- 1Perelman School of Medicine, University of Pennsylvania, Philadelphia, PA, United States
- 2Department of Psychiatry, University of Texas Southwestern Medical Center, Dallas, TX, United States
- 3Department of Anesthesiology and Critical Care Medicine, Children’s Hospital of Philadelphia, Philadelphia, PA, United States
- 4University of Pennsylvania, Philadelphia, PA, United States
- 5Department of Neurology and Neurological Therapeutics, University of Texas Southwestern Medical Center, Dallas, TX, United States
- 6Department of Neuroscience, Perelman School of Medicine, Mahoney Institute for Neurosciences, University of Pennsylvania, Philadelphia, PA, United States
Astronauts during interplanetary missions will be exposed to galactic cosmic radiation, including charged particles like 56Fe. Most preclinical studies with mature, “astronaut-aged” rodents suggest space radiation diminishes performance in classical hippocampal- and prefrontal cortex-dependent tasks. However, a rodent cognitive touchscreen battery unexpectedly revealed 56Fe radiation improves the performance of C57BL/6J male mice in a hippocampal-dependent task (discrimination learning) without changing performance in a striatal-dependent task (rule-based learning). As there are conflicting results on whether the female rodent brain is preferentially injured by or resistant to charged particle exposure, and as the proportion of female vs. male astronauts is increasing, further study on how charged particles influence the touchscreen cognitive performance of female mice is warranted. We hypothesized that, similar to mature male mice, mature female C57BL/6J mice exposed to fractionated whole-body 56Fe irradiation (3 × 6.7cGy 56Fe over 5 days, 600 MeV/n) would improve performance vs. Sham conditions in touchscreen tasks relevant to hippocampal and prefrontal cortical function [e.g., location discrimination reversal (LDR) and extinction, respectively]. In LDR, 56Fe female mice more accurately discriminated two discrete conditioned stimuli relative to Sham mice, suggesting improved hippocampal function. However, 56Fe and Sham female mice acquired a new simple stimulus-response behavior and extinguished this acquired behavior at similar rates, suggesting similar prefrontal cortical function. Based on prior work on multiple memory systems, we next tested whether improved hippocampal-dependent function (discrimination learning) came at the expense of striatal stimulus-response rule-based habit learning (visuomotor conditional learning). Interestingly, 56Fe female mice took more days to reach criteria in this striatal-dependent rule-based test relative to Sham mice. Together, our data support the idea of competition between memory systems, as an 56Fe-induced decrease in striatal-based learning is associated with enhanced hippocampal-based learning. These data emphasize the power of using a touchscreen-based battery to advance our understanding of the effects of space radiation on mission critical cognitive function in females, and underscore the importance of preclinical space radiation risk studies measuring multiple cognitive processes, thereby preventing NASA’s risk assessments from being based on a single cognitive domain.
Introduction
As space agencies plan for impending interplanetary missions—such as to Mars—understanding potential hazards associated with galactic cosmic radiation (GCR) exposure becomes a priority (Vazquez, 1998; Schimmerling et al., 2003; Setlow, 2003; National Research Council et al., 2008; Chancellor et al., 2014; Cucinotta, 2015; Kokhan et al., 2016; Nelson et al., 2016; National Academies of Sciences, Engineering, and Medicine, 2018). GCR is composed of fast-moving low and high- (H) atomic number (Z) and high-energy (E) particles, such as 56Fe, and cannot be effectively blocked by modern spacecraft shielding (Cucinotta et al., 2006; Spillantini et al., 2007; Durante, 2014; Nelson, 2016; Zeitlin and La Tessa, 2016; Patel et al., 2020; Willey et al., 2021). Rodent data suggest HZE particle exposure is detrimental to brain physiology and functional cognitive output, with noted negative impact on hippocampal function and also on operant behavior (Rabin et al., 2004, 2007b; Blakely and Chang, 2007; Rabin, 2012; Cucinotta et al., 2014; Kokhan et al., 2016; Nelson, 2016; Cekanaviciute et al., 2018; Jandial et al., 2018; Cucinotta and Cacao, 2019; Kiffer et al., 2019; Limoli, 2020; Britten et al., 2021b; Davis et al., 2021). Therefore, HZE particle exposure appears to pose an unavoidable threat to astronaut well-being and mission success. Specifically, a central theme emerging from rodent space radiation literature (which overwhelmingly has used 56Fe particles) is that exposure to HZE particles may be harmful to astronaut cognition and brain health.
A thorough review of the literature, however, does not support a uniformly negative impact of HZE particles on rodent brain and behavior (Kiffer et al., 2019; Britten et al., 2021b). Two even more recent studies highlight that 56Fe particle exposure can have seemingly beneficial effects on the mouse hippocampus, a brain region critical for memory and mood regulation. One study showed exposure to whole-body 56Fe particle irradiation (IRR) improves hippocampal-dependent spatial learning 12 and 20 months (mon) post-IRR in male and female mice (Miry et al., 2021). Another study exposed 6-mon-old (“astronaut-age”) male mice to whole-body 56Fe particles (Whoolery et al., 2020) and used a rodent touchscreen platform to probe the functional integrity of brain circuits (Oomen et al., 2013; Hvoslef-Eide et al., 2016; Kangas and Bergman, 2017), drawing similarity to the way astronauts undergo touchscreen testing (Basner et al., 2017; Moore et al., 2017). This study found mice exposed to 56Fe particles had better discrimination learning (location discrimination, LD) vs. Sham mice, suggesting astronauts may show an improvement in this mission-critical skill. However, 56Fe mice were not different from Sham mice in many other tasks (pairwise discrimination, PD; visuospatial/associative-learning, Paired Associates Learning, PAL; stimulus-response habit or “rule-based” learning, visuomotor conditional learning, VMCL; cognitive flexibility, PD reversal) (Whoolery et al., 2020). Taken together, these studies suggest caution in concluding that HZE particle exposure decreases rodent cognition; the reality is likely that there are time-, task-, species- dose-, and energy-, etc., dependent effects (Miry et al., 2021). In addition, these studies point out the importance—recently underscored for the space radiation field (Britten et al., 2021b)—of measuring multiple cognitive processes in rodents, thereby preventing NASA’s risk assessment from being based on a single cognitive domain.
Another important factor to consider in assessing the impact of HZE particle exposure on cognition is biological sex. As of 2019, <10% of preclinical studies assessing the cognitive effects of HZE particle exposure used female rodents (Kiffer et al., 2019). Human research is only slightly better; the available data from astronauts is heavily skewed in favor of males (n = 477) vs. females (n = 57). Thus there is an urgent need for studies to determine the role of biological sex in the body’s response to space flight stressors (Mark et al., 2014), including exposure to GCR and HZE particles. While some work suggests female rodents are more susceptible than males to space radiation exposure, other preclinical work suggests the female rodent brain may be protected from radiation-induced immune and cognitive deficits (Villasana et al., 2006, 2010; Cherry et al., 2012; Krukowski et al., 2018; Hinkle et al., 2019; Liu et al., 2019; Parihar et al., 2020). Considering the astronaut class is 40% women (Mark et al., 2014) and the translational relevance of the rodent touchscreen platform (Oomen et al., 2013; Hvoslef-Eide et al., 2016; Kangas and Bergman, 2017), it is striking that the touchscreen platform has not yet been used to assess how female rodent cognition is influenced by whole-body exposure to an HZE particle, such as 56Fe.
To address this knowledge gap, mature “astronaut aged” C57BL/6J female mice received either Sham or whole-body 56Fe particle IRR (3 × 6.7cGy 56Fe, 600 MeV/n) and were assessed on a battery of operant touchscreen and classical behavior tasks to probe aspects of cognition. These radiation exposure parameters are identical to those that reportedly improve location discrimination in mature male mice (Whoolery et al., 2020). This dose was chosen as it is submaximal to that predicted for a Mars mission (Cucinotta and Durante, 2006; Hellweg et al., 2007). The fractionation regimen was selected based on its relevance for a Mars mission and its known impact on rodent brain structure and function (Rivera et al., 2013; Whoolery et al., 2017, 2020). For the present study, female Sham and IRR mice were tested for touchscreen performance of instrumental learning, discrimination learning, extinction learning, and stimulus-response habit (rule-based) learning. Given literature suggesting the female rodent brain may be spared from the negative impact of HZE particle exposure (Rabin et al., 2013; Krukowski et al., 2018), we hypothesized that whole-body 56Fe IRR would spare or even improve their performance in touchscreen-based behaviors, especially hippocampal-reliant discrimination learning. This touchscreen battery revealed an unexpected finding: improved discrimination learning, but worse stimulus-response habit learning in 56Fe-irradiated vs. Sham mice. We also tested several classical behaviors to examine these anxiety, stress response and repetitive behaviors, but both Sham and IRR mice performed similarly in those tests. Taken together with pre-planned, in-depth analysis of key aspects of their touchscreen performance on this and many other tasks, these data suggest whole-body exposure to 56Fe particle IRR in mature female mice may support or enhance hippocampal tasks like discrimination learning, but may diminish striatum-dependent tasks like stimulus-response habit learning.
Materials and Methods
The ARRIVE 2.0 guidelines were used to design and report this study (Percie du Sert et al., 2020). A protocol was prepared for this study prior to experimentation, but this protocol was not registered.
Animals
Two-month-old female C57BL/6J mice were purchased from Jackson Laboratories (stock #000664) and housed at Children’s Hospital of Philadelphia (CHOP, Figure 1A) or UT Southwestern Medical Center (UTSW, Figure 1B) and shipped to Brookhaven National Laboratories (BNL) for IRR at 6-mon of age. Experiments were performed at these two different institutions due to the Eisch Lab moving institutions. Data collected at these two institutions are both presented here given the well-documented reliability of the operant touchscreen platform (Beraldo et al., 2019; Dumont et al., 2020; Sullivan et al., 2021). Housing conditions at all facilities are 3–4/cage, light on 06:00, lights off 18:00, UTSW/CHOP: room temperature 68–79°F, room humidity 30–70%, BNL: room temperature 70–74°F and room humidity 30–70%. During shipping and housing at BNL, mice were provided Shepherd Shacks (Bio-Serv); no other enrichment was provided during housing. After IRR, mice were transferred to either UTSW (Sham n = 16, IRR n = 16) or CHOP (Sham n = 11, IRR n = 9). At both facilities, food (CHOP and BNL: LabDiet #5015; UTSW: Envigo Teklad global 16% protein) and water were provided ad libitum except during the appetitive behavior tasks. When placed in CHOP quarantine after return from IRR (below), all mice received modified chow (Test Diet, Cat#1813527, Modified LabDiet 5058 with 13 ppm Ivermectin and 150 ppm Fenbendazole) as required by CHOP’s Department of Veterinary Research. Animal procedures and husbandry were in accordance with the National Institutes of Health Guide for the Care and Use of Laboratory Animals, and performed in IACUC-approved facilities at UTSW [Dallas TX; AAALAC Accreditation #000673, PHS Animal Welfare Assurance D16-00296, Office of Laboratory Animal Welfare (OLAW) A3472-01], CHOP [Philadelphia, PA; AAALAC Accreditation #000427, PHS Animal Welfare Assurance D16-00280 (OLAW A3442-01)] and BNL [Upton NY; AAALAC Accreditation #000048, PHS Animal Welfare Assurance D16-00067 (OLAW A3106-01)]. Of the 52 total mice used for this study, 3 mice (n = 2 Sham, n = 1 IRR) had to be euthanized for reaching a humane endpoint (lethargy, hunched posture, and coat unkempt). None of the other mice used in this study warranted us employing our established interventions for reducing pain, suffering, or distress.
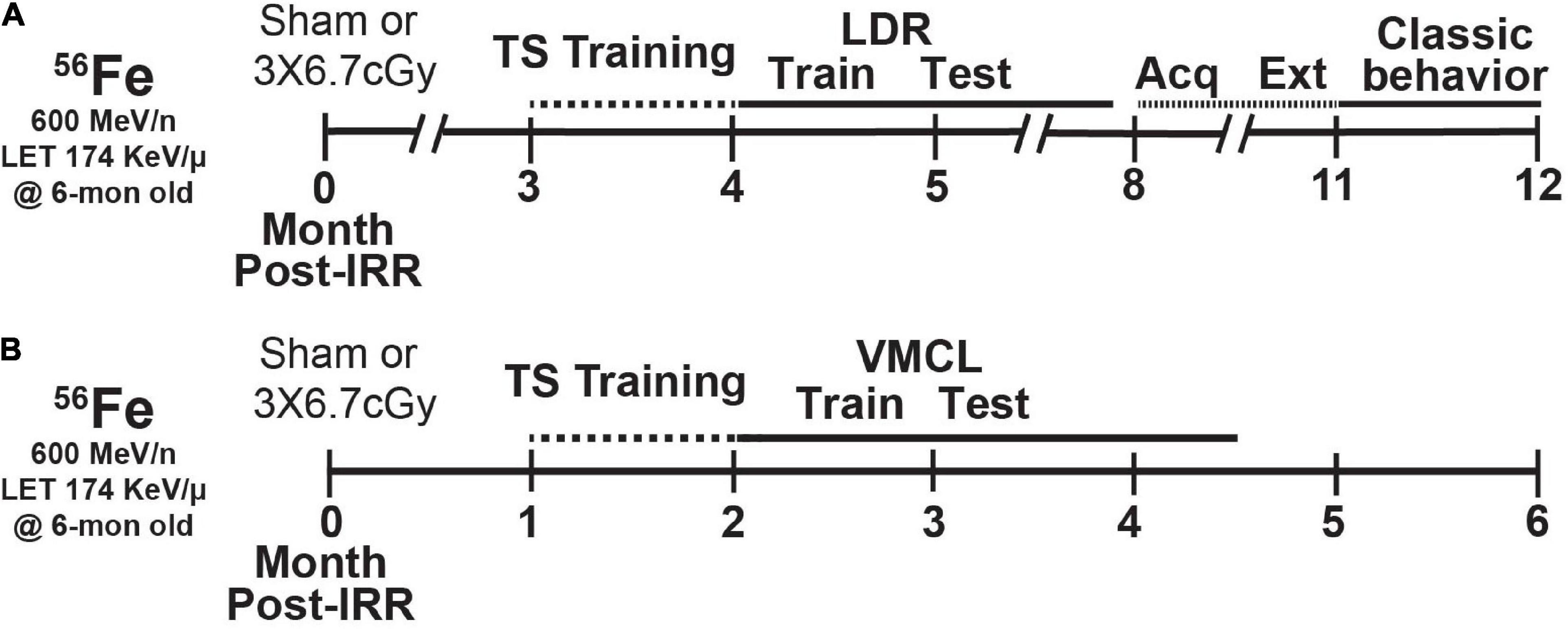
Figure 1. Timeline of experimental groups and overview of behavior tests. Six-month-old C57BL/6J female mice received whole-body exposure to 56Fe [0-Month (Mon) Post-Irradiation (IRR)] and subsequently were run on a variety of touchscreen and non-touchscreen behavioral tests, including (A) touchscreen training with a twelve-window (2 × 6) grid followed by LDR Train and Test, Acquisition and Extinction of stimulus-response habit learning, and classic behavior tests (EPM, MB, OF, SI, and FST) or (B) touchscreen training on a 3-window (1 × 3) grid followed by VMCL Train and Test. Acq, acquisition; Ext, extinction; LDR, location discrimination reversal; Test, testing; Train, training; TS, touchscreen; VMCL, visuomotor conditioning learning.
Particle Irradiation
Mice received whole-body HZE particle IRR at BNL’s NASA Space Radiation Laboratory (NSRL) during NSRL campaigns 17B and 18A. The 56Fe ion beams were produced by the AGS Booster Accelerator at BNL and transferred to the experimental beam line in the NSRL. Dosimetry and beam uniformity was provided by NSRL staff. Delivered doses were ±0.5% of the requested value. All mice—regardless of whether control (Sham) or experimental (56Fe)—were singly-placed for 15 min in modified clear polystyrene rectangular containers (AMAC Plastics, Cat #100C, W 5.8 × L 5.8 × H 10.7 cm; modified with ten 5-mm air holes). Although confined to a container, mice had room to move freely and turn around during confinement. A maximum of six containers were placed perpendicular to the beam for each cave entry. Mice received either Sham exposure (placed in cubes Monday, Wednesday, Friday, but received no 56Fe exposure) or Fractionated (Frac) 20 cGy 56Fe IRR (600 MeV/n, LET 174 KeV/μ, dose rate 20 cGy/min; placed in cubes and received 6.7 cGy on Monday, Wednesday, and Friday). Post-IRR, mice were returned to UTSW (Figure 1B) or CHOP (Figure 1A) and housed in quarantine for 1–1.5 mon prior to initiation of touchscreen behavior testing (Figure 1). Body weights (Figure 2A) were taken multiple times: prior to IRR, at IRR, and at least weekly post-IRR until collection of brain tissue. This dose of 56Fe was selected as it is submaximal to that predicted for a Mars mission (Cucinotta and Durante, 2006; Hellweg et al., 2007) and the fractionation interval (48 h) was determined by the inter-fraction period for potential repair processes (Thames, 1985).
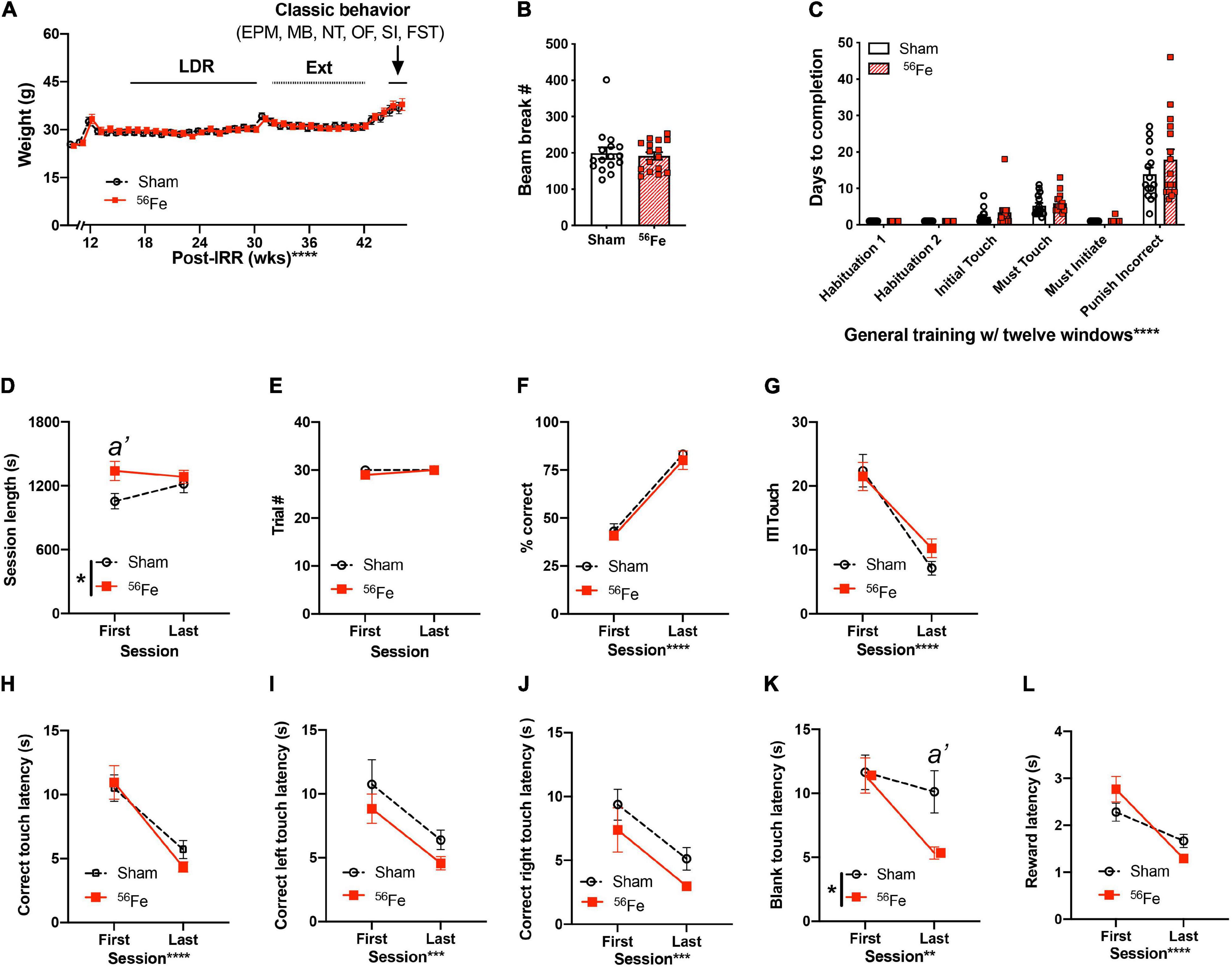
Figure 2. Weights, locomotion, and general touchscreen training learning are generally unaffected in 6-mon old female mice exposed to whole-body 56Fe IRR compared to Sham. (A) No gross weekly weight difference was detected between Sham or 56Fe mice during touchscreen testing. (B) Beam breaks measured in the novel TS operant chambers in Habituation 1 revealed no gross baseline difference in locomotion after exposure to Sham or 56Fe IRR. (C) Sham and 56Fe IRR groups performed similarly in each of the first six steps of general touchscreen training with twelve windows: Habituation 1 and 2, Initial Touch, Must Touch, Must Initiate, and Punish Incorrect. (D–L) During the Punish Incorrect stage of general TS training, 56Fe IRR female mice had a longer first, but not last, training session vs. Sham mice (D). However, Sham and 56Fe IRR mice did not differ in the number of completed trials (E),% correct (F), total ITI touches (G), correct touch latency (H), and correct left or right touch latency (I,J). (K) 56Fe IRR female mice were ∼5 s faster vs. Sham mice to touch a blank window in the final session of testing. Aside from these differences, IRR and Sham mice had similar reward collection latency (L). Error bars depict mean ± SEM. Mixed-effects analysis was used in panel (A) main effects: Time F36,1067 = 66.05, p < 0.0001 and Treatment F1,30 = 0.02450, p = 0.8767; interaction: Treatment × Time F36,1067 = 1.586, p = 0.0161, post hoc: all p > 0.05. Unpaired t-test was used in panel (B); p = 0.6979. Two-way RM ANOVA was used in panels (C–L): main effect *p < 0.05; **p < 0.01; ***p < 0.001; ****p < 0.0001, Bonferroni’s post hoc analysis a’ p < 0.05. In panel (D), main effects: Session F1,29 = 0.5688, p = 0.4568 and Treatment F1,29 = 4.532, p = 0.0419 (post hoc: a’ p = 0.0228, in Sham vs. 56Fe, ωp2 = 0.07) and interaction: Session × Treatment F1,29 = 2.345, p = 0.1365. In panel (K), main effects: Session F1,29 = 9.015, p < 0.0055, ωp2 = 0.12 and Treatment F1,29 = 3.842, p = 0.05 (post hoc a’ p = 0.0203 in Sham vs. 56Fe) and interaction: Session × Treatment F1,29 = 3.234, p = 0.0825. EPM, elevated plus maze; FST, forced swim test; IRR, irradiation; MB, marble burying; OF, open field; SI, social interaction; TS, touchscreen; s, seconds; wks, weeks. Complete and detailed statistical information provided in Supplementary Table 1.
Overview of Behavioral Testing
Mice exposed to HZE particles in NSRL campaigns 18A and 17B were divided into parallel groups (Figures 1A,B, respectively) that underwent touchscreen behavioral testing 1–3 mon post-IRR. Touchscreen experiments were performed between 08:00 and 14:00 during weekdays. As is standard in most rodent touchscreen experiments, mice were food restricted during touchscreen experiments. Mouse chow was removed from each cage at 17:00 the day prior to training or testing. Each cage was given ad libitum access to chow for 3 h (minimum) to 4 h (maximum) immediately following daily touchscreen training/testing, and from completion of training/testing on Friday until Sunday 5 p.m. Mice were weighed each Wednesday to ensure weights > 80% initial body weight. While weights below this threshold merited removal of the mouse from the study, zero mice reached this threshold (Mar et al., 2013; Oomen et al., 2013). Luminescence emits from the touchscreen chamber screen and reward magazine, and from the house light during one stage of general touchscreen training, and thus the mice were not performing in darkness. In one group of mice (Figure 1A), mice began touchscreen behavioral testing 3-mon post-IRR. Operant touchscreen platform procedures included general touchscreen training (with 2 × 6 window grid), Location Discrimination Reversal (LDR, Train and Test), and Extinction (Ext, training/“Acquisition” and testing). Total beam breaks as a measure of baseline locomotor activity were gathered in touchscreen operant chambers during general touchscreen training (Habituation 1). After all animals completed LDR Test, mice received unrestricted food pellets for 2 weeks before beginning Ext to allow them to recover from potential stress associated with food restriction. Following Ext testing, mice were tested in a variety of non-touchscreen tests classified here as “classical behavior tests” (Figures 1A, 2A). These tests measure anxiety- [elevated plus maze (EPM), open field (OF)] or repetitive/compulsive-like behaviors [marble burying (MB)], locomotion (OF), sociability [social interaction (SI)], and despair-like behaviors [forced swim test (FST)], methods which are provided below. Classical behavior tests were performed between 14:00 and 17:00 during weekdays under red light (45–65 lux) except for the forced swim test which was done under white and red light simultaneously. Mice were habituated to the testing suite under red light for 1 h prior to testing. In the second group of mice (Figure 1B), mice began touchscreen behavioral testing 1-mon post-IRR. Operant touchscreen platform procedures performed on this group were general touchscreen training (with 1 × 3 window grid) and Visuomotor Conditional Learning (VMCL) Train and Test. Subject number for each group in each figure panel is provided in Supplementary Table 1.
General Touchscreen Training
General Touchscreen Training (prior to LDR) consists of six stages, as previously published (Whoolery et al., 2020): Habituation 1, Habituation 2, Initial Touch, Must Touch, Must Initiate, and Punish Incorrect (PI). Methods for each stage are described in turn below. Mice went through general touchscreen training with twelve windows (2 × 6) for the LDR experiment.
Habituation
Mice are individually placed in a touchscreen chamber for 30-min (max) with the magazine light turned on (LED Light, 75.2 lux). For the initial reward in each habituation session, a tone is played [70 decibel (dB) at 500 Hz, 1,000 ms] at the same time as a priming reward (150-μl Ensure Original Strawberry Nutrition Shake) is dispensed to the reward magazine. After a mouse inserts and removes her head from the magazine, the magazine light turns off and a 10-s delay begins. At the end of the delay, the magazine light is turned on and the tone is played again as a standard amount of the reward (7-μl Ensure) is dispensed. If the mouse’s head remains in the magazine at the end of the 10-s delay, an additional 1-s delay is added. A mouse completes Habituation training after they collect 25 rewards (25 × 7 μl) within 30 min. Mice that achieve habituation criteria in <30 min are removed from the chamber immediately after their 25th reward in order to minimize extinction learning. The measure reported for Habituation is days to completion.
Initial touch
A 2 × 6 window grid is placed in front of the touchscreen for the remaining stages of training. At the start of the session, an image (a lit white square) appears in a pseudo-random location in one of the 12 windows on the touchscreen. The mouse has 30 s to touch the lit square (typically with their nose). If the mouse does not touch the image, it is removed, a reward (7 μl Ensure) is delivered into the illuminated magazine on the opposite wall from the touchscreen, and a tone is played. After the reward is collected, the magazine light turns off and a 20-s intertrial interval (ITI) begins. If the mouse touches the image while it is displayed, the image is removed and the mouse receives three times the normal reward (21-μl Ensure, magazine is illuminated, tone is played). For subsequent trials, the image appears in another of the 12 windows on the touchscreen, and never in the same location more than three consecutive times. Mice reach criteria and advance past Initial Touch training when they complete 25 trials (irrespective of reward level received) within 30 min. Mice that achieve Initial Touch criteria in <30 min are removed from the chamber immediately after their 25th trial. The measure reported for Initial Touch is days to completion.
Must touch
Similar to Initial Touch training, an image appears, but now the window remains lit until it is touched. If the mouse touches the lit square, the mouse receives a reward (7-μl Ensure, magazine is illuminated, tone is played). If the mouse touches one of the blank windows, there is no response (no reward is dispensed, the magazine is not illuminated, and no tone is played). Mice reach criteria and advance past Must Touch training after they complete 25 trials within 30 min. Mice that achieve Must Touch criteria in <30 min are removed from the chamber immediately after their 25th trial. The measure reported for Must Touch is days to completion.
Must initiate
Must Initiate training is similar to Must Touch training, but a mouse is now required to initiate the training by placing its head into the already-illuminated magazine. A random placement of the image (lit white square) will then appear on the screen, and the mouse must touch the image to receive a reward (7-μl Ensure, magazine lit, tone played). Following the collection of the reward, the mouse must remove its head from the magazine and then reinsert its head to initiate the next trial. Mice advance from Must Initiate training after they complete 25 trials within 30 min. Mice that achieve Must Initiate criteria in <30 min are removed from the chamber immediately after their 25th trial. The measure reported for Must Initiate is days to completion.
Punish incorrect
PI training builds on Must Initiate training, but here if a mouse touches a portion of the screen that is blank (does not have a lit white square), the overhead house light turns on and the lit white square disappears from the screen. After a 5-s timeout period, the house light turns off and the mouse has to initiate a correction trial where the lit white square appears in the same location on the screen. The correction trials are repeated until the mouse successfully presses the lit white square; however, correction trials are not counted toward the final percent correct criteria. Mice reach criteria and advance past PI training and onto Location Discrimination Reversal Train/Test after they complete 30 trials within 30 min at ≥76% (≥19 correct) on day 1 and >80% (>24 correct) on day 2 over two consecutive days. Mice that achieve PI criteria in <30 min are removed from the chamber immediately after their 30th trial. As with the other stages, a measure reported for PI is days to completion (to reach criteria). However, since the PI stage also contains a metric of accuracy, more measures were analyzed relative to the other five stages. Therefore, other measures reported for PI are session length, trial number, percent correct responses, ITI, latency to make a correct touch (for total touches, left touches, and right touches) and an incorrect touch (touching a blank window), and latency to collect a reward.
Location Discrimination Reversal
Location Discrimination Reversal (LDR; program LD1 choice reversal v3; ABET II software, Cat #89546-6) tests the ability to discriminate two conditioned stimuli that are separated either by a large or small separation. The reversal component of LDR is used here and in classic LDR studies (Clelland et al., 2009; Oomen et al., 2013) tests cognitive flexibility; prior work showing space radiation improved LD function in male mice used LD, not LDR (Whoolery et al., 2020). Taken together, LDR is a hippocampal-dependent task (Clelland et al., 2009; Oomen et al., 2013) which allows assessment of both discrimination ability as well as cognitive flexibility. In our timeline (Figure 1A), mice received one additional training step (“LDR Train”) prior to the actual 2-choice LDR Test.
Location discrimination reversal train
In LDR train, mice initiated the trial, which led to the display of two identical white squares (25 × 25 pixels, Figure 3A) presented with two blank (unlit) squares between them, a separation which was termed “intermediate” (8th and 11th windows in 2 × 6 high grid-bottom row). One of the left (L) or right (R) locations of the squares was rewarded (i.e., L+) and the other is not (R-), and the initial rewarded location (left or right) was counterbalanced within-group. On subsequent days, the rewarded square location was switched based on the previous day’s performance (L+ becomes L- and R- becomes R+, then L- becomes L+ and R+ becomes R-, etc.). A daily LDR Train session is complete once the mouse touches either L+ or R- 50 times or when 30 min has passed. Once 7 out of 8 trials had been correctly responded to, on a rolling basis, the rewarded square location was switched (becomes L-), then L+, then L-, etc.; this is termed a “reversal.” Once the mouse reached >1 reversal in 3 out of 4 consecutive testing sessions, the mouse advanced to the LDR Test. A daily training is considered a “session.” Measures reported for LDR Train are: percent of each group reaching criteria over time (survival curve), days to completion, trial number, and percent correct during trials to the 1st reversal.
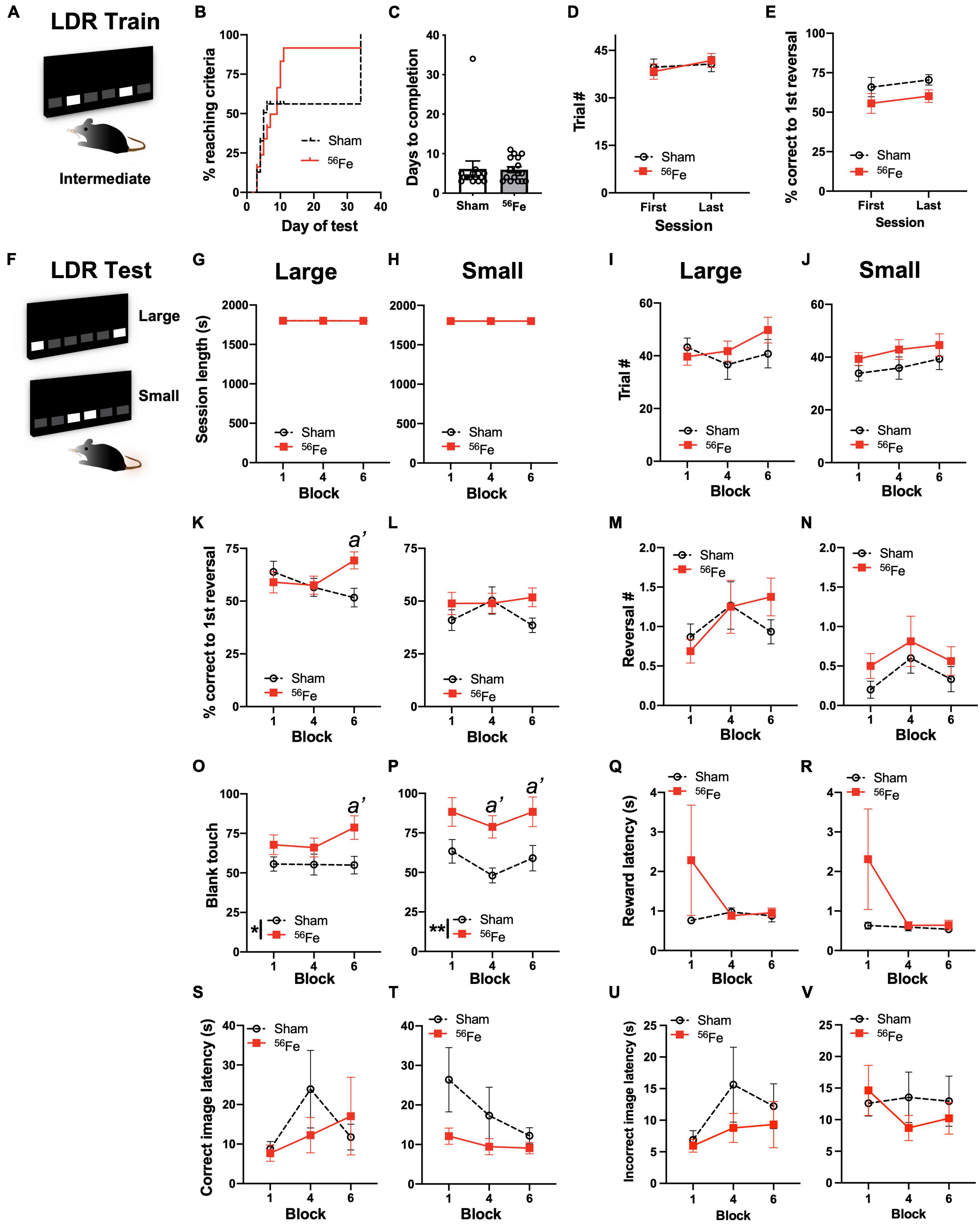
Figure 3. On an appetitive, touchscreen discrimination learning task, female mice exposed to whole-body 56Fe IRR at 6-mon of age perform better than Sham mice in discriminating the location of two identical visual cues. (A) Schematic of the lit squares and their Intermediate separation which are used for location discrimination reversal training (LDR Train). (B–E) Sham and 56Fe IRR mice performed similarly in LDR Train based on (B) distribution of subjects reaching criteria (the visual difference in percent of Sham and 56Fe subjects reaching criteria was rejected by survival curve analysis), (C) days to completion, (D) trials completed, and (E) % correct to 1st reversal. (F) Schematic of the lit squares and their Large and Small separation used for LDR testing (LDR Test). (G–V) When mice underwent LDR Test with squares maximally-separated (Large separation, G,I,K,M,O,Q,S,U) or minimally-separated (Small separation, H,J,L,N,P,R,T,V), there was no difference between IRR and Sham mice in session length (G,H) or number of completed trials (I,J) on the last day of the 1st, 4th, and 6th 2-day block. However, in the Large - but not Small -separation trials, 56Fe IRR female mice had greater accuracy vs. Sham mice in trials to the first reversal (K,L). Sham and IRR groups did not differ in the number of reversals completed (M,N). Both Large and Small separation trials revealed that 56Fe IRR female mice made more blank touches (to non-stimuli windows) vs. Sham mice (O,P), specifically during the 6th (last) block of Large separation trials and during the 4th and 6th blocks of Small separation trials. IRR and Sham mice had similar reward collection latency (Q,R), correct image response latency (S,T), and incorrect image response latency (U,V) in the Large and Small separation trials. Error bars depict mean ± SEM. Statistical analysis was performed in panel (B): Mantel-Cox test, in panel (C): unpaired t-test and in panels (D–V): Two-way RM ANOVA: main effect *p < 0.05, **p < 0.01. In panel (K), main effects: main effects: Block F2,58 = 0.5836, p = 0.5611 and Treatment F1,29 = 1.230, p = 0.2765; interaction: Block × Treatment F2,58 = 3.761, p = 0.0291, post hoc: a’ p = 0.0220 in Sham vs. 56Fe, ωp2 = 0.05; Small; in panel (O), main effect: Block F2,58 = 0.7784, p = 0.4639 and Treatment F1,29 = 6.262, p = 0.0182, post hoc: a’ p = 0.0232, ωp2 = 0.09; interaction of Block × Treatment F2,58 = 0.9088, p = 0.4087; in panel (P), main effects: Block F2,58 = 2.185, p = 0.1216 and Treatment F1,29 = 11.49, p = 0.0020, post hoc: a’ p = 0.0204 at Block 4, a’ p = 0.0292 at Block 6, ωp2 = 0.17; interaction: Block × Treatment F2,58 = 0.1156, p = 0.8910. LDR, location discrimination reversal; s, seconds. Complete and detailed statistical information provided in Supplementary Table 1.
Location discrimination reversal test
In LDR test, mice initiated the trial, which led to the display of two identical white squares, either with four black squares between them [“large” separation, two at maximum separation (7th and 12th windows in the bottom row of a 2 × 6 grid)] or directly next to each other [“small” separation, two at minimum separation (9th and 10th windows in the bottom row of a 2 × 6 grid; Figure 3F)]. As in LDR Train, only one of the square locations (right-most or left-most) was rewarded (L+, same side for both large and small separation, and counterbalanced within-groups). The rewarded square location was reversed based on the previous day’s performance (L+ becomes L-, then L+, then L-, etc.). Once 7 out of 8 trials had been correctly responded to, on a rolling basis, the rewarded square location was reversed (becomes L-, then L+, then L-, etc.). Each mouse was exposed to only one separation type during a daily LDR Test session (either large or small) and the separation type changed every 2 days (2 days of large, then 2 days of small, 2 days of large, etc.). A daily LDR Test session was completed once the mouse touched either L+ or R- 81 times or when 30 min had passed. LDR Test data are analyzed by block (1 block = 4 days LDR Test counterbalanced with two Large and two Small separation daily sessions). Once 24 testing sessions (12 days of Large, 12 days of Small separation) were completed, mice received 2 weeks of normal feeding prior to extinction testing. Measures reported for LDR Test are all presented for both Large and Small separation: session length, trial number, percent correct during trials to the 1st reversal, number of reversal, number of blank touches (touching an un-lit square), reward collection latency, latency to touch the correct image on the last day of the 1st, 4th, and 6th 2-day block were reported, and latency to touch the incorrect image (touching the incorrect lit square; does not include blank window touches) on the last day of the 1st, 4th, and 6th 2-day block (to allow assessment in the last day in Large or Small separation testing blocks).
Extinction Learning (Ext; ABET II Software, Cat #89547)
Acquisition of simple stimulus-response learning (schedule name: extinction pt 1)
Acquisition of simple stimulus-response learning (schedule name: Extinction pt 1) is the first part of the extinction test, and is a task that involves the amygdala (Fernando et al., 2013). The start of acquisition was marked by the magazine light turning on and the delivery of a free reward. Mice initiate the trial, which leads to the display of an image (lit white square stimulus) in the center window (middle square in 1 × 3 grid; Figure 4A; Mar et al., 2013). The mouse must touch the stimulus displayed in the center window to elicit tone/food response. The two side windows were left blank throughout the experiment. No response ensued if the mouse touches a blank part of the screen. A daily acquisition session was complete once the mouse touched the center window 30 times or when 30 min had passed. Once the mouse completed 30 trials within 15 min on each of five consecutive sessions (criteria for acquisition), the mouse advanced to the extinction test. Measures reported for Acquisition are: percent of each group reaching criteria over time (survival curve), days to completion, session length, and number of correct responses.
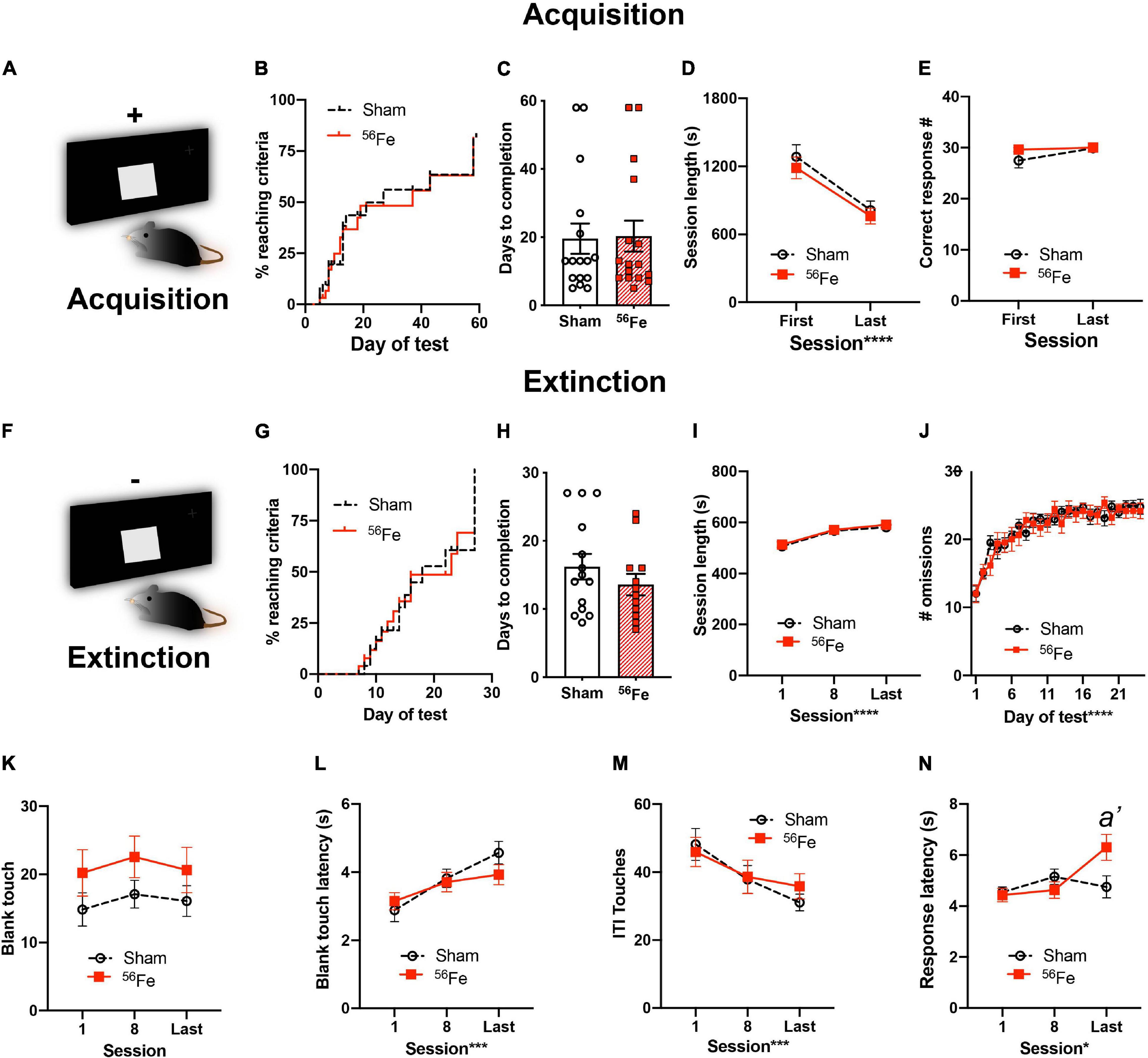
Figure 4. Six-month-old female mice exposed to whole-body 56Fe IRR and Sham mice perform similarly in acquisition and extinction of simple stimulus-response operant tasks. (A) Schematic of the single, large lit square image used for Acquisition of stimulus-response operant learning. Plus (+) sign indicates that the mouse is rewarded for touching the single, large, lit square. (B–E) Sham and 56Fe IRR mice performed similarly during stimulus-response Acquisition, based on (B) distribution of subjects reaching criteria, (C) days to completion, (D) session length, and (E) number of correct responses. (F) Schematic of the single, large lit square image used for extinction of the operant stimulus-response learning. Minus (-) sign indicates that the mouse receives no reward during extinction. (H)56Fe IRR and Sham mice took similar time to extinguish the previously-acquired stimulus-response contingent behavior, and the performance of Sham vs. IRR mice was similar based on session length (I), number of omissions (J), number of blank touches (K), blank touch latency (L), and ITI touches (M). 56Fe IRR mice showed a higher response touch latency than Sham mice in the last session of extinction testing (N). Error bars depict mean ± SEM. Statistical analysis was performed in panels (B,G): Mantel-Cox test, in panels (C,H): Unpaired, two-tailed t-tests, and in panel (J): mixed effects analysis, and in panels (D,E,I,K–N): Two-way RM ANOVA, main effect *p < 0.05, ***p < 0.001, ****p < 0.0001. Bonferroni’s post hoc analysis a’ p < 0.05. In panel (N), main effects: Session F2,50 = 3.835, p = 0.0282, ωp2 = 0.08 and Treatment F1,25 = 1.512, p = 0.2302; interaction of Session × Treatment F2,50 = 4.173, p = 0.0211, post hoc: a’ p = 0.0088 in Sham vs. 56Fe, ωp2 = 0.08. s, seconds. Complete and detailed statistical information provided in Supplementary Table 1.
Extinction test (schedule name: extinction pt 2)
Extinction test (schedule name: Extinction pt 2) is a test that involves the prefrontal cortex (PFC) (Mar et al., 2013). A 5-s ITI marked the start of extinction. Following the initial ITI, an image (the lit white square stimulus) was presented in the center window (middle window in 1 × 3 grid; Figure 4F). The stimulus display was held on the screen for 10 s during which the mouse could elicit or omit its learned response to the square. The two side windows were left blank throughout the experiment. If the mouse touched the blank window of the screen, no response occurred. If the white square was touched, no food delivery was made but the image was removed, the magazine light was illuminated, a tone was played and the ITI period (10 s) was started; this is the “correct” action, even though no reward is provided. If the white square was not touched, then the image was removed and the ITI period started. Mouse entry into the reward magazine during the ITI would turn off the magazine light. Following an ITI, the magazine light was turned off and the next trial began automatically. A daily extinction session was complete once the mouse was presented with the white square stimulus 30 times. When the mouse reached ≥80% response omissions on each of at least three out of four consecutive sessions, the mouse was considered to have reached daily criteria. Mice that reached criteria first continued to be tested daily until all of the mice’s performance was synchronized and completed before advancing to classical behavior battery testing. Measures reported for Extinction are: percent of each group reaching criteria over time (survival curve), days to completion, and number of omissions across testing days as well as session length, number of touches and latency to touch to a blank part of the touchscreen, number of touches during the ITI, and latency to make a correct response on the last day of the first, 8th, and last testing session.
Visuomotor Conditional Learning
Visuomotor Conditional Learning (VMCL, ABET software, Cat #89542) is a stimulus-response habit (or rule-based) learning task reliant on the striatum (Horner et al., 2013; Delotterie et al., 2015).
General touchscreen training (prior to VMCL)
General Touchscreen Training (prior to VMCL) occurred as in section “General Touchscreen Training (Prior to LDR)” with two differences: mice went through only one habituation stage (Habituation 2) and training occurred with a three window grid (1 × 3). As in section “General Touchscreen Training (Prior to LDR),” the measure reported for these five general touchscreen stages is days to completion (to reach criteria). Due to computer issues, “days to completion” was the only metric extracted for this group, precluding in-depth accuracy analyses of Punish Incorrect as was done in the LDR cohort. After these five training stages, mice then went through VMCL Train and finally VMCL Test.
Visuomotor conditional learning train (schedule name: punish incorrect II)
Visuomotor Conditional Learning Train was designed to teach the mouse to touch two images (both lit white squares) on the screen in a specific order and in rapid succession. The first touch must be to an image presented in the center of the screen, and the second touch must be to an image presented either on the left or right of the screen. Specifically, after trial initiation, the mouse must touch a center white square (200 × 200 pixels; Figure 5A), which then disappears after it is touched. A second white square immediately appears on either the left or right side of the screen in a pseudorandom style, such that a square was located on each side 5 out of 10 times, but not more than three times in a row. If the mouse selected the location with the second white square, a reward (7 μl) was provided, and a 20-s ITI began. However, if the mouse selected the location without a lit white square, then the second stimulus was removed, the house light was illuminated for 5 s to indicate a timeout period, and then finally a 20-s ITI occurred. Then the mouse was presented with a correction trial which must be completed prior to a new set of locations being displayed. VMCL Train was complete when the mouse completed 2 out of 3 consecutive days of 25 trials in 30 min with ≥75% correct. Measures reported for VMCL Train are: days to completion and distribution of percent of mice which reach criteria over time (survival curve), as well as session length, trial number, % correct responses, and number of correction trials on the first and last VMCL Train session.
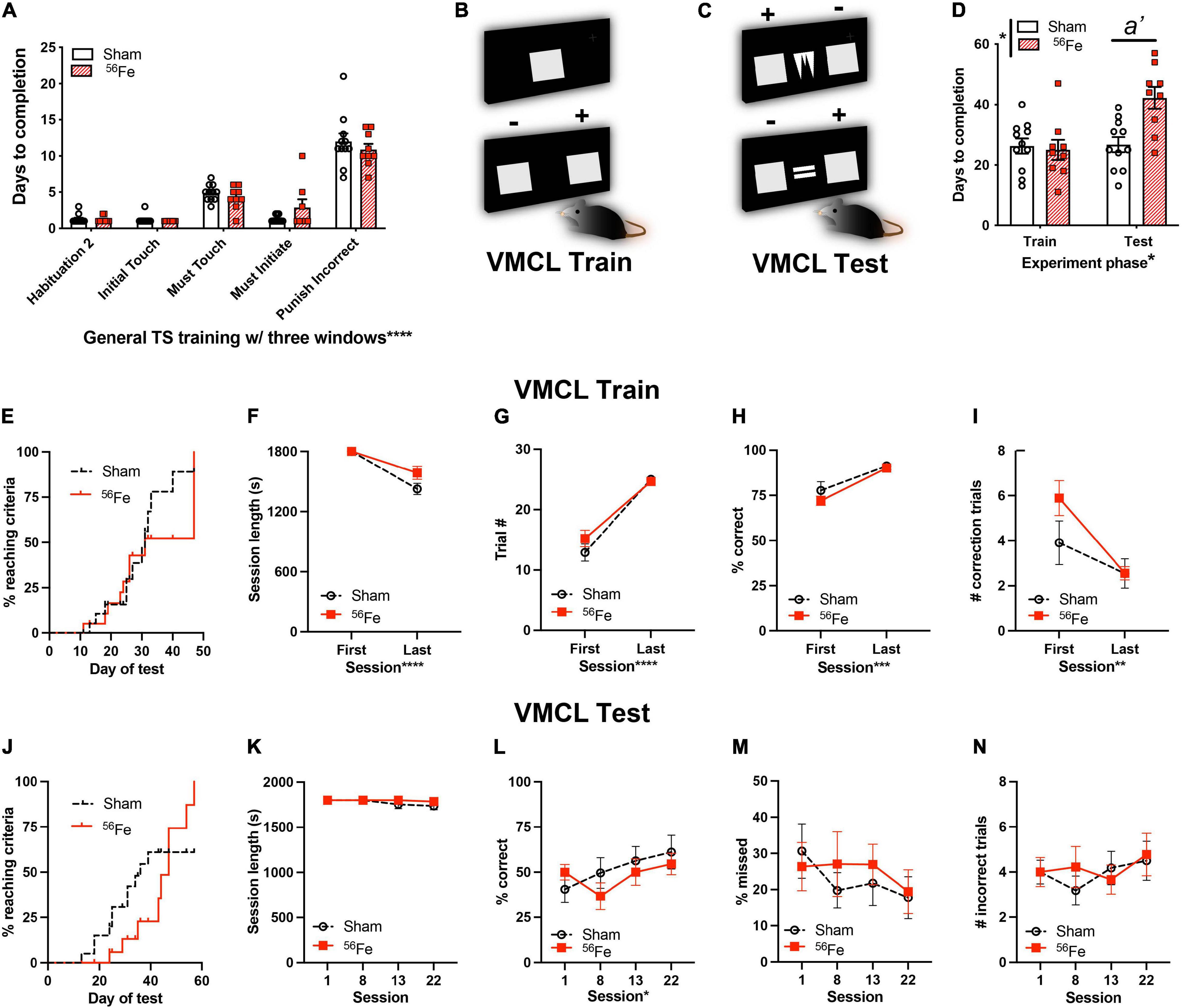
Figure 5. Six-month-old female mice exposed to whole-body 56Fe IRR perform worse than Sham in tests of stimulus-response habit learning. (A) Sham and 56Fe IRR groups performed similarly in each of the five steps of general touchscreen training with three windows: Habituation 2, Initial Touch, Must Touch, Must Initiate, and Punish Incorrect. (B) Schematic of the single, large lit square image (top) and two, large lit squares used for Visuomotor Conditional Learning (VMCL) Train. The mouse learns that touching one square (+) is rewarded while the other (-) is not rewarded. (C) Schematic of the two, large lit squares flanking samples used for VMCL Test. The mouse learns that touching one square (+) is rewarded while the other (-) is not rewarded depending on the center image shown. (D)56Fe IRR mice took a similar number of days to complete VMCL Train - but took more days to complete the VMCL Test - compared to Sham mice. (E) Cumulative distribution function showed no difference between groups in days required to complete VMCL Train. (F–I) 56Fe IRR mice performed similarly as Sham mice in VMCL Train (F: Session length, G: completed trials, H: % correct, I: # correction trials). (J) Cumulative distribution function showed no difference between groups in days required to complete VMCL Test. (K–N) All parameters (K: session length, L: % correct, M: % missed, N: # incorrect trials) between 56Fe IRR and Sham mice were similar. Error bars depict mean ± SEM. Statistical analysis was performed in panels (A,D,K–N): Mixed-effect analysis, in panels (E,J): Mantel-Cox test, in panels (F–I): Two-way RM ANOVA, main effect *p < 0.05, **p < 0.01, ***p < 0.001, ****p < 0.0001. In panel (D), main effects: Experiment phase F1,18 = 8.145, p = 0.0105 and Treatment F1,18 = 6.334, p = 0.0215; interaction: Experiment phase × Treatment F1,18 = 7.329, p = 0.0144, post hoc: a’ p = 0.0014 in Sham vs.56Fe in test, ωp2 = 0.11. s, seconds; TS, touchscreen; VMCL, visuomotor conditional learning. Complete and detailed statistical information provided in Supplementary Table 1.
Visuomotor conditional learning test
Mice were provided with one of two black-and-white images (spikes or horizontal bars, Figure 5C) placed in the center window. Once touched, the center image disappeared and white squares simultaneously appeared on the right and left of the screen. For this task, the center image of the spikes signaled that the mouse should touch the right square, while the center image of the horizontal bars signaled that the mouse should touch the left square. The two center images were presented pseudorandomly for an equal number of times, and the mice had 2 s to touch the white square on the either right or left side of the central image depending on the center image type. If the mouse touched the appropriate image (right or left side), this was considered a correct trial, and the mouse received a reward (7 μl) and then a 20-s ITI occurred. If the mouse touched the inappropriate image (right or left side), this was considered an incorrect trial, and the house light was illuminated for a 5-s timeout period followed by a 20-s ITI. If the mouse did not touch the white square (right or left side) within 2 s, this was considered a “missed” trial, and the house light was illuminated for a 5-s timeout period followed by a 20-s ITI. After either an incorrect or missed trial, a correction trial was run to protect against side bias. VMCL Test was completed when the mouse completed two consecutive days of 25 trials in 30 min with ≥80% correct responses. Measures reported for VMCL Test are: days to completion and distribution of percent of mice which reach criteria over time (survival curve), as well as session length, percent of responses that were correct, percent of missed responses, and number of incorrect trials on the 1st (first), 8th, 13th (intermediate), and 22nd (later) testing sessions (22nd day chosen based on average days to meet the criteria of VMCL Test in Sham group).
Classic Behavior Testing
Elevated plus maze
Elevated Plus Maze (EPM) is a test for anxiety-like behavior (Yun et al., 2018). The maze contained two open arms and two closed arms each with a length of 67 cm and a width of 6 cm with the opaque walls of the closed arms being 17 cm tall (Harvard Apparatus, #760075). Mice were placed on the end of the open arms at the start of the behavior and allowed free movement throughout the maze for 5 min. The parameters of EPM (total distance of movement, entries and duration in the open arms, entries and duration in the closed arms) were scored via EthoVision software (Noldus Information Technology) using nose-center-tail tracking to determine position.
Marble burying
Marble Burying (MB) is a test of repetitive or compulsive-like behavior (Angoa-Pérez et al., 2013; Tran et al., 2020). Transparent polycarbonate cages (25.7 cm × 48.3 cm × 15.2 cm) with filter-top lids (Allentown Inc. #PC10196HT) were used as marble burying arenas. 4–5 cm of wood chip bedding (Beta Chip Bedding, Animal Specialties and Provisions, #NOR301) was evenly distributed to cover the bottom of the cage and 20 glass marbles were laid gently on top of the bedding (four rows with five marbles in each row, evenly spaced). Mice were placed in the marble burying arena and given 20 min to explore and interact with the marbles. After 20 min of testing, marbles were scored by two independent observers and only marbles that were two-thirds or more covered in bedding were counted. The measure reported for this test is percent of marbles buried.
Open field
Open Field (OF) is a test for exploration and anxiety-like behavior (Yun et al., 2018; Tran et al., 2020). The open field arena measured 42 cm × 42 cm × 42 cm (opaque white Plexiglas, customer design Nationwide Plastics). The center zone was established in EthoVision as 14 cm × 14 cm and corner periphery zones were set as 5 cm × 5 cm each. Each mouse was placed in the arena and allowed free movement of the novel environment with recording for 5 min. The parameters of open field (total distance of movement, entries and duration in the center zone, entries and duration in the periphery zone) were scored via EthoVisionXT software (Noldus Information Technology) using nose-center-tail tracking to determine position.
Social interaction
Social Interaction (SI) is a test of exploration, locomotion, and sociability (Yun et al., 2018). Test mice were individually placed in a white open-field chamber (42 cm × 42 cm × 42 cm) that had a discrete interaction zone against one wall (26 cm × 14 cm) inside of which there was an empty plastic and wire mesh container (10 cm × 6 cm). For the first trial, the mouse was placed randomly into either corner of the box opposite to the interaction zone, and the movements of the mouse were tracked using Ethovision software (Noldus Information Technology). Specifically, the time the mouse spent either in the interaction zone or in corners opposite to the interaction zone during a 2.5 min trial was quantified. For the second trial, which began ∼5 min after the first trial, an unfamiliar age and sex-matched C57BL/6J mouse was placed into the plastic and wire mesh container and the container was placed in the interaction zone. Again, the time the mouse spent either in the interaction zone or in corners opposite to the interaction zone during a 2.5 min trial was quantified. Measures reported for Social Interaction are time spent in the interaction zone without and with another mouse placed inside the plastic and wire mesh container.
Forced swim test
Forced Swim Test (FST) is a test of despair-like responses (Yun et al., 2018). The FST was performed to evaluate behavioral withdrawal induced by stress. Mice were placed in a 5L beaker (Corning Inc. Life Sciences, Lowell, MA, United States) filled with 4 L of 25 ± 2°C water. The movements of the mouse were tracked using Ethovision software (Noldus Information Technology) for the entirety of the 6-min session. Mice each went through two, 6-min sessions on consecutive days. Immobile time was measured and the last 4-min of data are reported.
Rigor, Sex as a Biological Variable, Additional ARRIVE 2.0 Details, and Statistical Analysis
The experimental unit in this study is a single mouse. For behavioral studies, mice were randomly assigned to groups. Steps were taken at each experimental stage to minimize potential confounds. For example, mice from the two experimental groups (Sham and 56Fe IRR) were interspersed throughout housing racks at UTSW, CHOP, and BNL (to prevent effects of cage location) and were interdigitated for all weighing and behaviors (to prevent an order effect). In regard to sex as a biological variable, this study only used females due to equipment limitations. Specifically, only eight touchscreen chambers were available (enabling us to train/test a maximum of 56 mice/day) and the chambers were in use 5–6 days/week for several months. Mice had to be trained/tested continuously (preventing us from alternating sexes on different days or running females in the first few months and males in the later months). Male mice irradiated at the same time were tested in classical behavior tests and are the focus of another study. Thus, this study design was intended to examine the impact of space radiation on female mice, not to examine sex differences in response to space radiation. Sample sizes were pre-determined via power analysis and confirmed on the basis of extensive laboratory experience and consultation with CHOP and PennMed statisticians as previously reported (Whoolery et al., 2020). Exact sample number for each group in each figure panel is provided in Supplementary Table 1. Data for each group are reported as mean ± SEM. All analyses were hypothesis-based and therefore pre-planned, unless otherwise noted. Testing of data assumptions (normal distribution, similar variation between control and experimental groups, etc.) and statistical analyses were performed in GraphPad Prism (ver. 9.0.0). Normality was tested via Quantile–Quantile (Q–Q) plots followed by the Shapiro-Wilk test if needed. Since all data were found to be normally distributed, parametric tests were used for further statistical analysis. Analyses with two groups were performed using an unpaired, two-tailed Student’s t-test. Analyses with more than two variables were performed using two-way ANOVA or Mixed-effects analysis with Bonferroni post hoc test; repeated measures (RM) were used where appropriate. Analysis of the distribution of subjects reaching criteria between control and experimental groups (survival curve) was performed with the Mantel-Cox test and significance was defined as ∗p < 0.05. Following best practices to move beyond null hypothesis significance testing and reliance on the p-value and to incorporate estimation statistics (Lakens, 2013; Halsey et al., 2015; Born, 2019; Calin-Jageman and Cumming, 2019; Makin and de Xivry, 2019; Wasserstein et al., 2019; Drummond, 2020), statistical approaches and results including statistical analysis significance (p-values) and effect size (when RM two-way ANOVA p < 0.05: partial omega-squared ωp2 where ≤0.05 small, ≥0.06 medium, ≥0.14 large) are provided in Supplementary Table 1. All data that are helpful for interpreting touchscreen performance are provided in main figures to enable consideration of “positive” data (p < 0.05) in the context of “negative” data. Detailed statistical results are also provided in figure legends for panels in which there is a main effect of Treatment and/or an interaction. A total of n = 8 mice (n = 5 Sham, n = 3 IRR) were outliers based on a priori established experimental reasons (n = 1 Sham did not complete the “Punish Incorrect” stage even by Day 53; n = 1 Sham and n = 1 IRR did not complete “Acquisition” even by Day 58; n = 3 Sham and n = 2 56Fe did not complete “Extinction”) and the data from these mice were excluded from LDR, Acquisition, and Extinction analyses, respectively. Due to health issues, n = 2 Sham and n = 1 IRR mice were not run on classical behavior tests. Experimenters were blinded to treatment until analysis was complete.
Scripts
Prior to statistical analysis, extinction and acquisition data were sorted and extracted. We used a custom Python 3.8.3, SQLite3 2.6.0, and Tkinter 8.6 script developed by the Eisch Lab to extract, calculate needed values, and compile the data into a database. Extracting the data into an output CSV file was managed with another custom script, and these outputs were verified manually. Following this verification, the data were analyzed using GraphPad Prism 8 according to the tests detailed in the Statistical Analysis section. These scripts along with sample data files are available at https://github.com/EischLab/18AExtinction.
Results
Whole-Body 56Fe Particle Irradiation Does Not Change Body Weight or Locomotor Activity and Has Modest Effects on Operant Learning in Female Mice
Six-month-old female C57BL/6J mice received either Sham IRR or Frac whole-body 20 cGy 56Fe (3 exposures of 6.7 cGy every-other day, total 20 cGy; Figure 1). This total dose is submaximal to that predicted for a Mars mission, and the fractionation interval (48 h) was used to allow potential repair processes to occur (Thames, 1985; Cucinotta and Durante, 2006; Hellweg and Baumstark-Khan, 2007). Consistent with a prior report (Whoolery et al., 2020), this dose and fractionation interval of 56Fe do not interfere with normal weight gain between groups (Figure 2A) or locomotor activity (Figure 2B, unpaired t-test, t30 = 0.3919, P = 0.6979; Supplementary Table 1).
Beginning 3-month post-IRR, Sham and 56Fe IRR female mice began training on a touchscreen platform extensively validated in rodents (Figure 1A; Horner et al., 2013; Oomen et al., 2013; Hvoslef-Eide et al., 2016). Mice initially went through six stages of general touchscreen testing (Figure 1A), with performance reflecting operant learning. Sham and 56Fe IRR mice completed all stages of general touchscreen training in similar periods of time (Figure 2C and Supplementary Table 1). Thus, there was no gross difference in operant performance between these Sham and 56Fe IRR mice.
The final stage of general touchscreen testing, Punish Incorrect (PI, where an incorrect trial results in timeout), was next analyzed to a greater extent for two reasons. First, PI is the sole general touchscreen training stage that has an accuracy criterion (% correct). Second, PI is the stage that takes the longest to learn. We measured relevant to accuracy on first vs. last PI day [including individual session length, trial #, % correct, correct touches and latency, intertrial interval (ITI) touches, blank window touches, and reward latency, Figures 2D–L and Supplementary Table 1] to see how 56Fe influenced operant learning (via changes in speed, impulsivity, motivation, and side bias, etc.) (Mar et al., 2013; Swan et al., 2014). 56Fe IRR mice took longer than Sham mice to complete a maximum of 30 trials on the first—but not the last—PI session (Figure 2D). However, on the first and last PI day Sham and 56Fe IRR mice performed similarly in many other PI metrics, including number of trials completed (Figure 2E), percent correct (Figure 2F), ITI touches (Figure 2G), latency of total correct touches to the left and right side of screen (Figure 2H), left correct touch latency (Figure 2I), and right correct touch latency (Figure 2J). In regard to touching a blank window when the stimulus was presented, 56Fe IRR mice had a nearly 5s shorter latency vs. Sham mice during the last session of PI (Figure 2K), suggesting late-developing impulsivity in 56Fe IRR mice. Motivation did not appear changed, as Sham and 56Fe IRR mice had similar reward collection latencies (Figure 2L). This further analysis of PI training stage suggests that 56Fe IRR has modest effects on certain aspects of operant learning (IRR mice are slower to finish in daily PI session and may be more impulsive late in PI), but in many other ways perform indistinguishably from Sham IRR mice in PI and all other operant learning stages.
Female Mice Given Whole-Body 56Fe Particle Irradiation Perform Better Than Sham Particle Irradiation Mice in an Appetitive-Based Location Discrimination Reversal Touchscreen Task
As previously reported, male mice given whole-body 56Fe IRR perform better than Sham IRR mice in an appetitive-based location discrimination (LD) touchscreen task, suggesting improved behavioral discrimination or behavioral pattern separation (Whoolery et al., 2020). The effect of 56Fe IRR exposure on translationally-relevant female mouse touchscreen performance is unknown, and specifically its effect on discrimination and cognitive flexibility is unknown. These are important knowledge gaps, as the proportion of United States female astronauts is increasing and some preclinical work shows the female rodent brain may be less susceptible after charged particle exposure vs. the male rodent brain (Krukowski et al., 2018; Parihar et al., 2020).
To test if whole-body 56Fe IRR improves discrimination learning and impacts cognitive flexibility in adult female mice, Sham and 56Fe female mice were trained and tested on LDR performance (Figure 1). In the LDR Train sessions (Figures 3A–E and Supplementary Table 1; presentation of a two-choice stimulus response with lit squares intermediately-separated), there was a visual difference in percent of Sham and 56Fe subjects reaching criteria, but this difference was rejected by survival curve analysis (Figure 3B). Sham and 56Fe also had similar average days to complete LDR Train (Figure 3C) and completed a similar number of LDR Train trials (Figure 3D) and also had similar accuracy before the first reversal (Figure 3E), indicating similar competency during the overall LDR Train sessions.
Sham and 56Fe IRR female mice were then assessed on overall LDR performance (LDR Test, Figures 3F–V), considering performance when the LDR Test squares were maximally separated (Large separation, Figures 3G,I,K,M,O,Q,S,U) or minimally separated (Small separation, Figures 3H,J,L,N,P,R,T,V), and are analyzed by block (1 block = 4 days LDR counterbalanced with two Large and two Small separation daily sessions, Supplementary Table 1). Sham and 56Fe IRR took a similar amount of time to complete sessions for both the Large (Figure 3G) and Small separation LDR Test trials (Figure 3H), completed a similar number of trials for both Large (Figure 3I) and Small separation LDR Test trials (Figure 3J). Sham and 56Fe IRR mice performance was also assessed for location discrimination reversal learning, which provides insight into both discrimination learning and cognitive flexibility (Vivar et al., 2012; Swan et al., 2014; Graf et al., 2018). First, we assessed discrimination learning by analyzing the percent correct trials made in each group prior to the 1st reversal. In Large – but not Small – separation trials, 56Fe IRR female mice were ∼34% more accurate vs. Sham mice in percent correct trials prior to the 1st reversal (Large: Figure 3K; Small: Figure 3L). However, Sham and 56Fe IRR mice had a similar number of reversals (an index of cognitive flexibility) in both Large (Figure 3M) and Small separation LDR Test trials (Figure 3N). Together these data suggest 56Fe IRR mice have better discrimination learning than Sham mice, but 56Fe IRR mice and Sham have similar cognitive flexibility.
We next probed how 56Fe IRR mice were achieving greater accuracy in the Large separation LDR Test sessions (via possible changes in impulsivity, motivation, and side bias, etc.) (Mar et al., 2013; Swan et al., 2014). 56Fe IRR female mice touched the blank, non-stimulus window more than Sham mice during the 6th (last) block of the Large separation LDR Test block (Figure 3O) and the 4th and 6th blocks of the Small separation LDR Test block (Figure 3P), implying IRR-induced increased impulsivity. 56Fe IRR and Sham mice had similar reward collection latencies in both the Large (Figure 3Q) and Small separation LDR Test blocks (Figure 3R). 56Fe IRR and Sham mice took just a long to press the correct selection on the display in the Large (Figure 3S) and Small separation LDR Test blocks (Figure 3T) as well as the incorrect selection in the Large (Figure 3U) and Small separation LDR Test blocks (Figure 3V). Together these data suggest 56Fe IRR mice have improved accuracy (yet increased impulsivity) in Large separation trials late in LDR Test, but no change in attention or motivation vs. Sham mice. 56Fe IRR mice also have increased impulsivity in Small separation trials late in LDR Test, but no change in performance. These results suggest 56Fe IRR mice are better than Sham IRR mice in key aspects of discrimination learning, despite showing impulsivity.
Whole-Body 56Fe Particle Irradiation Does Not Change Acquisition or Extinction Learning of a Simple Stimulus-Response Task
To determine whether the observed IRR-induced increase in cognitive performance was limited to hippocampal-dependent tasks, we next tested for PFC-dependent executive function (Figure 4 and Supplementary Table 1). The same cohort of Sham and 56Fe IRR female mice underwent simple stimulus-response learning (acquisition; Figures 1, 4A) followed by extinction of acquired learning (Figure 4F). Stimulus-response learning was similar between the groups (Figure 4A) as >75% of mice in both Sham and 56Fe IRR groups reached criteria by 60 days (Figure 4B) and both groups completed the task in a similar number of days (Figure 4C). In addition, when looking at general performance over the course of acquisition, Sham and 56Fe-IRR mice gave a similar number of correct responses to a stimulus (Figure 4E) in comparable times (Figure 4D), again suggesting similar simple stimuli-response learning between groups.
In extinction testing (Figure 4F), Sham and 56Fe IRR mice took a similar number of days to reach criteria, indicating no difference in the rate of extinction learning (Figure 4H). Sham and 56Fe IRR mice also had similar individual session length (Figure 4I) and reached a stable omission criteria (>24 out of 30 response omissions) over the course of testing (Figure 4J). To assess whether these same Touchscreen-experienced mice differed in measures of potential impulsivity or general engagement with the screen, we analyzed blank touches, blank touch latency, and ITI touches (Figures 4K–M). Sham and 56Fe IRR mice had a similar number of blank touches (Figure 4K), speed to make a blank touch (Figure 4L), and ITI touch’s number (Figure 4M). Together these results suggest no effect of 56Fe IRR on task-specific impulsivity. However, in the last extinction session, 56Fe IRR mice took ∼1.5 sec longer to give a correct response vs. Sham mice (Figure 4N). This small but significant increase in latency for 56Fe IRR mice to give a correct response did not influence extinction performance. Therefore, taken together, these data suggest 56Fe IRR does not influence extinction performance.
Female Mice Given Whole-Body 56Fe Particle Irradiation Took Twice as Long as Sham Particle Irradiation Mice to Reach Stimulus-Response Habit Learning Criteria
It has been suggested that systems of “declarative” vs. “habit” memory—which rely on the medial temporal lobe (e.g., hippocampus) and basal ganglia (e.g., caudate-putamen), respectively—compete with one another during behavioral tasks (Poldrack and Packard, 2003). These systems are proposed to be identifiably separable and function simultaneously, “overriding” one another during various learning tasks. To assess whether the observed IRR-induced increase in hippocampal-dependent discrimination learning occurs at the expense of striatal memory circuit functional integrity, a parallel group of mice was used to assess the influence of 56Fe IRR on visuomotor conditional learning (VMCL; Figures 1, 5 and Supplementary Table 1). VMCL reflects stimulus-response habit or “rule-based” learning and relies on intact circuits of the striatum and posterior cingulate cortex (Horner et al., 2013).
Similar to what was seen with the parallel cohort of mice (Figure 2C), during general touchscreen training this cohort of Sham and 56Fe IRR mice completed each training phase in a similar number of days (Figure 5A). Thus, in two parallel cohorts – one assessed 3-mon post-IRR and the other 1-mon post-IRR – there was no overt effect of 56Fe IRR on operant learning. Unfortunately in-depth accuracy analysis of the last stage (Punish Incorrect) was not possible due to computer file inaccessibility. In VMCL Train – an intermediate training phase prior to VMCL Test – Sham and 56Fe IRR mice also did not differ in completion days (26.27 vs. 25 days, respectively, Figures 5B,D). However, in the VMCL Test (Figure 5C), 56Fe IRR mice took nearly twice as many days vs. Sham to reach criteria (Figure 5D). These data suggest a 56Fe IRR-induced impairment in the rate of striatal-mediated learning.
To assess whether a slower rate of VMCL learning in 56Fe IRR mice could be explained by behavioral deficits evident in earlier training stages, we analyzed VMCL Train performance in-depth (Figures 5B,E–I). In VMCL Train, a similar proportion of Sham and 56Fe IRR mice reached criteria over time (50% subjects reached criteria at 31 days in both Sham and 56Fe IRR mice; Figure 5E). Sham and 56Fe IRR mice also had similar length of training sessions (Figure 5F), number of training trials (Figure 5G), response accuracy (Figure 5H), and number of correction trials following an incorrect response (Figure 5I). Taken together, these results suggest no gross impact of 56Fe IRR on the ability to complete VMCL Train.
In VMCL Test (Figure 5C), a similar distribution of the proportion of Sham and 56Fe IRR mice reach criteria over the entire VMCL Test period (Figure 5J). Sham and 56Fe IRR mice had similar VMCL Test performance as indicated by similar session length (Figure 5K), accuracy (Figure 5L), percentage of trials missed due to inactivity (Figure 5M), and number of incorrect trials made during the initial choice stage (Figure 5N). Therefore, while 56Fe IRR mice took more days to complete VMCL Test at certain accuracy vs. Sham mice, this was not due to a difference in other VMCL Train and Test performance measures.
Whole-Body 56Fe Particle Irradiation Does Not Change Measures Relevant to Anxiety, Depression, Repetitive Behavior, and Sociability
56Fe IRR-induced improvements in discrimination learning and the increased number of blank touches during LDR Test could be explained by increased compulsivity or other stereotypic behaviors, or alterations in anxiety- or despair-like behaviors. To assess these possibilities, the same touchscreen-experienced Sham and 56Fe IRR mice were run on a variety of classic non-touchscreen behavior tests including elevated plus maze, marble burying, open field, social interaction, and forced swim test (Figures 1, 6A).
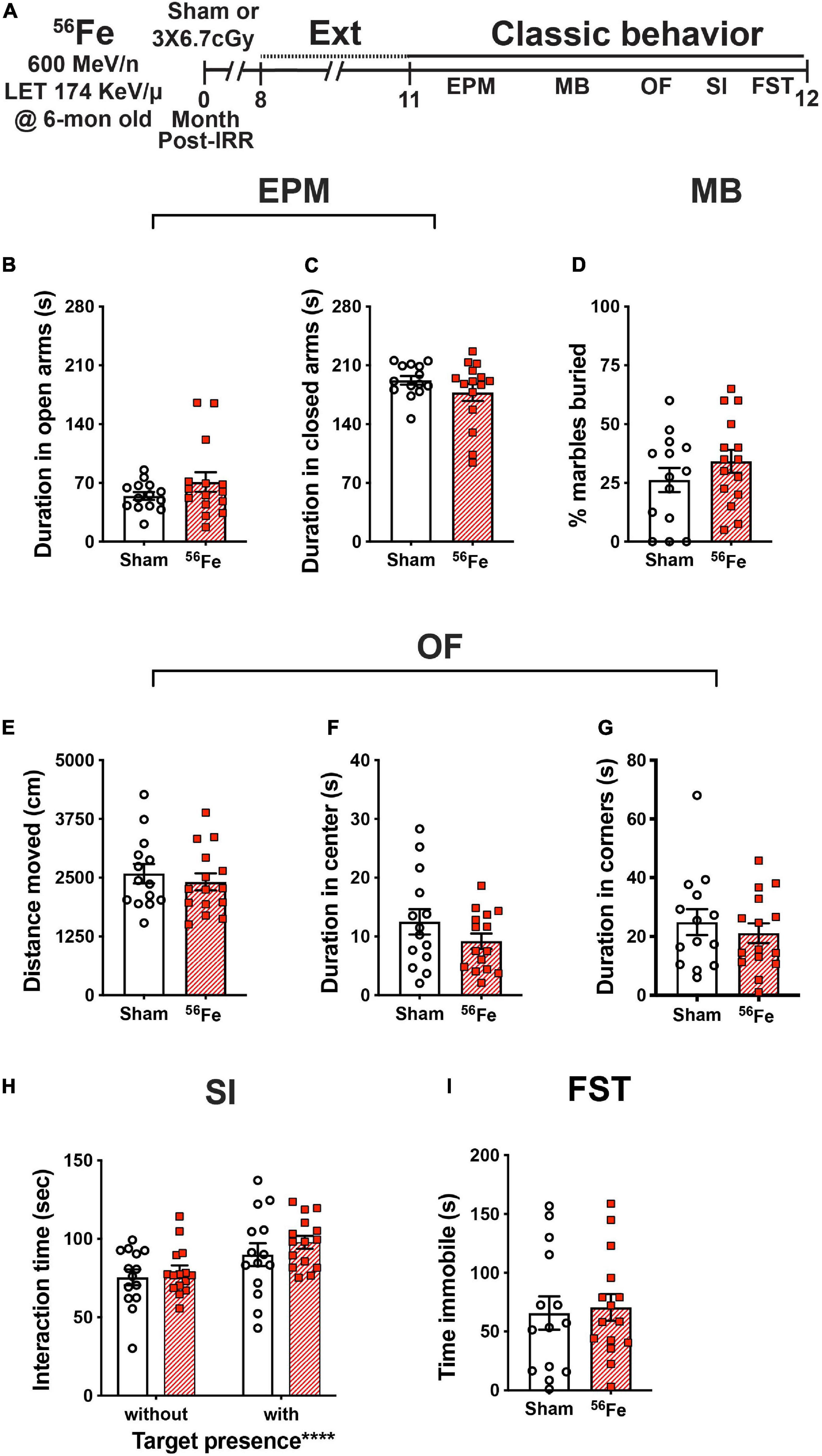
Figure 6. Timeline and results of classical behavior tests show anxiety-, compulsive-like behavior, and despair-like behavior and sociability are generally unaffected in female mice exposed to whole-body 56Fe IRR at 6-mon-old compared to Sham. (A) Six-month old C57BL/6J female mice that received whole-body exposure to 56Fe [0-Month Post-Irradiation (IRR)] and finished touchscreen LDR and Ext were then run on a variety of non-touchscreen classic behavioral tests. (B,C) Sham and 56Fe IRR mice showed no difference in anxiety-like behavior as shown by the time spent in either the open arms (B) or closed arms (C) of the elevated plus maze (EPM). (D) Sham and 56Fe IRR mice showed no difference in compulsive-like behavior as shown by percent of marbles buried in the marble burying (MB) task. (E–G) Sham and 56Fe IRR mice showed no difference in anxiety-like behavior as shown by the distance moved (E) and time spent in the center (F) or corner (G) of the open field (OF). (H) Sham and 56Fe IRR mice showed similar sociability when exposed to an age- and sex-matched conspecific in the social interaction (SI) test. Both groups spent more time in the interaction zone in the presence of a social target vs. in the absence of a social target. (I) Sham and 56Fe IRR mice had similar despair-like behavior as they spent a similar time immobile time in the forced swim test (FST). Error bars depict mean ± SEM. Statistical analysis was performed in panels (B–G,I): Unpaired and two-tailed t-tests in panel (H): Two-way RM ANOVA, main effect of target presence, ****p < 0.0001. Bonferroni’s post hoc analyses showed no difference between Sham and 56Fe IRR groups. cm, centimeters; EPM, elevated plus maze; Ext, extinction; FST, forced swim test; IRR, irradiation; LDR, location discrimination reversal; MB, marble burying; Mon; month; OF, open field; s, seconds; SI, social interaction. Complete and detailed statistical information provided in Supplementary Table 1.
To analyze and compare anxiety-like behavior between Sham and 56Fe IRR groups, mice were exposed to the elevated plus maze and open field, both well-validated anxiety tests in rodent models. In the elevated plus maze, Sham, and 56Fe IRR mice spent a similar amount of time in both the open and closed arms (Figures 6B,C and Supplementary Table 1). In the open field, Sham and 56Fe IRR mice traveled a similar total distance and spent a similar amount of time in both predefined center and corner areas (Figures 6E–G).
An additional test for anxiety-like behavior that also provides an index of repetitive, compulsive-like behavior is marble burying (Thomas et al., 2009; Angoa-Pérez et al., 2013; de Brouwer et al., 2019). Thus, the same female mice also were assessed in a marble burying test. Both Sham and 56Fe IRR mice buried a similar percentage of marbles during a 30-min session, which implies a lack of potentially pathological 56Fe IRR-induced stereotypic and compulsive behavior (Figure 6D).
In certain transgenic rodent models of autism, mice show improved behavioral “pattern separation” alongside social deficits (Benevento et al., 2017). To assess whether 56Fe IRR-induced improvements in discrimination learning shown here were accompanied by social deficits, Sham and 56Fe IRR female mice were exposed to an age-, sex-matched conspecific in a social interaction test. Sham and 56Fe IRR mice spent a similar amount of time in a predefined interaction zone in the absence and presence of a conspecific target (in a plastic and wire mesh enclosure), and spent relatively more time in the interaction zone in the presence vs. absence of a conspecific (Figure 6H). These data indicate no effect of 56Fe IRR on sociability.
We finally looked at whether 56Fe IRR-induced improvement in learning related to behavior shown under conditions that mimic despair (Lezak et al., 2017) by exposing the mice to the forced swim test, which is often used to assess anti-depressive-like efficacy and stress coping (Armario, 2021). Both Sham and 56Fe IRR mice spent a similar amount of time immobile during the 6-min test (Figure 6I), suggesting no 56Fe IRR-induced despair-like phenotype.
Discussion
Here we provide a behavioral profile of female C57BL/6J mice that received fractionated exposure to a Mars mission-relevant dose of whole-body 56Fe IRR at 6-mon of age. From 7- to 18-mon of age, 56Fe IRR mice and their Sham counterparts—which received every experimental manipulation but placement in front of the beam line—were examined via touchscreen and classical behavior tests to assess a range of cognitive abilities. We leveraged the power of the touchscreen platform to provide a holistic, multi-dimensional perspective on mouse behavior, performing in-depth analyses that have become the gold standard in the field (Horner et al., 2013; Oomen et al., 2013; Beraldo et al., 2019). Six differences emerged between Sham and 56Fe IRR mice. Relative to Sham female mice, 56Fe IRR female mice (1) took longer (27% longer) to complete the first session of the last stage of general training; (2) took ∼5 s less (71% less time) to touch a blank window during the last stage of general training; (3) had a greater percent of correct trials (34% more) when distinguishing conditioned stimuli separated by a large (but not small) distance, specifically prior to their first reversal and late in location discrimination reversal testing; (4) touched a blank window more when distinguishing conditioned stimuli separated by a large or small distance (range of 43–63% more), specifically late in location discrimination reversal testing; (5) took ∼1 s longer (34% longer) to touch the lit window (an incorrect response) in the last extinction session; and (6) took more than twice as many days (57% as many) to reach criteria in the visuomotor conditioned learning test. Sham and 56Fe IRR female mice were similar in the other many touchscreen and classical behavior metrics collected. Below we discuss these findings in mice as they relate to cognitive domains, indicate the strengths and limitations of our work, and speculate what these findings mean for NASA’s risk assessment for female astronauts in future deep space missions.
Our touchscreen data show that 56Fe IRR female mice had ∼34% more correct trials relative to Sham mice during Large separation trials prior to their first reversal in LDR Test. The effect size for this 56Fe IRR-induced increase in location discrimination is small (Supplementary Table 1), and it is only seen in trials that use a Large, not Small, separation. In fact, both Sham and 56Fe IRR female mice perform just above chance (∼50% correct prior to first reversal, which is expected given the challenge of the task and the age of the mice at testing), and it is only in the last block that the 56Fe IRR mice perform better. These caveats aside, these data suggest 56Fe IRR improves location discrimination reversal learning in female mice vs. Sham female mice. Given the well-described role of the hippocampus in LD and LDR (Clelland et al., 2009; McTighe et al., 2009; Swan et al., 2014), one interpretation of these data is that 56Fe IRR improves hippocampal function or perhaps integrity. There are three notable aspects of this interpretation. First, while we cannot make any direct sex comparisons or claim sex-specific effects of fractionated 56Fe exposure on cognition, it is appropriate to mention prior work performed with male mice. Prior work with male mice reported that the same 56Fe IRR parameters used here improved discrimination learning in an LD test (Whoolery et al., 2020); LDR was not assessed in that study. In that study, male mice had a higher percentage of accurate responses and reached LD criteria in fewer days relative to Sham male mice. While there are additional distinctions between these studies, it is notable that both female and male mice exposed to 56Fe IRR show indices of improved LD or LDR—and thus perhaps improved hippocampal function—vs. Sham mice. Second, it is interesting to compare the interpretation of the present data (56Fe IRR female mice have improved LDR and hippocampal function) to prior literature on the impact of space radiation on hippocampal function. Many rodent studies suggest HZE particle exposure is detrimental to brain physiology and functional cognitive output, with noted negative impact on hippocampal function and also on operant behavior (Rabin et al., 2004, 2007b; Blakely and Chang, 2007; Rabin, 2012; Cucinotta et al., 2014; Kokhan et al., 2016; Nelson, 2016; Cekanaviciute et al., 2018; Jandial et al., 2018; Cucinotta and Cacao, 2019; Kiffer et al., 2019; Limoli, 2020; Britten et al., 2021b; Davis et al., 2021). It is only relatively recently that rodents have been irradiated at “astronaut age,” that low doses of space radiation have been used to perturb hippocampal function, and that female rodents have been more commonly studied. Indeed, some work suggests female rodents are more susceptible than males to space radiation, while other preclinical work suggests the female rodent brain may be protected from radiation-induced immune and cognitive deficits (Villasana et al., 2006, 2010; Cherry et al., 2012; Krukowski et al., 2018; Hinkle et al., 2019; Liu et al., 2019; Parihar et al., 2020). With these studies in mind, it is notable that touchscreen analysis of both female and male mice (of “astronaut age” at time of exposure) shows 56Fe IRR improves LDR (present results) or LD (Whoolery et al., 2020), respectively, vs. Sham exposure without influencing other cognitive domains (exceptions in female mice are discussed below). However, in contrast to our present work in female mice, another study with mature male mice exposed to a single bolus of whole-body low dose 56Fe showed a dose- and time-dependent impact on a non-touchscreen hippocampal-dependent task: novel object recognition (Impey et al., 2016). Specifically, 2 weeks post-IRR, male mice exposed to 0.1 or 0.4 cGy spent a similar percentage of time investigating a familiar and novel object, while mice exposed to 0.2 cGy spent a greater percentage time investigating a novel object. Twenty weeks post-IRR, all IRR mice spent a greater percentage of time investigating a novel object. Future studies are needed to assess whether female mice have a similar disruption of hippocampal-dependent function soon after IRR, and to understand how results from a behavioral test that takes relatively few days to run (novel object) relate to a behavioral test that requires weeks to months to run (touchscreen training and testing). A third perspective on these data with 56Fe IRR improving LDR in female mice is highlighted in recent work showing that the rodent brain has a compensatory, dynamic, time-dependent response to 56Fe IRR (Miry et al., 2021). More longitudinal studies are needed to clarify the time course of the LDR “improvement” reported here in 56Fe IRR female mice.
Another outcome of our touchscreen data is that 56Fe IRR female mice take more days to reach criteria relative to Sham mice in VMCL Test, suggesting impaired in stimulus-response habit learning. Given the reliance of stimulus-response rule-based habit learning on intact striatal circuits (Horner et al., 2013; Delotterie et al., 2015), our data suggest 56Fe IRR female mice have striatal/basal ganglia dysfunction. Of note, while we did not observe behavioral changes that are indicative of gross striatal dysfunction (normal locomotor, and marble burying, etc.), both high and low doses of HZE particles can produce maladaptive striatal plasticity and/or compromise the dopaminergic system of rodents (Joseph et al., 1992, 1993, 1994; Kiffer et al., 2019). Our finding that 56Fe IRR female mice have impaired stimulus-response rule-based habit learning in an operant touchscreen task may call to mind work in retired breeder male rats where exposure to ≤15 cGy of 600 MeV/n 56Fe particles impairs the acquisition—but not the long-term memory—of rules in the attentional set-shifting assay (Jewell et al., 2018). However, the operant nature of the touchscreen task used in the present work is in contrast to the associations that must be made in attentional set-shifting, thereby limiting comparisons between these studies. On a more comparable level, our finding that 56Fe IRR female mice have impaired stimulus-response habit learning is distinct from what is seen in male mice, as 56Fe IRR male mice perform similarly to Sham mice on VMCL (Whoolery et al., 2020). These results are of course not directly comparable since the interval between IRR and touchscreen training/testing was 2 mon for females but 4 mon for males. A 56Fe IRR-induced deficit in stimulus-response rule-based habit learning in female mice is actually opposite of our hypothesis, which was fueled by studies suggesting the female rodent brain may be spared from the negative impact of HZE particle exposure (Rabin et al., 2013; Krukowski et al., 2018). More specifically, when exposed to space radiation, female mice—do not show deficits in social interaction or novel social and object recognition memory, do not show anxiety-like phenotypes, and do not have the microglia activation and hippocampal synaptic losses seen in IRR male mice (Krukowski et al., 2018; Parihar et al., 2020). Thus, our data presented here add to the growing literature that whole-body exposure to HZE particles—such as 56Fe—affects cognition of female mice in a circuit-specific manner.
While there are many manuscripts that report the influence of HZE particle exposure on rodent operant behavior Rabin et al., 2002, 2005a,b,c, 2007a, 2011, 2012, 2013, 2014a,b, 2015a,b, 2018, 2019a,b; Shukitt-Hale et al., 2007; Cahoon et al., 2020; Whoolery et al., 2020), few use Mars-relevant doses of HZE (<1Gy) and delivery (e.g., whole-body exposure) (Rabin et al., 2014b; Cahoon et al., 2020; Whoolery et al., 2020), and only one uses rodents that are “astronaut age” at irradiation (∼6 mon old at time of exposure) (Whoolery et al., 2020). “Operant behavior” is an umbrella term, and these three publications test different types of operant behavior. Despite this and other distinct experimental parameters (e.g., rodent species, radiation particle used), it may be useful to compare the impact of space radiation on operant behavior as reported in these three studies. In studies with young male rats (Rabin et al., 2014b; Cahoon et al., 2020), whole-body exposure to doses of 16O (1, 5, 10, and 25 cGy) decreased performance of a striatal-dependent operant task (responding on an ascending fixed-ratio reinforcement schedule) 2-mon post-IRR, while 56Fe had dose- and time-post-IRR-dependent effects; both 25 and 50 cGy decreased performance 3-mon post-IRR, and 25cGy actually improved performance 11-mon post-IRR. In the study with mature (“astronaut age”) male mice (Whoolery et al., 2020), whole-body fractionated exposure to 56Fe did not change performance on either an operant task that engages PFC-perirhinal cortex-striatal circuits (pairwise discrimination) 2-mon post-IRR or a task that engages the striatum (VMCL) 4-mon post-IRR, but improved performance on LD. The present work is the only study to test operant behavior in mature female rodents after whole-body exposure to a Mars-relevant space radiation regimen. Clearly more work is needed to understand how space radiation influences the very broad spectrum of operant performance in both female and male rodents.
Taken together with our data presented here on the performance of female mice on LDR, it is notable that female mice perform “worse” on a striatal-dependent task, VMCL, but “better” on a hippocampal-dependent task, LDR. The present VMCL Test results are therefore interesting in regard to the theory of multiple memory systems (Nadel, 1992; Poldrack and Packard, 2003). Human and non-human memory studies suggest memory formation and consolidation are dependent on both hippocampal and non-hippocampal (i.e., basal ganglia or striatal) cooperative networks or memory systems. These systems encode for different memory types, with hippocampal circuits encoding relational memory for declarative past events and striatal circuits encoding for acquisition of stimulus-response rule-based habit learning and some forms of Pavlovian conditioning (Poldrack and Packard, 2003). These networks also compete. In amnesic patients with partial temporal lobe damage, hippocampal-reliant recognition memory is decreased while striatal-mediated motor learning is spared (Tranel et al., 1994). Conversely, in patients with basal ganglia damage, striatal memory function is decreased while hippocampal memory function is spared (Heindel et al., 1989). Here we report an 56Fe IRR-induced improvement in hippocampal-based discrimination learning in female mice yet deficits in striatal-dependent rule-based learning. We advocate for more specific evaluation of striatal-reliant behavioral patterns after HZE exposure using other touchscreen (i.e., autoshaping) (Horner et al., 2013) or other operant paradigms (rodent psychomotor vigilance test) (Davis et al., 2016), as such studies may clarify whether the improved discrimination learning shown here is accompanied by general basal ganglia-learning deficits. Additional study is also needed to determine if the results presented here in female mice—improved hippocampal-based LDR, worse striatal-based VMCL—are a result of memory system competition.
In addition to the improved performance in LDR and decreased performance in VMCL, there are two other aspects of our data worth discussing. One, 56Fe IRR and Sham female mice in general performed similarly in Acquisition and Extinction, suggesting no difference in prefrontal cortical function. An exception is response latency; 56Fe IRR female mice take ∼1.5 s longer than Sham female mice to press the image. While this difference did not influence any other metric in Extinction, it is notable since the “correct” response in Extinction is to not touch the image. Here the 56Fe IRR female mice still press the image (which is an incorrect response) but take slightly longer to press it. Future studies will be needed to assess whether this longer latency means the 56Fe IRR mice are “in conflict” about making a response (but press it anyways). Two, there are indications that 56Fe IRR mice may be more impulsive. In the last and longest stage of general touchscreen training (PI), 56Fe IRR mice tested 3-mon post-IRR took 71% less time to touch blank windows in the last session vs. Sham mice. This is notable in that 56Fe IRR mice initially had 27% longer sessions early in PI. This faster blank touch in 56Fe IRR mice is not due to changes in attention, locomotor ability, or motivation since there are no differences between 56Fe IRR and Sham mice in the latency to touch the correct image or collect the reward. We interpret the shorter latency to touch a blank window in the last PI session 3-mon post-IRR as 56Fe IRR-induced impulsivity. It is unclear if the faster blank touch latency in 56Fe IRR mice late in PI is due to time post-IRR; we were unable to assess latency and other accuracy metrics in Sham and 56Fe IRR mice tested 1-mon post-IRR due to computer file issues. Another suggestion of impulsivity from our data is that 56Fe IRR mice touched blank windows more in both Large and Small separation trials near the end of LDR Test. As research suggests striatal circuits can also be involved in impulsive as well as habit behaviors (Fineberg et al., 2010; Lipton et al., 2019), it will be interesting for future space radiation studies to more specifically target assessment of impulsivity as it relates to striatal function and integrity.
The mechanism underlying 56Fe IRR-induced improvement in discrimination learning and decrement in stimulus-response rule-based is unknown, although the hippocampus and striatum, respectively, are linked to these functions (Clelland et al., 2009; McTighe et al., 2009; Horner et al., 2013; Oomen et al., 2013; Delotterie et al., 2015). Interestingly, a recent study in both female and male mice reports 56Fe IRR-induced changes in hippocampal cellular, synaptic, and behavioral plasticity 2-mon post-IRR normalize 6-mon post-IRR, and are actually enhanced 12-mon post-IRR (Miry et al., 2021). Therefore a reasonable hypothesis is that the improved hippocampal-dependent discrimination learning and decreased striatal-based habit learning shown here in female mice are due to dynamic and compensatory processes post-IRR that are brain-region specific. Future assessment of this hypothesis ideally would continue to include behavioral tests reliant on other brain regions (such as the PFC) as we have done here and as others have done as well (e.g., Britten et al., 2016; Parihar et al., 2018; Acharya et al., 2019; Liu et al., 2019; Allen et al., 2020; Whoolery et al., 2020; Miry et al., 2021).
There are limitations to the present study. The first limitation is our use of a fractionated exposure. In principle, in vivo whole-body fractionated exposure to single (or mixed) particles of space radiation has translational relevance even beyond NASA (Held et al., 2016; Simonsen et al., 2020). In the brain, there is a limited literature on the effect of fractionated vs. non-fractionated in vivo whole-body exposure to a space radiation-relevant single particle; more studies have been done in other systems, such as the cardiac system (Leith et al., 1982; Chang et al., 2007; Rivera et al., 2013; Whoolery et al., 2017, 2020; Davis et al., 2021; Mao et al., 2021). An increasing number of in vivo studies on space radiation and cognition deliver fractions of mixed—rather than single—beams (Kiffer et al., 2018; Krukowski et al., 2018; Raber et al., 2019; Holden et al., 2021). However, HZE particle exposure is stochastic, making it challenging for the field to agree on an in vivo fractionation exposure paradigm. This challenge likely has contributed to fractionation being underutilized in in vivo basic science experiments, which raises another obstacle: the difficulty in comparing data from fractionation experiments to studies where a similar dose and energy of radiation are given in a non-fractionated manner (e.g., single bonus to the whole-body). On a related note, the present work uses a fractionation interval (48 h) that putatively allows potential repair processes to occur (Thames, 1985; Cucinotta and Durante, 2006; Hellweg and Baumstark-Khan, 2007). While we and others have published indices of DNA damage (e.g., 53BP1) in brain tissue after exposure to space radiation (DeCarolis et al., 2014) and DNA damage indices are evident in normal brain tissue and after injury or radiation (Robbins et al., 2012; Watson and Tsai, 2017; Davis and Vemuganti, 2021), further research is needed to determine if this 48 h fractionation interval is applicable to the brain and if a model of DNA damage and repair that is highly-influential in radiobiology has relevance to brain tissue and cognitive function (Curtis, 1986). While no fractionation regimen will suit all scientists, for relevance to deep space missions future in vivo studies examining the influence of space radiation on the brain may benefit from using protracted low-dose per fraction regimens or just chronic exposure, as have been used in rodents (Brown et al., 2005; Acharya et al., 2019; Borak et al., 2019; Britten et al., 2021a; Krishnan et al., 2021). A second limitation is that the two cohorts of female mice assessed here (tested on LDR/Ext/classical behaviors vs. VMCL, Figure 1A vs. 1B) underwent touchscreen testing at two different institutions due to the lab moving institutions. Since the experiments were distinct between the two institutions, it was not possible to perform an analysis in which institution was a covariate. Ideally the behavioral experiments here would be performed again in the future at a single institution, and with sufficient resources and equipment to enable several dependent measures to be assessed in both groups at the same time post-IRR. However, the reliability (including inter-institutional reliability) of the operant touchscreen platform is well documented (Beraldo et al., 2019; Dumont et al., 2020; Sullivan et al., 2021), and therefore we felt it appropriate to present these data in the same work. Third, due to equipment limitations, this study focuses only on female mice. It is inappropriate for us to compare the female performance reported here with our prior work on male mice irradiated with similar exposure parameters (Whoolery et al., 2020), as that prior work used a distinct LD paradigm relative to the one used here. For example, in that prior work with male mice, the CS+ alternated at the beginning of each day, while in the present work the CS+ “reversed” throughout each test after criteria was met. Also, those male mice received a random mix of large and small separation stimuli within each day of testing, while in the present work female mice received 2 days of large separation and two subsequent days of small separation. While the goal of the present study was to examine the impact of space radiation on female mice (not to examine sex differences in response to space radiation) and thus only female mice were examined, ideally future mechanistic studies would assess both female and male mice in parallel. Fourth, the touchscreen and radiation work shown here in mice and elsewhere in rats are appetitive tasks, typically employing food (or water) restriction to increase the rodent’s motivation to perform (e.g., Davis et al., 2014; Hadley et al., 2015; Jewell et al., 2018; Cahoon et al., 2020). In male mice, food restriction and touchscreen training transiently increase corticosterone levels in fecal boli (Mallien et al., 2016). Notably, levels of this stress hormone return to baseline after 2–6 weeks, a period of time during which general touchscreen training can be completed. While the benefits of a reward-based test (where aversive stimuli are avoided but food restriction is employed) may outweigh the drawbacks (Bussey et al., 2012; Horner et al., 2013), the influence of food or water restriction on stress is a limitation that should be kept in mind when interpreting these touchscreen behavior results (as well as results from any appetitive tasks that use food restriction). A final limitation of this work is the classical behavior tests in the present work were performed months after the touchscreen testing. The length of time between these two types of testing makes it difficult to know what effect space radiation would have on classical behavior if tested at the same time post-IRR as touchscreen testing. Ideally future experiments will test if there is indeed a lack of IRR-induced change in classical behavior performance by examining parallel groups of mice tested in classic vs. touchscreen behaviors.
In conclusion, we have used a translationally-relevant rodent touchscreen battery to analyze the functional integrity of female mouse cognitive domains and associated brain circuits following exposure to the HZE particle 56Fe, a major component of space radiation that is a potential threat to the success of future crewed interplanetary missions. Our data in female mice: (1) suggest an IRR-induced competition between memory systems, as we see improved hippocampal-dependent memory and decreased striatal-dependent memory, (2) show that IRR induces sex-specific changes in cognition, (3) suggest the power that extensive multimodal behavioral analyses would have in helping standardize reporting of results from disparate behavioral experiments, and (4) underscore the importance of measuring multiple cognitive processes in preclinical space radiation risk studies, thereby preventing NASA’s risk assessments from being based on a single cognitive domain.
Data Availability Statement
Data will be made available on written request to the corresponding authors.
Ethics Statement
The animal study was reviewed and approved by three Ethics committees [the Institutional Animal Care and Use Committees at the University of Texas Southwestern Medical Center (UTSW), Children’s Hospital of Philadelphia (CHOP), and Brookhaven National Laboratories (BNL)]. Specifically, animal procedures and husbandry were in accordance with the National Institutes of Health Guide for the Care and Use of Laboratory Animals, and performed in IACUC-approved facilities at UT Southwestern Medical Center [UTSW, Dallas TX; AAALAC Accreditation #000673, PHS Animal Welfare Assurance D16-00296, Office of Laboratory Animal Welfare (OLAW) A3472-01], Children’s Hospital of Philadelphia [CHOP, Philadelphia, PA; AAALAC Accreditation #000427, PHS Animal Welfare Assurance D16-00280 (OLAW A3442-01)] and Brookhaven National Laboratories [BNL, Upton NY; AAALAC Accreditation #000048, PHS Animal Welfare Assurance D16-00067 (OLAW A3106-01)].
Author Contributions
IS, SY, CW, and AE: conceptualization and methodology. YR: software. IS, SY, PK, and AE: validation, writing – original draft, and visualization. IS, SY, and PK: formal analysis and data curation. IS, SY, CW, FT, RR, MD, and AG: investigation. SY and AE: resources and projection administration. IS, SY, PK, FK, and AE: writing – review and editing. SY, AS, and AE: supervision. SY, AS, FK, and AE: funding acquisition. All authors contributed to the article and approved the submitted version.
Funding
IS was supported by the UPenn Post Baccalaureate Research Education Program (PennPREP) which is supported by a grant from the NIH (R25GM071745, PI: K. L. Jordan-Sciutto) and additional funding from Biomedical Graduate Studies at the University of Pennsylvania. SY was supported by an NIH Institutional Training Grant (MH076690, PI: C. A. Tamminga), NNX15AE09G (PI AE), MH107945 (PI AE), a 2018 PENN McCabe Pilot grant, a 2019 IBRO travel grant, and is currently supported by 2019 NARSAD Young Investigator Grant from the Brain and Behavior Research Foundation, a 2020 PENN Undergraduate Research Foundation grant, and a 2021 NASA HERO grant (80NSSC21K0814). CW was supported by an NIH Institutional Training grant (DA007290, PI AE). YR was supported by an Undergraduate Translational Research Internship Program under Penn’s Institute for Translational Medicine and Therapeutics which is supported by an NIH Institutional Clinical and Translational Science Award TR001878, PI: G. A. Fitzgerald). AS was supported by the American Heart Association (14SDG18410020), NIH/NINDS (NS088555), the Dana Foundation David Mahoney Neuroimaging Program, and The Haggerty Center for Brain Injury and Repair (UTSW). FK was supported by the Translational Research Institute for Space Health (TRISH) through NASA cooperative agreement NNX16AO69A. This research was also supported by NASA grants NNX07AP84G (co-I AE), NNX12AB55G (co-I AE), and NNX15AE09G (PI AE), NIH grants DA007290, DA023555, DA016765, and MH107945 to AE and R15 MH117628 (PI K. G. Lambert), and a pilot grant from the University of Pennsylvania Perelman School of Medicine Department of Radiation Oncology (co-PI with Y. Fan). The content of this work is solely the responsibility of the authors and does not necessarily represent the official views of the NIH or NASA.
Conflict of Interest
The authors declare that the research was conducted in the absence of any commercial or financial relationships that could be construed as a potential conflict of interest.
Publisher’s Note
All claims expressed in this article are solely those of the authors and do not necessarily represent those of their affiliated organizations, or those of the publisher, the editors and the reviewers. Any product that may be evaluated in this article, or claim that may be made by its manufacturer, is not guaranteed or endorsed by the publisher.
Acknowledgments
We thank many scientists for technical support and helpful conversations including Kyung Jin Ahn, Lyles Clark, Shibani Mukherjee, Guillermo Palchik, Ann Stowe, Vanessa Torres, Angela K. Walker, and Kielen Zuurbier. We also thank staff members of the Brookhaven National Laboratory and the NASA Space Radiation Laboratory, including Adam Rusek (Physics team leader), MaryAnn Petry (animal support director), Peter Guida (organization and technical support director) as well as all of their team members who help make our experiments possible.
Supplementary Material
The Supplementary Material for this article can be found online at: https://www.frontiersin.org/articles/10.3389/fnbeh.2021.722780/full#supplementary-material
References
Acharya, M. M., Baulch, J. E., Klein, P. M., Baddour, A. A. D., Apodaca, L. A., Kramár, E. A., et al. (2019). New concerns for neurocognitive function during deep space exposures to chronic, low dose-rate, neutron radiation. eNeuro 6:ENEURO.0094-19.2019. doi: 10.1523/ENEURO.0094-19.2019
Allen, B. D., Syage, A. R., Maroso, M., Baddour, A. A. D., Luong, V., Minasyan, H., et al. (2020). Mitigation of helium irradiation-induced brain injury by microglia depletion. J. Neuroinflam. 17:159. doi: 10.1186/s12974-020-01790-9
Angoa-Pérez, M., Kane, M. J., Briggs, D. I., Francescutti, D. M., and Kuhn, D. M. (2013). Marble burying and nestlet shredding as tests of repetitive, compulsive-like behaviors in mice. J. Vis. Exp. 82:50978. doi: 10.3791/50978
Armario, A. (2021). The forced swim test: Historical, conceptual and methodological considerations and its relationship with individual behavioral traits. Neurosci. Biobehav. Rev. 128, 74–86. doi: 10.1016/j.neubiorev.2021.06.014
Basner, M., Savitt, A., Moore, T. M., Port, A. M., McGuire, S., Ecker, A. J., et al. (2017). Cognition Test Battery. Washington, DC: PsycTESTS Dataset.
Benevento, M., Oomen, C. A., Horner, A. E., Amiri, H., Jacobs, T., Pauwels, C., et al. (2017). Haploinsufficiency of EHMT1 improves pattern separation and increases hippocampal cell proliferation. Sci. Rep. 7:40284. doi: 10.1038/srep40284
Beraldo, F. H., Palmer, D., Memar, S., Wasserman, D. I., Lee, W.-J. V., Liang, S., et al. (2019). MouseBytes, an open-access high-throughput pipeline and database for rodent touchscreen-based cognitive assessment. Elife 8:e49630. doi: 10.7554/eLife.49630
Blakely, E. A., and Chang, P. Y. (2007). A review of ground-based heavy ion radiobiology relevant to space radiation risk assessment: Cataracts and CNS effects. Adv. Space Res. 40, 1307–1319. doi: 10.1016/j.asr.2007.03.070
Borak, T. B., Heilbronn, L. H., Krumland, N., and Weil, M. M. (2019). Design and dosimetry of a facility to study health effects following exposures to fission neutrons at low dose rates for long durations. Int. J. Radiat. Biol. 97, 1063–1076. doi: 10.1080/09553002.2019.1688884
Born, R. T. (2019). Banishing “Black/White Thinking”: A trio of teaching tricks. eNeuro 6:ENEURO.0456-19.2019. doi: 10.1523/ENEURO.0456-19.2019
Britten, R. A., Wellman, L. L., and Sanford, L. D. (2021b). Progressive increase in the complexity and translatability of rodent testing to assess space-radiation induced cognitive impairment. Neurosci. Biobehav. Rev. 126, 159–174. doi: 10.1016/j.neubiorev.2021.01.027
Britten, R. A., Duncan, V. D., Fesshaye, A. S., Wellman, L. L., Fallgren, C. M., and Sanford, L. D. (2021a). Sleep fragmentation exacerbates executive function impairments induced by protracted low dose rate neutron exposure. Int. J. Radiat. Biol. 97, 1077–1087. doi: 10.1080/09553002.2019.1694190
Britten, R. A., Miller, V. D., Hadley, M. M., Jewell, J. S., and Macadat, E. (2016). Performance in hippocampus- and PFC-dependent cognitive domains are not concomitantly impaired in rats exposed to 20cGy of 1GeV/n (56)Fe particles. Life Sci. Space Res. 10, 17–22. doi: 10.1016/j.lssr.2016.06.005
Brown, W. R., Thore, C. R., Moody, D. M., Robbins, M. E., and Wheeler, K. T. (2005). Vascular damage after fractionated whole-brain irradiation in rats. Radiat. Res. 164, 662–668. doi: 10.1667/RR3453.1
Bussey, T. J., Holmes, A., Lyon, L., Mar, A. C., McAllister, K. A. L., Nithianantharajah, J., et al. (2012). New translational assays for preclinical modelling of cognition in schizophrenia: the touchscreen testing method for mice and rats. Neuropharmacology 62, 1191–1203. doi: 10.1016/j.neuropharm.2011.04.011
Cahoon, D. S., Shukitt-Hale, B., Bielinski, D. F., Hawkins, E. M., Cacioppo, A. M., and Rabin, B. M. (2020). Effects of partial- or whole-body exposures to 56Fe particles on brain function and cognitive performance in rats. Life Sci. Space Res. 27, 56–63. doi: 10.1016/j.lssr.2020.07.006
Calin-Jageman, R. J., and Cumming, G. (2019). Estimation for better inference in neuroscience. eNeuro 6:ENEURO.0205-19.2019. doi: 10.1523/ENEURO.0205-19.2019
Cekanaviciute, E., Rosi, S., and Costes, S. V. (2018). Central nervous system responses to simulated galactic cosmic rays. Int. J. Mol. Sci. 19:3669. doi: 10.3390/ijms19113669
Chancellor, J. C., Scott, G. B. I., and Sutton, J. P. (2014). Space radiation: the number one risk to astronaut health beyond low earth orbit. Life 4, 491–510. doi: 10.3390/life4030491
Chang, P. Y., Bakke, J., and Puey, A. (2007). Fractionated exposure of high energy iron ions has a sparing effect in vivo. Adv. Space Res. 40, 568–575. doi: 10.1016/j.asr.2007.05.034
Cherry, J. D., Liu, B., Frost, J. L., Lemere, C. A., Williams, J. P., Olschowka, J. A., et al. (2012). Galactic cosmic radiation leads to cognitive impairment and increased aβ plaque accumulation in a mouse model of Alzheimer’s disease. PLoS One 7:e53275. doi: 10.1371/journal.pone.0053275
Clelland, C. D., Choi, M., Romberg, C., Clemenson, G. D. Jr., Fragniere, A., Tyers, P., et al. (2009). A functional role for adult hippocampal neurogenesis in spatial pattern separation. Science 325, 210–213. doi: 10.1126/science.1173215
Cucinotta, F. A. (2015). Review of NASA approach to space radiation risk assessments for mars exploration. Health Phys. 108, 131–142. doi: 10.1097/hp.0000000000000255
Cucinotta, F. A., Alp, M., Sulzman, F. M., and Wang, M. (2014). Space radiation risks to the central nervous system. Life Sci. Space Res. 2, 54–69. doi: 10.1016/j.lssr.2014.06.003
Cucinotta, F. A., and Cacao, E. (2019). Risks of cognitive detriments after low dose heavy ion and proton exposures. Int. J. Radiat. Biol. 95, 985–998. doi: 10.1080/09553002.2019.1623427
Cucinotta, F. A., and Durante, M. (2006). Cancer risk from exposure to galactic cosmic rays: implications for space exploration by human beings. Lancet Oncol. 7, 431–435. doi: 10.1016/S1470-2045(06)70695-7
Cucinotta, F. A., Kim, M.-H. Y., and Ren, L. (2006). Evaluating shielding effectiveness for reducing space radiation cancer risks. Radiat. Meas. 41, 1173–1185. doi: 10.1016/j.radmeas.2006.03.011
Curtis, S. B. (1986). Lethal and potentially lethal lesions induced by radiation — A unified repair model. Radiat. Res. 106, 252–270. doi: 10.2307/3576798
Davis, C. K., and Vemuganti, R. (2021). DNA damage and repair following traumatic brain injury. Neurobiol. Dis. 147:105143. doi: 10.1016/j.nbd.2020.105143
Davis, C. M., Allen, A. R., and Bowles, D. E. (2021). Consequences of space radiation on the brain and cardiovascular system. J. Environ. Sci Health C Toxicol. Carcinog. 39, 180–218. doi: 10.1080/26896583.2021.1891825
Davis, C. M., Roma, P. G., and Hienz, R. D. (2016). The rodent Psychomotor Vigilance Test (rPVT): A method for assessing neurobehavioral performance in rats and mice. J. Vis. Exp. 118:54629 doi: 10.3791/54629
Davis, C. M., Roma, P. G., Armour, E., Gooden, V. L., Brady, J. V., Weed, M. R., et al. (2014). Effects of X-ray radiation on complex visual discrimination learning and social recognition memory in rats. PLoS One 9:e104393. doi: 10.1371/journal.pone.0104393
de Brouwer, G., Fick, A., Harvey, B. H., and Wolmarans, D. W. (2019). A critical inquiry into marble-burying as a preclinical screening paradigm of relevance for anxiety and obsessive-compulsive disorder: Mapping the way forward. Cogn. Affect. Behav. Neurosci. 19, 1–39. doi: 10.3758/s13415-018-00653-4
DeCarolis, N. A., Rivera, P. D., Ahn, F., Amaral, W. Z., LeBlanc, J. A., Malhotra, S., et al. (2014). (56)Fe particle exposure results in a long-lasting increase in a cellular index of genomic instability and transiently suppresses adult hippocampal neurogenesis in vivo. Life Sci. Space Res. 2, 70–79. doi: 10.1016/j.lssr.2014.06.004
Delotterie, D. F., Mathis, C., Cassel, J.-C., Rosenbrock, H., Dorner-Ciossek, C., and Marti, A. (2015). Touchscreen tasks in mice to demonstrate differences between hippocampal and striatal functions. Neurobiol. Learn. Mem. 120, 16–27. doi: 10.1016/j.nlm.2015.02.007
Drummond, G. (2020). A world beyond P: policies, strategies, tactics and advice. Exp. Physiol. 105, 13–16. doi: 10.1113/EP088040
Dumont, J. R., Salewski, R., and Beraldo, F. (2020). Critical mass: The rise of a touchscreen technology community for rodent cognitive testing. Genes Brain Behav. 20:e12650. doi: 10.1111/gbb.12650
Durante, M. (2014). Space radiation protection: destination mars. Life Sci. Space Res. 1, 2–9. doi: 10.1016/j.lssr.2014.01.002
Fernando, A. B. P., Murray, J. E., and Milton, A. L. (2013). The amygdala: securing pleasure and avoiding pain. Front. Behav. Neurosci. 7:190. doi: 10.3389/fnbeh.2013.00190
Fineberg, N. A., Potenza, M. N., Chamberlain, S. R., Berlin, H. A., Menzies, L., Bechara, A., et al. (2010). Probing compulsive and impulsive behaviors, from animal models to endophenotypes: a narrative review. Neuropsychopharmacology 35, 591–604. doi: 10.1038/npp.2009.185
Graf, R., Longo, J. L., and Hughes, Z. A. (2018). The location discrimination reversal task in mice is sensitive to deficits in performance caused by aging, pharmacological and other challenges. J. Psychopharmacol. 32, 1027–1036. doi: 10.1177/0269881118779383
Hadley, M. M., Davis, L. K., Jewell, J. S., Miller, V. D., and Britten, R. A. (2015). Exposure to mission-relevant doses of 1 GeV/n (48)Ti particles impairs attentional set-shifting performance in retired breeder rats. Radiat. Res. 185, 13–19. doi: 10.1667/RR14086.1
Halsey, L. G., Curran-Everett, D., Vowler, S. L., and Drummond, G. B. (2015). The fickle P value generates irreproducible results. Nat. Methods 12, 179–185. doi: 10.1038/nmeth.3288
Heindel, W. C., Salmon, D. P., Shults, C. W., Walicke, P. A., and Butters, N. (1989). Neuropsychological evidence for multiple implicit memory systems: a comparison of Alzheimer’s, Huntington’s, and Parkinson’s disease patients. J. Neurosci. 9, 582–587. doi: 10.1523/JNEUROSCI.09-02-00582.1989
Held, K. D., Blakely, E. A., Story, M. D., and Lowenstein, D. I. (2016). Use of the NASA space radiation laboratory at brookhaven national laboratory to conduct charged particle radiobiology studies relevant to ion therapy. Radiat. Res. 185, 563–567. doi: 10.1667/RR14412.1
Hellweg, C. E., and Baumstark-Khan, C. (2007). Getting ready for the manned mission to Mars: the astronauts’ risk from space radiation. Naturwissenschaften 94, 517–526. doi: 10.1007/s00114-006-0204-0
Hellweg, C. E., Arenz, A., and Baumstark-Khan, C. (2007). Assessment of space environmental factors by cytotoxicity bioassays. Acta Astronaut. 60, 525–533. doi: 10.1016/j.actaastro.2006.09.017
Hinkle, J. J., Olschowka, J. A., Love, T. M., Williams, J. P., and O’Banion, M. K. (2019). Cranial irradiation mediated spine loss is sex-specific and complement receptor-3 dependent in male mice. Sci. Rep. 9:18899. doi: 10.1038/s41598-019-55366-6
Holden, S., Perez, R., Hall, R., Fallgren, C. M., Ponnaiya, B., Garty, G., et al. (2021). Effects of acute and chronic exposure to a mixed field of neutrons and photons and single or fractionated simulated galactic cosmic ray exposure on behavioral and cognitive performance in mice. Radiat. Res. 196, 31–39. doi: 10.1667/RADE-20-00228.1
Horner, A. E., Heath, C. J., Hvoslef-Eide, M., Kent, B. A., Kim, C. H., Nilsson, S. R. O., et al. (2013). The touchscreen operant platform for testing learning and memory in rats and mice. Nat. Protoc. 8, 1961–1984. doi: 10.1038/nprot.2013.122
Hvoslef-Eide, M., Nilsson, S. R. O., Saksida, L. M., and Bussey, T. J. (2016). Cognitive translation using the rodent touchscreen testing approach. Curr. Top. Behav. Neurosci. 28, 423–447. doi: 10.1007/7854_2015_5007
Impey, S., Jopson, T., Pelz, C., Tafessu, A., Fareh, F., Zuloaga, D., et al. (2016). Short- and long-term effects of 56Fe irradiation on cognition and hippocampal DNA methylation and gene expression. BMC Genomics 17:825. doi: 10.1186/s12864-016-3110-7
Jandial, R., Hoshide, R., Waters, J. D., and Limoli, C. L. (2018). Space-brain: The negative effects of space exposure on the central nervous system. Surg. Neurol. Int. 9:9. doi: 10.4103/sni.sni_250_17
Jewell, J. S., Duncan, V. D., Fesshaye, A., Tondin, A., Macadat, E., and Britten, R. A. (2018). Exposure to ≤15 cGy of 600 MeV/n 56Fe particles impairs rule acquisition but not long-term memory in the attentional set-shifting assay. Radiat. Res. 190, 565–575. doi: 10.1667/RR15085.1
Joseph, J. A., Hunt, W. A., Rabin, B. M., and Dalton, T. K. (1992). Possible “Accelerated Striatal Aging” induced by heavy-particle irradiation: implications for manned space flights. Radiat. Res. 130, 88–93. doi: 10.2307/3578484
Joseph, J. A., Hunt, W. A., Rabin, B. M., Dalton, T. K., and Harris, A. H. (1993). Deficits in the sensitivity of striatal muscarinic receptors induced by heavy-particle irradiation: Further “Age-Radiation” Parallels. Radiat. Res. 135, 257–261. doi: 10.2307/3578303
Joseph, J. A., Villalobos-Molina, R., Rabin, B. M., Dalton, T. K., Harris, A., and Kandasamy, S. (1994). Reductions of 56Fe heavy-particle irradiation-induced deficits in striatal muscarinic receptor sensitivity by selective cross-activation/inhibition of second-messenger systems. Radiat. Res. 139, 60–66. doi: 10.2307/3578733
Kangas, B. D., and Bergman, J. (2017). Touchscreen technology in the study of cognition-related behavior. Behav. Pharmacol. 28, 623–629. doi: 10.1097/FBP.0000000000000356
Kiffer, F., Boerma, M., and Allen, A. (2019). Behavioral effects of space radiation: A comprehensive review of animal studies. Life Sci. Space Res. 21, 1–21. doi: 10.1016/j.lssr.2019.02.004
Kiffer, F., Carr, H., Groves, T., Anderson, J. E., Alexander, T., Wang, J., et al. (2018). Effects of 1H + 16O charged particle irradiation on short-term memory and hippocampal physiology in a murine model. Radiat. Res. 189, 53–63. doi: 10.1667/RR14843.1
Kokhan, V. S., Matveeva, M. I., Mukhametov, A., and Shtemberg, A. S. (2016). Risk of defeats in the central nervous system during deep space missions. Neurosci. Biobehav. Rev. 71, 621–632. doi: 10.1016/j.neubiorev.2016.10.006
Krishnan, B., Natarajan, C., Bourne, K. Z., Alikhani, L., Wang, J., Sowa, A., et al. (2021). Chronic low dose neutron exposure results in altered neurotransmission properties of the hippocampus-prefrontal cortex axis in both mice and rats. Int. J. Mol. Sci. 22:3668. doi: 10.3390/ijms22073668
Krukowski, K., Grue, K., Frias, E. S., Pietrykowski, J., Jones, T., Nelson, G., et al. (2018). Female mice are protected from space radiation-induced maladaptive responses. Brain Behav. Immun. 74, 106–120. doi: 10.1016/j.bbi.2018.08.008
Lakens, D. (2013). Calculating and reporting effect sizes to facilitate cumulative science: a practical primer for t-tests and ANOVAs. Front. Psychol. 4:863. doi: 10.3389/fpsyg.2013.00863
Leith, J. T., McDonald, M., Powers-Risius, P., Bliven, S. F., and Howard, J. (1982). Response of rat spinal cord to single and fractionated doses of accelerated heavy ions. Radiat. Res. 89, 176–193. doi: 10.2307/3575694
Lezak, K. R., Missig, G., and Carlezon, W. A. Jr. (2017). Behavioral methods to study anxiety in rodents. Dialogues Clin. Neurosci. 19, 181–191. doi: 10.31887/DCNS.2017.19.2/wcarlezon
Limoli, C. (2020). Can a comparison of clinical and deep space irradiation scenarios shed light on the radiation response of the brain? Br. J. Radiol. 93:20200245. doi: 10.1259/bjr.20200245
Lipton, D. M., Gonzales, B. J., and Citri, A. (2019). Dorsal striatal circuits for habits, compulsions and addictions. Front. Syst. Neurosci. 13:28. doi: 10.3389/fnsys.2019.00028
Liu, B., Hinshaw, R. G., Le, K. X., Park, M.-A., Wang, S., Belanger, A. P., et al. (2019). Space-like 56Fe irradiation manifests mild, early sex-specific behavioral and neuropathological changes in wildtype and Alzheimer’s-like transgenic mice. Sci. Rep. 9:12118. doi: 10.1038/s41598-019-48615-1
Makin, T. R., and de Xivry, J.-J. O. (2019). Science Forum: Ten common statistical mistakes to watch out for when writing or reviewing a manuscript. Elife 8:e48175. doi: 10.7554/eLife.48175.005
Mallien, A. S., Palme, R., Richetto, J., Muzzillo, C., Richter, S. H., Vogt, M. A., et al. (2016). Daily exposure to a touchscreen-paradigm and associated food restriction evokes an increase in adrenocortical and neural activity in mice. Horm. Behav. 81, 97–105. doi: 10.1016/j.yhbeh.2016.03.009
Mao, X.-W., Stanbouly, S., Jones, T., and Nelson, G. (2021). Evaluating ocular response in the retina and optic nerve head after single and fractionated high-energy protons. Life 11:849. doi: 10.3390/life11080849
Mar, A. C., Horner, A. E., Nilsson, S. R. O., Alsiö, J., Kent, B. A., Kim, C. H., et al. (2013). The touchscreen operant platform for assessing executive function in rats and mice. Nat. Protoc. 8, 1985–2005. doi: 10.1038/nprot.2013.123
Mark, S., Scott, G. B. I., Donoviel, D. B., Leveton, L. B., Mahoney, E., Charles, J. B., et al. (2014). The impact of sex and gender on adaptation to space: executive summary. J. Womens. Health 23, 941–947. doi: 10.1089/jwh.2014.4914
McTighe, S. M., Mar, A. C., Romberg, C., Bussey, T. J., and Saksida, L. M. (2009). A new touchscreen test of pattern separation: effect of hippocampal lesions. Neuroreport 20, 881–885. doi: 10.1097/WNR.0b013e32832c5eb2
Miry, O., Zhang, X.-L., Vose, L. R., Gopaul, K. R., Subah, G., Moncaster, J. A., et al. (2021). Life-long brain compensatory responses to galactic cosmic radiation exposure. Sci. Rep. 11, 1–14. doi: 10.1038/s41598-021-83447-y
Moore, T. M., Basner, M., Nasrini, J., Hermosillo, E., Kabadi, S., Roalf, D. R., et al. (2017). Validation of the cognition test battery for spaceflight in a sample of highly educated adults. Aerosp. Med. Hum. Perform 88, 937–946. doi: 10.3357/AMHP.4801.2017
Nadel, L. (1992). Multiple memory systems: what and why. J. Cogn. Neurosci. 4, 179–188. doi: 10.1162/jocn.1992.4.3.179
National Academies of Sciences, Engineering, and Medicine. (2018). Review of NASA’s Evidence Reports on Human Health Risks: 2017 Letter Report. Washington, DC: National Academies Press.
National Research Council, Division on Engineering and Physical Sciences, Aeronautics and Space Engineering Board, and Committee on the Evaluation of Radiation Shielding for Space Exploration. (2008). Managing Space Radiation Risk in the New Era of Space Exploration. Washington, DC: National Academies Press.
Nelson, G. A. (2016). Space radiation and human exposures, a primer. Radiat. Res. 185, 349–358. doi: 10.1667/RR14311.1
Nelson, G. A., Simonsen, L., and Huff, J. L. (2016). NASA Technical Reports Server (NTRS). Available Online at: https://ntrs.nasa.gov/search.jsp?R=20160004368 (accessed June 2, 2021).
Oomen, C. A., Hvoslef-Eide, M., Heath, C. J., Mar, A. C., Horner, A. E., Bussey, T. J., et al. (2013). The touchscreen operant platform for testing working memory and pattern separation in rats and mice. Nat. Protoc. 8, 2006–2021. doi: 10.1038/nprot.2013.124
Parihar, V. K., Angulo, M. C., Allen, B. D., Syage, A., Usmani, M. T., Passerat de la Chapelle, E., et al. (2020). Sex-specific cognitive deficits following space radiation exposure. Front. Behav. Neurosci. 14:535885. doi: 10.3389/fnbeh.2020.535885
Parihar, V. K., Maroso, M., Syage, A., Allen, B. D., Angulo, M. C., Soltesz, I., et al. (2018). Persistent nature of alterations in cognition and neuronal circuit excitability after exposure to simulated cosmic radiation in mice. Exp. Neurol. 305, 44–55. doi: 10.1016/j.expneurol.2018.03.009
Patel, Z. S., Brunstetter, T. J., Tarver, W. J., Whitmire, A. M., Zwart, S. R., Smith, S. M., et al. (2020). Red risks for a journey to the red planet: The highest priority human health risks for a mission to Mars. NPJ Microgravity 6:33. doi: 10.1038/s41526-020-00124-6
Percie du Sert, N., Hurst, V., Ahluwalia, A., Alam, S., Avey, M. T., Baker, M., et al. (2020). The ARRIVE guidelines 2.0: updated guidelines for reporting animal research. J. Physiol. 598, 3793–3801. doi: 10.1113/JP280389
Poldrack, R. A., and Packard, M. G. (2003). Competition among multiple memory systems: converging evidence from animal and human brain studies. Neuropsychologia 41, 245–251. doi: 10.1016/S0028-3932(02)00157-4
Raber, J., Yamazaki, J., Torres, E. R. S., Kirchoff, N., Stagaman, K., Sharpton, T., et al. (2019). Combined effects of three high-energy charged particle beams important for space flight on brain, behavioral and cognitive endpoints in B6D2F1 female and male mice. Front. Physiol. 10:179. doi: 10.3389/fphys.2019.00179
Rabin, B. (2012). An introduction to behavior testing for the radiobiologist. Three Health Risks Extraterr. Environ. 11, 1–11.
Rabin, B. M., Buhler, L. L., Joseph, J. A., Shukitt-Hale, B., and Jenkins, D. G. (2002). Effects of exposure to 56Fe particles or protons on fixed-ratio operant responding in rats. J. Radiat. Res. 43, S225–S228. doi: 10.1269/jrr.43.S225
Rabin, B. M., Carrihill-Knoll, K. L., and Shukitt-Hale, B. (2011). Operant responding following exposure to HZE particles and its relationship to particle energy and linear energy transfer. Adv. Space Res. 48, 370–377. doi: 10.1016/j.asr.2011.03.008
Rabin, B. M., Carrihill-Knoll, K. L., and Shukitt-Hale, B. (2015a). Comparison of the effectiveness of exposure to low-LET HELIUM PARTICles ((4)He) and Gamma Rays ((137)Cs) on the disruption of cognitive performance. Radiat. Res. 184, 266–272. doi: 10.1667/RR14001.1
Rabin, B. M., Carrihill-Knoll, K. L., Carey, A., Shukitt-Hale, B., and Joseph, J. A. (2005a). Effect of diet on the disruption of operant responding at different ages following exposure to 56Fe particles. Age 27, 69–73. doi: 10.1007/s11357-005-4000-0
Rabin, B. M., Carrihill-Knoll, K. L., Long, L. V., Pitts, S. C., and Hale, B. S. (2013). Effects of 17β-estradiol on cognitive performance of ovariectomized female rats exposed to space radiation. J. Behav. Brain Sci. 03, 67–73. doi: 10.4236/jbbs.2013.31007
Rabin, B. M., Carrihill-Knoll, K. L., Miller, M. G., and Shukitt-Hale, B. (2018). Age as a factor in the responsiveness of the organism to the disruption of cognitive performance by exposure to HZE particles differing in linear energy transfer. Life Sci. Space Res. 16, 84–92. doi: 10.1016/j.lssr.2017.12.001
Rabin, B. M., Heroux, N. A., Shukitt-Hale, B., Carrihill-Knoll, K. L., Beck, Z., and Baxter, C. (2015b). Lack of reliability in the disruption of cognitive performance following exposure to protons. Radiat. Environ. Biophys. 54, 285–295. doi: 10.1007/s00411-015-0597-2
Rabin, B. M., Joseph, J. A., and Shukitt-Hale, B. (2004). Heavy particle irradiation, neurochemistry and behavior: thresholds, dose–response curves and recovery of function. Adv. Space Res. 33, 1330–1333. doi: 10.1016/j.asr.2003.09.051
Rabin, B. M., Joseph, J. A., and Shukitt-Hale, B. (2005b). A longitudinal study of operant responding in ratsirradiated when 2 months old. Radiat. Res. 164, 552–555. doi: 10.1667/RR3349.1
Rabin, B. M., Joseph, J. A., and Shukitt-Hale, B. (2005c). Effects of age and diet on the heavy particle-induced disruption of operant responding produced by a ground-based model for exposure to cosmic rays. Brain Res. 1036, 122–129. doi: 10.1016/j.brainres.2004.12.041
Rabin, B. M., Joseph, J. A., Shukitt-Hale, B., and Carrihill-Knoll, K. L. (2012). Interaction between age of irradiation and age of testing in the disruption of operant performance using a ground-based model for exposure to cosmic rays. Age 34, 121–131. doi: 10.1007/s11357-011-9226-4
Rabin, B. M., Miller, M. G., Larsen, A., Spadafora, C., Zolnerowich, N. N., Dell’Acqua, L. A., et al. (2019a). Effects of exposure to 12C and 4He particles on cognitive performance of intact and ovariectomized female rats. Life Sci. Space Res. 22, 47–54. doi: 10.1016/j.lssr.2019.07.005
Rabin, B. M., Poulose, S. M., Bielinski, D. F., and Shukitt-Hale, B. (2019b). Effects of head-only or whole-body exposure to very low doses of 4He (1000 MeV/n) particles on neuronal function and cognitive performance. Life Sci. Space Res. 20, 85–92. doi: 10.1016/j.lssr.2019.02.001
Rabin, B. M., Shukitt-Hale, B., and Carrihill-Knoll, K. L. (2014a). Effects of age on the disruption of cognitive performance by exposure to space radiation. J. Behav. Brain Sci. 04, 297–307. doi: 10.4236/jbbs.2014.47031
Rabin, B. M., Shukitt-Hale, B., Carrihill-Knoll, K. L., and Gomes, S. M. (2014b). Comparison of the effects of partial- or whole-body exposures to 16O particles on cognitive performance in rats. Radiat. Res. 181, 251–257. doi: 10.1667/RR13469.1
Rabin, B. M., Shukitt-Hale, B., Joseph, J., and Todd, P. (2007b). Diet as a factor in behavioral radiation protection following exposure to heavy particles. Gravit. Space Biol. Bull. 18, 71–77.
Rabin, B. M., Shukitt-Hale, B., Joseph, J. A., Carrihill-Knoll, K. L., Carey, A. N., and Cheng, V. (2007a). Relative effectiveness of different particles and energies in disrupting behavioral performance. Radiat. Environ. Biophys. 46, 173–177. doi: 10.1007/s00411-006-0071-2
Rivera, P. D., Shih, H.-Y., Leblanc, J. A., Cole, M. G., Amaral, W. Z., Mukherjee, S., et al. (2013). Acute and fractionated exposure to high-LET (56)Fe HZE-particle radiation both result in similar long-term deficits in adult hippocampal neurogenesis. Radiat. Res. 180, 658–667. doi: 10.1667/RR13480.1
Robbins, M., Greene-Schloesser, D., Peiffer, A., Shaw, E., Chan, M., and Wheeler, K. (2012). Radiation-induced brain injury: A review. Front. Oncol. 2:73. doi: 10.3389/fonc.2012.00073
Schimmerling, W., Cucinotta, F. A., and Wilson, J. W. (2003). Radiation risk and human space exploration. Adv. Space Res. 31, 27–34. doi: 10.1016/s0273-1177(02)00653-1
Setlow, R. B. (2003). The hazards of space travel. EMBO Rep. 4, 1013–1016. doi: 10.1038/sj.embor.embor7400016
Shukitt-Hale, B., Carey, A. N., Jenkins, D., Rabin, B. M., and Joseph, J. A. (2007). Beneficial effects of fruit extracts on neuronal function and behavior in a rodent model of accelerated aging. Neurobiol. Aging 28, 1187–1194. doi: 10.1016/j.neurobiolaging.2006.05.031
Simonsen, L. C., Slaba, T. C., Guida, P., and Rusek, A. (2020). NASA’s first ground-based galactic cosmic ray simulator: enabling a new era in space radiobiology research. PLoS Biol. 18:e3000669. doi: 10.1371/journal.pbio.3000669
Spillantini, P., Casolino, M., Durante, M., Mueller-Mellin, R., Reitz, G., Rossi, L., et al. (2007). Shielding from cosmic radiation for interplanetary missions: Active and passive methods. Radiat. Meas. 42, 14–23. doi: 10.1016/j.radmeas.2006.04.028
Sullivan, J. A., Dumont, J. R., Memar, S., Skirzewski, M., Wan, J., Mofrad, M. H., et al. (2021). New frontiers in translational research: Touchscreens, open science, and the mouse translational research accelerator platform. Genes Brain Behav. 20:e12705. doi: 10.1111/gbb.12705
Swan, A. A., Clutton, J. E., Chary, P. K., Cook, S. G., Liu, G. G., and Drew, M. R. (2014). Characterization of the role of adult neurogenesis in touch-screen discrimination learning. Hippocampus 24, 1581–1591. doi: 10.1002/hipo.22337
Thames, H. D. (1985). An “Incomplete-repair” model for survival after fractionated and continuous irradiations. Int. J. Radiat. Biol. Relat. Stud. Phys. Chem. Med. 47, 319–339. doi: 10.1080/09553008514550461
Thomas, A., Burant, A., Bui, N., Graham, D., Yuva-Paylor, L. A., and Paylor, R. (2009). Marble burying reflects a repetitive and perseverative behavior more than novelty-induced anxiety. Psychopharmacology 204, 361–373. doi: 10.1007/s00213-009-1466-y
Tran, F. H., Spears, S. L., Ahn, K. J., Eisch, A. J., and Yun, S. (2020). Does chronic systemic injection of the DREADD agonists clozapine-N-oxide or Compound 21 change behavior relevant to locomotion, exploration, anxiety, and depression in male non-DREADD-expressing mice? Neurosci. Lett. 739:135432. doi: 10.1016/j.neulet.2020.135432
Tranel, D., Damasio, A. R., Damasio, H., and Brandt, J. P. (1994). Sensorimotor skill learning in amnesia: additional evidence for the neural basis of nondeclarative memory. Learn. Mem. 1, 165–179.
Vazquez, M. E. (1998). Neurobiological problems in long-term deep space flights. Adv. Space Res. 22, 171–183. doi: 10.1016/S0273-1177(98)80009-4
Villasana, L., Acevedo, S., Poage, C., and Raber, J. (2006). Sex- and APOE isoform-dependent effects of radiation on cognitive function. Radiat. Res. 166, 883–891. doi: 10.1667/RR0642.1
Villasana, L., Rosenberg, J., and Raber, J. (2010). Sex-dependent effects of 56Fe irradiation on contextual fear conditioning in C57BL/6J mice. Hippocampus 20, 19–23. doi: 10.1002/hipo.20659
Vivar, C., Potter, M. C., Choi, J., Lee, J.-Y., Stringer, T. P., Callaway, E. M., et al. (2012). Monosynaptic inputs to new neurons in the dentate gyrus. Nat. Commun. 3:1107. doi: 10.1038/ncomms2101
Wasserstein, R. L., Schirm, A. L., and Lazar, N. A. (2019). Moving to a World Beyond “p < 0.05.”. Am. Stat. 73, 1–19. doi: 10.1080/00031305.2019.1583913
Watson, L. A., and Tsai, L.-H. (2017). In the loop: how chromatin topology links genome structure to function in mechanisms underlying learning and memory. Curr. Opin. Neurobiol. 43, 48–55. doi: 10.1016/j.conb.2016.12.002
Whoolery, C. W., Walker, A. K., Richardson, D. R., Lucero, M. J., Reynolds, R. P., Beddow, D. H., et al. (2017). Whole-body exposure to 28Si-radiation dose-dependently disrupts dentate gyrus neurogenesis and proliferation in the short term and new neuron survival and contextual fear conditioning in the long term. Radiat. Res. 188, 532–551. doi: 10.1667/RR14797.1
Whoolery, C. W., Yun, S., Reynolds, R. P., Lucero, M. J., Soler, I., Tran, F. H., et al. (2020). Multi-domain cognitive assessment of male mice shows space radiation is not harmful to high-level cognition and actually improves pattern separation. Sci. Rep. 10:2737. doi: 10.1038/s41598-020-59419-z
Willey, J. S., Britten, R. A., Blaber, E., Tahimic, C. G. T., Chancellor, J., Mortreux, M., et al. (2021). The individual and combined effects of spaceflight radiation and microgravity on biologic systems and functional outcomes. J. Environ. Sci. Health C Toxicol. Carcinog. 39, 129–179. doi: 10.1080/26896583.2021.1885283
Yun, S., Reynolds, R. P., Petrof, I., White, A., and Rivera, P. D. (2018). Stimulation of entorhinal cortex–dentate gyrus circuitry is antidepressive. Nat. Med. 24, 658–666. doi: 10.1038/s41591-018-0002-1
Keywords: dentate gyrus, prefrontal cortex, striatum, hippocampus, behavioral pattern separation, rodent touchscreen, galactic cosmic radiation, HZE particle fractionation
Citation: Soler I, Yun S, Reynolds RP, Whoolery CW, Tran FH, Kumar PL, Rong Y, DeSalle MJ, Gibson AD, Stowe AM, Kiffer FC and Eisch AJ (2021) Multi-Domain Touchscreen-Based Cognitive Assessment of C57BL/6J Female Mice Shows Whole-Body Exposure to 56Fe Particle Space Radiation in Maturity Improves Discrimination Learning Yet Impairs Stimulus-Response Rule-Based Habit Learning. Front. Behav. Neurosci. 15:722780. doi: 10.3389/fnbeh.2021.722780
Received: 09 June 2021; Accepted: 08 September 2021;
Published: 11 October 2021.
Edited by:
Francis A. Cucinotta, University of Nevada, Las Vegas, United StatesReviewed by:
Jacob Raber, Oregon Health and Science University, United StatesRichard Britten, Eastern Virginia Medical School, United States
Copyright © 2021 Soler, Yun, Reynolds, Whoolery, Tran, Kumar, Rong, DeSalle, Gibson, Stowe, Kiffer and Eisch. This is an open-access article distributed under the terms of the Creative Commons Attribution License (CC BY). The use, distribution or reproduction in other forums is permitted, provided the original author(s) and the copyright owner(s) are credited and that the original publication in this journal is cited, in accordance with accepted academic practice. No use, distribution or reproduction is permitted which does not comply with these terms.
*Correspondence: Sanghee Yun, eXVuc0BjaG9wLmVkdQ==; Amelia J. Eisch, ZWlzY2hAdXBlbm4uZWR1
†These authors have contributed equally to this work and share first authorship
‡Present address: Ivan Soler, Nash Family Department of Neuroscience, Friedman Brain; Institute, Graduate School of Biomedical Sciences, Icahn School of Medicine at Mount Sinai, New York, NY, United States; Ryan P. Reynolds, Center for Hypothalamic Research, UT Southwestern Medical Center, Dallas, TX, United States; Ann M. Stowe, Department of Neurology, University of Kentucky, Lexington, KY, United States
§These authors have contributed equally to this work and share second authorship