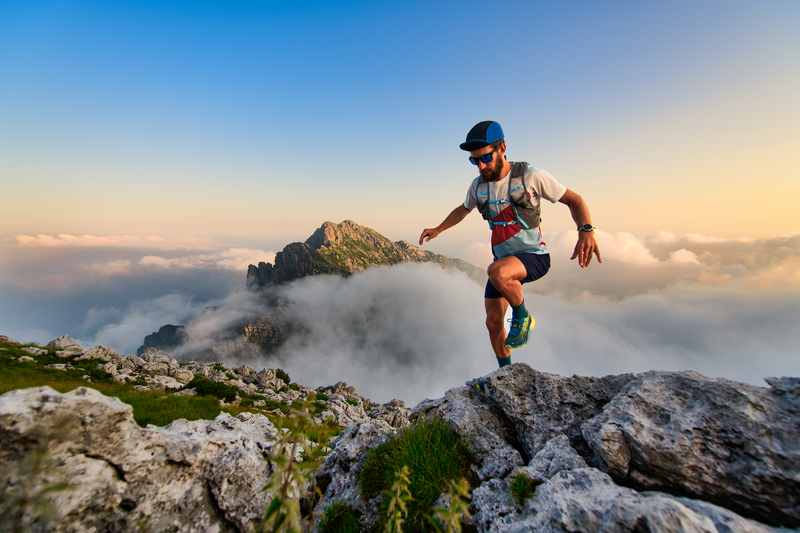
95% of researchers rate our articles as excellent or good
Learn more about the work of our research integrity team to safeguard the quality of each article we publish.
Find out more
ORIGINAL RESEARCH article
Front. Behav. Neurosci. , 18 March 2014
Sec. Emotion Regulation and Processing
Volume 8 - 2014 | https://doi.org/10.3389/fnbeh.2014.00092
This article is part of the Research Topic Modeling Affective Disorders and Addiction in Animals View all 16 articles
The fine-tuning of neuronal excitability relies on a tight control of Ca2+ homeostasis. The low voltage-activated (LVA) T-type calcium channels (Cav3.1, Cav3.2 and Cav3.3 isoforms) play a critical role in regulating these processes. Despite their wide expression throughout the central nervous system, the implication of T-type Cav3.2 isoform in brain functions is still poorly characterized. Here, we investigate the effect of genetic ablation of this isoform in affective disorders, including anxiety, cognitive functions as well as sensitivity to drugs of abuse. Using a wide range of behavioral assays we show that genetic ablation of the cacna1h gene results in an anxiety-like phenotype, whereas novelty-induced locomotor activity is unaffected. Deletion of the T-type channel Cav3.2 also triggers impairment of hippocampus-dependent recognition memories. Acute and sensitized hyperlocomotion induced by d-amphetamine and cocaine are dramatically reduced in T-type Cav3.2 deficient mice. In addition, the administration of the T-type blocker TTA-A2 prevented the expression of locomotor sensitization observed in wildtype mice. In conclusion, our data reveal that physiological activity of this specific Ca2+ channel is required for affective and cognitive behaviors. Moreover, our work highlights the interest of T-type channel blockers as therapeutic strategies to reverse drug-associated alterations.
Affective and cognitive functions constitute a complex ensemble of behavioral skills strongly dependent on the fine regulation of intracellular homeostasis and neuronal excitability. The fine regulation of calcium (Ca2+) signaling is critical for basic neuronal functions including action potential generation, neurotransmitter release and synaptic plasticity (Berridge, 1998). Ca2+ is the most common signal transduction element in neurons and its entry is tightly regulated by two major classes of voltage-gated calcium channels (VGCCs): the high-voltage activated (HVA) (L-, P/Q and N-type) and the low-voltage activated (LVA) (T-type) calcium channels.
Three subtypes of T-type channel have been cloned, namely, Cav3.1 (a1G), Cav3.2 (a1H) and Cav3.3 (a1I) (Cribbs et al., 1998, 2000; Lee et al., 1999; Zhuang et al., 2000; Gomora et al., 2002). T-type VGCCs are widely expressed throughout the body and, given their ability to switch neuronal firing patterns and modulate burst-firing activity in the central nervous system they have been implicated in several physiological aspects including sleep regulation, body weight maintenance and pain (Huguenard, 1996; Cribbs et al., 1998, 2000; Talley et al., 1999; Nilius et al., 2006; Bourinet et al., 2014). Disruption of calcium-dependent low-threshold currents mediated by T-type calcium channels have been associated with a wide range of neurological and neuropsychiatric disorders including epilepsy, insomnia, Parkinson’s disease, depression and schizophrenia as well as chronic pain syndromes (Huguenard and Prince, 1994; Kim et al., 2001, 2003; Anderson et al., 2005; Bourinet et al., 2005; Choi et al., 2007; Llinas et al., 2007; Uebele et al., 2009; Miwa et al., 2011; Francois et al., 2013; Park et al., 2013). Interestingly, several classes of drugs currently used in therapy (antipsychotics, antidepressants and antiepileptics) also show activity on T-type channels (Heady et al., 2001; Santi et al., 2002; Traboulsie et al., 2006; Kraus et al., 2007), thus suggesting T-type channels as a potential target for some brain dysfunctions. In addition, other calcium channel blockers including mibefradil, nicardipine, 2-octanol and amilodipine, which non-specifically act also on T-type channels, have been shown to reduce the psychomotor effects of cocaine, methamphetamine and phencyclidine (PCP; Popoli et al., 1993; Hori et al., 1998; Bisagno et al., 2010). More recently, the novel and specific blocker of T-type channels TTA-A2 has been reported to efficiently alleviate psychomotor effects induced by MK-801 and amphetamine as well as conditioned avoidance responding (Kraus et al., 2010; Uslaner et al., 2012), thus strengthening the strategic interest of studying the T-type channels physiology and pharmacology.
Although the implication of T-type channels in neuronal excitability has been extensively studied (Nelson et al., 2006; Shin et al., 2008; Iftinca and Zamponi, 2009; Cheong and Shin, 2013), very little is known about the role of T-type channels in motor, affective and cognitive functions. Based on the fact that Cav3.2 channels are widely expressed in the basal ganglia circuit, in limbic as well as in cortical areas (Talley et al., 1999) and that such circuits are critical components for the correct computation of many of these behaviors, we therefore decided to investigate the involvement of T-type Cav3.2 channels in spontaneous and drugs-altered performances. Understanding the physiological role of T-type calcium channels has been for long time limited by the lack of selective pharmacological compounds (Perez-Reyes, 2003), but new T-type antagonists have recently been reported (Barrow et al., 2007; Shipe et al., 2008; Yang et al., 2008). In this study, we deciphered the implication of Cav3.2 calcium channel in anxiety and memory disorders as well as in sensitivity to psychostimulants using Cav3.2 deficient mice.
Cav3.2 knockout mice (Chen et al., 2003) were maintained in the C57BL/6J background. Both WT and KO littermates were gene-rated from Cav3.2 heterozygous breeders. 8–12 weeks old male mice were used and maintained in a 12 h light/dark cycle, in stable conditions of temperature (22°C) and humidity (60%), with food and water ad libitum. All experiments were in accordance with the guidelines of the French Agriculture and Forestry Ministry for handling animals (D34-172-13).
Cocaine hydrochloride (15 mg/kg, Sigma-Aldrich) and d-amphetamine sulfate (2 mg/kg, Sigma-Aldrich) were dissolved in 0.9% physiological saline and injected intraperitoneally (i.p.) in a body volume of 10 ml/kg. The T-type blocker TTA-A2 (1 mg/kg, p.o., Merck) was dissolved in a solution containing 0.05% methylcellulose in H2O and 10% Tween80.
The light/dark conflict test, which is believed to measure anxiety-related behavior (Crawley and Goodwin, 1980), was performed by placing the mouse in a cage (35 cm width × 50 cm length × 20 cm height) that has two chambers, one bigger and bright (2/3), and the other smaller and dark (1/3). The animal was initially placed in the lighted side, and transitions between sides and the time spent in each division were recorded for 10 min. The apparatus was wiped with 70% ethanol between sessions.
Spontaneous exploratory behavior was monitored in an open field (white plastic arena with 35 cm width × 50 cm length × 20 cm height) for 10 min. The open field was wiped with 70% ethanol between sessions. The center zone was defined as a virtual perimeter within 5 cm from the sides of the arena. Experiments were videotaped and an observer scored the time spent in the center (four paws inside the center zone) and the number of transitions in the center zone.
The elevated-plus maze (EPM) was elevated 1 m above the floor and was constructed of black plastic with 2 open arms (5 cm width × 35 cm length × 0.5 cm height of the walls) and two closed arms (5 width × 35 cm length × 15 cm height of the walls). Mice were placed in the center of an EPM facing one of the open arms and were allowed to explore the maze for 10 min. The EPM was wiped with 70% ethanol between sessions. Experiments were videotaped and scored for entries and time spent in the closed arms (four paws within closed arm) or open arms (four paws within open arms).
Balance and motor coordination as well as motor learning were assessed using a mouse accelerating rotarod (Ugo Basile, Comerio, Italy). Mice were placed on the rotating drum that accelerated from 4 to 40 rpm over 5 min for four trials a day, for four consecutive days. The trial interval was 45 min for all the mice. Rotarod scores were scored for latency to either fall or ride the rod around.
The grip-strength apparatus (BioSeb, Chaville, France) comprised of a wire grid (8 × 8 cm) connected to an isometric force transducer (dynamometer). Mice were lifted by the tail so that their forepaws could grasp the grid. The mice were then gently pulled backward by the tail until the grid was released. The mean of three consecutive measurements for each mouse was calculated. The muscular strength was expressed in Newton (N) as mean ± SEM.
A cage grid is held at least 35 cm above a mouse cage containing 5–7 cm of soft bedding. Each hang period begins with all four paws of the mouse grasping the greed. The hang time is measured from the time the grid is inverted to the time that the mouse falls off the wire grid. A fixed limit of 60 s is applied for the hanging period.
Locomotor activity was measured as described previously (Brami-Cherrier et al., 2005; Gangarossa et al., 2013). Horizontal and vertical activity was measured in a circular corridor (Imetronic, Pessac, France). Counts for horizontal activity were incremented by consecutive interruption of two adjacent beams placed at a height of 1 cm per 90° sector of the corridor (mice moving through one-quarter of the circular corridor) and counts for vertical activity (rearings) as interruption of beams placed at a height of 7.5 cm along the corridor (mice stretching upwards). All mice were habituated to the test apparatus, handling, and procedure for three consecutive days before the actual experiment. In this habituation procedure mice were placed for 30 min in the activity box, received a first injection of saline, and were placed back in the box for 2 h. For the acute drug injection, the handling was identical, except that the saline injection was replaced by administration of cocaine (15 mg/kg, i.p.) and d-amphetamine (2 mg/kg, i.p). In cocaine and amphetamine sensitization experiments, mice were treated daily with cocaine (15 mg/kg, i.p.) and amphetamine (2 mg/kg, i.p.) for five consecutive days. This repeated exposure was followed by 8 days of withdrawal and by a challenge injection of cocaine (15 mg/kg) or d-amphetamine (2 mg/kg). Locomotor activity was measured as described above. The day after the challenge, wildtype and Cav3.2 deficient mice were treated with TTA-A2 (1 mg/kg) or its vehicle 30 min prior the administration of d-amphetamine or cocaine. The degree of psychostimulants-induced locomotor sensitization was determined from the slope of the curve corresponding to the 5 days of d-amphetamine or cocaine administration.
The Y-maze apparatus, made of Plexiglas had three identical arms (40 × 9 × 16 cm) placed at 120° with respect to each other. Each mouse was placed at the end of one arm and allowed to explore freely the apparatus for 5 min. Spontaneous alternation performance (SAP) was assessed by scoring the pattern of entries into each arm during the 5 min of the test. Alternations were defined as successive entries into each of the three arms as on overlapping triplet sets (i.e., ABC, BCA, ‥.). Percent of spontaneous alternations was defined as the ratio of actual (= total alternations) to possible (= total arm entries −2) number of alternations × 100. Total entries were scored as an index of ambulatory activity in the Y-maze.
Mice were habituated to a V-maze with two identical arms (34 × 6 × 15 cm, at 90°) for 10 min. The following day, mice were allowed to freely explore two objects (A and B) located at the end of the two arms. Object interaction was defined as approaching the object with the nose closer than 1 cm. Following a retention interval of 24 h, mice underwent a 5 min recall session during which the V-maze contained a familiar object (A) and a novel object (C). Following each session, the objects and the open field were cleaned with 70% ethanol. The experiments were videotaped and the time spent exploring the objects was scored. The percentage of exploration was calculated using the formula % exploration = ((Time C)/(Time A + Time C)) × 100.
Mice were habituated to an arena for 10 min. The following day, mice were allowed to freely explore two different objects (A and B) located in the same site of the arena. Object interaction was defined as approaching the object with the nose closer than 1 cm. Following a retention interval of 24 h, mice underwent a 5 min recall session during which the arena contained one of the two objects displaced in the other site of the arena (novel place). Following each session, the objects and the open field were cleaned with 70% ethanol. The experiments were videotaped and the time spent exploring the objects was scored. The percentage of exploration was calculated using the formula % exploration = ((Time B)/(Time A + Time B)) × 100.
Mice were placed in the center of a clear plastic cage containing 5 cm of sawdust bedding with 24 glass marbles (1.5 cm diameter) on top, arranged in four rows of six marbles. The lid of the cage was used to prevent mice from escaping. Mice were then allowed to explore the testing chamber for 20 min. The number of marbles buried at least two-thirds deep were counted.
The data were analyzed using one-way or two-way ANOVA followed by Bonferroni post-hoc test for specific comparisons. Student’s t-test with equal variances was used for groups of two, when relevant. In all cases, significance threshold was set at p < 0.05. Statistical analyses were performed using GraphPad Prism 5.0 (GraphPad Prism Software Inc., San Diego, USA).
We first investigated the spontaneous locomotor activity of Cav3.2 deficient mice in response to novelty, in a non-stressful environment (low luminosity). No differences in the initial horizontal activity or in the habituation phase (3 days) were observed between WT and Cav3.2 KO mice (Figures 1A–C), thus suggesting intact exploratory drive. In addition, despite a mild but significant attenuation in vertical locomotor activity (rearing behavior) observed in Cav3.2 deficient mice during the first day (Figures 1D and E), both genotypes showed habituation to the testing environment (Figure 1F).
Figure 1. Spontaneous locomotor activity in Cav3.2 deficient mice. (A) Horizontal locomotor activity of Cav3.2 null mice (n = 22) and their WT littermates (n = 26) in a novel non-stressful environment. Data (means ± SEM) were analyzed using two-way ANOVA: (Time × Genotype: F(14, 644) = 1.607, P = 0.0724; Time: F(14, 644) = 157.8, P < 0.0001; Genotype: F(1, 46) = 0.0268, P = 0.8707). (B) Cumulative locomotor activity of WT and Cav3.2 KO mice over a 150 min period. Data (means ± SEM) were analyzed using Student’s t-test: NS. (C) Environmental habituation over a period of 3 days measured as a reduction of spontaneous horizontal locomotor activity. Data (means ± SEM) were analyzed using two-way ANOVA: (Time × Genotype: F(2, 92) = 0.8412, P = 0.4345; Time: F(2, 92) = 161.4, P < 0.0001; Genotype: F(1, 46) = 0.9561, P = 0.3333). Specific comparisons: *** p < 0.001 (WT-Day3 vs. WT-Day1) and °°° p < 0.001 (KO-Day3 vs. KO-Day1). (D) Rearing activity (vertical locomotor activity) of Cav3.2 null mice (n = 22) and their WT littermates (n = 26) in a novel non-stressful environment. Data (means ± SEM) were analyzed using two-way ANOVA: (Time × Genotype: F(14, 644) = 3.751, P < 0.0001; Time: F(14, 644) = 70.86, P < 0.0001; Genotype: F(1, 46) = 4.601, P = 0.0373). Specific comparisons: ** p < 0.01 and * p < 0.05 (KO vs. WT). (E) Cumulative rearing activity of WT and Cav3.2 KO mice over a 150 min period. Data (means ± SEM) were analyzed using Student’s t-test: * p < 0.05. (F) Environmental habituation over a period of 3 days measured as a reduction of spontaneous rearing activity. Data (means ± SEM) were analyzed using two-way ANOVA: (Time × Genotype: F(2, 92) = 1.139, P = 0.3246; Time: F(2, 92) = 76.78, P < 0.0001; Genotype: F(1, 46) = 3.874, P = 0.05). Specific comparisons: *** p < 0.001 (WT-Day3 vs. WT-Day1), °°° p < 0.001 (KO-Day3 vs. KO-Day1) and # p < 0.05 (KO-Day1 vs. WT-Day1).
To investigate whether the genetic ablation of Cacna1h gene might induce muscular alteration that can ultimately impact on the spontaneous behavior of mice, we assessed muscular strength using two essays: the grip-strength and the grid test. Both tests revealed no major differences between genotypes. Grip-strength test: WT (1.68 N ± 0.076, n = 15) and KO (1.85 N ± 0.084, n = 14). Grid test: WT (59.87 sec ± 0.13, n = 15) and KO (59.79 sec ± 0.15, n = 14).
Taken together our results show that Cacna1h gene deletion does not alter spontaneous locomotor performance and exploratory drive.
Because of the reduced vertical locomotor activity, we next investigated anxiety-related behaviors in Cav3.2 deficient mice using well-established behavioral paradigms: the light/dark conflict context, the EPM and the open field tests. In the light/dark test, KO mice spent less time in the light compartment and more time in the dark compartment compared to WT mice (Figure 2A). Cav3.2 deficient mice were also slightly less active than WT as demonstrated by the reduced number of entries in both compartments (light and dark) (Figure 2B). In the EPM test, Cav3.2 deficient mice spent less time in the open arms compared to their WT littermates confirming an increased anxiety-related phenotype (Figure 2C). No differences were observed in the number of entries in both closed and open arms suggesting that this effect is not the result of an altered locomotor response (Figure 2D). To further extend these behavioral observations, we measured the spontaneous behavior of Cav3.2 deficient mice to explore a novel and stressful environment using the open field test. As shown in Figure 2E, Cav3.2 deficient mice spent less time in the center of the arena compared to their WT littermates and made an equal number of transitions in the center zone compared to WT mice therefore indicating increased anxiety rather than a general locomotor deficit (Figure 2F).
Figure 2. Anxiety-like behavior in Cav3.2 deficient mice. Mice were tested in three anxiety-related behavioral paradigms: the ligh/dark conflict test, the elevated plus maze test (EPM) and the open field test. (A) Histograms show the percentage of time Cav3.2 KO (n = 14) and WT mice (n = 15) spent in the light and the dark compartments. (B) Histograms indicate the number of entries of Cav3.2 KO (n = 14) and WT mice (n = 15) in the light and the dark compartments. (A and B) Data (means ± SEM) were analyzed using Student’s t-test: * p < 0.05; ** p < 0.01. (C) Histograms show the percentage of time Cav3.2 KO (n = 17) and WT mice (n = 19) spent in the open arms of the EPM. Data (means ± SEM) were analyzed using Student’s t-test: * p < 0.05. (D) Histograms indicate the number of entries of Cav3.2 KO (n = 17) and WT mice (n = 19) in open and closed arms of EPM. Data (means ± SEM) were analyzed using student’s t-test: ns. (E) Histograms show the percentage of time Cav3.2 KO (n = 14) and WT mice (n = 15) spent in the center zone of the open field. Data (means ± SEM) were analyzed using student’s t-test: * p < 0.05. (F) Histograms indicate the number of transitions Cav3.2 KO (n = 14) and WT mice (n = 15) made in the center zone of the open field. Data (means ± SEM) were analyzed using student’s t-test: ns.
Anxiety disorders are often exacerbated by repetitive and compulsive behaviors, such as in obsessive-compulsive disorder (OCD), in which abnormal repetitive behaviors can take place to alleviate recurrent and generalized anxiety. The marble burying test was then performed to assess whether Cav3.2 null mice displayed some forms of compulsivity. No differences were observed in the number of marbles buried: WT (15.15 ± 1.5, n = 13) and KO (16.31 ± 0.5, n = 13).
Altogether these data strongly suggest that T-type Cav3.2 channel influence anxiety-related behaviors, which do not seem being associated with repetitive and compulsive behaviors. Further studies should be performed to investigate whether T-type channels participate to altered behaviors observed in OCD.
Previous studies have shown that T-type Cav3.2 is critical for hippocampal LTP, cued-context fear conditioning and passive avoidance tasks (Chen et al., 2012). We then investigated two hippocampal recognition memory tasks, the novel and the spatial object recognition (NOR and SOR, respectively). As shown in Figures 3A, B, Cav3.2 deficient mice do not show preference for the novel or the relocated object during the recall session 24 h later as opposed to the WT. This altered response is not the result of an impairment of the exploratory drive since the exploration of the two objects is not significantly different from the WT animal during the familiarization phase (data not shown). Interestingly, the spatial working memory, assessed using the spontaneous alternation in the Y-Maze, was unaffected in Cav3.2 deficient mice (Figure 3C).
Figure 3. Memory functions in Cav3.2 deficient mice. (A) Graph shows the percentage of exploration time of Cav3.2 KO (n = 14) and WT (n = 15) mice in the NOR test. Data (means ± SEM) were analyzed using two-way ANOVA: (Object exploration × Genotype: F(1, 44) = 25.80, P < 0.0001; Object exploration: F(1, 44) = 37.58, P < 0.0001; Genotype: F(1, 44) = 3.245e-014, P = 0.99). Specific comparisons: *** p < 0.001 (WT-new vs. WT-familiar). (B) Graph shows the percentage of exploration time of Cav3.2 KO (n = 14) and WT (n = 15) mice in the SOR test. Data (means ± SEM) were analyzed using two-way ANOVA: (Object exploration × Genotype: F(1, 44) = 31.80, P < 0.0001; Object exploration: F(1, 44) = 48.81, P < 0.0001; Genotype: F(1, 44) = 1.324e-013, P = 0.99). Specific comparisons: *** p < 0.001 (WT-new vs. WT-familiar). (C) Histogram indicates the percentage of spontaneous alternation of Cav3.2 KO (n = 14) and WT (n = 15) in the Y-maze test. The red dotted line represents the chance level of alternation (random, 50%). Data (means ± SEM) were analyzed using Student’s t-test: ns. (D) Curves show coordination and motor learning in WT (n = 22) and Cav3.2 KO (n = 22) mice over a training period of 4 days. Data (means ± SEM) were analyzed using two-way ANOVA (Time × Genotype: F(3, 168) = 2.017, P = 0.1134; Time: F(3, 168) = 19.03, P < 0.0001; Genotype: F(1, 168) = 20.12, P < 0.0001). Specific comparisons: ** p < 0.01 (KO-Day4 vs. WT-Day4). (E) Percentage of motor learning in WT (n = 22) and Cav3.2 KO (n = 22). Data (means ± SEM) were analyzed using two-way ANOVA (Time × Genotype: F(1, 84) = 5.643, P < 0.05; Time: F(1, 84) = 5.654, P < 0.05; Genotype: F(1, 84) = 49.11, P < 0.0001). Specific comparisons: *** p < 0.001 (WT-Day4 vs. WT-Day1), ** p < 0.01 (KO-Day4 vs. KO-Day1) and °° p < 0.01 (KO-Day4 vs. WT-Day4).
Motor skill learning was also investigated in Cav3.2 deficient mice using the accelerating rotarod. No differences were observed during the first day of rotarod training, thus suggesting unaltered motor coordination in Cav3.2 deficient mice (Figures 3D, E). Although Cav3.2 KO mice showed a significant reduction of the motor performance compared to their WT littermates (Figures 3D, E), both genotypes gradually acquired motor skills following extensive training (Figures 3D, E).
Altogether, these data strongly suggest that T-type Cav3.2 channels influence preferentially memory recognition tasks, but spares working memory and motor learning.
The psychomotor effects of cocaine, methamphetamine and PCP have been shown to partly involve the activity of T-type calcium channels (Popoli et al., 1993; Hori et al., 1998; Bisagno et al., 2010). To test the specific contribution of T-type Cav3.2 channels in these locomotor responses, the acute hyperlocomotion induced by d-amphetamine (2 mg/kg, i.p.) and cocaine (15 mg/kg, i.p.) was evaluated in WT and Cav3.2 deficient mice. As shown in Figure 4A, the total locomotor activity over a 120 min period induced by a single injection of d-amphetamine was dramatically reduced in Cav3.2 deficient mice compared to their WT littermates. A similar effect was also observed when mice were treated with cocaine (Figure 4B).
Figure 4. Psychomotor effects of d-amphetamine and cocaine in Cav3.2 deficient mice. (A) Locomotor activity induced by single administration of d-amphetamine (2 mg/kg, i.p.) in WT (n = 17) and Cav3.2 KO (n = 15) mice. Data (means ± SEM) were analyzed using two-way ANOVA: (Treatment × Genotype: F(14, 420) = 4.805, P < 0.0001; Treatment: F(14, 420) = 8.34, P < 0.0001; Genotype: F(1, 30) = 15.85, P < 0.001). (B) Locomotor activity induced by single administration of cocaine (15 mg/kg, i.p.) in WT (n = 9) and Cav3.2 KO (n = 7) mice. Data (means ± SEM) were analyzed using two-way ANOVA: (Treatment × Genotype: F(14, 196) = 12.09, P < 0.0001; Treatment: F(14, 196) = 17.53, P < 0.0001; Genotype: F(1, 14) = 20.92, P < 0.001). (C) Locomotor activity induced by repeated administration (sensitization protocol) of d-amphetamine (2 mg/kg, i.p.) in WT (n = 17) and Cav3.2 KO (n = 15). Data (means ± SEM) were analyzed using two-way ANOVA: (Treatment × Genotype: F(6, 210) = 5.280, P < 0.001; Treatment: F(6, 210) = 43.34, P < 0.0001; Genotype: F(1, 210) = 147.3, P < 0.001). Specific comparisons: *** p < 0.001 (KO vs. WT), °°° p < 0.001 (WT-Day5 vs. WT-Day1) and ## p < 0.01 (KO-Day5 vs. KO-Day1). (D) Degree of d-amphetamine-induced sensitization measured as a slope of sensitization curves in WT (n = 17) and Cav3.2 KO (n = 15). Data (means ± SEM) were analyzed using Student’s t-test: * p < 0.05. (E) Locomotor activity induced by repeated administration (sensitization protocol) of cocaine (15 mg/kg, i.p.) in WT (n = 9) and Cav3.2 KO (n = 7). Data (means ± SEM) were analyzed using two-way ANOVA: (Treatment × Genotype: F(6, 98) = 9.086, P < 0.0001; Treatment: F(6, 98) = 37.96, P < 0.0001; Genotype: F(1, 98) = 301.3, P < 0.0001). Specific comparisons: *** p < 0.001 (KO vs. WT), °°° p < 0.001 (WT-Day5 vs. WT-Day1) and # p < 0.05 (KO-Day5 vs. KO-Day1). (F) Degree of cocaine-induced sensitization measured as a slope of sensitization curves in WT (n = 9) and Cav3.2 KO (n = 7). Data (means ± SEM) were analyzed using student’s t-test: * p < 0.05.
We next determine whether Cav3.2 KO mice could still undergo locomotor sensitization despite the reduced acute locomotor response. Repeated exposure to d-amphetamine (2 mg/kg) results in a progressive and persistent enhancement of the locomotor response in WT mice (Figure 4C). Cav3.2 deficient mice also displayed locomotor sensitization but this response remained lower than in WT mice even when the mice were challenged after a withdrawal period of 8 days (Figure 4C). Interestingly, taking into account the slope sensitization, the locomotor sensitization to d-amphetamine was less pronounced in Cav3.2 deficient mice than in their WT controls (Figure 4D). Similar results have been obtained when mice were repeatedly injected with cocaine (Figures 4E, F). Altogether, these results show that Cav3.2 null mice displayed a reduction of sensitized responses to d-amphetamine and cocaine.
Finally, we examined whether a pharmacological acute blockade of T-type Ca2+ channel could affect the expression of locomotor sensitization in WT animals and therefore recapitulate the phenotype of Cav3.2 null mice. To test this possibility, WT mice sensitized to d-amphetamine (Figures 5A–B) or cocaine (Figures 5D–E) were challenged with either vehicle or TTA-A2 (1 mg/kg, p.o.), a specific but non-selective T-type blocker prior to the last psychostimulant treatment (day 14). TTA-A2 blocked the expression of d-amphetamine and cocaine locomotor sensitization (Figures 5A, B, D, E). When the identical treatment regimen was performed in sensitized Cav3.2 deficient mice, TTA-A2 had no effect on the expression of either d-amphetamine or cocaine locomotor sensitization (Figures 5A, C, D, F). Taken together, these results suggest that the effect of this T-type Ca2+ channel blocker on the expression of sensitization observed in WT mice was most likely attributable to the specific inhibition of the Cav3.2 isoform.
Figure 5. Effect of the T-type calcium channel inhibitor TTA-A2 on psychostimulants-induced locomotor activity. (A) Histograms show the effect of TTA-A2 (1 mg/kg, p.o.) on d-amphetamine-sensitized mice. Data (means ± SEM) were analyzed using two-way ANOVA: A (Treatment × Genotype: F(1, 28) = 26.47, P < 0.0001; Treatment: F(1, 28) = 2.595, P = 0.1185; Genotype: F(1, 28) = 53.99, P < 0.0001). Specific comparisons: *** p < 0.001 (WT-TTA-A2+d-amph vs. WT-Veh+d-amph) and °°° p < 0.001 (KO-Veh+d-amph vs. WT-Veh+d-amph). (B and C) Curves showing the behavioral profile of T-type channels blocker TTA-A2 in WT (B, n = 9) and Cav3.2 KO (C, n = 7) d-amphetamine-treated mice. Data (means ± SEM) were analyzed using two-way ANOVA: B (Treatment × Time: F(14, 224) = 9.302, P < 0.0001; Time: F(14, 224) = 34.60, P < 0.0001; Treatment: F(1, 16) = 83.58, P < 0.0001); C (Treatment × Time: F(14, 168) = 2.717, P < 0.01; Time: F(14, 168) = 85.72, P < 0.0001; Treatment: F(1, 12) = 2.108, P = 0.1722). (D) Histograms show the effect of TTA-A2 (1 mg/kg, p.o.) on cocaine-sensitized mice. Data (means ± SEM) were analyzed using two-way ANOVA: A (Treatment × Genotype: F(1, 28) = 24.15, P < 0.0001; Treatment: F(1, 28) = 27.96, P < 0.0001; Genotype: F(1, 28) = 34.54, P < 0.0001). Specific comparisons: *** p < 0.001 (WT-TTA-A2+Coc vs. WT-Veh+Coc) and °°° p < 0.001 (KO-Veh+Coc vs. WT-Veh+Coc). (E and F) Curves showing the behavioral profile of T-type calcium channels blocker TTA-A2 in WT (E, n = 9) and Cav3.2 KO (F, n = 7) cocaine-treated mice. Data (means ± SEM) were analyzed using two-way ANOVA: E (Treatment × Time: F(14, 224) = 2.31, P < 0.01; Time: F(14, 224) = 37.34, P < 0.0001; Treatment: F(1, 16) = 50.35, P < 0.0001); F (Treatment × Time: F(14, 168) = 1.768, P < 0.05; Time: F(14, 168) = 29.27, P < 0.0001; Treatment: F(1, 12) = 0.8157, P = 0.3842).
In the present study, we evaluate the effect of genetic ablation of the Cacna1h gene in several behavioral paradigms related to motor, affective and cognitive functions. T-type calcium channels mediate calcium-dependent low-threshold currents and they are associated with rhythmic burst-firing activities of some brain regions (Beurrier et al., 1999; Park et al., 2010; Tai et al., 2011; Bourinet et al., 2014). By regulating Ca2+ homeostasis and neuronal activation, T-type calcium channels (Cav3.1, Cav3.2 and Cav3.3) represent an interesting and promising pharmacological target. T-type calcium channels are widely expressed in the brain (Talley et al., 1999) and the T-type Cav3.2 isoform is found in key regions involved in affective and cognitive behaviors. However, due to the lack of selective compounds for each T-type isoforms, little is known about their respective specific implications in the above-mentioned functions. Here, we focused on studying the T-type Cav3.2 channel using Cav3.2 deficient mice. Our findings reveal that the lack of Cacna1h gene promotes anxiety-related behavior, impairs learning and memory formation and results in a reduced sensitivity to psychostimulants (d-amphetamine and cocaine).
The involvement of T-type calcium channels in anxiety-related disorders is poorly documented. Our study reveals that mice lacking the T-type Cav3.2 showed elevated anxiety across a range of behavioral tests including the light/dark conflict test, the EPM and the open field. These phenotypes were not the result of a general motor impairment since Cav3.2 deficient showed unaltered locomotor response when placed in a novel environment (see also Choi et al., 2007). However, our results contrast with this previous study reporting a lack of anxiety-related phenotype in Cav3.2 KO mice in the light/dark conflict test (Choi et al., 2007). Different parameters could explain this apparent discrepancy. First, the genetic background of the Cav3.2 deficient mice employed in both studies was different. While our mice were on a C57BL/6J background the previous study used mice on a mixed 129/sv and C57BL/6J background (Choi et al., 2007). C57BL/6 and 129 mouse lines are the most commonly used background strains in research. However, these two strains show markedly differences in learning and memory, anxiety and sensitivity to drugs (Homanics et al., 1999; Contet et al., 2001; Cook et al., 2001, 2002; Murphy et al., 2001; Bouwknecht and Paylor, 2002). It is worth to mention that, in the light/dark conflict test, C57BL/6 show higher transition frequency and reduced time in the dark compartment compared to the 129 mouse line (Bouwknecht and Paylor, 2002). The second aspect is related to the behavioral procedure used to perform the light/dark conflict test. In the present study, mice were always placed in the light compartment at the beginning of the session (Crawley and Goodwin, 1980; Gao and Cutler, 1992; Bourin and Hascoët, 2003). This contrasts with the study of Choi and colleagues and could explain why different results have been observed between the two studies (Choi et al., 2007). Furthermore, the behavioral responses we observed in the EPM and the open field test strongly support an implication of Cav3.2 T-type calcium channels in anxiety-related behaviors. Even though our results suggest that the an-xious phenotype observed in KO mice is not associated with repetitive behavior, a study clearly aiming at deciphering the role of T-type calcium channels in OCD-like behavior need to be undertaken.
Calcium-dependent signaling is important for LTP and learning and memory functions. NMDA-independent influx of Ca2+ through VGCCs has been shown to be crucial for both hippocampus- and amygdala-dependent tasks. Thus, HVA L-type Cav1.2 and Cav1.3 mutant mice showed altered spatial memory and impaired consolidation of fear conditioning, respectively (Moosmang et al., 2005; McKinney and Murphy, 2006; White et al., 2008). In addition, ablation of HVA P/Q-type Ca2+ channels impaired memory functions (Mallmann et al., 2013). Interestingly, disruption of the T-type calcium channel activity has been reported to strongly alter physiological induction and maintenance of LTP in the hippocampus, visual cortex and cerebellum (Yoshimura et al., 2008; Chen et al., 2012; Ly et al., 2013) and mutations of the human cacna1h gene have been associated with autism spectrum disorder (ASD; Splawski et al., 2006). Moreover, the demonstration that T-type calcium channels are interacting with the neurotransmitter release machinery reinforces the notion of their impact on synaptic transmission (Tang et al., 2011; Weiss et al., 2012a,b). Our study further highlights the emerging role of Cav3.2 channels in learning and memory functions. Thus, while the working memory function is spared, memory recognition assessed by using the NOR and SOR is strongly impaired in Cav3.2 deficient mice. This impairment is not the result of a deficit of exploratory drive since Cav3.2 null mice and WT animals showed similar object exploration during the familiarization phases of the two behavioral tasks. In contrast, in the Morris water maze, memory formation and retrieval are not altered in Cav3.2 deficient mice (Chen et al., 2012). In addition, we also found that, although less efficient, Cav3.2 null mice were able to acquired motor skills suggesting the neural systems involved in these processes are functional. Altogether these functional data strongly suggest that T-type Cav3.2 channels influence a subset of specific learning and memory processes.
The contribution of VGCCs in the action of psychostimulant drugs has been extensively documented (Karler et al., 1991; Ansah et al., 1993; Schechter, 1993; Pierce and Kalivas, 1997; Pierce et al., 1998; Licata et al., 2000; Newton et al., 2004, 2008; Rajadhyaksha and Kosofsky, 2005; Chartoff et al., 2006; Bisagno et al., 2010; Giordano et al., 2010). However, little is known about the contribution of T-type calcium channels. Here, we found that T-type Cav3.2 channels are necessary for acute psychomotor effects induced by cocaine and d-amphetamine. These data extend previous results obtained in rats showing that the pharmacological blockade of T-type calcium channels achieved with TTA-A2, strongly reduced d-amphetamine-induced psychomotor effects (Bisagno et al., 2010; Uslaner et al., 2012). Interestingly, this initial study suggested that this effect would result from an alteration of the glutamatergic transmission since TTA-A2 inhibits glutamate release in the nucleus accumbens leaving unaltered dopamine influx (Uslaner et al., 2012). However, functional coupling of T-type currents to calcium-activated-potassium channels has been proposed to modify firing pattern of midbrain DA neurons (Wolfart and Roeper, 2002), a mechanism that may contribute to reward-based processes.
Our study also highlights that T-type Cav3.2 channels participate to psychostimulant-induced development and expression of drug sensitization. Interestingly, previous studies pointed out that Cav1.3 L-type calcium channels (LTCCs) mediate development of sensitization whereas Cav1.2 LTCCs mediate expression of the psychostimulant-induced sensitized response (Giordano et al., 2010). Furthermore, our pharmacological experiment, using TTA-A2, strongly suggests that the Cav3.2 channel is the only member of the T-type family required for the expression of sensitization, a crucial finding for future relevance in clinical treatments of addictions. Given the high expression of Cav3.2 mRNA in the striatum, the nucleus accumbens and other nuclei that composed the rewarding systems (Talley et al., 1999), it is tempting to speculate that the effects observed are associated with altered functions of this T-type isoform within the circuit. Future experiments are necessary to identify whether the altered acute responses are associated with the function of the Cav3.2 channel in a particular cell-type of the system mentioned above.
Follow up explorations would be needed in the next future to precise the cellular basis of the underlying circuits involved, especially when cell and tissue specific conditional knockout mice will be available. Moreover, elucidation of the cellular and subcellular Cav3.2 expression patterns is still pending to the availability of faithful antibodies. We should also mention that brain regions (i.e., cortex, hippocampus, striatum, midbrain) known to be important for the behaviors here explored express high level of Cav3.2 transcripts (Talley et al., 1999), and that functional presence of Cav3.2 like T-type currents is well established in these regions (Perez-Reyes, 2003).
Our study shows that T-type Cav3.2 calcium channels are strongly involved in affective and cognitive functions. In depth behavioral characterization of Cav3.2 deficient mice revealed an anxiety-like phenotype although general exploratory drive was unaltered. We further demonstrate an impairment of recognition memory functions but intact working memory processing in Cav3.2-ablated mice. In addition, we also provide evidence for an involvement of specific T-type Cav3.2 calcium channels in sensitization processes triggered by commonly abused psychostimulant drugs.
However, it should be mentioned that mechanisms of functional compensations by other T-type calcium channels might occur due to global knockout of Cav3.2 although no compensation in Cav3.1 and Cav3.3 has been detected at the level of the hippocampus (see GEO1).
In conclusion, our data highlight that physiological activity of the specific T-type Cav3.2 calcium channel is required for affective and cognitive behaviors suggesting potential functional implications of this calcium channel in many brain disorders. The emergence of T-type selective pharmacology is therefore very promising and holds many clinical perspectives.
The authors declare that the research was conducted in the absence of any commercial or financial relationships that could be construed as a potential conflict of interest.
We are grateful to Dr. V. Uebele (Merck and Co., Inc.) for the TTA-A2 molecule. This work was supported by Inserm and grants from ATIP-Avenir (Inserm), Sanofi-Aventis R and D, from the Agence Nationale de la Recherche (ANR-2010-JCJC-1412) to Valjent Emmanuel, and by grants from the ANR (ANR-09-MNPS-037) and the AFM (AFM-PainT) to Bourinet Emmanuel. Laffray Sophie is supported by fellowships from the FRM and the Labex ICST.
Anderson, M. P., Mochizuki, T., Xie, J., Fischler, W., Manger, J. P., Talley, E. M., et al. (2005). Thalamic Cav3.1 T-type Ca2+ channel plays a crucial role in stabilizing sleep. Proc. Natl. Acad. Sci. U S A 102, 1743–1748. doi: 10.1073/pnas.0409644102
Ansah, T. A., Wade, L. H., and Shockley, D. C. (1993). Effects of calcium channel entry blockers on cocaine and amphetamine-induced motor activities and toxicities. Life Sci. 53, 1947–1956. doi: 10.1016/0024-3205(93)90016-v
Barrow, J. C., Bieber, K. A. S., Cube, R. V., Mattern, M. C., Reger, T. S., Shu, Y., et al. (2007). Pyridyl amide T-type calcium channels. http://patentscope.wipo.int/search/en/detail.jsf?docId=WO2007120729&recNum=24&docAn=US2007008977&queryString=%28IN/%2522Barrow,%2520James%2522%29%2520&maxRec=52
Berridge, M. J. (1998). Neuronal calcium signaling. Neuron 21, 13–26. doi: 10.1016/S0896-6273(00)80510-3
Beurrier, C., Congar, P., Bioulac, B., and Hammond, C. (1999). Subthalamic nucleus neurons switch from single-spike activity to burst-firing mode. J. Neurosci. 19, 599–609.
Bisagno, V., Raineri, M., Peskin, V., Wikinski, S. I., Uchitel, O. D., Llinas, R. R., et al. (2010). Effects of T-type calcium channel blockers on cocaine-induced hyperlocomotion and thalamocortical GABAergic abnormalities in mice. Psychopharmacology (Berl) 212, 205–214. doi: 10.1007/s00213-010-1947-z
Bourin, M., and Hascoët, M. (2003). The mouse light/dark box test. Eur. J. Pharmacol. 463, 55–65. doi: 10.1016/s0014-2999(03)01274-3
Bourinet, E., Alloui, A., Monteil, A., Barrere, C., Couette, B., Poirot, O., et al. (2005). Silencing of the Cav3.2 T-type calcium channel gene in sensory neurons demonstrates its major role in nociception. EMBO J. 24, 315–324. doi: 10.1038/sj.emboj.7600515
Bourinet, E., Altier, C., Hildebrand, M. E., Trang, T., Salter, M. W., and Zamponi, G. W. (2014). Calcium-permeable ion channels in pain signaling. Physiol. Rev. 94, 81–140. doi: 10.1152/physrev.00023.2013
Bouwknecht, J. A., and Paylor, R. (2002). Behavioral and physiological mouse assays for anxiety: a survey in nine mouse strains. Behav. Brain Res. 136, 489–501. doi: 10.1016/s0166-4328(02)00200-0
Brami-Cherrier, K., Valjent, E., Herve, D., Darragh, J., Corvol, J. C., Pages, C., et al. (2005). Parsing molecular and behavioral effects of cocaine in mitogen- and stress-activated protein kinase-1-deficient mice. J. Neurosci. 25, 11444–11454. doi: 10.1523/jneurosci.1711-05.2005
Chartoff, E. H., Pliakas, A. M., and Carlezon, W. A. Jr. (2006). Microinjection of the L-type calcium channel antagonist diltiazem into the ventral nucleus accumbens shell facilitates cocaine-induced conditioned place preferences. Biol. Psychiatry 59, 1236–1239. doi: 10.1016/j.biopsych.2005.09.024
Chen, C. C., Lamping, K. G., Nuno, D. W., Barresi, R., Prouty, S. J., Lavoie, J. L., et al. (2003). Abnormal coronary function in mice deficient in alpha1H T-type Ca2+ channels. Science 302, 1416–1418. doi: 10.1126/science.1089268
Chen, C. C., Shen, J. W., Chung, N. C., Min, M. Y., Cheng, S. J., and Liu, I. Y. (2012). Retrieval of context-associated memory is dependent on the Ca(v)3.2 T-type calcium channel. PLoS One 7:e29384. doi: 10.1371/journal.pone.0029384
Cheong, E., and Shin, H. S. (2013). T-type Ca2+ channels in normal and abnormal brain functions. Physiol. Rev. 93, 961–992. doi: 10.1152/physrev.00010.2012
Choi, S., Na, H. S., Kim, J., Lee, J., Lee, S., Kim, D., et al. (2007). Attenuated pain responses in mice lacking Ca(V)3.2 T-type channels. Genes Brain Behav. 6, 425–431. doi: 10.1111/j.1601-183x.2006.00268.x
Contet, C., Rawlins, J. N., and Deacon, R. M. (2001). A comparison of 129S2/SvHsd and C57BL/6JOlaHsd mice on a test battery assessing sensorimotor, affective and cognitive behaviours: implications for the study of genetically modified mice. Behav. Brain Res. 124, 33–46. doi: 10.1016/s0166-4328(01)00231-5
Cook, M. N., Bolivar, V. J., McFadyen, M. P., and Flaherty, L. (2002). Behavioral differences among 129 substrains: implications for knockout and transgenic mice. Behav. Neurosci. 116, 600–611. doi: 10.1037/0735-7044.116.4.600
Cook, M. N., Williams, R. W., and Flaherty, L. (2001). Anxiety-related behaviors in the elevated zero-maze are affected by genetic factors and retinal degeneration. Behav. Neurosci. 115, 468–476. doi: 10.1037//0735-7044.115.2.468
Crawley, J., and Goodwin, F. K. (1980). Preliminary report of a simple animal behavior model for the anxiolytic effects of benzodiazepines. Pharmacol. Biochem. Behav. 13, 167–170. doi: 10.1016/0091-3057(80)90067-2
Cribbs, L. L., Gomora, J. C., Daud, A. N., Lee, J. H., and Perez-Reyes, E. (2000). Molecular cloning and functional expression of Ca(v)3.1c, a T-type calcium channel from human brain. FEBS Lett. 466, 54–58. doi: 10.1016/s0014-5793(99)01756-1
Cribbs, L. L., Lee, J. H., Yang, J., Satin, J., Zhang, Y., Daud, A., et al. (1998). Cloning and characterization of alpha1H from human heart, a member of the T-type Ca2+ channel gene family. Circ. Res. 83, 103–109. doi: 10.1161/01.res.83.1.103
Francois, A., Kerckhove, N., Meleine, M., Alloui, A., Barrere, C., Gelot, A., et al. (2013). State-dependent properties of a new T-type calcium channel blocker enhance Ca(V)3.2 selectivity and support analgesic effects. Pain 154, 283–293. doi: 10.1016/j.pain.2012.10.023
Gangarossa, G., Perroy, J., and Valjent, E. (2013). Combinatorial topography and cell-type specific regulation of the ERK pathway by dopaminergic agonists in the mouse striatum. Brain Struct. Funct. 218, 405–419. doi: 10.1007/s00429-012-0405-6
Gao, B., and Cutler, M. G. (1992). Effects of acute administration of the 5-HT3 receptor antagonist, BRL 46470A, on the behaviour of mice in a two compartment light-dark box and during social interactions in their home cage and an unfamiliar neutral cage. Neuropharmacology 31, 743–748. doi: 10.1016/0028-3908(92)90035-n
Giordano, T. P., Tropea, T. F., Satpute, S. S., Sinnegger-Brauns, M. J., Striessnig, J., Kosofsky, B. E., et al. (2010). Molecular switch from L-type Ca v 1.3 to Ca v 1.2 Ca2+ channel signaling underlies long-term psychostimulant-induced behavioral and molecular plasticity. J. Neurosci. 30, 17051–17062. doi: 10.1523/JNEUROSCI.2255-10.2010
Gomora, J. C., Murbartian, J., Arias, J. M., Lee, J. H., and Perez-Reyes, E. (2002). Cloning and expression of the human T-type channel Ca(v)3.3: insights into prepulse facilitation. Biophys. J. 83, 229–241. doi: 10.1016/s0006-3495(02)75164-3
Heady, T. N., Gomora, J. C., Macdonald, T. L., and Perez-Reyes, E. (2001). Molecular pharmacology of T-type Ca2+ channels. Jpn. J. Pharmacol. 85, 339–350. doi: 10.1254/jjp.85.339
Homanics, G. E., Quinlan, J. J., and Firestone, L. L. (1999). Pharmacologic and behavioral responses of inbred C57BL/6J and strain 129/SvJ mouse lines. Pharmacol. Biochem. Behav. 63, 21–26. doi: 10.1016/s0091-3057(98)00232-9
Hori, Y., Takeda, H., Tsuji, M., and Matsumiya, T. (1998). Differentiation of the inhibitory effects of calcium antagonists on abnormal behaviors induced by methamphetamine or phencyclidine. Pharmacology 56, 165–174. doi: 10.1159/000028195
Huguenard, J. R. (1996). Low-threshold calcium currents in central nervous system neurons. Annu. Rev. Physiol. 58, 329–348. doi: 10.1146/annurev.physiol.58.1.329
Huguenard, J. R., and Prince, D. A. (1994). Intrathalamic rhythmicity studied in vitro: nominal T-current modulation causes robust antioscillatory effects. J. Neurosci. 14, 5485–5502.
Iftinca, M. C., and Zamponi, G. W. (2009). Regulation of neuronal T-type calcium channels. Trends Pharmacol. Sci. 30, 32–40. doi: 10.1016/j.tips.2008.10.004
Karler, R., Turkanis, S. A., Partlow, L. M., and Calder, L. D. (1991). Calcium channel blockers and behavioral sensitization. Life Sci. 49, 165–170. doi: 10.1016/0024-3205(91)90029-b
Kim, D., Park, D., Choi, S., Lee, S., Sun, M., Kim, C., et al. (2003). Thalamic control of visceral nociception mediated by T-type Ca2+ channels. Science 302, 117–119. doi: 10.1126/science.1088886
Kim, D., Song, I., Keum, S., Lee, T., Jeong, M. J., Kim, S. S., et al. (2001). Lack of the burst firing of thalamocortical relay neurons and resistance to absence seizures in mice lacking alpha(1G) T-type Ca(2+) channels. Neuron 31, 35–45. doi: 10.1016/S0896-6273(01)00343-9
Kraus, R. L., Li, Y., Gregan, Y., Gotter, A. L., Uebele, V. N., Fox, S. V., et al. (2010). In vitro characterization of T-type calcium channel antagonist TTA-A2 and in vivo effects on arousal in mice. J. Pharmacol. Exp. Ther. 335, 409–417. doi: 10.1124/jpet.110.171058
Kraus, R. L., Li, Y., Jovanovska, A., and Renger, J. J. (2007). Trazodone inhibits T-type calcium channels. Neuropharmacology 53, 308–317. doi: 10.1016/j.neuropharm.2007.05.011
Lee, J. H., Daud, A. N., Cribbs, L. L., Lacerda, A. E., Pereverzev, A., Klockner, U., et al. (1999). Cloning and expression of a novel member of the low voltage-activated T-type calcium channel family. J. Neurosci. 19, 1912–1921.
Licata, S. C., Freeman, A. Y., Pierce-Bancroft, A. F., and Pierce, R. C. (2000). Repeated stimulation of L-type calcium channels in the rat ventral tegmental area mimics the initiation of behavioral sensitization to cocaine. Psychopharmacology (Berl) 152, 110–118. doi: 10.1007/s002130000518
Llinas, R. R., Choi, S., Urbano, F. J., and Shin, H. S. (2007). Gamma-band deficiency and abnormal thalamocortical activity in P/Q-type channel mutant mice. Proc. Natl. Acad. Sci. U S A 104, 17819–17824. doi: 10.1073/pnas.0707945104
Ly, R., Bouvier, G., Schonewille, M., Arabo, A., Rondi-Reig, L., Lena, C., et al. (2013). T-type channel blockade impairs long-term potentiation at the parallel fiber-Purkinje cell synapse and cerebellar learning. Proc. Natl. Acad. Sci. U S A 110, 20302–20307. doi: 10.1073/pnas.1311686110
Mallmann, R. T., Elgueta, C., Sleman, F., Castonguay, J., Wilmes, T., Van Den Maagdenberg, A., et al. (2013). Ablation of CaV2.1 voltage-gated Ca(2+) channels in mouse forebrain generates multiple cognitive impairments. PLoS One 8:e78598. doi: 10.1371/journal.pone.0078598
McKinney, B. C., and Murphy, G. G. (2006). The L-Type voltage-gated calcium channel Cav1.3 mediates consolidation, but not extinction, of contextually conditioned fear in mice. Learn. Mem. 13, 584–589. doi: 10.1101/lm.279006
Miwa, H., Koh, J., Kajimoto, Y., and Kondo, T. (2011). Effects of T-type calcium channel blockers on a parkinsonian tremor model in rats. Pharmacol. Biochem. Behav. 97, 656–659. doi: 10.1016/j.pbb.2010.11.014
Moosmang, S., Haider, N., Klugbauer, N., Adelsberger, H., Langwieser, N., Muller, J., et al. (2005). Role of hippocampal Cav1.2 Ca2+ channels in NMDA receptor-independent synaptic plasticity and spatial memory. J. Neurosci. 25, 9883–9892. doi: 10.1523/jneurosci.1531-05.2005
Murphy, N. P., Lam, H. A., and Maidment, N. T. (2001). A comparison of morphine-induced locomotor activity and mesolimbic dopamine release in C57BL6, 129Sv and DBA2 mice. J. Neurochem. 79, 626–635. doi: 10.1046/j.1471-4159.2001.00599.x
Nelson, M. T., Todorovic, S. M., and Perez-Reyes, E. (2006). The role of T-type calcium channels in epilepsy and pain. Curr. Pharm. Des. 12, 2189–2197. doi: 10.2174/138161206777585184
Newton, P. M., Orr, C. J., Wallace, M. J., Kim, C., Shin, H. S., and Messing, R. O. (2004). Deletion of N-type calcium channels alters ethanol reward and reduces ethanol consumption in mice. J. Neurosci. 24, 9862–9869. doi: 10.1523/jneurosci.3446-04.2004
Newton, P. M., Zeng, L., Wang, V., Connolly, J., Wallace, M. J., Kim, C., et al. (2008). A blocker of N- and T-type voltage-gated calcium channels attenuates ethanol-induced intoxication, place preference, self-administration and reinstatement. J. Neurosci. 28, 11712–11719. doi: 10.1523/JNEUROSCI.3621-08.2008
Nilius, B., Talavera, K., and Verkhratsky, A. (2006). T-type calcium channels: the never ending story. Cell Calcium 40, 81–88. doi: 10.1016/j.ceca.2006.04.011
Park, Y. G., Kim, J., and Kim, D. (2013). The potential roles of T-type Ca2+ channels in motor coordination. Front. Neural Circuits 7:172. doi: 10.3389/fncir.2013.00172
Park, Y. G., Park, H. Y., Lee, C. J., Choi, S., Jo, S., Choi, H., et al. (2010). Ca(V)3.1 is a tremor rhythm pacemaker in the inferior olive. Proc. Natl. Acad. Sci. U S A 107, 10731–10736. doi: 10.1073/pnas.1002995107
Perez-Reyes, E. (2003). Molecular physiology of low-voltage-activated t-type calcium channels. Physiol. Rev. 83, 117–161. doi: 10.1152/physrev.00018.2002
Pierce, R. C., and Kalivas, P. W. (1997). Repeated cocaine modifies the mechanism by which amphetamine releases dopamine. J. Neurosci. 17, 3254–3261.
Pierce, R. C., Quick, E. A., Reeder, D. C., Morgan, Z. R., and Kalivas, P. W. (1998). Calcium-mediated second messengers modulate the expression of behavioral sensitization to cocaine. J. Pharmacol. Exp. Ther. 286, 1171–1176.
Popoli, P., Pezzola, A., and Scotti de Carolis, A. (1993). Influence of non-L-type calcium channel antagonists on phencyclidine-induced effects in rats. Life Sci. 52, 2055–2061. doi: 10.1016/0024-3205(93)90690-5
Rajadhyaksha, A. M., and Kosofsky, B. E. (2005). Psychostimulants, L-type calcium channels, kinases and phosphatases. Neuroscientist 11, 494–502. doi: 10.1177/1073858405278236
Santi, C. M., Cayabyab, F. S., Sutton, K. G., Mcrory, J. E., Mezeyova, J., Hamming, K. S., et al. (2002). Differential inhibition of T-type calcium channels by neuroleptics. J. Neurosci. 22, 396–403.
Schechter, M. D. (1993). Cocaine discrimination is attenuated by isradipine and CGS 10746B. Pharmacol. Biochem. Behav. 44, 661–664. doi: 10.1016/0091-3057(93)90183-t
Shin, H. S., Cheong, E. J., Choi, S., Lee, J., and Na, H. S. (2008). T-type Ca2+ channels as therapeutic targets in the nervous system. Curr. Opin. Pharmacol. 8, 33–41. doi: 10.1016/j.coph.2007.12.003
Shipe, W. D., Barrow, J. C., Yang, Z. Q., Lindsley, C. W., Yang, F. V., Schlegel, K. A., et al. (2008). Design, synthesis, and evaluation of a novel 4-aminomethyl-4-fluoropiperidine as a T-type Ca2+ channel antagonist. J. Med. Chem. 51, 3692–3695. doi: 10.1021/jm800419w
Splawski, I., Yoo, D. S., Stotz, S. C., Cherry, A., Clapham, D. E., and Keating, M. T. (2006). CACNA1H mutations in autism spectrum disorders. J. Biol. Chem. 281, 22085–22091. doi: 10.1074/jbc.m603316200
Tai, C. H., Yang, Y. C., Pan, M. K., Huang, C. S., and Kuo, C. C. (2011). Modulation of subthalamic T-type Ca(2+) channels remedies locomotor deficits in a rat model of Parkinson disease. J. Clin. Invest. 121, 3289–3305. doi: 10.1172/JCI46482
Talley, E. M., Cribbs, L. L., Lee, J. H., Daud, A., Perez-Reyes, E., and Bayliss, D. A. (1999). Differential distribution of three members of a gene family encoding low voltage-activated (T-type) calcium channels. J. Neurosci. 19, 1895–1911.
Tang, A. H., Karson, M. A., Nagode, D. A., Mcintosh, J. M., Uebele, V. N., Renger, J. J., et al. (2011). Nerve terminal nicotinic acetylcholine receptors initiate quantal GABA release from perisomatic interneurons by activating axonal T-type (Cav3) Ca(2)(+) channels and Ca(2)(+) release from stores. J. Neurosci. 31, 13546–13561. doi: 10.1523/JNEUROSCI.2781-11.2011
Traboulsie, A., Chemin, J., Kupfer, E., Nargeot, J., and Lory, P. (2006). T-type calcium channels are inhibited by fluoxetine and its metabolite norfluoxetine. Mol. Pharmacol. 69, 1963–1968. doi: 10.1124/mol.105.020842
Uebele, V. N., Gotter, A. L., Nuss, C. E., Kraus, R. L., Doran, S. M., Garson, S. L., et al. (2009). Antagonism of T-type calcium channels inhibits high-fat diet-induced weight gain in mice. J. Clin. Invest. 119, 1659–1667. doi: 10.1172/JCI36954
Uslaner, J. M., Smith, S. M., Huszar, S. L., Pachmerhiwala, R., Hinchliffe, R. M., Vardigan, J. D., et al. (2012). T-type calcium channel antagonism produces antipsychotic-like effects and reduces stimulant-induced glutamate release in the nucleus accumbens of rats. Neuropharmacology 62, 1413–1421. doi: 10.1016/j.neuropharm.2010.11.015
Weiss, N., Hameed, S., Fernandez-Fernandez, J. M., Fablet, K., Karmazinova, M., Poillot, C., et al. (2012a). A Ca(v)3.2/syntaxin-1A signaling complex controls T-type channel activity and low-threshold exocytosis. J. Biol. Chem. 287, 2810–2818. doi: 10.1074/jbc.M111.290882
Weiss, N., Zamponi, G. W., and De Waard, M. (2012b). How do T-type calcium channels control low-threshold exocytosis? Commun. Integr. Biol. 5, 377–380. doi: 10.4161/cib.19997
White, J. A., Mckinney, B. C., John, M. C., Powers, P. A., Kamp, T. J., and Murphy, G. G. (2008). Conditional forebrain deletion of the L-type calcium channel Ca V 1.2 disrupts remote spatial memories in mice. Learn. Mem. 15, 1–5. doi: 10.1101/lm.773208
Wolfart, J., and Roeper, J. (2002). Selective coupling of T-type calcium channels to SK potassium channels prevents intrinsic bursting in dopaminergic midbrain neurons. J. Neurosci. 22, 3404–3413.
Yang, Z. Q., Barrow, J. C., Shipe, W. D., Schlegel, K. A., Shu, Y., Yang, F. V., et al. (2008). Discovery of 1,4-substituted piperidines as potent and selective inhibitors of T-type calcium channels. J. Med. Chem. 51, 6471–6477. doi: 10.1021/jm800830n
Yoshimura, Y., Inaba, M., Yamada, K., Kurotani, T., Begum, T., Reza, F., et al. (2008). Involvement of T-type Ca2+ channels in the potentiation of synaptic and visual responses during the critical period in rat visual cortex. Eur. J. Neurosci. 28, 730–743. doi: 10.1111/j.1460-9568.2008.06384.x
Keywords: T-type Ca2+ channels, anxiety, memory and learning, psychostimulants, drugs of abuse, spontaneous behavior
Citation: Gangarossa G, Laffray S, Bourinet E and Valjent E (2014) T-type calcium channel Cav3.2 deficient mice show elevated anxiety, impaired memory and reduced sensitivity to psychostimulants. Front. Behav. Neurosci. 8:92. doi: 10.3389/fnbeh.2014.00092
Received: 15 January 2014; Accepted: 03 March 2014;
Published online: 18 March 2014.
Edited by:
Benjamin Adam Samuels, Columbia University/Research Foundation for Mental Hygeine, USAReviewed by:
Gerald Zamponi, University of Calgary, CanadaCopyright © 2014 Gangarossa, Laffray, Bourinet and Valjent. This is an open-access article distributed under the terms of the Creative Commons Attribution License (CC BY). The use, distribution or reproduction in other forums is permitted, provided the original author(s) or licensor are credited and that the original publication in this journal is cited, in accordance with accepted academic practice. No use, distribution or reproduction is permitted which does not comply with these terms.
*Correspondence: Gangarossa Giuseppe and Valjent Emmanuel, Institut de Génomique Fonctionnelle, CNRS, UMR-5203, INSERM U661, Montpellier F-34094, France e-mail:cGVwcGUuZ2FuZ2FAZ21haWwuY29t;
ZW1tYW51ZWwudmFsamVudEBnbWFpbC5jb20=
Disclaimer: All claims expressed in this article are solely those of the authors and do not necessarily represent those of their affiliated organizations, or those of the publisher, the editors and the reviewers. Any product that may be evaluated in this article or claim that may be made by its manufacturer is not guaranteed or endorsed by the publisher.
Research integrity at Frontiers
Learn more about the work of our research integrity team to safeguard the quality of each article we publish.