- Department of Neuroscience, University of Minnesota, Minneapolis, MN, United States
Our social relationships determine our health and well-being. In rodent models, there is now strong support for the rewarding properties of aggressive or assertive behaviors to be critical for the expression and development of adaptive social relationships, buffering from stress and protecting from the development of psychiatric disorders such as depression. However, due to the false belief that aggression is not a part of the normal repertoire of social behaviors displayed by females, almost nothing is known about the neural mechanisms mediating the rewarding properties of aggression in half the population. In the following study, using Syrian hamsters as a well-validated and translational model of female aggression, we investigated the effects of aggressive experience on the expression of markers of postsynaptic structure (PSD-95, Caskin I) and excitatory synaptic transmission (GluA1, GluA2, GluA4, NR2A, NR2B, mGluR1a, and mGluR5) in the nucleus accumbens (NAc), caudate putamen and prefrontal cortex. Aggressive experience resulted in an increase in PSD-95, GluA1 and the dimer form of mGluR5 specifically in the NAc 24 h following aggressive experience. There was also an increase in the dimer form of mGluR1a 1 week following aggressive experience. Aggressive experience also resulted in an increase in the strength of the association between these postsynaptic proteins and glutamate receptors, supporting a common mechanism of action. In addition, 1 week following aggressive experience there was a positive correlation between the monomer of mGluR5 and multiple AMPAR and NMDAR subunits. In conclusion, we provide evidence that aggressive experience in females results in an increase in the expression of postsynaptic density, AMPARs and group I metabotropic glutamate receptors, and an increase in the strength of the association between postsynaptic proteins and glutamate receptors. This suggests that aggressive experience may result in an increase in excitatory synaptic transmission in the NAc, potentially encoding the rewarding and behavioral effects of aggressive interactions.
Introduction
Approximately one in five adults in the United States now suffer from a diagnosable mental disorder, such as depression, schizophrenia, anxiety disorder, and autism spectrum disorder (National Institute of Mental Health website: statistics). Importantly, common and overlapping traits key to many of these disorders are deficits in social processes (Henry et al., 2016; Novacek et al., 2016; Pegg et al., 2019; Snyder-Mackler et al., 2020). Hence, the rewarding properties of social interactions are critical for the expression of adaptive social behaviors (Darwin, 1859; Skinner, 1938; Trezza et al., 2011; Borland et al., 2017) and the development and maintenance of social relationships (Young and Wang, 2004; Krach et al., 2010; Borland et al., 2019b). These relationships can serve as a buffer to stress (Morrison et al., 2014; Smith and Wang, 2014) and reduce susceptibility to psychiatric and addictive disorders (Bodenmann and Randall, 2013; Nagy and Moore, 2017; Fett et al., 2019). Social interactions come in a variety of forms, including affiliation, aggression, parental and sexual behaviors, each with distinct molecular signatures (Young and Wang, 2004; Shahrokh et al., 2010; Albers, 2012; Staffend and Meisel, 2012; Staffend et al., 2014; Bredewold et al., 2015); yet due to the false belief that aggression is not a part of the normal repertoire of social behaviors displayed by females (Buss, 1961; Blanchard et al., 1988; Albers et al., 2002; Björkqvist, 2018), very little is known about the neural mechanisms mediating aggression in this sex (Been et al., 2019).
It is important to note that while previous studies of aggression have primarily focused on its maladaptive and destructive nature (Siever, 2008; Golden et al., 2019b), aggression under many circumstances can be advantageous (Crombach and Elbert, 2014; Wrangham, 2018). For example, assertiveness is key to protecting one’s rights and privileges (e.g., resist “bullying”). It is in this context that the positive rewarding effects of resisting intrusions can help develop a positive level of self-esteem that supports mental health (Morrison et al., 2014; Snyder-Mackler et al., 2016; Komori et al., 2019). Indeed, the pervasive expression of agonistic encounters, dominant-subordinate relationships, and ultimately social hierarchies across the animal kingdom is evidence of the beneficial, adaptive and rewarding properties of aggression (Meisel and Joppa, 1994; Abbott et al., 1998; Edwards and Spitzer, 2006; Pettinger et al., 2011; Albers, 2012; Gil et al., 2013). These adaptive processes are critical for resource allocation, reproductive success and thus survival for males and females (Digby and McLean Stevens, 2007; Nelson and Trainor, 2007; Lindenfors and Tullberg, 2011; Stockley and Bro-Jorgensen, 2011; Giebel et al., 2013).
The apex in the central nervous system for reward processing is the nucleus accumbens (NAc) (Koob, 1996; Salamone et al., 2009; Mohebi et al., 2019). Changes in the electrical transmission of neurons in this region underlie reward processing (Schultz et al., 1997; Kourrich et al., 2007). Using female Syrian hamsters, a well validated animal model for studying aggression in this sex (Drickamer and Vandenbergh, 1973), our lab recently showed that aggressive experience results in an increase of mature mushroom-like head dendritic spines on NAc medium spiny neurons (MSNs) (Staffend and Meisel, 2012). Although the molecular and physiological mechanisms mediating these postsynaptic changes are unknown, follow-up studies from our lab suggest PSD-95 and mGluR5 play a functional role in this synaptic structural plasticity (Peterson et al., 2015; Been et al., 2016; Gross et al., 2016). Furthermore, previous literature suggests that increases in mushroom head spines may be associated with increases in excitatory synaptic transmission and long-term potentiation; more specifically an increase in AMPAR expression (Malinow and Malenka, 2002; Muddashetty et al., 2007; Takahashi et al., 2009; Bencsik et al., 2019).
The glutamate system is universal in neuronal excitatory plasticity, consisting of a diverse assortment of ionotropic (AMPA/NMDA) and metabotropic (mGluR1-8) receptors with various kinetic and physiological properties (Reiner and Levitz, 2018; Goncalves et al., 2020). We postulate that increases in excitatory synaptic transmission in the NAc will likely encode the rewarding properties of aggression, similar to the effects of addictive drug exposure (Kourrich et al., 2007; Ebner et al., 2018; Turner et al., 2018a; Stagkourakis et al., 2020). To begin to develop this line of research, we investigated the effects of female aggressive experience on the expression of proteins associated with postsynaptic plasticity (PSD-95 and Caskin I) and excitatory synaptic transmission, AMPARs (GluA1, GluA2, and GluA4), NMDARs (NR2A and NR2B) and group I mGluRs (Gq-protein coupled monomer and dimer forms of mGluR1a and mGluR5) in the NAc. Furthermore, to gain insight into potential mechanisms mediating changes in postsynaptic density and excitatory synaptic transmission, we investigated the strength of associations between the various postsynaptic structure proteins and glutamate receptors analyzed, and potential effects of aggressive experience on these relationships. We hypothesized that aggressive experience results in the strengthening of the relationships between postsynaptic proteins and glutamate receptors in the NAc. In addition, based on previous literature (Been et al., 2016), we predict mGluR5 expression in particular to play a central role in this aggression mediated postsynaptic plasticity.
Materials and Methods
Subjects
Adult female (n = 36) and male Syrian hamsters (n = 24) (Mesocricetus auratus) were purchased from Charles River Laboratories (Wilmington, MA, United States) at approximately 60 days of age (120–130 g). Subject females were housed individually while intruder males were pair-housed in polycarbonate cages (females: 50.8 cm × 40.6 cm × 20.3 cm; males: 43.2 cm × 22.9 cm × 20.3 cm). These semi-natural housing arrangements maximize aggression in female subjects, while decreasing aggression in male intruders (Brain, 1972; Grelk et al., 1974; Gattermann et al., 2001; Ross et al., 2017). All animals were maintained on a reversed 14:10 light/dark photoperiod (lights off at 1300 h) and all behavioral testing occurred during the first 3 h of the dark phase. The animal room was maintained at a controlled temperature of 22°C and food and water were available ad libitum. Hamsters acclimated for 2 weeks before experiments. All animal procedures were carried out in accordance with the National Institutes of Health Guide for the Care and Use of Laboratory Animals (NIH Publications No. 80-23; revised 2011) and approved by the University of Minnesota Institutional Animal Care and Use Committee.
Ovariectomy
To maximize aggressive behavior in females, circulating estradiol levels were maintained at low levels via bilateral ovariectomy (Meisel et al., 1988) under sterile conditions. Surgery was conducted under sodium pentobarbital anesthesia (Nembutal, 8.5 mg/100 g body weight, i.p.). Analgesic (Meloxicam, 1 mg/kg, s.c., Fort Dodge Animal Health, Overland Park, KS, United States) was administered for 3 days of post-operative pain management. Two subjects were preemptively sacrificed due to complications during surgery.
Behavioral Testing and Scoring
Females were handled daily for at least 4 days prior to the first aggressive interaction session. A different adult male stimulus hamster was placed into a female subject’s home cage for five min each day for a five-day period. Each session was video recorded and later scored for two main criteria: (1) the latency to the subject’s first attack and (2) the total number of attacks. A two-step process was used to verify scoring (live scoring followed by video scoring). Attacks were operationally defined as the subject biting, or clearly attempting to bite, the intruder male. Intruder males were used at most every other day and for each test individual females were paired with a different male to minimize the likelihood that submission by the male would influence the behavior of the females (Huhman et al., 2003). No social interaction control females did not interact with males but did undergo the same handling and transport experiences as experimental groups.
Tissue Collection
Twenty-four hours or 1 week following the fifth aggression test females were given an overdose of sodium pentobarbital (Beuthanasia-D, 0.25 ml i.p.,/animal, Schering, Union, NJ, United States) and sacrificed by rapid decapitation. Control subjects that did not experience social interaction also had tissue collected at these two time points. Coronal sections (approximately 1 – 2 mm) containing the NAc, caudate putamen (CPu) and medial prefrontal cortex (PFC) were taken with the aid of a brain matrix and bilateral tissue punches (1 mm diameter) were immediately collected from each area. NAc punches included the anterior commissure to bias the punches toward the NAc core, where we have previously found increases in dendritic spine density following aggressive experience (Staffend and Meisel, 2012). Dorsal medial CPu punches were taken to evaluate the regional specificity of the biochemical changes within the striatum following aggressive experience. Punches were flash-frozen and stored in an −80°C freezer.
Tissue Preparation
To prepare tissue for Western blot analysis, flash frozen tissue was first transferred into 1.5 mL protein lysate tubes with metallic beads. 100 μl of ice-cold RIPA buffer (250 mM NaCl and 50 mM Tris pH 8) and 1 μl of HALT phosphatase and 1 μl of HALT protease inhibitors (Thermo Fisher, Waltham, MA, United States) were added to each lysate tube. Tissue samples were then homogenized in a bullet blender (Next Advance, Inc., Troy, NY, United States) for 6 min. 21 μl of detergent master mix (per 16 ml: 1 ml 100% triton, 10 ml of 10% sodium dodecyl sulfate (SDS) and 5 ml of 10% sodium deoxycholate) was then added to tubes with homogenized tissue. Samples were then rotated for 45 min with a sample mixer at 4°C. Samples were centrifuged at 4°C for 20 min (12,000 rpm). Finally, the supernatant was aspirated (∼80–100 μl) and placed in a fresh tube. Approximately 6 μl per sample was used for protein analysis and the rest was stored in a −80°C freezer until used for Western blots. Protein was quantified using Bio-Rad protein DC assay (Bio-Rad Laboratories, Berkeley, CA, United States). Samples were run in triplicate at 20, 10 and 6.7 dilution factors (1.0–3.0 μl of sample and 19.0–17.0 μl of 1% SDS). Standards were run in duplicate in eight serial dilutions of 1.5–0.05 mg/ml (Bio-Rad Laboratories).
Western Blot
For each subject twenty or thirty μg of total protein were heated for 10 min on a 90°C hot plate. Samples were then loaded into a 12–15% polyacrylamide gradient gel in 1% SDS running buffer (Mini-PROTEAN TGX Precast Mini Gel, Bio-Rad Laboratories, 110 mV for ∼90 min). Proteins from the gel were then transferred to a nitrocellulose membrane (0.45 μm pore size, Bio-Rad Laboratories, Cat. # 1620115, 100 mV for 55 min). After transfer, membranes were then blocked in Odyssey Blocking Buffer (Li-Cor Biosiences, Lincoln, NE, United States) for 1 h. Membranes were then incubated overnight in primary antibodies against mouse anti-PSD-95 (1:1000; Invitrogen, Rockford, IL, United States, REF MA 1-045), rabbit anti-Caskin I (1:2000; Synaptic Systems, Göttingen, Germany, Cat. # 185 002), rabbit anti-GluA1 (1:2000; Abcam, Cambridge, MA, United States, ab31232), rabbit anti-GluA2 (1:1000; Cell Signaling, Danvers, MA, United States, Ref: 04/2019 5306S), rabbit anti-GluA4 (1:1000; Abcam, Cambridge, MA, United States, ab109431), rabbit anti-NR2A (1:500; Abcam, Cambridge, MA, United States, ab124913), rabbit anti-NR2B (1:1000; Abcam, Cambridge, MA, United States, ab65783), rabbit anti-mGluR1a (1:1000; Millipore, Billerica, MA, United States, 07-617), rabbit anti-mGluR5 (1:2000; Millipore, Billerica, MA, United States, AB5675) and mouse anti-GAPDH (1:10,000; Abcam, Cambridge, MA, United States, ab8245). The following day, the membranes were washed in TBST for six 3-min washes. Membranes were then incubated in a Li-Cor incubation box for 1 h in the appropriate secondary antibodies (goat anti-rabbit 680RD or 800CW IgG and goat anti-mouse 800CW IgG, 1:20,000, Li-Cor Biosciences, Lincoln, NE, United States) and then repeat washed in TBST six more times for 3 min each. Finally, membranes were imaged and analyzed using the Odyssey imaging system (Li-Cor Biosciences), as previous described (Been et al., 2016). Within each lane/subject, protein of interest was normalized to GAPDH. Within each gel, these relative expression values were then normalized to the average expression of subjects that did not experience social interaction (no social control) to reveal a fold change. Multiple proteins were assessed on the same gel: PSD-95 and mGluR5 (monomer and dimer), GluA1 and NR2A, GluA2 and NR2B, GluA4 and mGluR1a (monomer and dimer), and thus were normalized to the same GAPDH band (Supplementary Figure 2).
Data Analysis
For behavioral analyses, mixed repeated-measures (RM) ANOVAs with Tukey’s post hoc tests were used to detect significant differences in aggressive behaviors between groups (tissue collected at 24 h versus 1 week after social experience) and across testing days (days 1 through 5), p < 0.05. For protein analyses, significant differences between groups were detected using one-way ANOVAs with LSD post hoc tests, p < 0.05 (Statistics are in Supplementary Tables 1–3). A Kruskal-Wallis test was performed to detect if aggressive experience resulted in a shift in the quality and strength of relationships between the different proteins in the NAc (categorized 1 through 7; strong ± 1.00 > r > 0.75, moderate 0.74 > r > 0.50, weak 0.49 > r > 0.25, none 0.24 > r), p < 0.05. Correlation matrices for each treatment examined the positive or negative relationships between each protein, p < 0.05 or p < 0.01. For correlation analyses fold change was normalized to 0. Data were analyzed using GraphPad Prism 8.3.0 for Windows. Two subjects were removed, as they did not display aggressive behaviors toward male intruders. Statistical outliers were removed using the ROUT method (maximum False Discovery Rate, Q = 1%). All data were examined to determine if the assumptions of parametric statistical tests were met, [normality (Bartlett’s test) and equal variance (Brown-Forsythe test)]. If assumptions were not met, Kruskal-Wallis (non-parametric) test was performed (along with Dunn’s post hoc tests). All tests were two-tailed, and results considered statistically significant if p < 0.05 unless specified otherwise. All data are presented as mean ± standard error of the mean.
Results
The Rewarding Properties of Five Consecutive Days of Aggressive Behavior
Female Syrian hamsters displayed a steady decrease in the latency to initiate an attack from the first to the fifth social behavior test session (mixed RM-ANOVA: F = 4.403, p = 0.005, df = 4, 92) (Figure 1A). Specifically, the latency to the first attack was shorter on the fourth (Tukey’s: d = 0.630, p = 0.038, df = 23) and fifth (Tukey’s: d = 0.664, p = 0.026, df = 23) days compared to the first testing day. However, there were no differences in the total number of attacks over the five test days (mixed RM-ANOVA: F = 1.554, p > 0.05, df = 4, 92) (Figure 1B). Following the five days of social interaction testing, brain tissue was collected for protein analysis either 24 h or 1 week later. There were no differences in either the latency to attack (mixed RM-ANOVA: F = 0.6688, p > 0.05, df = 1, 22) or the total number of attacks (mixed RM-ANOVA: F = 0.7522, p > 0.05, df = 1, 22) between subjects in which tissue was collected 24 h versus 1 week after behavior testing (Supplementary Figure 1).
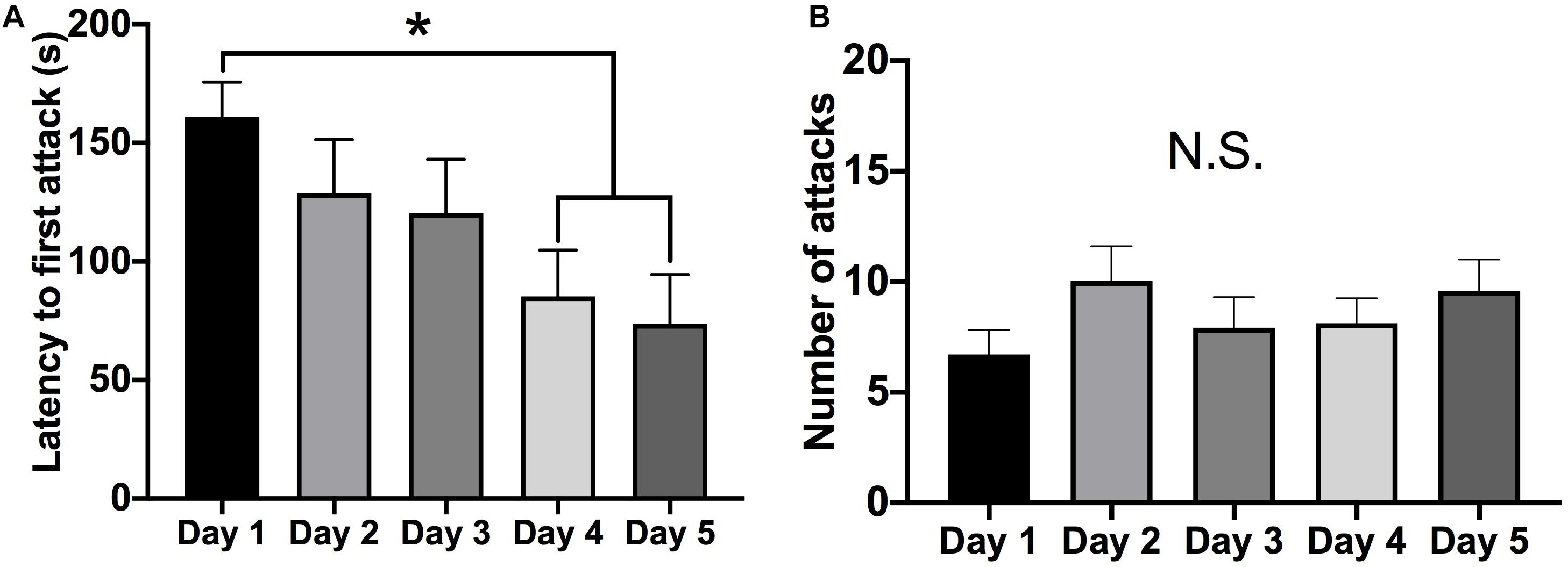
Figure 1. Aggressive behavior over five consecutive tests (5-min each). (A) There was a decrease in the latency to attack on the four and fifth test day compared to the first test day (p < 0.05). (B) There was no change in the average number of attacks over the five social interaction test days. (Days 1–5 n = 24). (*Indicates significant difference between groups, p < 0.05).
Effect of Aggressive Experience on Markers of Postsynaptic Morphology in the NAc
Representative lanes for Western blot analysis can be found in Supplementary Figures 2A–E. Western blot analysis revealed that aggressive experience had a main effect on PSD-95 protein expression in the NAc (Kruskal-Wallis: H = 8.101, p = 0.017, df = 2) (Figure 2A). An increase in PSD-95 in the NAc 24 h after aggressive experience significantly decreased to that of control females 1 week later (Dunn’s test: d = 1.165, p = 0.013, df = 26). Finally, Caskin I protein expression was examined to assess if these changes in postsynaptic structural density may be linked to changes in specifically mushroom-like spines (Bencsik et al., 2019). There was no effect of aggressive experience on Caskin I expression in the NAc (one-way ANOVA: F = 0.668, p > 0.05, df = 2, 25) (Figure 2B).
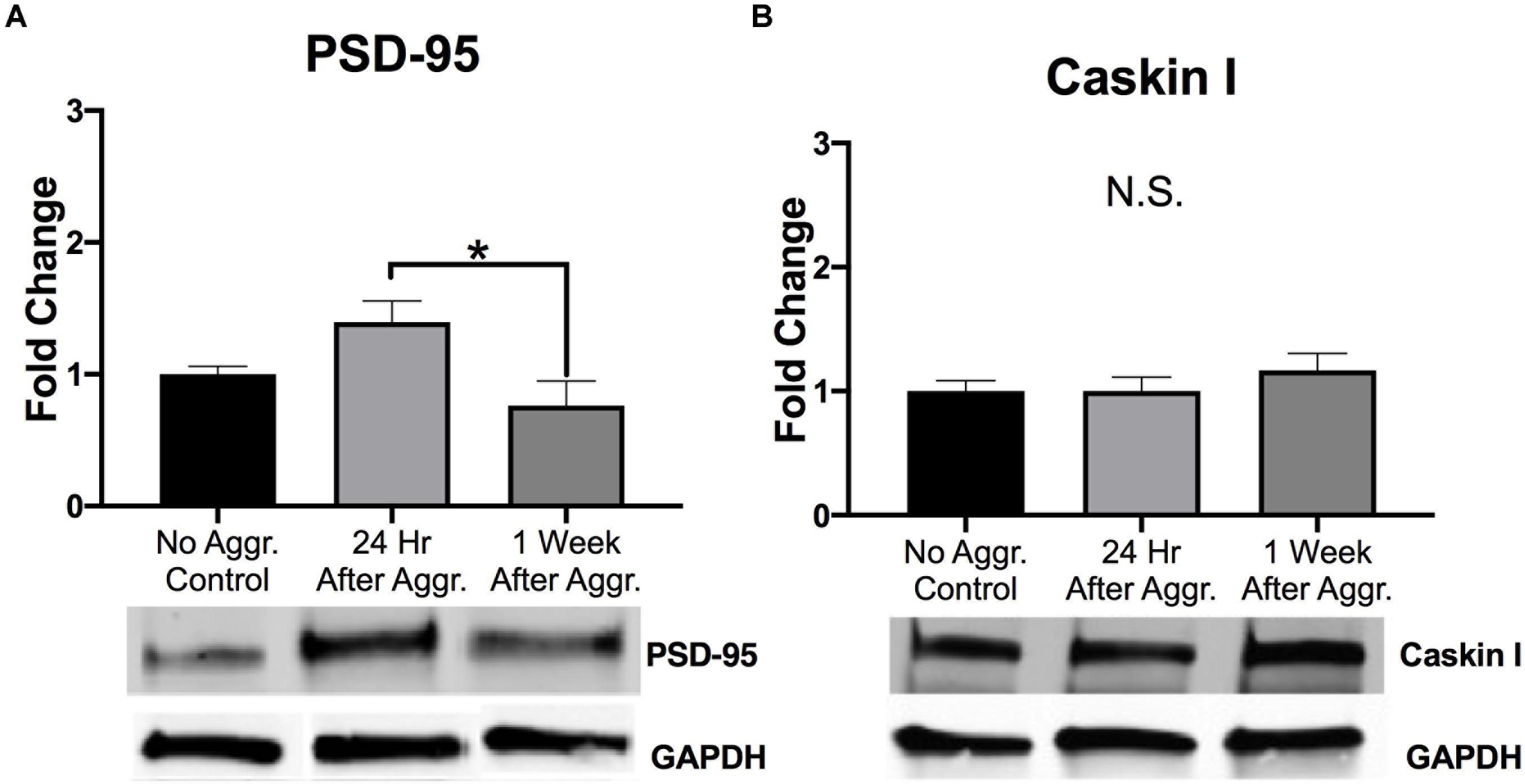
Figure 2. The effect of aggressive behavior on the expression of markers of postsynaptic plasticity in the NAc. (A) For subjects that had aggressive experience, there was an increase in PSD-95 expression in the NAc 24 h, compared to expression levels 1 week following aggression (p < 0.05). (B) Aggressive experience had no overall effect on Caskin I expression in the NAc. (Control n = 8–9, 24 h n = 11, 1 Week n = 9). (*Indicates significant difference between groups, p < 0.05).
Effect of Aggressive Experience on AMPARs and NMDARs in the NAc
To examine if aggressive experience results in changes in AMPAR expression in the NAc, we examined expression levels of different AMPAR (GluA1, GluA2, and GluA4) subunits. There was a trend for GluA1 protein expression to be increased 24 h following aggressive experience (Dunn’s test: d = 1.042, p = 0.058, df = 25) compared to no social controls (Kruskal-Wallis: H = 5.572, p = 0.062, df = 2) (Figure 3A). This increase in GluA1 24 h following aggressive experience was attenuated 1 week later (Dunn’s test: d = 0.806, p = 0.033, df = 25). There was no main effect of aggressive experience on GluA2 expression in the NAc (one-way ANOVA: F = 1.308, p > 0.05, df = 2, 24) (Figure 3B). Similarly, there was no main effect of aggressive experience on GluA4 expression in the NAc (Kruskal-Wallis: H = 2.138, p > 0.05, df = 2), although the original parametric test (the data was not normally distributed) did detect a significant increase in GluA4 expression 1 week following aggressive experience (one-way ANOVA: F = 3.560, p = 0.043, df = 2, 26) compared to just 24 h following aggressive experience (Fisher’s LSD: d = 1.062, p = 0.032, df = 26) and compared to no social control subjects (Fisher’s LSD: d = 0.926, p = 0.025, df = 26) (Figure 3C). To examine if aggressive experience results in changes in NMDAR expression in the NAc, we examined expression levels of the NR2A and NR2B subunits. Aggressive experience had no effect on either NR2A (one-way ANOVA: F = 1.795, p > 0.05, df = 2, 27) or NR2B (one-way ANOVA: F = 0.709, p > 0.05, df = 2, 26) expression in the NAc (Figures 4A,B). In sum, there was an increase in GluA1 expression in the NAc 24 h versus 1 week following the final aggression experience.
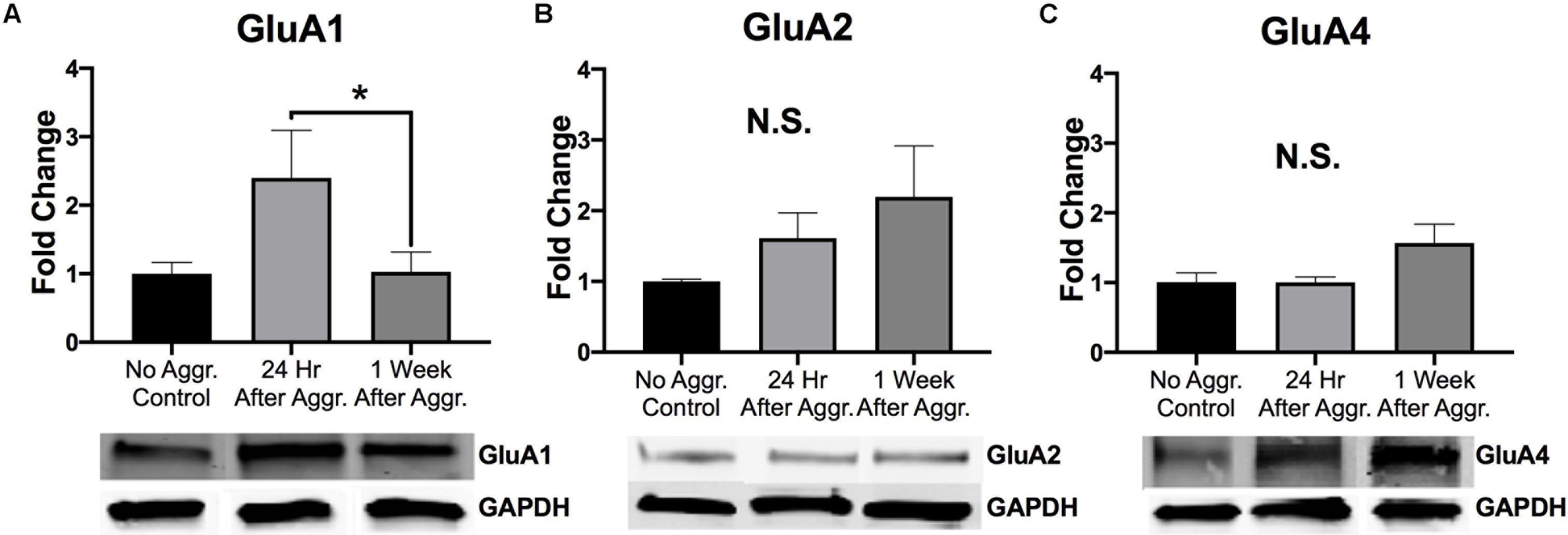
Figure 3. The effect of aggression on AMPAR subunit expression in the NAc. (A) Aggression resulted in an increase in GluA1 expression in the NAc 24 h following aggressive experience compared to 1 week following aggressive experience (p < 0.05). (B) There was no effect of aggressive experience on GluA2 expression. (C) There was no effect of aggressive experience on GluA4 expression. (Control n = 7–9, 24 h n = 10–11, 1 Week n = 9). (*Indicates significant difference between groups, p < 0.05).
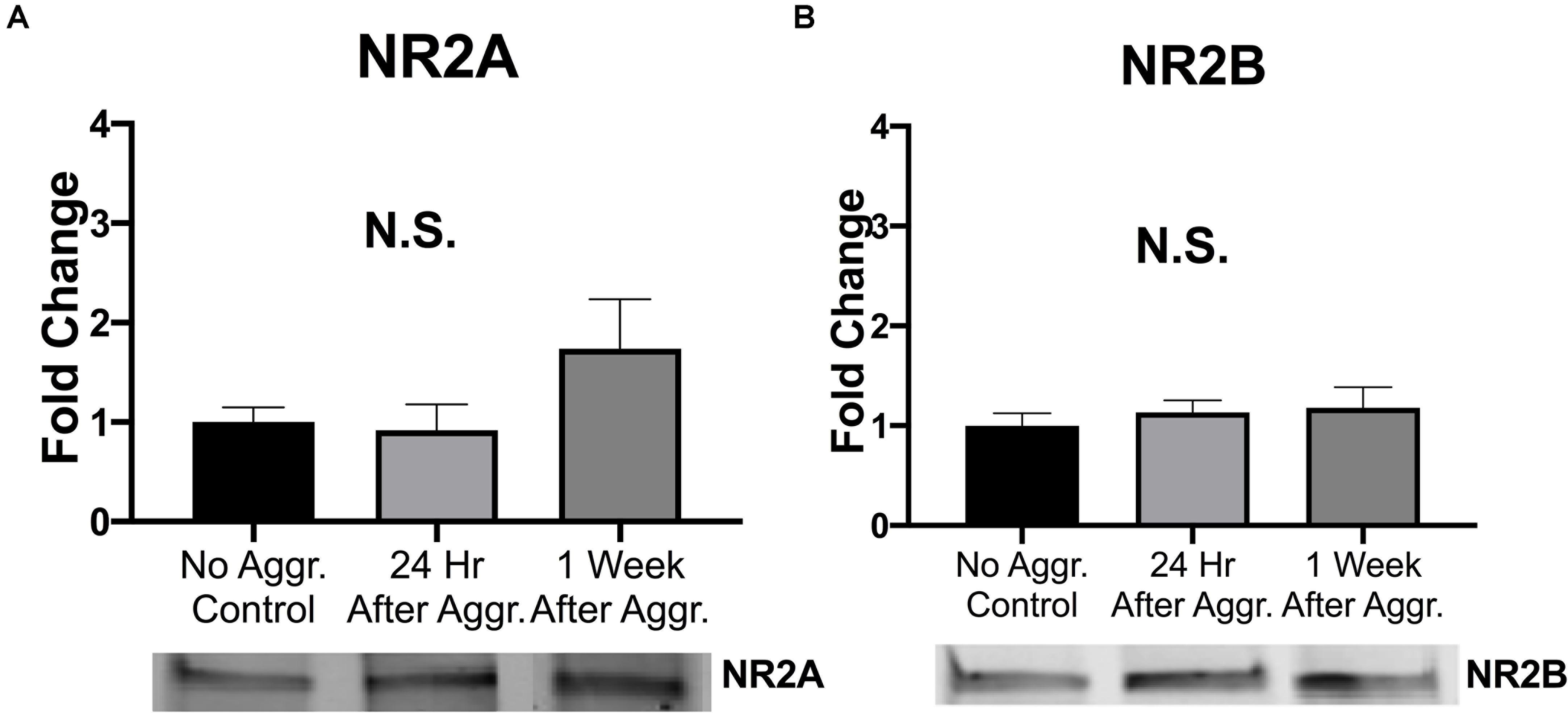
Figure 4. Aggression did not alter NMDAR subunit expression in the NAc. (A) Aggressive experience had no effect on overall NR2A expression in the NAc. (B) Aggressive experience had no effect on overall NR2B expression in the NAc. (Control n = 9, 24 h n = 11, 1 Week n = 9–10). (p > 0.05).
Effect of Aggressive Experience on mGluRs in the NAc
To investigate if aggressive experience affects group I metabotropic glutamate receptor expression we examined expression levels of mGluR5 and mGluR1a, respectively. Aggressive experience resulted in an increase in the dimer (activated) form of mGluR5 in the NAc 24 h (Fisher’s LSD: d = 1.587, p = 0.002, df = 27) but not 1 week (p > 0.05) after aggressive experience as compared to control subjects that did not experience aggression (one-way ANOVA: F = 5.638, p = 0.009, df = 2, 27) (Figure 5B). Aggressive experience resulted in an increase in the dimer (activated) form of mGluR1a 1 week (Dunn’s test: d = 1.107, p = 0.007, df = 24), but not 24 h (p > 0.05) after aggressive experience compared to no social controls (Kruskal-Wallis: H = 7.305, p = 0.026, df = 2) (Figure 5D). For both of these receptors, these effects were observed only for the dimer form of the receptor. No changes were detected for the monomer forms of mGluR5 (one-way ANOVA: F = 1.204, p > 0.05, df = 2, 27) and mGluR1a (one-way ANOVA: F = 0.179, p > 0.05, df = 2, 26) (Figures 5A,C).
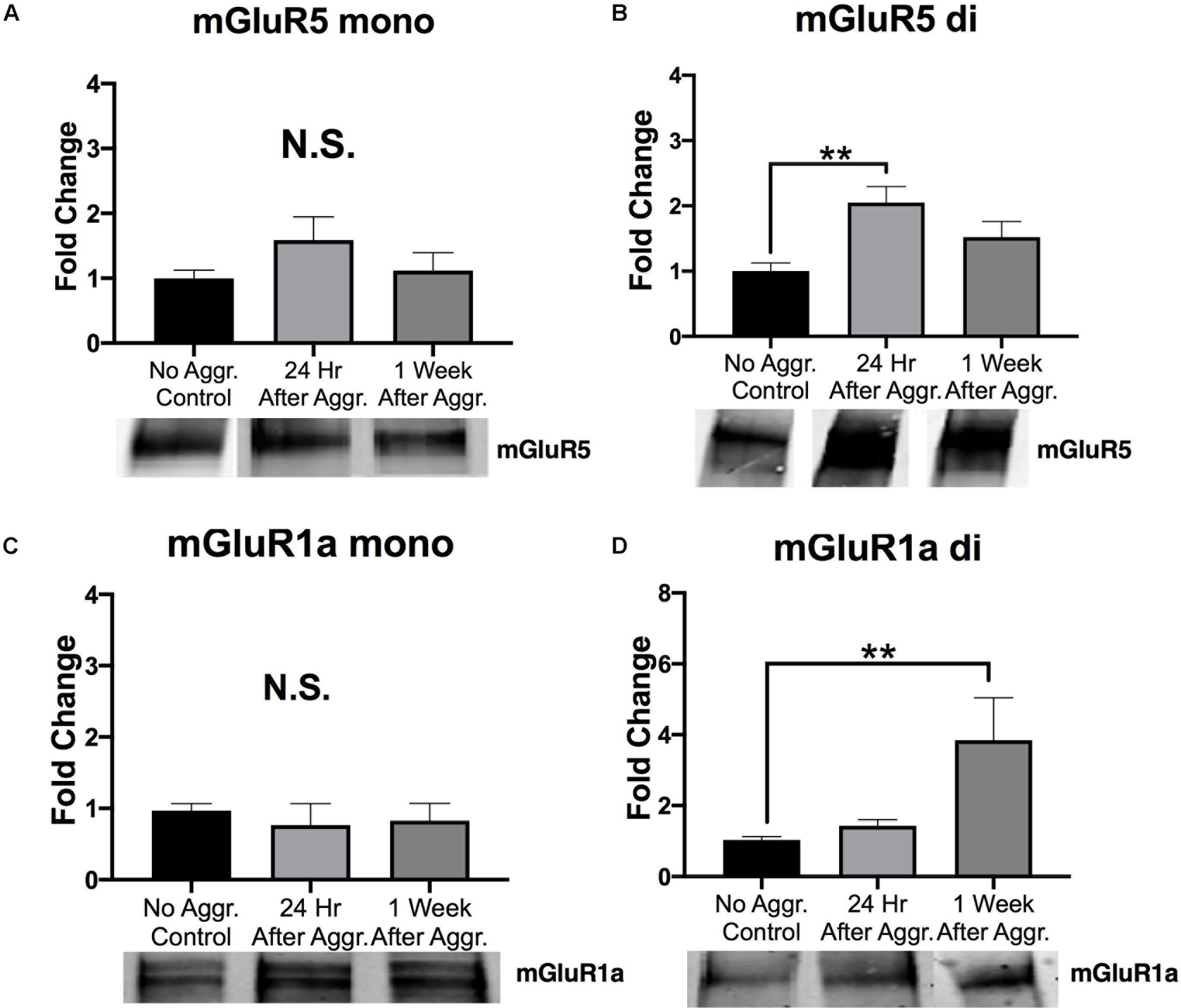
Figure 5. The effect of aggression on group I metabotropic glutamate receptor expression in the NAc. (A) There was no effect of aggressive experience on the expression of the monomer form (mono) of mGluR5 in the NAc. (B) There was an increase in the dimer form (di) of mGluR5 in the NAc 24 h following aggressive experience compared to subjects that did not have social experience (p < 0.05). (C) There was no effect of aggressive experience on the expression of the monomer form of mGluR1a. (D) There was an increase in the dimer form of mGluR1a in the NAc 1 week following aggressive experience compared to subjects that did not have social experience (p < 0.05). (Control n = 8-9, 24 h n = 9–12, 1 Week n = 9–10). (*Indicates significant difference between groups, p < 0.05; **p < 0.01).
These changes in expression were selective to the NAc, as aggressive experience did not affect the overall expression levels of any of the proteins (PSD-95, GluA1, GluA2, GluA4, NR2A, NR2B, mGluR5, and mGluR1a) in tissue collected from the CPu or the PFC (one-way ANOVA: F = 0.037–2.099, p > 0.05, df = 2, 25–27) (Supplementary Figures 3, 4). Summary of NAc statistics can be found in Supplementary Table 1. Summary of CPu and PFC statistics can be found in Supplementary Tables 2, 3. In sum, aggressive experience results in an increase in the expression of PSD-95, GluA1 and the dimer forms of group I mGluRs in the NAc.
Effect of Aggressive Experience on the Relationship and Strength of Association Between Postsynaptic Proteins and Glutamate Receptors in the NAc
To investigate if these increases in PSD-95, GluA1 and mGluR5 dimer are correlated with each other, supporting a common mechanism of action, and if aggressive experience results in changes in the strength of the relationship between postsynaptic structural proteins and glutamate receptors, correlation matrices were calculated for each treatment condition in the NAc (Figures 6A–C). Aggressive experience resulted in a shift in the quality of the relationships between the different postsynaptic proteins and glutamate receptors analyzed (positive relationship: strong 1.00 > r > 0.75, moderate 0.74 > r > 0.50, weak 0.49 > r > 0.25; negative relationship: strong −1.00 > r > −0.75, moderate −0.74 > r > −0.50, weak −0.49 > r > −0.25; no relationship 0.24 > r > −0.24) (Kruskal-Wallis: H = 31.38, p < 0.001, df = 2). There was an increase in the strength of association between the different postsynaptic proteins and glutamate receptors analyzed 1 week following aggressive experience compared to no social controls (Dunn’s test: d = 0.747, p < 0.001, df = 162) and compared to 24 h following aggressive experience (Dunn’s test: d = 1.364, p < 0.001, df = 162) (Figure 7).
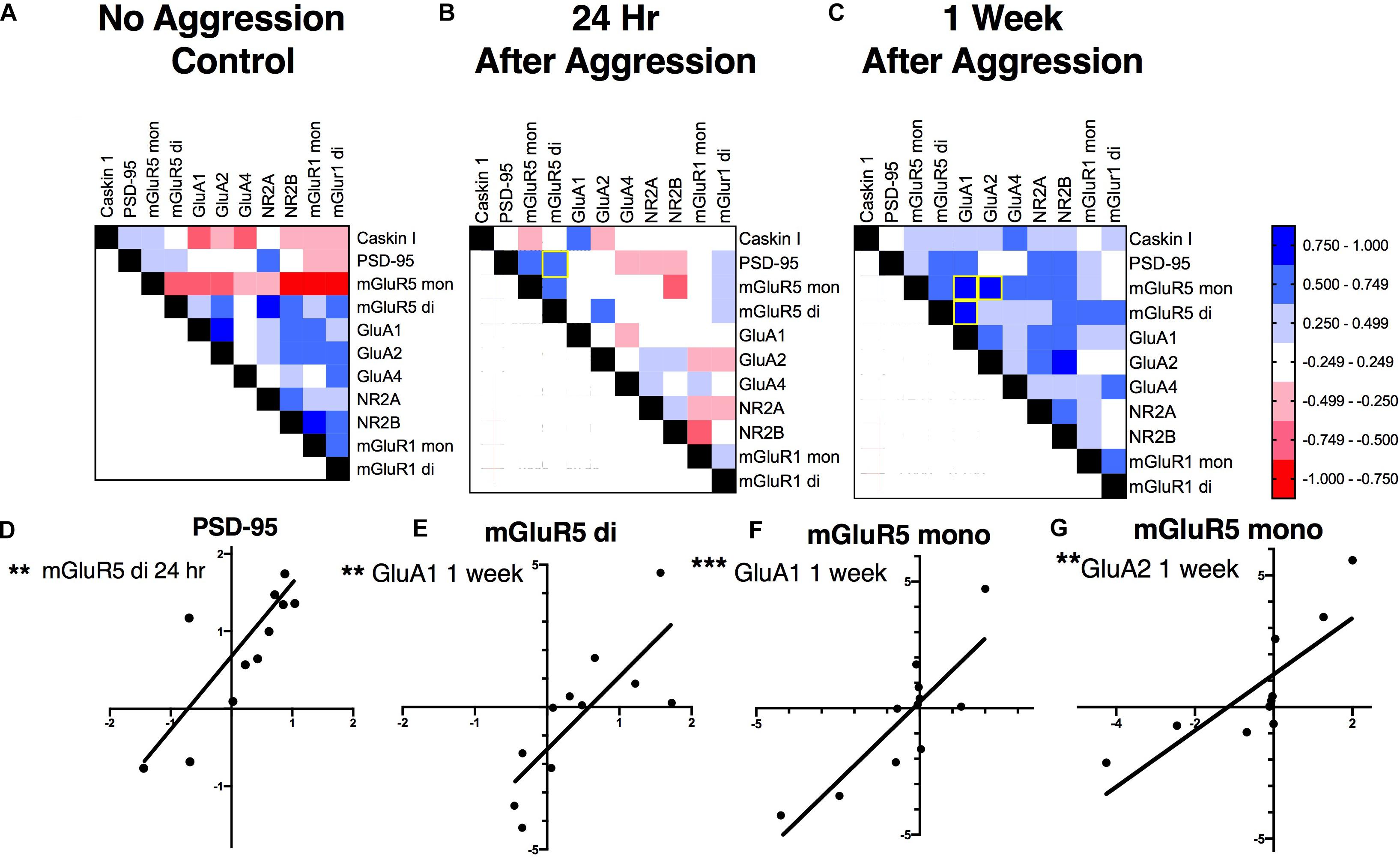
Figure 6. The effect of aggressive experience on the relationships between postsynaptic proteins and glutamate receptors in the NAc: correlation matrices. (A–C) One week following aggressive experience there is an increase in the strength of positive associations between the postsynaptic proteins and glutamate receptors analyzed (p < 0.001) (also see Figure 7). (A) Relationships between postsynaptic proteins and glutamate receptors in the NAc in subjects that did not have social experience. (B) Relationships in the NAc 24 h following aggressive experience. (C) Relationships in the NAc 1 week following aggressive experience. [Positive relationship: strong 1.00 > r > 0.75, moderate 0.74 > r > 0.50, weak 0.49 > r > 0.25 (blue); negative relationship: strong –1.00 > r > –0.75, moderate –0.74 > r > –0.50, weak –0.49 > r > –0.25 (red); no relationship 0.24 > r > –0.24 (white)]. (D–G) Representative correlations: (D) the expression of the dimer form of mGluR5 was positively correlated with PSD-95 expression 24 h following aggressive experience (p < 0.01), but not 1 week following aggressive experience, nor in subjects that did not have social experience (p > 0.05). (E) The mGluR5 dimer was positively correlated with GluA1 1 week following aggressive experience (p < 0.01). (F) The mGluR5 monomer was positively correlated with GluA1 and (G) GluA2 1 week following aggressive experience (p < 0.01). (**Indicates significant difference between groups, p < 0.01; ***p < 0.001).
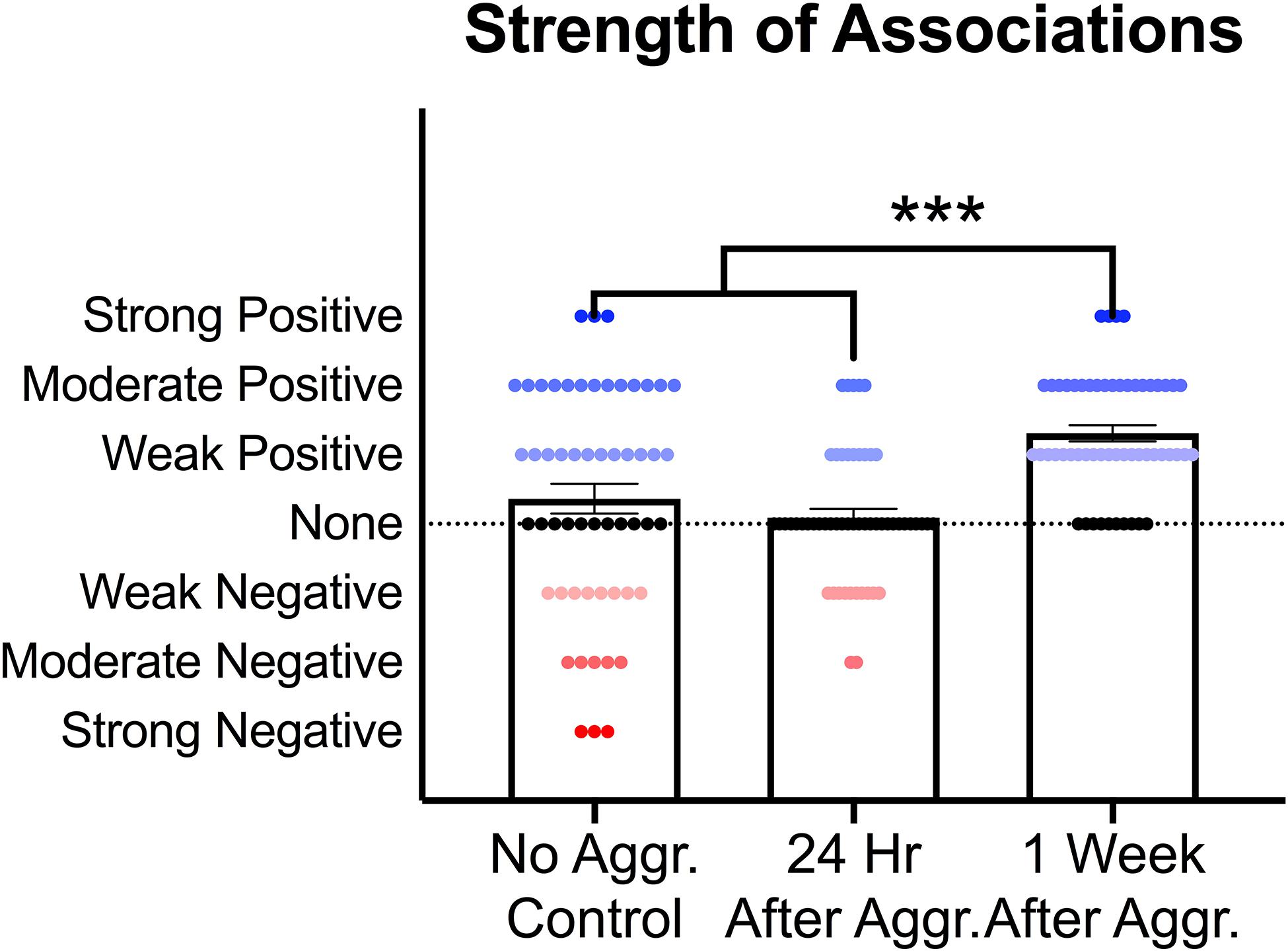
Figure 7. Effect of aggressive experience on the strength of relationships between postsynaptic proteins and glutamate receptors in the NAc. Aggressive experience resulted in an increase in the strength of positive associations between the proteins analyzed at 1 week compared to the strength of relationships 24 h following aggressive experience and compared to no social control subjects (*** = p < 0.001).
More specifically, at baseline (no aggressive experience), the mGluR5 dimer was positively correlated with NR2A (Pearson correlation coefficient: r = 0.925, p = 0.004, df = 7), the mGluR5 monomer was negatively correlated with NR2B (r = −0.869, p = 0.002, df = 7) and the mGluR1a monomer (r = −0.812, p = 0.008, df = 7) and dimer (r = −0.777, p = 0.014, df = 7), and the mGluR1a monomer was positively correlated with NR2B (r = 0.925, p < 0.001, df = 7) expression. Finally, the GluA1 subunit was positively correlated with GluA2 expression [r = 0.907, p = 0.007, df = 7] (Figure 8B). Twenty-four hours following aggressive experience: the mGluR5 dimer was positively correlated with PSD-95 (r = 0.748, p = 0.005, df = 10, Figure 6D) and the mGluR5 monomer (r = 0.648, p = 0.023, df = 10), and PSD-95 was positively correlated with the mGluR5 monomer (r = 0.650, p = 0.022, df = 10). Finally, Caskin I was positively correlated with GluA1 (r = 0.706, p = 0.015, df = 9) (Figure 8C). One week following aggressive experience: the mGluR5 monomer was positively correlated with the mGluR5 dimer (r = 0.639, p = 0.034, df = 9), GluA1 (r = 0.849, p = 0.001, df = 9, Figure 6F), GluA2 (r = 0.806, p = 0.005, df = 8, Figure 6G), NR2A (r = 0.604, p = 0.049, df = 9) and NR2B (r = 0.727, p = 0.017, df = 8), the mGluR5 dimer was positively correlated with GluA1 (r = 0.787, p = 0.004, df = 9, Figure 6E), GluA1 was positively correlated with GluA2 (r = 0.650, p = 0.042, df = 8) and NR2A (r = 0.712, p = 0.014, df = 9), and the GluA2 subunit was positively correlated with NR2B (r = 0.814, p = 0.004, df = 8). Finally, the NR2A subunit was positively correlated with NR2B (r = 0.657, p = 0.039, df = 8) and PSD-95 (r = 0.602, p = 0.050, df = 9), and the mGluR1a dimer was positively correlated with its monomer form (r = 0.726, p = 0.018, df = 8) (Figure 8D). In conclusion, aggressive experience results in a positive synchronization in the amount of expression between almost all the different glutamate receptors analyzed, and in particular, the mGluR5 monomer was central.
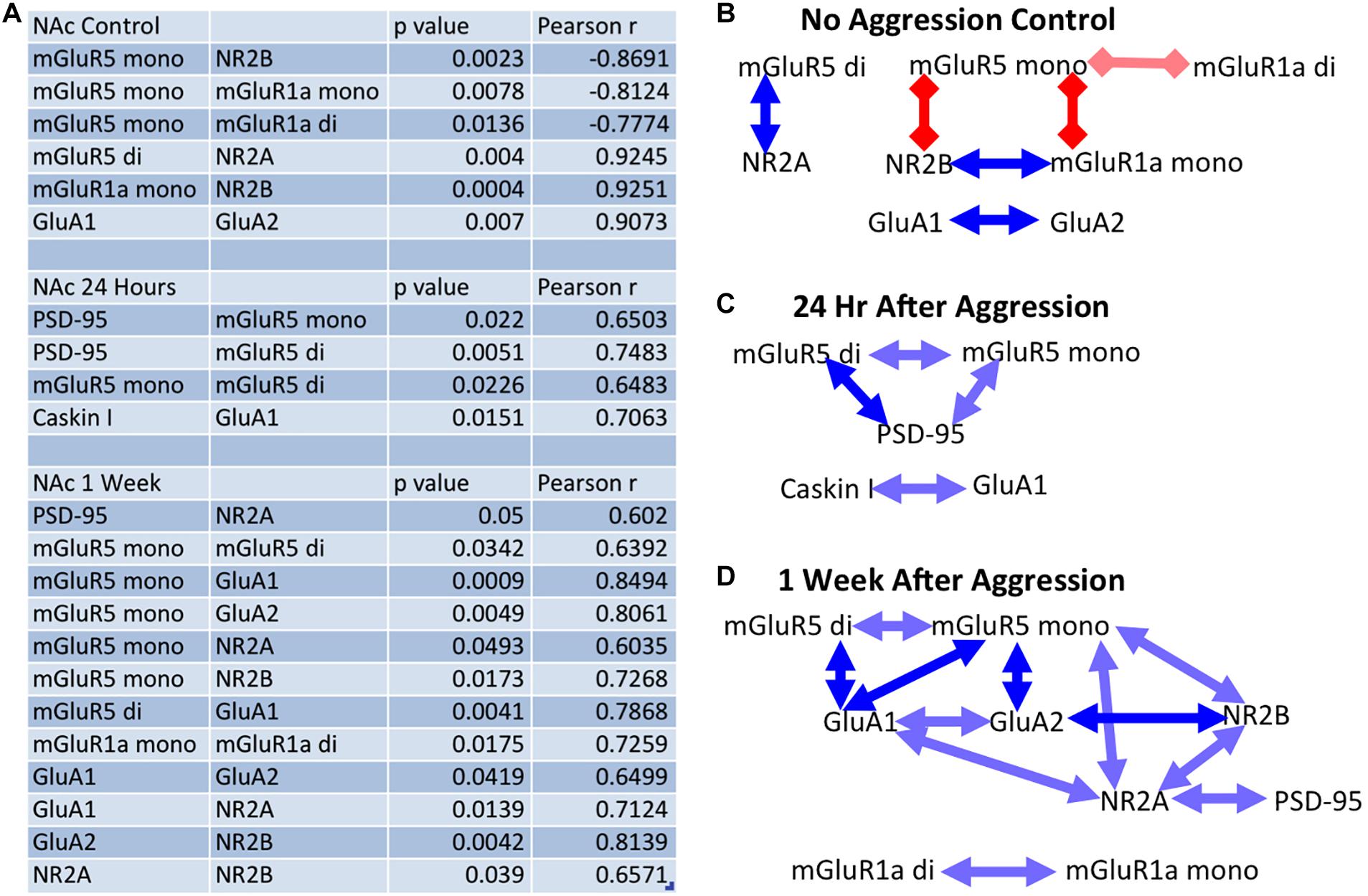
Figure 8. The effect of aggressive experience on the relationships between postsynaptic proteins and glutamate receptors in the NAc: significance table. (A) Table of significant correlations between proteins in the NAc in subjects that either did not encounter a social stimulus or subjects that had aggressive experience and had tissue collected either 24 h or 1 week later. (B) Significant relationships between proteins in the NAc in subjects that did not encounter a social stimulus. (Red square lines = negative/inverse relationship; blue arrow lines = positive/direct relationship; dark = p < 0.01, light = 0.01 < p < 0.05). At baseline: the mGluR5 dimer is positively correlated with NR2A, the mGluR5 monomer is negatively correlated with NR2B and the mGluR1a monomer and dimer, and mGluR1a monomer is positively correlated with NR2B expression. Finally, GluA1 is positively correlated with GluA2 expression. (C) Twenty-four hours following aggressive experience: the mGluR5 dimer is positively correlated with PSD-95 and the mGluR5 monomer, and PSD-95 is positively correlated with the mGluR5 monomer. Caskin I is positively correlated with GluA1. (D) One week following aggressive experience: the mGluR5 monomer is positively correlated with the mGluR5 dimer, GluA1, GluA2, NR2B, and NR2A, the mGluR5 dimer is positively correlated with GluA1, GluA1 is positively correlated with GluA2 and NR2A, GluA2 is positively correlated with NR2B, NR2A is positively correlated with NR2B and PSD-95, and the mGluR1a dimer is positively correlated with its monomer form.
Discussion
The overall goal of this study was to begin to characterize responses of components of glutamate neurotransmission following aggressive experience in a female model. We previously investigated the functional necessity of mGluR5 activity on parameters associated with repeated aggressive experience in females (Been et al., 2016). Those results made us take a step back to provide an extensive analysis of protein changes in glutamate receptors and postsynaptic proteins following aggressive experience.
The Rewarding Properties of Aggressive Experience in Female Syrian Hamsters
There is an accumulating body of literature investigating the neural mechanisms underlying the rewarding properties of aggressive behavior (Fish et al., 2005; Couppis and Kennedy, 2008; Gray et al., 2015; Golden et al., 2017, 2019a; Aleyasin et al., 2018; Panasiuk et al., 2019). However, these studies have been both primarily focused on the role of dopaminergic neurotransmission and heavily biased toward only studies of males. Thus, the overarching goal of this study was to bridge these gaps by (1), investigating the role of glutamatergic transmission and synaptic plasticity in aggression reward processing, and (2), focusing on the neural mechanisms mediating aggression in females. In sum, in this study, female aggressive experience resulted in dynamic changes in the expression of postsynaptic proteins including glutamate receptors in the NAc.
We first wanted to provide evidence that aggressive experience can affect parameters of future encounters in females. Female Syrian hamsters displayed a steady decrease in the latency to initiate an offensive attack against an intruding male over the five consecutive days of testing. In contrast, there was no change in the overall number of attacks. This supports the hypothesis that aggressive behavior comprises a feed-forward process involving reward processing, increasing behavioral efficiency (Thorndike, 1905; Skinner, 1938; Meisel and Joppa, 1994). It is theorized that increases in the strength of synaptic transmission underlie the rewarding consequences of stimuli (Kasai et al., 2003; Malenka, 2003; Ebner et al., 2018; Turner et al., 2018a). Thus, this study was the first to investigate if aggression might result in increases in molecular markers of postsynaptic plasticity and excitatory synaptic transmission and increases in the strength of the associations between these postsynaptic proteins and glutamate receptors in the NAc (Pitchers et al., 2012; Bredewold et al., 2015; Garcia-Pardo et al., 2019; Zoicas and Kornhuber, 2019).
Overall Changes in Molecular Markers of Excitatory Transmission in the NAc
In line with previous literature, aggressive experience resulted in a transient increase in PSD-95 expression in the NAc 24 h following aggressive experience (Staffend and Meisel, 2012; Been et al., 2016). This effect was attenuated 1 week later. Thus, aggressive behavior is likely resulting in an increase in postsynaptic structural density in the NAc. To assess if these postsynaptic changes are specifically related to changes in mature mushroom-like spines, we investigated Caskin I protein expression. Interestingly, we did not detect any changes in Caskin I protein expression. Thus, the postsynaptic structural changes may have been independent of changes specifically in mushroom-like spines or our experimental procedure may not have been selective or sensitive enough to detect more subtle changes in expression (Bencsik et al., 2019). We next investigated if these changes in markers of postsynaptic structural density correlate with changes in excitatory synaptic transmission in the NAc.
Similar to PSD-95, there was an increase in the expression of the GluA1 subunit in the NAc 24 h following aggressive experience. The AMPA subunit GluA1 is highly associated with and implicated in the formation of mature mushroom-like spines and excitatory synaptic plasticity (Diering and Huganir, 2018). Interestingly, although GluA2 subunit membrane trafficking is implicated in calcium impermeability and spine stabilization (Henley and Wilkinson, 2013; Briand et al., 2016; Diering and Huganir, 2018), no significant differences in GluA2 were detected. Suggesting that the increases in AMPAR are calcium permeable, as has been seen with cocaine experience (Wolf and Tseng, 2012). There was a trend for an increase in GluA2 expression 1 week following aggression experience. Furthermore, although GluA4 has been primarily only implicated in neurodevelopment, we also observed a non-significant trend for increased expression in GluA4 1 week following aggressive experience, suggesting that it may play a role in the long-lasting behavioral effects of aggression (Huupponen et al., 2016; Luchkina et al., 2017; Atanasova et al., 2019).
Gating of AMPAR membrane trafficking and plasticity is synonymous with activation (Ca2 + influx) of NMDARs. Thus, we next investigated the effects of aggressive experience on the expression of the NR2A and NR2B NMDAR subunits. No overall changes in NR2A and NR2B expression were detected in the NAc. However, the NMDA receptor can still play a critical role in AMPAR trafficking, synaptic plasticity and long-term potentiation without actual changes in expression levels (i.e., second messenger effects) (Fox et al., 2006; Chang et al., 2015). AMPARs cluster in domains that surround a main NMDAR domain at the postsynaptic density (Goncalves et al., 2020). Further studies should investigate if aggressive experience results in changes in the activity, i.e., phosphorylation of NMDARs in the NAc, or if systemic treatment of an NMDAR antagonist blocks the molecular, morphological and behavioral effects of aggression in females. Similar to the GluA2 and GluA4 subunits, there was a trend for an increase in the expression of the NR2A subunit 1 week following aggression experience.
Activation, trafficking and translation of metabotropic glutamate receptors (a Gq-protein coupled receptor) have also been shown to play a critical role in the regulation of synaptic plasticity, AMPAR and NMDAR expression; and thus long-term potentiation and learning and memory (Menard and Quirion, 2012). There was a transient increase in the expression of the dimer form of mGluR5 24 h, but not 1 week, following aggressive experience. Interestingly, there was an increase in the dimer form of mGluR1a 1 week, but not 24 h, following aggressive experience. There were no significant changes in the overall expression levels of the monomer forms of mGluR5 and mGluR1a in the NAc. Collectively, aggressive experience likely results in an increase in postsynaptic structural density (potentially mushroom-like spines: evidence provided by an increase in GluA1), and excitatory synaptic transmission in the NAc (Staffend and Meisel, 2012; Meyer et al., 2014; Been et al., 2016; Diering and Huganir, 2018).
An important note, even though the mGluR5 and mGluR1a subunits may form heterodimers with each other (Pandya et al., 2016). There was an increase in mGluR5 dimer, but not mGluR1a dimer, 24 h following aggression, with an increase in the mGluR1a dimer 1 week following aggression, without any increase in mGluR5 dimer expression. These dissociations support the interpretation of homodimerization of the mGluRs. A recent study suggests that allosteric interactions and not heterodimerization may underlie the interactions between mGluR5 and mGluR1a (Kammermeier and McCullock, 2020).
All of the significant effects observed in the NAc were exclusive to the NAc, as there were no overall changes in any of the proteins analyzed in the CPu or PFC. However, it is not known if the increases in PSD-95, GluA1 and mGluR5 observed in the NAc are correlated with each other, supporting a common or linked mechanism of action; or not, supporting independent mechanisms of action. Thus, this study then investigated associations between these postsynaptic structural proteins and various glutamate receptors. Furthermore, this study was the first to investigate if aggressive experience results in changes in the strength of associations between postsynaptic proteins and glutamate receptors.
Synaptic Protein and Glutamate Receptor Relationships in the NAc, Effects of Aggressive Experience
Aggressive experience resulted in an increase in the strength of relationships between the different postsynaptic proteins and glutamate receptors analyzed in the NAc. At baseline, without aggressive experience, there was an inverse relationship between mGluR5 and mGluR1a. Furthermore, mGluR5 was positively associated with the NR2A subunit, while mGluR1a was positively associated with the NR2B subunit of the NMDA receptor. Collectively, these results further provide evidence for an inverse role between mGluR1a and mGluR5 (Gross et al., 2016; Turner et al., 2018b), a reciprocal role between NR2A and NR2B in long-term potentiation and long-term depression (Massey et al., 2004; Bartlett et al., 2007), and differences in NMDAR function and learning and memory (Bar-Shira et al., 2015). In other words, these results provide support for the theory that mGluR1a and mGluR5 activation have opposite roles in NAc synaptic morphology, neuronal transmission and behavior (Dewing et al., 2007; Staffend and Meisel, 2012; Staffend et al., 2014; Been et al., 2016; Gross et al., 2016; Pitchers et al., 2016) through differences in their association with specific NMDAR subunits (Yashiro and Seki, 2017; Zoicas and Kornhuber, 2019). In agreement with previous literature, there was also a positive correlation in the expression of GluA1 and GluA2 (Diering and Huganir, 2018).
Twenty-four hours following aggressive experience, when the expression of PSD-95 and mGluR5 dimer and GluA1 are all increased in the NAc, the expression of both the mGluR5 dimer and monomer are positively correlated with PSD-95 expression, and the GluA1 subunit is positively correlated with Caskin I expression. These correlations are not observed in females that did not have aggressive experience. Collectively, PSD-95, Caskin I, GluA1, and mGluR5 expression are all associated with increases in mature mushroom-like spines and increases in excitatory postsynaptic transmission (Malinow and Malenka, 2002; Muddashetty et al., 2007; Takahashi et al., 2009; Peterson et al., 2015; Been et al., 2016; Bencsik et al., 2019). Thus, the increases in PSD-95, GluA1, and mGluR5 observed in the NAc are correlated together, further supporting a common or linked mechanism of action (Goncalves et al., 2020).
Finally, 1 week following aggressive experience, there was a distinct increase in the strength of positive correlations between almost all of the proteins analyzed, a “positive synchronization.” In particular, the monomer form of mGluR5 was positively correlated with the expression of the dimer form of mGluR5, the GluA1, GluA2, NR2A, and NR2B subunits; and more generally, all these glutamate receptor subunits, along with PSD-95, become positively associated with each other. Previous studies have also implicated mGluR5 in the NAc in synaptic plasticity and social reward processing (Stoker et al., 2012; Been et al., 2016; Gross et al., 2018). However, this is the first study to directly correlate the monomer form of mGluR5 with markers of postsynaptic structure (PSD-95) and multiple ionotropic glutamate receptors (GluA1, GluA2, NR2A, and NR2B) following social experience. This is particularly interesting considering that the dimer form of mGluRs is widely regarded as the active, functional or membrane trafficked form of the receptor, while the monomer form is regarded as the inactive or cytoplasm trafficked form of the receptor (Robbins et al., 1999; Lum et al., 2016). However, the monomer mGluR5 may also be a more direct measure of the translation of mGluR5. Thus, the broad correlation of the mGluR5 monomer with the various ionotropic glutamate receptor subunits 1 week following aggressive experience may be an indication of a common epigenetic messenger linked to mGluR5 activation regulating the translation of AMPARs, NMDARs and mGluR5 (Menard and Quirion, 2012; Sweatt, 2016). While the overall increases in the mGluR5 dimer may be an indication of increased postsynaptic membrane trafficking of mGluR5. Collectively, these findings implicate the group I mGluR5 in the regulation of excitatory postsynaptic plasticity in the NAc for female aggressive experience.
This is the first study to our knowledge that has comprehensively assessed and demonstrated that aggressive experience in females results in dynamic changes in the expression of both ionotropic and metabotropic glutamate receptors, and that these changes are also tightly associated and coupled with changes in postsynaptic proteins. These changes in markers of postsynaptic plasticity and glutamate receptors likely represent increases in excitatory input that could mediate a heightened response in synaptic transmission, potentially encoding the rewarding effects of aggressive experience (increased efficiency in the display of aggressive behavior) (Weiss et al., 1992; Kasai et al., 2003; Kourrich et al., 2007; Stagkourakis et al., 2020). In this regard, aggressive experience in male mice results in a persistent increase in excitatory synaptic transmission of estrogen receptor expressing neurons in the ventrolateral ventromedial hypothalamus (Stagkourakis et al., 2020). Future studies should investigate if this structural plasticity and increases in glutamate receptor expression in the NAc translates to an increase in excitatory sensitivity and transmission of MSNs that may mediate a heightened response to future activation in females (Beloate and Coolen, 2017; Turner et al., 2018a; Venniro et al., 2018).
Translational Implications
For this study we were specifically focused on elucidating the molecular mechanisms mediating aggressive behavior in females, as previous studies of aggression are heavily biased toward males. There is evidence to suggest that there may be both conserved and divergent molecular mechanisms underlying aggression reward between males and females (Terranova et al., 2016; Borland et al., 2019a). There is also strong evidence that there are both conserved (Wallace et al., 2008; Hedges et al., 2009; Lobo et al., 2013; Pitchers et al., 2013; Aleyasin et al., 2018; Hamilton et al., 2018; Heshmati et al., 2018; Golden et al., 2019a) and divergent (Staffend and Meisel, 2012; Staffend et al., 2014) mechanisms underlying different social behaviors and animal species. We hypothesize that the recruitment of the glutamate system in the NAc for encoding social reward is conserved between males and females and across different species (Lei et al., 2020; Stagkourakis et al., 2020). Indeed, it has been previously postulated that glutamate terminals in the NAc regulate the coordinated activity of the oxytocin (via presynaptic OTRs on dorsal raphe 5-HT terminals) and serotonin system (via presynaptic 5-HT1b receptors on glutamate terminals) in gating social reward in male mice (Dolen et al., 2013; Walsh et al., 2018; Nardou et al., 2019). Furthermore, Stagkourakis et al. (2020) demonstrated that aggressive experience in male mice results in long-lasting increases in the excitatory synaptic transmission of ventrolateral ventromedial hypothalamus estrogen receptor expressing neurons. However, no studies to date have investigated if changes in glutamate receptor expression/plasticity in the NAc are critical for social reward processing.
Thus, our study is the first to provide direct evidence that plasticity in glutamate receptor protein expression may indeed be directly involved in social reward processing and synaptic plasticity in female Syrian hamsters. More specifically, we identify PSD-95, GluA1, and mGluR5 in the immediate, but perhaps not long-term effects of social reward in the NAc. The mGluR1a and perhaps GluA2, GluA4, and NR2A receptor subunit may underlie the long-term encoding of social reward. Furthermore, we provide evidence that there may be a coordinated recruitment of these different glutamate receptor components for encoding increases in excitatory synaptic transmission in MSNs in the NAc. Thus, future studies should investigate if the coordinated recruitment of these glutamate components in the NAc observed for female Syrian hamsters are also linked with the long-term potentiation mechanisms observed for social reward in male mice (Dolen et al., 2013; Stagkourakis et al., 2020). Once there is a sufficient representation of neurobiological studies of female aggression that issues of sex differences and commonalities in specific mechanisms should be explored.
Regarding comparisons with other natural reward, we have published a series of studies with sexual experience in female Syrian hamsters suggesting a casual link for synaptic plasticity and morphology in the NAc in the rewarding properties of sex behavior (Hedges et al., 2009; Been et al., 2013; Staffend et al., 2014; Moore et al., 2019). Coolen’s group has published similar findings for male sex behavior in rats (Pitchers et al., 2010, 2013). Thus, a collection of evidence, strongly suggest that the changes in the markers of synaptic structure and glutamate receptor expression in the NAc play a critical role in the rewarding effects of naturally occurring social behaviors in rodents.
Conclusion
In conclusion, this study supports the hypothesis that aggression results in an increase in excitatory postsynaptic plasticity and transmission in the NAc and that this excitatory transmission may underlie the rewarding properties of social interactions in females (Been et al., 2016). Furthermore, there is potential that these social processes interact with the same neural mechanisms and pathways involving addictive behavior and drugs of abuse (Kourrich et al., 2007; Venniro et al., 2018). Thus, social interaction, or more specifically assertiveness, may serve as an effective and drug free treatment strategy for drug addiction and psychiatric disorders like depression (Francis and Lobo, 2017; Nagy and Moore, 2017; Komori et al., 2019). Syrian hamsters are the ideal model for studies of the reinforcing properties of aggressive interactions because like primates, both males and females, naturally and reliably establish dominance relationships (Digby and Kahlenberg, 2002; Stockley and Bro-Jorgensen, 2011; Been et al., 2019). Furthermore, recent studies of social reward in Syrian hamsters have had significant translational application to studies in humans (Terranova et al., 2017; Borland et al., 2019b). As previous studies of the mechanisms regulating social reward have been heavily biased toward males, the overall goal of this study was to further our understanding of the molecular mechanisms regulating the rewarding properties of aggression in females. This is one of the first studies to implicate excitatory postsynaptic plasticity and potentially long-term potentiation in the NAc in the rewarding properties of aggressive behavior in females.
Disclosure
The content is solely the responsibility of the authors and does not necessarily represent the official views of the National Science Foundation and National Institutes of Health. The authors report no biomedical financial interests or potential conflicts of interest.
Data Availability Statement
The raw data supporting the conclusions of this article will be made available by the authors, without undue reservation.
Ethics Statement
The animal study was reviewed and approved by the University of Minnesota Institutional Care and Use Committee.
Author Contributions
JB, SS, and EK conducted all experiments. JB analyzed the data and wrote the first draft of this manuscript. RM supervised all aspects of this study including the data analysis and contributed significantly in the experimental design, and preparation and editing of the manuscript. SS, EK, PR, and PM also provided vital input in the experimental design, and the preparation and editing of the manuscript.
Funding
Research presented here was supported by NSF IOS 1856724 to RM and NIH R01 DA048946 to PR. JB was supported by the National Institute on Drug Abuse of the National Institutes of Health under Award Number T32DA007234 to PM.
Conflict of Interest
The authors declare that the research was conducted in the absence of any commercial or financial relationships that could be construed as a potential conflict of interest.
Acknowledgments
We would like to thank Anna Peyla for assisting with the experiments.
Supplementary Material
The Supplementary Material for this article can be found online at: https://www.frontiersin.org/articles/10.3389/fnbeh.2020.583395/full#supplementary-material
References
Abbott, D. H., Saltzman, W., Schultz-Darken, N. J., and Tannenbaum, P. L. (1998). Adaptations to subordinate status in female marmoset monkeys. Compar. Biochem. Physiol. Part C Pharmacol. Toxicol. Endocrinol. 119, 261–274. doi: 10.1016/s0742-8413(98)00015-2
Albers, H. E. (2012). The regulation of social recognition, social communication and aggression: vasopressin in the social behavior neural network. Hormones Behav. 61, 283–292. doi: 10.1016/j.yhbeh.2011.10.007
Albers, H. E., Huhman, K. L., and Meisel, R. L. (2002). “Hormonal basis of social conflict and communication,” in Hormones, Brain and Behavior, eds D. Pfaff, A. P. Arnold, A. Etgen, S. E. Fahrbach, and R. T. Rubin (Amsterdam: Academic Press), 393–433. doi: 10.1016/b978-012532104-4/50008-1
Aleyasin, H., Flanigan, M. E., Golden, S. A., Takahashi, A., Menard, C., Madeline, L. P., et al. (2018). Cell-Type-Specific Role of DeltaFosB in Nucleus Accumbens In Modulating Intermale Aggression. J. Neurosci. 38, 5913–5924. doi: 10.1523/jneurosci.0296-18.2018
Atanasova, T., Kharybina, Z., Kaarela, T., Huupponen, J., Luchkina, N. V., Taira, T., et al. (2019). GluA4 Dependent Plasticity Mechanisms Contribute to Developmental Synchronization of the CA3-CA1 Circuitry in the Hippocampus. Neurochem. Res. 44, 562–571. doi: 10.1007/s11064-017-2392-8
Bar-Shira, O., Maor, R., and Chechik, G. (2015). Gene Expression Switching of Receptor Subunits in Human Brain Development. PLoS Comput. Biol. 11:e1004559. doi: 10.1371/journal.pcbi.1004559
Bartlett, T. E., Bannister, N. J., Collett, V. J., Dargan, S. L., Massey, P. V., Bortolotto, Z. A., et al. (2007). Differential roles of NR2A and NR2B-containing NMDA receptors in LTP and LTD in the CA1 region of two-week old rat hippocampus. Neuropharmacology 52, 60–70. doi: 10.1016/j.neuropharm.2006.07.013
Been, L. E., Gibbons, A. B., and Meisel, R. L. (2019). Towards a neurobiology of female aggression. Neuropharmacology 156, 107451. doi: 10.1016/j.neuropharm.2018.11.039
Been, L. E., Hedges, V. L., Vialou, V., Nestler, E. J., and Meisel, R. L. (2013). DeltaJunD overexpression in the nucleus accumbens prevents sexual reward in female Syrian hamsters. Genes Brain Behav. 12, 666–672. doi: 10.1111/gbb.12060
Been, L. E., Moore, K. M., Kennedy, B. C., and Meisel, R. L. (2016). Metabotropic Glutamate Receptor and Fragile X Signaling in a Female Model of Escalated Aggression. Biol. Psychiatry 79, 685–692. doi: 10.1016/j.biopsych.2015.07.021
Beloate, L. N., and Coolen, L. M. (2017). Influences of social reward experience on behavioral responses to drugs of abuse: Review of shared and divergent neural plasticity mechanisms for sexual reward and drugs of abuse. Neurosci. Biobehav. Rev. 83, 356–372. doi: 10.1016/j.neubiorev.2017.10.024
Bencsik, N., Pusztai, S., Borbely, S., Fekete, A., Dulk, M., Kis, V., et al. (2019). Dendritic spine morphology and memory formation depend on postsynaptic Caskin proteins. Sci. Rep. 9:16843.
Blanchard, R. J., Flannelly, K. J., and Blanchard, D. C. (1988). Life-span studies of dominance and aggression in established colonies of laboratory rats. Physiol. Behav. 43, 1–7. doi: 10.1016/0031-9384(88)90089-3
Bodenmann, G., and Randall, A. K. (2013). Close relationships in psychiatric disorders. Curr. Opin. Psychiatry 26, 464–467. doi: 10.1097/yco.0b013e3283642de7
Borland, J. M., Aiani, L. M., Norvelle, A., Grantham, K. N., O’Laughlin, K., Terranova, I., et al. (2019a). Sex-dependent regulation of social reward by oxytocin receptors in the ventral tegmental area. Neuropsychopharmacology 44, 785–792. doi: 10.1038/s41386-018-0262-y
Borland, J. M., Frantz, K. J., Aiani, L. M., Grantham, K. N., Song, Z., and Albers, H. E. (2017). A novel operant task to assess social reward and motivation in rodents. J. Neurosci. Methods 287, 80–88. doi: 10.1016/j.jneumeth.2017.06.003
Borland, J. M., Rilling, J. K., Frantz, K. J., and Albers, H. E. (2019b). Sex-dependent regulation of social reward by oxytocin: an inverted U hypothesis. Neuropsychopharmacology 44, 97–110. doi: 10.1038/s41386-018-0129-2
Brain, P. F. (1972). Effects of isolation/grouping on endocrine function and fighting behavior in male and female golden hamsters. (Mesocricetus auratus Waterhouse). Behav. Biol. 7, 349–357. doi: 10.1016/s0091-6773(72)80106-8
Bredewold, R., Schiavo, J. K., van der Hart, M., Verreij, M., and Veenema, A. H. (2015). Dynamic changes in extracellular release of GABA and glutamate in the lateral septum during social play behavior in juvenile rats: Implications for sex-specific regulation of social play behavior. Neuroscience 307, 117–127. doi: 10.1016/j.neuroscience.2015.08.052
Briand, L. A., Deutschmann, A. U., Ellis, A. S., and Fosnocht, A. Q. (2016). Disrupting GluA2 phosphorylation potentiates reinstatement of cocaine seeking. Neuropharmacology 111, 231–241. doi: 10.1016/j.neuropharm.2016.09.010
Chang, C. H., Hsiao, Y. H., Chen, Y. W., Yu, Y. J., and Gean, P. W. (2015). Social isolation-induced increase in NMDA receptors in the hippocampus exacerbates emotional dysregulation in mice. Hippocampus 25, 474–485. doi: 10.1002/hipo.22384
Couppis, M. H., and Kennedy, C. H. (2008). The rewarding effect of aggression is reduced by nucleus accumbens dopamine receptor antagonism in mice. Psychopharmacology 197, 449–456. doi: 10.1007/s00213-007-1054-y
Crombach, A., and Elbert, T. (2014). The benefits of aggressive traits: a study with current and former street children in Burundi. Child Abuse Neglect 38, 1041–1050. doi: 10.1016/j.chiabu.2013.12.003
Darwin, C. (1859). On the Origin of Species by Means of Natural Selection. London: John Murray. 502.
Dewing, P., Boulware, M. I., Sinchak, K., Christensen, A., Mermelstein, P. G., and Micevych, P. (2007). Membrane estrogen receptor-alpha interactions with metabotropic glutamate receptor 1a modulate female sexual receptivity in rats. J. Neurosci. 27, 9294–9300. doi: 10.1523/jneurosci.0592-07.2007
Diering, G. H., and Huganir, R. L. (2018). The AMPA Receptor Code of Synaptic Plasticity. Neuron 100, 314–329. doi: 10.1016/j.neuron.2018.10.018
Digby, L. J., and Kahlenberg, S. M. (2002). Female dominance in blue-eyed black lemurs(Eulemur macaco flavifrons). Primates J. Primatol. 43, 191–199. doi: 10.1007/bf02629647
Digby, L., and McLean Stevens, A. (2007). Maintenance of female dominance in blue-eyed black lemurs (Eulemur macaco flavifrons) and gray bamboo lemurs (Hapalemur griseus griseus) under semi-free-ranging and captive conditions. Zoo Biol. 26, 345–361. doi: 10.1002/zoo.20140
Dolen, G., Darvishzadeh, A., Huang, K. W., and Malenka, R. C. (2013). Social reward requires coordinated activity of nucleus accumbens oxytocin and serotonin. Nature 501, 179–184. doi: 10.1038/nature12518
Drickamer, L. C., and Vandenbergh, J. G. (1973). Predictors of social dominance in the adult female golden hamster (Mesocricetus auratus). Anim. Behav. 21, 564–570. doi: 10.1016/s0003-3472(73)80017-x
Ebner, S. R., Larson, E. B., Hearing, M. C., Ingebretson, A. E., and Thomas, M. J. (2018). Extinction and Reinstatement of Cocaine-seeking in Self-administering Mice is Associated with Bidirectional AMPAR-mediated Plasticity in the Nucleus Accumbens Shell. Neuroscience 384, 340–349. doi: 10.1016/j.neuroscience.2018.05.043
Edwards, D. H., and Spitzer, N. (2006). 6. Social dominance and serotonin receptor genes in crayfish. Curr. Topics Dev Biol 74, 177–199. doi: 10.1016/s0070-2153(06)74006-6
Fett, A. J., Mouchlianitis, E., Gromann, P. M., Vanes, L., Shergill, S. S., and Krabbendam, L. (2019). The neural mechanisms of social reward in early psychosis. Soc. Cog Affect. Neurosci. 14, 861–870. doi: 10.1093/scan/nsz058
Fish, E. W., DeBold, J. F., and Miczek, K. A. (2005). Escalated aggression as a reward: corticosterone and GABA(A) receptor positive modulators in mice. Psychopharmacology 182, 116–127. doi: 10.1007/s00213-005-0064-x
Fox, C. J., Russell, K. I., Wang, Y. T., and Christie, B. R. (2006). Contribution of NR2A and NR2B NMDA subunits to bidirectional synaptic plasticity in the hippocampus in vivo. Hippocampus 16, 907–915. doi: 10.1002/hipo.20230
Francis, T. C., and Lobo, M. K. (2017). Emerging Role for Nucleus Accumbens Medium Spiny Neuron Subtypes in Depression. Biol. Psychiatry 81, 645–653. doi: 10.1016/j.biopsych.2016.09.007
Garcia-Pardo, M. P., Minarro, J., Llansola, M., Felipo, V., and Aguilar, M. A. (2019). Role of NMDA and AMPA glutamatergic receptors in the effects of social defeat on the rewarding properties of MDMA in mice. Eur. J. Neurosci. 50, 2623–2634. doi: 10.1111/ejn.14190
Gattermann, R., Fritzsche, P., Neumann, K., Al-Hussein, I., Kayser, A., Abiad, M., et al. (2001). Notes on the current distribution and the ecology of wild golden hamsters (Mesocricetus auratus). J. Zool. 154, 359–365. doi: 10.1017/s0952836901000851
Giebel, G., Weierstall, R., Schauer, M., and Elbert, T. (2013). Female attraction to appetitive-aggressive men is modulated by women’s menstrual cycle and men’s vulnerability to traumatic stress. Evol. Psychol. 11, 248–262.
Gil, M., Nguyen, N. T., McDonald, M., and Albers, H. E. (2013). Social reward: interactions with social status, social communication, aggression, and associated neural activation in the ventral tegmental area. Eur. J. Neurosci. 38, 2308–2318. doi: 10.1111/ejn.12216
Golden, S. A., Heins, C., Venniro, M., Caprioli, D., Zhang, M., Epstein, D. H., et al. (2017). Compulsive Addiction-like Aggressive Behavior in Mice. Biol. Psychiatry 82, 239–248. doi: 10.1016/j.biopsych.2017.03.004
Golden, S. A., Jin, M., and Shaham, Y. (2019b). Animal Models of (or for) Aggression Reward, Addiction, and Relapse: Behavior and Circuits. J. Neurosci. 39, 3996–4008. doi: 10.1523/jneurosci.0151-19.2019
Golden, S. A., Jin, M., Heins, C., Venniro, M., Michaelides, M., and Shaham, Y. (2019a). Nucleus Accumbens Drd1-Expressing Neurons Control Aggression Self-Administration and Aggression Seeking in Mice. J. Neurosci. 39, 2482–2496. doi: 10.1523/jneurosci.2409-18.2019
Goncalves, J., Bartol, T. M., Camus, C., Levet, F., Menegolla, A. P., Sejnowski, T. J., et al. (2020). Nanoscale co-organization and coactivation of AMPAR, NMDAR, and mGluR at excitatory synapses. Proc. Natl. Acad. Sci. U S A. 117, 14503–14511. doi: 10.1073/pnas.1922563117
Gray, C. L., Norvelle, A., Larkin, T., and Huhman, K. L. (2015). Dopamine in the nucleus accumbens modulates the memory of social defeat in Syrian hamsters (Mesocricetus auratus). Behav. Brain Res. 286, 22–28. doi: 10.1016/j.bbr.2015.02.030
Grelk, D. F., Papson, B. A., Cole, J. E., and Rowe, F. A. (1974). The influence of caging conditions and hormone treatments on fighting in male and female hamsters. Horm. Behav. 5, 355–366. doi: 10.1016/0018-506x(74)90021-x
Gross, K. S., Brandner, D. D., Martinez, L. A., Olive, M. F., Meisel, R. L., and Mermelstein, P. G. (2016). Opposite Effects of mGluR1a and mGluR5 Activation on Nucleus Accumbens Medium Spiny Neuron Dendritic Spine Density. PloS One 11:e0162755. doi: 10.1371/journal.pone.0162755
Gross, K. S., Moore, K. M., Meisel, R. L., and Mermelstein, P. G. (2018). mGluR5 Mediates Dihydrotestosterone-Induced Nucleus Accumbens Structural Plasticity, but Not Conditioned Reward. Front. Neurosci. 12:855. doi: 10.3389/fnins.2018.00855
Hamilton, P. J., Burek, D. J., Lombroso, S. I., Neve, R. L., Robinson, A. J., Nestler, E. J., et al. (2018). Cell-Type-Specific Epigenetic Editing at the Fosb Gene Controls Susceptibility to Social Defeat Stress. Neuropsychopharmacology 43, 272–284. doi: 10.1038/npp.2017.88
Hedges, V. L., Chakravarty, S., Nestler, E. J., and Meisel, R. L. (2009). Delta FosB overexpression in the nucleus accumbens enhances sexual reward in female Syrian hamsters. Genes Brain Behavior 8, 442–449. doi: 10.1111/j.1601-183x.2009.00491.x
Henley, J. M., and Wilkinson, K. A. (2013). AMPA receptor trafficking and the mechanisms underlying synaptic plasticity and cognitive aging. Dial. Clin. Neurosci. 15, 11–27. doi: 10.31887/dcns.2013.15.1/jhenley
Henry, J. D., von Hippel, W., Molenberghs, P., Lee, T., and Sachdev, P. S. (2016). Clinical assessment of social cognitive function in neurological disorders. Nat. Rev. Neurol. 12, 28–39. doi: 10.1038/nrneurol.2015.229
Heshmati, M., Aleyasin, H., Menard, C., Christoffel, D. J., Flanigan, M. E., Pfau, M. L., et al. (2018). Cell-type-specific role for nucleus accumbens neuroligin-2 in depression and stress susceptibility. Proc. Natl. Acad. Sci. U S A. 115, 1111–1116. doi: 10.1073/pnas.1719014115
Huhman, K. L., Solomon, M. B., Janicki, M., Harmon, A. C., Lin, S. M., Israel, J. E., et al. (2003). Conditioned defeat in male and female Syrian hamsters. Horm. Behav. 44, 293–299. doi: 10.1016/j.yhbeh.2003.05.001
Huupponen, J., Atanasova, T., Taira, T., and Lauri, S. E. (2016). GluA4 subunit of AMPA receptors mediates the early synaptic response to altered network activity in the developing hippocampus. J. Neurophysiol. 115, 2989–2996. doi: 10.1152/jn.00435.2015
Kammermeier, P. J., and McCullock, T. W. (2020). Probing the unusual functional interaction between mGluR1 and mGluR5. J. Feder. Am. Soc. Exp. Biol. 34, 1–1. doi: 10.1096/fasebj.2020.34.s1.06395
Kasai, H., Matsuzaki, M., Noguchi, J., Yasumatsu, N., and Nakahara, H. (2003). Structure-stability-function relationships of dendritic spines. Trends Neurosci. 26, 360–368. doi: 10.1016/s0166-2236(03)00162-0
Komori, T., Makinodan, M., and Kishimoto, T. (2019). Social status and modern-type depression: A review. Brain Behav. 9:e01464.
Kourrich, S., Rothwell, P. E., Klug, J. R., and Thomas, M. J. (2007). Cocaine experience controls bidirectional synaptic plasticity in the nucleus accumbens. J. Neurosci. 27, 7921–7928. doi: 10.1523/jneurosci.1859-07.2007
Krach, S., Paulus, F. M., Bodden, M., and Kircher, T. (2010). The rewarding nature of social interactions. Front. Behav. Neurosci. 4:22. doi: 10.3389/fnbeh.2010.00022
Lei, G., Liu, F., Liu, P., Jiao, T., Yang, L., Chu, Z., et al. (2020). Does genetic mouse model of constitutive Hint1 deficiency exhibit schizophrenia-like behaviors? Schizophr. Res. S0920-9964, 30270–X.
Lindenfors, P., and Tullberg, B. S. (2011). Evolutionary aspects of aggression the importance of sexual selection. Adv. Genet. 75, 7–22. doi: 10.1016/b978-0-12-380858-5.00009-5
Lobo, M. K., Zaman, S., Damez-Werno, D. M., Koo, J. W., Bagot, R. C., DiNieri, J. A., et al. (2013). DeltaFosB induction in striatal medium spiny neuron subtypes in response to chronic pharmacological, emotional, and optogenetic stimuli. J. Neurosci. 33, 18381–18395. doi: 10.1523/jneurosci.1875-13.2013
Luchkina, N. V., Coleman, S. K., Huupponen, J., Cai, C., Kivisto, A., Taira, T., et al. (2017). Molecular mechanisms controlling synaptic recruitment of GluA4 subunit-containing AMPA-receptors critical for functional maturation of CA1 glutamatergic synapses. Neuropharmacology 112, 46–56. doi: 10.1016/j.neuropharm.2016.04.049
Lum, J. S., Fernandez, F., Matosin, N., Andrews, J. L., Huang, X. F., Ooi, L., et al. (2016). Neurodevelopmental Expression Profile of Dimeric and Monomeric Group 1 mGluRs: Relevance to Schizophrenia Pathogenesis and Treatment. Sci. Rep. 6:34391.
Malenka, R. C. (2003). Synaptic plasticity and AMPA receptor trafficking. Ann. N Y Acad. Sci. 1003, 1–11. doi: 10.1196/annals.1300.001
Malinow, R., and Malenka, R. C. (2002). AMPA receptor trafficking and synaptic plasticity. Ann. Rev. Neurosci. 25, 103–126. doi: 10.1146/annurev.neuro.25.112701.142758
Massey, P. V., Johnson, B. E., Moult, P. R., Auberson, Y. P., Brown, M. W., Molnar, E., et al. (2004). Differential roles of NR2A and NR2B-containing NMDA receptors in cortical long-term potentiation and long-term depression. J. Neurosci. 24, 7821–7828. doi: 10.1523/jneurosci.1697-04.2004
Meisel, R. L., and Joppa, M. A. (1994). Conditioned place preference in female hamsters following aggressive or sexual encounters. Physiol. Behav. 56, 1115–1118. doi: 10.1016/0031-9384(94)90352-2
Meisel, R. L., Sterner, M. R., and Diekman, M. A. (1988). Differential hormonal control of aggression and sexual behavior in female Syrian hamsters. Horm. Behav. 22, 453–466. doi: 10.1016/0018-506x(88)90050-5
Menard, C., and Quirion, R. (2012). Group 1 metabotropic glutamate receptor function and its regulation of learning and memory in the aging brain. Front. Pharmacol. 3:182. doi: 10.3389/fphar.2012.00182
Meyer, D., Bonhoeffer, T., and Scheuss, V. (2014). Balance and stability of synaptic structures during synaptic plasticity. Neuron 82, 430–443. doi: 10.1016/j.neuron.2014.02.031
Mohebi, A., Pettibone, J. R., Hamid, A. A., Wong, J. T., Vinson, L. T., Patriarchi, T., et al. (2019). Dissociable dopamine dynamics for learning and motivation. Nature 570, 65–70. doi: 10.1038/s41586-019-1235-y
Moore, K. M., Oelberg, W. L., Glass, M. R., Johnson, M. D., Been, L. E., and Meisel, R. L. (2019). Glutamate Afferents From the Medial Prefrontal Cortex Mediate Nucleus Accumbens Activation by Female Sexual Behavior. Front. Behav. Neurosci. 13:227. doi: 10.3389/fnbeh.2019.00227
Morrison, K. E., Bader, L. R., Clinard, C. T., Gerhard, D. M., Gross, S. E., and Cooper, M. A. (2014). Maintenance of dominance status is necessary for resistance to social defeat stress in Syrian hamsters. Behav. Brain Res. 270, 277–286. doi: 10.1016/j.bbr.2014.05.041
Muddashetty, R. S., Kelic, S., Gross, C., Xu, M., and Bassell, G. J. (2007). Dysregulated metabotropic glutamate receptor-dependent translation of AMPA receptor and postsynaptic density-95 mRNAs at synapses in a mouse model of fragile X syndrome. J. Neurosci. 27, 5338–5348. doi: 10.1523/jneurosci.0937-07.2007
Nagy, E., and Moore, S. (2017). Social interventions: An effective approach to reduce adult depression? J. Affect. Disord. 218, 131–152. doi: 10.1016/j.jad.2017.04.043
Nardou, R., Lewis, E. M., Rothhaas, R., Xu, R., Yang, A., Boyden, E., et al. (2019). Oxytocin-dependent reopening of a social reward learning critical period with MDMA. Nature 569, 116–120. doi: 10.1038/s41586-019-1075-9
Nelson, R. J., and Trainor, B. C. (2007). Neural mechanisms of aggression. Nature Rev. Neurosci. 8, 536–546.
Novacek, D. M., Gooding, D. C., and Pflum, M. J. (2016). Hedonic Capacity in the Broader Autism Phenotype: Should Social Anhedonia Be Considered a Characteristic Feature? Front. Psychol. 7:666. doi: 10.3389/fpsyg.2016.00666
Panasiuk, M., Hertz, A., and Gale-Grant, O. (2019). Nucleus Accumbens Dopamine Receptor 1 Expressing Neurons Are Instrumental in Appetitive Aggression. J. Neurosci. 39, 6610–6612. doi: 10.1523/jneurosci.0772-19.2019
Pandya, N. J., Klaassen, R. V., van der Schors, R. C., Slotman, J. A., Houtsmuller, A., Smit, A. B., et al. (2016). Group 1 metabotropic glutamate receptors 1 and 5 form a protein complex in mouse hippocampus and cortex. Proteomics 16, 2698–2705. doi: 10.1002/pmic.201500400
Pegg, S., Ethridge, P., Shields, G. S., Slavich, G. M., Weinberg, A., and Kujawa, A. (2019). Blunted Social Reward Responsiveness Moderates the Effect of Lifetime Social Stress Exposure on Depressive Symptoms. Front. Behav. Neurosci. 13:178. doi: 10.3389/fnbeh.2019.00178
Peterson, B. M., Mermelstein, P. G., and Meisel, R. L. (2015). Estradiol mediates dendritic spine plasticity in the nucleus accumbens core through activation of mGluR5. Brain Struct. Func. 220, 2415–2422. doi: 10.1007/s00429-014-0794-9
Pettinger, A. M., Steiger, S., Muller, J. K., Sakaluk, S. K., and Eggert, A. K. (2011). Dominance status and carcass availability affect the outcome of sperm competition in burying beetles. Behav. Ecol. 22, 1079–1087.
Pitchers, K. K., Di Sebastiano, A. R., and Coolen, L. M. (2016). mGluR5 activation in the nucleus accumbens is not essential for sexual behavior or cross-sensitization of amphetamine responses by sexual experience. Neuropharmacology 107, 122–130. doi: 10.1016/j.neuropharm.2016.03.002
Pitchers, K. K., Frohmader, K. S., Vialou, V., Mouzon, E., Nestler, E. J., Lehman, M. N., et al. (2010). DeltaFosB in the nucleus accumbens is critical for reinforcing effects of sexual reward. Genes Brain Behav. 9, 831–840. doi: 10.1111/j.1601-183x.2010.00621.x
Pitchers, K. K., Schmid, S., Di Sebastiano, A. R., Wang, X., Laviolette, S. R., Lehman, M. N., et al. (2012). Natural reward experience alters AMPA and NMDA receptor distribution and function in the nucleus accumbens. PloS One 7:e34700. doi: 10.1371/journal.pone.0034700
Pitchers, K. K., Vialou, V., Nestler, E. J., Laviolette, S. R., Lehman, M. N., and Coolen, L. M. (2013). Natural and drug rewards act on common neural plasticity mechanisms with deltaFosB as a key mediator. J. Neurosci. 33, 3434–3442. doi: 10.1523/jneurosci.4881-12.2013
Reiner, A., and Levitz, J. (2018). Glutamatergic Signaling in the Central Nervous System: Ionotropic and Metabotropic Receptors in Concert. Neuron 98, 1080–1098. doi: 10.1016/j.neuron.2018.05.018
Robbins, M. J., Ciruela, F., Rhodes, A., and McIlhinney, R. A. (1999). Characterization of the dimerization of metabotropic glutamate receptors using an N-terminal truncation of mGluR1alpha. J. Neurochem. 72, 2539–2547. doi: 10.1046/j.1471-4159.1999.0722539.x
Ross, A. P., Norvelle, A., Choi, D. C., Walton, J. C., Albers, H. E., and Huhman, K. L. (2017). Social housing and social isolation: Impact on stress indices and energy balance in male and female Syrian hamsters (Mesocricetus auratus). Physiol. Behav. 177, 264–269. doi: 10.1016/j.physbeh.2017.05.015
Salamone, J. D., Correa, M., Farrar, A. M., Nunes, E. J., and Pardo, M. (2009). Dopamine, behavioral economics, and effort. Front. Behav. Neurosci. 3:13. doi: 10.3389/neuro.08.013
Schultz, W., Dayan, P., and Montague, P. R. (1997). A neural substrate of prediction and reward. Science 275, 1593–1599. doi: 10.1126/science.275.5306.1593
Shahrokh, D. K., Zhang, T. Y., Diorio, J., Gratton, A., and Meaney, M. J. (2010). Oxytocin-dopamine interactions mediate variations in maternal behavior in the rat. Endocrinology 151, 2276–2286. doi: 10.1210/en.2009-1271
Smith, A. S., and Wang, Z. (2014). Hypothalamic oxytocin mediates social buffering of the stress response. Biological Psychiatry 76, 281–288. doi: 10.1016/j.biopsych.2013.09.017
Snyder-Mackler, N., Burger, J. R., Gaydosh, L., Belsky, D. W., Noppert, G. A., Campos, F. A., et al. (2020). Social determinants of health and survival in humans and other animals. Science. 368:eaax9553. doi: 10.1126/science.aax9553
Snyder-Mackler, N., Sanz, J., Kohn, J. N., Brinkworth, J. F., Morrow, S., Shave, A. O., et al. (2016). Social status alters immune regulation and response to infection in macaques. Science 354, 1041–1045. doi: 10.1126/science.aah3580
Staffend, N. A., and Meisel, R. L. (2012). Aggressive experience increases dendritic spine density within the nucleus accumbens core in female Syrian hamsters. Neuroscience 227, 163–169. doi: 10.1016/j.neuroscience.2012.09.064
Staffend, N. A., Hedges, V. L., Chemel, B. R., Watts, V. J., and Meisel, R. L. (2014). Cell-type specific increases in female hamster nucleus accumbens spine density following female sexual experience. Brain Struct. Fun. 219, 2071–2081. doi: 10.1007/s00429-013-0624-5
Stagkourakis, S., Spigolon, G., Liu, G., and Anderson, D. J. (2020). Experience-dependent plastcitiy in an innate social behavior is mediated by hypothalamic LTP. Proc. Natl. Acad. Sci. U S A. 117, 25789–25799. doi: 10.1073/pnas.2011782117
Stockley, P., and Bro-Jorgensen, J. (2011). Female competition and its evolutionary consequences in mammals. Biol. Rev. Camb. Phil. Soc. 86, 341–366. doi: 10.1111/j.1469-185x.2010.00149.x
Stoker, A. K., Olivier, B., and Markou, A. (2012). Involvement of metabotropic glutamate receptor 5 in brain reward deficits associated with cocaine and nicotine withdrawal and somatic signs of nicotine withdrawal. Psychopharmacology 221, 317–327. doi: 10.1007/s00213-011-2578-8
Sweatt, J. D. (2016). Dynamic DNA methylation controls glutamate receptor trafficking and synaptic scaling. J. Neurochem. 137, 312–330. doi: 10.1111/jnc.13564
Takahashi, H., Yamazaki, H., Hanamura, K., Sekino, Y., and Shirao, T. (2009). Activity of the AMPA receptor regulates drebrin stabilization in dendritic spine morphogenesis. J. Cell Sci. 122, 1211–1219. doi: 10.1242/jcs.043729
Terranova, J. I., Ferris, C. F., and Albers, H. E. (2017). Sex Differences in the Regulation of Offensive Aggression and Dominance by Arginine-Vasopressin. Front. Endocrinol. 8:308. doi: 10.3389/fendo.2017.00308
Terranova, J. I., Song, Z., Larkin, T. E. II, Hardcastle, N., Norvelle, A., Riaz, A., et al. (2016). Serotonin and arginine-vasopressin mediate sex differences in the regulation of dominance and aggression by the social brain. Proc. Natl. Acad. Sci. U S A. 113, 13233–13238. doi: 10.1073/pnas.1610446113
Trezza, V., Campolongo, P., and Vanderschuren, L. J. (2011). Evaluating the rewarding nature of social interactions in laboratory animals. Developmental Cog. Neurosci. 1, 444–458. doi: 10.1016/j.dcn.2011.05.007
Turner, B. D., Kashima, D. T., Manz, K. M., Grueter, C. A., and Grueter, B. A. (2018a). Synaptic Plasticity in the Nucleus Accumbens: Lessons Learned from Experience. ACS Chem. Neurosci. 9, 2114–2126. doi: 10.1021/acschemneuro.7b00420
Turner, B. D., Rook, J. M., Lindsley, C. W., Conn, P. J., and Grueter, B. A. (2018b). mGlu1 and mGlu5 modulate distinct excitatory inputs to the nucleus accumbens shell. Neuropsychopharmacology 43, 2075–2082. doi: 10.1038/s41386-018-0049-1
Venniro, M., Zhang, M., Caprioli, D., Hoots, J. K., Golden, S. A., Heins, C., et al. (2018). Volitional social interaction prevents drug addiction in rat models. Nat. Neurosci. 21, 1520–1529. doi: 10.1038/s41593-018-0246-6
Wallace, D. L., Vialou, V., Rios, L., Carle-Florence, T. L., Chakravarty, S., Kumar, A., et al. (2008). The influence of DeltaFosB in the nucleus accumbens on natural reward-related behavior. J Neurosci. 28, 10272–10277.
Walsh, J. J., Christoffel, D. J., Heifets, B. D., Ben-Dor, G. A., Selimbeyoglu, A., Hung, L. W., et al. (2018). 5-HT release in nucleus accumbens rescues social deficits in mouse autism model. Nature. 560, 589–594. doi: 10.1038/s41586-018-0416-4
Weiss, F., Paulus, M. P., Lorang, M. T., and Koob, G. F. (1992). Increases in extracellular dopamine in the nucleus accumbens by cocaine are inversely related to basal levels: effects of acute and repeated administration. J. Neurosci. 12, 4372–4380. doi: 10.1523/jneurosci.12-11-04372.1992
Wolf, M. E., and Tseng, K. Y. (2012). Calcium-permeable AMPA receptors in the VTA and nucleus accumbens after cocaine exposure: when, how, and why? Front. Mol. Neurosci. 5:72. doi: 10.3389/fnmol.2012.00072
Wrangham, R. W. (2018). Two types of aggression in human evolution. Proc. Natl. Acad. Sci. U S A. 115, 245–253. doi: 10.1073/pnas.1713611115
Yashiro, S., and Seki, K. (2017). Association of social defeat stress-induced anhedonia-like symptoms with mGluR1-dependent decrease in membrane-bound AMPA-GluR1 in the mouse ventral midbrain. Stress 20, 404–418. doi: 10.1080/10253890.2017.1336534
Young, L. J., and Wang, Z. (2004). The neurobiology of pair bonding. Nat. Neurosci. 7, 1048–1054. doi: 10.1038/nn1327
Keywords: social behavior, synaptic plasiticty, nucleus accumbens, metabotropic Glu receptors, female aggression
Citation: Borland JM, Kim E, Swanson SP, Rothwell PE, Mermelstein PG and Meisel RL (2020) Effect of Aggressive Experience in Female Syrian Hamsters on Glutamate Receptor Expression in the Nucleus Accumbens. Front. Behav. Neurosci. 14:583395. doi: 10.3389/fnbeh.2020.583395
Received: 27 July 2020; Accepted: 30 October 2020;
Published: 23 November 2020.
Edited by:
Richard G. Hunter, University of Massachusetts Boston, United StatesReviewed by:
Antonio Luchicchi, VU University Medical Center, NetherlandsHeng-Ye Man, Boston University, United States
Copyright © 2020 Borland, Kim, Swanson, Rothwell, Mermelstein and Meisel. This is an open-access article distributed under the terms of the Creative Commons Attribution License (CC BY). The use, distribution or reproduction in other forums is permitted, provided the original author(s) and the copyright owner(s) are credited and that the original publication in this journal is cited, in accordance with accepted academic practice. No use, distribution or reproduction is permitted which does not comply with these terms.
*Correspondence: Johnathan M. Borland, Ym9ybGEwNDBAdW1uLmVkdQ==