- 1Department of Biomedical Sciences, Colorado State University, Fort Collins, CO, United States
- 2Department of Basic Medical Sciences, University of Arizona College of Medicine, Phoenix, AZ, United States
The hypothalamic-pituitary-adrenal axis is a complex system of neuroendocrine pathways and feedback loops that function to maintain physiological homeostasis. Abnormal development of the hypothalamic-pituitary-adrenal (HPA) axis can further result in long-term alterations in neuropeptide and neurotransmitter synthesis in the central nervous system, as well as glucocorticoid hormone synthesis in the periphery. Together, these changes can potentially lead to a disruption in neuroendocrine, behavioral, autonomic, and metabolic functions in adulthood. In this review, we will discuss the regulation of the HPA axis and its development. We will also examine the maternal-fetal hypothalamic-pituitary-adrenal axis and disruption of the normal fetal environment which becomes a major risk factor for many neurodevelopmental pathologies in adulthood, such as major depressive disorder, anxiety, schizophrenia, and others.
Introduction
Humans and animals respond to environmental perturbations with a stress response that allows physiological adaptation to the stressor to maintain homeostasis. A major component of the homeostatic response is the hypothalamic-pituitary-adrenal (HPA) axis, an intricate, yet robust, neuroendocrine mechanism that mediates the effects of stressors by regulating numerous physiological processes, such as metabolism, immune responses, and the autonomic nervous system (ANS). The HPA axis consists of a cascade of endocrine pathways that respond to specific negative feedback loops involving the hypothalamus, anterior pituitary gland, and adrenal gland.
There are several critical developmental stages must be attained to ensure proper functionality of the HPA axis and appropriate behavioral and physiological stress-responses in adulthood. The HPA axis begins to develop as early as fetal life and becomes sexually dimorphic during puberty due to differing levels of gonadal hormones. Early life exposure of the offspring to excess fetal glucocorticoid (GC) hormones or environmental perturbations, such as maternal stressors, can alter normal neuropeptide synthesis and lead to a disruption in the development of the HPA axis. This may become detrimental to the fetus later in life as it leads to abnormal physiological function in adulthood, thereby increasing the risk for adult disease.
In this review, we discuss the HPA axis as the central regulator of various physiological responses to stressors. We also examine the activational and organizational effects of hormones during critical periods of development that result in the sexually dimorphic responses of the HPA axis in adults. The effect of environmental perturbations, such as prenatal stress or prenatal exposure to synthetic GC hormones, and the associated susceptibility to stress-related neuropathologies in adulthood are also addressed.
Components of the HPA Axis
Morphology and Development of the Paraventricular Nucleus (PVN)
The paraventricular nucleus (PVN) houses three functional neuronal types that act as central regulators of the stress response: parvocellular, neurosecretory magnocellular, and long-projecting neurons. These neurons are characterized by their unique electrophysiological properties (Tasker and Dudek, 1991). Parvocellular neurons display small low threshold depolarizations, while long-projecting neurons generate large low-threshold depolarizations. In contrast, magnocellular neurons do not display low-threshold potentials but are characterized by a distinct return to baseline after depolarizing stimuli (Hernandez et al., 2015; Israel et al., 2016). Further investigation is necessary to identify specific mechanisms that lead to these unique properties.
Neurosecretory parvocellular neurons send their axons to the external zone of the median eminence to regulate the secretion of releasing factors [e.g., corticotropin-releasing hormone (CRH), thyrotropin-releasing hormone (TRH)] into the hypothalamohypophyseal portal vasculature to control the secretion of corresponding anterior pituitary hormones. An anterior parvocellular division extends from the rostral boundary of the PVN to the rostral boundary of the medial magnocellular division, just lateral to the periventricular area. The medial parvocellular division lies lateral to the periventricular area and medial to the medial magnocellular division. Neurons in the anterior and medial parvocellular groups project to the median eminence or other hypothalamic and extrahypothalamic regions.
Neurosecretory magnocellular neurons project to the neurohypophysis to regulate the secretion of oxytocin (OT) and arginine vasopressin (AVP) directly into the general circulation (Vandesande and Dierickx, 1975; Rhodes et al., 1981; Swanson and Sawchenko, 1983). The rat is the most studied species for characterizing PVN morphology and in the rat PVN, magnocellular neurons distribute into two distinct areas. The medial magnocellular division lies anteromedially within the PVN and contains mostly OT expressing neurons. The lateral magnocellular division is largely comprised of AVP-expressing neurons that are surrounded by a loop of OT neurons. Long-projecting neurons send their axons to the brainstem and spinal cord regions to control autonomic and somatosensory function.
The hypothalamus is derived from the anteroventral neuroectoderm during early development (Takata et al., 2017). Mapping of gene expression along the rostral-caudal axis shows that the early hypothalamic primordium differentiates into the floor plate, basal plate, and alar plate. The dorsal-most portion of the alar plate gives rise to the PVN and supraoptic nucleus (SON) and is identified by the expression of Brn-2, Otp, and Sim1 genes and the absence of Dlx, Arx, Gad67, Isl1, and Vax1 genes that are found in the subregion immediately below the PVN (Morales-Delgado et al., 2014).
The transcription factor, Brn-2 (POU-homeodomain protein BRIN-2), is endogenously expressed in both parvocellular and magnocellular neurons (He et al., 1989). Brn-2 null mutations in rodents demonstrate a failure to differentiate between CRH parvocellular neurons and OT and AVP magnocellular neurons, suggesting it is necessary for terminal differentiation of these hypothalamic cells (Schonemann et al., 1995).
The homeobox gene, Otp, transcribes a transcription factor that helps regulate differentiation and maturation of the neurosecretory PVN neurons expressing TRH, AVP, and OT. In mice, the induction of a missense mutation in the Otp gene causes acute onset obesity and increased anxiety, phenotypes that have similarly been shown to be modulated by AVP and OT. Moreover, Otp seems to be necessary for regulating the transcriptional activity of PVN neurons (Moir et al., 2017).
The Sim1 transcription factor acts as another key regulatory gene of the PVN, encoding a protein that also regulates AVP, TRH, and OT expression, as well as CRH and somatostatin (Michaud et al., 1998). Sim1 knock-out mice show severe loss of AVP, TRH, CRH, OT, and somatostatin neurons and rarely survive to adulthood (Michaud, 2001), while heterozygous mice display early obesity, hyperinsulinemia, hyperphagia, and hyperleptinemia, phenotypes that are associated with PVN neurosecretory neurons (Michaud, 2001). The Sim1 protein has been shown to dimerize with Aryl hydrocarbon receptor nuclear translocator 2 (ARNT2), which is thought to differentiate PVN and SON neurons. Brn2, a downstream target of the Sim1/ARNT2 dimer, also mediates Sim1 function. Brn2 promotes the expression of AVP, OT, and CRH in the PVN, and decreased numbers of these cell phenotypes are seen in Brn2 knock-out mice (Schonemann et al., 1995).
Morphology and Development of the Pituitary Gland
The pituitary gland functions largely in response to releasing factors from the hypothalamus. The pituitary gland is divided into two structures: the adenohypophysis (anterior pituitary) and the neurohypophysis (posterior pituitary; Dorton, 2000). The adenohypophysis constitutes 80% of the pituitary gland and houses specialized hormone-producing cells that synthesize and secrete several hormones, including, but not limited to, growth hormone (GH), thyroid-stimulating hormone (TSH), follicle-stimulating hormone (FSH), luteinizing hormone (LH), prolactin and adrenocorticotropic hormone (ACTH). These hormones target various types of tissues to mediate several physiological processes in response to stress (Scanes, 2015). Annexin-1 (formerly known as lipocortin 1) is another protein that exists in the anterior pituitary. While it is not directly involved in the synthesis of hormones discussed above, it is an important regulator of their secretion through inhibitory pathways (for a more detailed description of the actions of annexin-1, see reviews by John et al., 2004; Denef, 2008).
The adenohypophysis can be further divided into the pars distalis, pars intermedia, and the pars tuberalis. The pars distalis is composed of chromaffin and chromophobe cells and is where most hormone synthesis occurs. The pars tuberalis is an extension of the pars distalis and houses epithelial cells and the hypophyseal portal vessels that connect the anterior pituitary to the hypothalamus. The pars intermedia, located between the pars distalis and neurohypophysis, secretes products produced by the proopiomelanocortin (POMC) gene, particularly melanocyte-stimulating hormone (MSH; Ilahi and Ilahi, 2020).
In contrast to the adenohypophysis, the neurohypophysis is directly connected to the hypothalamus by axonal projections of magnocellular neurons originating from either the PVN or SON. The posterior pituitary stores OT and AVP synthesized by these neurons and secretes them into the general circulation in response to various hypothalamic releasing factors. OT is required for lactation, while AVP is involved in the regulation of osmotic balance (Borrow et al., 2019). Peptide hormones synthesized in the SON and PVN travel along axons to their terminals in the posterior pituitary where they are released into the general circulation in response to signals from their hypothalamic cell bodies (Ohbuchi et al., 2015).
The development of the pituitary gland is complex, yet unique because of its dual origin.
In humans, during the fourth week of gestation, cells of the oral portion of the ectoderm begin to thicken to form the hypophyseal placode (Ericson et al., 1998). The hypophyseal placode elongates to form Rathke’s pouch. At 6–8 weeks of development, the base of Rathke’s pouch is separated from the oral epithelium. The rapid proliferation of cells of the anterior wall of the pouch forms the anterior lobe of the pituitary (pars distalis) and the slower proliferation of cells of the posterior wall give rise to the intermediate lobe or pars intermedia. By contrast, a specific portion of the ventral diencephalon located dorsal to Rathke’s pouch gives rise to the infundibulum from which posterior pituitary originates (Amar and Weiss, 2003; Zhu et al., 2007).
The development of the pituitary gland is mediated by several cellular transcription factors. Sonic hedgehog, expressed in the oral ectoderm, and bone morphogenic protein 4, and fibroblast growth factor 8, found in the ventral diencephalon, are all important signaling genes that initiate cellular proliferation of pituitary cells. These genes have also been shown to affect the expression of transcription factors that contribute to the differentiation of specific pituitary lineages, however specific mechanisms are not yet known (Ericson et al., 1998). Furthermore, the transcription factor, Tpit, is critical for the expression of the POMC gene. POMC is expressed in corticotrophs, the first pituitary cell type to terminally differentiate (about gestation day 12.5 in the mouse; Pulichino et al., 2003). A deficiency of Tpit blocks terminal differentiation, but not a commitment to the corticotroph lineage (Pulichino et al., 2003). Somatotrophs, lactotrophs, and thyrotrophs are differentiated through the influence of transcription factors, Prop1 and Pit-1 whereas gonadotrophs require GATA-2 and SF1 signaling molecules for terminal differentiation. Although these terminal cell lineages are found in the pituitary gland, the existence of a common ancestral precursor pool is unclear (Zhao et al., 2001).
Morphology and Development of the Adrenal Gland
The adrenal gland of adult mammals is surrounded by a fibrous capsule and is composed of two regions with differing embryological origins (Bornstein et al., 1991). While the adrenal medulla, responsible for catecholamine production, derives from neuroectoderm, the steroid-hormone producing adrenal cortex has an embryonic origin from the adrenogonadal primordium (Sun et al., 2018).
The adrenal medulla is composed of chromaffin cells that secrete epinephrine and norepinephrine following sympathetic stimulation. They can be considered as a grouping of modified postganglionic neurons that are directly innervated by preganglionic neurons from the central nervous system (CNS; McCorry, 2007). Thus, the adrenal medulla is an important component of the ANS and responds very rapidly to stressors, releasing epinephrine and norepinephrine into the bloodstream to affect heart rate, blood pressure, metabolism, and others (Vinson et al., 1994). These hormones are classically involved in the “fight or flight” response. The effects of epinephrine and norepinephrine on various physiological systems are emphasized by changes noted in patients with pheochromocytoma, a catecholamine secreting neuroendocrine tumor of the adrenal chromaffin cells (Pacak, 2011). Symptoms include sweating, heart palpitations, markedly elevated blood pressure, nausea, tremors, and weight loss (Parenti et al., 2012).
Histologically, the adrenal cortex is composed of three zones. The outer zona glomerulosa produces aldosterone, which is involved in water and mineral balance through its actions on the kidney and colon (Rakova et al., 2017). The intermediate zona fasciculata is the thickest region of the adrenal cortex and synthesizes corticosteroids (primarily cortisol in the human, corticosterone in most rodents) and androgens. Similarly, the innermost zona reticularis also synthesizes adrenal androgens (Longcope, 1986). Of note, dehydroepiandrosterone (DHEA) is the most abundant circulating adrenal androgen in adult humans, whereas these are very low in adult rats and mice (Dumontet et al., 2019). Both the zona reticularis and fasciculata are regulated by ACTH and in the absence of ACTH, these zones atrophy, whereas following chronic ACTH stimulation, these zones hypertrophy (Gallo-Payet et al., 2017).
The adrenal cortex is derived from mesoderm and is dependent upon several transcription factors such as SF-1 (steroidogenic factor-1) and DAX-1 (dosage-sensitive sex reversal-adrenal hypoplasia). Deletion of either of these genes results in the absence of adrenocortical development in mice (Hammer et al., 2005). During human gestation, an inner fetal adrenal zone makes up the bulk of the adrenal gland, and an adult-like “definitive” zone, a group of small tightly packed cells is also present (Coulter, 2004). The human fetal adrenal responds to ACTH, but because of the absence of the 3-hydroxysteroid dehydrogenase enzyme, the fetal adrenal mainly produces DHEA and DHEA sulfate (Ishimoto and Jaffe, 2011). These fetal adrenal steroids serve as precursors of maternal placental estrogens. The definitive zone is the major producer of fetal cortisol in response to ACTH stimulation.
By contrast, the developing rodent adrenal is quiescent. It is questionable whether the rodent adrenal contains a fetal adrenal zone per se, although some studies indicate a transient fetal adrenal zone based on the presence of fetal adrenal enhancer elements (Zubair et al., 2006). While the adult cortex of rodents increases in size from late gestation through puberty, the fetal zone cells disappear gradually and accumulate along the boundary with the adrenal medulla (Morohashi and Zubair, 2011). However, even after the adult zones are developed, the adrenal gland of the rodent fetus does not yet express aldosterone synthase nor does it respond to stimulation by increasing mineralocorticoid or GC synthesis (Ehrhart-Bornstein et al., 1998).
The Physiology of Stress and Central Regulation of the HPA Axis
It is well established that animals and humans respond to threats to their welfare by activating neurons that control neuroendocrine and autonomic responses. For the HPA axis, the endocrine response is characterized by the secretion of GCs from the adrenal cortex. Circulating GCs act on a variety of tissues to mobilize energy stores, induce lipolysis and proteolysis, potentiate vasoconstriction driven by the ANS, suppress reproduction, and alter stress-related behaviors, to allow homeostasis (Papadimitriou and Priftis, 2009). Most of the physiological responses to acute elevations in GCs that occur following stressors, such as enhanced cognition and metabolism and inhibition of immune function must be beneficial, as they permit the “fight or flight” response. By contrast, although some benefits to chronic stress exist, chronic activation of the HPA axis has deleterious effects on immune, cardiovascular, metabolic, and neural functions and may decrease the resilience of neurons and glia to subsequent insults (McEwen, 1998; Jauregui-Huerta et al., 2010; Heck et al., 2020), resulting in increased risk for several diseases (see Figure 1 for summary describing effects of chronic stress on health conditions). Whether these are direct or indirect effects of glucocorticoids remains to be determined.
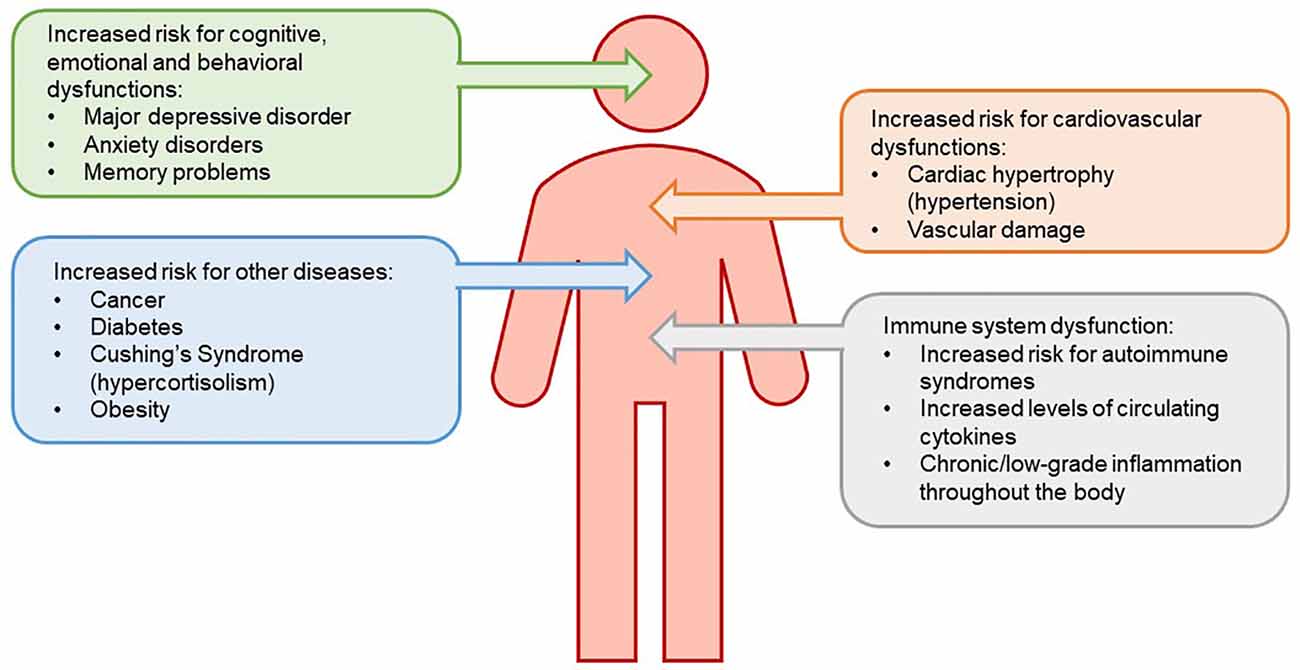
Figure 1. Chronic stress leads to reduced sensitivity of the negative feedback system that governs the hypothalamic-pituitary-adrenal (HPA) axis. The loss of this negative feedback system is due to an increased level of circulating glucocorticoids (GCs). HPA axis dysregulation results in downstream physiological consequences, increasing risk for immune system dysfunction, mood disorders, metabolic disease, and cardiovascular disease.
Negative Feedback Circuitry
The HPA axis is governed by a closed-loop GC-dependent negative feedback system that is essential for the termination of the stress response. Normal HPA function is highly influenced by the dose and duration of GC exposure (Abe and Critchlow, 1980; Sapolsky et al., 2000). For example, adrenalectomy decreases GC secretion, which increases PVN neuropeptide expression and secretion in both basal and stress-induced states (Sawchenko, 1987; Imaki et al., 1991). Negative feedback can also act at the level of the PVN, the anterior pituitary, and indirectly via brain regions that project to the PVN (Akana et al., 1986; Sawchenko, 1987; Bradbury et al., 1994). Notably, primary sites of negative feedback differ between endogenous and synthetic GC. Endogenous GCs, such as corticosterone, primarily induce negative feedback at the level of the PVN, while the synthetic GC, dexamethasone (DEX), functions as a glucocorticoid receptor (GR) agonist to inhibit GC release at the level of the anterior pituitary gland (Spiga et al., 2017).
GC-dependent negative feedback has further been shown to rely on the rhythmic release of GC in diurnal and ultradian patterns that are fundamental to the termination of the stress response (Sapolsky et al., 2000; Gjerstad et al., 2018). In all vertebrates, a peak of circulating corticosterone occurs just before the onset of daily activity. The diurnal rhythm of HPA axis activity is driven by the central circadian pacemaker, the suprachiasmatic nucleus (SCN), which has major inputs to the PVN to regulate daily rhythms of GC output (Lightman and Conway-Campbell, 2010). An ultradian variation in GC secretion is composed of the pulsatile release of corticosterone and ACTH that occur in response to differential timing of the stimulus and feedback signals within the HPA axis (Walker et al., 2012). In contrast to the circadian rhythm, the ultradian rhythm is not under central regulation of the SCN but is likely generated by a pituitary-adrenal feed-forward—feedback loop under constant CRH infusion. Moreover, when high levels of CRH were infused, the ultradian pattern was disrupted and corticosterone oscillations were dampened, further suggesting a dose-dependent effect of CRH on the patterns of GC secretion (Rankin et al., 2012).
Since circulating GCs can bind to both GR and mineralocorticoid receptor (MR), both receptors are involved in the negative feedback regulation of the HPA axis. MRs have a greater affinity for corticosterone (cortisol in humans; Reul and de Kloet, 1985) and consequently, they are predominantly bound during low or basal secretion of corticosteroid (Reul and de Kloet, 1985). Adrenalectomy increases basal CRH and ACTH levels, suggesting that a decrease in circulating corticosterone removes the negative feedback signal (Dallman et al., 1985, 1987; Rabadan-Diehl et al., 1997) whereas corticosterone replacement at doses that bind MR selectively, returns ACTH levels to baseline (Bradbury et al., 1994). Moreover, the hippocampus (HC), a primary site for HPA regulation during basal states, expresses MR at high levels. MR antagonists administered directly to the rat HC elevate basal ACTH and corticosterone levels like those seen after adrenalectomy (Van Haarst et al., 1997). Studies using transgenic mice that overexpress forebrain MR show reductions in the corticosterone response to restraint and decreases in anxiety-like behaviors (Rozeboom et al., 2007). Together, such data suggest that the ratio of MR:GR are as influential as absolute levels for regulating stress-induced HPA axis activity and stress-related behaviors.
In contrast to MR, GR has a lesser affinity for corticosterone and is thought to be the primary target for negative feedback when GC levels are elevated (Ruel and de Kloet, 1985). GRs remain mostly unoccupied during the basal state but are quickly occupied after a stress-induced increase in circulating GCs (Reul and de Kloet, 1985). This supports the hypothesis that GR activation the return of HPA activity to baseline following high amplitude secretion of corticosteroids after an acute stressor. Like MR, GR is highly expressed in the HC, as well as in the PVN and adenohypophysis (Ahima and Harlan, 1990; Morimoto et al., 1996). Initiation of HPA axis negative feedback occurs via GR expressing neurons in the HC (Sapolsky et al., 1984) and the hypothalamus (Weidenfeld et al., 2002), following stress-induced elevations in corticosterone. At the PVN, this first acts upon AVP neurons (Kovács et al., 2000). The importance of GR in negative feedback regulation is further demonstrated using a transgenic mouse model. Selective knockout of forebrain GR causes an increase in basal and stress-induced corticosterone levels (Kolber and Muglia, 2009), further implicating GC binding sites in the forebrain. In comparison, Wei et al. (2004) showed that the overexpression of forebrain GR does not alter basal ACTH or corticosterone levels, indicating that the forebrain GR is not the only player in regulating the baseline activity of the HPA axis. Selective disruption of GR in the PVN increased CRH immunoreactivity in the PVN, with corresponding increases in levels of plasma ACTH and corticosterone, supporting the hypothesis that GR is involved in negative feedback (Solomon et al., 2015). Furthermore, GR has been reported to be absent in the SCN, suggesting an alternate circadian-like feedback mechanism where GC influences HPA axis activity as discussed previously.
Regulation of CRH in the Brain and Pituitary
CRH is abundantly produced in neurons of the PVN as well as other brain areas and is highly conserved between humans, rats, and mice (Wamsteeker-Cusulin et al., 2013). While the predominant site of CRH expression is the PVN, CRH is expressed in other brain areas including the central n. of the amygdala (AMY; CeA), the bed n. of the stria terminalis (BNST), and the cortex and HC. It is well-known that stressors can similarly increase crh expression in the PVN and CeA (Herman and Tasker, 2016) with increases in the primary transcript (heterologous nuclear RNA) for crh, rising within minutes following the application of a stressor (Evans et al., 2013). This is followed by subtler increases in crh mRNA (Vazquez et al., 2006). Following enhanced cellular activity such as following a kainic acid-induced seizure, crh expression also increases and numerous CRH-ir neurons can be visualized in brain areas that normally express modest levels of CRH, including the HC, BNST, and globus pallidus (Foradori et al., 2007). Within the PVN, CRH is expressed by both pre-autonomic neurons that project to the brainstem and spinal cord (Swanson and Kuypers, 1980), as well as neuroendocrine neurons that project to the median eminence.
The actions of CRH are mediated by two receptor types, CRF-R1 and CRF-R2. The cloning and characterization of the CRH receptor were originally reported by Chen et al. (1993). This receptor-bound CRH with high affinity and selectivity and was coupled to adenylate cyclase to increase intracellular cyclic adenosine monophosphate (cAMP). Subsequently, a second CRH receptor, CRF-R2, was found to possess approximately 70% homology to CRF-R1. CRF-R2 had a different distribution in the brain, being found in subcortical regions, with the greatest expression in the lateral septum and ventromedial n. (VMN), in contrast to the CRF-R1 which was found to be highly expressed in neocortical and cerebellar regions. In the adenohypophysis, CRF-R1 was expressed at very high levels whereas CRF-R2 was expressed at much lower levels (Chalmers et al., 1995). Such studies indicate widespread effects of CRH with physiological responses selectively mediated by two different receptors.
CRH action at the pituitary is primarily mediated by CRF-R1, yet, its actions can be modified by a CRH binding protein (CRH-BP) which is highly expressed by both pituitary and hypothalamus, although only at low levels in the PVN (Chalmers et al., 1995). CRH-BP is found in cells expressing CRH receptors and in plasma and rapidly binds CRH with high affinity, thereby acting as a competitive inhibitor of CRH actions (Kalin, 2018). A sex difference in CRH-BP mRNA has been shown in the mouse pituitary with females having much greater levels than males (Speert et al., 2002). Moreover, stress increased CRH-BP mRNA and protein expression in POMC neurons in females more than males. Whereas data show that CRH-BP deficient mice exhibit elevated corticosterone levels (Speert et al., 2002), indicating that CRH-BP is functionally involved in preventing the actions of CRH to reduce the HPA response to stress, this is inconsistent with the greater basal and stress-induced levels of plasma corticosterone in females, suggesting other mechanisms are also involved in setting up sex differences in HPA function.
CRH as a Regulator of ACTH
ACTH is synthesized from high molecular weight (266 amino acids) precursor protein, POMC, in the anterior pituitary (Harno et al., 2018). The POMC gene consists of three exons and two introns. All functional peptide products of the POMC gene are encoded in exon 3 including N-terminal glycopeptide, Υ-MSH, ACTH, α-MSH, CLIP, β-lipoprotein, Υ-lipoprotein, β-MSH, and β-endorphin. Their evolutionary importance is indicated by the fact that the regions encoding α-MSH, ACTH, and β−endorphin are highly homologous between mammalian species including humans. Consistent with the important role of ACTH in mediating the neuroendocrine stress response, POMC mRNA levels in the anterior pituitary are upregulated by CRH and inhibited by GCs (Deng et al., 2015, 2017). By contrast, in the intermediate lobe, there is no effect of GCs on POMC mRNA levels due to the very low expression of GR (Wang et al., 2015).
AVP and OT as a Regulator of ACTH
While CRH is the primary regulator of ACTH secretion by the adenohypophysis, supporting roles of AVP and OT as co-secretagogues have also been shown (Herman et al., 1992). Although AVP has been described as regulating osmotic balance, and OT as a principal hormone for parturition, both neuropeptides are co-expressed in about half of the parvocellular CRH-expressing neurons of the PVN after adrenalectomy (Sawchenko et al., 1984). Both are thought to be co-released with CRH (Bondy et al., 1989; Raadsheer et al., 1993) to potentiate CRH’s secretagogue activity at the corticotroph (Rivier and Vale, 1983; Schlosser et al., 1994). Moreover, both AVP and OT can stimulate ACTH secretion even in the absence of CRH (Gillies et al., 1982; Schlosser et al., 1994) by activating the V1b receptor on corticotrophs (Schlosser et al., 1994). In contrast, when applied to the PVN, or following intracerebroventricular injection, OT and AVP inhibit HPA axis responses (Neumann et al., 2000; Landgraf and Neumann, 2004). It has also been shown that these neuropeptides modify PVN function in a paracrine fashion through local dendritic release within the PVN (Neumann, 2007), the net result being regulatory effects on the PVN that are very different from actions on the adenohypophysis.
In the adenohypophysis, AVP is hypothesized to also arise by collateral projections to the median eminence from magnocellular neurons that target the posterior pituitary, or through vessels from the posterior pituitary that connect to the adenohypophysis. Interestingly, the mouse does not seem to co-express AVP in CRH neurons to the same extent as the rat (Biag et al., 2012) suggesting that co-release with crh may be minimal in the mouse. Nonetheless, AVP drives the rapid release of ACTH from anterior pituitary corticotrophs, although, by itself, AVP alone causes only a small ACTH response. However, AVP potentiates CRH-driven ACTH release in vivo and in vitro, when cells are first exposed to CRH. In contrast, if cells are first exposed to AVP, CRH does not potentiate AVP-stimulated ACTH secretion (Roper et al., 2011). Thus, it appears that AVP and CRH can cooperate, but not substitute for each other to regulate ACTH secretion.
Neuroendocrine Stress Responses and Central Inputs
To cope with the physiological changes following a stressor, parvocellular neurons of the PVN integrate neural or hormonal input from a variety of sources leading to a physiologic and metabolic response. Direct inputs arising from the brainstem are essential to integrating HPA reactions to systemic stressors. Projections originating from noradrenergic and adrenergic neurons in the nucleus of the solitary tract (NTS), locus coeruleus, and the ventrolateral medulla that innervate the parvocellular PVN have been identified (Kítazawa et al., 1987; Hornby and Piekut, 1989; Cunningham et al., 1990). CRH neurons of the medial parvocellular PVN receive noradrenergic innervation from A2 adrenergic cell groups of the NTS (Kítazawa et al., 1987; Hornby and Piekut, 1989). The co-expression of alpha(1) and alpha(2) receptors in medial parvocellular CRH neurons (Cummings and Seybold, 1988; Day et al., 1997, 1999) allows norepinephrine to rapidly increase crh mRNA (Itoi et al., 1999; Cole and Sawchenko, 2002; Khan et al., 2007). Alpha(1) adrenergic receptors may be primarily responsible for the stimulatory effects of norepinephrine (Cummings and Seybold, 1988; Kiss and Aguilera, 1992; Khan et al., 2007), whereas alpha(2) adrenergic receptors, specifically alpha(2A) and alpha(2C), may be essential in inhibition of norepinephrine release at the presynaptic membrane (Bucheler et al., 2002).
Another circuit that strongly influences HPA responses to stress are projections from the median and dorsal raphe nuclei. Serotonergic fibers to the parvocellular PVN (Sawchenko et al., 1983; Zhang and Fogel, 2002) stimulate the HPA axis (Van de Kar and Blair, 1999). Serotonin (5-HT) 2C receptors have been implicated in 5-HT-induced activation of the HPA axis (Heisler et al., 2007). However, 5-HT1A receptors have also been shown to increase ACTH secretion (Rossi et al., 2010) and knockout of 5-HT1b receptors causes a 50% reduction in the diurnal rise in plasma corticosterone (Sollars et al., 2014). Restraint-induced elevations in ACTH and corticosterone can be increased by blocking 5-HT7 receptors (García-Iglesias et al., 2013), while 5-HT can inhibit GABAergic synaptic transmission at the PVN (Lee et al., 2008) providing evidence that the effect of 5-HT on PVN neurons vary depending on where the afferents terminate.
Limbic Pathways Regulate CRH Neuron Function
Important information to PVN neurons also arises from a variety of limbic structures, including the BNST, a group of related subnuclei that directly project to the parvocellular PVN (Cullinan et al., 1993; Dong et al., 2001). BNST neurons express androgen receptor (AR) and estrogen receptor (ER; Simerly, 1993), and play a crucial role in gonadal steroid regulation of HPA function. The majority of these neurons are GABAergic (Cullinan et al., 1993) and their activity can be enhanced by collateral CRH afferents (Kash and Winder, 2006; Dabrowska et al., 2011). The BNST also contains CRH neurons that project to the PVN (Dong et al., 2001; Dong and Swanson, 2006) and contain ARs (Heck and Handa, 2019a). Lesion studies show that BNST GABAergic neurons inhibit CRH mRNA levels, and inhibit the corticosterone responses to stress (Dunn, 1987; Herman et al., 1994) as does treatment with androgens (Lund et al., 2005). However, not all BNST neurons are inhibitory since selective lesions of the anterior or lateral BNST can decrease ACTH secretion (Gray et al., 1993; Herman et al., 1994). Nonetheless, the BNST represents an important modulating region that is gonadal steroid hormone-sensitive.
Other limbic regions known to modulate stress-responsive HPA axis activity include the HC, prefrontal cortex (PFC), CeA, medial AMY (MeA), and lateral septum. These regions do not directly innervate parvocellular PVN but relay through areas such as the BNST or peri-PVN (Dong et al., 2001; McKlveen et al., 2015). The predominantly glutamatergic projections from HC and PFC (Walaas and Fonnum, 1980) are translated to inhibitory actions on the HPA axis via the GABAergic nature of these relays (Diorio et al., 1993; Herman et al., 1998; Figueiredo et al., 2003). By contrast, AMY to BNST and peri-PVN projections are largely GABAergic. Thus, reducing the inhibitory tone is an effective mechanism to increase HPA axis activity (Swanson and Petrovich, 1998).
Development of Neuropeptides and Their Regulation
Development of CRH
In rats, Crh mRNA is detected as early as embryonic day 17 in the PVN (Grino et al., 1989), while the peptide is found to be expressed the following embryonic day 18 (Bugnon et al., 1982). In contrast to the CRH peptide, Crh mRNA is robustly expressed during embryonic days 18 and 19, followed by a reduction on days 20 and 21. CRH finally reaches adult levels of expression by the end of postnatal day (PND) 7 (Grino et al., 1989). In the mouse hypothalamus, CRH expression is detected initially on embryonic day 13, but mimics the same trend as the rat and decreases just before the time of birth (embryonic day 17–18 in mice), followed by an increase to adult levels thereafter (Schmidt et al., 2003).
Development of AVP and OT
In all species examined, AVP mRNA has been detected during development before OT mRNA, and in greater quantities (Wolf et al., 1984). In rodent studies, immunohistochemistry (Whitnall et al., 1985), quantitative PCR (Lipari et al., 2001), quantitative immunoassay (Sinding et al., 1980), and in situ hybridization assays (Laurent et al., 1989), show that AVP is consistently found in the developing fetus’ brain, while OT is not significantly expressed until 1–2 days following parturition (Yamashita et al., 1988; Laurent et al., 1989). Interestingly, more recently, Tamborski et al. (2016) detected OT mRNA as early as embryonic day 16 in females, but not until PND 2 in males, indicating a sex difference in the development of central oxytocin that may contribute to sex differences in adulthood. The AVP circuitry also becomes sexually dimorphic with the greater synthesis of AVP in males than females during early postnatal stages (for more details, see reviews: Szot and Dorsa, 1993; Rood and De Vries, 2011; Rood et al., 2013). In adulthood, many AVP populations of neurons are androgen-responsive (de Vries and Al-Shamma, 1990). Both AVP and OT expressing-neurons continue to increase postnatally in the PVN, and SON of the hypothalamus (Van Tol et al., 1987; Almazan et al., 1989; for further details of OT and AVP development, see reviews: di Scala-Guenot et al., 1990; Bales and Perkeybile, 2012).
Neurodevelopment of the HPA Axis
Estrogens and Androgens Have Activational Actions on the HPA Axis
Gonadal steroids are crucial hormones in the regulation of the adult HPA axis, resulting in stark differences in responsiveness of the axis between sexes. Studies show that testosterone generally depresses the stress response (Viau and Meaney, 1996) while estradiol can either enhance or inhibit it (Handa et al., 2009; Zuloaga et al., 2014). The actions of estrogens are controlled by two major types of ER, ERα and ERβ. ERs are ligand-activated transcription factors that bind to estrogen response elements (ERE) in gene promoters, thereby providing a link between gonadal hormones and transcriptional responses of the HPA axis (McEwen et al., 1999). ERα and ERβ both have unique yet overlapping expression patterns in the brain. ERα is highest in the VMN, arcuate n. (ARC) and medial preoptic area (MPOA), whereas ERβ is highly expressed in the PVN, SCN, and SON (in the rat). Overlapping brain regions containing both ERs include BNST, MeA, and MPOA (Hiroi et al., 2013). The unique expression of ERα and ERβ accounts for various physiological functions, specifically the HPA response to acute stress considering that EREs exist upstream of AVP, OT, and CRH gene promoters and can directly regulate gene transcription (Shapiro et al., 2000; Miller et al., 2004; Pak et al., 2007; Hiroi et al., 2013).
Estrogens have been shown to augment HPA axis activity and the release of the stress-related secretagogues at several sites due to the broad expression of ERs. In the adrenal gland, estradiol increases the adrenal response to ACTH administration (Patchev et al., 1996). Similarly, reports have shown that at the level of the adenohypophysis, estradiol results in a greater response to CRH demonstrated by increased ACTH secretion. Ovariectomy decreases HPA axis stress-responses and these effects can be reversed by replacement of estradiol to females (Seale et al., 2004). Increased PVN CRH immunoreactivity following estradiol treatment has also been shown (Patchev et al., 1996), with more recent studies displaying an overlap of ERβ expression in CRH neurons of the mouse PVN, further suggesting a possible mechanism for augmentation of HPA axis function by estrogens (Oyola et al., 2017). By contrast, some studies have demonstrated that estradiol decreases HPA axis activity or has no effect (Young et al., 2001; Ochedalski et al., 2007). Treatment with estradiol decreased neuronal activation in the PVN (Figueiredo et al., 2007), as well as reduced ACTH secretion (Young et al., 2001) and expression of Crh (Ochedalski et al., 2007). Varying reports of the effects of estradiol on the HPA axis could be explained by differing experimental conditions, such as a dose or duration-dependent effect (Figueiredo et al., 2007). Opposing effects of estradiol may also occur due to different signaling mechanisms of ERα and ERβ (Tsigos and Chrousos, 2002). In ovariectomized female rats, ERα agonist, propylpyrazoletriol, increases stress-induced GC secretion while diarylpropionitrile, an ERβ agonist, decreases stress-induced GC secretion (Weiser and Handa, 2009). It has also been proposed that ERα works by reducing the inhibitory tone of GABAergic neurons that project to the PVN, such as those in the BNST, hippocampus, and peri-PVN (Handa and Weiser, 2014) but has limited expression in PVN-specific neurons (Oyola et al., 2017), implicating indirect effects on the HPA axis (Weiser and Handa, 2009). Meanwhile, ERβ is proposed to directly alter HPA axis function since it is found to be co-expressed with several PVN neuropeptides, such as CRH, OT, and AVP (Lund et al., 2006; Oyola et al., 2017).
Androgens are consistently reported to inhibit HPA axis activation and activity (Rosinger et al., 2018; Heck and Handa, 2019a,b). Castration of male rodents removes endogenous androgens, increasing stress-induced secretion of ACTH and corticosterone (Handa et al., 2013). Further, testosterone or dihydrotestosterone replacement is consistently shown to reverse the inhibitory effects, reducing the ACTH and corticosterone response to an acute stressor, suggesting the inhibitory role of testosterone on the HPA axis (Williamson and Viau, 2008). The alterations in ACTH and corticosterone were not accompanied by alterations to CRH sensitivity in the pituitary, suggesting a more central-mediated effect of androgens. Recent studies (Seale et al., 2005b) show that the HPA axis is inhibited by testosterone directly through ARs or indirectly through metabolism with other co-regulatory elements that bind ARs or ERs.
Notably, the effects of dihydrotestosterone, a potent androgenic metabolite of testosterone, are important in the suppression of the HPA axis and GC secretion following stress. Central administration of a 5α-reductase inhibitor, finasteride, blunted the effects of testosterone, and not dihydrotestosterone, implicating a central role for the enzyme, 5α-reductase, in the reduction of testosterone to dihydrotestosterone in HPA reactivity to stress (Handa et al., 2013). Consistent with a study by Handa et al. (2013), dihydrotestosterone injected near the PVN in a gonadectomized, adult male decreases ACTH and corticosterone secretion (Lund et al., 2006). Androgens additionally are reported to decrease CRH response to stress in castrated males, indicating indirect action of testosterone on CRH synthesis since ARs are not expressed by hypophysiotropic CRH neurons (Bingaman et al., 1994). In rodent studies, dihydrotestosterone is metabolized intracellularly to 5α-androstane 3β, 17β-diol (3β-diol), which subsequently binds ERβ to inhibit the HPA axis. Thus, although interactions between androgen and estrogen actions can occur at the level of ligand identity, interactions on a molecular level are harder to identify. Recently, Mahfouz et al. (2016) showed a significant overlap between AR and ER in MPOA and hippocampus in rodents, using genome-wide spatial co-expression of AR and ER targets. Whether this overlap indicates functional interactions at a cellular level in rodents remains to be determined, with the caveat that additional factors may modify these cellular mechanisms in humans.
It has been proposed that sex differences arise, in part, due to varying levels of GR and MR and the availability of corticosteroids in the brain. Corticosteroid-binding globulin (CBG), a glycoprotein produced by the liver, binds to circulating corticosterone. CBG enhances corticosteroid stability during transport to target tissues, but it also prevents corticosteroids from binding to GR or MR (de Kloet et al., 2005). Only free corticosterone can exert physiological effects through its actions on its receptors. Thus, it is important to make a distinction when comparing total plasma corticosterone levels and available corticosterone. In females, CBG is found at levels that are 2-fold higher than in males, but levels of total corticosterone are also significantly higher. Therefore, the increased levels of CBG may help buffer the increased amount of total plasma corticosterone, contributing to the lack of a sex difference in free corticosterone levels (McCormick et al., 2002). Moreover, CBG binds acute stress-induced corticosterone, resulting in a delayed free corticosterone response in comparison to total plasma corticosterone, and implicating CBG as an important buffer for available corticosteroids (Qian et al., 2011). The greater levels of CBG in females likely contributes to the increased HPA axis activity when compared to males, whereas, low levels of CBG in males may lead to higher availability of free corticosterone and a more robust negative feedback on the HPA axis (Viau and Meaney, 1996; Tinnikov, 1999).
Evidence for Organizational Actions of Gonadal Steroids on HPA Axis
The sex differences in adult stress responses may also be programmed by neonatal exposure to gonadal steroids suggesting an organizational effect on the neural circuitry controlling patterns of corticosteroid secretion (Seale et al., 2005a,b) and shaping brain morphology in some brain sites controlling HPA axis function in adulthood (Green and McCormick, 2016). The mechanism(s) by which gonadal steroid hormones act to influence HPA function has not been completely resolved but evidence for androgens and estrogens modulating adrenal (Kitay, 1965), pituitary (Coyne and Kitay, 1969; Viau and Meaney, 2004) and hypothalamic functions (Handa et al., 1994; Viau and Meaney, 1996; Viau et al., 2001) has been reported. The considerable overlap in gonadal and adrenal steroid hormone receptor expression within the neural circuitry of the PVN supports this as a mechanism (Figure 2; see review Goel et al., 2014 for a more in-depth overview).
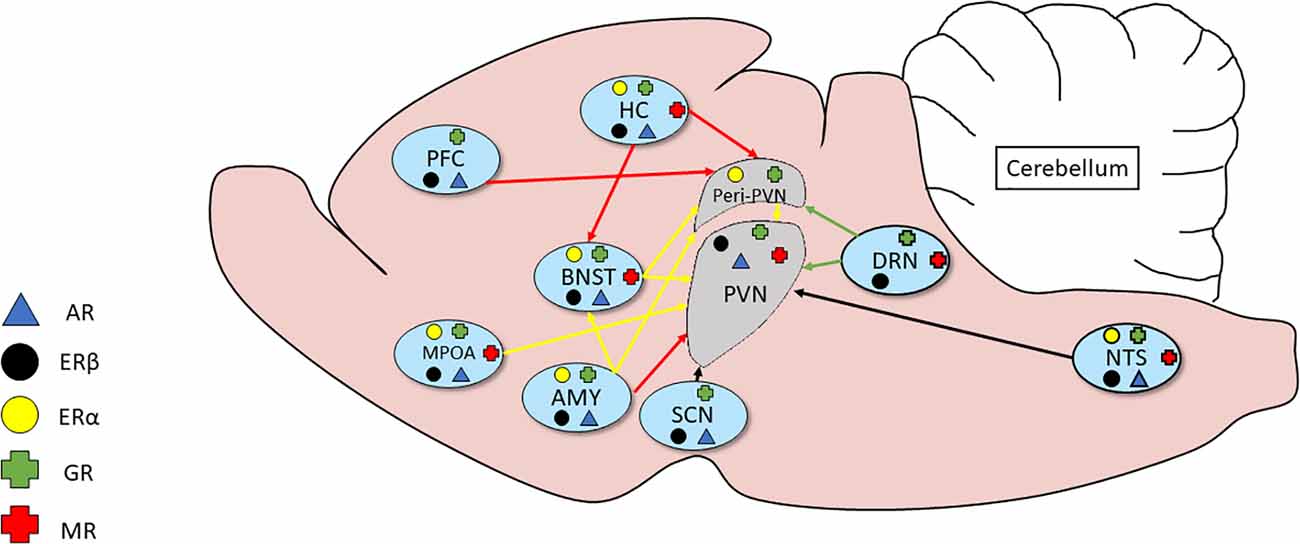
Figure 2. Relationship of inputs of adrenal and gonadal steroid hormone receptors to the circuitry of the HPA axis. Several brain regions secrete adrenal and gonadal hormones that act on receptors in the peri-PVN and paraventricular nucleus (PVN). Much of the expression shows considerable overlap.
There are two important periods during development in which a surge of testosterone has been reported to defeminize and masculinize the brain of male rodents: late gestation and shortly after birth (Weisz and Ward, 1980). Whereas many studies have examined the sexual differentiation of reproductive components of the brain, much less has been published regarding the organizational differentiation of the HPA axis. Postnatal gonadectomy of male rats has been reported to cause a more female-like HPA axis activity in adulthood, characterized by increased basal and stress-induced corticosterone secretion (Patchev et al., 1995). Further, inhibiting the aromatase enzyme to prevent the conversion of testosterone to estradiol in neonatal males, has similar lasting consequences of increased basal and stress-induced corticosterone in adulthood. These results implicate the actions of estradiol, as the result of the aromatization of testosterone in the male on the organization of sex differences in the HPA axis (Lucion et al., 1996; de Kloet et al., 1998). The organizational actions of perinatal steroids have been further supported by studies examining the pulsatile patterns of corticosterone secretion throughout the day, where males show a lower amplitude and frequency of corticosteroid pulses compared to females (Seale et al., 2005a). Administration of an AR antagonist in a perinatal male increases the amplitude and frequency of corticosteroid pulses to resemble that of adult females. Moreover, perinatal gonadectomy of males also leads to a female-like pulsatile pattern in adulthood, while a single dose of testosterone following gonadectomy reverses this effect. Moreover, females treated with testosterone within 24 h of birth show a male-like pattern of corticosterone secretion (Seale et al., 2005a,b). These studies implicate an organizational action of testosterone directly through AR or indirectly through ER, via the aromatization of testosterone to estradiol, on the HPA axis (Seale et al., 2005a,b).
Development of the HPA Axis During Early Life
During pregnancy, the stress response of the fetus is immature and relies heavily on inputs from the maternal and placental systems (Gunn et al., 2013). During late gestation, the fetus becomes capable of secreting CRH and ACTH in response to maternal stress, resulting in corticosterone production (Gunn et al., 2013). Basal levels of corticosterone during this time are similar to those of adults (Meaney et al., 1985b), suggesting functional HPA axis activity. From PND4 to PND14, basal corticosteroid levels drop, accompanied by substantially decreased ACTH and corticosterone production in response to stress (Schmidt et al., 2003). This period is known as the “stress hypo-responsive period” (SHRP; Buschdorf and Meaney, 2016). During this time, the expression of GR and MR mRNA is significantly increased (Yi et al., 1994). Accompanied by the lower levels of corticosterone, these changes are thought to dampen the HPA axis responses. Stress exposure during the SHRP induces a slight increase of expression of c-Fos mRNA in the PVN but does not influence ACTH or peripheral corticosterone secretion (Levine, 1994). Such data suggests an adrenal insensitivity may also play a role in the SHRP, but further mechanisms have not yet been determined.
Recent studies show that SHRP can be maintained through the influence of maternal care (Buschdorf and Meaney, 2016). Maternal care, quantified through observations of pup licking and maternal arch-backed nursing, are highly correlated with each other (Caldji et al., 1998). Dams categorized by levels of maternal care show a causal relationship to epigenetic reprogramming that alters negative feedback sensitivity through changes in DNA methylation and histone modifications (Weaver et al., 2004; Buschdorf and Meaney, 2016). Several studies suggest this may be related to the transcription factor, nerve growth factor-inducible protein A (NGFI-A) which binds to a promoter region on exon 1–7 of GR (Caldji et al., 1998) consisting of two CpG dinucleotides on opposing ends of the response element that are methylated on the day of birth. Offspring that received high maternal care show higher amounts of demethylation of 5′ CpG sites in the NGFI-A binding region in adulthood (Buschdorf and Meaney, 2016). Offspring that received low levels of maternal care displayed no change in methylation. Point mutation studies indicate that the NGFI-A binding strength and gene expression is determined by the presence of a methyl group at the 5′ CpG site, where a mutated 5′ CpG site resulted in increased transcription of NR3C1, the gene encoding GR (Weaver et al., 2004). These studies support the actions of low maternal care to program increased GR expression and increase feedback sensitivity of adult offspring (Liu et al., 1997). Additional studies show decreased corticosterone and ACTH responses to acute stress in adulthood of high maternal care-exposed offspring. Such data further support epigenetic reprogramming of the 5′ CpG site. A genome analysis of chromosome 18 containing NR3C1 found that varying amounts of maternal care correlated with changes in protocadherin loci (McGowan et al., 2011) which regulate the development of the CNS (McGowan et al., 2011) thereby implicating maternal care during the SHRP for proper brain development. However, it is important to note that the promoter region on exon 1–7 of GR only accounts for about 1% of all GR mRNA transcripts in the hippocampus. Reports examining promoter region on exon 1–7 of GR methylation found that upregulation of NGFI-A did not alter stress-induced activation of the promoter region on exon 1–7 of GR transcription or total expression of GR (Makino et al., 1995). Consistent with this, Witzmann et al. (2012) showed low methylation levels at the 5′ CpG site following acute stress, further indicating that NGFI may not drive the promoter region on exon 1–7 of GR transcription nor play a role during the acute stress response (Witzmann et al., 2012). Thus, while epigenetic reprogramming is altered through maternal care, specific mechanisms in which this occurs are still unclear.
Maternal separation during fetal development is another variable that influences HPA axis development and adult patterns of stress-reactivity (Boccia and Pederson, 2001). Prolonged separation from the dam is associated with a hyperactive HPA axis and increased anxiety- and depressive-like behaviors in adult offspring (Liu et al., 1997). In contrast, brief separation increases maternal attentiveness to pups, resulting in better attenuation of the stress-response (Boccia and Pederson, 2001). Rodent studies have demonstrated that these changes are partially a consequence of alterations in the dopaminergic system since prolonged maternal separation caused decreased dopamine uptake associated with changes in dopamine transporter expression (Curley et al., 2011). This is thought to lead to increased stress-induced dopamine activity resulting in a hyperactive HPA axis. 5-HT signaling has also been shown to be altered by maternal separation with decreased metabolism in the AMY and enhanced concentrations of 5-HT and associated metabolites in the dorsal raphe nucleus and cingulate cortex (Arborelius and Eklund, 2007). Long-term consequences include the altered function of 5-HT receptors and transporters, as well as decreased expression of 5-HT receptor subtypes in the PFC and hypothalamus (Ladd et al., 2005). These changes correlate with increased anxiety- and depressive-like behaviors, suggesting that a signaling pathway linking the dopaminergic and serotonergic systems with stress responses exists, however, specific mechanisms have yet to be elucidated (Zakharova et al., 2009).
Paternal influences on the stress axis of adult mice have also been reported. Males exposed to 6 weeks of chronic variable stress before breeding had offspring of both sexes with reduced HPA axis activation to acute restraint in adulthood (Rodgers et al., 2013). This correlated with gene transcription changes in the PVN and BNST of offspring suggesting the possibility of epigenetic reprogramming through the male lineage. Studies have also investigated paternal retrieval and grooming effects in offspring. Testosterone levels were decreased in offspring from rats with increased paternal retrieval, as was AVP expression in the BNST, and this correlated with reduced aggressiveness in social interaction tests, such as the resident-intruder test (Frazier et al., 2006). Such data suggests an important hormonal link between paternal care, testosterone levels, and aggression. AVP immunoreactivity in the PVN was also found to be increased with reduced paternal care (Rodgers et al., 2013), correlating with increased stress-induced corticosterone secretion, further linking paternal care and HPA axis responses. Nonetheless, specific mechanisms of how paternal transmission to the offspring occurs have yet to be elucidated.
The Development of the HPA Axis at Puberty
Puberty is a unique developmental event, influenced largely by the maturation of the hypothalamic-pituitary-gonadal (HPG) axis, which is responsible for gonadal maturation and adult hormone secretory patterns (Ojeda and Urbanski, 1994). Some reports also suggest that this represents a second critical period for organizational actions of gonadal hormones that further sculpt the HPA axis into its adult-like characteristics (Romeo, 2003; see review Romeo, 2010 for a more thorough analysis of age-dependent changes in the HPA axis).
Importantly, HPA axis reactivity is significantly greater before puberty than after puberty. Rat studies in males show an increased and prolonged stress-responsive release of ACTH and GC prepubertally in comparison to post-pubertal animals (Goldman et al., 1973; Romeo et al., 2004a). Similarly, the stress-induced activity of CRH neurons in the prepubertal PVN is greater than that of adults, demonstrating that the prolonged prepubertal pattern of corticosterone and ACTH may be driven by increased hypothalamic CRH synthesis (Romeo et al., 2004b; Viau et al., 2005) and altered by the onset of male puberty. These findings indicate a blunted GC-dependent negative feedback in prepubertal males (Romeo et al., 2004a).
Studies in pre-pubertal male rodents show elevated HPA activation, with increases in CRH activation following restraint in comparison to adults, indicating that PVN CRH neuron activity changes across puberty (Romeo et al., 2006). Following a corticosterone injection, Romeo and McEwen (2006) showed increased GR expression in regions of the brain, such as HC, AMY, and PFC, in adolescents compared to adults. This observation further indicated that puberty represents a critical period during development that renders the brain more vulnerable to environmental perturbations and increases the risk of HPA-related neuropathologies (Romeo and McEwen, 2006). The changes in the HPA axis do not appear to be the consequence of pubertal rises in testosterone (Romeo, 2003). However, because the initial increase of gonadotropin-releasing hormone (GnRH) secretion and kisspeptin occurs near the onset of puberty, one possibility is that changes in the HPA axis observed across puberty are preprogrammed developmental events that are independent of changes in gonadal hormones (Romeo, 2003).
Some reports further suggest that the pubertal rise in estradiol may also play a role in shaping the adult HPA axis. Studies in pre-pubertal females show an inhibitory effect of estradiol on stress-induced HPA axis function, while estradiol treatment in post-pubertal females shows a stimulatory effect of estradiol during the acute stress response (Evuarherhe et al., 2009). Further, regardless of whether females were ovariectomized before or after puberty, administration of estradiol consistently elevated basal and stress-induced GC secretion, as well as GC pulse amplitude and frequency (Evuarherhe et al., 2009). Data suggests there is a reversal effect of estradiol on HPA axis function during puberty where estradiol is inhibitory before puberty and stimulatory post-puberty, implying an estradiol-independent mechanism in the development of the HPA axis during puberty in adult females (Evuarherhe et al., 2009).
Disruption of the Maternal-Fetal HPA Axis and Adult Disease Risk
A growing body of studies has described fetal risk factors for adult diseases that form the basis for the hypothesis of the Developmental Origins of Health and Disease (DOHaD; Sandman et al., 2015). The DOHaD postulates that there is a critical period of development where the fetus is most sensitive to certain environmental influences that significantly impact short- and long-term health (Harris and Seckl, 2011). Such environmental influences include maternal stress, which is a likely correlate of fetal overexposure to GC, implying a common pathway in which environmental insults become linked between mother and fetus (Edwards et al., 1993; Cottrell and Seckl, 2009).
Commonly, administration of synthetic GCs has been a common clinical treatment for women at risk for preterm labor, to improve survival of the newborn by allowing proper lung maturation (Liggins and Howie, 1972; Crowther et al., 2019). Betamethasone or DEX is often used (Roberts and Dalziel, 2006) in the clinic and they do not appear to differ in efficacy (Crowther et al., 2019). DEX has also been used to treat female fetuses diagnosed with congenital adrenal hyperplasia (CAH; Speiser et al., 2010), an autosomal recessive disorder that results in the deficiency of 21-hydroxylase that impairs the synthesis of corticosterone and causes impaired synthesis of adrenal enzymes (Speiser et al., 2010). Therefore, steroid metabolism is redirected to adrenal androgens, resulting in abnormal masculinization of genital development and behaviors in females. Consequently, dexamethasone is administered to female CAH fetuses to inhibit adrenal androgen production and minimize these effects. Notably, these females represent a population that is exposed to dexamethasone early in gestation, a critical period for fetal development. More recent studies have begun to elucidate long- and short- term consequences of prenatal exposure to excess GC, causing programming effects in the HPA axis of the fetus that result in dysregulation of important physiological functions in adulthood.
A key player mediating the consequences of maternal elevations of GC from stress is the placenta. During pregnancy, GC concentrations in maternal blood are higher than those of the fetus. This is a result of 11β-Hydroxysteroid dehydrogenase type 2 (11β-HSD2) expression by the placenta (Stroud et al., 2016). 11β-HSD2 oxidizes maternal corticosteroids into its inactive 11-keto derivatives (Benediktsson et al., 1997; Holmes et al., 2006), which buffers the increased levels of maternal GCs to the fetus. Levels of 11β-HSD2 are known to increase during early and mid-gestation, followed by a drop in late gestation to allow GC mediated fetal lung maturation. Furthermore, rodent studies suggest that the drop in 11β-HSD2 during late gestation allows increased transport of maternal GC to the fetus, increasing the vulnerability of the fetus during this period to unwanted programming effects (Edwards et al., 1993). Inhibition of 11β-HSD2 with carbenoxolone during late gestation shows effects similar to excess GC, further suggesting the importance of 11β-HSD2 in protecting the fetus from overexposure of GC (Brown et al., 1993; Lindsay et al., 1996; Holmes et al., 2006). Importantly, the administration of synthetic GCs bypasses the 11β-HSD system of the placenta since synthetic GCs are not a substrate for the enzyme (Figure 3; Low et al., 1994; Walker et al., 1994).
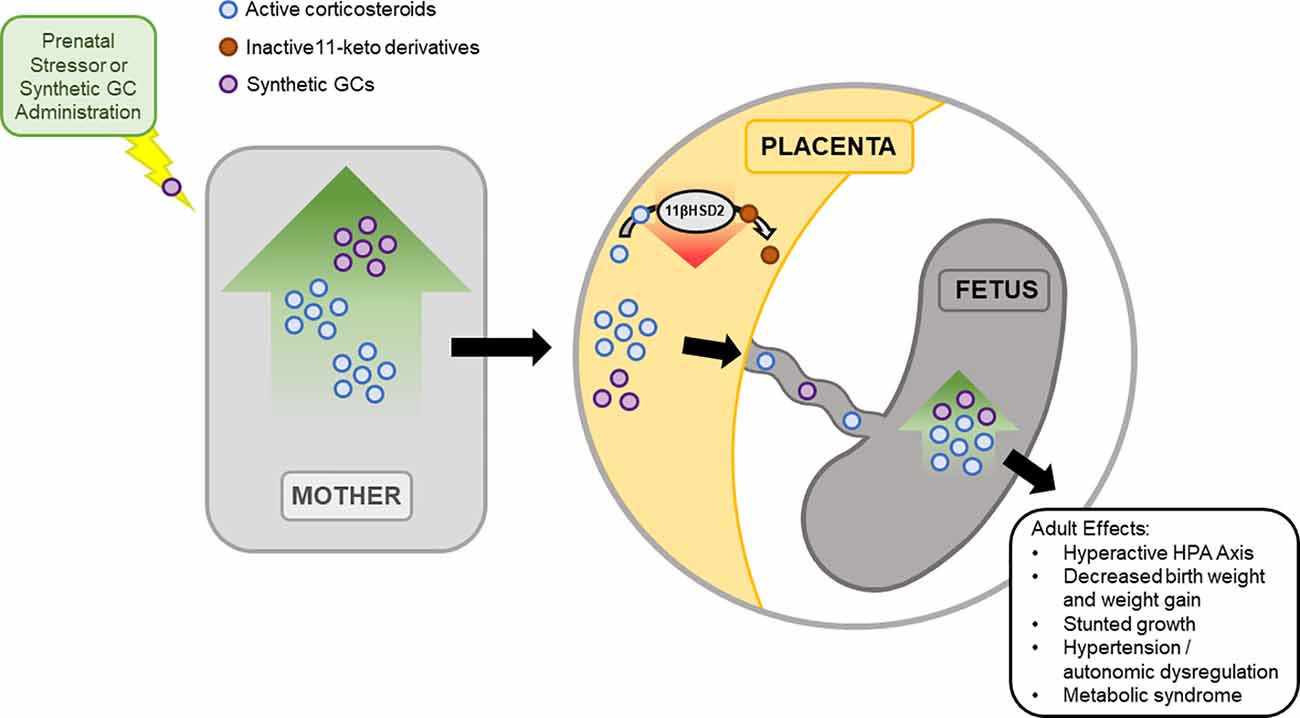
Figure 3. Prenatal exposure to excess GCs has short- and long- term effects. During pregnancy, 11-β hydroxysteroid dehydrogenase type 2 (11β-HSD2) expression in the placenta mediates the fetus’ exposure to excess maternal GC. 11β-HSD2 regulates the level of active GCs through the oxidation of maternal corticosteroids into inactive 11-keto derivatives, reducing fetal exposure to active GCs. While 11β-HSD2 concentration in the placenta is high during most of gestation, 11β-HSD2 levels drop during late gestation. As a result, fetal exposure to maternal GCs increases, leading to short term effects, observed immediately at birth into adulthood. To note, synthetic GC does not act as a substrate for 11β-HSD2 and bypasses this system to the fetus.
The short- and long- term effects on the fetus of maternal elevations of GC due to prenatal stress or prenatal exposure to synthetic GC have been found to depend on the length of exposure and time during development at which the insult occurs (Barbazanges et al., 1996). Fujioka et al. (1999) highlighted duration-dependent effects in rodents exposed to excess GCs in CRH-expressing PVN neurons, where chronic prenatal stress caused degeneration and apoptosis in these neurons (Baquedano et al., 2011). However, brief prenatal stress did not cause a change in CRH-expressing PVN neurons, suggesting that the duration of the stressor is important for impacting the normal development of PVN neurons (Fujioka et al., 1999) and perhaps neurons controlling the HPA axis (Insel et al., 1988).
Other studies have investigated the time-dependent effects of fetal exposure to excess GC. In rodents, in utero exposure to elevated GCs during late gestation has been shown to cause hyperactivity of the HPA axis in adulthood with elevated levels of basal GC and ACTH and decreased CRH expression (Kapoor et al., 2006), perhaps suggesting reduced negative feedback. Increased peaks and prolonged secretion of these hormones in response to stressors have also been observed (Muneoka et al., 1997; Shoener et al., 2006). In contrast, Kamphuis et al. (2002) demonstrated that postnatal exposure to a synthetic GC reduced stress-induced HPA activity in adulthood, further suggesting that the timing of GC elevation plays a crucial role in the developing stress response. Importantly, studies have also shown that treatment with allopregnanolone and 3β-diol can reverse the hyperactive HPA response to immune challenges in adult rats that were prenatally stressed (Brunton et al., 2015). This raises the possibility that the HPA axis can retain sufficient plasticity postnatally to allow reversal of maternal HPA axis hyperactivity in the offspring.
Offspring of rodent dams prenatally treated with GC during late gestation typically exhibit reduced birth weights that were consistently reduced throughout life compared to control offspring (Carbone et al., 2012). Reductions in birth weight have been suggested to be a direct consequence of a lack of placental 11β-HSD2 activity and excessive GC exposure on the fetus (Holmes et al., 2006), rather than due to malnutrition or poor maternal care (Nyirenda et al., 2001). Carbone et al. (2012) further observed reduced long bone lengths through PND21 in female, but not male, offspring prenatally exposed to DEX. This was thought to be linked to a dysregulation in growth hormone signaling, thereby reducing transcription of the Igf-1 gene (Carbone et al., 2012). Similarly, Ghrh mRNA transcripts in the ARC of female, but not male, offspring were reduced (Carbone et al., 2012). Together, such data suggest a sex-specific mechanism in which prenatal GC exposure causes a reduction in Ghrh to decrease circulating GH, resulting in lower plasma IGF-1, and reduced birth weights. Evidence for molecular mechanisms underlying these events remains to be determined.
It is well-established that prenatal insults can impact the autonomic system in adult offspring exposed to fetal GC. Such long-term consequences include cardiovascular function (O’Regan et al., 2008). Angiotensinogen is an important component of the renin-angiotensin-system (RAS) that is upregulated by GCs (Tamura et al., 1995). Estrogens are found to have inhibitory influences over angiotensinogen gene expression (Dzau and Hermann, 1982), implying sex-dependent programming effects could be correlated with increased sensitivity to GC-mediated hypertension in females (O’Regan et al., 2004). Moreover, adult female offspring exposed to prenatal stress display a more prolonged increase in systolic blood pressure and a longer recovery period when subjected to restraint stress than males (Igosheva et al., 2007). Such data are consistent with recent observations of altered R-R interval variability (Heart rate variability; HRV) in adult offspring exposed to elevated fetal GCs. Adult female, but not male offspring of prenatal GC treated dams exhibit a reduction in high-frequency power when compared to control (Madhavpeddi et al., 2020). Because high-frequency power represents parasympathetic activity (Akselrod et al., 1981), these effects suggest that prenatal GC treatment programs the parasympathetic nervous system, which, in female offspring, is responsible for altered pressor and tachycardic responses. Whether this is the same as the responses of prenatal stress remains to be determined but implicate sex-specific programming effects of the ANS due to excess prenatal GC exposure.
In addition to alterations in ANS and neuroendocrine function in adult offspring exposed to elevated GC levels in development, the risk for adult metabolic dysfunction is also increased. Female rats prenatally exposed to high levels of GCs are hyperinsulinemic after oral glucose administration, with alterations in the expression of genes that mediate GC and lipid metabolism in subcutaneous fat (Brunton et al., 2013). By contrast, prenatal GC exposure was found to cause hyperglycemia following oral glucose in male offspring (Nyirenda et al., 1998), with alterations in the expression of genes that mediate GC and lipid metabolism in skeletal muscle and liver. These observations suggest sex-specific mechanisms where fetal exposure to GCs programs the stress response, leading to a dysregulation of glucose-insulin homeostasis (Brunton et al., 2013) and increased risk for diabetes mellitus type 2 in adulthood (Nyirenda et al., 1998). Moreover, prenatal exposure to GC alters hepatic gene expression, with decreased mRNA transcripts encoding Pparα and Pgc1α, key regulators of lipid and energy metabolism, and an increase of plasma triglyceride concentrations in offspring (Drake et al., 2010; Brunton et al., 2013). These observations suggest a mechanism in which fetal GCs affect important genes in fatty acid metabolism and increase the risk for hepatic steatosis in adulthood (Carbone et al., 2012).
Future Studies on DOHaD
The developmental origins of the disease model posit that events during fetal and early-life correlate with long-term consequences that encompass the development of neuroendocrine signaling and ultimately susceptibility to neuropsychological and neuropathological diseases in adulthood. Improper development of the HPA axis is commonly suggested as the primary neuroendocrine system affected by alterations in the prenatal environment.
A growing body of evidence further suggests the placenta’s important role in mediating maternal-fetal interactions during the prenatal period. Placental hormones and cytokines are thought to regulate the effects of maternal stress on the fetal HPA axis, but it is important to consider the nature and timing of prenatal insults, as evidence suggests exposure to prenatal stress during various gestational periods and varying lengths of time exerts different effects on HPA axis activity (Sandman et al., 2015). However, precise mechanisms in which prenatal stressors influence neuroendocrine signaling between the maternal-placental-fetal interface are still unclear. In humans, a potential prospect is placental CRH, which is upregulated by maternal and fetal cortisol. Studies implicate placental CRH to directly mediate fetal pituitary-adrenal growth and steroid production, factors that are known to significantly affect HPA axis function during development and in adulthood (Green et al., 2016). Notably, more recent evidence examines the effects of fetal sex in placental crosstalk. In rodents, placentas of female fetuses show greater expression of genes associated with immune and endocrine function, while placentas of male fetuses tend to express more genes involved in inflammation (Cvitic et al., 2013). Moreover, the inherent sex differences in fetal metabolic needs and timing of GC administration related to maturation and decline of placental function towards the end of gestation could help us understand mechanisms by which fetal sex influences the maternal-placental-fetal interface, a primary regulator of the development of the HPA axis (Lassance et al., 2015; Sun et al., 2020).
Although there is a long history of research behind the HPA axis and development, much remains to be revealed. It is well known the HPA axis holds a fundamental role in maintaining proper neuroendocrine function and a large body of research strongly correlates the dysregulation of the HPA axis to neuropsychological and physiological disease risk. Therefore, it is reasonable to suggest a strong association between the development of the HPA axis to such diseases, emphasizing the importance of future developmental studies to address this large gap in our current knowledge.
Author Contributions
RH and TH conceived of topic. RH wrote original outline. JS, NB, and MR wrote original manuscript. SM conceived of and constructed figures. AB proofread the manuscript and updated references. RH and TH edited and performed final proofreading. All authors contributed to the article and approved the submitted version.
Funding
This work was supported by National Institute of Diabetes and Digestive and Kidney Diseases (NIDDK) R01 DK105826 (RH) and National Institute of Mental Health (NIMH) U54 MH118919 (TH and RH).
Conflict of Interest
The authors declare that the research was conducted in the absence of any commercial or financial relationships that could be construed as a potential conflict of interest.
Abbreviations
11β-HSD2, 11-β hydroxysteroid dehydrogenase type 2; 5-HT, Serotonin; ACTH, Adrenocorticotropic hormone; AMY, Amygdala; ANS, Autonomic nervous system; AR, Androgen receptor; ARC, Arcuate n.; ARNT2, Aryl hydrocarbon receptor nuclear translocator 2; AVP, Arginine Vasopressin; BNST, Bed n. of the stria terminalis; CAH, Congenital Adrenal Hyperplasia; cAMP, cyclic adenosine monophosphate; CBG, Corticosteroid-binding globulin; CeA, Central n. of the amygdala; CNS, Central nervous system; CRH, Corticotropin releasing hormone; CRH-BP, Corticotropin releasing hormone binding protein; DEX, Dexamethasone; DHEA, Dehydroepiandrosterone; DOHaD, Developmental Origins of Health and Disease; ER, Estrogen Receptor; ERE, Estrogen response element; ERα, Estrogen receptor alpha; ERβ, Estrogen receptor beta; FSH, Follicle stimulating hormone; GC, Glucocorticoid; GH, Growth hormone; GR, Glucocorticoid receptor; GnRH, Gonadotropin-Releasing Hormone; HC, Hippocampus; HPA, Hypothalamic-pituitary-adrenal; HPG, Hypothalamic-pituitary-gonadal; LH, Luteinizing hormone; MeA, Medial Amygdala; MPOA, Medial Preoptic Area; MR, Mineralocorticoid receptor; MSH, Melanocyte stimulating hormone; NGFI-A, Nerve growth factor-inducible protein A; NTS, Nucleus of Solitary Tract; OT, Oxytocin; PFC, Prefrontal Cortex; PND, Postnatal day; POMC, Proopiomelanocortin; PVN, Paraventricular nucleus; SCN, Suprachiasmatic nucleus; SHRP, Stress hypo-responsive period; SON, Supraoptic nucleus; TRH, Thyrotropin releasing hormone; TSH, Thyroid stimulating hormone; VMN, Ventromedial n.
References
Abe, K., and Critchlow, V. (1980). Delayed feedback inhibition of stress-induced activation of pituitary-adrenal function: effects of varying dose, rate and duration of corticosterone administration and of telencephalon removal. Neuroendocrinology 31, 349–354. doi: 10.1159/000123100
Ahima, R. S., and Harlan, R. E. (1990). Charting of type II glucocorticoid receptor-like immunoreactivity in the rat central nervous system. Neuroscience 39, 579–604. doi: 10.1016/0306-4522(90)90244-x
Akana, S. F., Cassio, C. S., Du, J. Z., Levin, N., and Dallman, M. F. (1986). Reset of feedback in the adrenocortical system: an apparent shift in sensitivity of adrenocorticotropin to inhibition by corticosterone between morning and evening. Endocrinology 119, 2325–2332. doi: 10.1210/endo-119-5-2325
Akselrod, S., Gordon, D., Ubel, F. A., Shannon, D. C., Barger, A. C., and Cohen, R. J. (1981). Power spectrum analysis of heart rate fluctuations: a quantitative probe of beat-to-beat cardiovascular control. Science 213, 220–222. doi: 10.1126/science.6166045
Almazan, G., Lefebvre, D. L., and Zingg, H. H. (1989). Ontogeny of hypothalamic vasopressin, oxytocin and somatostatin gene expression. Dev. Brain Res. 45, 69–75. doi: 10.1016/0165-3806(89)90008-4
Amar, A., and Weiss, M. (2003). Pituitary anatomy and physiology. Neurosurg. Clin. N. Am. 14, 11–23. doi: 10.1016/s1042-3680(02)00017-7
Arborelius, L., and Eklund, M. B. (2007). Both long and brief maternal separation produces persistent changes in tissue levels of brain monoamines in middle-aged female rats. Neuroscience 145, 738–750. doi: 10.1016/j.neuroscience.2006.12.007
Bales, K. L., and Perkeybile, A. M. (2012). Developmental experiences and the oxytocin receptor system. Horm. Behav. 61, 313–319. doi: 10.1016/j.yhbeh.2011.12.013
Baquedano, E., García-Cáceres, C., Diz-Chaves, Y., Lagunas, N., Calmarza-Font, I., Azcoitia, I., et al. (2011). Prenatal stress induces long-term effects in cell turnover in the hippocampus-hypothalamus-pituitary axis in adult male rats. PLoS One 6, 1–9. doi: 10.1371/journal.pone.0027549
Barbazanges, A., Piazza, P. V., Moal, M. L., and Maccari, S. (1996). Maternal glucocorticoid secretion mediates long-term effects of prenatal stress. J. Neurosci. 16, 3943–3949. doi: 10.1523/JNEUROSCI.16-12-03943.1996
Benediktsson, R., Calder, A. A., Edwards, C. R., and Seckl, J. R. (1997). Placental 11 beta-hydroxysteroid dehydrogenase: a key regulator of fetal glucocorticoid exposure. Clin. Endocrinol. 46, 161–166. doi: 10.1046/j.1365-2265.1997.1230939.x
Biag, J., Huang, Y., Gou, L., Hintiryan, H., Askarinam, A., Hahn, J. D., et al. (2012). Cyto- and chemoarchitecture of the hypothalamic paraventricular nucleus in the C57BL/6J male mouse: a study of immunostaining and multiple fluorescent tract tracing. J. Comp. Neurol. 520, 6–33. doi: 10.1002/cne.22698
Bingaman, E. W., Magnuson, D. J., Gray, T. S., and Handa, R. J. (1994). Androgen inhibits the increases in hypothalamic corticotropin-releasing hormone (CRH) and CRH-immunoreactivity following gonadectomy. Neuroendocrinology 59, 228–234. doi: 10.1159/000126663
Boccia, M. L., and Pederson, C. A. (2001). Brief vs. long maternal separations in infancy: contrasting relationships with adult maternal behavior and lactation levels of aggression and anxiety. Psychoneuroendocrinology 26, 657–672. doi: 10.1016/s0306-4530(01)00019-1
Bondy, C. A., Whitnall, M. H., Brady, L. S., and Gainer, H. (1989). Coexisting peptides in hypothalamic neuroendocrine systems: some functional implications. Cell. Mol. Neurobiol. 9, 427–446. doi: 10.1007/BF00712791
Bornstein, S. R., Ehrhart-Bornstein, M., Usadel, H., Böckmann, M., and Scherbaum, W. A. (1991). Morphological evidence for a close interaction of chromaffin cells with cortical cells within the adrenal gland. Cell Tissue Res. 265, 1–9. doi: 10.1007/BF00318133
Borrow, A. P., Stover, S. A., Bales, N. J., and Handa, R. J. (2019). “Posterior pituitary hormones,” in Hormonal Signaling in Biology and Medicine: Comprehensive Modern Endocrinology, ed. G. Litwack (Cambridge: Academic Press), 203–226.
Bradbury, M. J., Akana, S. F., and Dallman, M. F. (1994). Roles of type I and II corticosteroid receptors in regulation of basal activity in the hypothalamo-pituitary-adrenal axis during the diurnal trough and the peak: evidence for a non-additive effect of combined receptor occupation. Endocrinology 134, 1286–1296. doi: 10.1210/endo.134.3.8119168
Brown, R. W., Chapman, K. E., Edwards, C. R., and Seckl, J. R. (1993). Human placental 11 beta-hydroxysteroid dehydrogenase: evidence for and partial purification of a distinct NAD-dependent isoform. Endocrinology 132, 2614–2621. doi: 10.1210/endo.132.6.8504762
Brunton, P. J., Donadio, M. V., Yao, S. T., Greenwood, M., Seckl, J. R., Murphy, D., et al. (2015). 5A-reduced neurosteroids sex-dependently reverse central prenatal programming of neuroendocrine stress responses in rats. J. Neurosci. 35, 666–677. doi: 10.1523/JNEUROSCI.5104-13.2015
Brunton, P. J., Sullivan, K. M., Kerrigan, D., Russell, J. A., Seckl, J. R., and Drake, A. J. (2013). Sex-specific effects of prenatal stress on glucose homoeostasis and peripheral metabolism in rats. J. Endocrinol. 217, 161–173. doi: 10.1530/JOE-12-0540
Bucheler, M. M., Hadamek, K., and Hein, L. (2002). Two α2 adrenergic receptor subtypes, α2A and α2C, inhibit transmitter release in the brain of gene-targeted mice. Neuroscience 109, 819–826. doi: 10.1016/s0306-4522(01)00531-0
Bugnon, D., Fellmann, A., Gouget, A., and Cardot, J. (1982). Corticoliberin in rat brain: immunocytochemical identification and localization of a novel neuroglandular system. Neurosci. Lett. 30, 25–30. doi: 10.1016/0304-3940(82)90006-4
Buschdorf, J. P., and Meaney, M. J. (2016). Epigenetics/programming in the HPA axis. Compr. Physiol. 6, 87–110. doi: 10.1002/cphy.c140027
Caldji, C., Tannenbaum, B., Sharma, S., Francis, D., Plotsky, P. M., and Meaney, M. J. (1998). Maternal care during infancy regulates the development of neural systems mediating the expression of fearfulness in the rat. Proc. Natl. Acad. Sci. U S A 95, 5335–5340. doi: 10.1073/pnas.95.9.5335
Carbone, D. L., Zuloaga, D. G., Hirori, R., Foradori, C. D., Legare, M. E., and Handa, R. J. (2012). Prenatal dexamethasone exposure potentiates diet-induced hepatosteatosis and decreases plasma IGF-I in a sex-specific fashion. Endocrinology 153, 295–306. doi: 10.1210/en.2011-1601
Chalmers, D. T., Lovenberg, T. W., and De Souza, E. B. (1995). Localization of novel corticotropin-releasing factor receptor (CRF2) MRNA expression to specific subcortical nuclei in rat brain: comparison with CRF1 receptor MRNA expression. J. Neurosci. 15, 6340–6350. doi: 10.1523/JNEUROSCI.15-10-06340.1995
Chen, R., Lewis, K. A., Perrin, M. H., and Vale, W. W. (1993). Expression cloning of a human corticotropin-releasing-factor receptor. Proc. Natl. Acad. Sci. U S A 90, 8967–8971. doi: 10.1073/pnas.90.19.8967
Cole, R. L., and Sawchenko, P. E. (2002). Neurotransmitter regulation of cellular activation and neuropeptide gene expression in the paraventricular nucleus of the hypothalamus. J. Neurosci. 22, 959–969. doi: 10.1523/JNEUROSCI.22-03-00959.2002
Cottrell, E. C., and Seckl, J. R. (2009). Prenatal stress, glucocorticoids and the programming of adult disease. Front. Behav. Neurosci. 3:19. doi: 10.3389/neuro.08.019.2009
Coulter, C. L. (2004). Functional biology of the primate fetal adrenal gland: advances in technology provide new insight. Clin. Exp. Pharmacol. Physiol. 31, 475–484. doi: 10.1111/j.1440-1681.2004.04031.x
Coyne, M. D., and Kitay, J. I. (1969). Effect of ovariectomy on pituitary secretion of ACTH. Endocrinology 85, 1097–1102. doi: 10.1210/endo-85-6-1097
Crowther, C. A., Middleton, P. F., Voysey, M., Askie, L., Zhang, S., Martlow, T. K., et al. (2019). Effects of repeat prenatal corticosteroids given to women at risk of preterm birth: an individual participant data meta-analysis. PLoS Med. 16, 1–25. doi: 10.1371/journal.pmed.1002771
Cullinan, W. E., Herman, J. P., and Watson, S. J. (1993). Ventral subicular interaction with the hypothalamic paraventricular nucleus: evidence for a relay in the bed nucleus of the stria terminalis. J. Comp. Neurol. 332, 1–20. doi: 10.1002/cne.903320102
Cummings, S., and Seybold, V. (1988). Relationship of alpha-1- and alpha-2-adrenergic-binding sites to regions of the paraventricular nucleus of the hypothalamus containing corticotropin-releasing factor and vasopressin neurons. Neuroendocrinology 47, 523–532. doi: 10.1159/000124965
Cunningham, E. T., Bohn, M. C., and Sawchenko, P. E. (1990). Organization of adrenergic inputs to the paraventricular and supraoptic nuclei of the hypothalamus in the rat. J. Comp. Neurol. 292, 651–667. doi: 10.1002/cne.902920413
Curley, J. P., Jensen, C. L., Mashoodh, R., and Champagne, F. A. (2011). Social influences on neurobiology and behavior: epigenetic effects during development. Psychoneuroendocrinology 36, 352–371. doi: 10.1016/j.psyneuen.2010.06.005
Cvitic, S., Longtine, M. S., Hackl, H., Wagner, K., Nelson, M. D., Desoye, G., et al. (2013). The human placental sexome differs between trophoblast and epithelium and villous vessel endothelium. PLoS One 8:e79233. doi: 10.1371/journal.pone.0079233
Dabrowska, J., Hazra, R., Ahern, T. H., Guo, J., McDonald, A. J., Mascagni, F., et al. (2011). Neuroanatomical evidence for reciprocal regulation of the corticotrophin-releasing factor and oxytocin systems in the hypothalamus and the bed nucleus of the stria terminalis of the rat: implications for balancing stress and affect. Psychoneuroendocrinology 36, 1312–1326. doi: 10.1016/j.psyneuen.2011.03.003
Dallman, M. F., Akana, S. F., Jacobson, L., Levin, N., Cascio, C. S., and Shinsako, J. (1987). Characterization of corticosterone feedback regulation of ACTH secretion. Ann. N. Y. Acad. Sci. 512, 402–414. doi: 10.1111/j.1749-6632.1987.tb24976.x
Dallman, M. F., Makara, G. B., Roberts, J. L., Levin, N., and Blum, M. (1985). Corticotrope response to removal of releasing factors and corticosteroids in vivo. Endocrinology 117, 2190–2197. doi: 10.1210/endo-117-5-2190
Day, H. E. W., Campeau, S., Watson, S. J., and Akil, H. (1997). Distribution of α1a-, α1b- and α1d-adrenergic receptor MRNA in the rat brain and spinal cord. J. Chem. Neuroanat. 13, 115–139. doi: 10.1016/s0891-0618(97)00042-2
Day, H. E. W., Campeau, S., Watson, S. J., and Akil, H. (1999). Expression of α1b adrenoceptor MRNA in corticotropin-releasing hormone-containing cells of the rat hypothalamus and its regulation by corticosterone. J. Neurosci. 19,10098–10106. doi: 10.1523/JNEUROSCI.19-22-10098.1999
de Kloet, E. R., Joëls, M., and Holsboer, F. (2005). Stress and the brain: from adaptation to disease. Nat. Rev. Neurosci. 6, 463–475. doi: 10.1038/nrn1683
de Kloet, E. R., Vreugdenhil, E., Oitzl, M. S., and Joels, M. (1998). Brain corticosteroid receptor balance in health and disease. Endocr. Soc. 19, 269–301. doi: 10.1210/edrv.19.3.0331
de Vries, G. J., and Al-Shamma, H. A. (1990). Sex differences in hormonal responses of vasopressin pathways in the rat brain. J. Neurobiol. 21, 686–693. doi: 10.1002/neu.480210503
Denef, C. (2008). Paracrinicty: the story of 30 years of cellular pituitary crosstalk. J. Neuroendocrinol. 20, 1–70. doi: 10.1111/j.1365-2826.2007.01616.x
Deng, Q., Riquelme, D., Trinh, L., Low, M. J., Tomić, M., Stojilkovic, S., et al. (2015). Rapid glucocorticoid feedback inhibition of ACTH secretion involves ligand-dependent membrane association of glucocorticoid receptors. Endocrinology 156, 3215–3227. doi: 10.1210/EN.2015-1265
Deng, Q., Zhang, Z., and Wu, Y. (2017). The pulsatility of ACTH secretion in the rat anterior pituitary cell perifusion system. Cell. Physiol. Biochem. 41, 154–162. doi: 10.1159/000455984
di Scala-Guenot, D., Strosser, M. T., Sarlieve, L. L., Legros, J. J., and Richard, P. (1990). Development of neurophysin-containing neurons in primary cultures of rat hypothalami is related to the age of the embryo: morphological study and comparison of in vivo and in vitro neurophysins, oxytocin and vasopressin content. J. Neurosci. Res. 25, 94–102. doi: 10.1002/jnr.490250112
Diorio, D., Viau, V., and Meaney, M. J. (1993). The role of the medial prefrontal cortex (cingulate gyrus) in the regulation of hypothalamic-pituitary-adrenal responses to stress. J. Neurosci. 13, 3839–3847. doi: 10.1523/JNEUROSCI.13-09-03839.1993
Dong, H. W., Petrovich, G. D., and Swanson, L. W. (2001). Basic organization of projections from the oval and fusiform nuclei of the bed nuclei of the stria terminalis in adult rat brain. J. Comp. Neurol. 436, 430–455. doi: 10.1002/cne.1079
Dong, H. W., and Swanson, L. W. (2006). Projections from bed nuclei of the stria terminalis, anteromedial area: cerebral hemisphere integration of neuroendocrine, autonomic and behavioral aspects of energy balance. J. Comp. Neurol. 494, 142–178. doi: 10.1002/cne.20788
Dorton, A. M. (2000). The pituitary gland: embryology, physiology and pathophysiology. Neonatal Netw. 19, 9–17. doi: 10.1891/0730-0832.19.2.9
Drake, A. J., Raubenheimer, P. J., Kerrigan, D., McInnes, K. J., Seckl, J. R., and Walker, B. R. (2010). Prenatal dexamethasone programs expression of genes in liver and adipose tissue and increased hepatic lipid accumulation but not obesity on a high-fat diet. Endocrinology 151, 1581–1587. doi: 10.1210/en.2009-1088
Dumontet, T., Sahut-Barnola, I., Septier, A., Montanier, N., Plotton, I., Roucher-Boulez, F., et al. (2019). PKA signaling drives reticularis differentiation and sexually dimorphic adrenal cortex renewal. JCI Insight 3:398394. doi: 10.1172/jci.insight.98394
Dunn, J. D. (1987). Plasma corticosterone responses to electrical stimulation of the bed nucleus of the stria terminalis. Brain Res. 407, 327–331. doi: 10.1016/0006-8993(87)91111-5
Dzau, V. J., and Hermann, H. C. (1982). Hormonal control of angiotensinogen production. Life Sci. 30, 577–584. doi: 10.1016/0024-3205(82)90272-7
Edwards, C. R., Benediktsson, R., Lindsay, R. S., and Seckl, J. R. (1993). Dysfunction of placental glucocorticoid barrier: link between fetal environment and adult hypertension? Lancet 341, 355–357. doi: 10.1016/0140-6736(93)90148-a
Ehrhart-Bornstein, M., Hinson, J. P., Bornstein, S. R., Scherbaum, W. A., and Vinson, G. P. (1998). Intraadrenal interactions in the regulation of adrenocortical steroidogenesis. Endocr. Rev. 19, 101–143. doi: 10.1210/edrv.19.2.0326
Ericson, J., Norlin, S., Jessell, T. M., and Edlund, T. (1998). Integrated FGF and BMP signaling controls the progression of progenitor cell differentiation and the emergence of pattern in the embryonic anterior pituitary. Development 125, 1005–1015.
Evans, A. N., Liu, Y., Macgregor, R., Huang, V., and Aguilera, G. (2013). Regulation of hypothalamic corticotropin-releasing hormone transcription by elevated glucocorticoids. Mol. Endocrinol. 27, 1796–1807. doi: 10.1210/me.2013-1095
Evuarherhe, O., Leggett, J., Waite, E., Kershaw, Y., and Lightman, S. (2009). Reversal of the hypothalamic-pituitary-adrenal response to oestrogens around puberty. J. Endocrinol. 202, 279–285. doi: 10.1677/JOE-09-0175
Figueiredo, H. F., Bruestle, A., Bodie, B., Dolgas, C. M., and Herman, J. P. (2003). The medial prefrontal cortex differentially regulates stress-induced c-fos expression in the forebrain depending on type of stressor. Eur. J. Neurosci. 18, 2357–2364. doi: 10.1046/j.1460-9568.2003.02932.x
Figueiredo, H. F., Ulrich-Lai, Y. M., Choi, D. C., and Herman, J. P. (2007). Estrogen potentiates adrenocortical responses to stress in female rats. AJP Endocrinol. Metab. 292, E1173–E1182. doi: 10.1152/ajpendo.00102.2006
Foradori, C. D., Werner, S. B., Sandau, U. S., Clapp, T. R., and Handa, R. J. (2007). Activation of the androgen receptor alters the intracellular calcium response to glutamate in primary hippocampal neurons and modulates sarco/endoplasmic reticulum calcium ATPase 2 transcription. Neuroscience 149, 155–164. doi: 10.1016/j.neuroscience.2007.06.054
Frazier, C. R., Trainor, B. C., Cravens, C. J., Whitney, T. K., and Marler, C. A. (2006). Paternal behavior influences development of aggression and vasopressin expression in male california mouse offspring. Horm. Behav. 50, 699–707. doi: 10.1016/j.yhbeh.2006.06.035
Fujioka, T., Sakata, Y., Yamaguchi, K., Shibasaki, T., Kato, H., and Nakamura, S. (1999). The effects of prenatal stress on the development of hypothalamic paraventricular neurons in fetal rats. Neuroscience 92, 1079–1088. doi: 10.1016/s0306-4522(99)00073-1
Gallo-Payet, N., Martinez, A., and Lacroix, A. (2017). Editorial: ACTH action in the adrenal cortex: from molecular biology to pathophysiology. Front. Endocrinol. 8:101. doi: 10.3389/fendo.2017.00101
García-Iglesias, B. B., Mendoza-Garrido, M. E., Gutiérrez-Ospina, G., Rangel-Barajas, C., Noyola-Díaz, M., and Terrón, J. A. (2013). Sensitization of restraint-induced corticosterone secretion after chronic restraint in rats: involvement of 5-HT7 receptors. Neuropharmacology 71, 216–227. doi: 10.1016/j.neuropharm.2013.03.013
Gillies, G. E., Linton, E. A., and Lowry, P. J. (1982). Corticotropin releasing activity of the new CRF is potentiated several times by vasopressin. Nature 299, 355–357. doi: 10.1038/299355a0
Gjerstad, J. K., Lightman, S. L., and Spiga, F. (2018). Role of glucocorticoid negative feedback in the regulation of HPA axis pulsatility. Stress 21, 403–416. doi: 10.1080/10253890.2018.1470238
Goel, N., Workman, J. L., Lee, T. T., Innala, L., and Viau, V. (2014). Sex differences in the HPA axis. Comp. Physiol. 4, 1121–1155. doi: 10.1002/cphy.c130054
Goldman, J., Winget, C., Hollingshead, G. W., and Levine, S. (1973). Postweaning development of negative feedback in the pituitary-adrenal system of the rat. Neuroendocrinology 12, 199–211. doi: 10.1159/000122169
Gray, T. S., Piechowski, R. A., Yracheta, J. M., Rittenhouse, P. A., Bethea, C. L., and Van de Kar, L. D. (1993). Ibotenic acid lesions in the bed nucleus of the stria terminalis attenuate conditioned stress-induced increases in prolactin, ACTH and corticosterone. Neuroendocrinology 57, 517–524. doi: 10.1159/000126400
Green, M., and McCormick, C. (2016). Sex and stress steroids in adolescence: gonadal regulation of hypothalamic-pituitary-adrenal axis in the rat. Gen. Comp. Endocrinol. 234, 110–116. doi: 10.1016/j.ygcen.2016.02.004
Green, M. R., Nottrodt, R., Simone, J. J., and McCormick, C. M. (2016). Glucocorticoid receptor translocation and expression of relevant genes in hippocampus in adolescent and adult male rats. Psychoneuroendocrinology 73, 32–41. doi: 10.1016/j.psyneuen.2016.07.210
Grino, M., Young, W. S., and Burgunder, J. (1989). Ontogeny of expression of the corticotropin-releasing factor gene in the hypothalamic paraventricular nucleus and of the proopiomelanocortin gene in rat pituitary. Endocrinology 124, 60–68. doi: 10.1210/endo-124-1-60
Gunn, B. G., Cunningham, L., Cooper, M. A., Corteen, N. L., Seifi, M., Swinny, J. D., et al. (2013). Dysfunctional astrocytic and synaptic regulation of hypothalamic glutamatergic transmission in a mouse model of early-life adversity: relevance to neurosteroids and programming of the stress response. J. Neurosci. 33, 19534–19554. doi: 10.1523/JNEUROSCI.1337-13.2013
Hammer, G. D., Parker, K. L., and Schimmer, B. P. (2005). Minireview: transcriptional regulation of adrenocortical development. Endocrinology 146, 1018–1024. doi: 10.1210/en.2004-1385
Handa, R. J., Kudwa, A. E., Donner, N. C., McGivern, R. F., and Brown, R. (2013). Central 5-alpha reduction of testosterone is required for testosterone’s inhibition of the hypothalamo-pituitary-adrenal axis response to restraint stress in adult male rats. Brain Res. 1529, 74–82. doi: 10.1016/j.brainres.2013.07.021
Handa, R. J., Nunley, K. M., Lorens, S. A., Louie, J. P., McGivern, R. F., and Bollnow, M. R. (1994). Androgen regulation of adrenocorticotropin and corticosterone secretion in the male rat following novelty and foot shock stressors. Physiol. Behav. 55, 117–124. doi: 10.1016/0031-9384(94)90018-3
Handa, R. J., and Weiser, M. J. (2014). Gonadal steroid hormones and the hypothalamo-pituitary-adrenal axis. Front. Neuroendocrinol. 35, 197–220. doi: 10.1016/j.yfrne.2013.11.001
Handa, R. J., Weiser, M. J., and Zuloaga, D. G. (2009). A role for the androgen metabolite, 5α-androstane-3β, 17β-diol, in modulating oestrogen receptor β-mediated regulation of hormonal stress reactivity. J. Neuroendocrinol. 21, 351–358. doi: 10.1111/j.1365-2826.2009.01840.x
Harno, E., Ramamoorthy, T. G., Coll, A. P., and White, A. (2018). POMC: the physiological power of hormone processing. Physiol. Rev. 98, 2381–2430. doi: 10.1152/physrev.00024.2017
Harris, A., and Seckl, J. (2011). Glucocorticoids, prenatal stress and the programming of disease. Horm. Behav. 59, 278–289. doi: 10.1016/j.yhbeh.2010.06.007
He, X., Traecy, M. N., Simmons, D. M., Ingraham, H. A., Swanson, L. S., and Rosenfeld, M. G. (1989). Expression of a large family of POU-domain regulatory genes in mammalian brain development. Nature 340, 35–41. doi: 10.1038/340035a0
Heck, A. L., and Handa, R. J. (2019a). Androgens drive sex biases in hypothalamic corticotropin-releasing hormone gene expression after adrenalectomy of mice. Endocrinology 160, 1757–1770. doi: 10.1210/en.2019-00238
Heck, A. L., and Handa, R. J. (2019b). Sex differences in the hypothalamic-pituitary-adrenal axis’ response to stress: an important role for gonadal hormones. Neuropsychopharmacology 44, 45–58. doi: 10.1038/s41386-018-0167-9
Heck, A. L., Sheng, J. A., Miller, A. M., Stover, S. A., Bales, N. J., Tan, S. M. L., et al. (2020). Social isolation alters hypothalamic pituitary adrenal axis activity after chronic variable stress in male C57BL/6 mice. Stress 23, 457–465. doi: 10.1080/10253890.2020.1733962
Heisler, L. K., Pronchuk, N., Nonogaki, K., Zhou, L., Raber, J., Tung, L., et al. (2007). Serotonin activates the hypothalamic-pituitary-adrenal axis via serotonin 2C receptor stimulation. J. Neurosci. 27, 6956–6964. doi: 10.1523/JNEUROSCI.2584-06.2007
Herman, J. P., Cullinan, W. E., and Watson, S. J. (1994). Involvement of the bed nucleus of the stria terminalis in tonic regulation of paraventricular hypothalamic CRH and AVP MRNA expression. J. Neuroendocrinol. 6, 433–442. doi: 10.1111/j.1365-2826.1994.tb00604.x
Herman, J. P., Dolgas, C. M., and Carlson, S. L. (1998). Ventral subiculum regulates hypothalamo-pituitary-adrenocortical and behavioural responses to cognitive stressors. Neuroscience 86, 449–459. doi: 10.1016/s0306-4522(98)00055-4
Herman, J. P., Schafer, M. K., Thompson, R. C., and Watson, S. J. (1992). Rapid regulation of corticotropin-releasing hormone gene transcription in vivo. Mol. Endocrinol. 6, 1061–1069. doi: 10.1210/mend.6.7.1324419
Herman, J. P., and Tasker, J. G. (2016). Paraventricular hypothalamic mechanisms of chronic stress adaptation. Front. Endocrinol. 7:137. doi: 10.3389/fendo.2016.00137
Hernandez, V. S., Vazquez-Jaurez, E., Marquez, M. M., Jauregui-Huerta, F., Barrio, R. A., and Zhang, L. (2015). Extra-neurohypophyseal axonal projections from individual vasopressin-containing magnocellular neurons in rat hypothalamus. Front. Neuroanat. 9:130. doi: 10.3389/fnana.2015.00130
Hiroi, R., Lacagnina, A. F., Hinds, L. R., Carbone, D. G., Uht, R. M., and Handa, R. J. (2013). The androgen metabolite, 5α-androstane-3β,17β-diol (3β-diol), activates the oxytocin promoter through an estrogen receptor-β pathway. Endocrinology 154, 1802–1812. doi: 10.1210/en.2012-2253
Holmes, M. C., Abrahamsen, C. T., French, K. L., Paterson, J. M., Mullins, J. J., and Seckl, J. R. (2006). The mother or the fetus? 11β-hydroxysteroid dehydrogenase type 2 null mice provide evidence for direct fetal programming of behavior by endogenous glucocorticoids. J. Neurosci. 26, 3840–3844. doi: 10.1523/JNEUROSCI.4464-05.2006
Hornby, P. J., and Piekut, D. T. (1989). Opiocortin and catecholamine input to CRF-immunoreactive neurons in rat forebrain. Peptides 10, 1139–1146. doi: 10.1016/0196-9781(89)90005-3
Igosheva, N., Taylor, P. D., Poston, L., and Glover, V. (2007). Prenatal stress in the rat results in increased blood pressure responsiveness to stress and enhanced arterial reactivity to neuropeptide Y in adulthood. J. Physiol. 582, 665–674. doi: 10.1113/jphysiol.2007.130252
Ilahi, S., and Ilahi, T. B. (2020). Anatomy, Adenohypophysis (Pars Anterior, Anterior Pituitary). Treasure Island, FL: StatPearls Publishing.
Imaki, T., Nahan, J. L., Rivier, C., Sawchenko, P. E., and Vale, W. (1991). Differential regulation of corticotropin-releasing factor MRNA in rat brain regions by glucocorticoids and stress. J. Neurosci. 11, 585–599. doi: 10.1523/JNEUROSCI.11-03-00585.1991
Insel, T. R., Battaglia, G., Fairbanks, D. W., and De Souza, E. B. (1988). The ontogeny of brain receptors for corticotropin-releasing factor and the development of their functional association with adenylate cyclase. J. Neurosci. 8, 4151–4158. doi: 10.1523/JNEUROSCI.08-11-04151.1988
Ishimoto, H., and Jaffe, R. B. (2011). Development and function of the human fetal adrenal cortex: a key component in the feto-placental unit. Endocr. Rev. 32, 317–355. doi: 10.1210/er.2010-0001
Israel, J., Oliet, S. H., and Ciofi, P. (2016). Electrophysiology of hypothalamic magnocellular neurons in vitro: a rhythmic drive in organotypic cultures and acute slices. Front. Neurosci. 10:109. doi: 10.3389/fnins.2016.00109
Itoi, K., Helmreich, D. L., Lopez-Figueroa, M. O., and Watson, S. J. (1999). Differential regulation of corticotropin-releasing hormone and vasopressin gene transcription in the hypothalamus by norepinephrine. J. Neurosci. 19, 5464–5472. doi: 10.1523/JNEUROSCI.19-13-05464.1999
Jauregui-Huerta, F., Ruvalcaba-Delgadillo, Y., Gonzalez-Castañeda, R., Garcia-Estrada, J., Gonzalez-Perez, O., and Luquin, S. (2010). Responses of glial cells to stress and glucocorticoids. Curr. Immunol. Rev. 6, 195–204. doi: 10.2174/157339510791823790
John, C. D., Christian, H. C., Morris, J. F., Flower, R. J., Solito, E., and Buckingham, J. C. (2004). Annexin 1 and the regulation of endocrine function. Trends Endocrinol. Metab. 15, 103–109. doi: 10.1016/j.tem.2004.02.001
Kalin, N. H. (2018). Corticotropin-releasing hormone binding protein: stress, psychopathology and antidepressant treatment response. Am. J. Psychiatry 175, 204–206. doi: 10.1176/appi.ajp.2018.18010059
Kamphuis, P. J., Bakker, J. M., Broekhoven, M. H., Kunne, C., Croiset, G., Lentjes, E. G., et al. (2002). Enhanced glucocorticoid feedback inhibition of hypothalamo-pituitary-adrenal responses to stress in adult rats neonatally treated with dexamethasone. Neuroendocrinology 76, 159–169. doi: 10.1159/000064526
Kapoor, A., Dunn, E., Kostaki, A., Andrews, M. H., and Matthews, S. G. (2006). Fetal programming of hypothalamo-pituitary-adrenal function: prenatal stress and glucocorticoids. J. Physiol. 572, 31–44. doi: 10.1113/jphysiol.2006.105254
Kash, T. L., and Winder, D. G. (2006). Neuropeptide Y and corticotropin-releasing factor bi-directionally modulate inhibitory synaptic transmission in the bed nucleus of the stria terminalis. Neuropharmacology 51, 1013–1022. doi: 10.1016/j.neuropharm.2006.06.011
Khan, A. M., Ponzio, T. A., Sanchez-Watts, G., Stanley, B. G., Hatton, G. I., and Watts, A. G. (2007). Catecholaminergic control of mitogen-activated protein kinase signaling in paraventricular neuroendocrine neurons: a proposed role during glycemic challenges. J. Neurosci. 27:7344. doi: 10.1523/JNEUROSCI.0873-07.2007
Kiss, A., and Aguilera, G. (1992). Participation of A1-adrenergic receptors in the secretion of hypothalamic corticotropin-releasing hormone during stress. Neuroendocrinology 56, 153–160. doi: 10.1159/000126223
Kitay, J. I. (1965). Effects of oophorectomy and various doses of estradiol-17β on corticosterone production by rat adrenal slices. Proc. Soc. for Exp. Biol. Med. 120, 193–196. doi: 10.3181/00379727-120-30483
Kítazawa, S., Shioda, S., and Nakai, Y. (1987). Catecholaminergic innervation of neurons containing corticotropin-releasing factor in the paraventricular nucleus of the rat hypothalamus. Acta Anat. 129, 337–343.
Kolber, B. J., and Muglia, L. J. (2009). Defining brain region-specific glucocorticoid action during stress by conditional gene disruption in mice. Brain Res. 1293, 85–90. doi: 10.1016/j.brainres.2009.03.061
Kovács, K., Földes, A., and Sawchenko, P. E. (2000). Glucocorticoid negative feedback selectively targets vasopressin transcription in parvocellular neurosecretory neurons. J. Neurosci. 20, 3843–3852. doi: 10.1523/JNEUROSCI.20-10-03843.2000
Ladd, C. O., Thrivikraman, K. V., Huot, R. L., and Plotsky, P. M. (2005). Differential neuroendocrine responses to chronic variable stress in adult long evans rats exposed to handling-maternal separation as neonates. Psychoneuroendocrinology 30, 520–533. doi: 10.1016/j.psyneuen.2004.12.004
Landgraf, R., and Neumann, I. D. (2004). Vasopressin and oxytocin release within the brain: a dynamic concept of multiple and variable modes of neuropeptide communication. Front. Neuroendocrinol. 25, 150–176. doi: 10.1016/j.yfrne.2004.05.001
Lassance, L., Haghiac, M., Leahy, P., Basu, S., Minium, J., Zhou, J., et al. (2015). Identification of early transcriptome signatures in placenta exposed to insulin and obesity. Am. J. Obstet. Gynecol. 212, 647.e1–647.e11. doi: 10.1016/j.ajog.2015.02.026
Laurent, F. M., Hindelang, C., Klein, M. J., Stoeckel, M. E., and Felix, J. M. (1989). Expression of the oxytocin and vasopressin genes in the rat hypothalamus during development: an in situ hybridization study. Dev. Brain Res. 46, 145–154. doi: 10.1016/0165-3806(89)90152-1
Lee, K. S., Han, T. H., Jo, J. Y., Kang, G., Lee, S. Y., Ryu, P. D., et al. (2008). Serotonin inhibits GABA synaptic transmission in presympathetic paraventricular nucleus neurons. Neurosci. Lett. 439, 138–142. doi: 10.1016/j.neulet.2008.05.012
Levine, S. (1994). The ontogeny of the hypothalmo-pituitary-adrenal axis: the influence of maternal factors. Ann. N. Y. Acad. Sci. 746, 275–288. doi: 10.1111/j.1749-6632.1994.tb39245.x
Liggins, G. C., and Howie, R. N. (1972). A controlled trial of antepartum glucocorticoid treatment prevention of the respiratory distress syndrome in premature infants. Pediatrics 50, 515–525.
Lightman, S. L., and Conway-Campbell, B. L. (2010). The crucial role of pulsatile activity of the HPA axis for continuous dynamic equilibration. Nat. Rev. Neurosci. 11, 710–718. doi: 10.1038/nrn2914
Lindsay, R. S., Lindsay, R. M., Waddel, B. J., and Seckl, J. R. (1996). Prenatal glucocorticoid exposure leads to offspring hyperglycaemia in the rat: studies with the 11 beta-hydroxysteroid dehydrogenase inhibitor carbenoxolone. Diabetologia 39, 1299–1305. doi: 10.1007/s001250050573
Lipari, E. F., Lipari, D., Gerbino, A., Di Liberto, D., Bellafiore, M., Catalono, M., et al. (2001). The Hypothalamic magnocellular neurosecretory system in developing rats. Eur. J. Histochem. 45, 163–168. doi: 10.4081/1626
Liu, D., Diorio, J., Tannenbaum, B., Caldji, C., Francis, D., Freedman, A., et al. (1997). Maternal care, hippocampal glucocorticoid receptors and hypothalamic-pituitary-adrenal response to stress. Science 277, 1659–1662. doi: 10.1126/science.277.5332.1659
Longcope, C. (1986). Adrenal and gonadal androgen secretion in normal females. Clin. Endocrinol. Metab. 15, 213–228. doi: 10.1016/s0300-595x(86)80021-4
Low, S. C., Moisan, M. P., Noble, J. M., Edwards, C. R., and Seckl, J. R. (1994). Glucocorticoids regulate hippocampal 11 beta-hydroxysteroid dehydrogenase activity and gene expression in vivo in the rat. J. Neuroendocrinol. 6, 285–290. doi: 10.1111/j.1365-2826.1994.tb00584.x
Lucion, A. B., Charchat, H., Pereira, G. A. M., and Rasia-Filho, A. A. (1996). Influence of early postnatal gonadal hormones on anxiety in adult male rats. Physiol. Behav. 60, 1419–1423. doi: 10.1016/s0031-9384(96)00246-6
Lund, T. D., Hinds, L. R., and Handa, R. J. (2006). The Androgen 5α-dihydrotestosterone and its metabolite 5α-androstan-3β,17β-diol inhibit the hypothalamo-pituitary- adrenal response to stress by acting through estrogen receptor beta-expressing neurons in the hypothalamus. J. Neurosci. 26, 1448–1456. doi: 10.1523/JNEUROSCI.3777-05.2006
Lund, T. D., Rovis, T., Chung, W. C. J., and Handa, R. J. (2005). Novel actions of estrogen receptor-beta on anxiety-related behaviors. Endocrinology 146, 797–807. doi: 10.1210/en.2004-1158
Madhavpeddi, L., Hammond, B., Carbone, D., Handa, R. J., and Hale, T. M. (2020). Impact of prenatal dexamethasone on adult cardiovascular autonomic regulation. FASEB J. 34:1. doi: 10.1096/fasebj.2020.34.s1.05817
Mahfouz, A., Lelieveldt, B. P. F., Grefhorst, A., van Weert, L. T. C. M., Mol, I. M., Sips, H. C. M., et al. (2016). Genome-wide coexpression of steroid receptors in the mouse brain: identifying signaling pathways and functionally coordinated regions. Proc. Natl. Acad. Sci. U S A 113, 2738–2743. doi: 10.1073/pnas.1520376113
Makino, S., Schulkin, J., Smith, M. A., Pacak, K., Palkovits, M., and Gold, P. W. (1995). Regulation of corticotropin-releasing hormone receptor messenger ribonucleic acid in the rat brain and pituitary by glucocorticoids and stress. Endocrinology 136, 4517–4525. doi: 10.1210/endo.136.10.7664672
McCormick, C. M., Linkroum, W., Sallinen, B. J., and Miller, N. W. (2002). Peripheral and central sex steroids have differential effects on the HPA axis of male and female rats. Stress 5, 135–247. doi: 10.1080/1025389021000061165
McCorry, L. K. (2007). Physiology of the autonomic nervous system. Am. J. Pharm. Educ. 71:78. doi: 10.5688/aj710478
McEwen, B. S. (1998). “Stress, adaptation and disease: allostasis and allostatic load,” in Molecular Aspects, Integrative Systems and Clinical Advances, eds S. M. McCann, J. M. Lipton, E. M. Sternberg, G. P. Chrousos, P. W. Gold, and C. C. Smith (New York, NY: New York Academy of Sciences), 33–44.
McEwen, B. S., Harold, S. E. A., and Milliken, M. (1999). Estrogen actions in the central nervous system. Endocr. Rev. 20, 279–307. doi: 10.1210/edrv.20.3.0365
McGowan, P. O., Suderman, M., Sasaki, A., Huang, T. C. T., Hallett, M., Meaney, M. J., et al. (2011). Broad epigenetic signature of maternal care in the brain of adult rats. PLoS One 6:e14739. doi: 10.1371/journal.pone.0014739
McKlveen, J. M., Myers, B., and Herman, J. P. (2015). The medial prefrontal cortex: coordinator of autonomic, neuroendocrine and behavioural responses to stress. J. Neuroendocrinol. 27, 446–456. doi: 10.1111/jne.12272
Michaud, J. L. (2001). The developmental program of the hypothalamus and its disorders. Clin. Genet. 60, 255–263. doi: 10.1034/j.1399-0004.2001.600402.x
Michaud, J. L., Rosenquist, T., May, N. R., and Fan, C. M. (1998). Development of neuroendocrine lineages requires the BHLH-PAS transcription factor SIM1. Genes Dev. 12, 3264–3275. doi: 10.1101/gad.12.20.3264
Miller, W. J. S., Suzuki, S., Miller, L. K., Handa, R. J., and Uht, R. M. (2004). Estrogen receptor (ER)β isoforms rather than ERα regulate corticotropin-releasing hormone promoter activity through an alternate pathway. J. Neurosci. 24, 10628–19635. doi: 10.1523/JNEUROSCI.5540-03.2004
Moir, L., Bochukova, E. G., Dumbell, R., Banks, G., Bains, R. S., Nolan, P. M., et al. (2017). Disruption of the homeodomain transcription factor orthopedia homeobox (Otp) is associated with obesity and anxiety. Mol. Metab. 6, 1419–1428. doi: 10.1016/j.molmet.2017.08.006
Morales-Delgado, N., Castro-Robles, B., Ferrán, J. L., Martinez-de-la-Torre, M., Puelles, L., and Díaz, C. (2014). Regionalized differentiation of CRH, TRH and GHRH peptidergic neurons in the mouse hypothalamus. Brain Struct. Funct. 219, 1083–1111. doi: 10.1007/s00429-013-0554-2
Morimoto, M., Morita, N., Ozawa, H., Yokoyama, K., and Kawata, M. (1996). Distribution of glucocorticoid receptor immunoreactivity and MRNA in the rat brain: an immunohistochemical and in situ hybridization study. Neurosci. Res. 26, 235–269. doi: 10.1016/s0168-0102(96)01105-4
Morohashi, K., and Zubair, M. (2011). The fetal and adult adrenal cortex. Mol. Cell. Endocrinol. 336, 193–197. doi: 10.1016/j.mce.2010.11.026
Muneoka, K., Ogawa, T., Kamei, K., Muraoka, S., Tomiyoshi, R., Mimura, Y., et al. (1997). Prenatal nicotine exposure affects the development of the central serotonergic system as well as the dopaminergic system in rat offspring: involvement of route of drug administrations. Dev. Brain Res. 102, 117–126. doi: 10.1016/s0165-3806(97)00092-8
Neumann, I. D. (2007). Stimuli and consequences of dendritic release of oxytocin within the brain. Biochem. Soc. Trans. 35, 1252–1257. doi: 10.1042/BST0351252
Neumann, I. D., Krömer, S. A., Toschi, N., and Ebner, K. (2000). Brain oxytocin inhibits the (re)activity of the hypothalamo-pituitary-adrenal axis in male rats: involvement of hypothalamic and limbic brain regions. Regul. Pept. 96, 31–38. doi: 10.1016/s0167-0115(00)00197-x
Nyirenda, M. J., Lindsay, R. S., Kenyon, C. J., Burchell, A., and Seckl, J. R. (1998). Glucocorticoid exposure in late gestation permanently programs rat hepatic phosphoenolpyruvate carboxykinase and glucocorticoid receptor expression and causes glucose intolerance in adult offspring. J. Clin. Invest. 101, 2174–2181. doi: 10.1172/JCI1567
Nyirenda, M. J., Welberg, L. A., and Seckl, J. R. (2001). Programming hyperglycaemia in the rat through prenatal exposure to glucocorticoids-fetal effect or maternal influence? J. Endocrinol. 170, 653–660. doi: 10.1677/joe.0.1700653
O’Regan, D., Kenyon, C. J., Seckl, J. R., and Holmes, M. C. (2004). Glucocorticoid exposure in late gestation in the rat permanently programs gender-specific differences in adult cardiovascular and metabolic physiology. Am. J. Physiol. 287, E863–E870. doi: 10.1152/ajpendo.00137.2004
O’Regan, D., Kenyon, C. J., Seckl, J. R., and Holmes, M. C. (2008). Prenatal dexamethasone “programmes” hypotension, but stress-induced hypertension in adult offspring. J. Endocrinol. 196, 343–352. doi: 10.1677/JOE-07-0327
Ochedalski, T., Subbaraju, S., Wynn, P. C., and Aguilera, G. (2007). Interaction between oestrogen and oxytocin on hypothalamic-pituitary-adrenal axis activity. J. Neuroendocrinol. 19, 189–197. doi: 10.1111/j.1365-2826.2006.01525.x
Ohbuchi, T., Haam, J., and Tasker, J. G. (2015). Regulation of neuronal activity in hypothalamic vasopressin neurons. Interdiscip. Inf. Sci. 21, 225–234. doi: 10.4036/iis.2015.B.07
Ojeda, S. R., and Urbanski, H. F. (1994). “Puberty in the rat,” in The Physiology of Reproduction, eds E. Knobil and J. D. Neill (New York, NY: Raven Press, Ltd.), 363–409.
Oyola, M. G., Thompson, M. K., Handa, A. Z., and Handa, R. J. (2017). Distribution of chemical composition of estrogen receptor β neurons in the paraventricular nucleus of the female and male mouse hypothalamus. J. Comp. Neurol. 525, 3666–3682. doi: 10.1002/cne.24295
Pacak, K. (2011). Phaeochromocytoma: a catecholamine and oxidative stress disorder. Endocr. Regul. 45, 65–90. doi: 10.4149/endo_2011_02_65
Pak, T. R., Chung, W. C. J., Hinds, L. R., and Handa, R. J. (2007). Estrogen receptor-beta mediates dihydrotestosterone-induced stimulation of the arginine vasopressin promoter in neuronal cells. Endocrinology 148, 3371–3382. doi: 10.1210/en.2007-0086
Papadimitriou, A., and Priftis, K. N. (2009). Regulation of the hypothalamic-pituitary-adrenal axis. Neuroimmunomodulation 16, 265–271. doi: 10.1159/000216184
Parenti, G., Zampetti, B., Rapizzi, E., Ercolino, T., Giachè, V., and Mannelli, M. (2012). Updated and new perspectives on diagnosis, prognosis and therapy of malignant pheochromocytoma/paraganglioma. J. Oncol. 2012:872713. doi: 10.1155/2012/872713
Patchev, V. K., Hassan, A. H., Holsboer, D. F., and Almeida, O. F. (1996). The neurosteroid tetrahydroprogesterone attenuates the endocrine response to stress and exerts glucocorticoid-like effects on vasopressin gene transcription in the rat hypothalamus. Neuropsychopharmacology 15, 533–540. doi: 10.1016/S0893-133X(96)00096-6
Patchev, V. K., Hayashi, S., Orikasa, C., and Almeida, O. F. (1995). Implications of estrogen-dependent brain organization for gender differences in hypothalmo-pituitary-adrenal regulation. FASEB J. 9, 419–423. doi: 10.1096/fasebj.9.5.7896013
Pulichino, A., Vallette-Kasic, S., Couture, C., Gauthier, Y., Brue, T., David, M., et al. (2003). Human and mouse TPIT gene mutations cause early onset pituitary ACTH deficiency. Genes Dev. 17, 711–716. doi: 10.1101/gad.1065603
Qian, X., Droste, S. K., Gutièrrez-Mecinas, M., Collins, A., Kersanté, F., Reul, J. M. H. M., et al. (2011). A rapid release of corticosteroid-binding globulin from the liver restrains the glucocorticoid hormone response to acute stress. Endocrinology 152, 3738–3748. doi: 10.1210/en.2011-1008
Raadsheer, F. C., Sluiter, A. A., Ravid, R., Tilders, F. J. H., and Swaab, D. F. (1993). Localization of corticotropin-releasing hormone (CRH) neurons in the paraventricular nucleus of the human hypothalamus; age-dependent colocalization with vasopressin. Brain Res. 615, 50–62. doi: 10.1016/0006-8993(93)91113-7
Rabadan-Diehl, C., Makara, G., Kiss, A., Zelena, D., and Aguilera, G. (1997). Regulation of pituitary corticotropin releasing hormone (CRH) receptor MRNA and CRH binding during adrenalectomy: role of glucocorticoids and hypothalamic factors. J. Neuroendocrinol. 9, 689–697. doi: 10.1046/j.1365-2826.1997.00626.x
Rakova, N., Kitada, K., Lerchl, K., Dahlmann, A., Birukov, A., Daub, S., et al. (2017). Increased salt consumption induces body water conservation and decreases fluid intake. J. Clin. Invest. 127, 1932–1943. doi: 10.1172/JCI88530
Rankin, J., Walker, J. J., Windle, R., Lightman, S. L., and Terry, J. R. (2012). Characterizing dynamic interactions between ultradian glucocorticoid rhythmicity and acute stress using the phase response curve. PLoS One 7:e30978. doi: 10.1371/journal.pone.0030978
Reul, J. M., and de Kloet, E. R. (1985). Two receptor systems for corticosterone in rat brain: microdistribution and differential occupation. Endocrinology 117, 2505–2511. doi: 10.1210/endo-117-6-2505
Rhodes, C. H., Morriell, J. I., and Pfaff, D. W. (1981). Immunohistochemical analysis of magnocellular elements in rat hypothalamus: distribution and numbers of cells containing neurophysin, oxytocin and vasopressin. J. Comp. Neurol. 198, 45–64. doi: 10.1002/cne.901980106
Rivier, C., and Vale, W. (1983). Modulation of stress-induced ACTH release by corticotropin-releasing factor, catecholamines and vasopressin. Nature 305, 325–327. doi: 10.1038/305325a0
Roberts, D., and Dalziel, S. (2006). Antenatal corticosteroids for accelerating fetal lung maturation for women at risk of preterm birth. Cochrane Database Syst. Rev. 19:CD004454. doi: 10.1002/14651858.CD004454.pub2
Rodgers, A. B., Morgan, C. P., Bronson, S. L., Revello, S., and Bale, T. L. (2013). Paternal stress exposure alters sperm microRNA content and reprograms offspring HPA stress axis regulation. J. Neurosci. 33, 9003–9012. doi: 10.1523/JNEUROSCI.0914-13.2013
Romeo, R. D. (2003). Puberty: a period of both organizational and activational effects of steroid hormones on neurobehavioural development. J. Neuroendocrinol. 15, 1185–1192. doi: 10.1111/j.1365-2826.2003.01106.x
Romeo, R. D. (2010). Pubertal maturation and programming of hypothalamic-pituitary-adrenal reactivity. Front. Neuroendocrinol. 31, 232–240. doi: 10.1016/j.yfrne.2010.02.004
Romeo, R. D., Bellani, R., Karatsoreos, I. N., Chhua, N., Vernov, M., Conrad, C. D., et al. (2006). Stress history and pubertal development interact to shape hypothalamic pituitary adrenal axis plasticity. Endocrinology 147, 1667–1674. doi: 10.1210/en.2005-1432
Romeo, R. D., Lee, S. J., Chhua, N., McPherson, C. R., and McEwen, B. S. (2004a). Testosterone cannot activate an adult-like stress response in prepubertal male rats. Neuroendocrinology 79, 125–132. doi: 10.1159/000077270
Romeo, R. D., Lee, S. J., and McEwen, B. S. (2004b). Differential stress reactivity in intact and ovariectomized prepubertal and adult female rats. Neuroendocrinology 80, 387–393. doi: 10.1159/000084203
Romeo, R. D., and McEwen, B. S. (2006). Stress and the adolescent brain. Ann. N. Y. Acad. Sci. 1094, 202–214. doi: 10.1196/annals.1376.022
Rood, B. D., and De Vries, G. J. (2011). Vasopressin innervation of the mouse (Mus musculus) brain and spinal cord. J. Comp. Neurol. 519, 2434–2474. doi: 10.1002/cne.22635
Rood, B. D., Stott, R. T., You, S., Smith, C. J. W., Woodbury, M. E., and De Vries, G. J. (2013). Site of origin of and sex differences in the vasopressin innervation of the mouse (Mus musculus) brain. J. Comp. Neurol. 521, 2321–2358. doi: 10.1002/cne.23288
Roper, J. A., O’Carroll, A.-M., Young, W. S. III, and Lolait, S. J. (2011). The vasopressin avpr1b receptor: molecular and pharmacological studies. Stress 14, 98–115. doi: 10.3109/10253890.2010.512376
Rosinger, Z. J., Jacobskind, J. S., Bulanchuk, N., Malone, M., Fico, D., Justice, N. J., et al. (2018). Characterization and gonadal hormone regulation of a sexually dimorphic corticotropin releasing factor receptor 1 cell group. J. Comp. Neurol. 527, 1056–1069. doi: 10.1002/cne.24588
Rossi, D. V., Dai, Y., Thomas, P., Carrasco, G. A., DonCarlos, L. L., Muma, N. A., et al. (2010). Estradiol-induced desensitization of 5-HT1A receptor signaling in the paraventricular nucleus of the hypothalamus is independent of estrogen receptor-beta. Psychoneuroendocrinology 35, 1023–1033. doi: 10.1016/j.psyneuen.2010.01.003
Rozeboom, A. M., Akil, H., and Seasholtz, A. F. (2007). Mineralocorticoid receptor overexpression in forebrain decreases anxiety-like behavior and alters the stress response in mice. Proc. Natl. Acad. Sci. U S A 104, 4688–4693. doi: 10.1073/pnas.0606067104
Ruel, J. M., and de Kloet, E. R. (1985). Two receptor systems for corticosterone in rat brain: microdistribution and differential occupation. Endocrinology 117, 2505–2511. doi: 10.1210/endo-117-6-2505
Sandman, C. A., Buss, C., Head, K., and Davis, E. P. (2015). Fetal exposure to maternal depressive symptoms is associated with cortical thickness in late childhood. Biol. Psychiatry 77, 324–334. doi: 10.1016/j.biopsych.2014.06.025
Sapolsky, R. M., Krey, L. C., and McEwen, B. S. (1984). Stress down-regulates corticosterone receptors in a site-specific manner in the brain. Endocrinology 114, 287–292. doi: 10.1210/endo-114-1-287
Sapolsky, R. M., Romero, L. M., and Munck, A. U. (2000). How Do glucocorticoids influence stress responses? Integrating, suppressive, stimulatory and preparative actions. Endocr. Rev. 21, 55–89. doi: 10.1210/edrv.21.1.0389
Sawchenko, P. E. (1987). Evidence for a local site of action for glucocorticoids in inhibiting CRF and vasopressin expression in the paraventricular nucleus. Brain Res. 403, 213–223. doi: 10.1016/0006-8993(87)90058-8
Sawchenko, P. E., Swanson, L. W., Steinbusch, H. W., and Verhofstad, A. A. (1983). The distribution and cells of origin of serotonergic inputs to the paraventricular and supraoptic nuclei of the rat. Brain Res. 277, 355–360. doi: 10.1016/0006-8993(83)90945-9
Sawchenko, P. E., Swanson, L. W., and Vale, W. W. (1984). Co-expression of corticotropin-releasing factor and vasopressin immunoreactivity in parvocellular neurosecretory neurons of the adrenalectomized rat. Proc. Natl. Acad. Sci. U S A 81, 1883–1887. doi: 10.1073/pnas.81.6.1883
Scanes, C. G. (2015). Pituitary Gland. Sturkie’s Avian Physiology, 6th edition. Milwaukee, WI: Academic Press.
Schlosser, S. F., Almeida, O. F., Patchev, V. K., Yassouridis, A., and Elands, J. (1994). Oxytocin-stimulated release of adrenocorticotropin from the rat pituitary is mediated by arginine vasopressin receptors of the V1b Type. Endocrinology 135, 2058–2063. doi: 10.1210/endo.135.5.7956927
Schmidt, M. V., Enthoven, L., van der Mark, M., Levine, S., de Kloet, E. R. and Oitzl, M. S. (2003). The postnatal development of the hypothalamic–pituitary–adrenal axis in the mouse. Int. J. Dev. Neurosci. 21, 125–132. doi: 10.1016/S0736-5748(03)00030-3
Schonemann, M. D., Ryan, A. K., McEvilly, R. J., O’Connell, S. M., Arias, C. A., Kalla, K. A., et al. (1995). Development and survival of the endocrine hypothalamus and posterior pituitary gland requires the neuronal POU domain factor Brn-2. Genes Dev. 9, 3122–3135. doi: 10.1101/gad.9.24.3122
Seale, J. V., Wood, S. A., Atkinson, H. C., Bate, E., Lightman, S. L., Ingram, C. D., et al. (2004). Gonadectomy reverses the sexually diergic patterns of circadian and stress-induced hypothalamic-pituitary-adrenal axis activity in male and female rats. J. Neuroendocrinol. 16, 516–524. doi: 10.1111/j.1365-2826.2004.01195.x
Seale, J. V., Wood, S. A., Atkinson, H. C., Harbuz, M. S., and Lightman, S. L. (2005a). Postnatal masculinization alters the HPA axis phenotype in the adult female rat. J. Physiol. 563, 265–274. doi: 10.1113/jphysiol.2004.078212
Seale, J. V., Wood, S. A., Atkinson, H. C., Lightman, S. L., and Harbuz, M. S. (2005b). Organizational role for testosterone and estrogen on adult hypothalamic-pituitary-adrenal axis activity in the male rat. Endocrinology 146, 1973–1982. doi: 10.1210/en.2004-1201
Shapiro, R. A., Xu, C., and Dorsa, D. M. (2000). Differential transcriptional regulation of rat vasopressin gene expression by estrogen receptor α and β. Endocrinology 141, 4056–4064. doi: 10.1210/endo.141.11.7796
Shoener, J. A., Baig, R., and Page, K. C. (2006). Prenatal exposure to dexamethasone alters hippocampal drive on hypothalamic-pituitary-adrenal axis activity in adult male rats. Am. J. Physiol. Regl. Integr. Comp. Physiol. 290, R1366–R1373. doi: 10.1152/ajpregu.00757.2004
Simerly, R. B. (1993). Distribution and regulation of steroid hormone receptor gene expression in the central nervous system. Adv. Neurol. 59, 207–226.
Sinding, C., Czernichow, P., Seif, S. M., and Robinson, A. G. (1980). Quantitative changes in neurohypophyseal peptides in the developing brain. Peptides 1, 45–50. doi: 10.1016/0196-9781(80)90102-3
Sollars, P. J., Weiser, M. J., Kudwa, A. E., Bramley, J. R., Ogilvie, M. D., Spencer, R. L., et al. (2014). Altered entrainment to the day/night cycle attenuates the daily rise in circulating corticosterone in the mouse. PLoS One 9:e111944. doi: 10.1371/journal.pone.0111944
Solomon, M. B., Loftspring, M., de Kloet, A. D., Ghosal, S., Jankord, R., Flak, J. N., et al. (2015). Neuroendocrine function after hypothalamic depletion of glucocorticoid receptors in male and female mice. Endocrinology 156, 2843–2853. doi: 10.1210/en.2015-1276
Speert, D. B., MCClennen, S. J., and Seasholtz, A. F. (2002). Sexually dimorphic expression of corticotropin-releasing hormone-binding protein in the mouse pituitary. Endocrinology 143, 4730–4731. doi: 10.1210/en.2002-220556
Speiser, P. W., Azziz, R., Baskin, L. S., Lucia, G., Hensle, T. W., Merke, D. P., et al. (2010). Congenital adrenal hyperplasia due to steroid 21-hydroxylase deficiency: an endocrine society clinical practice guideline. J. Clin. Endocrinol. Metab. 95, 4133–4160. doi: 10.1210/jc.2018-01865
Spiga, F., Zavala, E., Walker, J. J., Zhao, Z., Terry, J. R., and Lightman, S. L. (2017). Dynamic responses of the adrenal steroidogenic regulatory network. PNAS 114, E6466–E6474. doi: 10.1073/pnas.1703779114
Stroud, L. R., Papandonatos, G. D., Parade, S. H., Salisbury, A. L., Phipps, M. G., Lester, B. M., et al. (2016). Prenatal major depressive disorder, placenta glucocorticoid and serotonergic signaling and infant cortisol response. Psychosom. Med. 78, 979–990. doi: 10.1097/PSY.0000000000000410
Sun, T., Gonzalez, T. L., Deng, N., DiPentino, R., Clark, E. L., Lee, B., et al. (2020). Sexually dimorphic crosstalk at the maternal-fetal interface. J. Clin. Endocrinol. Metab. 105, e4831–e4847. doi: 10.1210/clinem/dgaa503
Sun, N., Wu, Y., Nanba, K., Sbiera, S., Kircher, S., Kunzke, T., et al. (2018). High-resolution tissue mass spectrometry imaging reveals a refined functional anatomy of the human adult adrenal gland. Endocrinology 159, 1511–1524. doi: 10.1210/en.2018-00064
Swanson, L. W., and Kuypers, H. G. (1980). The paraventricular nucleus of the hypothalamus: cytoarchitectonic subdivisions and organization of projections to the pituitary, dorsal vagal complex and spinal cord as demonstrated by retrograde fluorescence double-labeling methods. J. Comp. Neurol. 194, 555–570. doi: 10.1002/cne.901940306
Swanson, L. W., and Petrovich, G. D. (1998). What is the amygdala? Trends Neurosci. 21, 323–331. doi: 10.1016/s0166-2236(98)01265-x
Swanson, L. W., and Sawchenko, P. E. (1983). Hypothalamic integration: organization of the paraventricular and supraoptic nuclei. Ann. Rev. Neurosci. 6, 269–324. doi: 10.1146/annurev.ne.06.030183.001413
Szot, P., and Dorsa, D. M. (1993). Differential timing and sexual dimorphism in the expression of the vasopressin gene in the developing rat brain. Brain Res. Dev. Brain Res. 73, 177–183. doi: 10.1016/0165-3806(93)90136-x
Takata, N., Sakakura, E., Eiraku, M., Kasukawa, T., and Sasai, Y. (2017). Self-patterning of rostral-caudal neuroectoderm requires dual role of fgf signaling for localized wnt antagonism. Nat. Commun. 8:1339. doi: 10.1038/s41467-017-01105-2
Tamborski, S., Mintz, E. M., and Caldwell, H. K. (2016). Sex differences in the embryonic development of the central oxytocin system in mice. J. Neuroendocrinol. 28. doi: 10.1111/jne.12364
Tamura, K., Umemura, S., Fukamizu, A., Ishii, M., and Murakami, K. (1995). Recent advances in the study of renin and angiotensinogen genes: from molecules to the whole body. Hypertens Res. 18, 7–18. doi: 10.1291/hypres.18.7
Tasker, J. G., and Dudek, F. E. (1991). Electrophysical properties of neurones in the region of the paraventricular nucleus in slices of rat hypothalamus. J. Physiol. 443, 271–293.
Tinnikov, A. A. (1999). Responses of serum corticosterone and corticosteroid-binding globulin to acute and prolonged stress in the rat. Endocrine 11, 145–150. doi: 10.1385/ENDO:11:2:145
Tsigos, C., and Chrousos, G. P. (2002). Hypothalamic-pituitary-adrenal axis, neuroendocrine factors and stress. J. Psychosom. Res. 53, 865–871. doi: 10.1016/s0022-3999(02)00429-4
Van de Kar, L. D., and Blair, M. L. (1999). Forebrain pathways mediating stress-induced hormone secretion. Front. Neuroendocrinol. 20, 1–48. doi: 10.1006/frne.1998.0172
Van Haarst, A. D., Oitzl, M. S., and de Kloet, E. R. (1997). Facilitation of feedback inhibition through blockade of glucocorticoid receptors in the hippocampus. Neurochem. Res. 22, 1323–1328. doi: 10.1023/a:1022010904600
Van Tol, H. H. M., Voorhius, D. T. H. A. M., and Burbach, J. P. H. (1987). Oxytocin gene expression in discrete hypothalamic magnocellular cell groups is stimulated by prolonged salt loading. Endocrinology 120, 71–76. doi: 10.1210/endo-120-1-71
Vandesande, F., and Dierickx, K. (1975). Identification of the vasopressin producing and of the oxytocin producing neurons in the hypothalamic magnocellular neurosecretory system of the rat. Cell Tissue Res. 164, 153–162. doi: 10.1007/BF00218970
Vazquez, D. M., Bailey, C., Dent, G. W., Okimoto, D. K., Steffek, A., López, J. F., et al. (2006). Brain corticotropin-releasing hormone (CRH) circuits in the developing rat: effect of maternal deprivation. Brain Res. 1121, 83–94. doi: 10.1016/j.brainres.2006.08.104
Viau, V., Bingham, B., Davis, J., Lee, P., and Wong, M. (2005). Gender and puberty interact on the stress-induced activation of parvocellular neurosecretory neurons and corticotropin-releasing hormone messenger ribonucleic acid expression in the rat. Endocrinology 146, 137–146. doi: 10.1210/en.2004-0846
Viau, V., and Meaney, M. J. (1996). The inhibitory effect of testosterone on hypothalamic-pituitary-adrenal responses to stress is mediated by the medial preoptic area. J. Neurosci. 16, 1866–1876. doi: 10.1523/JNEUROSCI.16-05-01866.1996
Viau, V., and Meaney, M. J. (2004). Testosterone-dependent variations in plasma and intrapituitary corticosteroid binding globulin and stress hypothalamic-pituitary-adrenal activity in the male rat. J. Endocrinol. 181, 223–231. doi: 10.1677/joe.0.1810223
Viau, V., Soriano, L., and Dallman, M. F. (2001). Androgens alter corticotropin releasing hormone and arginine vasopressin mRNA within forebrain sites known to regulate activity in the hypothalamic-pituitary-adrenal axis. J. Neuroendocrinol. 13, 442–452. doi: 10.1046/j.1365-2826.2001.00653.x
Vinson, G. P., Hinson, J. P., and Tóth, I. E. (1994). The neuroendocrinology of the adrenal cortex. J. Neuroendocrinol. 6, 235–246. doi: 10.1111/j.1365-2826.1994.tb00578.x
Walaas, I., and Fonnum, F. (1980). Biochemical evidence for glutamate as a transmitter in hippocampal efferents to the basal forebrain and hypothalamus in the rat brain. Neuroscience 5, 1691–1698. doi: 10.1016/0306-4522(80)90088-3
Walker, J. J., Spiga, F., Waite, E., Zhao, Z., Kershaw, Y., Terry, J. R., et al. (2012). The origin of glucocorticoid hormone oscillations. PLoS Biol. 10:e1001341. doi: 10.1371/journal.pbio.1001341
Walker, B. R., Williams, B. C., and Edwards, C. R. (1994). Regulation of 11 beta-hydroxysteroid dehydrogenase activity by the hypothalamic-pituitary-adrenal axis in the rat. J. Endocrinol. 141, 467–472. doi: 10.1677/joe.0.1410467
Wamsteeker-Cusulin, J. I., Füzesi, T., Watts, A. G., and Bains, J. S. (2013). Characterization of corticotropin-releasing hormone neurons in the paraventricular nucleus of the hypothalamus of Crh-IRES-Cre mutant mice. PLoS One 8:64943. doi: 10.1371/journal.pone.0064943
Wang, D., He, X., Zhao, Z., Feng, Q., Lin, R., Sun, Y., et al. (2015). Whole-brain mapping of the direct inputs and axonal projections of POMC and AgRP neurons. Front. Neuroanat. 9:40. doi: 10.3389/fnana.2015.00040
Weaver, I. C. G., Cervoni, N., Champagne, F. A., D’Alessio, A. C., Sharma, S., Seckl, J. R., et al. (2004). Epigenetic programming by maternal behavior. Nat. Neurosci. 7, 847–854. doi: 10.1038/nn1276
Wei, Q., Lu, X., Liu, L., Schafer, G., Shieh, K., Burke, S., et al. (2004). Glucocorticoid receptor overexpression in forebrain: a mouse model of increased emotional lability. Proc. Natl. Acad. Sci. U S A 101, 11851–11856. doi: 10.1073/pnas.0402208101
Weidenfeld, J., Feldman, S., Itzik, A., Van de Kar, L. D., and Newman, M. E. (2002). Evidence for a mutual interaction between noradrenergic and serotonergic agonists in stimulation of ACTH and corticosterone secretion in the rat. Brain Res. 941, 113–117. doi: 10.1016/s0006-8993(02)02641-0
Weiser, M. J., and Handa, R. J. (2009). Estrogen impairs glucocorticoid dependent negative feedback on the hypothalamic-pituitary-adrenal axis via estrogen receptor alpha within the hypothalamus. Neuroscience 159, 883–895. doi: 10.1016/j.neuroscience.2008.12.058
Weisz, J., and Ward, I. L. (1980). Plasma testosterone and progesterone titers of pregnant rats, their male and female fetuses and neonatal offspring. Endocrinology 106, 306–316. doi: 10.1210/endo-106-1-306
Whitnall, M. H., Key, S., Ben-Barak, Y., Ozato, K., and Gainer, H. (1985). Immunocytochemical studies of the ontogeny of oxytocinergic and vasopressinergic neurons’. J. Neurosci. 5, 98–109. doi: 10.1523/JNEUROSCI.05-01-00098.1985
Williamson, M., and Viau, V. (2008). Selective contributions of the medial preoptic nucleus to testosteronedependant regulation of the paraventricular nucleus of the hypothalamus and the HPA axis. Am. J. Physiol. Regul. Integr. Comp. Physiol. 295, 1020–1030. doi: 10.1152/ajpregu.90389.2008
Witzmann, S. R., Turner, J. D., Meriaux, S. B., Meijer, O. C., and Muller, C. P. (2012). Epigenetic regulation of the glucocorticoid receptor promoter 17 in adult rats. Epigenetics 7, 1290–1301. doi: 10.4161/epi.22363
Wolf, D., Harris, N., and Rotter, V. (1984). Reconstitution of P53 expression in a nonproducer Ab-MuLV-transformed cell line by transfection of a functional P53 gene. Cell 38, 119–126. doi: 10.1016/0092-8674(84)90532-4
Yamashita, T., Tomoo, K. K., and Kawashima, S. (1988). Arginine vasopressin contents of the hypothalamus and pituitary during fetal and postnatal development in the mouse. Dev. Growth Differ. 30, 563–571. doi: 10.1111/j.1440-169X.1988.00563.x
Yi, S. J., Masters, J. N., and Baram, T. Z. (1994). Glucocorticoid receptor mRNA ontogeny in the fetal and postnatal rat forebrain. Mol. Cell. Neursci. 5, 385–393. doi: 10.1006/mcne.1994.1048
Young, E. A., Altemus, M., Parkinson, V., and Shastry, S. (2001). Effects of estrogen antagonists and agonists on the ACTH response to restraint stress in female rats. Neuropsychopharmacology 25, 881–891. doi: 10.1016/S0893-133X(01)00301-3
Zakharova, E., Miller, J., Unterwald, E., Wade, D., and Izenwasser, S. (2009). Social and physical environment alter cocaine conditioned place preference and dopaminergic markers in adolescent male rats. Neuroscience 163, 890–897. doi: 10.1016/j.neuroscience.2009.06.068
Zhang, X., and Fogel, R. (2002). Glutamate mediates an excitatory influence of the paraventricular hypothalamic nucleus on the dorsal motor nucleus of the vagus. J. Neurophysiol. 88, 49–63. doi: 10.1152/jn.2002.88.1.49
Zhao, L., Bakke, M., Krimkevich, Y., Cushman, L. J., Parlow, A. F., Camper, S. A., et al. (2001). Steroidogenic factor 1 (SF1) is essential for pituitary gonadotrope function. Development 128, 147–154.
Zhu, X., Gleiberman, A. S., and Rosenfeld, M. G. (2007). Molecular physiology of pituitary development: signaling and transcriptional networks. Physiol. Rev. 87, 933–963. doi: 10.1152/physrev.00006.2006
Zubair, M., Ishihara, S., Oka, S., Okumura, K., and Morohashi, K. (2006). Two-step regulation of Ad4BP/SF-1 gene transcription during fetal adrenal development: initiation by a hox-Pbx1-Prep1 complex and maintenance via autoregulation by Ad4BP/SF-1. Mol. Cell. Biol. 26, 4111–4121. doi: 10.1128/MCB.00222-06
Keywords: estrogen, androgens, glucocorticoid, sex differences, hypothalamus, development, homeostasis, HPA axis
Citation: Sheng JA, Bales NJ, Myers SA, Bautista AI, Roueinfar M, Hale TM and Handa RJ (2021) The Hypothalamic-Pituitary-Adrenal Axis: Development, Programming Actions of Hormones, and Maternal-Fetal Interactions. Front. Behav. Neurosci. 14:601939. doi: 10.3389/fnbeh.2020.601939
Received: 02 September 2020; Accepted: 10 December 2020;
Published: 13 January 2021.
Edited by:
Juan M. Dominguez, University of Texas at Austin, United StatesReviewed by:
Tomoko Soga, Monash University Malaysia, MalaysiaOnno Meijer, Leiden University, Netherlands
Copyright © 2021 Sheng, Bales, Myers, Bautista, Roueinfar, Hale and Handa. This is an open-access article distributed under the terms of the Creative Commons Attribution License (CC BY). The use, distribution or reproduction in other forums is permitted, provided the original author(s) and the copyright owner(s) are credited and that the original publication in this journal is cited, in accordance with accepted academic practice. No use, distribution or reproduction is permitted which does not comply with these terms.
*Correspondence: Robert J. Handa, YmhhbmRhQGNvbG9zdGF0ZS5lZHU=