- Department of Psychiatry and Behavioral Sciences, Stanford University School of Medicine, Stanford, CA, United States
Sleep is fundamental to life, and poor sleep quality is linked to the suboptimal function of the neural circuits that process and respond to emotional stimuli. Wakefulness (“arousal”) is chiefly regulated by circadian and homeostatic forces, but affective mood states also strongly impact the balance between sleep and wake. Considering the bidirectional relationships between sleep/wake changes and emotional dynamics, we use the term “emotional arousal” as a representative characteristic of the profound overlap between brain pathways that: (1) modulate wakefulness; (2) interpret emotional information; and (3) calibrate motivated behaviors. Interestingly, many emotional arousal circuits communicate using specialized signaling molecules called neuropeptides to broadly modify neural network activities. One major neuropeptide-enriched brain region that is critical for emotional processing and has been recently implicated in sleep regulation is the bed nuclei of stria terminalis (BNST), a core component of the extended amygdala (an anatomical term that also includes the central and medial amygdalae, nucleus accumbens shell, and transition zones betwixt). The BNST encompasses an astonishing diversity of cell types that differ across many features including spatial organization, molecular signature, biological sex and hormonal milieu, synaptic input, axonal output, neurophysiological communication mode, and functional role. Given this tremendous complexity, comprehensive elucidation of the BNST neuropeptide circuit mechanisms underlying emotional arousal presents an ambitious set of challenges. In this review, we describe how rigorous investigation of these unresolved questions may reveal key insights to enhancing psychiatric treatments and global psychological wellbeing.
Introduction
Precise control of wakefulness (“arousal”) is essential for generating the adaptive forms of reward-seeking and stress resilience that encourage healthy survival (Tsujino and Sakurai, 2009; Eban-Rothschild et al., 2017). Thus, neuronal wakefulness systems were shaped by evolution to confer high sensitivity for detecting, interpreting, and acting upon emotional stimuli. Healthy sleep/wake cycles are essential for optimal cognition and emotion, and poor sleep quality can lead to deleterious changes in the physiological function of brain circuits that gate behavioral responses to emotional stimuli (Koob and Colrain, 2020). In mental health conditions of addiction, anxiety, and depression, maladaptive responses to hedonically-valenced stimuli are linked to distinct activity patterns in brain arousal pathways. Indeed, stress is a major factor driving insomnia (inability to sleep), and various sleep-related disturbances are common among individuals enduring stress-related psychiatric conditions. On the other hand, experiencing pleasure and anticipating future reward can also extend wakefulness and prevent healthy sleep, highlighting the ability of both positive and negative hedonically-valenced stimuli to shift the thresholds of arousal (Eban-Rothschild et al., 2018). A further fascinating example of the link between emotion and arousal is the sleep disorder narcolepsy with cataplexy, in which powerful feelings of euphoria or aversion can interrupt wakefulness by triggering rapid intrusion of a sleep-like state (Adamantidis et al., 2020). These profound neuroscientific mysteries hint at the commonalities among (and/or interactions between) brain pathways that calibrate wakefulness, process emotional information, and generate motivated behaviors.
Intriguingly, many emotional arousal circuits use specialized modulatory signaling molecules called neuropeptides to fine-tune the coordination of broad neural network activity (Ryabinin et al., 2012; Schank et al., 2012; Giardino and de Lecea, 2014; Kash et al., 2015; Li et al., 2017). One major neuropeptide-enriched emotional processing network is the extended amygdala (an anatomical term referring to neurons spanning the bed nuclei of stria terminalis (BNST), central and medial amygdalae (CeA, MeA), nucleus accumbens shell (NAcSh), and the transition zones betwixt; Alheid, 2003). While communication via neuropeptide signaling likely allows the BNST to perform sophisticated control of emotional arousal circuitry, the primary mechanisms underlying changes in synthesis, storage, and release of peptide neuromodulators from BNST neurons remain largely undescribed. This is due in part to the complex patterns of more than 10 discrete neuropeptides that are distributed in varying combinations of multi-neuropeptide co-expression amongst up to forty unique cellular subpopulations (Moffitt et al., 2018; Welch et al., 2019; Rodriguez-Romaguera et al., 2020). The BNST encompasses a particularly astonishing diversity of cell types that differ along spectrums of several features, including spatial organization, molecular signature, biological sex and hormonal milieu, synaptic input, axonal output, neurophysiological messaging, and functional role (Kash et al., 2015; Lebow and Chen, 2016; Vranjkovic et al., 2017; Ch’ng et al., 2018; Beyeler and Dabrowska, 2020).
Historically, an all-encompassing framework for the BNST cell groups and connections driving emotional behaviors was limited by existing pharmacological and neurochemical approaches. Recent advances in genetic, optical, and computational tools for mapping, manipulating, and monitoring brain activity have revolutionized functional annotation of behavioral neurocircuits (Saunders et al., 2015; Nectow and Nestler, 2020; Xia and Kheirbek, 2020). Nevertheless, comprehensive elucidation of the BNST neuropeptide mechanisms underlying emotional arousal presents an ambitious set of challenges. In this review, we describe how rigorous investigation of these unresolved questions may reveal key insights to enhancing psychiatric treatments and global psychological wellbeing.
Spatially-Defined BNST Cell Types
The BNST is a ventromedial forebrain complex surrounded on all sides by the hypothalamus, thalamus, striatum, septum, and lateral ventricles. Given the wide-ranging descriptions of “BNST”, we primarily discuss the multiple distinct neuronal populations corresponding to those encompassed within adult mouse (Mus musculus) brain stereotaxic coordinates approximately +0.45 to −0.35 mm anterior/posterior (A/P), 0.40 to 1.20 mm medial/lateral (M/L) bilaterally off the midline, and −4.0 to −5.0 mm dorsal/ventral (D/V). Various systems of nomenclature have been proposed for labeling unique BNST subcompartments, but the classification of BNST cellular populations based solely on spatial location remains unstandardized and highly subjective (Bota et al., 2012; Lebow and Chen, 2016; Barbier et al., 2021). This persisting lack of consensus for definitive BNST spatial subdivisions reflects the challenges faced by early anatomists, who first divided BNST on the M/L axis, only to be challenged by developmental biologists who inferred a predominantly A/P axis, followed by synaptic physiologists, neurochemists, and others who emphasized a D/V axis (corresponding to divergent patterns of monoaminergic innervation, for example; De Olmos and Ingram, 1972; Krettek and Price, 1978; Weller and Smith, 1982; Bayer and Altman, 1987; Dong et al., 2000; Egli and Winder, 2003; Bota et al., 2012; McElligott et al., 2013; Radley and Johnson, 2018). Although functional associations with BNST divisions across each of the anatomical axes have sparked valuable hypotheses, variability in the degree to which unique BNST features differ across distinct spatial dimensions limits the holistic impact of relying solely on such descriptors to functionally parcellate the BNST.
For example, the term “ventral BNST” commonly refers to neurons located directly ventral to (beneath) the anterior commissure (a prominent white matter tract that forms a wide horizontal band when viewed in the coronal plane). However, pioneering neuroanatomists acknowledged more than 30 years ago that, while the commissure may be a useful landmark for dividing general areas of the BNST, “it does not necessarily always define strict cytoarchitectonic boundaries, since a component of the dorsal area may well be separated and come to lie in the ventral area” (Ju and Swanson, 1989). In other words, the commissure forms a wide horizontal shape only at certain points along the rodent BNST A/P axis, and the commissure’s departure from view in the caudal BNST reveals contiguous cellular populations that may have been “divided” on a D/V axis in rostral sections purely incidentally. Indeed, Ju and Swanson (1989) noted that “Immunohistochemical studies with antisera to several peptides also indicate very similar staining patterns within these (D/V) regions. It seems clear to us, therefore, that the anterior commissure simply passes through the BNST” (Ju and Swanson, 1989).
Upon eschewing the commissure as a monolithic landmark, Swanson and colleagues identified at least five different BNST cellular populations residing ventrally to the commissure (Ju and Swanson, 1989; Ju et al., 1989; Dong et al., 2001a,b; Dong and Swanson, 2003, 2004a,b, 2006a,b,c). Although they originally used the abbreviation “vBNST” to refer only to a particular ventralmost subnucleus within the ventral BNST complex (Ju and Swanson, 1989; Ju et al., 1989; Dong et al., 2000, 2001a,b; Dong and Swanson, 2003, 2004a,b, 2006a,b,c), modern widespread usage of the term “vBNST” generally translates to “ventral BNST writ large”, and the commissure remains a major dividing line for ascribing any readily identifiable characteristics that may be distinguished along the D/V BNST axis. Supplementary Table 1 displays the incongruity of stereotaxic coordinates used to target the mouse “ventral BNST” in recent behavioral neuroscience publications, reflecting the limitations of relying on ambiguous spatial descriptors (Jennings et al., 2013b; Dedic et al., 2018; Kim et al., 2018; Hardaway et al., 2019; Chen et al., 2020; Girven et al., 2020). Especially given the renewed interest in adjacent bordering structures (i.e., ventral pallidum, substantia innominata, preoptic area; McHenry et al., 2017; Gordon-Fennell et al., 2019; Ottenheimer et al., 2019; Stephenson-Jones et al., 2020), investigators may decide to refine their definitions when examining ventrally-located BNST neuronal populations. Of course, “ventral BNST” is simply one of many instances of imperfect BNST anatomical nomenclature. Numerous additional examples of incongruous systems for spatially labeling neuronal subtypes serve only to further strengthen the rationale for adopting a BNST framework that heavily incorporates non-spatial defining features (Ju and Swanson, 1989; Jennings et al., 2013a, b; Kim et al., 2013; Giardino et al., 2018; Barbier et al., 2021).
Although beyond the scope of this review, potential differences in the spatial organization of BNST cell types between various rodent and primate species also require serious consideration. In addition to the anatomical literature cited above, we refer the reader to foundational work from Bales, Blackford, Fox, Fudge, Luyten, Shackman, Trainor, Zahm, and others (Zahm, 1998; Fudge and Haber, 2001; Zahm et al., 2003; Hostetler et al., 2011; Avery et al., 2014, 2016; Fox et al., 2015; Luyten et al., 2016; Shackman and Fox, 2016; Fudge et al., 2017; Oler et al., 2017; Raymaekers et al., 2017; Reichard et al., 2017; Theiss et al., 2017; Duque-Wilckens et al., 2018; Fox and Shackman, 2019; Luyck et al., 2019, 2020; Flook et al., 2020; Luyten, 2020). Indeed, most in vivo data generated from monkey and human BNST thus far has been collected using methods with a low spatial resolution like functional magnetic resonance imaging and deep brain stimulation. Within these mental health contexts, reliable in vivo parcellation of human BNST subcompartments remains a lofty goal. Keeping this in mind, we encourage others to acknowledge the possibility that acquiring enhanced spatial resolution of the BNST in human patients may turn out to be largely inconsequential for improving overall psychiatric and neurological outcomes. In doing so, we posit that emphasis on non-spatial aspects of BNST neurons (such as molecular markers, physiological features, long-range projection targets, and sources of upstream neural inputs) may hold the key for accelerating discovery on functional contributions of BNST circuitry to behavior and sleep/wake arousal states.
Molecularly-Defined BNST Cell Types
Similar to the rest of the extended amygdala, the BNST contains many cell types marked by a myriad of neurotransmitters, neuropeptides, receptors, enzymes, and regulatory proteins (Bota et al., 2012). The BNST primarily consists of subpopulations of inhibitory neurons marked by the GABA transporter Vgat, as well as excitatory populations marked by the glutamate transporter Vglut2, and a mixed excitatory/inhibitory population marked by co-expression of Vgat and Vglut3 (Kudo et al., 2012; Jennings et al., 2013b). Although these various GABAergic and glutamatergic populations are widely dispersed, mapping molecularly-defined cell types to different spatial areas of the BNST may help clarify how different subregions regulate emotional arousal behaviors (Figure 1).
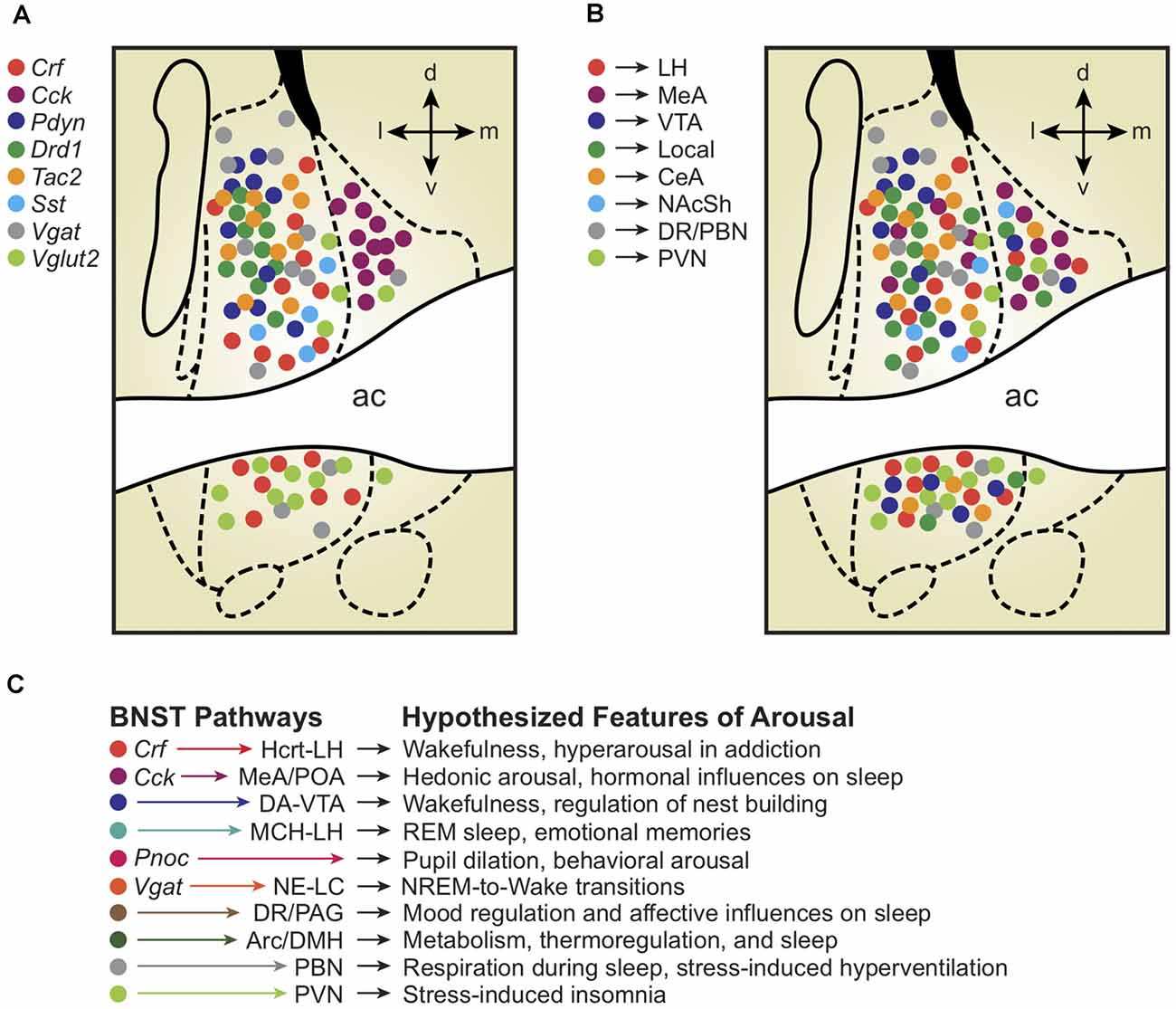
Figure 1. (A) Depiction of molecularly-defined bed nuclei of stria terminalis (BNST) cell types and their distribution across BNST subregions. Note the remarkable compartmentalization of some cell-types compared with others (Cck vs. Crf vs. Vglut2). (B) Depiction of projection-defined BNST cell types and their approximate distributions across BNST subregions. Interestingly, some (but not all) projection-defined cell types roughly map onto corresponding molecularly-defined subpopulations. (C) Depiction of BNST cell types, pathways, and their hypothesized relationships with distinct features of arousal and sleep/wake regulation. Colors of each hypothesized pathway reflect the molecularly- and projection-defined BNST cell types when consistent with panels (A) and (B). Distinct colors represent hypothesized BNST pathways with unknown molecular traits and spatial distributions across the BNST.
To survey BNST neurons defined by molecular markers other than GABA/glutamate transporters, multiple studies first used Cre driver rodent lines with Ai9/Ai14 tdTomato fluorescent reporter mice or viral labeling techniques and combined anatomical analyses with immunohistochemical staining (Chen et al., 2015; Pomrenze et al., 2015; Nguyen et al., 2016; Giardino et al., 2018; Walker et al., 2019). The global population of Vgat-BNST neurons was found to encompass several molecularly-defined subgroups, including neurons expressing genetic and protein markers for the neuropeptides corticotropin-releasing factor (Crf; Dabrowska et al., 2013, 2016) and cholecystokinin (Cck; Giardino et al., 2018). Crf and Cck are non-overlapping GABAergic subpopulations that occupy separate lateral vs. medial subdivisions, co-residing in adjacent compartments throughout the middle of the BNST A/P axis, approximately +0.2 to −0.2 mm from Bregma in the mouse. In addition to Crf and Cck, the neuropeptides dynorphin (Pdyn), enkephalin (Penk), neurotensin (Nts), neuropeptide Y (Npy), nociceptin (Pnoc), somatostatin (Sst), substance P (Tac1), neurokinin B (Tac2), and vasopressin (Avp) are also found in neurons throughout the BNST (Malsbury and McKay, 1987; Walter et al., 1991; Poulin et al., 2009; Kudo et al., 2014; Crowley et al., 2016; Ahrens et al., 2018; Giardino et al., 2018; Zelikowsky et al., 2018; Kovner et al., 2019; Rigney et al., 2019; Rodriguez-Romaguera et al., 2020; Smith et al., 2020; Whylings et al., 2020; Xiao et al., 2020; Figure 1A). Labeling for the calcium-binding protein calretinin appears selective for the dorsolateral (dl)BNST, whereas dopamine receptor type-1 (Drd1) and protein kinase C delta (Pkcd) neurons cluster more specifically within the oval nucleus (ovBNST, a discrete subnucleus within the larger dlBNST subregion; Kim et al., 2013; Nguyen et al., 2016; Pomrenze et al., 2019a; Wang et al., 2019).
Breakthrough efforts to characterize the entire genetic diversity of the BNST and surrounding regions at the single-cell level provided evidence for up to 37 distinct neuronal subtypes, although an exhaustive discussion of this data is beyond the scope of our review (Moffitt et al., 2018; Welch et al., 2019). A more recent single-cell RNA sequencing study targeted specifically in the dorsal (d)BNST identified several neuronal clusters, including those marked by expected genes (e.g., Pkcd, Sst, Npy), but also some surprising markers (e.g., Lmo4; Rodriguez-Romaguera et al., 2020).
Numerous BNST neurons (particularly in the posteromedial [pm]BNST) express markers for actions of gonadal steroid hormones, including the androgen receptor (AR), progesterone receptor (PR), estrogen receptors, and aromatase (Aro), the enzyme that converts androgens to estrogens (Bayless and Shah, 2016). Aro-BNST neurons have been well-studied in contexts of sexually dimorphic behaviors (Bayless et al., 2019), and pmBNST neurons expressing AR and PR are more numerous in males vs. females (Juntti et al., 2010; Yang et al., 2013), highlighting the importance of sex differences and hormonal interactions when studying BNST contributions to emotional arousal (Bangasser and Shors, 2008; Bangasser et al., 2019).
Concerning the potential wake-modulating effects of molecularly-defined BNST subpopulations, the reported pupil dilatory effects of Pnoc-BNST neuron stimulation (Rodriguez-Romaguera et al., 2020) suggest that such rapid arousal responses may also regulate sleep/wake state transitions, but this has not been explicitly tested. Furthermore, while genetic markers in the BNST have thus far been primarily used to simply define the type of cell rather than determine the role of the corresponding protein product, future progress will shift toward understanding the physiological actions of the molecule itself. For example, it will be essential to assess whether modern neurotechnological approaches used to stimulate CRF-expressing BNST neurons will be sufficient to accurately recapitulate the sleep/wake changes resulting from the natural release of the CRF neuropeptide from BNST neurons.
Projection-Defined BNST Cell Types
A major property of the BNST is its connections with a plethora of downstream target brain regions, providing a useful framework for conceptualizing the potential contributions of BNST neurons to distinct features of sleep/wake arousal states (Figure 1B). For example, by examining BNST projection neurons that form synapses with cells in limbic regions regulating emotional behavior, One landmark article characterized the role of three different BNST pathways in separate features of anxiety (Kim et al., 2013). The authors showed that neurons in the anterodorsal (ad)BNST target the lateral hypothalamus (LH), ventral tegmental area (VTA), and parabrachial nucleus (PBN) to drive anxiolysis, reward, and decreased respiratory rate, respectively. Retrograde tracing determined minimal overlap between cells projecting to the three different downstream regions, collectively implying unique anxiety-relevant functions for different BNST cell types based in part on their projection targets. Given the rich literature describing roles for the LH (Li et al., 2017, 2018), VTA (Eban-Rothschild et al., 2016, 2020), and PBN (Kaur and Saper, 2019) in various aspects of sleep/wake regulation, we speculate that such BNST axonal outputs controlling separable components of emotional behavior may also modulate distinct physiological aspects of sleep/wake arousal states (Figure 1C).
In earlier studies of BNST neuroanatomy in rodents, VTA-projecting BNST neurons had been characterized by the Watanabe group, who identified a double-inhibitory pathway in which GABAergic BNST neurons preferentially target VTA-GABA neurons (Kudo et al., 2012). Kudo et al. (2012, 2014) also identified VTA-projecting BNST neurons marked by the glutamate transporters Vglut2 and Vglut3, as well as the opioid peptide Penk, providing multiple diverse mechanisms for modulating the activity of GABA and dopamine (DA) neurons in the VTA. Jennings et al. (2013b) reported that Vgat and Vglut2 neurons specifically in the ventral (v)BNST synapse onto GABA and DA VTA neurons, where they control distinct motivational states. Numerous other studies also focused on VTA-projecting BNST neurons, including those labeled by Crf and Pdyn (Briand et al., 2010; Silberman et al., 2013; Marcinkiewcz et al., 2016; Pina and Cunningham, 2017; Rinker et al., 2017; Companion and Thiele, 2018; Dedic et al., 2018; Fellinger et al., 2020).
Given recent evidence that VTA-DA neurons drive wakefulness and regulate nest-building (a critical sleep preparatory sequence in mice), BNST→VTA-DA circuits may therefore be a key mechanism influencing ethologically-relevant behavioral arousal (Eban-Rothschild et al., 2016, 2017; Eban-Rothschild and de Lecea, 2017). Furthermore, the activity of the VTA-GABA neuron population was found to be positively correlated with high-frequency gamma signal (30–80 Hz) during wakefulness, providing another measure of physiological arousal likely impacted by BNST→VTA projections (Eban-Rothschild et al., 2020).
Separate efforts on LH-projecting neurons identified a large Vgat-BNST population that preferentially targeted Vglut2-LH neurons downstream (Jennings et al., 2013a). Later studies determined that LH-projecting Vgat-BNST neurons include both Crf and Cck subpopulations that exhibit divergent preferences for downstream target cell types, with Crf-BNST neurons displaying a particularly high level of connectivity with LH neurons containing the arousal-promoting neuropeptide hypocretin (Hcrt; also known as orexin; Giardino et al., 2018). Hcrt-LH neurons comprise a subset of glutamatergic LH neurons, consistent with the interpretation that Crf-BNST→Hcrt-LH connections represent a subset of the larger Vgat-BNST→Vglut2-LH pathway. González et al. (2016) also investigated BNST→LH connectivity, finding that Vgat-BNST neurons synapse onto both Hcrt/orexin and melanin-concentrating hormone (MCH) neurons of the LH. This discovery of BNST neurons interacting with the MCH system is notable based on several studies showing that Mch-LH neurons promote rapid eye movement (REM) sleep (Bandaru et al., 2020).
In addition to their LH projections, Crf and Cck BNST neurons innervate (to varying degrees) the paraventricular nucleus of the hypothalamus (PVN), medial amygdala (MeA), CeA, medial preoptic area (mPOA), NAcSh, ventral premammillary nucleus (PMv), and ventrolateral periaqueductal gray (vlPAG; Dabrowska et al., 2016; Giardino et al., 2018). BNST projections to the PVN are particularly relevant given recent findings that glutamatergic neurons in this region are critical for the control of wakefulness (Liu et al., 2020). Further pursuing questions of BNST→hypothalamus connectivity, Barbier et al. (2021) traced the long-range projections of dlBNST and dorsomedial (dm)BNST neurons in exquisite detail, finding that the dlBNST projects especially to the LH and tuberomammillary nucleus (TMN; the site of wake-promoting histamine neurons; Barbier et al., 2021). In contrast to the dlBNST, the dmBNST preferentially innervates the PVN, the arcuate nucleus (Arc), and dorsomedial hypothalamus (DMH) regions that may influence sleep pressure via regulation of metabolic and thermoregulatory processes (Barbier et al., 2021).
In addition to targeted investigations, serendipitous retrograde labeling of upstream neurons has yielded surprising BNST outputs and circuit motifs. For example, monosynaptic rabies tracing from either “patch” or “matrix” neurons in the dorsal striatum revealed a major input from the dBNST to patch neurons that were largely absent from matrix neurons (Smith et al., 2016). These dBNST neurons form inhibitory synapses with striatal patch neurons who in turn project to DA neurons in the substantia nigra. Similarly, a GABAergic projection from Sst-BNST neurons was recently characterized and shown to form synapses with parvalbumin interneurons in the NAcSh (Xiao et al., 2020). Therefore, in addition to directly targeting the VTA, the BNST is capable of modulating mesolimbic DA function through indirect mechanisms at sites of midbrain innervation in the striatum.
Monosynaptic rabies tracing also identified BNST neurons that project directly to serotonin and GABA neurons in the dorsal raphé nucleus (DR; Weissbourd et al., 2014). Interestingly, BNST→DRN neurons preferentially target GABA cells over serotonin cells. While the majority of the BNST→DR cells were GABAergic at mouse coordinates +0.14 mm A/P from Bregma, a smaller set of BNST→DR neurons were labeled by Vglut2 at +0.02 mm A/P from Bregma. Separate rabies tracing studies also revealed BNST inputs to noradrenergic locus coeruleus neurons (Schwarz et al., 2015), DA and GABA VTA neurons (Beier et al., 2015), and CRF receptor 1 (CRFR1) PVN neurons (Jiang et al., 2018).
Beyond its rich collection of long-range projection neurons, the BNST contains several species of short-range interneurons. The Deisseroth group showed that ovBNST neurons project locally between subregions of the adBNST where they release GABA (Kim et al., 2013). In line with this, ovBNST neurons secrete dynorphin to inhibit excitatory basolateral amygdala (BLA) fibers innervating the adBNST (Crowley et al., 2016). Neurons in ovBNST also send dense axons to the vBNST (Dong et al., 2001; Wang et al., 2019). Aside from inhibitory local connections, many studies raised the possibility that neuropeptide modulators are also released locally from within the BNST. For example, NPY enhances inhibitory transmission in Crf-BNST neurons (Pleil et al., 2015) and although this study did not identify the source of NPY, the BNST contains several NPY-expressing neurons capable of local release. Another study from the Kash group found a complex microcircuit in the BNST comprised of Crf interneurons that are modulated by serotonin and regulate the activity of separate long-range BNST projection neurons (Marcinkiewcz et al., 2016). Collectively, these studies demonstrate the complexity of both long-range and local connectivity in the BNST and point to another dimension of inherent organization arising from gene expression and neurotransmitter phenotype.
Physiology-Defined BNST Cell Types
The BNST contains multiple neuronal cell types that have been classified according to their electrophysiological properties, most prominently in rats by Hammack and others (Hammack et al., 2007; Hazra et al., 2011; Dabrowska et al., 2013; Rodríguez-Sierra et al., 2013; Silberman et al., 2013; Nagano et al., 2015; Yamauchi et al., 2018). While originally described primarily within the ovBNST, these three types (Type I, II, and III) have also been identified in the non-oval areas of adBNST, as well as anteroventral (av)BNST. Type I neurons exhibit an Ih-like current in response to hyperpolarizing current injection and a regular firing pattern in response to depolarizing current. Type II neurons exhibit a similar Ih-like current but burst fire in response to depolarizing current. Type III neurons do not exhibit an Ih-like current but instead, show a fast rectification in response to hyperpolarizing current and exhibit a regular firing pattern when depolarized. Coupled with the diverse synaptic inputs, these physiological characteristics likely play a strong role in modulating varied forms of BNST output.
Regarding distinct output modes of neurophysiological communication, most outgoing physiological signals from the BNST have been recorded simply as GABAergic/glutamatergic inhibitory/excitatory postsynaptic currents. However, the existence of co-expressed neuropeptides in Vgat-BNST neurons suggests the likelihood of multiplexed modes of signaling in which single cells may influence downstream activity via a combination of slow-acting neuropeptide release and fast-acting classical neurotransmitter release. Indeed, a growing suite of fluorescent sensor tools for detecting receptor signaling with the cell-type resolution at rapid timescales may prove revolutionary for elucidating the sleep/wake mechanisms of neuropeptide release and actions across synaptic and extrasynaptic sites of the BNST circuitry (Gizowski et al., 2016; Patriarchi et al., 2018; Sun et al., 2018).
Functionally-Defined BNST Cell Types
Hedonic Valence
The BNST is known to impact both positive and negative states of reward and stress, and early studies relied primarily on electrolytic and neurochemical lesions to generate several opposing hypotheses regarding the bi-valent emotional behaviors generated by activity in the BNST (Bangasser et al., 2005; Pezuk et al., 2008; Resstel et al., 2008). Access to molecularly-defined cell types enabled by modern neurotechnology has ushered in several recent advances in understanding the sources of hedonic valence in the BNST, beginning with studies from the Stuber group showing that optical stimulation of Vgat-BNST and Vglut2-BNST neurons produced approach and avoidance behaviors, respectively (Jennings et al., 2013a, b).
Going beyond the separation of large populations of GABAergic vs. glutamatergic neurons, additional tools for recording and modulating cell-specific neural activity in vivo revealed that dlBNST Crf neurons preferentially respond to aversive stimuli and drive behavioral avoidance, whereas dmBNST Cck neurons are activated by rewarding stimuli and generate behavioral preference/approach (Giardino et al., 2018). Mirroring this lateral/medial functional distinction, Drd1 neurons in the ovBNST/dlBNST and Six3 neurons in the dmBNST also drive avoidance and approach, respectively (Giardino et al., 2018). Because activation of the global Vgat-BNST population promotes positive valence (Jennings et al., 2013a, b; Giardino et al., 2018), we hypothesize that Crf marks a specialized subgroup of lateral Vgat-BNST neurons with opposing functional properties from the larger set of combined lateral and medial Vgat-BNST cells. In other words, global Vgat-BNST stimulation may more closely resemble activation of medial Vgat-BNST neurons (like Cck) rather than lateral Vgat-BNST neurons (like Crf).
It should be noted that the vast majority of data on BNST circuits driving hedonic valence has been generated only from male mice. Given that the BNST is a major site for integrating signals from centrally-circulating gonadal steroids, intense investigation of hormonal influences on the function of extended amygdala pathways may be key for understanding sex differences in the stress response and reward sensitivity. Future studies may also seek to titrate levels of stressor exposure, drug consumption, and other variables to determine whether such experiential factors can influence the hedonic valence associated with mobilization of particular BNST subpopulations.
Anxiety
Despite the distinct hedonic valences associated with stimulating Crf and Cck BNST neurons, optogenetic or chemogenetic activation of either Crf or Cck BNST neurons led to increased indices of anxiety-like behavior in multiple paradigms (Giardino et al., 2018), suggesting that standard measures of “anxiety” in rodents may reflect generalized arousal states independent of hedonic valence per se. Consistent with this interpretation, activation of Vgat-BNST neurons (Mazzone et al., 2018), Drd1-ovBNST neurons (Kim et al., 2013), Pdyn-BNST→VTA neurons (Fellinger et al., 2020), dlBNST→CeA neurons (Yamauchi et al., 2018), and Crf-CeA→dlBNST neurons (Pomrenze et al., 2019b) all increased anxiety-like behaviors. Yet, separate studies found that stimulation of adBNST→LH neurons (Kim et al., 2013), Vgat-vBNST→VTA neurons (Jennings et al., 2013b), and Sst-BNST→NAcSh neurons (Xiao et al., 2020) had the opposite effect of reducing anxiety, revealing some unresolved issues regarding how BNST microcircuits and long-range pathways regulate stress-related “anxiety” phenotypes. Anxiogenic circumstances like fear learning and stress-induced social deficits also strongly engage the BNST (Bjorni et al., 2020; Emmons et al., 2021), providing multiple perspectives for approaching the study of BNST in anxiety-related emotional arousal.
The BNST in Sleep, Wake, and Emotional Arousal
Only a handful of studies have explicitly investigated the BNST within the context of sleep/wake arousal states and corresponding neurophysiological rhythms. Beginning in 1995, in vivo recordings of 63 single units in the BNST of cats revealed that 72% of neurons fired more frequently during wakefulness and rapid eye movement (REM) sleep than during “quiet sleep” (presumably non-REM/slow-wave sleep; NREM; Terreberry et al., 1995). These findings are consistent with more recent data indicating that excitatory projections from the BNST may activate REM-active neurons in the sublaterodorsal tegmental nucleus (SLD) of the brainstem (Boissard et al., 2003; Rodrigo-Angulo et al., 2008). In the BNST of rats, sleep deprivation increased cFos and CRF protein expression (Duan et al., 2005; Deurveilher et al., 2008) and M3 muscarinic acetylcholine receptor gene expression (Kushida et al., 1995), suggesting possible neuropeptidergic and cholinergic mechanisms for homeostatic sleep drive in the BNST.
In 2014, an intriguing arousal-related physiological signature was discovered in the extended amygdala by Haufler and Pare (2014), who recorded high-frequency oscillations (HFOs; 110–160 Hz local field potentials) that appeared with a significantly higher incidence in the BNST and CeA relative to surrounding areas (striatum, pallidum, septum; Haufler and Pare, 2014). HFOs in hippocampal, cortical, and subthalamic brain regions are thought to have functional consequences in memory-processing, epileptic seizures, and symptoms of Parkinson’s disease, respectively. Whereas the role of HFOs in the BNST remains unclear, their ability to entrain large populations of neurons (with greater power during REM vs. non-REM sleep; Haufler and Pare, 2014) provides a fascinating example of the network-level changes in extended amygdala activity that might profoundly influence discrete states of arousal. Future endeavors will revolutionize understanding of how arousal state transitions may be aligned to physiological activity changes and/or receptor signaling events in a BNST cell type-specific manner by implementing combinatorial approaches like EEG/EMG sleep monitoring in tandem with next-generation neural recording technologies (fiber photometry, miniscope, and two-photon imaging).
To directly investigate the BNST as a potential node in the arousal circuitry, Kodani et al. (2017, 2019) utilized optogenetic methods and reported that they could generate immediate transitions from NREM sleep to wakefulness by stimulating the global GABAergic BNST population in Gad67-Cre mice. Gad67-BNST arousal was associated with activation of norepinephrine neurons in the locus coeruleus (NE-LC), an established wakefulness-promoting center. Gad67-BNST arousal was also associated with activation of Hcrt-LH neurons and required signaling of Hcrt receptors (Kodani et al., 2017), consistent with the reported contributions of BNST→LH circuits to emotional arousal and related behaviors (Giardino and de Lecea, 2014; González et al., 2016; Giardino et al., 2018; Barbier et al., 2021). Of course, the precise directionalities of the relationships between endogenous BNST activity, emotional behaviors, and changes in sleep and wakefulness remain mostly uncharacterized. For example, whereas states of stress might be hypothesized to drive insomnia via chronically elevated BNST hyperactivity, additional experiments are required to determine whether excess BNST activity is truly a contributing factor or rather an indirect consequence of stress-induced arousal.
In addition to providing outputs to wake-promoting downstream targets in the LH, VTA, and PVN, the BNST also receives inputs from arousal-promoting neurons such as calretinin cells of the paraventricular thalamus (PVT; Hua et al., 2018). Hua et al. (2018) recently found that starvation promotes a state of hyperarousal via activation of the PVT-calretinin circuit, which can be blocked by chemogenetic inhibition of the PVT→BNST pathway. Despite this exciting progress, a complete understanding of the arousal-promoting abilities of the global GABAergic BNST population requires additional studies. Future experiments will aim to determine whether distinct molecularly-defined or pathway-specific BNST outputs are necessary and/or sufficient for regulating various forms of behavioral arousal and natural sleep-to-wake transitions (see hypothesized functions in Figure 1C).
On this note, it will be important to causally determine which BNST neurocircuits have experience-dependent effects on sleep/wake as a function of negative vs. positive hedonic valence. This can be accomplished by monitoring the activities of extended amygdala pathways and corresponding changes in arousal following emotional experiences with ethologically aversive or rewarding stimuli. For example, the discovery that reward-promoting Cck-BNST neurons are densely connected with the medial amygdala (MeA; Giardino et al., 2018) suggests that these pathways powerfully influence arousal changes linked to innately rewarding consummatory behaviors. In contrast to the Cck-BNST→MeA circuit, Crf-BNST→Hcrt-LH connections likely drive stress-induced insomnia following psychosocial challenges.
Given the well-established role of the BNST in drug-seeking behavior (Vranjkovic et al., 2017), attention should be placed on the idea that addiction-related sleep disturbances are driven by specific extended amygdala pathways. Core features of drug addiction (e.g., craving, relapse, and withdrawal) are each associated with maladaptive neuroplasticity in homeostatic circuits that regulate sleep/wake cycles and stress sensitivity, illustrating how addiction can be viewed as a condition of pathological hyperarousal (Koob, 2013; Koob and Colrain, 2020). Substance use disorders are highly co-morbid with other arousal-related psychiatric conditions (e.g., insomnia, anxiety, PTSD, panic), and the BNST may be a common substrate linking dysregulated hedonic processing to chronic sleep disruption.
Finally, the BNST may be an important and overlooked link in emotional arousal about the sleep disorder narcolepsy with cataplexy (in which powerful feelings of euphoria or aversion can interrupt wakefulness by triggering rapid intrusion of a sleep-like state). Intriguingly, narcolepsy is associated with selective loss of Hcrt neurons in the LH. Based on existing evidence for BNST→LH connections driving motivated behaviors, detailed studies are warranted on BNST circuit contributions to emotionally-triggered arousal destabilization. Of course, the existence of putative sleep-promoting BNST neurons remains to be seen. However, BNST connections with hypothalamic nuclei regulating the release of prolactin, oxytocin, and vasopressin suggest that experiments linking BNST activity to arousal changes following binge eating (“food coma”) and sexual behavior (mating) may be successful in revealing the neurocircuitry of mysterious phenomena like post-ingestive sleep, post-copulatory sleep, and narcolepsy with cataplexy.
Conclusions
Altogether, our understanding of extended amygdala circuits regulating affective behaviors will have direct relevance to therapeutic strategies aimed at modulating motivation and sleep/wake regulation. Before the advent of tools permitting cell type-specific and pathway-specific labeling and manipulation, the inherent complexity of extended amygdala pathways hindered progress in understanding their roles in emotional arousal. As BNST circuits continue to be disentangled at the genetic, synaptic, and systems levels, new anatomical and functional frameworks are taking shape that illustrates the multifaceted nature of the BNST. Data of this kind are extremely valuable for developing new therapeutic approaches for a range of neuropsychiatric conditions, highlighting the extraordinary relevance of emotional arousal circuits to researchers and clinicians across the fields of neuroscience, genetics, psychology, and mental health interventions. We anticipate that as studies on BNST function become more precise, effective clinical treatment strategies will be successfully developed.
Author Contributions
All authors listed have made a substantial, direct and intellectual contribution to the work, and approved it for publication.
Funding
This study received funding from NIH/NIAAA K99/R00 AA025677 (WG) and NIH/NIDA T32 DA035165 (MP).
Conflict of Interest
The authors declare that the research was conducted in the absence of any commercial or financial relationships that could be construed as a potential conflict of interest.
Acknowledgments
We thank D. W. Bayless, J. R. Knoedler, D. C. Castro, T. L. Kash, L. R. Halladay, and others for insightful discussions.
Supplementary Material
The Supplementary Material for this article can be found online at: https://www.frontiersin.org/articles/10.3389/fnbeh.2021.613025/full#supplementary-material.
References
Adamantidis, A. R., Schmidt, M. H., Carter, M. E., Burdakov, D., Peyron, C., and Scammell, T. E. (2020). A circuit perspective on narcolepsy. Sleep 43:zsz296. doi: 10.1093/sleep/zsz296
Ahrens, S., Wu, M. V., Furlan, A., Hwang, G. R., Paik, R., Li, H., et al. (2018). A central extended amygdala circuit that modulates anxiety. J. Neurosci. 38, 5567–5583. doi: 10.1523/JNEUROSCI.0705-18.2018
Alheid, G. F. (2003). Extended amygdala and basal forebrain. Ann. N Y Acad. Sci. 985, 185–205. doi: 10.1111/j.1749-6632.2003.tb07082.x
Avery, S. N., Clauss, J. A., and Blackford, J. U. (2016). The human BNST: functional role in anxiety and addiction. Neuropsychopharmacology 41, 126–141. doi: 10.1038/npp.2015.185
Avery, S. N., Clauss, J. A., Winder, D. G., Woodward, N., Heckers, S., and Blackford, J. U. (2014). BNST neurocircuitry in humans. NeuroImage 91, 311–323. doi: 10.1016/j.neuroimage.2014.01.017
Bandaru, S. S., Khanday, M. A., Ibrahim, N., Naganuma, F., and Vetrivelan, R. (2020). Sleep-wake control by melanin-concentrating hormone (MCH) neurons: a review of recent findings. Curr. Neurol. Neurosci. Rep. 20:55. doi: 10.1007/s11910-020-01075-x
Bangasser, D. A., Eck, S. R., and Ordoñes Sanchez, E. (2019). Sex differences in stress reactivity in arousal and attention systems. Neuropsychopharmacology 44, 129–139. doi: 10.1038/s41386-018-0137-2
Bangasser, D. A., Santollo, J., and Shors, T. J. (2005). The bed nucleus of the stria terminalis is critically involved in enhancing associative learning after stressful experience. Behav. Neurosci. 119, 1459–1466. doi: 10.1037/0735-7044.119.6.1459
Bangasser, D. A., and Shors, T. J. (2008). The bed nucleus of the stria terminalis modulates learning after stress in masculinized but not cycling females. J. Neurosci. 28, 6383–6387. doi: 10.1523/JNEUROSCI.0831-08.2008
Barbier, M., Gonzalez, J. A., Houdayer, C., Burdakov, D., Risold, P. Y., and Croizier, S. (2021). Projections from the dorsomedial division of the bed nucleus of the stria terminalis to hypothalamic nuclei in the mouse. J. Comp. Neurol. 529, 929–956. doi: 10.1002/cne.24988
Bayer, S. A., and Altman, J. (1987). Development of the preoptic area: time and site of origin, migratory routes and settling patterns of its neurons. J. Comp. Neurol. 265, 65–95. doi: 10.1002/cne.902650106
Bayless, D. W., and Shah, N. M. (2016). Genetic dissection of neural circuits underlying sexually dimorphic social behaviours. Philos. Trans. R. Soc. Lond. B Biol. Sci. 371:20150109. doi: 10.1098/rstb.2015.0109
Bayless, D. W., Yang, T., Mason, M. M., Susanto, A. A. T., Lobdell, A., and Shah, N. M. (2019). Limbic neurons shape sex recognition and social behavior in sexually naive males. Cell 176, 1190.e20–1205.e20. doi: 10.1016/j.cell.2018.12.041
Beier, K. T., Steinberg, E. E., DeLoach, K. E., Xie, S., Miyamichi, K., Schwarz, L., et al. (2015). Circuit architecture of VTA dopamine neurons revealed by systematic input-output mapping. Cell 162, 622–634. doi: 10.1016/j.cell.2015.07.015
Beyeler, A., and Dabrowska, J. (2020). Neuronal diversity of the amygdala and the bed nucleus of the stria terminalis. Handb. Behav. Neurosci. 26, 63–100. doi: 10.1016/b978-0-12-815134-1.00003-9
Bjorni, M., Rovero, N. G., Yang, E. R., Holmes, A., and Halladay, L. R. (2020). Phasic signaling in the bed nucleus of the stria terminalis during fear learning predicts within- and across-session cued fear expression. Learn. Mem. 27, 83–90. doi: 10.1101/lm.050807.119
Boissard, R., Fort, P., Gervasoni, D., Barbagli, B., and Luppi, P. H. (2003). Localization of the GABAergic and non-GABAergic neurons projecting to the sublaterodorsal nucleus and potentially gating paradoxical sleep onset. Eur. J. Neurosci. 18, 1627–1639. doi: 10.1046/j.1460-9568.2003.02861.x
Bota, M., Sporns, O., and Swanson, L. W. (2012). Neuroinformatics analysis of molecular expression patterns and neuron populations in gray matter regions: the rat BST as a rich exemplar. Brain Res. 1450, 174–193. doi: 10.1016/j.brainres.2012.02.034
Briand, L. A., Vassoler, F. M., Pierce, R. C., Valentino, R. J., and Blendy, J. A. (2010). Ventral tegmental afferents in stress-induced reinstatement: the role of cAMP response element-binding protein. J. Neurosci. 30, 16149–16159. doi: 10.1523/JNEUROSCI.2827-10.2010
Chen, Y., Molet, J., Gunn, B. G., Ressler, K., and Baram, T. Z. (2015). Diversity of reporter expression patterns in transgenic mouse lines targeting corticotropin-releasing hormone-expressing neurons. Endocrinology 156, 4769–4780. doi: 10.1210/en.2015-1673
Chen, A.-X., Yan, J.-J., Zhang, W., Wang, L., Yu, Z.-X., Ding, X.-J., et al. (2020). Specific hypothalamic neurons required for sensing conspecific male cues relevant to inter-male aggression. Neuron 108, 763.e6–774.e6. doi: 10.1016/j.neuron.2020.08.025
Ch’ng, S., Fu, J., Brown, R. M., McDougall, S. J., and Lawrence, A. J. (2018). The intersection of stress and reward: BNST modulation of aversive and appetitive states. Prog. Neuropsychopharmacol. Biol. Psychiatry 87, 108–125. doi: 10.1016/j.pnpbp.2018.01.005
Companion, M. A., and Thiele, T. E. (2018). Assessment of ventral tegmental area-projecting GABAergic neurons from the bed nucleus of the stria terminalis in modulating binge-like ethanol intake. Eur. J. Neurosci. 48, 3335–3343. doi: 10.1111/ejn.14222
Crowley, N. A., Bloodgood, D. W., Hardaway, J. A., Kendra, A. M., McCall, J. G., Al-Hasani, R., et al. (2016). Dynorphin controls the gain of an amygdalar anxiety circuit. Cell Rep. 14, 2774–2783. doi: 10.1016/j.celrep.2016.02.069
Dabrowska, J., Hazra, R., Guo, J. D., Dewitt, S., and Rainnie, D. G. (2013). Central CRF neurons are not created equal: phenotypic differences in CRF-containing neurons of the rat paraventricular hypothalamus and the bed nucleus of the stria terminalis. Front. Neurosci. 7:156. doi: 10.3389/fnins.2013.00156
Dabrowska, J., Martinon, D., Moaddab, M., and Rainnie, D. G. (2016). Targeting corticotropin-releasing factor projections from the oval nucleus of the bed nucleus of the stria terminalis using cell-type specific neuronal tracing studies in mouse and rat brain. J. Neuroendocrinol. 28:12. doi: 10.1111/jne.12442
De Olmos, J. S., and Ingram, W. R. (1972). The projection field of the stria terminalis in the rat brain. An experimental study. J. Comp. Neurol. 146, 303–334. doi: 10.1002/cne.901460303
Dedic, N., Kühne, C., Jakovcevski, M., Hartmann, J., Genewsky, A. J., Gomes, K. S., et al. (2018). Chronic CRH depletion from GABAergic, long-range projection neurons in the extended amygdala reduces dopamine release and increases anxiety. Nat. Neurosci. 21, 803–807. doi: 10.1038/s41593-018-0151-z
Deurveilher, S., Cumyn, E. M., Peers, T., Rusak, B., and Semba, K. (2008). Estradiol replacement enhances sleep deprivation-induced c-Fos immunoreactivity in forebrain arousal regions of ovariectomized rats. Am. J. Physiol. Regul. Integr. Comp. Physiol. 295, R1328–R1340. doi: 10.1152/ajpregu.90576.2008
Dong, H., Petrovich, G. D., and Swanson, L. W. (2000). Organization of projections from the juxtacapsular nucleus of the BST: a PHAL study in the rat. Brain Res. 859, 1–14. doi: 10.1016/s0006-8993(99)02246-5
Dong, H.-W., Petrovich, G. D., and Swanson, L. W. (2001a). Topography of projections from amygdala to bed nuclei of the stria terminalis. Brain Res. Rev. 38, 192–246. doi: 10.1016/s0165-0173(01)00079-0
Dong, H.-W., Petrovich, G. D., Watts, A. G., and Swanson, L. W. (2001b). Basic organization of projections from the oval and fusiform nuclei of the bed nuclei of the stria terminalis in adult rat brain. J. Comp. Neurol. 436, 430–455. doi: 10.1002/cne.1079
Dong, H.-W., and Swanson, L. W. (2003). Projections from the rhomboid nucleus of the bed nuclei of the stria terminalis: implications for cerebral hemisphere regulation of ingestive behaviors. J. Comp. Neurol. 463, 434–472. doi: 10.1002/cne.10758
Dong, H.-W., and Swanson, L. W. (2004a). Organization of axonal projections from the anterolateral area of the bed nuclei of the stria terminalis. J. Comp. Neurol. 468, 277–298. doi: 10.1002/cne.10949
Dong, H.-W., and Swanson, L. W. (2004b). Projections from bed nuclei of the stria terminalis, posterior division: implications for cerebral hemisphere regulation of defensive and reproductive behaviors. J. Comp. Neurol. 471, 396–433. doi: 10.1002/cne.20002
Dong, H.-W., and Swanson, L. W. (2006a). Projections from bed nuclei of the stria terminalis, anteromedial area: cerebral hemisphere integration of neuroendocrine, autonomic, and behavioral aspects of energy balance. J. Comp. Neurol. 494, 142–178. doi: 10.1002/cne.20788
Dong, H.-W., and Swanson, L. W. (2006b). Projections from bed nuclei of the stria terminalis, magnocellular nucleus: implications for cerebral hemisphere regulation of micturition, defecation, and penile erection. J. Comp. Neurol. 494, 108–141. doi: 10.1002/cne.20789
Dong, H.-W., and Swanson, L. W. (2006c). Projections from bed nuclei of the stria terminalis, dorsomedial nucleus: implications for cerebral hemisphere integration of neuroendocrine, autonomic, and drinking responses. J. Comp. Neurol. 494, 75–107. doi: 10.1002/cne.20790
Duan, X.-L., Wang, B.-R., Yang, T.-B., Yu, H.-M., Zhu, Z.-H., Xu, Z., et al. (2005). Preparation of polyclonal and monoclonal antibodies against CRF and change of CRF in the sleep-deprived rat brain. Xi Bao Yu Fen Zi Mian Yi Xue Za Zhi 21, 476–479.
Duque-Wilckens, N., Steinman, M. Q., Busnelli, M., Chini, B., Yokoyama, S., Pham, M., et al. (2018). Oxytocin receptors in the anteromedial bed nucleus of the stria terminalis promote stress-induced social avoidance in female california mice. Biol. Psychiatry 83, 203–213. doi: 10.1016/j.biopsych.2017.08.024
Eban-Rothschild, A., Appelbaum, L., and de Lecea, L. (2018). Neuronal mechanisms for sleep/wake regulation and modulatory drive. Neuropsychopharmacology 43, 937–952. doi: 10.1038/npp.2017.294
Eban-Rothschild, A., Borniger, J. C., Rothschild, G., Giardino, W. J., Morrow, J. G., and de Lecea, L. (2020). Arousal state-dependent alterations in VTA-GABAergic neuronal activity. eNeuro 7:ENEURO.0356-19.2020. doi: 10.1523/ENEURO.0356-19.2020
Eban-Rothschild, A., and de Lecea, L. (2017). Neuronal substrates for initiation, maintenance and structural organization of sleep/wake states. F1000Res. 6:212. doi: 10.12688/f1000research.9677.1
Eban-Rothschild, A., Giardino, W. J., and de Lecea, L. (2017). To sleep or not to sleep: neuronal and ecological insights. Curr. Opin. Neurobiol. 44, 132–138. doi: 10.1016/j.conb.2017.04.010
Eban-Rothschild, A., Rothschild, G., Giardino, W. J., Jones, J. R., and de Lecea, L. (2016). VTA dopaminergic neurons regulate ethologically relevant sleep-wake behaviors. Nat. Neurosci. 19, 1356–1366. doi: 10.1038/nn.4377
Egli, R. E., and Winder, D. G. (2003). Dorsal and ventral distribution of excitable and synaptic properties of neurons of the bed nucleus of the stria terminalis. J. Neurophysiol. 90, 405–414. doi: 10.1152/jn.00228.2003
Emmons, R., Sadok, T., Rovero, N. G., Belnap, M. A., Henderson, H. J. M., Quan, A. J., et al. (2021). Chemogenetic manipulation of the bed nucleus of the stria terminalis counteracts social behavioral deficits induced by early life stress in C57BL/6J mice. J. Neurosci. Res. 99, 90–109. doi: 10.1002/jnr.24644
Fellinger, L., Jo, Y. S., Hunker, A. C., Soden, M. E., and Zweifel, L. S. (2020). A midbrain dynorphin circuit promotes threat generalization. bioRxiv [Preprint]. doi: 10.1101/2020.04.21.053892
Flook, E. A., Feola, B., Avery, S. N., Winder, D. G., Woodward, N. D., Heckers, S., et al. (2020). BNST-insula structural connectivity in humans. NeuroImage 210:116555. doi: 10.1016/j.neuroimage.2020.116555
Fox, A. S., Oler, J. A., Tromp do, P. M., Fudge, J. L., and Kalin, N. H. (2015). Extending the amygdala in theories of threat processing. Trends Neurosci. 38, 319–329. doi: 10.1016/j.tins.2015.03.002
Fox, A. S., and Shackman, A. J. (2019). The central extended amygdala in fear and anxiety: closing the gap between mechanistic and neuroimaging research. Neurosci. Lett. 693, 58–67. doi: 10.1016/j.neulet.2017.11.056
Fudge, J. L., and Haber, S. N. (2001). Bed nucleus of the stria terminalis and extended amygdala inputs to dopamine subpopulations in primates. Neuroscience 104, 807–827. doi: 10.1016/s0306-4522(01)00112-9
Fudge, J. L., Kelly, E. A., Pal, R., Bedont, J. L., Park, L., and Ho, B. (2017). Beyond the classic VTA: extended amygdala projections to DA-striatal paths in the primate. Neuropsychopharmacology 42, 1563–1576. doi: 10.1038/npp.2017.38
Giardino, W. J., and de Lecea, L. (2014). Hypocretin (orexin) neuromodulation of stress and reward pathways. Curr. Opin. Neurobiol. 29, 103–108. doi: 10.1016/j.conb.2014.07.006
Giardino, W. J., Eban-Rothschild, A., Christoffel, D. J., Li, S. B., Malenka, R. C., and de Lecea, L. (2018). Parallel circuits from the bed nuclei of stria terminalis to the lateral hypothalamus drive opposing emotional states. Nat. Neurosci. 21, 1084–1095. doi: 10.1038/s41593-018-0198-x
Girven, K. S., Aroni, S., Navarrete, J., Marino, R. A. M., McKeon, P. N., Cheer, J. F., et al. (2020). Glutamatergic input from the insula to the ventral bed nucleus of the stria terminalis controls reward-related behavior. Addict. Biol. doi: 10.1111/adb.12961
Gizowski, C., Zaelzer, C., and Bourque, C. W. (2016). Clock-driven vasopressin neurotransmission mediates anticipatory thirst prior to sleep. Nature 537, 685–688. doi: 10.1038/nature19756
González, J. A., Iordanidou, P., Strom, M., Adamantidis, A., and Burdakov, D. (2016). Awake dynamics and brain-wide direct inputs of hypothalamic MCH and orexin networks. Nat. Commun. 7:11395. doi: 10.1038/ncomms11395
Gordon-Fennell, A. G., Will, R. G., Ramachandra, V., Gordon-Fennell, L., Dominguez, J. M., Zahm, D. S., et al. (2019). The lateral preoptic area: a novel regulator of reward seeking and neuronal activity in the ventral tegmental area. Front. Neurosci. 13:1433. doi: 10.3389/fnins.2019.01433
Hammack, S. E., Mania, I., and Rainnie, D. G. (2007). Differential expression of intrinsic membrane currents in defined cell types of the anterolateral bed nucleus of the stria terminalis. J. Neurophysiol. 98, 638–656. doi: 10.1152/jn.00382.2007
Hardaway, J. A., Halladay, L. R., Mazzone, C. M., Pati, D., Bloodgood, D. W., Kim, M., et al. (2019). Central amygdala prepronociceptin-expressing neurons mediate palatable food consumption and reward. Neuron 102:1088. doi: 10.1016/j.neuron.2019.04.036
Haufler, D., and Pare, D. (2014). High-frequency oscillations are prominent in the extended amygdala. J. Neurophysiol. 112, 110–119. doi: 10.1152/jn.00107.2014
Hazra, R., Guo, J.-D., Ryan, S. J., Jasnow, A. M., Dabrowska, J., and Rainnie, D. G. (2011). A transcriptomic analysis of type I–III neurons in the bed nucleus of the stria terminalis. Mol. Cell. Neurosci. 46, 699–709. doi: 10.1016/j.mcn.2011.01.011
Hostetler, C. M., Kowalczyk, A. S., Griffin, L. L., and Bales, K. L. (2011). CART peptide following social novelty in the prairie vole (Microtus ochrogaster). Brain Res. 1414, 32–40. doi: 10.1016/j.brainres.2011.07.040
Hua, R., Wang, X., Chen, X., Wang, X., Huang, P., Li, P., et al. (2018). Calretinin neurons in the midline thalamus modulate starvation-induced arousal. Curr. Biol. 28, 3948.e4–3959.e4. doi: 10.1016/j.cub.2018.11.020
Jennings, J. H., Rizzi, G., Stamatakis, A. M., Ung, R. L., and Stuber, G. D. (2013a). The inhibitory circuit architecture of the lateral hypothalamus orchestrates feeding. Science 341, 1517–1521. doi: 10.1126/science.1241812
Jennings, J. H., Sparta, D. R., Stamatakis, A. M., Ung, R. L., Pleil, K. E., Kash, T. L., et al. (2013b). Distinct extended amygdala circuits for divergent motivational states. Nature 496, 224–228. doi: 10.1038/nature12041
Jiang, Z., Rajamanickam, S., and Justice, N. J. (2018). Local corticotropin-releasing factor signaling in the hypothalamic paraventricular nucleus. J. Neurosci. 38, 1874–1890. doi: 10.1523/JNEUROSCI.1492-17.2017
Ju, G., and Swanson, L. W. (1989). Studies on the cellular architecture of the bed nuclei of the stria terminalis in the rat: I. Cytoarchitecture. J. Comp. Neurol. 280, 587–602. doi: 10.1002/cne.902800409
Ju, G., Swanson, L. W., and Simerly, R. B. (1989). Studies on the cellular architecture of the bed nuclei of the stria terminalis in the rat: II. Chemoarchitecture. J. Comp. Neurol. 280, 603–621. doi: 10.1002/cne.902800410
Juntti, S. A., Tollkuhn, J., Wu, M. V., Fraser, E. J., Soderborg, T., Tan, S., et al. (2010). The androgen receptor governs the execution, but not programming, of male sexual and territorial behaviors. Neuron 66, 260–272. doi: 10.1016/j.neuron.2010.03.024
Kash, T. L., Pleil, K. E., Marcinkiewcz, C. A., Lowery-Gionta, E. G., Crowley, N., Mazzone, C., et al. (2015). Neuropeptide regulation of signaling and behavior in the BNST. Mol. Cells 38, 1–13. doi: 10.14348/molcells.2015.2261
Kaur, S., and Saper, C. B. (2019). Neural circuitry underlying waking up to hypercapnia. Front. Neurosci. 13:401. doi: 10.3389/fnins.2019.00401
Kim, S.-Y., Adhikari, A., Lee, S. Y., Marshel, J. H., Kim, C. K., Mallory, C. S., et al. (2013). Diverging neural pathways assemble a behavioral state from separable features in anxiety. Nature 496, 219–223. doi: 10.1038/nature12018
Kim, B., Yoon, S., Nakajima, R., Lee, H. J., Lim, H. J., Lee, Y. K., et al. (2018). Dopamine D2 receptor-mediated circuit from the central amygdala to the bed nucleus of the stria terminalis regulates impulsive behavior. Proc. Natl. Acad. Sci. U S A 115, E10730–E10739. doi: 10.1073/pnas.1811664115
Kodani, S., Soya, S., and Sakurai, T. (2017). Excitation of GABAergic neurons in the bed nucleus of the stria terminalis triggers immediate transition from non-rapid eye movement sleep to wakefulness in mice. J. Neurosci. 37, 7164–7176. doi: 10.1523/JNEUROSCI.0245-17.2017
Kodani, S., Soya, S., and Sakurai, T. (2019). Optogenetic manipulation of neural circuits during monitoring sleep/wakefulness states in mice. J. Vis. Exp. 19:148. doi: 10.3791/58613
Koob, G. F. (2013). Addiction is a reward deficit and stress surfeit disorder. Front. Psychiatry 4:72. doi: 10.3389/fpsyt.2013.00072
Koob, G. F., and Colrain, I. M. (2020). Alcohol use disorder and sleep disturbances: a feed-forward allostatic framework. Neuropsychopharmacology 45, 141–165. doi: 10.1038/s41386-019-0446-0
Kovner, R., Fox, A. S., French, D. A., Roseboom, P. H., Oler, J. A., Fudge, J. L., et al. (2019). Somatostatin gene and protein expression in the non-human primate central extended amygdala. Neuroscience 400, 157–168. doi: 10.1016/j.neuroscience.2018.12.035
Krettek, J. E., and Price, J. L. (1978). Amygdaloid projections to subcortical structures within the basal forebrain and brainstem in the rat and cat. J. Comp. Neurol. 178, 225–254. doi: 10.1002/cne.901780204
Kudo, T., Konno, K., Uchigashima, M., Yanagawa, Y., Sora, I., Minami, M., et al. (2014). GABAergic neurons in the ventral tegmental area receive dual GABA/enkephalin-mediated inhibitory inputs from the bed nucleus of the stria terminalis. Eur. J. Neurosci. 39, 1796–1809. doi: 10.1111/ejn.12503
Kudo, T., Uchigashima, M., Miyazaki, T., Konno, K., Yamasaki, M., Yanagawa, Y., et al. (2012). Three types of neurochemical projection from the bed nucleus of the stria terminalis to the ventral tegmental area in adult mice. J. Neurosci. 32, 18035–18046. doi: 10.1523/JNEUROSCI.4057-12.2012
Kushida, C. A., Zoltoski, R. K., and Gillin, J. C. (1995). The expression of m1–m3 muscarinic receptor mRNAs in rat brain following REM sleep deprivation. NeuroReport 6, 1705–1708. doi: 10.1097/00001756-199508000-00027
Lebow, M. A., and Chen, A. (2016). Overshadowed by the amygdala: the bed nucleus of the stria terminalis emerges as key to psychiatric disorders. Mol. Psychiatry 21, 450–463. doi: 10.1038/mp.2016.1
Li, S.-B., Giardino, W. J., and de Lecea, L. (2017). Hypocretins and arousal. Curr. Top. Behav. Neurosci. 33, 93–104. doi: 10.1007/7854_2016_58
Li, S.-B., Nevárez, N., Giardino, W. J., and de Lecea, L. (2018). Optical probing of orexin/hypocretin receptor antagonists. Sleep 41:zsy141. doi: 10.1093/sleep/zsy141
Liu, Y., Li, Y., Yang, B., Yu, M., Zhang, X., Bi, L., et al. (2020). Glutamatergic neurons of the paraventricular nucleus are critical for the control of wakefulness. Neuroscience 446, 137–144. doi: 10.1016/j.neuroscience.2020.08.024
Luyck, K., Arckens, L., Nuttin, B., and Luyten, L. (2020). It takes two: bilateral bed nuclei of the stria terminalis mediate the expression of contextual fear, but not of moderate cued fear. Prog. Neuropsychopharmacol. Biol. Psychiatry 101:109920. doi: 10.1016/j.pnpbp.2020.109920
Luyck, K., Goode, T. D., Lee Masson, H., and Luyten, L. (2019). Distinct activity patterns of the human bed nucleus of the stria terminalis and amygdala during fear learning. Neuropsychol. Rev. 29, 181–185. doi: 10.1007/s11065-018-9383-7
Luyten, L. (2020). The bed nucleus of the stria terminalis: translational deep brain stimulation to reduce anxiety. Neuroscientist 26, 278–284. doi: 10.1177/1073858419898381
Luyten, L., Hendrickx, S., Raymaekers, S., Gabriels, L., and Nuttin, B. (2016). Electrical stimulation in the bed nucleus of the stria terminalis alleviates severe obsessive-compulsive disorder. Mol. Psychiatry 21, 1272–1280. doi: 10.1038/mp.2015.124
Malsbury, C. W., and McKay, K. (1987). A sex difference in the pattern of substance P-like immunoreactivity in the bed nucleus of the stria terminalis. Brain Res. 420, 365–370. doi: 10.1016/0006-8993(87)91258-3
Marcinkiewcz, C. A., Mazzone, C. M., D’Agostino, G., Halladay, L. R., Hardaway, J. A., DiBerto, J. F., et al. (2016). Serotonin engages an anxiety and fear-promoting circuit in the extended amygdala. Nature 537, 97–101. doi: 10.1038/nature19318
Mazzone, C. M., Pati, D., Michaelides, M., DiBerto, J., Fox, J. H., Tipton, G., et al. (2018). Acute engagement of Gq-mediated signaling in the bed nucleus of the stria terminalis induces anxiety-like behavior. Mol. Psychiatry 23, 143–153. doi: 10.1038/mp.2016.218
McElligott, Z. A., Fox, M. E., Walsh, P. L., Urban, D. J., Ferrel, M. S., Roth, B. L., et al. (2013). Noradrenergic synaptic function in the bed nucleus of the stria terminalis varies in animal models of anxiety and addiction. Neuropsychopharmacology 38, 1665–1673. doi: 10.1038/npp.2013.63
McHenry, J. A., Otis, J. M., Rossi, M. A., Robinson, J. E., Kosyk, O., Miller, N. W., et al. (2017). Hormonal gain control of a medial preoptic area social reward circuit. Nat. Neurosci. 20, 449–458. doi: 10.1038/nn.4487
Moffitt, J. R., Bambah-Mukku, D., Eichhorn, S. W., Vaughn, E., Shekhar, K., Perez, J. D., et al. (2018). Molecular, spatial, and functional single-cell profiling of the hypothalamic preoptic region. Science 362:eaau5324. doi: 10.1126/science.aau5324
Nagano, Y., Kaneda, K., Maruyama, C., Ide, S., Kato, F., and Minami, M. (2015). Corticotropin-releasing factor enhances inhibitory synaptic transmission to type III neurons in the bed nucleus of the stria terminalis. Neurosci. Lett. 600, 56–61. doi: 10.1016/j.neulet.2015.05.059
Nectow, A. R., and Nestler, E. J. (2020). Viral tools for neuroscience. Nat. Rev. Neurosci. 21, 669–681. doi: 10.1038/s41583-020-00382-z
Nguyen, A. Q., Dela Cruz, J. A., Sun, Y., Holmes, T. C., and Xu, X. (2016). Genetic cell targeting uncovers specific neuronal types and distinct subregions in the bed nucleus of the stria terminalis. J. Comp. Neurol. 524, 2379–2399. doi: 10.1002/cne.23954
Oler, J. A., Tromp, D. P., Fox, A. S., Kovner, R., Davidson, R. J., Alexander, A. L., et al. (2017). Connectivity between the central nucleus of the amygdala and the bed nucleus of the stria terminalis in the non-human primate: neuronal tract tracing and developmental neuroimaging studies. Brain Struct. Funct. 222, 21–39. doi: 10.1007/s00429-016-1198-9
Ottenheimer, D. J., Wang, K., Haimbaugh, A., Janak, P. H., and Richard, J. M. (2019). Recruitment and disruption of ventral pallidal cue encoding during alcohol seeking. Eur. J. Neurosci. 50, 3428–3444. doi: 10.1111/ejn.14527
Patriarchi, T., Cho, J. R., Merten, K., Howe, M. W., Marley, A., Xiong, W. H., et al. (2018). Ultrafast neuronal imaging of dopamine dynamics with designed genetically encoded sensors. Science 360:eaat4422. doi: 10.1126/science.aat4422
Pezuk, P., Aydin, E., Aksoy, A., and Canbeyli, R. (2008). Effects of BNST lesions in female rats on forced swimming and navigational learning. Brain Res. 1228, 199–207. doi: 10.1016/j.brainres.2008.06.071
Pina, M. M., and Cunningham, C. L. (2017). Ethanol-seeking behavior is expressed directly through an extended amygdala to midbrain neural circuit. Neurobiol. Learn. Mem. 137, 83–91. doi: 10.1016/j.nlm.2016.11.013
Pleil, K. E., Rinker, J. A., Lowery-Gionta, E. G., Mazzone, C. M., McCall, N. M., Kendra, A. M., et al. (2015). NPY signaling inhibits extended amygdala CRF neurons to suppress binge alcohol drinking. Nat. Neurosci. 18, 545–552. doi: 10.1038/nn.3972
Pomrenze, M. B., Giovanetti, S. M., Maiya, R., Gordon, A. G., Kreeger, L. J., and Messing, R. O. (2019a). Dissecting the roles of gaba and neuropeptides from rat central amygdala CRF neurons in anxiety and fear learning. Cell Rep. 29, 13.e14–21.e14. doi: 10.1016/j.celrep.2019.08.083
Pomrenze, M. B., Tovar-Diaz, J., Blasio, A., Maiya, R., Giovanetti, S. M., Lei, K., et al. (2019b). A corticotropin releasing factor network in the extended amygdala for anxiety. J. Neurosci. 39, 1030–1043. doi: 10.1523/JNEUROSCI.2143-18.2018
Pomrenze, M. B., Millan, E. Z., Hopf, F. W., Keiflin, R., Maiya, R., Blasio, A., et al. (2015). A transgenic rat for investigating the anatomy and function of corticotrophin releasing factor circuits. Front. Neurosci. 9:487. doi: 10.3389/fnins.2015.00487
Poulin, J.-F., Arbour, D., Laforest, S., and Drolet, G. (2009). Neuroanatomical characterization of endogenous opioids in the bed nucleus of the stria terminalis. Prog. Neuropsychopharmacol. Biol. Psychiatry 33, 1356–1365. doi: 10.1016/j.pnpbp.2009.06.021
Radley, J. J., and Johnson, S. B. (2018). Anteroventral bed nuclei of the stria terminalis neurocircuitry: towards an integration of HPA axis modulation with coping behaviors—Curt Richter Award Article 2017. Psychoneuroendocrinology 89, 239–249. doi: 10.1016/j.psyneuen.2017.12.005
Raymaekers, S., Vansteelandt, K., Luyten, L., Bervoets, C., Demyttenaere, K., Gabriels, L., et al. (2017). Long-term electrical stimulation of bed nucleus of stria terminalis for obsessive-compulsive disorder. Mol. Psychiatry 22, 931–934. doi: 10.1038/mp.2016.124
Reichard, R. A., Subramanian, S., Desta, M. T., Sura, T., Becker, M. L., Ghobadi, C. W., et al. (2017). Abundant collateralization of temporal lobe projections to the accumbens, bed nucleus of stria terminalis, central amygdala and lateral septum. Brain Struct. Funct. 222, 1971–1988. doi: 10.1007/s00429-016-1321-y
Resstel, L. B., Alves, F. H., Reis, D. G., Crestani, C. C., Correa, F. M., and Guimaraes, F. S. (2008). Anxiolytic-like effects induced by acute reversible inactivation of the bed nucleus of stria terminalis. Neuroscience 154, 869–876. doi: 10.1016/j.neuroscience.2008.04.007
Rigney, N., Whylings, J., Mieda, M., de Vries, G., and Petrulis, A. (2019). Sexually dimorphic vasopressin cells modulate social investigation and communication in sex-specific ways. eNeuro 6:ENEURO.0415-18.2019. doi: 10.1523/ENEURO.0415-18.2019
Rinker, J. A., Marshall, S. A., Mazzone, C. M., Lowery-Gionta, E. G., Gulati, V., Pleil, K. E., et al. (2017). Extended amygdala to ventral tegmental area corticotropin-releasing factor circuit controls binge ethanol intake. Biol. Psychiatry 81, 930–940. doi: 10.1016/j.biopsych.2016.02.029
Rodrigo-Angulo, M. L., Heredero, S., Rodriguez-Veiga, E., and Reinoso-Suarez, F. (2008). GABAergic and non-GABAergic thalamic, hypothalamic and basal forebrain projections to the ventral oral pontine reticular nucleus: their implication in REM sleep modulation. Brain Res. 1210, 116–125. doi: 10.1016/j.brainres.2008.02.095
Rodriguez-Romaguera, J., Ung, R. L., Nomura, H., Otis, J. M., Basiri, M. L., Namboodiri, V. M. K., et al. (2020). Prepronociceptin-expressing neurons in the extended amygdala encode and promote rapid arousal responses to motivationally salient stimuli. Cell Rep. 33:108362. doi: 10.1016/j.celrep.2020.108362
Rodríguez-Sierra, O. E., Turesson, H. K., and Pare, D. (2013). Contrasting distribution of physiological cell types in different regions of the bed nucleus of the stria terminalis. J. Neurophysiol. 110, 2037–2049. doi: 10.1152/jn.00408.2013
Ryabinin, A. E., Tsoory, M. M., Kozicz, T., Thiele, T. E., Neufeld-Cohen, A., Chen, A., et al. (2012). Urocortins: CRF’s siblings and their potential role in anxiety, depression and alcohol drinking behavior. Alcohol 46, 349–357. doi: 10.1016/j.alcohol.2011.10.007
Saunders, B. T., Richard, J. M., and Janak, P. H. (2015). Contemporary approaches to neural circuit manipulation and mapping: focus on reward and addiction. Philos. Trans. R. Soc. Lond. B Biol. Sci. 370:20140210. doi: 10.1098/rstb.2014.0210
Schank, J. R., Ryabinin, A. E., Giardino, W. J., Ciccocioppo, R., and Heilig, M. (2012). Stress-related neuropeptides and addictive behaviors: beyond the usual suspects. Neuron 76, 192–208. doi: 10.1016/j.neuron.2012.09.026
Schwarz, L. A., Miyamichi, K., Gao, X. J., Beier, K. T., Weissbourd, B., DeLoach, K. E., et al. (2015). Viral-genetic tracing of the input-output organization of a central noradrenaline circuit. Nature 524, 88–92. doi: 10.1038/nature14600
Shackman, A. J., and Fox, A. S. (2016). Contributions of the central extended amygdala to fear and anxiety. J. Neurosci. 36, 8050–8063. doi: 10.1523/JNEUROSCI.0982-16.2016
Silberman, Y., Matthews, R. T., and Winder, D. G. (2013). A corticotropin releasing factor pathway for ethanol regulation of the ventral tegmental area in the bed nucleus of the stria terminalis. J. Neurosci. 33, 950–960. doi: 10.1523/JNEUROSCI.2949-12.2013
Smith, M. A., Choudhury, A. I., Glegola, J. A., Viskaitis, P., Irvine, E. E., de Campos Silva, P. C. C., et al. (2020). Extrahypothalamic GABAergic nociceptin-expressing neurons regulate AgRP neuron activity to control feeding behavior. J. Clin. Invest. 130, 126–142. doi: 10.1172/JCI130340
Smith, J. B., Klug, J. R., Ross, D. L., Howard, C. D., Hollon, N. G., Ko, V. I., et al. (2016). Genetic-based dissection unveils the inputs and outputs of striatal patch and matrix compartments. Neuron 91, 1069–1084. doi: 10.1016/j.neuron.2016.07.046
Stephenson-Jones, M., Bravo-Rivera, C., Ahrens, S., Furlan, A., Xiao, X., Fernandes-Henriques, C., et al. (2020). Opposing contributions of GABAergic and glutamatergic ventral pallidal neurons to motivational behaviors. Neuron 105, 921.e25–933.e25. doi: 10.1016/j.neuron.2019.12.006
Sun, F., Zeng, J., Jing, M., Zhou, J., Feng, J., Owen, S. F., et al. (2018). A genetically encoded fluorescent sensor enables rapid and specific detection of dopamine in flies, fish, and mice. Cell 174, 481.e19–496.e19. doi: 10.1016/j.cell.2018.06.042
Terreberry, R. R., Oguri, M., and Harper, R. M. (1995). State-dependent respiratory and cardiac relationships with neuronal discharge in the bed nucleus of the stria terminalis. Sleep 18, 139–144. doi: 10.1093/sleep/18.3.139
Theiss, J. D., Ridgewell, C., McHugo, M., Heckers, S., and Blackford, J. U. (2017). Manual segmentation of the human bed nucleus of the stria terminalis using 3T MRI. NeuroImage 146, 288–292. doi: 10.1016/j.neuroimage.2016.11.047
Tsujino, N., and Sakurai, T. (2009). Orexin/hypocretin: a neuropeptide at the interface of sleep, energy homeostasis, and reward system. Pharmacol. Rev. 61, 162–176. doi: 10.1124/pr.109.001321
Vranjkovic, O., Pina, M., Kash, T. L., and Winder, D. G. (2017). The bed nucleus of the stria terminalis in drug-associated behavior and affect: a circuit-based perspective. Neuropharmacology 122, 100–106. doi: 10.1016/j.neuropharm.2017.03.028
Walker, L. C., Cornish, L. C., Lawrence, A. J., and Campbell, E. J. (2019). The effect of acute or repeated stress on the corticotropin releasing factor system in the CRH-IRES-Cre mouse: a validation study. Neuropharmacology 154, 96–106. doi: 10.1016/j.neuropharm.2018.09.037
Walter, A., Mai, J. K., Lanta, L., and Görcs, T. (1991). Differential distribution of immunohistochemical markers in the bed nucleus of the stria terminalis in the human brain. J. Chem. Neuroanat. 4, 281–298. doi: 10.1016/0891-0618(91)90019-9
Wang, Y., Kim, J., Schmit, M. B., Cho, T. S., Fang, C., and Cai, H. (2019). A bed nucleus of stria terminalis microcircuit regulating inflammation-associated modulation of feeding. Nat. Commun. 10:2769. doi: 10.1038/s41467-019-10715-x
Weissbourd, B., Ren, J., DeLoach, K. E., Guenthner, C. J., Miyamichi, K., and Luo, L. (2014). Presynaptic partners of dorsal raphe serotonergic and GABAergic neurons. Neuron 83, 645–662. doi: 10.1016/j.neuron.2014.06.024
Welch, J. D., Kozareva, V., Ferreira, A., Vanderburg, C., Martin, C., and Macosko, E. Z. (2019). Single-cell multi-omic integration compares and contrasts features of brain cell identity. Cell 177, 1873.e17–1887.e17. doi: 10.1016/j.cell.2019.05.006
Weller, K. L., and Smith, D. A. (1982). Afferent connections to the bed nucleus of the stria terminalis. Brain Res. 232, 255–270. doi: 10.1016/0006-8993(82)90272-4
Whylings, J., Rigney, N., Peters, N. V., de Vries, G. J., and Petrulis, A. (2020). Sexually dimorphic role of BNST vasopressin cells in sickness and social behavior in male and female mice. Brain Behav. Immun. 83, 68–77. doi: 10.1016/j.bbi.2019.09.015
Xia, F., and Kheirbek, M. A. (2020). Circuit-based biomarkers for mood and anxiety disorders. Trends Neurosci. 43, 902–915. doi: 10.1016/j.tins.2020.08.004
Xiao, Q., Zhou, X., Wei, P., Xie, L., Han, Y., Wang, J., et al. (2020). A new GABAergic somatostatin projection from the BNST onto accumbal parvalbumin neurons controls anxiety. Mol. Psychiatry [Epub ahead of print]. doi: 10.1038/s41380-020-0816-3
Yamauchi, N., Takahashi, D., Sugimura, Y. K., Kato, F., Amano, T., and Minami, M. (2018). Activation of the neural pathway from the dorsolateral bed nucleus of the stria terminalis to the central amygdala induces anxiety-like behaviors. Eur. J. Neurosci. 48, 3052–3061. doi: 10.1111/ejn.14165
Yang, C. F., Chiang, M. C., Gray, D. C., Prabhakaran, M., Alvarado, M., Juntti, S. A., et al. (2013). Sexually dimorphic neurons in the ventromedial hypothalamus govern mating in both sexes and aggression in males. Cell 153, 896–909. doi: 10.1016/j.cell.2013.04.017
Zahm, D. S. (1998). Is the caudomedial shell of the nucleus accumbens part of the extended amygdala? A consideration of connections. Crit. Rev. Neurobiol. 12, 245–265. doi: 10.1615/critrevneurobiol.v12.i3.50
Zahm, D. S., Grosu, S., Irving, J. C., and Williams, E. A. (2003). Discrimination of striatopallidum and extended amygdala in the rat: a role for parvalbumin immunoreactive neurons? Brain Res. 978, 141–154. doi: 10.1016/s0006-8993(03)02801-4
Keywords: bed nuclei of the stria terminalis, extended amygdala, neuropeptide, arousal, circuit, sleep, wakefulness, bed nucleus of stria terminalis (BNST)
Citation: Giardino WJ and Pomrenze MB (2021) Extended Amygdala Neuropeptide Circuitry of Emotional Arousal: Waking Up on the Wrong Side of the Bed Nuclei of Stria Terminalis. Front. Behav. Neurosci. 15:613025. doi: 10.3389/fnbeh.2021.613025
Received: 01 October 2020; Accepted: 15 January 2021;
Published: 09 February 2021.
Edited by:
Yuval Silberman, Pennsylvania State University, United StatesReviewed by:
Dennis Sparta, University of Maryland, Baltimore, United StatesAntonio Figueiredo, University of Maryland, Baltimore, United States in collaboration with reviewer DS
Yanhua Huang, University of Pittsburgh, United States
Copyright © 2021 Giardino and Pomrenze. This is an open-access article distributed under the terms of the Creative Commons Attribution License (CC BY). The use, distribution or reproduction in other forums is permitted, provided the original author(s) and the copyright owner(s) are credited and that the original publication in this journal is cited, in accordance with accepted academic practice. No use, distribution or reproduction is permitted which does not comply with these terms.
*Correspondence: William J. Giardino, d2lsbGdpYXJAc3RhbmZvcmQuZWR1; Matthew B. Pomrenze, cG9tcmVuemVAc3RhbmZvcmQuZWR1
† These authors have contributed equally to this work