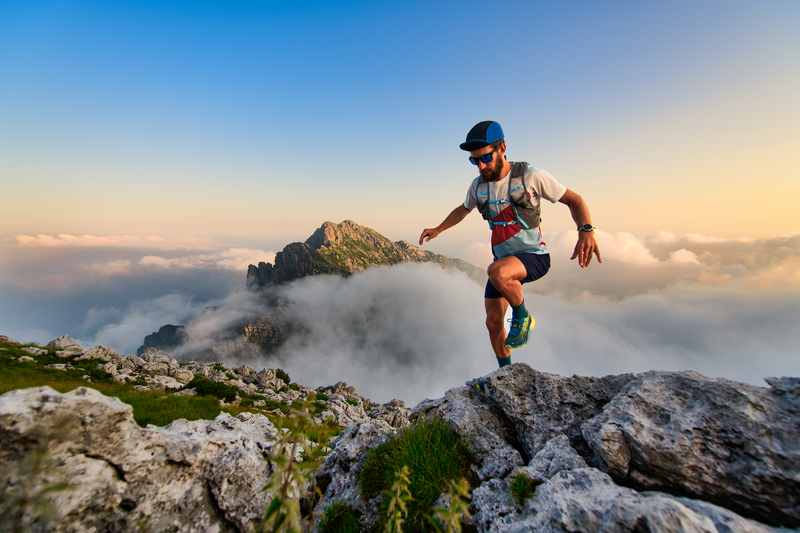
95% of researchers rate our articles as excellent or good
Learn more about the work of our research integrity team to safeguard the quality of each article we publish.
Find out more
ORIGINAL RESEARCH article
Front. Behav. Neurosci. , 02 March 2022
Sec. Emotion Regulation and Processing
Volume 16 - 2022 | https://doi.org/10.3389/fnbeh.2022.789524
This article is part of the Research Topic The Burden of Stress and Depression – New Insight Into Faster and Efficient Treatment View all 14 articles
Accumulating evidence suggests that glutamate clearance plays a critical role in the pathophysiology and treatment of depression. Preclinical and clinical studies have demonstrated that ketamine provides an immediate and sustained antidepressant effect. However, the precise mechanism of its action remains to be elucidated. Glutamate transporter 1 (GLT1) participates in glutamate clearance; therefore, we hypothesized that GLT1 may play an important role in the antidepressant effect of ketamine. In this study, we determined that GLT1 inhibition blocks the antidepressant-like properties of ketamine and alters the phosphorylation of the mammalian target of rapamycin (mTOR) in the prefrontal cortex (PFC). Our results show that pretreatment with dihydrokainic acid (DHK), a GLT1 inhibitor, alleviated the antidepressant-like effect of ketamine, and decreased the level of phosphorylated mTOR (pmTOR) in mice (which is normally upregulated by ketamine). In addition, inhibition of α-amino-3-hydroxy-5-methyl-4-isoxazole-propionic acid (AMPA) receptor and L-type voltage-dependent calcium channel (L-VDCC) significantly abolished the antidepressant-like effect of ketamine. Moreover, inhibition of L-VDCC significantly blocked the upregulation of GLT1 and BDNF in the PFC of mice. The inhibition of the AMPA receptor only significantly alleviated BDNF. Our results provide insight into the role of GLT1 as the critical presynaptic molecule participating in the pathophysiological mechanism of depression and contributing to the antidepressant-like effect of ketamine. In addition, our study confirms that both AMPA receptor and L-VDCC are crucial factors in the immediate antidepressant-like effect of ketamine.
Depression is one of the most prevalent psychiatric disorders, characterized by high incidence and treatment resistance. However, currently available antidepressants have several major drawbacks, such as low response rates and delayed therapeutic effects (Gaynes et al., 2009). The pathophysiology of depression as well as targets of pharmacological treatments have been defined by the monoamine hypothesis of depression for the last decades. Antidepressants aiming at these previously defined targets normally require from 3 to 8 weeks to produce a therapeutic response; however, they affect neurotransmitters immediately (Machado-Vieira et al., 2008). A single sub-anesthetic dose of ketamine, a glutamate N-methyl-D-aspartate (NMDA) receptor antagonist, based on two meta-analyses of randomized placebo-controlled trials (Fond et al., 2014; McGirr et al., 2015), meets the needs of a rapid-acting antidepressant treatment. However, the mechanism underlying this immediate antidepressant effect in animal models remains largely unknown (Kavalali and Monteggia, 2015).
Ketamine has been described as a powerful antagonist of NMDA receptors, however, researchers have shown that the mechanisms underlying this response are likely to be more complex than a selective blockade of NMDA receptors (Naughton et al., 2014; Chaki and Fukumoto, 2015). Glutamatergic systems have been found to play an important role in the rapid antidepressant effect of ketamine (Krystal et al., 2013; Lener et al., 2017; Machado-Vieira et al., 2017). Glial cells regulate glutamatergic systems by clearing glutamate from extracellular space via excitatory amino acid transporter (EAAT). The glutamate is then recycled in the glutamate-glutamine cycle. Without the activity of glutamate transporters, glutamate builds up and kills cells in a process called excitotoxicity. It has been reported that β-lactam antibiotic ceftriaxone increases the uptake of glutamate by upregulating the expression of EAATs, and therefore exerts neuroprotective (Rothstein et al., 2005) and antidepressant effects (Mineur et al., 2007). There are five subtypes of EAATs in humans, as well as rodents. Subtypes EAAT1-2 are found in the membranes of glial cells (Lehre et al., 1995). EAAT2, also known as glutamate transporter 1 (GLT1), is responsible for over 90% of glutamate reuptake in the central nervous system (CNS) (Matsugami et al., 2006; Holmseth et al., 2009; Rao et al., 2015). GLT1 has been reported to play a critical role in the antidepressant effect of ketamine, and its mechanism of action may be associated with brain-derived neurotrophic factor (BDNF)/tropomyosin receptor kinase B (TrkB) signaling (Liu et al., 2016). However, the upstream regulatory mechanism by which GLT1 participates in the antidepressant effect of ketamine remains to be clarified.
In the present study, we examined the effects of GLT1 on the rapid antidepressant-like effect of ketamine in chronic unpredictable mild stress (CUMS) mice and explored the pathways that may participate in the regulation of GLT1 in the prefrontal cortex (PFC) of mice. First, we inhibited the activity of GLT1 with dihydrokainic acid (DHK) in CUMS mice. We then determined the level of mammalian target of rapamycin (mTOR), which is responsible for the rapid action of ketamine (Jernigan et al., 2011). Furthermore, we aimed to clarify the roles of the α-amino-3-hydroxy-5-methyl-4-isoxazolepropionic acid receptor (AMPAR) and L-type voltage-dependent calcium channel (L-VDCC) in the regulation of GLT1. These two proteins participate in the upstream pathways of BDNF as well as TrkB, and have been reported to play an important role in the rapid antidepressant-like effects of ketamine (Duman et al., 2012). Additionally, BDNF and TrkB activation regulated by AMPAR and L-VDCC are required for the rapid antidepressant effects of ketamine (Autry et al., 2011), and BDNF is a potent endogenous activator of mTOR, which has also been suggested to underlie the antidepressant action of ketamine (Li et al., 2010).
The adult male C57BL/6J mice (6–8 weeks) used for the experiment were supplied by the Laboratory Animal Center of the Southern Medical University (Guangzhou, China). The animals were housed in an air-conditioned room at 22 ± 3°C and 60 ± 5% relative humidity under a 12 h light/12 h dark cycle (lights on at 7:00 a.m.) with ad libitum access to food and water. All experiments were carried out in accordance with the principles of the “NIH Guide for the Care and Use of Laboratory Animals” (NIH Publications No. 80–23, revised 1996). The procedures were approved by the Animal Care and Use Committee of the Southern Medical University. Ketamine hydrochloride, NBQX, and DHK used in this work were obtained from Sigma (St. Louis, MO or Shanghai, China). Verapamil was purchased from Aladdin Ltd., (Shanghai, China). All of them were dissolved in saline to the required concentration.
The first aim of the present study was to explore the role of GLT1 in ketamine-induced rapid-acting antidepressant-like effects in CUMS mice. The selective inhibitor of GLT-1, DHK (1.0 μg), or vehicle was microinjected by intracerebroventricular (i.c.v.) injection in mice. Intraperitoneal injection of ketamine (10 mg/kg, i.p) was administered 30 min after the DHK treatment, and then the open field test (OFT) and forced swim test (FST) were conducted successively in the light phase between 8:00 a.m. and 4:00 p.m. in day 1 and 2 (as shown in Figure 1A).
Figure 1. Schedule of drug treatment and behavioral tasks orders. The experiment design of GLT1 inhibition in the rapid antidepressant-like effect of Ketamine (A) and the involvement of AMPAR/L-VDCC in the regulation of Ketamine on GLT1 (B).
In addition, to assess the roles of AMPAR and L-VDCC in the antidepressant-like actions of ketamine, the NBQX (0.5 μg, i.c.v) and Verapamil (5.0 nmol, i.c.v) were pretreatment 30 min before the ketamine administration, respectively, in mice. And then the OFT and FST were conducted successively in the same day (as shown in Figure 1B).
Intracerebroventricular cannulation implantation was performed as described (Li et al., 2017). The stereotaxic coordinates for the lateral ventricle were carried out in accordance with the Paxinos/Franklin mouse atlas (Paxinos and Franklin, 2001). All mice were anesthetized with 2% isoflurane and mounted on a stereotactic frame (R WD Kopf Instruments, Shenzhen, China) with a Kopf model mouse adaptor. Stainless steel guide cannula were implanted in the lateral ventricle at 0.70 mm posterior to the bregma, 1.30 mm lateral to the midline, and 2.00 mm below the skull surface. The guide cannula was anchored to the skull with dental cement and a stainless-steel stylet was inserted to maintain patency for microinjection. Animals were housed individually and allowed 7 days for recovery.
During the microinfusion, the mice were indisposed with a gaseous anesthetic and woke up 10∼20 min after infusion, and could be applied to behavior tests to explore the rapid antidepressant-like effects of ketamine in mice. The infusions were performed via a 10-μl Hamilton microsyringe connected to the microinfusion cannula via 0.26-mm ID polyethylene tubing. Microinfusions were carried out over 5 min with an infusion pump at 1 μL/min, and the cannulas were left in place for 3 additional minutes to avoid backflow. To verify the correct placement of the cannula after i.c.v drug delivery, mice were sacrificed after behavioral tests and cryostat sections of lateral ventricle cut through the cortex determine the cannula track. Only animals with the correct cannula placement were used for further analysis. Mice were sacrificed by dislocated spine method under anesthesia (ether), the tissues used for western blot analysis were collected 6 h after ketamine treatment.
This animal model of stress consists of chronic exposure to variable unpredictable stressors, none of which is sufficient alone to induce long-lasting effects. Briefly, the CUMS procedure (Huang et al., 2017) involved 12 different stressors that were randomly arranged throughout the day and night over 56 consecutive days. The stressors were (1) 24 h of food deprivation, (2) 1 h of exposure to 4°C room (3) 24 h of exposure to a 45° cage tilt, (4) overnight illumination, (5) 24 h of exposure to a wet cage (100 ml of water per individual cage, which is enough to make the sawdust bedding wet), (6) 5 min of swimming in 6∼8°C water, (7) tail clamp for 5 min, (8) 24 h of water deprivation, (9) unpredictable shocks for 5 min (15 mA, one shock/5, 10 s duration), (10) Swimming for 15 min, (11) 4 h of restricted movement, and (12) 4 h of disrupting the cage. The behavioral tests were performed and scored by trained and experienced observers who were blinded to the animals’ conditions.
Briefly, the Open Field Test (OFT) was conducted according to the previous protocols that we recently reported (Li et al., 2017; Lv et al., 2018). The 50 × 50-cm arena with 39-cm high walls is made of a white Plexiglas box. Two black lines were drawn on the floor. Mice were placed into the center of the arena and allowed to explore the apparatus for 5 min. The number of the line crossings and rearings were considered parameters of locomotor activity and recorded over a 5-min period by a digital system.
The FST was conducted in a sound-attenuated room according to the previous studies with minor changes (Wu et al., 2016; Zanos et al., 2016; Lv et al., 2018). Briefly, mice were placed individually for 6 min into a clear plastic cylinder (diameter 10 cm, height 25 cm) containing 10 cm of fresh water, maintained at 23 ± 2°C. The immobility time was recorded over the following 4 min of the 6-min testing duration. The immobility time was defined as time when a mouse floated with only the bare minimum activity necessary to keep their heads above the water.
Frozen PFC tissues in each group (n = 3) of mice were homogenized in ice-cold radio-immunoprecipitation assay (RIPA) lysis buffer containing protease and phosphatase inhibitors cocktail (Pierce Biotechnology, Rockford, IL, United States). Lysates were centrifuged at 12,000 × g for 30 min at 4°C. The protein concentration of each sample lysate was determined with the BCA kit (Thermo Scientific, Rockford, IL). Each sample (25 μg total protein) was separated on 10% SDS polyacrylamide gel electrophoresis (PAGE) gels and transferred to PVDF membranes (0.22 μm; Millipore, CA). Membranes were then incubated with anti-mTOR (1:1,000, Cell Signaling), anti-phospho-mTOR (1:1,000, Cell Signaling), anti-GLT1 (1:1,000, Santa Cruze), BDNF (1:800, Abcam, United States) and anti-GAPDH (1:2,000, Millipore, CA) at 4°C overnight. The membranes were then incubated with Alexa Fluor800-conjugated antibody (1:10,000, Invitrogen, Eugene, OR) for 60 min. Target bands were captured with the fluorescence scanner (Odyssey Infrared Imaging System, LI-COR Biotechnology, Lincoln, NE) and quantified with Image J.
Data are presented as mean ± standard error of the mean (SEM). Statistical analysis of the data was performed using one-way analysis of variance (ANOVA) followed by Tukey’s post-hoc test as appropriate using GraphPad Prism software (Version 5.0, Prism software for PC, GraphPad). Values of P < 0.05 were considered statistically significant.
The CUMS exposure did not alter the locomotor activities of the mice in OFT [F(4, 51) = 1.712, P = 0.1616, Figure 2A]. While, the immobility time of FST was significantly increased by CUMS in day 1 [F(4, 51) = 5.228, P < 0.01, Figure 2B] and day 2 [F(4, 51) = 5.447, P < 0.01, Figure 2C]. A single administration of ketamine (10 mg/kg) significantly reversed these two alterations in CUMS procedure (both P < 0.01). However, the decrease of immobility time produced by ketamine was only significantly blocked by intracerebroventricular (i.c.v.) injection of DHK on day 1 (P < 0.01, Figure 2B), suggesting that GLT1 plays a critical role in the rapid-acting antidepressant-like effects of ketamine in mice. We did not find the antidepressant-like actions of ketamine was abolished by DHK 24 h after infusion in the FST (Day 2, Figure 2C). As shown in Supplementary Figure 1, the different doses of ketamine (3, 10, and 30 mg/kg, i.p) did not significantly change the locomotor activities. However, these Three doses of ketamine produced significant antidepressant-like actions in the FST and TST of mice (Supplementary Figure 2).
Figure 2. The influence of DHK on the rapid antidepressant effect of Ketamine. The crossing of mice in OFT (A); the immobility time of mice in day 1 (B); the immobility time of mice in day 2 (C). The data are expressed as means ± SEM, n = 10–12. **P < 0.01 compared with vehicle + vehicle + non-stressed group; ##P < 0.01 compared with vehicle + vehicle + CUMS group; @@P < 0.01 compared with ketamine + vehicle + CUMS group.
To investigate whether the activation of mTOR produced by ketamine in an animal model of depression was abolished by DHK, the expressions of phosphorylation of mTOR (pmTOR) and total mTOR were determined in the PFC of mice (Figure 3A). As shown in Figure 3B, the CUMS exposure resulted in a significant decrease in protein levels of pmTOR when compared with the control group (P < 0.05). In comparison with the CUMS group, ketamine treatment significantly elevated pmTOR protein expression (P < 0.01). However, pretreatment with DHK, the up-regulation on the ratio of pmTOR in the PFC of mice produced by ketamine was significantly abolished (P < 0.01). Figure 3C showed the changes of mTOR, there was no significant change among all groups [F(4, 10) = 0.1079, P = 0.9770].
Figure 3. The influence of DHK on the mTOR phosphorylation in the PFC of mice. mTOR and pmTOR protein expression were determined by Western blot. Representative western blot (A) and quantification (B,C) of fold changes in the protein levels of pmTOR and mTOR in the PFC of mice. The data are expressed as means ± SEM; n = 3; *P < 0.05 compared with vehicle + vehicle + non-stressed group; ##P < 0.01 compared with vehicle + vehicle + CUMS group; @@P < 0.01 compared with ketamine + vehicle + CUMS group.
Given the growing evidence that AMPAR and L-VDCC may involve in the rapid-acting antidepressants (Duman et al., 2012; Lepack et al., 2014; Zanos et al., 2016), we aimed to explore whether the GLT1 and BDNF were regulated by AMPAR and L-VDCC in the antidepressant-like actions of ketamine in mice. As shown in Figures 4A,C, the locomotor activities of the mice were not changed in the line crossings [NBQX, F(4, 45) = 1.523, P = 0.2115, Figure 4A; Verapamil, F(4, 45) = 0.8936, P = 0.4757, Figure 4C] of OFT by all treatments. However, both pretreatment with NBQX [F(4, 45) = 22.56, P < 0.001, Figure 4B] and verapamil [F(4, 45) = 10.03, P < 0.001, Figure 4D] can completely reversed the antidepressant-like effects of ketamine in the FST of mice. As shown in Supplementary Figure 3. Single treatment with NBQX (microinjection) and verapamil (i.p.) had no effects alone in the OFT and FST of mice.
Figure 4. The roles of AMPAR and L-VDCC played in the rapid antidepressant effect of Ketamine. The crossing of mice in an open field test in AMPAR blocked (A) and L-VDCC inhibition (C); the immobility time of mice in forced swimming test in AMPAR blocked (B) and L-VDCC inhibition (D). The data are expressed as means ± SEM, n = 10. **P < 0.01 compared with vehicle + vehicle + non-stressed group; ##P < 0.01 compared with vehicle + vehicle + CUMS group; @@P < 0.01 compared with ketamine + vehicle + CUMS group.
Growing evidence has shown that the AMPAR and L-VDCC are activated by rapid-acting antidepressants, resulting in the fast release of BDNF and activation of downstream pathways (Lepack et al., 2014; Yao et al., 2017; Yu et al., 2017, 2018; Ghosal et al., 2018). To evaluate the roles of AMPAR and L-VDCC in the regulation of GLT1 and BDNF by ketamine, we analyzed levels of the GLT1 and BDNF by western blot analysis in the PFC of mice (Figure 5A). After 56 days of CUMS exposure, the levels of GLT1 [F(4, 10) = 17.01, P = 0.0002, Figure 5B] and BDNF [F(4, 10) = 13.63, P = 0.0005, Figure 5B] were significantly decreased in the PFC of mice. However, a single treatment with ketamine rapidly and significantly reversed these molecular changes (GLT1, P < 0.01; BDNF, P < 0.01, Figure 5B). In contrast, the levels of BDNF (NBQX, P < 0.05; verapamil, P < 0.001) in the PFC of mice were significantly abolished by pretreatment with verapamil, respectively. Notably, the up-regulation on the GLT1 of ketamine was significantly alleviated by verapamil in the PFC of mice (P < 0.05). Interestingly, pretreatment with NBQX, the up-regulation on the GLT1 by ketamine was not significantly abolished compared with single treatment with ketamine in the PFC of mice.
Figure 5. The roles of AMPAR and L-VDCC in the regulation of Ketamine on GLT1 and BDNF in the PFC of mice. GLT1 and BDNF protein expression were determined by Western blot. Representative western blot (A) and quantification (B) of fold changes in the protein levels of GLT1, and BDNF in the PFC of mice. The data are expressed as means ± SEM; n = 3; **P < 0.01 compared with vehicle + vehicle + non-stressed group; ##P < 0.01 compared with vehicle + vehicle + CUMS group; @P < 0.05, @@P < 0.01 compared with ketamine + vehicle + CUMS group.
A variety of studies in patients have shown that glutamatergic and rodent models dysregulation is involved in depression (Li et al., 2019). GLT1 is responsible for the majority (90%) of extracellular and synaptic glutamate clearance in the CNS. Previous studies have suggested that a decrease in the level of GLT1 in the brain is a possible cause of depression (Cui et al., 2014; Rappeneau et al., 2016). Specifically, infusion of DHK (GLT1 inhibitor) into the brain has been shown to alter the levels of amino acids (Fallgren and Paulsen, 1996) and induce both anxiety and depressive-like symptoms (John et al., 2015; Gasull-Camos et al., 2017a). In the above reports, DHK exhibited its effects within 5–15 min, and biological assays and behavior tests were completed in 24 h, similar to the behavior tests in our study. GLT1 levels in the PFC corresponding to the effect after 48 h of DHK infusion were established by western blot analyses.
In our experiments, GLT1 expression levels in the PFC were significantly decreased following CUMS stimulation and returned to normal by ketamine treatment, which was consistent with the results of previous studies (Choudary et al., 2005; Liu et al., 2016). GLT1 inhibition is known to induce depression-like behaviors (Bechtholt-Gompf et al., 2010), whereas upregulation of GLT1 can have an antidepressant effect (Rothstein et al., 2005; Sanacora et al., 2007; Bechtholt-Gompf et al., 2010; Ding et al., 2017). Our current work confirmed that pretreatment with the GLT1 inhibitor DHK significantly alleviated the rapid antidepressant-like effect of ketamine infusion, which was consistent with the results of previous studies (Choudary et al., 2005; Liu et al., 2016). The decrease in astrocytic Glu uptake due to the decreased level of GLT1 might lead to a shortage of Gln in astrocytes, which is responsible for the release of Glu into the presynaptic neuron, which may support our findings. Our findings may be also confirmed by previous studies in which the infusion of DHK into the brain has been shown to change the levels of amino acids (Fallgren and Paulsen, 1996) and induce both anxiety and depressive-like symptoms (John et al., 2015; Gasull-Camos et al., 2017a).
Activation of the mTOR signaling pathway has been recently shown to be a critical factor in the antidepressant effect of ketamine (Hoeffer and Klann, 2010). The rapid induction of mTOR phosphorylation occurs within 30 min of ketamine administration. This, in turn, leads to the activation of mTOR-dependent protein synthesis (Duman et al., 2012). Here, we assessed changes in the level of the phosphorylated mTOR (pmTOR) after DHK treatment and found that the downregulation of GLT1 significantly inhibited the regulatory effect of ketamine on pmTOR. Therefore, it can be concluded that the regulatory action of ketamine on GLT1 influences mTOR activity. GLT1 as the major glial glutamate transporter is located in the membranes of pre-synaptic astrocytes and is responsible for more than 90% of glutamate uptake.
Glutamate is an important excitatory neurotransmitter in the CNS. This molecule as well as its cognate receptors have been described as new targets for rapid antidepressant action (Dutta et al., 2015; Machado-Vieira et al., 2017). Here, we speculated that GLT1 indirectly influences the level of mTOR phosphorylation through the regulation of glutamate levels during the rapid antidepressant effect of ketamine.
AMPAR and L-VDCC have both been demonstrated to play important roles in the rapid antidepressant effect of ketamine as one of the critical downstream fast-acting factors. This finding has been confirmed by behavior tests in the current study. We also measured BDNF expression levels and found that ketamine regulated the levels of BDNF in CUMS mice. Both NBQX and verapamil pretreatment abolished the effects of ketamine on BDNF, consistent with previous reports (Jourdi et al., 2009; Lepack et al., 2014; Zhou et al., 2014). It is likely that L-VDCC may play a key role in the regulatory effect of ketamine on GLT1. However, the effect of L-VDCC inhibition on the level of GLT1 expression was different from that of AMPAR inhibition. AMPAR antagonist treatment increased GLT1 expression. We believe that this effect may be due to the participation of AMPA in the serotonergic activity in the PFC. It was reported that DHK and S-AMPA microinfusion in IL evoked similar antidepressant-like effects in the FST at 10 min post-administration (Gasull-Camos et al., 2018). Moreover, the GLT1 inhibition has been reported to induce a rapid increase in serotonergic activity in IL, which was blocked by NBQX (Gasull-Camos et al., 2017b). However, in our experiments we only detected the total GLT1 expressed in the PFC (not IL); therefore, further research is needed to clarify the changes in GLT1 expression in the IL after AMPA inhibition. The signaling pathway induced by the communication between glial and neuronal cells is often difficult to study. Data suggest that astrocytes signal to neurons through the Ca2+-dependent release of glutamate (Haydon and Carmignoto, 2006; Fiacco et al., 2009). Additionally, our biochemical studies revealed that the calcium channel blocker verapamil significantly inhibited the effect of ketamine in CUMS mice.
This may be evidence of the influence of calcium channels on GLT1 expression. Growing evidence suggests that the antidepressant-like effects of ketamine and scopolamine in rodent models are caused by an influx of extracellular glutamate, elevated levels of BDNF, and activation of L-VDCC (Lepack et al., 2014; Wohleb et al., 2017; Ghosal et al., 2018; Yu et al., 2018). This may explain why the regulation of GLT1 by ketamine was blocked by t e calcium channel antagonist verapamil.
This study has some limitations. We found that GLT1 expression changed in both the hippocampus and PFC in mice under CUMS. As the PFC is an integral region in the top-down regulation of behavior and control of stress reactivity (Arnsten, 2015), we studied the biological changes in this area as a representation of the brain region. Accumulating evidence indicates that the hippocampus and nucleus accumbens (NAc) are also involved in depression-like phenotypes (Tsankova et al., 2006; Zhang et al., 2014; Yang et al., 2015). Thus, it is necessary to clarify the changes in these areas in future studies.
In conclusion, we showed that the antidepressant-like effect of ketamine on CUMS mice was prevented by GLT1 inhibition and that the regulation of mTOR phosphorylation in the PFC of mice affected the action of GLT1. Our results also indicated that L-VDCC in the PFC may influence the regulatory role of GLT1 in the therapeutic mechanism of ketamine.
The raw data supporting the conclusions of this article will be made available by the authors, without undue reservation.
The animal study was reviewed and approved by the Animal Care and Use Committee of the Southern Medical University.
YC, MS, XL, JX, and CW performed the experiments and article preparation. CW wrote the first draft of the manuscript, which all other authors reviewed. All authors approved publication.
This work was supported by grants from the Natural Science Funds for Distinguished Young Scholar of Zhejiang (LR20H090001), the National Natural Science Foundation of China (82171527), the Education and Research Projects for Young and Middle-aged Teachers of Fujian Educational Department (JAT190237), and the School Supervision Subject of Fujian University of Traditional Chinese Medicine (X2019007-platform). Funding organizations had no role in study design, data collection, and analysis, decision to publish, or preparation of the manuscript.
The authors declare that the research was conducted in the absence of any commercial or financial relationships that could be construed as a potential conflict of interest.
All claims expressed in this article are solely those of the authors and do not necessarily represent those of their affiliated organizations, or those of the publisher, the editors and the reviewers. Any product that may be evaluated in this article, or claim that may be made by its manufacturer, is not guaranteed or endorsed by the publisher.
The Supplementary Material for this article can be found online at: https://www.frontiersin.org/articles/10.3389/fnbeh.2022.789524/full#supplementary-material
Arnsten, A. (2015). Stress weakens prefrontal networks: molecular insults to higher cognition. Nat. Neurosci. 18, 1376–1385. doi: 10.1038/nn.4087
Autry, A. E., Adachi, M., Nosyreva, E., Na, E. S., Los, M. F., Cheng, P.-F., et al. (2011). NMDA receptor blockade at rest triggers rapid behavioural antidepressant responses. Nature 475:91. doi: 10.1038/nature10130
Bechtholt-Gompf, A. J., Walther, H. V., Adams, M. A., Carlezon, W. A. Jr., Ongur, D., and Cohen, B. M. (2010). Blockade of astrocytic glutamate uptake in rats induces signs of anhedonia and impaired spatial memory. Neuropsychopharmacology 35, 2049–2059. doi: 10.1038/npp.2010.74
Chaki, S., and Fukumoto, K. (2015). Potential of glutamate-based drug discovery for next generation antidepressants. Pharmaceuticals 8, 590–606. doi: 10.3390/ph8030590
Choudary, P. V., Molnar, M., Evans, S. J., Tomita, H., Li, J. Z., Vawter, M. P., et al. (2005). Altered cortical glutamatergic and GABAergic signal transmission with glial involvement in depression. Proc. Natl. Acad. Sci. U.S.A. 102, 15653–15658. doi: 10.1073/pnas.0507901102
Cui, W., Mizukami, H., Yanagisawa, M., Aida, T., Nomura, M., Isomura, Y., et al. (2014). Glial dysfunction in the mouse habenula causes depressive-like behaviors and sleep disturbance. J. Neurosci. 34, 16273–16285. doi: 10.1523/JNEUROSCI.1465-14.2014
Ding, X.-F., Li, Y.-H., Chen, J.-X., Sun, L.-J., Jiao, H.-Y., Wang, X.-X., et al. (2017). Involvement of the glutamate/glutamine cycle and glutamate transporter GLT-1 in antidepressant-like effects of Xiao Yao san on chronically stressed mice. BMC Complement. Altern. Med. 17:326. doi: 10.1186/s12906-017-1830-0
Duman, R. S., Li, N., Liu, R. J., Duric, V., and Aghajanian, G. (2012). Signaling pathways underlying the rapid antidepressant actions of ketamine. Neuropharmacology 62, 35–41. doi: 10.1016/j.neuropharm.2011.08.044
Dutta, A., McKie, S., and Deakin, J. F. (2015). Ketamine and other potential glutamate antidepressants. Psychiatry Res. 225, 1–13. doi: 10.1016/j.psychres.2014.10.028
Fallgren, ÅB., and Paulsen, R. E. (1996). A microdialysis study in rat brain of dihydrokainate, a glutamate uptake inhibitor. Neurochem. Res. 21, 19–25. doi: 10.1007/BF02527667
Fiacco, T. A., Agulhon, C., FauMcCarthy, K. D., and McCarthy, K. D. (2009). Sorting out astrocyte physiology from pharmacology. Annu. Rev. Pharmacol. Toxicol. 49, 151–174. doi: 10.1146/annurev.pharmtox.011008.145602
Fond, G., Loundou, A., Rabu, C., Macgregor, A., Lancon, C., Brittner, M., et al. (2014). Ketamine administration in depressive disorders: a systematic review and meta-analysis. Psychopharmacology 231, 3663–3676. doi: 10.1007/s00213-014-3664-5
Gasull-Camos, J., Martinez-Torres, S., Tarres-Gatius, M., Ozaita, A., Artigas, F., and Castane, A. (2018). Serotonergic mechanisms involved in antidepressant-like responses evoked by GLT-1 blockade in rat infralimbic cortex. Neuropharmacology 139, 41–51. doi: 10.1016/j.neuropharm.2018.06.029
Gasull-Camos, J., Soto-Montenegro, M. L., Casquero-Veiga, M., Desco, M., Artigas, F., and Castane, A. (2017a). Differential patterns of subcortical activity evoked by glial GLT-1 blockade in prelimbic and infralimbic cortex: relationship to antidepressant-like effects in rats. Int. J. Neuropsychopharmacol. 20, 988–993. doi: 10.1093/ijnp/pyx067
Gasull-Camos, J., Tarres-Gatius, M., Artigas, F., and Castane, A. (2017b). Glial GLT-1 blockade in infralimbic cortex as a new strategy to evoke rapid antidepressant-like effects in rats. Transl. Psychiatry 7:e1038. doi: 10.1038/tp.2017.7
Gaynes, B. N., Warden, D., Trivedi, M. H., Wisniewski, S. R., Fava, M., and Rush, A. J. (2009). What did STAR*D teach us? Results from a large-scale, practical, clinical trial for patients with depression. Psychiatr. Serv. 60, 1439–1445. doi: 10.1176/ps.2009.60.11.1439
Ghosal, S., Bang, E., Yue, W., Hare, B. D., Lepack, A. E., Girgenti, M. J., et al. (2018). Activity-Dependent brain-derived neurotrophic factor release is required for the rapid antidepressant actions of scopolamine. Biol. Psychiatry 83, 29–37. doi: 10.1016/j.biopsych.2017.06.017
Haydon, P. G., and Carmignoto, G. (2006). Astrocyte control of synaptic transmission and neurovascular coupling. Physiol. Rev. 86, 1009–1031. doi: 10.1152/physrev.00049.2005
Hoeffer, C. A., and Klann, E. (2010). MTOR signaling: at the crossroads of plasticity, memory and disease. Trends Neurosci. 33, 67–75. doi: 10.1016/j.tins.2009.11.003
Holmseth, S., Scott, H. A., Real, K., Lehre, K. P., Leergaard, T. B., Bjaalie, J. G., et al. (2009). The concentrations and distributions of three C-terminal variants of the GLT1 (EAAT2; slc1a2) glutamate transporter protein in rat brain tissue suggest differential regulation. Neuroscience 162, 1055–1071. doi: 10.1016/j.neuroscience.2009.03.048
Huang, P., Dong, Z., Huang, W., Zhou, C., Zhong, W., Hu, P., et al. (2017). Voluntary wheel running ameliorates depression-like behaviors and brain blood oxygen level-dependent signals in chronic unpredictable mild stress mice. Behav. Brain Res. 330, 17–24. doi: 10.1016/j.bbr.2017.05.032
Jernigan, C. S., Goswami, D. B., Austin, M. C., Iyo, A. H., Chandran, A., Stockmeier, C. A., et al. (2011). The mTOR signaling pathway in the prefrontal cortex is compromised in major depressive disorder. Prog. Neuro Psychopharmacol. Biol. psychiatry 35, 1774–1779. doi: 10.1016/j.pnpbp.2011.05.010
John, C. S., Sypek, E. I., Carlezon, W. A., Cohen, B. M., Ongur, D., and Bechtholt, A. J. (2015). Blockade of the GLT-1 transporter in the central nucleus of the amygdala induces both anxiety and depressive-like symptoms. Neuropsychopharmacology 40, 1700–1708. doi: 10.1038/npp.2015.16
Jourdi, H., Hsu, Y. T., Zhou, M., Qin, Q., Bi, X., and Baudry, M. (2009). Positive AMPA receptor modulation rapidly stimulates BDNF release and increases dendritic mRNA translation. J. Neurosci. 29, 8688–8697. doi: 10.1523/JNEUROSCI.6078-08.2009
Kavalali, E. T., and Monteggia, L. M. (2015). How does ketamine elicit a rapid antidepressant response? Curr. Opin. Pharmacol. 20, 35–39. doi: 10.1016/j.coph.2014.11.005
Krystal, J. H., Sanacora, G., and Duman, R. S. (2013). Rapid-acting glutamatergic antidepressants: the path to ketamine and beyond. Biol. Psychiatry 73, 1133–1141. doi: 10.1016/j.biopsych.2013.03.026
Lehre, K. P., Levy, L. M., Ottersen, O. P., Storm-Mathisen, J., and Danbolt, N. C. (1995). Differential expression of two glial glutamate transporters in the rat brain: quantitative and immunocytochemical observations. J. Neurosci. 15(3 Pt 1), 1835–1853. doi: 10.1523/JNEUROSCI.15-03-01835.1995
Lener, M. S., Kadriu, B., and Zarate, C. A. Jr. (2017). Ketamine and beyond: investigations into the potential of glutamatergic agents to treat depression. Drugs 77, 381–401. doi: 10.1007/s40265-017-0702-8
Lepack, A. E., Fuchikami, M., Dwyer, J. M., Banasr, M., and Duman, R. S. (2014). BDNF release is required for the behavioral actions of ketamine. Int. J. Neuropsychopharmacol. 18:33. doi: 10.1093/ijnp/pyu033
Li, C., Li, M., Yu, H., Shen, X., Wang, J., Sun, X., et al. (2017). Neuropeptide VGF C-Terminal peptide TLQP-62 alleviates lipopolysaccharide-induced memory deficits and anxiety-like and depression-like behaviors in mice: the role of BDNF/TrkB signaling. ACS Chem. Neurosci. 8, 2005–2018. doi: 10.1021/acschemneuro.7b00154
Li, M. X., Li, Q., Sun, X. J., Luo, C., Li, Y., Wang, Y. N., et al. (2019). Increased Homer1-mGluR5 mediates chronic stress-induced depressive-like behaviors and glutamatergic dysregulation via activation of PERK-eIF2alpha. Prog. Neuro Psychopharmacol. Biol. Psychiatry 95:109682. doi: 10.1016/j.pnpbp.2019.109682
Li, N., Lee, B., Liu, R. J., Banasr, M., Dwyer, J. M., Iwata, M., et al. (2010). MTOR-dependent synapse formation underlies the rapid antidepressant effects of NMDA antagonists. Science 329, 959–964. doi: 10.1126/science.1190287
Liu, W. X., Wang, J., Xie, Z. M., Xu, N., Zhang, G. F., Jia, M., et al. (2016). Regulation of glutamate transporter 1 via BDNF-TrkB signaling plays a role in the anti-apoptotic and antidepressant effects of ketamine in chronic unpredictable stress model of depression. Psychopharmacology 233, 405–415. doi: 10.1007/s00213-015-4128-2
Lv, D., Chen, Y., Shen, M., Liu, X., Zhang, Y., Xu, J., et al. (2018). Mechanisms underlying the rapid-acting antidepressant-like effects of neuropeptide VGF (non-acronymic) C-terminal peptide TLQP-62. Neuropharmacology 143, 317–326. doi: 10.1016/j.neuropharm.2018.09.046
Machado-Vieira, R., Henter, I. D., and Zarate, C. A. Jr. (2017). New targets for rapid antidepressant action. Prog. Neurobiol. 152, 21–37. doi: 10.1016/j.pneurobio.2015.12.001
Machado-Vieira, R., Salvadore, G., Luckenbaugh, D. A., Manji, H. K., and Zarate, C. A. Jr. (2008). Rapid onset of antidepressant action: a new paradigm in the research and treatment of major depressive disorder. J. Clin. psychiatry 69, 946–958. doi: 10.4088/jcp.v69n0610
Matsugami, T. R., Tanemura, K., Mieda, M., Nakatomi, R., Yamada, K., Kondo, T., et al. (2006). From the cover: indispensability of the glutamate transporters GLAST and GLT1 to brain development. Proc. Natl. Acad. Sci. U.S.A. 103, 12161–12166. doi: 10.1073/pnas.0509144103
McGirr, A., Berlim, M. T., Bond, D. J., Fleck, M. P., Yatham, L. N., and Lam, R. W. (2015). A systematic review and meta-analysis of randomized, double-blind, placebo-controlled trials of ketamine in the rapid treatment of major depressive episodes. Psychol. Med. 45, 693–704. doi: 10.1017/S0033291714001603
Mineur, Y. S., Picciotto, M. R., and Sanacora, G. (2007). Antidepressant-like effects of ceftriaxone in male C57BL/6J mice. Biol. Psychiatry 61, 250–252. doi: 10.1016/j.biopsych.2006.04.037
Naughton, M., Clarke, G., O’Leary, O. F., Cryan, J. F., and Dinan, T. G. (2014). A review of ketamine in affective disorders: current evidence of clinical efficacy, limitations of use and pre-clinical evidence on proposed mechanisms of action. J. Affect. Disord. 156, 24–35. doi: 10.1016/j.jad.2013.11.014
Paxinos, G., and Franklin, K. (2001). The Mouse Brain In Stereotaxic Coordinates. Amsterdam: Elsevier.
Rao, P., Yallapu, M. M., Sari, Y., Fisher, P. B., and Kumar, S. (2015). Designing Novel nanoformulations targeting glutamate transporter excitatory amino acid transporter 2: implications in treating drug addiction. J. Pers. Nanomed. 1, 3–9.
Rappeneau, V., Blaker, A., Petro, J. R., Yamamoto, B. K., and Shimamoto, A. (2016). Disruption of the glutamate-glutamine cycle involving astrocytes in an animal model of depression for males and females. Front. Behav. Neurosci. 10:231. doi: 10.3389/fnbeh.2016.00231
Rothstein, J. D., Patel, S., Regan, M. R., Haenggeli, C., Huang, Y. H., Bergles, D. E., et al. (2005). β-Lactam antibiotics offer neuroprotection by increasing glutamate transporter expression. Nature 433:73. doi: 10.1038/nature03180
Sanacora, G., Kendell, S. F., Levin, Y., Simen, A. A., Fenton, L. R., Coric, V., et al. (2007). Preliminary evidence of riluzole efficacy in antidepressant-treated patients with residual depressive symptoms. Biol. Psychiatry 61, 822–825. doi: 10.1016/j.biopsych.2006.08.037
Tsankova, N. M., Berton, O., Renthal, W., Kumar, A., Neve, R. L., and Nestler, E. J. (2006). Sustained hippocampal chromatin regulation in a mouse model of depression and antidepressant action. Nat. Neurosci. 9, 519–525. doi: 10.1038/nn1659
Wohleb, E. S., Gerhard, D., Thomas, A., and Duman, R. S. (2017). Molecular and cellular mechanisms of rapid-acting antidepressants ketamine and scopolamine. Curr. Neuropharmacol. 15, 11–20. doi: 10.2174/1570159x14666160309114549
Wu, R., Tao, W., Zhang, H., Xue, W., Zou, Z., Wu, H., et al. (2016). Instant and persistent antidepressant response of gardenia yellow pigment is associated with acute protein synthesis and delayed upregulation of BDNF expression in the hippocampus. ACS Chem. Neurosci. 7, 1068–1076. doi: 10.1021/acschemneuro.6b00011
Yang, C., Shirayama, Y., Zhang, J. C., Ren, Q., and Hashimoto, K. (2015). Regional differences in brain-derived neurotrophic factor levels and dendritic spine density confer resilience to inescapable stress. Int. J. Neuropsychopharmacol. 18:yu121. doi: 10.1093/ijnp/pyu121
Yao, N., Skiteva, O., Zhang, X., Svenningsson, P., and Chergui, K. (2017). Ketamine and its metabolite (2R,6R)-hydroxynorketamine induce lasting alterations in glutamatergic synaptic plasticity in the mesolimbic circuit. Mol. Psychiatry 23, 2066–2077. doi: 10.1038/mp.2017.239
Yu, H., Li, M., Shen, X., Lv, D., Sun, X., Wang, J., et al. (2018). The requirement of L-Type voltage-dependent calcium channel (L-VDCC) in the rapid-acting antidepressant-like effects of scopolamine in mice. Int. J. Neuropsychopharmacol. 21, 175–186. doi: 10.1093/ijnp/pyx080
Yu, H., Li, M., Zhou, D., Lv, D., Liao, Q., Lou, Z., et al. (2017). Vesicular glutamate transporter 1 (VGLUT1)-mediated glutamate release and membrane GluA1 activation is involved in the rapid antidepressant-like effects of scopolamine in mice. Neuropharmacology 131, 209–222. doi: 10.1016/j.neuropharm.2017.12.028
Zanos, P., Moaddel, R., Morris, P. J., Georgiou, P., Fischell, J., Elmer, G. I., et al. (2016). NMDAR inhibition-independent antidepressant actions of ketamine metabolites. Nature 533, 481–486. doi: 10.1038/nature17998
Zhang, J. C., Wu, J., Fujita, Y., Yao, W., Ren, Q., Yang, C., et al. (2014). Antidepressant effects of TrkB ligands on depression-like behavior and dendritic changes in mice after inflammation. Int. J. Neuropsychopharmacol. 18:77. doi: 10.1093/ijnp/pyu077
Keywords: glutamate transporter 1, depression, ketamine, α-Amino-3-hydroxy-5-methyl-4-isoxazolepropionic acid receptor, L-type voltage-dependent calcium channel
Citation: Chen Y, Shen M, Liu X, Xu J and Wang C (2022) The Regulation of Glutamate Transporter 1 in the Rapid Antidepressant-Like Effect of Ketamine in Mice. Front. Behav. Neurosci. 16:789524. doi: 10.3389/fnbeh.2022.789524
Received: 05 October 2021; Accepted: 17 January 2022;
Published: 02 March 2022.
Edited by:
Alon Shamir, Mazor Mental Health Center, IsraelReviewed by:
Qi-Gang Zhou, Nanjing Medical University, ChinaCopyright © 2022 Chen, Shen, Liu, Xu and Wang. This is an open-access article distributed under the terms of the Creative Commons Attribution License (CC BY). The use, distribution or reproduction in other forums is permitted, provided the original author(s) and the copyright owner(s) are credited and that the original publication in this journal is cited, in accordance with accepted academic practice. No use, distribution or reproduction is permitted which does not comply with these terms.
*Correspondence: Jiangping Xu, anB4QHNtdS5lZHUuY24=, orcid.org/0000-0002-0447-9229; Chuang Wang, d2FuZ2NodWFuZ0BuYnUuZWR1LmNu, d2FuZ2xvdmVjaHVhbmdAMTYzLmNvbQ==, orcid.org/0000-0002-3816-230X
†These authors have contributed equally to this work
Disclaimer: All claims expressed in this article are solely those of the authors and do not necessarily represent those of their affiliated organizations, or those of the publisher, the editors and the reviewers. Any product that may be evaluated in this article or claim that may be made by its manufacturer is not guaranteed or endorsed by the publisher.
Research integrity at Frontiers
Learn more about the work of our research integrity team to safeguard the quality of each article we publish.