- 1Center for Neuroscience Research, Children’s National Hospital, Washington, DC, United States
- 2Center for Genomic Medicine, Children’s National Hospital, Washington, DC, United States
- 3Center for Cancer and Immunology Research, Children’s National Hospital, Washington, DC, United States
In humans, mutations in the transcription factor encoding gene, FOXP2, are associated with language and Autism Spectrum Disorders (ASD), the latter characterized by deficits in social interactions. However, little is known regarding the function of Foxp2 in male or female social behavior. Our previous studies in mice revealed high expression of Foxp2 within the medial subnucleus of the amygdala (MeA), a limbic brain region highly implicated in innate social behaviors such as mating, aggression, and parental care. Here, using a comprehensive panel of behavioral tests in male and female Foxp2+/– heterozygous mice, we investigated the role Foxp2 plays in MeA-linked innate social behaviors. We reveal significant deficits in olfactory processing, social interaction, mating, aggressive, and parental behaviors. Interestingly, some of these deficits are displayed in a sex-specific manner. To examine the consequences of Foxp2 loss of function specifically in the MeA, we conducted a proteomic analysis of microdissected MeA tissue. This analyses revealed putative sex differences expression of a host of proteins implicated in neuronal communication, connectivity, and dopamine signaling. Consistent with this, we discovered that MeA Foxp2-lineage cells were responsive to dopamine with differences between males and females. Thus, our findings reveal a central and sex-specific role for Foxp2 in social behavior and MeA function.
Introduction
In humans, the function of the transcription factor encoding gene, FOXP2, was first primarily associated with speech, language production, and vocal communication. Several different mutations in FOXP2 result in difficulty in coordinating speech-related movements (developmental verbal dyspraxia, dysarthria, and/or apraxia of speech), as well as deficits in receptive and expressive verbal abilities (dysphasia), first described in the KE family (Vargha-Khadem et al., 1998; Lai et al., 2001; MacDermot et al., 2005; Feuk et al., 2006; Shriberg et al., 2006; Zeesman et al., 2006; Fisher and Scharff, 2009; Morgan et al., 2017). Based on these findings, FOXP2 was initially dubbed “the language gene.” These findings prompted a myriad of investigations in mouse models into the function of Foxp2, as well as in other species including songbirds, bats, and non-human primates. The majority of these studies focused on sensory and motor-related brain regions implicated in language/verbal communication such as the basal ganglia (caudate nucleus and striatum), cerebellum, and the cerebral cortex, all regions with high expression of Foxp2 (Haesler et al., 2004; Shu et al., 2005; Vargha-Khadem et al., 2005; Li et al., 2007; Fujita et al., 2008; Groszer et al., 2008; Enard et al., 2009; Konopka et al., 2009, 2012; Gaub et al., 2010; Hilliard et al., 2012; Castellucci et al., 2016).
In addition to these studies, another line of investigation has unveiled interesting correlations between FOXP2 mutations and social impairment. Large chromosome mutations comprising the FOXP2 region or polymorphisms in the FOXP2 gene have been associated with social deficits and autistic phenotypes; some of them concomitant with language deficits (Gong et al., 2004; Li et al., 2005; Marui et al., 2005; Feuk et al., 2006; Laroche et al., 2008; Konopka, 2013; Medvedeva, 2015; Chien et al., 2017; Morgan et al., 2017; and see DECIPHER and SFARI databases). The exact role of FOXP2 in Autism Spectrum Disorders (ASD) remains to be fully determined, as several rounds of large scale genome-wide sequencing studies showed no association with ASD (Newbury et al., 2002; Gauthier et al., 2003; Kumar, 2008; Marshall et al., 2008; Weiss et al., 2008; Wang et al., 2009; Abrahams and Geschwind, 2010; Anney et al., 2010; Krishnan et al., 2016). However, more recent studies, most notably the large-scale sequencing effort by Satterstrom et al. (2020) have uncovered a correlation between FOXP2 mutations and ASD (Lim et al., 2017; Guo et al., 2019; Munnich et al., 2019; Satterstrom et al., 2020). Although more and larger sequencing studies will be informative, based on the current evidence, the Simons Foundation Autism Research Initiative (SFARI GENE) ranks FOXP2 as a category 1, “high confidence” ASD gene (following the highest confidence category of “Syndromic”). Consistent with the role of Foxp2 in social behavior, studies in mouse models also reported diminished social function in several Foxp2 mutants. For instance, heterozygous Foxp2+/– mice were less frequently in group contact in the modified Hole Board test (Shu et al., 2005; Enard et al., 2009). Also, cortical-specific Foxp2 conditional mutant mice showed deficits in approach towards conspecifics (Medvedeva, 2015; Medvedeva et al., 2019).
Despite the above described extensive studies of Foxp2 in different brain areas mainly involved in language, studies of Foxp2 function in the amygdala, a central brain region implicated in social behavior and communication, have been lacking. In the amygdala, Foxp2 is highly expressed in both the intercalated neurons (ITCs), which are associated with fear conditioning, and the medial subnucleus (MeA), which is essential for processing olfactory cues that trigger innate (unlearned) behaviors such as mating, aggression, parenting, and predator avoidance (Lischinsky et al., 2017; Kuerbitz et al., 2018; Lischinsky and Lin, 2020). Our previous studies using either immunohistochemistry to label Foxp2+ cells or Cre-based fate mapping to tag Foxp2+ cells and their descendants revealed that Foxp2+ and Foxp2-lineage neurons in the MeA are a molecular and electrophysiologically distinct subpopulation of inhibitory output neurons (Carney et al., 2010; Lischinsky et al., 2017; Matos et al., 2020). Our work further revealed that Foxp2+ and Foxp2-lineage neurons are activated by innate reproductive and aggressive behaviors in a sex-specific manner and display molecular and intrinsic electrophysiological differences between males and females (Lischinsky et al., 2017; Matos et al., 2020).
In addition to our findings, other studies have revealed sex differences in Foxp2 function in different brain regions, demonstrating that at least some of the roles of Foxp2 are sex-specific. For instance, in rats, the level of Foxp2 expression correlates with male and female differences in ultrasonic vocal communication by pups, leading to a dam’s preferential retrieval of male pups (Bowers et al., 2013). In addition, the male sex hormone androgen promotes Foxp2 expression in the striatum, cerebellar vermis, and cortex (Bowers et al., 2014).
Based on these findings, we explored the putative role Foxp2 plays in the modulation of MeA driven innate social and non-social behaviors in males and females by examining Foxp2+/– mice. We found sex differences in a subset of innate behaviors, including most strikingly opposite effects on aggressive behaviors in males and females. Correlating with these sex differences in behavioral alterations, we found putative male/female differences in alterations at the protein level in Foxp2+/– mice in the MeA. These include changes in proteins implicated in DA-signalling, which has previously been associated with MeA-driven motivational behaviors (Miller et al., 2019; Nordman and Li, 2020). We further found that MeA Foxp2-lineage cells are highly responsive to dopamine, also in a sex-specific manner. Thus, our study uncovers a previously unknown role for Foxp2 in regulating innate behaviors and potentially in motivational states in male and female mice; processes perhaps contributed via Foxp2 function in the MeA.
Materials and Methods
Animals and Housing
Mice were housed in the temperature and light-controlled (12 h light-dark cycle) animal care facility at Children’s National Hospital and given food and water ad libitum. All animal procedures were approved by the Children’s National Hospital Institutional Animal Care and Use Committee (IACUC) and conformed to NIH Guidelines for animal use. Foxp2+/– mutant mice used in this study were previously generated by replacing exons 12 and 13 with a neomycin cassette resulting in a complete loss of function in homozygous Foxp2−/− mice (Shu et al., 2005). As Foxp2−/− mice are early postnatal lethal, only Foxp2+/– mice were used here. Foxp2+/– mice were maintained on the C57BL/6J background. Foxp2+/– mice were generated from crossing to Foxp2+/– males to C57BL/6J females. Foxp2+/– mice are termed “mutant” (mt) with controls (ctr) wild-type littermates or C57BL/6J mice. Foxp2Cre mice (JAX labs Stock No: 030541; Rousso et al., 2016) and GCaMP5G-tdTm mice (Jax Labs Stock No: 024477; Gee et al., 2014) were obtained from Jackson Labs. Mice were genotyped by Transnetyx Inc. Genotyping Services. Mouse chow was standard diet (Teklad global rodent diets, #2918, 18% protein, Envigo, Indianapolis, IN, USA) and bedding was Corncob 1/4, #7097 (Envigo, Indianapolis, IN, USA).
Behavioral Experiments
Behavioral experiments were conducted using two to three separate cohorts of 3–4 month-old male and female Foxp2+/– mutants and control mice with a total n ≥ 10 animals per group (exact n’s noted in the Results and/or Figure Legends). For behavioral analyses, each cohort roughly included 3–5 pups/sex/genotype/litter from approximately 5 to 6 litters. Video tracking was used in the analysis of social behavior (Dold Labs and Engineering software) and the other behaviors tracked by visual observation and scoring behavioral data by an observer blind to the genotype of the animals. Experiments were carried out in the Children’s National DC-IDDRC (1U54HD090257) supported Animal Neurobehavioral Evaluation Core (ANEC).
Social Interaction Assay
Social behavior was evaluated using an automated 3-chamber device (Dold Labs and Engineering, Seguin, TX, USA), as previously described (McFarlane et al., 2008). The socialization chamber is composed of three partitions separated by retractable doorways. On test days, a single Foxp2+/– or ctr mouse was placed in the middle chamber and allowed to explore freely for 10 min. After 10 min, the mouse was given free access to the other two chambers for 10 min. After this exploratory period, an inverted empty wire cup (novel object) was placed in one of the chambers, and an unfamiliar (novel) C57BL/6J sex- and age-matched mouse (i.e., males with males and females with females) was placed inside an identical inverted wire cup in the other chamber. In order to control for the possibility of innate side preferences, the wire cups with and without the novel mouse were randomly placed on the left or right chambers, and the placement was changed between mice (Wang et al., 2015). Animal behaviors were video-recorded for 10 min and the total time the experimental mouse spent sniffing the novel mouse, the novel object, and the total time spent in each chamber were scored.
Olfactory Habituation and Discrimination Assay
Urine pooled from multiple female mice across the estrus cycle, pooled male urine, peanut butter, or water were swabbed onto a cotton swab. Each odor-swabbed cotton swab was presented to Foxp2+/– or ctr mice three times each for 2 min, with 2 min between each inter-trial interval. Active sniffing was scored each time the animal’s nose was oriented towards and less than 2 cm from the tip of the swab. The cumulative time spent investigating a particular odor during the 2 min trial was scored.
Mating Assay
Sexually naïve experimental Foxp2+/– and control males and females were singly housed for 1 week prior to testing. A sexually experienced C57BL/6J female or male, respectively, was placed into the test male or female home-cage. Each test trial was recorded for 30 min. Trials were repeated three times, separated by at least 1 week (total trials: 60 for males and 66 trials for females). The number, duration, and latency of the mating parameters of mounting, intromission, and ejaculation were scored using criteria previously described by us (Sokolowski et al., 2015).
Maternal Aggression Assay
Singly housed Foxp2+/– or control female mice were impregnated via cross to a C57BL/6J male and gave birth to a litter of pups. Tests were conducted when pups were between P3-P10. On the test day, pups were removed from the home-cage, and an intruder C57BL/6J male was introduced. Each trial consisted of 15 min of interaction. The time and number of nips (pinching with the mouth) and attacks were recorded and scored. Nips were defined as pinching with the mouth, without other motor components. Attacks were defined as the full repertoire of the motor components of aggression such as biting combined with clinching and tussling. The pups were returned to the home-cage after the intruder was removed. Trials were repeated two times, separated by at least 1 week.
Resident-Intruder Aggression Assay
Each resident Foxp2+/– or control male was singly housed and undisturbed for at least 1 week prior to testing. After this time, a smaller novel C57BL/6J male was introduced into the home-cage of the resident. Each trial was recorded for 10 min. The time and number of social explorations (moving directly toward conspecific, chasing, or sniffing) and attacks (fighting and clinching in an upright position) were scored.
Pup Retrieval Test
Foxp2+/– or control mice gave birth to a litter of pups. In each trial, the dam was temporarily removed from the cage, and the litter of P3-P10 pups was taken from the nest and placed in a far corner of the home-cage. The dam was returned to the cage, and the time required for her to retrieve the first and second pup back to the nest was scored. Trials were repeated two times, separated by at least 1 week.
Predator Avoidance Assay
The predator odor consisted of a plastic petri dish containing rat bedding (12g), introduced into the home-cage. The benign odor consisted of a petri dish containing clean unsoiled mouse bedding (12g). To habituate the mice to the dish, a novel object, Foxp2+/– and control mice were exposed to benign odor for 15 min. Then, the predator odor rat bedding in a petri dish was presented into the home-cage for 15 min. The escape behaviors (climbs) and chemoinvestigative behaviors (risk assessments, as defined by cautious investigation, and sniffs) were scored.
Proteomics: Relative Protein Quantitation Using TMT Isobaric Mass Tagging
Adult 3-month-old male and female Foxp2+/– and control mice were euthanized, and the MeA encompassing both the MePD and MePV (Bregma −1.58 to −2.06; Mouse Brain Atlas, 3rd edition; Franklin and Paxinos, 2008) was microdissected manually using Lumdsen microdissection scissors. The MeA is observed as a teardrop structure distinct from nearby amygdala nuclei. Care was taken to avoid dissecting nearby amygdala nuclei (CeA, CoA), notably avoiding Foxp2+ intercalated neurons that are found adjacent to the basolateral amygdala which is bounded by clearly demarcating white matter tracts. Dissected tissue was snap-frozen in liquid nitrogen and stored at −80°C until processed. MeA tissue from three animals (two amygdala/animal for a total of six) was pooled together and considered a single sample. A total of n = 2 samples were analyzed for each sex (male and female) and genotype (Foxp2+/– and control). MeA tissue samples were homogenized in 8 M urea and sonicated for 30 s. The resultant lysate was centrifuged at 16K rpm for 30 min at 4°C and cleared supernatant was collected. Protein concentration was determined by BCA assay (PierceTM, Thermo Scientific), and 100 μg of protein lysate was processed for each sample. The proteins were treated with TCEP and Iodoacetamide as described in TMT Isobaric Mass Tags labeling protocol (Thermo Scientific), extracted with methanol: chloroform, air dried, dissolved in 50 mM TEAB, and digested overnight with sequencing grade Trypsin (Thermo Scientific) at 37°C. The resulting peptides were labeled with TMT Label Reagent (Sixplex kit, Thermo Scientific), each with one specific tag (as per manufacturer’s protocol). Following labeling, the samples were pooled together, desalted, and fractionated to eight fractions using Pierce high-pH fractionation kit (Thermo Scientific). The peptide mixtures from each fraction were sequentially analyzed by LC-MS/MS using nano-LC system (Easy nLC1000) connected to Q Exactive HF mass spectrometer (Thermo Scientific). The platform is configured with nano-electrospray ion source (Easy-Spray, Thermo Scientific), Acclaim PepMap 100 C18 nanoViper trap column (3 μm particle size, 75 μm ID x 20 mm length), EASY-Spray C18 analytical column (2 μm particle size, 75 μm ID x 500 mm length). The peptides were eluted at a flow rate of 300 nl/min using linear gradients of 7–25% Acetonitrile (in aqueous phase and 0.1% Formic Acid) for 80 min, followed by 45% for 25 min, and static flow at 90% for 15 min. The mass spectrometry data was collected in a data-dependent manner switching between one full scan MS mode (m/z 380–1,400, resolution 60K, AGC 3e6, max ion time 20 ms), and 10 MS/MS scans (resolution 15K, AGC 1e5, max ion time 120 ms, nCE 32) of the top 10 target ions. The ions were sequenced once and dynamically excluded from the list for 30 s. The MS raw data sets were analyzed using Thermo Proteome Discoverer Software (version 2.3), and searched against the Mouse UniProt database using Sequest HT algorithm at precursor mass tolerance of 10 ppm and fragment mass tolerance of 0.02 Da. The peptides and proteins were filtered using a Percolator at a target FDR of 0.01 (thus at 99% confidence level). The relative quantitation of proteins was determined based on the intensities of reporter mass tags. We used open-source Perseus software for data filtration to exclude any false hits and to select only high confidence quantified proteins. Heatmap visualization of the dataset based on relative protein abundance ratio between the two groups of animals was done by Hierarchical clustering analyses using Euclidean distance, with maximum of 10 iterations. As we are using three animals in each group, the abundance represents the average of three animals. Proteins with similar abundance between the two independent groups of analyses were selected for downstream analyses. We note that in this study we performed global quantitative proteomics analyses only in two technical replicates, thus the results from this experiment should be viewed as a hypothesis-generating analysis rather than one that conclusively validates the differences in specific proteins.
Dopamine Activation of MeA Foxp2-Lineage Cells Ex vivo
Acute Brain Slice Preparation
Three- to 7-month-old male and female Foxp2cre;GCaMP5G-tdTM mice were euthanized, and brains rapidly removed and immersed in ice-cold cutting solution (230 mM sucrose, 2.5 mM KCl, 0.5 mM CaCl2, 10 mM MgCl2, 26 mM NaHCO3, 1.25 mM NaH2PO4, 0.04 mM Na-Ascorbate, and 10 mM glucose, pH 7.2–7.4). Coronal slices containing the MeA (Bregma −1.58 to −2.06) were cut with a vibratome (Leica VT1000S) at 300 μm and transferred to artificial cerebral spinal fluid (aCSF; 126 mM NaCl, 4 mM KCl, 2 mM CaCl2, 2 mM MgCl2, 26 mM NaHCO3, 1.25 mM NaH2PO4, 0.04 mM Na-Ascorbate, and 10 mM glucose, pH 7.2–7.4, osmolarity = 310 mOsm/l) bubbled with 95% O2 and 5% CO2. Slices were allowed to recover in oxygenated aCSF for 1 h at room temperature before acute slice imaging. During imaging, slices were placed in a perfusion chamber and superfused with oxygenated aCSF at 30° to 32°C for the duration of the experiment. The cells were visualized with a 20× immersion objective (Olympus Optical, New York, NY) and epifluorescence.
Ca2+ Imaging and Analysis
Two-Photon Ca2+ imaging was performed with an Olympus (Tokyo, Japan) FluoView FVMPE-RS Multiphoton Microscope imaging system using FluoView software and a Ti: Sapphire laser source emitting 140 fs pulses at an 80 MHz repetition rate with a wavelength adjustable for 690–1,040 nm (Maitai DeepSee pulsed, infrared laser). The full field of view was acquired with XY raster scanning using the 20× 0.95 NA water-immersion objective. Changes in fluorescence (ΔF) were quantified using ImageJ (NIH) software and expressed as a percentage of baseline (%ΔF/F). Time-lapse images of cellular Ca2+ signaling were recorded at a frame rate of 1 Hz. ROIs were selected based on the appearance of GCaMP5G Ca2+ transients in the time-lapse images. To trigger Ca2+ transients, dopamine (100 μM) was dissolved in aCSF and delivered locally by a pressure pulse (10 psi; 100–500 ms) using a Picospritzer III (Parker Instrumentation, Chicago, IL).
Statistical Analyses
Behavioral Experiments
Statistical analyses and graph plots were carried out using Microsoft Excel and GraphPad (Prism9). Data were represented by the mean ± S.E.M. (standard error of the mean). Significant differences were revealed by either unpaired t-test for 2-group mean comparisons or by ANOVA for multiple comparisons using Tukey’s post hoc test, after verifying normality and homoscedasticity by Shapiro–Wilk’s and Levene’s test, respectively.
Proteomics
Proteomics analysis was carried out in two samples per condition, i.e., per male and female Foxp2+/– mutants (mt) and controls (wild type siblings). Each sample represented pooled MeA from n = 3 animals. For analyzing differential protein expression in Foxp2+/– mutant vs. wild type siblings (ctr) in males and females, relative expression was assessed by generating the ratios: mtM1/ctrM1, mtM1/ctrM1 and mtF/mtM. Log2FC ≥ |1.11| change was considered significant. Proteins were clustered based on similarity of expression between the experimental and control groups, which is indicated by the dendrogram. The color key and histogram panel indicates the Z-scores of expression and the expression counts. The related pathways, biological processes, and ontology of the differentially expressed proteins were determined using Ingenuity Pathway Analysis software (IPA, Qiagen vs. 2020) and the Cytoscape (vs. 3.5.1) plug-in ClueGO (vs. 2.5.5). Heatmaps were generated using R (version 3.6.2) with the heatmap.2 function in the gplots package (version 3.1.1). Map colors used the RColorBrewer palette (version 1.1-2). Proteins were clustered based on similarity of expression between the experimental and control groups, indicated by the dendrogram. Highly expressed proteins are shown in red, while those with lower expression are shown in green. Differentially expressed proteins in the MeA of Foxp2+/– mutants were then compared with categories “Syndromic”, “1. High confidence”, “2. Strong candidate” and “3. Suggestive evidence” of the publicly available SFARI list reporting autism-susceptibility genes (version release 04-13-2020, by the Simons Foundation Autism Research Initiative), as well as with the largest exome sequencing study of ASD to date (Satterstrom et al., 2020). To visualize overlap between comparisons, Venn diagrams were created using an online tool (Oliveros, 2007).
Dopamine Activation
Activation of Foxp2-lineage cells by dopamine was investigated in at least two MeA slices from five Foxp2cre;GCaMP5G- tdTM per sex. All values are expressed as means ± S.E.M. Normality of the data was evaluated with Shapiro–Wilk test with p = 0.05. Differences between the two means were assessed by Student’s test (unpaired; Mann–Whitney post hoc test). Statistical significances (p* ≤ 0.05) were calculated using GraphPad Prism 6.0 (GraphPad Software, La Jolla, CA, USA).
Results
Alterations in Social and Non-social Innate Behaviors in Foxp2+/– Mice
Previous studies revealed that Foxp2 is expressed in areas of the brain, such as the amygdala, that regulate social behavior (Co et al., 2020). Therefore, we wanted to examine whether Foxp2+/– mutant mice display alterations in amygdala regulated social and non-social innate behaviors and if so, whether there are differences across males and females. To test this, we conducted the following tasks: the 3-chamber social test, olfactory habituation/dishabituation, mating/reproduction, female maternal and male territorial resident-intruder aggression, pup retrieval, and predator odor avoidance. As described below, we found robust deficits in a number of behavioral paradigms tested, a subset which also differed between males and females.
Three-Chamber Social Test
First, to determine whether Foxp2 is required for social discrimination, we used the 3-chambered social task, which is considered the gold standard behavioral assay to assess sociability (McFarlane et al., 2008; Wang et al., 2015; Kazdoba et al., 2016). We compared the preference of the test mouse (Foxp2+/– or C57BL/6J control) to a social object (male or female mouse) vs. a non-social object (wire cup). In this assay, control mice typically show a greater preference for engaging a novel mouse as opposed to a novel object. The test mouse was placed in the center chamber and allowed to explore freely. Placed in the other chambers were either a wire cup without a mouse and a wire cup with the same sex mouse. Our results showed that female Foxp2+/– mice spent less time in the presence of another mouse from the same sex, and instead spent more time in the presence of the object, indicative of deficits in sociability (Figure 1). In contrast, we observed no differences in sociability in male mice. Thus, in the 3-chamber tests, social behavior in Foxp2+/– mice was impaired in females, but not in males.
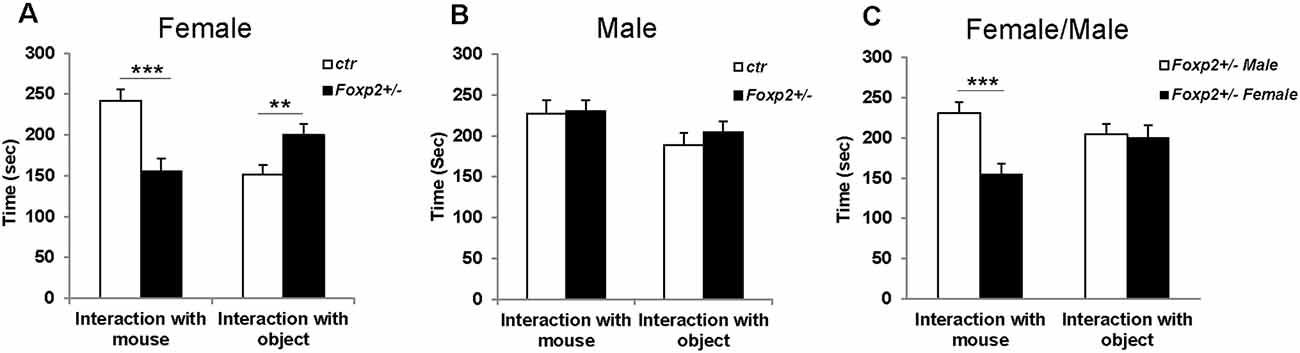
Figure 1. Decreased social interaction in female Foxp2+/– mice in the 3-chambered task. Compared to control mice, female Foxp2+/– mice show a significant decrease in time spent in the presence of a social cue (mouse; p = 0.0004, Foxp2+/– n = 13, ctr n = 12), with a correspondingly significant increase in time spent in the presence of a non-social object (wire cup; p = 0.01) (A). In contrast, male Foxp2+/– mice showed no significant changes in time spent in social or non-social interactions compared to control (p = 0.89, Foxp2+/– n = 13, ctr n = 12) (B). When directly comparing male and female Foxp2+/– mice, a significant decrease was observed in social interaction in females compared to males, with no change in non-social interaction (C; p** ≤ 0.01; p*** ≤ 0.001).
Olfactory Habituation/Dishabituation
Using the well-established habituation/dishabituation test (Yang and Crawley, 2009), we next assessed the ability of Foxp2+/– mice to detect and discriminate between non-social and social odors. In this task, a mouse is presented with a series of neutral odor (water) followed by attractive odors (peanut butter, urine) to which the animal typically spends more time investigating via sniffing. It is expected that mice will habituate to a previously displayed cue with a decrease in sniffing upon repeated presentation of the same cue, and dishabituate with an increase in sniffing when a novel odor is presented. In this assay, both female and male Foxp2+/– mice displayed decreased time sniffing olfactory cues; however, this deficit appeared more pronounced in females (Figure 2). Female Foxp2+/– mice spent less time than control littermates sniffing both non-social and social motivating odors, and interestingly also water, the neutral odor. However, while female Foxp2+/– mice displayed less interaction time with a given cue, habituation/dishabituation appeared to follow the same pattern as control. In contrast, male Foxp2+/– mice displayed a significant defect only in response to peanut butter, an appetitive cue, with no differences in time spent sniffing male or female urine. Thus, while habituation/dishabituation still occurred, both female and male Foxp2+/– mice appear to have complex deficits in olfactory processing that may relate to deficits in motivation to investigate novel cues and/or a diminished ability to process specific olfactory cues.
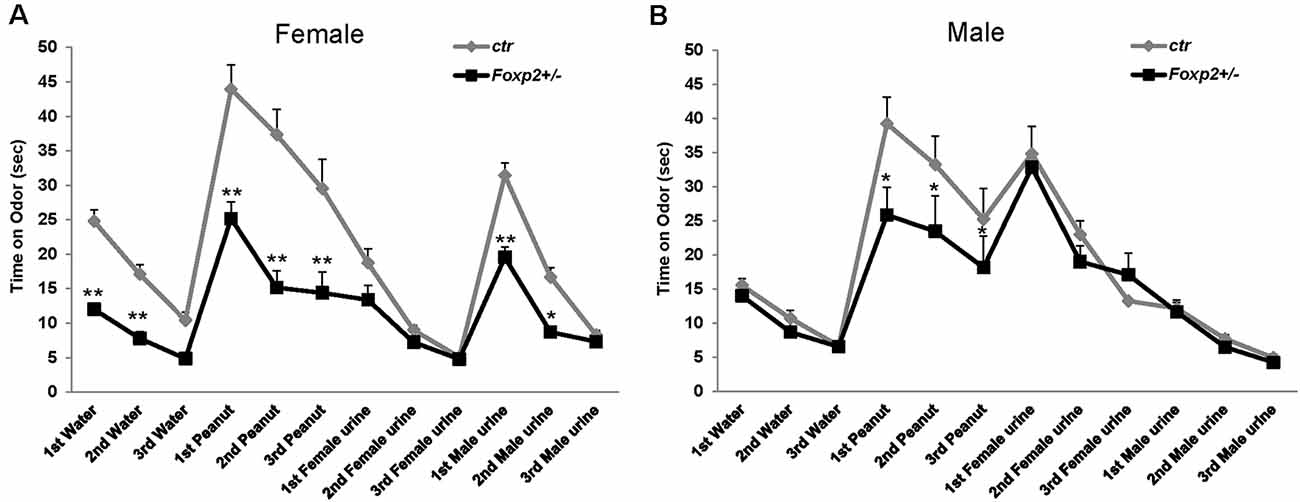
Figure 2. Female and male Foxp2+/– mice display decreased time spent investigating different odors. Mice were exposed to different odors (e.g. neutral: water; non-social: peanut butter; and social: female or male urine) three times each for 2 min (with intervals of 2 min between odor exposure). Both female and male Foxp2+/– mice spent significantly less time sniffing a non-social motivating odor (peanut) than control mice (A,B). Additionally, female Foxp2+/– mice spent significantly less time sniffing both a neutral odor and a social motivating odor (male urine) (B). The experiment was repeated in two different cohorts (Foxp2+/– n = 13, ctr n = 12; p* ≤ 0.05; p** ≤ 0.01).
Mating Assays
We next wanted to assess the ability of Foxp2+/– mice to execute the mating repertoire. In mice, mating behavior is characterized by stereotypical steps of male chemoinvestigation, followed by mounting, intromission and ejaculation. While both female and male Foxp2+/– mice were able to mate, each displayed deficits in specific aspects of the mating repertoire, with females and males displaying different deficits (Figure 3). Specifically, female Foxp2+/– mice displayed deficits in latency to mount. In contrast, the number and duration of mounts were significantly lower in Foxp2+/– males.
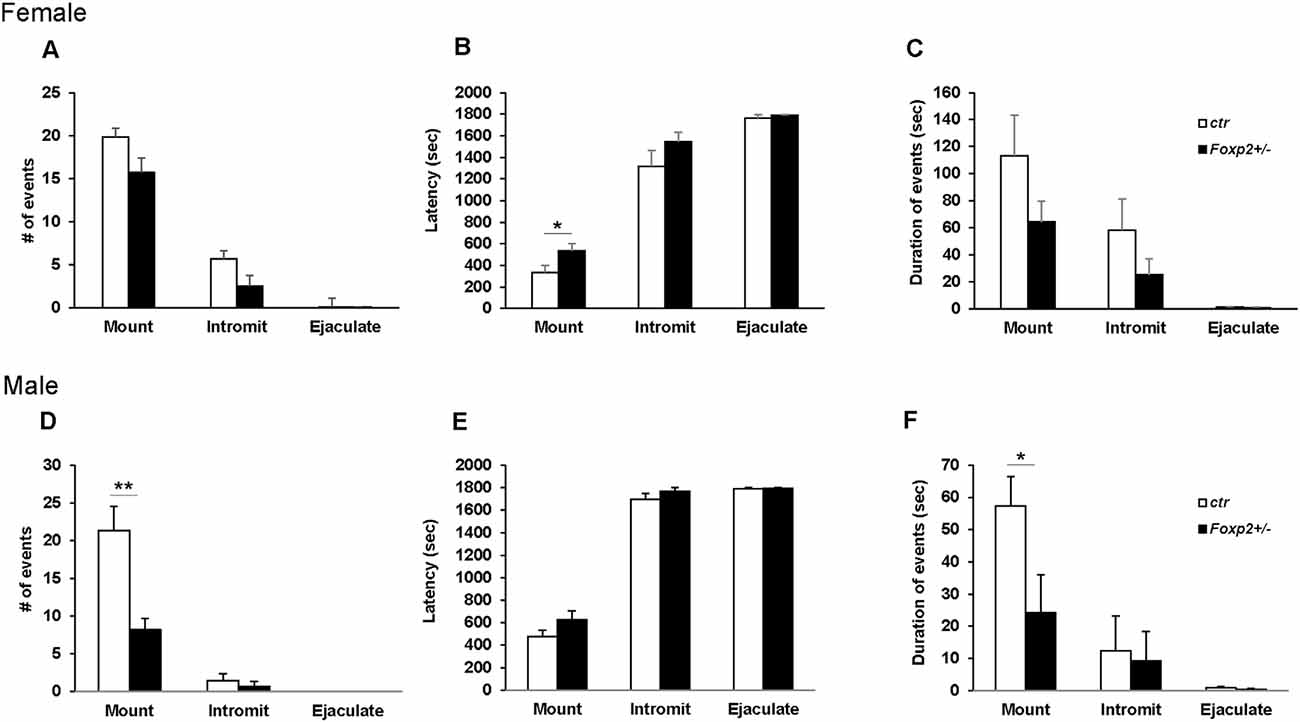
Figure 3. Impaired mating behavior in Foxp2+/– mice. The mating repertoire of mounting, intromission, and ejaculation was recorded over a 30 min period. While the number of mounts was unchanged in female Foxp2+/– mice, male Foxp2+/– mice displayed a decreased number of mounts compared to controls (A–D). In contrast, female Foxp2+/– mice displayed a greater latency to mount than controls, a phenotype that was not observed in male Foxp2+/– mice (B,E). The duration of events was unchanged in female Foxp2+/– mice (C), with a decrease in the duration of mounts in male Foxp2+/– mice compared (F). All other parameters were unchanged (A–F). Experiment was repeated in two different cohorts (total females: Foxp2+/– n = 14, ctr n = 13; total males: Foxp2+/– n = 10, ctr n = 10). Trials were repeated three times separated by at least 1 week (total of 81 trials for females, 60 trials for males). p* ≤ 0.05; p** ≤ 0.01.
Female and Male Aggression
We next explored maternal aggression and resident-intruder aggression in which female and male mice display aggressive behaviors, respectively (Silverman et al., 2010). Maternal aggression was assessed by placing an intruder mouse in the cage of a dam with pups removed (Figure 4). In this assay, we observed that Foxp2+/– dams were more aggressive showing less latency to first attack and attacking more times than the C57BL/6J controls. This was combined with fewer nips, a less aggressive display than a full attack. Thus, female Foxp2+/– mice were more overly aggressive to an intruder than controls. In contrast, male Foxp2+/– mice showed significantly less aggression when compared to controls, with a decrease in the number and duration of the attacks compared to controls (Figure 5). Interestingly, this decreased aggression was not due to differences in latency to first attack or changes in social exploration, as male Foxp2+/– mice investigated the intruder in a similar manner as controls. Thus, female and male Foxp2+/– mice display strikingly opposite alterations in aggressive behavior.
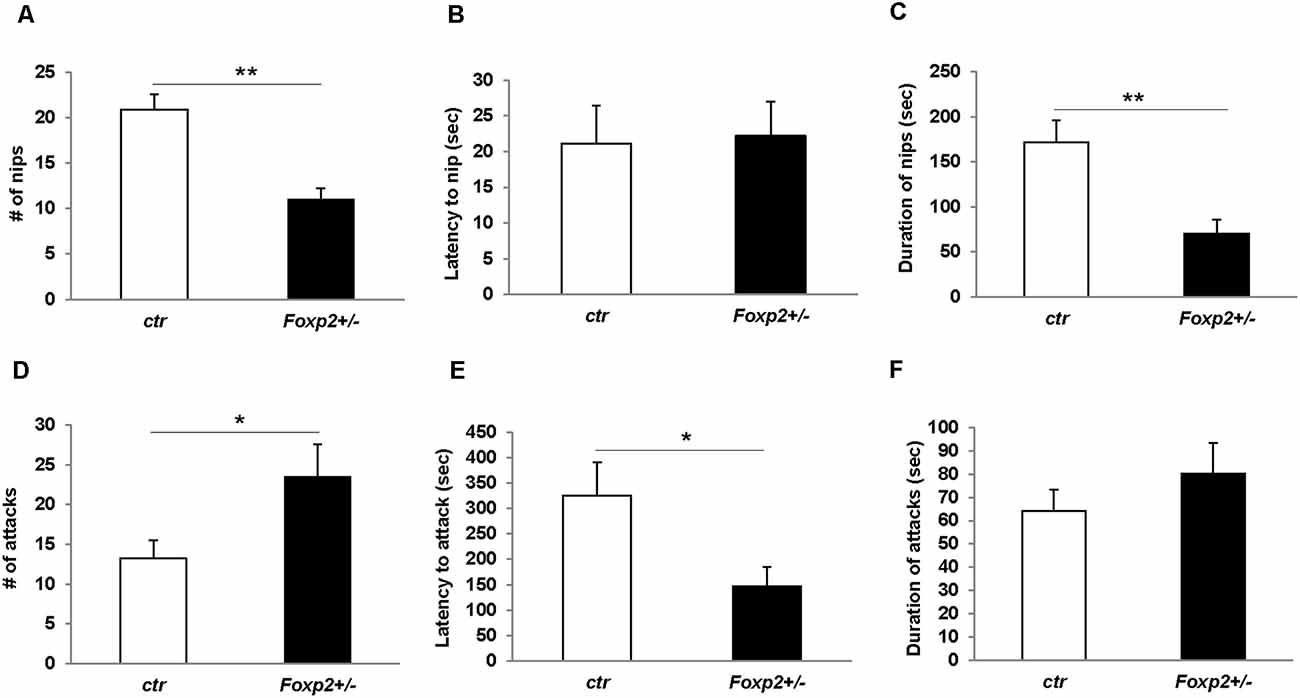
Figure 4. Increased maternal aggression in Foxp2+/– mice. An intruder C57BL/6J male was introduced for 15 min to the home-cage of either a lactating Foxp2+/– or ctr female mice after removal of pups. Female Foxp2+/– mice displayed a decreased number and duration of nips (A,C), but no change in the latency of nips compared to control (B). In contrast, the number and latency of attacks was significantly increased (D,E) with no change in the duration of attacks (F). Trials were repeated two times separated by at least 1 week (Foxp2+/– n = 10, ctr n = 12). p* ≤ 0.05; p** ≤ 0.01.
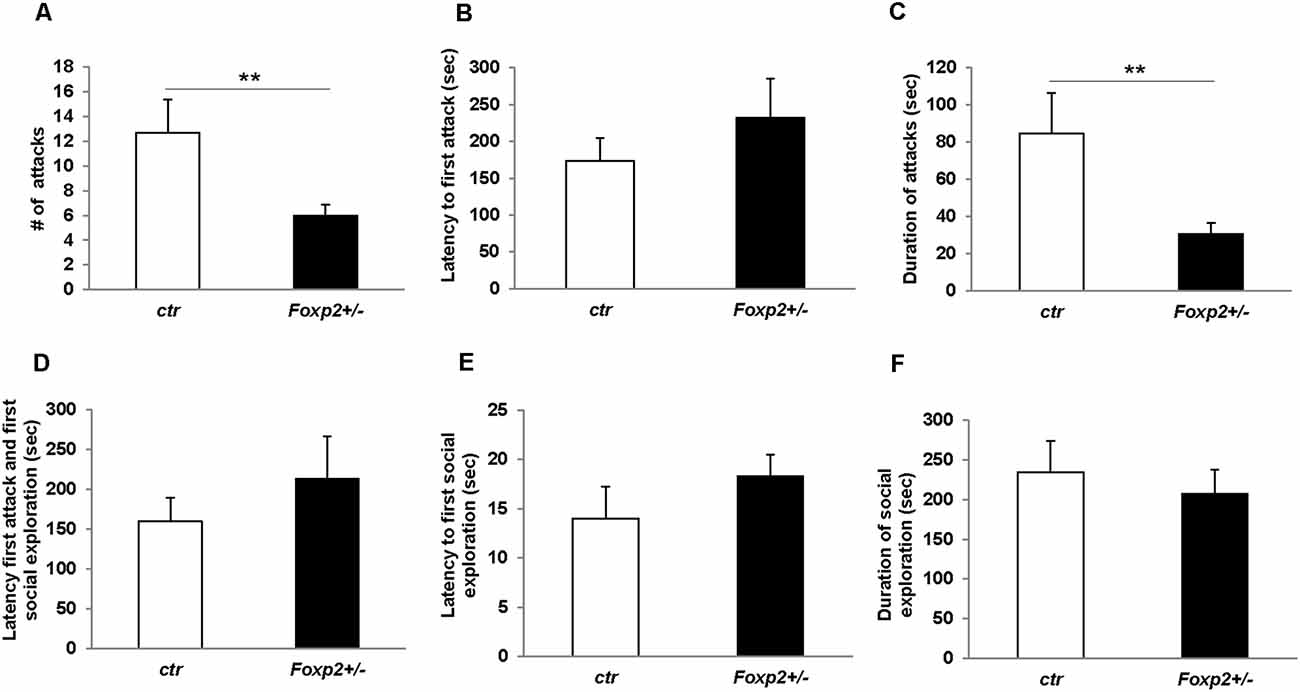
Figure 5. Decreased male territorial aggression in Foxp2+/– mice. An intruder C57BL/6J male was introduced into the home-cage of either a Foxp2+/– or ctr male for 10 min. Male Foxp2+/– mice displayed a significant decrease in both the number and duration of attacks compared to ctr (A,C). However, male Foxp2+/– mice did not display any differences in latency to first attack or social exploration, or difference in latency to first attack or social exploration compared to ctr (B,D,E). The total duration of social exploration was also unchanged (F) (Foxp2+/– n = 10, ctr n = 10). p** ≤ 0.01.
Pup Retrieval and Maternal Care
The ability of a dam to retrieve pups back to her nest is a well-characterized assay for maternal care (Silverman et al., 2010). Using this assay we tested Foxp2+/– females’ maternal behavior. We observed that the time to retrieve either one or two pups and bring them back to the nest was significantly greater in Foxp2+/– females (Figure 6). This indicates a deficit in motivation to retrieve pups and/or deficits in perceiving or processing sensory input related to pup calls. We further found a decrease in the number of pups per litter from a Foxp2+/– dam (Figure 6). We also anecdotally observed more pups found dead in the cage of Foxp2+/– dams than with control mice. Together, these results suggest diminished maternal care in Foxp2+/– females.
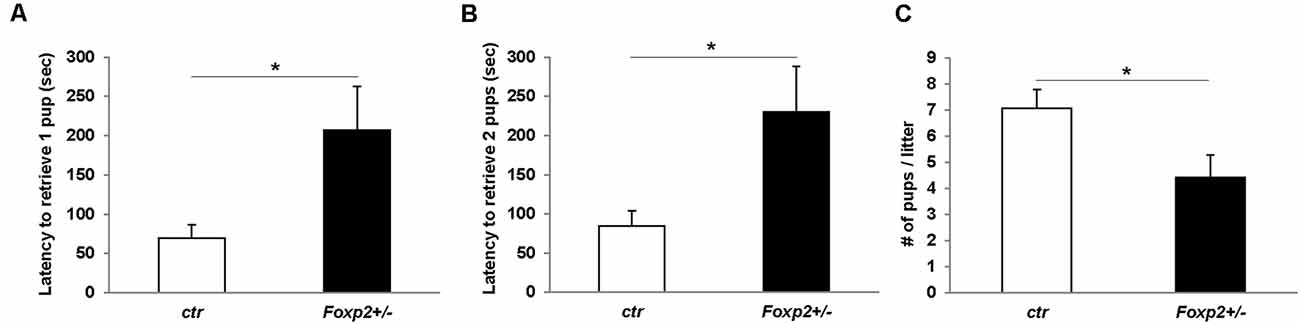
Figure 6. Female Foxp2+/– mice display deficits in maternal behavior. To examine pup retrieval, a Foxp2+/– or ctr dam was removed from the cage and their pups were removed from the nest and placed in a far corner. When placed back into the home-cage, Foxp2+/– dams displayed a significantly greater latency to retrieve one or two pups than ctr dams (A,B). Additionally, female Foxp2+/– mice had significantly less pups per litter (C). For (A,B). Trials were repeated two times separated by at least 1 week in two different cohorts (total number of dams: Foxp2+/– n = 10, ctr n = 13; one litter per dam). p* ≤ 0.05.
Predator Odor Avoidance
Predator odor avoidance is an innate behavior in which an animal displays avoidance to olfactory cues of a predator, and when in a confined environment this behavior is characterized by increased risk assessment (cautious investigation) and decreased sniffing of predator olfactory cue followed by stereotypical escape responses such as climbing (Apfelbach et al., 2005). In this assay, we used rat bedding as a predator odor, which is typically highly aversive to mice (Sokolowski et al., 2015; Figure 7). In our hands, control male mice did not display the typical avoidance response (data not shown), whereas female control mice displayed the stereotypical reduced sniffing and increased risk assessment behavior and climbs (Figure 7). Therefore, here we present only the data for females. Foxp2+/– female mice displayed an overall diminished predator avoidance response as indicated by no change in risk assessment, sniffing, and/or climbs in the presence of rat odor compared to benign odor. This indicates that Foxp2+/– mutant females did not interpret rat odor as aversive.
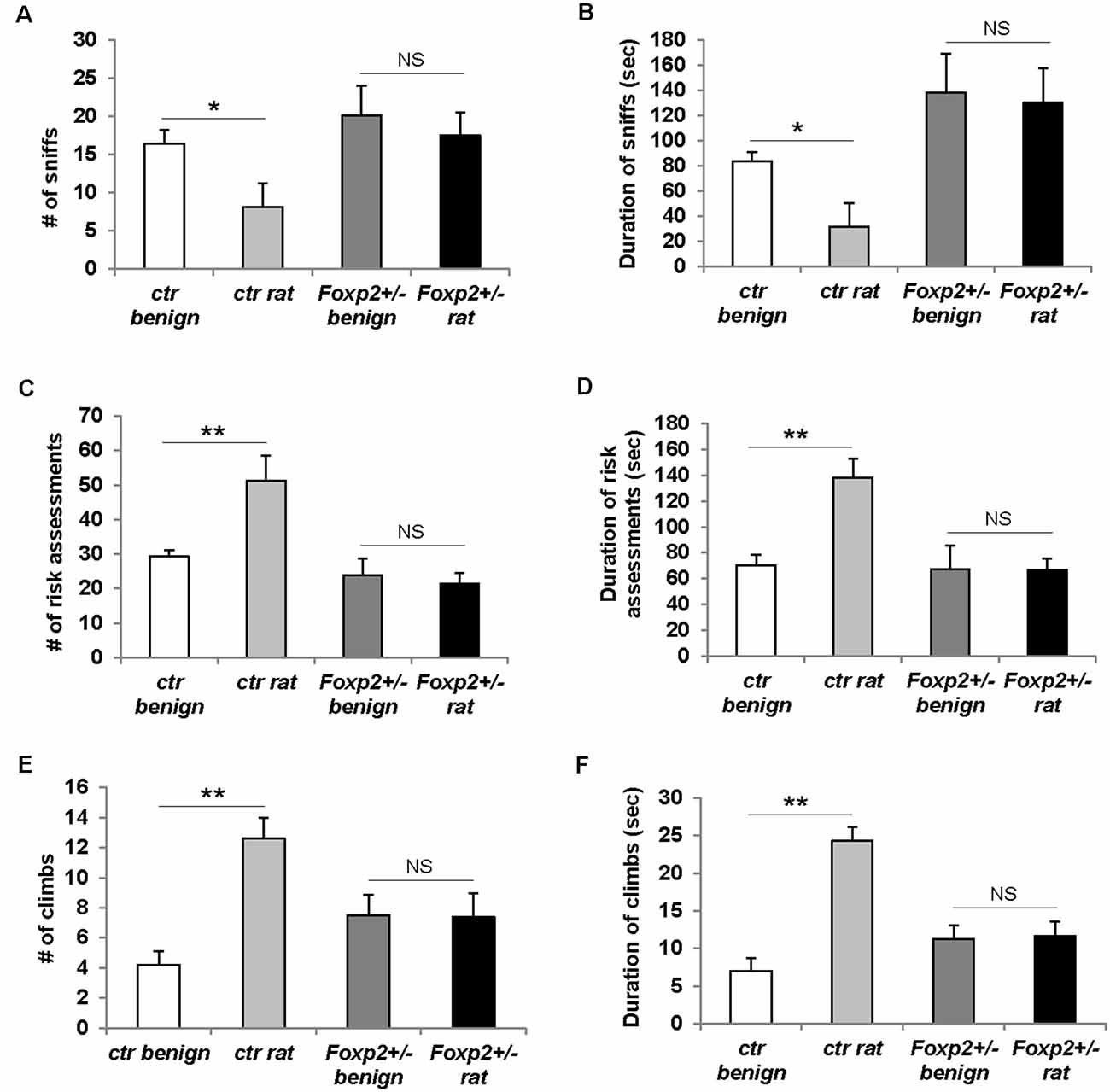
Figure 7. Females Foxp2+/– mice display impaired predator odor avoidance. Ctr female mice exposed to the aversive odor of rat bedding display significantly decreased number (A) and duration (B) of sniffs to the aversive odor when compared to a benign odor (unsoiled bedding). In contrast, female Foxp2+/– mice do not display a change in either number (A) or duration (B) of sniffs to rat bedding compared to a benign odor. Ctr female mice also display the stereotypical risk assessment behavior to rat bedding, as defined by an increased number (C) and duration (D) of risk assessments as compared to benign odor. In contrast, female Foxp2+/– mice do not display risk assessment behavior (C,D). Ctr female mice also display stereotypical escape behaviors to rat bedding, observed as a significant increase in the number of climbs (E) and duration of climbing (F) compared to benign odor. In contrast, this escape behavior is not observed in female Foxp2+/– mice (E,F). The experiment was repeated in four different cohorts (total: Foxp2+/– n = 10, ctr n = 10). p* ≤ 0.05; p** ≤ 0.01. NS, not significant.
In sum, we found that Foxp2+/– mice displayed impaired social interaction, olfactory discrimination/motivation, mating/reproduction, aggression against an intruder, and predator odor avoidance, with social interaction and aggression manifesting in a sex-specific manner (Table 1).
MeA Proteomic Changes in Female and Male Foxp2+/– Mice
As we observed robust differences in multiple behaviors in Foxp2+/– mutant mice, with a subset displaying in a sex-specific manner, we next explored underlying proteomic changes correlating with these behavioral alterations in males and females. Based on the known role of the MeA in each of the social and non-social behaviors we tested (Kling and Brothers, 1992; Choleris et al., 2007; Sokolowski and Corbin, 2012; Bergan et al., 2014) and that the MeA is comprised of a large population of Foxp2+ cells (Carney et al., 2010; Lischinsky et al., 2017), we focused our proteomic analysis on this structure. MeA tissue from three animals (two amygdala/animal for a total of n = 6) was pooled together and considered a single sample. A total of n = 2 samples were analyzed for each sex (male and female) and genotype (Foxp2+/– and control). We conducted TMT Isobaric Mass Tagging proteomics analysis to assess the full repertoire of proteins differentially expressed in the MeA of female and male Foxp2+/– mutant mice. We compared female and male Foxp2+/– mutants to their respective controls in three sets of analyses (Figures 8, 9). First, comparing female Foxp2+/– mutants to female control, we observed 107 differentially expressed proteins (Figure 9A, Supplementary Table 1). Second, comparing male Foxp2+/– mutants to male control, we observed 97 differentially expressed proteins (Figure 9B, Supplementary Table 1). Third, comparing male Foxp2+/– mutants to female Foxp2+/– mutants, we observed 107 differentially expressed proteins (Figure 9C, Supplementary Table 1). Two proteins, ALDH1A1 and CRYM, were found in all comparisons, with other protein sets common to two comparisons or only in one comparison (Figure 9). In addition to protein changes that are common across more than one comparison, a large number of protein changes were solely observed in each comparison (36 for Foxp2+/– mutant F vs. control F; 64 for Foxp2+/– mutant M vs. control M; 52 for Foxp2+/– mutant M vs. Foxp2+/– mutant F). Thus, these protein sets represent both mutant vs. control differences within the same sex, as well as sex-specific signatures of Foxp2 function in the MeA. It is important to note that as we performed global quantitative proteomics analyses in only two technical replicates, these results will need future replication in larger sample sets and validation of candidates of interest on tissue. Despite this caveat, as described below, our analysis suggests alterations in interesting pathways.
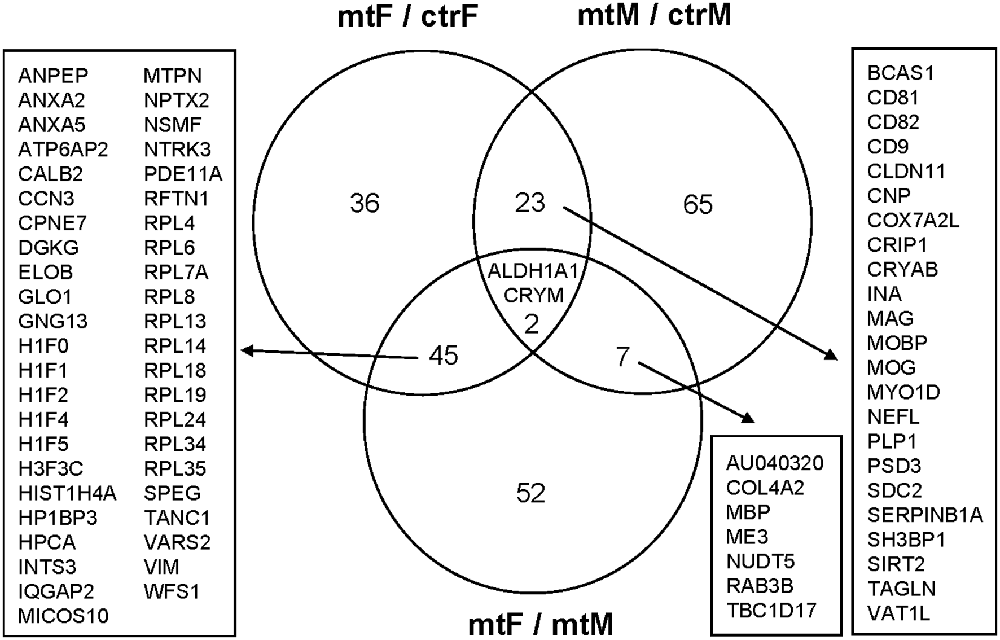
Figure 8. Overlapping and non-overlapping changes in protein expression in the MeA of female and male Foxp2+/– mice. Venn Diagram of the differentially expressed proteins in the three comparisons from Figure 8: Foxp2+/– female vs. ctr female (mtF/ctrF), Foxp2+/– male vs. ctr male (mtM/ctrM), and Foxp2+/– female vs. Foxp2+/– male (mtF/mtM; Log2F C≥ |1.11|). The signature of proteins and neuromodulators in the amygdala of Foxp2+/– mice was sex-specific.
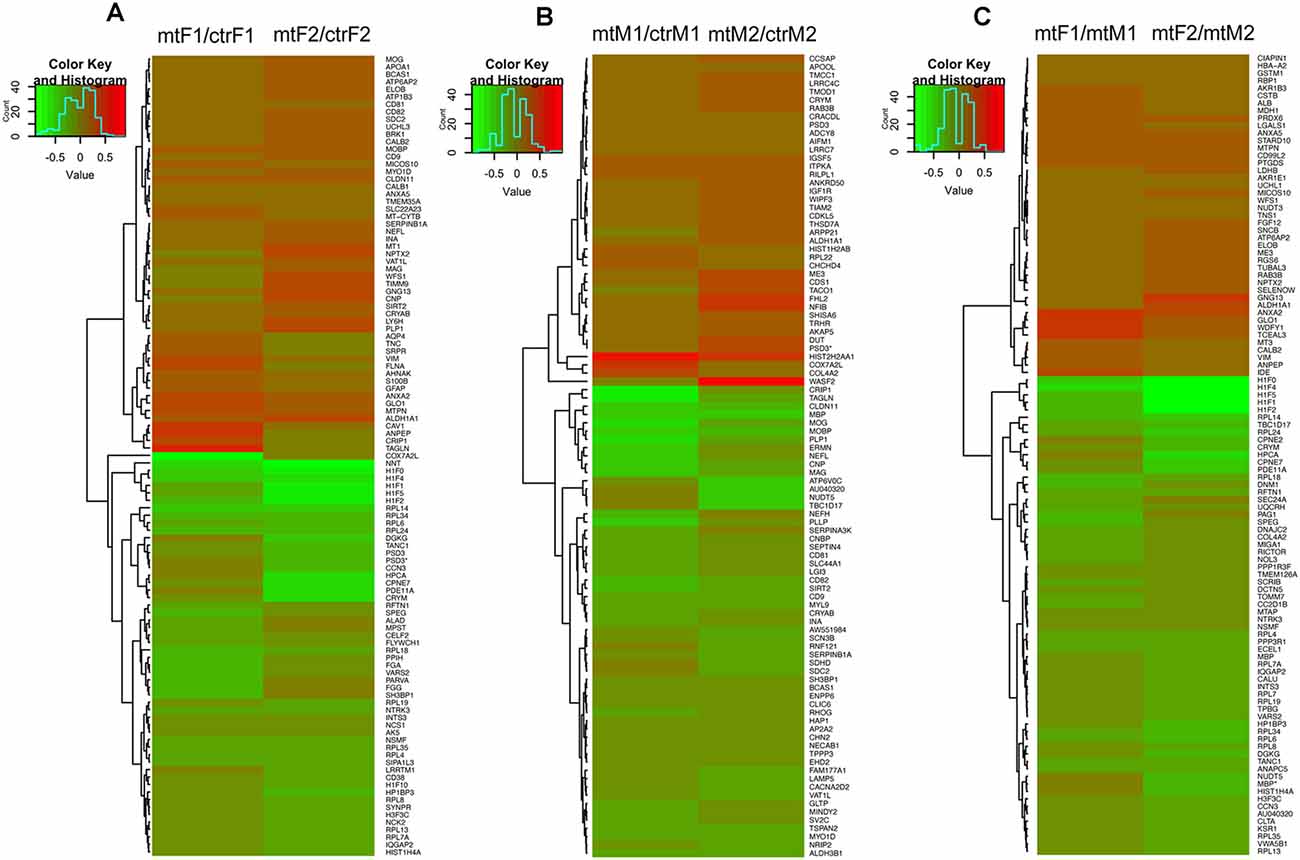
Figure 9. Heatmaps of differentially expressed proteins in the MeA of female and male Foxp2+/– mice. Pooled microdissected MeA tissue from thee brains was run in duplicate. Ratios for each duplicate are shown in the x-axis and protein names (symbols) of differentially expressed proteins are displayed on the y-axis on the right. Panels (A–C) show proteins significantly upregulated (red) or downregulated (green) in three comparisons: Foxp2+/– female vs. ctr female (mtF/ctrF) (A), Foxp2+/– male vs. ctr male (mtM/ctrM) (B), Foxp2+/– female vs. Foxp2+/– male (mtF/mtM) (C) (Log2 fold change ≥ |1.11|). Proteins were clustered based on similarity of expression between the experimental and control groups, which is indicated by the dendrogram. The color key and histogram panel indicates the Z-scores of expression and the expression counts. See Supplementary Table 1 for a full list of altered proteins in each comparison.
Enriched ontology (GO) analyses revealed common pathway alterations in neuronal communication and protein transport (Figure 10, Table 2). Indeed, within the enriched GO terms for the proteins differentially expressed in the MeA across comparisons, the term “myelin sheath” was common across all three comparisons, and six other terms were common in two comparisons: “NAD binding”, “ensheathment of neurons” and “cellular aldehyde metabolic process” (in comparisons Figures 10A,B), and “association of DFF40 with chromatin”, “cytosolic large ribosomal subunit” and “extracellular exosome” (in comparisons Figures 10A,C). Among them, “association of DFF40 with chromatin” was the most enriched term (41%), when comparing female to male mutants (Figure 10). Other prominent GO-enriched terms based on the terms per group with the highest percentages were related to chromatin regulation, cell cycle, and catecholamine metabolic process (Table 2).
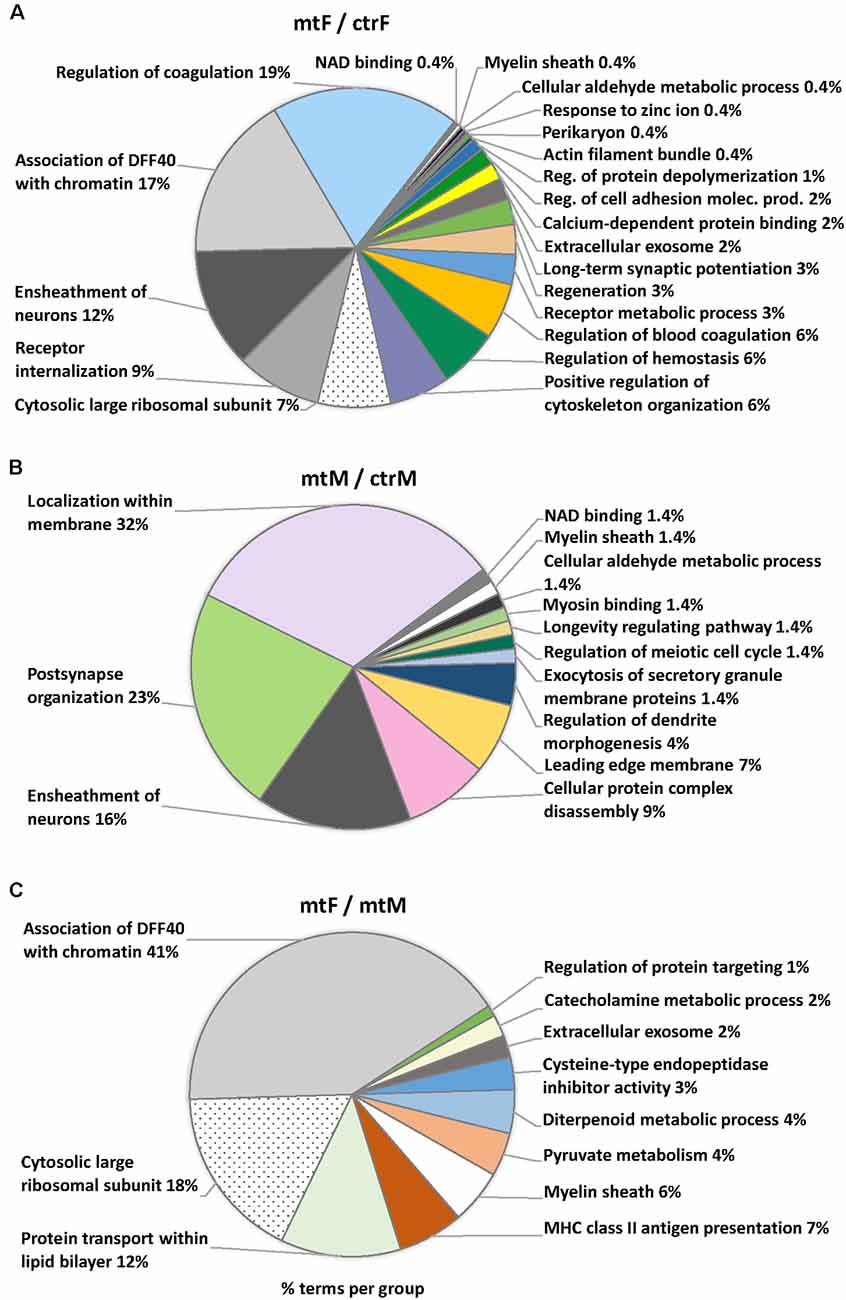
Figure 10. Pie charts of enriched Ontology (GO) terms for the proteins differentially expressed in the MeA of female and male Foxp2+/– mice. Ontology analysis in the three comparisons: Foxp2+/– female vs. ctr female (mtF/ctrF), Foxp2+/– male vs. ctr male (mtM/ctrM), Foxp2+/– female vs. Foxp2+/– male (mtF/mtM). Myelin sheath was a common term for the three comparisons (colored in white). Common terms across (A,B; i.e., Ensheathment of neurons, NAD binding, Cellular aldehyde metabolic process) and (A,C; Association of DFF40 with chromatin, Cytosolic large ribosomal subunit, Extracellular exosome) are colored in scales of gray. Selected terms and related metrics were determined using ClueGO, based on the terms per group with the highest percentages.
One interpretation of our above described behavioral analyses (Figures 1–7) is that deficits in Foxp2+/– mutant behavior may reflect an alteration in the motivational drive. As dopamine (DA) is a prime neurotransmitter implicated in motivational states and previous studies have revealed dopaminergic inputs to the MeA (Poulin et al., 2018), we assessed whether there were protein changes in the expression of known DA pathway/DA regulated proteins (Table 3). Across comparisons, we found 3 DA pathway proteins to have altered expression (FLNA, GFAP, DMN1).
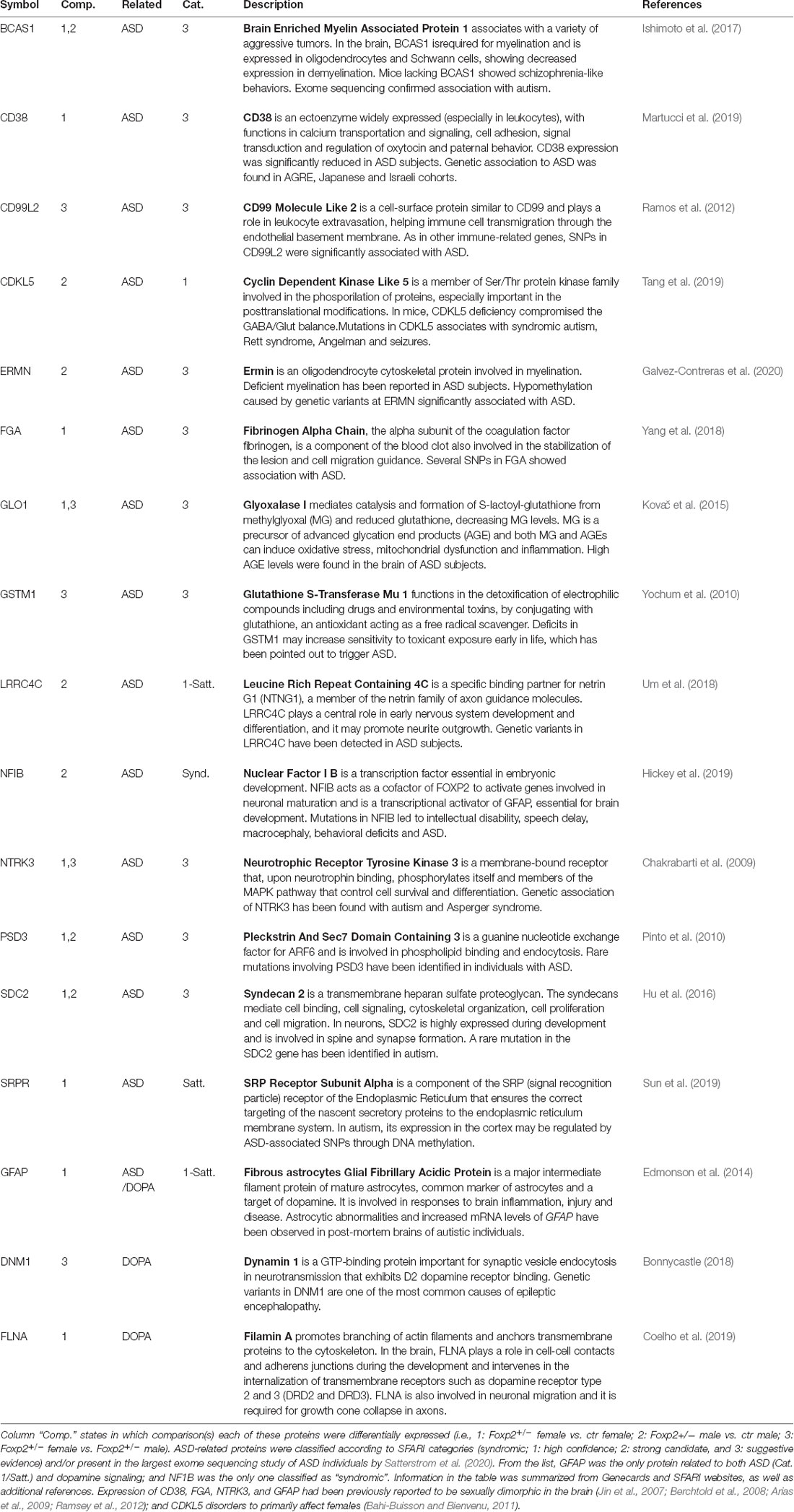
Table 3. Detailed information on the biological function of the 15 ASD and three dopamine related proteins differentially expressed in the MeA.
Mutations in FOXP2 in humans have been consistently associated with Autism Spectrum Disorders (ASD), and as such, FOXP2 is ranked category 1 (high confidence) in the SFARI autism gene database1. Comparing proteomic changes in Foxp2+/– mutant mice to both the SFARI gene database and the results of the most comprehensive ASD sequencing effort to date (Satterstrom et al., 2020), we found 15 proteins implicated in ASD (Table 3) in at least one comparison, with one of these, the nuclear factor NFIB, being syndromic.
Sex Differences in Dopamine Activation of Foxp2-Lineage Cells in the MeA
Previous studies have identified DA-ergic inputs to the MeA (Poulin et al., 2018). The above described proteomic analysis revealed putative alterations in DA signaling, however, it is not known if Foxp2+ cells can respond directly to DA. To test this, we crossed Foxp2cre mice (Rousso et al., 2016) to GCaMP5G-tdTM reporter mice (Gee et al., 2014) allowing us to assess evoked Ca2+ transients in Foxp2+ cells in acute brain slices (Figures 11A,B). Focal application of DA (100 μm) evoked cytosolic Ca2+ increases in multiple morphologically distinct cell types resembling astrocytes, neurons, and ependyma. We further found Foxp2+ activation patterns in response to DA differed in females and males, with less activation in females (Figure 11C). Thus, this analysis reveals that MeA Foxp2+ cells can be activated by dopamine, a neurotransmitter involved in social behavior, motivation, and reward.
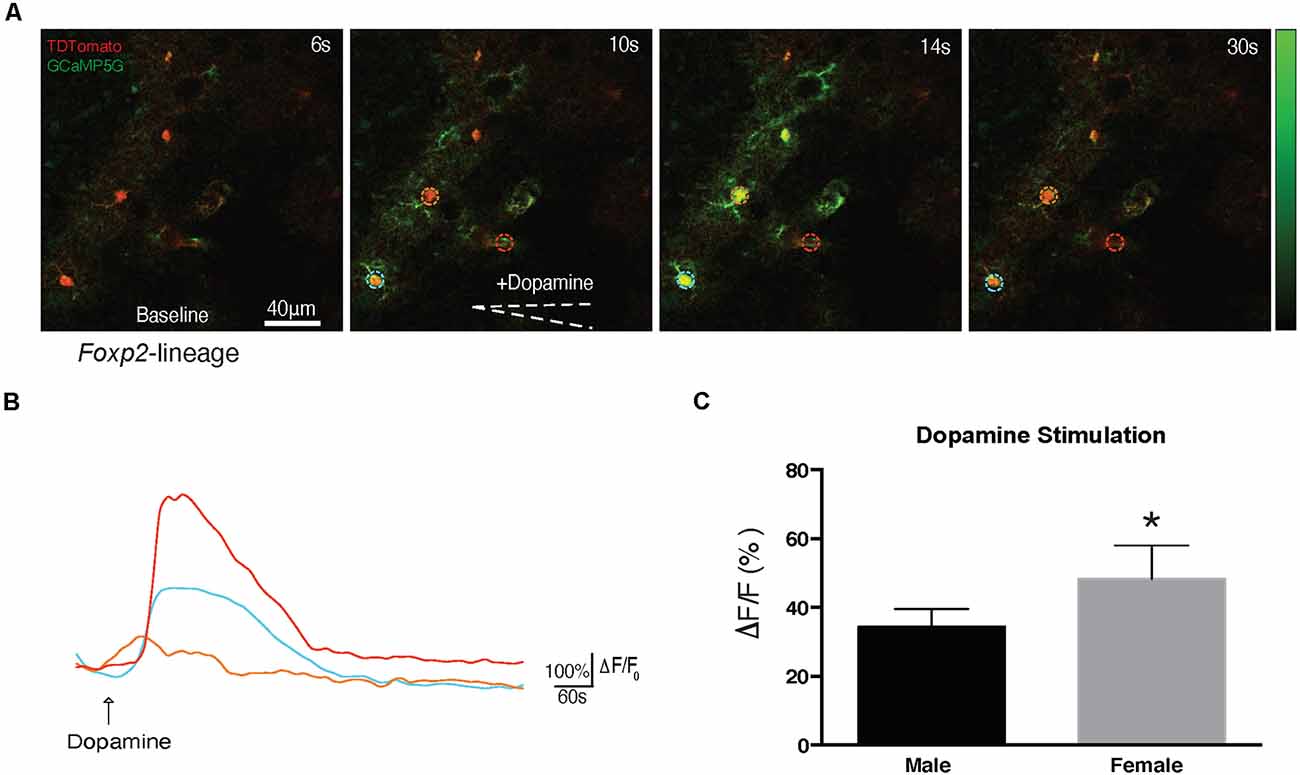
Figure 11. Foxp2-lineage cells in MeA are more responsive to dopamine in females. Dopamine evoked Ca2+ transients were assessed in 300 μm MeA slices from Foxp2cre;GCaMP5G-tdTM mice. Time series of Ca2+ increases in Foxp2-lineage cells by focal application of dopamine (100 mM) from a glass pipette (white dotted line) is shown: in red the recombined Foxp2-lineage cells, in green the Ca++ transient (A). The pseudocolor scale displays changes in GCaMP5G △F/F. Representative individual traces of GCaMP5G fluorescence changes (△F/F) in response to dopamine (B). The color-coding of the traces matches the ROI of the colored circles in (A). Dopamine evoked significantly more activation in Foxp2-lineage cells in females than in males (C). Analysis was carried out in n = 5 females, n = 4 males in at least two slices per animal containing the MeA. *p ≤ 0.05 (p = 0.0294).
Discussion
In this study, we found that heterozygous Foxp2+/– knockout mice display altered behavior across a host of innate social and non-social behaviors (summarized in Table 1). The brain regions modulating these behaviors are well documented and most prominently include the olfactory bulb (both main and accessory), the cortical and medial nuclei of the amygdala (MeA), bed nucleus of the stria terminalis (BNST), and multiple nuclei of the hypothalamus (Gross and Canteras, 2012; Sokolowski and Corbin, 2012; Chen and Hong, 2018; Li and Dulac, 2018). Of these regions, Foxp2+ cells are found in high densities in the olfactory bulb (both main and accessory), and MeA, with fewer cells in the hypothalamus2. Thus, Foxp2+ neurons are well-positioned to modulate social and non-social innate behaviors, and deficits in Foxp2 gene function in any of these regions may underlie the innate behavioral deficits observed here. Our previous work (Lischinsky et al., 2017) revealed that the Foxp2+ cells of the MeA comprise a subpopulation of inhibitory output neurons. As the MeA is directly involved in processing olfactory sensory information for appropriate behavioral outputs, largely via connections with the BNST and hypothalamus, the MeA represents a likely site of deficit for the altered behavioral processing we observed in Foxp2+/– mice. Consistent with this role, via cFos expression, we previously observed that MeA Foxp2+ cells respond to a host of innate behavioral cues (Lischinsky et al., 2017). However, it is important to note that while we hypothesize that deficits in the MeA are at least in part causally linked to our behavioral phenotypes observed in Foxp2+/– mice, deficits in other brain regions cannot be ruled out at this time. Conditional Foxp2 mutagenesis in the olfactory bulb, MeA, and hypothalamus will likely prove highly informative in dissecting the region-specific role of Foxp2 in innate behavioral processing.
Of the behaviors we explored, we interestingly found sex differences mainly in two: social interaction and aggression. We observed decreased social interaction in female Foxp2+/– mice, with no change in male Foxp2+/– mice. Furthermore, and perhaps most striking, were the opposite consequences on aggressive behavior. Female Foxp2+/– mice displayed enhanced aggression, while male Foxp2+/– mice displayed decreased aggression. Although it is important to note that male territorial aggression is considered offensive aggression, while maternal aggression is considered defensive, we find the opposite results in Foxp2+/– mice particularly intriguing. Thus, it appears that Foxp2 gene function plays behaviorally separable roles in the male and female brain. As aggression manifests differently and in different contexts in male and female mice, this result may reflect either male/female differences in Foxp2+ circuitry and/or neuronal function. Consistent with this, the core aggression circuit across species is sexually dimorphic (Hashikawa et al., 2018; Li and Dulac, 2018). In mice, there are known sex differences in olfactory inputs to the MeA, as well as sex differences in MeA neuronal population activation in response to various olfactory cues. In the fruit fly, Drosophila, recent studies have revealed that males and females have different neurons for regulating aggression (Chiu et al., 2021). Although the circuit and/or neuronal mechanism of sex differences in aggressive and social interactive behaviors that we observed in Foxp2+/– mice remains to be uncovered, our proteomic analyses provide us preliminary clues. The major difference when comparing female to male mutants is in the GO term “association with DFF40 with chromatin.” DFF40 is associated with apoptotic cell death (Liu et al., 1998), perhaps indicating male/female differences in the requirement for Foxp2 in cell survival/apoptosis either in a cell-autonomous or non-cell-autonomous manner. In addition, based on our GO analyses, several other Foxp2-dependent processes may be involved, such as membrane protein transport and cytosolic large ribosomal transport, which together may suggest sex differences in protein trafficking and/or translation. In agreement with our results, extensive previous work has implicated Foxp2 gene function in the multi-fold processes of chromatin regulation, activity-dependent plasticity, and dendritic morphology (French and Fisher, 2014; Co et al., 2020). As the MeA is a highly sexually dimorphic brain region (Gegenhuber and Tollkuhn, 2020; Matos et al., 2020), it is possible that Foxp2 may be required to regulate these processes differently in males and females. Unraveling the underlying neuronal and circuit mechanisms of the Foxp2-dependent control of innate behaviors as well as the sex differences in the function of Foxp2 will likely be an exciting area of future investigation.
Our extensive behavioral analyses of Foxp2+/– mice reveal robust deficits across a number of innate behavioral domains, typically displayed as a blunted behavioral response across various behaviors (with the notable exception of aggression in female Foxp2+/– mice, which is enhanced). One interpretation of this data is that Foxp2 is required for proper motivation to execute a given behavior. In this context, one apparent candidate pathway to explain our behavioral data would be alterations in dopamine (DA) signaling, which is highly implicated in motivation and reward-seeking behavior. Focusing on DA signaling, from our proteomics analyses, we found that Foxp2+/– mice have alterations in the expression of DA-signalling/DA-responsive proteins FLNA, GFAP, and DMN1. However, as our proteomics analysis was done on whole MeA tissue, thus containing both Foxp2-positive and Foxp2-negative cells, we cannot determine if this deficit is cell-autonomous or cell non-autonomous. To investigate whether Foxp2+ cells can directly respond to DA, we show via 2-Photon Ca2+ imaging that Foxp2+ cells are highly responsive to DA, and notably with differences between males and females. It will be interesting to explore if/how DA signaling is altered in Foxp2+ cells in Foxp2+/– mutants. In the striatum, Foxp2 is expressed in many medium spiny neurons, major targets of dopaminergic inputs (Ferland et al., 2003; Lai et al., 2003; Takahashi et al., 2003; Scharff and Haesler, 2005; Wijchers et al., 2006). Consistent with a putative Foxp2-dependent role for DA, Enard et al. (2009) observed increased DA levels in heterozygous Foxp2+/– mice across a number of brain regions (though this study did not include the amygdala). In addition, while the cellular target was not identified, previous axonal tracing studies have shown that there are major DA inputs to the MeA (Poulin et al., 2018), and MeA neurons express DR1 receptors (Miller et al., 2019). Consistent with this, in humans, dopamine modulates the amygdala network (also comprising some areas of the prefrontal cortex and the nucleus accumbens) to mediate mother-infant bonding (Atzil et al., 2017). It is therefore plausible that alterations in DA signaling may be a contributing component to the behavioral deficits we observed in Foxp2+/– mice.
In humans, FOXP2 has been most closely linked to language disorders (Morgan et al., 2017). Despite conflicting genetic evidence, many studies have found an association between FOXP2 mutations and ASD (Gong et al., 2004; Li et al., 2005; Marui et al., 2005; Feuk et al., 2006; Laroche et al., 2008; Chien et al., 2017; Lim et al., 2017; Guo et al., 2019; Munnich et al., 2019; Satterstrom et al., 2020). ASD is a common multi-genic neurodevelopmental disorder affecting 1 in 68 individuals (with a higher prevalence in males), with little known regarding the underlying neurobiology of sex differences in diagnosis. We interestingly observed that Foxp2+/– female, but not male, mice display deficits in the 3-chambered social task. This task is one of the major assays for face validity in ASD-animal models, suggesting ASD-like behavior in female Foxp2+/– mice. Consistent with this, we found that in the MeA, Foxp2+/– mice differentially expressed 15 ASD-risk proteins. This is perhaps not surprising as amygdala dysfunction has been highly implicated in the pathophysiology of ASD (Baron-Cohen et al., 2000; Lim et al., 2017; Avino et al., 2018). One protein of particular interest is the syndromic nuclear factor NFIB, which was upregulated in Foxp2+/– males. This protein is a coactivator of FOXP2 and is able to regulate androgen and estrogen receptor activity (Grabowska et al., 2014; Becker-Santos et al., 2017; Hickey et al., 2019). Another interesting protein is GFAP, a common marker for astrocytes which is also related to both ASD (SFARI: Score 1 = high-confidence; Edmonson et al., 2014; Herrero et al., 2020; Satterstrom et al., 2020) and dopamine signaling (Sands and Chronwall, 1996; Galloway et al., 2018). Notably, GFAP was only differentially expressed when comparing Foxp2+/– mutant females vs. ctr females, perhaps suggesting a role for this protein in the sex-specific differences associated with deficiency of Foxp2+/– in females. Thus, while Foxp2+/– mice are not a model for syndromic ASD, our findings may provide a window into differential gene and protein regulation in the male and female brain in relation to ASD.
Data Availability Statement
The original contributions presented in the study are included in the article/Supplementary Material, further inquiries can be directed to the corresponding author/s. The mass spectrometry proteomics data have been deposited to the ProteomeXchange Consortium via the PRIDE partner repository (Perez-Riverol et al., 2019) with the dataset identifier PXD026978.
Ethics Statement
The animal study was reviewed and approved by Children’s National Hospital Institutional Animal Care and Use Committee (IACUC), conformed to NIH Guidelines for animal use.
Author Contributions
MH: conceptualized the study, conducted microdissections, protein isolation, oversaw proteomic screen and with AP analyzed the proteomics data; managed the mouse colony and coordinated behavioral experiments with LW, and co-wrote the manuscript with JC. LW: designed and conducted the experiments on behavior, data analysis, and co-wrote behavior materials and methods and results. DH-P: animal care and handling and assisted with data analysis. PB: assisted with proteomic data analysis and plotting heatmaps. HM: assisted in calcium imaging experiment and brain slice preparation. MG: assisted with animal colony maintenance and genotyping. AP: oversaw proteomic quantitation, conducted mass spec experiments and with MH proteomic data analysis. NS: conducted experiment on calcium imaging, data analysis, and writing of materials and methods and results. JC: conceptualized and supervised the study, managed collaborations, provided funding, and co-wrote the manuscript with MH. All authors contributed to the article and approved the submitted version.
Funding
This work was supported by NIH R01DA020140 and the Florence Nesh Charitable Trust through the PNC Charitable Trusts Grant Review Committee (JC), the Philippe Foundation (MH), and NIH K01 NS110981 (NS). Further support was provided by the District of Columbia Intellectual and Developmental Disabilities Research Center (DC-IDDRC), U54HD090257 and the Clinical and Translational Science Institute at Childrens National, UL1TR001876. The animal behavior work was conducted within the NIH DC-IDDRC funded Animal Neurobehavior Core (U54HD090257).
Conflict of Interest
The authors declare that the research was conducted in the absence of any commercial or financial relationships that could be construed as a potential conflict of interest.
Publisher’s Note
All claims expressed in this article are solely those of the authors and do not necessarily represent those of their affiliated organizations, or those of the publisher, the editors and the reviewers. Any product that may be evaluated in this article, or claim that may be made by its manufacturer, is not guaranteed or endorsed by the publisher.
Acknowledgments
We kindly acknowledge the donation of Foxp2+/– mice from laboratory Dr. Joseph D. Buxbaum at the Mt. Sinai School of Medicine and Dr. Gregg A. Castellucci in the McCormick Lab at Yale University for coordinating the shipment of Foxp2+/– mice. We also acknowledge the dedication of our colleagues in the Children’s National Animal Facility directed by JanNean Bradford, Daniel Bobb, and Dr. Crisanto Escano. We thank members of the Corbin, Haydar and Triplett Labs for helpful input during the course of the project. We thank Dr. Hiroki Morizono, the Children’s National Research Institute Bioinformatics Unit and the DC-IDDRC Genomics and Bioinformatics Core for their help on bioinformatics analyses.
Footnotes
Supplementary Material
The Supplementary Material for this article can be found online at: https://www.frontiersin.org/articles/10.3389/fnbeh.2021.706079/full#supplementary-material.
Supplementary Table 1 | List of differentially expressed proteins in the MeA of Foxp2+/– female and male mice. Differentially expressed proteins are listed from the three comparisons: Foxp2+/– female vs. ctr female (mtF/ctrF), Foxp2+/– male vs. ctr male (mtM/ctrM), Foxp2+/– female vs. Foxp2+/– male (mtF/mtM). The total number of differentially expressed proteins for each of the three comparisons was respectively: 107 proteins (54 downregulated, 53 upregulated); 97 proteins (57 downregulated, 40 upregulated), and 107 proteins (64 downregulated, 43 upregulated).
References
Abrahams, B. S., and Geschwind, D. H. (2010). Connecting genes to brain in the autism spectrum disorders. Arch. Neurol. 67, 395–399. doi: 10.1001/archneurol.2010.47
Anney, R., Klei, L., Pinto, D., Regan, R., Conroy, J., Magalhaes, T. R., et al. (2010). A genome-wide scan for common alleles affecting risk for autism. Hum. Mol. Genet. 19, 4072–4082. doi: 10.1093/hmg/ddq307
Apfelbach, R., Blanchard, C. D., Blanchard, R. J., Hayes, R. A., and McGregor, I. S. (2005). The effects of predator odors in mammalian prey species: a review of field and laboratory studies. Neurosci. Biobehav. Rev. 29, 1123–1144. doi: 10.1016/j.neubiorev.2005.05.005
Arias, C., Zepeda, A., Hernández-Ortega, K., Leal-Galicia, P., Lojero, C., and Camacho-Arroyo, I. (2009). Sex and estrous cycle-dependent differences in glial fibrillary acidic protein immunoreactivity in the adult rat hippocampus. Horm. Behav. 55, 257–263. doi: 10.1016/j.yhbeh.2008.10.016
Atzil, S., Touroutoglou, A., Rudy, T., Salcedo, S., Feldman, R., Hooker, J. M., et al. (2017). Dopamine in the medial amygdala network mediates human bonding. Proc. Natl. Acad. Sci. U S A 114, 2361–2366. doi: 10.1073/pnas.1612233114
Avino, T. A., Barger, N., Vargas, M. V., Carlson, E. L., Amaral, D. G., Bauman, M. D., et al. (2018). Neuron numbers increase in the human amygdala from birth to adulthood, but not in autism. Proc. Natl. Acad. Sci. U S A 115, 3710–3715. doi: 10.1073/pnas.1801912115
Bahi-Buisson, N., and Bienvenu, T. (2011). CDKL5-related disorders: from clinical description to molecular genetics. Mol. Syndromol. 2, 137–152. doi: 10.1159/000331333
Baron-Cohen, S., Ring, H. A., Bullmore, E. T., Wheelwright, S., Ashwin, C., and Williams, S. C. R. (2000). The amygdala theory of autism. Neurosci. Biobehav. Rev. 24, 355–364. doi: 10.1016/s0149-7634(00)00011-7
Becker-Santos, D. D., Lonergan, K. M., Gronostajski, R. M., and Lam, W. L. (2017). Nuclear factor I/B: a master regulator of cell differentiation with paradoxical roles in cancer. EBioMedicine 22, 2–9. doi: 10.1016/j.ebiom.2017.05.027
Berchtold, N. C., Cribbs, D. H., Coleman, P. D., Rogers, J., Head, E., Kim, R., et al. (2008). Gene expression changes in the course of normal brain aging are sexually dimorphic. Proc. Natl. Acad. Sci. U S A 105, 15605–15610. doi: 10.1073/pnas.0806883105
Bergan, J. F., Ben-Shaul, Y., and Dulac, C. (2014). Sex-specific processing of social cues in the medial amygdala. eLife 3:e02743. doi: 10.7554/eLife.02743
Bonnycastle, K. (2018). Synaptic vesicle recycling in preclinical models of intellectual disability, autism spectrum disorder and epilepsy. Available online at: https://era.ed.ac.uk/handle/1842/31344. Accessed January 10, 2021.
Bowers, J. M., Perez-Pouchoulen, M., Edwards, N. S., and McCarthy, M. M. (2013). Foxp2 mediates sex differences in ultrasonic vocalization by rat pups and directs order of maternal retrieval. J. Neurosci. 33, 3276–3283. doi: 10.1523/JNEUROSCI.0425-12.2013
Bowers, J. M., Perez-Pouchoulen, M., Roby, C. R., Ryan, T. E., and McCarthy, M. M. (2014). Androgen modulation of Foxp1 and Foxp2 in the developing rat brain: impact on sex specific vocalization. Endocrinology 155, 4881–4894. doi: 10.1210/en.2014-1486
Carney, R. S. E., Mangin, J.-M., Hayes, L., Mansfield, K., Sousa, V. H., Fishell, G., et al. (2010). Sonic hedgehog expressing and responding cells generate neuronal diversity in the medial amygdala. Neural Dev. 5:14. doi: 10.1186/1749-8104-5-14
Castellucci, G. A., McGinley, M. J., and McCormick, D. A. (2016). Knockout of Foxp2 disrupts vocal development in mice. Sci. Rep. 6:23305. doi: 10.1038/srep23305
Chakrabarti, B., Dudbridge, F., Kent, L., Wheelwright, S., Hill-Cawthorne, G., Allison, C., et al. (2009). Genes related to sex steroids, neural growth and social-emotional behavior are associated with autistic traits, empathy and asperger syndrome. Autism Res. 2, 157–177. doi: 10.1002/aur.80
Chen, P., and Hong, W. (2018). Neural circuit mechanisms of social behavior. Neuron 98, 16–30. doi: 10.1016/j.neuron.2018.02.026
Chien, Y.-L., Wu, Y.-Y., Chen, H.-I., Tsai, W.-C., Chiu, Y.-N., Liu, S.-K., et al. (2017). The central nervous system patterning gene variants associated with clinical symptom severity of autism spectrum disorders. J. Formos. Med. Assoc. 116, 755–764. doi: 10.1016/j.jfma.2016.11.015
Chiu, H., Hoopfer, E. D., Coughlan, M. L., Pavlou, H. J., Goodwin, S. F., and Anderson, D. J. (2021). A circuit logic for sexually shared and dimorphic aggressive behaviors in Drosophila. Cell 184, 507–520.e16. doi: 10.1016/j.cell.2020.11.048
Choleris, E., Little, S. R., Mong, J. A., Puram, S. V., Langer, R., and Pfaff, D. W. (2007). Microparticle-based delivery of oxytocin receptor antisense DNA in the medial amygdala blocks social recognition in female mice. Proc. Natl. Acad. Sci. U S A 104, 4670–4675. doi: 10.1073/pnas.0700670104
Co, M., Anderson, A. G., and Konopka, G. (2020). FOXP transcription factors in vertebrate brain development, function and disorders. Wiley Interdiscip. Rev. Dev. Biol. 9:e375. doi: 10.1002/wdev.375
Coelho, M. C. A., Vasquez, M. L., Wildemberg, L. E., Vázquez-Borrego, M. C., Bitana, L., Camacho, A. H. da S., et al. (2019). Clinical significance of filamin a in patients with acromegaly and its association with somatostatin and dopamine receptor profiles. Sci. Rep. 9:1122. doi: 10.1038/s41598-018-37692-3
Edmonson, C., Ziats, M. N., and Rennert, O. M. (2014). Altered glial marker expression in autistic post-mortem prefrontal cortex and cerebellum. Mol. Autism 5:3. doi: 10.1186/2040-2392-5-3
Enard, W., Gehre, S., Hammerschmidt, K., Hölter, S. M., Blass, T., Somel, M., et al. (2009). A humanized version of Foxp2 affects cortico-basal ganglia circuits in mice. Cell 137, 961–971. doi: 10.1016/j.cell.2009.03.041
Ferland, R. J., Cherry, T. J., Preware, P. O., Morrisey, E. E., and Walsh, C. A. (2003). Characterization of Foxp2 and Foxp1 mRNA and protein in the developing and mature brain. J. Comp. Neurol. 460, 266–279. doi: 10.1002/cne.10654
Feuk, L., Kalervo, A., Lipsanen-Nyman, M., Skaug, J., Nakabayashi, K., Finucane, B., et al. (2006). Absence of a paternally inherited FOXP2 gene in developmental verbal dyspraxia. Am. J. Hum. Genet. 79, 965–972. doi: 10.1086/508902
Fisher, S. E., and Scharff, C. (2009). FOXP2 as a molecular window into speech and language. Trends Genet. 25, 166–177. doi: 10.1016/j.tig.2009.03.002
Franklin, K., and Paxinos, G. (2008). The Mouse Brain in Stereotaxic Coordinates, Compact, 3rd Edn. New York, NY: Academic Press.
French, C. A., and Fisher, S. E. (2014). What can mice tell us about Foxp2 function? Curr. Opin. Neurobiol. 28, 72–79. doi: 10.1016/j.conb.2014.07.003
Fujita, E., Tanabe, Y., Shiota, A., Ueda, M., Suwa, K., Momoi, M. Y., et al. (2008). Ultrasonic vocalization impairment of Foxp2 (R552H) knockin mice related to speech-language disorder and abnormality of Purkinje cells. Proc. Natl. Acad. Sci. U S A 105, 3117–3122. doi: 10.1073/pnas.0712298105
Galloway, A., Adeluyi, A., O’Donovan, B., Fisher, M. L., Rao, C. N., Critchfield, P., et al. (2018). Dopamine triggers CTCF-dependent morphological and genomic remodeling of astrocytes. J. Neurosci. 38, 4846–4858. doi: 10.1523/JNEUROSCI.3349-17.2018
Galvez-Contreras, A. Y., Zarate-Lopez, D., Torres-Chavez, A. L., and Gonzalez-Perez, O. (2020). Role of oligodendrocytes and myelin in the pathophysiology of autism spectrum disorder. Brain Sci. 10:951. doi: 10.3390/brainsci10120951
Gaub, S., Groszer, M., Fisher, S. E., and Ehret, G. (2010). The structure of innate vocalizations in Foxp2-deficient mouse pups. Genes Brain Behav. 9, 390–401. doi: 10.1111/j.1601-183X.2010.00570.x
Gauthier, J., Joober, R., Mottron, L., Laurent, S., Fuchs, M., De Kimpe, V., et al. (2003). Mutation screening of FOXP2 in individuals diagnosed with autistic disorder. Am. J. Med. Genet. A 118, 172–175. doi: 10.1002/ajmg.a.10105
Gee, J. M., Smith, N. A., Fernandez, F. R., Economo, M. N., Brunert, D., Rothermel, M., et al. (2014). Imaging Activity in Neurons and Glia with a Polr2a-Based and Cre-Dependent GCaMP5G-IRES-tdTomato Reporter Mouse. Neuron 83, 1058–1072. doi: 10.1016/j.neuron.2014.07.024
Gegenhuber, B., and Tollkuhn, J. (2020). Signatures of sex: sex differences in gene expression in the vertebrate brain. Wiley Interdiscip. Rev. Dev. Biol. 9:e348. doi: 10.1002/wdev.348
Gong, X., Jia, M., Ruan, Y., Shuang, M., Liu, J., Wu, S., et al. (2004). Association between the FOXP2 gene and autistic disorder in Chinese population. Am. J. Med. Genet. B Neuropsychiatr. Genet. 127, 113–116. doi: 10.1002/ajmg.b.20162
Grabowska, M. M., Elliott, A. D., DeGraff, D. J., Anderson, P. D., Anumanthan, G., Yamashita, H., et al. (2014). NFI transcription factors interact with FOXA1 to regulate prostate-specific gene expression. Mol. Endocrinol. 28, 949–964. doi: 10.1210/me.2013-1213
Gross, C. T., and Canteras, N. S. (2012). The many paths to fear. Nat. Rev. Neurosci. 13, 651–658. doi: 10.1038/nrn3301
Groszer, M., Keays, D. A., Deacon, R. M. J., de Bono, J. P., Prasad-Mulcare, S., Gaub, S., et al. (2008). Impaired synaptic plasticity and motor learning in mice with a point mutation implicated in human speech deficits. Curr. Biol. 18, 354–362. doi: 10.1016/j.cub.2008.01.060
Guo, H., Duyzend, M. H., Coe, B. P., Baker, C., Hoekzema, K., Gerdts, J., et al. (2019). Genome sequencing identifies multiple deleterious variants in autism patients with more severe phenotypes. Genet. Med. 21, 1611–1620. doi: 10.1038/s41436-018-0380-2
Haesler, S., Wada, K., Nshdejan, A., Morrisey, E. E., Lints, T., Jarvis, E. D., et al. (2004). FoxP2 expression in avian vocal learners and non-learners. J. Neurosci. 24, 3164–3175. doi: 10.1523/JNEUROSCI.4369-03.2004
Hashikawa, K., Hashikawa, Y., Lischinsky, J., and Lin, D. (2018). The neural mechanisms of sexually dimorphic aggressive behaviors. Trends Genet. 34, 755–776. doi: 10.1016/j.tig.2018.07.001
Herrero, M. J., Velmeshev, D., Hernandez-Pineda, D., Sethi, S., Sorrells, S., Banerjee, P., et al. (2020). Identification of amygdala-expressed genes associated with autism spectrum disorder. Mol. Autism 11:39. doi: 10.1186/s13229-020-00346-1
Hickey, S. L., Berto, S., and Konopka, G. (2019). Chromatin decondensation by FOXP2 promotes human neuron maturation and expression of neurodevelopmental disease genes. Cell Rep. 27, 1699–1711.e9. doi: 10.1016/j.celrep.2019.04.044
Hilliard, A. T., Miller, J. E., Fraley, E., Horvath, S., and White, S. A. (2012). Molecular microcircuitry underlies functional specification in a basal ganglia circuit dedicated to vocal learning. Neuron 73, 537–552. doi: 10.1016/j.neuron.2012.01.005
Hu, H.-T., Umemori, H., and Hsueh, Y.-P. (2016). Postsynaptic SDC2 induces transsynaptic signaling via FGF22 for bidirectional synaptic formation. Sci. Rep. 6:33592. doi: 10.1038/srep33592
Ishimoto, T., Ninomiya, K., Inoue, R., Koike, M., Uchiyama, Y., and Mori, H. (2017). Mice lacking BCAS1, a novel myelin-associated protein, display hypomyelination, schizophrenia-like abnormal behaviors and upregulation of inflammatory genes in the brain. Glia 65, 727–739. doi: 10.1002/glia.23129
Jin, D., Liu, H.-X., Hirai, H., Torashima, T., Nagai, T., Lopatina, O., et al. (2007). CD38 is critical for social behaviour by regulating oxytocin secretion. Nature 446, 41–45. doi: 10.1038/nature05526
Kazdoba, T. M., Leach, P. T., and Crawley, J. N. (2016). Behavioral phenotypes of genetic mouse models of autism. Genes Brain Behav. 15, 7–26. doi: 10.1111/gbb.12256
Kling, A. S., and Brothers, L. A. (1992). “The amygdala and social behavior,” in The Amygdala: Neurobiological Aspects of Emotion, Memory and Mental Dysfunction, (New York, NY: Wiley-Liss), 353–377.
Konopka, G. (2013). FOXP2: Linking Language and Autism. Available online at: https://oxfordmedicine.com/view/10.1093/med/9780199744312.001.0001/med-9780199744312-chapter-19. Accessed November 24, 2020.
Konopka, G., Bomar, J. M., Winden, K., Coppola, G., Jonsson, Z. O., Gao, F., et al. (2009). Human-specific transcriptional regulation of CNS development genes by FOXP2. Nature 462, 213–217. doi: 10.1038/nature08549
Konopka, G., Friedrich, T., Davis-Turak, J., Winden, K., Oldham, M. C., Gao, F., et al. (2012). Human-specific transcriptional networks in the brain. Neuron 75, 601–617. doi: 10.1016/j.neuron.2012.05.034
Kovač, J., Podkrajšek, K. T., Lukšič, M. M., and Battelino, T. (2015). Weak association of glyoxalase 1 (GLO1) variants with autism spectrum disorder. Eur. Child. Adolesc. Psychiatry 24, 75–82. doi: 10.1007/s00787-014-0537-8
Krishnan, A., Zhang, R., Yao, V., Theesfeld, C. L., Wong, A. K., Tadych, A., et al. (2016). Genome-wide prediction and functional characterization of the genetic basis of autism spectrum disorder. Nat. Neurosci. 19, 1454–1462. doi: 10.1038/nn.4353
Kuerbitz, J., Arnett, M., Ehrman, S., Williams, M. T., Vorhees, C. V., Fisher, S. E., et al. (2018). Loss of intercalated cells (ITCs) in the mouse amygdala of Tshz1 mutants correlates with fear, depression and social interaction phenotypes. J. Neurosci. 38, 1160–1177. doi: 10.1523/JNEUROSCI.1412-17.2017
Kumar, S. (2008). The Role of Tumour Suppressor Tyrosine Kinase SYK in Glioblastoma and Breast Cancer. München, Germany: Universitätsbibliothek der TU München.
Lai, C. S. L., Gerrelli, D., Monaco, A. P., Fisher, S. E., and Copp, A. J. (2003). FOXP2 expression during brain development coincides with adult sites of pathology in a severe speech and language disorder. Brain 126, 2455–2462. doi: 10.1093/brain/awg247
Lai, C. S., Fisher, S. E., Hurst, J. A., Vargha-Khadem, F., and Monaco, A. P. (2001). A forkhead-domain gene is mutated in a severe speech and language disorder. Nature 413, 519–523. doi: 10.1038/35097076
Laroche, F., Ramoz, N., Leroy, S., Fortin, C., Rousselot-Paillet, B., Philippe, A., et al. (2008). Polymorphisms of coding trinucleotide repeats of homeogenes in neurodevelopmental psychiatric disorders. Psychiatr. Genet. 18, 295–301. doi: 10.1097/YPG.0b013e3283060fa5
Li, G., Wang, J., Rossiter, S. J., Jones, G., and Zhang, S. (2007). Accelerated FoxP2 evolution in echolocating bats. PLoS One 2:e900. doi: 10.1371/journal.pone.0000900
Li, H., Yamagata, T., Mori, M., and Momoi, M. Y. (2005). Absence of causative mutations and presence of autism-related allele in FOXP2 in Japanese autistic patients. Brain Dev. 27, 207–210. doi: 10.1016/j.braindev.2004.06.002
Li, Y., and Dulac, C. (2018). Neural coding of sex-specific social information in the mouse brain. Curr. Opin. Neurobiol. 53, 120–130. doi: 10.1016/j.conb.2018.07.005
Lim, E. T., Uddin, M., De Rubeis, S., Chan, Y., Kamumbu, A. S., Zhang, X., et al. (2017). Rates, distribution and implications of postzygotic mosaic mutations in autism spectrum disorder. Nat. Neurosci. 20:1217. doi: 10.1038/nn.4598
Lischinsky, J. E., and Lin, D. (2020). Neural mechanisms of aggression across species. Nat. Neurosci. 23, 1317–1328. doi: 10.1038/s41593-020-00715-2
Lischinsky, J. E., Sokolowski, K., Li, P., Esumi, S., Kamal, Y., Goodrich, M., et al. (2017). Embryonic transcription factor expression in mice predicts medial amygdala neuronal identity and sex-specific responses to innate behavioral cues. eLife 6:e21012. doi: 10.7554/eLife.21012
Liu, X., Li, P., Widlak, P., Zou, H., Luo, X., Garrard, W. T., et al. (1998). The 40-kDa subunit of DNA fragmentation factor induces DNA fragmentation and chromatin condensation during apoptosis. Proc. Natl. Acad. Sci. U S A 95, 8461–8466. doi: 10.1073/pnas.95.15.8461
MacDermot, K. D., Bonora, E., Sykes, N., Coupe, A.-M., Lai, C. S. L., Vernes, S. C., et al. (2005). Identification of FOXP2 truncation as a novel cause of developmental speech and language deficits. Am. J. Hum. Genet. 76, 1074–1080. doi: 10.1086/430841
Marshall, C. R., Noor, A., Vincent, J. B., Lionel, A. C., Feuk, L., Skaug, J., et al. (2008). Structural variation of chromosomes in autism spectrum disorder. Am. J. Hum. Genet. 82, 477–488. doi: 10.1016/j.ajhg.2007.12.009
Martucci, L. L., Amar, M., Chaussenot, R., Benet, G., Bauer, O., Zélicourt, A. D., et al. (2019). A multiscale analysis in CD38−/− mice unveils major prefrontal cortex dysfunctions. FASEB J. 33, 5823–5835. doi: 10.1096/fj.201800489R
Marui, T., Koishi, S., Funatogawa, I., Yamamoto, K., Matsumoto, H., Hashimoto, O., et al. (2005). No association of FOXP2 and PTPRZ1 on 7q31 with autism from the japanese population. Neurosci. Res. 53, 91–94. doi: 10.1016/j.neures.2005.05.003
Matos, H. Y., Hernandez-Pineda, D., Charpentier, C. M., Rusk, A., Corbin, J. G., and Jones, K. S. (2020). Sex differences in biophysical signatures across molecularly defined medial amygdala neuronal subpopulations. eNeuro 7:ENEURO.0035-20.2020. doi: 10.1523/ENEURO.0035-20.2020
McFarlane, H. G., Kusek, G. K., Yang, M., Phoenix, J. L., Bolivar, V. J., Crawley, J. N., et al. (2008). Autism-like behavioral phenotypes in BTBR T+tf/J mice. Genes Brain Behav. 7, 152–163. doi: 10.1111/j.1601-183X.2007.00330.x
Medvedeva, V. (2015). Characterization of Foxp2 functions in the mouse cortex. Available online at: http://www.theses.fr/2015PA066118. Accessed November 20, 2020.
Medvedeva, V. P., Rieger, M. A., Vieth, B., Mombereau, C., Ziegenhain, C., Ghosh, T., et al. (2019). Altered social behavior in mice carrying a cortical Foxp2 deletion. Hum. Mol. Genet. 28, 701–717. doi: 10.1093/hmg/ddy372
Miller, S. M., Marcotulli, D., Shen, A., and Zweifel, L. S. (2019). Divergent medial amygdala projections regulate approach-avoidance conflict behavior. Nat. Neurosci. 22, 565–575. doi: 10.1038/s41593-019-0337-z
Morgan, A., Fisher, S. E., Scheffer, I., and Hildebrand, M. (2017). “FOXP2-related speech and language disorders,” in GeneReviews®, eds M. P. Adam, H. H. Ardinger, R. A. Pagon, S. E. Wallace, L. J. Bean, K. Stephens et al. (Seattle, WA: University of Washington).
Munnich, A., Demily, C., Frugère, L., Duwime, C., Malan, V., Barcia, G., et al. (2019). Impact of on-site clinical genetics consultations on diagnostic rate in children and young adults with autism spectrum disorder. Mol. Autism 10:33. doi: 10.1186/s13229-019-0284-2
Newbury, D. F., Bonora, E., Lamb, J. A., Fisher, S. E., Lai, C. S. L., Baird, G., et al. (2002). FOXP2 is not a major susceptibility gene for autism or specific language impairment. Am. J. Hum. Genet. 70, 1318–1327. doi: 10.1159/000516667
Nordman, J., and Li, Z. (2020). The dorsal raphe regulates the duration of attack through the medial orbitofrontal cortex and medial amygdala. eNeuro 7:ENEURO.0331-20.2020. doi: 10.1523/ENEURO.0331-20.2020
Oliveros, J. C. (2007). Venny. An interactive tool for comparing lists with Venn’s diagrams. Available online at: https://bioinfogp.cnb.csic.es/tools/venny_old/venny.php.
Perez-Riverol, Y., Csordas, A., Bai, J., Bernal-Llinares, M., Hewapathirana, S., Kundun, D. J., et al. (2019). The PRIDE database and related tools and resources in 2019: improving support for quantification data. Nucleic Acids Res. 47, 442–450. doi: 10.1093/nar/gky1106
Pinto, D., Pagnamenta, A. T., Klei, L., Anney, R., Merico, D., Regan, R., et al. (2010). Functional impact of global rare copy number variation in autism spectrum disorders. Nature 466, 368–372. doi: 10.1038/nature09146
Poulin, J.-F., Caronia, G., Hofer, C., Cui, Q., Helm, B., Ramakrishnan, C., et al. (2018). Mapping projections of molecularly defined dopamine neuron subtypes using intersectional genetic approaches. Nat. Neurosci. 21, 1260–1271. doi: 10.1038/s41593-018-0203-4
Ramos, P. S., Sajuthi, S., Langefeld, C. D., and Walker, S. J. (2012). Immune function genes CD99L2, JARID2 and TPO show association with autism spectrum disorder. Mol. Autism 3:4. doi: 10.1186/2040-2392-3-4
Ramsey, J. M., Schwarz, E., Guest, P. C., van Beveren, N. J. M., Leweke, F. M., Rothermundt, M., et al. (2012). Molecular sex differences in human serum. PLoS One 7:e51504. doi: 10.1371/journal.pone.0051504
Rousso, D. L., Qiao, M., Kagan, R. D., Yamagata, M., Palmiter, R. D., and Sanes, J. R. (2016). Two pairs of ON and OFF retinal ganglion cells are defined by intersectional patterns of transcription factor expression. Cell Rep. 15, 1930–1944. doi: 10.1016/j.celrep.2016.04.069
Sands, S. A., and Chronwall, B. M. (1996). GFAP expression induced by dopamine D(2) receptor agonists in the rat pituitary intermediate lobe. Endocrine 4, 35–42. doi: 10.1007/BF02738872
Satterstrom, F. K., Kosmicki, J. A., Wang, J., Breen, M. S., De Rubeis, S., An, J.-Y., et al. (2020). Large-scale exome sequencing study implicates both developmental and functional changes in the neurobiology of autism. Cell 180, 568.e23–584.e23. doi: 10.1016/j.cell.2019.12.036
Scharff, C., and Haesler, S. (2005). An evolutionary perspective on FoxP2: strictly for the birds. Curr. Opin. Neurobiol. 15, 694–703. doi: 10.1016/j.conb.2005.10.004
Shriberg, L. D., Ballard, K. J., Tomblin, J. B., Duffy, J. R., Odell, K. H., and Williams, C. A. (2006). Speech, prosody and voice characteristics of a mother and daughter with a 7;13 translocation affecting FOXP2. J. Speech Lang. Hear. Res. 49, 500–525. doi: 10.1044/1092-4388(2006/038)
Shu, W., Cho, J. Y., Jiang, Y., Zhang, M., Weisz, D., Elder, G. A., et al. (2005). Altered ultrasonic vocalization in mice with a disruption in the Foxp2 gene. Proc. Natl. Acad. Sci. U S A 102, 9643–9648. doi: 10.1073/pnas.0503739102
Silverman, J. L., Yang, M., Lord, C., and Crawley, J. N. (2010). Behavioural phenotyping assays for mouse models of autism. Nat. Rev. Neurosci. 11, 490–502. doi: 10.1038/nrn2851
Sokolowski, K., and Corbin, J. G. (2012). Wired for behaviors: from development to function of innate limbic system circuitry. Front. Mol. Neurosci 5:55. doi: 10.3389/fnmol.2012.00055
Sokolowski, K., Esumi, S., Hirata, T., Kamal, Y., Tran, T., Lam, A., et al. (2015). Specification of select hypothalamic circuits and innate behaviors by the embryonic patterning gene Dbx1. Neuron 86, 403–416. doi: 10.1016/j.neuron.2015.03.022
Sun, Y., Yao, X., March, M. E., Meng, X., Li, J., Wei, Z., et al. (2019). Target genes of autism risk loci in brain frontal cortex. Front. Genet. 10:707. doi: 10.3389/fgene.2019.00707
Takahashi, K., Liu, F.-C., Hirokawa, K., and Takahashi, H. (2003). Expression of Foxp2, a gene involved in speech and language, in the developing and adult striatum. J. Neurosci. Res. 73, 61–72. doi: 10.1002/jnr.10638
Tang, S., Terzic, B., Wang, I.-T. J., Sarmiento, N., Sizov, K., Cui, Y., et al. (2019). Altered NMDAR signaling underlies autistic-like features in mouse models of CDKL5 deficiency disorder. Nat. Commun. 10:2655. doi: 10.1038/s41467-019-10689-w
Um, S. M., Ha, S., Lee, H., Kim, J., Kim, K., Shin, W., et al. (2018). NGL-2 Deletion Leads to Autistic-like Behaviors Responsive to NMDAR Modulation. Cell Rep. 23, 3839–3851. doi: 10.1016/j.celrep.2018.05.087
Vargha-Khadem, F., Gadian, D. G., Copp, A., and Mishkin, M. (2005). FOXP2 and the neuroanatomy of speech and language. Nat. Rev. Neurosci. 6, 131–138. doi: 10.1038/nrn1605
Vargha-Khadem, F., Watkins, K. E., Price, C. J., Ashburner, J., Alcock, K. J., Connelly, A., et al. (1998). Neural basis of an inherited speech and language disorder. Proc. Natl. Acad. Sci. U S A 95, 12695–12700. doi: 10.1073/pnas.95.21.12695
Wang, K., Zhang, H., Ma, D., Bucan, M., Glessner, J. T., Abrahams, B. S., et al. (2009). Common genetic variants on 5p14.1 associate with autism spectrum disorders. Nature 459, 528–533. doi: 10.1038/nature07999
Wang, L., Almeida, L. E. F., Spornick, N. A., Kenyon, N., Kamimura, S., Khaibullina, A., et al. (2015). Modulation of social deficits and repetitive behaviors in a mouse model of autism: the role of the nicotinic cholinergic system. Psychopharmacology (Berl) 232, 4303–4316. doi: 10.1007/s00213-015-4058-z
Weiss, L. A., Shen, Y., Korn, J. M., Arking, D. E., Miller, D. T., Fossdal, R., et al. (2008). Association between microdeletion and microduplication at 16p11.2 and autism. N. Engl. J. Med. 358, 667–675. doi: 10.1056/NEJMoa075974
Wijchers, P. J. E. C., Hoekman, M. F. M., Burbach, J. P. H., and Smidt, M. P. (2006). Identification of forkhead transcription factors in cortical and dopaminergic areas of the adult murine brain. Brain Res. 1068, 23–33. doi: 10.1016/j.brainres.2005.11.022
Yang, J., Chen, Y., Xiong, X., Zhou, X., Han, L., Ni, L., et al. (2018). Peptidome analysis reveals novel serum biomarkers for children with autism spectrum disorder in china. Proteomics Clin. Appl. 12:e1700164. doi: 10.1002/prca.201700164
Yang, M., and Crawley, J. N. (2009). Simple behavioral assessment of mouse olfaction. Curr. Protoc. Neurosci. 8:24. doi: 10.1002/0471142301.ns0824s48
Yochum, C. L., Bhattacharya, P., Patti, L., Mirochnitchenko, O., and Wagner, G. C. (2010). Animal model of autism using GSTM1 knockout mice and early post-natal sodium valproate treatment. Behav. Brain Res. 210, 202–210. doi: 10.1016/j.bbr.2010.02.032
Keywords: Foxp2, medial amygdala (MeA), social behavior, aggression, sex-specific differences, proteomics
Citation: Herrero MJ, Wang L, Hernandez-Pineda D, Banerjee P, Matos HY, Goodrich M, Panigrahi A, Smith NA and Corbin JG (2021) Sex-Specific Social Behavior and Amygdala Proteomic Deficits in Foxp2+/– Mutant Mice. Front. Behav. Neurosci. 15:706079. doi: 10.3389/fnbeh.2021.706079
Received: 06 May 2021; Accepted: 29 June 2021;
Published: 05 August 2021.
Edited by:
James Cherry, Boston University, United StatesReviewed by:
Brian Trainor, University of California, Davis, United StatesMaarten Van Den Buuse, La Trobe University, Australia
Copyright © 2021 Herrero, Wang, Hernandez-Pineda, Banerjee, Matos, Goodrich, Panigrahi, Smith and Corbin. This is an open-access article distributed under the terms of the Creative Commons Attribution License (CC BY). The use, distribution or reproduction in other forums is permitted, provided the original author(s) and the copyright owner(s) are credited and that the original publication in this journal is cited, in accordance with accepted academic practice. No use, distribution or reproduction is permitted which does not comply with these terms.
*Correspondence: Li Wang, bHdhbmdAY2hpbGRyZW5zbmF0aW9uYWwub3Jn; Joshua G. Corbin, amNvcmJpbkBjaGlsZHJlbnNuYXRpb25hbC5vcmc=
† These authors have contributed equally to this work