz100
Metha, J. A., Brian, M. L., Oberrauch, S., Barnes, S. A., Featherby, T. J., Bossaerts, P., et al. (2020). Separating Probability and Reversal Learning in a Novel Probabilistic Reversal Learning Task for Mice. Front. Behav. Neurosci. 13:270. doi: 10.3389/fnbeh.2019.00270
Misiak, B., Krefft, M., Bielawski, T., Moustafa, A. A., Sąsiadek, M. M., and Frydecka, D. (2017). Toward a unified theory of childhood trauma and psychosis: A comprehensive review of epidemiological, clinical, neuropsychological and biological findings. Neurosci. Biobehav. Rev. 75, 393–406. doi: 10.1016/j.neubiorev.2017.02.015
Mota, N., Tsai, J., Kirwin, P. D., Harpaz-Rotem, I., Krystal, J. H., Southwick, S. M., et al. (2016). Late-Life Exacerbation of PTSD Symptoms in US Veterans: Results From the National Health and Resilience in Veterans Study. J. Clin. Psychiatr. 77:10211. doi: 10.4088/JCP.15m10101
Myers, C. E., Moustafa, A. A., Sheynin, J., VanMeenen, K. M., Gilbertson, M. W., Orr, S. P., et al. (2013). Learning to Obtain Reward, but Not Avoid Punishment, Is Affected by Presence of PTSD Symptoms in Male Veterans: Empirical Data and Computational Model. PLoS One 8:e72508. doi: 10.1371/journal.pone.0072508
Nikolova, Y., Bogdan, R., and Pizzagalli, D. A. (2012). Perception of a Naturalistic Stressor Interacts with 5-HTTLPR/rs25531 Genotype and Gender to Impact Reward Responsiveness. Neuropsychobiology 65, 45–54. doi: 10.1159/000329105
Petkus, A. J., Lenze, E. J., Butters, M. A., Twamley, E. W., and Wetherell, J. L. (2018). Childhood Trauma is Associated with Poorer Cognitive Performance in Older Adults. J. Clin. Psychiatr. 79:16m11021. doi: 10.4088/JCP.16m11021
Planchez, B., Surget, A., and Belzung, C. (2019). Animal models of major depression: drawbacks and challenges. J. Neural. Transm. 126, 1383–1408. doi: 10.1007/s00702-019-02084-y
Pruessner, M., Bechard-Evans, L., Pira, S., Joober, R., Collins, D. L., Pruessner, J. C., et al. (2017). Interplay of hippocampal volume and hypothalamus-pituitary-adrenal axis function as markers of stress vulnerability in men at ultra-high risk for psychosis. Psychol. Med. 47, 471–483. doi: 10.1017/S0033291716002658
Reber, S. O., Langgartner, D., Foertsch, S., Postolache, T. T., Brenner, L. A., Guendel, H., et al. (2016). Chronic subordinate colony housing paradigm: A mouse model for mechanisms of PTSD vulnerability, targeted prevention, and treatment—2016 Curt Richter Award Paper. Psychoneuroendocrinol. 74, 221–230. doi: 10.1016/j.psyneuen.2016.08.031
Reinen, J. M., Whitton, A. E., Pizzagalli, D. A., Slifstein, M., Abi-Dargham, A., McGrath, P. J., et al. (2021). Differential reinforcement learning responses to positive and negative information in unmedicated individuals with depression. Eur. Neuropsychopharmacol. 53, 89–100. doi: 10.1016/j.euroneuro.2021.08.002
Roesch, M. R., Singh, T., Brown, P. L., Mullins, S. E., and Schoenbaum, G. (2009). Ventral Striatal Neurons Encode the Value of the Chosen Action in Rats Deciding between Differently Delayed or Sized Rewards. J. Neurosci. 29, 13365–13376. doi: 10.1523/JNEUROSCI.2572-09.2009
Roehr, B. (2013). American psychiatric association explains DSM-5. BMJ 346:f3591. doi: 10.1136/bmj.f3591
Ross, R. A., Foster, S. L., and Ionescu, D. F. (2017). The Role of Chronic Stress in Anxious Depression. Chronic Stress 1:247054701668947. doi: 10.1177/2470547016689472
Rothgeb, C. L. (1971). Standard Edition of the Complete Psychological Works of Sigmund Freud. Available online at: https://eric.ed.gov/?id=ED062645. (accessed February 25, 2022).
Saavedra-Rodríguez, L., and Feig, L. A. (2013). Chronic Social Instability Induces Anxiety and Defective Social Interactions Across Generations. Biol. Psychiatr. 73, 44–53. doi: 10.1016/j.biopsych.2012.06.035
Salcioglu, E., Urhan, S., Pirinccioglu, T., and Aydin, S. (2017). Anticipatory fear and helplessness predict PTSD and depression in domestic violence survivors. Psychol. Trauma 9, 117–125. doi: 10.1037/tra0000200
Schenkel, L. S., Spaulding, W. D., DiLillo, D., and Silverstein, S. M. (2005). Histories of childhood maltreatment in schizophrenia: Relationships with premorbid functioning, symptomatology, and cognitive deficits. Schizophrenia Res. 76, 273–286. doi: 10.1016/j.schres.2005.03.003
Schreiber, A. L., McGinn, M. A., Edwards, S., and Gilpin, N. W. (2019). Predator Odor Stress Blunts Alcohol Conditioned Aversion. Neuropharmacology 144, 82–90. doi: 10.1016/j.neuropharm.2018.10.019
Seib, D. R., Espinueva, D. F., Floresco, S. B., and Snyder, J. S. (2020). A role for neurogenesis in probabilistic reward learning. Behav. Neurosci. 134, 283–295. doi: 10.1037/bne0000370
Shannon, C., Douse, K., McCusker, C., Feeney, L., Barrett, S., and Mulholland, C. (2011). The Association Between Childhood Trauma and Memory Functioning in Schizophrenia. Schizophrenia Bull. 37, 531–537. doi: 10.1093/schbul/sbp096
Sharhabani-Arzy, R., Amir, M., Kotler, M., and Liran, R. (2003). The Toll of Domestic Violence: PTSD among Battered Women in an Israeli Sample. J. Interpers. Violence 18, 1335–1346. doi: 10.1177/0886260503256842
Silverman, B. W. (2018). Density Estimation for Statistics and Data Analysis. New York, NY: Routledge.
Simon, G. E., Von Korff, M., Saunders, K., Miglioretti, D. L., Crane, P. K., van Belle, G., et al. (2006). Association Between Obesity and Psychiatric Disorders in the US Adult Population. Arch. Gen. Psychiatr. 63, 824–830. doi: 10.1001/archpsyc.63.7.824
Sinha, R. (2008). Chronic Stress, Drug Use, and Vulnerability to Addiction. Ann.N.Y Acad. Sci. 1141, 105–130. doi: 10.1196/annals.1441.030
Streeck-Fischer, A., and van der Kolk, B. A. (2000). Down will come baby, cradle and all: diagnostic and therapeutic implications of chronic trauma on child development. Aus. N.Z. J. Psychiatr. 34, 903–918. doi: 10.1080/000486700265
Toyoda, A. (2017). Social defeat models in animal science: What we have learned from rodent models. Anim. Sci. J. 88, 944–952 doi: 10.1111/asj.12809
Vasilevski, V., and Tucker, A. (2016). Wide-ranging cognitive deficits in adolescents following early life maltreatment. Neuropsychology 30, 239–246. doi: 10.1037/neu0000215
Veeser, J., Barkmann, C., Schumacher, L., Zindler, A., Schön, G., and Barthel, D. (2021). Post-traumatic stress disorder in refugee minors in an outpatient care center: prevalence and associated factors. Eur. Child Adolesc. Psychiatr. [Epub ahead of print]. doi: 10.1007/s00787-021-01866-8
Venzala, E., García-García, A. L., Elizalde, N., Delagrange, P., and Tordera, R. M. (2012). Chronic social defeat stress model: behavioral features, antidepressant action, and interaction with biological risk factors. Psychopharmacology 224, 313–325. doi: 10.1007/s00213-012-2754-5
Wang, W., Liu, W., Duan, D., Bai, H., Wang, Z., and Xing, Y. (2021). Chronic social defeat stress mouse model: Current view on its behavioral deficits and modifications. Behav. Neurosci. 135, 326–335. doi: 10.1037/bne0000418
Whitaker, A. M., Gilpin, N. W., and Edwards, S. (2014). Animal Models of Post-Traumatic Stress Disorder and Recent Neurobiological Insights. Behav. Pharmacol. 25, 398–409. doi: 10.1097/FBP.0000000000000069
Keywords: reinforcement learning, trauma, PTSD, predator odor, chronic stress
Citation: Bielawski T, Drapała J, Krowicki P, Stańczykiewicz B and Frydecka D (2022) Trauma Disrupts Reinforcement Learning in Rats—A Novel Animal Model of Chronic Stress Exposure. Front. Behav. Neurosci. 16:903100. doi: 10.3389/fnbeh.2022.903100
Received: 23 March 2022; Accepted: 25 April 2022;
Published: 17 May 2022.
Edited by:
Adebobola Imeh-Nathaniel, North Greenville University, United StatesReviewed by:
Ayodeji O. Ipinmoroti, Alabama State University, United StatesCopyright © 2022 Bielawski, Drapała, Krowicki, Stańczykiewicz and Frydecka. This is an open-access article distributed under the terms of the Creative Commons Attribution License (CC BY). The use, distribution or reproduction in other forums is permitted, provided the original author(s) and the copyright owner(s) are credited and that the original publication in this journal is cited, in accordance with accepted academic practice. No use, distribution or reproduction is permitted which does not comply with these terms.
*Correspondence: Tomasz Bielawski, dG9tYXN6YmllbGF3c2tpOTBAZ21haWwuY29t
Disclaimer: All claims expressed in this article are solely those of the authors and do not necessarily represent those of their affiliated organizations, or those of the publisher, the editors and the reviewers. Any product that may be evaluated in this article or claim that may be made by its manufacturer is not guaranteed or endorsed by the publisher.
Research integrity at Frontiers
Learn more about the work of our research integrity team to safeguard the quality of each article we publish.