- 1Graduate School of Informatics and Engineering, The University of Electro-Communications, Chofu, Japan
- 2Center for Education in Liberal Arts and Sciences, Osaka University, Osaka, Japan
- 3Graduate School of Sports and Health Science, Fukuoka University, Fukuoka, Japan
- 4Physical Fitness Research Institute, Meiji Yasuda Life Foundation of Health and Welfare, Shinjuku City, Japan
- 5Extreme Environments Laboratory, School of Sport, Health & Exercise Science, University of Portsmouth, Portsmouth, United Kingdom
- 6Faculty of Sports and Health Science, Fukuoka University, Fukuoka, Japan
The role of peripheral biomarkers following acute physical exercise on cognitive improvement has not been systematically evaluated. This study aimed to explore the role of peripheral circulating biomarkers in executive performance following acute aerobic and resistance exercise. Nineteen healthy males completed a central executive (Go/No-Go) task before and after 30-min of perceived intensity matched aerobic and resistance exercise. In the aerobic condition, the participants cycled an ergometer at 40% peak oxygen uptake. In the resistance condition, they performed resistance exercise using elastic bands. Before and after an acute bout of physical exercise, venous samples were collected for the assessment of following biomarkers: adrenaline, noradrenaline, glucose, lactate, cortisol, insulin-like growth hormone factor 1, and brain-derived neurotrophic factor. Reaction time decreased following both aerobic exercise and resistance exercise (p = 0.04). Repeated measures correlation analysis indicated that changes in reaction time were not associated with the peripheral biomarkers (all p > 0.05). Accuracy tended to decrease in the resistance exercise condition (p = 0.054). Accuracy was associated with changes in adrenaline [rrm(18) = −0.51, p = 0.023], noradrenaline [rrm(18) = −0.66, p = 0.002], lactate [rrm(18) = −0.47, p = 0.035], and brain-derived neurotrophic factor [rrm(17) = −0.47, p = 0.044] in the resistance condition. These findings suggest that these peripheral biomarkers do not directly contribute to reduction in reaction time following aerobic or resistance exercise. However, greater sympathoexcitation, reflected by greater increase in noradrenaline, may be associated with a tendency for a reduction in accuracy after acute resistance exercise.
Introduction
Acute aerobic exercise at light/moderate intensity improves cognitive performance (Chang et al., 2012; McMorris, 2021). The effects of acute resistance exercise on cognitive performance have received increasing attention, and recent reviews have suggested that resistance exercise also has the potential to improve cognitive performance (Soga et al., 2018; Wilke et al., 2019). It is widely speculated that an increase in arousal is responsible for these improvements in cognitive performance (Chang et al., 2012; Ando et al., 2020; McMorris, 2021). However, the mechanism(s) responsible for cognitive improvement following acute aerobic and resistance exercise remain unclear. Specifically, the role of peripheral biomarkers following acute physical exercise on cognitive improvement has not been systematically evaluated.
Adrenaline and noradrenaline are important for the adaptive response to physiological stressors through the activation of the sympathoadrenomedullary system (Danese et al., 2018). In this context, there is some evidence in the literature that acute physical exercise increases circulating catecholamine concentrations (Pontifex et al., 2019; McMorris, 2021). Although peripheral adrenaline and noradrenaline do not easily traverse the blood-brain barrier (Cornford et al., 1982), increased circulating adrenaline and noradrenaline activate β-adrenoceptors on the afferent vagus nerve, which terminates in the nucleus tractus solitarii (NTS) within the blood-brain barrier (McGaugh et al., 1996). Noradrenergic cells in the NTS also project to the locus coeruleus and stimulate noradrenaline synthesis and release to other regions of the brain (McMorris, 2016). Thus, increased circulating adrenaline and noradrenaline may, at least in part, lead to an improvement in executive performance.
Blood glucose is the primary source of energy for the brain (Gold, 1995) and recent studies indicate that increased blood lactate after high-intensity interval exercise is associated with attentional or executive performance (Tsukamoto et al., 2016; Hashimoto et al., 2018; Herold et al., 2022). These findings suggest that enhanced lactate metabolism may contribute to executive improvements. However, the role of blood lactate following acute light intensity aerobic and resistance exercise is relatively unknown. Similarly, in response to acute stress, cortisol is released by the adrenal cortex and is regulated by the hypothalamic–pituitary–adrenal (HPA) (Costello et al., 2018). Tsai and colleagues reported that alterations in cortisol level after resistance exercise are associated with electrophysiological activity (i.e., P3 amplitude) (Tsai et al., 2014). These findings suggest that alterations in circulating cortisol level could be linked to executive performance following acute physical exercise.
Acute resistance exercise increases peripheral insulin-like growth hormone factor 1 (IGF-1) (Rubin et al., 2005; Rojas Vega et al., 2010; Tsai et al., 2014, 2018). Acute aerobic (Huang et al., 2014; Tsai et al., 2018), and resistance (Marston et al., 2017) exercise increases peripheral brain-derived neurotrophic factor (BDNF). Rodent studies suggest that increases in IGF-1 are related to improvements in learning and spatial memory after a period of training (Ding et al., 2006; Cassilhas et al., 2012). In contrast to adrenaline and noradrenaline, BDNF crosses the blood–brain barrier in both directions (Pan et al., 1998) and it has also been speculated that elevated BDNF might contribute to improvements in executive performance/memory after acute physical exercise (Ferris et al., 2007; Griffin et al., 2011; Piepmeier and Etnier, 2015; Borror, 2017). Indeed, improvement in executive performance was associated with exercise-related changes in BDNF (Hwang et al., 2016). However, IGF-1 and BDNF appears to play a crucial role in angiogenesis, synaptogenesis, and neurogenesis following long-term exercise (Cotman and Berchtold, 2002; Voss et al., 2011; Nieto-Estevez et al., 2016) and further studies are necessary to understand whether transient increases in IGF-1 and BDNF contribute to an improvement in executive performance following acute physical exercise.
Acute light aerobic exercise has been suggested to improve executive performance (Chang et al., 2012), and peripheral adrenaline and noradrenaline concentrations increase after acute light aerobic exercise (i.e., 40% maximal oxygen uptake) (McMurray et al., 1987). If changes in peripheral biomarkers, such as adrenaline and noradrenaline, are associated with alterations of the performance of executive functioning, it seems reasonable to hypothesize that an association between peripheral biomarkers and executive functioning can be observed even after light-intensity physical exercise. Lactate production in response to acute exercise depends on type of exercise (i.e., aerobic or resistance) (Hashimoto et al., 2021). Acute light aerobic exercise does not increase blood lactate concentration (Ivy et al., 1980). Conversely, resistance exercise at low intensity using elastic bands appear to increase blood lactate (Yasuda et al., 2014). Furthermore, an acute bout of resistance exercise, but not aerobic exercise, is a physiological stimulus for acute increases in IGF-1 (Gregory et al., 2013). These suggest that changes in some peripheral biomarkers (e.g., lactate and IGF-1) may be greater after resistance exercise relative to aerobic exercise. Hence, comparing the effects of aerobic and resistance exercise on executive performance and peripheral biomarkers can help to elucidate the common or divergent molecular mechanisms driving changes in cognitive performance after different types of acute physical exercises (i.e., aerobic vs. resistance exercises).
Accordingly, this study sought to investigate the relationship between peripheral circulating biomarkers and executive performance following acute, intensity matched, aerobic and resistance training exercise. We tested the hypothesis that: (i) acute aerobic and resistance exercise would improve executive performance, (ii) peripheral biomarkers would be associated with this performance, and (iii) the differential effects of aerobic and resistance exercise on peripheral biomarkers would delineate the specific contribution of these biomarkers to improvement in executive performance.
Materials and Methods
Participants
Nineteen healthy males volunteered to participate in this study [age: 22.5 ± 2.3 year; body weight: 1.71 ± 0.06 m; body mass: 66.2 ± 7.6 kg; peak oxygen uptake (V̇O2peak): 48.2 ± 7.1 ml kg min–1]. All participants were physically active and met the following criteria: (i) right handed as assessed by the Edinburgh Handedness Inventory (Oldfield, 1971); (ii) low-risk status for physical exercise-related adverse events assessed by Physical Activity Readiness Questionnaire (Warburton et al., 2011); and (iii) no history of cardiovascular, cerebrovascular, or respiratory disease (self-report). All participants gave their written informed consent prior to participation. Sample size was calculated using G-power (version 3.1) (Faul et al., 2009) based on our pilot data which suggested that a reduction in reaction time (RT) after aerobic exercise at 40% V̇O2peak was ∼28 ms (Cohen’s day effect size of 0.4). Accordingly, a minimum of 15 participants were required to achieve a power of 80% with an alpha of 0.05. The participants were instructed to abstain from any strenuous exercise for 24 h and food, caffeine and alcohol for 12 h prior to the laboratory visit. All experimental procedures adhered to the standards set by the latest revision of the declaration of Helsinki, except for registration in a database, and were approved by the ethics committee of Fukuoka University (2015-09-01).
Cognitive Task
Central executive function was assessed using a Go/No-Go task. The task was completed on a laptop computer (Let’s note CF-R4, Panasonic, Osaka, Japan) placed 80 cm from the participants. The participants performed the cognitive task sitting on a chair. The details of the cognitive task are described in detail elsewhere (Ando et al., 2013). Briefly, the participants were required to either respond (Go trial) or not (No-Go trial) according to the stimulus. A shift-key on the keyboard was used to perform the cognitive task. The participants pressed the key using the right index finger. A total of 30 trials were completed. Both RT and accuracy of the task were used to assess executive performance. Omitting a response in a Go trial or performing an incorrect response in a No-Go trial was regarded as an error trial. Accuracy of the task was calculated as number of correct response/total number of trials.
Experimental Procedure
This study employed a within-participants pre-test post-test crossover comparison in line with the taxonomy provided by Pontifex et al. (2019). The experiment was performed over three non-consecutive days with intervals of at least 3 days between experimental sessions. Throughout the experiment, the ambient temperature was maintained at 22°C and the relative humidity was controlled approximately at 50%. On the first day, the participants practiced the cognitive task until they were familiar with the task to minimize the impact of a learning effect. Thereafter, the participants performed a maximal exercise test to exhaustion on a cycle ergometer (75XLII, COMBI Wellness, Tokyo, Japan). After a warm-up period at 10 W for 1 min, the test was initiated with 20 W increments every minute in a ramp manner. Participants were instructed to maintain a cadence of 60 revolutions per min (rpm), and the test was terminated when they were unable to maintain a cadence of >40 rpm. Minute ventilation, oxygen uptake, fraction of end-tidal CO2, and O2 were recorded using a gas analysis system (ARCO-2000, ARCO System, Chiba, Japan), and V̇O2peak was determined as the highest value attained over the course of 1 min. Exercise intensity at 40% V̇O2peak (90 ± 13 watts) was subsequently calculated for aerobic exercise.
On the second and third days of the experiment, the experiments were performed on the same time to minimize circadian effects. At the beginning of the experiment, venous blood sample was collected from the antecubital vein for the analyses of adrenaline, noradrenaline, cortisol, IGF-1, and BDNF analysis. The left earlobe was pricked with a safety lancet and 2 μL capillary blood was collected for glucose and lactate analysis. Systolic blood pressure and diastolic blood pressure were measured from the right arm in a sitting position (HEM-705IT, Omron Healthcare, Kyoto, Japan). Mean arterial pressure (MAP) was subsequently calculated. Then, the participants performed the first cognitive task. After the cognitive task, the participants performed either aerobic or resistance exercise for 30 min. The order of exercise type was randomly counterbalanced. Immediately after the exercise, venous and capillary blood samples were collected, and blood pressure was measured. Then, the participants performed the second cognitive task.
Several studies have compared the effects of aerobic and resistance exercise on cognitive performance using a randomized crossover design (Pontifex et al., 2009; Alves et al., 2012; Harveson et al., 2016; Dunsky et al., 2017). However, exercise intensity is one of the key factors that determine the exercise-cognition interaction (Chang et al., 2012) and these previous studies did not attempt to match heart rate (HR) between the aerobic and resistance exercise. This is most likely attributed to the challenges associated with matching HR during both aerobic and resistance exercise. Thus, following extensive pilot tests, we attempted to match ratings of perceived exertion (RPE) (6–20 Borg scale) (Borg, 1975) between aerobic and resistance exercise. In the aerobic exercise condition, the intensity was set at 40% V̇O2peak. Mean HR corresponded to 58.7 ± 6.0% age-predicted maximal HR in the aerobic condition and 52.7 ± 7.5% in the resistance condition. Thus, exercise intensity of the aerobic exercise was considered light according to the ACSM guidelines (Riebe et al., 2018). In the resistance condition, the participants performed resistance exercise using elastic bands (Spoband 55, YKC, Tokyo, Japan). We used elastic bands for the resistance exercise as they are low-cost, portable, have a low risk of injury, and are easily accessible. The findings may be practically useful for exercise prescription at home, or in other settings. The validity of intervention was confirmed by a previous study (Roh et al., 2020). The resistance exercise program was designed to use all major muscle groups (Hofmann et al., 2016). The resistance of the band was 10.3 kg when the length of the band was doubled. The participants performed an exercise program incorporating 42 difference exercises (see Supplementary Table 1). This program targeted the following muscle groups: shoulder, chest, back, arms, abdomen, hip, and legs, and included three types of muscle contractions (concentric, eccentric, and isometric) and both multi- and single joint movements. After brief instruction for each program, and participants completed 10 reps of each exercise. The duration of the exercise program was 30 min.
Measurement
Heart rate was recorded continuously using a heart rate monitor (RS800CX; Polar Electro Oy, Kempele, Finland). RPE was recorded before and immediately after exercise. Blood glucose concentration was measured by glucose oxidase method using blood glucose monitor (Glutest Ace, Sanwa Kagaku, Nagoya, Japan). Blood lactate concentration was determined by the lactate oxidase method, using an automated analyzer (Lactate Pro, Arkray, Kyoto, Japan). Blood sample volume was ∼15 ml for each measurement, and both plasma and serum samples were collected. Plasma samples were obtained from heparinized blood samples by centrifugation at 3,000 rpm for 15 min and stored at −80°C until analysis. Plasma adrenaline and noradrenaline concentrations were determined using a high-performance liquid chromatography system (Shimadzu, Kyoto, Japan). Serum samples were obtained from the venous blood by centrifugation at 3,000 rpm for 15 min and stored at −80°C until analysis. Serum cortisol was measured by commercial radioimmunoassay kit (Immunotech, Marseille, France). Serum IGF-1 concentration was determined using an immunoradiometric assay (IGF-1 IRMA Daiichi, TFB, Tokyo, Japan) and a Wallac 1460 Gamma Counter (Wallac, Turku, Finland). Serum BDNF concentration was measured using the Quantikine Human BDNF Immunoassay (R&D systems, Minneapolis, United States). Due to collection issues resulting in too small sample volumes, the concentration changes of BDNF from two participants (one in the aerobic condition and one in the resistance condition) could not be determined. Adrenaline, noradrenaline, cortisol, IGF-1, and BDNF concentrations were measured at the SRL Clinical Laboratory (Tokyo, Japan). All samples were analyzed in duplicate. Intra- and inter-assay coefficients of variance were 2.8 and 2.6% for adrenaline, 1.0 and 1.4% for noradrenaline, 1.7 and 1.6% for cortisol, and 3.5 and 3.0% for IGF-1.
Data and Statistical Analysis
The distribution of data was assessed using descriptive methods (skewness, outliers, and distribution plots) and inferential statistics (Shapiro-Wilk test). We performed a two-way repeated-measures ANOVA [Exercise Type (aerobic, resistance) × Time (pre, post)] for all variables, followed by Bonferroni-corrected paired t-tests for normally distributed data or the Wilcoxon signed rank test for non-normally distributed data. All the RT values were plausible, and within the expected ranges, based on our previous studies (Ando et al., 2013; Komiyama et al., 2015). Effect sizes are presented as partial eta-squared (ηp2) in the main effects and interactions. Statistical analyses were performed using SPSS (Statistical Package for the Social Sciences) version 25.0 (SPSS Inc., Chicago, IL, United States). We also performed repeated measures correlation analysis (Bakdash and Marusich, 2017; Marusich and Bakdash, 2021) between executive performance (both RT and Accuracy) and peripheral biomarkers. Raincloud plots were created using the JASP version 0.16 (JASP team, Amsterdam, Netherlands). Data are expressed as mean ± SD or median (interquartile range). The significance level was set at p < 0.05.
Results
Figure 1 illustrates RT and accuracy of the Go/No-Go task. A significant main effect of Time was observed in RT [F(1,18) = 5.02, p = 0.038, ηp2 = 0.22]; however, no effect of Exercise Type [F(1,18) = 0.07, p = 0.792, ηp2 = 0.004], or interaction [F(1,18) = 0.06, p = 0.812, ηp2 = 0.003] was observed. There were no significant main effects of Exercise Type [F(1,18) = 0.41, p = 0.530, ηp2 = 0.02] and Time [F(1,18) = 0.01, p = 0.939, ηp2 = 0.000] on the accuracy of the Go/No-Go task. However, there was a trend toward a significant interaction effect [F(1,18) = 4.41, p = 0.050, ηp2 = 0.20].
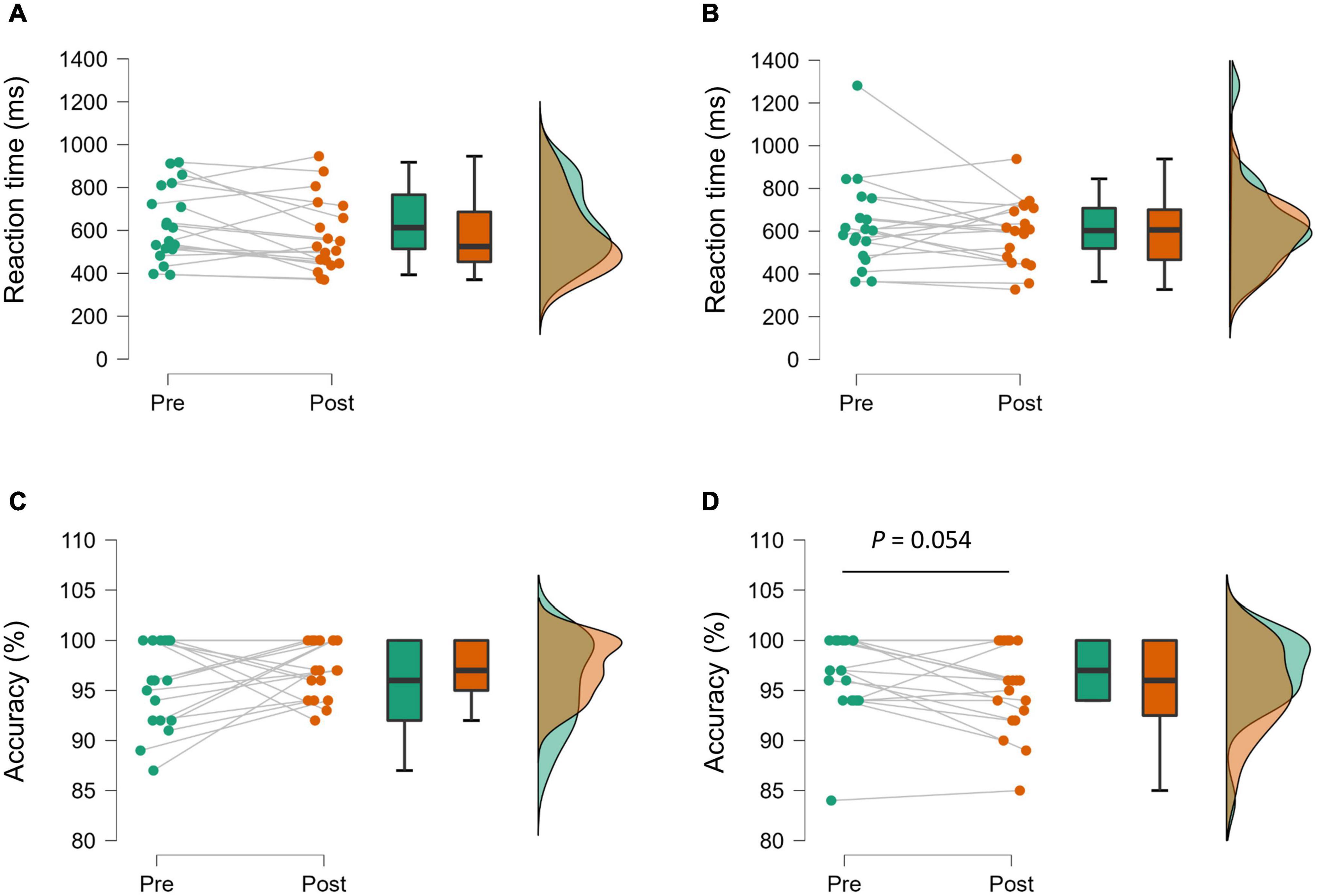
Figure 1. Raincloud plots showing the distribution of reaction time [(A) aerobic condition, (B) resistance condition] and accuracy of the Go/No-Go task [(C) aerobic condition, (D) resistance condition]. Plots and lines represent individual data (left). The lower and upper hinges on the boxplots represent the first and third quartile with the median (center). Illustration of data distribution (right).
Table 1 summarizes HR, RPE, MAP, and peripheral biomarkers. For HR, we found a significant interaction between Exercise Type and Time, and HR was significantly greater during aerobic exercise than that during resistance exercise (p = 0.004). RPE increased after aerobic and resistance exercise. Both adrenaline and noradrenaline increased after both aerobic and resistance exercise, but there were no differences between the modalities (all p > 0.05). Glucose significantly decreased after aerobic and resistance exercise (main effect of Time, p = 0.001). Although lactate increased after both aerobic (p = 0.045) and resistance (p < 0.001) exercise, it was higher after resistance exercise (p < 0.001). Cortisol decreased after aerobic and resistance exercise (main effect of Time, p = 0.002). IGF-1 was elevated following resistance exercise (p = 0.003), but not after the aerobic exercise (p = 0.36). There was a trend for BDNF to be higher after exercise (main effect of Time, p = 0.06).
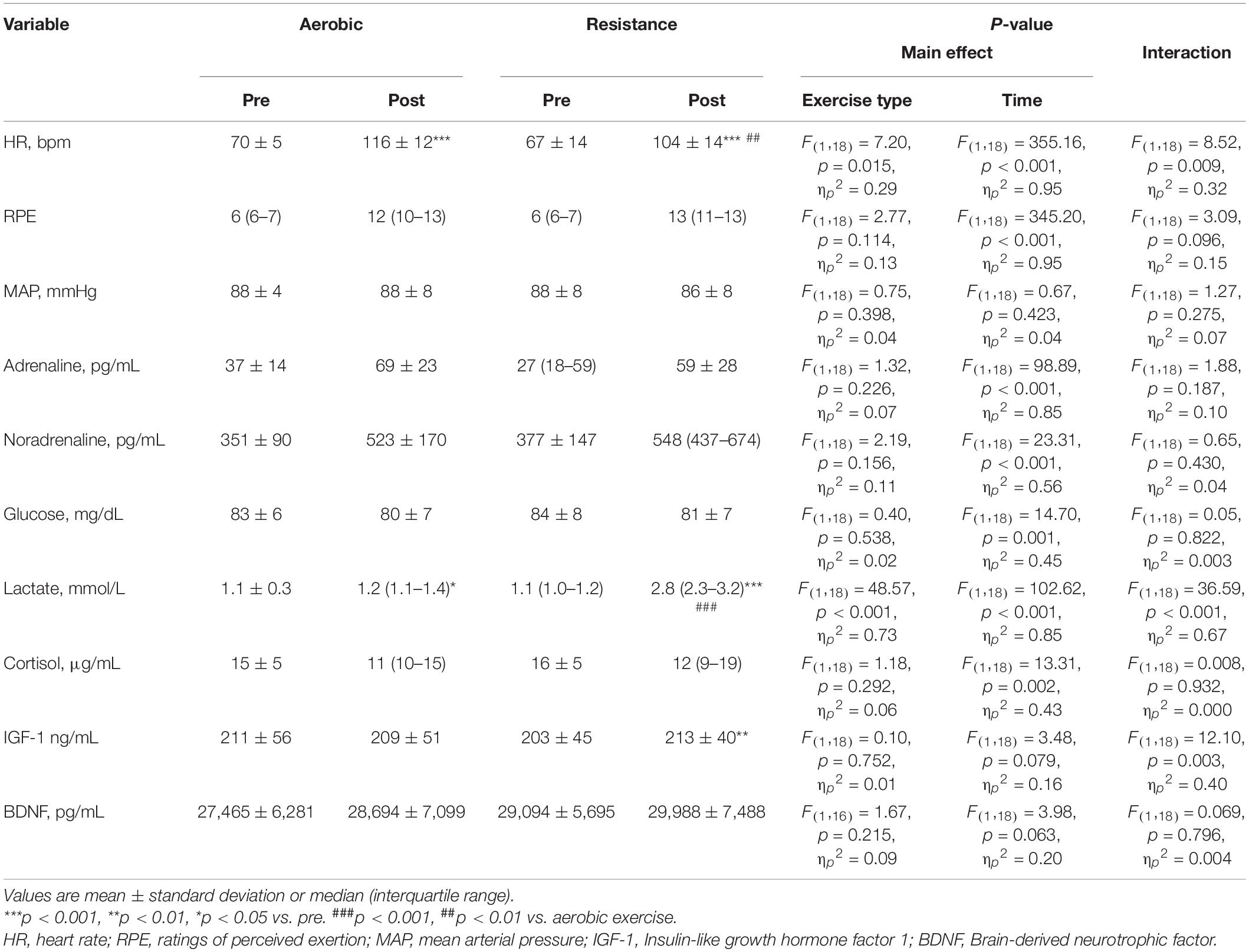
Table 1. Heart rate (HR), MAP, and peripheral biomarkers before and after acute aerobic and resistance exercise.
Table 2 displays the results of repeated measures correlation analysis. Reduction in RT was not associated with changes in any of the circulating biomarkers (all p > 0.05). Conversely, accuracy was associated with changes in adrenaline [rrm(18) = −0.51, p = 0.023], noradrenaline [rrm(18) = −0.66, p = 0.002], lactate [rrm(18) = −0.47, p = 0.035], and BDNF [rrm(17) = −0.47, p = 0.044] in the resistance (Figure 2), but not aerobic (all p > 0.05), condition.
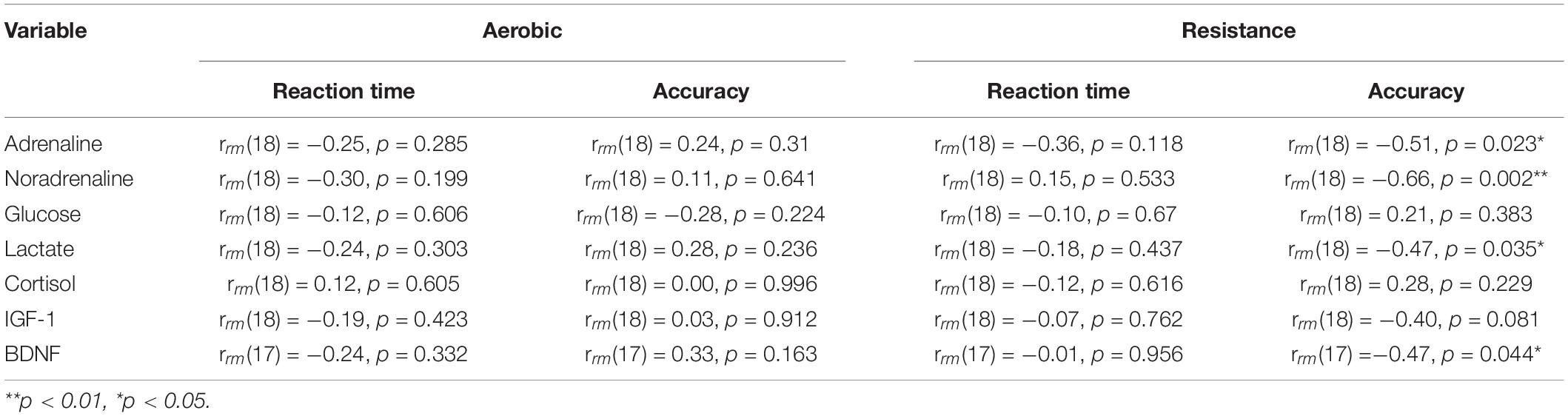
Table 2. Repeated measures correlation between cognitive performance (reaction time and accuracy) and peripheral biomarkers.
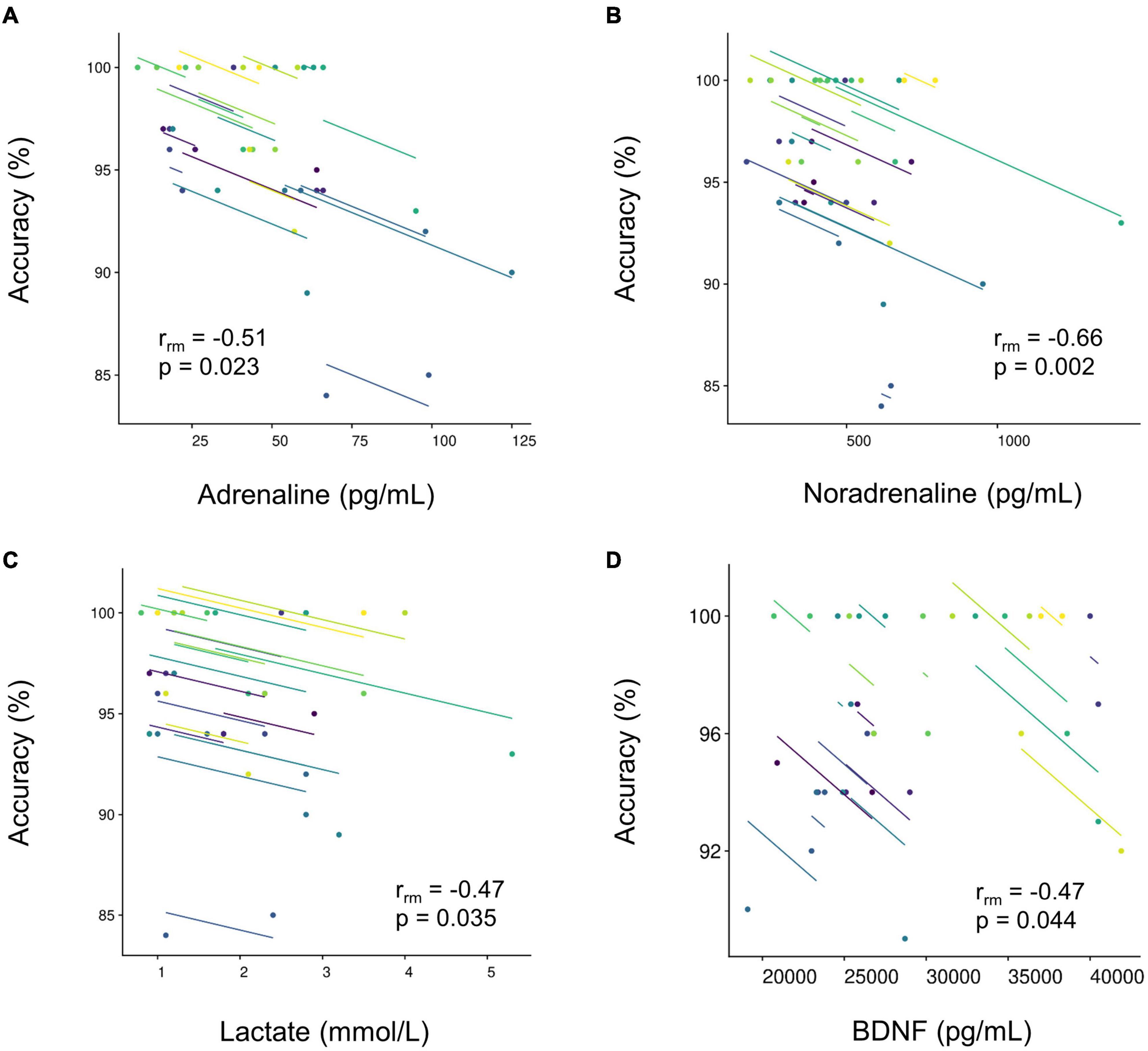
Figure 2. Repeated measure correlations between the accuracy of the Go/No-Go task and peripheral circulating biomarkers [(A) adrenaline, n = 19; (B) noradrenaline, N = 19; (C) lactate, N = 19; and (D) brain-derived neurotrophic factor, N = 18] in the resistance condition. Plots represent individual data. Lines show the rrm fit for the participants. Same colors represent data from the same participants.
Discussion
The major findings of this study were: (1) executive performance improved (i.e., reduced RT) after acute RPE-matched aerobic and resistance exercise, while accuracy of the executive task tended to be impaired after resistance exercise; (2) acute physical exercise resulted in alterations in peripheral biomarkers; (3) reduced RT was not correlated with alterations in peripheral biomarkers; and (4) accuracy was associated with increases in peripheral adrenaline, noradrenaline, lactate, and BDNF after resistance, but not aerobic, exercise.
Previous studies using electroencephalogram suggest increases in arousal following both aerobic (Kamijo et al., 2004) and resistance (Tsai et al., 2014) exercise. Acute physical exercise has also been implicated in altering brain circuits involving neurotransmitters (Pontifex et al., 2019; McMorris, 2021). This suggests that executive improvement after acute physical exercise may be related to increased central neurochemical activity. We observed reduced RTs and increases in adrenaline and noradrenaline after both aerobic and resistance exercise. As the increases in both adrenaline and noradrenaline were comparable after aerobic and resistance exercise, it is plausible that the activation of the sympathoadrenomedullary system was also relatively similar following the two types of exercise. Nevertheless, alterations in RTs were not correlated with alterations in peripheral adrenaline and noradrenaline. The catecholamine hypothesis describing the exercise-cognition interaction is intriguing (Cooper, 1973). However, the specific mechanism(s) by which acute physical exercise improves executive performance warrant further investigation. We observed a tendency for accuracy to be decreased after resistance exercise (p = 0.054). Furthermore, lower accuracy was associated with a greater increase in peripheral adrenaline and noradrenaline after resistance exercise. Although we did not assess adrenaline and noradrenaline level directly in the brain, excess noradrenaline appears to impair prefrontal cortex function including cognitive function in the brain (Arnsten, 2011). Therefore, the present findings may suggest that greater sympathoexcitation has the potential to be associated with accuracy in a Go/No-Go task.
Executive performance improves during exercise after skipping breakfast (Komiyama et al., 2016), which suggests that energy substrates may compensate for reduced availability of blood glucose. It is possible that lactate is used by the brain (Quistorff et al., 2008), and increases in blood lactate concentration appear to provide energy that contributes to improvements in attentional and executive performance after high intensity exercise (Tsukamoto et al., 2016; Hashimoto et al., 2018; Herold et al., 2022). Conversely, Coco et al. (2009, 2020) have shown that increase in blood lactate concentration have adverse effects on attentional and executive performances. We observed no associations between reduction in RT and alterations in lactate or glucose. The findings are inconsistent with others (Tsukamoto et al., 2016; Hashimoto et al., 2018; Herold et al., 2022). It is likely that these heterogeneous findings are attributable to methodological differences. First, the exercise intensity was higher (high intensity interval exercise: 80—90% and 50–60% of maximal workload) in previous studies (Tsukamoto et al., 2016; Hashimoto et al., 2018), compared to the intensity used in the current study (40% V̇O2peak). Given that blood lactate substantially increases after high intensity, contribution of blood lactate to cognitive improvement after acute physical exercise may be dependent on the degree of increase in blood lactate. Second, Hashimoto et al. (2018) directly measured brain blood lactate uptake, while blood lactate was measured from the antecubital vein in the present study. This may also explain the lack of a significant correlation between executive improvement and increase in blood lactate.
In the resistance condition, the trend toward impaired accuracy was modestly associated with blood lactate. Blood lactate uptake in the brain becomes significantly elevated when arterial lactate increases, for instance, in response to strenuous physical exercises (Quistorff et al., 2008). In the present study, increases in blood lactate was limited up to around 3 mmol L–1 in most cases. Hence, the amount of blood lactate uptake in the brain is presumably minimal, and it is less likely that blood lactate directly impaired the accuracy. Our findings suggest that there is a large inter-individual variability in accuracy of a task probing executive functioning after acute resistance exercises (see Figure 1D). Furthermore, accuracy seems to be more impaired in individuals who showed more pronounced physiological alterations (i.e., higher level of peripheral blood lactate) in response to an acute bout of resistance exercises (see also Figure 2C). In general, the latter finding fits to the observations of Coca and colleagues who reported that high levels of lactate have detrimental effects on attentional and executive performance (Coco et al., 2009, 2020). However, given the inconclusive results in the literature, further studies are warranted to investigate whether lactate acts as a possible mediator of exercise-induced changes in executive performance.
The HPA system is sensitive to acute physiological stress including exercise (Gaffey et al., 2016; Williams et al., 2019). In the present study, cortisol decreased after both aerobic and resistance exercise. Hill et al. (2008) reported that low intensity aerobic exercise (i.e., 40% maximal oxygen uptake) decreased circulating cortisol level (Hill et al., 2008). Tsai et al. (2014) also reported decrease in cortisol level after moderate resistance exercise (Tsai et al., 2014). The present results are in line with these observations. We observed no association between executive improvement and in the change in cortisol after acute aerobic or resistance exercise. Tsai et al. (2014) suggested that arousal level might be modulated by alteration in cortisol. However, alterations in cortisol were not associated with alterations in executive performance. These findings suggest cortisol may not be directly associated with executive performance after acute physical exercise.
Consistent with the literature acute resistance, but not aerobic, exercise increased peripheral IGF-1 (Rubin et al., 2005; Rojas Vega et al., 2010; Tsai et al., 2014; Tsai et al., 2018). BDNF also tended to increase after both aerobic and resistance exercise. Although this is not always the case, previous studies have reported that BDNF increases after exercise at higher intensities (Ferris et al., 2007; Winter et al., 2007). Therefore, given the relatively low exercise intensity of the present study, the absence of significant increases in BDNF are perhaps not surprising. We observed no association between executive performance and alterations in IGF-1 and BDNF. Previous studies reported no association between alterations in IGF-1 and executive performance after acute resistance exercise (Tsai et al., 2014, 2018). Similarly, increases in IGF-1 after exhaustive exercise have previously been shown to be unrelated with executive performance (Sudo et al., 2017) and alterations in BDNF were not associated with executive performance and neurophysiological variables after acute physical exercise (Tsai et al., 2016). In contrast, significant associations were observed between alteration in BDNF and performance in executive and memory tasks after acute physical exercise (Winter et al., 2007; Lee et al., 2014; Skriver et al., 2014). Thus, although alterations in BDNF appears not to be directly associated with improvement in executive performance after acute physical exercise, BDNF may contribute to memory performance. Given that IGF-1 and BDNF are associated with angiogenesis, synaptogenesis, and neurogenesis after long-term exercise (Cotman and Berchtold, 2002; Voss et al., 2011; Nieto-Estevez et al., 2016), it is reasonable to think that alterations in IGF-1 or BDNF may play a minor role in executive performance following acute physical exercise. Nevertheless, further studies are required to clarify the contribution of IGF-1 and BDNF to cognitive performance (e.g., memory performance) after acute physical exercise. In the resistance condition, the trend toward an impairment in accuracy was moderately associated with BDNF. Previous studies indicated that increase in BDNF is dependent on exercise intensity (Ferris et al., 2007; Winter et al., 2007). Thus, like the association between impaired accuracy and blood lactate, accuracy could be more impaired in those individuals showing more pronounced alterations in specific biomarkers in response to a given exercise intensity.
The present study was not without limitation. First, despite attempting to match exercise intensity between the aerobic and resistance exercise, there were significant differences in HR (∼12 beats min–1). We also found inter-individual differences in HR across both conditions. Second, we observed a trend toward an impairment in accuracy and the association between accuracy and peripheral biomarkers only in the resistance condition. Given that dose-response is important to examine exercise-cognition interaction (Herold et al., 2019, 2020), the results may be attributable to these differences in relative exercise intensity. Third, since we did not include a control condition, we cannot rule out the possibility that time-related factors including practice effects and circadian change of peripheral biomarkers. However, the participants were familiarized with the cognitive task and exercise duration was only 30 min. Thus, the effects may be small, if any. Fourth, we estimated sample size based on the primary outcome (i.e., RT). This might lead to low statistical power to detect the association between cognitive performance and peripheral biomarkers. Indeed, we observed no significant correlations between the reductions in RTs and peripheral biomarkers following acute aerobic and resistance exercise. The present results suggest that these peripheral circulating biomarkers are not capable of indicating the level of executive performance after exercise. However, the effects of acute physical exercise on cognitive performance are multifaceted and probably determined by the integration of several physiological factors. Perhaps, some of these peripheral biomarkers may contribute to executive improvement in a synergistic manner. Fifth, we did not take consider the impact of other potential confounding factors including nutritional status, habitual physical activity, and psychological factors (e.g. motivation, concentration, and fatigue). Finally, we collected venous and capillary blood samples immediately after aerobic and resistance exercise. The timing of the measurement is commonly used in the literature. However, concentrations of peripheral biomarkers appear to change depending on the time elapsed after physical exercises (e.g., Griffin et al., 2011; Gregory et al., 2013; Hashimoto et al., 2018), and the association between executive performance and peripheral biomarkers are likely to be influenced by the timing of measurement (e.g., immediately or 15 min after exercise). This should be considered in future studies.
Conclusion
In conclusion, despite observing a reduction in RT in the Go/No-Go task following both aerobic exercise and resistance exercise, we observed no significant correlations between these reductions and peripheral adrenaline, noradrenaline, glucose, lactate, cortisol, IGF-1, or BDNF. These results suggest that alterations in these peripheral circulating biomarkers do not directly contribute to improved RT after aerobic and resistance exercise. However, greater sympathoexcitation, reflected by greater increase in noradrenaline, may be associated with a tendency for a reduction accuracy after acute resistance exercise.
Data Availability Statement
The raw data supporting the conclusions of this article will be made available by the authors, without undue reservation.
Ethics Statement
The studies involving human participants were reviewed and approved by the Ethics Committee of Fukuoka University. The patients/participants provided their written informed consent to participate in this study.
Author Contributions
SA and TK contributed to the conception and design of the study and drafted the manuscript. SA, TK, YT, and MS acquired the data and performed the data analysis. JC, YU, and YH edited and revised the manuscript. All authors interpreted the results, read, and approved the final version of the manuscript.
Funding
This study was supported in part by a grant from Descente and Ishimoto Memorial Foundation for Promotion of Sports Science and the Japan Society for the Promotion of Science KAKENHI (Grant Number: 16H03230).
Conflict of Interest
The authors declare that the research was conducted in the absence of any commercial or financial relationships that could be construed as a potential conflict of interest.
Publisher’s Note
All claims expressed in this article are solely those of the authors and do not necessarily represent those of their affiliated organizations, or those of the publisher, the editors and the reviewers. Any product that may be evaluated in this article, or claim that may be made by its manufacturer, is not guaranteed or endorsed by the publisher.
Acknowledgments
We acknowledge Hiroaki Tanaka for his support. We are also grateful to Kisou Kubota for providing software to assess cognitive performance, and to Kenji Ando for data acquisition.
Supplementary Material
The Supplementary Material for this article can be found online at: https://www.frontiersin.org/articles/10.3389/fnbeh.2022.853150/full#supplementary-material
References
Alves, C. R., Gualano, B., Takao, P. P., Avakian, P., Fernandes, R. M., Morine, D., et al. (2012). Effects of acute physical exercise on executive functions: a comparison between aerobic and strength exercise. J. Sport Exerc. Psychol. 34, 539–549. doi: 10.1123/jsep.34.4.539
Ando, S., Hatamoto, Y., Sudo, M., Kiyonaga, A., Tanaka, H., and Higaki, Y. (2013). The effects of exercise under hypoxia on cognitive function. PLoS One 8:e63630. doi: 10.1371/journal.pone.0063630
Ando, S., Komiyama, T., Sudo, M., Higaki, Y., Ishida, K., Costello, J. T., et al. (2020). The interactive effects of acute exercise and hypoxia on cognitive performance: a narrative review. Scand. J. Med. Sci. Sports 30, 384–398. doi: 10.1111/sms.13573
Arnsten, A. F. (2011). Catecholamine influences on dorsolateral prefrontal cortical networks. Biol. Psychiatry 69, e89–e99. doi: 10.1016/j.biopsych.2011.01.027
Bakdash, J. Z., and Marusich, L. R. (2017). Repeated Measures Correlation. Front. Psychol. 8:456. doi: 10.3389/fpsyg.2017.00456
Borror, A. (2017). Brain-derived neurotrophic factor mediates cognitive improvements following acute exercise. Med. Hypotheses 106, 1–5. doi: 10.1016/j.mehy.2017.06.024
Cassilhas, R. C., Lee, K. S., Fernandes, J., Oliveira, M. G., Tufik, S., Meeusen, R., et al. (2012). Spatial memory is improved by aerobic and resistance exercise through divergent molecular mechanisms. Neuroscience 202, 309–317. doi: 10.1016/j.neuroscience.2011.11.029
Chang, Y. K., Labban, J. D., Gapin, J. I., and Etnier, J. L. (2012). The effects of acute exercise on cognitive performance: a meta-analysis. Brain Res. 1453, 87–101. doi: 10.1016/j.brainres.2012.02.068
Coco, M., Buscemi, A., Guerrera, C. S., Di Corrado, D., Cavallari, P., Zappala, A., et al. (2020). Effects of a Bout of Intense Exercise on Some Executive Functions. Int. J. Environ. Res. Public Health 17:898. doi: 10.3390/ijerph17030898
Coco, M., Di Corrado, D., Calogero, R. A., Perciavalle, V., Maci, T., and Perciavalle, V. (2009). Attentional processes and blood lactate levels. Brain Res. 1302, 205–211. doi: 10.1016/j.brainres.2009.09.032
Cooper, C. J. (1973). Anatomical and physiological mechanisms of arousal, with special reference to the effects of exercise. Ergonomics 16, 601–609. doi: 10.1080/00140137308924551
Cornford, E. M., Braun, L. D., Oldendorf, W. H., and Hill, M. A. (1982). Comparison of lipid-mediated blood-brain-barrier penetrability in neonates and adults. Am. J. Physiol. 243, C161–C168. doi: 10.1152/ajpcell.1982.243.3.C161
Costello, J. T., Rendell, R. A., Furber, M., Massey, H. C., Tipton, M. J., Young, J. S., et al. (2018). Effects of acute or chronic heat exposure, exercise and dehydration on plasma cortisol, IL-6 and CRP levels in trained males. Cytokine 110, 277–283. doi: 10.1016/j.cyto.2018.01.018
Cotman, C. W., and Berchtold, N. C. (2002). Exercise: a behavioral intervention to enhance brain health and plasticity. Trends Neurosci. 25, 295–301. doi: 10.1016/s0166-2236(02)02143-4
Danese, E., Tarperi, C., Salvagno, G. L., Guzzo, A., Sanchis-Gomar, F., Festa, L., et al. (2018). Sympatho-adrenergic activation by endurance exercise: effect on metanephrines spillover and its role in predicting athlete’s performance. Oncotarget 9, 15650–15657. doi: 10.18632/oncotarget.24584
Ding, Q., Vaynman, S., Akhavan, M., Ying, Z., and Gomez-Pinilla, F. (2006). Insulin-like growth factor I interfaces with brain-derived neurotrophic factor-mediated synaptic plasticity to modulate aspects of exercise-induced cognitive function. Neuroscience 140, 823–833. doi: 10.1016/j.neuroscience.2006.02.084
Dunsky, A., Abu-Rukun, M., Tsuk, S., Dwolatzky, T., Carasso, R., and Netz, Y. (2017). The effects of a resistance vs. an aerobic single session on attention and executive functioning in adults. PLoS One 12:e0176092. doi: 10.1371/journal.pone.0176092
Faul, F., Erdfelder, E., Buchner, A., and Lang, A. G. (2009). Statistical power analyses using G*Power 3.1: tests for correlation and regression analyses. Behav. Res. Methods 41, 1149–1160. doi: 10.3758/BRM.41.4.1149
Ferris, L. T., Williams, J. S., and Shen, C. L. (2007). The effect of acute exercise on serum brain-derived neurotrophic factor levels and cognitive function. Med. Sci. Sports Exerc. 39, 728–734. doi: 10.1249/mss.0b013e31802f04c7
Gaffey, A. E., Bergeman, C. S., Clark, L. A., and Wirth, M. M. (2016). Aging and the HPA axis: stress and resilience in older adults. Neurosci. Biobehav. Rev. 68, 928–945. doi: 10.1016/j.neubiorev.2016.05.036
Gold, P. E. (1995). Role of glucose in regulating the brain and cognition. Am. J. Clin. Nutr. 61, 987S–995S. doi: 10.1093/ajcn/61.4.987S
Gregory, S. M., Spiering, B. A., Alemany, J. A., Tuckow, A. P., Rarick, K. R., Staab, J. S., et al. (2013). Exercise-induced insulin-like growth factor I system concentrations after training in women. Med. Sci. Sports Exerc. 45, 420–428. doi: 10.1249/MSS.0b013e3182750bd4
Griffin, E. W., Mullally, S., Foley, C., Warmington, S. A., O’mara, S. M., and Kelly, A. M. (2011). Aerobic exercise improves hippocampal function and increases BDNF in the serum of young adult males. Physiol. Behav. 104, 934–941. doi: 10.1016/j.physbeh.2011.06.005
Harveson, A. T., Hannon, J. C., Brusseau, T. A., Podlog, L., Papadopoulos, C., Durrant, L. H., et al. (2016). Acute Effects of 30 Minutes Resistance and Aerobic Exercise on Cognition in a High School Sample. Res. Q. Exerc. Sport 87, 214–220. doi: 10.1080/02701367.2016.1146943
Hashimoto, T., Tsukamoto, H., Ando, S., and Ogoh, S. (2021). Effect of Exercise on Brain Health: the Potential Role of Lactate as a Myokine. Metabolites 11:813. doi: 10.3390/metabo11120813
Hashimoto, T., Tsukamoto, H., Takenaka, S., Olesen, N. D., Petersen, L. G., Sorensen, H., et al. (2018). Maintained exercise-enhanced brain executive function related to cerebral lactate metabolism in men. FASEB J. 32, 1417–1427. doi: 10.1096/fj.201700381RR
Herold, F., Behrendt, T., Meißner, C., Müller, N., and Schega, L. (2022). The Influence of Acute Sprint Interval Training on Cognitive Performance of Healthy Younger Adults. Int. J. Environ. Res. Public Health 19:613. doi: 10.3390/ijerph19010613
Herold, F., Muller, P., Gronwald, T., and Muller, N. G. (2019). Dose-Response Matters! - A Perspective on the Exercise Prescription in Exercise-Cognition Research. Front. Psychol. 10:2338. doi: 10.3389/fpsyg.2019.02338
Herold, F., Torpel, A., Hamacher, D., Budde, H., and Gronwald, T. (2020). A Discussion on Different Approaches for Prescribing Physical Interventions - Four Roads Lead to Rome, but Which One Should We Choose? J. Pers. Med. 10:55. doi: 10.3390/jpm10030055
Hill, E. E., Zack, E., Battaglini, C., Viru, M., Viru, A., and Hackney, A. C. (2008). Exercise and circulating cortisol levels: the intensity threshold effect. J. Endocrinol. Invest. 31, 587–591. doi: 10.1007/BF03345606
Hofmann, M., Schober-Halper, B., Oesen, S., Franzke, B., Tschan, H., Bachl, N., et al. (2016). Effects of elastic band resistance training and nutritional supplementation on muscle quality and circulating muscle growth and degradation factors of institutionalized elderly women: the Vienna Active Ageing Study (VAAS). Eur. J. Appl. Physiol. 116, 885–897. doi: 10.1007/s00421-016-3344-8
Huang, T., Larsen, K. T., Ried-Larsen, M., Moller, N. C., and Andersen, L. B. (2014). The effects of physical activity and exercise on brain-derived neurotrophic factor in healthy humans: a review. Scand. J. Med. Sci. Sports 24, 1–10. doi: 10.1111/sms.12069
Hwang, J., Brothers, R. M., Castelli, D. M., Glowacki, E. M., Chen, Y. T., Salinas, M. M., et al. (2016). Acute high-intensity exercise-induced cognitive enhancement and brain-derived neurotrophic factor in young, healthy adults. Neurosci. Lett. 630, 247–253. doi: 10.1016/j.neulet.2016.07.033
Ivy, J. L., Withers, R. T., Van Handel, P. J., Elger, D. H., and Costill, D. L. (1980). Muscle respiratory capacity and fiber type as determinants of the lactate threshold. J. Appl. Physiol. Respir. Environ. Exerc. Physiol. 48, 523–527. doi: 10.1152/jappl.1980.48.3.523
Kamijo, K., Nishihira, Y., Hatta, A., Kaneda, T., Kida, T., Higashiura, T., et al. (2004). Changes in arousal level by differential exercise intensity. Clin. Neurophysiol. 115, 2693–2698. doi: 10.1016/j.clinph.2004.06.016
Komiyama, T., Sudo, M., Higaki, Y., Kiyonaga, A., Tanaka, H., and Ando, S. (2015). Does moderate hypoxia alter working memory and executive function during prolonged exercise? Physiol. Behav. 139, 290–296. doi: 10.1016/j.physbeh.2014.11.057
Komiyama, T., Sudo, M., Okuda, N., Yasuno, T., Kiyonaga, A., Tanaka, H., et al. (2016). Cognitive function at rest and during exercise following breakfast omission. Physiol. Behav. 157, 178–184. doi: 10.1016/j.physbeh.2016.02.013
Lee, J. K., Koh, A. C., Koh, S. X., Liu, G. J., Nio, A. Q., and Fan, P. W. (2014). Neck cooling and cognitive performance following exercise-induced hyperthermia. Eur. J. Appl. Physiol. 114, 375–384. doi: 10.1007/s00421-013-2774-9
Marston, K. J., Newton, M. J., Brown, B. M., Rainey-Smith, S. R., Bird, S., Martins, R. N., et al. (2017). Intense resistance exercise increases peripheral brain-derived neurotrophic factor. J. Sci. Med. Sport 20, 899–903. doi: 10.1016/j.jsams.2017.03.015
Marusich, L. R., and Bakdash, J. Z. (2021). rmcorrShiny: a web and standalone application for repeated measures correlation. F1000Res. 10:697. doi: 10.12688/f1000research.55027.2
McGaugh, J. L., Cahill, L., and Roozendaal, B. (1996). Involvement of the amygdala in memory storage: interaction with other brain systems. Proc. Natl. Acad. Sci. U. S. A. 93, 13508–13514. doi: 10.1073/pnas.93.24.13508
McMorris, T. (2016). “Beyond the catecholamine hypothesis for an acute exercise-cognition interaction: a neurochemical perspective” in Exercise-Cognition Interaction: neuroscience Perspective. ed. T. Mcmorris (London: Elsevier).
McMorris, T. (2021). The acute exercise-cognition interaction: from the catecholamines hypothesis to an interoception model. Int. J. Psychophysiol. 170, 75–88. doi: 10.1016/j.ijpsycho.2021.10.005
McMurray, R. G., Forsythe, W. A., Mar, M. H., and Hardy, C. J. (1987). Exercise intensity-related responses of beta-endorphin and catecholamines. Med. Sci. Sports Exerc. 19, 570–574.
Nieto-Estevez, V., Defterali, C., and Vicario-Abejon, C. (2016). IGF-I: a Key Growth Factor that Regulates Neurogenesis and Synaptogenesis from Embryonic to Adult Stages of the Brain. Front. Neurosci. 10:52. doi: 10.3389/fnins.2016.00052
Oldfield, R. C. (1971). The assessment and analysis of handedness: the Edinburgh inventory. Neuropsychologia 9, 97–113. doi: 10.1016/0028-3932(71)90067-4
Pan, W., Banks, W. A., Fasold, M. B., Bluth, J., and Kastin, A. J. (1998). Transport of brain-derived neurotrophic factor across the blood-brain barrier. Neuropharmacology 37, 1553–1561. doi: 10.1016/s0028-3908(98)00141-5
Piepmeier, A. T., and Etnier, J. L. (2015). Brain-derived neurotrophic factor (BDNF) as a potential mechanism of the effects of acute exercise on cognitive performance. J. Sport Health Sci. 4, 14–23.
Pontifex, M. B., Hillman, C. H., Fernhall, B., Thompson, K. M., and Valentini, T. A. (2009). The effect of acute aerobic and resistance exercise on working memory. Med. Sci. Sports Exerc. 41, 927–934. doi: 10.1249/MSS.0b013e3181907d69
Pontifex, M. B., Mcgowan, A. L., Chandler, M. C., Gwizdala, K. L., Parks, A. C., Fenn, K., et al. (2019). A primer on investigating the after effects of acute bouts of physical activity on cognition. Psychol. Sport Exerc. 40, 1–22.
Quistorff, B., Secher, N. H., and Van Lieshout, J. J. (2008). Lactate fuels the human brain during exercise. FASEB J. 22, 3443–3449. doi: 10.1096/fj.08-106104
Riebe, D., Ehrman, J. K., Liguori, G., and Magal, M. American College of Sports Medicine (2018). ACSM’s Guidelines for Exercise Testing and Prescription. 10th Edn. Philadelphia: Wolters Kluwer.
Roh, H. T., Cho, S. Y., and So, W. Y. (2020). A Cross-Sectional Study Evaluating the Effects of Resistance Exercise on Inflammation and Neurotrophic Factors in Elderly Women with Obesity. J. Clin. Med. 9:842. doi: 10.3390/jcm9030842
Rojas Vega, S., Knicker, A., Hollmann, W., Bloch, W., and Struder, H. K. (2010). Effect of resistance exercise on serum levels of growth factors in humans. Horm. Metab. Res. 42, 982–986. doi: 10.1055/s-0030-1267950
Rubin, M. R., Kraemer, W. J., Maresh, C. M., Volek, J. S., Ratamess, N. A., Vanheest, J. L., et al. (2005). High-affinity growth hormone binding protein and acute heavy resistance exercise. Med. Sci. Sports Exerc. 37, 395–403. doi: 10.1249/01.mss.0000155402.93987.c0
Skriver, K., Roig, M., Lundbye-Jensen, J., Pingel, J., Helge, J. W., Kiens, B., et al. (2014). Acute exercise improves motor memory: exploring potential biomarkers. Neurobiol. Learn. Mem. 116, 46–58. doi: 10.1016/j.nlm.2014.08.004
Soga, K., Masaki, H., Gerber, M., and Ludyga, S. (2018). Acute and Long-term Effects of Resistance Training on Executive Function. J. Cogn. Enhanc. 2, 200–207. doi: 10.1007/s41465-018-0079-y
Sudo, M., Komiyama, T., Aoyagi, R., Nagamatsu, T., Higaki, Y., and Ando, S. (2017). Executive function after exhaustive exercise. Eur. J. Appl. Physiol. 117, 2029–2038. doi: 10.1007/s00421-017-3692-z
Tsai, C. L., Pan, C. Y., Chen, F. C., Wang, C. H., and Chou, F. Y. (2016). Effects of acute aerobic exercise on a task-switching protocol and brain-derived neurotrophic factor concentrations in young adults with different levels of cardiorespiratory fitness. Exp. Physiol. 101, 836–850. doi: 10.1113/EP085682
Tsai, C. L., Ukropec, J., Ukropcova, B., and Pai, M. C. (2018). An acute bout of aerobic or strength exercise specifically modifies circulating exerkine levels and neurocognitive functions in elderly individuals with mild cognitive impairment. Neuroimage Clin. 17, 272–284. doi: 10.1016/j.nicl.2017.10.028
Tsai, C. L., Wang, C. H., Pan, C. Y., Chen, F. C., Huang, T. H., and Chou, F. Y. (2014). Executive function and endocrinological responses to acute resistance exercise. Front. Behav. Neurosci. 8:262. doi: 10.3389/fnbeh.2014.00262
Tsukamoto, H., Suga, T., Takenaka, S., Tanaka, D., Takeuchi, T., Hamaoka, T., et al. (2016). Repeated high-intensity interval exercise shortens the positive effect on executive function during post-exercise recovery in healthy young males. Physiol. Behav. 160, 26–34. doi: 10.1016/j.physbeh.2016.03.029
Voss, M. W., Nagamatsu, L. S., Liu-Ambrose, T., and Kramer, A. F. (2011). Exercise, brain, and cognition across the life span. J. Appl. Physiol. 111, 1505–1513. doi: 10.1152/japplphysiol.00210.2011
Warburton, D. E., Gledhill, N., Jamnik, V. K., Bredin, S. S., Mckenzie, D. C., Stone, J., et al. (2011). Evidence-based risk assessment and recommendations for physical activity clearance: consensus Document 2011. Appl. Physiol. Nutr. Metab. 36, S266–S298. doi: 10.1139/h11-062
Wilke, J., Giesche, F., Klier, K., Vogt, L., Herrmann, E., and Banzer, W. (2019). Acute Effects of Resistance Exercise on Cognitive Function in Healthy Adults: a Systematic Review with Multilevel Meta-Analysis. Sports Med. 49, 905–916. doi: 10.1007/s40279-019-01085-x
Williams, T. B., Corbett, J., McMorris, T., Young, J. S., Dicks, M., Ando, S., et al. (2019). Cognitive performance is associated with cerebral oxygenation and peripheral oxygen saturation, but not plasma catecholamines, during graded normobaric hypoxia. Exp. Physiol. 104, 1384–1397. doi: 10.1113/EP087647
Winter, B., Breitenstein, C., Mooren, F. C., Voelker, K., Fobker, M., Lechtermann, A., et al. (2007). High impact running improves learning. Neurobiol. Learn. Mem. 87, 597–609. doi: 10.1016/j.nlm.2006.11.003
Keywords: cognition, brain, reaction time, executive function, catecholamines
Citation: Ando S, Komiyama T, Tanoue Y, Sudo M, Costello JT, Uehara Y and Higaki Y (2022) Cognitive Improvement After Aerobic and Resistance Exercise Is Not Associated With Peripheral Biomarkers. Front. Behav. Neurosci. 16:853150. doi: 10.3389/fnbeh.2022.853150
Received: 12 January 2022; Accepted: 21 February 2022;
Published: 15 March 2022.
Edited by:
Chong Chen, Yamaguchi University Graduate School of Medicine, JapanReviewed by:
Zhaowei Kong, University of Macau, Macao SAR, ChinaKazuya Suwabe, Ryutsu Keizai University, Japan
Fabian Herold, German Center for Neurodegeneratives, Helmholtz Association of German Research Centers (HZ), Germany
Copyright © 2022 Ando, Komiyama, Tanoue, Sudo, Costello, Uehara and Higaki. This is an open-access article distributed under the terms of the Creative Commons Attribution License (CC BY). The use, distribution or reproduction in other forums is permitted, provided the original author(s) and the copyright owner(s) are credited and that the original publication in this journal is cited, in accordance with accepted academic practice. No use, distribution or reproduction is permitted which does not comply with these terms.
*Correspondence: Soichi Ando, c29pY2hpLmFuZG9AdWVjLmFjLmpw