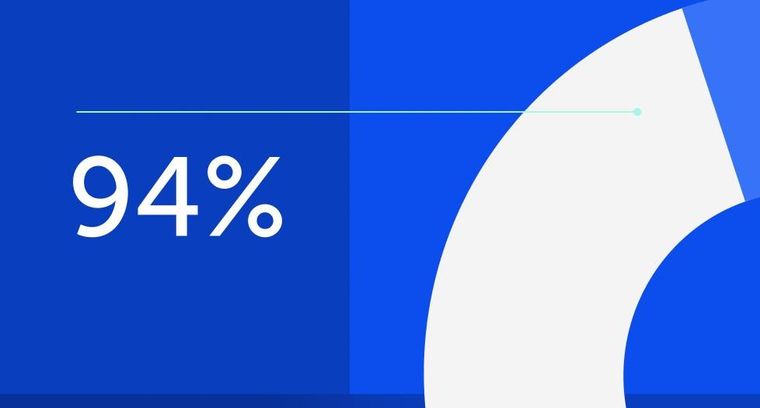
94% of researchers rate our articles as excellent or good
Learn more about the work of our research integrity team to safeguard the quality of each article we publish.
Find out more
MINI REVIEW article
Front. Behav. Neurosci., 12 January 2023
Sec. Learning and Memory
Volume 16 - 2022 | https://doi.org/10.3389/fnbeh.2022.1082158
This article is part of the Research TopicUpdates on Memory Modulation in Health and DiseaseView all 5 articles
Whenever we navigate through different contexts, we build a cognitive map: an internal representation of the territory. Spatial navigation is a complex skill that involves multiple types of information processing and integration. Place cells and grid cells, collectively with other hippocampal and medial entorhinal cortex neurons (MEC), form a neural network whose activity is critical for the representation of self-position and orientation along with spatial memory retrieval. Furthermore, this activity generates new representations adapting to changes in the environment. Though there is a normal decline in spatial memory related to aging, this is dramatically increased in pathological conditions such as Alzheimer’s disease (AD). AD is a multi-factorial neurodegenerative disorder affecting mainly the hippocampus-entorhinal cortex (HP-EC) circuit. Consequently, the initial stages of the disease have disorientation and wandering behavior as two of its hallmarks. Recent electrophysiological studies have linked spatial memory deficits to difficulties in spatial information encoding. Here we will discuss map impairment and remapping disruption in the HP-EC network, as a possible circuit mechanism involved in the spatial memory and navigation deficits observed in AD, pointing out the benefits of virtual reality as a tool for early diagnosis and rehabilitation.
The passing of time affects the body, and it also has effects on the brain, these being highly variable among individuals. In particular, memory loss becomes more frequent with aging but this will not translate into dramatic changes in the life quality of most people. However, a part of the population suffers typical age-related cognitive deficits and others might experience pathological impairments such as mild cognitive impairment, or Alzheimer’s disease (AD) (Grundman et al., 2004; Hort et al., 2007; Wilson et al., 2011; Boccia et al., 2016). AD is a multi-factorial disease characterized by the progressive deterioration of many cognitive skills (Wilson et al., 2011). Though its onset and development might differ between individuals, it has three associated abnormalities: brain volume reduction (Du et al., 2007), pathological accumulation of amyloid plaques (Zhang et al., 2022), and hyperphosphorylated tau protein neurofibrillary tangles (Armstrong, 2009).
In AD patients, there is amyloid-β (Aβ) plaque deposition derived from the amyloid precursor protein (APP), which may progressively lead to tau pathology and the deterioration of brain circuit functions and cellular degeneration over several years (Sasaguri et al., 2017). The accumulation of Aβ generates high-toxicity Aβ soluble oligomers, which then aggregate into plaques of fibrillar amyloid (Drummond and Wisniewski, 2017).
Besides, in AD and the aging brain, tau proteins are hyperphosphorylated and abnormally folded. In normal physiological conditions, tau proteins stabilize the microtubules in the axon, contributing to axonal transport, development, and growth (Barbier et al., 2019). The loss of tau function is coupled with increased aggregation properties (Avila et al., 2004; DeTure and Dickson, 2019) and abnormal tau is linked to the spreading of tau pathology throughout the brain in AD (DeTure and Dickson, 2019).
The clinical diagnosis of AD is based on an observable cognitive decline and complementary PET scans which correlate with amyloid plaque deposition. While amyloid plaque deposition is one of the characteristics that define this pathology, there is a proportion of the elder population (from 5% in the 50–60 years old group up to 50% in the 70–80 years old group) with elevated plaque levels that show no cognitive impairment. For this reason, it is fundamental that the PET scan is interpreted in an adequate clinical context (Marcus et al., 2014). Finally, the definite confirmation of this pathology requires the post-mortem assessment of both amyloid plaques and tau neurofibrillary tangles (Drummond and Wisniewski, 2017).
To assess cognitive decline in AD, common clinical evaluations include tests of episodic memory, executive function, and general orientation and cognition (Rajan et al., 2015). Learning and memory are central topics in cognitive aging and AD research, yet fewer studies have investigated changes in spatial navigation (Lester et al., 2017). Remarkably, spatial disorientation is one of the first landmarks that lead to AD diagnosis. In fact, more than 60% of AD patients suffer from spatial memory deficits and wandering behaviors (Hope et al., 1994).
Here we will discuss evidence from transgenic rodent AD models with altered firing properties of hippocampal and entorhinal neurons that lead to disrupted cognitive maps and, in consequence, spatial memory deficits. Besides, we will introduce findings derived from the use of virtual reality in humans highlighting its potential use as a tool to enable an earlier diagnosis of AD or for rehabilitation.
The ability to navigate an environment depends on the processing of several modalities of information. It requires the interpretation of multi-sensorial inputs and the generation of an internal representation of the space based on the animal’s prior knowledge. This was called “cognitive map” and it was first introduced by Tolman (1940). Tolman’s work had an influential value when single neuron activity with map-like representations was discovered in the hippocampal-entorhinal circuit (HP-EC) (Moser et al., 2015). The HP-EC contains a variety of neurons encoding different aspects of space crucial for its representation and for navigating through it. Hippocampal place cells are one of the best-known of all of them, having a preferential firing in precise coordinates of space (O’Keefe and Nadel, 1978). Grid cells of the MEC fire at several coordinates following a regular grid of triangles covering the entire surface of an environment (Hafting et al., 2005). This grid pattern of activity supports the representation of the distance between spatial locations, providing a spatial metric for the cognitive map. There is a vast diversity of other functional cell types in the HP-EC with a map-like organization: band cells (Krupic et al., 2012), head direction cells (Taube et al., 1990), border cells (Solstad et al., 2008; Lever et al., 2009), object cells (Høydal et al., 2019), speed cells (Kropff et al., 2015; Hinman et al., 2016), goal cells (Hok et al., 2007), reward cells (Gauthier and Tank, 2018), and even cells that encode conspecific location on the map (Danjo et al., 2018; Omer et al., 2018). The existence of all these functional types of neurons with map-like computations supports the idea that the HP-EC circuit could form the cognitive map necessary for navigation through space and daily experiences.
Under some conditions, associative memory networks need to decorrelate overlapping inputs before storing them. In response to different contexts, place cells and grid cells can change their activity, a process called remapping (Leutgeb et al., 2005). By remapping, they create specific representations for different environments. When animals explore markedly different contexts, place cells and grid cells undergo a complete reorganization of their activity (global remapping) (Muller and Kubie, 1987; Hafting et al., 2005). In response to subtle changes, place cells maintain their place code but alter their firing rate (rate remapping) (Leutgeb et al., 2005). In the HP-EC circuit, remapping could be the process that enables the neuronal pattern separation of overlapping events (Colgin et al., 2008).
For the encoding of the environment, grid cells and place cells need a reference to external landmarks. This association is likely formed during the initial visit to the territory. If the environment is modified, the grid cells reorganize their fields to encode the new dimensions (Wernle et al., 2018). Hippocampal place cells can flexibly encode changes in the environment (e.g., the addition of barriers or spatial compartmentalization), however, they do not remap after changes in the connectivity between compartments (Duvelle et al., 2021).
Place cells were also described in humans, with electrode recordings of the frontal and medial temporal lobes of patients while they performed a virtual-navigation task. These cells displayed responses to specific locations and views of different landmarks associated with goals within a task (Ekstrom et al., 2003). Path cells were described in electrode recordings of the EC of presurgical epilepsy patients (Jacobs et al., 2010). Moreover, grid-like activity was observed in fMRI recordings of the EC of patients performing a virtual reality (VR) navigation task analog to rodents’ foraging tasks (Doeller et al., 2010). Not only this hexagonally distributed fMRI signal is present when subjects actively navigate a virtual environment but also when they imagine moving through it (Horner et al., 2016).
In humans, grid-like representations that support spatial memory were found in the HP-EC system, which is associated with autobiographical memory and imagery (Doeller et al., 2010; Bellmund et al., 2016; Horner et al., 2016) and in the EC and medial prefrontal cortex in the processing of odor landscape (Bao et al., 2019). Grid-like activity was also found in goal-directed tasks not related to spatial navigation, where abstract non-spatial relationships were coded into maps resembling the ones used for spatial navigation (Garvert et al., 2017). Given that a cognitive map is a way of organizing information to build an internal representation of the environment, it is highly likely that grid coding underlies other cognitive processes besides topographical representations (Ekstrom et al., 2020; Peer et al., 2021).
In sum, the neurons in the HP-EC circuit can encode multiple aspects of an experience besides the position in space, suggesting that they have a crucial role not only in spatial navigation but also in episodic memories. Further, they exhibit pattern separation/completion activity that allows spatial disambiguation between similar memories, which in turn enables the successful retrieval of mnemonic traces (Kyle et al., 2015). Hence, they are a unique target to study spatial memory impairment and wandering behavior that AD patients suffer.
The histopathological post-mortem analysis of AD patients shows that amyloid plaques and tau pathology are present together, however, these are less commonly found in AD models because of the technical limitations to obtaining consistent and abundant expression of both plaques and tangles (Drummond and Wisniewski, 2017). No animal models can fully recapitulate AD, nevertheless, they allow a better understanding of the mechanisms that underlie navigation impairment, disorientation, and wandering. Recent findings in transgenic mice with amyloid plaques or neurofibrillary tangles in the HP-EC circuit show deficits in place-cell and grid-cell activity, leading to altered cognitive maps and difficulties in solving spatial tasks, pointing to these neurons as key players in the navigational and memory decline observed in AD (For summary, see Table 1).
Place cell abnormalities have been reported in different mouse models of AD. rTg4510 mice (7–8 months) with advanced tau pathology and progressive neurodegeneration showed disrupted place cell coding. Although place cells did not show place specificity, they displayed robust firing sequences. However, these sequences were similar among different environments, suggesting that they lost their ability to represent the animal’s current surroundings (Cheng and Ji, 2013). Further work with this model showed spatial memory deficits accompanied by alterations in the intrinsic properties of CA1 pyramidal neurons leading to aberrant CA1 network oscillations and altered place cell firing properties (Booth et al., 2016).
Disturbed CA1 local oscillatory activity and impaired place coding were also described in the APP-PS1 model. Six months APP-PS1 mice have increased amounts of soluble and insoluble Aβ and show degraded spatial information processing and inflexible representations in their place cells during reversal learning in an operant conditioning task (Cayzac et al., 2015). At 11 months Aβ-expressing APP mice did not improve their place cell coding through learning, producing spatial codes of lower resolution that correlate with delayed learning (Zhao et al., 2014). Similarly, place cells with a degraded neuronal representation of the environment were also reported in aged – but not in young – Tg2576 mice and were associated with deficits in spatial memory tasks (Cacucci et al., 2008). In accordance with these findings, 3xTg mice develop a progressive accumulation of plaques and tangles, and show disrupted coordination of place cell firing by hippocampal theta and gamma rhythms (Mably et al., 2017). Theta and gamma rhythms are involved in many hippocampal cognitive processes, such as encoding and retrieval of episodic memories (Vertes et al., 2004; Nyhus and Curran, 2010). Network dysfunctions have been proposed to underlie some of the fluctuations in neurological functions observed in AD (Palop et al., 2006). Accordingly, theta and gamma dysfunctions were observed in several AD models, being reduced gamma and theta power a common feature among them (Villette et al., 2010; Rubio et al., 2012; Ittner et al., 2014; Schneider et al., 2014; Gillespie et al., 2016; Iaccarino et al., 2016).
Unimpaired grid coding in the HP-EC circuit is necessary for path integration in spatial navigation. The ablation of NMDA glutamate receptors in the retro-hippocampal region reduced spatial selectivity and grid spatial periodicity of the MEC principal cells (Gil et al., 2018). Likewise, mice lacking GluA1-containing AMPA receptors of the MEC showed difficulties in a homing task when allocentric cues were reduced (Allen et al., 2014). Furthermore, in transgenic models of tau pathology, excitatory neuronal loss and grid cell dysfunction in the MEC were associated with poor spatial coding. In 30-month-old EC-tau transgenic mice, accumulated tangles altered the network properties: grid cell firing rate was reduced, grid fields were destabilized, spatial information content was diminished and theta power was enhanced. At the stage where these alterations appeared, mice showed deficits in spatial learning and memory tasks, such as Morris Water Maze and T-maze test (Fu et al., 2017). Remarkably, the disturbances were observed in dorsal MEC, following previous work that showed that dorsal MEC, and not ventral, is preferentially vulnerable to pathological tau (Booth et al., 2016).
Grid coding was impaired in APP-KI and J20 APP mice lines expressing Aβ protein in the hippocampus and the MEC. Spatial memory deficits are observed in >6 months male and female mice and, though they present a mild deterioration in their place cells’ activity, the most adverse effect is observed in the grid cells of the MEC and it has an earlier onset (Jun et al., 2020; Ying et al., 2022). In particular, APP-KI mice had impairments in remapping that emerged first in grid cells and then in place cells. As with the amyloid models, in the tauopathy model, rTg4510 mice lost grid cell periodicity and, as a result, exhibited impaired path integration. Hippocampal place cell coding was less affected than the grid cell coding of the MEC (Ridler et al., 2020).
Remapping deficits were also observed in a mice model of familial AD (APPSwe + PSEN1/ΔE9). Pattern separation and pattern completion were affected in a regional way, where pattern separation was reduced in the dentate gyrus, and pattern completion was reduced in the CA3 region (Rechnitz et al., 2021). Although it is not clear whether deficits in CA3 are a consequence of corrupted inputs (from the DG or EC) or arise as an independent regional deficit, these results suggest a network-level information processing impairment.
TgF344 female rats with increased gliosis, amyloid plaques, and neurofibrillary tangles showed working and reference memory impairments in the radial-arm maze and Morris Water maze following a linear development (6, 9, and 12 months). Remarkably, the 9 months group conserved its working memory and had a distinctive correlation between physiological and brain outcomes, putting forward that critical processes might be taking place at this intermediate point in the course of the disease (Bernaud et al., 2022). Another work with this model showed that the behavioral deficits observed at 12 months were accompanied by disrupted place cell coding (Galloway et al., 2018), in consonance with evidence in mice models.
Together, these results show that place cell and grid cell perturbations are a common feature of the vast majority of AD models and, in most cases, they are accompanied by a decline in spatial navigation and memory performance. Interestingly, in mice models where both place and grid aberrant activity was reported, the most adverse effects were found in grid cells. Moreover, the effect on grid cells had an earlier onset, resembling what has been described in patients (Braak and Braak, 1991). Overall, evidence from transgenic models suggests that hippocampal place cells can form place fields without entorhinal cortex input in these AD models. Thus, deficient place representation could first arise from the lack of the allocentric reference provided by grid coding and, as the pathology progresses, place cell coding becomes disrupted too. In humans, the deterioration of EC activity could be at the basis of spatial disorientation and wandering.
The use of virtual navigation tasks alongside fMRI enabled the discovery of grid-like activity in humans (Ekstrom et al., 2003). VR correlates highly with real-world navigation (Puthusseryppady et al., 2020) and allows the representation of large areas into a smaller space. Though that VR lacks vestibular and proprioceptive activity, visual, planning, and memory systems are engaged in these types of tasks (Epstein et al., 2017).
Younger and older adults show different navigational skills in a VR homing task, which could be driven by increased noise in spatial representations in the HP-EC network (Bates and Wolbers, 2014). Using fMRI and VR, Kunz et al. (2015) studied neural correlates of spatial navigation in the EC in control study participants and individuals at risk of developing AD. This group showed reduced grid-cell–like activity and altered navigation many decades before the onset of the disease. Moreover, in an immersive VR Object-Location test, patients at risk of developing AD had poorer performance than their age-matching controls, making it a potential tool for the diagnosis of the development of the disease (Castegnaro et al., 2022). Accordingly, AD patients showed impairments in VR tasks designed to study navigational skills, however, their performance could not be used to predict the disorientation degree they may have in the real world (Coughlan et al., 2020).
Virtual reality offers a controlled secure environment with multimodal stimulation that can be standardized and used for navigation and memory assessment in elder patients. As it allows repetitive practice, feedback on the performance, and the incorporation of different stimuli VR also opens up the possibility of generating rehabilitating tools to improve spatial memories and navigational skills (Serino and Riva, 2013; Colombo et al., 2017). Thus, VR represents a unique tool that could be used for early diagnosis and rehabilitation as well.
The earliest stages of AD are characterized by the formation of mature tangles in the EC and are accompanied by disorientation and confusion when navigating familiar places. Several mechanisms contribute to the deterioration of the HP-MEC circuit in AD. In pathological conditions, excessive amyloid plaque deposition and neurofibrillary tangles affect neuronal metabolism, axonal transport, and growth, and increase neurotoxicity and inflammation, among other phenomena (Domínguez-Álvaro et al., 2021; Kobro-Flatmoen et al., 2021). Though AD models may not reproduce the pathological development in its entirety, they bring extremely valuable information on the evolution of the disease. For instance, both clinical evidence and transgenic models show that damage to the grid cells in the MEC is detrimental to navigation and it starts earlier than in the hippocampal place cells (Jun et al., 2020; Richter et al., 2020; Ying et al., 2022). Remarkably, MEC’s neurons are among the first to exhibit degeneration as shown by anatomical studies in AD patients (Van Hoesen et al., 1991; Gómez-Isla et al., 1996).
Grid coding has a key role in cognitive map formation. Cognitive maps are representational systems that allow navigating the environment but also physical, social, and conceptual spaces. Given all these characteristics, the impairment in cognitive maps has multiple profound effects on the lives of AD patients. The development of biomarkers (Holbrook et al., 2020; Richter et al., 2020; Matuskova et al., 2021; Billette et al., 2022) together with cognitive (Vlcek and Laczo, 2014) and VR tests (Bates and Wolbers, 2014; Coughlan et al., 2018, 2020; Castegnaro et al., 2022) that can detect early changes in the MEC could be crucial for the prevention and early diagnosis of AD. Considering spatial navigation is less attached to verbal, cultural, and educational biases than other tests (Coughlan et al., 2018) and the possibility to translate VR to different locations, there is a unique window of opportunity toward a global approach in the diagnostic and treatment of AD.
Both authors listed have made a substantial, direct, and intellectual contribution to the work, and approved it for publication.
This work was funded with research grants from Fondo para la Investigación Científica y Tecnológica (FONCYT), PICT 2017-2465, granted to MM.
The authors declare that the research was conducted in the absence of any commercial or financial relationships that could be construed as a potential conflict of interest.
All claims expressed in this article are solely those of the authors and do not necessarily represent those of their affiliated organizations, or those of the publisher, the editors and the reviewers. Any product that may be evaluated in this article, or claim that may be made by its manufacturer, is not guaranteed or endorsed by the publisher.
Allen, K., Gil, M., Resnik, E., Toader, O., Seeburg, P., and Monyer, H. (2014). Impaired path integration and grid cell spatial periodicity in mice lacking GluA1-containing AMPA receptors. J. Neurosci. 34, 6245–6259. doi: 10.1523/JNEUROSCI.4330-13.2014
Armstrong, R. (2009). The molecular biology of senile plaques and neurofibrillary tangles in Alzheimer’s disease. Folia Neuropathol. 47, 289–299.
Avila, J., Lucas, J. J., Perez, M., and Hernandez, F. (2004). Role of tau protein in both physiological and pathological conditions. Physiol. Rev. 84, 361–384. doi: 10.1152/physrev.00024.2003
Bao, X., Gjorgieva, E., Shanahan, L. K., Howard, J. D., Kahnt, T., and Gottfried, J. A. (2019). Grid-like neural representations support olfactory navigation of a two-dimensional odor space. Neuron 102, 1066–1075.e5. doi: 10.1016/j.neuron.2019.03.034
Barbier, P., Zejneli, O., Martinho, M., Lasorsa, A., Belle, V., Smet-Nocca, C., et al. (2019). Role of tau as a microtubule-associated protein: Structural and functional aspects. Front. Aging Neurosci. 11:204. doi: 10.3389/fnagi.2019.00204
Bates, S. L., and Wolbers, T. (2014). How cognitive aging affects multisensory integration of navigational cues. Neurobiol. Aging 35, 2761–2769. doi: 10.1016/j.neurobiolaging.2014.04.003
Bellmund, J. L., Deuker, L., Navarro Schröder, T., and Doeller, C. F. (2016). Grid-cell representations in mental simulation. Elife 5:e17089. doi: 10.7554/eLife.17089
Bernaud, V. E., Bulen, H. L., Peña, V. L., Koebele, S. V., Northup-Smith, S. N., Manzo, A. A., et al. (2022). Task-dependent learning and memory deficits in the TgF344-AD rat model of Alzheimer’s disease: Three key timepoints through middle-age in females. Sci. Rep. 12:14596. doi: 10.1038/s41598-022-18415-1
Billette, O. V., Ziegler, G., Aruci, M., Schütze, H., Kizilirmak, J., Richter, A., et al. (2022). Novelty-Related fMRI responses of precuneus and medial temporal regions in individuals at risk for Alzheimer disease. Neurology 99, e775–e788. doi: 10.1212/WNL.0000000000200667
Boccia, M., Silveri, M. C., Sabatini, U., Guariglia, C., and Nemmi, F. (2016). Neural underpinnings of the decline of topographical memory in mild cognitive impairment. Am. J. Alzheimer’s Dis. Other Dement. 31, 618–630. doi: 10.1177/1533317516654757
Booth, C. A., Witton, J., Nowacki, J., Tsaneva-Atanasova, K., Jones, M. W., Randall, A. D., et al. (2016). Altered intrinsic pyramidal neuron properties and pathway-specific synaptic dysfunction underlie aberrant hippocampal network function in a mouse model of tauopathy. J. Neurosci. 36, 350–363. doi: 10.1523/JNEUROSCI.2151-15.2016
Braak, H., and Braak, E. (1991). Neuropathological stageing of Alzheimer-related changes. Acta Neuropathol. 82, 239–259. doi: 10.1007/BF00308809
Cacucci, F., Yi, M., Wills, T. J., Chapman, P., and O’Keefe, J. (2008). Place cell firing correlates with memory deficits and amyloid plaque burden in Tg2576 Alzheimer mouse model. Proc. Natl. Acad. Sci. U.S.A. 105, 7863–7868. doi: 10.1073/pnas.0802908105
Castegnaro, A., Howett, D., Li, A., Harding, E., Chan, D., Burgess, N., et al. (2022). Assessing mild cognitive impairment using object-location memory in immersive virtual environments. Hippocampus 32, 660–678. doi: 10.1002/hipo.23458
Cayzac, S., Mons, N., Ginguay, A., Allinquant, B., Jeantet, Y., and Cho, Y. H. (2015). Altered hippocampal information coding and network synchrony in APP-PS1 mice. Neurobiol. Aging 36, 3200–3213. doi: 10.1016/j.neurobiolaging.2015.08.023
Cheng, J., and Ji, D. (2013). Rigid firing sequences undermine spatial memory codes in a neurodegenerative mouse model. Elife 2:e00647. doi: 10.7554/eLife.00647
Colgin, L. L., Moser, E. I., and Moser, M.-B. (2008). Understanding memory through hippocampal remapping. Trends Neurosci. 31, 469–477. doi: 10.1016/j.tins.2008.06.008
Colombo, D., Serino, S., Tuena, C., Pedroli, E., Dakanalis, A., Cipresso, P., et al. (2017). Egocentric and allocentric spatial reference frames in aging: A systematic review. Neurosci. Biobehav. Rev. 80, 605–621. doi: 10.1016/j.neubiorev.2017.07.012
Coughlan, G., Laczó, J., Hort, J., Minihane, A. M., and Hornberger, M. (2018). Spatial navigation deficits - overlooked cognitive marker for preclinical Alzheimer disease? Nat. Rev. Neurol. 14, 496–506. doi: 10.1038/s41582-018-0031-x
Coughlan, G., Puthusseryppady, V., Lowry, E., Gillings, R., Spiers, H., Minihane, A. M., et al. (2020). Test-retest reliability of spatial navigation in adults at-risk of Alzheimer’s disease. PLoS One 15:e0239077. doi: 10.1371/journal.pone.0239077
Danjo, T., Toyoizumi, T., and Fujisawa, S. (2018). Spatial representations of self and other in the hippocampus. Science 359, 213–218. doi: 10.1126/science.aao3898
DeTure, M. A., and Dickson, D. W. (2019). The neuropathological diagnosis of Alzheimer’s disease. Mol. Neurodegener. 14:32. doi: 10.1186/s13024-019-0333-5
Doeller, C. F., Barry, C., and Burgess, N. (2010). Evidence for grid cells in a human memory network. Nature 463, 657–661. doi: 10.1038/nature08704
Domínguez-Álvaro, M., Montero-Crespo, M., Blazquez-Llorca, L., Plaza-Alonso, S., Cano-Astorga, N., DeFelipe, J., et al. (2021). 3D Analysis of the synaptic organization in the entorhinal cortex in Alzheimer’s disease. eNeuro 8, ENEURO.504–ENEURO.520. doi: 10.1523/ENEURO.0504-20.2021
Drummond, E., and Wisniewski, T. (2017). Alzheimer’s disease: Experimental models and reality. Acta Neuropathol. 133, 155–175. doi: 10.1007/s00401-016-1662-x
Du, A., Schuff, N., Kramer, J., Rosen, H., Gorno-Tempini, M., Rankin, K., et al. (2007). Different regional patterns of cortical thinning in Alzheimer’s disease and frontotemporal dementia. Brain 130(Pt 4) 1159–1166. doi: 10.1093/brain/awm016
Duvelle, É, Grieves, R., Liu, A., Jedidi-Ayoub, S., Holeniewska, J., Harris, A., et al. (2021). Hippocampal place cells encode global location but not connectivity in a complex space. Curr. Biol. 31, 1221-1233.e9. doi: 10.1016/j.cub.2021.01.005
Ekstrom, A. D., Harootonian, S. K., and Huffman, D. J. (2020). Grid coding, spatial representation, and navigation: Should we assume an isomorphism?. Hippocampus 30, 422–432. doi: 10.1002/hipo.23175
Ekstrom, A., Kahana, M., Caplan, J., Fields, T., Isham, E., Newman, E., et al. (2003). Cellular networks underlying human spatial navigation. Nature 425, 184–188. doi: 10.1038/nature01964
Epstein, R. A., Patai, E. Z., Julian, J. B., and Spiers, H. J. (2017). The cognitive map in humans: Spatial navigation and beyond. Nat. Neurosci. 20, 1504–1513. doi: 10.1038/nn.4656
Fu, H., Rodriguez, G. A., Herman, M., Emrani, S., Nahmani, E., Barrett, G., et al. (2017). Tau pathology induces excitatory neuron loss, grid cell dysfunction, and spatial memory deficits reminiscent of early Alzheimer’s disease. Neuron 93, 533.e–541.e. doi: 10.1016/j.neuron.2016.12.023
Galloway, C. R., Ravipati, K., Singh, S., Lebois, E. P., Cohen, R. M., Levey, A. I., et al. (2018). Hippocampal place cell dysfunction and the effects of muscarinic M1 receptor agonism in a rat model of Alzheimer’s disease. Hippocampus 28, 568–585. doi: 10.1002/hipo.22961
Garvert, M. M., Dolan, R. J., and Behrens, T. E. (2017). A map of abstract relational knowledge in the human hippocampal–entorhinal cortex. Elife 6:e17086. doi: 10.7554/eLife.17086
Gauthier, J. L., and Tank, D. W. (2018). A dedicated population for reward coding in the hippocampus. Neuron 99, 179.e–193.e. doi: 10.1016/j.neuron.2018.06.008
Gil, M., Ancau, M., Schlesiger, M. I., Neitz, A., Allen, K., De Marco, R. J., et al. (2018). Impaired path integration in mice with disrupted grid cell firing. Nat. Neurosci. 21, 81–91. doi: 10.1038/s41593-017-0039-3
Gillespie, A. K., Jones, E. A., Lin, Y. H., Karlsson, M. P., Kay, K., Yoon, S. Y., et al. (2016). Apolipoprotein E4 causes age-dependent disruption of slow gamma oscillations during hippocampal sharp-wave ripples. Neuron 90, 740–751. doi: 10.1016/j.neuron.2016.04.009
Gómez-Isla, T., Price, J. L., McKeel, D. W. Jr., Morris, J. C., Growdon, J. H., and Hyman, B. T. (1996). Profound loss of layer II entorhinal cortex neurons occurs in very mild Alzheimer’s disease. J. Neurosci. 16, 4491–4500. doi: 10.1523/JNEUROSCI.16-14-04491.1996
Grundman, M., Petersen, R. C., Ferris, S. H., Thomas, R. G., Aisen, P. S., Bennett, D. A., et al. (2004). Mild cognitive impairment can be distinguished from Alzheimer disease and normal aging for clinical trials. Arch. Neurol. 61, 59–66. doi: 10.1001/archneur.61.1.59
Hafting, T., Fyhn, M., Molden, S., Moser, M., and Moser, E. (2005). Microstructure of a spatial map in the entorhinal cortex. Nature 436, 801–806. doi: 10.1038/nature03721
Hinman, J., Brandon, M., Climer, J., Chapman, G., and Hasselmo, M. (2016). Multiple running speed signals in medial entorhinal cortex. Neuron 91, 666–679. doi: 10.1016/j.neuron.2016.06.027
Hok, V., Lenck-Santini, P., Roux, S., Save, E., Muller, R., and Poucet, B. (2007). Goal-related activity in hippocampal place cells. J. Neurosci. 27, 472–482. doi: 10.1523/JNEUROSCI.2864-06.2007
Holbrook, A. J., Tustison, N. J., Marquez, F., Roberts, J., Yassa, M. A., Gillen, D. L., et al. (2020). Anterolateral entorhinal cortex thickness as a new biomarker for early detection of Alzheimer’s disease. Alzheimer’s Dement. 12:e12068. doi: 10.1002/dad2.12068
Hope, T., Tilling, K., Gedling, K., Keene, J., Cooper, S., and Fairburn, C. (1994). The structure of wandering in dementia. Int. J. Geriatr. Psychiatry 9, 149–155. doi: 10.1002/gps.930090209
Horner, A. J., Bisby, J. A., Zotow, E., Bush, D., and Burgess, N. (2016). Grid-like processing of imagined navigation. Curr. Biol. 26, 842–847. doi: 10.1016/j.cub.2016.01.042
Hort, J., Laczó, J., Vyhnálek, M., Bojar, M., Bures, J., and Vlcek, K. (2007). Spatial navigation deficit in amnestic mild cognitive impairment. Proc. Natl. Acad. Sci. U.S.A. 104, 4042–4047. doi: 10.1073/pnas.0611314104
Høydal, Ø, Skytøen, E., Andersson, S., Moser, M., and Moser, E. (2019). Object-vector coding in the medial entorhinal cortex. Nature 568, 400–404. doi: 10.1038/s41586-019-1077-7
Iaccarino, H. F., Singer, A. C., Martorell, A. J., Rudenko, A., Gao, F., Gillingham, T. Z., et al. (2016). Gamma frequency entrainment attenuates amyloid load and modifies microglia. Nature 540, 230–235. doi: 10.1038/nature20587
Ittner, A. A., Gladbach, A., Bertz, J., Suh, L. S., and Ittner, L. M. (2014). p38 MAP kinase-mediated NMDA receptor-dependent suppression of hippocampal hypersynchronicity in a mouse model of Alzheimer’s disease. Acta Neuropathol. Commun. 2:149. doi: 10.1186/s40478-014-0149-z
Jacobs, J., Kahana, M. J., Ekstrom, A. D., Mollison, M. V., and Fried, I. (2010). A sense of direction in human entorhinal cortex. Proc. Natl. Acad. Sci. U.S.A. 107, 6487–6492. doi: 10.1073/pnas.0911213107
Jun, H., Bramian, A., Soma, S., Saito, T., Saido, T. C., and Igarashi, K. M. (2020). Disrupted place cell remapping and impaired grid cells in a knockin model of Alzheimer’s disease. Neuron 107, 1095.e–1112.e. doi: 10.1016/j.neuron.2020.06.023
Kobro-Flatmoen, A., Lagartos-Donate, M. J., Aman, Y., Edison, P., Witter, M. P., and Fang, E. F. (2021). Re-emphasizing early Alzheimer’s disease pathology starting in select entorhinal neurons, with a special focus on mitophagy. Ageing Res. Rev. 67:101307. doi: 10.1016/j.arr.2021.101307
Kropff, E., Carmichael, J., Moser, M., and Moser, E. (2015). Speed cells in the medial entorhinal cortex. Nature 523, 419–424. doi: 10.1038/nature14622
Krupic, J., Burgess, N., and O’Keefe, J. (2012). Neural representations of location composed of spatially periodic bands. Science 337, 853–857. doi: 10.1126/science.1222403
Kunz, L., Schröder, T. N., Lee, H., Montag, C., Lachmann, B., Sariyska, R., et al. (2015). Reduced grid-cell-like representations in adults at genetic risk for Alzheimer’s disease. Science 350, 430–433. doi: 10.1126/science.aac8128
Kyle, C. T., Stokes, J. D., Lieberman, J. S., Hassan, A. S., and Ekstrom, A. D. (2015). Successful retrieval of competing spatial environments in humans involves hippocampal pattern separation mechanisms. Elife 4:e10499. doi: 10.7554/eLife.10499
Lester, A., Moffat, S., Wiener, J., Barnes, C., and Wolbers, T. (2017). The aging navigational system. Neuron 95, 1019–1035. doi: 10.1016/j.neuron.2017.06.037
Leutgeb, J., Leutgeb, S., Treves, A., Meyer, R., Barnes, C., McNaughton, B., et al. (2005). Progressive transformation of hippocampal neuronal representations in “morphed” environments. Neuron 48, 345–358. doi: 10.1016/j.neuron.2005.09.007
Lever, C., Burton, S., Jeewajee, A., O’Keefe, J., and Burgess, N. (2009). Boundary vector cells in the subiculum of the hippocampal formation. J. Neurosci. 29, 9771–9777. doi: 10.1523/JNEUROSCI.1319-09.2009
Mably, A. J., Gereke, B. J., Jones, D. T., and Colgin, L. L. (2017). Impairments in spatial representations and rhythmic coordination of place cells in the 3xTg mouse model of Alzheimer’s disease. Hippocampus 27, 378–392. doi: 10.1002/hipo.22697
Marcus, C., Mena, E., and Subramaniam, R. M. (2014). Brain PET in the diagnosis of Alzheimer’s disease. Clin. Nucl. Med. 39, e413–e426. doi: 10.1097/RLU.0000000000000547
Matuskova, V., Ismail, Z., Nikolai, T., Markova, H., Cechova, K., Nedelska, Z., et al. (2021). Mild behavioral impairment is associated with atrophy of entorhinal cortex and hippocampus in a memory clinic cohort. Front. Aging Neurosci. 13:643271. doi: 10.3389/fnagi.2021.643271
Moser, M., Rowland, D., and Moser, E. (2015). Place cells, grid cells, and memory. Cold Spring Harb. Perspect. Biol. 7:a021808. doi: 10.1101/cshperspect.a021808
Muller, R., and Kubie, J. (1987). The effects of changes in the environment on the spatial firing of hippocampal complex-spike cells. J. Neurosci. 7, 1951–1968. doi: 10.1523/JNEUROSCI.07-07-01951.1987
Nyhus, E., and Curran, T. (2010). Functional role of gamma and theta oscillations in episodic memory. Neurosci. Biobehav. Rev. 34, 1023–1035. doi: 10.1016/j.neubiorev.2009.12.014
O’Keefe, J., and Nadel, L. (1978). The hippocampus as a cognitive Map. Oxford: Oxford University Press.
Omer, D., Maimon, S., Las, L., and Ulanovsky, N. (2018). Social place-cells in the bat hippocampus. Science 359, 218–224. doi: 10.1126/science.aao3474
Palop, J. J., Chin, J., and Mucke, L. (2006). A network dysfunction perspective on neurodegenerative diseases. Nature 443, 768–773. doi: 10.1038/nature05289
Peer, M., Brunec, I. K., Newcombe, N. S., and Epstein, R. A. (2021). Structuring knowledge with cognitive maps and cognitive graphs. Trends Cogn. Sci. 25, 37–54. doi: 10.1016/j.tics.2020.10.004
Puthusseryppady, V., Emrich-Mills, L., Lowry, E., Patel, M., and Hornberger, M. (2020). Spatial disorientation in Alzheimer’s disease: The missing path from virtual reality to real world. Front. Aging Neurosci. 12:550514. doi: 10.3389/fnagi.2020.550514
Rajan, K., Wilson, R., Weuve, J., Barnes, L., and Evans, D. (2015). Cognitive impairment 18 years before clinical diagnosis of Alzheimer disease dementia. Neurology 85, 898–904. doi: 10.1212/WNL.0000000000001774
Rechnitz, O., Slutsky, I., Morris, G., and Derdikman, D. (2021). Hippocampal sub-networks exhibit distinct spatial representation deficits in Alzheimer’s disease model mice. Curr. Biol. 31, 3292.e–3302.e. doi: 10.1016/j.cub.2021.05.039
Richter, N., Bischof, G. N., Dronse, J., Nellessen, N., Neumaier, B., Langen, K. J., et al. (2020). Entorhinal tau predicts hippocampal activation and memory deficits in Alzheimer’s disease. J. Alzheimer’s Dis. 78, 1601–1614. doi: 10.3233/JAD-200835
Ridler, T., Witton, J., Phillips, K. G., Randall, A. D., and Brown, J. T. (2020). Impaired speed encoding and grid cell periodicity in a mouse model of tauopathy. Elife 9:e59045. doi: 10.7554/eLife.59045
Rubio, S. E., Vega-Flores, G., Martínez, A., Bosch, C., Pérez-Mediavilla, A., del Río, J., et al. (2012). Accelerated aging of the GABAergic septohippocampal pathway and decreased hippocampal rhythms in a mouse model of Alzheimer’s disease. FASEB J. 26, 4458–4467. doi: 10.1096/fj.12-208413
Sasaguri, H., Nilsson, P., Hashimoto, S., Nagata, K., Saito, T., De Strooper, B., et al. (2017). APP mouse models for Alzheimer’s disease preclinical studies. EMBO J. 36, 2473–2487. doi: 10.15252/embj.201797397
Schneider, F., Baldauf, K., Wetzel, W., and Reymann, K. G. (2014). Behavioral and EEG changes in male 5xFAD mice. Physiol. Behav. 135, 25–33. doi: 10.1016/j.physbeh.2014.05.041
Serino, S., and Riva, G. (2013). Getting lost in Alzheimer’s disease: A break in the mental frame syncing. Med. Hypotheses 80, 416–421. doi: 10.1016/j.mehy.2012.12.031
Solstad, T., Boccara, C. N., Kropff, E., Moser, M. B., and Moser, E. I. (2008). Representation of geometric borders in the entorhinal cortex. Science 322, 1865–1868. doi: 10.1126/science.1166466
Taube, J., Muller, R., and Ranck, J. (1990). Head-direction cells recorded from the postsubiculum in freely moving rats. I. Description and quantitative analysis. J. Neurosci. 10, 420–435. doi: 10.1523/JNEUROSCI.10-02-00420.1990
Tolman, E. C. (1940). Spatial angle and vicarious trial and error. J. Comp. Psychol. 30, 129–135. doi: 10.1037/h0062577
Van Hoesen, G. W., Hyman, B. T., and Damasio, A. R. (1991). Entorhinal cortex pathology in Alzheimer’s disease. Hippocampus 1, 1–8. doi: 10.1002/hipo.450010102
Vertes, R. P., Hoover, W. B., and Viana Di Prisco, G. (2004). Theta rhythm of the hippocampus: Subcortical control and functional significance. Behav. Cogn. Neurosci. Rev. 3, 173–200. doi: 10.1177/1534582304273594
Villette, V., Poindessous-Jazat, F., Simon, A., Léna, C., Roullot, E., Bellessort, B., et al. (2010). Decreased rhythmic GABAergic septal activity and memory-associated theta oscillations after hippocampal amyloid-beta pathology in the rat. J. Neurosci. 30, 10991–11003. doi: 10.1523/JNEUROSCI.6284-09.2010
Vlcek, K., and Laczo, J. (2014). Neural correlates of spatial navigation changes in mild cognitive impairment and Alzheimer’s disease. Front. Behav. Neurosci. 8:89. doi: 10.3389/fnbeh.2014.00089
Wernle, T., Waaga, T., Mørreaunet, M., Treves, A., Moser, M., and Moser, E. (2018). Integration of grid maps in merged environments. Nat. Neurosci. 21, 92–101. doi: 10.1038/s41593-017-0036-6
Wilson, R. S., Leurgans, S. E., Boyle, P. A., and Bennett, D. A. (2011). Cognitive decline in prodromal Alzheimer disease and mild cognitive impairment. Arch. Neurol. 68, 351–356. doi: 10.1001/archneurol.2011.31
Ying, J., Keinath, A. T., Lavoie, R., Vigneault, E., El Mestikawy, S., and Brandon, M. P. (2022). Disruption of the grid cell network in a mouse model of early Alzheimer’s disease. Nat. Commun. 13:886. doi: 10.1038/s41467-022-28551-x
Zhang, H., Jiang, X., Ma, L., Wei, W., Li, Z., Chang, S., et al. (2022). Role of Aβ in Alzheimer’s-related synaptic dysfunction. Front. Cell Dev. Biol. 26:964075. doi: 10.3389/fcell.2022.964075
Keywords: Alzheimer’s disease, cognitive map, navigation, hippocampus, entorhinal cortex, remapping
Citation: Silva A and Martínez MC (2023) Spatial memory deficits in Alzheimer’s disease and their connection to cognitive maps’ formation by place cells and grid cells. Front. Behav. Neurosci. 16:1082158. doi: 10.3389/fnbeh.2022.1082158
Received: 27 October 2022; Accepted: 28 December 2022;
Published: 12 January 2023.
Edited by:
Marcelo Giachero, Fundación INECO, ArgentinaReviewed by:
Rafal Czajkowski, Nencki Institute of Experimental Biology (PAN), PolandCopyright © 2023 Silva and Martínez. This is an open-access article distributed under the terms of the Creative Commons Attribution License (CC BY). The use, distribution or reproduction in other forums is permitted, provided the original author(s) and the copyright owner(s) are credited and that the original publication in this journal is cited, in accordance with accepted academic practice. No use, distribution or reproduction is permitted which does not comply with these terms.
*Correspondence: María Cecilia Martínez, bWNtYXJ0aW5lekBmbWVkLnViYS5hcg==
†These authors have contributed equally to this work
Disclaimer: All claims expressed in this article are solely those of the authors and do not necessarily represent those of their affiliated organizations, or those of the publisher, the editors and the reviewers. Any product that may be evaluated in this article or claim that may be made by its manufacturer is not guaranteed or endorsed by the publisher.
Research integrity at Frontiers
Learn more about the work of our research integrity team to safeguard the quality of each article we publish.