- 1Department of Marine Science and Fisheries, College of Agricultural and Marine Sciences, Sultan Qaboos University, Al Khoud, Oman
- 2Nanotechnology Research Center, Sultan Qaboos University, Muscat, Oman
- 3Department of Physics, College of Science, Sultan Qaboos University, Muscat, Oman
- 4Department of Petroleum and Chemical Engineering, College of Engineering, Sultan Qaboos University, Muscat, Oman
- 5UNESCO Chair in Marine Biotechnology, Centre of Excellence in Marine Biotechnology, Sultan Qaboos University, Muscat, Oman
Biofouling in the cooling systems of greenhouses in arid climates is a major problem. The current study describes the usage of coatings composed of copper oxide microparticles (CuO MPs) and zinc oxide nanoparticles (ZnO NPs) in greenhouse cooling systems for biofouling protection. A simple spray coating method was employed to coat cooling cardboard with the CuO MPs and ZnO NPs. Biofouling properties of coated cardboard were investigated as antimicrobial activities in laboratory experiments under light and dark conditions using different types of Gram-positive and Gram-negative bacteria isolated from the greenhouse. CuO MPs coating showed the strongest antimicrobial activity compared to ZnO NPs in Gram-positive bacteria (Bacillus infantis) under both conditions. Almost the same antimicrobial activity was observed for CuO MPs and ZnO NPs with Gram-negative (Escherichia coli) bacteria. Furthermore, the antialgal activity of the coatings was investigated against Scenedesmus sp. and Pinnularia sp. isolated from the greenhouse. Results demonstrated that CuO MPs exhibited the strongest antialgal activity. The observed antifouling activity was mainly due to the production of reactive oxygen species (ROS) and ions from ZnO NPs and CuO MPs coatings. The results of this study suggest that CuO MPs coatings can be applicable for antifouling protection of greenhouse cooling systems.
1 Introduction
The global wastewater discharge rate is 400 billion cubic meters (m3) per year, contaminating approximately 5,500 billion m3 of water each year (Zhang and Shen, 2019). Ineffective discharge of non-treated wastewater into the environment may cause contamination by pollutants and the discharge and growth of harmful pathogens (Dacewicz and Chmielowski, 2018). In dry and semiarid areas, one of the possible uses of treated wastewater after treatment is irrigation, particularly in dry and semiarid areas, due to the low availability of water and high content of nutrients like nitrogen and phosphorus (Zhang and Shen, 2019). Moreover, treated wastewater contains sufficient levels of nutrients for plant growth, thus promoting plant growth and thereby reducing the need for chemical fertilizers (Ghernaout, 2018). Treated wastewater irrigation is already utilized in many regions of the world, primarily for orchards and landscaping. However, treated wastewater irrigation of vegetables is prohibited due to the potential health dangers that such water may produce pathogens for consumers of fresh vegetables (Obayomi et al., 2019). Moreover, treated wastewater contains sufficient nutrients for plant growth, thus reducing the use of chemical fertilizers.
The Sultanate of Oman is an arid and semiarid country with temperatures exceeding 45°C in summer and an average annual rainfall of 100 mm per year (Al-Ismaili and Jayasuriya, 2016). Therefore, Oman’s weather is not highly suitable for open-field cultivation, especially in the summertime, which is almost 8 months of the year (Al-Ismaili and Jayasuriya, 2016). Besides, around 52% of the agricultural lands were banned due to salt accumulation that came from saline irrigation which mainly happened in the Al-Batinah region due to seawater intrusion. To overcome the problem of hot weather, Controlled Environment Agriculture (CEA) was widely adopted for vegetable crops throughout the country. It aims to improve the quality and quantity of crops through a controlled environment in greenhouses. Al-Ismaili and Jayasuriya (2016) reported that greenhouse production rises 12 times more than open field-grown crops in Oman due to CEA.
To reduce the temperature in a greenhouse in an arid climate, an air heat exchanger and fogging (an evaporative cooling system) are used to control the interior temperature and humidity (Hegazy et al., 2022). Thus, greenhouse cooling pads are constructed in the ventilation opening of the pad-and-fan framework, so that practically all incoming ventilation air travels through the pads before reaching the greenhouse area. Typically, these pads are made of cardboard and are stuck together in a way that air can pass through them while at the same time maintaining the optimum contact area between the air and the material of the wet pad (Figure 1). Water is drained to the top of the pads and released through small holes along the whole of the supply pipe. The spacing of the hole is designed to ensure that the passing water wets the entire pad surface consistently without allowing the patches to dry out. Water at the bottom of the pads is collected and returned to a storage tank to be reused (Both, 2007). When the warm, dry air flows through the pad, some of the water evaporates. The air passing over the pad is thus cooled and humidified at the same time due to the evaporation process, which requires no additional energy source. As a result, the greenhouse atmosphere’s humidity rises and the temperature falls (Jain and Hindoliya, 2011). Such a system allows for a reduction in the freshwater consumed by 50% and the interior temperature reduced from 50 to 35o C. To reduce the amount of freshwater used in such greenhouses, it is proposed to use treated wastewater to cool greenhouses. However, compared to freshwater, treated wastewater is rich in nutrients that could support algal growth and cause significant biofouling in the cooling system of the greenhouse (Al-Busaidi et al., 2022).
Biofouling is the undesirable deposition and growth of microorganisms on wetted surfaces (Flemming, 2002). Biofouling blocks the cooling pads, affects the efficiency of the greenhouse cooling system and reduces the lifetime and efficiency of the cardboard used in the cooling system. Moreover, it is unclear if the treated water could be a source of any diseases by spreading harmful microbes inside the greenhouse, affecting human health and contaminating crops. Although wastewater treatment systems are designed to minimize the concentration of contaminants, many wastewater treatment plants release such effluents without chlorination, resulting in the accumulation of pathogens (Iwane et al., 2001). Examples of such bacteria include Escherichia coli which has been commonly used in aquatic environments as a fecal pollution predictor. Most strains of this bacterium are considered nonpathogenic, while some strains may be pathogenic (Iwane et al., 2001). Also, Pseudomonas mendocina, Enterobacter kobei, and Pseudomonas alcaligenes are soil and water pathogenic bacteria (Wehr et al., 2015; Brooke, 2012). Thus, the development of an effective antifouling and antimicrobial treatment for the greenhouse cooling system is required.
To prevent biofouling growth in industrial applications various methods are used (Davidson et al., 2021). These methods range from using toxic biocides, like copper, to non-toxic approaches that leverage the physical properties of surfaces including microtopography and wettability (Weber and Esmaeili, 2023). However, while toxic methods can effectively prevent biofouling, they pose significant environmental risks (Weber and Esmaeili, 2023). Conversely, physical methods are not always effective, highlighting the need for better biofouling management.
To deal with biofouling problems, it is important to develop non-toxic or low-toxic antifouling methods (Bora and Dutta, 2014). Nanotechnology in recent years has been used to provide a solution for wastewater treatment as well as the prevention of biofouling (Kumar et al., 2021). Nanotechnology uses nanoscale materials with dimensions ranging from a few nanometers to approximately 100 nm in any or all dimensions (Bora and Dutta, 2014). Nanomaterials usually have a high degree of reactivity and functionalization, a broad higher specific surface area, and size-dependent properties, among other things, making them ideal for wastewater treatment and water purification. For example, zinc oxide nanoparticles (ZnO NPs) have antibacterial, antifouling, and anti-algal properties and low toxicity (Dobretsov et al., 2020; Al-Fori et al., 2014; Sathe et al., 2017). It was proven that ZnO NPs have lower toxicity to different trophic-level organisms than Zn ions (Dobretsov et al., 2020; Falfushynska et al., 2019). Similarly, copper oxide nano and microparticles (CuO MPs) have antimicrobial properties (Chapman et al., 2013). CuO MPs is less toxic compare to CuO NPs. Thus, it is possible that ZnO NPs and CuO MPs can prevent biofouling in the cooling systems of greenhouses.
The main aim of this study was to develop CuO microparticles (MPs) and ZnO nanoparticles (NPs) coatings on the cardboard of a greenhouse cooling system and test their antifouling properties. The specific objectives of this study were to: 1) make CuO MPs and ZnO NPs coatings on the cooling cardboard and characterize them; 2) test the antifouling properties of CuO MPs and ZnO NPs coatings using freshwater Gram-positive (B. infantis) and Gram-negative bacteria (E. coli) and microalgae (Scenedesmus sp. and Pinnularia sp.) isolated from a greenhouse cooling system; and 3) investigate leaching of metal ions and the production of reactive oxygen species (ROS) from CuO MPs and ZnO NPs coatings. The results of this work have the potential for the prevention of biofouling in greenhouse cooling of agricultural systems.
2 Materials and methods
2.1 Materials
Copper (II) sulfate pentahydrate and ascorbic acid were purchased from Fisher Scientific, Loughborough, United Kingdom. Sodium hydroxide was procured from Honeywell, Germany. Absolute ethanol and terephthalic acid were obtained from Merck, Darmstadt, Germany. Standard deionized water (DI) was used throughout the experiment. Nutrient broth was obtained from Sigma-Aldrich. In this experiment, research grade zinc oxide nanoparticles (>99%) were obtained from US Research Nanomaterials Inc., United States (Houston, TX, United States) and used without any further modification but copper oxide microparticles were synthesized using the procedure shown below. An evaporating air-cooling system cardboard for a greenhouse was obtained from China Anhui Jimei Air Treatment Equipment Co. (Beijing, China).
2.2 Synthesis of CuO microparticles
The green chemical reduction process was used to synthesize CuO microparticles (MPs) (Izwanie et al., 2019). To do this, 0.1 M copper (II) sulfate pentahydrate solution was added to 1.44 g of starch that dissolved in 100 mL of deionized water under continuous stirring for half an hour (30 min). Then, 50 mL of 0.2 M ascorbic acid solution was added to the synthesis solution. After that, 30 mL of 1 M sodium hydroxide solution was slowly added to the prepared solution and heated at 80°C for 2 h with constant stirring until the color of the solution changed from yellow to ocher. Then, the solution was removed from the hot plate and kept at room temperature overnight to allow the particles to precipitate and the supernatant solution was carefully discarded. A vacuum filtration system with 0.2 µm pore size and 47 mm diameter filter paper was used to separate the precipitates from the solution. To remove the excess starch bound with the particles, the precipitates were washed with deionized water and ethanol 3 times. Finally, the microparticles were dried in a desiccator for 2 days and stored in a glass vial for further analysis (Khan et al., 2016).
2.3 Spray coating of cardboards with Cu microparticles and ZnO nanoparticles
To make coatings, 0.025 g of commercial ZnO NPs with the size of 30–45 nm and the synthesized CuO MPs particles were dispersed separately in 50 mL of ethanol (Al-Belushi et al., 2020). The solution was sprayed by using a commercial spray gun on cooling system cardboard (30 cm × 15 cm x 5 cm). After spraying, the cardboard was dried at room temperature and covered with aluminum foil for further use. These cardboards were used for antibacterial and anti-algal testing, respectively (see below).
2.4 Characterizations
The crystal structure of CuO MPs and ZnO NPs was characterized using X-ray diffraction (Rigaku Miniflex 600, Tokyo, Japan). Surface morphology and elemental composition were observed by field emission scanning electron microscopy (JEOL JSM 7800F, Tokyo, Japan) attached with energy dispersive X-ray spectroscopy (Oxford instrument, United Kingdom). Zeta potential measurements of ZnO NPs and CuO MPs were conducted using Zeta Analyzer (NICOMPTM 380 ZLS, United States).
2.5 Antibacterial testing
The antibacterial activity of CuO MPs and ZnO NPs coatings was tested in the laboratory. Gram-positive and Gram-negative freshwater bacteria were used. First, the bacteria B. infantis and E. coli were isolated from the fouled cardboard of the greenhouse (Sultan Qaboos University, Agricultural and Experimental Station, Muscat, Oman). Bacteria were identified using a MALDI-TOF (matrix-assisted laser desorption ionization/time of flight) biotyper (Bruker Daltonics, Leipzig, Germany). This method identifies bacteria based on a unique proteomic fingerprint. Second, the bacteria were incubated at 37°C for 24 h in a sterile nutrient broth (Sigma-Aldrich, St. Louis, MO, United States). Third, a small piece (1 cm × 1 cm) of the cardboard coated with CuO MPs and ZnO NPs was placed in a multi-well plate (Thermo Fisher Scientific, Waltham, MA, United States). Uncoated cardboard was used as a control. Fourth, 3 mL of sterile nutrient broth was mixed with the bacterial culture and added to each well. There were three replicates in this experiment. Two experiments were conducted: in the dark and in the light. Light conditions were 30.0–31.5 kLux light intensity generated by white luminescence lamps. To make the dark conditions (0 Lux), all samples were covered with aluminum foil. For B. infantis, a bacterial culture of around 10 CFU/mL was combined with 3 mL of sterile marine broth and put into each well. For E. coli, a bacterial culture of around 12 CFU/mL was combined with 3 mL of sterile nutrient broth and added to each well. In both experiments, plates were incubated at 37°C for 24 h. The optical density (OD at 600 nm) of all treatments was measured after 0 and 24 h using a spectrophotometer (Thermo Fisher Scientific United States, Waltham, MA, United States). Antibacterial activity of CuO MPs, ZnO NPs, and control (uncoated cardboard) was counted as Log CFU/mL using 23.018*OD + 0.0326 equation for B. infantis and 23.846*OD + 0.0732 for E. coli.
2.6 Anti-algal test
Freshwater algae (Scenedesmus sp., Chlorophyta and Pinnularia sp., Bacillariophyta) from the treated wastewater cooling system of the greenhouse (Sultan Qaboos University, Agricultural and Experimental Station, Muscat, Oman) were used in this study. Algae were isolated according to Lee et al. (2014). Before the experiment, algae were cultivated for 7 days in F2 media at 27°C with a photoperiod of 12/12 (Light/Dark, h) and light intensity of 5 kLux. For the experiment, small pieces (1 cm × 1 cm) of cardboard coated with CuO MPs, ZnO NPs, and an uncoated one (control) were used. There were three replicates for each treatment. All samples were incubated at 27°C and in a 12 h light: dark photoperiod (Intensity: 30.0–31.5 kLux). The experiment was conducted for 72 h. The growth of algae was measured as the OD at the wavelength of 664 nm after 72 h.
2.7 Leaching test
To conduct a leaching test, 1 × 1 cm of cardboard coated with CuO MPs, ZnO NPs, and uncoated ones were immersed in 100 mL of deionized water (DI). 10 mL from each sample were collected after 72 h. There were three replicates for each treatment. Inductively coupled plasma-optical emission spectrometry (ICP-OES, Perkin Elmer 8000 DV, Waltham, MA, United States) was utilized to analyze the concentration of Zn2+ and Cu2+ ions from the leaching test.
2.8 Reactive oxygen species test
To probe reactive oxygen species (ROS) from ZnO NPs and CuO MPs, 0.5 mM terephthalic acid (TA) was prepared in 0.2 mM aqueous NaOH solution. ZnO NPs and CuO MPs deposited cardboard, and was cut to 1 cm2 and kept in the 5 mL TA solution. The samples with TA solutions were placed under the solar-simulated light at 1000 W/m2 (Sciencetech, Canada). The emission light was measured using a photoluminescence spectrometer (PerkinElmer LS 55, United States) with an excitation wavelength of 315 nm after 1 h of visible light irradiation to the samples.
2.9 Data analysis
Means and standard errors were calculated for bacterial density and algal density using Excel software. The errors were calculated using the standard deviation based on three multiple measurements. The differences between densities of bacteria and algae in different treatments were analyzed using ANOVA followed by Tukey’s post hoc test. Before the test, the normality and homogeneity of the data were verified by the Shapiro-Wilk and Levene tests. In all cases, the significant difference was P < 0.05.
3 Results
3.1 X-ray diffraction (XRD) and zeta potential
XRD patterns of CuO MPs and ZnO NPs on cardboard show different diffraction peaks at specific degrees. CuO MPs have diffraction peaks at 43.16o, 50.27o, and 83.97o which can be assigned as (110), (200) and (311) planes of monoclinic CuO crystal structure (Khashan et al., 2018; Menamo et al., 2017; ElFaham et al., 2021) (Figure 2a). However, the ZnO NPs have diffraction peaks at 31.7°, 34.35°, 36.18°, 47.42°, 56.52°, 62.77°, 66.28°, 67.88°, 69.02, 72.50° and 76.90° which can be assigned as (100), (002), (101), (102), (110), (103), (200), (112), (201), (004) and (201) planes of hexagonal wurtzite ZnO crystal structure (Ashkarran, 2012) (Figure 2b). in Figure 1a and b showed sharp diffraction peaks indicating well crystalline structure of CuO MPs and ZnO NPs.
The average zeta potentials of ZnO NPs and CuO MPs were found to be −17.23 mV and −50.21 mV, respectively.
3.2 CuO MPs and ZnO NPs coated cardboard surface: surface morphology and elemental analysis
CuO MPs and ZnO NPs were successfully deposited on the cooling system cardboard (Figure 3). Some unwanted debris was observed in pristine uncoated cardboard surfaces, and EDS analysis displayed the elements of O, C, Si, Al, Fe, K, Mg, and Na (Figures 3a, b). These materials might come from the environment and the cardboard materials themselves (Figure 3b). For CuO MPs coated on the cardboard surface, CuO MPs existed as in agglomerated form with a mean diameter of ∼ 2–8 µm (Figure 3c), which comprised C, O, and Cu, respectively. Apart from the expected elements (C, O, and Cu), no other elements were detected. This could be due to the removal of other components from the original surface while spraying CuO MPs onto the cardboard (Figure 3d). On the other hand, ZnO NPs with a diameter of ∼30–45 nm (Figure 3e) were randomly coated on the cardboard in an aggregated way, and the EDS result showed the presence of C, O, and Zn on the surface (Figure 3f).
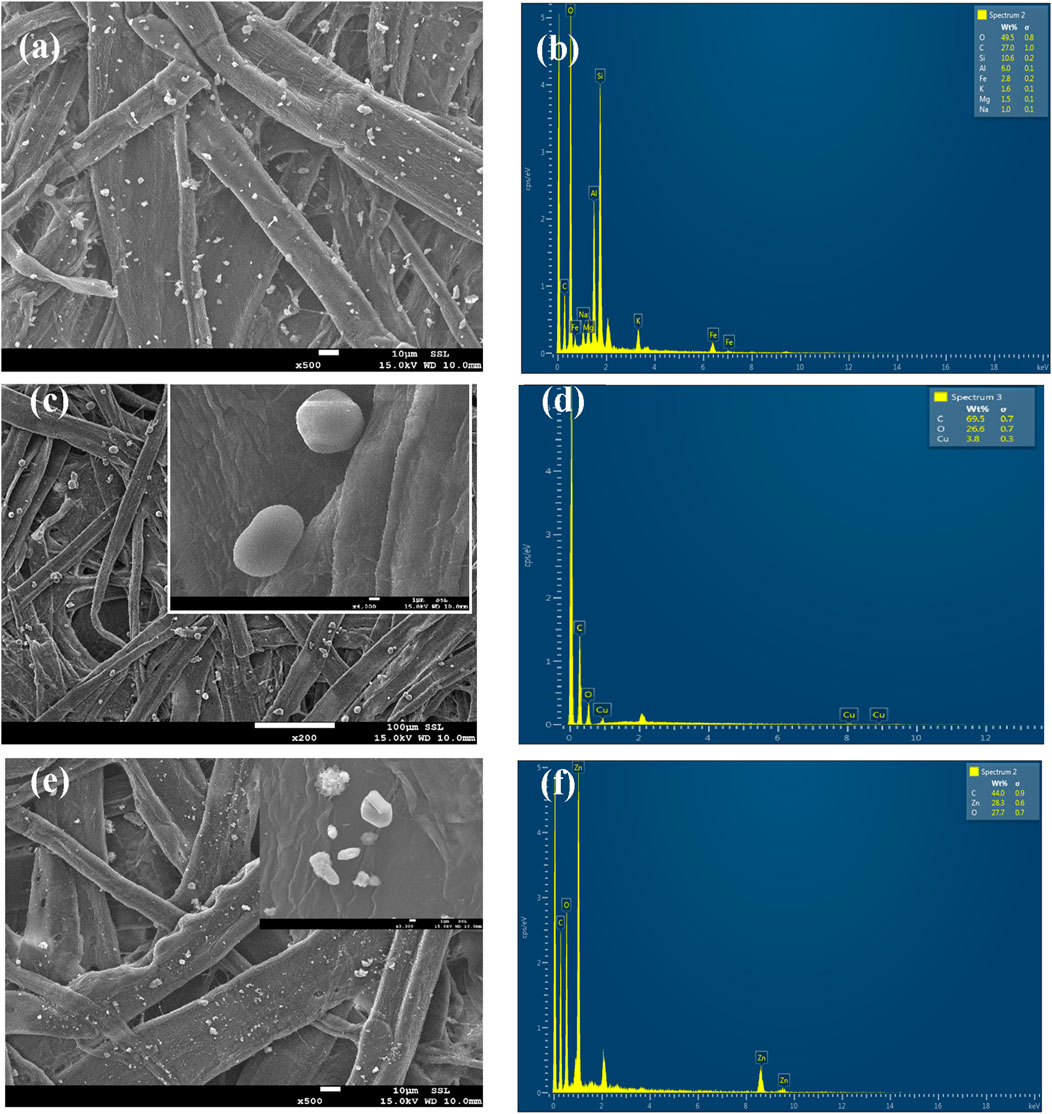
Figure 3. The scanning electron microscope (SEM) images and energy-dispersive X-ray spectroscopy of the cooling system cardboard with different coatings: (a) and (b) pristine, uncoated cardboard, (c, d) CuO MPs, and (e) and (f) ZnO NPs coatings on cardboard. The ZnO NPs and CuO MPs are randomly distributed on the cardboard surface.
3.3 Antibacterial test with gram-positive bacteria under different treatments
Under light conditions, the average growth of Gram-positive bacteria B. infantis bacteria was increased in all treatments. After 24h, the growth of B. infantis in the presence of CuO MPs coating was lower by 39.0% than ZnO NPs, and by 44.0% than the control (bacteria and carboard) (Figure 4). It can be seen that there was a significant difference in bacterial growth between treatments (ANOVA, P = 0.0005) (Supplementary Table S1). There were significant differences in the growth of B. infantis between CuO MPs and ZnO NPs and between CuO MPs and the control (Tukey post hoc, p < 0.05) (Supplementary Table S2). The lowest growth was observed in the case of CuO MPs coating.
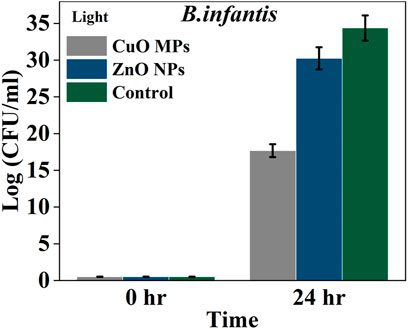
Figure 4. Average density Log(CFU/mL) of B. infantis in the presence of CuO MPs, ZnO NPs, and control (bacteria and cardboard) light conditions.
In the dark condition, the average growth of Gram-positive bacteria B. infantis was decreased after 24 h in CuO MPs and ZnO NPs treatments, while it increased in the control (bacteria and cardboard) (Figure 5). The growth of B. infantis in the presence of CuO MPs coating after 24 h was lower by 68.1% than ZnO NPs and by 89.3% than the control (Figure 5). It can be seen that there was a significant difference in bacterial growth between treatments (ANOVA, p = 0.0003) (Supplementary Table S3). Additionally, there were significant differences in the growth of B. infantis between ZnO NPs and control and between CuO MPs and control in the dark (Tukey post hoc, p < 0.05) (Supplementary Table S4).
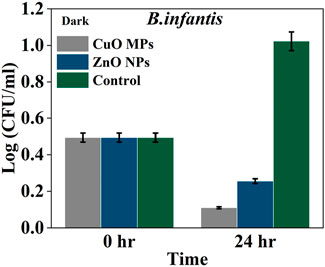
Figure 5. Average density Log(CFU/mL) of B. infantis in the presence of CuO MPs, ZnO NPs, and control (bacteria and cardboard) in dark conditions.
3.4 Antibacterial test with gram-negative bacteria under different treatments
Under light conditions, the average growth of Gram-negative E. coli bacteria was increased after 24 h in all treatments (Figure 6). The growth of E. coli was lower in CuO MPs treatment by 56.0% than ZnO NPs and 61.0% than the control (bacteria and cardboard). It can be seen that there was a significant difference in bacteria growth between treatments (ANOVA, p = 0.0046) (Supplementary Table S5). There were significant differences in the growth of E. coli between ZnO NPs and the control and between CuO MPs and the control (p < 0.05) (Tukey post hoc, Supplementary Table S6). The lowest growth was observed in the case of CuO MPs coating.
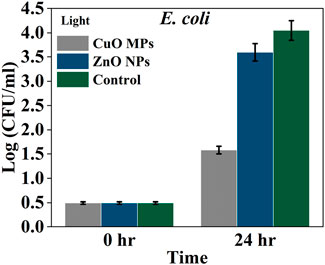
Figure 6. Average density Log(CFU/mL) of E. coli in the presence of CuO MPs, ZnO NPs, and control (bacteria and cardboard) in light conditions.
In the dark condition, the growth of all Gram-negative bacteria was increased after 24 h in all treatments (Figure 7). The lowest growth was in the presence of CuO MPs coating, followed by ZnO NPs and control treatments. The growth of E. coli was lower than that in CuO MPs treatment by 16.2% than ZnO NPs and 53.5% than in control (Figure 7). It can be seen that there was no significant difference in the growth of bacteria between all treatments (ANOVA, p = 0.0007) (Supplementary Table S7). However, there were significant differences in the growth of E. coli between ZnO NPs and control treatments and between CuO MPs and control treatments (Tukey post hoc, p < 0.05) (Supplementary Table S8). Both ZnO NPs and CuO MPs coatings were effective in reducing E. coli growth in the dark condition.
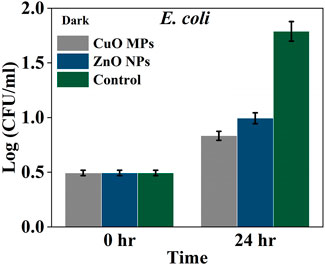
Figure 7. Average density Log(CFU/mL) of E. coli in the presence of CuO MPs, ZnO NPs and control (bacteria and cardboard) in dark conditions.
3.5 Anti-algal test with growth of algae in different treatments
The average growth of the green alga Scenedesmus sp. (Chlorophyceae) was slightly increased after 72 h in CuO MPs and ZnO NPs and significantly in the control treatment (Figure 8a). However, the growth of Scenedesmus sp. expressed as optical density (OD) was significantly lower in the case of CuO MPs by 87.8% and ZnO NPs by 84.2% compared to control treatments (algae with cardboard) (Supplementary Tables S9, S10).
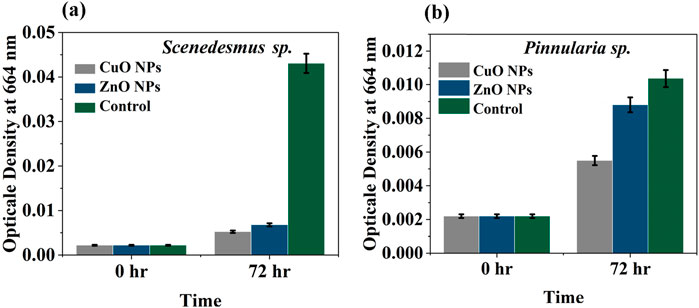
Figure 8. Average growth of algae expressed as OD664 after 72 h in the presence of Cu NPs, ZnO NPs and control (algae and cardboard) against Scenedesmus sp. (a) and Pinnularia sp. (b).
The growth of a diatom Pinnularia sp. (Bacillariophyceae) in all treatments was increased after 72 h (Figure 8b). The lowest growth was observed in the presence of CuO MPs cardboard, while the highest growth was found in the control treatment. The growth of Pinnularia sp. increased in ZnO NPs and the control treatments by 37.5% and 46.9%, respectively, compared to the CuO MPs treatment (Figure 7b). In the case of CuO MPs, the growth of Pinnularia sp. was significantly lower compared to the control. There was no difference in Pinnularia sp. growth between ZnO and control treatments (ANOVA, P = 0.235) (Supplementary Table S10).
3.6 Leaching test and ROS production
As expected, Zn2+ and Cu2+ ions were released from ZnO NPs and CuO MPs coatings, correspondingly (Figure 9a). The leaching test demonstrated the presence of Cu2+ ions in all samples including control ones (Figure 9a). This suggests that the cardboard surface contains Cu-based compounds that leached during the experiment. The highest ion concentration was observed in the case of ZnO NPs (Zn2+ = 0.038 ppm). The average leaching of Cu2+ ions was higher in the case of CuO MPs by 57.1% compared to the control treatment. Cu2+ ions released in the case of ZnO NPs coatings could be due to the presence of Cu in cardboard. There was a significant difference in the leaching of Zn2+ and Cu2+ ions between different treatments (ANOVA, P = 1E-20) (Supplementary Table S11).
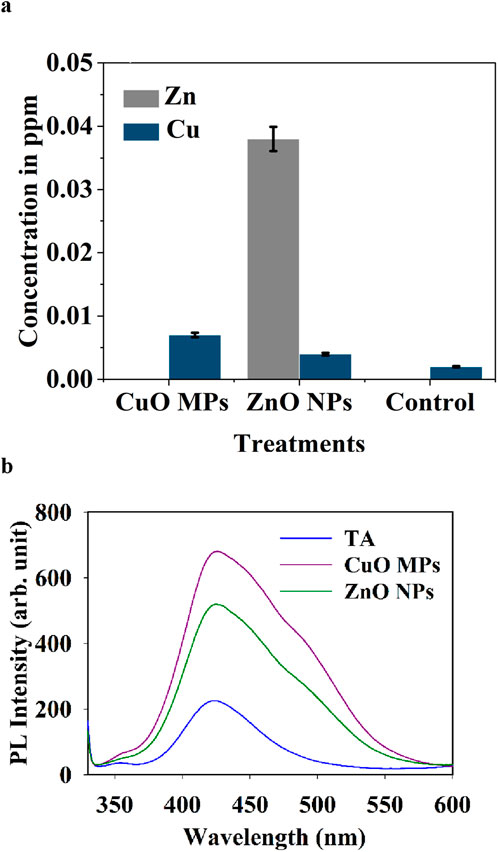
Figure 9. (a) Average concentrations (ppm) of Cu2+ and Zn2+ ions in the presence CuO MPs, ZnO NPs coatings and control (cardboard) after 72 h (b). Photoluminescence emission spectra of terephthalic acid (TA) reacts with (•OH) produced from the ZnO NPs and CuO MPs coatings under visible light irradiation.
ROS presence was confirmed by observing the production of •OH from the ZnO NPs and CuO MPs surfaces in PL measurement (Figure 9b). Although the size of CuO MPs is bigger compared with ZnO NPs, the PL emission results show that CuO MPs produce more (•OH) production than ZnO NPs.
4 Discussion
To grow crops, countries with arid and semi-arid climates use greenhouses with an evaporative cooling system to reduce the temperature and increase humidity (Hegazy et al., 2022). However, biofouling of evaporative pads of the cooling system of greenhouses impacted their performance and increased the cost of operation. In this study, we isolated bacteria and microalgae from a greenhouse and tested the antifouling properties of copper oxide microparticles (CuO MPs) and zinc oxide nanoparticles (ZnO NPs) coatings of cooling system evaporative cardboards of greenhouses. The CuO MPs used in this study were synthesized using a green chemistry approach. This environmentally friendly method results in the formation of micro-sized particles rather than nanoparticles. Additionally, CuO MPs are less toxic than CuO NPs (Maciel-Magalhães et al., 2020) and have a reduced environmental impact.
4.1 Bacteria and microalgae isolated from the greenhouse
In this study, the bacteria B. infantis and E. coli from treated wastewater and fouled cardboard of the greenhouse were isolated. B. infantis is a Gram-positive bacterium that has been previously isolated from waste (Moumita and Tapobrata, 2024), soil (Saggu and Mishra, 2017), and marine waters (Soni et al., 2023). According to Vaz-Moreira et al. (2012) and Kumar et al. (2016), B. infantis is commonly found in freshwater, drinking water treatment plants, and treated wastewater treatment plants. This bacterium could be pathogenic and isolated from the blood of newborns (Ko et al., 2006) and could induce heart autoimmunity (Massilamany et al., 2016). To our knowledge, this is the first record of the isolation of B. infantis from a greenhouse cooling system and it could be due to the use of waste-treated water. On the opposite, E. coli is a Gram-negative bacterium. It is usually harmless; however, since this bacterium is associated with the lower intestine, its presence indicates water contamination. E. coli was previously found in soil (Sharma et al., 2016), water (Lopez-Galvez et al., 2016), as well as fruits and vegetables in greenhouses (Shaw et al., 2015). The presence of this bacterium is probably due to the use of wastewater for cooling in our study. The health risks posed by B. infantis and E. coli in greenhouse settings are significant because they directly impact food safety, human health, and the performance of the heat exchanger of greenhouses.
In addition, the green alga Scenedesmus sp. and the diatom Pinnularia sp. were isolated in this study. Scenedesmus sp. is primarily found in freshwater environments (Xin et al., 2011). These algae are characterized by their non-motile nature and typically form colonies consisting of 2–32 cells surrounded with mucus. Under some conditions, this alga can adhere to surfaces and form biofilms (Tsavatopoulou and Manariotis, 2020). Previously, it was reported that Scenedesmus is responsible for biofouling of an oil produced water treatment plant (Johnson et al., 2016). These publications could explain the presence of this alga on cooling pads in the greenhouse. Pinnularia sp. is a unicellular diatom alga and a common biofouling species. It is commonly found in freshwater environments with high levels of organic pollution attached to different substrata (Brake and Hasiotis, 2010). The presence of nutrients in wastewater in our study could explain the growth of this alga on cooling pads. However, neither Scenedesmus nor Pinnularia were recorded in the cooling pads of greenhouses before this study. According to our observations, Scenedesmus sp. and Pinnularia sp. directly impact the performance of greenhouses.
4.2 Antibacterial and antialgal activity of coatings
Under the light conditions, the lowest density of Gram-positive bacteria B. infantis was observed in CuO MPs coating, while the highest density was found on ZnO NPs coating and control (not coated cardboard). Similarly, CuO MPs coating showed a significant reduction in the growth of Gram-negative bacteria E. coli in comparison to the control. In comparison to the light condition, in the dark, bacterial growth was lower. In the dark conditions, both CuO MPs and ZnO NPs coatings significantly reduced the growth of bacteria. However, the growth of Gram-positive B. infantis was reduced strongly than Gram-positive E. coli. Differences in the cell wall structure of Gram-positive and Gram-negative bacteria can explain the different activity of ZnO and CuO against B. infantis and E. coli (Xiao et al., 2015). Copper can change the Bacillus biofilm hydrophobicity and make them more sensitive to antibiotics and biocides (Falcón García et al., 2020). According to Perelshtein et al. (2013), Cu2+ and Zn2+ ions are positively charged, and they interact with negatively charged lipid-based bacterial membranes. During this process, the permeability of the Gram-negative membranes could change and, as a result, reduce bacterial cell growth and cell viability.
The cooling system cardboard coating with CuO MPs and ZnO NPs significantly inhibited the growth of freshwater algae Scenedesmus sp. and Pinnularia sp. compared to the control in laboratory experiments. The effects of heavy metal ions on different algal species are different (Hugo et al., 2007). This could explain why the coatings had different antifouling effects on Scenedesmus sp. and Pinnularia sp. algae. Both coatings were equally effective against Scenedesmus sp. On the opposite end, according to Terry and Stone, 2002, Scenedesmus species can tolerate heavy metals and could be used to remove heavy metals from the water via biosorption. The difference between this and our results could be explained by several hypotheses. First, the concentration of heavy metals in our study is different from the study of Terry and Stone, 2002. Second, CuO MPs and ZnO NPs coatings not only produce heavy metal ions but also release ROS under light conditions (see mechanism of action).
4.3 Mechanism of antifouling action
The antifouling activity of coatings could be explained by the production of reactive oxygen species (ROS) and metal ions. CuO MPs and ZnO NPs can produce reactive oxygen species under light conditions through water photocatalysis (Ivask et al., 2010; Sirelkhatim et al., 2015; Al-Belushi et al., 2020). The ROS presence was confirmed by observing the production of hydroxyl radicals (•OH) from the ZnO NPs and CuO MPs in the PL measurement. When ZnO NPs and CuO MPs are exposed to visible light, they undergo photoexcitation, where photons excite the electrons in the valence band to the conduction band, leaving behind holes in the valence band (Al-Belushi et al., 2020). The conduction band electrons and valence band holes could react with water in the environment, generating ROS such as •OH, superoxide ions (O2•-), and hydrogen peroxide (H2O2). ROS are well-known for degrading contaminants and inhibiting bacterial and algal growth by causing damage to cell membranes, mitochondria, and DNA, resulting in bacterial and algal death (Al-Belushi et al., 2020). According to Franklin et al. (2007), under light conditions, ZnO NPs produce ROS that are harmful to the freshwater microalga Pseudokricheriella subcapitata.
Additionally, both tested coatings release ions that could kill bacteria and microalgae under dark and light conditions. This is supported by our ICP-MS results. The concentrations of ions observed in our study were enough to kill bacteria and algae but lower than the ones that caused the mortality of human T cells (Reddy et al., 2007). This suggests the potential safety of using tested coatings in greenhouse applications. The release of Cu2+ ions was detected in our study, even in the case of the control (clean cardboard). This could suggest that the manufacturer used copper to prevent the growth of bacteria on cooling cardboard or possible environmental contamination. However, the concentration of Cu2+ ions released by the cardboard was not enough to inhibit the growth of Gram-positive and negative bacteria and microalgae used in this study. This demonstrated the necessity of additional antifouling defense of the cooling cardboard of greenhouses.
4.4 Differences between antifouling activity of CuO MPs and ZnO NPs
There were differences in the antifouling activity of CuO MPs and ZnO NPs coatings. CuO MPs coating was more effective in the inhibition of the growth of Gram-positive and Gram-negative bacteria, the diatom Pinnularia sp., and the green alga Scenedesmus sp. compared to ZnO NPs coating. The size of micro- and nano-particles could change the surface area, thus affecting the production of ROS and the release of ions. Smaller particles tend to leach more ions, which explains why ZnO NPs coatings released more ions than CuO MPs. However, the antifouling effect of CuO MPs was higher than that of ZnO NPs coatings. It could be due to the higher toxicity of Cu2+ compared to Zn2+ ions (Terry and Stone, 2002; Zhang et al., 2016). The accumulation of Cu2+ ions leads to metabolic alkalosis (Karlsson et al., 2008). According to Hugo et al. (2007), Cu2+ is toxic to chromosomes, causes DNA damage and oxidative DNA lesions, and disrupts the mitosis cycle, which could explain their higher activity in this study.
The selection of CuO MPs was because CuO NPs have significantly higher toxicity and environmental impact (Maciel-Magalhães et al., 2020). Although the size of CuO MPs was bigger compared with ZnO NPs, the production of ROS by CuO MPs was higher. Additionally, CuO MPs had higher zeta potential compared to ZnO NPs. This suggests that CuO MPs are likely to be more stable in suspension due to greater electrostatic repulsion. Zeta potential plays a crucial role in the antimicrobial activity of nanoparticles (NPs) and microparticles (MPs) because it influences particle interactions with microbial cells (Jastrzębska et al., 2015). Higher ROS generation and greater stability in the suspension of CuO MPs coatings compared to ZnO NPs explain the improved antimicrobial and antialgal properties of CuO MPs.
4.5 Conclusion
Biofouling is a common problem and critical issue in the cooling systems of greenhouses in arid climates. It can compromise the efficiency of cooling systems essential for maintaining optimal conditions. In this study, the use of innovative coatings, such as CuO MPs and ZnO NPs, to address the practical biofouling problems of greenhouses was studied. CuO MPs and ZnO NPs coatings provide several advantages over traditional cleaning methods, such as chlorine treatment or physical cleaning, particularly when considering their efficiency, scalability, and cost-effectiveness. Traditional methods like chlorine cleaning can release harmful byproducts, which can be environmentally damaging and require careful disposal. This study demonstrated the ability of CuO MPs and ZnO NPs coatings to effectively reduce the growth of Gram-positive and Gram-negative bacteria, as well as microalgae isolated from the greenhouse cooling system. The antifouling effect was due to ROS and ion production. The application of CuO MPs coatings was more effective than ZnO NPs coatings in the prevention of biofouling of greenhouse cooling systems. However, care must be taken in the use of these antifouling coatings in greenhouse cooling systems for longer periods. Further studies are needed to test the durability of the coatings and the efficiency of antifouling solutions in field experiments. Additionally, future investigations are required to test long-term Cu and Zn accumulation risks in soil and water systems, as well as their potential health impacts. Furthermore, the findings suggest that CuO MPs coatings can lead to more sustainable agricultural practices by minimizing chemical treatments traditionally used for biofouling control.
Data availability statement
The raw data supporting the conclusions of this article will be made available by the authors, without undue reservation.
Author contributions
AA-B: Conceptualization, Data curation, Formal Analysis, Investigation, Methodology, Software, Validation, Visualization, Writing – original draft, Writing – review and editing. RA-M: Data curation, Formal Analysis, Investigation, Validation, Writing–original draft, Writing – review and editing. HK: Formal Analysis, Investigation, Software, Validation, Writing – original draft, Writing – review and editing. MM: Data curation, Formal Analysis, Investigation, Visualization, Writing – original draft, Writing – review and editing. MA-A: Conceptualization, Funding acquisition, Project administration, Supervision, Validation, Writing – original draft, Writing – review and editing. SD: Conceptualization, Data curation, Funding acquisition, Investigation, Methodology, Project administration, Resources, Supervision, Validation, Writing – original draft, Writing – review and editing.
Funding
The author(s) declare that financial support was received for the research and/or publication of this article. The authors acknowledge funding from Sultan Qaboos University IG/AGR/FISH/24/01 and the Ministry of Higher Education and Innovation RC/GRG-AGR/FISH/24/01.
Acknowledgments
The authors acknowledge the help of Dr. Ahmed Al-Busaidi (Sultan Qaboos University) in conducting part of this study.
Conflict of interest
The authors declare that the research was conducted in the absence of any commercial or financial relationships that could be construed as a potential conflict of interest.
Generative AI statement
The author(s) declare that no Generative AI was used in the creation of this manuscript.
Publisher’s note
All claims expressed in this article are solely those of the authors and do not necessarily represent those of their affiliated organizations, or those of the publisher, the editors and the reviewers. Any product that may be evaluated in this article, or claim that may be made by its manufacturer, is not guaranteed or endorsed by the publisher.
Supplementary material
The Supplementary Material for this article can be found online at: https://www.frontiersin.org/articles/10.3389/fnano.2025.1545224/full#supplementary-material
References
Al-Belushi, M. A., Myint, M. T. Z., Kyaw, H. H., Al-Naamani, L., Al-Mamari, R., Al-Abri, M., et al. (2020). ZnO nanorod-chitosan composite coatings with enhanced antifouling properties. Int. J. Biol. Macromol. 162, 1743–1751. doi:10.1016/j.ijbiomac.2020.08.096
Al-Busaidi, A., Al-Busaidi, A., Dobretsov, S., and Ahmed, M. (2022). Risks associated with treated wastewater in greenhouse cooling system. Water Treat. 263 (2021), 98–107. doi:10.5004/dwt.2022.28210
Al-Fori, M., Dobretsov, S., Myint, M. T. Z., and Dutta, J. (2014). Antifouling properties of zinc oxide nanorod coatings. Biofouling 30 (7), 871–882. doi:10.1080/08927014.2014.942297
Al-Ismaili, A. M., and Jayasuriya, H. (2016). Seawater greenhouse in Oman: a sustainable technique for freshwater conservation and production. Renew. Sustain. Energy Rev. 54, 653–664. doi:10.1016/j.rser.2015.10.016
Ashkarran, A. A. (2012). A twice liquid arc discharge approach for synthesis of visible-light-active nanocrystalline Ag:ZnO photocatalyst. Appl. Phys. A Mater. Sci. Process. 107 (2), 401–410. doi:10.1007/s00339-012-6797-6
Bora, T., and Dutta, J. (2014). Applications of nanotechnology in wastewater treatment-A review. J. Nanosci. Nanotechnol. 14 (1), 613–626. doi:10.1166/jnn.2014.8898
Brake, S. S., and Hasiotis, S. T. (2010). Eukaryote-dominated biofilms and their significance in acidic environments. Geomicrobiol. J. 27 (6-7), 534–558. doi:10.1080/01490451003702966
Brooke, J. S. (2012). Stenotrophomonas maltophilia: an emerging global opportunistic pathogen. Clin. Microbiol. Rev. 25 (1), 2–41. doi:10.1128/cmr.00019-11
Chapman, J., Le Nor, L., Brown, R., Kitteringham, E., Russell, S., Sullivan, T., et al. (2013). Antifouling performances of macro-to micro-to nano-copper materials for the inhibition of biofouling in its early stages. J. Mater. Chem. B 1 (45), 6194–6200. doi:10.1039/c3tb21285h
Dacewicz, E., and Chmielowski, K. (2018). The importance of media in wastewater treatment. Sewage. doi:10.5772/intechopen.75625
Davidson, I., Cahill, P., Hinz, A., Kluza, D., Scianni, C., and Georgiades, E. (2021). A review of biofouling of ships’ internal seawater systems. In Front. Mar. Sci., (8). doi:10.3389/fmars.2021.761531
Dobretsov, S., Sathe, P., Bora, T., Barry, M., Myint, M. T. Z., and Abri, M.Al. (2020). Toxicity of different zinc oxide nanomaterials at 3 trophic levels: implications for development of low-toxicity antifouling agents. Environ. Toxicol. Chem. 39 (7), 1343–1354. doi:10.1002/etc.4720
ElFaham, M. M., Mostafa, A. M., and Toghan, A. (2021). Facile synthesis of Cu2O nanoparticles using pulsed laser ablation method for optoelectronic applications. Colloids Surfaces A Physicochem. Eng. Aspects 630 (July), 127562. doi:10.1016/j.colsurfa.2021.127562
Falcón García, C., Kretschmer, M., Lozano-Andrade, C. N., Schönleitner, M., Dragoŝ, A., Kovács, Á. T., et al. (2020). Metal ions weaken the hydrophobicity and antibiotic resistance of Bacillus subtilis NCIB 3610 biofilms. npj Biofilms Microbiomes 6 (1), 1. doi:10.1038/s41522-019-0111-8
Falfushynska, H. I., Wu, F., Ye, F., Kasianchuk, N., Dutta, J., Dobretsov, S., and Sokolova, I. (2019). The effects of ZnO nanostructures of different morphology on bioenergetics and stress response biomarkers of the blue mussels Mytilus edulis. Sci. Total Environ. 694, 133717. doi:10.1016/j.scitotenv.2019.133717
Flemming, H. C. (2002). Biofouling in water systems - cases, causes and countermeasures. Appl. Microbiol. Biotechnol. 59 (6), 629–640. doi:10.1007/s00253-002-1066-9
Franklin, N. M., Rogers, N. J., Apte, S. C., Batley, G. E., Gadd, G. E., and Casey, P. S. (2007). Comparative toxicity of nanoparticulate ZnO, bulk ZnO, and ZnCl2 to a freshwater microalga (pseudokirchneriella subcapitata): the importance of particle solubility. Environ. Sci. Technol. 41, 8484–8490. doi:10.1021/es071445r
Ghernaout, D. (2018.). Increasing trends towards drinking water reclamation from treated wastewater. 3, 1, doi:10.11648/j.wjac.20180301.11
Hegazy, A., Farid, M., Subiantoro, A., and Norris, S. (2022). Sustainable cooling strategies to minimize water consumption in a greenhouse in a hot arid region. Agric. Water Manag. 274, 107960. doi:10.1016/j.agwat.2022.107960
Hugo, V.P.-V., González-Moreno, S., Montes-Horcasitas, C., and Cañizares-Villanueva, R. O. (2007). Growth, photosynthetic and respiratory responses to sub-lethal copper concentrations in Scenedesmus incrassatulus. Chlorophyceae 67 (11), 0–2281. doi:10.1016/j.chemosphere.2006.11.036
Ivask, A., Bondarenko, O., Jepihhina, N., and Kahru, A. (2010). Profiling of the reactive oxygen species-related ecotoxicity of CuO, ZnO, TiO2, silver and fullerene nanoparticles using a set of recombinant luminescent Escherichia colistrains: differentiating the impact of particles and solubilised metals. Anal. Bioanal. Chem. 398 (2), 701–716. doi:10.1007/s00216-010-3962-7
Iwane, T., Urase, T., and Yamamoto, K. (2001). Possible impact of treated wastewater discharge on incidence of antibiotic resistant bacteria in river water. Water Sci. Technol. 43 (2), 91–99. doi:10.2166/wst.2001.0077
Izwanie, N., Basri, H., and Harun, Z. (2019). Biosynthesized nanoparticles from aloe vera: brief review towards membrane technology. Malaysian Journal of Fundamental and Applied Sciences 15(6), 895–902.
Jain, J. K., and Hindoliya, D. A. (2011). Experimental performance of new evaporative cooling pad materials. Sustain. Cities Soc. 1 (4), 252–256. doi:10.1016/j.scs.2011.07.005
Jastrzębska, A. M., Kurtycz, P., Olszyna, A., Karwowska, E., Miaśkiewicz-Pęska, E., Załęska-Radziwiłł, M., et al. (2015). The impact of zeta potential and physicochemical properties of TiO2-based nanocomposites on their biological activity. Int. J. Appl. Ceram. Technol. 12 (6), 1157–1173. doi:10.1111/ijac.12340
Johnson, R. J., Jurawan, I., Frenzel, M., and Price, A. C. (2016). The identification and mechanism of a Scenedesmus spp. causing bio-fouling of an oil field produced water treatment plant. Int. Biodeterior. and Biodegrad. 108, 207–213. doi:10.1016/j.ibiod.2015.05.023
Karlsson, H. L., Cronholm, P., Gustafsson, J., and Möller, L. (2008). Copper oxide nanoparticles are highly toxic: a comparison between metal oxide nanoparticles and carbon nanotubes. Chem. Res. Toxicol. 21 (9), 1726–1732. doi:10.1021/tx800064j
Khan, A., Rashid, A., Younas, R., and Chong, R. (2016). A chemical reduction approach to the synthesis of copper nanoparticles. Int. Nano Lett. 6 (1), 21–26. doi:10.1007/s40089-015-0163-6
Khashan, K. S., Jabbar, N. A., and Abdulameer, F. A. (2018). Preparation and characterization of copper oxide nanoparticles decorated carbon nanoparticles using laser ablation in liquid. Journal of Physics: Conference Series. 1003, 012100.
Ko, K. S., Oh, W. S., Lee, M. Y., Lee, J. H., Lee, H., Peck, K. R., et al. (2006). Bacillus infantis sp. nov. and Bacillus idriensis sp. nov., isolated from a patient with neonatal sepsis. Int. J. Syst. Evol. Microbiol. 56 (11), 2541–2544. doi:10.1099/ijs.0.64213-0
Kumar, G., Suraj, A., and Mukherji, S. (2016). Performance of an algal-bacterial system for treatment of biomass gasifier wastewater in a 16-L RBC at 36 h HRT. Mater. Today Proc. 3 (10), 3418–3426. doi:10.1016/j.matpr.2016.10.022
Kumar, S., Ye, F., Dobretsov, S., and Dutta, J. (2021). Nanocoating is a new way for biofouling prevention. Front. Nanotechnol. 3, 771098. doi:10.3389/fnano.2021.771098
Lee, K., Eisterhold, M. L., Rindi, F., Palanisami, S., and Nam, P. K. (2014). Isolation and screening of microalgae from natural habitats in the Midwestern United States of America for biomass and biodiesel sources. Journal of Natural Science, Biology and Medicine. 5(2), 333–339. doi:10.4103/0976-9668.136178
Lopez-Galvez, F., Gil, M. I., Pedrero-Salcedo, F., Alarcón, J. J., and Allende, A. (2016). Monitoring generic Escherichia coli in reclaimed and surface water used in hydroponically cultivated greenhouse peppers and the influence of fertilizer solutions. Food control. 67, 90–95. doi:10.1016/j.foodcont.2016.02.037
Maciel-Magalhães, M., Medeiros, R. J., Bravin, J. S., Patricio, B. F. C., Rocha, H. V. A., Paes-de-Almeida, E. C., et al. (2020). Evaluation of acute toxicity and copper accumulation in organs of Wistar rats, 14 days after oral exposure to copper oxide (II) nano- and microparticles. J. Nanopart Res. 22 (2), 2. doi:10.1007/s11051-019-4721-0
Massilamany, C., Mohammed, A., Loy, J. D., Purvis, T., Krishnan, B., Basavalingappa, R. H., et al. (2016). Whole genomic sequence analysis of Bacillus infantis: defining the genetic blueprint of strain NRRL B-14911, an emerging cardiopathogenic microbe. BMC Genomics 17, 511. doi:10.1186/s12864-016-2900-2
Menamo, D. S., Ayele, D. W., and Ali, M. T. (2017). Green synthesis, characterization and antibacterial activity of copper nanoparticles using L-ascorbic acid as a reducing agent. Ethiop. J. Sci. Technol. 10 (3), 209. doi:10.4314/ejst.v10i3.5
Moumita, B., and Tapobrata, D. (2024). Growth kinetics of lead resistant Bacillus infantis isolated from battery industry waste. J. Environ. Eng. Sci. 19, 132–139. doi:10.1680/jenes.23.00035
Obayomi, O., Bernstein, N., Edelstein, M., Vonshak, A., Ghazayarn, L., Ben-Hur, M., et al. (2019). Importance of soil texture to the fate of pathogens introduced by irrigation with treated wastewater. Sci. Total Environ. 653, 886–896. doi:10.1016/j.scitotenv.2018.10.378
Perelshtein, I., Ruderman, E., Perkas, N., Tzanov, T., Beddow, J., Joyce, E., et al. (2013). Chitosan and chitosan–ZnO-based complex nanoparticles: formation, characterization, and antibacterial activity. J. Mater. Chem. B 1 (14), 1968. doi:10.1039/C3TB00555K
Reddy, K. M., Feris, K., Bell, J., Wingett, D. G., Hanley, C., and Punnoose, A. (2007). Selective toxicity of zinc oxide nanoparticles to prokaryotic and eukaryotic systems. Appl. Phys. Lett. 90, 2139021–2139023. doi:10.1063/1.2742324
Saggu, S. K., and Mishra, P. C. (2017). Characterization of thermostable alkaline proteases from Bacillus infantis SKS1 isolated from garden soil. PLoS ONE 12 (11), e0188724. doi:10.1371/journal.pone.0188724
Sathe, P., Laxman, K., Myint, M. T. Z., Dobretsov, S., Richter, J., and Dutta, J. (2017). Bioinspired nanocoatings for biofouling prevention by photocatalytic redox reactions. Sci. Rep. 7 (1), 3624. doi:10.1038/s41598-017-03636-6
Sharma, M., Millner, P. D., Hashem, F., Camp, M., Whyte, C., Graham, L., et al. (2016). Survival and persistence of nonpathogenic Escherichia coli and attenuated Escherichia coli O157:H7 in soils amended with animal manure in a greenhouse environment. J. Food Prot. 79 (6), 913–922. doi:10.4315/0362-028X.JFP-15-421
Shaw, K. S., Rosenberg Goldstein, R. E., He, X., Jacobs, J. M., Crump, B. C., and Sapkota, A. R. (2015). Growth of Escherichia coli O157:H7, Non-O157 Shiga Toxin–Producing Escherichia coli, and Salmonella in Water and Hydroponic Fertilizer Solutions. Journal of Food Protection 78 (10), 1811–1820. doi:10.4315/0362-028X.JFP-16-073
Sirelkhatim, A., Mahmud, S., Seeni, A., Kaus, N. H. M., Ann, L. C., Bakhori, S. K. M., et al. (2015). Review on zinc oxide nanoparticles: antibacterial activity and toxicity mechanism. Nano-Micro Lett. 7 (3), 219–242. doi:10.1007/s40820-015-0040-x
Soni, N., Dhandhukia, P., and Thakker, J. N. (2023). Carotenoid from marine Bacillus infantis: production, extraction, partial characterization, and its biological activity. Arch Microbiol. Arch. Microbiol. 205, 161. doi:10.1007/s00203-023-03505-z
Terry, P. A., and Stone, W. (2002). Biosorption of cadmium and copper contaminated water by Scenedesmus abundans. Chemosphere 47 (3), 249–255. doi:10.1016/s0045-6535(01)00303-4
Tsavatopoulou, V. D., and Manariotis, I. D. (2020). The effect of surface properties on the formation of Scenedesmus rubescens biofilm. Algal Res. 52, 102095. doi:10.1016/j.algal.2020.102095
Vaz-Moreira, I., Figueira, V., Lopes, A. R., Lobo-da-Cunha, A., Sproer, C., Schumann, P., et al. (2012). Bacillus purgationiresistans sp. nov., isolated from a drinking-water treatment plant. Int. J. Syst. Evol. Microbiol. 62 (1), 71–77. doi:10.1099/ijs.0.028605-0
Weber, F., and Esmaeili, N. (2023). Marine biofouling and the role of biocidal coatings in balancing environmental impacts. Biofouling 39 (6), 661–681. doi:10.1080/08927014.2023.2246906
Wehr, J. D., Wehr, J. D., Sheath, R. G., and Kociolek, J. P. (2015). Freshwater algae of North America: ecology and classification. Waltham, MA: Elsevier.
Xiao, G., Zhang, X., Zhang, W., Zhang, S., Su, H., and Tan, T. (2015). Visible-light-mediated synergistic photocatalytic antimicrobial effects and mechanism of Ag-nanoparticles@chitosan–TiO2 organic–inorganic composites for water disinfection. Appl. Catal. B Environ. 170-171, 255–262. doi:10.1016/j.apcatb.2015.01.042
Xin, L., Hong-ying, H., and Yu-ping, Z. (2011). Growth and lipid accumulation properties of a freshwater microalga Scenedesmus sp. under different cultivation temperature. Bioresour. Technol. 102 (3), 3098–3102. doi:10.1016/j.biortech.2010.10.055
Zhang, W., Liu, X., Bao, S., Xiao, B., and Fang, T. (2016). Evaluation of nano-specific toxicity of zinc oxide, copper oxide, and silver nanoparticles through toxic ratio. J. Nanopart Res. 18, 372. doi:10.1007/s11051-016-3689-2
Keywords: biofouling, antifouling, copper oxide microparticles, zinc oxide nanoparticles, antimicrobial activity, algae, bacteria
Citation: Al-Busaidi A, Al-Mamari R, Kyaw HH, Myint MTZ, Al-Abri M and Dobretsov S (2025) Antifouling properties of copper oxide microparticles and zinc oxide nanoparticles in greenhouse cooling systems. Front. Nanotechnol. 7:1545224. doi: 10.3389/fnano.2025.1545224
Received: 14 December 2024; Accepted: 17 March 2025;
Published: 01 April 2025.
Edited by:
Bala Subramanian, Anna University, IndiaReviewed by:
V. Mahendran, Institut Jožef Stefan (IJS), SloveniaSahil Tahiliani, Applied Materials, United States
Copyright © 2025 Al-Busaidi, Al-Mamari, Kyaw, Myint, Al-Abri and Dobretsov. This is an open-access article distributed under the terms of the Creative Commons Attribution License (CC BY). The use, distribution or reproduction in other forums is permitted, provided the original author(s) and the copyright owner(s) are credited and that the original publication in this journal is cited, in accordance with accepted academic practice. No use, distribution or reproduction is permitted which does not comply with these terms.
*Correspondence: Sergey Dobretsov, c2VyZ2V5QHNxdS5lZHUub20=