- 1Neurobiology Laboratory, Department of Health and Human Services, National Institute of Environmental Health Sciences, National Institutes of Health, Durham, NC, United States
- 2Center on Compulsive Behaviors, National Institutes of Health, Bethesda, MD, United States
- 3Department of Psychiatry and Carolina Institute for Developmental Disabilities, University of North Carolina, Chapel Hill, NC, United States
- 4Department of Health and Human Services, Neurobehavioral Core, National Institute of Environmental Health Sciences, National Institutes of Health, Durham, NC, United States
Introduction: Altered signaling or function of acetylcholine (ACh) has been reported in various neurological diseases, including Alzheimer’s disease, Tourette syndrome, epilepsy among others. Many neurons that release ACh also co-transmit the neurotransmitter gamma-aminobutyrate (GABA) at synapses in the hippocampus, striatum, substantia nigra, and medial prefrontal cortex (mPFC). Although ACh transmission is crucial for higher brain functions such as learning and memory, the role of co-transmitted GABA from ACh neurons in brain function remains unknown. Thus, the overarching goal of this study was to investigate how a systemic loss of GABA co-transmission from ACh neurons affected the behavioral performance of mice.
Methods: To do this, we used a conditional knock-out mouse of the vesicular GABA transporter (vGAT) crossed with the ChAT-Cre driver line to selectively ablate GABA co-transmission at ACh synapses. In a comprehensive series of standardized behavioral assays, we compared Cre-negative control mice with Cre-positive vGAT knock-out mice of both sexes.
Results: Loss of GABA co-transmission from ACh neurons did not disrupt the animal’s sociability, motor skills or sensation. However, in the absence of GABA co-transmission, we found significant alterations in social, spatial and fear memory as well as a reduced reliance on striatum-dependent response strategies in a T-maze. In addition, male conditional knockout (CKO) mice showed increased locomotion.
Discussion: Taken together, the loss of GABA co-transmission leads to deficits in higher brain functions and behaviors. Therefore, we propose that ACh/GABA co-transmission modulates neural circuitry involved in the affected behaviors.
Introduction
In agreement with Dale’s principle, most synaptic vesicles (SVs) contain only a single neurotransmitter type defined by the vesicular transporters located within its membrane (Gutierrez, 2009; Upmanyu et al., 2022). In addition to neurotransmitters, SVs co-release other signaling molecules such as protons, ATP, and in some cases, Zn2+ ions (Burnstock, 1976; Ellis and Burnstock, 1989; Soto et al., 2018; Li et al., 2020; Upmanyu et al., 2022). Rarely, different neurotransmitters or neuropeptides are co-released from the same SVs (Wojcik et al., 2006; Tritsch et al., 2016). More often, separate pools of SVs with different neurotransmitters and different intrinsic properties exist at and are released from the same synapse or neuron during a process called co-transmission (Gutierrez, 2009; Takacs et al., 2018).
Acetylcholine- (ACh) releasing neurons exist both as local interneurons as well as projection neurons and they form complex axonal arborizations with vast numbers of synapses which transmit signals through ionotropic nicotinic ACh receptors (nAChR) or metabotropic muscarinic ACh receptors (mAChR; Ballinger et al., 2016). The expression of nAChR and mAChR depends on pre- and/or postsynaptic location, cell type, cell location, and brain region (Guillem et al., 2011; Poorthuis et al., 2013). Furthermore, the effects of ACh transmission strongly depend on the timing of the ACh signal and other firing events (Gu and Yakel, 2011; Unal et al., 2015). Individual ACh neurons form synapses with many target neurons, even within different brain regions (Nelson and Mooney, 2016; Li et al., 2018). The cholinergic system coordinates the activity within a large network of neurons in the brain by modulating neuron firing properties, SV release probability, and morphology, ACh signals can directly trigger the release of neuromodulators such as dopamine (DA) from axonal varicosities (Lozada et al., 2012; Picciotto et al., 2012; Morley and Mervis, 2013; Cheng and Yakel, 2015; Shin et al., 2017; Urban-Ciecko et al., 2018; Liu et al., 2022; Steinecke et al., 2022). The varied effects of ACh transmission are required to coordinate oscillatory events of computational processes within the hippocampus or cortical areas where large neuron populations fire synchronously (Marrosu et al., 1995; Hasselmo and Mcgaughy, 2004; Gu et al., 2017, 2020).
The role of ACh release in cognition is well-established (Ballinger et al., 2016). It is, however, unclear why large subpopulations of ACh neurons co-express markers (e.g., GAD1/2, vGAT, Lhx6, VIP) which are typically found in gamma-amino-butyrate (GABA)-ergic neurons (Lee et al., 2010; Saunders et al., 2015a, b; Sethuramanujam et al., 2016; Lozovaya et al., 2018; Takacs et al., 2018; Obermayer et al., 2019; Granger et al., 2020). These GABAergic ACh neurons can release GABA at their synapses in the retina, hippocampus, striatum, medial prefrontal cortex (mPFC), lateral septum, and substantia nigra pars compacta (SNc, Lee et al., 2010; Saunders et al., 2015a, b; Sethuramanujam et al., 2016; Estakhr et al., 2017; Lozovaya et al., 2018; Takacs et al., 2018; Obermayer et al., 2019; Granger et al., 2020; Hunt et al., 2022; Le Gratiet et al., 2022). There are indications that both neurotransmitters are co-transmitted at the same ACh synapses by distinct mechanisms (Lee et al., 2010; Estakhr et al., 2017; Takacs et al., 2018). In fact, GABA release at ACh synapses has faster kinetics, requires less calcium, and relies mostly on CaV 2.1 channels indicating tight SV coupling; ACh release, in contrast, has a higher calcium dependence and relies more on CaV 2.2 channels indicating loose SV coupling (Neher and Sakaba, 2008; Lee et al., 2010; Takacs et al., 2018).
Co-transmission of ACh and GABA is implicated in different brain functions but due to the inherent limitations of current tools, the effects of co-transmitted GABA are hard to separate from those of ACh or GABA release from other neurons. In the striatum, ACh/GABA interneurons (CGINs) are more strongly involved in the pause response and more sensitive to local inhibition (Lozovaya et al., 2018). In a model of Parkinson’s disease (PD), changes in chloride homeostasis cause co-transmitted GABA to have excitatory effects (Lozovaya et al., 2018). This excitatory drive increases the activity of CGIN and enlarged the dendritic fields of CGINs, causing an increased CGIN-CGIN connectivity and firing (Lozovaya et al., 2018). In the hippocampus, ACh and GABA are co-transmitted from the medial septum/diagonal band of Broca projections onto oriens lacunosum moleculare (OLM) interneurons (Takacs et al., 2018). While the release of GABA is sufficient to suppress sharp-wave ripples and epileptiform activity within the hippocampus, it is unknown whether co-transmitted GABA from ACh neurons is required for these processes, as well (Takacs et al., 2018). The mPFC receives inputs from ACh/GABA neurons in the external segment of the globus pallidus (GPe) and from the adjacent part of the nucleus basalis of Meynert (NB) mostly onto L1 interneurons (Saunders et al., 2015a, b). Moreover, there seem to be differences between mice and rats regarding mPFC VIP+/ChAT+ interneurons abundance and innervations (Obermayer et al., 2019; Granger et al., 2020). In the mPFC of rats, GABA co-transmission from VIP+/ChAT+ interneurons modulates the spike timing in target neurons and contributes to long-term attention during animal behavior assays (Obermayer et al., 2019). Lastly, SNc DA neurons receive co-transmitted ACh and GABA from the lateral dorsal tegmental (LDT) as well as the pedunculopontine nuclei (PPN) ACh neurons which affect their excitability (Estakhr et al., 2017; Le Gratiet et al., 2022).
However, little is known about the importance of GABA co-transmission from ACh neurons for animal behavior. Therefore, we assessed whether mice without GABA co-transmission from ACh neurons showed any behavioral deficits. We ablated GABAergic transmission from ACh neurons by knocking out the vesicular GABA transporter (vGAT, Slc32a1) specifically in ACh neurons, and then evaluated the conditional knockout (CKO) mice in a battery of standardized behavior assays. We found that vGAT CKO mice had impaired social and spatial memory as well as minor alterations in striatal response learning and fear renewal. Furthermore, male CKO mice had increased locomotor activity. Taken together, these alterations indicate the involvement of GABA co-transmission from ACh neurons in complex behaviors that require hippocampal, striatal, and mPFC circuitry.
Materials and Methods
Animals
Transgenic animal lines were purchased from The Jackson Laboratories and subsequently maintained and bred in-house. Offspring were group housed (<5 per cage, separated by sex) whenever possible in a regular 12 h light/dark cycle. Food and water were supplied ad libitum. To delete GABAergic co-transmission from cholinergic neurons, we crossed vGAT-flox mice (Jax# 012897, RRID:IMSR_JAX:012897), with ChAT-IRES-Cre mice (Jax# 006410, RRID:IMSR_JAX:006410, Tong et al., 2008; Rossi et al., 2011). Mice were bred with homozygous vGAT-flox alleles and heterozygous ChAT-IRES-Cre alleles to obtain Cre-negative (ctrl) as well as heterozygous Cre-positive littermates (CKO) for behavior experiments.
All animal care and procedures were conducted in strict compliance with the animal welfare policies set by the National Institutes of Health. All procedures were approved and performed in compliance with the NIEHS/NIH Humane Care and Use of Animals Protocols, and, where applicable, by the UNC Institutional Animal Care and Use Committee and by the University of North Carolina at Chapel Hill (UNC).
Animal behavior experiments
We used three independent cohorts of mice for behavioral assays (Table 1): general behavioral phenotyping, T-maze, and additional behaviors. The general behavioral phenotyping was performed at the UNC Behavioral Phenotyping Laboratory and all mice in this cohort went through all assays unless otherwise stated (see Table 2 for a detailed timeline). The T-maze and the additional behavior were performed at the NIEHS animal facility. For the T-maze, the mice were placed in a 12 h reverse-light cycle room and put on a food-restriction schedule to gradually reduce and maintain the body weight at >85%. During a period of 7–10 days, the animals were weighed and handled (10 min/day) daily. Before the start of the behavioral testing, animals were acclimated to the room for >30 min. Behavioral testing was conducted during the animals’ dark cycle. The additional behavior included an automated home-cage discrimination and reversal learning test, and fear conditioning, extinction, as well as renewal. All mice in this cohort went through both assays unless otherwise stated. Both tasks were separated by about 4 weeks.
Three-chamber choice test
The procedure was comprised of three 10-min phases: habituation, sociability test, and social novelty preference test. During the sociability test, mice had a choice between proximity to an unfamiliar, sex-matched C57BL/6J adult mouse (“stranger 1”) and being alone. During the social novelty test, mice were to choose between the already-investigated stranger 1, and a new unfamiliar mouse (“stranger 2”). A rectangular, 3-chambered clear Plexiglas box with dividing walls and doorways to each chamber was used to perform the test. An automated image tracking system (Ethovision7, Noldus, Leesburg, VA, USA, RRID:SCR_000441) provided measures of time spent on each side and entries into each side of the social test box.
During the first phase of the test, the mouse was placed in the middle chamber and allowed to explore for 10 min, with the doorways open into both side chambers. After the habituation phase, the test mouse was enclosed in the center compartment of the social test box, and stranger 1 was placed in one of the side chambers. The stranger mouse was enclosed in a small Plexiglas cage drilled with holes to allow nose contact. An identical empty Plexiglas cage was placed in the opposite chamber. After the placement of stranger 1 and the empty cage, the doors were re-opened, and the subject was allowed to explore the social test box for another 10-min session. At the end of the sociability phase, stranger 2 was placed in the empty Plexiglas cage, and the subject was given an additional 10 min to explore the social test box.
Morris water maze
Spatial and reversal learning, swimming ability, and vision were assessed by performance in the Morris water maze. A large circular pool (122 cm diameter) was partially filled with water (45 cm depth, 24–26°C), located in a room with numerous visual cues. The test consisted of three different phases: the visible platform test, acquisition in the hidden platform test, and the reversal learning test.
During the visible platform test, every mouse performed four trials per day on two consecutive days, to swim to an escape platform cued by a patterned cylinder extending above the water. The mouse started each trial in the pool at one of four possible locations at random and was given 60 s to find the visible platform. If the mouse reached the platform, the trial ended, and the animal remained on the platform for 10 s before the next trial began. In case the platform was not found, the mouse was placed on the platform for 10 s, and then given the next trial. Latency to reach the platform and swimming speed was measured via an automated tracking system (Ethovision 15, Noldus).
Following the visible platform test, mice were tested for the ability to find a submerged, hidden escape platform (diameter = 12 cm). Each mouse performed 4 × 1 min trials per day, to swim to the hidden platform. In the present study, all groups reached the learning criterion on day 4 (15 s or less to locate the platform) and were given a 1-min probe trial in the pool without any platform on the same day. The selective search for the correct location was assessed by comparing the number of swim paths crossing over the quadrant where the platform (target) was positioned during training with the corresponding area in the opposite quadrant.
Following the hidden platform phase, mice were tested for reversal learning. During this phase, the same procedure was used as described above but with the hidden platform transferred to the opposite quadrant in the pool. As during the visible platform test, the latency to find the platform was assessed. On day 4 of testing, the platform was removed from the pool, and every mouse performed a probe trial to evaluate reversal learning. Quadrant preference was assessed by comparing swim paths over the target and opposite quadrants.
T-maze
A plus maze with a white polypropylene floor (Med Associates) with four identical arms (37.47 × 8.92 × 14.68 cm) was raised to 17.46 cm and placed in a black-curtained area. All trials were recorded on video and tracked with Ethovision 15. Animals were trained during their dark cycle in the presence of dim white illumination with large white fabric cues (triangle, oval, rectangle, square) affixed to the curtain behind every arm. During habituation and training trials, the maze arm facing the start arm was blocked to create a T-maze. Before the training, every mouse performed two 5 min habituation trials on each of two consecutive days to adjust to the maze environment. To avoid a direction bias, about half of the animals were trained to turn right to gain the reward of two sugar pellets (Dustless Precision Rodent Pellets, F05684, Bio-Serv, Flemington, NJ) located at the end of the right arm. The other half were trained to turn left for the reward. On each trial, the mouse was placed at the end of the start arm facing the center of the maze and given 2 min to find and eat the reward. After 2 min or when the pellets were eaten, the mouse was returned to an empty cage for 30 s before the next trial was started. Every animal performed four trials per day. If the mouse turned into the baited arm first, the trial was counted as successful. After 7 days of training, the first probe trial was performed. During the probe trial, the training start arm was blocked, and mice entered the maze at the end of the initially blocked arm. If the mouse turned in the arm that was correct during training, the mouse was considered a “place learner”. If the mouse turned in the direction that was not correct during training, the mouse was considered a “response learner”. After another 7 days of training, the second probe trial was performed. In total every mouse performed 4× habituation, 56× training, and 2× probe trials.
Automated home-cage discrimination and reversal learning test
An automated home-cage platform (Phenotyper, Noldus) was used to monitor spontaneous behavior as well as discrimination and reversal learning in absence of human intervention. Mice were monitored by video and tracked throughout the complete experiment using Ethovision 16 (Noldus). Up to 16 mice were tested in parallel. Before the start of the experiment, animals were single-housed in regular cages on white cellulose ALPHA-DRI bedding for 4–5 days and habituated to the rewarded sugar pellets (Dustless Precision Rodent Pellets, F05684, Bio-Serv, Flemington, NJ) with regular feed and water ad libitum. The lights were controlled by the automated home cage, the dark cycle was from 6 pm to 6 am. On the day of the experiment start, mice were transferred to the Phenotype cages (L = 30 × W = 30 × H = 35 cm) and habituated to the novel environment for 6 h. The Cognition Wall was introduced about 30 min (~4:00 pm) before the start of the discrimination learning experiment (~4.30 pm). The Cognition Wall, an opaque wall with three holes, allowed the mice to pass through to retrieve food pellets, as previously described (Remmelink et al., 2016). Water was provided ad libitum throughout the protocol. Standard feed was absent from the cage during the trial but a “free” pellet reward was dispensed to create the association between Cognition Wall entry and pellet reward. During a 48-h discrimination learning (DL) test, mice were trained to discriminate the left entrance hole as the “correct” hole and received a pellet reward. “Correct” hole entries were detected by the software and automatically triggered pellet dispensation. DL success was measured by the rate of establishing a preference for the rewarded entrance. If the mouse did not reach the criterion of >100 dispensed pellets within 48 h, it was withdrawn from the study and returned to its home cage. After the DL phase, the reversal learning (RL) phase was conducted within the following 48 h. During RL, the rewarded entrance shifted from “left” to “right” entrance. The rate of a shift in preference for the new entrance was used as a measure for reversal learning. During DL and RL, a pellet reward was dispensed for every fifth entry through the correct hole (FR5 schedule of reinforcement) to avoid the accumulation of non-consumed pellet rewards in the cage (Remmelink et al., 2016). Mice did not have to make five consecutive correct entries. The results of the Cognition Wall experiments were independently analyzed by Sylics (Synaptologics BV, Bilthoven, The Netherlands).
Acquisition, extinction, renewal of cued and contextual fear learning
Mice were tested for fear learning and memory in fear conditioning boxes (MED Associates, St. Albans, VT, USA). The experiment had these three phases: acquisition on day 1, fear extinction on day 2–4, and a test for context-dependent/renewal learning on day 5.
During the training on day 1, each mouse was put in the test chamber designated as context A (standard grid floor with isopropanol/simple green scent) within a sound-attenuating box and allowed to explore for 3 min. Then, the mice were exposed to a 75 dB 2,800 Hz pure tone for 30 s that co-terminated with a 2 s scrambled foot shock (0.5 mA). Mice received two additional shock-tone pairings, with an 80 s pause between each pairing.
During the fear extinction phase on day 2–4, mice were placed into a modified chamber arranged as context B (black A-frame insert, white floor, ethanol/windex scent) for a test of extinction of the cued fear response in absence of the foot shock. After 3 min in the chamber, the animal was presented with a massed extinction protocol, where the same auditory tone from the acquisition day (30 s 75 dB 2,800 Hz) was presented 20 times separated by 5 s. During the context-dependent/renewal learning phase on day 5, the mouse was returned to the original acquisition context conditioning chamber arranged as context A. After the exploration phase, the 75 dB 2,800 Hz pure tone was presented 3× for 30 s per repetition using the protocol used for acquisition with the shock omitted. Freezing, defined as complete immobility except that necessitated by breathing was scored using the Video Freeze software (activity threshold 19 for 1 s). During the extinction phase, tone presentations were binned by five tone presentations to facilitate graphing and analysis.
Statistics
The experimenters were blinded for the mouse genotype during behavioral testing. Statview (SAS, Cary, NC, RRID:SCR_017411), Microsoft Excel (Redmond WA, USA, RRID:SCR_016137), Igor Pro 8.04 (Wavemetrics, Lake Oswego, OR, USA, RRID:SCR_000325), Prism 9 (GraphPad, San Diego, CA, USA, RRID:SCR_002798), and R (version 3.6.3) were used for data analysis. For the behavioral data, two-way or repeated measures analysis of variance (ANOVA) was used to determine the effects of genotype and sex, followed by separate analyses for males and females, to determine genotype effects within each sex. Post-hoc comparisons were conducted using Fisher’s Protected Least Significant Difference (PLSD) tests only when a significant F value was found in the ANOVA. Within-genotype comparisons were used to determine side preference in the 3-chamber test and quadrant selectivity in the water maze. If no significant F value was found in the ANOVA for sex, animals were pooled by genotype.
For the T maze, no side bias was detected using graphical inspection. The success rate to enter goal arms, was analyzed by a Repeated Measures Proportional Odds Logistic Regression model using the repolr package (version 3.41). The daily success rate from day 1 to day 14 was fit using generalized estimating equations with sex, genotype, day, and their interactions as well as an AR (1) covariance structure to reflect temporal correlation. The T-maze probe trial results as well as strategy transitions from probe trial 1 to probe trial 2 were analyzed using a Fisher’s exact test. For all comparisons, significance was set at p < 0.05.
Results
Loss of GABA co-transmission from ACh neurons does not affect general health, anxiety-like behavior, or motor and sensory skills in mice
The co-transmission of ACh and GABA has been described previously in the hippocampus, striatum, mPFC, lateral septum, SNc, as well as the retina (Lee et al., 2010; Saunders et al., 2015a, b; Sethuramanujam et al., 2016; Estakhr et al., 2017; Lozovaya et al., 2018; Takacs et al., 2018; Obermayer et al., 2019; Granger et al., 2020; Hunt et al., 2022; Le Gratiet et al., 2022). Although these reports provided many insights into how ACh/GABA co-transmission is embedded into the individual circuits or contributes to pathogenicity in disease, the role of GABA co-transmission with AC as it relates to circuit function remains unknown. Therefore, the primary goal of our experiments was to assess how a systemic loss of GABA co-transmission from ACh neurons affected the behavioral performance of mice during a battery of standardized behavioral tests (Table 2). We found that in absence of GABA co-transmission from ACh neurons, mice showed no impairments in general health, motor activity (locomotion in a simple environment, rearing, swimming, motor coordination), sensory abilities (vision, hearing, olfaction), anxiety-like behavior, sensorimotor gating, fear memory acquisition, or fear extinction (Supplementary Figures 1–4, Supplementary Table 1). However, we found several genotype or sex-dependent effects on more complex cognitive processes, such as: social novelty preference, spatial memory, context-dependent locomotion, competing learning strategies, and fear renewal (Figures 1–5, Supplementary Tables 2–6).
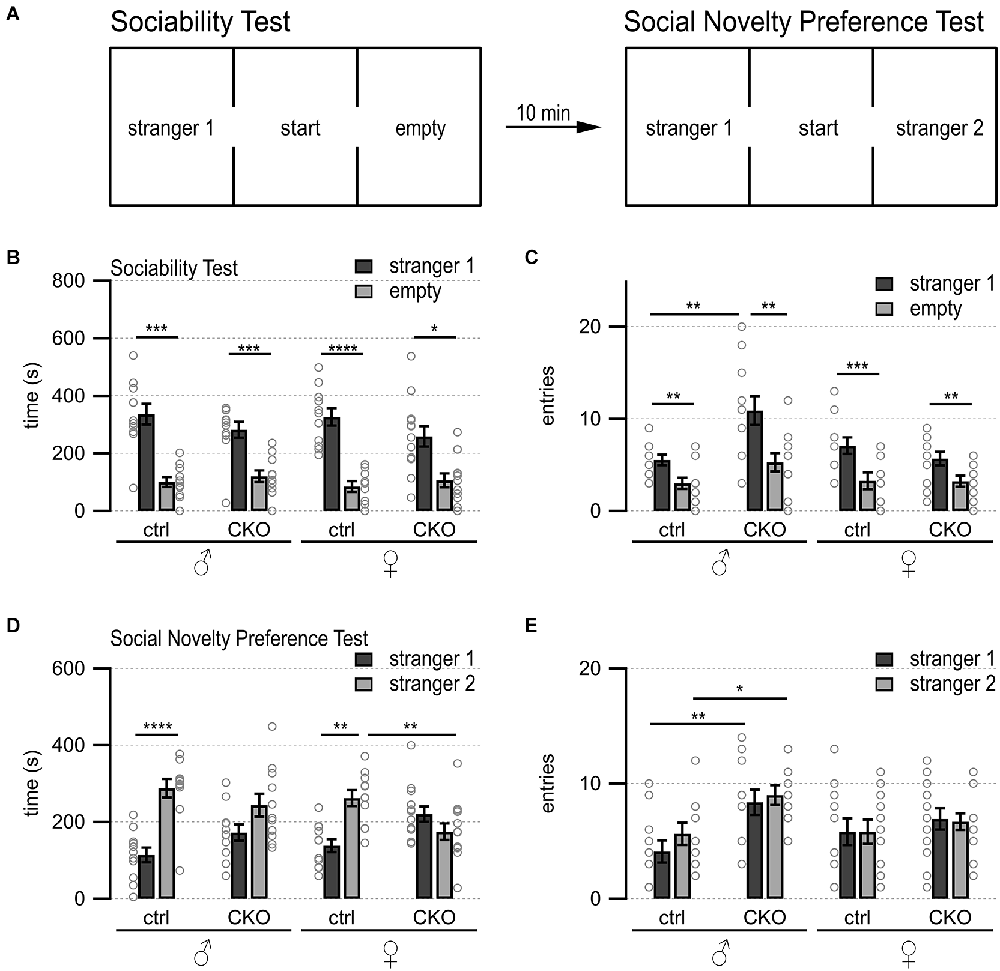
Figure 1. Loss of GABA co-transmission from ACh neurons results in loss of social novelty preference independent of sex as well as increased chamber entries in males. Loss of GABA co-transmission leads to a loss of social novelty preference in mice as well as increased chamber entries in males but does not affect sociability. (A) Schematic showing setup of sociability and social novelty preference test. Mice habituated for 10 min in the cage with both side chambers empty. Before the sociability test, an unfamiliar sex-matched mouse (stranger 1) was introduced into the left chamber. After 10 min of the sociability test, before the start of the social novelty preference test, an unfamiliar sex-matched mouse (stranger 2) was introduced into the right chamber. Time spent in outer chambers and chamber entries were measured. (B,C) Sociability test results. Time spent in (B) and entries (C) into side chambers shown for male, female control (ctrl), and CKO mice. (D,E) Social novelty test results. Time spent in (D) and entries (E) into side chambers shown for male, female control (ctrl), and CKO mice. Values represent mean ± SEM. Individual data points are depicted as open circles. Repeated measures ANOVA followed by Fisher’s protected least-significant difference tests (*p < 0.05, **p < 0.01, ***p < 0.001, ****p < 0.0001). See Supplementary Table 2 for data.
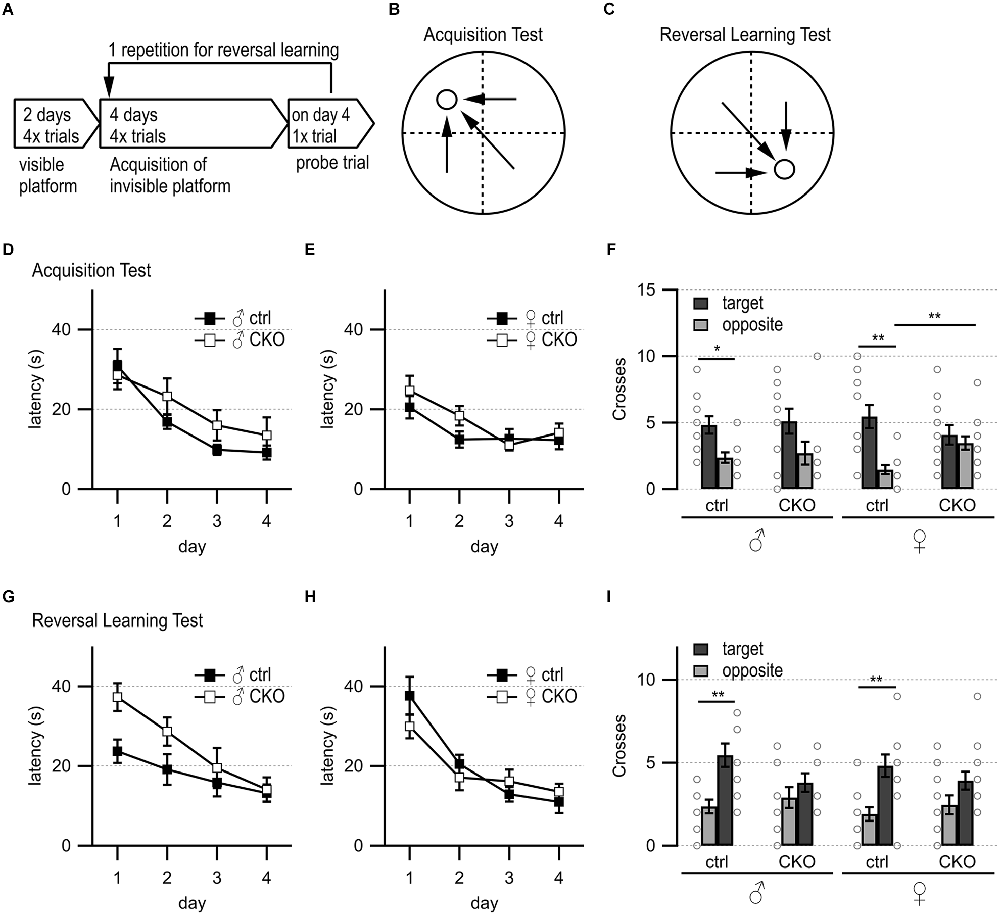
Figure 2. Loss of GABA co-transmission from ACh neurons results in spatial memory deficits. Loss of GABA co-transmission leads to spatial memory impairments. (A) Timeline of Morris water maze experiment. Visible platform test, acquisition test using hidden platform followed by one probe trial without platform, reversal learning test with hidden platform in the opposite quadrant, and one probe trial without platform. (B) Water maze setup for acquisition test. Visible/hidden platform placed at the circle. Start points chosen in arbitrarily random order. Theoretical escape pathway to hidden platform indicated by arrows. (C) Water maze setup for reversal test with hidden platform placed in opposite quadrant. (D,E) Latency to reach the hidden platform during acquisition learning for males (D) and females (E). (F) Probe trial results after acquisition learning phase in absence of the platform. Counts of target and opposite quadrant crosses. (G,H) Latency to reach the hidden platform during reversal learning for males (D) and females (E). (I) Probe trial results after reversal learning phase in absence of the platform. Counts of swimming path crosses over the platform area in target quadrant and corresponding area in the opposite quadrant. Values represent mean ± SEM. Individual data points are depicted as open circles. Repeated measures ANOVA followed by Fisher’s protected least-significant difference (*p < 0.05, **p < 0.01). See Supplementary Table 3 for data.
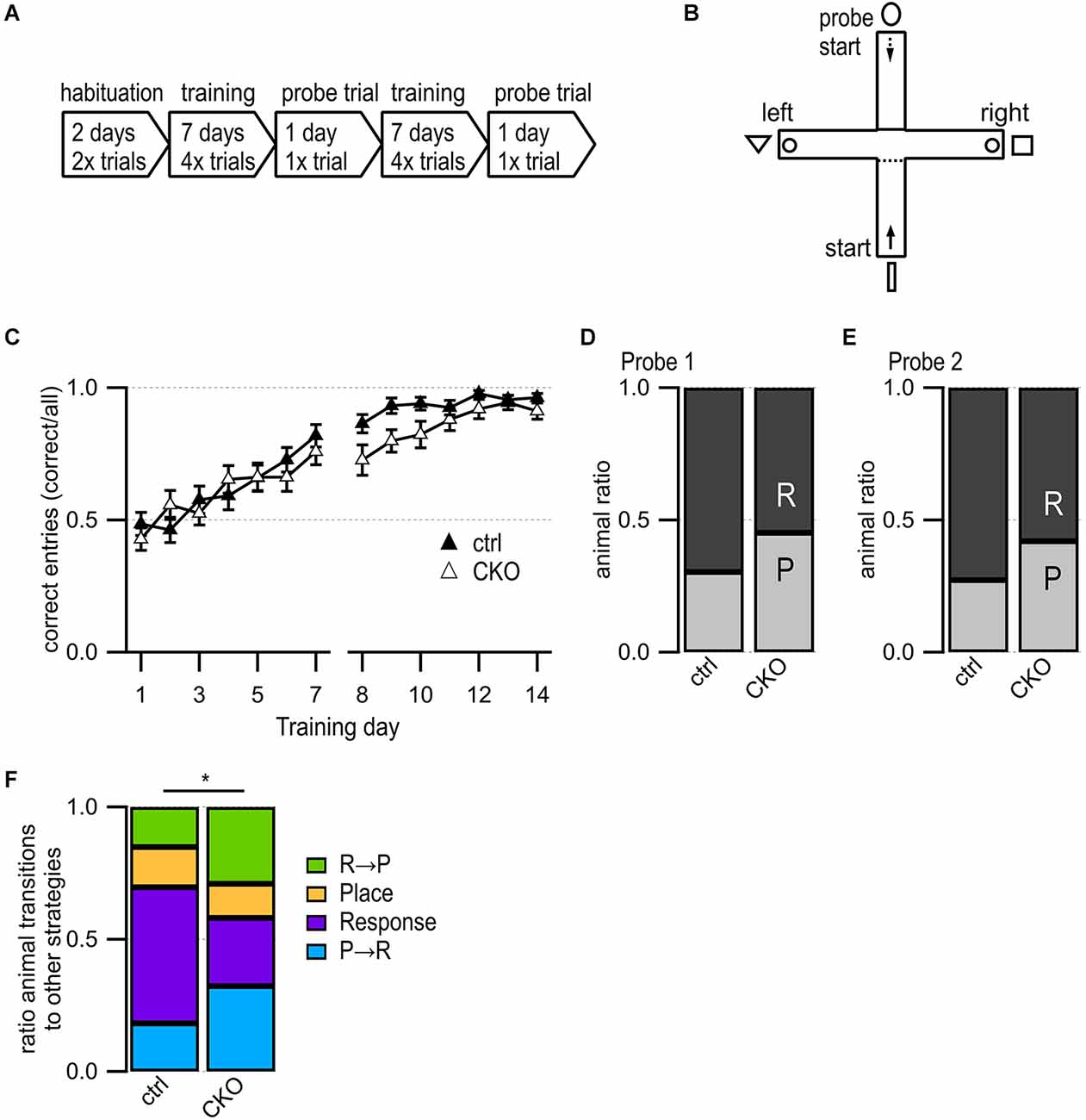
Figure 3. Loss of GABA co-transmission from ACh neurons leads to changes in usage of competing learning strategies. (A) Timeline of T maze experiment. Habituation phase for 2 days, training phase for 7 days followed by one probe trial day. Repetition of the training phase for another 7 days followed by one probe trial day. (B) T-maze setup. Animals start in the south arm during habituation and training but from the north arm during probe trials. Visible fabric cues attached to the curtained walls outside of the maze. Food bowls (circle) were placed at ends of both goal arms. Start points for training indicated by continuous arrow and for probe trials by dashed arrow. (C) Training success indicated as correct entries into goal arms for every training day. (D,E) Results for Probe trial day 1 (D) and Probe trial day 2 (E) indicating the relative number of response (R) and place (P) learners. (F) Animal ratios were sorted by learning strategy and strategy transitions from probe trial 1 to probe trial 2: place transitioner (R→P, green), consistent place (orange), consistent response (purple), response transitioner (P→R, turquoise). Values represent mean ± SEM. Repeated Measures Proportional Odds Logistic Regression model (C) and Fisher’s exact test (F) (*p < 0.05). See Supplementary Table 4 for data.
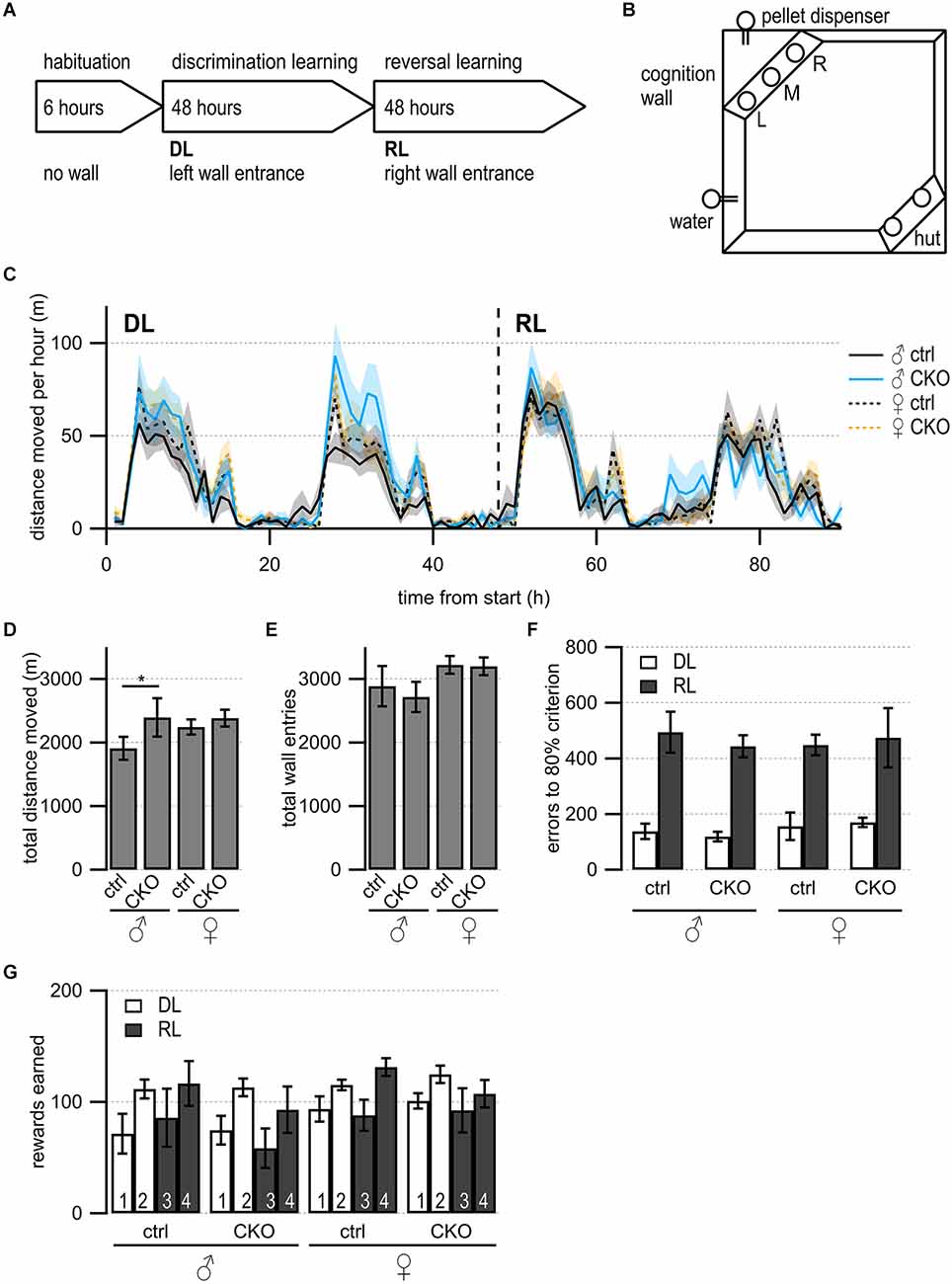
Figure 4. Loss of GABA co-transmission from ACh neurons results in increased locomotion in males but no impairments in discrimination or reversal learning. (A) Timeline of Cognition Wall experiment. Habituation phase for 6 h without Cognition Wall. Introduction of the Cognition Wall ~30 min before the start of discrimination learning phase (DL) for 2 days followed by reversal learning phase (RL) for another 2 days. During DL, the animal trained to enter left wall entrance for food reward. During RL, the animal trained to enter right wall entrance for food reward. (B) Cognition Wall setup. Hut for shelter in bottom right, water bottle in bottom left, Cognition Wall in top left covering the food dispenser. Left (L), middle (M), right (R) entrance of Cognition Wall. (C) The total distance moved during experiment per 1 h bin. (D) The total distance moved during experiment. (E) The total wall entries during experiment. (F) The number of error entries before reaching 80% learning criterion during DL and RL. (G) The number of reward pellets earned per day during DL and RL. Values represent mean ± SEM. Two-way ANOVA followed by Fisher’s protected least-significant difference (*p < 0.05). See Supplementary Table 5 for data.
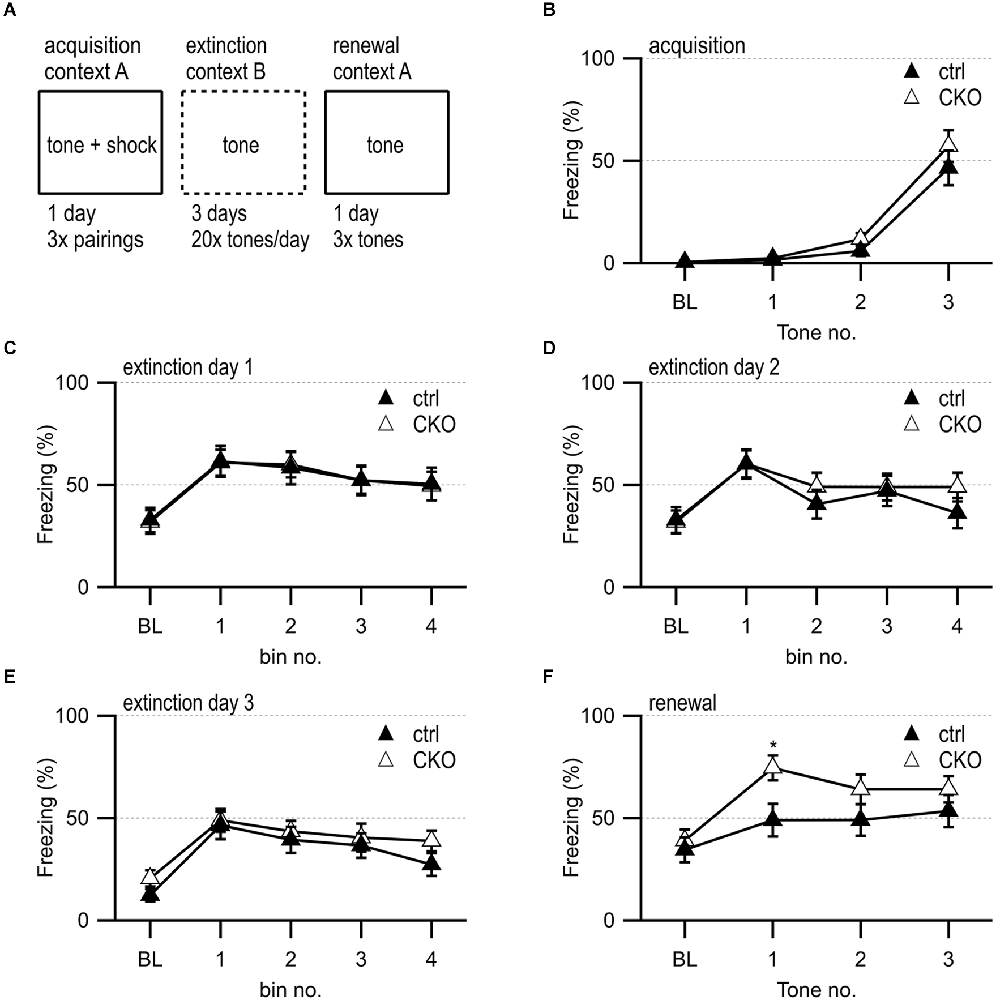
Figure 5. Loss of GABA co-transmission from ACh neurons leads to transient increased freezing during contextual fear renewal. (A) Timeline of fear conditioning experiment. Acquisition of fear memory on day 1 by 3× pairing of 75 dB 2,800 Hz pure tone with 2 s 0.5 mV foot shock in context A. During 3 days of extinction, animal was presented with 20 tones/day in context B. During the renewal of the fear memory, animal was presented with three tones in context A. (B) Percent of freezing during acquisition phase at baseline (BL) and per tone/foot shock pairing. (C–E) Percent of freezing during extinction phase at baseline (BL) and per five tone bin on day 1 (C), day 2 (D), and day 3 (E). (F) Percent of freezing during renewal phase at baseline (BL) and per tone presentation. Values represent mean ± SEM. Two-way ANOVA followed by Fisher’s protected least-significant difference test (*p < 0.05). See Supplementary Table 6 for data.
GABA co-transmission from ACh neurons is required for social novelty preference independent of sex and exploratory behavior in males
Many psychiatric diseases, such as autism, bipolar disorder, and schizophrenia, are accompanied by impaired social abilities (Moy et al., 2004, 2009, 2013; O’tuathaigh et al., 2007; Carter et al., 2011; Pietropaolo et al., 2011; Sidhu et al., 2014; Brunner et al., 2015; Jaramillo et al., 2017; Kim et al., 2017; Lu et al., 2018; Olaya et al., 2018; Nakazawa et al., 2019; Win-Shwe et al., 2021). Using the three-chamber choice test (Figure 1A), we assessed whether a loss of GABA co-transmission from ACh neurons leads to impairments in the natural inclination of mice to investigate a novel social stimulus. Sociability, or the preference for an animal to investigate another animal compared with an empty chamber, was unaffected by loss of GABA co-transmission from ACh neurons (Figure 1B, main effect of side, males, F(1,20) = 54.92, p < 0.0001; females, F(1,22) = 33.5, p < 0.0001).
During the social novelty preference phase, only the ctrl but not the CKO mice showed significant social novelty preference as assessed by investigation times (Figure 1D, genotype × side, males F(1,20) = 20.46, p = 0.0002; females F(1,22) = 11.23, p = 0.0029). However, male CKO mice transitioned about twice as often into the two outer chambers during the sociability phase (Figure 1C, genotype, F(1,20) = 9.46, p = 0.006; genotype × side, F(1,20) = 4.58, p = 0.0448). Chamber entries during the social novelty preference phase were increased in male CKO mice, as well (Figure 1E, genotype, F(1,20) = 11.44, p = 0.003). Taken together, these data indicate that loss of GABA co-transmission from ACh neurons impaired social novelty preference, but not sociability, independent of sex. Additionally, we saw increased chamber entries in male CKO mice.
GABA co-transmission from ACh neurons is required for spatial learning and memory
Previous work has shown that stimulation of septohippocampal ACh neuron terminals evoked biphasic ACh/GABA postsynaptic currents in oriens lacunosum moleculare (OLM) interneurons, which play an important role in information flow through the hippocampus/entorhinal cortex circuitry (EC, Haam et al., 2018; Takacs et al., 2018). Thus, we wanted to test whether a loss of the co-transmitted GABA from ACh neurons affected hippocampal function. We used the Morris water maze to assess swimming ability, spatial learning, and memory, as well as reversal learning (Figures 2A–C, Brandeis et al., 1989; Hollup et al., 2001; Vorhees and Williams, 2014). On day 1 of the visible platform test, female CKO mice needed significantly more time to escape (genotype, F(1,22) = 9.46, p = 0.0055; genotype × side, F(1,22) = 7.33, p = 0.0129, Supplementary Table 3). However, on the following day, all groups learned the visible platform test similarly well with comparable swim speed (Supplementary Table 3). The escape latencies and the number of training days were not significantly different for either sex or genotype during the hidden platform test (Figures 2D,E). During the first probe trial, ctrl mice showed a significant preference for swim path crossings over the platform area in the target quadrant compared to the corresponding area in the opposite quadrant (Figure 2F, genotype × quadrant, males, F(1,19) = 6.72; p = 0.0179, females, F(1,22) = 10.53, p = 0.0037). In contrast, CKO mice did not show a preference for the target quadrant over the opposite quadrant (Figure 2F). Furthermore, female CKO mice had significantly more crossings over the incorrect area in the opposite quadrant than female ctrl mice (Figure 2F, genotype × quadrant, F(1,22) = 5.67, p = 0.0264).
During the reversal learning phase, male CKO and female mice showed a tendency to escape more slowly onto the hidden platform (Figures 2G,H). However, all animals escaped to the hidden platform within the 15 s criterion at the end of the 4-day reversal learning phase. Although CKO mice failed to demonstrate the preference for the target quadrant during the second probe (Figure 2I, males, the main effect of the quadrant, F(1,19) = 16.9, p = 0.0006; genotype × quadrant, F(1,19) = 5.09, p = 0.036; females, the main effect of the quadrant, F(1,22) = 16.9, p = 0.0005), the initial spatial memory deficit likely overshadowed any potential effects on reversal learning. Taken together, these data indicate that GABA co-transmission from ACh neurons is required for spatial memory.
GABA co-transmission from ACh neurons is not required for reward learning but stabilizes usage of response learning
The results of the Morris water maze test were suggestive of spatial learning deficits in CKO mice while the locomotive hyperactivity in the 3-chamber test suggests possible striatal impairments involving inhibitory behavioral control. In order to assess whether these deficits affected learning, we utilized a classic T-maze task that can be solved via either a hippocampus-dependent place strategy or a striatum-dependent response strategy and utilizes probe tests to determine which strategy is dominant (Packard and Mcgaugh, 1996). Recently, impaired inhibition in the dentate gyrus has been implied in decreased spatial learning during foraging behaviors (Albrecht et al., 2022). Because ACh release levels in the hippocampus and striatum can be used as predictors of which strategy is dominant, we hypothesized that the loss of GABA co-transmission may lead to changes in reward learning and learning strategy usage (Chang and Gold, 2003).
We did not find any differences in distance traveled during the two days of habituation (Supplementary Table 4). During the 14 days of training, we found no sex differences, and no differences in success rate except for a slight deficit trend on day 9 after the first probe trial (Figure 3C, p = 0.096, Repeated Measures Proportional Odds Logistic Regression with Bonferroni correction). The first probe trial revealed an overall preference for the response learning strategy but no significant differences between ctrl and CKO mice (Figure 3D, p = 0.303, Fisher’s exact test). During the second probe trial, strategy preferences roughly followed those of the first probe trial, with no significant differences between ctrl and CKO mice (Figure 3E, p = 0.294, Fisher’s exact test). We assessed the stability of the response vs. place strategy usage further by assigning every animal to one of these four groups (Figure 3F): place transitioner (“R→P”), consistent place learner (“Place”), consistent response learner (“Response”), response transitioner (“P→R”). There were significantly more consistent response learners among ctrl mice compared to CKO mice (p = 0.0434, Fisher’s exact test). This indicates that ctrl mice quickly adopted a striatal learning strategy by probe trial 1 and persisted in this strategy until probe trial 2, whereas CKO mice were less likely to maintain a consistent strategy. Taken together, these data indicate that a loss of GABA co-transmission from ACh neurons caused only minor changes in reward-associated learning in the T maze. However, the loss of GABA co-transmission from ACh neurons caused instability in hippocampal vs. striatal strategy selection. Since our results are not consistent with findings in rats, further studies are required to optimize the T maze task for mice.
GABA co-transmission for ACh neurons is not required for discrimination and reversal learning but regulates locomotion in males
We wanted to further assess the spontaneous behaviors as well as learning performance, and cognitive flexibility of mice in the absence of human interference (Remmelink et al., 2016). During a 4-day discrimination and reversal learning paradigm in an automated home-cage (Figure 4A), mice were trained to discriminate between rewarded or unrewarded response options followed by a reversal learning phase. During the discrimination learning (DL) phase, mice had to enter the correct (left) entrance into the Cognition Wall to receive food rewards (Figure 4B). After the first two days, the reversal learning (RL) phase started with the correct response option changed from the left to the right entrance.
First, we looked at the circadian rhythmicity of activity by assessing the distance moved over time (Figure 4C). All mice showed comparable activity patterns with the highest movement activity during the dark cycle. We investigated animal activity by comparing the total distance moved and found that male CKO mice moved significantly more than male ctrl mice during the experiment (Figure 4D, genotype, F(89,1260) = 27.66, p = 0.0409). Female ctrl mice showed higher activity compared to male ctrl mice (Figure 4D, sex, F (3,3060) = 113.5, p = 0.0106). Female CKO mice did not move significantly more than female ctrl mice. However, the total wall entry count was not significantly different between any of the groups (Figure 4E). During DL and RL, all groups reached the 80% learning criterion within a similar number of errors (Figure 4F) comparable to C57BL/6J mice (Remmelink et al., 2016). We did not find differences in rewards earned per day (Figure 4G).
Taken together, these data indicate that the loss of GABA co-transmission from ACh neurons does not affect discrimination or reversal learning in an automated home-cage experiment. However, we detected higher locomotive activity in male CKO mice without affecting wall entry or rewards earned. We, therefore, propose GABA co-transmission from ACh neurons may be required for locomotive or exploratory behaviors in males.
GABA co-transmission from ACh neurons is not required for acquisition or extinction of fear memories, but for context-dependent renewal of fear to a discrete auditory cue
During the initial behavioral assessment, CKO mice did not exhibit substantial alterations in the acquisition and retention of fear memories (Supplementary Figure 4). The other behavioral deficits in CKO mice, however, pointed towards disturbed communication between the hippocampus, the mPFC, and the striatum after the loss of GABA co-transmission from ACh neurons. The mPFC contributes to the modulation of the fear response, particularly through the counteracting roles of infralimbic cortex (IL) and prelimbic cortex (PL, Marek et al., 2018; Likhtik and Johansen, 2019; Vasquez et al., 2019). Since prior data indicated GABA/ACh co-transmission involvement in mPFC circuitry function, we wanted to further assess fear extinction and context-dependent fear renewal (Saunders et al., 2015a; Obermayer et al., 2019; Granger et al., 2020).
We found no sex differences and again observed normal acquisition (Figure 5B) as well as a similar reduction in freezing during repeated tone presentations during fear extinction (Figures 5C–E). However, when placed back into the original training context for a test of context-dependent fear renewal, the CKO mice showed a transient elevation of freezing during the first tone presentation (Figure 5F, a priori planned comparison of tone 1, genotype: F(1,35) = 5.293, p = 0.027). These findings indicate that GABA co-transmission from ACh neurons may contribute to fear renewal.
Discussion
Here, we studied the role of GABA co-transmission from ACh neurons in behavior. In the absence of GABA co-transmission from ACh neurons, mice showed substantial impairments in social and spatial memory, less stability in learning strategy usage, as well as context-dependent fear renewal. Furthermore, we discovered a task-specific increase in locomotion exclusively in males.
Impairments in social novelty preference
The 3-chamber test revealed a loss in social novelty preference in CKO mice, but not a loss in sociability. Social novelty preference requires social recognition and involves computation by the hippocampal region dorsal CA2 before excitatory projections transfer information to the ventral CA1 (Alexander et al., 2016; Okuyama et al., 2016; Meira et al., 2018). CA2 receives inputs from the supramammillary nucleus, which is activated by novelty and influences θ oscillations during the exploration of novel stimuli (Vertes et al., 2004; Jeewajee et al., 2008; Ito et al., 2009). In addition, social novelty preference requires ACh stimulation from basal forebrain neurons to the hippocampus where α7 nAChRs on GABAergic neurons lead to disinhibition in CA2 (Nacer et al., 2021; Pimpinella et al., 2021). Other signaling cascades are involved in CA2 function and social memory, such as glucocorticoids, enkephalins, and neuropeptides like oxytocin and vasopressin (Pagani et al., 2015; Raam et al., 2017; Mccann et al., 2021; Leroy et al., 2022). Hippocampal sharp-wave ripples and output to the mPFC through γ oscillations depend on CA2 activity (Alexander et al., 2018; Joo and Frank, 2018). Although it is unclear whether GABA co-transmission is directly required for γ oscillations, blocking GABA transmission was sufficient to block sharp-wave ripples in vitro (Takacs et al., 2018).
Furthermore, social behaviors require adequate crosstalk from the ventral hippocampus to layer 5 of the mPFC (Phillips et al., 2019). The mPFC function or information input are disturbed in mouse models for the study of autism, Alzheimer’s disease, or other brain dysfunctions (Riedel et al., 2009; Gordon, 2011; Johnson et al., 2013; Li et al., 2015; Franklin et al., 2017; Kim et al., 2017; Liang et al., 2018; Phillips et al., 2019; Poppe et al., 2019; Bicks et al., 2020; Huang et al., 2020; Liu et al., 2020; Scheggia et al., 2020). Given the ACh/NMDA receptor interplay in mPFC glutamatergic signaling and social behaviors, GABA co-transmission may contribute to neuron tuning and is disrupted in autism models in mice and higher-order mammals (Brigman et al., 2009; Avale et al., 2011; Finlay et al., 2015; Liang et al., 2018; Okada et al., 2021; Cools and Arnsten, 2022; Sun et al., 2022). Additional processing of social information requires ventral CA1 projections to the nucleus accumbens (NAc), LDT projections to NAc, or down-stream processing in the lateral septum (Okuyama et al., 2016; Fernandez et al., 2018; Coimbra et al., 2019; Menon et al., 2022; Hunt et al., 2022). Taken together, the impairment of social novelty preference in CKO mice may be caused by impaired signaling in several brain regions, including the hippocampus or the mPFC.
Impairments in spatial learning and memory
The Morris water maze revealed spatial memory impairments in CKO mice. The hippocampus/EC circuitry is essential for allocentric navigation which is required for spatial learning and memory (O’keefe and Lynn, 1978; Leutgeb et al., 2005; Buzsaki and Moser, 2013; Chersi and Burgess, 2015). The hippocampal function requires well-timed ACh modulation (Hasselmo and Mcgaughy, 2004; Gu and Yakel, 2011). During exploration, the hippocampus and associated brain regions exhibit large type 1 θ oscillations involving M1 mAChR on hippocampal pyramidal neurons as well as α7 nAChR on OLM interneurons (Gu et al., 2017, 2020). Because OLM interneurons receive ACh/GABA co-transmission from MS/DBB inputs, co-transmitted GABA may also modulate hippocampal spike timing and output through OLM interneurons (Haam et al., 2018; Takacs et al., 2018).
The formation and consolidation of spatial memories require additional hippocampal and mPFC interplay through VIP+ neurons (Maviel et al., 2004; Lee et al., 2019; Malik et al., 2022). Although only ~10% of mPFC VIP+ neurons are ACh+, GABA co-transmission may modulate disinhibition or cause other effects in the mPFC (Obermayer et al., 2019; Granger et al., 2020). Taken together, loss of GABA co-transmission from ACh neurons likely impaired spatial memory through altering the hippocampus, mPFC, or both.
Task-specific increases in locomotive activity in males
We observed increased locomotion in male CKO mice only in the 3-chamber test and the automated home-cage assay. Consistent with the literature, however, female ctrl mice were generally more active than male ctrl mice in the automated home-cage assay (Caldarone et al., 2008; Rosenfeld, 2017; Warncke et al., 2021; Holcomb et al., 2022; Stevanovic et al., 2022). Changes in locomotion have been reported in drug abuse models, in response to disturbed striatal DA transport and receptor function, and in patients with bipolar disorders or schizophrenia potentially through ventral striatum nAChR signaling or ventral pallidum (Napier, 1992; Jerlhag et al., 2006; Perry et al., 2009; Singer et al., 2012; Moreno et al., 2013; Moy et al., 2013; Amitai et al., 2014; DeLong and Wichmann, 2015). Furthermore, locomotion has been associated with PPN or LDTmodulation of SNcDA neuron firing (Dautan et al., 2016b; Estakhr et al., 2017; Le Gratiet et al., 2022). Therefore, the loss of GABA co-transmission from ACh neurons may affect locomotor activity by disrupting DA signaling in the brain.
Role of GABA co-transmission in competing learning strategies
The animal’s ability to employ place or response learning to solve a goal-directed task has intrigued researchers for a long time (Chersi and Burgess, 2015; Goodman, 2020). Overall, mice showed a preference for striatal response learning in the T maze, but CKO mice were less consistent in their strategic choices.
During striatal learning, the dorsolateral striatum undergoes changes in neuron firing, cell signaling, as well as epigenetic modifications (Aosaki et al., 1994b; Jog et al., 1999; Kheirbek et al., 2009; Zhang and Cragg, 2017; Malvaez et al., 2018). These changes likely enable the synchronization between the striatum and hippocampus leading to increased mPFC activity (Doeller and Burgess, 2008; Doeller et al., 2008; Rich and Shapiro, 2009; Goldenberg et al., 2020). Given the importance of ACh neurons in striatal learning and function, loss of GABA co-transmission may disrupt corticostriatal inhibition, striatal output, strengthen striatal extinction mechanisms, or reduce synchrony between the hippocampus, the mPFC, and striatum (Aosaki et al., 1994a; Chang and Gold, 2003; Lozovaya et al., 2018; Goldenberg et al., 2020; Fleming et al., 2022). We, therefore, conclude that GABA co-transmission is a minor regulator of response learning with possible relevance to altered learning strategies in addiction, autism spectrum disorders, schizophrenia, or PD (Graybiel and Rauch, 2000; Redgrave et al., 2010).
Role of GABA co-transmission in fear renewal
Disturbances in the underlying neural circuitry of fear response are associated with phobias or post-traumatic stress disorder (PTSD; Herry et al., 2010). We did not see changes in acquisition or fear extinction but found transient increases in context-dependent fear renewal in CKO mice.
Disturbances in ACh signaling in neurons or glia in the hippocampus, amygdala, and mPFC were found in cue encoding, spatial processing, aversive learning, extinction, and fear renewal (Lotfipour et al., 2013, 2017; Kutlu et al., 2018; Likhtik and Johansen, 2019; Titus et al., 2019; Chen et al., 2020; Kellis et al., 2020; Mineur et al., 2020; Oliveros-Matus et al., 2020; Miguelez Fernandez et al., 2021; Mooney-Leber et al., 2021; Zhang et al., 2021; Yanpallewar et al., 2022). For example, feed-forward inhibition from the ventral hippocampus to PL is sufficient to decrease contextual fear renewal, while feed-forward inhibition from the ventral hippocampus to IL increases fear renewal (Marek et al., 2018; Vasquez et al., 2019). Moreover, fear extinction and fear renewal are improved by GABABR inhibition at the beginning of the extinction period independent of the dorsal hippocampus or BLA (Adkins et al., 2021). We, therefore, propose that GABA co-transmission from ACh neurons may inhibit fear renewal in mice by coordinating hippocampus-mPFC crosstalk. Given that the changes in fear responses were transient, GABA co-transmission from ACh neurons likely has only a minor role in regulating contextual fear renewal to a conditioned tone.
Limitations of this study
Further studies are needed to assess the role of GABA co-transmission from ACh neurons in the brain. Recent work has shown that homozygous ChAT-IRES-Cre mice exhibit behavioral deficits when compared to C57BL/6J mice (Chen et al., 2018; Lhopitallier et al., 2022). While all mice in our experiments were littermates and either Cre-negative or carried a heterozygous Cre allele, we cannot rule out off-target effects of Cre expression. Although it has been shown that ChAT expression is not affected in heterozygous ChAT-IRES-Cre mice in the hippocampus, we cannot rule out that ChAT expression is unchanged in other tissues, and whether this has an impact on ACh neuron function in the brain or other tissues (Chen et al., 2018).
The efficacy of the vGAT knock-out mouse has been verified by various groups in ACh and other neurons in the brain or other tissues but it remains unknown how the loss of vGAT affects ACh neuron function and survival (Tong et al., 2008; Saunders et al., 2015a; Hirano et al., 2016; Lin et al., 2018; Holly et al., 2019; Duan et al., 2020; Granger et al., 2020; Hanson et al., 2020; Liu et al., 2020). Further studies need to assess whether the loss of vGAT in ACh neurons causes negative or compensatory effects such as excessive ACh release, or degeneration of ACh axons and synapses.
In addition, we cannot rule out that glycinergic signaling is negatively affected by the loss of vGAT in ACh neurons (Kumamoto and Murata, 1996; Cassell, 1999). According to single cell transcriptomics data of striatal interneurons, striatal ACh neurons do not express glycine transporters (Slc6a5, Slc6a9) but ionotropic glycine receptor subunits (Glra2, Glrb, Munoz-Manchado et al., 2018). However, it remains to be assessed whether glycinergic signaling is changed due to the loss of vGAT in ACh neurons and negatively affects brain function or development (Avila et al., 2013).
Lastly, if loss of vGAT caused dysfunctions in other ACh neuron populations, thalamo-cortical as well as thalamo-striatal circuitry may be affected by changes in ACh signaling from the PPN or the LDT (Dautan et al., 2014; 2016a,b; 2020; Huerta-Ocampo et al., 2020, 2021). Changes in locomotion may be caused by altered ACh signaling in spinal cordmotor neurons (Mille et al., 2021). Therefore, further studies are required to elucidate how ACh neurons and particularly signaling molecules co-transmitted from ACh neurons are embedded into brain circuitry and contribute to brain function.
Role of GABA co-transmission in the brain
Taken together, our findings support the importance of GABA co-transmission from ACh projection and interneurons in the brain and agree with previously published work (see Figure 6 for a simplified model). Loss of GABA co-transmission from ACh neurons may impair hippocampal functions associated with spatial and social memory due to alterations in OLM interneuron function (Takacs et al., 2018). This likely affects both memory formation and consolidation through dysregulation of the dorsal hippocampus/EC as well as the ventral hippocampus-mPFC or nucleus accumbens outputs (Phillips et al., 2019; Sosa et al., 2020). Furthermore, synchronized oscillatory events associated with social or spatial information may have been altered (Gu et al., 2017, 2020; Alexander et al., 2018; Haam et al., 2018). Since oscillatory activities in different brain regions are highly correlated, altered hippocampal output may negatively affect neural activity in downstream targets as well as feedback to the hippocampus (Lee et al., 2019; Sosa et al., 2020; Malik et al., 2022).
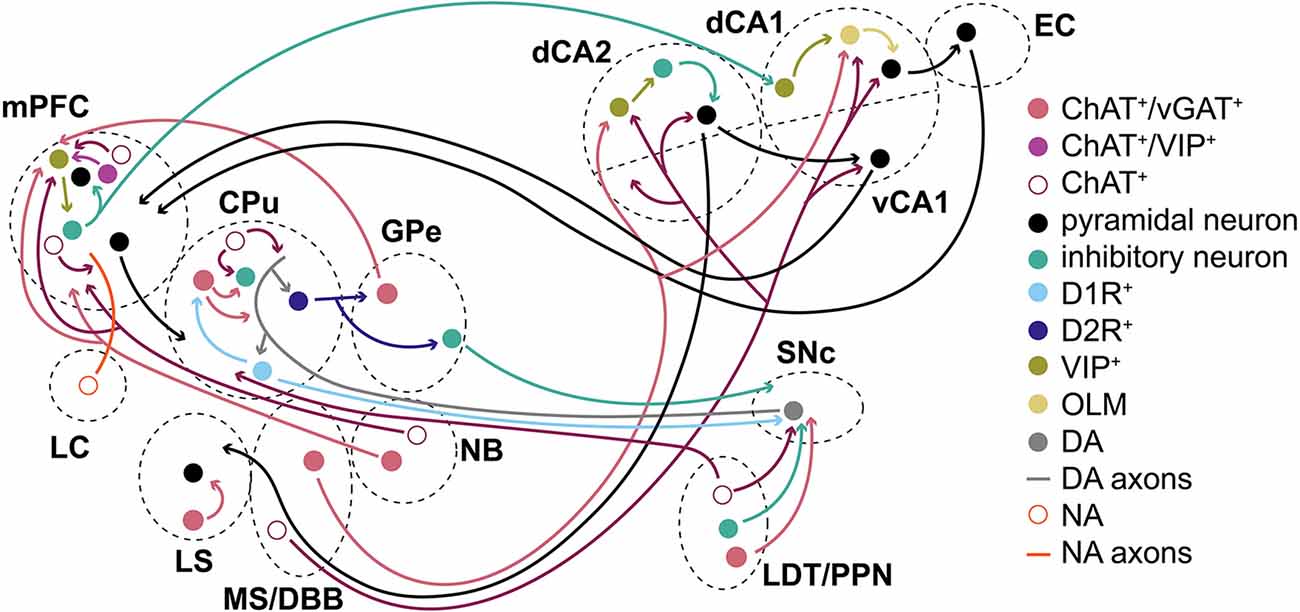
Figure 6. Summary/simplified model. Simplified model of how ACh/GABA co-transmission is embedded in the circuitry of cortical and subcortical structures. ACh/GABA projections from the basal forebrain (MS/DBB and NB) form synapses in the hippocampus and mPFC, respectively. The hippocampal outputs vary based on dorsal or ventral location of the neurons to mPFC or EC, among others. Basal ganglia structures such as CPu or GPe contain either local ACh/GABA interneurons, receive inputs from LDT/PPN, or project to the mPFC. The mPFC contains local ACh/GABA interneurons and receives noradrenergic (NA) inputs from the locus coeruleus (LC). SNc DA neurons receive ACh/GABA projections from LDT/PPN.
The striatal function was likely impaired by decreased cortical disinhibition through GABA+/ACh+ interneurons during the pause response of ACh neurons and may have implications in PD or Tourette syndrome (Lennington et al., 2016; Lozovaya et al., 2018). Alternatively, LDT and PPN ACh/GABA co-transmission may modulate SNc DA neurons or thalamo-striatal functions (Dautan et al., 2016a; Estakhr et al., 2017; Le Gratiet et al., 2022). The role of GABA co-transmission from ACh neurons in GPe and NB in circuit function remains unclear (Saunders et al., 2015a, b). While the GPe output to the mPFC could provide negative feedback from the striatum through the indirect pathway during habit formation, NB may add spike timing refinement in mPFC L1. Similarly, local mPFC GABA co-transmission mostly targets L1 interneurons (Obermayer et al., 2019; Granger et al., 2020). Given the sparsity with which L1 mPFC interneurons receive inputs, one may wonder about the physiological relevance in adult mice. However, GABA co-transmission may further shape the circuit during critical windows in development, as recently shown for GABAergic transmission in the mPFC or motor cortex (Bicks et al., 2020; Steinecke et al., 2022). Higher-order mammals, such as rats, could potentially co-transmit more GABA from ACh neurons (Bayraktar et al., 1997; Obermayer et al., 2019; Dienel et al., 2021).
In the future, it may be beneficial to assess how GABA co-transmitting ACh neurons are integrated into the brain circuitry. By studying the anatomy of cell distributions and synaptic projections as well as the functional consequences for pre- or postsynaptic targets, GABA co-transmission from ACh neurons may be better understood. The behavioral relevance of GABA co-transmission could be further elucidated by restricting its loss to specific brain regions or cell populations. Lastly, future studies may help to determine the developmental role of GABA co-transmission during neurocircuit formation.
Data Availability Statement
The raw data supporting the conclusions of this article will be made available by the authors, without undue reservation.
Ethics Statement
The animal study was reviewed and approved by NIEHS Animal Care and Use Committee, UNC Institutional Animal Care and Use Committee.
Author Contributions
RG, JY, JC, and SM: conceptualization. RG, KH, BB, and SF: data curation. RG, SM, JC, and PL: analysis. RG: figures and writing. All authors contributed to the article and approved the submitted version.
Funding
This research was supported by the Intramural Research Program of the National Institutes of Health (NIH; grant number Z01ES090089 to JY), National Institute of Environmental Health Sciences, the Center on Compulsive Behaviors, NIH via NIH Director’s Challenge Award (RG), and the UNC Intellectual and Developmental Disabilities Research Center (NICHD; P50 HD103573; PI: Joseph Piven).
Acknowledgments
We thank for statistical or bioinformatics support by Drs. Min Shi and Jian-Liang Li at NIEHS as well as Drs. Sandra McBride, Guanhua Xie, and Kathryn Konrad (contract #: GS-00F-173CA/75N96022F00055). We are grateful for assistance with animal husbandry/feeding/care provided by Scotty D. Dowdy, Maria G. Barrientos Sandoval, Steven E. Butler, Rodriguez Q. Sutton, as well as the NIEHS Comparative Medicine Branch. We thank Makayla Wood for technical assistance. We thank Drs. Shiyi Wang, Serena M. Dudek, as well as Yakel lab members for comments on the manuscript. The content of the manuscript has previously appeared online as a preprint: https://www.biorxiv.org/content/10.1101/2022.10.07.511349v.
Conflict of Interest
The authors declare that the research was conducted in the absence of any commercial or financial relationships that could be construed as a potential conflict of interest.
Publisher’s Note
All claims expressed in this article are solely those of the authors and do not necessarily represent those of their affiliated organizations, or those of the publisher, the editors and the reviewers. Any product that may be evaluated in this article, or claim that may be made by its manufacturer, is not guaranteed or endorsed by the publisher.
Footnotes
Supplementary Material
The Supplementary Material for this article can be found online at: https://www.frontiersin.org/articles/10.3389/fnbeh.2022.1067409/full#supplementary-material.
References
Adkins, J. M., Lynch, J., Gray, M. 3rd, and Jasnow, A. M. (2021). Presynaptic GABAB receptor inhibition sex dependently enhances fear extinction attenuates fear renewal. Psychopharmacology (Berl) 238, 2059–2071. doi: 10.1007/s00213-021-05831-w
Albrecht, A., Müller, I., Weiglein, A., Pollali, E., Çalşkan, G., and Stork, O. (2022). Choosing memory retrieval strategies: a critical role for inhibition in the dentate gyrus. Neurobiol. Stress 20:100474. doi: 10.1016/j.ynstr.2022.100474
Alexander, G. M., Brown, L. Y., Farris, S., Lustberg, D., Pantazis, C., Gloss, B., et al. (2018). CA2 neuronal activity controls hippocampal low gamma and ripple oscillations. eLife 7:e38052. doi: 10.7554/eLife.38052
Alexander, G. M., Farris, S., Pirone, J. R., Zheng, C., Colgin, L. L., Dudek, S. M., et al. (2016). Social novel contexts modify hippocampal CA2 representations of space. Nat. Commun. 7:10300. doi: 10.1038/ncomms10300
Amitai, N., Young, J. W., Higa, K., Sharp, R. F., Geyer, M. A., Powell, S. B., et al. (2014). Isolation rearing effects on probabilistic learning cognitive flexibility in rats. Cogn. Affect. Behav. Neurosci. 14, 388–406. doi: 10.3758/s13415-013-0204-4
Aosaki, T., Graybiel, A. M., and Kimura, M. (1994a). Effect of the nigrostriatal dopamine system on acquired neural responses in the striatum of behaving monkeys. Science 265, 412–415. doi: 10.1126/science.8023166
Aosaki, T., Tsubokawa, H., Ishida, A., Watanabe, K., Graybiel, A. M., Kimura, M., et al. (1994b). Responses of tonically active neurons in the primate’s striatum undergo systematic changes during behavioral sensorimotor conditioning. J. Neurosci. 14, 3969–3984. doi: 10.1523/JNEUROSCI.14-06-03969.1994
Avale, M. E., Chabout, J., Pons, S., Serreau, P., De Chaumont, F., Olivo-Marin, J. C., et al. (2011). Prefrontal nicotinic receptors control novel social interaction between mice. FASEB J. 25, 2145–2155. doi: 10.1096/fj.10-178558
Avila, A., Nguyen, L., and Rigo, J. M. (2013). Glycine receptors brain development. Front. Cell. Neurosci. 7:184. doi: 10.3389/fncel.2013.00184
Ballinger, E. C., Ananth, M., Talmage, D. A., and Role, L. W. (2016). Basal forebrain cholinergic circuits signaling in cognition cognitive decline. Neuron 91, 1199–1218. doi: 10.1016/j.neuron.2016.09.006
Bayraktar, T., Staiger, J. F., Acsady, L., Cozzari, C., Freund, T. F., Zilles, K., et al. (1997). Co-localization of vasoactive intestinal polypeptide, γ-aminobutyric acid choline acetyltransferase in neocortical interneurons of the adult rat. Brain Res. 757, 209–217. doi: 10.1016/s0006-8993(97)00218-7
Bicks, L. K., Yamamuro, K., Flanigan, M. E., Kim, J. M., Kato, D., Lucas, E. K., et al. (2020). Prefrontal parvalbumin interneurons require juvenile social experience to establish adult social behavior. Nat. Commun. 11:1003. doi: 10.1038/s41467-020-14740-z
Brandeis, R., Brandys, Y., and Yehuda, S. (1989). The use of the morris water maze in the study of memory learning. Int. J. Neurosci. 48, 29–69. doi: 10.3109/00207458909002151
Brigman, J. L., Ihne, J., Saksida, L. M., Bussey, T. J., and Holmes, A. (2009). Effects of subchronic phencyclidine (PCP) treatment on social behaviors operant discrimination reversal learning in C57BL/6J mice. Front. Behav. Neurosci. 3:2. doi: 10.3389/neuro.08.002.2009
Brunner, D., Kabitzke, P., He, D., Cox, K., Thiede, L., Hanania, T., et al. (2015). Comprehensive analysis of the 16p11.2 deletion null cntnap2 mouse models of autism spectrum disorder. PLoS One 10:e0134572. doi: 10.1371/journal.pone.0134572
Burnstock, G. (1976). Do some nerve cells release more than one transmitter? Neuroscience 1, 239–248. doi: 10.1016/0306-4522(76)90054-3
Buzsaki, G., and Moser, E. I. (2013). Memory, navigation theta rhythm in the hippocampal-entorhinal system. Nat. Neurosci. 16, 130–138. doi: 10.1038/nn.3304
Caldarone, B. J., King, S. L., and Picciotto, M. R. (2008). Sex differences in anxiety-like behavior locomotor activity following chronic nicotine exposure in mice. Neurosci. Lett. 439, 187–191. doi: 10.1016/j.neulet.2008.05.023
Carter, M. D., Shah, C. R., Muller, C. L., Crawley, J. N., Carneiro, A. M., Veenstra-Vanderweele, J., et al. (2011). Absence of preference for social novelty increased grooming in integrin β3 knockout mice: initial studies future directions. Autism Res. 4, 57–67. doi: 10.1002/aur.180
Cassell, M. D. (1999). Glycine receptor (gephyrin) immunoreactivity is present on cholinergic neurons in the dorsal vagal complex. Neuroscience 95, 489–497. doi: 10.1016/s0306-4522(99)00454-6
Chang, Q., and Gold, P. E. (2003). Switching memory systems during learning: changes in patterns of brain acetylcholine release in the hippocampus striatum in rats. J. Neurosci. 23, 3001–3005. doi: 10.1523/JNEUROSCI.23-07-03001.2003
Chen, E., Lallai, V., Sherafat, Y., Grimes, N. P., Pushkin, A. N., Fowler, J. P., et al. (2018). Altered baseline nicotine-mediated behavioral cholinergic profiles in ChAT-Cre mouse lines. J. Neurosci. 38, 2177–2188. doi: 10.1523/JNEUROSCI.1433-17.2018
Chen, S., Lin, Z., Tan, K. L., Chen, R., Su, W., Zhao, H., et al. (2020). Enhanced contextual fear memory elevated astroglial glutamate synthase activity in hippocampal CA1 BChE shRNA knockdown mice. Front. Psychiatry 11:564843. doi: 10.3389/fpsyt.2020.564843
Cheng, Q., and Yakel, J. L. (2015). Activation of α7 nicotinic acetylcholine receptors increases intracellular cAMP levels via activation of AC1 in hippocampal neurons. Neuropharmacology 95, 405–414. doi: 10.1016/j.neuropharm.2015.04.016
Chersi, F., and Burgess, N. (2015). The cognitive architecture of spatial navigation: hippocampal striatal contributions. Neuron 88, 64–77. doi: 10.1016/j.neuron.2015.09.021
Coimbra, B., Soares-Cunha, C., Vasconcelos, N. A. P., Domingues, A. V., Borges, S., Sousa, N., et al. (2019). Role of laterodorsal tegmentum projections to nucleus accumbens in reward-related behaviors. Nat. Commun. 10:4138. doi: 10.1038/s41467-019-11557-3
Cools, R., and Arnsten, A. F. T. (2022). Neuromodulation of prefrontal cortex cognitive function in primates: the powerful roles of monoamines acetylcholine. Neuropsychopharmacology 47, 309–328. doi: 10.1038/s41386-021-01100-8
Dautan, D., Hacioglu Bay, H., Bolam, J. P., Gerdjikov, T. V., and Mena-Segovia, J. (2016a). Extrinsic sources of cholinergic innervation of the striatal complex: a whole-brain mapping analysis. Front. Neuroanat. 10:1. doi: 10.3389/fnana.2016.00001
Dautan, D., Souza, A. S., Huerta-Ocampo, I., Valencia, M., Assous, M., Witten, I. B., et al. (2016b). Segregated cholinergic transmission modulates dopamine neurons integrated in distinct functional circuits. Nat. Neurosci. 19, 1025–1033. doi: 10.1038/nn.4335
Dautan, D., Huerta-Ocampo, I., Gut, N. K., Valencia, M., Kondabolu, K., Kim, Y., et al. (2020). Cholinergic midbrain afferents modulate striatal circuits shape encoding of action strategies. Nat. Commun. 11:1739. doi: 10.1038/s41467-020-15514-3
Dautan, D., Huerta-Ocampo, I., Witten, I. B., Deisseroth, K., Bolam, J. P., Gerdjikov, T., et al. (2014). A major external source of cholinergic innervation of the striatum nucleus accumbens originates in the brainstem. J. Neurosci. 34, 4509–4518. doi: 10.1523/JNEUROSCI.5071-13.2014
DeLong, M. R., and Wichmann, T. (2015). Basal ganglia circuits as targets for neuromodulation in parkinson disease. JAMA Neurol. 72, 1354–1360. doi: 10.1001/jamaneurol.2015.2397
Dienel, S. J., Ciesielski, A. J., Bazmi, H. H., Profozich, E. A., Fish, K. N., Lewis, D. A., et al. (2021). Distinct laminar cellular patterns of gaba neuron transcript expression in monkey prefrontal visual cortices. Cereb. Cortex 31, 2345–2363. doi: 10.1093/cercor/bhaa341
Doeller, C. F., and Burgess, N. (2008). Distinct error-correcting incidental learning of location relative to landmarks boundaries. Proc. Natl. Acad. Sci. U S A 105, 5909–5914. doi: 10.1073/pnas.0711433105
Doeller, C. F., King, J. A., and Burgess, N. (2008). Parallel striatal hippocampal systems for landmarks boundaries in spatial memory. Proc. Natl. Acad. Sci. U S A 105, 5915–5920. doi: 10.1073/pnas.0801489105
Duan, Z. R. S., Che, A., Chu, P., Modol, L., Bollmann, Y., Babij, R., et al. (2020). GABAergic restriction of network dynamics regulates interneuron survival in the developing cortex. Neuron 105, e575–e592. doi: 10.1016/j.neuron.2019.10.008
Ellis, J. L., and Burnstock, G. (1989). Angiotensin neuromodulation of adrenergic purinergic co-transmission in the guinea-pig vas deferens. Br. J. Pharmacol. 97, 1157–1164. doi: 10.1111/j.1476-5381.1989.tb12574.x
Estakhr, J., Abazari, D., Frisby, K., Mcintosh, J. M., and Nashmi, R. (2017). Differential control of dopaminergic excitability locomotion by cholinergic inputs in mouse substantia nigra. Curr. Biol. 27, e41900–e41914. doi: 10.1016/j.cub.2017.05.084
Fernandez, S. P., Broussot, L., Marti, F., Contesse, T., Mouska, X., Soiza-Reilly, M., et al. (2018). Mesopontine cholinergic inputs to midbrain dopamine neurons drive stress-induced depressive-like behaviors. Nat. Commun. 9:4449. doi: 10.1038/s41467-018-06809-7
Finlay, J. M., Dunham, G. A., Isherwood, A. M., Newton, C. J., Nguyen, T. V., Reppar, P. C., et al. (2015). Effects of prefrontal cortex hippocampal NMDA NR1-subunit deletion on complex cognitive social behaviors. Brain Res. 1600, 70–83. doi: 10.1016/j.brainres.2014.10.037
Fleming, W., Lee, J., Briones, B. A., Bolkan, S. S., and Witten, I. B. (2022). Cholinergic interneurons mediate cocaine extinction in male mice through plasticity across medium spiny neuron subtypes. Cell Rep. 39:110874. doi: 10.1016/j.celrep.2022.110874
Franklin, T. B., Silva, B. A., Perova, Z., Marrone, L., Masferrer, M. E., Zhan, Y., et al. (2017). Prefrontal cortical control of a brainstem social behavior circuit. Nat. Neurosci. 20, 260–270. doi: 10.1038/nn.4470
Goldenberg, J. E., Lentzou, S., Ackert-Smith, L., Knowlton, H., and Dash, M. B. (2020). Interindividual differences in memory system local field potential activity predict behavioral strategy on a dual-solution T-maze. Hippocampus 30, 1313–1326. doi: 10.1002/hipo.23258
Goodman, J. (2020). Place vs. response learning: history, controversy neurobiology. Front. Behav. Neurosci. 14:598570. doi: 10.3389/fnbeh.2020.598570
Gordon, J. A. (2011). Oscillations hippocampal-prefrontal synchrony. Curr. Opin. Neurobiol. 21, 486–491. doi: 10.1016/j.conb.2011.02.012
Granger, A. J., Wang, W., Robertson, K., El-Rifai, M., Zanello, A. F., Bistrong, K., et al. (2020). Cortical ChAT+ neurons co-transmit acetylcholine GABA in a target- brain-region-specific manner. eLife 9:e57749. doi: 10.7554/eLife.57749
Graybiel, A. M., and Rauch, S. L. (2000). Toward a neurobiology of obsessive-compulsive disorder. Neuron 28, 343–347. doi: 10.1016/s0896-6273(00)00113-6
Gu, Z., Alexander, G. M., Dudek, S. M., and Yakel, J. L. (2017). Hippocampus entorhinal cortex recruit cholinergic NMDA receptors separately to generate hippocampal theta oscillations. Cell Rep. 21, 3585–3595. doi: 10.1016/j.celrep.2017.11.080
Gu, Z., and Yakel, J. L. (2011). Timing-dependent septal cholinergic induction of dynamic hippocampal synaptic plasticity. Neuron 71, 155–165. doi: 10.1016/j.neuron.2011.04.026
Gu, Z., Smith, K. G., Alexander, G. M., Guerreiro, I., Dudek, S. M., Gutkin, B., et al. (2020). Hippocampal interneuronal α7 nAChRs modulate theta oscillations in freely moving mice. Cell Rep. 31:107740. doi: 10.1016/j.celrep.2020.107740
Guillem, K., Bloem, B., Poorthuis, R. B., Loos, M., Smit, A. B., Maskos, U., et al. (2011). Nicotinic acetylcholine receptor β2 subunits in the medial prefrontal cortex control attention. Science 333, 888–891. doi: 10.1126/science.1207079
Haam, J., Zhou, J., Cui, G., and Yakel, J. L. (2018). Septal cholinergic neurons gate hippocampal output to entorhinal cortex via oriens lacunosum moleculare interneurons. Proc. Natl. Acad. Sci. U S A 115, E1886–E1895. doi: 10.1073/pnas.1712538115
Hanson, E., Swanson, J., and Arenkiel, B. R. (2020). GABAergic input from the basal forebrain promotes the survival of adult-born neurons in the mouse olfactory bulb. Front. Neural Circuits 14:17. doi: 10.3389/fncir.2020.00017
Hasselmo, M. E., and Mcgaughy, J. (2004). High acetylcholine levels set circuit dynamics for attention encoding low acetylcholine levels set dynamics for consolidation. Prog. Brain Res. 145, 207–231. doi: 10.1016/S0079-6123(03)45015-2
Herry, C., Ferraguti, F., Singewald, N., Letzkus, J. J., Ehrlich, I., Luthi, A., et al. (2010). Neuronal circuits of fear extinction. Eur. J. Neurosci. 31, 599–612. doi: 10.1111/j.1460-9568.2010.07101.x
Hirano, A. A., Liu, X., Boulter, J., Grove, J., Perez De Sevilla Muller, L., Barnes, S., et al. (2016). Targeted deletion of vesicular GABA transporter from retinal horizontal cells eliminates feedback modulation of photoreceptor calcium channels. eNeuro 3:ENEURO.0148–15.2016. doi: 10.1523/ENEURO.0148-15.2016
Holcomb, L. E., Rowe, P., O’neill, C. C., Dewitt, E. A., and Kolwicz, S. C., Jr. (2022). Sex differences in endurance exercise capacity skeletal muscle lipid metabolism in mice. Physiol. Rep. 10:e15174. doi: 10.14814/phy2.15174
Hollup, S. A., Molden, S., Donnett, J. G., Moser, M. B., and Moser, E. I. (2001). Place fields of rat hippocampal pyramidal cells spatial learning in the watermaze. Eur. J. Neurosci. 13, 1197–1208. doi: 10.1046/j.0953-816x.2001.01487.x
Holly, E. N., Davatolhagh, M. F., Choi, K., Alabi, O. O., Vargas Cifuentes, L., Fuccillo, M. V., et al. (2019). Striatal low-threshold spiking interneurons regulate goal-directed learning. Neuron 103, e692–e6101. doi: 10.1016/j.neuron.2019.04.016
Huang, W. C., Zucca, A., Levy, J., and Page, D. T. (2020). Social behavior is modulated by valence-encoding mPFC-amygdala sub-circuitry. Cell Rep. 32:107899. doi: 10.1016/j.celrep.2020.107899
Huerta-Ocampo, I., Dautan, D., Gut, N. K., Khan, B., and Mena-Segovia, J. (2021). Whole-brain mapping of monosynaptic inputs to midbrain cholinergic neurons. Sci. Rep. 11:9055. doi: 10.1038/s41598-021-88374-6
Huerta-Ocampo, I., Hacioglu-Bay, H., Dautan, D., and Mena-Segovia, J. (2020). Distribution of midbrain cholinergic axons in the thalamus. eNeuro 7:ENEURO.0454–19.2019. doi: 10.1523/ENEURO.0454-19.2019
Hunt, P. J., Kochukov, M., Pekarek, B. T., Belfort, B. D. W., Romero, J. M., Swanson, J. L., et al. (2022). Co-transmitting neurons in the lateral septal nucleus exhibit features of neurotransmitter switching. IBRO Neurosci. Rep. 12, 390–398. doi: 10.1016/j.ibneur.2022.05.003
Ito, M., Shirao, T., Doya, K., and Sekino, Y. (2009). Three-dimensional distribution of Fos-positive neurons in the supramammillary nucleus of the rat exposed to novel environment. Neurosci. Res. 64, 397–402. doi: 10.1016/j.neures.2009.04.013
Jaramillo, T. C., Speed, H. E., Xuan, Z., Reimers, J. M., Escamilla, C. O., Weaver, T. P., et al. (2017). Novel Shank3 mutant exhibits behaviors with face validity for autism altered striatal hippocampal function. Autism Res. 10, 42–65. doi: 10.1002/aur.1664
Jeewajee, A., Lever, C., Burton, S., O’keefe, J., and Burgess, N. (2008). Environmental novelty is signaled by reduction of the hippocampal theta frequency. Hippocampus 18, 340–348. doi: 10.1002/hipo.20394
Jerlhag, E., Egecioglu, E., Dickson, S. L., Andersson, M., Svensson, L., Engel, J. A., et al. (2006). Ghrelin stimulates locomotor activity accumbal dopamine-overflow via central cholinergic systems in mice: implications for its involvement in brain reward. Addict. Biol. 11, 45–54. doi: 10.1111/j.1369-1600.2006.00002.x
Jog, M. S., Kubota, Y., Connolly, C. I., Hillegaart, V., and Graybiel, A. M. (1999). Building neural representations of habits. Science 286, 1745–1749. doi: 10.1126/science.286.5445.1745
Johnson, A. W., Jaaro-Peled, H., Shahani, N., Sedlak, T. W., Zoubovsky, S., Burruss, D., et al. (2013). Cognitive motivational deficits together with prefrontal oxidative stress in a mouse model for neuropsychiatric illness. Proc. Natl. Acad. Sci. U S A 110, 12462–12467. doi: 10.1073/pnas.1307925110
Joo, H. R., and Frank, L. M. (2018). The hippocampal sharp wave-ripple in memory retrieval for immediate use consolidation. Nat. Rev. Neurosci. 19, 744–757. doi: 10.1038/s41583-018-0077-1
Kellis, D. M., Kaigler, K. F., Witherspoon, E., Fadel, J. R., and Wilson, M. A. (2020). Cholinergic neurotransmission in the basolateral amygdala during cued fear extinction. Neurobiol. Stress 13:100279. doi: 10.1016/j.ynstr.2020.100279
Kheirbek, M. A., Britt, J. P., Beeler, J. A., Ishikawa, Y., Mcgehee, D. S., Zhuang, X., et al. (2009). Adenylyl cyclase type 5 contributes to corticostriatal plasticity striatum-dependent learning. J. Neurosci. 29, 12115–12124. doi: 10.1523/JNEUROSCI.3343-09.2009
Kim, K. C., Cho, K. S., Yang, S. M., Gonzales, E. L., Valencia, S., Eun, P. H., et al. (2017). Sex differences in autism-like behavioral phenotypes postsynaptic receptors expression in the prefrontal cortex of TERT transgenic mice. Biomol. Ther. (Seoul) 25, 374–382. doi: 10.4062/biomolther.2016.242
Kumamoto, E., and Murata, Y. (1996). Glycine current in rat septal cholinergic neuron in culture: monophasic positive modulation by Zn2+. J. Neurophysiol. 76, 227–241.
Kutlu, M. G., Tumolo, J. M., Cann, C., and Gould, T. J. (2018). Differential effects of α4β2 nicotinic receptor antagonists partial-agonists on contextual fear extinction in male C57BL/6 mice. Psychopharmacology (Berl) 235, 1211–1219. doi: 10.1007/s00213-018-4837-4
Le Gratiet, K. L., Anderson, C. K., Puente, N., Grandes, P., Copas, C., Nahirney, P. C., et al. (2022). Differential subcellular distribution release dynamics of co-transmitted cholinergic gabaergic synaptic inputs modifies dopaminergic neuronal excitability. J. Neurosci. 235. [Online ahead of print]. doi: 10.1523/JNEUROSCI.2514-21.2022.
Lee, A. T., Cunniff, M. M., See, J. Z., Wilke, S. A., Luongo, F. J., Ellwood, I. T., et al. (2019). VIP interneurons contribute to avoidance behavior by regulating information flow across hippocampal-prefrontal networks. Neuron 102, e41223–e41234. doi: 10.1016/j.neuron.2019.04.001
Lee, S., Kim, K., and Zhou, Z. J. (2010). Role of ACh-GABA cotransmission in detecting image motion motion direction. Neuron 68, 1159–1172. doi: 10.1016/j.neuron.2010.11.031
Lennington, J. B., Coppola, G., Kataoka-Sasaki, Y., Fernandez, T. V., Palejev, D., Li, Y., et al. (2016). Transcriptome analysis of the human striatum in tourette syndrome. Biol. Psychiatry 79, 372–382. doi: 10.1016/j.biopsych.2014.07.018
Leroy, F., De Solis, C. A., Boyle, L. M., Bock, T., Lofaro, O. M., Buss, E. W., et al. (2022). Enkephalin release from VIP interneurons in the hippocampal CA2/3a region mediates heterosynaptic plasticity social memory. Mol. Psychiatry 27, 2879–2900. doi: 10.1038/s41380-021-01124-y
Leutgeb, S., Leutgeb, J. K., Moser, M. B., and Moser, E. I. (2005). Place cells, spatial maps the population code for memory. Curr. Opin. Neurobiol. 15, 738–746. doi: 10.1016/j.conb.2005.10.002
Lhopitallier, C., Perrault, C., Chauveau, F., Saurini, F., Berrard, S., Granon, S., et al. (2022). Characterization of social behavior in young middle-aged ChAT-IRES-Cre mouse. PLoS One 17:e0272141. doi: 10.1371/journal.pone.0272141
Li, F., Eriksen, J., Finer-Moore, J., Chang, R., Nguyen, P., Bowen, A., et al. (2020). Ion transport regulation in a synaptic vesicle glutamate transporter. Science 368, 893–897. doi: 10.1126/science.aba9202
Li, M., Long, C., and Yang, L. (2015). Hippocampal-prefrontal circuit disrupted functional connectivity in psychiatric neurodegenerative disorders. Biomed. Res. Int. 2015:810548. doi: 10.1155/2015/810548
Li, X., Yu, B., Sun, Q., Zhang, Y., Ren, M., Zhang, X., et al. (2018). Generation of a whole-brain atlas for the cholinergic system mesoscopic projectome analysis of basal forebrain cholinergic neurons. Proc. Natl. Acad. Sci. U S A 115, 415–420. doi: 10.1073/pnas.1703601115
Liang, B., Zhang, L., Barbera, G., Fang, W., Zhang, J., Chen, X., et al. (2018). Distinct dynamic on off neural ensembles in the prefrontal cortex code social exploration. Neuron 100, e9700–e9714. doi: 10.1016/j.neuron.2018.08.043
Likhtik, E., and Johansen, J. P. (2019). Neuromodulation in circuits of aversive emotional learning. Nat. Neurosci. 22, 1586–1597. doi: 10.1038/s41593-019-0503-3
Lin, T. W., Tan, Z., Barik, A., Yin, D. M., Brudvik, E., Wang, H., et al. (2018). Regulation of synapse development by vgat deletion from ErbB4-positive interneurons. J. Neurosci. 38, 2533–2550. doi: 10.1523/JNEUROSCI.0669-17.2018
Liu, C., Cai, X., Ritzau-Jost, A., Kramer, P. F., Li, Y., Khaliq, Z. M., et al. (2022). An action potential initiation mechanism in distal axons for the control of dopamine release. Science 375, 1378–1385. doi: 10.1126/science.abn0532
Liu, L., Xu, H., Wang, J., Li, J., Tian, Y., Zheng, J., et al. (2020). Cell type-differential modulation of prefrontal cortical GABAergic interneurons on low gamma rhythm social interaction. Sci. Adv. 6:eaay4073. doi: 10.1126/sciadv.aay4073
Lotfipour, S., Byun, J. S., Leach, P., Fowler, C. D., Murphy, N. P., Kenny, P. J., et al. (2013). Targeted deletion of the mouse α2 nicotinic acetylcholine receptor subunit gene (Chrna2) potentiates nicotine-modulated behaviors. J. Neurosci. 33, 7728–7741. doi: 10.1523/JNEUROSCI.4731-12.2013
Lotfipour, S., Mojica, C., Nakauchi, S., Lipovsek, M., Silverstein, S., Cushman, J., et al. (2017). α2* Nicotinic acetylcholine receptors influence hippocampus-dependent learning memory in adolescent mice. Learn. Mem. 24, 231–244. doi: 10.1101/lm.045369.117
Lozada, A. F., Wang, X., Gounko, N. V., Massey, K. A., Duan, J., Liu, Z., et al. (2012). Induction of dendritic spines by β2-containing nicotinic receptors. J. Neurosci. 32, 8391–8400. doi: 10.1523/JNEUROSCI.2467-11.2012
Lozovaya, N., Eftekhari, S., Cloarec, R., Gouty-Colomer, L. A., Dufour, A., Riffault, B., et al. (2018). GABAergic inhibition in dual-transmission cholinergic GABAergic striatal interneurons is abolished in Parkinson disease. Nat. Commun. 9:1422. doi: 10.1038/s41467-018-03802-y
Lu, D. H., Liao, H. M., Chen, C. H., Tu, H. J., Liou, H. C., Gau, S. S., et al. (2018). Impairment of social behaviors in Arhgef10 knockout mice. Mol. Autism 9:11. doi: 10.1186/s13229-018-0197-5
Malik, R., Li, Y., Schamiloglu, S., and Sohal, V. S. (2022). Top-down control of hippocampal signal-to-noise by prefrontal long-range inhibition. Cell 185, e171602–e171617. doi: 10.1016/j.cell.2022.04.001
Malvaez, M., Greenfield, V. Y., Matheos, D. P., Angelillis, N. A., Murphy, M. D., Kennedy, P. J., et al. (2018). Habits are negatively regulated by histone deacetylase 3 in the dorsal striatum. Biol. Psychiatry 84, 383–392. doi: 10.1016/j.biopsych.2018.01.025
Marek, R., Jin, J., Goode, T. D., Giustino, T. F., Wang, Q., Acca, G. M., et al. (2018). Hippocampus-driven feed-forward inhibition of the prefrontal cortex mediates relapse of extinguished fear. Nat. Neurosci. 21, 384–392. doi: 10.1038/s41593-018-0073-9
Marrosu, F., Portas, C., Mascia, M. S., Casu, M. A., Fa, M., Giagheddu, M., et al. (1995). Microdialysis measurement of cortical hippocampal acetylcholine release during sleep-wake cycle in freely moving cats. Brain Res. 671, 329–332. doi: 10.1016/0006-8993(94)01399-3
Maviel, T., Durkin, T. P., Menzaghi, F., and Bontempi, B. (2004). Sites of neocortical reorganization critical for remote spatial memory. Science 305, 96–99. doi: 10.1126/science.1098180
Mccann, K. E., Lustberg, D. J., Shaughnessy, E. K., Carstens, K. E., Farris, S., Alexander, G. M., et al. (2021). Novel role for mineralocorticoid receptors in control of a neuronal phenotype. Mol. Psychiatry 26, 350–364. doi: 10.1038/s41380-019-0598-7
Meira, T., Leroy, F., Buss, E. W., Oliva, A., Park, J., Siegelbaum, S. A., et al. (2018). A hippocampal circuit linking dorsal CA2 to ventral CA1 critical for social memory dynamics. Nat. Commun. 9:4163. doi: 10.1038/s41467-018-06501-w
Menon, R., SÜß, T., Oliveira, V. E. D. M., Neumann, I. D., and Bludau, A. (2022). Neurobiology of the lateral septum: regulation of social behavior. Trends Neurosci. 45, 27–40. doi: 10.1016/j.tins.2021.10.010
Miguelez Fernandez, A. M. M., Molla, H. M., Thomases, D. R., and Tseng, K. Y. (2021). Prefrontal α7 nAChR signaling differentially modulates afferent drive trace fear conditioning behavior in adolescent adult rats. J. Neurosci. 41, 1908–1916. doi: 10.1523/JNEUROSCI.1941-20.2020
Mille, T., Quilgars, C., Cazalets, J. R., and Bertrand, S. S. (2021). Acetylcholine spinal locomotor networks: the insider. Physiol. Rep. 9:e14736. doi: 10.14814/phy2.14736
Mineur, Y. S., Ernstsen, C., Islam, A., Lefoli Maibom, K., and Picciotto, M. R. (2020). Hippocampal knockdown of α2 nicotinic or M1 muscarinic acetylcholine receptors in C57BL/6J male mice impairs cued fear conditioning. Genes Brain Behav. 19:e12677. doi: 10.1111/gbb.12677
Mooney-Leber, S. M., Zeid, D., Garcia-Trevizo, P., Seemiller, L. R., Bogue, M. A., Grubb, S. C., et al. (2021). Genetic differences in dorsal hippocampus acetylcholinesterase activity predict contextual fear learning across inbred mouse strains. Front. Psychiatry 12:737897. doi: 10.3389/fpsyt.2021.737897
Moreno, M., Economidou, D., Mar, A. C., Lopez-Granero, C., Caprioli, D., Theobald, D. E., et al. (2013). Divergent effects of D(2)/(3) receptor activation in the nucleus accumbens core shell on impulsivity locomotor activity in high low impulsive rats. Psychopharmacology (Berl) 228, 19–30. doi: 10.1007/s00213-013-3010-3
Morley, B. J., and Mervis, R. F. (2013). Dendritic spine alterations in the hippocampus parietal cortex of α7 nicotinic acetylcholine receptor knockout mice. Neuroscience 233, 54–63. doi: 10.1016/j.neuroscience.2012.12.025
Moy, S. S., Ghashghaei, H. T., Nonneman, R. J., Weimer, J. M., Yokota, Y., Lee, D., et al. (2009). Deficient NRG1-ERBB signaling alters social approach: relevance to genetic mouse models of schizophrenia. J. Neurodev. Disord. 1, 302–312. doi: 10.1007/s11689-009-9017-8
Moy, S. S., Nadler, J. J., Perez, A., Barbaro, R. P., Johns, J. M., Magnuson, T. R., et al. (2004). Sociability preference for social novelty in five inbred strains: an approach to assess autistic-like behavior in mice. Genes Brain Behav. 3, 287–302. doi: 10.1111/j.1601-1848.2004.00076.x
Moy, S. S., Nonneman, R. J., Shafer, G. O., Nikolova, V. D., Riddick, N. V., Agster, K. L., et al. (2013). Disruption of social approach by MK-801, amphetamine fluoxetine in adolescent C57BL/6J mice. Neurotoxicol. Teratol. 36, 36–46. doi: 10.1016/j.ntt.2012.07.007
Munoz-Manchado, A. B., Bengtsson Gonzales, C., Zeisel, A., Munguba, H., Bekkouche, B., Skene, N. G., et al. (2018). Diversity of interneurons in the dorsal striatum revealed by single-cell RNA sequencing patchseq. Cell Rep. 24, e72179–e72190. doi: 10.1016/j.celrep.2018.07.053
Nacer, S. A., Letsinger, A. C., Otto, S., Defilipp, J. S., Nikolova, V. D., Riddick, N. V., et al. (2021). Loss of α7 nicotinic acetylcholine receptors in GABAergic neurons causes sex-dependent decreases in radial glia-like cell quantity impairments in cognitive social behavior. Brain Struct. Funct. 226, 365–379. doi: 10.1007/s00429-020-02179-3
Nakazawa, H., Suzuki, Y., Ishikawa, Y., Bando, Y., Yoshida, S., Shiosaka, S., et al. (2019). Impaired social discrimination behavior despite normal social approach by kallikrein-related peptidase 8 knockout mouse. Neurobiol. Learn. Mem. 162, 47–58. doi: 10.1016/j.nlm.2019.04.014
Napier, T. C. (1992). Evaluations of ventral pallidal dopamine receptor activation in behaving rats. Neuroreport 3, 609–611. doi: 10.1097/00001756-199207000-00016
Neher, E., and Sakaba, T. (2008). Multiple roles of calcium ions in the regulation of neurotransmitter release. Neuron 59, 861–872. doi: 10.1016/j.neuron.2008.08.019
Nelson, A., and Mooney, R. (2016). The basal forebrain motor cortex provide convergent yet distinct movement-related inputs to the auditory cortex. Neuron 90, 635–648. doi: 10.1016/j.neuron.2016.03.031
Obermayer, J., Luchicchi, A., Heistek, T. S., De Kloet, S. F., Terra, H., Bruinsma, B., et al. (2019). Prefrontal cortical ChAT-VIP interneurons provide local excitation by cholinergic synaptic transmission control attention. Nat. Commun. 90:5280. doi: 10.1038/s41467-019-13244-9
Okada, K., Nishizawa, K., Kobayashi, T., Sakata, S., Hashimoto, K., Kobayashi, K., et al. (2021). Different cholinergic cell groups in the basal forebrain regulate social interaction social recognition memory. Sci. Rep. 11:13589. doi: 10.1038/s41598-021-93045-7
Okuyama, T., Kitamura, T., Roy, D. S., Itohara, S., and Tonegawa, S. (2016). Ventral CA1 neurons store social memory. Science 353, 1536–1541. doi: 10.1126/science.aaf7003
Olaya, J. C., Heusner, C. L., Matsumoto, M., Sinclair, D., Kondo, M. A., Karl, T., et al. (2018). Overexpression of neuregulin 1 type III confers hippocampal mRNA alterations schizophrenia-like behaviors in mice. Schizophr. Bull. 44, 865–875. doi: 10.1093/schbul/sbx122
Oliveros-Matus, P., Perez-Urrutia, N., Alvarez-Ricartes, N., Echeverria, F., Barreto, G. E., Elliott, J., et al. (2020). Cotinine enhances fear extinction astrocyte survival by mechanisms involving the nicotinic acetylcholine receptors signaling. Front. Pharmacol. 11:303. doi: 10.3389/fphar.2020.00303
O’tuathaigh, C. M., Babovic, D., O’sullivan, G. J., Clifford, J. J., Tighe, O., Croke, D. T., et al. (2007). Phenotypic characterization of spatial cognition social behavior in mice with “knockout” of the schizophrenia risk gene neuregulin 1. Neuroscience 147, 18–27. doi: 10.1016/j.neuroscience.2007.03.051
Packard, M. G., and Mcgaugh, J. L. (1996). Inactivation of hippocampus or caudate nucleus with lidocaine differentially affects expression of place response learning. Neurobiol. Learn. Mem. 65, 65–72. doi: 10.1006/nlme.1996.0007
Pagani, J. H., Zhao, M., Cui, Z., Avram, S. K., Caruana, D. A., Dudek, S. M., et al. (2015). Role of the vasopressin 1b receptor in rodent aggressive behavior synaptic plasticity in hippocampal area CA2. Mol. Psychiatry 20, 490–499. doi: 10.1038/mp.2014.47
Perry, W., Minassian, A., Paulus, M. P., Young, J. W., Kincaid, M. J., Ferguson, E. J., et al. (2009). A reverse-translational study of dysfunctional exploration in psychiatric disorders: from mice to men. Arch. Gen. Psychiatry 66, 1072–1080. doi: 10.1001/archgenpsychiatry.2009.58
Phillips, M. L., Robinson, H. A., and Pozzo-Miller, L. (2019). Ventral hippocampal projections to the medial prefrontal cortex regulate social memory. eLife 8:e44182. doi: 10.7554/eLife.44182
Picciotto, M. R., Higley, M. J., and Mineur, Y. S. (2012). Acetylcholine as a neuromodulator: cholinergic signaling shapes nervous system function behavior. Neuron 76, 116–129. doi: 10.1016/j.neuron.2012.08.036
Pietropaolo, S., Guilleminot, A., Martin, B., D’amato, F. R., and Crusio, W. E. (2011). Genetic-background modulation of core variable autistic-like symptoms in fMR1 knock-out mice. PLoS One 6:e17073. doi: 10.1371/journal.pone.0017073
Pimpinella, D., Mastrorilli, V., Giorgi, C., Coemans, S., Lecca, S., Lalive, A. L., et al. (2021). Septal cholinergic input to CA2 hippocampal region controls social novelty discrimination via nicotinic receptor-mediated disinhibition. eLife 10:e65580. doi: 10.7554/eLife.65580
Poorthuis, R. B., Bloem, B., Schak, B., Wester, J., De Kock, C. P., Mansvelder, H. D., et al. (2013). Layer-specific modulation of the prefrontal cortex by nicotinic acetylcholine receptors. Cereb. Cortex 23, 148–161. doi: 10.1093/cercor/bhr390
Poppe, L., Rue, L., Timmers, M., Lenaerts, A., Storm, A., Callaerts-Vegh, Z., et al. (2019). EphA4 loss improves social memory performance alters dendritic spine morphology without changes in amyloid pathology in a mouse model of Alzheimer’s disease. Alzheimers Res. Ther. 11:102. doi: 10.1186/s13195-019-0554-4
Raam, T., Mcavoy, K. M., Besnard, A., Veenema, A. H., and Sahay, A. (2017). Hippocampal oxytocin receptors are necessary for discrimination of social stimuli. Nat. Commun. 8:2001. doi: 10.1038/s41467-017-02173-0
Redgrave, P., Rodriguez, M., Smith, Y., Rodriguez-Oroz, M. C., Lehericy, S., Bergman, H., et al. (2010). Goal-directed habitual control in the basal ganglia: implications for Parkinson’s disease. Nat. Rev. Neurosci. 11, 760–772. doi: 10.1038/nrn2915
Remmelink, E., Smit, A. B., Verhage, M., and Loos, M. (2016). Measuring discrimination- reversal learning in mouse models within 4 days without prior food deprivation. Learn. Mem. 23, 660–667. doi: 10.1101/lm.042085.116
Rich, E. L., and Shapiro, M. (2009). Rat prefrontal cortical neurons selectively code strategy switches. J. Neurosci. 29, 7208–7219. doi: 10.1523/JNEUROSCI.6068-08.2009
Riedel, G., Kang, S. H., Choi, D. Y., and Platt, B. (2009). Scopolamine-induced deficits in social memory in mice: reversal by donepezil. Behav. Brain Res. 204, 217–225. doi: 10.1016/j.bbr.2009.06.012
Rosenfeld, C. S. (2017). Sex-dependent differences in voluntary physical activity. J. Neurosci. Res 95, 279–290. doi: 10.1002/jnr.23896
Rossi, J., Balthasar, N., Olson, D., Scott, M., Berglund, E., Lee, C. E., et al. (2011). Melanocortin-4 receptors expressed by cholinergic neurons regulate energy balance glucose homeostasis. Cell Metab. 13, 195–204. doi: 10.1016/j.cmet.2011.01.010
Saunders, A., Granger, A. J., and Sabatini, B. L. (2015a). Corelease of acetylcholine GABA from cholinergic forebrain neurons. eLife 4:e06412. doi: 10.7554/eLife.06412
Saunders, A., Oldenburg, I. A., Berezovskii, V. K., Johnson, C. A., Kingery, N. D., Elliott, H. L., et al. (2015b). A direct GABAergic output from the basal ganglia to frontal cortex. Nature 521, 85–89. doi: 10.1038/nature14179
Scheggia, D., Manago, F., Maltese, F., Bruni, S., Nigro, M., Dautan, D., et al. (2020). Somatostatin interneurons in the prefrontal cortex control affective state discrimination in mice. Nat. Neurosci. 23, 47–60. doi: 10.1038/s41593-019-0551-8
Sethuramanujam, S., Mclaughlin, A. J., Derosenroll, G., Hoggarth, A., Schwab, D. J., Awatramani, G. B., et al. (2016). A central role for mixed acetylcholine/GABA transmission in direction coding in the retina. Neuron 90, 1243–1256. doi: 10.1016/j.neuron.2016.04.041
Shin, J. H., Adrover, M. F., and Alvarez, V. A. (2017). Distinctive modulation of dopamine release in the nucleus accumbens shell mediated by dopamine acetylcholine receptors. J. Neurosci. 37, 11166–11180. doi: 10.1523/JNEUROSCI.0596-17.2017
Sidhu, H., Dansie, L. E., Hickmott, P. W., Ethell, D. W., and Ethell, I. M. (2014). Genetic removal of matrix metalloproteinase 9 rescues the symptoms of fragile X syndrome in a mouse model. J. Neurosci. 34, 9867–9879. doi: 10.1523/JNEUROSCI.1162-14.2014
Singer, B. F., Scott-Railton, J., and Vezina, P. (2012). Unpredictable saccharin reinforcement enhances locomotor responding to amphetamine. Behav. Brain Res. 226, 340–344. doi: 10.1016/j.bbr.2011.09.003
Sosa, M., Joo, H. R., and Frank, L. M. (2020). Dorsal ventral hippocampal sharp-wave ripples activate distinct nucleus accumbens networks. Neuron 105, 725–741.e8. doi: 10.1016/j.neuron.2019.11.022
Soto, E., Ortega-Ramirez, A., and Vega, R. (2018). Protons as messengers of intercellular communication in the nervous system. Front. Cell. Neurosci. 12:342. doi: 10.3389/fncel.2018.00342
Steinecke, A., Bolton, M. M., and Taniguchi, H. (2022). Neuromodulatory control of inhibitory network arborization in the developing postnatal neocortex. Sci. Adv. 8:eabe7192. doi: 10.1126/sciadv.abe7192
Stevanovic, K. D., Fry, S. A., Defilipp, J. M. S., Wu, N., Bernstein, B. J., Cushman, J. D., et al. (2022). Assessing the importance of sex in a hippocampus-dependent behavioral test battery in C57BL/6NTac mice. Learn. Mem. 29, 203–215. doi: 10.1101/lm.053599.122
Sun, Q., Zhang, J., Li, A., Yao, M., Liu, G., Chen, S., et al. (2022). Acetylcholine deficiency disrupts extratelencephalic projection neurons in the prefrontal cortex in a mouse model of Alzheimer’s disease. Nat. Commun. 13:998. doi: 10.1038/s41467-022-28493-4
Takacs, V. T., Cserep, C., Schlingloff, D., Posfai, B., Szonyi, A., Sos, K. E., et al. (2018). Co-transmission of acetylcholine GABA regulates hippocampal states. Nat. Commun. 9:2848. doi: 10.1038/s41467-018-05136-1
Titus, D. J., Johnstone, T., Johnson, N. H., London, S. H., Chapalamadugu, M., Hogenkamp, D., et al. (2019). Positive allosteric modulation of the α7 nicotinic acetylcholine receptor as a treatment for cognitive deficits after traumatic brain injury. PLoS One 14:e0223180. doi: 10.1371/journal.pone.0223180
Tong, Q., Ye, C. P., Jones, J. E., Elmquist, J. K., and Lowell, B. B. (2008). Synaptic release of GABA by AgRP neurons is required for normal regulation of energy balance. Nat. Neurosci. 11, 998–1000. doi: 10.1038/nn.2167
Tritsch, N. X., Granger, A. J., and Sabatini, B. L. (2016). Mechanisms functions of GABA co-release. Nat. Rev. Neurosci. 17, 139–145. doi: 10.1038/nrn.2015.21
Unal, C. T., Pare, D., and Zaborszky, L. (2015). Impact of basal forebrain cholinergic inputs on basolateral amygdala neurons. J. Neurosci. 35, 853–863. doi: 10.1523/JNEUROSCI.2706-14.2015
Upmanyu, N., Jin, J., Emde, H. V., Ganzella, M., Bosche, L., Malviya, V. N., et al. (2022). Colocalization of different neurotransmitter transporters on synaptic vesicles is sparse except for VGLUT1 ZnT3. Neuron 110, e71483–e71497. doi: 10.1016/j.neuron.2022.02.008
Urban-Ciecko, J., Jouhanneau, J. S., Myal, S. E., Poulet, J. F. A., and Barth, A. L. (2018). Precisely timed nicotinic activation drives SST inhibition in neocortical circuits. Neuron 97, e5611–e5625. doi: 10.1016/j.neuron.2018.01.037
Vasquez, J. H., Leong, K. C., Gagliardi, C. M., Harland, B., Apicella, A. J., Muzzio, I. A., et al. (2019). Pathway specific activation of ventral hippocampal cells projecting to the prelimbic cortex diminishes fear renewal. Neurobiol. Learn. Mem. 161, 63–71. doi: 10.1016/j.nlm.2019.03.003
Vertes, R. P., Hoover, W. B., and Viana Di Prisco, G. (2004). Theta rhythm of the hippocampus: subcortical control functional significance. Behav. Cogn. Neurosci. Rev. 3, 173–200. doi: 10.1177/1534582304273594
Vorhees, C. V., and Williams, M. T. (2014). Assessing spatial learning memory in rodents. ILAR J. 55, 310–332. doi: 10.1093/ilar/ilu013
Warncke, U. O., Toma, W., Meade, J. A., Park, A. J., Thompson, D. C., Caillaud, M., et al. (2021). Impact of dose, sex strain on oxaliplatin-induced peripheral neuropathy in mice. Front. Pain Res. (Lausanne) 2:683168. doi: 10.3389/fpain.2021.683168
Win-Shwe, T. T., Kyi-Tha-Thu, C., Fujitani, Y., Tsukahara, S., and Hirano, S. (2021). Perinatal exposure to diesel exhaust-origin secondary organic aerosol induces autism-like behavior in rats. Int. J. Mol. Sci. 22:538. doi: 10.3390/ijms22020538
Wojcik, S. M., Katsurabayashi, S., Guillemin, I., Friauf, E., Rosenmund, C., Brose, N., et al. (2006). A shared vesicular carrier allows synaptic corelease of GABA glycine. Neuron 50, 575–587. doi: 10.1016/j.neuron.2006.04.016
Yanpallewar, S., Tomassoni-Ardori, F., Palko, M. E., Hong, Z., Kiris, E., Becker, J., et al. (2022). TrkA-cholinergic signaling modulates fear encoding extinction learning in PTSD-like behavior. Transl. Psychiatry 12:111. doi: 10.1038/s41398-022-01869-2
Zhang, K., Forster, R., He, W., Liao, X., Li, J., Yang, C., et al. (2021). Fear learning induces α7-nicotinic acetylcholine receptor-mediated astrocytic responsiveness that is required for memory persistence. Nat. Neurosci. 24, 1686–1698. doi: 10.1038/s41593-021-00949-8
Keywords: acetylcholine, GABA, co-transmission, animal behavior, transgenic mice
Citation: Goral RO, Harper KM, Bernstein BJ, Fry SA, Lamb PW, Moy SS, Cushman JD and Yakel JL (2022) Loss of GABA co-transmission from cholinergic neurons impairs behaviors related to hippocampal, striatal, and medial prefrontal cortex functions. Front. Behav. Neurosci. 16:1067409. doi: 10.3389/fnbeh.2022.1067409
Received: 11 October 2022; Accepted: 04 November 2022;
Published: 24 November 2022
Edited by:
Satoru Otani, Institut National de la Santé et de la Recherche Médicale (INSERM), FranceReviewed by:
Dautan Daniel, Karolinska Institutet (KI), SwedenLisa M. Savage, Binghamton University, United States
Copyright © 2022 Goral, Harper, Bernstein, Fry, Lamb, Moy, Cushman and Yakel. This is an open-access article distributed under the terms of the Creative Commons Attribution License (CC BY). The use, distribution or reproduction in other forums is permitted, provided the original author(s) and the copyright owner(s) are credited and that the original publication in this journal is cited, in accordance with accepted academic practice. No use, distribution or reproduction is permitted which does not comply with these terms.
*Correspondence: Jerrel L. Yakel, eWFrZWxAbmllaHMubmloLmdvdg==