- Shobhaben Pratapbhai Patel School of Pharmacy & Technology Management, SVKM’s Narsee Monjee Institute of Management Studies, Mumbai, India
Alzheimer’s disease (AD) has become increasingly prevalent in the elderly population across the world. It’s pathophysiological markers such as overproduction along with the accumulation of amyloid beta (Aβ) plaques and neurofibrillary tangles (NFT) are posing a serious challenge to novel drug development processes. A model which simulates the human neurodegenerative mechanism will be beneficial for rapid screening of potential drug candidates. Due to the comparable neurological network with humans, zebrafish has emerged as a promising AD model. This model has been thoroughly validated through research in aspects of neuronal pathways analogous to the human brain. The cholinergic, glutamatergic, and GABAergic pathways, which play a role in the manifested behavior of the zebrafish, are well defined. There are several behavioral models in both adult zebrafish and larvae to establish various aspects of cognitive impairment including spatial memory, associative memory, anxiety, and other such features that are manifested in AD. The zebrafish model eliminates the shortcomings of previously recognized mammalian models, in terms of expense, extensive assessment durations, and the complexity of imaging the brain to test the efficacy of therapeutic interventions. This review highlights the various models that analyze the changes in the normal behavioral patterns of the zebrafish when exposed to AD inducing agents. The mechanistic pathway adopted by drugs and novel therapeutic strategies can be explored via these behavioral models and their efficacy to slow the progression of AD can be evaluated.
Introduction
Globally there has been a rise in the occurrence of neurological disorders such as Alzheimer’s disease (AD), an advancing multifaceted neurodegenerative disorder, is the leading cause for 70% of dementia worldwide (Mayeux and Stern, 2012). The number of patients suffering from AD is predicted to reach 78 million globally by 2030 and expected rise to 139 million by 2050 (World Health Organization, 2021). The two main pathophysiological characteristic features of AD are formation of amyloid beta (Aβ) plaques and intracellular neurofibrillary tangles (NFT) (Sajjad et al., 2018; Hampel et al., 2021). Extracellular deposits of amyloid plaques form in the brain parenchyma and cerebral blood vessels, a condition termed as congophilic angiopathy or cerebral amyloid angiopathy (CAA)(Thanvi and Robinson, 2006; Jäkel et al., 2021). NFT are intracellular, large paired helical filaments of hyperphosphorylated tau proteins which cause synaptic and neural loss (Moloney et al., 2021). The predominant regions of the human brain which display AD pathology are the association areas of the cerebral cortex and the hippocampus (Wang et al., 2010). The vulnerable neurons among these regions are in the layer II of the entorhinal cortex, the subiculum and the CA1 region of the hippocampus since they are susceptible to display high levels of NFT and are first to be lost in early phases of the disease (Hyman et al., 1984; Kordower et al., 2001; Price et al., 2001). AD associated neuronal loss is observed, at specific cortical and subcortical brain sites such as the trans-entorhinal and entorhinal regions, hippocampus, amygdala, medial septal nucleus, nuclei of the diagonal band of Broca, basal nucleus of Meynert, compact part of the substantia nigra, locus coeruleus, midbrain, and pontine raphe nuclei. The neuronal loss is predominantly due to intra-neuronal deposits and extra-neuronal deposits of abnormal protein, which constitute the irregularly phosphorylated tau protein and the insoluble beta amyloid protein, respectively (Arnold et al., 1991; Stratmann et al., 2016). Further, dysregulation of neurochemicals such as acetylcholine (Ach), dopamine, glutamate, gamma amino butyric acid (GABA), serotonin and noradrenaline, due to neuronal loss in these critical regions has been observed in AD (Kaur et al., 2019). The manifested behavior in AD patients depend on the nature of development of the disease and progressively includes memory lapses, difficulty in organizing and planning of tasks, confusion, disorientation, changes in sleep patterns, anxiety, hallucinations, delusions, paranoia and lack of physical control (Mega et al., 1996; Serda, 2013). The neuropsychiatric symptoms (NPS) are based on disruptions in frontal-subcortical circuits (involving frontal cortex, basal ganglia, thalamus), cortico-cortical network (with hippocampus and amygdala as the epicenters), and the monoaminergic system [comprising of the neuronal cell bodies producing serotonin, norepinephrine, or dopamine located primarily in the brain stem (midbrain, pons, and medulla) and diffusely projected via long axons to virtually all parts of the brain to mediate human behavior] (Geda et al., 2013). These three neurobiological models form the foundations of neuroimaging and biomarker research in AD. It can be characterized into two main types, familial AD (FAD) and sporadic AD (SAD) (Piaceri et al., 2013).
Previously, AD animal models were developed to reproduce the brain lesions formed due to Aβ plaques and NFTs with the assumption that the models would authentically reproduce the human clinical condition. However, many therapeutics which have shown promise in the animal models have failed to show efficacy in clinical trials (Franco and Cedazo-Minguez, 2014). The challenges to translatability of the experiments include lack of good predictable animal models, lack of good biomarkers to indicate disease progression and diversity of genetic susceptibility of the target population under clinical trials (Franco and Cedazo-Minguez, 2014). The drawbacks of AD animal models such as rodents (including transgenics), canines, non-human primates for translation research are well discussed by Vitek and colleagues (Vitek et al., 2020) and will not be reiterated here. Emphasis, is on the development of current AD models that accelerate the translatability of the therapeutic interventions to check the progression of the disease (Götz et al., 2018; Li et al., 2019).
Zebrafish (Danio rerio) which belongs to the infraclass of teleost fishes is found to have certain features that makes it a suitable model for the study of AD and evaluation of therapeutic agents. Zebrafish possesses the following features; genes orthologous to the genes known to be involved in AD (Newman et al., 2011), a neuroanatomic alignment like humans (Guo, 2009; Kozol et al., 2016; Gupta et al., 2018) comparable neural signaling (Horzmann and Freeman, 2016) and the propensity of adult zebrafish and larval zebrafish to serve as models to study behavior (Norton and Bally-Cuif, 2010; Fero et al., 2011). These similarities make zebrafish an exceptional model for studying various neurodegenerative diseases comparable to in vivo and in vitro mammalian models for therapeutic drug screening (Michael Stewart and Kalueff, 2012). In recent years, the zebrafish model has proved to be useful in the study of neurodegeneration due to AD (Thawkar and Kaur, 2021). This review highlights the behavioral model development in both adults and larval zebrafish which enable the comprehension of AD progression mechanisms and efficacy of therapeutic interventions.
Zebrafish as an Alzheimer’s Disease Model
Zebrafish has several characteristics that make it a suitable model for drug discovery. The zebrafish female can produce 200–250 eggs per mating, the embryogenesis is rapid i.e., the entire body structure is made 24 h post fertilization (hpf) and the internal organs like heart, liver and kidney develop 96 hpf. The development of the zebrafish can be visualized in vivo due to the transparency of the larvae. These fishes can be genetically manipulated and the gene expressions can be studied using adequate photo-imaging tools. Further, zebrafish embryos can be used to screen potential compounds in 50 microliter (μl) volumes (Chakraborty et al., 2009) (similar to an in vitro cellular model). Compounds added to water containing zebrafish, undergo the processes of absorption, distribution, metabolism and excretion similar to an in vivo model (Bhusnure et al., 2015). Zebrafish being a vertebrate, is evolutionary closer to humans than that of drosophila or the nematode models which are popular in neuroscientific and genetic studies (Shams et al., 2018). Using zebrafish in research, in lieu of animal studies involving mammals, is advantageous since it fulfils the principle of 3R’s; replacement, refinement and reduction. The European Commission Directive in 2010, exempts the studies with larval zebrafish up to 5 days post fertilization (dpf) from regulatory protection (Cassar et al., 2020). Therefore, studies with zebrafish larvae up to 5 dpf can be carried out as an alternative to testing in higher animals.
Thus, zebrafish applications in neurodegenerative disease research and neuropharmacology are greatly expanding due to lower economic costs, the small size of the organism, a sequenced characterized genome, and well described anatomical structures (Basnet et al., 2019). The most advantageous characteristics of zebrafish as a model would be the optical clarity of the embryo which would allow phenotypic visualization of the effects of genes all through the developmental process and the ease of introducing transient genetic manipulations and chemical manipulations (Koster and Sassen, 2015). All of these characteristics coupled with the presence of several orthologous genes in humans make zebrafish an ideal model to study AD as compared to rodents (Saleem and Kannan, 2018).
Neuroanatomical Comparisons Between Zebrafish and Mammalian Brain
Cognition includes all the mental processing by which knowledge is acquired, retained and used in perception, learning, memory and thinking. Despite not having a defined cortex or hippocampus like the mammalian brain, zebrafishes can perform required learning and memory tasks via various parts in brain that are functionally equivalent to these structures (Calvo and Schluessel, 2021; Figure 1 and Table 1.
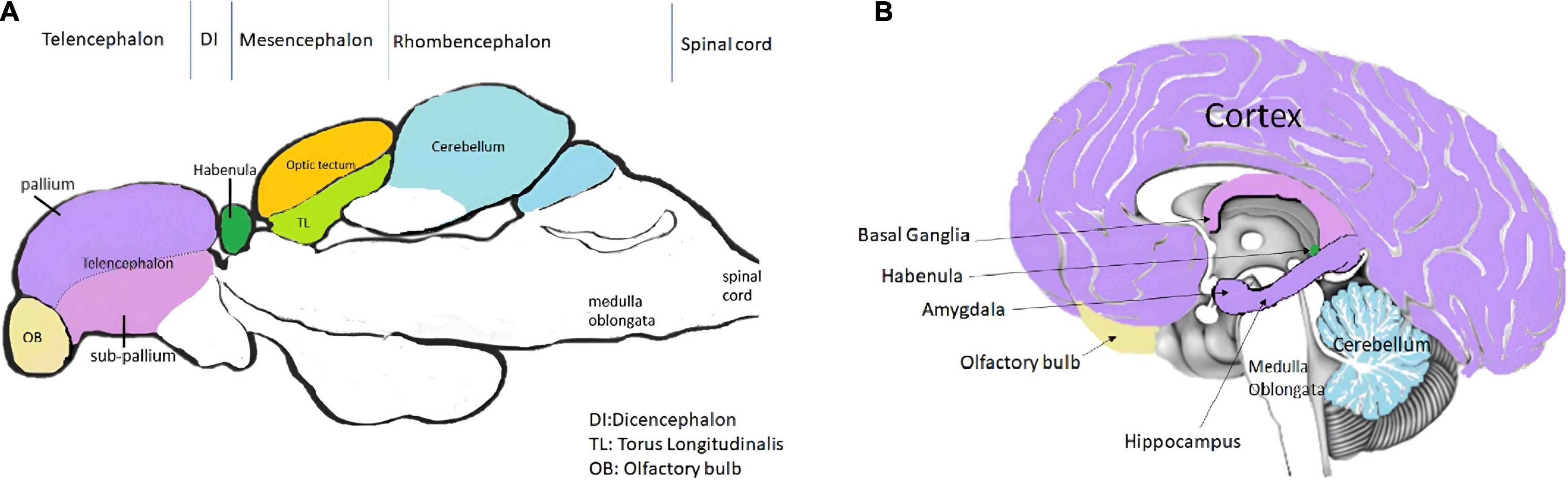
Figure 1. Comparison of panel (A) (a representative of the mid-sagittal section of zebrafish brain) with (B) (a representative of mid-sagittal section of human brain). The figure attempts to represent the important areas involved in learning and memory in humans and zebrafish (Bally-Cuif and Vernier, 2010; Kozol et al., 2016; Langova et al., 2020).
From a structural perspective, the zebrafish brain is similarly aligned as the mammalian brain possessing a forebrain (anterior most part), midbrain, hind brain (posterior most part) and a spinal cord (Guo, 2009). These develop at 24 hpf in the zebrafish. The forebrain of the zebrafish embryo further forms the telencephalon (composed by the pallium, the sub-pallium and the olfactory bulbs), the diencephalon, the hypothalamus and the retina (Vaz et al., 2019). The sub-pallium is the ventral telencephalon while the pallium is the dorsal telencephalon. The ventral telencephalon is sub-divided into two brain nuclei; the ventral nucleus (Vv) and the dorsal nucleus (Vd) of the ventral telencephalon. The dorsal telencephalon is much more complex and is composed of different brain nuclei or regions; the central zone (Dc), the dorsomedial zone (Dm), the lateral zone (Dl) and the posterior zone (Dp) of the dorsal telencephalon (Wullimann et al., 1996; Diotel et al., 2020). The zebrafish brain develops by eversion rather than inversion, due to which some classical regions of the mammalian brain, such as the hippocampus, amygdala, and substantia nigra are not present but the fish brain has structures which can carry out similar functions (Schmidt et al., 2013). Among the estimated 16 neurogenic niches of the zebrafish telencephalon, the Vv of the subpallium is comparable to the sub-ventricular zone (SVZ) of the lateral ventricle of mammals and the Dl, Dp are thought to be equivalent to sub-granular zone (SGZ) between the dentate gyrus (DG) and hilum of the mammalian hippocampus (Diotel et al., 2020; Ghaddar et al., 2021). The lateral pallium (LP) is predicted to be important for spatial learning, whereas the medial pallium (MP) is integral for avoidance learning in the zebrafish (Calvo and Schluessel, 2021). The diencephalon comprises of the thalamus, pineal body, and habenula (Vaz et al., 2019). The forebrain is responsible for receiving and processing sensory information and directing behavior. Telencephalon regulates social behavior, memory and emotion (Broglio et al., 2011) while the diencephalon addresses attention, alertness and circadian actions. Though the structures of telencephalon and diencephalon of the teleost brain are not seen in mammalian brain, some regions of the zebrafish and mammalian forebrain are conserved with respect to architecture and function (Vaz et al., 2019).
The midbrain (mesencephalon) of the zebrafish is important for vision and hearing (Vaz et al., 2019). It lies between the forebrain and hindbrain, containing the optic tectum, torus semicircularis, torus longitudinalis and the midbrain tegmentum (Zebrafish UCL, 2022). The region in mammalian brain functionally similar to the optic tectum, which has a role in vision in zebrafish, is known as the superior colliculus. The inferior colliculus of mammalian brain can be considered to be comparable to the torus longitudinalis which is responsible for auditory sensations in the zebrafish (Wullimann et al., 1996).
The hindbrain (rhombencephalon) of the zebrafish is composed of the posteriorly located medulla oblongata, the ventro-anterior pons and the dorso-anterior cerebellum (Moens and Prince, 2002). The cerebellum of the zebrafish is sub-divided into three parts; the vestibulolateralis lobe, the corpus cerebelli and the valvula cerebelli (Wullimann et al., 1996). The cerebellum controls motor reflexes and plays a role in emotional learning and spatial cognition in teleost fishes (Rodríguez et al., 2005). The fourth ventricle of the brain is formed by the medulla and pons which clubbed with the mid brain are referred to as the “brain stem.” The brain stem regulates respiratory, circulatory and wakefulness activities in the teleost (Moens and Prince, 2002).
Functionally analogous regions in the brains of zebrafish and humans are illustrated and outlined in Figure 1 (Bally-Cuif and Vernier, 2010; Kozol et al., 2016; Langova et al., 2020).
Neurotransmitter Pathways in Mammals and the Zebrafish
AD in humans is caused by excessive neuronal loss predominantly in the hippocampus and the cerebral cortex resulting into cognitive dysfunction, memory loss and behavioral changes in the patient. The symptoms of this disease are exacerbated when there is a massive loss of cholinergic neurons that synthesize Ach, a neurotransmitter involved in memory consolidation (Kaur et al., 2019). The currently approved therapy for AD includes acetylcholinesterase inhibitors (rivastigmine, galantamine, and donepezil), and a N-methyl D-aspartate receptor antagonist (NMDA) (e.g. memantine), and these are thought to preserve cholinergic neurotransmission (Guzior et al., 2014; Kaur et al., 2019) Besides ACh (Tohgi et al., 1994) several other neurotransmitters such as gamma amino butyric acid (GABA) (Zimmer et al., 1984), glutamate (Kuiper et al., 2000), serotonin (Volicer, 1985), dopamine (Pinessi et al., 1987) are present in reduced levels in the cerebrospinal fluid (Kaur et al., 2019) of AD patients and may contribute to the pathology of the disease.
Some AD patients exhibit extrapyramidal symptoms suggesting a loss of dopaminergic neurons (Lopez et al., 1997; Martorana and Koch, 2014). Aβ plaques, NFT, neuronal loss, and a reduction in dopamine content were observed in the neurons constituting the nigrostriatal Pathway. Thus, indicating that dopamine is clearly involved in the pathogenesis of AD. The zebrafish central nervous system uses similar neurotransmitters as the mammalian brain (GABA, glutamate, dopamine, noradrenaline, serotonin, histamine, and ACh) in both interneuron systems and in nerve pathways (Panula et al., 2006, 2010).
The differences and similarities in the metabolic and synthetic routes of these neurotransmitters in zebrafish and mammals are well reviewed by Horzmann and Freeman (2016) and Wasel and Freeman (2020). Three well researched neurotransmitter pathways i.e., cholinergic, glutamatergic, and GABAergic implicated in AD in zebrafish and humans (Santana et al., 2012) are described briefly.
Cholinergic Pathway
Ach is a significant neurotransmitter that is quite widespread in the human central nervous system (CNS) (Bierer et al., 1995). It is essential in learning processes and functions of memory and also helps in regulating the release of other neurotransmitters such as GABA. AD symptoms are manifested after a loss in cholinergic transmission in hippocampal region and the basal areas of the forebrain (Auld et al., 2002; Geula et al., 2021). The presence of acetylcholinesterase neurons in the telencephalon suggests the prevalence of a cholinergic system in the teleost. The telencephalon is a key area in the zebrafish brain for memory consolidation (Flood et al., 1976). The presence of cholinergic neurotransmission pathways has been validated in zebrafish by previous research as well (Williams and Messer, 2004; Mans et al., 2019). Studies using electrophysiological methods, histological methods, and antibody binding methods have indicated that the cholinergic system is widely distributed in the zebrafish brain (Mueller et al., 2004).
Glutamatergic Pathway
Glutamatergic pathway involving the NMDA receptor is one of the important excitatory pathways in vertebrates and is essential in learning, memory, and synaptic plasticity (Li and Tsien, 2009). However, increased amounts of glutamate in synaptic cleft can be neurotoxic (Maragakis and Rothstein, 2004).
Glutamate is a ligand to some classes of metabotropic receptors and ionotropic receptors. Human ionotropic glutamate receptors (iGluRs) include, NMDA, amino-3-hydroxy-5-methyl-4-isoxazolepropionic acid (AMPA), and kainate receptors (Hu et al., 2012). Among ionotropic receptors, the NMDA receptor has been well characterized in zebrafish. Studies further proved that the telencephalon is sensitive to antagonists of NMDA receptors and that its long-term stimulation affects both memory and learning skills (Nam et al., 2004).
Reports on a family of excitatory amino acid transporters (EAATs) in humans, which transport the glutamate away from the synapse, have indicated that, neutralization of excess glutamate could reduce the excitotoxicity of the neuroreceptor (Danbolt, 2001). The presence of EAAT-orthologous genes in zebrafish makes it an ideal model to study this pathway (Rico et al., 2010).
GABAergic Pathway
GABA is an inhibitory neurotransmitter which regulates neural functions via modulating the activity of postsynaptic cells (Bowery and Smart, 2006). Defects in GABA neurotransmission plays a major role in CNS disorders (Staley et al., 1995). The GABAergic system is extensively present throughout the brain and plays a major role in balancing excitatory signals with inhibitory signals (Rissman et al., 2007). The equilibrium between the two is important in synchronization of various CNS functions. Through various pre-clinical and clinical studies it has been established that dysfunction of GABAergic system causes imbalance in excitatory and inhibitory signals. This imbalance is one of the potential markers for the initial stages of AD (Zheng et al., 2021). Neurodegenerative diseases can result from dysfunctional brain cells leading to changes in signaling systems (Lanctôt et al., 2004). The presence of GABAergic neurons and GABAA and GABAC receptors in zebrafish has been reported in the telencephalon, hypothalamus, tectum striatum, and olfactory bulb (Sadamitsu et al., 2021). Glutamic acid decarboxylase (GAD) is an enzyme that is conserved over species (Bosma et al., 1999). Reports of genes similar to human GAD genes in zebrafish were recorded and also their early stage expression was observed. GAD enzyme was majorly concentrated in the areas of the telencephalon medial longitudinal fasciculus in the midbrain, and at the border regions of the rhombomeres in the rostral hindbrain (Martin et al., 1998).
Administration of drugs and chemicals can modify the neurotransmission pathways by targeting various molecular mechanisms. Several compounds induce AD in mammals via one of these pathways (Cholinergic, Glutamatergic, and GABAergic). Figure 2 elaborates the possible pathways by which certain inducers dysregulate signaling to promote AD.
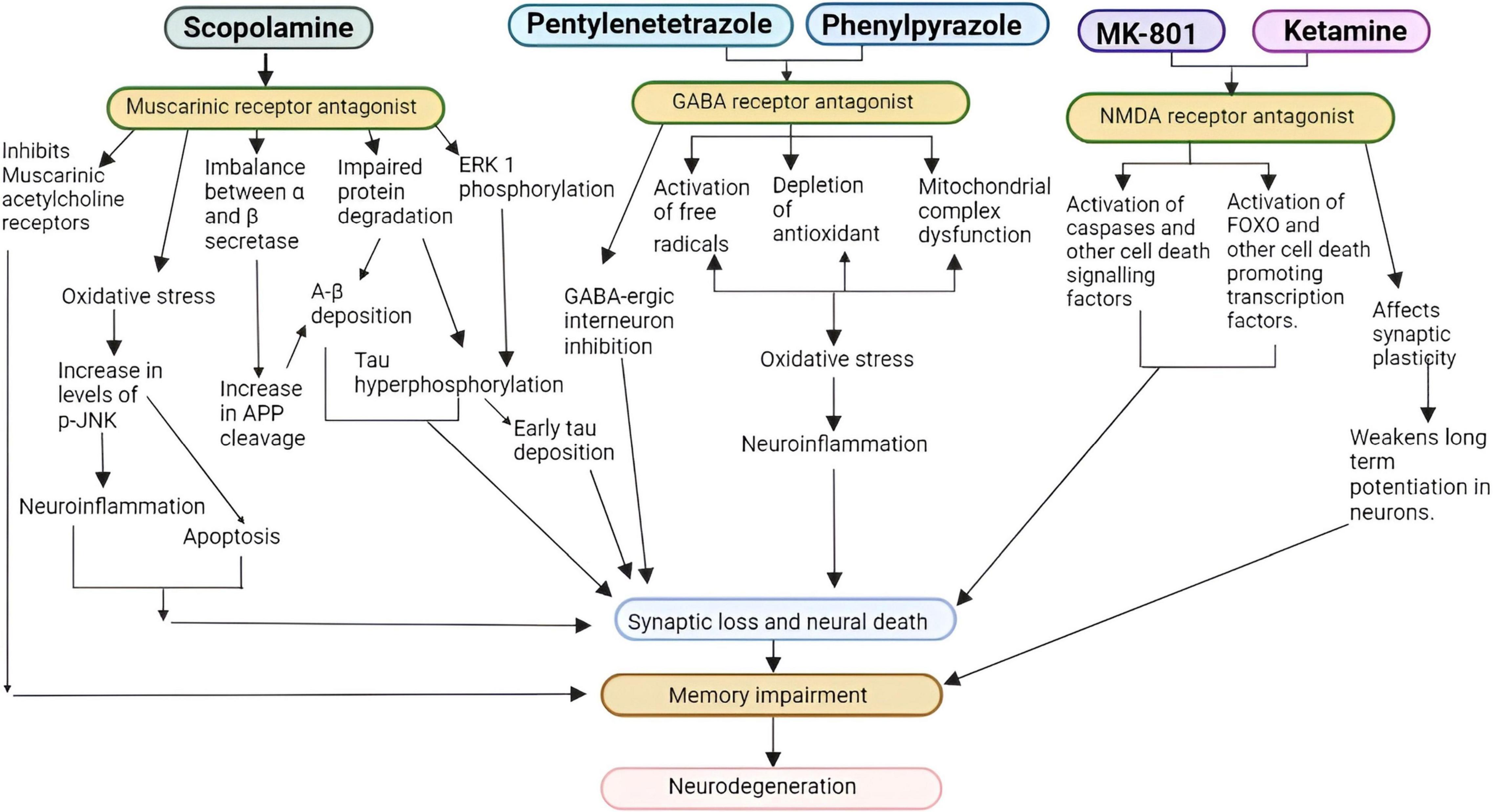
Figure 2. Pathways for cholinergic, glutamatergic, and GABAergic inducers (FOXO, forkhead box transcription factor, p-JNK, p-Jun N-terminal kinase, APP, amyloid precursor protein, NMDA, N-methyl-D- aspartate, GABA, γ-amino butyric acid ERK1, Extracellular signal-regulated kinase 1) (Guan, 2008; Chen and Yeong, 2020).
Genes Implicated in Human and Zebrafish Alzheimer’s Disease
Multiple studies have discovered that in FAD autosomal dominant mutations are mainly in three genes that are presenilin 1 (PS1), presenilin 2 (PS2), and Aβ precursor protein (APP) (Soto-Ospina et al., 2021). The expressed proteins of these genes are involved in ensuring functional cleavage of APP genes or in the formation of soluble Aβ protein. When mutated these proteins lead to the formation of insoluble Aβ which leads to Aβ plaque accumulation (O’Brien and Wong, 2011). SAD is correlated with the expression of the apolipoprotein E (ApoE) variant, ε4 (Xia, 2010). In zebrafish, various homologous gene encoding such as PS1, PS2, APP, and ApoE have been identified (Newman et al., 2014; Kiper and Freeman, 2021). Table 2 enlists the genes that have a prominent role in AD and their orthologs in zebrafish.
Behavioral Models of Alzheimer’s Disease in Zebrafish
Symptoms of AD in humans span from cognitive deficits (evidenced by decrease in memory, spatial recognition, problem solving, and language), abnormal motor movements (tremors, loss of co-ordination, incontinence, eating troubles) to behavioral and emotional issues (depression, agitation, anxiety, depression, tendency to hallucinate) (Voisin and Vellas, 2009; Blanc et al., 2014). The zebrafish when exposed to an AD inducing drug also manifest cognitive and memory impairments. The behavioral responses in the zebrafish can be grouped into basic motor responses which is inclusive of observed sensorimotor responses which in turn is encompassed by the higher learning and memory related reactions of the fish. The types of fish responses and the observed endpoints for behavioral assessments are well described in the publication by Tierney (2011). Major behavioral endpoints are conducted on the zebrafish 3–4 days post fertilization except learning or memory dependent endpoints (Tierney, 2011). The behavioral tests that can be performed on the zebrafish are shown in Figure 3. The AD inducing agents, effects of the moieties counter-acting AD in zebrafish and the behavioral model used for assessment are outlined in Table 3.
Models for Adult Zebrafish
Y-Maze Test
Over the last couple of decades, there has been a significant rise in interest in the behavioral features of zebrafish. However, the current protocols are based on reinforcement/reward or avoidance and have long training periods. The advantages of Y-maze are that, it is simple and has quick training sessions, allowing specific training of memory as it does not include conditioned learning. It also minimizes factors that might influence performance such as emotional and motivational states. This task assesses the natural tendency of the fish to explore a novel arm when the mnemonic demand is less. As the retention time does last for more than a couple of hours, the performance of the fish can be evaluated multiple times (Benvenutti et al., 2021). Advanced free movement pattern (FMP) Y-maze assay is a combination of existing aspects of the Y-maze assay with certain modifications in the analysis. In a study conducted by Cleal et al., the effects of periodic doses of D-Amphetamine and nicotine on cognitive behavior in zebrafish in the FMP-Y maze were observed for 14 days. The observed tetragram based on the alteration patterns were analyzed. It was observed that there was an improved memory function in the zebrafish in initial stages after a short withdrawal period along with a decrease in cognitive flexibility which was observed on D-amphetamine administration but not when treated with nicotine (Cleal et al., 2021).
The apparatus used for this test is a three-armed glass tank whose length, width, and height are 25, 8, and 15 cm, respectively. Each arm can be associated with different visual cues and it should be ensured that zebrafish is not biased toward a particular clue (Cognato et al., 2012). Two arms of the maze are opened during initial training and one arm remains blocked. It is observed that the novel arm once opened for exploration, zebrafish normally tend to spend more time in this arm (Zanandrea et al., 2018). This indicates that they are recognizing the novel arm and are willing to explore it. For disease-induced (via inducers such as scopolamine) zebrafishes, it has been observed that they have a lower intention of exploration and tend to float around in a particular arm. To create an unbias environment with regards to the cues the maze is rotated for each experiment (Valu et al., 2021b).
Spontaneous alterations analysis allows the measurement of spatial memory of the animals. The following is used to calculate the same:
The higher the percentage of alteration the higher is the tendency to explore the novel arm which leads to an inference of low anxiety and good spatial memory (Kraeuter et al., 2019).
Hole Board Test
Hole board is a method normally used for the screening of prospective anxiolytic drugs. It is based on the hypothesis that the anxiety state of an animal is inversely proportional to its intention to look for baited holes. Initially, the animals are allowed to explore the experimental tank for 15 mins without any external visual cues in the open field and baited holes in the hole board. After habituation for a period of 4 days, training of zebrafish is commenced where only one hole of the whole board is baited. The time required to find the hole with the bait is noted and to avoid fixed directional swimming the fish is released into the tank from various locations. The maximum time given to each fish to find the baited hole is 3 mins (van der Staay et al., 2012; Bailey et al., 2015; Ruhl et al., 2015). This experiment tests the spatial cognition of the fish and therefore is an important model for testing of AD novel drug therapies.
T-Maze Test
T-maze is a method of assessing spatial memory, associative memory and learning in rodents and fishes. It has also been used in the evaluation of the pharmacological results of drugs in animal behavioral models. In zebrafish, this model is based on how quickly it can grasp a certain behavior through discrimination training which contains multiple sessions of training and contains a stimulus or food reward. The fish is trained to choose an arm through pavlovian conditioning (Gould, 2011; Bault et al., 2015).
The apparatus has one long arm which is the starting zone for the zebrafish and two short arms among which one is the favorable zone and the other one is the unfavorable zone These zones can also be color-coded. During training, the zebrafish is allowed to acclimatize within the two arms it can choose. If it chooses the wrong arm, it meets unfavorable conditions such as disturbance. Upon choosing the correct arm the zebrafish does not face any disturbance or can get a positive reinforcement such as food. On the day of acquisition, time spent in unfavorable and favorable zones is noted down. The control zebrafish will spend more time in the favorable zone and the AD model will not be able to develop such a bias. In the recent research conducted by Moreira et al., the effects of oxybenzone on behavior and cognition was tested by using various behavioral models including T-maze test. The results showed a decrease in the explorative instincts of the animal and impairment of the animal’s memory (Moreira and Luchiari, 2022).
Inhibitory Avoidance Paradigm
The apparatus consists of an aquarium which is of the dimensions 60 cm × 30 cm × 30 cm and contains about 10 cm water level. This aquarium is to be divided into two compartments with the help of a manually operating sliding door and the surfaces of each compartment are white and black, respectively. The black compartment is wired to a power source of 1-9V (AC). After allowing the fishes to acclimatize in both the compartments, an electric shock is given in the dark compartment for about 5 s and then the fish is returned to its home tank (Manuel et al., 2014). The following day the trained fish’s ability to avoid the dark compartment is observed. The fishes that do not enter the dark compartment in 3 mins are marked as successful in exercising their memory to avoid making the adverse choice and in the AD model, the fishes would show no such bias due to memory loss (Manuel et al., 2014).
Novel Tank Diving Test
For several animals, including zebrafish, new surroundings can be anxiogenic. This test has been considerably used in the modeling of anxiety and assessing the effects of anxiolytics. As this test is based on the idea of a novel surrounding, it is equivalent to the open field test generally performed in rodents. In this test, the initial response of an adult zebrafish is to stay at the bottom of the tank until they get accustomed to the rest of the tank (Stewart et al., 2012; Haghani et al., 2019).
In this method, the fishes are paired and transferred into a behavioral examination tank. After holding the fishes in the behavioral tanks, they are transferred into separate 100 ml beakers containing 25–30 mL water for immediate transportation into observational tanks. After the transfer, the fishes are observed in the new environment. The ability of the fishes to explore the novel tank is investigated. The more amount of time a fish spends at the bottom of the tank indicates high anxiety levels, which is a common manifestation in neurodegenerative disease models (Haghani et al., 2019).
Anwer et al. (2021) have recently hypothesized that this test when conducted in taller tanks yields a high repeatability.
Light Dark Test
The light-dark test is commonly used in the quantification of anxiety in rodents but in recent years has been also used for screening adult zebrafish. This model uses the nature of phototaxis displayed in fishes to evaluate the anxiety (Facciol et al., 2017). It is a straightforward and efficient method which does not require pre-training and enables understanding of pharmacological modifications in zebrafish. Further pharmacological studies observed that the method is sensitive to anxiolytic but not to the panicolytic drugs. In nature, a preference for the dark allows them to evade predators rather than being exposed in a white background. Anxiety inducing drugs alter zebrafish’s tendency to explore the tank and they stay in the dark region of the tank for longer periods compared to control, displaying negligible or low explorative preferences. The light-dark apparatus that is used for rodents is modified accordingly for application in zebrafishes. The tank has dimensions of 15 cm height × 30 cm length × 16 cm width (Stewart et al., 2011). The major differences in the apparatus are that it is sealed to avoid leakage of water which is filled to a height of 12 cm, there is also no sliding door between the two sides (light and dark) hence the fish are free to swim in both the compartments (Stewart et al., 2011). Currently several inducers of neurological diseases are being investigated to test for manifestations of symptoms like anxiety using this model. Decynium-22 was tested by Maximino et al. for inducing anxiety using the light dark test model (Maximino, 2021).
Models for Larvae Zebrafish
Startle Response
Zebrafish larvae when exposed to abrupt stimuli of unexpected touch (tactile), loud sound (acoustic), or sudden bright light (visual) tend to react by a swimming burst to escape from the threatening stimuli (Fetcho and McLean, 2009). Startle response is important to understand whether the larvae have proper sensory and motor stimuli which are critical for survival (Troconis et al., 2017; Faria et al., 2019). The different times for development of different responses are listed in Table 4.
This behavioral model is important for neuropharmacological research to observe if the drugs being administered can restore the normal response to startling stimulus in larvae (Basnet et al., 2019; Banono and Esguerra, 2020). Locomotion analysis in larvae via high-throughput methods will be able to provide observations required for testing and identification of neuroactive compounds. Recent research by Png et al. (2021) used acoustic startle response with the help of 36 continuous mechanical taps, to observe the impairment of cognitive ability in response to 0.8 uM morphine administration for 5 days starting from 5 h post fertilization.
Optokinetic Response
The optokinetic response is first observed in zebrafish 73 h post-fertilization and it slowly develops until 4 days post fertilization where it reaches to 9/10th of an adult goldfish (Easter and Nicola, 1997). Around the time zebrafish larvae start hunting for food, the response is completely developed. There are various types of optokinetic responses but the horizontal optokinetic response is the only one studied in zebrafish and it is also commonly studied in other species. This response signifies a stereotyped eye movement in response to any form of movement within the field of vision (Huang and Neuhauss, 2008). To evoke an optokinetic response in zebrafish larvae an LCD screen displaying moving graphics or a rotating drum containing black and white stripes is used (Cameron et al., 2013).
Effect on the optokinetic response via chemicals that affect the central nervous system allows the study of Alzheimer’s induction and treatment by considering this response as an analysis parameter (Barbereau et al., 2021). This behavioral model has been used to study the effect of drugs that reduce anxiety such as fluoxetine. It was observed that the anxiolytic drug expunged the consequences (Hodges, 2021).
Thigmotaxis
When exposed to a new environment animals tend to have a natural tendency of moving to the periphery of the novel environment. This behavior, also known as the wall-hugging behavior, is used to estimate anxiety and it is conserved across all species (Simon et al., 1994; Colwill and Creton, 2011). Zebrafish larva shows thigmotaxis in response to novel environments at 5 days post fertilization. Exposure to sudden darkness or sudden light can elucidate this behavior in zebrafish (Liu et al., 2016). The test should be performed in an arena where there is enough space to distinguish between inner and outer zones so the choice is usually a 24 well plate (Schnörr et al., 2012).
This test can be used to study anxiety-like behavior in the zebrafish (Cho et al., 2012). Thus, using this behavioral model one can study the induction of Alzheimer’s like phenotype and efficacy of anxiolytic drugs (Ahmad and Richardson, 2013). Recent studies have been conducted to test the anxiolytic effect of compounds like Buspirone hydrochloride by observing thigmotaxic behavior in zebrafish as it was a responsive and straightforward way for evaluating the efficacy of similar anxiety reducing drugs (Abozaid and Gerlai, 2022).
Optomotor Response
Zebrafish can be exposed to aversive and non-aversive visual cues on an LCD screen monitor. For responsive cues, 15 zebrafish larvae are placed in a single petri dish and then placed on a monitor. The zebrafish is exposed to moving red and white stripes 5 days post-fertilization (Fleisch and Neuhauss, 2006; Creton, 2009; Nery et al., 2017). The directions of the stripes are alternated every 1 min with a 5-s interval, where the cue is faded (Karaduman et al., 2021). The animals usually tend to swim in a direction similar to the movement of the stripes. The petri plate is divided into three zones to observe the number of fishes present in the correct zone. In aversive cues, the LCD monitor displays a red bouncing ball. The animals will try to swim to the non-stimulated area of the petri dish to escape the bouncing ball (Pelkowski et al., 2011; Nery et al., 2014). These tests help in determining the cognitive performance of animals (Karaduman et al., 2021). Quinpirole, a compound that induced anxiety-like symptoms by targeting dopaminergic signaling, was observed to impair optomotor response (Nabinger et al., 2021).
Prey Capture
After hatching, zebrafish embryos start swimming toward the prey, a behavior that is essential for survival (Muto and Kawakami, 2013). To test this behavior a small air bubble released in the test arena is utilized as prey (Gahtan et al., 2005). In the assay, the latent period before the attack on the prey, the number of attacked or captured prey, and the efficiency with which the prey is captured are the parameters measured (Tierney, 2011). The convergence of eye movement marks the onset of hunting behavior and the prey attack is defined by the biting motion of larvae (Bianco et al., 2011). This behavior involves decision making therefore can be used to assess cognitive behavior (Formella et al., 2018).
Conclusion and Future Prospects
The zebrafish model is a robust system for the physiological and genetic study of AD (Xia, 2010). The advantages of the zebrafish models are that they allow sizable forward genetic screening, investigation of different properties such as temporal, spatial, and also real-time observation of the pathological changes in vivo, exploration of behavioral and pharmaceutical in both larvae and adults (Lorent et al., 2004). Over the years, extensive research has provided abundant evidence and essential information about the correlation between disease and its development in zebrafish (Brun et al., 2021). Due to advancements in modern technology, the zebrafish system can be established as a substitute to mammalian models in drug development, target identification, and validation along with providing shorter routes for novel therapeutic strategies (Chakraborty et al., 2009). The larva-adult duality in the zebrafish model has a large-scale utilization for preclinical studies before rodent models for validating novel therapies (Bailey et al., 2015). The article sheds light on the use of behavioral models of both the larva and adult zebrafish along with the different neurotransmitter pathways that regulate them but strategies to overcome the complexities are yet to be established (Santana et al., 2012; Saleem and Kannan, 2018). Zebrafish as a model could assist in further understanding neuroanatomical circuits and their role in neurodegenerative disorders (Stewart et al., 2015). Further research in regard to zebrafish telencephalon is required to gain an understanding of the neurological and molecular mechanism at the onset of AD. This study would allow us to extrapolate the observations and corelate them to the early biomarkers of mammalian AD commencement in the hippocampus. This would assist in the progress of novel preventive therapies. It can be concluded that the zebrafish is a promising model for gaining a perception of mechanisms of AD but requires further optimization to be indispensable to novel drug screening.
Author Contributions
GK and AU visualized the presented idea, contributed to the manuscript writing, supervised the project and corrected, revised, and approved the manuscript. MB, SB-P, and AS contributed equally to doing literature searches and in the preparation of the manuscript. All authors contributed to the article and approved the submitted version.
Funding
We are acknowledged for the financial support to research work by the core research grant of DST SERB, Government of India (CRG/2020/003316).
Conflict of Interest
The authors declare that the research was conducted in the absence of any commercial or financial relationships that could be construed as a potential conflict of interest.
Publisher’s Note
All claims expressed in this article are solely those of the authors and do not necessarily represent those of their affiliated organizations, or those of the publisher, the editors and the reviewers. Any product that may be evaluated in this article, or claim that may be made by its manufacturer, is not guaranteed or endorsed by the publisher.
Acknowledgments
We are thankful to Shobhaben Pratapbhai Patel School of Pharmacy & Technology Management, SVKM’s NMIMS for providing the facility.
References
Abozaid, A., and Gerlai, R. (2022). Behavioral effects of buspirone in Juvenile zebrafish of two different genetic backgrounds. Toxics 10:22. doi: 10.3390/toxics10010022
Ahmad, F., and Richardson, M. K. (2013). Exploratory behaviour in the open field test adapted for larval zebrafish: impact of environmental complexity. Behav. Process. 92, 88–98. doi: 10.1016/J.BEPROC.2012.10.014
Amo, R., Aizawa, H., Takahoko, M., Kobayashi, M., Takahashi, R., Aoki, T., et al. (2010). Identification of the zebrafish ventral habenula as a homolog of the mammalian lateral habenula. J. Neurosci. 30, 1566–1574.
Anwer, H., Mason, D., Zajitschek, S., Noble, D. W. A., Hesselson, D., Morris, M. J., et al. (2021). An efficient new assay for measuring zebrafish anxiety: tall tanks that better characterize between-individual differences. J. Neurosci. Methods 356:109138. doi: 10.1016/J.JNEUMETH.2021.109138
Arnold, S. E., Hyman, B. T., Flory, J., Damasio, A. R., and van Hoesen, G. W. (1991). The topographical and neuroanatomical distribution of neurofibrillary tangles and neuritic plaques in the cerebral cortex of patients with Alzheimer’s disease. Cereb. Cortex 1, 103–116.
Auld, D. S., Kornecook, T. J., Bastianetto, S., and Quirion, R. (2002). Alzheimer’s disease and the basal forebrain cholinergic system: relations to beta-amyloid peptides, cognition, and treatment strategies. Prog. Neurobiol. 68, 209–245. doi: 10.1016/S0301-0082(02)00079-5
Babin, P. J., Thisse, C., Durliat, M., Andre, M., Akimenko, M.-A., and Thisse, B. (1997). Both apolipoprotein E and A-I genes are present in a nonmammalian vertebrate and are highly expressed during embryonic development (brainembryo nutritionlipoproteinyolk syncytial layerzebrafish). Proc. Natl. Acad. Sci. U.S.A. 94, 8622–8627.
Bai, X.-C., Yan, C., Yang, G., Lu, P., Ma, D., Sun, L., et al. (2015). An atomic structure of human γ-secretase. Nature 525, 212–217. doi: 10.1038/nature14892
Bailey, J. M., Oliveri, A. N., and Levin, E. D. (2015). Pharmacological analyses of learning and memory in zebrafish (Danio rerio). Pharmacol. Biochem. Behav. 139:103. doi: 10.1016/J.PBB.2015.03.006
Bally-Cuif, L., and Vernier, P. (2010). Organization and physiology of the zebrafish nervous system. Fish Physiol. 29, 25–80. doi: 10.1016/S1546-5098(10)02902-X
Banono, N. S., and Esguerra, C. v. (2020). Pharmacological validation of the prepulse inhibition of startle response in larval zebrafish using a commercial automated system and software. JoVE J. Vis. Exp. 161:e61423. doi: 10.3791/61423
Barbereau, C., Cubedo, N., Maurice, T., and Rossel, M. (2021). Zebrafish models to study new pathways in Tauopathies. Int. J. Mol. Sci. 22:4626. doi: 10.3390/IJMS22094626
Basnet, R. M., Zizioli, D., Taweedet, S., Finazzi, D., and Memo, M. (2019). Zebrafish larvae as a behavioral model in neuropharmacology. Biomedicines 7:23.
Bault, Z. A., Peterson, S. M., and Freeman, J. L. (2015). Directional and color preference in adult zebrafish: implications in behavioral and learning assays in neurotoxicology studies. J. Appl. Toxicol. 35, 1502–1510. doi: 10.1002/jat.3169
Baumkotter, F., Schmidt, N., Vargas, C., Schilling, S., Weber, R., Wagner, K., et al. (2014). Amyloid precursor protein dimerization and synaptogenic function depend on copper binding to the growth factor-like domain. J. Neurosci. 34, 11159–11172. doi: 10.1523/JNEUROSCI.0180-14.2014
Benvenutti, R., Marcon, M., Gallas-Lopes, M., de Mello, A. J., Herrmann, A. P., and Piato, A. (2021). Swimming in the maze: an overview of maze apparatuses and protocols to assess zebrafish behavior. Neurosci. Biobehav. Rev. 127, 761–778. doi: 10.1016/j.neubiorev.2021.05.027
Best, J. D., Berghmans, S., Hunt, J. J. F. G., Clarke, S. C., Fleming, A., Goldsmith, P., et al. (2007). Non-associative learning in larval zebrafish. Neuropsychopharmacology 33, 1206–1215.
Bhusnure, O. G., Mane, J. M., and Gholve, S. B. (2015). Drug target screening and its validation by zebrafish as a novel tool. Pharm. Anal. Acta 6:426. doi: 10.4172/2153-2435.1000426
Bianco, I. H., Kampff, A. R., and Engert, F. (2011). Prey capture behavior evoked by simple visual stimuli in larval zebrafish. Front. Syst. Neurosci. 5:101. doi: 10.3389/fnsys.2011.00101
Bierer, L. M., Haroutunian, V., Gabriel, S., Knott, P. J., Carlin, L. S., Purohit, D. P., et al. (1995). Neurochemical correlates of dementia severity in Alzheimer’s disease: relative importance of the cholinergic deficits. J. Neurochem. 64, 749–760. doi: 10.1046/J.1471-4159.1995.64020749.X
Blanc, F., Noblet, V., Philippi, N., Cretin, B., Foucher, J., Armspach, J.-P., et al. (2014). Right anterior insula: core region of hallucinations in cognitive neurodegenerative diseases. PLoS One 9:e114774. doi: 10.1371/journal.pone.0114774
Boiangiu, R. S., Mihasan, M., Gorgan, D. L., Stache, B. A., and Hritcu, L. (2021). Anxiolytic, promnesic, anti-acetylcholinesterase and antioxidant effects of cotinine and 6-hydroxy-l-nicotine in scopolamine-induced zebrafish (Danio rerio) model of Alzheimer’s disease. Antioxidants 10, 1–28. doi: 10.3390/antiox10020212
Bosma, P. T., Blázquez, M., Collins, M. A., Bishop, J. D. D., Drouin, G., Priede, I. G., et al. (1999). Multiplicity of glutamic acid decarboxylases (GAD) in vertebrates: molecular phylogeny and evidence for a new GAD paralog. Mol. Biol. Evol. 16, 397–404.
Bowery, N. G., and Smart, T. G. (2006). GABA and glycine as neurotransmitters: a brief history. Br. J. Pharmacol. 147, S109–S119. doi: 10.1038/sj.bjp.0706443
Broglio, C., Gómez, A., Durán, E., Salas, C., and Rodríguez, F. (2011). “Brain and cognition in teleost fish,” in Fish Cognition and Behavior, eds C. Brown, K. Laland, and J. Krause (Oxford: Wiley-Blackwell), 325–358.
Brun, N. R., Panlilio, J. M., Zhang, K., Zhao, Y., Ivashkin, E., Stegeman, J. J., et al. (2021). Developmental exposure to non-dioxin-like polychlorinated biphenyls promotes sensory deficits and disrupts dopaminergic and GABAergic signaling in zebrafish. Commun. Biol. 4, 1–12. doi: 10.1038/s42003-021-02626-9
Bühler, A., and Carl, M. (2021). Zebrafish tools for deciphering habenular network-linked mental disorders. Biomolecules 11, 1–18. doi: 10.3390/biom11020324
Calvo, R., and Schluessel, V. (2021). Neural substrates involved in the cognitive information processing in teleost fish. Anim. Cogn. 24, 923–946. doi: 10.1007/S10071-021-01514-3
Cameron, D. J., Rassamdana, F., Tam, P., Dang, K., Yanez, C., Ghaemmaghami, S., et al. (2013). The optokinetic response as a quantitative measure of visual acuity in zebrafish. J. Vis. Exp. JoVE 80:50832. doi: 10.3791/50832
Campbell, W. A., Yang, H., Zetterberg, H., Baulac, S., Sears, J. A., Liu, T., et al. (2006). Zebrafish lacking Alzheimer presenilin enhancer 2 (Pen-2) demonstrate excessive p53-dependent apoptosis and neuronal loss. J. Neurochem. 96, 1423–1440. doi: 10.1111/j.1471-4159.2006.03648.x
Capatina, L., Todirascu-Ciornea, E., Napoli, E. M., Ruberto, G., Hritcu, L., and Dumitru, G. (2020). Thymus vulgaris essential oil protects zebrafish against cognitive dysfunction by regulating cholinergic and antioxidants systems. Antioxidants 9, 1–18. doi: 10.3390/antiox9111083
Cassar, S., Adatto, I., Freeman, J. L., Gamse, J. T., Iturria, I., Lawrence, C., et al. (2020). Use of zebrafish in drug discovery toxicology. Chem. Res. Toxicol. 33, 95–118. doi: 10.1021/acs.chemrestox.9b00335
Chakraborty, C., Hsu, C., Wen, Z., Lin, C., and Agoramoorthy, G. (2009). Zebrafish: a complete animal model for in vivo drug discovery and development. Curr. Drug Metab. 10, 116–124. doi: 10.2174/138920009787522197
Chen, M., Martins, R. N., and Lardelli, M. (2009). Complex splicing and neural expression of duplicated tau genes in zebrafish embryos. J. Alzheimers Dis. 18, 305–317. doi: 10.3233/JAD-2009-1145
Chen, W. N., and Yeong, K. Y. (2020). Scopolamine, a toxin-induced experimental model, used for research in Alzheimer’s disease. CNS Neurol. Disord. Drug Targets 19, 85–93. doi: 10.2174/1871527319666200214104331
Cheng, R. K., Jesuthasan, S. J., and Penney, T. B. (2014). Zebrafish forebrain and temporal conditioning. Philos. Trans. R. Soc. Lond. B Biol. Sci. 369:20120462. doi: 10.1098/rstb.2012.0462
Cho, H., Lee, C. J., Choi, J., Hwang, J., and Lee, Y. (2012). Anxiolytic effects of an acetylcholinesterase inhibitor, physostigmine, in the adult zebrafish. Anim. Cells Syst. 16, 198–206. doi: 10.1080/19768354.2011.642084
Cleal, M., Fontana, B. D., and Parker, M. O. (2021). The cognitive and behavioral effects of D-amphetamine and nicotine sensitization in adult zebrafish. Psychopharmacology 238, 2191–2200.
Cognato, G., de, P., Bortolotto, J. W., Blazina, A. R., Christoff, R. R., Lara, D. R., et al. (2012). Y-Maze memory task in zebrafish (Danio rerio): the role of glutamatergic and cholinergic systems on the acquisition and consolidation periods. Neurobiol. Learn. Mem. 98, 321–328. doi: 10.1016/J.NLM.2012.09.008
Colwill, R. M., and Creton, R. (2011). Imaging escape and avoidance behavior in zebrafish larvae. Rev. Neurosci. 22, 63–73. doi: 10.1515/RNS.2011.008
Creton, R. (2009). Automated analysis of behavior in zebrafish larvae. Behav. Brain Res. 203, 127–136. doi: 10.1016/j.bbr.2009.04.030
Diotel, N., Lübke, L., Strähle, U., and Rastegar, S. (2020). Common and distinct features of adult neurogenesis and regeneration in the telencephalon of zebrafish and mammals. Front. Neurosci. 14:568930. doi: 10.3389/fnins.2020.568930
Dumitru, G., El-Nashar, H. A. S., Mostafa, N. M., Eldahshan, O. A., Boiangiu, R. S., Todirascu-Ciornea, E., et al. (2019). Agathisflavone isolated from Schinus polygamus (Cav.) Cabrera leaves prevents scopolamine-induced memory impairment and brain oxidative stress in zebrafish (Danio rerio). Phytomedicine 58:152889. doi: 10.1016/j.phymed.2019.152889
Easter, S. S., and Nicola, G. N. (1997). The development of eye movements in the zebrafish (Danio rerio). Dev. Psychobiol. 31, 267–276.
Edbauer, D., Winkler, E., Regula, J. T., Pesold, B., Steiner, H., and Haass, C. (2003). Reconstitution of gamma-secretase activity. Nat. Cell Biol. 5, 486–488. doi: 10.1038/ncb960
Esterházy, D., Stützer, I., Wang, H., Rechsteiner, M. P., Beauchamp, J., Döbeli, H., et al. (2011). Bace2 is a β cell-enriched protease that regulates pancreatic β cell function and mass. Cell Metab. 14, 365–377. doi: 10.1016/j.cmet.2011.06.018
Facciol, A., Tran, S., and Gerlai, R. (2017). Re-examining the factors affecting choice in the light–dark preference test in zebrafish. Behav. Brain Res. 327, 21–28. doi: 10.1016/j.bbr.2017.03.040
Fagan, A. M., Bu, G., Sun, Y., Daugherty, A., and Holtzman, D. M. (1996). Apolipoprotein E-containing high density lipoprotein promotes neurite outgrowth and is a ligand for the low density lipoprotein receptor-related protein. J. Biol. Chem. 271, 30121–30125. doi: 10.1074/jbc.271.47.30121
Faria, M., Prats, E., Novoa-Luna, K. A., Bedrossiantz, J., Gómez-Canela, C., Gómez-Oliván, L. M., et al. (2019). Development of a vibrational startle response assay for screening environmental pollutants and drugs impairing predator avoidance. Sci. Total Environ. 650, 87–96. doi: 10.1016/J.SCITOTENV.2018.08.421
Feng, J., Song, G., Wu, Y., Chen, X., Pang, J., Xu, Y., et al. (2021). Plasmalogens improve swimming performance by modulating the expression of genes involved in amino acid and lipid metabolism, oxidative stress, and Ferroptosis in an Alzheimer’s disease zebrafish model. Food Funct. 12, 12087–12097. doi: 10.1039/d1fo01471d
Fero, K., Yokogawa, T., and Burgess, H. A. (2011). “The behavioral repertoire of larval zebrafish,” in Zebrafish Models in Neurobehavioral Research. Neuromethods, Vol. 52, eds A. Kalueff and J. Cachat (Totowa, NJ: Humana Press), 249–291. doi: 10.1007/978-1-60761-922-2_12
Fetcho, J. R., and McLean, D. L. (2009). “Startle response,” in Encyclopedia of Neuroscience, ed. L. R. Squire (Amsterdam: Elsevier Ltd), 375–379. doi: 10.1016/B978-008045046-9.01973-2
Fleisch, V. C., and Neuhauss, S. C. F. (2006). Visual behavior in zebrafish. Zebrafish 3, 191–201. doi: 10.1089/ZEB.2006.3.191
Flood, N. C., Bruce Overmier, J., Savage, G. E., Overmier, J. B., and Savage Teleost, G. E. (1976). THEORETICAL REVIEW teleost telencephalon and learning: an interpretive review of data and hypotheses 1. Physiol. Behav. 16, 783–788.
Formella, I., Svahn, A. J., Radford, R. A. W., Don, E. K., Cole, N. J., Hogan, A., et al. (2018). Real-time visualization of oxidative stress-mediated neurodegeneration of individual spinal motor neurons in vivo. Redox Biol. 19, 226–234. doi: 10.1016/J.REDOX.2018.08.011
Fraering, P. C., Ye, W., Strub, J. M., Dolios, G., LaVoie, M. J., Ostaszewski, B. L., et al. (2004). Purification and characterization of the human gamma-secretase complex. Biochemistry 43, 9774–9789. doi: 10.1021/BI0494976
Franco, R., and Cedazo-Minguez, A. (2014). Successful therapies for Alzheimer’s disease: why so many in animal models and none in humans? Front. Pharmacol. 5:146. doi: 10.3389/fphar.2014.00146
Gahtan, E., Tanger, P., and Baier, H. (2005). Visual prey capture in larval zebrafish is controlled by identified reticulospinal neurons downstream of the Tectum. J. Neurosci. 25, 9294–9303. doi: 10.1523/JNEUROSCI.2678-05.2005
Ganz, J., Kroehne, V., Freudenreich, D., Machate, A., Geffarth, M., Braasch, I., et al. (2015). Subdivisions of the adult zebrafish pallium based on molecular marker analysis. F1000Res. 3:308. doi: 10.12688/f1000research.5595.2
Ganzen, L., Venkatraman, P., Pang, C., Leung, Y. F., and Zhang, M. (2017). Utilizing zebrafish visual behaviors in drug screening for retinal degeneration. Int. J. Mol. Sci. 18:1185. doi: 10.3390/ijms18061185
Gao, X., Zhang, P., Chen, J., Zhang, L., Shang, N., Chen, J., et al. (2022). Necrostatin-1 relieves learning and memory deficits in a zebrafish model of Alzheimer’s disease induced by aluminum. Neurotox. Res. 40, 198–214. doi: 10.1007/s12640-021-00463-6
Gawel, K., Kukula-koch, W., Banono, N. S., Nieoczym, D., Targowska-duda, K. M., Czernicka, L., et al. (2021). 6-gingerol, a major constituent of Zingiber officinale rhizoma, exerts anticonvulsant activity in the pentylenetetrazole- induced seizure model in larval zebrafish. Int. J. Mol. Sci. 22:7745. doi: 10.3390/ijms22147745
Geda, Y. E., Schneider, L. S., Gitlin, L. N., Miller, D. S., Smith, G. S., Bell, J., et al. (2013). Neuropsychiatric symptoms in Alzheimer’s disease: past progress and anticipation of the future. Alzheimers Dement. 9, 602–608. doi: 10.1016/j.jalz.2012.12.001
Geula, C., Dunlop, S. R., Ayala, I., Kawles, A. S., Flanagan, M. E., Gefen, T., et al. (2021). Basal forebrain cholinergic system in the dementias: vulnerability, resilience, and resistance. J. Neurochem. 158, 1394–1411. doi: 10.1111/JNC.15471
Ghaddar, B., Lübke, L., Couret, D., Rastegar, S., and Diotel, N. (2021). Cellular mechanisms participating in brain repair of adult zebrafish and mammals after injury. Cells 10:391. doi: 10.3390/cells10020391
Götz, J., Bodea, L.-G., and Goedert, M. (2018). Rodent models for Alzheimer disease. Nat. Rev. Neurosci. 19, 583–598. doi: 10.1038/s41583-018-0054-8
Gould, G. G. (2011). Modified associative learning T-maze test for zebrafish (Danio rerio) and other small teleost fish. Neuromethods 51, 61–73. doi: 10.1007/978-1-60761-953-6_5
Groth, C., Nornes, S., McCarty, R., Tamme, R., and Lardelli, M. (2002). Identification of a second presenilin gene in zebrafish with similarity to the human Alzheimer’s disease presenilin2. Dev. Genes Evol. 212, 486–490. doi: 10.1007/s00427-002-0269-5
Guan, Z. Z. (2008). Cross-talk between oxidative stress and modifications of cholinergic and glutaminergic receptors in the pathogenesis of Alzheimer’s disease. Acta Pharmacol. Sin. 29, 773–780. doi: 10.1111/j.1745-7254.2008.00819.x
Guo, S. (2009). Using zebrafish to assess the impact of drugs on neural development and function. Expert Opin. Drug Discov. 4, 715–726. doi: 10.1517/17460440902988464
Gupta, T., Marquart, G. D., Horstick, E. J., Tabor, K. M., Pajevic, S., and Burgess, H. A. (2018). Morphometric analysis and neuroanatomical mapping of the zebrafish brain. Methods 150, 49–62. doi: 10.1016/j.ymeth.2018.06.008
Guzior, N., Wieckowska, A., Panek, D., and Malawska, B. (2014). Recent development of multifunctional agents as potential drug candidates for the treatment of Alzheimer’s disease. Curr. Med. Chem. 22, 373–404. doi: 10.2174/0929867321666141106122628
Haghani, S., Karia, M., Cheng, R. K., and Mathuru, A. S. (2019). An automated assay system to study novel tank induced anxiety. Front. Behav. Neurosci. 13:180. doi: 10.3389/fnbeh.2019.00180
Hampel, H., Hardy, J., Blennow, K., Chen, C., Perry, G., Kim, S. H., et al. (2021). The amyloid-β pathway in Alzheimer’s disease. Mol. Psychiatry 26, 5481–5503. doi: 10.1038/s41380-021-01249-0
Hodges, K. (2021). Anxiolytic Effects of Fluoxetine and Anxiogenic Effects of D-Amphetamine on Associative and Non-Associative Memory. Dissertation. Macomb, IL: Western Illinois University.
Horzmann, K. A., and Freeman, J. L. (2016). Zebrafish get connected: investigating neurotransmission targets and alterations in chemical toxicity. Toxics 4:19. doi: 10.3390/TOXICS4030019
Hu, N. W., Ondrejcak, T., and Rowan, M. J. (2012). Glutamate receptors in preclinical research on Alzheimer’s disease: update on recent advances. Pharmacol. Biochem. Behav. 100, 855–862. doi: 10.1016/J.PBB.2011.04.013
Hu, Y.-H., Yang, J., Zhang, Y., Liu, K.-C., Liu, T., Sun, J., et al. (2019). Synthesis and biological evaluation of 3–(4-aminophenyl)-coumarin derivatives as potential anti-Alzheimer’s disease agents. J. Enzyme Inhib. Med. Chem. 34, 1083–1092. doi: 10.1080/14756366.2019.1615484
Huang, Y. Y., and Neuhauss, S. C. F. (2008). The optokinetic response in zebrafish and its applications. Front. Biosci. 13:1899–1916. doi: 10.2741/2810
Huang, Y., and Mahley, R. W. (2014). Apolipoprotein E: structure and function in lipid metabolism, neurobiology, and Alzheimer’s diseases. Neurobiol. Dis. 72(Pt. A), 3–12. doi: 10.1016/j.nbd.2014.08.025
Hussain, I., Powell, D., Howlett, D. R., Tew, D. G., Meek, T. D., Chapman, C., et al. (1999). Identification of a novel aspartic protease (Asp 2) as beta-secretase. Mol. Cell. Neurosci. 14, 419–427. doi: 10.1006/mcne.1999.0811
Hyman, B. T., van Hoesen, G. W., Damasio, A. R., and Barnes, C. L. (1984). Alzheimer’s disease: cell-specific pathology isolates the hippocampal formation. Science 225, 1168–1170. doi: 10.1126/science.6474172
Jäkel, L., de Kort, A. M., Klijn, C. J. M., Schreuder, F. H. B. M., and Verbeek, M. M. (2021). Prevalence of cerebral amyloid angiopathy: a systematic review and meta-analysis. Alzheimers Dement. 18, 10–28. doi: 10.1002/ALZ.12366
Javed, I., Peng, G., Xing, Y., Yu, T., Zhao, M., Kakinen, A., et al. (2019). Inhibition of amyloid beta toxicity in zebrafish with a chaperone-gold nanoparticle dual strategy. Nat. Commun. 10:3780. doi: 10.1038/s41467-019-11762-0
Karaduman, A., Karoglu-Eravsar, E. T., Kaya, U., Aydin, A., Adams, M. M., and Kafaligonul, H. (2021). The optomotor response of aging zebrafish reveals a complex relationship between visual motion characteristics and cholinergic system. Neurobiol. Aging 98, 21–32. doi: 10.1016/J.NEUROBIOLAGING.2020.10.018
Karunakaran, K. B., Thiyagaraj, A., and Santhakumar, K. (2022). Novel insights on acetylcholinesterase inhibition by Convolvulus pluricaulis, scopolamine and their combination in zebrafish. Nat. Prod. Bioprospect. 12:6. doi: 10.1007/s13659-022-00332-5
Kaur, S., DasGupta, G., and Singh, S. (2019). Altered neurochemistry in Alzheimer’s disease: targeting neurotransmitter receptor mechanisms and therapeutic strategy. Neurophysiology 51, 293–309. doi: 10.1007/s11062-019-09823-7
Kim, Y. H., Lee, Y., Kim, D., Jung, M. W., and Lee, C. J. (2010). Scopolamine-induced learning impairment reversed by physostigmine in zebrafish. Neurosci. Res. 67, 156–161. doi: 10.1016/j.neures.2010.03.003
Kimberly, W. T., LaVoie, M. J., Ostaszewski, B. L., Ye, W., Wolfe, M. S., and Selkoe, D. J. (2003). Gamma-secretase is a membrane protein complex comprised of presenilin, nicastrin, Aph-1, and Pen-2. Proc. Natl. Acad. Sci. U.S.A. 100, 6382–6387. doi: 10.1073/pnas.1037392100
Kiper, K., and Freeman, J. L. (2021). Use of zebrafish genetic models to study etiology of the amyloid-beta and neurofibrillary tangle pathways in Alzheimer’s disease. Curr. Neuropharmacol. 20, 524–539. doi: 10.2174/1570159X19666210524155944
Kockx, M., Traini, M., and Kritharides, L. (2018). Cell-specific production, secretion, and function of apolipoprotein E. J. Mol. Med. (Berlin, Germany) 96, 361–371. doi: 10.1007/s00109-018-1632-y
Koehler, D., Shah, Z. A., and Williams, F. E. (2019). The GSK3β inhibitor, TDZD-8, rescues cognition in a zebrafish model of okadaic acid-induced Alzheimer’s disease. Neurochem. Int. 122, 31–37. doi: 10.1016/j.neuint.2018.10.022
Koehler, D., Shah, Z. A., Hensley, K., and Williams, F. E. (2018). Lanthionine ketimine-5-ethyl ester provides neuroprotection in a zebrafish model of okadaic acid-induced Alzheimer’s disease. Neurochem. Int. 115, 61–68. doi: 10.1016/j.neuint.2018.02.002
Kordower, J. H., Chu, Y., Stebbins, G. T., DeKosky, S. T., Cochran, E. J., Bennett, D., et al. (2001). Loss and atrophy of layer II entorhinal cortex neurons in elderly people with mild cognitive impairment. Ann. Neurol. 49, 202–213.
Koster, R., and Sassen, W. A. (2015). A molecular toolbox for genetic manipulation of zebrafish. Adv. Genomics Genet. 5, 151–163. doi: 10.2147/agg.s57585
Kowal, R. C., Herz, J., Goldstein, J. L., Esser, V., and Brown, M. S. (1989). Low density lipoprotein receptor-related protein mediates uptake of cholesteryl esters derived from apoprotein E-enriched lipoproteins. Proc. Natl. Acad. Sci. U.S.A. 86, 5810–5814. doi: 10.1073/pnas.86.15.5810
Kozol, R. A., Abrams, A. J., James, D. M., Buglo, E., Yan, Q., and Dallman, J. E. (2016). Function over form: modeling groups of inherited neurological conditions in zebrafish. Front. Mol. Neurosci. 9:55. doi: 10.3389/fnmol.2016.00055
Kraeuter, A. K., Guest, P. C., and Sarnyai, Z. (2019). The Y-maze for assessment of spatial working and reference memory in mice. Methods Mol. Biol. 1916, 105–111. doi: 10.1007/978-1-4939-8994-2_10
Kuiper, M. A., Teerlink, T., Visser, J. J., Bergmans, P. L. M., Scheltens, P., and Wolters, E. C. (2000). L-Glutamate, L-arginine and L-citrulline levels in cerebrospinal fluid of Parkinson’s disease, multiple system atrophy, and Alzheimer’s disease patients. J. Neural Transm. 107, 183–189. doi: 10.1007/s007020050016
Lanctôt, K. L., Herrmaan, N., Mazzotta, P., Khan, L. R., and Ingber, N. (2004). GABAergic function in Alzheimer’s disease: evidence for dysfunction and potential as a therapeutic target for the treatment of behavioural and psychological symptoms of dementia. Can. J. Psychiatry Rev. Can. Psychiatrie 49, 439–453. doi: 10.1177/070674370404900705
Langova, V., Vales, K., Horka, P., and Horacek, J. (2020). The role of zebrafish and laboratory rodents in schizophrenia research. Front. Psychiatry 11:703. doi: 10.3389/fpsyt.2020.00703
Leimer, U., Lun, K., Romig, H., Walter, J., Grünberg, J., Brand, M., et al. (1999). Zebrafish (Danio rerio) presenilin promotes aberrant amyloid β-peptide production and requires a critical aspartate residue for its function in amyloidogenesis. Biochemistry 38, 13602–13609. doi: 10.1021/bi991453n
Li, C., Briner, A., and Götz, J. (2019). The search for improved animal models of Alzheimer’s disease and novel strategies for therapeutic intervention. Future Med. Chem. 11, 1853–1857. doi: 10.4155/fmc-2019-0150
Li, F., and Tsien, J. Z. (2009). Memory and the NMDA receptors. N. Engl. J. Med. 361, 302–303. doi: 10.1056/nejmcibr0902052
Li, Y. Q., Chen, Y., Fang, J. Y., Jiang, S. Q., Li, P., and Li, F. (2020). Integrated network pharmacology and zebrafish model to investigate dual-effects components of Cistanche tubulosa for treating both Osteoporosis and Alzheimer’s Disease. J. Ethnopharmacol. 254:112764. doi: 10.1016/j.jep.2020.112764
Lim, A., Moussavi Nik, S. H., Ebrahimie, E., and Lardelli, M. (2015). Analysis of nicastrin gene phylogeny and expression in zebrafish. Dev. Genes Evol. 225, 171–178. doi: 10.1007/s00427-015-0500-9
Lin, X., Koelsch, G., Wu, S., Downs, D., Dashti, A., and Tang, J. (2000). Human aspartic protease memapsin 2 cleaves the beta-secretase site of beta-amyloid precursor protein. Proc. Natl. Acad. Sci. U.S.A. 97, 1456–1460. doi: 10.1073/pnas.97.4.1456
Liu, X., Lin, J., Zhang, Y., Peng, X., Guo, N., and Li, Q. (2016). Effects of diphenylhydantoin on locomotion and thigmotaxis of larval zebrafish. Neurotoxicol. Teratol. 53, 41–47. doi: 10.1016/J.NTT.2015.11.008
Lopez, O. L., Wisnieski, S. R., Becker, J. T., Boiler, F., and DeKosky, S. T. (1997). Extrapyramidal signs in patients with probable Alzheimer disease. Arch. Neurol. 54, 969–975. doi: 10.1001/archneur.1997.00550200033007
Lorent, K., Yeo, S. Y., Oda, T., Chandrasekharappa, S., Chitnis, A., Matthews, R. P., et al. (2004). Inhibition of Jagged-mediated Notch signaling disrupts zebrafish biliary development and generates multi-organ defects compatible with an Alagille syndrome phenocopy. Development (Cambridge, England) 131, 5753–5766. doi: 10.1242/DEV.01411
Lu, P., Bai, X.-C., Ma, D., Xie, T., Yan, C., Sun, L., et al. (2014). Three-dimensional structure of human γ-secretase. Nature 512, 166–170. doi: 10.1038/nature13567
Mans, R. A., Hinton, K. D., Payne, C. H., Powers, G. E., Scheuermann, N. L., and Saint-Jean, M. (2019). Cholinergic stimulation of the adult zebrafish brain induces phosphorylation of glycogen synthase kinase-3β and extracellular signal-regulated kinase in the telencephalon. Front. Mol. Neurosci. 12:91. doi: 10.3389/fnmol.2019.00091
Manuel, R., Gorissen, M., Piza Roca, C., Zethof, J., van de Vis, H., Flik, G., et al. (2014). Inhibitory avoidance learning in zebrafish (Danio rerio): effects of shock intensity and unraveling differences in task performance. Zebrafish 11, 341–352. doi: 10.1089/zeb.2013.0970
Maragakis, N. J., and Rothstein, J. D. (2004). Glutamate transporters: animal models to neurologic disease. Neurobiol. Dis. 15, 461–473. doi: 10.1016/j.nbd.2003.12.007
Martin, S. C., Heinrich, G., and Sandell, J. H. (1998). Sequence and expression of glutamic acid decarboxylase isoforms in the developing zebrafish. J. Comp. Neurol. 396, 253–266.
Martorana, A., and Koch, G. (2014). Is dopamine involved in Alzheimer’s disease. Front. Aging Neurosci. 6:252. doi: 10.3389/fnagi.2014.00252
Maximino, C. (2021). Decynium-22 affects behavior in the zebrafish light/dark test. bioRxiv [preprint] doi: 10.1101/2021.01.14.426728
Mayeux, R., and Stern, Y. (2012). Epidemiology of Alzheimer disease. Cold Spring Harb. Perspect. Med. 2, 137–152.
Medina, L., Abellán, A., Vicario, A., and Desfilis, E. (2014). Evolutionary and developmental contributions for understanding the organization of the Basal Ganglia. Brain Behav. Evol. 83, 112–125. doi: 10.1159/000357832
Mega, M. S., Cummings, J. L., Fiorello, T., and Gornbein, J. (1996). The spectrum of behavioral changes in Alzheimer’s disease. Neurology 46, 130–135. doi: 10.1212/WNL.46.1.130
Michael Stewart, A., and Kalueff, A. v. (2012). The developing utility of zebrafish models for cognitive enhancers research. Curr. Neuropharmacol. 10, 263–271. doi: 10.2174/157015912803217323
Moens, C. B., and Prince, V. E. (2002). Constructing the hindbrain: insights from the zebrafish. Dev. Dyn. 224, 1–17. doi: 10.1002/dvdy.10086
Moloney, C. M., Lowe, V. J., and Murray, M. E. (2021). Visualization of neurofibrillary tangle maturity in Alzheimer’s disease: a clinicopathologic perspective for biomarker research. Alzheimers Dement. 17, 1554–1574. doi: 10.1002/ALZ.12321
Moreira, A. L. P., and Luchiari, A. C. (2022). Effects of oxybenzone on zebrafish behavior and cognition. Sci. Total Environ. 808:152101. doi: 10.1016/J.SCITOTENV.2021.152101
Moussavi Nik, S. H., Wilson, L., Newman, M., Croft, K., Mori, T. A., Musgrave, I., et al. (2012). The BACE1-PSEN-AβPP regulatory axis has an ancient role in response to low oxygen/oxidative stress. J. Alzheimers Dis. 28, 515–530. doi: 10.3233/JAD-2011-110533
Mueller, T., Vernier, P., and Wullimann, M. F. (2004). The adult central nervous cholinergic system of a neurogenetic model animal, the zebrafish Danio rerio. Brain Res. 1011, 156–169. doi: 10.1016/J.BRAINRES.2004.02.073
Musa, A., Lehrach, H., and Russo, V. E. A. (2001). Distinct expression patterns of two zebrafish homologues of the human APP gene during embryonic development. Dev. Genes Evol. 211, 563–567. doi: 10.1007/s00427-001-0189-9
Muto, A., and Kawakami, K. (2013). Prey capture in zebrafish larvae serves as a model to study cognitive functions. Front. Neural Circuits 7:110. doi: 10.3389/fncir.2013.00110
Nabinger, D. D., Altenhofen, S., Peixoto, J. V., da Silva, J. M. K., and Bonan, C. D. (2021). Long-lasting behavioral effects of quinpirole exposure on zebrafish. Neurotoxicol. Teratol. 88:107034. doi: 10.1016/J.NTT.2021.107034
Nam, R. H., Kim, W., and Lee, C. J. (2004). NMDA receptor-dependent long-term potentiation in the telencephalon of the zebrafish. Neurosci. Lett. 370, 248–251. doi: 10.1016/j.neulet.2004.08.037
Nery, L. R., Eltz, N. S., Hackman, C., Fonseca, R., Altenhofen, S., Guerra, H. N., et al. (2014). Brain intraventricular injection of amyloid-β in zebrafish embryo impairs cognition and increases tau phosphorylation, effects reversed by lithium. PLoS One 9:e105862. doi: 10.1371/journal.pone.0105862
Nery, L. R., Silva, N. E., Fonseca, R., and Vianna, M. R. M. (2017). Presenilin-1 targeted morpholino induces cognitive deficits, increased brain Aβ1-42 and decreased synaptic marker PSD-95 in zebrafish larvae. Neurochem. Res. 42, 2959–2967. doi: 10.1007/S11064-017-2327-4
Newman, M., Ebrahimie, E., and Lardelli, M. (2014). Using the zebrafish model for Alzheimer’s disease research. Front. Genet. 5:189. doi: 10.3389/fgene.2014.00189
Newman, M., Verdile, G., Martins, R. N., and Lardelli, M. (2011). Zebrafish as a tool in Alzheimer’s disease research. Biochim. Biophys. Acta (BBA) Mol. Basis Dis. 1812, 346–352. doi: 10.1016/j.bbadis.2010.09.012
Norton, W., and Bally-Cuif, L. (2010). Adult zebrafish as a model organism for behavioural genetics. BMC Neurosci. 11:90. doi: 10.1186/1471-2202-11-90
O’Brien, R. J., and Wong, P. C. (2011). Amyloid precursor protein processing and Alzheimer’s disease. Annu. Rev. Neurosci. 34, 185–204. doi: 10.1146/annurev-neuro-061010-113613
Okada, H., Zhang, W., Peterhoff, C., Hwang, J. C., Nixon, R. A., Ryu, S. H., et al. (2010). Proteomic identification of sorting nexin 6 as a negative regulator of BACE1-mediated APP processing. FASEB J. 24, 2783–2794. doi: 10.1096/fj.09-146357
Pan, H., Zhang, J., Wang, Y., Cui, K., Cao, Y., Wang, L., et al. (2019). Linarin improves the dyskinesia recovery in Alzheimer’s disease zebrafish by inhibiting the acetylcholinesterase activity. Life Sci. 222, 112–116. doi: 10.1016/j.lfs.2019.02.046
Panula, P., Chen, Y. C., Priyadarshini, M., Kudo, H., Semenova, S., Sundvik, M., et al. (2010). The comparative neuroanatomy and neurochemistry of zebrafish CNS systems of relevance to human neuropsychiatric diseases. Neurobiol. Dis. 40, 46–57. doi: 10.1016/J.NBD.2010.05.010
Panula, P., Sallinen, V., Sundvik, M., Kolehmainen, J., Torkko, V., Tiittula, A., et al. (2006). Modulatory neurotransmitter systems and behavior: towards zebrafish models of neurodegenerative diseases. Zebrafish 3, 235–247. doi: 10.1089/zeb.2006.3.235
Pelkowski, S. D., Kapoor, M., Richendrfer, H. A., Wang, X., Colwill, R. M., and Creton, R. (2011). A novel high-throughput imaging system for automated analyses of avoidance behavior in zebrafish larvae. Behav. Brain Res. 223, 135–144. doi: 10.1016/j.bbr.2011.04.033
Piaceri, I., Nacmias, B., and Sorbi, S. (2013). Genetics of familial and sporadic Alzheimer’s disease. Front. Biosci. 5:167–177. doi: 10.2741/e605
Pinessi, L., Rainero, I., de Gennaro, T., Gentile, S., Portaleone, P., and Bergamasco, B. (1987). Biogenic amines in cerebrospinal fluid and plasma of patients with dementia of Alzheimer type. Funct. Neurol. 2, 51–58.
Png, W. Y., Mok, S. Y., Tang, P. Y., Ogawa, S., and Parhar, I. (2021). “Morphine-induced cognitive dysfunction model in zebrafish,” in Proceedings of the 2020 IEEE EMBS Conference on Biomedical Engineering and Sciences, IECBES 2020 (Piscataway, NJ: IEEE), 68–71. doi: 10.1109/IECBES48179.2021.9398834
Price, J. L., Ko, A. I., Wade, M. J., Tsou, S. K., McKeel, D. W., and Morris, J. C. (2001). Neuron number in the entorhinal cortex and CA1 in preclinical Alzheimer disease. Arch. Neurol. 58:1395. doi: 10.1001/archneur.58.9.1395
Pusceddu, M. M., Hernandez-Baixauli, J., Puiggrós, F., Arola, L., Caimari, A., del Bas, J. M., et al. (2022). Mediterranean natural extracts improved cognitive behavior in zebrafish and healthy rats and ameliorated lps-induced cognitive impairment in a sex dependent manner. Behav. Brain Funct. 18:5. doi: 10.1186/s12993-022-00190-8
Reinhardt, L., Kordes, S., Reinhardt, P., Glatza, M., Baumann, M., Drexler, H. C. A., et al. (2019). Dual inhibition of GSK3β and CDK5 protects the cytoskeleton of neurons from neuroinflammatory-mediated degeneration in vitro and in vivo. Stem Cell Rep. 12, 502–517. doi: 10.1016/j.stemcr.2019.01.015
Richetti, S. K., Blank, M., Capiotti, K. M., Piato, A. L., Bogo, M. R., Vianna, M. R., et al. (2011). Quercetin and rutin prevent scopolamine-induced memory impairment in zebrafish. Behav. Brain Res. 217, 10–15. doi: 10.1016/j.bbr.2010.09.027
Rico, E. P., de Oliveira, D. L., Rosemberg, D. B., Mussulini, B. H., Bonan, C. D., Dias, R. D., et al. (2010). Expression and functional analysis of Na+-dependent glutamate transporters from zebrafish brain. Brain Res. Bull. 81, 517–523. doi: 10.1016/j.brainresbull.2009.11.011
Rissman, R. A., de Blas, A. L., and Armstrong, D. M. (2007). GABA(A) receptors in aging and Alzheimer’s disease. J. Neurochem. 103, 1285–1292. doi: 10.1111/J.1471-4159.2007.04832.X
Rodríguez, F., Durán, E., Gómez, A., Ocaña, F. M., Álvarez, E., Jiménez-Moya, F., et al. (2005). Cognitive and emotional functions of the teleost fish cerebellum. Brain Res. Bull. 66, 365–370. doi: 10.1016/j.brainresbull.2004.11.026
Rodríguez, F., Quintero, B., Amores, L., Madrid, D., Salas-Peña, C., and Salas, C. (2021). Spatial cognition in teleost fish: strategies and mechanisms. Animals 11:2271. doi: 10.3390/ani11082271
Ruhl, T., Jonas, A., Seidel, N. I., Prinz, N., Albayram, O., Bilkei-Gorzo, A., et al. (2015). Oxidation and cognitive impairment in the aging zebrafish. Gerontology 62, 47–57. doi: 10.1159/000433534
Sadamitsu, K., Shigemitsu, L., Suzuki, M., Ito, D., Kashima, M., and Hirata, H. (2021). Characterization of zebrafish GABAA receptor subunits. Sci. Rep. 11, 1–11. doi: 10.1038/s41598-021-84646-3
Sajjad, R., Arif, R., Shah, A. A., Manzoor, I., and Mustafa, G. (2018). Pathogenesis of Alzheimer’s disease: role of amyloid-beta and hyperphosphorylated tau protein. Indian J. Pharm. Sci. 80, 581–591. doi: 10.4172/PHARMACEUTICAL-SCIENCES.1000397
Saleem, S., and Kannan, R. R. (2018). Zebrafish: an emerging real-time model system to study Alzheimer’s disease and neurospecific drug discovery. Cell Death Discov. 4, 1–13. doi: 10.1038/s41420-018-0109-7
Sandberg, A., Ling, H., Gearing, M., Dombroski, B., Cantwell, L., R’Bibo, L., et al. (2020). Fibrillation and molecular characteristics are coherent with clinical and pathological features of 4-repeat tauopathy caused by MAPT variant G273R. Neurobiol. Dis. 146:105079. doi: 10.1016/j.nbd.2020.105079
Sang, Z., Wang, K., Han, X., Cao, M., Tan, Z., and Liu, W. (2019). Design, synthesis, and evaluation of novel ferulic acid derivatives as multi-target-directed ligands for the treatment of Alzheimer’s disease. ACS Chem. Neurosci. 10, 1008–1024. doi: 10.1021/acschemneuro.8b00530
Sang, Z., Wang, K., Shi, J., Cheng, X., Zhu, G., Wei, R., et al. (2020). Apigenin-rivastigmine hybrids as multi-target-directed liagnds for the treatment of Alzheimer’s disease. Eur. J. Med. Chem. 187:111958. doi: 10.1016/j.ejmech.2019.111958
Santana, S., Rico, E. P., and Burgos, J. S. (2012). Can zebrafish be used as animal model to study Alzheimer’s disease? Am. J. Neurodegener. Dis. 1:32.
Schmidt, R., Strähle, U., and Scholpp, S. (2013). Neurogenesis in zebrafish – from embryo to adult. Neural Dev. 8:3. doi: 10.1186/1749-8104-8-3
Schnörr, S. J., Steenbergen, P. J., Richardson, M. K., and Champagne, D. L. (2012). Measuring thigmotaxis in larval zebrafish. Behav. Brain Res. 228, 367–374. doi: 10.1016/J.BBR.2011.12.016
Sehayek, E., and Eisenberg, S. (1991). Mechanisms of inhibition by apolipoprotein C of apolipoprotein E-dependent cellular metabolism of human triglyceride-rich lipoproteins through the low density lipoprotein receptor pathway. J. Biol. Chem. 266, 18259–18267.
Serda, M. (2013). Synteza i Aktywność Biologiczna Nowych Analogów Tiosemikarbazonowych Chelatorów Żelaza. Ph.D. thesis. Katowice: Uniwersytet Śląski, 343–354. doi: 10.2/JQUERY.MIN.JS
Shams, S., Rihel, J., Ortiz, J. G., and Gerlai, R. (2018). The zebrafish as a promising tool for modeling human brain disorders: a review based upon an IBNS Symposium. Neurosci. Biobehav. Rev. 85, 176–190. doi: 10.1016/j.neubiorev.2017.09.002
Simon, P., Dupuis, R., and Costentin, J. (1994). Thigmotaxis as an index of anxiety in mice. Influence of dopaminergic transmissions. Behav. Brain Res. 61, 59–64.
Singsai, K., Ladpala, N., Dangja, N., Boonchuen, T., Jaikhamfu, N., and Fakthong, P. (2021). Effect of Streblus asper leaf extract on scopolamine-induced memory deficits in zebrafish: the model of Alzheimer’s disease. Adv. Pharmacol. Pharm. Sci. 2021:6666726. doi: 10.1155/2021/6666726
Soto-Ospina, A., Araque Marín, P., Bedoya, G., de, J., and Villegas Lanau, A. (2021). Structural predictive model of presenilin-2 protein and analysis of structural effects of familial Alzheimer’s disease mutations. Biochem. Res. Int. 2021:9542038. doi: 10.1155/2021/9542038
Staley, K. J., Soldo, B. L., and Proctor, W. R. (1995). Ionic mechanisms of neuronal excitation by inhibitory GABAA receptors. Science (New York, N.Y.) 269, 977–981. doi: 10.1126/SCIENCE.7638623
Stewart, A. M., Gaikwad, S., Kyzar, E., and Kalueff, A. v. (2012). Understanding spatio-temporal strategies of adult zebrafish exploration in the open field test. Brain Res. 1451, 44–52. doi: 10.1016/j.brainres.2012.02.064
Stewart, A. M., Ullmann, J. F. P., Norton, W. H. J., Parker, M. O., Brennan, C. H., Gerlai, R., et al. (2015). Molecular psychiatry of zebrafish. Mol. Psychiatry 20, 2–17. doi: 10.1038/MP.2014.128
Stewart, A., Maximino, C., Marques de Brito, T., Herculano, A. M., Gouveia, A., Morato, S., et al. (2011). “Neurophenotyping of adult zebrafish using the light/dark box paradigm,” in Zebrafish Neurobehavioral Protocols. Neuromethods, Vol. 51, eds A. Kalueff and J. Cachat (Totowa, NJ: Humana Press), 157–167. doi: 10.1007/978-1-60761-953-6_13
Stratmann, K., Heinsen, H., Korf, H.-W., del Turco, D., Ghebremedhin, E., Seidel, K., et al. (2016). Precortical phase of Alzheimer’s disease (AD)-related tau cytoskeletal pathology. Brain Pathol. 26, 371–386. doi: 10.1111/bpa.12289
Thanvi, B., and Robinson, T. (2006). Sporadic cerebral amyloid angiopathy—an important cause of cerebral haemorrhage in older people. Age Ageing 35, 565–571. doi: 10.1093/ageing/afl108
Thawkar, B. S., and Kaur, G. (2021). Zebrafish as a promising tool for modeling neurotoxin-induced Alzheimer’s disease. Neurotox. Res. 39, 949–965. doi: 10.1007/s12640-021-00343-z
Tierney, K. B. (2011). Behavioural assessments of neurotoxic effects and neurodegeneration in zebrafish. Biochim. Biophys. Acta (BBA) Mol. Basis Dis. 1812, 381–389. doi: 10.1016/J.BBADIS.2010.10.011
Tohgi, H., Abe, T., Hashiguchi, K., Saheki, M., and Takahashi, S. (1994). Remarkable reduction in acetylcholine concentration in the cerebrospinal fluid from patients with Alzheimer type dementia. Neurosci. Lett. 177, 139–142. doi: 10.1016/0304-3940(94)90064-7
Troconis, E. L., Ordoobadi, A. J., Sommers, T. F., Aziz-Bose, R., Carter, A. R., and Trapani, J. G. (2017). Intensity-dependent timing and precision of startle response latency in larval zebrafish. J. Physiol. 595, 265–282. doi: 10.1113/JP272466
Tu, H., Nelson, O., Bezprozvanny, A., Wang, Z., Lee, S. F., Hao, Y. H., et al. (2006). Presenilins form ER Ca2+ leak channels, a function disrupted by familial Alzheimer’s disease-linked mutations. Cell 126, 981–993. doi: 10.1016/J.CELL.2006.06.059
Tucker Edmister, S., Ibrahim, R., Kakodkar, R., Kreiling, J. A., and Creton, R. (2022). A zebrafish model for calcineurin-dependent brain function. Behav. Brain Res. 416:113544. doi: 10.1016/j.bbr.2021.113544
Valu, M.-V., Ducu, C., Moga, S., Negrea, D., Hritcu, L., Boiangiu, R. S., et al. (2021a). Effects of the hydroethanolic extract of Lycopodium selago L. on scopolamine-induced memory deficits in zebrafish. Pharmaceuticals 14:568. doi: 10.3390/ph14060568
Valu, M.-V., Soare, L. C., Ducu, C., Moga, S., Negrea, D., Vamanu, E., et al. (2021b). Hericium erinaceus (Bull.) Pers. ethanolic extract with antioxidant properties on scopolamine-induced memory deficits in a zebrafish model of cognitive impairment. J. Fungi 7:477. doi: 10.3390/jof7060477
van Bebber, F., Hruscha, A., Willem, M., Schmid, B., and Haass, C. (2013). Loss of Bace2 in zebrafish affects melanocyte migration and is distinct from Bace1 knock out phenotypes. J. Neurochem. 127, 471–481. doi: 10.1111/jnc.12198
van der Staay, F. J., Gieling, E. T., Pinzón, N. E., Nordquist, R. E., and Ohl, F. (2012). The appetitively motivated “cognitive” holeboard: a family of complex spatial discrimination tasks for assessing learning and memory. Neurosci. Biobehav. Rev. 36, 379–403. doi: 10.1016/j.neubiorev.2011.07.008
Vargas, J. P., López, J. C., and Portavella, M. (2009). What are the functions of fish brain Pallium? Brain Res. Bull. 79, 436–440. doi: 10.1016/j.brainresbull.2009.05.008
Vaz, R., Hofmeister, W., and Lindstrand, A. (2019). Zebrafish models of neurodevelopmental disorders: limitations and benefits of current tools and techniques. Int. J. Mol. Sci. 20:1296. doi: 10.3390/ijms20061296
Vitek, M. P., Araujo, J. A., Fossel, M., Greenberg, B. D., Howell, G. R., Rizzo, S. J. S., et al. (2020). Translational animal models for Alzheimer’s disease: an Alzheimer’s association business consortium think tank. Alzheimers Dement. Transl. Res. Clin. Interv. 6:e12114. doi: 10.1002/trc2.12114
Voisin, T., and Vellas, B. (2009). Diagnosis and treatment of patients with severe Alzheimer’s disease. Drugs Aging 26, 135–144. doi: 10.2165/0002512-200926020-00005
Volicer, L. (1985). Serotoninergic system in dementia of the Alzheimer type. Arch. Neurol. 42:1158. doi: 10.1001/archneur.1985.04060110040013
Wang, K., Shi, J., Zhou, Y., He, Y., Mi, J., Yang, J., et al. (2021). Design, synthesis and evaluation of cinnamic acid hybrids as multi-target-directed agents for the treatment of Alzheimer’s disease. Bioorg. Chem. 112:104879. doi: 10.1016/j.bioorg.2021.104879
Wang, X., Michaelis, M. L., and Michaelis, E. K. (2010). Functional genomics of brain aging and Alzheimers disease: focus on selective neuronal vulnerability. Curr. Genomics 11, 618–633. doi: 10.2174/138920210793360943
Wasel, O., and Freeman, J. L. (2020). Chemical and genetic zebrafish models to define mechanisms of and treatments for dopaminergic neurodegeneration. Int. J. Mol. Sci. 21:5981. doi: 10.3390/ijms21175981
Williams, F. E., and Messer, W. S. Jr. (2004). Muscarinic acetylcholine receptors in the brain of the zebrafish (Danio rerio) measured by radioligand binding techniques. Comp. Biochem. Physiol. C Toxicol. Pharmacol. 137, 349–353. doi: 10.1016/j.cca.2004.03.002
World Health Organization (2021). Dementia [Online]. Available online at: https://www.who.int/news-room/fact-sheets/detail/dementia (accessed April 23, 2022).
Wullimann, M. F., Rupp, B., and Reichert, H. (1996). Neuroanatomy of the Zebrafish Brain. Berlin: Springer. doi: 10.1007/978-3-0348-8979-7
Xia, W. (2010). Exploring Alzheimer’s disease in zebrafish. J. Alzheimers Dis. 20, 981–990. doi: 10.3233/JAD-2010-1412
Yang, J., Zhang, P., Hu, Y., Liu, T., Sun, J., and Wang, X. (2019). Synthesis and biological evaluation of 3-arylcoumarins as potential anti-Alzheimer’s disease agents. J. Enzyme Inhib. Med. Chem. 34, 651–656. doi: 10.1080/14756366.2019.1574297
Yoshida, H., and Goedert, M. (2012). Phosphorylation of microtubule-associated protein tau by AMPK-related kinases. J. Neurochem. 120, 165–176. doi: 10.1111/j.1471-4159.2011.07523.x
Yu, G., Nishimura, M., Arawaka, S., Levitan, D., Zhang, L., Tandon, A., et al. (2000). Nicastrin modulates presenilin-mediated notch/glp-1 signal transduction and betaAPP processing. Nature 407, 48–54. doi: 10.1038/35024009
Zanandrea, R., Abreu, M. S., Piato, A., Barcellos, L. J. G., and Giacomini, A. C. V. V. (2018). Lithium prevents scopolamine-induced memory impairment in zebrafish. Neurosci. Lett. 664, 34–37. doi: 10.1016/j.neulet.2017.11.010
Zebrafish UCL (2022). Midbrain — Zebrafish UCL. Available online at: http://zebrafishucl.org/midbrain (accessed April, 2022).
Zheng, Q., Bi, R., Xu, M., Zhang, D. F., Tan, L. W., Lu, Y. P., et al. (2021). Exploring the Genetic Association of the ABAT Gene with Alzheimer’s Disease. Mol. Neurobiol. 58, 1894–1903. doi: 10.1007/S12035-020-02271-Z
Zhou, R., Yang, G., Guo, X., Zhou, Q., Lei, J., and Shi, Y. (2019). Recognition of the amyloid precursor protein by human γ-secretase. Science (New York, N.Y.) 363:eaaw0930. doi: 10.1126/science.aaw0930
Keywords: zebrafish, Alzheimer’s disease, behavior, glutamatergic, cholinergic
Citation: Shenoy A, Banerjee M, Upadhya A, Bagwe-Parab S and Kaur G (2022) The Brilliance of the Zebrafish Model: Perception on Behavior and Alzheimer’s Disease. Front. Behav. Neurosci. 16:861155. doi: 10.3389/fnbeh.2022.861155
Received: 24 January 2022; Accepted: 21 April 2022;
Published: 13 June 2022.
Edited by:
Adebobola Imeh-Nathaniel, North Greenville University, United StatesReviewed by:
Robert Knepp, North Greenville University, United StatesAyodeji O. Ipinmoroti, Alabama State University, United States
Copyright © 2022 Shenoy, Banerjee, Upadhya, Bagwe-Parab and Kaur. This is an open-access article distributed under the terms of the Creative Commons Attribution License (CC BY). The use, distribution or reproduction in other forums is permitted, provided the original author(s) and the copyright owner(s) are credited and that the original publication in this journal is cited, in accordance with accepted academic practice. No use, distribution or reproduction is permitted which does not comply with these terms.
*Correspondence: Ginpreet Kaur, Z2lucHJlZXQuYW5lamFAZ21haWwuY29t