- 1Division of Anatomical Science, Department of Functional Morphology, Nihon University School of Medicine, Itabashi, Japan
- 2Cognitive Neuroscience Section, Primate Research Institute, Kyoto University, Inuyama, Japan
- 3Biomedical Research Institute, National Institute of Advanced Industrial Science and Technology, Ikeda, Japan
Rodents acquire more information from the sense of smell than humans because they have a nearly fourfold greater variety of olfactory receptors. They use olfactory information not only for obtaining food, but also for detecting environmental dangers. Predator-derived odor compounds provoke instinctive fear and stress reactions in animals. Inbred lines of experimental animals react in an innate stereotypical manner to predators even without prior exposure. Predator odors have also been used in models of various neuropsychiatric disorders, including post-traumatic stress disorder following a life-threatening event. Although several brain regions have been reported to be involved in predator odor-induced stress responses, in this mini review, we focus on the functional role of inhibitory neural circuits, especially in the anterior piriform cortex (APC). We also discuss the changes in these neural circuits following innate reactions to odor exposure. Furthermore, based on the three types of modulation of the stress response observed by our group using the synthetic fox odorant 2,5-dihydro-2,4,5-trimethylthiazoline, we describe how the APC interacts with other brain regions to regulate the stress response. Finally, we discuss the potential therapeutic application of odors in the treatment of stress-related disorders. A clearer understanding of the odor–stress response is needed to allow targeted modulation of the monoaminergic system and of the intracerebral inhibitory networks. It would be improved the quality of life of those who have stress-related conditions.
Introduction
Rodents use odors as cues for evaluating their surroundings, for locating food, social interactions and breeding, and the detection of threats in the environment. With respect to threat detection, the primary focus of this review, rapid and accurate detection of threat in its proximity is critical for survival (e.g., cat, fox, and ferret). The odors produced by these predators, known as kairomones, which are the interspecific chemical signals that cause a disadvantage to the source of release, rapidly induce fear and stress responses in rodents (Vernet-Maury et al., 1984; Apfelbach et al., 2005; Takahashi, 2014; Janitzky et al., 2015; Masuo et al., 2021). While the stress responses are critical for survival, dysregulation of these responses can result in psychiatric disorders in humans, such as anxiety and mood disorders, phobias, and post-traumatic stress disorder (PTSD) (Rosen et al., 2008; Staples, 2010; Zoladz et al., 2012; Wilson et al., 2014; Dopfel et al., 2019).
There is an extensive neural network that responds to predator odors. A constituent odorant of fox feces, 2,5-dihydro-2,4,5-trimethylthiazoline (TMT), which mimics the anal gland secretions of the fox, is one of the most effective at inducing innate stress responses in rodents (Vernet-Maury et al., 1984; Day et al., 2004). The detection of TMT appears to be predominantly through the piriform cortex (PC) via the olfactory bulb (OB) which receives input signals from olfactory sensory neurons activated in the olfactory epithelium (OE) (Takahashi, 2014). Although the anterior piriform cortex (APC) has known to involve both detection of TMT and induction of TMT-induced stress responses, the underlying mechanisms including the related neural network and modulatory system have thought to be different (Kobayakawa et al., 2007). One of the immediate early genes, c-fos, is a biomarker of neural activity, and is associated with stress responses. Based on c-fos expression, it has been shown that various brain regions, including the APC, medial part of the bed nucleus of the stria terminalis (mBST), and amygdalopiriform transition area (AmyPir), are involved in the stress response to predator odor (Day et al., 2004; Kobayakawa et al., 2007; Janitzky et al., 2009; Kondoh et al., 2016). In this review, we describe the effects of odor in the modulation of stress responses, focusing on the role of the inhibitory circuits in the APC. We also discuss how a better understanding of the regulation of stress responses is important for treating stress-related illnesses.
Stress and Odor
Responses to Stress and Fear
There are autonomic, endocrine, and behavioral responses to fear and stress. The autonomic nervous system, which provides a rapid and short-term response to stress, raises heart rate and blood pressure, enabling a rapid response to threats (Ulrich-Lai and Herman, 2009). The endocrine response, which involves activation of the hypothalamic–pituitary–adrenal (HPA) axis, is less rapid, but is sustained for a longer period (Ulrich-Lai and Herman, 2009). Activation of the HPA axis stimulates endocrine release, including adrenocorticotropic hormone (ACTH), cortisol, and corticosterone, which can be used as biomarkers of stress. In addition, behavioral responses to stress and fear may include freezing, attack/avoidance and other behaviors, and these too can be used to assess anxiety.
Moreover, monoaminergic neuromodulation system has thought to be involved in the regulation of stress-related responses. Although definitive biomarkers of PTSD remain elusive, dysregulation of various neurotransmitter systems has been implicated in the disorder (Arora et al., 1993; Geracioti, Baker et al., 2001; Krystal and Neumeister, 2009; Southwick and Charney, 2012). Monoamines, including dopamine (DA), norepinephrine (NE), and serotonin (5-HT), are synthesized in specific regions of the brain and regulate neural activities in numerous target regions. Moreover, predator odors can increase the release of monoamines in the mouse brain (Hayley et al., 2001; Smith et al., 2006) and activate the locus coeruleus (LC), from which noradrenergic fibers innervate the cerebral cortex, amygdala, and hippocampus (Day et al., 2004; Curtis et al., 2012; Isosaka et al., 2015; Janitzky et al., 2015). These monoamines play important roles in behavioral responses to innate and learned fears and stresses by modulating motor control, motivation, reward, learning, and associative memory.
Modulation of Stress by Odor
Innate Responses
We previously showed that natural odors can be classified into three types: (1) odors that cause innate fear/stress reactions (innate stressors) such as predator odors; (2) odors that innately reduce stress signaling (innate relaxants) such as rose and hinokitiol odors; and (3) odors that do not affect stress responses (neutral odors) such as caraway odor (Matsukawa et al., 2011; Murakami et al., 2012). Next, we describe the effects and associated systems for each type of odor.
Innate Stressors
In a number of studies, predator odors, such as those of cats and ferrets, have also been used to activate stress pathways in rats and mice (Takahashi, 2014). Despite the absence of a real threat, TMT activated stress responses, which are to increase plasma ACTH levels and to heighten the activities in the predator odor-related neural pathways (Day et al., 2004; Matsukawa et al., 2011). In addition to TMT, predator urines have been shown to induce neural activation in the main olfactory system (MOS) via the main OB (MOB) (Takahashi, 2014). Furthermore, stress responses are strongly linked with learning and memory processes, and thus may adapt to predator odors over time (Staples, 2010). The stimulus intensity of predator odors (concentration and repeated administration) is known to impact habituation and extinction (Takahashi et al., 2005). Moreover, neonatal exposure to TMT odor in mice can reduce avoidance, immobility and freezing behaviors in adulthood (Hacquemand et al., 2010). In addition to odors from external threats, a number of studies have shown that stressed animals can release odors called warning pheromones that cause anxiety-related behaviors in other conspecifics in their proximity (Masuo et al., 2021).
Innate Relaxants and Neutral Odors
Conversely, several odors have been shown to improve mood or signs of stress or anxiety (Haze et al., 2002; Lehrner et al., 2005; Ito et al., 2009; Masuo et al., 2021). In addition, some odors can decrease responses to stressor odors. For example, rose odor reduces plasma ACTH levels during TMT-induced stress responses by decreasing c-fos upregulation-associated neural activation in the ventrorostral part of the APC (APCvr) and the mBST in mice (Table 1; Matsukawa et al., 2011). Notably, robust odor-induced feedforward inhibitory signals are sent from the APCvr to the entire APC (Ishikawa et al., 2007). Hinokitiol odor, a woody scent, but not S(+)-carvone (caraway odor), prevents the TMT-induced upregulation of plasma ACTH by increasing c-fos-associated neural activation in the lateral part of the BST (lBST) when presented in combination with TMT odor (Table 1; Murakami et al., 2012). This demonstrates that animals have innate responses to specific odors, and that some odors, such as rose and hinokitiol odors, reduce stress responses without prior learning, while others, like caraway odor, are innately neutral and do not significantly impact the stress response.
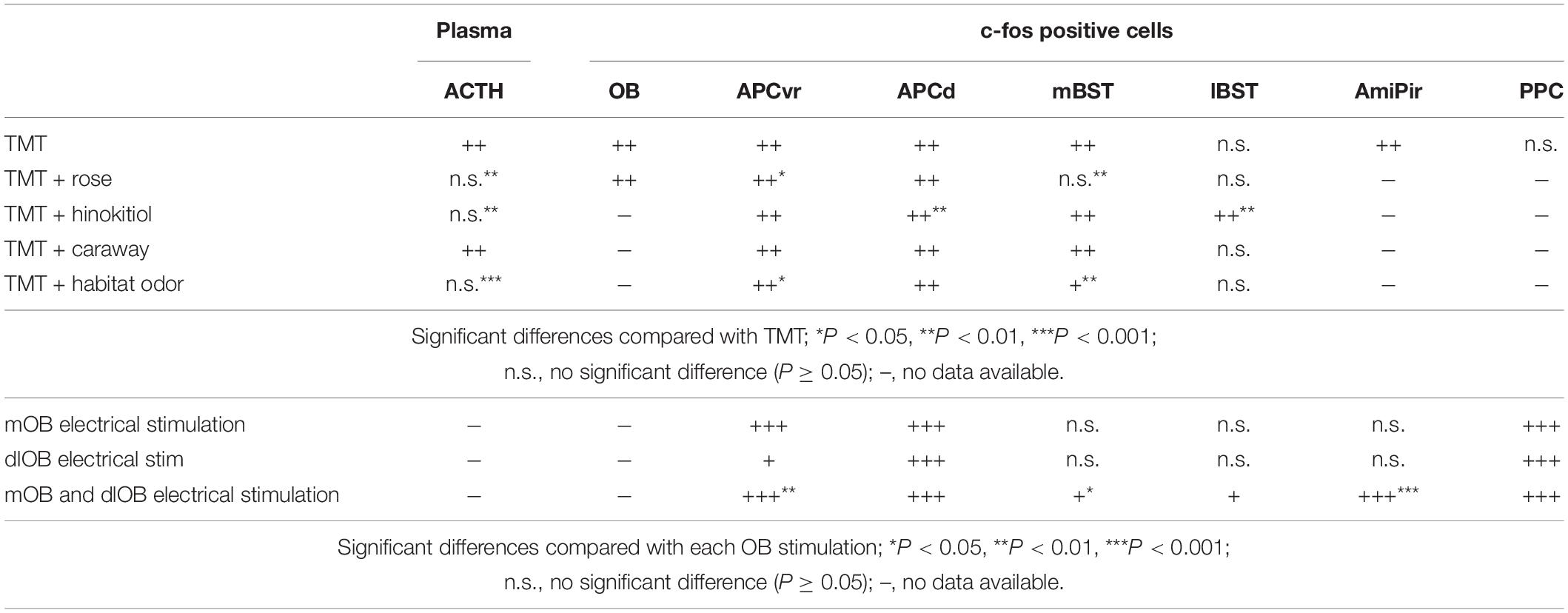
Table 1. Changes in the number of c-fos-positive cells in each brain region following each odor presentation (upper part) and following each electrical stimulation (lower part).
Apparently, there are multiple mechanisms that induce the innate inhibitory response. When combined with TMT odor, hinokitiol odor may activate the olfactory system broadly and robustly, thereby making the specific effect of TMT impossible to distinguish at the neural level (Table 1; Murakami et al., 2012). These findings suggest that there may be at least two distinct mechanisms that reduce TMT odor-induced stress responses: (1) direct and selective mechanisms that inhibit TMT odor-induced activation of stress-related networks, as seen with rose odor; and (2) mechanisms that obscure the selective effect of TMT odor, as seen with hinokitiol odor.
Conditioned Responses
Experiences can change the innate reactions. We previously demonstrated that even an artificial odor, classified as a neutral odor, could alleviate the predator odor-induced stress responses in adulthood in mice when they experience and are habituated to the artificial odor early in life (Matsukawa et al., 2016). This suggests that animals can be conditioned to experience stress-relieving effects from odors later in life when experienced in the absence of a real threat. This also means that experience can modify innate reactions. When combined with a habitat odor, TMT odor-induced neural activation is reduced in the APCvr and the mBST, similar to the effect of rose odor (Table 1; Matsukawa et al., 2016). These stress-reducing effects suggest that a selective inhibitory system is present in brain regions that participate in predator odor-induced stress responses.
The Mechanisms of Odor-Induced Stress Reactions: Olfaction and Stress Responses
Neural Substrate of Olfactory Information Processing
In mammals, the olfactory system is composed of two distinct pathways—the MOS and the accessory olfactory system (AOS). The MOS is considered to be primarily involved in the detection of environmental cues such as foods and predators (Takahashi, 2014; Masuo et al., 2021), whereas the AOS is more involved in the detection of intraspecific chemical stimuli such as pheromones (Wyatt, 2017; Mohrhardt et al., 2018). The MOS comprises the main OE, which expresses odorant and trace amine-associated receptors. These detect environmental cues and convey the information to the olfactory cortex [APC, posterior piriform cortex (PPC), AmyPir, entorhinal cortex (EC)] and other areas, including the amygdala, to modify physiological and behavioral responses (Figure 1A; Takahashi, 2014; Isosaka et al., 2015; Kondoh et al., 2016). Both the amygdala and the EC have bidirectional innervation to the hippocampus, and bidirectional connections exist between the amygdala and extended amygdala, including the BST.
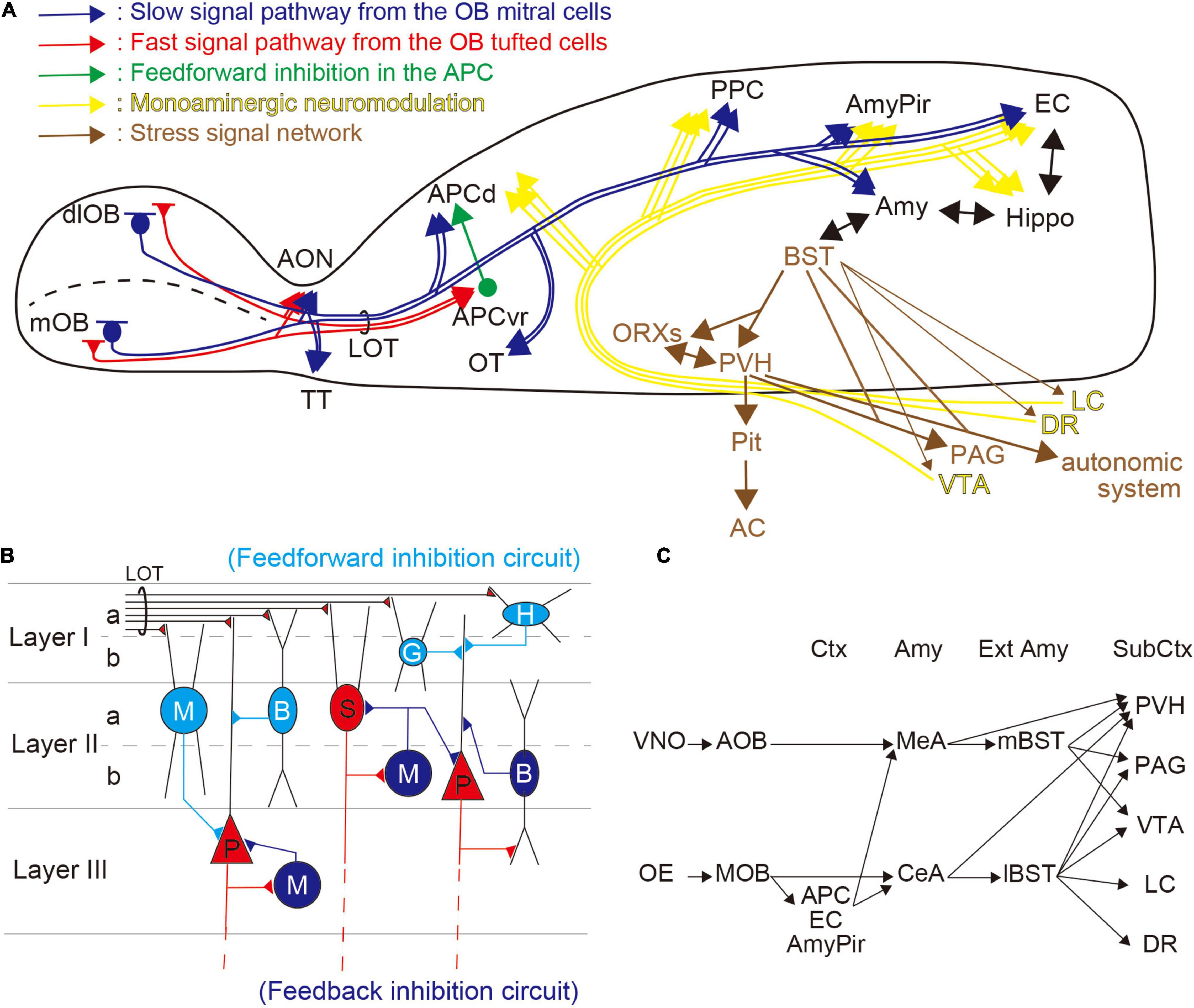
Figure 1. Olfactory information processing and stress-related networks. (A) Overview of the regulation of the odor–stress response. Distinct olfactory bulb (OB) projection neurons are shown in red [tufted cells (TCs)] and blue [mitral cells (MCs)] lines, and show fast and slow pathways, respectively. TCs project to the anterior olfactory nucleus (AON) and the ventrorostral part of the anterior piriform cortex (APCvr). MCs innervate the AON, the olfactory tubercle (OT), the APCd, the posterior piriform cortex (PPC), the amygdalopiriform transition area (AmyPir), the entorhinal cortex (EC), and the amygdala (Amy). Feedforward inhibitory circuit from the APCvr to the APCd is shown as a green line. Stress-related networks are illustrated in brown; from the bed nucleus of the stria terminalis (BST), the information passes to the hypothalamus (PVH and ORXs) where it can activate the hypothalamic–pituitary–adrenal (HPA) axis. Many brainstem regions, including the periaqueductal gray (PAG) and autonomic system, receive information from the BST and hypothalamus. The yellow lines show monoaminergic modulatory networks. DA projections from the ventral tegmental area (VTA), NE projections from the locus coeruleus (LC), and serotonin (5-HT) projections from the dorsal raphe (DR) are distributed widely in the brain. (B) Inhibitory pathways in the piriform cortex (PC). In the PC, olfactory information from the OB is obtained via the lateral olfactory tract (LOT). Horizontal cells (H), neurogliaform cells (G), bitufted cells (B), and multipolar cells (M), which are inhibitory interneurons in the superficial layer (illustrated in light blue), have feedforward connections to projection neurons [semilunar cells (S) and pyramidal cells (P); illustrated in red] in the PC. Inhibitory cells in mid to deep layers (B and M; illustrated in blue) feedback onto S and P cells. (C) Putative networks for odor-induced stress responses. The accessory olfactory system (AOS) from the vomeronasal organ (VNO) provides inputs to the extended amygdala (Ext Amy) via the medial amygdala (MeA). In contrast, the main olfactory system (MOS) from the olfactory epithelium (OE) provides inputs to the Ext Amy directly from the central amygdala (CeA) and indirectly via olfactory cortices, including the APC, EC, and AmyPir. Both the Amy and Ext Amy have projections to subcortical regions (Sub Ctx) including the PVH, PAG, VTA, LC, and DR.
Relationship Between Olfaction and Stress Responses
The Piriform Cortex
The PC, which is the largest area in the primary olfactory cortex, consists of three layers (Layers I, II, and III) and is divided into two regions, the APC and the PPC. These two regions have different roles in olfactory information processing. The APC encodes odorant information, perception, and odor-associated values, while the PPC encodes associated information (e.g., odor similarity, quality) (Litaudon et al., 2003; Kadohisa and Wilson, 2006; Calu et al., 2007; Roesch et al., 2007). A recent study showed that the PPC has a key role in spatial representation, including the formation of odor–place associations and guided olfactory-based spatial navigation (Poo et al., 2022). The APC and the PPC are also neuroanatomically different—the APC receives greater input from olfactory regions than the PPC, while the latter receives comparatively more projections from the hippocampus and cerebral nuclei (Wang et al., 2020). In addition, the APC can be subdivided into two distinct parts, the APCvr and the dorsal part of the APC (APCd), by morphological analysis (Ekstrand et al., 2001). In the MOB, there are two distinct projection neurons—mitral cells (MCs) and tufted cells (TCs). The axonal projections of MCs and TCs differ, with MCs sparsely projecting to the APCd, and the TCs robustly projecting to the APCvr (Figure 1A; Igarashi et al., 2012).
Despite its relatively simple trilaminar cortical structure, the PC has many inhibitory interneurons for both feedforward and feedback connections (Figure 1B; Gavrilovici et al., 2010; Suzuki and Bekkers, 2012; Bekkers and Suzuki, 2013; Large et al., 2016). The feedforward inhibition usually occurs when excitatory inputs activate inhibitory cells, which then inhibit postsynaptic excitatory neurons (Kee et al., 2015). In contrast, feedback inhibition generally occurs when excitatory neurons activate inhibitory cells that then recurrently inhibit them (Kee et al., 2015). In the PC, the interneurons responsible for feedforward inhibition are localized superficially (Layers I–II), and those responsible for feedback connections are distributed in deep layers (Layers II–III) (Figure 1B).
The Bed Nucleus of the Stria Terminalis
The BST, known as the extended amygdala, has an important role in stress and fear responses. This forebrain region, made up of more than 10 subregions, comprises distinct neuroanatomical and neurochemical populations. AOS information from the vomeronasal organ (VNO) provides input to the mBST via the medial amygdala (MeA), and MOS information from the OE provides input to both the mBST, via the MeA, and the lBST, via the central amygdala (CeA). In addition, the BST outputs project to the hypothalamus [e.g., the paraventricular hypothalamic nucleus (PVH), orexin neurons (ORXs)] and brainstem regions [e.g., the periaqueductal gray (PAG), and the origins of monoaminergic innervation, including the ventral tegmental area (VTA), dorsal raphe nucleus (DR), and LC] (Figure 1C; Takahashi, 2014; Fox et al., 2015; Lebow and Chen, 2016). The HPA axis may be stimulated, via the mBST, following presentation of the predator odor (Kobayakawa et al., 2007). In addition, the BST appears to play a role in conditioned fear responses with a temporal aspect (Goode and Maren, 2017). Moreover, recent studies suggest that the BST modulates the response to weak, uncertain threats (Glover et al., 2020; Bruzsik et al., 2021).
Inhibitory Circuits and the Piriform Cortex
2,5-Dihydro-2,4,5-trimethylthiazoline odor information is conveyed from the MOB to the APC by several neural networks. The OE innervates spatially distinct glomeruli forming mirror-image maps in the MOB (Nagao et al., 2000; Uchida et al., 2000; Inaki et al., 2002; Mori et al., 2006; Matsumoto et al., 2010; Murthy, 2011). We demonstrated that stress-related neural activities were induced only following simultaneous stimulation in the mirror-image-organized medial and dorso-lateral walls of the MOB (mOB and dlOB, respectively), but not following stimulation of the mOB or dlOB individually (Table 1; Matsukawa et al., 2020). The TCs, whose firing rates during burst discharges are about 100 Hz, provide rapid input to the APCvr (fast pathway), whereas the MCs, whose firing rates during burst discharges are about 40 Hz, provide slow input to the APCd (slow pathway) (Figure 1A; Nagayama et al., 2004; Igarashi et al., 2012). We previously suggested that the association of beta-band (15–40 Hz) oscillations from MCs in both mOB and dlOB should be needed for stress-induced activation of the APCd (Matsukawa et al., 2020). In addition, through the inhibitory circuit from the APCvr, the fast pathway component can regulate the association of oscillatory activities in the APCd (Ishikawa et al., 2007; Sato et al., 2008). It has been shown that the integration and processing of the mOB and dlOB inputs in the APC are important for the expression of what is termed the odor–stress response (Matsukawa et al., 2020). Furthermore, we showed that the selective inhibitory system involved in reducing the predator odor-induced stress response is likely to be in the APC rather than in the OB (Matsukawa et al., 2011, 2016, 2020).
The Therapeutic Importance of Odor Modulation of Stress Responses
Monoamine neurotransmitters have been the primary target of therapeutic strategies for the treatment of neuropsychiatric diseases such as PTSD (Krystal and Neumeister, 2009). In animal models of PTSD, lower 5-HT and elevated NE levels have been observed in the prefrontal cortex and hippocampus (Wilson et al., 2014). In addition, we demonstrated changes in hippocampal NE concentrations following TMT presentation (Matsukawa et al., 2016). These studies suggest that increased hippocampal NE concentrations are important in the stress response following exposure to a life-threatening event/cue. Higher concentrations of NE in the hippocampus have also been shown to affect the regulation of stress responses and learning under stress (de Kloet et al., 2005; Joëls et al., 2006; Ulrich-Lai and Herman, 2009). Moreover, hippocampal NE concentrations, under stress conditions, have also been shown to affect memory consolidation via the amygdala (Roozendaal et al., 2009; Galliot et al., 2010; Hubert and Muly, 2014). Recent studies have shown that medial prefrontal areas, including the prelimbic, infralimbic, and anterior cingulate cortices, can regulate the response to predatory threats (Glover et al., 2020; de Lima et al., 2022). Dopaminergic innervation from the VTA has also been reported in these brain regions, where the number of synapses is regulated by DA in Layer I via D1 receptor as well as by NE in Layer II/III (Imai et al., 2004). Together, these observations suggest that monoamines, which can modify the neural activities in broad regions of the brain, participate in odor-induced stress responses.
In addition to monoaminergic modulation, there are many intrinsic inhibitory circuits that use a variety of inhibitory neurotransmitters in the APC (Gavrilovici et al., 2010; Suzuki and Bekkers, 2012; Bekkers and Suzuki, 2013; Large et al., 2016), as well as inhibitory connections among subregions in the APC (Ishikawa et al., 2007; Sato et al., 2008). However, many issues remain to be addressed, such as whether the selective inhibition system in the APC is a specific response to TMT odor or common to other predator odors. A better understanding of the inhibitory mechanisms that selectively suppress the odor-induced stress response in the APC may be important for the therapeutic modulation of odor–stress relationships.
Conclusion and Future Studies
Odor-induced stress responses, which involve autonomic, endocrine, and behavioral responses, can be modulated by innate and conditioned odors. This modulation of the response to stress-inducing stimuli such as predator odors likely involves inhibitory pathways in the APC. These pathways have potential as therapeutic targets for conditions such as chronic stress, anxiety, phobias, and PTSD. However, many questions remain unresolved, including which inhibitory neural circuits and inhibitory neurotransmitters are involved in a condition-dependent manner. Moreover, a recent study suggests that transient receptor potential ankyrin 1 (TRPA1) participates in predator odor-evoked innate fear responses (Wang et al., 2018). TRPA1, a chemoreceptor for noxious stimuli, such as formaldehyde (McNamara et al., 2007), has an important role in nociception (Story et al., 2003). In addition, a recent study suggests that innate fear stimuli orchestrate hypothermia and anti-hypoxia via TRPA1 activation (Matsuo et al., 2021). Elucidation of stress-related neural circuits, their selective inhibitory systems, and related chemoreceptors is necessary for the future development of effective therapeutic interventions.
Author Contributions
MM planned and conducted most of these studies with co-authors. MY planned and conducted collection of brain samples from experimental animals and investigation for c-fos study. NK planned and conducted experiments for effects of electrical stimulations in mirror-arranged glomeruli in the OB. SA designed and performed the collection of plasma samples and investigation of odor-induced stress responses. TS designed and conducted studies on the effects of different odors on TMT-induced stress-related responses in mice. MM and TS wrote the manuscript. All authors analyzed the data and discussed the results.
Funding
This research was supported, in part, by a Grant-in-Aid for Scientific Research from the Japan Society for the Promotion of Science #20500195 (to MM), #23500264 (to MM), #15K00381 (to MM), #18K11507 (to MM), #21K12092 (to MM), and by a Nihon University Research Grant #kyo09-010 (to MM and NK).
Conflict of Interest
The authors declare that the research was conducted in the absence of any commercial or financial relationships that could be construed as a potential conflict of interest.
Publisher’s Note
All claims expressed in this article are solely those of the authors and do not necessarily represent those of their affiliated organizations, or those of the publisher, the editors and the reviewers. Any product that may be evaluated in this article, or claim that may be made by its manufacturer, is not guaranteed or endorsed by the publisher.
Acknowledgments
We are grateful to the staff in the laboratory animals section (for help with animal research) at the Medical Research Support Center, Nihon University School of Medicine. We are also grateful to Miyuki Yuda for contributions to immunohistochemical studies. We thank Barry Patel, from Edanz (https://jp.edanz.com/ac), for editing a draft of this manuscript.
References
Apfelbach, R., Blanchard, C. D., Blanchard, R. J., Hayes, R. A., and McGregor, I. S. (2005). The effects of predator odors in mammalian prey species: a review of field and laboratory studies. Neurosci. Biobehav. Rev. 29, 1123–1144. doi: 10.1016/j.neubiorev.2005.05.005
Arora, R. C., Fichtner, C. G., O’Connor, F., and Crayton, J. W. (1993). Paroxetine binding in the blood platelets of post-traumatic stress disorder patients. Life Sci. 53, 919–928. doi: 10.1016/0024-3205(93)90444-8
Bekkers, J. M., and Suzuki, N. (2013). Neurons and circuits for odor processing in the piriform cortex. Trends Neurosci. 36, 429–438. doi: 10.1016/j.tins.2013.04.005
Bruzsik, B., Biro, L., Sarosdi, K. R., Zelena, D., Sipos, E., Szebik, H., et al. (2021). Neurochemically distinct populations of the bed nucleus of stria terminalis modulate innate fear response to weak threat evoked by predator odor stimuli. Neurobiol. Stress 15:100415. doi: 10.1016/j.ynstr.2021.100415
Calu, D. J., Roesch, M. R., Stalnaker, T. A., and Schoenbaum, G. (2007). Associative encoding in posterior piriform cortex during odor discrimination and reversal learning. Cereb. Cortex 17, 1342–1349. doi: 10.1093/cercor/bhl045
Curtis, A. L., Leiser, S. C., Snyder, K., and Valentino, R. J. (2012). Predator stress engages corticotropin-releasing factor and opioid systems to alter the operating mode of locus coeruleus norepinephrine neurons. Neuropharmacology 62, 1737–1745. doi: 10.1016/j.neuropharm.2011.11.020
Day, H. E. W., Masini, C. V., and Campeau, S. (2004). The pattern of brain c-fos mRNA induced by a component of fox odor, 2,5-dihydro-2,4,5-Trimethylthiazoline (TMT), in rats, suggests both systemic and processive stress characteristics. Brain Res. 1025, 139–151. doi: 10.1016/j.brainres.2004.07.079
de Kloet, E. R., Joëls, M., and Holsboer, F. (2005). Stress and the brain: from adaptation to disease. Nature Rev. Neurosci. 6, 463–475. doi: 10.1038/nrn1683
de Lima, M. A. X., Baldo, M. V. C., Oliveira, F. A., and Canteras, N. S. (2022). The anterior cingulate cortex and its role in controlling contextual fear memory to predatory threats. eLife 11:e67007. doi: 10.7554/eLife.67007
Dopfel, D., Perez, P. D., Verbitsky, A., Bravo-Rivera, H., Ma, Y., Quirk, G. J., et al. (2019). Individual variability in behavior and functional networks predicts vulnerability using an animal model of PTSD. Nat. Commun. 10:2372. doi: 10.1038/s41467-019-09926-z
Ekstrand, J. J., Domroese, M. E., Johnson, D. M., Feig, S. L., Knodel, S. M., Behan, M., et al. (2001). A new subdivision of anterior piriform cortex and associated deep nucleus with novel features of interest for olfaction and epilepsy. J Comp. Neurol. 434, 289–307. doi: 10.1002/cne.1178
Fox, A. S., Oler, J. A., Tromp do, P. M., Fudge, J. L., and Kalin, N. H. (2015). Extending the amygdala in theories of threat processing. Trends Neurosci. 38, 319–329. doi: 10.1016/j.tins.2015.03.002
Galliot, E., Levaillant, M., Beard, E., Millot, J.-L., and Pourié, G. (2010). Enhancement of spatial learning by predator odor in mice: involvement of amygdala and hippocampus. Neurobiol. Learn. Mem. 93, 196–202. doi: 10.1016/j.nlm.2009.09.011
Gavrilovici, C., D’Alfonso, S., and Poulter, M. O. (2010). Diverse interneuron populations have highly specific interconnectivity in the rat piriform cortex. J. Comp. Neurol. 518, 1570–1588. doi: 10.1002/cne.22291
Geracioti, T. D., Baker, D. G., Ekhator, N. N., West, S. A., Hill, K. K., Bruce, A. B., et al. (2001). CSF norepinephrine concentrations in posttraumatic stress disorder. Am. J. Psychiatr. 158, 1227–1230. doi: 10.1176/appi.ajp.158.8.1227
Glover, L. R., McFadden, K. M., Bjorni, M., Smith, S. R., Rovero, N. G., Oreizi-Esfahani, S., et al. (2020). A prefrontal-bed nucleus of the stria terminalis circuit limits fear to uncertain threat. eLife. 9:e60812. doi: 10.7554/eLife.60812
Goode, T. D., and Maren, S. (2017). Role of the bed nucleus of the stria terminalis in aversive learning and memory. Learn. Mem. 24, 480–491. doi: 10.1101/lm.044206.116
Hacquemand, R., Pourie, G., Jacquot, L., and Brand, G. (2010). Postnatal exposure to synthetic predator odor (TMT) induces quantitative modification in fear-related behaviors during adulthood without change in corticosterone levels. Behav. Brain Res. 215, 58–62. doi: 10.1016/j.bbr.2010.06.024
Hayley, S., Borowski, T., Merali, Z., and Anisman, H. (2001). Central monoamine activity in genetically distinct strains of mice following a psychogenic stressor: effects of predator exposure. Brain Res. 892, 293–300. doi: 10.1016/S0006-8993(00)03262-5
Haze, S., Sakai, K., and Gozu, Y. (2002). Effects of fragrance inhalation on sympathetic activity in normal adults. Jpn. J. Pharmacol. 90, 247–253. doi: 10.1254/jjp.90.247
Hubert, G. W., and Muly, E. C. (2014). Distribution of AMPA receptor subunit glur1 in the bed nucleus of the stria terminalis and effect of stress. Synapse 68, 194–201. doi: 10.1002/syn.21729
Igarashi, K. M., Ieki, N., An, M., Yamaguchi, Y., Nagayama, S., Kobayakawa, K., et al. (2012). Parallel mitral and tufted cell pathways route distinct odor information to different targets in the olfactory cortex. J. Neurosci. 32, 7970–7985. doi: 10.1523/jneurosci.0154-12.2012
Imai, H., Matsukawa, M., and Okado, N. (2004). Lamina-selective changes in the density of synapses following perturbation of monoamines and acetylcholine in the rat medial prefrontal cortex. Brain Res. 1012, 138–145. doi: 10.1016/j.brainres.2004.03.039
Inaki, K., Takahashi, Y. K., Nagayama, S., and Mori, K. (2002). Molecular-feature domains with posterodorsal-anteroventral polarity in the symmetrical sensory maps of the mouse olfactory bulb: mapping of odorant-induced Zif268 expression. Eur. J. Neurosci. 15, 1563–1574. doi: 10.1046/j.1460-9568.2002.01991.x
Ishikawa, T., Sato, T., Shimizu, A., Tsutsui, K., de Curtis, M., and Iijima, T. (2007). Odor-driven activity in the olfactory cortex of an in vitro isolated guinea pig whole brain with olfactory epithelium. J. Neurophysiol. 97, 670–679. doi: 10.1152/jn.01366.2005
Isosaka, T., Matsuo, T., Yamaguchi, T., Funabiki, K., Nakanishi, S., Kobayakawa, R., et al. (2015). Htr2a-expressing cells in the central amygdala control the hierarchy between innate and learned fear. Cell 163, 1153–1164. doi: 10.1016/j.cell.2015.10.047
Ito, A., Miyoshi, M., Ueki, S., Fukada, M., Komaki, R., and Watanabe, T. (2009). “Green odor” inhalation by rats down-regulates stress-induced increases in Fos expression in stress-related forebrain regions. Neurosci. Res. 65, 166–174. doi: 10.1016/j.neures.2009.06.012
Janitzky, K., D’Hanis, W., Kröber, A., and Schwegler, H. (2015). TMT predator odor activated neural circuit in C57BL/6J mice indicates TMT-stress as a suitable model for uncontrollable intense stress. Brain Res. 1599, 1–8. doi: 10.1016/j.brainres.2014.12.030
Janitzky, K., Stork, O., Lux, A., Yanagawa, Y., Schwegler, H., and Linke, R. (2009). Behavioral effects and pattern of brain c-fos mRNA induced by 2,5-dihydro-2,4,5-trimethylthiazoline, a component of fox feces odor in GAD67-GFP knock-in C57BL/6 mice. Behav. Brain Res 202, 218–224. doi: 10.1016/j.bbr.2009.03.038
Joëls, M., Pu, Z., Wiegert, O., Oitzl, M. S., and Krugers, H. J. (2006). Learning under stress: how does it work? Trends Cogn. Sci. 10, 152–158. doi: 10.1016/j.tics.2006.02.002
Kadohisa, M., and Wilson, D. A. (2006). Separate encoding of identity and similarity of complex familiar odors in piriform cortex. Proc. Natl. Acad. Sci. U S A 103, 15206–15211. doi: 10.1073/pnas.0604313103
Kee, T., Sanda, P., Gupta, N., Stopfer, M., and Bazhenov, M. (2015). Feed-forward versus feedback inhibition in a basic olfactory circuit. PLoS Comput. Biol. 11:e1004531. doi: 10.1371/journal.pcbi.1004531
Kobayakawa, K., Kobayakawa, R., Matsumoto, H., Oka, Y., Imai, T., Ikawa, M., et al. (2007). Innate versus learned odour processing in the mouse olfactory bulb. Nature 450, 503–508. doi: 10.1038/nature06281
Kondoh, K., Lu, Z., Ye, X., Olson, D. P., Lowell, B. B., and Buck, L. B. (2016). A specific area of olfactory cortex involved in stress hormone responses to predator odours. Nature 532, 103–106. doi: 10.1038/nature17156
Krystal, J. H., and Neumeister, A. (2009). Noradrenergic and serotonergic mechanisms in the neurobiology of posttraumatic stress disorder and resilience. Brain Res. 1293, 13–23. doi: 10.1016/j.brainres.2009.03.044
Large, A. M., Vogler, N. W., Mielo, S., and Oswald, A.-M. M. (2016). Balanced feedforward inhibition and dominant recurrent inhibition in olfactory cortex. Proc. Natl. Acad. Sci. U S A 113, 2276–2281. doi: 10.1073/pnas.1519295113
Lebow, M. A., and Chen, A. (2016). Overshadowed by the amygdala: the bed nucleus of the stria terminalis emerges as key to psychiatric disorders. Mol. Psychiat. 21, 450–463. doi: 10.1038/mp.2016.1
Lehrner, J., Marwinski, G., Lehr, S., Johren, P., and Deecke, L. (2005). Ambient odors of orange and lavender reduce anxiety and improve mood in a dental office. Physiol. Behav. 86, 92–95. doi: 10.1016/j.physbeh.2005.06.031
Litaudon, P., Amat, C., Bertrand, B., Vigouroux, M., and Buonviso, N. (2003). Piriform cortex functional heterogeneity revealed by cellular responses to odours. Eur. J. Neurosci. 17, 2457–2461. doi: 10.1046/j.1460-9568.2003.02654.x
Masuo, Y., Satou, T., Takemoto, H., and Koike, K. (2021). Smell and stress response in the brain: review of the connection between chemistry and neuropharmacology. Molecules 26:2571. doi: 10.3390/molecules26092571
Matsukawa, M., Imada, M., Aizawa, S., and Sato, T. (2016). Habitat odor can alleviate innate stress responses in mice. Brain Res. 1631, 46–52. doi: 10.1016/j.brainres.2015.11.020
Matsukawa, M., Imada, M., Murakami, T., Aizawa, S., and Sato, T. (2011). Rose odor can innately counteract predator odor. Brain Res. 1381, 117–123. doi: 10.1016/j.brainres.2011.01.053
Matsukawa, M., Katsuyama, N., Imada, M., Aizawa, S., and Sato, T. (2020). Simultaneous activities in both mirror-image glomerular maps in the olfactory bulb may have an important role in stress-related neuronal responses in mice. Brain Res. 1732:146676. doi: 10.1016/j.brainres.2020.146676
Matsumoto, H., Kobayakawa, K., Kobayakawa, R., Tashiro, T., Mori, K., Sakano, H., et al. (2010). Spatial arrangement of glomerular molecular-feature clusters in the odorant-receptor class domains of the mouse olfactory bulb. J. Neurophysiol. 103, 3490–3500. doi: 10.1152/jn.00035.2010
Matsuo, T., Isosaka, T., Hayashi, Y., Tang, L., Doi, A., Yasuda, A., et al. (2021). Thiazoline-related innate fear stimuli orchestrate hypothermia and anti-hypoxia via sensory TRPA1 activation. Nat. Commun 12:2074. doi: 10.1038/s41467-021-22205-0
McNamara, C. R., Mandel-Brehm, J., Bautista, D. M., Siemens, J., Deranian, K. L., Zhao, M., et al. (2007). TRPA1 mediates formalin-induced pain. Proc. Natl. Acad. Sci. USA 104, 13525–13530. doi: 10.1073/pnas.0705924104
Mohrhardt, J., Nagel, M., Fleck, D., Ben-Shaul, Y., and Spehr, M. (2018). Signal detection and coding in the accessory olfactory system. Chem. Senses 43, 667–695. doi: 10.1093/chemse/bjy061
Mori, K., Takahashi, Y. K., Igarashi, K. M., and Yamaguchi, M. (2006). Maps of odorant molecular features in the mammalian olfactory bulb. Physiol. Rev. 86, 409–433. doi: 10.1152/physrev.00021.2005
Murakami, T., Matsukawa, M., Katsuyama, N., Imada, M., Aizawa, S., and Sato, T. (2012). Stress-related activities induced by predator odor may become indistinguishable by hinokitiol odor. NeuroReport 23, 1071–1076. doi: 10.1097/WNR.0b013e32835b373b
Murthy, V. N. (2011). Olfactory maps in the brain. Annu. Rev. Neurosci. 34, 233–258. doi: 10.1146/annurev-neuro-061010-113738
Nagao, H., Yoshihara, Y., Mitsui, S., Fujisawa, H., and Mori, K. (2000). Two mirror-image sensory maps with domain organization in the mouse main olfactory bulb. Neurorep 11, 3023–3027. doi: 10.1097/00001756-200009110-00039
Nagayama, S., Takahashi, Y. K., Yoshihara, Y., and Mori, K. (2004). Mitral and tufted cells differ in the decoding manner of odor maps in the rat olfactory bulb. J. Neurophysiol. 91, 2532–3540. doi: 10.1152/jn.01266.2003
Poo, C., Agarwal, G., Bonacchi, N., and Mainen, Z. F. (2022). Spatial maps in piriform cortex during olfactory navigation. Nature 601, 595–599. doi: 10.1038/s41586-021-04242-3
Roesch, M. R., Stalnaker, T. A., and Schoenbaum, G. (2007). Associative encoding in anterior piriform cortex versus orbitofrontal cortex during odor discrimination and reversal learning. Cereb. Cortex 17, 643–652. doi: 10.1093/cercor/bhk009
Roozendaal, B., McEwen, B. S., and Chattarji, S. (2009). Stress, memory and the amygdala. Nature Rev. Neurosci. 10, 423–433. doi: 10.1038/nrn2651
Rosen, J. B., Pagani, J. H., Rolla, K. L. G., and Davis, C. (2008). Analysis of behavioral constraints and the neuroanatomy of fear to the predator odor trimethylthiazoline: a model for animal phobias. Neurosci. Biobehav. Rev. 32, 1267–1276. doi: 10.1016/j.neubiorev.2008.05.006
Sato, T., Hirono, J., Hamana, H., Ishikawa, T., Shimizu, A., Takashima, I., et al. (2008). Architecture of odor information processing in the olfactory system. Anat. Sci. Int. 83, 195–206. doi: 10.1111/j.1447-073x.2007.00215.x
Smith, D. G., Davis, R. J., Gehlert, D. R., and Nomikos, G. G. (2006). Exposure to predator odor stress increases efflux of frontal cortex acetylcholine and monoamines in mice: comparisons with immobilization stress and reversal by chlordiazepoxide. Brain Res. 1114, 24–30. doi: 10.1016/j.brainres.2006.07.058
Southwick, S. M., and Charney, D. S. (2012). The science of resilience: implications for the prevention and treatment of depression. Science 338, 79–82. doi: 10.1126/science.1222942
Staples, L. G. (2010). Predator odor avoidance as a rodent model of anxiety: learning-mediated consequences beyond the initial exposure. Neurobiol. Learn. Memory 94, 435–445. doi: 10.1016/j.nlm.2010.09.009
Story, G. M., Peier, A. M., Reeve, A. J., Eid, S. R., Mosbacher, J., Hricik, T. R., et al. (2003). ANKTM1, a TRP-like channel expressed in nociceptive neurons, is activated by cold temperatures. Cell 112, 819–829. doi: 10.1016/S0092-8674(03)00158-2
Suzuki, N., and Bekkers, J. M. (2012). Microcircuits mediating feedforward and feedback synaptic inhibition in the piriform cortex. J. Neurosci. 32, 919–931. doi: 10.1523/JNEUROSCI.4112-11.2012
Takahashi, L. K. (2014). Olfactory systems and neural circuits that modulate predator odor fear. Front. Behav. Neurosci. 8:1–13. doi: 10.3389/fnbeh.2014.00072
Takahashi, L. K., Nakashima, B. R., Hong, H., and Watanabe, K. (2005). The smell of danger: A behavioral and neural analysis of predator odor-induced fear. Neurosci Biobehav Rev 29, 1157–1167. doi: 10.1016/j.neubiorev.2005.04.008
Uchida, N., Takahashi, Y. K., Tanifuji, M., and Mori, K. (2000). Odor maps in the mammalian olfactory bulb: domain organization and odorant structural features. Nat. Neurosci. 3, 1035–1043. doi: 10.1038/79857
Ulrich-Lai, Y. M., and Herman, J. P. (2009). Neural regulation of endocrine and autonomic stress responses. Nature Rev. Neurosci. 10, 397–409. doi: 10.1038/nrn2647
Vernet-Maury, E., Polak, E. H., and Demael, A. (1984). Structure-activity relationship of stress-inducing odorants in the rat. J. Chem. Ecol. 10, 1007–1018. doi: 10.1007/BF00987509
Wang, L., Zhang, Z., Chen, J., Manyande, A., Haddad, R., Liu, Q., et al. (2020). Cell-type-specific whole-brain direct inputs to the anterior and posterior piriform cortex. Front. Neural Circuits 14:4. doi: 10.3389/fncir.2020.00004
Wang, Y., Cao, L., Lee, C.-Y., Matsuo, T., Wu, K., Asher, G., et al. (2018). Large-scale forward genetics screening identifies Trpa1 as a chemosensor for predator odor-evoked innate fear behaviors. Nature Commun. 9:2041. doi: 10.1038/s41467-018-04324-3
Wilson, C. B., Ebenezer, P. J., McLaughlin, L. D., and Francis, J. (2014). Predator exposure/psychosocial stress animal model of post-traumatic stress disorder modulates neurotransmitters in the rat hippocampus and prefrontal cortex. PLoS One 9:e89104. doi: 10.1371/journal.pone.0089104
Keywords: predator odor, stress, innate response, relaxant, feedforward inhibition, anterior piriform cortex, monoaminergic neuromodulation system
Citation: Matsukawa M, Yoshikawa M, Katsuyama N, Aizawa S and Sato T (2022) The Anterior Piriform Cortex and Predator Odor Responses: Modulation by Inhibitory Circuits. Front. Behav. Neurosci. 16:896525. doi: 10.3389/fnbeh.2022.896525
Received: 15 March 2022; Accepted: 07 April 2022;
Published: 28 April 2022.
Edited by:
Regina Marie Sullivan, NYU Grossman School of Medicine, United StatesReviewed by:
Roseanna M. Zanca, NYU Grossman School of Medicine, United StatesMax L. Fletcher, University of Tennessee Health Science Center (UTHSC), United States
Copyright © 2022 Matsukawa, Yoshikawa, Katsuyama, Aizawa and Sato. This is an open-access article distributed under the terms of the Creative Commons Attribution License (CC BY). The use, distribution or reproduction in other forums is permitted, provided the original author(s) and the copyright owner(s) are credited and that the original publication in this journal is cited, in accordance with accepted academic practice. No use, distribution or reproduction is permitted which does not comply with these terms.
*Correspondence: Mutsumi Matsukawa, bWF0c3VrYXdhLm11dHN1bWlAbmlob24tdS5hYy5qcA==
†Present address: Narumi Katsuyama, Cognitive Neuroscience Section, Center for the Evolutionary Origins of Human Behavior, Kyoto University, Inuyama, Japan