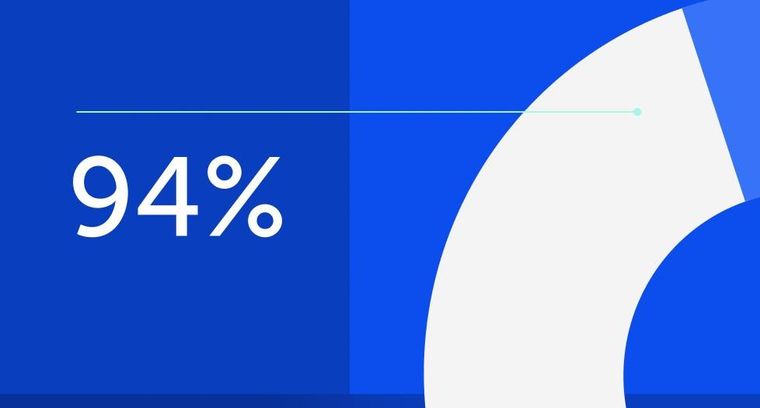
94% of researchers rate our articles as excellent or good
Learn more about the work of our research integrity team to safeguard the quality of each article we publish.
Find out more
REVIEW article
Front. Behav. Neurosci., 04 June 2021
Sec. Individual and Social Behaviors
Volume 15 - 2021 | https://doi.org/10.3389/fnbeh.2021.683780
This article is part of the Research TopicHome Cage-based Phenotyping in Rodents: Innovation, Standardization, Reproducibility and Translational ImprovementView all 24 articles
The use of animal models for substance use disorder (SUD) has made an important contribution in the investigation of the behavioral and molecular mechanisms underlying substance abuse and addiction. Here, we review a novel and comprehensive behavioral platform to characterize addiction-like traits in rodents using a fully automated learning system, the IntelliCage. This system simultaneously captures the basic behavioral navigation, reward preference, and aversion, as well as the multi-dimensional complex behaviors and cognitive functions of group-housed rodents. It can reliably capture and track locomotor and cognitive pattern alterations associated with the development of substance addiction. Thus, the IntelliCage learning system offers a potentially efficient, flexible, and sensitive tool for the high-throughput screening of the rodent SUD model.
Understanding the neural mechanisms of complex human behaviors and the behavioral anomalies accompanying neurobiological disorders, including substance use disorder (SUD; or substance addiction), represents one of the most formidable challenges in behavioral and cognitive neuroscience research at present (Lynch et al., 2010; Gulinello et al., 2019; Kuhn et al., 2019). The past decade has seen a resurgence of studies using laboratory rodents, coupled with an impressive array of genetic modifications, providing unprecedented opportunities to generate suitable rodent models to research human pathologies. In contrast, behavioral assays, and their application to large numbers of animals, trailed behind in terms of throughput if compared with genetic advances for rodent models. Notwithstanding, there is a growing need for reliable and robust high-throughput behavioral assessment platforms to elucidate the cognitive and behavioral performances in both wild-type and transgenic rodent strains.
To address this issue, we focus on the IntelliCage system, a home-cage-based rodent behavioral assessment platform, and specifically, its utility to investigate the neurobehavioral underpinnings of SUD in rodent models. This fully automated live-in environment approach helps eliminate the confounding effects and considerable stress from environmental and experimental variables that may obscure the behavioral measures. Owed to its low-dependency on human interference, the IntelliCage system also enables investigators to monitor the multi-dimensional processes in group-housed mice regulated over longer time scales in a straightforward, time-, and cost-effective manner (Lipp, 2005; Lipp et al., 2005; Spruijt and DeVisser, 2006; Wolfer et al., 2012; Kiryk et al., 2020). Such an approach may not only improve throughput, but also provide new insights into the regulation of rodent behaviors that is not as practical with conventional behavioral assays.
In this narrative review, we provide an overview on several commonly employed animal models of SUD, describe the IntelliCage system apparatus and its application in modeling human neurological disorders, and provide in-depth reviews of the related SUD studies utilizing the IntelliCage in the assessment of multi-symptomatic animal physiology, behaviors, and cognitive functions.
Substance use disorder is a chronically relapsing disorder characterized by compulsive and uncontrollable substance-seeking and use, which persists even in the face of negative consequences (Koob and Volkow, 2010, 2016; Uhl et al., 2019). Animal models of SUD are recognized as indispensable tools in defining our current knowledge of the neurobiology and pathophysiology of addiction, and the neuropharmacological aspects of substances of abuse (Koob, 2014; Venniro et al., 2016; Wingo et al., 2016; Müller, 2018; Kuhn et al., 2019). Although animal models may not fully emulate and reproduce the complex human experience, they nevertheless provide means for the researchers to conduct addiction research under highly controlled conditions that may not be possible or ethical to replicate in humans. Earlier animal models of SUD emphasized on the use of operant paradigms in non-human primates and the mechanisms of acute reward. However, recently, these paradigms have been extrapolated and utilized in small rodents (namely, laboratory mouse and rat). Current research has also shifted to include consequent neuroadaptations in long-term or chronic substance abuse paradigms. The use of rodent models, together with the recent advancement, has provided significant new knowledge and understanding in the neurobiology of SUD.
The behavioral sensitization model (i.e., experimenter-administered drug exposure) has been extensively used to assess drug-induced locomotor changes, and to identify key reward-related neurobiological substrates and the underlying neuroplasticity (Steketee and Kalivas, 2011; Kuhn et al., 2019). The model involves a progressive increase in the motor stimulatory effects that occur with a repeated, intermittent exposure to a specific drug. Depending on the experimenter’s timeline, sensitization can be rapidly induced to study the short-term drug-induced changes and/or long-term effects of chronic drug exposure. The development of behavioral sensitization has been hypothesized to represent a transition from drug “liking” to “wanting” that underlies compulsive substance use as reported to occur in response to morphine (Cheaha et al., 2017), amphetamine (Ridzwan et al., 2017), alcohol (Mitra and Nagaraja, 2020), nicotine, cocaine, and cannabinoids (Steketee and Kalivas, 2011; Venniro et al., 2016; Iman et al., 2017; Müller, 2018; Kuhn et al., 2019).
Current animal models of SUD emphasize on the addictive drugs actions as positive reinforcing stimuli, much like food, water, and other “natural” reinforcers. Laboratory animals can voluntarily self-administer these addictive substances leading to intoxication, which mimics the drug-taking behaviors seen in human addicts (Panlilio and Goldberg, 2007; Kuhn et al., 2019). In a commonly used paradigm, the animals (typically a mouse, rat, or monkey) are trained in an operant chamber to obtain a drug reward for short daily sessions (1–3 h), and even up to several months in a more complex chronic drug training. Drug delivery is made dependent on the performance of either a fixed or progressive ratio operant response; typically, lever press or nose-poke is used in rodents. Compared to the other models of SUD, these procedures provide the most likely representations with addictive behavior that occurs in the natural environment, as evidenced by the short-, intermittent, and/or long-access to emulate drug-taking and drug-seeking experimental designs. Hence, this self-administration paradigm has a high degree of face validity and is considered to be the gold standard in examining the reinforcing properties of addictive substances in rodents (Panlilio and Goldberg, 2007; Koob, 2014; Kuhn et al., 2019). Furthermore, this close correspondence allows the details of the procedure to be modified in a variety of ways to model specific aspects of addiction. The behavior observed is also highly sensitive to the manipulations of specific environmental and pharmacological variables. Thus, this makes the self-administration paradigm a suitable test for a better understanding of the factors to model drug seeking behavior leading to addiction, and they can also provide a means of testing potential therapeutic agents with anti-addictive properties or even evaluate the abuse potential of novel psychotropic candidates (Panlilio and Goldberg, 2007; Lynch et al., 2010; Spanagel, 2017).
Conditioned Place Preference (CPP) paradigm is a behavioral model commonly used to study the rewarding and/or aversive effects of natural and pharmacological stimuli, a learned behavior shown in many vertebrates, including humans (Huston et al., 2013). Although various designs and apparatuses are used to model CPP, the fundamental characteristic of this task involves the classical conditioning procedure where a particular environmental setting is associated with drug exposure, followed by the association of a different environment with the absence of the drug (or drug vehicle). After several environmental pairings, the drug-free animal is allowed to freely access both ends of the CPP paradigm, where the time spent in each environment will be measured. Theoretically, when addicted, the animals will exhibit a CPP for the environment paired with the drug reward that functions as a positive-reinforcer (i.e., spend more time in drug-paired vs non-drug environment) and avoid those that induce aversive states [i.e., conditioned place aversion (CPA)], frequently cued by a foot-shock punishment. This procedure permits the assessment of the conditioning of drug reinforcement, and provides information regarding the positive and negative reinforcing effects of drugs besides being relatively easy, quick, economical, and reproducible (Aris et al., 2012; Huston et al., 2013; Koob, 2014). Commonly abused substances such as morphine (Gibula-Tarlowska et al., 2019), cannabis (Clasen et al., 2017), amphetamines (Bardgett et al., 2020), cocaine (Carmack et al., 2013), nicotine (Muldoon et al., 2020), ethanol (Campos-Jurado et al., 2020), and 3,4-methylenedioxymethamphetamine (MDMA; Rodríguez-Arias et al., 2013) have been shown to readily establish a CPP and CPA paradigm in rodents. This paradigm is also considered a common and useful screening tool to assess the abuse liability of novel drugs due to its relative ease, economic, and reproducible set-ups (Huston et al., 2013).
The IntelliCage system (Figure 1) is a social-group environment developed by Hans-Peter Lipp and colleagues of the University of Zurich, Switzerland primarily for the use of Neural Plasticity & Repair, National Centre for Competence in Research (NCCR) research groups (Lipp, 2005; Lipp et al., 2005; Kiryk et al., 2020). IntelliCage is the first fully automated cage system designed for the assessment of spontaneous activity, spatial learning, memory, and cognitive abilities of rodents living in social groups. It allows the individual recording of the long-term and multi-dimensional behavioral patterns of up to 16 animals simultaneously. Various experimental paradigms and protocols can be freely programmed and executed with this system, thus, allowing maximum flexibility in the experimental design. Data are recorded while the animals are housed in the IntelliCage system, which provides considerably more information for analysis compared to any conventional method. The IntelliCage system was designed to circumvent practical issues often encountered with the standard behavioral test paradigms (as summarized in Table 1). The automated generation and collection of data by standardized procedures allow for high data comparability and reproducibility between labs thereafter, permitting a reduced number of animal replications needed to obtain reliable findings. This system also minimizes the need for human or experimenter’s handling, thus, reducing external artifacts that interfere with the animals’ activities throughout the desired period of monitoring (Lipp, 2005; Lipp et al., 2005; Kiryk et al., 2020).
Figure 1. An overview of the IntelliCage system. (A) The IntelliCage apparatus. (B) The IntelliCage apparatus is connected to a computer-based software used to design various behavioral protocols, as well as to measure and analyze mice behavioral patterns. (C) The motorized doors at each IntelliCage corner chamber which control access to water bottle nipples. (D) Schematic illustration of the IntelliCage. (E) Summary of IntelliCage parameters modified from Iman et al. (2017).
The IntelliCage system is a standard polycarbonate cage (55 cm width × 38 cm depth × 21 cm height) equipped with four triangular operant test chambers (15 × 15 × 21) fitted at each corner (Figure 1D). Animals are identified by the individual subcutaneously injected radio-frequency identification (RFID) tags (known as microtransponder; size: 12 mm × 2 mm) before being released into the IntelliCage system. Entry into each operant chamber is via the ring antenna which detects the animal’s unique RFID tags and records their visits. The round apertures on the walls of each chamber provide free access to water bottles. To date, mice have been reported to be supplied with tap water, sweetened water as a natural reward (i.e., sucrose or saccharin) (Radwanska and Kaczmarek, 2012; Iman et al., 2017; Heinla et al., 2018), aversive liquid (quinine solution) (Knapska et al., 2013; Smutek et al., 2014), or diluted liquid drug rewards (Radwanska and Kaczmarek, 2012; Marut et al., 2017; Skupio et al., 2017; Ajonijebu et al., 2018, 2019; Heinla et al., 2018). Small motorized doors at the aperture can be programmed too close to limit water access according to mice identification, time constraint, and conditioned action. Mice can be trained to perform a fixed or progressive ratio of nose-pokes at the door to allow access to water. The amount of liquid consumed is precisely measured by a lick-o-meter, while nose-pokes are measured by dedicated sensors. Three colored LEDs above the door in each corner provide visual cues. Aversive stimuli, or aversive reinforcement, employ the effective use of bitter tasting solution in one corner or brief air-puffs directed to the head of the mouse, therefore, eliminating the need for a more aggressive, painful, and fear-inducing stimulus (i.e., foot shock, vibration, loud noises). Four small triangular-shaped shelters are placed at the middle of the cage as a form of enrichment on which the mice could climb to reach for food (ad libitum). Shelters are red and transparent, but mice see red color as black so they are willing to hide inside which allows for their observation. The IntelliCage system also provides a continuous recording of the ambient variables (such as temperature and illumination) (Galsworthy et al., 2005; Lipp, 2005; Lipp et al., 2005).
The ability and efficiency for longitudinal and high-throughput behavioral monitoring allow researchers to develop animal models of human disorders using the IntelliCage system. In the last decade, increasingly sophisticated and specialized IntelliCage protocols had been employed and validated to characterize mouse models for Huntington’s disease (HD), Alzheimer’s disease (AD), Down syndrome, SUD, autism spectrum disorder (ASD), and other neurological and neuropsychiatric disorders (summarized in Table 2).
Table 2. Summary of selected studies using the IntelliCage system to model human neurological disorders.
Furthermore, a modified and adjusted prototype of the IntelliCage system for rats has recently been tested with transgenic HD (Urbach et al., 2014) and valproate-induced autistic-like rats (Pelsöczi et al., 2020). The automated phenotyping using the IntelliCage and Phenomaster systems for rats successfully replicated the previously described behavioral phenotypes from conventional tests, and traced the novel physiological and behavioral aspects of transgenic HD rats, including circadian activity, anxiety, and rearing (Urbach et al., 2014). In addition, Pelsöczi et al. (2020) had successfully demonstrated the disrupted locomotion, circadian activity, and social hierarchy in a rat model of ASD, further indicating the IntelliCage system’s reliability and validity to measure rat ethological and activated behaviors. While extensive validations of the IntelliCage protocols for mice models have been reported thus far, its validation development seems slower for rat models. Rats have been described to commonly show more cautious locomotor exploration and avoidance features when placed in open field and maze-testing paradigms (Bertoglio and Carobrez, 2000; Alstott and Timberlake, 2009), thereby, could limit the interpretation using the IntelliCage data and warrants further extensive validation to merit a wider acceptance for research use.
Substantial evidence has documented and recognized the practicality and effectiveness of the IntelliCage system for the short-term and/or long-term cognitive assessment of group-housed rodents. Earlier experiments with the IntelliCage system demonstrated its value for measuring spontaneous and simple conditioned behaviors. Ensuing studies developed and tested numerous parameters/protocols for the assessment of rodent social behaviors and cognitive functions, including spontaneous behavior and spatial navigation, learning and memory-related tasks, circadian activities, and place/drug preference or avoidance tasks. See Kiryk et al. (2020) for a more comprehensive description of the IntelliCage system parameters and protocols developed by approximately 80 research groups on a wide spectrum of rodent behaviors, to date.
Spontaneous behavior, or free exploration, is considered as the mandatory first-stage assessment of rodent in the IntelliCage system; during which, all drink bottles are always freely accessible for approximately one week. The free exploration paradigm provides a unique opportunity to systematically assess novel and general environment exploration, and provides an initial screen for neophobia, habituation, gross motor deficit, coordination, and cognitive states in rodents. A dynamic representation of these states are indispensable for establishing individual baselines and detecting behavioral anomalies as the indicators of the animal’s general well-being, health, and emotional state in an unrestricted open-field environment (Bailey and Crawley, 2009; Fonio et al., 2009; Jirkof, 2014; Hohlbaum et al., 2018), as provided with the IntelliCage system. One of the early works by Galsworthy et al. (2005) explored simple exploratory behaviors and learning paradigms between two sympatric wild-caught rodent species (i.e., wood mice and bank voles). Parameters included were initial exploration during the first 90 min of introduction into a novel arena, total habituated activity levels throughout the subsequent 8 days (based on the number of corner visits and water consumption), and circadian patterns. This study acknowledged the IntelliCage system as a valuable behavioral testing module for both wild and in-laboratory rodents, as well as for inter-species comparison (Galsworthy et al., 2005).
The IntelliCage system is reportedly efficient for the long-term monitoring of female mice, while males may eventually require supplementary compartment barriers, housing about three males (Lipp et al., 2005). Small enrichment shelters were then added to the system design to limit any male aggressive or stressful behaviors that may confound the behavioral analysis. However, many ensuing studies use females for their phenotyping strategies in an attempt to avoid male aggression and dominance issues typical in social-grouped mice (Kiryk et al., 2020), thereby, creating a potential female bias and overlooking the potential sex differences (Weber et al., 2017). Thus, the underrepresentation of males in the IntelliCage system research must not be disregarded to improve scientific validity.
In another study, the IntelliCage system statistically revealed the indistinguishable differences in standardized inter-laboratory tests of exploration and activity parameters of F1 B6D2, C57BL/6, and DBA/2 mice, compared to the open-field, elevated Null-maze, water maze, and object exploration tests (Lipp et al., 2005). Safi et al. (2006) successfully adapted a simple Vogel water-lick paradigm in the IntelliCage system to assess anxiety and anxiolytic drug effects of the control and Diazepam-treated C57BL/6 female mice. The study reported an efficient and robust analysis of the individual behavioral parameters indicative of anxiety elicited by an aversive stimulus (i.e., number and duration of visits, licks, and nose-pokes following air-puff punished visit) (Safi et al., 2006).
Knapska et al. (2006) tested the system for place preference (by the acquisition of sweetened water at a specific corner) and avoidance (by avoiding a corner associated with air-puff) tasks to balance aversive versus appetitive conditioning effects within the central amygdala of C57BL/6 female mice. Further refinement of the balanced appetitive/aversive training has been provided by the study by Knapska et al. (2013), in which discrimination learning between sweetened water vs. bitter-tasting water provided in the two bottles in one corner was compared. The IntelliCage system has also been used to investigate mouse physiology and behavioral phenotypes in various mouse models as part of the spontaneous behavior and spatial navigation (Goulding et al., 2008; Jaholkowski et al., 2009; Mechan et al., 2009; Krackow et al., 2010).
The most commonly used learning and memory-related protocol in the IntelliCage system is spatial/place learning for a specific IntelliCage corner associated with liquid reward. Thus far, results from the IntelliCage system, in the realm of cognitive and learning/memory functions, are parallel with those from standard behavioral assays, including Morris water maze and fear-conditioning tests (Kiryk et al., 2008; Konopka et al., 2010; Faizi et al., 2011; Vogel et al., 2020). A study by Onishchenko et al. (2007) focused on the long-term learning and memory effects of developmental exposure to methylmercury (MeHg) in pregnant C57BL/6 mice. The IntelliCage system was tested for spatial learning (learn to find water-rewarded corner) and reversal learning (learn to find newly placed water-rewarded corner) and patrolling behaviors. In the patrolling protocol, the water-reinforced corner was pre-programmed to change in a clockwise manner after each visit. Thus, mice had to learn to patrol to find the correct water-accessed corners, cued with a green LED light. This patrolling learning protocol in the IntelliCage system entails the involvement of mice visual discrimination, reference, and working memory challenge. This study also provided evidence that the IntelliCage system is more sensitive in the detection of behavioral alterations and learning paradigms in comparison to the Morris water maze, rotarod test, and forced swimming test (Onishchenko et al., 2007). The absence of mice social deprivation and any human interference with the IntelliCage system use may be the contributing factor to the sensitivity of the assessment.
More complex learning and memory protocols, including goal-directed behaviors (Gapp et al., 2014), serial reversal task (Endo et al., 2011; Kobayashi et al., 2013), chaining, and patrolling (Kobayashi et al., 2013), have also been successfully designed and employed using the IntelliCage system.
Systematic phenotyping of rodent models in automated home-cage systems is presently receiving considerable attention as an effective means of monitoring general and complex activity parameters, as well as detecting perturbations in the neural circuitry function. These complex tasks are achieved while eliminating the tedious and error prone bias of human assessment over extended periods, allowing researchers to address and recognize larger arrays of behavioral outputs than those traditionally assayed. Indeed, the automated home-cage monitoring is a promising frontier for improving translational neurobehavioral research in rodents (Jhuang et al., 2010; Mingrone et al., 2020; Voikar and Gaburro, 2020), including in SUD models. In addition to the IntelliCage system, there are several other automated home-cage monitoring systems available at present (as summarized in Table 3).
Table 3. Summary of the available home-cage monitoring systems and their use in SUD in rodent models.
Thus far, a growing body of literature has attested the practical utilities and importance of the IntelliCage system in addiction-related mouse models, primarily in alcoholism research. Each IntelliCage corner chamber permits a voluntary oral consumption of liquid reward (via nose-poke), which is useful for self-administration paradigms, as well as the application of operant and Pavlovian conditioning tasks for studying the rewarding properties of various substances of abuse.
The IntelliCage system allows for mimicking different aspects of human behavior to meet the addiction criteria defined in the Diagnostic and Statistical Manual V (DSM-V) of the American Psychiatric Association (Hasin et al., 2013). The DSM-V recognizes that individuals are not all equally vulnerable to developing SUD, and that SUD is a pattern of symptoms. DSM-V sets a diagnostic threshold of 2 or more out of 11 criteria to be met. The IntelliCage allows for examining the following criteria: withdrawal, tolerance, craving, amount of consumption, and time spent on seeking. By analyzing these measures, it is possible to differentiate animals in terms of level of compulsive drinking into low and high drinkers (Radwanska and Kaczmarek, 2012; Stefaniuk et al., 2017; Beroun et al., 2018; Skóra et al., 2020). Radwanska and Kaczmarek (2012) designed the first longitudinal study of animal models of addiction using extensive IntelliCage system parameters in BALB/cJ and C57BL/6 male mice. The study successfully elucidated the behavioral traits associated with alcohol addiction, such as: (i) novelty-seeking (number of corner visits in the novel IntelliCage system); (ii) impulsivity (inability to withhold nose-pokes at rewarding corners); (iii) anxiety (suppression of reward consumption at air-puff associated corners); (iv) motivation and persistence for natural reward (i.e., 10% sucrose); (v) withdrawal; and (vi) relapse in mice for a span of 128 days. This study corroborated the IntelliCage system as a reliable tool for an efficient, high-throughput screening of mice addiction-prone behavioral traits. The data suggested that high levels of anxiety-related traits (i.e., low novelty-seeking, low resistance to punishment, increased compulsivity and impulsivity) predicted addiction-like alcohol drinking in mice (Radwanska and Kaczmarek, 2012). Parkitna et al. (2013) later adapted this alcohol abuse model for a 3-month assessment of ethanol self-administration, abstinence, circadian pattern of chronic ethanol consumption, and cue-induced alcohol relapse. Ensuing studies utilized the IntelliCage system paradigms to develop more complex learning and memory procedures in alcohol addiction models, including intermittent-access schedule (Smutek et al., 2014; Koskela et al., 2018), delay-discounting impulsivity (Szumiec and Parkitna, 2016), motivation for alcohol-seeking behaviors (Stefaniuk et al., 2017), alcohol-deprivation-induced effects (Thomsen et al., 2017), and cue-induced conditioning procedures (Koskela et al., 2018).
Additionally, the IntelliCage system has been used for oral morphine self-administration (0.1–0.5 mg/ml) in a progressive ratio nose-pokes, with the co-administration of dexamethasone [a selective glucocorticoid receptor (GR) agonist], and CPP paradigms to evaluate the GR effects on the rewarding properties of morphine in mice. This model represents a novel approach for investigating the behavioral and molecular mechanisms underlying opioid addiction (Marut et al., 2017). In a follow-up study, Skupio et al. (2017) evaluated mice compulsive morphine self-administration features, including progressive ratio nose-pokes, intermittent-access schedule, enhanced resistance to punishment, withdrawal, and reinstatement of morphine-seeking behaviors, for over 100 days. More recently, to induce and assess symptoms of compulsive cocaine intake, similar paradigms were adapted to the IntelliCage by Ajonijebu et al. (2018). Compared with the control animals, cocaine-addicted C57BL/6J female mice exhibited a higher preference for natural reward and failure to discriminate rewarded from non-rewarded corners, suggestive of significant learning deficits with a prolonged cocaine exposure (Ajonijebu et al., 2018, 2019).
Overall, considering the flexible task design and longitudinal monitoring in a social cage environment, the IntelliCage system indicates invaluable and promising abilities to be a novel model for short-term and long-term SUD studies for other substances of abuse. Therefore, based on this knowledge, our laboratory had successfully designed a new protocol (Iman et al., 2017), which was an adaptation from Radwanska and Kaczmarek’s mice alcohol addiction model (Radwanska and Kaczmarek, 2012), for the study of extended behavioral and cognitive effects of socially interacting Swiss albino mice chronically exposed to the widely abused substances, i.e., morphine, Δ-9-tetrahydrocannabinol (THC), and mitragynine, a major alkaloid of Thai medicinal plant, kratom or Mitragyna speciosa Korth leaves, with psychostimulant and opioid-like properties (Suwanlert, 1975; Ahmad and Aziz, 2012; Hassan et al., 2013; Saingam et al., 2013; Iman et al., 2017). In brief, data collected from our IntelliCage sensitization model (Iman et al., 2017) effectively presented the behavioral and cognitive impairment evoked by the chronic administration of morphine, THC, and mitragynine, which are consistent with the reports from previous studies using conventional animal addiction assays (Justinova et al., 2009; Lu et al., 2010; O’Brien et al., 2013; Harvey-Lewis et al., 2015; Yusoff et al., 2016; Vanderschuren et al., 2017; Hassan et al., 2019).
In addition, the water bottles and programmable conditioning corners in the IntelliCage system would allow researchers to study hedonic behavior and multitudes of spatial learning and memory function in drug-addicted rodents, as well as compulsive behaviors after being punished with an air-puff. Different colored LEDs feature can be utilized to study cue-induced drug memory. The system also differs from conventional tests of SUD in that it examines behavior over an extended period of time. Nevertheless, it is important to note that the main drawbacks of the IntelliCage system include its upfront setting-up costs, regular maintenance, absence of visual tracking of in-cage behavior and social interaction, as well as male aggression issue in socially grouped rodents. However, extracting together the current data and the analysis from previous addiction-related IntelliCage studies, we can assert that the IntelliCage system provides an effective and reliable platform to detect and characterize addiction-related behavioral phenotypes of rodent, chiefly mice, in a social dimension.
In summary, current findings from the SUD mouse model characterize the IntelliCage system as a biologically valid, sensitive, and efficient system in the phenotypic detection of drug effects concurrently across multiple behavioral measures. Moreover, the IntelliCage system permits the assessments of behavior in a controlled environment for socially grouped rodents that minimizes human investigators’ interference. Concurrently, a fully valid model of substance addiction in the IntelliCage system can be further refined with more complex conditioning tasks and parameters, to complement other conventional behavioral assays. Thus, this platform can be beneficial in eliminating the bottleneck in rodent behavioral addiction studies. More importantly, it expands the opportunities to design better preclinical models of SUD to further elucidate the neurobiological mechanisms that contribute to addiction-related behaviors, as well as discovering related treatment options.
II, NY, and MMu conceptualized, drafted, and revised the manuscript. UT and NA contributed to the animal models of the SUD section. AN, JK, MMe, NJ, SM, MS, LK, and MMu critically reviewed the manuscript. All authors made substantial contribution to the review and approved the final manuscript.
This work was part of the research supported by Universiti Sains Malaysia (USM) Short Term Research grant (304/PPSP/6315252) and Research University grant (1001/PPSP/8012300) schemes awarded to MMu and NY. JK was supported by Universiti Kebangsaan Malaysia (UKM) Dana Fundamental (FF-2019-098) and MS was supported by a National Science Centre grant (2019/35/B/NZ4/04077).
The authors declare that the research was conducted in the absence of any commercial or financial relationships that could be construed as a potential conflict of interest.
II holds a scholarship from the Public Service Department of Malaysia.
Ahmad, K., and Aziz, Z. (2012). Mitragyna speciosa use in the northern states of Malaysia: a cross-sectional study. J. Ethnopharmacol. 141, 446–450. doi: 10.1016/j.jep.2012.03.009
Ajonijebu, D. C., Abboussi, O., Mabandla, M. V., and Daniels, W. M. (2018). Differential epigenetic changes in the hippocampus and prefrontal cortex of female mice that had free access to cocaine. Metab. Brain Dis. 33, 411–420. doi: 10.1007/s11011-017-0116-z
Ajonijebu, D. C., Abboussi, O., Mabandla, M. V., and Daniels, W. M. (2019). Cocaine-induced inheritable epigenetic marks may be altered by changing early postnatal fostering. Neuro Rep. 30, 1157–1165. doi: 10.1097/wnr.0000000000001332
Alstott, J., and Timberlake, W. (2009). Effects of rat sex differences and lighting on locomotor exploration of a circular open field with free-standing central corners and without peripheral walls. Behav. Brain Res. 196, 214–219. doi: 10.1016/j.bbr.2008.09.001
Aris, M., Mohd Moklas, M. A., Amom, Z., Taufik, M., Baharuldin, H., Mat Taib, C. N., et al. (2012). Chronic delta-9-tetrahydrocannabinol induces monoamine release but not conditioned place preference. Open Behav. Sci. J. 6, 31–36. doi: 10.2174/1874230001206010031
Bailey, K. R., and Crawley, J. N. (2009). Anxiety-Related Behaviors in Mice, 2nd Edn. Francis, Boca Raton (FL): CRC Press/Taylor &.
Balci, F., Oakeshott, S., Shamy, J. L., El-Khodor, B. F., Filippov, I., Mushlin, R., et al. (2013). High-throughput automated phenotyping of two genetic mouse models of Huntington’s disease. PLoS Curr. 5, 1–29. doi: 10.1371/currents.hd.124aa0d16753f88215776fba102ceb29
Bardgett, M. E., Downnen, T., Crane, C., Baltes Thompson, E. C., Muncie, B., Steffen, S. A., et al. (2020). Chronic risperidone administration leads to greater amphetamine-induced conditioned place preference. Neuropharmacology 179:108276. doi: 10.1016/j.neuropharm.2020.108276
Beroun, A., Nalberczak-Skóra, M., Harda, Z., Piechota, M., Ziółkowska, M., Cały, A., et al. (2018). Generation of silent synapses in dentate gyrus correlates with development of alcohol addiction. Neuropsychopharmacology 43, 1989–1999. doi: 10.1038/s41386-018-0119-4
Bertoglio, L. J., and Carobrez, A. P. (2000). Previous maze experience required to increase open arms avoidance in rats submitted to the elevated plus-maze model of anxiety. Behav. Brain Res. 108, 197–203. doi: 10.1016/S0166-4328(99)00148-5
Campos-Jurado, Y., Martí-Prats, L., Morón, J. A., Polache, A., Granero, L., and Hipólito, L. (2020). Dose-dependent induction of CPP or CPA by intra-pVTA ethanol: Role of mu opioid receptors and effects on NMDA receptors. Prog. Neuro Psychopharmacol. Biol. Psychiatry 100:109875. doi: 10.1016/j.pnpbp.2020.109875
Carmack, S. A., Kim, J. S., Sage, J. R., Thomas, A. W., Skillicorn, K. N., and Anagnostaras, S. G. (2013). The competitive NMDA receptor antagonist CPP disrupts cocaine-induced conditioned place preference, but spares behavioral sensitization. Behav. Brain Rese. 239, 155–163. doi: 10.1016/j.bbr.2012.10.042
Cheaha, D., Reakkamnuan, C., Nukitram, J., Chittrakarn, S., Phukpattaranont, P., Keawpradub, N., et al. (2017). Effects of alkaloid-rich extract from Mitragyna speciosa (Korth.) Havil. on naloxone-precipitated morphine withdrawal symptoms and local field potential in the nucleus accumbens of mice. J. Ethnopharmacol. 208, 129–137. doi: 10.1016/j.jep.2017.07.008
Clasen, M. M., Flax, S. M., Hempel, B. J., Cheng, K., Rice, K. C., and Riley, A. L. (2017). Antagonism of the kappa opioid receptor attenuates THC-induced place aversions in adult male Sprague-Dawley rats. Pharmacol. Biochem. Behav. 163, 30–35. doi: 10.1016/j.pbb.2017.10.010
Codita, A., Gumucio, A., Lannfelt, L., Gellerfors, P., Winblad, B., Mohammed, A. H., et al. (2010). Impaired behavior of female tg-ArcSwe APP mice in the IntelliCage: a longitudinal study. Behav. Brain Res. 215, 83–94. doi: 10.1016/j.bbr.2010.06.034
Endo, T., Maekawa, F., Võikar, V., Haijima, A., Uemura, Y., Zhang, Y., et al. (2011). Automated test of behavioral flexibility in mice using a behavioral sequencing task in IntelliCage. Behav. Brain Res. 221, 172–181. doi: 10.1016/j.bbr.2011.02.037
Faizi, M., Bader, P. L., Tun, C., Encarnacion, A., Kleschevnikov, A., Belichenko, P., et al. (2011). Comprehensive behavioral phenotyping of Ts65Dn mouse model of Down syndrome: activation of β1-adrenergic receptor by xamoterol as a potential cognitive enhancer. Neurobiol. Dis. 43, 397–413. doi: 10.1016/j.nbd.2011.04.011
Fischer, M., Cabello, V., Popp, S., Krackow, S., Hommers, L., Deckert, J., et al. (2017). Rsk2 knockout affects emotional behavior in the IntelliCage. Behav. Genet. 47, 434–448. doi: 10.1007/s10519-017-9853-3
Fonio, E., Benjamini, Y., and Golani, I. (2009). Freedom of movement and the stability of its unfolding in free exploration of mice. Proc. Natl. Acad. Sci. U.S.A. 106, 21335–21340. doi: 10.1073/pnas.0812513106
Galsworthy, M. J., Amrein, I., Kuptsov, P. A., Poletaeva, I. I., Zinn, P., Rau, A., et al. (2005). A comparison of wild-caught wood mice and bank voles in the Intellicage: assessing exploration, daily activity patterns and place learning paradigms. Behav. Brain Res. 157, 211–217. doi: 10.1016/j.bbr.2004.06.021
Gapp, K., Soldado-Magraner, S., Alvarez-Sánchez, M., Bohacek, J., Vernaz, G., Shu, H., et al. (2014). Early life stress in fathers improves behavioural flexibility in their offspring. Nat. Commun. 5:5466. doi: 10.1038/ncomms6466
Gibula-Tarlowska, E., Kedzierska, E., Piechura, K., Silberring, J., and Kotlinska, J. H. (2019). The influence of a new derivate of kisspeptin-10 – Kissorphin (KSO) on the rewarding effects of morphine in the conditioned place preference (CPP) test in male rats. Behav. Brain Res. 372:112043. doi: 10.1016/j.bbr.2019.112043
Goulding, E. H., Schenk, A. K., Juneja, P., MacKay, A. W., Wade, J. M., and Tecott, L. H. (2008). A robust automated system elucidates mouse home cage behavioral structure. Proc. Natl. Acad. Sci. U.S.A. 105, 20575–20582. doi: 10.1073/pnas.0809053106
Gulinello, M., Mitchell, H. A., Chang, Q., O’Brien, W. T., Zhou, Z., Abel, T., et al. (2019). Rigor and reproducibility in rodent behavioral research. Neurobiol. Learn. Memory 165:106780. doi: 10.1016/j.nlm.2018.01.001
Guo, H., Xie, Q., Cui, J., Xu, D., Deji, C., Chen, Y., et al. (2019). Naloxone reversed cognitive impairments induced by repeated morphine under heavy perceptual load in the 5-choice serial reaction time task. J. Neurosci. Res. 97, 1051–1065. doi: 10.1002/jnr.24427
Harvey-Lewis, C., Brisebois, A. D., Yong, H., and Franklin, K. B. (2015). Naloxone-precipitated withdrawal causes an increase in impulsivity in morphine-dependent rats. Behav. Pharmacol. 26, 326–329. doi: 10.1097/fbp.0000000000000106
Hasin, D. S., O’Brien, C. P., Auriacombe, M., Borges, G., Bucholz, K., Budney, A., et al. (2013). DSM-5 criteria for substance use disorders: recommendations and rationale. Am. J. Psychiatry 170, 834–851. doi: 10.1176/appi.ajp.2013.12060782
Hassan, Z., Muzaimi, M., Navaratnam, V., Yusoff, N. H., Suhaimi, F. W., Vadivelu, R., et al. (2013). From Kratom to mitragynine and its derivatives: physiological and behavioural effects related to use, abuse, and addiction. Neurosci. Biobehav. Rev. 37, 138–151. doi: 10.1016/j.neubiorev.2012.11.012
Hassan, Z., Suhaimi, F. W., Ramanathan, S., Ling, K.-H., Effendy, M. A., Müller, C. P., et al. (2019). Mitragynine (Kratom) impairs spatial learning and hippocampal synaptic transmission in rats. J. Psychopharmacol. 33, 908–918. doi: 10.1177/0269881119844186
Hay, E. A., McEwan, A., Wilson, D., Barrett, P., D’Agostino, G., Pertwee, R. G., et al. (2019). Disruption of an enhancer associated with addictive behaviour within the cannabinoid receptor-1 gene suggests a possible role in alcohol intake, cannabinoid response and anxiety-related behaviour. Psychoneuroendocrinology 109:104407. doi: 10.1016/j.psyneuen.2019.104407
Heinla, I., Åhlgren, J., Vasar, E., and Voikar, V. (2018). Behavioural characterization of C57BL/6N and BALB/c female mice in social home cage – Effect of mixed housing in complex environment. Physiol. Behav. 188, 32–41. doi: 10.1016/j.physbeh.2018.01.024
Hohlbaum, K., Bert, B., Dietze, S., Palme, R., Fink, H., and Thöne-Reineke, C. (2018). Systematic assessment of well-being in mice for procedures using general anesthesia. J. Vis. Exp. 133:57046.
Holgate, J. Y., Garcia, H., Chatterjee, S., and Bartlett, S. E. (2017). Social and environmental enrichment has different effects on ethanol and sucrose consumption in mice. Brain Behav. 7:e00767. doi: 10.1002/brb3.767
Huston, J. P., Silva, M. A., Topic, B., and Müller, C. P. (2013). What’s conditioned in conditioned place preference? Trends Pharmacol. Sci. 34, 162–166. doi: 10.1016/j.tips.2013.01.004
Iman, N. W. I., Nanthini, J., Mansor, S. M., Müller, C. P., and Muzaimi, M. (2017). Chronic mitragynine (kratom) enhances punishment resistance in natural reward seeking and impairs place learning in mice. Addiction Biol. 22, 967–976. doi: 10.1111/adb.12385
Jaholkowski, P., Kiryk, A., Jedynak, P., Ben Abdallah, N. M., Knapska, E., Kowalczyk, A., et al. (2009). New hippocampal neurons are not obligatory for memory formation; cyclin D2 knockout mice with no adult brain neurogenesis show learning. Learn. Memory 16, 439–451. doi: 10.1101/lm.1459709
Jhuang, H., Garrote, E., Yu, X., Khilnani, V., Poggio, T., Steele, A. D., et al. (2010). Automated home-cage behavioural phenotyping of mice. Nat. Commun. 1:68.
Jirkof, P. (2014). Burrowing and nest building behavior as indicators of well-being in mice. J. Neurosci. Methods 234, 139–146. doi: 10.1016/j.jneumeth.2014.02.001
Justinova, Z., Panlilio, L. V., and Goldberg, S. R. (2009). “Drug addiction,” in Behavioral Neurobiology of the Endocannabinoid System, eds D. Kendall and S. Alexander (Berlin: Springer), 309–346.
Kiryk, A., Aida, T., Tanaka, K., Banerjee, P., Wilczynski, G. M., Meyza, K., et al. (2008). Behavioral characterization of GLT1 (+/-) mice as a model of mild glutamatergic hyperfunction. Neurotoxicity Res. 13, 19–30. doi: 10.1007/BF03033364
Kiryk, A., Janusz, A., Zglinicki, B., Turkes, E., Knapska, E., Konopka, W., et al. (2020). IntelliCage as a tool for measuring mouse behavior–20 years perspective. Behav. Brain Res. 388:112620. doi: 10.1016/j.bbr.2020.112620
Kiryk, A., Mochol, G., K Filipkowski, R., Wawrzyniak, M., Lioudyno, V., Knapska, E., et al. (2011). Cognitive abilities of Alzheimer’s disease transgenic mice are modulated by social context and circadian rhythm. Curr. Alzheimer Res. 8, 883–892. doi: 10.2174/156720511798192745
Knapska, E., Lioudyno, V., Kiryk, A., Mikosz, M., Górkiewicz, T., Michaluk, P., et al. (2013). Reward learning requires activity of matrix metalloproteinase-9 in the central amygdala. J. Neurosci. 33, 14591–14600. doi: 10.1523/JNEUROSCI.5239-12.2013
Knapska, E., Walasek, G., Nikolaev, E., Neuhäusser-Wespy, F., Lipp, H.-P., Kaczmarek, L., et al. (2006). Differential involvement of the central amygdala in appetitive versus aversive learning. Learn. Memory 13, 192–200. doi: 10.1101/lm.54706
Kobayashi, Y., Sano, Y., Vannoni, E., Goto, H., Ikeda, T., Suzuki, H., et al. (2013). Genetic dissection of medial habenula–interpeduncular nucleus pathway function in mice. Front. Behav. Neurosci. 7:17. doi: 10.3389/fnbeh.2013.00017
Konopka, W., Kiryk, A., Novak, M., Herwerth, M., Parkitna, J. R., Wawrzyniak, M., et al. (2010). MicroRNA loss enhances learning and memory in mice. J. Neurosci. 30, 14835–14842. doi: 10.1523/jneurosci.3030-10.2010
Koob, G. F. (2014). “Neurocircuitry of alcohol addiction: Synthesis from animal models,” in Handbook of Clinical Neurology, Chap. 3, eds E. V. Sullivan and A. Pfefferbaum (Amsterdam: Elsevier), 33–54. doi: 10.1016/b978-0-444-62619-6.00003-3
Koob, G. F., and Volkow, N. D. (2010). Neurocircuitry of addiction. Neuropsychopharmacology 35, 217–238. doi: 10.1038/npp.2009.110
Koob, G. F., and Volkow, N. D. (2016). Neurobiology of addiction: a neurocircuitry analysis. Lancet Psychiatry 3, 760–773. doi: 10.1016/S2215-0366(16)00104-8
Koskela, M., Piepponen, T. P., Andressoo, J.-O., Võikar, V., and Airavaara, M. (2018). Towards developing a model to study alcohol drinking and craving in female mice housed in automated cages. Behav. Brain Res. 352, 116–124. doi: 10.1016/j.bbr.2018.03.027
Krackow, S., Vannoni, E., Codita, A., Mohammed, A. H., Cirulli, F., Branchi, I., et al. (2010). Consistent behavioral phenotype differences between inbred mouse strains in the IntelliCage. Genes Brain Behav. 9, 722–731. doi: 10.1111/j.1601-183x.2010.00606.x
Kuhn, B. N., Kalivas, P. W., and Bobadilla, A.-C. (2019). Understanding addiction using animal models. Front. Behav. Neurosci. 13:262. doi: 10.3389/fnbeh.2019.00262
Lan, W. C. J., Priestley, M., Mayoral, S. R., Tian, L., Shamloo, M., and Penn, A. A. (2011). Sex-specific cognitive deficits and regional brain volume loss in mice exposed to chronic, sublethal hypoxia. Pediatric Res. 70, 15–20. doi: 10.1203/PDR.0b013e31821b98a3
Lipp, H. P. (2005). High-throughput and automated behavioural screening of normal and genetically modified mice. Business Briefing Future Drug Discov. 8, 1–5.
Lipp, H. P., Litvin, O., Galsworthy, M., Vyssotski, D. L., Vyssotski, A. L., Zinn, P., et al. (2005). “Automated behavioral analysis of mice using INTELLICAGE: Inter-laboratory comparisons and validation with exploratory behavior and spatial learning,” in Proceedings of the 5th International Conference on Methods and Techniques in Behavioral Research, eds L. Noldus, F. Grieco, L. Loijens, and P. Zimmermann (Wageningen).
Lu, G., Zhou, Q.-X., Kang, S., Li, Q.-L., Zhao, L.-C., Chen, J.-D., et al. (2010). Chronic morphine treatment impaired hippocampal long-term potentiation and spatial memory via accumulation of extracellular adenosine acting on adenosine A1 receptors. J. Neurosci. 30, 5058–5070. doi: 10.1523/jneurosci.0148-10.2010
Lynch, W. J., Nicholson, K. L., Dance, M. E., Morgan, R. W., and Foley, P. L. (2010). Animal models of substance abuse and addiction: implications for science, animal welfare, and society. Comp. Med. 60, 177–188.
Marut, L., Skupio, U., and Przewłocki, R. (2017). Glucocorticoid receptor regulates rewarding properties of morphine. Eur. Neuropsychopharmacol. 27:S1062. doi: 10.1016/S0924-977X(17)31852-7
Masuda, A., Kobayashi, Y., Kogo, N., Saito, T., Saido, T. C., and Itohara, S. (2016). Cognitive deficits in single App knock-in mouse models. Neurobiol. Learn. Memory 135, 73–82. doi: 10.1016/j.nlm.2016.07.001
McGonigle, C. E., Nentwig, T. B., Wilson, D. E., Rhinehart, E. M., and Grisel, J. E. (2016). β-endorphin regulates alcohol consumption induced by exercise restriction in female mice. Alcohol 53, 51–60. doi: 10.1016/j.alcohol.2016.04.003
Mechan, A. O., Wyss, A., Rieger, H., and Mohajeri, M. H. (2009). A comparison of learning and memory characteristics of young and middle-aged wild-type mice in the IntelliCage. J. Neurosci. Methods 180, 43–51. doi: 10.1016/j.jneumeth.2009.02.018
Menalled, L. B., Kudwa, A. E., Miller, S., Fitzpatrick, J., Watson-Johnson, J., Keating, N., et al. (2012). Comprehensive behavioral and molecular characterization of a new knock-in mouse model of Huntington’s disease: zQ175. PLoS One 7:e49838. doi: 10.1371/journal.pone.0049838
Mingrone, A., Kaffman, A., and Kaffman, A. (2020). The promise of automated home-cage monitoring in improving translational utility of psychiatric research in rodents. Front. Neurosci. 14:618593. doi: 10.3389/fnins.2020.618593
Mitjans, M., Begemann, M., Ju, A., Dere, E., Wüstefeld, L., Hofer, S., et al. (2017). Sexual dimorphism of AMBRA1-related autistic features in human and mouse. Transl. Psychiatry 7:e1247. doi: 10.1038/tp.2017.213
Mitra, N. K., and Nagaraja, H. (2020). Effect of chronic ethanol exposure on the count of cerebellar Purkinje cells and motor coordination in adult mice. Eur. J. Anatomy 12, 67–71.
Muldoon, P. P., Akinola, L. S., Schlosburg, J. E., Lichtman, A. H., Sim-Selley, L. J., Mahadevan, A., et al. (2020). Inhibition of monoacylglycerol lipase reduces nicotine reward in the conditioned place preference test in male mice. Neuropharmacology 176:108170. doi: 10.1016/j.neuropharm.2020.108170
Müller, C. P. (2018). Animal models of psychoactive drug use and addiction: present problems and future needs for translational approaches. Behav. Brain Res. 352, 109–115. doi: 10.1016/j.bbr.2017.06.028
Muthuraju, S., Taha, S., Pati, S., Rafique, M., Jaafar, H., and Abdullah, J. M. (2013). Normabaric hyperoxia treatment improved locomotor activity of C57BL/6J mice through enhancing dopamine genes following fluid-percussion injury in striatum. Int. J. Biomed. Sci. IJBS 9:194.
O’Brien, L. D., Wills, K. L., Segsworth, B., Dashney, B., Rock, E. M., Limebeer, C. L., et al. (2013). Effect of chronic exposure to rimonabant and phytocannabinoids on anxiety-like behavior and saccharin palatability. Pharmacol. Biochem. Behav. 103, 597–602. doi: 10.1016/j.pbb.2012.10.008
Onishchenko, N., Tamm, C., Vahter, M., Hökfelt, T., Johnson, J. A., Johnson, D. A., et al. (2007). Developmental exposure to methylmercury alters learning and induces depression-like behavior in male mice. Toxicol. Sci. 97, 428–437. doi: 10.1093/toxsci/kfl199
Panlilio, L. V., and Goldberg, S. R. (2007). Self-administration of drugs in animals and humans as a model and an investigative tool. Addiction 102, 1863–1870. doi: 10.1111/j.1360-0443.2007.02011.x
Parkitna, J. R., Sikora, M., Gołda, S., Gołembiowska, K., Bystrowska, B., Engblom, D., et al. (2013). Novelty-seeking behaviors and the escalation of alcohol drinking after abstinence in mice are controlled by metabotropic glutamate receptor 5 on neurons expressing dopamine D1 receptors. Biol. Psychiatry 73, 263–270. doi: 10.1016/j.biopsych.2012.07.019
Pelsöczi, P., Kelemen, K., Csölle, C., Nagy, G., Lendvai, B., Román, V., et al. (2020). Disrupted social hierarchy in prenatally valproate-exposed autistic-like rats. Front. Behav. Neurosci. 13:295. doi: 10.3389/fnbeh.2019.00295
Puścian, A., Łęski, S., Górkiewicz, T., Meyza, K., Lipp, H. P., and Knapska, E. (2014). A novel automated behavioral test battery assessing cognitive rigidity in two genetic mouse models of autism. Front. Behav. Neurosci. 8:140. doi: 10.3389/fnbeh.2014.00140
Pushkin, A. N., Eugene, A. J., Lallai, V., Torres-Mendoza, A., Fowler, J., Chen, E., et al. (2019). Cannabinoid and nicotine exposure during adolescence induces sex-specific effects on anxiety-and reward-related behaviors during adulthood. PLoS One 14:e0211346. doi: 10.1371/journal.pone.0211346
Radwanska, K., and Kaczmarek, L. (2012). Characterization of an alcohol addiction-prone phenotype in mice. Addiction Biol. 17, 601–612. doi: 10.1111/j.1369-1600.2011.00394.x
Ridzwan, I. E., Suhaimi, M. S., Wasli, N. S., Kasmuri, A. R., Azzubaidi, M. S., Hashim, R., et al. (2017). The distinct role of kappa opioid receptor in attenuating relapse to morphine/methamphetamine (polydrug) dependence in mice. Br. J. Pharm. 2, 21–22.
Rodríguez-Arias, M., Valverde, O., Daza-Losada, M., Blanco-Gandía, M. C., Aguilar, M. A., and Miñarro, J. (2013). Assessment of the abuse potential of MDMA in the conditioned place preference paradigm: role of CB1 receptors. Prog. Neuro Psychopharmacol. Biol. Psychiatry 47, 77–84. doi: 10.1016/j.pnpbp.2013.07.013
Rudenko, O., Tkach, V., Berezin, V., and Bock, E. (2009). Detection of early behavioral markers of Huntington’s disease in R6/2 mice employing an automated social home cage. Behav. Brain Res. 203, 188–199. doi: 10.1016/j.bbr.2009.04.034
Safi, K., Neuhüusser-Wespy, F., Welzl, H., and Lipp, H.-P. (2006). Mouse anxiety models and an example of an experimental setup using unconditioned avoidance in an automated system - IntelliCage. Cogn. Creier Comportament Cogn. Brain, Behav. 10, 475–488.
Saingam, D., Assanangkornchai, S., Geater, A. F., and Balthip, Q. (2013). Pattern and consequences of krathom (Mitragyna speciosa Korth.) use among male villagers in southern Thailand: a qualitative study. Int. J. Drug Policy 24, 351–358. doi: 10.1016/j.drugpo.2012.09.004
Sekiguchi, K., Imamura, S., Yamaguchi, T., Tabuchi, M., Kanno, H., Terawaki, K., et al. (2011). Effects of yokukansan and donepezil on learning disturbance and aggressiveness induced by intracerebroventricular injection of amyloid β protein in mice. Phytother. Res. 25, 501–507. doi: 10.1002/ptr.3287
Skóra, M. N., Pattij, T., Beroun, A., Kogias, G., Mielenz, D., de Vries, T., et al. (2020). Personality driven alcohol and drug abuse: new mechanisms revealed. Neurosci. Biobehav. Rev. 116, 64–73. doi: 10.1016/j.neubiorev.2020.06.023
Skupio, U., Sikora, M., Korostynski, M., Wawrzczak-Bargiela, A., Piechota, M., Ficek, J., et al. (2017). Behavioral and transcriptional patterns of protracted opioid self-administration in mice. Addiction Biol. 22, 1802–1816. doi: 10.1111/adb.12449
Smutek, M., Turbasa, M., Sikora, M., Piechota, M., Zajdel, J., Przewlocki, R., et al. (2014). A model of alcohol drinking under an intermittent access schedule using group-housed mice. PLoS One 9:e96787. doi: 10.1371/journal.pone.0096787
Spruijt, B. M., and DeVisser, L. (2006). Advanced behavioural screening: automated home cage ethology. Drug Discov. Today Technol. 3, 231–237. doi: 10.1016/j.ddtec.2006.06.010
Stefaniuk, M., Beroun, A., Lebitko, T., Markina, O., Leski, S., Meyza, K., et al. (2017). Matrix metalloproteinase-9 and synaptic plasticity in the central amygdala in control of alcohol-seeking behavior. Biol. Psychiatry 81, 907–917. doi: 10.1016/j.biopsych.2016.12.026
Steketee, J. D., and Kalivas, P. W. (2011). Drug wanting: Behavioral sensitization and relapse to drug-seeking behavior. Pharmacol. Rev. 63, 348–365. doi: 10.1124/pr.109.001933
Szumiec, L., and Parkitna, J. R. (2016). A new model of impulsivity, social interactions and preference of alcohol in mice living in groups. Eur. Neuropsychopharmacol. 2:S270. doi: 10.1016/S0924-977X(16)31153-1
Thomsen, M., Dencker, D., Wörtwein, G., Weikop, P., Egecioglu, E., Jerlhag, E., et al. (2017). The glucagon-like peptide 1 receptor agonist Exendin-4 decreases relapse-like drinking in socially housed mice. Pharmacol. Biochem. Behav. 160, 14–20. doi: 10.1016/j.pbb.2017.07.014
Too, L. K., Ball, H. J., McGregor, I. S., and Hunt, N. H. (2014). A novel automated test battery reveals enduring behavioural alterations and cognitive impairments in survivors of murine pneumococcal meningitis. Brain Behav. Immunity 35, 107–124. doi: 10.1016/j.bbi.2013.09.007
Too, L. K., Li, K. M., Suarna, C., Maghzal, G. J., Stocker, R., McGregor, I. S., et al. (2016a). Deletion of TDO2, IDO-1 and IDO-2 differentially affects mouse behavior and cognitive function. Behav. Brain Res. 312, 102–117. doi: 10.1016/j.bbr.2016.06.018
Too, L. K., McGregor, I. S., Baxter, A. G., and Hunt, N. H. (2016b). Altered behaviour and cognitive function following combined deletion of Toll-like receptors 2 and 4 in mice. Behav. Brain Res. 303, 1–8. doi: 10.1016/j.bbr.2016.01.024
Too, L. K., Yau, B., Baxter, A. G., McGregor, I. S., and Hunt, N. H. (2019). Double deficiency of toll-like receptors 2 and 4 alters long-term neurological sequelae in mice cured of pneumococcal meningitis. Sci. Rep. 9, 1–13.
Uhl, G. R., Koob, G. F., and Cable, J. (2019). The neurobiology of addiction. Ann. N. Y. Acad. Sci. 1451:5. doi: 10.1111/nyas.13989
Urbach, Y. K., Raber, K. A., Canneva, F., Plank, A. C., Andreasson, T., Ponten, H., et al. (2014). Automated phenotyping and advanced data mining exemplified in rats transgenic for Huntington’s disease. J. Neurosci. Methods 234, 38–53. doi: 10.1016/j.jneumeth.2014.06.017
van Gurp, S., Hoog, J., Kalenscher, T., and van Wingerden, M. (2020). Vicarious reward unblocks associative learning about novel cues in male rats. Elife 9:e60755.
Vanderschuren, L. J. M. J., Minnaard, A. M., Smeets, J. A. S., and Lesscher, H. M. B. (2017). Punishment models of addictive behavior. Curr. Opin. Behav. Sci. 13, 77–84. doi: 10.1016/j.cobeha.2016.10.007
Venniro, M., Caprioli, D., and Shaham, Y. (2016). Animal models of drug relapse and craving: from drug priming-induced reinstatement to incubation of craving after voluntary abstinence. Prog. Brain Res. 224, 25–52. doi: 10.1016/bs.pbr.2015.08.004
Vogel, A., Wilken-Schmitz, A., Hummel, R., Lang, M., Gurke, R., Schreiber, Y., et al. (2020). Low brain endocannabinoids associated with persistent non-goal directed nighttime hyperactivity after traumatic brain injury in mice. Sci. Rep. 10, 1–22.
Voikar, V., and Gaburro, S. (2020). Three pillars of automated home-cage phenotyping of mice: novel findings, refinement, and reproducibility based on literature and experience. Front. Behav. Neurosci. 14:575434. doi: 10.3389/fnbeh.2020.575434
Weber, E. M., Dallaire, J. A., Gaskill, B. N., Pritchett-Corning, K. R., and Garner, J. P. (2017). Aggression in group-housed laboratory mice: why can’t we solve the problem? Lab Animal 46, 157–161. doi: 10.1038/laban.1219
Wingo, T., Nesil, T., Choi, J.-S., and Li, M. D. (2016). Novelty-seeking and drug addiction in humans and animals: from behavior to molecules. J. Neuroimmune Pharmacol. 11, 456–470. doi: 10.1007/s11481-015-9636-7
Wolfer, D. P., Võikar, V., Vannoni, E., Colacicco, G., and Lipp, H. P. (2012). “Mouse phenotyping in the IntelliCage: from spontaneous behavior to cognitive function,” in Proceedings of the 8th Measuring Behavior Conference 2012, (Utrecht).
Yusoff, N. H. M., Suhaimi, F. W., Vadivelu, R. K., Hassan, Z., Rümler, A., Rotter, A., et al. (2016). Abuse potential and adverse cognitive effects of mitragynine (kratom). Addiction Biol. 21, 98–110. doi: 10.1111/adb.12185
Keywords: IntelliCage system, substance use disorder (SUD), addiction, rodent model, behavior, home cage
Citation: Iman IN, Yusof NAM, Talib UN, Ahmad NAZ, Norazit A, Kumar J, Mehat MZ, Jayabalan N, Muthuraju S, Stefaniuk M, Kaczmarek L and Muzaimi M (2021) The IntelliCage System: A Review of Its Utility as a Novel Behavioral Platform for a Rodent Model of Substance Use Disorder. Front. Behav. Neurosci. 15:683780. doi: 10.3389/fnbeh.2021.683780
Received: 22 March 2021; Accepted: 05 May 2021;
Published: 04 June 2021.
Edited by:
Stefano Gaburro, Independent Researcher, Buguggiate, ItalyReviewed by:
Lars Lewejohann, Freie Universität Berlin, GermanyCopyright © 2021 Iman, Yusof, Talib, Ahmad, Norazit, Kumar, Mehat, Jayabalan, Muthuraju, Stefaniuk, Kaczmarek and Muzaimi. This is an open-access article distributed under the terms of the Creative Commons Attribution License (CC BY). The use, distribution or reproduction in other forums is permitted, provided the original author(s) and the copyright owner(s) are credited and that the original publication in this journal is cited, in accordance with accepted academic practice. No use, distribution or reproduction is permitted which does not comply with these terms.
*Correspondence: Mustapha Muzaimi, bW11emFpbWlAdXNtLm15
†These authors share first authorship
Disclaimer: All claims expressed in this article are solely those of the authors and do not necessarily represent those of their affiliated organizations, or those of the publisher, the editors and the reviewers. Any product that may be evaluated in this article or claim that may be made by its manufacturer is not guaranteed or endorsed by the publisher.
Research integrity at Frontiers
Learn more about the work of our research integrity team to safeguard the quality of each article we publish.