Linking adult olfactory neurogenesis to social behavior
- 1 Laboratory for Perception and Memory, Institut Pasteur, Paris, France
- 2 URA18, Centre National de la Recherche Scientifique, Paris, France
- 3 Laboratory for Quantitative Image Analysis, Institut Pasteur, Paris, France
- 4 URA2582, Centre National de la Recherche Scientifique, Paris, France
- 5 CEA, DSV, iRCM, SCSR, Laboratoire de Radiopathologie, INSERM U967, Fontenay-aux-Roses, France
- 6 Laboratoire d’Ethologie Expérimentale et Comparée, University of Paris 13, Villetaneuse, France
Adult-born neurons arrive to the olfactory bulb (OB) and integrate into the existing circuit throughout life. Despite the prevalence of this phenomenon, its functional impact is still poorly understood. Recent studies point to the importance of newly generated neurons to olfactory learning and memory. Adult neurogenesis is regulated by a variety of factors, notably by instances related to reproductive behavior, such as exposure to mating partners, pregnancy and lactation, and exposure to offspring. To study the contribution of olfactory neurogenesis to maternal behavior and social recognition, here we selectively disrupted OB neurogenesis using focal irradiation of the subventricular zone in adult female mice. We show that reduction of olfactory neurogenesis results in an abnormal social interaction pattern with male, but not female, conspecifics; we suggest that this effect could result from the inability to detect or discriminate male odors and could therefore have implications for the recognition of potential mating partners. Disruption of OB neurogenesis, however, neither impaired maternal-related behaviors, nor did it affect the ability of mothers to discriminate their own progeny from others.
Introduction
Adult neurogenesis is conserved in a variety of animals, ranging from insects to humans (reviewed in Lindsey and Tropepe, 2006; Gould, 2007), suggesting that this phenomenon is important to brain function. In the mammalian brain, two regions have been shown to receive neurons during adulthood: the olfactory bulb (OB) and the dentate gyrus (DG) of the hippocampus (reviewed in Alvarez-Buylla and Garcia-Verdugo, 2002; Li et al., 2009). Adult-born neurons that reach the olfactory system originate from neural precursors in the subventricular zone (SVZ) and migrate to the OB, where they differentiate into periglomerular and granule cells (PGs and GCs, respectively), two types of bulbar interneurons that are primarily GABAergic (Lledo and Saghatelyan, 2005). These newly generated neurons are functionally integrated into the OB circuitry, as demonstrated by recording the activity evoked by their synaptic partners (Carleton et al., 2003; Whitman and Greer, 2007), and by measuring their responses to odor stimulation (Magavi et al., 2005). Moreover, these adult-born granule cells also show unique properties: they exhibit enhanced synaptic plasticity (Nissant et al., 2009) and increased responsiveness to odors (Magavi et al., 2005), when compared to older granule cells.
Recent studies suggest that newly generated neurons play a role in learning and memory of olfactory information. Manipulations that alter the levels of neurogenesis affect olfactory behavior, but the effects depend on the nature of the manipulation as well as on the tasks used to assess olfactory function. For instance, rearing mice in an odor-enriched environment – a manipulation that doubles the number of newly arriving neurons in the OB but not the hippocampus – results in a longer-lasting odor memory (Rochefort et al., 2002). On the other hand, reducing or blocking olfactory neurogenesis affects behavior in a task-dependent manner: while olfactory discrimination ability seems to be unaffected (Imayoshi et al., 2008; Breton-Provencher et al., 2009; Lazarini et al., 2009), deficits in either long or short-term odor memory have been reported (Breton-Provencher et al., 2009; Lazarini et al., 2009; Valley et al., 2009; Sultan et al., 2010). In addition, in both perceptual and associative learning, newly generated neurons are differentially recruited to OB areas responsive to the odors learned (Alonso et al., 2006; Moreno et al., 2009; Sultan et al., 2010), suggesting that these neurons could contribute to changes in odor representations during learning.
A deeper understanding of the functional role of adult neurogenesis could come from studying the physiological conditions in which this process is regulated; such a modulation has been recently described in relation to reproductive behavior. In a study by Shingo et al. (2003) proliferation of neuronal precursors in the SVZ was shown to increase in female mice during the first week of both pregnancy and lactation, resulting in augmented levels of neurogenesis in the OB. This modulation of neurogenesis seems to be mediated solely by prolactin (PRL), a mammalian hormone essential to maternal behavior (Mann and Bridges, 2001). Other conditions regulate the levels of neurogenesis: exposing female mice to male pheromones produces an increase in neurogenesis in the females’ OB. Such male pheromone exposure also impacts female behavior: it is correlated with an advanced onset of maternal behavior (Larsen et al., 2008), and it is required for the establishment or expression of females’ preference for a dominant male (Mak et al., 2007). In addition, a recent study showed that neurogenesis in male OB is upregulated during the interaction of male mice with their offspring (Mak and Weiss, 2010). Notably, the increase of olfactory neurogenesis in all these settings is mediated by PRL.
Reproductive behaviors rely heavily on the use of odor cues. For instance, anosmic female mice are unable to distinguish normal from castrated males (Lin et al., 2005; Keller et al., 2006), and show abnormal hormonal cycles and impaired mating behavior (Vandenbergh, 1973). Olfaction is also fundamental to the establishment of maternal behavior, at least in certain species including sheep (Lévy et al., 1995) and mice (Gandelman et al., 1971; Vandenbergh, 1973). Ewes use odor cues to discriminate their own lambs from others, and provide selective care to their own progeny (Brennan and Kendrick, 2006). Mice, on the other hand, form communal nests and provide maternal care to own and alien pups. Nevertheless, mice are able to discriminate the odor of their pups from others (Ostermeyer and Elwood, 1983), and they have been shown to form nests preferentially with related individuals (Manning et al., 1992); moreover, the interaction with pups during the perinatal period seems essential for progeny recognition (Mak and Weiss, 2010). Signatures that identify different individuals are proposed to be provided by candidate molecules such as MHC peptides (Boehm and Zufall, 2006) or major urinary proteins (MUPs; Hurst, 2009). While MHC peptides are detected by both the main and accessory olfactory systems, MUPs are thought to act through activation of the vomeronasal organ (Morè, 2006; Chamero et al., 2007). Differences in MHC loci result in changes in the profile of peptides found in the urine (Singer et al., 1997). Notably, mice can even discriminate pups that differ only in a single MHC locus (Penn and Potts, 1998; Yamazaki et al., 2000).
Given the importance of olfactory cues to both mate and offspring recognition, it is intriguing that pregnancy and lactation, interaction with pups, and exposure to potential mating partners are all accompanied by the addition of new neurons to the olfactory circuit. Because adult neurogenesis results in a new set of bulbar interneurons, thought to be more responsive than preexisting cells (Magavi et al., 2005), and because interneurons are thought to play an important role in the discriminability of odors (Yokoi et al., 1995; Abraham et al., 2010), we hypothesized that the addition of newly generated neurons that are reaching maturity at the time of parturition could be important for the formation of the maternal bond and recognition of the progeny, both for providing maternal care and for avoiding inbreeding (Gandelman et al., 1971; Barnard and Fitzsimons, 1988; Potts et al., 1991; Manning et al., 1992; Pusey and Wolf, 1996). Here, we sought to investigate the contribution of adult olfactory neurogenesis to both maternal behavior and pup recognition. Using focal irradiation of the SVZ, we disrupted adult bulbar neurogenesis in female mice. We then compared maternal behavior between irradiated and non-irradiated females by daily recording mother–pup home cage interactions, and determining their maternal responsiveness in a retrieval test. To assess the contribution of new neurons to young and adult offspring recognition, we used habituation/dishabituation and social interaction tests. We show that disruption of bulbar neurogenesis resulted in abnormal social interaction patterns with adult males. By contrast, reduced olfactory neurogenesis neither affected maternal behavior nor the ability of female mice to discriminate between their own and alien pups. Thus, adult olfactory neurogenesis does not seem to be essential to the normal establishment or expression of maternal behavior, but our results point to a role of adult neurogenesis in social interaction, and possibly in gender discrimination.
Materials and Methods
Animals
C57BL/6J mice (Janvier, France) were used in this study. Animals were housed in groups of four until mating, and maintained in a 12:12 h light–dark cycle and at constant temperature (23°C). Food and water were available ad libitum. All procedures complied with the European Communities Council directives (86/609/EEC) and European guidelines, and were approved by the Institut Pasteur’s Institutional Animal Welfare Committee.
Focal Irradiation of SVZ
Seven- to eight-week-old female mice were irradiated using a medical Alcyon irradiator (gamma rays 60Co) as described (Lazarini et al., 2009). Briefly, mice were anesthetized with a ketamine (75 mg/kg, Merial) and medetomidine (1 mg/kg, Pfizer) mixture administered intraperitoneally (i.p.) before being placed in a stereotaxic frame (Stoelting) for cranial irradiation. Focal irradiation of the SVZ was achieved by exposing to the rays only a small column comprising the mouse SVZ (3 mm × 11 mm window centered at bregma AP: 1.5 mm), and shielding the rest of the brain and body using lead sheets (Figure 1A). Radiation was delivered at a rate of 1 Gy/min in three sessions of 5 Gy each, equally spaced over the course of 5 days (a total of 15 Gy). After gamma-ray exposure, mice were woken up by an i.p. injection of atipamezole (1 mg/kg, Pfizer). Control female mice underwent the same procedure, except that no radiation was delivered.
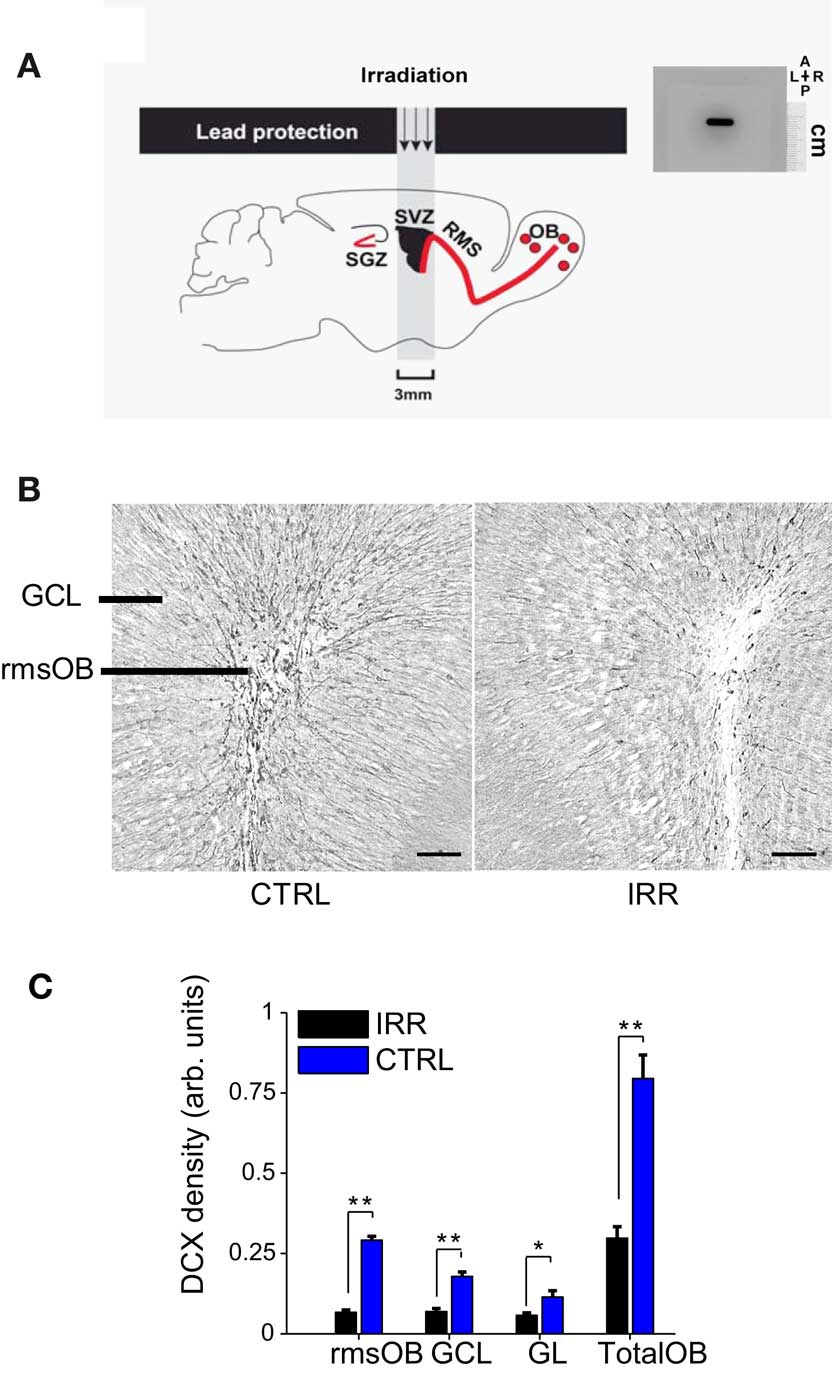
Figure 1. Reduced OB neurogenesis in SVZ-irradiated female mice. (A) Left, targeted irradiation was achieved by exposing a brain area encompassing the SVZ and protecting the rest of the brain with lead shields (see Materials and Methods and Lazarini et al., 2009) SGZ, subgranular zone; RMS, rostral migratory stream. Right, autoradiographic film showing the window of reach of irradiation (black staining). The film was positioned in the irradiator at the same place where mice were placed for irradiation. As shown by the film, irradiation was focal and restricted to a window of 3 mm × 11 mm. A, anterior; P, posterior; L, left; R, right. The scale is indicated. (B) Doublecortin (DCX) immunoreactivity in OB slices of IRR (right panel) and CTRL (left panel) females, 6.5 months after SVZ irradiation. rmsOB, rostral migratory stream at the OB; GCL, granule cell layer; GL, glomerular layer. Images are centered in the rmsOB. Scale bar, 100 μm. (C) DCX staining was quantified as optical density (OD; see Materials and Methods). Data are expressed as mean OD across mice in each treatment (IRR, n = 6; CTRL, n = 6), 6.5 months after irradiation, and for the different OB regions. For each mouse, OD was calculated for six slices and an average value was assigned to that mouse. Error bars represent SEM. *p < 0.05; **p < 0.01 (Mann–Whitney test, see Table A1 in Appendix).
Mice were allowed to recover from the treatment for 6–8 weeks to ensure that the brain recovered from potential inflammation damage occurring shortly after irradiation (Monje et al., 2002), before mating and behavioral experiments. Two groups of animals were used; in each group, one half of the animals was irradiated and the other half was used as controls. Only those animals that carried a successful pregnancy were included in the experiments (Group A, n = 7, Group B, n = 10, of an initial count of 20 and 16 respectively). The time course of the experiments is shown in Figure 2. Briefly, mice in group A were tested for maternal behavior and pup retrieval. The effect of the irradiation was analyzed in these mice by immunochemistry 6.5 months after treatment, at the time of the last behavioral experiment. Group B animals were tested for maternal behavior, pup retrieval, habituation/dishabituation, social interaction, object investigation, and open field.
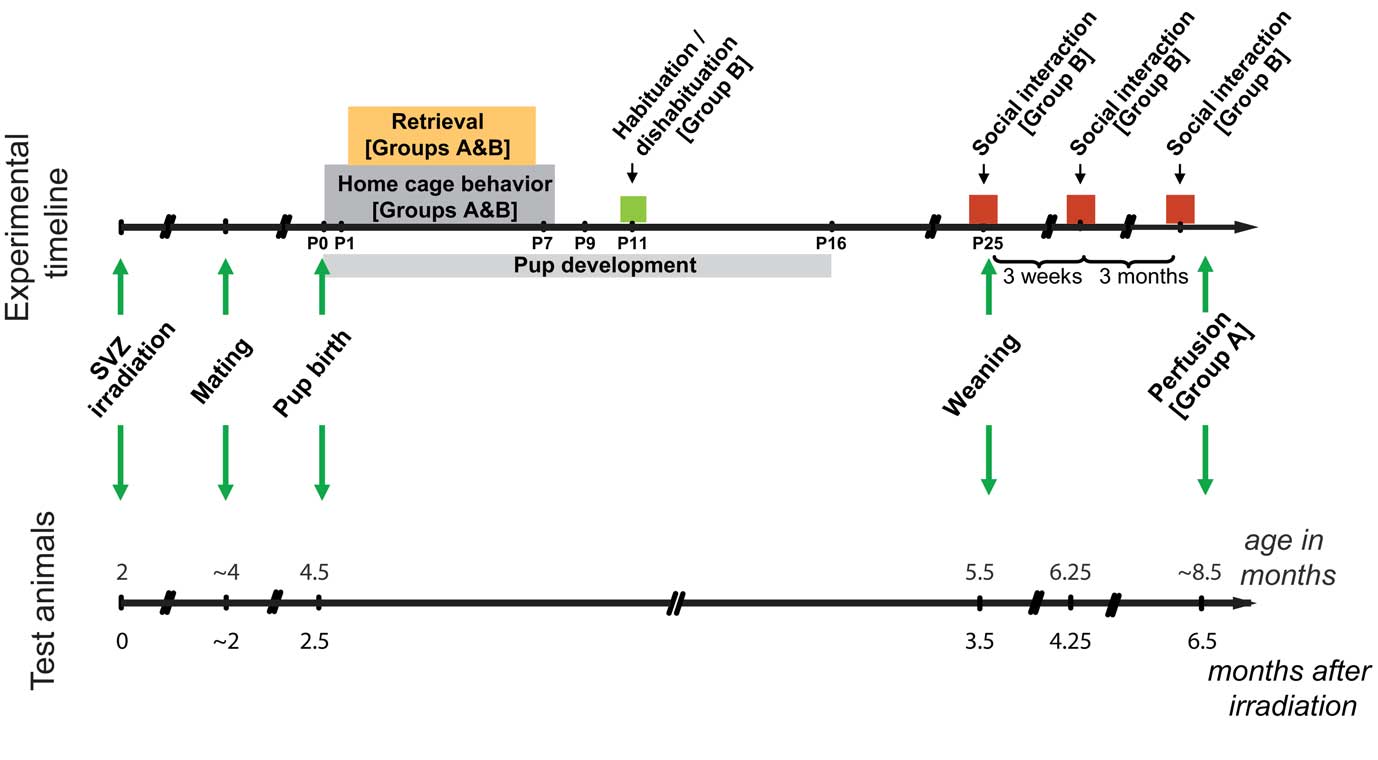
Figure 2. Experimental timeline. Timeline showing the sequence of events and behavioral testing the animals underwent. Color boxes represent behavioral testing; arrows indicate punctual events such as the time of irradiation, mating, birth, and weaning. Two groups of animals were used: all animals in Group B were included in the behavioral testing; for Group A, only a subset of animals was included in the behavior, and the whole group was sacrificed 6.5 months after irradiation for immunohistochemical analysis.
Immunohistochemistry
Mice were deeply anesthetized with sodium pentobarbital (100 mg/kg, Sanofi). Brains were dissected out after transcardiac perfusion with 0.9% NaCl containing heparin (5 × 103 U/ml) followed by a solution of paraformaldehyde (PFA, 4% in phosphate buffer) to fix the tissue. After dissection, brains were stored at 4°C in 4% PFA for a week, and then transferred to phosphate buffer saline (PBS) containing 0.2% sodium azide. Forty-micron thick coronal sections were made using a vibrating microtome (VT1000S, Leica). For doublecortin (DCX) immunohistochemistry, brain sections were first washed in PBS, incubated for 20 min in citrate buffer 0.1 M pH 9.0 at 80°C, and then treated with 0.2% Triton during 2 h. Sections were then incubated with rabbit polyclonal anti-DCX primary antibody (1:2000, Abcam ab18723) in 0.2% Triton, 4% bovine serum albumin (BSA, Sigma) and 2% goat serum overnight at 4°C. Labeled cells were detected using a donkey biotinylated secondary antibody (anti-rabbit IgG, 1:200; 711-065-152, Jackson) and developed using the ABC system (Vector Laboratories) and 3,3′-diaminobenzidine (0.05%, Sigma) as chromogen. Sections were mounted in Depex medium. Reconstructed images of the OB were taken using an Olympus BX51 microscope with a 20× objective and Compix Imaging software (Hamamatsu Photonics).
Doublecortin expression was used to assess the levels of neurogenesis, as DCX is considered a marker of young neurons (Brown et al., 2003). DCX staining was quantified by measuring optical density (OD) using custom-written QUIA software (http://www.bioimageanalysis.org) as previously described (Lazarini et al., 2009). For each animal, six sections 320 μm apart were selected, using the accessory OB as landmark, and quantified. Density was defined as pixels/surface area.
Mating
Prior to mating, virgin female mice (group A, n = 20; group B, n = 16) were exposed to soiled-bedding from male cages for 3 days in order to induce estrous cycle synchronization (the so-called Whitten effect; Whitten, 1966) and increase the chances of simultaneous pregnancy. At the end of the third day, each female was transferred to a new cage and paired with a male of the same strain for 3 days. At the end of this period, females were single-housed in clean cages until 3–4 days before estimated parturition, when they were transferred to clean observation cages (see below) in a separate room. Females were checked every 12 h to determine the day of pup birth (postnatal day 0, or P0). Because exposure to male pheromones induces an increase in SVZ neurogenesis (Mak et al., 2007; Larsen et al., 2008), we took care that all females were exposed to male pheromones or odorants for the same amount of time.
Video Recording
Video was recorded using custom-made software that allows recording video from eight cameras (Fire-i™ digital camera) simultaneously.
Observation of Maternal Behavior in the Home Cage
Pregnant females were single-housed in transparent cages (15 cm × 30 cm × 30 cm) for observation, and were relocated to a separate room with controlled light and temperature (12:12 h light–dark cycle, 23°C). A red, transparent “shelter” was provided to encourage females to build the nest in a fixed location (Figure A1A in Appendix). An observer assessed maternal behavior at the home cage during two 1-h sessions in the “light” phase (10–11 am; 2–3 pm). Observations were carried out during the light phase since mothers, at least in the case of rats, are more likely to nurse their litters during this phase of the day (Champagne et al., 2003). In each session, the behavior of each mother was recorded every 2 min. Observations began the day when birth was detected (P0), and continued until postnatal day 8 (P8) (Figure 2). The recorded behaviors were divided in two main categories, based on previous studies (Myers et al., 1989): behaviors occurring at the nest, or outside the nest. Behavior at nest was further classified as: mother in a nursing posture, regardless of whether pups were attached to the nipples or not, and which could be either active – the mother was arched over the pups in a position that could be considered to require some “effort,” i.e., with the abdomen actively elevated from the floor – or passive – lying on the pups or sideways – ; pups lactating, when at least half of the pups were attached to the nipples; mother licking or grooming the pups; mother self-grooming; mother performing nest maintenance; eating or resting (Figure A1B in Appendix illustrates some examples). The fraction of time (observations) that mice were engaged in each type of behavior was determined, and is expressed as the fraction of observed events of a given category relative to the total number of observations (30 in a 1-h session), or relative to the number of observations in which the female was at the nest (fraction of AT NEST time). Because maternal behavior is influenced by litter size (Lonstein and Fleming, 2002), we culled litters to seven to eight pups per mother.
Retrieval test
Retrieval tests were conducted daily from P1 to P7 (Figure 2), using a protocol adapted from previous studies (Myers et al., 1989; Lucas et al., 1998; Lonstein and Fleming, 2002). Tests took place in the afternoon (light phase), after the home cage observation session. Each day, cages were transferred to a test room, and pups were removed from the nest and kept warm by placing them on a heat pad. After 15 min of separation from their mothers, four pups were put back at the end of the cage opposite to the nest. We recorded the latency of the female to retrieve each pup. It is important to note that mothers were at the nest at the moment when pups were reintroduced in the cage.
Discrimination of Own vs. Alien pups
To assess the ability of females to discriminate their own pups from alien pups (pups coming from a different litter), we used a protocol similar to the habituation/dishabituation paradigm but where pups served as stimuli (Ostermeyer and Elwood, 1983). Two litters were removed from the cages and kept warm on a heat pad. Mothers were then left alone in the home cage for 15 min. Pups were sequentially presented in 3-min sessions, separated by 3 min of rest. The first four presentations consisted of pups belonging to the test female (O1–O4, Figure 7); in the fifth presentation, a pup from a different litter (alien, A) was introduced. Lastly, a pup belonging to the test female was presented (O5). All presentations were video-recorded and analyzed offline. The investigation time, defined as the amount of time spent sniffing or licking, of each pup was determined. The habituation/dishabituation test was performed on day 11 after delivery (P11; Figure 7 and Supplementary Video).
Social Interaction and Offspring Recognition
Female mice were subjected to three sessions of social recognition/interaction at different time points after weaning: 30 min, 3 weeks and 3 months after weaning of their pups. In all cases, the test was carried out in the home cage of the test female and each presentation lasted 3 min. In the first session, we assessed the differential interaction of females with a juvenile (separated 30 min before) male of their own litter compared to an alien juvenile male that were simultaneously introduced in the cage. In the other two sessions, mice coming from the test female’s litter or from a different litter were introduced sequentially (in a balanced order) in sessions separated by a 4-min interval. In these latter seassions, both female and male mice were used as stimuli. All sessions were video-recorded and analyzed offline. Interaction was defined to include behaviors of the test mouse directed toward the stimulus mouse, including olfactory investigation, allogrooming, pursuit, and sitting in close proximity (Winslow, 2003), and it was determined using a stopwatch. To control for differences in solicitation from the stimuli mice, we also determined their interaction directed toward experimental females (Figure A4 in Appendix).
Urine Collection
Urine was collected from four to five unrelated adult males and females by gently massaging the pelvic region. The urine was collected with a plastic pipette as soon as it was spontaneously voided above a glass Petri dish. Same-sex urine samples were subsequently pooled and stored at −20°C until the experiment.
Object/Odor Investigation
Exploration of an odorless object, male urine, female urine, and a non-social odorant was evaluated at the end of the experiment, 7.5–8 months after irradiation. A marble (object), a marble scented with male urine (20 μl), a marble scented with female urine (20 μl), and a marble scented with (-)-carvone (pure, 20 μl, Sigma) were used as stimuli. The different stimuli were presented on different days, and in the same order for each mouse. All tests were carried out in the home cage, and consisted of the presentation of the stimuli during 3 min. Behavior was video-recorded and analyzed offline. The time spent investigating the stimulus was measured using a stopwatch.
Open Field
Locomotor activity and anxiety-related behavior were evaluated in this paradigm, in 40-min sessions. Open field arenas were dark-gray plexiglass boxes measuring 40 cm × 40 cm, and were placed in a well-lit room. Mouse activity was video-recorded, and x–y position was extracted at a sampling rate of 5 Hz using the mouse-tracking feature of QUIA software. For analysis, the arena was virtually divided in 16 equally sized squares. The four central quadrants were defined as the center, an area approximately 11 cm away from all walls. Total traveled distance, number of quadrant crossings, time spent in the center (defined as the number of samples in the center divided by the total number of sampling points) and distance traveled in the center were determined. Distance was defined as the Euclidean distance between two sampling points and was calculated in relative units. Speed at each time point was calculated as distance/time.
Pup Development
To assess the impact of variations in maternal care on pup development, a few measurements that relate to pup growth and development were determined. Body weight and tail length were recorded daily from P1 to P14, immediately after the pups were separated from their mothers in preparation for the retrieval test; pups were then left undisturbed until testing. Eye opening day was also recorded.
Statistical Analyses
Data are expressed as mean ± SEM in bar graphs or time courses, unless otherwise stated. Boxplots represent median and interquartile ranges. Statistical analyses were performed using Statistica (StatSoft) or custom-written software (Matlab, MathWorks). Non-parametric tests were chosen when the data did not follow the assumptions for parametric tests. Density of DCX staining in control vs. irradiated brains was compared using a Mann–Whitney U test. For observations at the home cage and retrieval test, two-way repeated measures ANOVA (rmANOVA) was used to compare behavior between treatments (control vs. irradiated), and evaluate changes of behavior across days. p-values for the age factor were corrected for violations of sphericity (correlations in the repeated measures) using the Greenhouse–Geisser (G–G) correction. For interaction with own vs. alien pups, two-way rmANOVA was used to evaluate differences between treatments and stimulus type. For all other behavioral tests, non-parametric tests were used for comparing unpaired (Mann–Whitney or Wilcoxon unpaired) or paired samples (Wilcoxon matched-pairs signed-rank test) as described in the text or the corresponding figure legends. For pup weight and tail length, we used a two-way rmANOVA (with treatment and age as factors); for eye opening time, we used a Kolmogorov–Smirnov test. Statistical significance was set at p < 0.05. All statistical results are shown in detail in Tables A1 and A2 in Appendix.
Results
Irradiation of the SVZ as a Model to Study the Contribution of Adult-Generated Neurons to Maternal Behavior and Recognition
To assess the contribution of adult neurogenesis in the olfactory system to maternal behavior and social recognition, we evaluated different aspects of these behaviors when neurogenesis is disrupted. To decrease the level of adult-born neurons reaching the OB, we selectively exposed the SVZ of female mice to gamma irradiation, while sparing other brain regions (see Materials and Methods and Figure 1A; Lazarini et al., 2009). The effect of this treatment on olfactory neurogenesis was corroborated in a subset of animals (Group A) 6.5 months after irradiation by quantifying the expression of DCX. As previously shown (Lazarini et al., 2009), SVZ irradiation resulted in a marked reduction of newly generated neurons in the OB (Figures 1B,C; p < 0.05, Mann–Whitney U test, see Table A1 in Appendix). DCX immunoreactivity in the rostral migratory stream of the OB (rmsOB) of irradiated females was 23% of that of control, non-irradiated females; overall, there was a 63% reduction of neurogenesis in the OB after irradiation.
Females with Reduced OB Neurogenesis Spent More Time at the Nest
We compared the undisturbed, spontaneous maternal behavior at the home cage of irradiated and control females, from the day of pup birth (postnatal day 0, or P0; Figure 2) until postnatal day 8 (P8). Irradiated females spent significantly longer time at the nest compared to controls (Figure 3A; p = 0.0196; see Table A1 in Appendix). For both groups, the amount of time spent at the nest decreased as pups grew older (p = 0.0096, rmANOVA); importantly, the time course of this decrease in control females resembled that observed in other studies (Kimchi et al., 2007). The tendency of IRR females to stay longer at the nest was also evidenced by a decreased number of transitions in and out of the nest, as compared to CTRL females (Figure 3B; p = 0.0222). This apparent stillness observed in IRR females cannot be attributed to locomotor deficits or differences in anxiety levels, as the behavior of the IRR and CTRL groups was undistinguishable when tested in an open field (Figure A2 and Table A2 in Appendix).
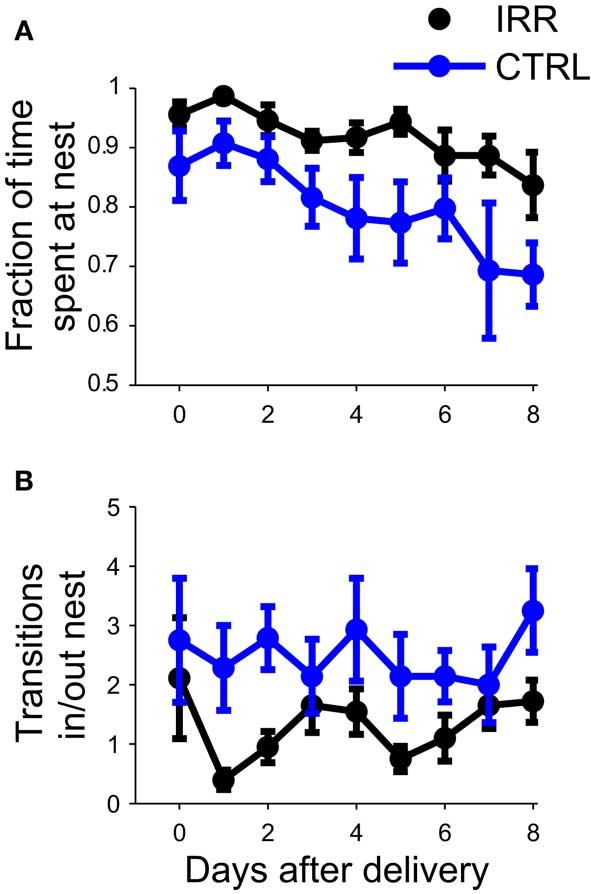
Figure 3. Irradiated females spent more time at the nest. (A) The fraction of time spent at the nest (number of observations at nest/total number of observations) was determined for eight consecutive days, starting at pup birth (P0). For each mouse, daily data is calculated as the average of two sessions. Data are represented as the mean across mice in each treatment (IRR, black, n = 10 mice; CTRL, blue, n = 7 mice). Error bars represent SEM. p(IRR vs. CTRL) = 0.0196, p(days after delivery) = 0.0096 with a two way rmANOVA. (B) Control mice shuttle more in and out of the nest. The number of transitions into or out of the nest was determined from P0 to P8. Data represent mean across mice, computed as in (A). p(IRR vs. CTRL) = 0.0222, p(days after delivery) > 0.05 with a two-way rmANOVA.
We examined the behavioral repertoire of these mice in more detail, focusing our analysis on how much time mothers spent engaging in different behaviors while at the nest (Figure 4). The proportion of time allocated to each of the behaviors recorded (see Materials and Methods and Figure A1B in Appendix) did not differ between CTRL and IRR females (p > 0.05 for all comparisons, rmANOVA; see Table A1 in Appendix). However, the longer presence of IRR females at the nest resulted in longer total time that their pups were observed lactating (Figure 5, compare A and B), although this difference did not reach statistical significance (p = 0.0506, rmANOVA).
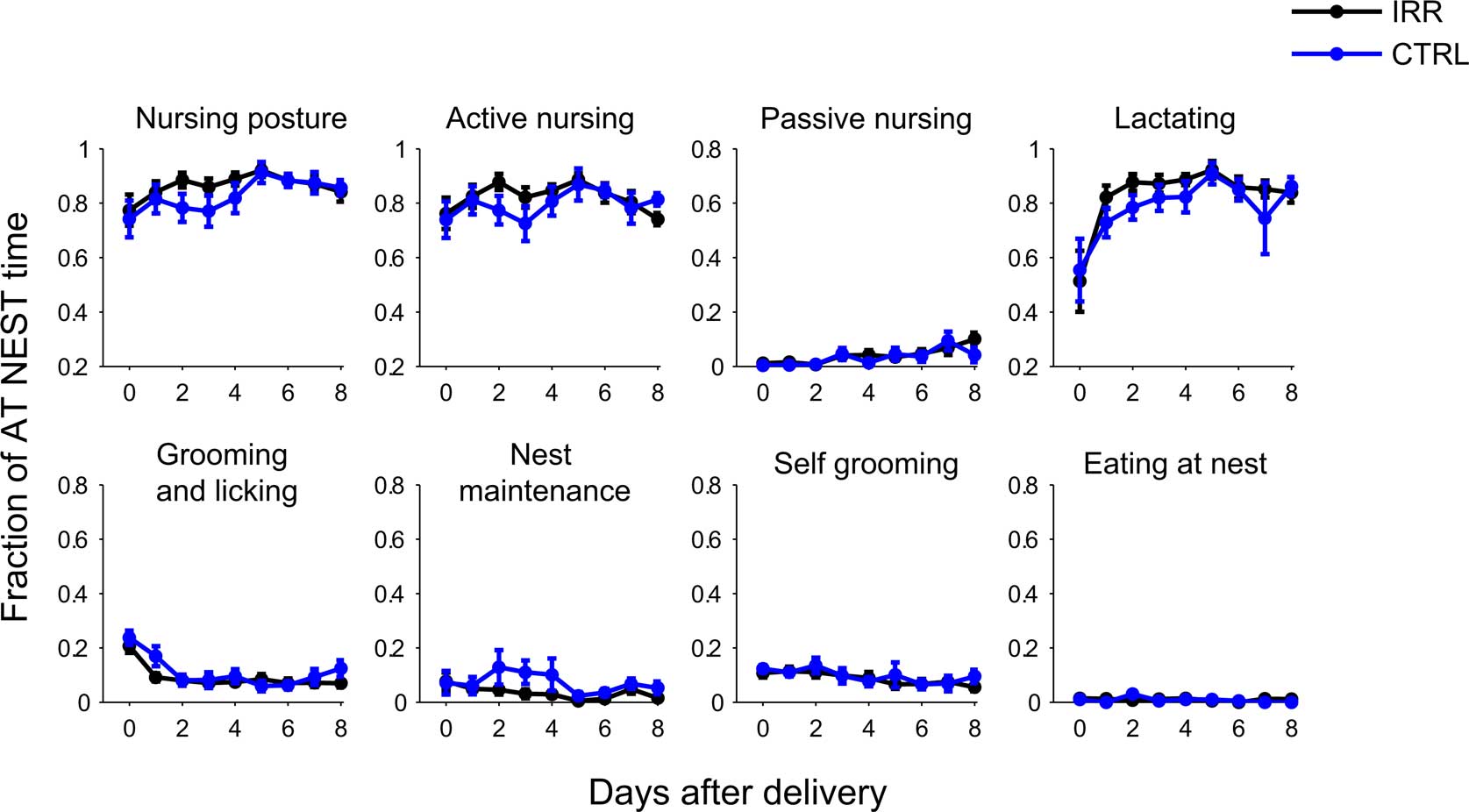
Figure 4. Irradiated and control females displayed indistinguishable behavioral repertoires while at the nest. Behavior at nest was further classified as: mother in a nursing posture, which could be an active or passive nursing posture, grooming and licking pups, doing nest maintenance, self-grooming or eating; and pups lactating (see Materials and Methods for a more detailed description of the behavioral categories and Figure A1B in Appendix for examples). Note that each behavior is scored as a fraction of the time the female was at the nest (number of observations of behavior/number observations at nest). Data represent mean across mice in each group; for each mouse, data is the average of two daily sessions. IRR (black), n = 10 mice; CTRL (blue), n = 7 mice. For none of the behaviors the time allocated (while at nest) differed between IRR and CTRL mice (p > 0.05 for all treatment comparisons, two-way rmANOVA).
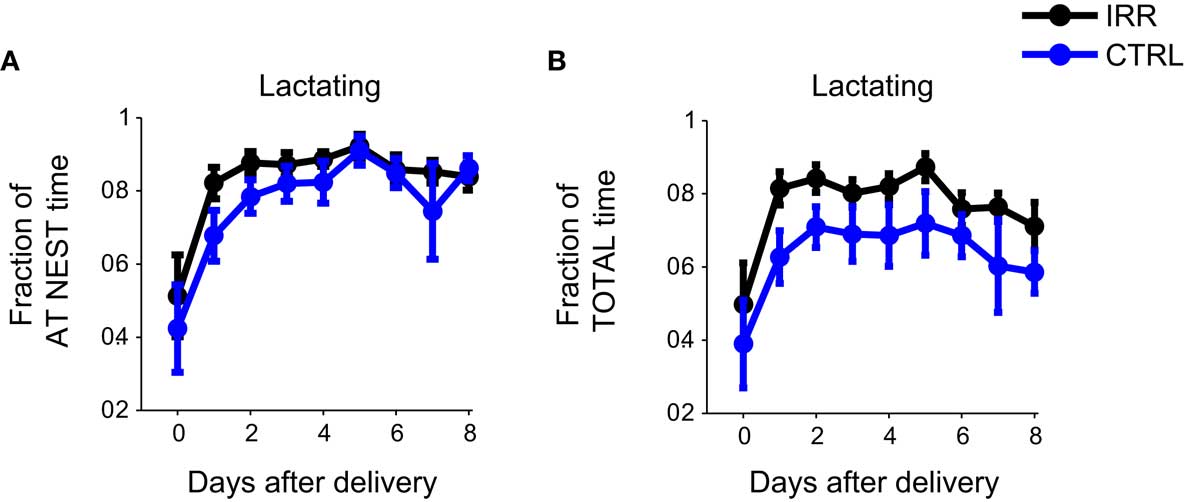
Figure 5. Longer times at the nest resulted in longer net lactating times for irradiated litters. Irradiated and control females, while at nest, spent the same proportion of time engaged in lactating (A, data is replicated from Figure 4). However, because IRR female mice spent more time at the nest (Figure 3A) they tended to show a net increase in total lactation time (B) compared to controls. p(IRR vs. CTRL) = 0.0506, p(days after delivery) = 0.0397, with a two-way rmANOVA.
To determine the possible influence of differences in nursing behavior on pup growth and development, we investigated a few parameters related to the latter. Body growth, measured as weight (Figure A3A in Appendix) and tail length (Figure A3B in Appendix), did not differ between litters of CTRL and IRR mothers, neither did the time of eye opening (Figure A3C in Appendix).
Overall, we observed little change in spontaneous maternal behavior when OB neurogenesis was reduced. Irradiated females spent longer time at the nest, and consequently they tended to spend more time nursing their litter; this difference, however, did not result in obvious changes in the development of the pups.
PUP Retrieval Did not Differ between Control and Irradiated Females
We next asked whether behavior in a pup retrieval test was affected in females with reduced OB neurogenesis. Retrieval behavior is an important component of maternal behavior, and retrieval tests have been widely used to evaluate it. Rodent models with altered maternal behavior show changes in the latency to retrieve pups to the nest, or in the time to complete the retrieval of all pups (Lucas et al., 1998; Bridges et al., 2001). We performed retrieval tests daily from days P1 to P7. Few females failed to retrieve their pups to the nest on the first day of testing, and the proportion of sessions with no retrievals did not differ between CTRL and IRR females (Figure 6A; IRR: one out of nine, CTRL; one out of seven, p ( 0.05 two-sided difference of proportions test). Latencies to retrieve the first and last (fourth) pup – the latter a measure of task completion – were unaffected in IRR females (Figures 6B,C; p = 0.4815, p = 0.2418, first and last pups, respectively; rmANOVA, see Table A1 in Appendix) in all tested days. Both groups showed shorter retrieval times with repeated testing (p = 0.0392 and 0.0105, first and last pups respectively; rmANOVA, see Table A1 in Appendix). Thus, reduced olfactory neurogenesis did not affect the rate at which pups were retrieved to the nest.
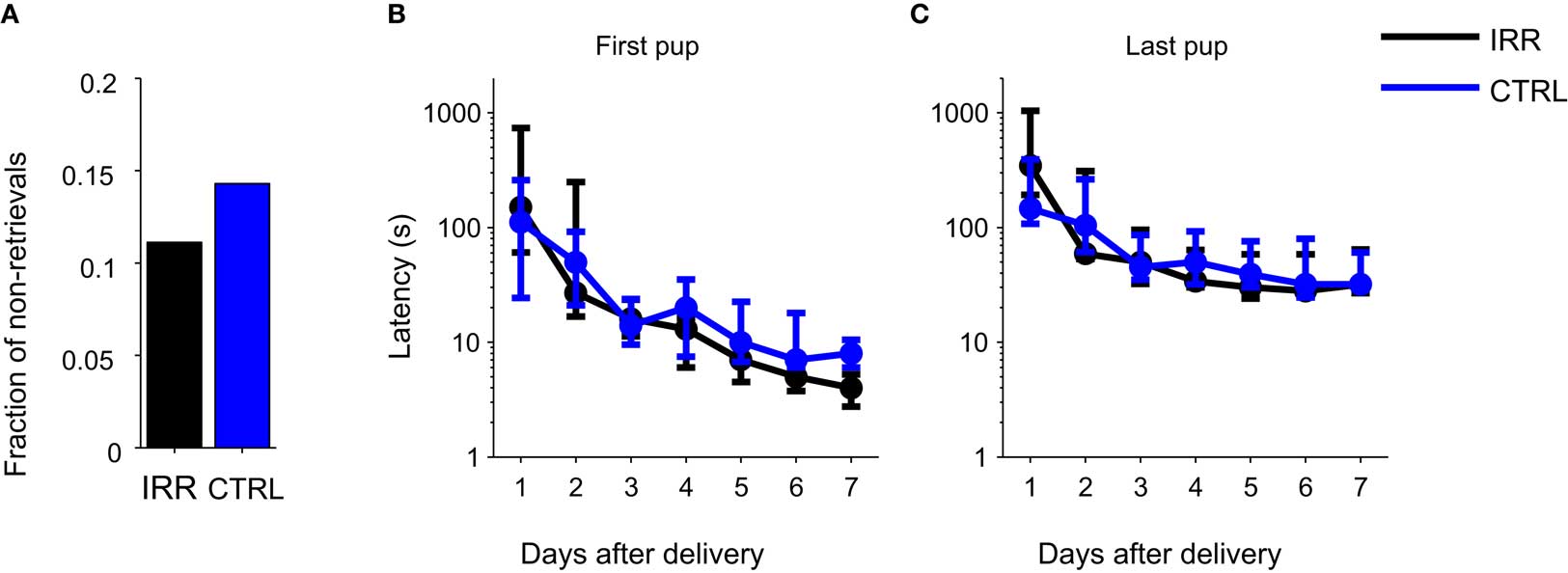
Figure 6. Irradiated and control females showed similar latencies in a pup retrieval test. (A) Fraction of females that failed to retrieve pups on the first day of testing (P1). IRR (black), n = 9 mice; CTRL (blue), n = 7 mice. (B,C) Latencies to retrieve the first (B) and last (C) pups. Latency to retrieve pups decreased across days (first pup: p = 0.0392, last pup: p = 0.0105) but did not differ between groups (first pup: p = 0.4815, last pup: p = 0.2418; two-way rmANOVA). Data are shown as median latencies, in logarithmic scale. Note that error bars represent 25th and 75th percentiles. IRR, n = 9 mice; CTRL, n = 7 mice.
Discrimination of Complex Social Odors is Unaltered in IRR Females
Both the maternal behavior at nest and the retrieval tests, although commonly used in studies of maternal behavior, are primarily related to motivational aspects in the establishment and maintenance of maternal behavior (Stern and Lonstein, 2001). We hypothesized that the addition of newly generated neurons during pregnancy could play a role in aiding the learning and discrimination of pup odors. In the next set of experiments, we tested the ability of IRR females to discriminate pups, as well as their differential interaction with own vs. alien pups or young adults.
The ability of female mice to spontaneously discriminate pups was tested at P11 in a protocol analogous to a habituation/dishabituation test but using pups as stimuli (Ostermeyer and Elwood, 1983). Pups belonging to the female’s litter were introduced sequentially. In each presentation, females immediately retrieved the pup to the nest, and engaged in sniffing and licking the retrieved pup (see Supplementary Video). As observed in habituation protocols, investigation time decreased after the first presentation in CTRL females (Figure 7; blue boxes, compare O1 and O2; p = 0.04, Wilcoxon matched-pairs test). These females were also able to discriminate between pups from their own or different litters, as evidenced by a large increase in investigation time when females were presented with an alien pup (Figure 7; blue boxes, compare O4 and alien; p = 0.04, Wilcoxon matched-pairs test), as previously shown for outbred mice (Ostermeyer and Elwood, 1983). Importantly, the habituation and discrimination ability in IRR females were indistinguishable from that of controls (Figure 7; compare blue and black boxes; O4 vs. alien (IRR): p = 0.04, Wilcoxon matched-paired test). Thus, decreased olfactory neurogenesis in irradiated females did not impair their ability to perform a fine, complex discrimination (that of offspring vs. non-offspring).
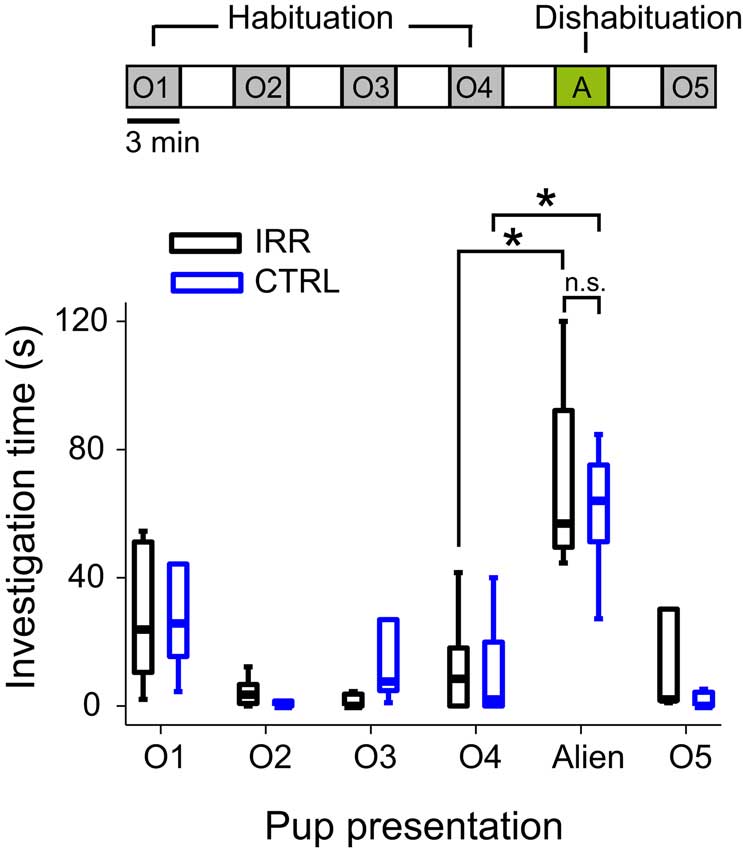
Figure 7. Irradiated females showed intact ability to discriminate pups in a habituation/dishabituation paradigm. Females were subjected sequentially (top scheme) to four 3-min presentations of a pup from their own litter (O1–O4, habituation phase), followed by a 3-min presentation of a pup from a different litter (alien, A, dishabituation), and another presentation of their own pup (O5). Presentations were separated by 3-min intervals. Investigation time is shown in boxplots (central line shows median investigation time; outliers are omitted). The time spent investigating own pups decreased after the first presentation for both CTRL (blue, n = 5) and IRR (black, n = 5) females (p < 0.05, Wilcoxon matched-pairs test). Presentation of an alien pup resulted in a marked increase in investigation time in females in both groups – compare alien to O4 (*p < 0.05, Wilcoxon matched-pairs test).
Irradiated Females Showed Enhanced Social Interaction with Male Conspecifics
Having shown that both CTRL and IRR females can discriminate between pups, we next asked whether this ability would result in a preferential interaction with own compared to alien pups in a test of social interaction, and whether there could be differences in preference between treated and untreated females. At the day of weaning, females were exposed simultaneously to two male pups, one of their own and one coming from another litter. Neither CTRL nor IRR females showed preferential interaction with own compared to alien pups (Figure 8; p(pupID) = 0.605, with a two-way rmANOVA). However, IRR females spent overall almost twice as much time as controls engaged in interaction with the pups introduced (Figure 8; p(IRR vs. CTRL) = 0.027, two-way rmANOVA).
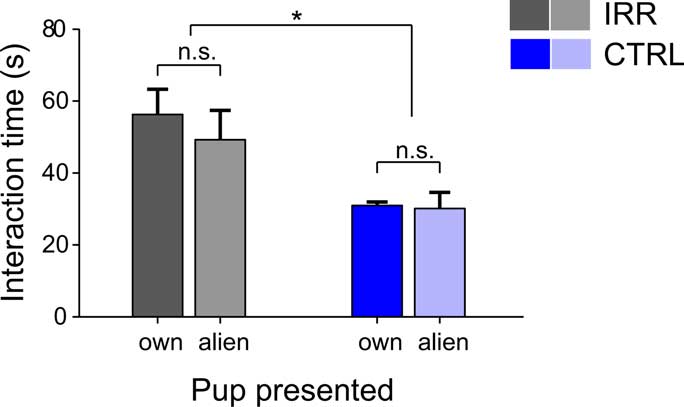
Figure 8. Irradiated females show elevated social interaction at weaning. Social interaction of experimental females toward different “stimulus” individuals was evaluated in 3-min sessions, 30 min after weaning. IRR, n = 5 mice; CTRL, n = 5 mice. Females did not show preference in their interaction with a juvenile male of their own litter (own) compared to a juvenile from another (alien) litter. However, irradiated females showed a marked increase in interaction time compared to controls (*p = 0.027, two-way rmANOVA). Data represent mean interaction time for each group.
We next asked whether social interaction would also be altered at later times. Experimental females were tested again for social interaction 3 weeks and 3 months (when they had become sexually mature adults) after they were separated from their pups (Figure 2). Females were exposed sequentially to both males and females from their litter (own) and unrelated litters (alien). As observed at weaning day, IRR females spent far more time in contact with the mice introduced, and this was true at both time points. Interestingly, this difference arose from an increased interaction of IRR mice with male adults (Figures 9A,B; p < 0.05), compared to the interaction of CTRL females with such subjects. It is important to note that the increased interaction of IRR females with male conspecifics did not result from a change in solicitation from part of the males, as the interaction of the latter with control and irradiated females was indistinguishable (Figure A4 and Table A2 in Appendix). Neither CTRL nor IRR females showed differential interaction with own vs. alien juveniles (data not shown), and thus the data is pooled together; this is in contrast with a recent study where male mice were shown to investigate differentially own vs. alien progeny, a difference that was dependent on intact neurogenesis (Mak and Weiss, 2010).
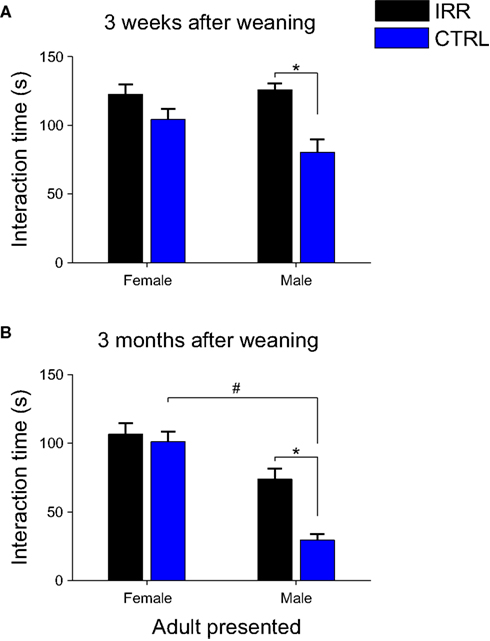
Figure 9. Increased social interaction persists at later times, and is the result of altered interaction with males. Social interaction of experimental females toward male and female adults was evaluated in 3-min sessions, 3 weeks (A) and 3 months (B) after weaning. IRR (black), n = 5 mice; CTRL (blue), n = 5 mice. (A) Irradiated females showed increased interaction with a male mouse when compared to controls (*p = 0.0367, IRR vs. CTRL, Mann–Whitney U test). However, interaction with a female intruder did not significantly differ between control and irradiated mice. Data represent the mean across experimental females; for each female, investigation times of own and alien pups did not differ (data not shown) and are averaged together. (B) Irradiated females showed significantly longer interactions with male stimuli compared to controls (*p < 0.05, Bonferroni). Note also that CTRL females showed a shorter interaction time with males than with females (#p < 0.05, Bonferroni). Data represent the mean across experimental females; for each female, investigation times of related (own) and unrelated (alien) young adults were averaged together.
To ensure that the differences we observed in the social behavior paradigms were due to social aspects of the test, and not differences in general exploration or odor investigation, we performed a series of tests to evaluate investigation of objects and odorants. The exploration/investigation time was determined for different “objects”: an unscented glass marble (to assess pure “object” investigation), or a marble scented with female urine, male urine, or a monomolecular odorant ((-)-carvone). Investigation time did not differ between IRR and CTRL females in any of the four conditions tested (Figure 10; p > 0.05 Mann–Whitney u test). It is important to note that changes in exploration time across days are probably due to habituation to the object or the testing procedure; nevertheless, this does not affect our comparisons as we focused on the differences between groups. This suggests that the differences we observe in the social interaction tests may be related to social odors (although a subset not present in the collected urine) or other aspect of the social encounter, but cannot be attributed to an increase in general object or odor exploration.
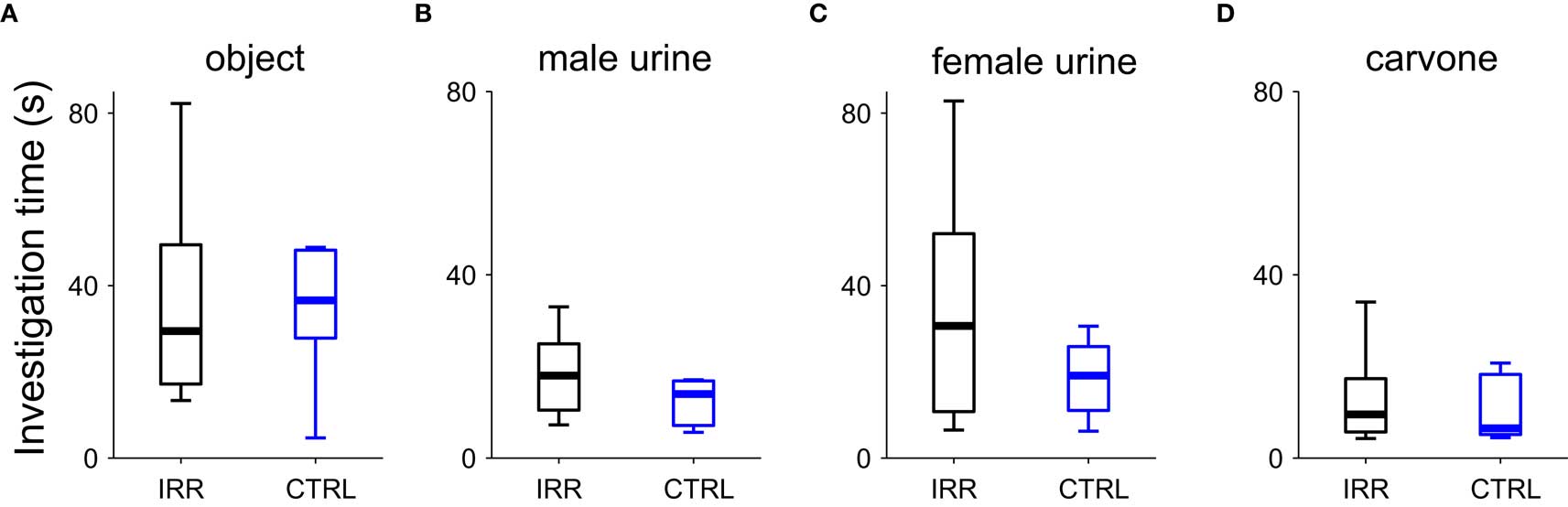
Figure 10. Object and odor investigation were unaltered in SVZ-irradiated females. Investigation time of an object (A), an object scented with male urine (B), an object scented with female urine (C), or an object scented with carvone (D) were determined. Data are shown in boxplots, where central lines represent the median investigation time. No differences in investigation times were found between groups (p > 0.05 for CTRL vs. IRR in all conditions, Mann–Whitney U test). IRR (black), n = 5 mice; CTRL (blue), n = 5 mice.
Altogether, the social interaction experiments show that IRR females display altered interaction with male, but not female, subjects. This suggests that IRR females may have a deficit in the detection or processing of male-related odors, which could result in altered gender recognition.
Discussion
In the present study we tested the effect of reducing the levels of adult neurogenesis on maternal behavior, offspring recognition, and social interaction. Females with impaired neurogenesis showed abnormal social interaction patterns with male conspecifics when compared to controls. When maternal behavior was evaluated, we observed that the reduction of olfactory neurogenesis had little effect on the behaviors analyzed. We further hypothesized that adult neurogenesis contributes to fine odor discrimination, and could therefore be important for pup recognition. Disruption of bulbar neurogenesis in female mice, however, did not affect their ability to discriminate their own pups from others. Thus, the addition of neurons in the olfactory system was not required for the establishment and expression of maternal behavior or pup discrimination, but rather played a role in social interaction.
Maternal Behavior was Largely Unaffected in Females with Impaired Neurogenesis
A growing number of studies shows that the levels of neurogenesis are modulated in the context of reproductive and social behavior, providing a framework for studying neurogenesis in ethologically relevant situations (Shingo et al., 2003; Mak et al., 2007; Larsen et al., 2008; Mak and Weiss, 2010).
Based on those studies, it has been hypothesized that adult-generated neurons in the olfactory system could play a role in parental behavior. To investigate whether a direct causal link between neurogenesis and maternal behavior exists, we evaluated the effect of reducing OB adult neurogenesis on several aspects of maternal behavior. Similarly, a recent study examined how maternal behavior is affected when surges of PRL, together with the concomitant increase in neurogenesis, are blocked during pregnancy (Larsen and Grattan, 2010). As in our study, the authors reported no difference in a retrieval test between low-PRL (i.e., low neurogenesis) and control females when the mothers were tested in their home cage. Our results extend these findings by showing that irradiation of the SVZ (a manipulation that specifically disrupts proliferation of neuronal progenitors) of female mice not only does not lead to deficits in pup retrieval, but also leaves overall maternal care unaffected.
The only difference we observed in irradiated mice was an increase in the time irradiated females spent at the nest with their litters. This observation is intriguing, as it does not seem to arise due to a lack of exploration or increased anxiety. It is possible that irradiated mice are less “interested” in exploring the environment due to an olfactory deficit, or that they are less sensitive to food odors and therefore less motivated to leave the nest; however, this seems unlikely given that both irradiated and control mice showed similar levels of odor exploration (as observed in the marble and habituation/dishabituation tests).
Altogether, disruption of olfactory neurogenesis had little effect on maternal behavior, at least for the behaviors evaluated. These observations could be explained by the fact that the behaviors tested are primarily related to motivational aspects of maternal care, and not necessarily require olfactory neurogenesis, although OB function is important for the normal establishment of maternal behavior in primiparous female mice (Gandelman et al., 1971). Alternatively, it is possible that the absence of a strong effect is related to limitations of the manipulation used. Although the irradiation protocol used has the important advantage of being highly focal, which allowed us to target the SVZ without affecting the hippocampus (Lazarini et al., 2009), a potential caveat of this method is its chronic nature: olfactory neurogenesis is reduced chronically, which could result in the appearance of compensatory mechanisms. In addition, despite the fact that the levels of neurogenesis were drastically reduced in irradiated females, it is possible that the remaining neurogenesis was sufficient to sustain normal behavior.
Discrimination of Social Odors
Upon birth, mothers are exposed for the first time to the odor of their offspring. Ewes, for instance, learn the odor of their lambs during the first hours after birth, and provide selective care to their progeny, rejecting others (Brennan and Kendrick, 2006). Female mice, despite forming communal nests and caring for own and alien pups, nest preferentially with other closely related females (Manning et al., 1992). We hypothesized that learning and discriminating the odors of a female’s own pups from pups coming from different litters could be important, at least in the wild. We further hypothesized that the addition of newly generated neurons during pregnancy could play a role in aiding the learning and discrimination of pup odors, and thus contribute to the recognition of a female’s own progeny. Indeed, a recent study shows that interaction with pups results in an increase of neurogenesis in male mice (Mak and Weiss, 2010), and that increase seems to be important for pup recognition by fathers.
However, we show here that the ability of female mice to discriminate their pups from alien pups did not depend on intact levels of olfactory neurogenesis, as irradiated females preserved their discrimination capacity. Because neurogenesis was not completely ablated using our irradiation protocol it is possible that the remaining fraction of newly generated neurons arriving at the OB could suffice to perform this discrimination. Alternatively, and although discrimination of individuals is believed to be mediated by olfactory cues (Hurst et al., 2001; Brennan and Kendrick, 2006), females could use other cues to discriminate pups, such as ultrasound vocalizations, although this seems unlikely. Ultrasound calls emitted by pups when removed from the nest did not differ qualitatively among litters (data not shown), arguing against a contribution of these signals to pup discrimination. Moreover, this mouse strain suffers from age-related hearing loss (Nemoto et al., 2004).
Social recognition studies suggest that there exist two types of discriminations: the fine discrimination of individuals within a strain, and the coarser discrimination of individuals belonging to different strains and thus genetically diverse (Macbeth et al., 2009b). It is important to note that here we used genetically identical mice, and therefore tested the discrimination within a strain, and showed that the ability of irradiated females to perform such a fine discrimination remained intact. However, we cannot rule out that irradiation could have affected the ability of mice to discriminate across strains. Although this discrimination seems at first glance an easier one, it is possible that irradiation compromised specifically the ability to discriminate among the genetically varying molecular profiles, such as those determined by MHC molecules or MUPs (Singer et al., 1997; Hurst, 2009), leaving odor discrimination intact. Intra and inter-strain discrimination could also rely on different neuronal circuits, which could have been differentially affected by our irradiation protocol.
Recognition of individuals and mating preferences are assessed behaviorally as a differential investigation of conspecifics (Barnard and Fitzsimons, 1988; Krackow and Matuschak, 1991; Macbeth et al., 2009a). Despite being able to discriminate their pups from others, females did not show a differential interaction with alien compared to own pups or juveniles when tested in a social interaction assay. This was true both for irradiated and control females. Although one would expect, in the light of inbreeding avoidance (Pusey and Wolf, 1996; Sherborne et al., 2007), that females would show some preference in the interaction with related vs. unrelated individuals, the lack of such a difference in our interaction test does not imply that, given the choice, female mice would mate indiscriminately with either male mouse. This remains an open question, as we did not test mating preferences, and the interaction test may be insufficient to uncover those. It is also possible that differences in social behavior would have been evident if we used wild-derived or outbred mice. Our results are in contrast with a recent study (Mak et al., 2007) that shows that male mice exposed to their pups are able to recognize their progeny later in adulthood, a recognition that is PRL-dependent and correlated with an increase in neurogenesis. The difference with our study could arise from differences in paternal vs. maternal interaction with progeny.
Remarkably, irradiated females spent more time engaged in social interaction, independently of the identity of the individual introduced. This increased investigation could be interpreted as irradiated females requiring longer investigation time to process information about the subject’s identity. However, this cannot be attributed to a general increase in exploration of odor stimuli (Figure 10). A possible caveat of this last experiment is that testing was done last in the series of behavioral experiments, at a point (7.5–8 months after IRR, that is, 9.5–10 months of age) where the difference in neurogenesis between CTRL and IRR could be lower due to the age-related decline of neurogenesis (Luo et al., 2006). However, our result is consistent with other studies that show that object investigation and recognition is unaffected in mice with ablated neurogenesis (Breton-Provencher et al., 2009). The increased interaction that we observed in females with reduced neurogenesis is in contrast with manipulations that render mice anosmic; such manipulations result in decreased investigation of opposite-sex conspecifics (Yoon et al., 2005) or odorants (Keller et al., 2006).
One interesting observation when comparing social interaction 3 weeks and 3 months after weaning was that control animals maintained an elevated interaction with female “intruders” but reduced their interaction with males. It is important to note that 3 weeks after weaning mice are still considered juveniles and are still sexually immature, whereas 3 months after weaning male mice have become fully mature and therefore constitute potential mates. Thus, as expected, control females develop a differential interaction with male or female stimuli when the stimuli are sexually mature subjects. However, the pattern observed in control females was altered in irradiated females, who spent similar amounts of time interacting with male and female conspecifics, suggesting that the processing of male odors involved in mate recognition is impaired in irradiated females (Hurst, 2009). In a previous study (Lazarini et al., 2009), some of us reported that SVZ-irradiated and control males spent a similar amount of time investigating male conspecifics; however, the interaction of male mice with females was not evaluated. In the present study, irradiated females showed an altered pattern of interaction with male conspecifics, but not with conspecifics of their same sex. Thus, it would be interesting to explore whether the deficits observed in the interaction are restricted to opposite-sex interactions, or is a deficit specific to female mice.
Notably, irradiated and control females did not differ in the investigation of urine from male conspecifics (Figure 10). This suggests that the differences observed in the interaction are likely to arise due to deficits in the detection or discrimination of social cues not present in urine, and points to the importance of other signals, and perhaps sensory modalities, available to the mice when interacting with the whole animal. Alternatively, some relevant volatile components in the urine could have been lost, although this seems unlikely as we froze the urine immediately after collection. Several substances have been implicated in mediating sex recognition and opposite-sex attraction in mice, acting on both the main and accessory olfactory systems (Ramm et al., 2008). Although most of the components characterized so far are present in the urine (Lin et al., 2005; Baum and Kelliher, 2009), other social cues important for sex recognition and sexual interaction are found in other body secretions, such as those from the lachrymal and submaxillary glands (Kimoto et al., 2005; Haga et al., 2010).
By ruling out differences in the investigation of urine (the marble-urine test) our observations narrow down the possible factors that could be involved in the differential interaction with males observed in irradiated females. Thus, it would be interesting to test whether newly generated neurons are responsive to candidate pheromones, and whether fewer neurons respond to this molecule in irradiated females. Although our results do not provide conclusive evidence for a deficit in gender discrimination in irradiated females, they are consistent with this hypothesis, and they point to this as an intriguing line for future experiments. Such an impairment in the processing of male-related odors could have consequences for the recognition of potential mating partners, and therefore for reproductive behavior. Further experiments are required, however, to explain the interaction pattern observed in irradiated females and elucidate the underlying mechanism.
Disruption of the OB Network: Fewer Plastic Cells or Altered Selection?
What is the effect of irradiation on the OB network? Both in this study in female mice, and in a previous study from our laboratory using male mice (Lazarini et al., 2009), we showed that SVZ irradiation results in a substantial reduction of young (DCX+) neurons reaching the OB. Because DCX is expressed transiently in immature neurons, determining DCX levels provides only a snapshot of the population of newly born neurons arriving in the OB. However, an important component of adult neurogenesis is the selection, integration and survival of adult-generated neurons (Lledo et al., 2006). In our previous study (Lazarini et al., 2009), we observed that the adult-generated neurons that did reach the OB in irradiated animals did not undergo the selection/survival process that normally occurs in control animals. In this scenario, the behavioral consequences that result from SVZ irradiation could be due to the lack of a population of neurons with specific properties (young, more plastic neurons; Nissant et al., 2009), or to the lack of selective pressure on the neurons that arrive in the bulb, or both. Further experiments are required to distinguish between these different hypotheses. In either case, we show here that disruption of normal adult neurogenesis in female mice results in altered social behavior.
Conclusions
Several lines of evidence have suggested a link between olfactory neurogenesis and maternal behavior. However, our experiments show that reduction of adult neurogenesis neither affects the establishment and expression of maternal behavior – to the extent of the parameters analyzed – nor does it compromise the ability of female mice to distinguish among pups. Rather, adult olfactory neurogenesis is important for normal social interaction. Our results suggest a possible role of adult neurogenesis in sex recognition, consistent with previous studies implicating neurogenesis in mate preference (Mak et al., 2007), and opens up new ends to tackle the contribution of adult neurogenesis to reproductive behavior.
Conflict of Interest Statement
The authors declare that the research was conducted in the absence of any commercial or financial relationships that could be construed as a potential conflict of interest.
Acknowledgments
We wish to thank Drs. Christian Machens and Zachary Mainen for critical reading of the manuscript, and Dr. Léa Zinck for helpful discussions. We thank Beatrice de Cougny for technical assistance with Figure 1A, Nicolas Torquet for assistance with behavioral experiments, and Drs. Marc-André Mouthon and Jean-Baptiste Lahaye for technical assistance with the irradiation procedure. This work was supported by the Agence Nationale de la Recherche (ANR-2007 SEST-01411), a Pasteur Institute Intramural Program (PTR n°319), the life insurance company “Novalis-Taitbout,” the Ecole des Neurosciences de Paris (ENP), and the Fondation pour la Recherche Médicale “Equipe FRM.” Claudia E. Feierstein was recipient of a postdoctoral fellowship from the ENP and the FRM. Sebastien Wagner was supported by the Letten Foundation.
References
Abraham, N. M., Egger, V., Shimshek, D. R., Renden, R., Fukunaga, I., Sprengel, R., Seeburg, P. H., Klugmann, M., Margrie, T. W., Schaefer, A. T., and Kuner, T. (2010). Synaptic inhibition in the olfactory bulb accelerates odor discrimination in mice. Neuron 65, 399–411.
Alonso, M., Viollet, C., Gabellec, M.-M., Meas-Yedid, V., Olivo-Marin, J.-C., and Lledo, P.-M. (2006). Olfactory discrimination learning increases the survival of adult-born neurons in the olfactory bulb. J. Neurosci. 26, 10508–10513.
Alvarez-Buylla, A., and Garcia-Verdugo, J. M. (2002). Neurogenesis in adult subventricular zone. J. Neurosci. 22, 629–634.
Barnard, C. J., and Fitzsimons, J. (1988). Kin recognition and mate choice in mice: the effects of kinship, familiarity and social interference on intersexual interaction. Anim. Behav. 36, 1078–1090.
Baum, M. J., and Kelliher, K. R. (2009). Complementary roles of the main and accessory olfactory systems in mammalian mate recognition. Annu. Rev. Physiol. 71, 141–160.
Boehm, T., and Zufall, F. (2006). MHC peptides and the sensory evaluation of genotype. Trends Neurosci. 29, 100–107.
Brennan, P. A., and Kendrick, K. M. (2006). Mammalian social odours: attraction and individual recognition. Philos. Trans. R. Soc. Lond., B, Biol. Sci. 361, 2061–2078.
Breton-Provencher, V., Lemasson, M., Peralta, M. R. III, and Saghatelyan, A. (2009). Interneurons produced in adulthood are required for the normal functioning of the olfactory bulb network and for the execution of selected olfactory behaviors. J. Neurosci. 29, 15245–15257.
Bridges, R. S., Rigero, B. A., Byrnes, E. M., Yang, L., and Walker, A. M. (2001). Central infusions of the recombinant human prolactin receptor antagonist, S179D-PRL, delay the onset of maternal behavior in steroid-primed, nulliparous female rats. Endocrinology 142, 730–739.
Brown, J. P., Couillard-Després, S., Cooper-Kuhn, C. M., Winkler, J., Aigner, L., and Kuhn, H. G. (2003). Transient expression of doublecortin during adult neurogenesis. J. Comp. Neurol. 467, 1–10.
Carleton, A., Petreanu, L. T., Lansford, R., Alvarez-Buylla, A., and Lledo, P.-M. (2003). Becoming a new neuron in the adult olfactory bulb. Nat. Neurosci. 6, 507–518.
Chamero, P., Marton, T. F., Logan, D. W., Flanagan, K., Cruz, J. R., Saghatelian, A., Cravatt, B. F., and Stowers, L. (2007). Identification of protein pheromones that promote aggressive behaviour. Nature 450, 899–902.
Champagne, F. A., Francis, D. D., Mar, A., and Meaney, M. J. (2003). Variations in maternal care in the rat as a mediating influence for the effects of environment on development. Physiol. Behav. 79, 359–371.
Gandelman, R., Zarrow, M. X., Denenberg, V. H., and Myers, M. (1971). Olfactory bulb removal eliminates maternal behavior in the mouse. Science 171, 210–211.
Haga, S., Hattori, T., Sato, T., Sato, K., Matsuda, S., Kobayakawa, R., Sakano, H., Yoshihara, Y., Kikusui, T., and Touhara, K. (2010). The male mouse pheromone ESP1 enhances female sexual receptive behaviour through a specific vomeronasal receptor. Nature 466, 118–122.
Hurst, J. L. (2009). Female recognition and assessment of males through scent. Behav. Brain Res. 200, 295–303.
Hurst, J. L., Payne, C. E., Nevison, C. M., Marie, A. D., Humphries, R. E., Robertson, D. H. L., Cavaggioni, A., and Beynon, R. J. (2001). Individual recognition in mice mediated by major urinary proteins. Nature 414, 631–634.
Imayoshi, I., Sakamoto, M., Ohtsuka, T., Takao, K., Miyakawa, T., Yamaguchi, M., Mori, K., Ikeda, T., Itohara, S., and Kageyama, R. (2008). Roles of continuous neurogenesis in the structural and functional integrity of the adult forebrain. Nat. Neurosci. 11, 1153–1161.
Keller, M., Douhard, Q., Baum, M. J., and Bakker, J. (2006). Destruction of the main olfactory epithelium reduces female sexual behavior and olfactory investigation in female mice. Chem. Senses 31, 315–323.
Kimchi, T., Xu, J., and Dulac, C. (2007). A functional circuit underlying male sexual behaviour in the female mouse brain. Nature 448, 1009–1014.
Kimoto, H., Haga, S., Sato, K., and Touhara, K. (2005). Sex-specific peptides from exocrine glands stimulate mouse vomeronasal sensory neurons. Nature 437, 898–901.
Krackow, S., and Matuschak, B. (1991). Mate choice for non-siblings in wild house mice: evidence from a choice test and a reproductive Test. Ethology 88, 99–108.
Larsen, C. M., and Grattan, D. R. (2010). Prolactin-induced mitogenesis in the subventricular zone of the maternal brain during early pregnancy is essential for normal postpartum behavioral responses in the mother. Endocrinology. 151, 3805–3814.
Larsen, C. M., Kokay, I. C., and Grattan, D. R. (2008). Male pheromones initiate prolactin-induced neurogenesis and advance maternal behavior in female mice. Horm. Behav. 53, 509–517.
Lazarini, F., Mouthon, M.-A., Gheusi, G., de Chaumont, F., Olivo-Marin, J.-C., Lamarque, S., Abrous, D. N., Boussin, F. D., and Lledo, P.-M. (2009). Cellular and behavioral effects of cranial irradiation of the subventricular zone in adult mice. PLoS ONE 4, e7017. doi: 10.1371/journal.pone.0007017.
Lévy, F., Locatelli, A., Piketty, V., Tillet, Y., and Poindron, P. (1995). Involvement of the main but not the accessory olfactory system in maternal behavior of primiparous and multiparous ewes. Physiol. Behav. 57, 97–104.
Li, Y., Mu, Y., and Gage, F. H. (eds) (2009). “Development of neural circuits in the adult hippocampus,” (Chapter 5) in Current Topics in Developmental Biology, Vol. 87 (San Diego, CA: Academic Press), 149–174.
Lin, D. Y., Zhang, S.-Z., Block, E., and Katz, L. C. (2005). Encoding social signals in the mouse main olfactory bulb. Nature 434, 470–477.
Lindsey, B. W., and Tropepe, V. (2006). A comparative framework for understanding the biological principles of adult neurogenesis. Prog. Neurobiol. 80, 281–307.
Lledo, P.-M., Alonso, M. and Grubb, M. S. (2006). Adult neurogenesis and functional plasticity in neuronal circuits. Nat Rev Neurosci. 7, 179–193.
Lledo, P.-M., and Saghatelyan, A. (2005). Integrating new neurons into the adult olfactory bulb: joining the network, life-death decisions, and the effects of sensory experience. Trends Neurosci. 28, 248–254.
Lonstein, J. S., and Fleming, A. S. (2002). Parental Behaviors in Rats and Mice. Current Protocols in Neuroscience New york: John Wiley & Sons, Inc., 8.15.1–8.15.26.
Lucas, B. K., Ormandy, C. J., Binart, N., Bridges, R. S., and Kelly, P. A. (1998). Null mutation of the prolactin receptor gene produces a defect in maternal behavior. Endocrinology 139, 4102–4107.
Luo, J., Daniels, S. B., Lennington, J. B., Notti, R. Q., and Conover, J. C. (2006). The aging neurogenic subventricular zone. Aging Cell 5, 139–152.
Macbeth, A. H., Edds, J. S., and Young, W. S. (2009a). Housing conditions and stimulus females: a robust social discrimination task for studying male rodent social recognition. Nat. Protoc. 4, 1574–1581.
Macbeth, A. H., Lee, H.-J., Edds, J., and Young, W. S. (2009b). Oxytocin and the oxytocin receptor underlie intrastrain, but not interstrain, social recognition. Genes Brain Behav. 8, 558–567.
Magavi, S. S. P., Mitchell, B. D., Szentirmai, O., Carter, B. S., and Macklis, J. D. (2005). Adult-born and preexisting olfactory granule neurons undergo distinct experience-dependent modifications of their olfactory responses in vivo. J. Neurosci. 25, 10729–10739.
Mak, G. K., Enwere, E. K., Gregg, C., Pakarainen, T., Poutanen, M., Huhtaniemi, I., and Weiss, S. (2007). Male pheromone-stimulated neurogenesis in the adult female brain: possible role in mating behavior. Nat. Neurosci. 10, 1003–1011.
Mak, G. K., and Weiss, S. (2010). Paternal recognition of adult offspring mediated by newly generated CNS neurons. Nat. Neurosci. 13, 753–758.
Mann, P. E., and Bridges, R. S. (2001). Lactogenic hormone regulation of maternal behavior. Prog. Brain Res. 133, 251–262.
Manning, C. J., Wakeland, E. K., and Potts, W. K. (1992). Communal nesting patterns in mice implicate MHC genes in kin recognition. Nature 360, 581–583.
Monje, M. L., Mizumatsu, S., Fike, J. R., and Palmer, T. D. (2002). Irradiation induces neural precursor-cell dysfunction. Nat. Med. 8, 955–962.
Morè, L. (2006). Mouse major urinary proteins trigger ovulation via the vomeronasal organ. Chem. Senses 31, 393–401.
Moreno, M. L. M., Linster, C., Escanilla, O., Sacquet, J. L., Didier, A., and Mandairon, N. (2009). Olfactory perceptual learning requires adult neurogenesis. Proc. Natl. Acad. Sci. U.S.A. 106, 17980–17985.
Myers, M. M., Brunelli, S. A., Squire, J. M., Shindeldecker, R. D., and Hofer, M. A. (1989). Maternal behavior of SHR rats ad its relationship to offspring blood pressures. Dev. Psychobiol. 22, 29–53.
Nemoto, M., Morita, Y., Mishima, Y., Takahashi, S., Nomura, T., Ushiki, T., Shiroishi, T., Kikkawa, Y., Yonekawa, H., and Kominami, R. (2004). Ahl3, a third locus on mouse chromosome 17 affecting age-related hearing loss. Biochem. Biophys. Res. Commun. 324, 1283–1288.
Nissant, A., Bardy, C., Katagiri, H., Murray, K., and Lledo, P.-M. (2009). Adult neurogenesis promotes synaptic plasticity in the olfactory bulb. Nat Neurosci. 12, 728–730.
Ostermeyer, M. C., and Elwood, R. W. (1983). Pup recognition in Mus musculus: parental discrimination between their own and alien young. Dev. Psychobiol. 16, 75–82.
Penn, D., and Potts, W. K. (1998). Untrained mice discriminate MHC-determined odors. Physiol. Behav. 63, 235–243.
Potts, W. K., Manning, C. J., and Wakeland, E. K. (1991). Mating patterns in seminatural populations of mice influenced by MHC genotype. Nature 352, 619–621.
Ramm, S. A., Cheetham, S. A., and Hurst, J. L. (2008). Encoding choosiness: female attraction requires prior physical contact with individual male scents in mice. Proc. R. Soc. Lond., B, Biol. Sci. 275, 1727–1735.
Rochefort, C., Gheusi, G., Vincent, J.-D., and Lledo, P.-M. (2002). Enriched odor exposure increases the number of newborn neurons in the adult olfactory bulb and improves odor memory. J. Neurosci. 22, 2679–2689.
Sherborne, A. L., Thom, M. D., Paterson, S., Jury, F., Ollier, W. E. R., Stockley, P., Beynon, R. J., and Hurst, J. L. (2007). The genetic basis of inbreeding avoidance in house mice. Curr. Biol. 17, 2061–2066.
Shingo, T., Gregg, C., Enwere, E., Fujikawa, H., Hassam, R., Geary, C., Cross, J. C., and Weiss, S. (2003). Pregnancy-stimulated neurogenesis in the adult female forebrain mediated by prolactin. Science 299, 117–120.
Singer, A. G., Beauchamp, G. K., and Yamazaki, K. (1997). Volatile signals of the major histocompatibility complex in male mouse urine. Proc. Natl. Acad. Sci. U.S.A. 94, 2210–2214.
Stern, J. M., and Lonstein, J. S. (2001). Neural mediation of nursing and related maternal behaviors. Prog. Brain Res. 133, 263–278.
Sultan, S., Mandairon, N., Kermen, F., Garcia, S., Sacquet, J., and Didier, A. (2010). Learning-dependent neurogenesis in the olfactory bulb determines long-term olfactory memory. FASEB J. 24, 2355–2363.
Valley, M. T., Mullen, T. R., Schultz, L. C., Sagdullaev, B. T., and Firestein, S. (2009). Ablation of mouse adult neurogenesis alters olfactory bulb structure and olfactory fear conditioning. Front Neurosci. 3, doi: 10.3389/neuro.22.003.2009.
Vandenbergh, J. G. (1973). Effects of central and peripheral anosmia on reproduction of female mice. Physiol. Behav. 10, 257–261.
Whitman, M. C., and Greer, C. A. (2007). Synaptic integration of adult-generated olfactory bulb granule cells: basal axodendritic centrifugal input precedes apical dendrodendritic local circuits. J. Neurosci. 27, 9951–9961.
Whitten, W. K. (1966). “Pheromones and mammalian reproduction,” in Advances in Reproductive Physiology, Vol. 1, ed. A. McLaren (New York: Academic Press), 155–177.
Winslow, J. T. (2003). Mouse social recognition and preference. Curr. Protoc. Neurosci. 22, 8.16.11–18.16.16.
Yamazaki, K., Beauchamp, G. K., Curran, M., Bard, J., and Boyse, E. A. (2000). Parental and progeny recognition as a function of MHC odortype identity. Proc. Natl. Acad. Sci. U.S.A. 97, 10500–10502.
Yokoi, M., Mori, K., and Nakanishi, S. (1995). Refinement of odor molecule tuning by dendrodendritic synaptic inhibition in the olfactory bulb. Proc. Natl. Acad. Sci. U.S.A. 92, 3371–3375.
Keywords: mate recognition, offspring recognition, maternal behavior, olfaction, neurogenesis, irradiation, social interaction
Citation: Feierstein CE, Lazarini F, Wagner S, Gabellec M-M, de Chaumont F, Olivo-Marin J-C, Boussin FD, Lledo P-M and Gheusi G (2010) Disruption of adult neurogenesis in the olfactory bulb affects social interaction but not maternal behavior. Front. Behav. Neurosci. 4:176. doi: 10.3389/fnbeh.2010.00176
Received: 22 July 2010;
Accepted: 27 October 2010;
Published online: 01 December 2010.
Edited by:
Serge Laroche, CNRS and University Paris-Sud, FranceReviewed by:
Silvia De Marchis, University of Turin, ItalyHeather A. Cameron, National Institute of Mental Health, USA
Copyright: © 2010 Feierstein, Lazarini, Wagner, Gabellec, de Chaumont, Olivo-Marin, Boussin, Lledo and Gheusi. This is an open-access article subject to an exclusive license agreement between the authors and the Frontiers Research Foundation, which permits unrestricted use, distribution, and reproduction in any medium, provided the original authors and source are credited.
*Correspondence: Pierre-Marie Lledo, Laboratory for Perception and Memory, Institut Pasteur, 25 rue du Dr. Roux, F-75015 Paris Cedex, France. e-mail: pmlledo@pasteur.fr; Claudia E. Feierstein, Champalimaud Neuroscience Programme, Instituto Gulbenkian de Ciência, Rua da Quinta Grande, 6, P-2780-156 Oeiras, Portugal. e-mail: claudia.feierstein@gmail.com