- 1 Department of Psychology, University of Virginia, Charlottesville, VA, USA
- 2 Department of Biology, University of Virginia, Charlottesville, VA, USA
- 3 Department of Neuroscience, Tufts University School of Medicine, Boston, MA, USA
- 4 Department of Cell Biology, Scripps Research Institute, San Diego, CA, USA
It is currently thought that memory formation requires the activation of NMDA receptors (NMDARs) in the hippocampus. However, recent studies indicate that these receptors are not necessary for all forms of learning. The current experiments examine this issue using context fear conditioning in mice. First, we show that context fear can be acquired without NMDAR activation in previously trained animals. Mice trained in one environment (context A) are subsequently able to learn about a second environment (context B) in the presence of NMDAR antagonists. Second, we demonstrate that NMDAR-independent learning requires the hippocampus and is dependent on protein synthesis. However, unlike NMDAR-dependent learning, it is not contingent on the expression of activity-regulated cytoskeleton-associated protein (Arc). Lastly, we present data that suggests NMDAR-independent learning is only observed when recently stimulated neurons are reactivated during conditioning. These data suggest that context fear conditioning modifies synaptic plasticity mechanisms in the hippocampus and allows subsequent learning to occur in the absence of NMDAR activation.
Introduction
The cellular basis of learning has been thoroughly characterized in the hippocampus. The N-methyl-D-aspartate receptor (NMDAR) plays an essential role in this process by mediating calcium influx and initiating intracellular events that lead to gene expression and synaptic strengthening (Elgersma and Silva, 1999; Malenka and Bear, 2004). Surprisingly then, several studies indicate that NMDAR activation is not necessary for learning. For example, spatial learning in the Morris water maze normally requires the activation of NMDARs in the hippocampus (Morris et al., 1986). However, animals that were previously trained on this task are able to acquire new spatial information in the presence of NMDAR antagonists (Bannerman et al., 1995; Saucier and Cain, 1995). The same phenomenon is observed using contextual fear conditioning (Sanders and Fanselow, 2003; Hardt et al., 2009; Wiltgen et al., 2010). Similar to the water maze, prior conditioning permits new context learning to occur in the absence of NMDAR activation. These studies demonstrate that the NMDAR is not necessary for all forms of learning, and suggest that prior behavioral experience alters synaptic plasticity mechanisms in the hippocampus.
Although several studies have observed NMDAR-independent learning, this unique form of conditioning has not been well characterized. The current experiments addressed this issue using context fear conditioning in mice. Animals were trained in two unique environments; context A followed several days later by conditioning in context B. Similar to previous reports, we found that NMDAR antagonists impaired initial learning in context A, but had no effect on subsequent conditioning in context B (Sanders and Fanselow, 2003; Hardt et al., 2009; Wiltgen et al., 2010). Follow-up experiments determined whether NMDAR-independent fear memories are stored in the hippocampus and require the synthesis of new proteins during learning. The unique contribution of activity-regulated cytoskeleton-associated protein (Arc), which is important for both synaptic plasticity and spatial learning, was also examined (Guzowski, 2002). Finally, several experiments were conducted to identify the specific behavioral conditions that give rise to NMDAR-independent context fear learning.
Materials and Methods
Subjects
Male B6129 F1 hybrids (8–12 weeks) from Taconic and TetTag transgenic mice (provided by Mark Mayford) were used in these experiments. Mice were housed two per cage, given free access to food and water and maintained on a 12-h light/dark cycle. Behavioral tests were performed during the light phase of the cycle. Double transgenic fos-histone/gfp mice (TetTag) were generated by crossing hemizygous transgenic mice expressing a gfp-tagged histone protein under control of tetO (tetO-histone/gfp) with hemizygous transgenic mice expressing tetracycline-transactivator (tTA) under control of the c-fos promoter (fos-tTA). The tetO-histone/gfp mice were purchased [The Jackson Laboratory, Bar Harbor, Maine; strain Tg(tetO-HIST1H2BJ/GFP)47Efu/J; stock number 005104]. TetTag animals were maintained in a C57BL/6J background. Generation of fos-tTA mice has been described previously (Reijmers et al., 2007). All experiments were approved by the University of Virginia Animal Research Committee.
Fear Conditioning
Mice were tested in individual conditioning chambers (32 cm wide, 25 cm high, 25 cm deep) encased in white sound-attenuated boxes (63.5 cm wide, 35.5 cm high, 76 cm deep; Med Associates Inc., St. Albans, VT, USA). The top and front of each chamber were made of clear polycarbonate, the back was white acrylic and the sides were stainless steel. Each chamber was equipped with a speaker in the side wall, a stainless steel grid floor (36 rods, each rod 2-mm diameter, 8-mm center to center) and a drop-pan. An overhead LED-based light source provided visible broad spectrum white light and near-infrared light. Video images were recorded via a progressive scan CCD video camera with a visible light filter that was contained within each chamber and connected to a computer in an adjacent room. The freezing response was measured using the automated VideoFreeze system (Med Associates Inc., St. Albans, VT, USA) as described previously (Anagnostaras et al., 2010).
In context A, visible light was turned on, a single grid floor was inserted, and the chambers and pans were cleaned with 95% ethanol prior to conditioning. In context B, visible light was turned off and a black plastic triangular tent translucent to only NIR light was placed inside the chamber. A staggered grid floor was inserted and the chambers and pans were cleaned with Saniwipes (Nice-Pak Products, Inc.). In Figures 3B,C, the Highly Similar context contained the same grid floor as context A, a triangular tent, visible light was turned on, and the chamber and pan were cleaned with 95% ethanol. The moderately similar context was the same as context B.
During training, B6129 mice were placed in the context and allowed to explore for 3 min prior to shock onset. Three shocks (0.5 mA, 2 s) were delivered, each separated by a 30-s intertrial interval. One minute after the final shock, the mice were removed from the conditioning chambers and returned to their homecages. TetTag mice were trained using a similar procedure except that five shocks (0.75 mA, 2 s) were delivered. A pilot experiment revealed that these training parameters were necessary to produce similar freezing levels to those observed in our B6129 mice. In experiments where mice were trained in two contexts, the conditioning sessions were separated by 5 days. Memory was tested 1 day after training by returning mice to the conditioning context for 5 min and measuring the freezing response. In the pre-exposure experiment, mice were allowed to explore the highly similar context for 10 min in the absence of shock. Five days later they were trained and tested in context A as described above.
Intra-Hippocampal Infusions
Mice were anesthetized with isoflurane and mounted in a stereotaxic apparatus (David Kopf Instruments, Tujunga, CA, USA). The scalp of each animal was incised and retracted, and the skull was adjusted to place bregma and lambda in the same horizontal plane. Small burr holes were drilled at the appropriate injection sites. Plastic guide cannule (22 gauge; Plastics One, Roanoke, VA, USA) were inserted bilaterally at the following positions relative to bregma (mm): AP: −2, ML: ±1.5, DV: −1 and affixed with dental cement (Harry J. Bosworth Company, Skokie, IL, USA). Dummy cannulae (28 gauge) were inserted into the guide cannulae following surgery. Mice were allowed to recover for 7–10 days prior to behavioral training in context A. Five days later they were trained in context B. Prior to training in each environment, the mice were lightly anesthetized with isoflurane and the dummy cannulae were removed and replaced with injection cannulae (28 gauge) that projected an additional 1 mm from the tip of the guide cannulae. Twenty minutes later, the NMDAR antagonist APV (Sigma-Aldrich, St. Louis, MO, USA; 2.5 μg/μl, 6.34 nmol/side) or saline (0.9%) was infused into the hippocampus (.5 μl/side; 0.1 μl/min). The injectors were left in place for 2 min after the end of the infusion to allow for diffusion.
Injections
For intraperitoneal (IP) injections, the NMDAR antagonist CPP and protein synthesis inhibitor anisomycin (Sigma-Aldrich, St. Louis, MO, USA) were dissolved in 0.9% saline (pH 7.4). CPP (10 mg/kg) was injected 30 min prior to training. Anisomycin (150 mg/kg) was injected immediately after training and again 2 h later.
Hippocampus Lesions
Mice were anesthetized with isoflurane and mounted in a stereotaxic apparatus (David Kopf Instruments, Tujunga, CA, USA). The scalp was incised and retracted and the skull adjusted to place bregma and lambda in the same horizontal plane. Small burr holes were drilled in the skull for the placement of stainless steel electrodes (size 00 insect pin insulated with Epoxylite except for the 0.5-mm tip). Mice were randomly assigned to receive a lesion or sham surgery. Lesioned mice received bilateral electrolytic lesions of the dorsal hippocampus by passing anodal current (3.0 mA for 5 s) at two sites (AP −1.8, ML ±2.0, DV−2.2). Sham mice were treated similarly but no current was passed.
Histology
Histological verification of cannulae placement and lesion location were performed at the end of behavioral testing. Mice were perfused transcardially with 0.1 M PB followed by 4% paraformaldehyde (PFA). Brains were post-fixed in 4% PFA for 24-h prior to sectioning. Coronal sections (40 μm) were cut on a vibratome and mounted on glass microscope slides. After drying, the sections were stained with cresyl violet to identify neuronal cell bodies. Lesions and cannulae placement were verified by visual inspection of the stained sections reconstructed on the mouse brain atlas (Dong, 2008).
Immunohistochemistry
Sixty minutes following behavioral training, mice were transcardially perfused with 0.1 M PB followed by 4% PFA. Brains were post-fixed in 4% PFA for 24 h. The next day, coronal sections (40 μm) were cut on a vibratome. Free-floating sections were stained using a protocol that combined an extended Avidin–Biotin/peroxidase blocking method, anti-Arc primary antibody, 1:1,000 dilution (SySy, Gottingen, Germany), biotinylated GT anti-rabbit secondary antibody, 1:500 dilution (Vector Laboratories, Burlingame, CA, USA), Cyanine 3 tyramide reagent, 1:50 dilution (Perkin-Elmer, Waltham, MA, USA), and DAPI, 1:500 dilution (Invitrogen, Carlsbad, CA, USA). A similar protocol was used for GFP TetTag section staining, with the exception of replacing Cyanine 3 with a Cyanine 5 tyramide reagent, 1:50 (Perkin-Elmer), to minimize fluorophore spectral overlap.
Widefield Fluorescence Microscopy
Bilateral CA1 images were captured at a Z frequency of 1 μm from five dorsal hippocampus sections per animal, ranging from Bregma −1.35 to −2.35 mm, using the 40× objective of a Nikon Eclipse 80i epifluorescence microscope and Nikon NIS Elements software (Nikon, Melville, NY, USA). The middle-most 1 μm step from each image was used to quantify the number of cells expressing nuclear Arc labeling as positive signals. A Nikon Elements macro was created to identify potential signals in the Cy3 channel as regions of interest (ROI) based on Arc signal intensity (>2 SD above mean intensity of Cy3 channel), size (≤μm2 area of a single DAPI-labeled nuclei), and a circularity factor similar to that of cell nuclei. Each ROI’s Z position (relative to adjacent cells) and signal quality were examined before a rater assigned cells as Arc positive. All quantification was performed by a rater blind to group assignment. DAPI cell bodies specific to the central-most plane were also counted. Total Arc-signals/total DAPI cell bodies were individually calculated for each CA1 image, and converted to percent positive cells.
Confocal Microscopy
GFP CA1 image stacks were taken at a Z frequency of 2 μm using a HCX PL APO CS 40X × 1.25 NA oil immersion objective and Leica SP5X Confocal Microscope fitted with a white light laser and laser diode module at 405 nm. Imaging parameters were optimized for the detection of DAPI-labeled cell bodies, Cy5 labeled Arc protein, and endogenous GFP with the avoidance of saturation. Pin hole (67.89 μm), PMT, laser power, gain (650), and offset settings were kept constant across experimental groups. Following collection, the middle-most section of each stack was analyzed for co-localization using the Time Series Analyzer V3c plug-in for Image J. DAPI bodies were first counted manually. GFP and Arc positive cells were then separately counted within their respective channels by tracing each cell expressing nuclear labeling with an ROI. The GFP and Cy5 channel ROI placements were then overlapped, and cells were classified as GFP positive, Arc positive, or GFP + Arc positive. All counting was performed blind to group assignment. Total GFP and Arc positive cells are reported as a percentage of DAPI-labeled cell bodies to account for variation in section size. CA1 images from both hemispheres of five sections were analyzed for each animal, giving a total of 10 images per animal for analysis.
qRT-PCR
Brains were extracted after behavioral training and flash frozen on dry ice. They were then placed in a brain block (kept at −20°C) and sliced at 2 mm sections from which the dorsal hippocampus was microdissected. Total RNA was extracted using an RNeasy Mini Kit and treated with DNase (Qiagen, Valencia, CA, USA) and reverse-transcribed into cDNA using oligo (dT) 20 primers and Superscipt III First-Strand Synthesis System (Invitrogen, Carlsbad, CA, USA). Expression of Arcand c-fos were determined by real-time PCR. Arc and c-fos primers were designed by Primer Express 2.0 (Applied Biosystems) and the following primer sequences were used: Arc, 5′ TATTCAGGCTGGGTCCTGTC 3′ (forward) and 5′ TGGAGCAGCTTATCCAGAGG 3′ (reverse); c-fos, 5′ TCACCCTGCCCCTTCTCA 3′ (forward) and 5′ CACGTTGCTGATGCTCTTGAC 3′ (reverse). Real-Time PCR was performed in an ABI PRISM 7900HT Sequence Detection System (Applied Biosystems, Foster City, CA, USA). Cycling parameters were as follows: initial denaturation at 95°C for 3 min followed by 40 cycles (95°C for 30 s and 60°C for 1 min). The data were quantified using the 2ΔCT method (Schmittgen and Livak, 2008) and mRNA expression was analyzed relative to that observed in homecage controls. The expression of 36B4 and HPRT genes were measured and used as housekeeping controls for all samples.
Statistics
In each experiment, a set of orthogonal contrasts was used to analyze the data (Edwards, 1972). ANOVA was used to analyze main effects and interactions. Post hoc tests and planned contrasts were used to make specific comparisons using Fisher’s PLSD.
Results
NMDAR-Independent Context Fear Learning
Prior studies suggest that context fear can be acquired in the absence of NMDAR activation in previously trained rats (Sanders and Fanselow, 2003; Hardt et al., 2009). We determined if the same effect is observed in mice by training them sequentially in two different environments. Mice were fear conditioned in context A on day 1 and then trained in context B 5 days later. Saline or the NMDAR antagonist APV was infused bilaterally into the dorsal hippocampus immediately before training in context A or context B (Figure 1A). Fear memory was assessed 24-h after each training session by measuring the freezing response (Anagnostaras et al., 2010). Similar to previous reports, we found that infusions of APV were more effective at blocking learning in context A than context B [context × drug interaction F(1, 13) = 9.33, p < 0.05; Figure 1B]. Post hoc tests (Fisher’s PLSD) revealed that APV impaired learning in context A (p = 0.05) but not context B (p > 0.05). These data indicate that NMDAR activation is required for initial learning in context A, but not subsequent learning in context B.
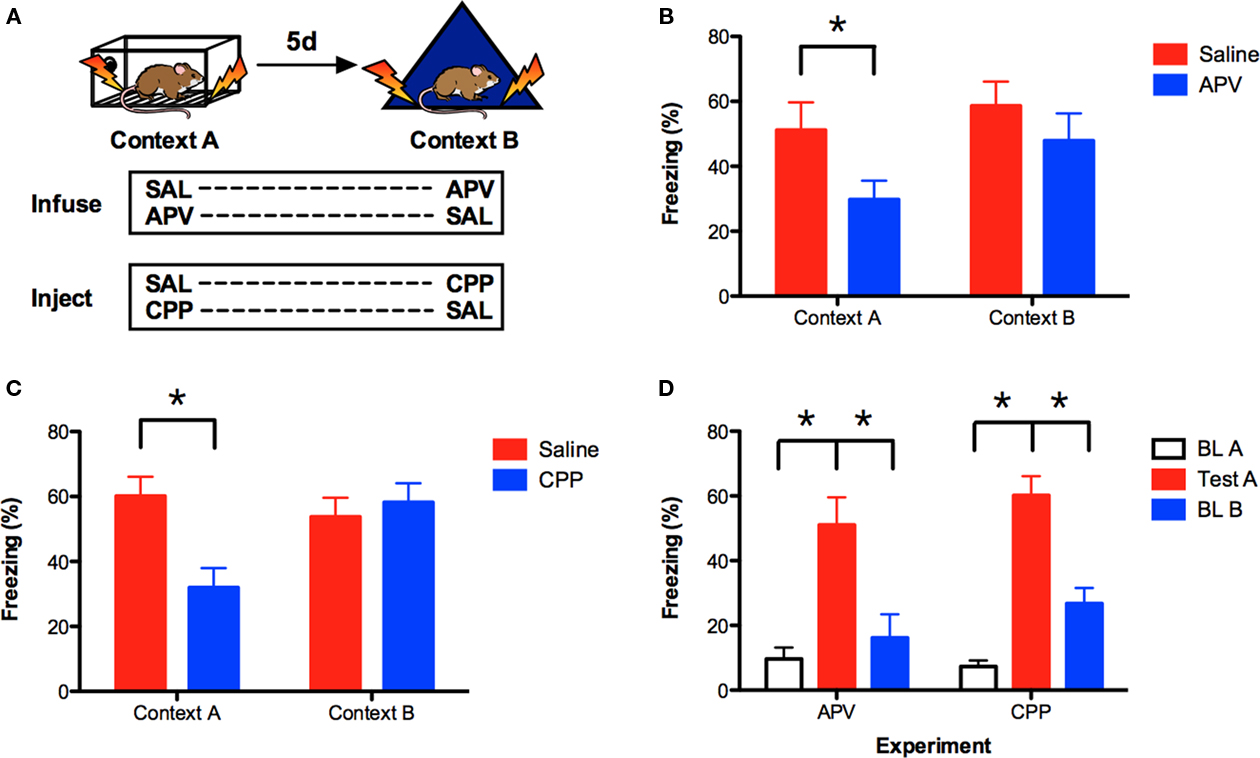
Figure 1. Prior conditioning produces NMDAR-independent context fear learning in the hippocampus. (A) Mice were fear conditioned in context A, and then trained in context B 5 days later. Saline or APV was infused bilaterally into the dorsal hippocampus immediately prior to training in context A (n = 7, n = 8) and context B (n = 8, n = 7). In a separate experiment, saline or CPP was administered IP 30 min prior to training in context A (n = 18, n = 21) and context B (n = 21, n = 18). Contextual fear memory (% freezing) was assessed 24 h after each training session. (B) APV impaired learning in context A but not context B. (C) CPP impaired learning in context A but not context B. (D) Comparison of freezing levels during baseline in context A, testing in context A and baseline in context B for the APV and CPP experiments. These data indicate that mice were able to discriminate between context A and context B. Error bars represent SEM. *p ≤ 0.05.
We next determined if IP injections of the NMDAR antagonist CPP produced the same effect. Mice were fear conditioned using the same behavioral design and IP injections of saline or CPP were administered 30 min prior to each training session. Similar to APV, injections of CPP were more disruptive in context A than context B [context × drug interaction F(1, 37) = 14.31, p < 0.05; Figure 1C]. Post hoc tests (Fisher’s PLSD) found that CPP impaired learning in context A (p < 0.05) but not context B (p > 0.05). These data are consistent with a previous report from our laboratory (Wiltgen et al., 2010).
It is possible that NMDAR antagonists are ineffective in context B because no learning occurs in this environment. This would be the case, for example, if mice were unable to discriminate between context A and context B. To address this issue, we compared the amount of freezing during the test in context A to baseline freezing levels in context B (prior to shock). If mice are unable to discriminate between these contexts, then the amount of freezing should be similar in both environments. Baseline freezing levels in context A are shown for comparison. Only mice that received saline during training in context A were evaluated. As expected, we found that mice in the APV and CPP experiments showed increased freezing during the test session in context A relative to baseline freezing in this same environment (main effect of session F(1, 23) = 63.09, p < 0.05); no experiment × session interaction F < 1). Freezing in context A was also significantly higher than baseline freezing in context B [main effect of context F(1, 23) = 25.95, p < 0.05; no context × experiment interaction F < 1]. Therefore, in both of our experiments, mice were able to discriminate between context A and context B. This suggests that new learning occurs in context B, a finding that is consistent with previous reports (Sanders and Fanselow, 2003; Hardt et al., 2009).
NMDAR-Independent Learning Depends on the Hippocampus
Under some conditions, context fear conditioning does not require the hippocampus (Maren et al., 1997; Frankland et al., 1998; Wiltgen et al., 2006). To ensure that memory for context B depends on this structure, we lesioned the hippocampus after training (Figure 2A). Mice were trained in context A and context B (as described above) and then received electrolytic lesions of the hippocampus 24-h later. Control animals underwent sham surgery. One week after surgery, animals were tested in both contexts. Hippocampus lesions produced significant amnesia for both context A and context B [main effect of lesion F(1, 26) = 39.55, p < 0.05; no context × lesion interaction F(1, 26) = 1.21, p > 0.05; Figure 2B]. Therefore, memory for both environments requires the hippocampus.
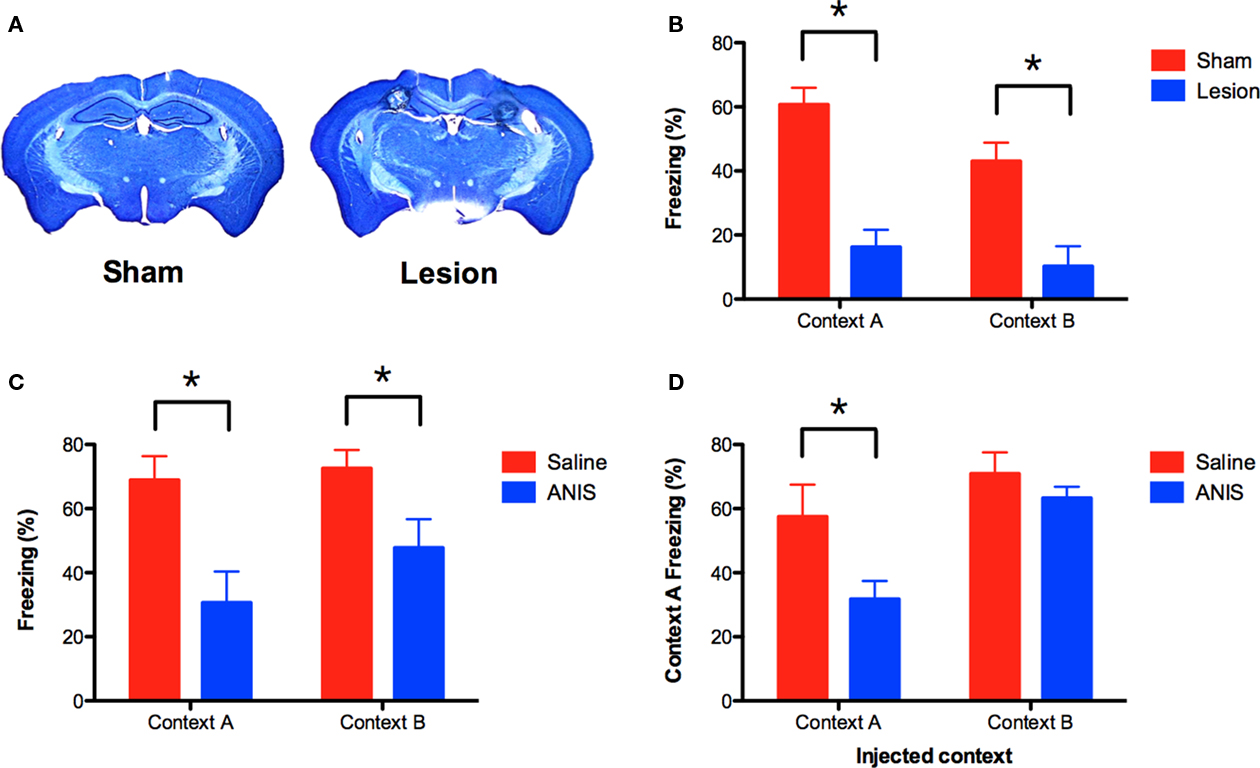
Figure 2. NMDAR-independent learning requires the hippocampus and the synthesis of new proteins. (A) Mice received electrolytic lesions of the hippocampus (n = 14) or sham surgery (n = 14) following completion of the A–B training protocol described in Figure 1A. Mice were tested for fear memory in both contexts 1 week after surgery. (B) Hippocampus lesions produced amnesia for both context A and B. (C) Anisomycin (ANIS) was administered IP after training in context A (n = 8) or context B (n = 10), and impaired memory in both contexts relative to saline controls (n = 8, n = 10). (D) Memory for context A was intact in animals that were injected with ANIS after training in context B. Mice that received ANIS in context A continued to show reduced freezing when re-tested in this context. Error bars represent SEM. *p ≤ 0.05.
NMDAR-Independent Learning Requires Protein Synthesis
Although learning in context B does not depend on NMDAR activation, it may require the synthesis of new proteins. To test this idea, we used the same behavioral design described above and injected the protein synthesis inhibitor anisomycin (ANIS) after training in context A or context B. We found that injections of ANIS impaired memory in both contexts [main effect of drug F(1, 32) = 15.32, p < 0.05, no context × drug interaction F < 1; Figure 2C]. These data indicate that protein synthesis is required for NMDAR-independent learning.
We next determined whether memory for context A remained intact in the mice that received ANIS after training in context B. If these memories are distinct, then blocking memory for context B should not affect memory for context A. To test this idea, we returned all mice to context A for a second memory test. Mice that had received ANIS in context A once again showed a deficit when re-tested in this environment (planned comparison, p < 0.05; Figure 2D, left side). In contrast, mice that had received ANIS after training in context B showed intact memory when tested in context A (planned comparison, p > 0.05; Figure 2D, right side). This result suggests that memory for context A and context B are distinct. If these memories were the same then ANIS injections in context B should also impair memory for context A.
A Model of NMDAR-Independent Learning in the Hippocampus
Using the current data, we developed a model to explain the emergence of NMDAR-independent learning. Training in context A activates and induces plasticity in a subset of hippocampal neurons. The induction of plasticity leads to an increase in synaptic strength and the expression of novel receptor proteins, some of which can mediate plasticity in the absence of NMDAR activation (Plant et al., 2006; Clem et al., 2008; Wiltgen et al., 2010). As a result, recently activated neurons are capable of NMDAR-independent plasticity. If these cells are subsequently reactivated in context B, they are able to mediate learning even in the presence of NMDAR antagonists (Figure 3A, shown in green). In contrast, plasticity in neurons that were not recently activated continues to require NMDAR activation (Figure 3A, shown in red).
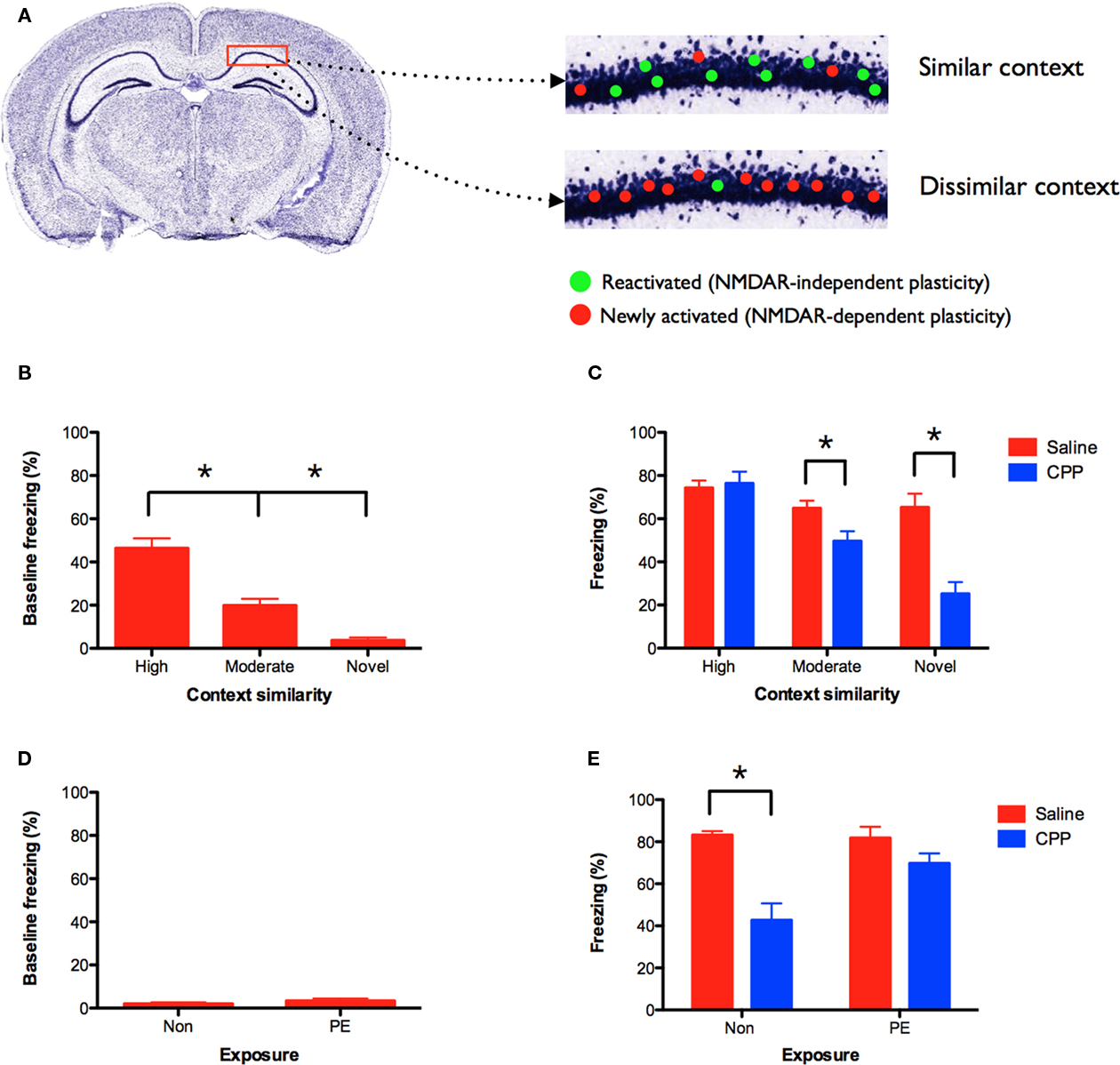
Figure 3. NMDAR-independent learning requires contextual similarity. (A) Model of NMDAR-independent learning: initial training in context A activates and induces NMDAR-dependent plasticity in a subset of hippocampal neurons. If these cells are subsequently reactivated in a similar context, then they are able to mediate learning in the presence of NMDAR antagonists (shown in green). Plasticity in neurons that were not recently activated in context A continues to require NMDAR activation (shown in red). NMDAR-independent learning should therefore be most robust when the training contexts share a degree of similarity. (B) Generalization changes with context similarity. Mice conditioned 5 days prior in an environment highly similar to context A (n = 15) showed the most generalization (i.e., baseline freezing prior to shock). Mice trained in a moderately similar context (n = 15) showed less generalization, and mice that did not receive prior training (novel) (n = 10) showed the least generalization. (C) Following pre-training, mice received CPP or saline injections prior to training in context A. CPP (n = 15) did not affect learning in mice that were previously trained in a highly similar environment relative to saline controls (n = 15). CPP significantly reduced learning in animals that were trained in a moderately similar context (n = 15) and those that received no prior training (n = 10) relative to saline controls (n = 15, n = 10). (D) Mice were pre-exposed to the highly similar context in the absence of shock, and then trained in context A 5-day later in the presence of saline or CPP. Pre-exposed mice (PE; n = 10) showed no baseline freezing in context A and froze at the same level as naïve mice (Non) (n = 10). (E) CPP impaired memory for context A in naïve (Non) (n = 9), but not pre-exposed (PE) mice (n = 10) relative to saline controls (n = 10, n = 10). Error bars represent SEM. *p ≤ 0.05.
According to this model, NMDAR-independent learning in context B depends on the reactivation of recently engaged neurons. In situ hybridization studies have shown that similar environments activate overlapping populations of cells in the hippocampus, while distinct environments activate unique populations (Guzowski et al., 1999; Vazdarjanova and Guzowski, 2004). Therefore, if context B is similar to context A, a number of neurons that are capable of NMDAR-independent plasticity will be reactivated (Figure 3A, similar context). These neurons will not be reactivated if context A and B are completely distinct (Figure 3A, dissimilar context). Consequently, NMDAR-independent learning should be most robust when the training contexts share a degree of similarity.
To examine this idea we trained three groups of mice. Two groups underwent context fear conditioning on day 1. One group was trained in an environment that was highly similar to context A. The other group was conditioned in an environment that was moderately similar to context A (see Materials and Methods for details). A third group received no conditioning on day 1. Five days later all three groups were trained in context A. As expected, mice that were previously fear conditioned in a highly similar environment showed the most generalization to context A (i.e., baseline freezing prior to shock; Figure 3B). Mice that were previously trained in a moderately similar context showed less generalization, and animals that did not receive prior training (novel) showed the least amount of generalization [main effect of context F(2, 37) = 23.56, p < 0.05; Fisher’s PLSD, Highly similar > Moderately similar > Novel, all p values < 0.05]. Using the same behavioral design, a separate set of mice received CPP injections prior to training in context A. When tested the following day, we found that CPP did not affect learning in mice that were previously trained in a highly similar environment (no effect of drug F < 1; Figure 3C). In contrast, CPP significantly reduced learning in mice that were previously trained in a moderately similar context (main effect of drug F(1, 28) = 7.127, p < 0.05) and in mice that received no prior training (main effect of drug F(1, 18) = 22.945, p < 0.05). These data are consistent with our model and suggest that NMDAR-independent learning is contingent on contextual similarity. NMDAR activation is not required for learning when training experiences are similar.
A potential complication with this experiment is that the groups showed different levels of baseline freezing in context A. It is possible that CPP-induced memory impairments are difficult to observe when freezing levels are close to ceiling. To avoid this problem we pre-exposed mice to the highly similar context in the absence of shock. Previous work has shown that exposure to a novel environment produces robust plasticity and learning in the hippocampus (Guzowski et al., 1999; Wiltgen et al., 2001; Wiltgen and Silva, 2007). Pre-exposed mice were then trained in context A (with shock) 5 days later in the presence of saline or CPP. As expected, pre-exposed mice showed no baseline freezing to context A (Figure 3D). They froze at the same level as naïve mice that were not pre-exposed to the context (no effect of exposure F(1, 18) = 1.25, p > 0.05). However, when these groups were tested the following day, we found that CPP did not impair memory for context A in pre-exposed mice, but produced large deficits in naïve animals [exposure × drug interaction F(1, 35) = 7.25, p < 0.05; Figure 3E]. Post hoc tests (Fisher’s PLSD) found that CPP reduced learning in naïve mice (p < 0.05), but not pre-exposed animals (p > 0.05). The difference between these groups cannot be attributed to different levels of baseline freezing in context A. This result demonstrates that prior exposure to a highly similar context (in the absence of shock) produces NMDAR-independent learning.
The Role of Immediate Early Gene Expression in NMDAR-Independent Learning
The immediate early gene (IEG) Arc (activity-regulated cytoskeleton-associated protein) is important for NMDAR-dependent learning and plasticity in the hippocampus (Guzowski, 2002). The current experiments determined whether Arc is also important for NMDAR-independent learning in previously trained animals. We first examined the expression of Arc after conditioning in context A. Training in this context produced a significant increase in Arc protein in the CA1 region of the hippocampus relative to homecage controls (planned comparison, Fisher’s PLSD, p < 0.05; Figures 4A,B). Injection of CPP 30-min prior to training significantly reduced the increase in Arc expression (planned comparison p < 0.05). We also quantified mRNA expression for Arcand another IEG (c-fos) using real-time reverse-transcription PCR (qRT-PCR). Similar to our immunohistochemistry results, conditioning in context A increased the expression of Arc and c-fos mRNA in the hippocampus relative to homecage controls (planned comparison p < 0.05; Figure 4C). This effect was significantly reduced by an injection of CPP (planned comparison p < 0.05).
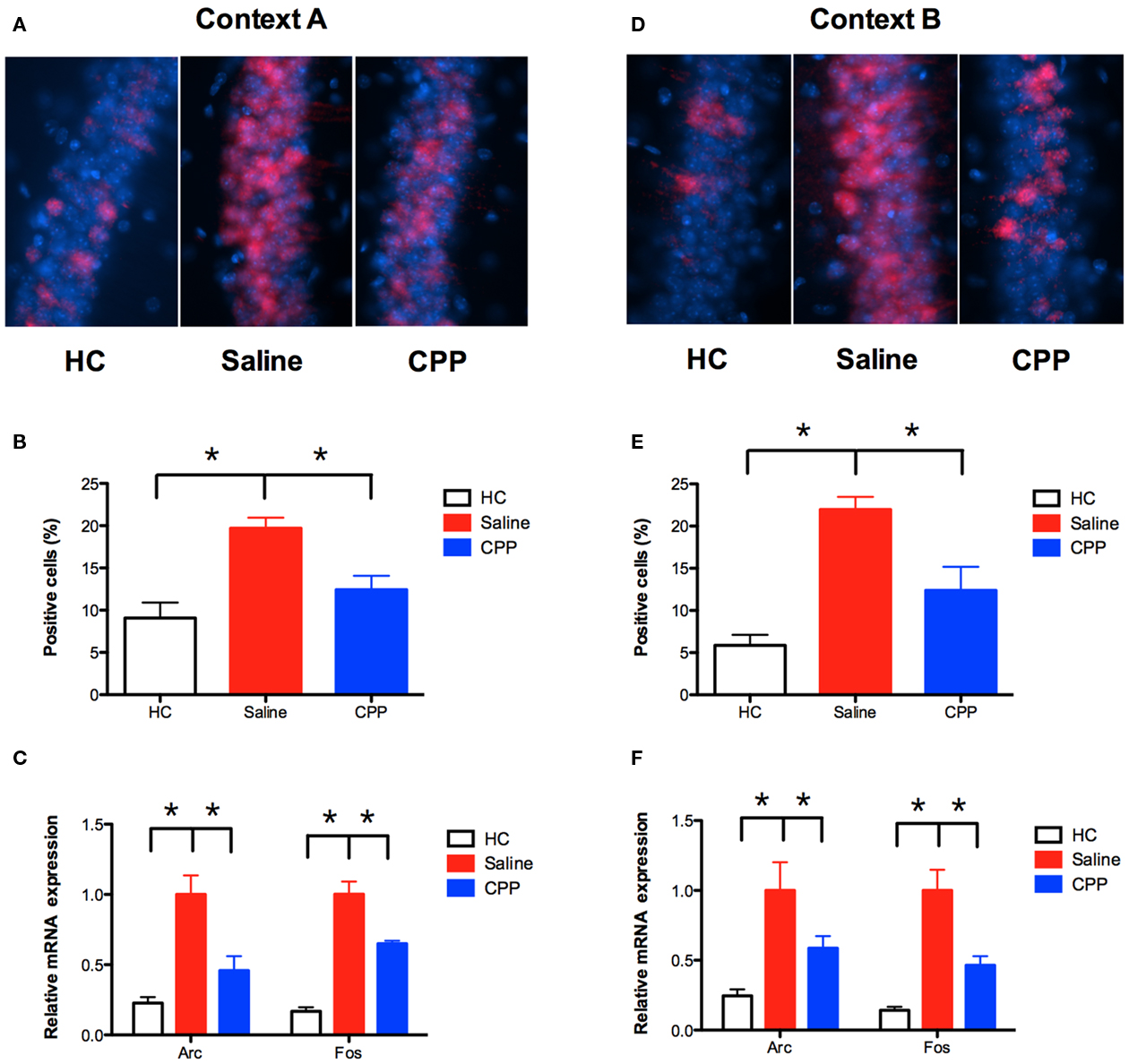
Figure 4. NMDAR activation is required for Arc and c-fos expression in the hippocampus. (A,B) Conditioning in context A significantly increased Arc protein in CA1 (n = 4) relative to homecage controls (HC; n = 4). Arc was detected using CY3 (red) and nuclei were stained with DAPI (blue). Injection of CPP 30 min prior to training (n = 4) significantly reduced Arc expression. Results are shown as percentage of CA1 cell nuclei expressing nuclear Arc labeling. (C) Conditioning in context A (n = 11) increased the expression of Arc and c-fos mRNA in the hippocampus relative to homecage controls (n = 8), as detected by qRT-PCR. This effect was reduced by an injection of CPP (n = 11) prior to training. (D,E) Mice trained in A, and context B 5 days later also demonstrated increased expression of Arc in CA1 (n = 4) relative to homecage controls (n = 2). This increase was reduced by an injection of CPP prior to training (n = 5). (F) Conditioning in context B (n = 6) increased the expression of Arc and c-fos mRNA relative to homecage controls (n = 8), as detected by qRT-PCR. This increase was reduced by an injection of CPP (n = 6) prior to training. Error bars represent SEM. *p ≤ 0.05.
Next, we examined the effects of NMDAR inactivation on Arc expression in context B. Mice were trained in context A, followed 5 days later by conditioning in context B. Training in context B produced an increase in the expression of Arc protein in the CA1 region of the hippocampus (planned comparison p < 0.05; Figures 4D,E). Similar to context A, the increase was reduced by an injection of CPP made prior to training (planned comparison p < 0.05). Consistent with these results, qRT-PCR found that conditioning in context B increased the expression of Arc and c-fos mRNA in the hippocampus relative to homecage controls (planned comparison p < 0.05; Figure 4F). This increase was reduced by an injection of CPP (planned comparison p < 0.05). These results indicate that NMDAR activation is necessary for the expression of Arc and c-fos in context B, even though this receptor is not required for learning. The implication is that Arc and c-fos expression are not required for NMDAR-independent learning. However, our model predicts that NMDAR-independent plasticity only occurs in previously activated cells (Figure 3). If this is the case, then CPP may not block IEG expression in context B if quantification is restricted to previously activated neurons. The following experiments examined this idea.
Activated Neurons Can be Identified with GFP in TetTag Mice
The goal of these experiments was to examine Arc expression in neurons that were previously activated by fear conditioning in context A. To accomplish this, we used a TetTag transgenic mouse (FosP-tTA × tetO-HIST1H2BJ/GFP) in which activated neurons are permanently labeled with green fluorescent protein (GFP). The TetTag mouse combines elements of the tetracycline-transactivator (tTA) system for transgene regulation with neuronal activity-induced activation of the c-fos promoter to tag activated neurons (Reijmers et al., 2007). In the absence of doxycycline (DOX), activation of the c-fos promoter leads to expression of HIST1H2BJ/GFP fusion protein, which is stable for several months after induction (unpublished data). In the presence of DOX, GFP expression is prevented. This regulation allows for the selective tagging of neurons activated by learning. To test the feasibility of this system, we raised TetTag animals on DOX chow until 4-days prior to fear conditioning in context A (Figure 5A). Control animals were also taken off of DOX, but remained in their homecages. Immediately after conditioning, all of the mice were put back on high concentration DOX chow to block subsequent expression of GFP. Five days later we perfused the animals and performed immunohistochemistry for GFP. In fear-conditioned mice, there was a significant increase in GFP expression in the CA1 region of the hippocampus relative to homecage controls [main effect of training F(1, 6) = 20.08, p < 0.05; Figure 5B]. This result suggests that neurons activated by learning in context A can be permanently tagged with GFP. In the next experiment, we used this system to determine if GFP labeled neurons are able to express Arc in the presence of CPP.
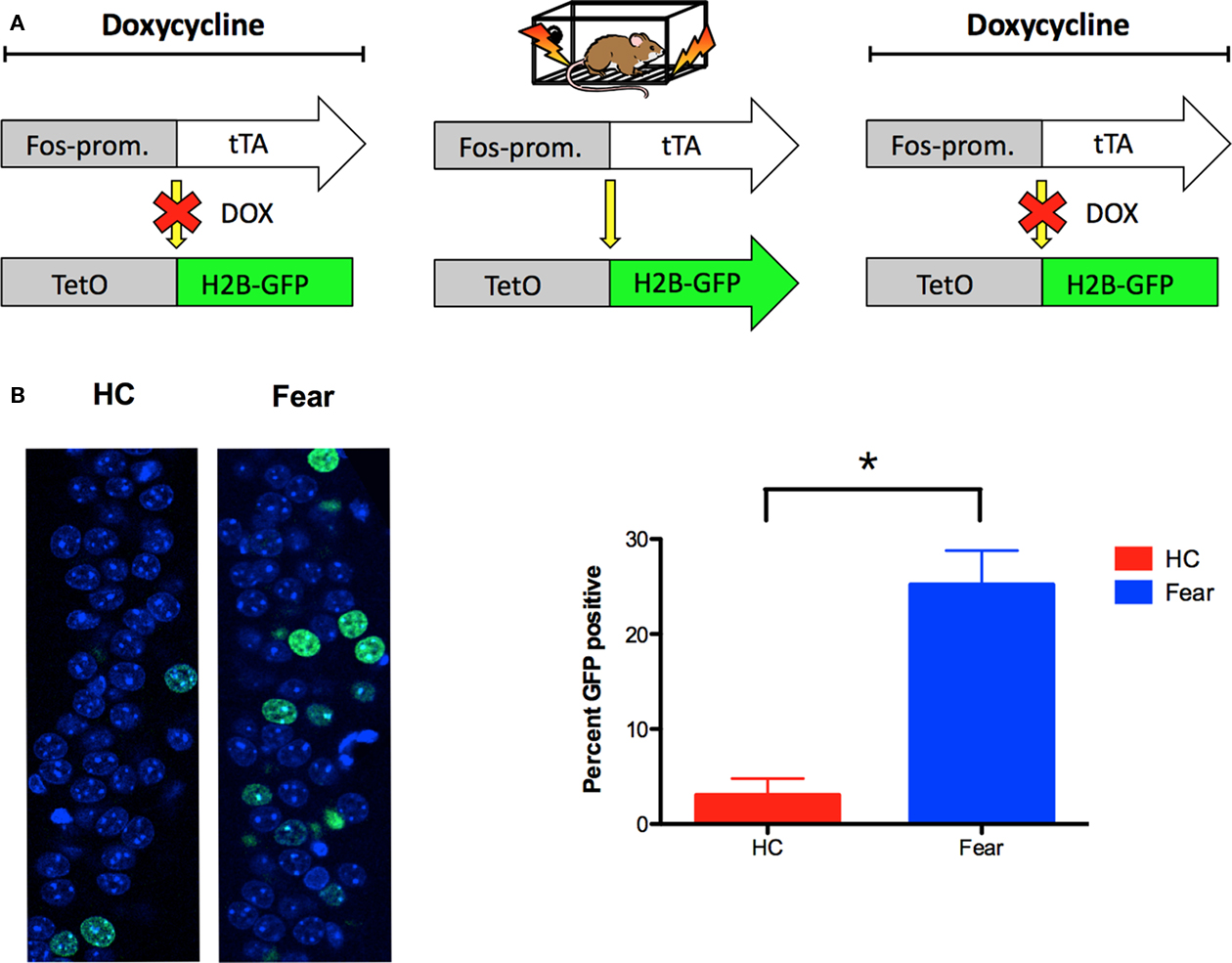
Figure 5. Neurons activated by learning can be tagged with GFP in TetTag mice. (A) The TetTag transgenic mouse combines a tetracycline-transactivator (tTA) system for transgene regulation with neuronal activity-induced activation of the c-fos promoter to tag activated neurons with GFP. In the presence of doxycycline (DOX), GFP expression is suppressed. In the absence of DOX, activation of the c-fos promoter leads to expression of histone-GFP fusion protein, which is stable for several months after induction. (B) Fear-conditioned TetTag mice (n = 5) demonstrated significantly more GFP expression in CA1 relative to off-DOX homecage controls (n = 3). GFP expression was detected 5 days after training (green), and counterstained using DAPI (blue). Results are shown as percentage of sampled CA1 cell nuclei expressing nuclear GFP labeling. Error bars represent SEM. *p ≤ 0.05.
NMDAR Activation is Required for Arc Expression in Previously Activated Neurons
To label neurons activated in context A with GFP, TetTag mice were raised on DOX until 4-days prior to fear conditioning. Immediately after training in context A mice were put on high concentration DOX chow. Testing in context A was not conducted the next day (as in our previous experiments) to avoid potential GFP labeling from this session. Five days later the same mice received an injection of saline or CPP and were conditioned in context B. To index Arc protein expression during this training session, mice were perfused 60 min after training.
Immunohistochemistry for GFP revealed that expression of this protein was increased in both saline and CPP groups relative to HC controls (planned comparisons, p < 0.05; Figure 6A). The amount of GFP labeling did not differ between saline and CPP groups (planned comparison, p > 0.05) because no injections were given prior to learning in context A. In contrast, Arc expression in context B was significantly reduced by injections of CPP as observed in our previous experiment (planned comparison, p < 0.05; Figure 6B). To determine if this reduction was observed in previously activated cells, we analyzed Arc protein separately in GFP positive (+) and GFP negative (−) neurons. We found that CPP reduced the expression of Arc in both GFP+ and GFP− neurons in the CA1 region (planned comparisons, p < 0.05; Figures 6C,E,F). Therefore, NMDAR activation is required for Arc expression even when quantification is restricted to previously activated cells. We also looked at the distribution of Arc expression in saline and CPP mice. We found that the majority of Arc protein was expressed in GFP− neurons (Saline = 64.35%, CPP = 64.92%) relative to GFP + neurons (Saline = 35.65%, CPP = 35.08%) in both groups (Figures 6D,E,F). This result provides further evidence that NMDAR inactivation does not limit Arc expression to previously activated GFP+ cells. If it did, then the majority of Arc protein would be observed in GFP+ cells in mice that received CPP. NMDAR antagonism, therefore, blocks the expression of Arc in context B, even though it does not prevent learning in this environment.
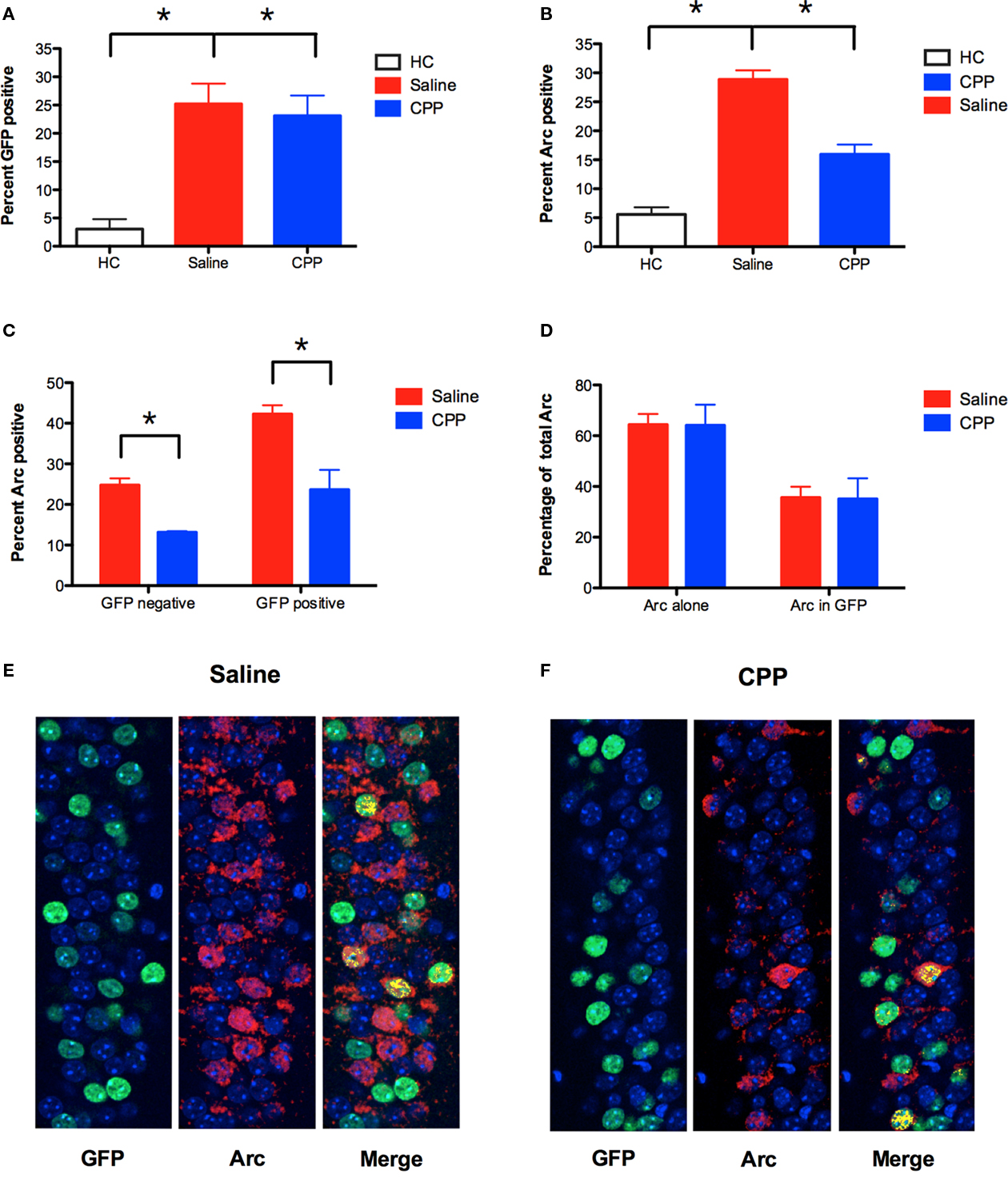
Figure 6. NMDAR activation is required for Arc expression in previously activated neurons. (A) Using the protocol described in Figure 5A, TetTag mice were conditioned off of Dox in context A, and conditioned in context B in the presence of saline (n = 5) or CPP (n = 3) 5 days later. Expression of GFP protein was increased in both saline and CPP groups relative to homecage controls (HC; n = 3). Results are shown as percentage of CA1 cell nuclei expressing GFP labeling. (B) Arc expression in context B was significantly reduced by an injection of CPP prior to training. Results are shown as percentage of CA1 cell nuclei expressing Arc labeling. (C) CPP reduced the expression of Arc in both GFP+ and GFP− neurons in CA1. Results are shown as percentage of GFP+ and GFP− cells that expressed nuclear Arc labeling. (D) Distribution of total Arc expression across GFP+ (Arc in GFP) and GFP− (Arc alone) neurons in saline and CPP injected mice. The majority of Arc protein was expressed in GFP− neurons (Saline = 64.35%, CPP = 64.92%) relative to GFP + neurons (Saline = 35.65%, CPP = 35.08%) in both groups. (E,F) Arc was detected using CY5 (red), and GFP labeling is shown in green. Nuclei expressing both Arc and GFP labeling are pseudo-colored (yellow). Counterstaining was performed using DAPI (blue). Error bars represent SEM. *p ≤ 0.05.
Discussion
NMDAR-mediated plasticity has been strongly linked to learning and memory in the hippocampus. Genetic deletion or pharmacological inactivation of the NMDAR blocks long-term potentiation (LTP) and impairs memory on several spatial and contextual learning tasks (Morris et al., 1986; Young et al., 1994; Tsien et al., 1996; Rampon et al., 2000). Despite these facts, several studies have found that the NMDAR is not always required for learning. This was first observed in the Morris water maze, where spatial pre-training alleviated the learning deficits typically produced by NMDAR antagonists (Bannerman et al., 1995; Saucier and Cain, 1995). We observed the same effect using context fear conditioning. Mice trained in one environment were subsequently able to learn about a second context in the presence of NMDAR antagonists. These data suggest that plasticity mechanisms in the hippocampus are dynamic and can be altered by prior behavioral experience.
NMDAR-independent learning in the water maze only emerged after spatial pre-training. This type of learning was not observed in animals that received non-spatial pre-training (Bannerman et al., 1995). Therefore, spatial tasks induce cellular changes in the hippocampus that alter the way future information is encoded. Based on these data, we hypothesized that NMDAR-independent learning will only occur when new learning activates many of the same neurons that were engaged by prior training. To test this idea, we fear-conditioned mice in two environments that differed in similarity. Recent work indicates that similar contexts activate overlapping populations of neurons in the hippocampus, while distinct environments activate different groups of cells (Vazdarjanova and Guzowski, 2004). Consistent with our hypothesis, we found that NMDAR-independent learning only occurred in contexts that were similar to a previously experienced environment. When contexts were distinct, NMDAR activation was once again required for learning. Taken together, these results suggest that the NMDAR plays a selective role in the encoding of novel environments. NMDAR-independent plasticity mechanisms may be used to encode spatial information that is similar to previously encountered environments.
A potential complication with these results is that new learning may not occur in contexts that are similar to previously experienced environments. If animals are unable to distinguish between context A and B, for example, then no new learning would occur in the latter environment. By this account, NMDAR antagonists are ineffective in context B because no learning occurs in this environment. Our data suggest that this is not the case, however, as mice were clearly able to distinguish between training contexts. Mice trained in context A froze significantly more in this environment than during their first exposure to context B (Figure 1D). We also found that injections of ANIS impaired memory for context B, suggesting that new learning occurred in this environment (Figure 2D). Therefore, consistent with previous studies, our data suggest that new learning can occur in the presence of NMDAR antagonists (Bannerman et al., 1995; Saucier and Cain, 1995; Sanders and Fanselow, 2003; Hardt et al., 2009).
Another possibility is that training in context B induces reconsolidation for the memory formed in context A (Nader et al., 2000; Debiec et al., 2002). However, our ANIS data suggest that this is not the case. In Figure 2C, one group was trained in context A followed 5 days later by training in context B. After training in context B, some animals received anisomycin, which impaired memory for this environment. It is possible that this deficit is due to a reconsolidation effect (i.e., reactivating the memory for context A induces reconsolidation which is blocked by ANIS). However, if this were true, then memory for context A should be impaired in these mice. This was not the case. When these mice were subsequently tested in context A their memory for this environment was normal (Figure 2D, right side). Consistent with our discrimination data, this result suggests that memory for context A and context B are distinct.
Based on the existing data, we propose a model whereby prior training activates a subset of hippocampal neurons and alters the mechanisms by which they become plastic (Figure 3A). This type of metaplasticity was recently observed in the mouse somatosensory cortex. In this system, potentiation of layer 4–2/3 synapses normally requires NMDAR activation. However, if animals receive prior whisker stimulation, then LTP can be produced in the presence of NMDAR antagonists (Clem et al., 2008). Follow-up studies found that whisker stimulation increased the expression of calcium-permeable AMPARs (CP-AMPARs) and metabotropic glutamate receptors (mGluRs), both of which can mediate LTP in the absence of NMDAR activation (Clem and Barth, 2006; Clem et al., 2008; Wiltgen et al., 2010). The influence of behavioral experience on NMDAR-independent plasticity mechanisms has not been examined in the hippocampus. However, recent studies found that LTP induction in this region increases the expression of CP-AMPARs and mGluRs (Plant et al., 2006; Cheyne and Montgomery, 2008; Guire et al., 2008). The contribution of these receptors to subsequent plasticity is unknown, although knockout studies show that CP-AMPARs can mediate LTP and learning in the hippocampus (Yang et al. 2010; Asrar et al., 2009; Wiltgen et al., 2010). Future studies in our laboratory will examine the contribution of these receptors to NMDAR-independent learning in the hippocampus.
The NMDAR is important for both excitatory learning and extinction (Quirk and Mueller, 2008). If NMDARs are blocked during extinction, animals cannot learn to inhibit fear to stimuli that no longer predict danger (Zimmerman and Maren, 2010). Similar to the current results, the role of the NMDAR in this inhibitory form of learning is also modulated by experience. Animals that have previously undergone extinction training do not require NMDAR activation for new extinction learning (a.k.a. re-extinction; Langton and Richardson, 2008, 2010; Laurent et al., 2008; Chan and McNally, 2009). Together with the Morris watermaze and contextual fear conditioning studies, these results suggest that the NMDAR plays a selective role in the acquisition of novel information in many different learning systems. However, in contrast to this idea, two previous studies reported that NMDAR-independent learning does not occur in the amygdala. In these experiments, rats received infusions of APV into the basolateral amygdala prior to fear conditioning (Lee and Kim, 1998; Laurent and Westbrook, 2009). APV was found to block new learning even in previously trained animals. This result is difficult to interpret, however, because APV is known to impair basal synaptic transmission in the amygdala (Li et al., 1995; Maren and Fanselow, 1995). To overcome this limitation, additional studies are needed with agents that block NMDAR-mediated plasticity but do not affect basal synaptic transmission (e.g., ifenprodil; Rodrigues et al., 2001).
Lastly, our studies indicate that NMDAR-independent learning requires the hippocampus and is dependent on the synthesis of new proteins, but is not contingent on the expression of Arc protein. Arc is an IEG that is expressed following NMDAR activation and contributes to plasticity and learning in the hippocampus (Guzowski, 2002). We found that an injection of the NMDAR antagonist CPP significantly reduced Arc and impaired learning in context A. The same injection impaired Arc expression in context B but did not affect learning in this environment.
This suggests that Arc expression is not required for NMDAR-independent learning. Interestingly, some forms of LTP in the hippocampus do not require the expression of Arc protein (Steward et al., 2007; Kuipers et al., 2009). It is possible that these Arc-independent forms of synaptic plasticity contribute to learning in context B. Another possibility is that learning in context B depends on Arc, but requires only a small amount of this protein to be made. In this case, learning will proceed normally as long as a minimal threshold of Arc expression is exceeded. The current study cannot rule out the latter scenario, as CPP did not completely block the expression of Arc protein in our experiments (Figures 4 and 6). To address this issue, subsequent studies will need to directly prevent Arc expression using targeted manipulations (e.g., genetic deletion, antisense oligodeoxynucleotides; Guzowski et al., 2000; Guzowski, 2002; Plath et al., 2006).
Conflict of Interest Statement
The authors declare that the research was conducted in the absence of any commercial or financial relationships that could be construed as a potential conflict of interest.
Acknowledgments
This work was supported by a Jeffress Memorial Trust research grant (Brian J. Wiltgen) and a National Science Foundation (NSF) graduate research fellowship (Kaycie Kuss Tayler). We thank Josselyn Newton, Kathryn O’Donnell, Pratik Patel, Zach Collier, and Ayushma Shrestha for their assistance with immunohistochemistry and Ammasi Periasamy (W. M. Keck Center for Cellular Imaging, University of Virginia, Charlottesville, VA, USA) for microscopy assistance.
References
Anagnostaras, S. G., Wood, S. C., Shuman, T., Cai, D. J., Leduc, A. D., Zurn, K. R., Zurn, J. B., Sage, J. R., and Herrera, G. M. (2010). Automated assessment of pavlovian conditioned freezing and shock reactivity in mice using the video freeze system. Front. Behav. Neurosci. 4:158. doi: 10.3389/fnbeh.2010.00158
Asrar, S., Zhou, Z., Ren, W., and Jia, Z. (2009). Ca2+ permeable AMPA receptor induced long-term potentiation requires PI3/MAP kinases but not Ca/CaM-dependent kinase II. PLoS ONE 4:e4339.
Bannerman, D. M., Good, M. A., Butcher, S. P., Ramsay, M., and Morris, R. G. (1995). Distinct components of spatial learning revealed by prior training and NMDA receptor blockade. Nature 378, 182–186.
Chan, W. Y., and McNally, G. P. (2009). Conditioned stimulus familiarity determines effects of MK-801 on fear extinction. Behav. Neurosci. 123, 303–314.
Cheyne, J. E., and Montgomery, J. M. (2008). Plasticity-dependent changes in metabotropic glutamate receptor expression at excitatory hippocampal synapses. Mol. Cell. Neurosci. 37, 432–439.
Clem, R. L., and Barth, A. (2006). Pathway-specific trafficking of native AMPARs by in vivo experience. Neuron 49, 663–670.
Clem, R. L., Celikel, T., and Barth, A. L. (2008). Ongoing in vivo experience triggers synaptic metaplasticity in the neocortex. Science 319, 101–104.
Debiec, J., LeDoux, J. E., and Nader, K. (2002). Cellular and systems reconsolidation in the hippocampus. Neuron 36, 527–538.
Edwards, A. L. (1972). Experimental Design in Psychological Research, 4th Edn. New York: Holt, Rinehart and Winston, Inc.
Elgersma, Y., and Silva, A. J. (1999). Molecular mechanisms of synaptic plasticity and memory. Curr. Opin. Neurobiol. 9, 209–213.
Frankland, P. W., Cestari, V., Filipkowski, R. K., McDonald, R. J., and Silva, A. J. (1998). The dorsal hippocampus is essential for context discrimination but not for contextual conditioning. Behav. Neurosci. 112, 863–874.
Guire, E. S., Oh, M. C., Soderling, T. R., and Derkach, V. A. (2008). Recruitment of calcium-permeable AMPA receptors during synaptic potentiation is regulated by CaM-kinase I. J. Neurosci. 28, 6000–6009.
Guzowski, J. F. (2002). Insights into immediate-early gene function in hippocampal memory consolidation using antisense oligonucleotide and fluorescent imaging approaches. Hippocampus 12, 86–104.
Guzowski, J. F., Lyford, G. L., Stevenson, G. D., Houston, F. P., McGaugh, J. L., Worley, P. F., and Barnes, C. A. (2000). Inhibition of activity-dependent arc protein expression in the rat hippocampus impairs the maintenance of long-term potentiation and the consolidation of long-term memory. J. Neurosci. 20, 3993–4001.
Guzowski, J. F., McNaughton, B. L., Barnes, C. A., and Worley, P. F. (1999). Environment-specific expression of the immediate-early gene Arc in hippocampal neuronal ensembles. Nat. Neurosci. 2, 1120–1124.
Hardt, O., Wang, S. H., and Nader, K. (2009). Storage or retrieval deficit: the yin and yang of amnesia. Learn. Mem. 16, 224–230.
Kuipers, S. D., Tiron, A., Soule, J., Messaoudi, E., Trentani, A., and Bramham, C. R. (2009). Selective survival and maturation of adult-born dentate granule cells expressing the immediate early gene Arc/Arg3.1. PLoS One 4, e4885. doi: 10.1371/journal.pone.0004885
Langton, J. M., and Richardson, R. (2008). D-cycloserine facilitates extinction the first time but not the second time: an examination of the role of NMDA across the course of repeated extinction sessions. Neuropsychopharmacology 33, 3096–3102.
Langton, J. M., and Richardson, R. (2010). The temporal specificity of the switch from NMDAr-dependent extinction to NMDAr-independent re-extinction. Behav. Brain Res. 208, 646–649.
Laurent, V., Marchand, A. R., and Westbrook, R. F. (2008). The basolateral amygdala is necessary for learning but not relearning extinction of context conditioned fear. Learn. Mem. 15, 304–314.
Laurent, V., and Westbrook, R. F. (2009). Infusion of the NMDA receptor antagonist, DL-APV, into the basolateral amygdala disrupts learning to fear a novel and a familiar context as well as relearning to fear an extinguished context. Learn. Mem. 16, 96–105.
Lee, H., and Kim, J. J. (1998). Amygdalar NMDA receptors are critical for new fear learning in previously fear-conditioned rats. J. Neurosci. 18, 8444–8454.
Li, X. F., Phillips, R., and LeDoux, J. E. (1995). NMDA and non-NMDA receptors contribute to synaptic transmission between the medial geniculate body and the lateral nucleus of the amygdala. Exp. Brain Res. 105, 87–100.
Maren, S., Aharonov, G., and Fanselow, M. S. (1997). Neurotoxic lesions of the dorsal hippocampus and Pavlovian fear conditioning in rats. Behav. Brain Res. 88, 261–274.
Maren, S., and Fanselow, M. S. (1995). Synaptic plasticity in the basolateral amygdala induced by hippocampal formation stimulation in vivo. J. Neurosci. 15, 7548–7564.
Morris, R. G., Anderson, E., Lynch, G. S., and Baudry, M. (1986). Selective impairment of learning and blockade of long-term potentiation by an N -methyl-D-aspartate receptor antagonist, AP5. Nature 319, 774–776.
Nader, K., Schafe, G. E., and Le Doux, J. E. (2000). Fear memories require protein synthesis in the amygdala for reconsolidation after retrieval. Nature 406, 722–726.
Plant, K., Pelkey, K. A., Bortolotto, Z. A., Morita, D., Terashima, A., McBain, C. J., Collingridge, G. L., and Isaac, J. T. (2006). Transient incorporation of native GluR2-lacking AMPA receptors during hippocampal long-term potentiation. Nat. Neurosci. 9, 602–604.
Plath, N., Ohana, O., Dammermann, B., Errington, M. L., Schmitz, D., Gross, C., Mao, X., Engelsberg, A., Mahlke, C., Welzl, H., Kobalz, U., Stawrakakis, A., Fernandez, E., Waltereit, R., Bick-Sander, A., Therstappen, E., Cooke, S. F., Blanquet, V., Wurst, W., Salmen, B., Bösl, M. R., Lipp, H. P., Grant, S. G., Bliss, T. V., Wolfer, D. P., and Kuhl, D. (2006). Arc/Arg3.1 is essential for the consolidation of synaptic plasticity and memories. Neuron 52, 437–444.
Quirk, G. J., and Mueller, D. (2008). Neural mechanisms of extinction learning and retrieval. Neuropsychopharmacology 33, 56–72.
Rampon, C., Tang, Y. P., Goodhouse, J., Shimizu, E., Kyin, M., and Tsien, J. Z. (2000). Enrichment induces structural changes and recovery from nonspatial memory deficits in CA1 NMDAR1-knockout mice. Nat. Neurosci. 3, 238–244.
Reijmers, L. G., Perkins, B. L., Matsuo, N., and Mayford, M. (2007). Localization of a stable neural correlate of associative memory. Science 317, 1230–1233.
Rodrigues, S. M., Schafe, G. E., and LeDoux, J. E. (2001). Intra-amygdala blockade of the NR2B subunit of the NMDA receptor disrupts the acquisition but not the expression of fear conditioning. J. Neurosci. 21, 6889–6896.
Sanders, M. J., and Fanselow, M. S. (2003). Pre-training prevents context fear conditioning deficits produced by hippocampal NMDA receptor blockade. Neurobiol. Learn. Mem. 80, 123–129.
Saucier, D., and Cain, D. P. (1995). Spatial learning without NMDA receptor-dependent long-term potentiation. Nature 378, 186–189.
Schmittgen, T. D., and Livak, K. J. (2008). Analyzing real-time PCR data by the comparative C(T) method. Nat. Protoc. 3, 1101–1108.
Steward, O., Huang, F., and Guzowski, J. F. (2007). A form of perforant path LTP can occur without ERK1/2 phosphorylation or immediate early gene induction. Learn. Mem. 14, 433–445.
Tsien, J. Z., Huerta, P. T., and Tonegawa, S. (1996). The essential role of hippocampal CA1 NMDA receptor-dependent synaptic plasticity in spatial memory. Cell 87, 1327–1338.
Vazdarjanova, A., and Guzowski, J. F. (2004). Differences in hippocampal neuronal population responses to modifications of an environmental context: evidence for distinct, yet complementary, functions of CA3 and CA1 ensembles. J. Neurosci. 24, 6489–6496.
Wiltgen, B. J., Royle, G., Gray, E. E., Abdipranoto, A., Thangthaeng, N., Jacobs, N., Saab, F., Tonegawa, S., Heinemann, S. F., O’Dell, T. J., Fanselow, M. S., and Vissel, B. (2010). A role for calcium-permeable AMPA receptors in synaptic plasticity and learning. PLoS ONE 5, e12818. doi: 10.1371/journal.pone.0012818
Wiltgen, B. J., Sanders, M. J., Anagnostaras, S. G., Sage, J. R., and Fanselow, M. S. (2006). Context fear learning in the absence of the hippocampus. J. Neurosci. 26, 5484–5491.
Wiltgen, B. J., Sanders, M. J., Behne, N. S., and Fanselow, M. S. (2001). Sex differences, context preexposure, and the immediate shock deficit in Pavlovian context conditioning with mice. Behav. Neurosci. 115, 26–32.
Wiltgen, B. J., and Silva, A. J. (2007). Memory for context becomes less specific with time. Learn. Mem. 14, 313–317.
Yang, Y., Wang, X. B., and Zhou, Q. (2010). Perisynaptic GluR2-lacking AMPA receptors control the reversibility of synaptic and spines modifications. Proc. Natl. Acad. Sci. U S A.
Young, S. L., Bohenek, D. L., and Fanselow, M. S. (1994). NMDA processes mediate anterograde amnesia of contextual fear conditioning induced by hippocampal damage: immunization against amnesia by context preexposure. Behav. Neurosci. 108, 19–29.
Keywords: fear, memory, synaptic plasticity, context conditioning, NMDA receptor, metaplasticity, spatial, CA1
Citation: Tayler KK, Lowry E, Tanaka K, Levy B, Reijmers L, Mayford M and Wiltgen BJ (2011) Characterization of NMDAR-independent learning in the hippocampus. Front. Behav. Neurosci. 5:28. doi: 10.3389/fnbeh.2011.00028
Received: 07 March 2011; Accepted: 09 May 2011;
Published online: 18 May 2011.
Edited by:
David M. Diamond, University of South Florida, USAReviewed by:
Phillip R. Zoladz, Ohio Northern University, USASeth Davin Norrholm, Emory University School of Medicine, USA
Richard G. M. Morris, The University of Edinburgh, UK
Copyright: © 2011 Tayler, Lowry, Tanaka, Levy, Reijmers, Mayford and Wiltgen. This is an open-access article subject to a non-exclusive license between the authors and Frontiers Media SA, which permits use, distribution and reproduction in other forums, provided the original authors and source are credited and other Frontiers conditions are complied with.
*Correspondence: Brian J. Wiltgen, Department of Psychology, University of Virginia, Charlottesville, VA 22904, USA. e-mail: bw4fh@virginia.edu