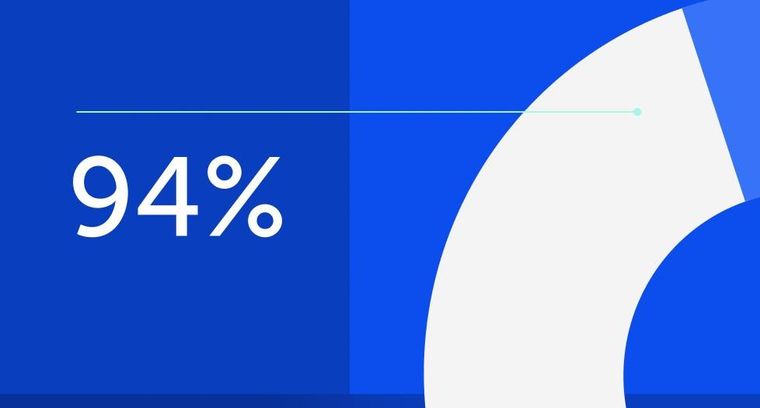
94% of researchers rate our articles as excellent or good
Learn more about the work of our research integrity team to safeguard the quality of each article we publish.
Find out more
REVIEW article
Front. Nanotechnol., 06 February 2025
Sec. Biomedical Nanotechnology
Volume 7 - 2025 | https://doi.org/10.3389/fnano.2025.1516180
This article is part of the Research TopicWomen in Nanotechnology: Volume IIView all articles
Plant genetic engineering is an evolving discipline that contributes to crop improvement by introducing desirable traits into crop plants, such as improved yield, enhanced nutrition value, and resistance to biotic and abiotic stresses. Plant transformation is carried out in two steps: Gene delivery into the plant cell and regeneration of the plant cell into the fertile plant. Gene delivery is an essential step in plant genetic transformation, and it is largely plant species-specific. Based on the mode of delivery the conventional plant gene delivery methods are divided into three main categories: biological (Agrobacterium-mediated transformation), physical (biolistic and electroporation), and chemical (Polyethylene glycol mediated and liposome-mediated gene delivery). Apart from species constraints, these methods have unique advantages and limitations, including random gene integration, low gene transfer efficiency, tissue damage, united gene alterations, time-consuming and labor-intensive plant regeneration protocols. Recent advancements in nanotechnology have introduced novel gene-delivery systems, utilizing micro and nanoparticles, which can overcome many limitations of conventional plant gene delivery methods by exhibiting superior transformation efficiency, demonstrate compatibility with biological systems, offer protection to different cargoes, and hold significant capability for enhancing plant regeneration. Nanoparticles are well recognized for its flexible size, shape, and cargo-binding properties, which enable them to surpass defensive primary cell wall barrier and it can be a promising candidate for plant gene delivery applications. However, delivering the nanoparticles and cargo complexes into plants is a critical step of the gene delivery process, and have not been thoroughly explored. In this review, we provide comprehensive insights into nano-delivery systems and detailed methods of introducing nanoparticle complexes into plant tissues. Further, we also discuss techniques such as syringe infiltration, vacuum infiltration, biolistic methods, magnetofection, ultrasound-mediated delivery, passive diffusion, cellular uptake, and spray method. This review serves as a valuable resource for advancing plant gene transformation using nanoparticles, offering guidance on the most effective delivery methods to enhance plant genetic engineering outcomes.
Global food security is increasingly threatened by the rapid growth of human populations and the escalating impacts of climate change (Molla et al., 2021). A critical approach to addressing these challenges is plant genetic engineering, which promotes the development of crops with desired characteristics, such as improved resistance to different stress conditions, efficient uptake of beneficial nutrients, resistance to herbicides, increased yield and nutritional value (Venugopal and Dively, 2017; Chung et al., 2021; Mora et al., 2022). This technique involves in the modification of internal genes within the species using genome editing techniques and incorporation of specific gene of interest into desired plant genome from other species, either through stable integration or transient expression, to achieve desired agronomic characteristics (Doudna and Charpentier, 2014). Several methods are developed to deliver exogenous genetic material into plants and rapidly generate genetically modified plant species (Narusaka et al., 2012). Some of the most widely employed techniques in plant genetics transformation includes: Agrobacterium-mediated gene delivery, biolistic gene delivery, electroporation-mediated gene delivery, and protoplast transfection (Men et al., 2003; Kant and Dasgupta, 2017). Despite their success, these methods are not cost-effective, and their usage is confined to the limited range of plant species (Duclercq et al., 2011). For the past few decades, one of the most successful and extensively used methods for manipulating plant nuclear genome is Agrobacterium-mediated transformation (AMT). AMT exploits the plant’s innate immune response to surpass the cellular defense to introduce DNA vectors (Gustafson et al., 2006; Kumar et al., 2012; Du and Pijut, 2009; Song et al., 2006). Most of the plant species are recalcitrant to ATM due to limited host range of agrobacterium, which limits its extensive use in genetic transformation in many plant species (Su et al., 2023; Kondo et al., 2000). Moreover, the current protocols for in-vitro plant regeneration are tediously lengthy (Sabbadini et al., 2019). Biolistic delivery represents a versatile and effective transformation tool, particularly for crops species, which are resistant to AMT (Shou et al., 2004). This method relies on applying motive force to accelerate biomolecule-coated microprojectiles into plant tissues, facilitating gene transfer (Ameen Rashid and Dhahir Lateef, 2016; Davies et al., 2013). Similar to AMT, the biolistic approach can achieve both stable and transient gene expression (Hamada et al., 2017; Miller et al., 2021). Notably, the biolistic delivery method has regarded to be a valuable approach for plant genetic transformation across wide range of crop species (Cho et al., 2004; Zhang et al., 2015). However, using the highly accelerated micro-particle could cause collateral damage to plant tissue and the nuclear genome (Altpeter et al., 2016). Biolistic delivery often leads to the possible integration of multiple copies of transgene and introduces off-target deletion (Liu et al., 2019) and delivering high amounts of exogenous DNA results in duplication or rearrangement of genes (Anami et al., 2013).
Electroporation leverages the electrical field-induced transit electropores to bypass the restrictive effect of the cell membrane for foreign DNA introduction (Ozyigit, 2020). Electroporation provides many advantages over the conventional AMT methods, with the relevance to transient and stable transfection of all cell types and the capability of transfecting a large group of cells at once in a short duration of time (Kotnik et al., 2012; Kar et al., 2018). However, electroporation is a variable process influenced by factors such as cell size, cell type, the conductivity of the surrounding medium, and osmolarity. Standardizing the protocols for protoplast preparation from diverse plant species is another barrier to electroporation (Wang et al., 2022). Also, high field pulse causes overheating, results in excessive cell death count, and requires more cells for the transformation (Kumar et al., 2019).
Protoplast transfection is an efficient alternate approach of introducing genes of interest to plant species (Krens et al., 1982). This method primarily involves the preparation of protoplast by stripping the cell wall. To deliver exogenous DNA, the protoplasts are incubated with a transfecting agent such as polyethylene glycol, which facilitates the temporary disruption of the plasma membrane, allowing the genetic material enter into protoplast (Wang et al., 2015; Yu et al., 2008; Moore et al., 2009). Protoplast transfection is not only used in delivering exogenous DNA but also for delivering other biomolecules including RNA and protein (Coy et al., 2022; Burris et al., 2016; Vanitharani et al., 2003). Protoplast transfection is successfully used in the preparation of transient and stable transgenic expression lines (Sun et al., 2015; Woo et al., 2015). Although protoplast transfection is more efficient compared to biolistic delivery, its application is limited to a handful of species with established protocols for protoplast regeneration (Squire et al., 2023). In summary, the field of plant genetic engineering has not advanced as quickly as animal systems. The effectiveness of plant genetic transformation is further limited by intracellular transport via cell walls, which poses a challenge to standard techniques of biomolecule delivery to plants. Plant biotechnology currently requires a technique that enables the passive delivery of different cargoes into various plant species and tissues without the need for external force or tissue harm. In order to address the limitation associated with the traditional gene delivery system in plants, nanoparticle mediated gene delivery into plant tissues is attaining significant attention in the field of plant biotechnology.
Nanoparticles (NPs) are characterized by ultrafine particles with diameters less than 100 nm; numerous amendment options allow them to deliver different cargoes, including drugs, proteins, and nucleic acids inside cells (Deng et al., 2014; Wang et al., 2017; Yin et al., 2017). NP mediated transformations facilitate the manipulation of plant cellular genomes to incorporate desirable traits to increase crop production and provides tolerance to different stress conditions (Yong et al., 2024). The NP-mediated gene delivery strategically operates the same magnitude of charge in proximity to a cellular membrane to overcome the plant cell wall barrier to introduce different cargoes into the plant tissues to promote transient or stable transformation (Lv et al., 2020). Additionally, NP-based gene delivery is not only used in nuclear genome editing but also used successfully to manipulate organellar genome transformation. Due to its non-invasive and diffusive nature, NP-mediated delivery is an attractive alternative to AMT (Campos et al., 2021). Additionally, with the utilization of passive diffusion-based gene delivery, where the nanoparticles loaded with molecular cargoes bypass the plant cell wall without any external agent (Cunningham et al., 2018), the NP-mediated gene delivery system is widely applicable in the genetic transformation of different plant species, including plant species resistant to AMT (Yan et al., 2023). Moreover, nanoparticles have low toxicity, potentially creating greater prospects for future biotechnological advancements (Hamers, 2017). For the past decades, various forms of nanoparticles have been used for sustained and controlled delivery of the exogenous DNA including carbon carriers (carbon dots, single- and multi-walled carbon nanotubes), metallic carriers (gold nanosphere, gold nanorods, gold nanoclusters, and iron oxide clusters), silicon carriers, and bio-inspired carriers (liposomes, and vesicles) (Cunningham et al., 2018).
Carbon dots (CDs) are carbon-based microscopic nanoparticles less than 10 nm in diameter. These CDs are categorized into three groups based on the chemical composition (1) graphene quantum dots (GQDs), (2) carbon nanodots (CNDs), and (3) polymer dots (PDs). CND can be divided further into two subgroups including carbon nanoparticles (CNPs) and carbon quantum dots (CQDs) (Lopes et al., 2022). Due to their exceptional tensile strength and small size CDs are used as carriers for genetic cargo reported in plants and suitable for bypassing the cell wall. CDs are covalently tethered with cationic charge polymers that help in grafting the negatively charged exogenous DNA to delivery into intact plants (Nayak and Das, 2021). CDs are efficiently used as a carrier for various exogenous nucleic acids, including plasmid DNA (Wang Z.-P. et al., 2020), siRNA (Schwartz et al., 2020), and dsRNA (Martin-Ortigosa et al., 2014), to manipulate plant nuclear and organellar genomes. Lipid exchange envelope penetration (LEEP) is a newly developed mathematical model that predicts the destination of NPs inside a cell, such as into the mitochondria, chloroplast, or remaining in the cytosol. LEEP relies on nanoparticles with adequate surface charge and size close to the destined cell membrane to create a potential difference across the membrane, in succession diffusional softening the lipid bilayer and inducing pores to internalize cargoes (Wong et al., 2016). Due to their small size, CDs were able to travel throughout the plant via vascular tissues. The plasmid-coated CDs approach was successfully used to manipulate nuclear and chloroplast genomes of Triticum aestivum, confirmed by fluorescence microscopy of GFP-SPO11 vector (Doyle et al., 2019). Besides gene manipulation studies, CDs are widely used in biomolecule sensing and increasing plant nutrition value via biofortification (Parra-Torrejón et al., 2023).
Carbon nanotubes (CNTs) are carbon-based nanoparticles with dimensions smaller than 4 nm × 0.5 μm. Based on the number of layers, CNTs are typically classified into two categories: single-walled carbon nanotubes (SWCNTs) and multi-walled carbon nanotubes (MWCNTs) (Dunbar et al., 2022). SWCNTs consist of single-layer graphene sheets rolled into a cylindrical shape with a diameter of around 0.5–2.0 nm. MWCNTs consist of nested SWCNTs rolled into tube-in-tube structures with a diameter of 220 nm (Lv et al., 2020). Using a high surface-to-volume ratio and exceptional tensile strength, CNTs could hold large amounts of plasmid DNA and can travel non-invasively through plant cell wall barriers to facilitate transient expression of exogenous nucleic acid in plant tissues. Moreover, CNTs are efficiently used in transient and stable plant gene expression studies. CNTs are effectively used for the passive internalization of exogenous nucleic acids, such as plasmid DNA and siRNA (Demirer et al., 2020), to manipulate the plant nuclear genome. Apart from nuclear genome manipulation, organellar membrane laminated LEEP-CNTs are successfully used in delivering exogenous biomolecules to organelles such as chloroplast and mitochondria for transient gene expression in different plant species without the aid of a gene gun (Kwak et al., 2019; Law et al., 2022).
Metallic nanoparticles (MNPs) are macro and nano-sized composite nanoparticles that have been used successfully to deliver cargoes in crop species. Depending upon their composition MNPs are divided into two groups: gold nanoparticles (gold nanospheres, gold nanorods, and gold nanoclusters; AuNPs) and metaloxide nanoparticles (MONPs), which are exclusively used in plant genetic transformations (Law et al., 2023). The average diameter of MNPs ranges from 5 nm to 14 nm (Squire et al., 2023). For the past few decades, AuNPs have been used in plant systems for delivering nucleic acids, showing a favorable in grafting the thiolated nucleic acids to its carriers, circumvents cargoes from nuclease degradation, and highly tunable to holding high amounts of cargo (Li, 2002). AuNPs delivery is one of the most efficient approaches after AMT in plant transformation for carrying the exogenous biomolecules into plant cells beyond the cell wall barrier. AuNP delivery created less damage to the plant tissue and displayed superior DNA delivery efficiency with ∼40%, when compared to macro-sized gold particles (Zhi et al., 2022). It was reported that the AuNP delivery greatly depends on the dimensions and types of biocompatible polymers. For example, gold nanoclusters and poly-disperse spherical gold NPs deliver siRNA to Arabidopsis protoplast and mature leaves of N. benthamiana with the efficiency of 80% knockdown of gene expression (Martin-Ortigosa et al., 2014). While spherical gold NPs ranging from 5 to 15 nm are not capable of internalizing into plant cells but are retained in the plant cell wall. In addition, spherical gold NPs with >5 nm and gold nanorods with 13 nm are efficiently diffused through the cell wall to deliver various biomolecules into the plant tissues (Squire et al., 2023; Zhang et al., 2023; Huang et al., 2013). MNOPs are fine crystalline supermagnetic nanoparticles with sizes ranging from 3 to 11 nm in diameter (Sharma et al., 2020). Exogenous DNA-coated MNOPs were primarily used in pollen genetic transformation through magnetofection, allowing gene manipulations in different crop species. Pollen genetic transformation is an efficient stable transformation approach enabling the transfer of exogenous nucleic acid into pollen grains to attain genomic edits transmitted directly to their progeny without involving the regeneration process (Park et al., 2024a). DNA-conjugated MNPs to intact pollen grains of Cucumis sp. and Gossypium sp. showed a stable expression validated by GUS staining (Park et al., 2024b; Zhang et al., 2019).
Bio-inspired nanoparticles (BiNPs) or biomimetic nanoparticles are synthesized by utilizing biological components such as plant extracts, biomolecules, bacteria, and viruses (Keum et al., 2023). BiNPs are primarily made up of metal or organic structures overlaid with biological components compatible with a sufficient magnitude of charge parallel to a cellular membrane to create enough potential difference to deliver cargoes into the plant cell. Due to their tunable properties, size, shape, structure, surface moieties, and surface charge BiNPs are efficiently used as nucleic acid and drug delivery carriers for both therapeutic and research applications. Liposomes or vesicles are closed spherical vesicles that consist of at least one lipid bilayer with an internal cavity. Liposomes such as cationic liposomes and polymer-based nanoparticles are often used to deliver molecular cargo such as plasmid DNA and siRNA. The positively charged cationic liposomes exploit physiological pH to make an assembly with negatively charged molecular cargos, such as nucleic acids and proteins, to form a nano-sized lipoplexes (Cunningham et al., 2020). These lipoplexes are primarily used to carry different forms of molecular cargo, including plasmid DNA, proteins, and ribonucleoproteins (RNP) complexes. Free lipofectamine-grafted Cas9 ribonucleoprotein (RNP) delivery showed successful gene edits in tobacco and citrus (Liu W. et al., 2020; Mahmoud et al., 2022). Polymer nanoparticles are solid nanoparticles made up of macromolecular polymers, with particle sizes ranging from 10 to 1,000 nm in diameter. Based on their composition, these polymer nanoparticles were categorized into two groups: naturally derived biopolymers and synthetic branched polymers, also called dendrimers. It was reported that direct delivery of poly (amidoamine) dendrimer-coated eGFP-plasmid showed transient expression in turfgrass cells for 2 days (Pasupathy et al., 2008).
Regardless of the method used for gene delivery, regeneration remains a significant hurdle in achieving stable plant transformation. However, nano-mediated delivery offers the potential to bypass regeneration-specific obstacles by enabling direct germline editing of plant tissues (Zhang et al., 2019; Wang et al., 2022). Additionally, nano-mediated delivery extends beyond nuclear genome modification, facilitating the transformation of organelle genomes as well (Law et al., 2022). While research on using nanoparticles as carriers for transient gene expression in plant tissues is still in its early stages, existing studies demonstrate their potential to overcome the limitations associated with traditional gene delivery methods. Many review articles have discussed the currently available nanoparticles for gene delivery, including their characterization and preparation methods. However, the success of nanoparticle-mediated delivery hinges not only on the type of nanoparticle used and its characteristics such as size, shape, emission/excitation wavelength, and surface charge but also on the suitable method of inserting the nanoparticle-cargo complex into plant tissues. Therefore, there is a need for a detailed examination of the available techniques for nanoparticle/cargo complex insertion. This will be crucial for the future application of nanoparticle-mediated gene delivery in plant transformation. The selection of an appropriate gene delivery technique significantly impacts the efficiency and efficacy of gene insertion. Various gene delivery techniques demonstrate differences in their gene insertion efficiencies contingent upon the type of plant species, type of plant tissue, and the dimensions of plant tissues. For example, the syringe infiltration technique is applicable solely to leaf tissues, while magnetofection has exhibited high efficiency exclusively in pollen transformation. Certain gene delivery techniques that employ external force and pressure may induce plant stress, adversely affecting the regeneration of plant tissues upon post-gene delivery. Additionally, the selection of appropriate delivery strategies is crucial for the scalability and accuracy of gene delivery. For instance, in case of gene delivery to leaf tissue, syringe infiltration and spray methods demonstrated superior performance regarding precision and scalability. Therefore, selection of appropriate techniques is crucial in plant genetic transformation. Hence, comprehensive review of the literature was conducted following the PRISMA guidelines (Moher et al., 2009). The literature search aimed to identify articles related to plant genetic transformation using both traditional and advanced gene delivery methods. Electronic databases, including PubMed, Scopus, Web of Science, and Google Scholar, were utilized to retrieve relevant publications up to 21 May 2024. Specific keywords such as “sustainability,” “plant transformation,” “gene delivery,” and “nanocarriers” were employed to ensure a focused search. A total of 170 articles were selected to present this review. The distribution of publications across time periods is as follows: approximately 51.7% were published between 2019 and 2024, 28.82% between 2010 and 2018, 16.47% between 2000 and 2009, and 2.94% between 1982 and 1999 (Supplementary Figure S1).
In this review, we provide a detailed explanation of the standard protocols and methods for inserting nanoparticle/cargo complexes into various plant tissues along with the advantages and disadvantages of each method (Table 1).
The process of genetic transformation is generally categorized into two primary types: stable transformation and transient transformation (Miki and Shimamoto, 2004). Stable transformation is a labor-intensive process with relatively low efficiency (Guidarelli and Baraldi, 2015; Vyacheslavova et al., 2012). In contrast, transient transformation offers a simpler and more efficient approach to gene delivery, addressing several limitations of stable transformation, such as low transformation efficiency, difficulties in selecting transformants, and challenges associated with plant regeneration (Toldi et al., 2009; Canto, 2016).
Syringe infiltration is recognized as an efficient technique for transient transformation, appreciated for its simplicity, speed, and versatility (Schöb et al., 1997; Wroblewski et al., 2005; Orzaez et al., 2006). This method involves using a needleless syringe to incorporate molecular cargo into matured intact leaves, making it broadly applicable for various plant biology studies (Santi et al., 2008; Sparkes et al., 2006; Xu et al., 2014; Bhaskar et al., 2009). The syringe infiltration process offers several key advantages, including the ability to introduce a single target gene across an entire leaf or insert multiple genes into different regions of a single leaf, thus enabling the execution of multiple experiments on the single leaf (Vaghchhipawala et al., 2011). Consequently, syringe infiltration has broad applications in plant biotechnology, enabling the evaluation of transient gene expression, gene knockdown, and gene knockout using siRNA, dsRNA, or CRISPR technologies, regardless of the carriers utilized (Přibylová et al., 2022; Kaur et al., 2021).
The delivery of nanoparticle-cargo complexes into leaf tissues begins with the preparation of nanoparticles. These nanoparticles are then mixed with the desired cargos, which may include plasmid DNA, dsRNA, siRNA, or CRISPR constructs, at the appropriate concentration. This mixture is incubated at for 30 min to 1 h to facilitate the conjugation of the nanoparticles with the cargos (Figure 1) (Demirer et al., 2019). After conjugation, the complexes are loaded into a needleless syringe for delivery. Injection into the leaf tissue occurs from the abaxial surface of intact leaves. To improve infiltration efficiency, a small puncture is created on the abaxial surface using a tip or small needle, and the conjugate enters through this puncture (Figure 2). Wipe any leftover solutions on the surfaces of leaves post-infiltration using a Kimwipe and delicately rub the leaf surface. If the leaves are infiltrated with plasmids containing fluorescent reporter genes such as GFP, RFP, and dsRed, it is preferable to transfer these plants in a growth chamber having low intensity light and room temperature instead of placing in a chamber with high-intensity light, as this may lead to photobleaching of the reporter genes. This approach allows for the efficient delivery of the nanoparticle-cargo complex into the leaf tissue (Demirer et al., 2019). Although fewer studies explore syringe infiltration for delivering nanoparticle and cargo complexes into leaf tissues compared to agroinfiltration, this technique holds significant potential for effectively delivering these complexes into plant leaf tissues. For example, the functionalization of quantum dots (QD) with β-cyclodextrin molecular baskets allowed the grafting and transportation of a variety of substances into plant chloroplasts. Nanoparticle coating with a guiding peptide allowed targeted delivery into Arabidopsis chloroplasts through syringe infiltration. Peptide biorecognition provided efficient and specific transport of QDs with chemical payloads into chloroplasts in living plant cells and resulted in a high delivery efficiency of 74.6% ± 10.8% (Santana et al., 2020; Kwak et al., 2019) successfully showed syringe infiltration of chitosan functionalized SWCNTs to deliver genes to chloroplasts in matured intact leaves arugula, watercress, spinach, and tobacco plants. This nanoparticle mediated approach allowed for targeted transgene expression and offered advantages over traditional methods such as Agrobacterium mediated transformation, offering a simpler, more convenient and cheaper alternative. This demonstrates syringe infiltration as a promising method for chloroplast transformation in mature plants and opens the door for plant bioengineering and research. Yong et al. (2022) demonstrated that 40-nm layered double hydroxide nanoparticles, when applied via needleless syringe infiltration, are rapidly absorbed by mature intact tobacco leaf cells and chloroplasts. Their study also elucidated the movement of these nanoparticles through the apoplast and vascular system. Additionally, they found that 40-nm LDH nanoparticles significantly increased the internalization of molecular cargo into tobacco leaf cells, achieving over 70% downregulation of the targeted transgenic mRNA within a single day after infiltration. Lei et al. (2020) synthesized gold nanoparticles (AuNPs) conjugated with branched polyethylenimine and combined them with small interfering RNAs (siRNAs) targeting the NPR1 gene, resulting in AuNPs-siRNANPR1 complexes. These were delivered into fully developed Arabidopsis thaliana leaves using a needleless syringe. The study demonstrated that AuNPs-siRNANPR1 effectively penetrated Arabidopsis cells, leading to an 80% reduction in NPR1 gene expression. Bacteriostatic and ion leakage assays further validated the successful gene silencing of NPR1 in Arabidopsis leaves following treatment with AuNPs-siRNANPR1. Similarly, several studies have employed syringe infiltration technology to deliver nanocarrier complexes into intact leaves (Table 2). Overall, syringe infiltration is a versatile and efficient technique for transient genetic modification and the delivery of nanoparticle-cargo complexes into plant tissues. Its simplicity, effectiveness, and broad applicability make it an essential tool in plant biotechnology, particularly for experiments involving rapid gene expression, gene knockdown, or precise nanoparticle-cargo delivery. Syringe infiltration holds great potential for advancing research in genetic engineering and nanotechnology. However, its use for delivering nanocarrier complexes has been limited to a narrow range of plant species. Expanding its application to a broader spectrum of species, with nanoparticles facilitating the delivery of diverse cargos, should be a priority for future research. Enhancing its applicability for large-scale studies will significantly increase its value in plant biotechnology, driving further advancements in genetic engineering and nanoparticle-mediated delivery systems.
Figure 2. Schematic representation of nanoparticle mediated gene delivery into plant tissues using syringe infiltration and vacuum infiltration.
Table 2. Evidence of successful delivery of various molecular cargoes into plant tissues using nanoparticles as carriers.
Vacuum infiltration is a versatile method used for transferring recombinant DNA using different carriers into plant tissues through an infiltration-mediated permeation pathway (Yan et al., 2012; Bian et al., 2024). Basically, there are two types of vacuum infiltration techniques available for the delivery of nanocarrier complex into various tissues. In one case the cargoes will be delivered into small tissues like small plants, leaves, pollens by placing the tissues in the infiltration solution containing the nanoparticle/cargo complex which is formed by mixing and incubating the desired concentration of nanoparticles and cargo for specific period of time, together in the large syringe. By drawing the plunger of the syringe, while obstructing its entrance, a negative pressure is created in both the syringe chamber and the infiltrated tissues. Upon unblocking the syringe aperture and releasing the plunger, the pressure in the tissues is effectively balanced by drawing the surrounding fluid into the intercellular gaps (Zheng et al., 2021; O’Leary et al., 2014; Chen et al., 2015; Matsuo et al., 2016). The main benefit of this technique is the cargo can be delivered into varies plant tissues without any specialized instruments, whereas in case of syringe infiltration the cargoes can be delivered only to matures leave. However, this method of vacuum infiltration can be applied only to small plant tissues. In another method of vacuum infiltration cargoes will be delivered into plant tissues using the vacuum pump (Simmons et al., 2009; Ranjbaran and Datta, 2019; Simmons et al., 2012). Plant parts, including flower buds, leaves, and seeds, are submerged in infiltration media containing nanoparticle/cargo complex. The submerged explants are then exposed to negative atmospheric pressure in a closed vacuum chamber. Negative pressure draws air from the submerged interstitial space of the explant. Releasing the vacuum permits nanoparticle/cargo complex in association with the interstitial spaces of the explant (Figure 2). This method allows the delivery of nanocomplex carriers into large plant tissues in contrast to syringe mediated vacuum infiltration. The infiltrated plant tissues are ideally transferred to a controlled environment with a temperature of 29°C and a light cycle of 14 h of light and 10 h of darkness. As similar to the syringe infiltration, few investigations have been reported, showing the successful implication of vacuum infiltration technique in nanoparticle mediated gene delivery compared to agrobacterium mediated gene delivery using vacuum infiltration. For instance, a nanomaterial synthesized by functionalizing polyethyleneimine with carbon dot can able to deliver the plasmid DNA containing GUS gene into mature rice embryo induced callus through vacuum infiltration method (Wang Z.-P. et al., 2020). CNTs carrying plasmid DNA can effectively penetrate plant cell walls, facilitating the expression of reporter genes. This was observed in excised embryos that were vacuum-infiltrated with a CNT-reporter gene complex at a pressure of 500 mm Hg for 5 min (Dunbar et al., 2022). Izuegbunam et al. (2021) demonstrated that the vacuum infiltration method significantly contributed to the successful uptake of a nano-biomimetic carrier containing plasmid DNA and the subsequent transient activation of a reporter gene in the leaves of Arabidopsis, common ice plant, and tobacco, as well as in the developing seed tissues of Arabidopsis, field mustard, barley, and wheat. This technique enabled efficient delivery of plasmid DNA into plant tissues. The study suggests that this nano-biomimetic transformation strategy, utilizing vacuum infiltration, may facilitate stable transformation in crop species without requiring multiple rounds of selection and breeding to eliminate the plasmid nanocarrier, a step often necessary with carbon-based nanocarriers. The transient activation of a reporter gene and effective uptake of the plasmid DNA carrier were also observed in the leaves of Arabidopsis, common ice plant, and tobacco, as well as in the seed tissues of Arabidopsis, field mustard, barley, and wheat. The study utilized vacuum infiltration at a pressure of −0.01 MPa for 1 minute to introduce the pDNA-R-nHA complex into the detached healthy leaves. The infiltration process for the seeds was repeated three times at 15-minute intervals, ensuring consistent delivery of the plasmid DNA (Izuegbunam et al., 2021). Similarly, few studies have employed vacuum infiltration technology to deliver nanocarrier complexes into plant tissues (Table 2). Overall, vacuum infiltration has proven to be a versatile and effective method for delivering nanoparticle/cargo complexes into various plant tissues, in contrast to syringe infiltration, which is primarily effective for gene delivery into only leaf tissues. While vacuum infiltration has been successfully employed for delivering plasmid DNA into plant tissues, there are no reported instances of its use for delivering siRNA or dsRNA, as seen with syringe infiltration. Additionally, syringe-mediated vacuum infiltration has not yet been explored for the delivery of nanocarrier complexes. Nonetheless, this technique holds significant potential, particularly for applications involving small tissues. Future research should investigate the use of siRNA, dsRNA, and CRISPR constructs as cargos, in combination with nanoparticles as carriers, delivered into plant tissues via both vacuum pump-mediated and syringe-mediated vacuum infiltration approaches. Such advancements could significantly expand the application of vacuum infiltration in plant genetic engineering, offering new possibilities for precision gene editing and crop improvement.
This physical method accelerates micro-sized, DNA-coated projectiles into plant cells or tissues under vacuum conditions (Maliga and Tungsuchat-Huang, 2014; Liu Q. et al., 2020). Utilizing gold or tungsten particles, the technique employs spermidine and CaCl₂ for the incorporation of gene of interest (Macklin et al., 1999). The optimal size of the microprojectiles for plant transformation typically ranges from 0.6 to 1.0 μm in diameter (Tian and Seguin, 2004). The biolistic method applies a selective mechanical force to deliver DNA-coated microprojectiles into plant cells, effectively minimizing damage to the surrounding plant tissue (Liu et al., 2019). As the biolistic approach majorly relies on physical force, this technique do not have species constraint and can deliver gene of interest into wide range of crop species (Cho et al., 2004; Zhang et al., 2015). Further, this method is effective across different plant tissues, including leaf tissues, callus, and even seeds. The biolistic method proved to be a rapid and efficient method of generating transgenic plants via transient and stable transformation (Abdallah et al., 2010; Lacroix and Citovsky, 2020). This approach facilitates the incorporation of multiple genes into plan cells at the same time, which could be challenging to other conventional transformation methods. This method is widely accepted in delivering various molecular cargoes into plant tissues such as plasmid DNA, siRNA, dsRNA, protein, and Cas9-RNP complex (Ueki et al., 2009; Klein et al., 1987; Kikkert et al., 2004; Wu et al., 2019). The utilization of the nanoparticle in the biolistic DNA delivery by functionalizing the microprojectiles with nanoparticles and using nanoparticle as substitute of gold or tungsten microcarriers has been attempted in the last decade, for instance, Torney et al. (2007) showed the first successful co-delivery of nanoparticles and DNA in Nicotiana benthamiana cotyledons using a biolistic approach. In this investigation gold-coated MSNs with a pore size of 3 nm and a diameter of 100–200 nm were employed. These MSNs were loaded with plasmid DNA encoding the reporter gene GFP and promoter, and the nanoparticle ends were capped with gold nanoparticles. Following biolistic delivery, the gold nanoparticle caps were removed, releasing a chemical inducer, β-estradiol, in the presence of dithiothreitol in the regeneration media. This release activated transient GFP expression in the cotyledons. Martin-Ortigosa et al. (2014) reported the development and evaluation of a novel platform composed of gold nanoparticles attached to mesoporous silica nanoparticles (Au-MSN) using a biolistic particle delivery mechanism. Specifically, the microprojectile, which consisted of gold nanoparticles functionalized with mesoporous silica nanoparticles (Au-MSN), was employed for this purpose. To demonstrate proof-of-concept, the study showed that Au-MSN with relatively large pore sizes (approximately 10 nm) could effectively carry and release both proteins and plasmid DNA within the same cell. This was achieved after the particles penetrated the plant cell wall through bombardment. This advancement represents the first instance of the biolistic-mediated co-delivery of proteins and plasmid DNA to plant cells using gold-functionalized mesoporous silica nanoparticles. In order to avoid the functionalization of microparticle with nanoparticle and to use nanoparticle alone as microcarrier in the biolistic DNA delivery in plants, Rajkumari et al. (2021) investigated the use of silver nanoparticles as substitute for gold microcarriers for biolistic gene transfer in tobacco. The study compared the efficacy of biolistic transformation using silver nanoparticles (100 nm) and gold microcarriers (0.6 microns) across various concentrations. The silver nanoparticles were delivered using helium pressure of 650 psi and a target distance of 9 cm, while the gold microcarriers were delivered at a pressure of 900 psi and a distance of 6 cm. The highest GUS expression was observed with both the gold microcarriers and silver nanoparticles. Notably, the use of silver nanoparticles resulted in significantly higher transformation efficiency compared to gold microcarriers. This improvement in efficiency also led to a substantial reduction in the cost of consumables, with expenses decreasing by up to 37.5 times. Additionally, in the biolistic DNA delivery method, relatively little attention has been given to the critical steps of DNA binding to and release from gold particles, both of which are essential for the success of the delivery process. A crucial element in these processes is the use of cationic molecules, as their positively charged amine groups enable electrostatic binding between negatively charged gold particles and DNA. Although various molecules contain amine groups, spermidine is almost universally used in biolistic protocols (Fadeev et al., 2006; Wang and Frame, 2009). Despite its widespread use, the formation of the DNA-gold complex has not been thoroughly investigated. Moreover, spermidine is expensive and cannot be synthesized under typical laboratory conditions, which adds to the cost and complexity of biolistic DNA delivery. Therefore, future research should focus on evaluating the efficiency of amine-functionalized nanoparticles as a potential alternative to spermidine for the effective binding of DNA to microcarriers. Additionally, studies should explore the use of amine-coated nanoparticles and silver nanoparticles, as suggested by Rajkumari et al. (2021) as cost-effective replacements for spermidine and gold microparticles, respectively (Figure 3). These advancements could significantly reduce the expenses associated with biolistic DNA delivery technology.
Figure 3. Schematic representation biolistic DNA delivery using amine coated nanoparticles such as carbon dots as a replacement for spermidine, which acts as binding agent between tungsten particles and plasmid DNA and biolistic DNA delivery using silver nanoparticles as a replacement for gold or tungsten microcarriers.
Magnetofection is a technique of incorporating exogenous nucleic acid into plant cells using a magnetic field (Gong et al., 2021; Wang B. et al., 2020). This method uses MNPs or MONPS, usually made up of Fe3O4, with sizes ranging from 5 nm to 14 nm in diameter (Alonso et al., 2013; Ludwig et al., 2017). The exogenous nucleic acid-coated magnetic nanoparticles are incubated with plant cell suspension to allow the nanoparticle to associate with the cells. Applying the magnetic field strength ranging from 0.1 to 1 T for a duration of 10 min to 1 h facilitates efficient uptake of exogenous nucleic acid-coated magnetic nanoparticles into plant cells (Figure 4). However, the strength and duration of the magnetic field greatly depend on plant species and type of nanoparticles (Wang et al., 2022b). Magnetofection is an effective method for stable pollen transformation, often called as pollen magnetofection, which involves the direct delivery of exogenous nucleic acid-coated magnetic nanoparticles into pollen grains to attain genomic edits transmitted directly to their progeny without involving the regeneration process (Park et al., 2024b). For instance, Zhao et al. (2017) introduced an innovative transformation platform known as pollen magnetofection, which enables the direct production of transgenic seeds without the need for regeneration. This method involves using a magnetic field to deliver magnetic nanoparticles carrying exogenous DNA into pollen. When plants are pollinated with this magnetofected pollen, transgenic plants are directly produced from the transformed seeds. The exogenous DNA is successfully integrated into the plant genome, expressed, and stably passed on to subsequent generations. Wang et al. (2022) presented an innovative, culture-free, and genotype-independent method for maize transfection. To improve DNA delivery efficiency while maintaining high pollen viability, MNPs coated with plasmid DNA encoding the reporter genes like RFP, GUS, EGFP, or the bialaphos resistance gene were introduced into maize pollen grains using the magnetofection technique. After pollination of female florets from maize inbred lines with the magnetofected pollen, red fluorescence was observed in 22% of the transfected pollen grains, and GUS staining was detected in 55% of embryos 18 days after pollination. This method demonstrates the potential for efficient gene delivery in maize, offering a promising tool for plant genetic engineering. Zhang et al. (2023) introduced an optimized transient transformation method using pollen magnetofection in Lilium regale. The study achieved the highest pollen germination rate (85.73%) and transformation efficiency (88.32%) with a specific ratio of nanomagnetic beads to DNA plasmid and a short transformation time. Transgenic seedlings showed strong GUS activity, indicating successful gene transfer. However, transformation efficiency varied among lily germplasm, suggesting species or cultivar specificity in the effectiveness of this method. George (2018) explored the use of magnetofection as a transformation system for tobacco mesophyll protoplasts, optimizing conditions and assessing the impact on protoplast viability. By applying a strong external magnetic field, magnetofectins were directed towards protoplasts, achieving transient GUS gene expression 48 h post-transfection. The highest transformation efficiency was observed with MNP weight ratios of 1:5 and 1:10. However, increasing the density of MNPs negatively impacted protoplast viability. An optimal MNP density of 1.0 μg/mL was identified, which supported gene expression without compromising protoplast viability. Farooq et al. (2022) reported the synthesis of green iron nanoparticles using Camellia sinensis leaf extract and iron chloride. These nanoparticles were employed to develop a novel method for gene transformation in okra (Abelmoschus esculentus) plants, incorporating various magnetofection factors. The study found that the highest gene transformation efficiency was achieved with a DNA-to-iron nanoparticle ratio of 1:20, by rotating the mixture of plasmid DNA, iron nanoparticles, and seed embryos at 800 rpm for 5 h during the magnetofection process. This method successfully facilitated the transformation of the GFP gene in okra plants, highlighting the effectiveness of this approach for plant genetic engineering. Overall, the delivery of nanoparticle-cargo complexes into plant tissues through magnetofection technology shows great promise for achieving both transient and stable gene expression. However, this gene delivery technique has predominantly been used to introduce genes of interest into pollen, with limited studies exploring its application in other plant tissues, such as protoplasts and seeds. Therefore, future research should focus on expanding the use of magnetofection to deliver cargos into various plant tissues, including protoplasts, seeds, and embryos. Moreover, successful magnetofection requires careful optimization of several parameters, including the size and coating of magnetic nanoparticles, the strength of the magnetic field, and the duration of exposure. Fine-tuning these factors is essential to enhance the efficiency and reliability of this technology for broader applications in plant biotechnology.
Figure 4. Schematic representation of nanoparticle mediated gene delivery into pollen grains or seeds using magnetofection technique.
Ultrasound-based delivery is an innovative technique for introducing genetic material into protoplasts or intact plant cells using high-frequency sound waves (Choudhary and Chin, 1995). Sonication induces acoustic cavitation, producing transient pores in cell membranes, which facilitates the entry of DNA or RNA into the cells. In this process, plant parts are mixed with exogenous nucleic acids in an appropriate buffer or carrier solution. The entire suspension is then exposed to ultrasound waves at various frequencies for a certain duration, minimizing excessive damage to plant cells. This technique has been utilized in nanoparticle-mediated gene delivery to increase the efficacy of gene transfer (Lo et al., 2014) (Figure 5). When the nanoparticle and cargo complex solution is subjected to ultrasound waves, tiny pores form on the cell surfaces, leading to the internalization of the nanocarrier complex into the plant tissues. In comparison to direct DNA delivery methods, sonication (ultrasound) provides a more straightforward and cost-efficient approach that is not limited by plant species. However, it may lead to cell damage or rupture. Thus, optimizing ultrasound conditions is essential to improve DNA uptake without harming the cells. Studies have shown that moderate ultrasound irradiation of 20 kHz–50 kHz is an effective method for transfection, both in vitro and in vivo (Liu et al., 2006). Particularly in gene delivery using sonication technology, it is crucial to prevent DNA degradation caused by ultrasound exposure (Sanzari et al., 2019). This can be achieved by encapsulating or loading the genetic material onto a suitable carrier. At present, a range of nanoparticles, including metal oxides, polymers, and carbon or silica-based nanomaterials, are used as nano-carriers (Li et al., 2019). Sonication enhances the efficiency of cargo delivery by promoting the internalization of the nanocarrier complex through cavitation in plant cells. As a result, the process is mutually beneficial. Zolghadrnasab et al. (2021) developed a highly efficient method for gene transfection in plant suspension cells by employing pDNA-loaded PEI-MSNs in combination with ultrasonic treatment. The results demonstrated that PEI-MSNs could adsorb a significant amount of DNA. When assessing the impact of ultrasonic treatment on the viability of tobacco plant cells, it was found that increasing the ultrasonic intensity from 160 W to 320 W, and then to 640 W, led to substantial cell damage, while extending the duration of the treatment caused less severe degradation. The evaluation of gene transfection efficiency under various conditions indicated that the use of PEI-MSNs as a gene carrier was essential for effective gene delivery into plant cells. Additionally, the incorporation of ultrasonic treatment had a synergistic effect, significantly enhancing gene transfection efficiency. Amani et al. (2018) explored the potential of cationic polyamidoamine (PAMAM) dendrimers, specifically hyperbranched PAMAM (hPAMAM)-G2 with a diethylenetriamine core, as nanocarriers for gene delivery. They assessed the capacity of these dendrimers to bind with DNA, shield it from ultrasonic damage, and facilitate its delivery into alfalfa cells. The study found that without the aid of nanocarriers, naked DNA was unable to penetrate the cell walls and membranes of alfalfa cells, likely due to its morphology, size, and charge. However, when sonication was applied in conjunction with hPAMAM dendrimers, it facilitated the successful delivery of DNA into the cells, highlighting the critical role of sonication in enhancing the efficiency of nanocarrier-based gene delivery. Sangwan et al. (2023) developed 60 nm core–shell piezoelectric nanoparticles, specifically barium titanate nanoparticles (BTNPs) coated with a silica shell, for efficient nucleic acid delivery into monocot and dicot suspension cells using sonoporation. The study optimized ultrasonic treatment times 5 min for dicots and 8 min for monocots at a constant intensity of 160 W and frequency of 40 kHz, ensuring cell viability. BTNPs demonstrated a DNA adsorption capacity of 40.1 μg per mg. GUS analysis showed significant enhancements in transfection efficiency, with increases of 56.9% and 72.6% in dicot and monocot cells, respectively, compared to naked pDNA delivery. This approach shows promise for genotype-independent transfection. Overall, the use of nanoparticle-mediated gene delivery into plant cells via sonication is a promising and straightforward technique that enhances the efficiency of nanocarrier complex uptake. Nanocarriers protect DNA from degradation, while sonication facilitates cavitation, promoting the efficient internalization of the nanocarrier complex. However, despite its potential, the application of sonoporation technology in nanoparticle-mediated gene delivery remains limited. Most studies have focused on plasmid DNA, leaving a gap in research on the use of other genetic materials such as siRNA, dsRNA, and CRISPR constructs. Future investigations should explore these materials with nanoparticle-mediated delivery and sonication to broaden the applicability and effectiveness of this gene delivery approach.
Figure 5. A schematic illustration shows nanoparticle-mediated gene delivery into plant cells using ultrasound (sonication), highlighting the delivery mechanism.
This is an innovative approach that involves the integration of exogenous DNA into plants through the application of a spray with a transformation solution. In spray method generally genetic material is blended with carrier solution containing suitable surfactants or adjuvants to enhance the uptake of genetic material (Wang and Jin, 2017a; Rank and Koch, 2021). The transformed solution is applied to the plant surfaces using a standard spray bottle or atomizer. The surfactants soften the lipid bilayer and open pores for internalization of genetic material (Thagun et al., 2022; Wang and Jin, 2017b). While in nanoparticle mediated gene delivery using the spray method, initially the nanoparticle solution would be mixed with the desired concentration of cargos and the mixture is mixed well and subject to the incubation to form a nanoparticle and cargo complex. Then the conjugate would spray to the plant surface, enabling their absorption through stomata or cuticular pores (Figure 6). After being absorbed by the plant, nanoparticles go through the walls and membranes of plant cells, releasing cargo that becomes part of the plant’s cellular machinery, after spraying the formulation the plant should ideally transferred to the growth chamber having the temperature of 25°C. This process has the capability to activate expression of gene of interest in the plant (Mitter et al., 2017). As it is a non-invasive technique and easier to use in a wide range of crop species, this method can be applied to whole plants or large areas of plants, which is helpful for field trials and large-scale applications. This method is used to deliver various molecular cargoes into plant tissues such as dsRNA and siRNA to achive gene knock down in combination with the nanoparticle sinstead of exogenous application od DsRNA or siRNA. Although earlier studies suggested that naked RNAs applied externally could effectively silence genes in plants (Dubrovina et al., 2019), more recent research has demonstrated that this approach is generally ineffective. The likely reason for this ineffectiveness is the negative charge of RNA molecules, which is due to their phosphate groups. This negative charge makes it difficult for RNA to cross the plant cell’s plasma membrane, which is also have a negative charge. This barrier highlights the need for alternative delivery strategies, such as nanoparticle carriers, to increase the molecular cargo uptake and achieve effective gene knockdown in plants (Zhang et al., 2021; Zhang et al., 2022). Nanoparticle/cargo2 complexes with neutralized surfaces have been shown to effectively silence genes, likely due to their capability to cross the plasma membrane. These complexes have demonstrated high efficacy in the knockdown of gene of interest within living plant cells, making them a promising tool for gene regulation (Azeem et al., 2021; Demirer et al., 2021). Therefore, the application of dsRNA or siRNA using nanoparticle as carrier has been using as powefull method of gene regulation using different insertion techniques, more importantly using spraying technique. For instance, Functionalized carbon dots were complexed with screened double-stranded RNAs (dsRNA-CDs) through electrostatic interactions, facilitating spray-induced gene silencing to control Phytophthora species. The spray application of dsRNA-CD complexes substantially increased the control efficiency against Phytophthora infestans, Phytophthora sojae, and both wild-type and fungicide-resistant P. capsici (Wang et al., 2023). In another investigation, a spray technique was used to deliver layered double hydroxide (LDH) nanoparticles complexed with dsRNA to Nicotiana benthamiana leaves. The virus-specific dsRNA-LDH complexes, applied through spraying, successfully activated the plant’s RNA interference (RNAi) machinery, reducing the accumulation of the Tomato Yellow Leaf Curl Virus genome and mitigating symptom development (Hernandez, 2021). Polymer-functionalized graphene oxide nanoparticles (GONs) were also utilized in a spray method to deliver siRNA into intact leaf cells. The spray-applied GON-siRNA complexes penetrated the cell wall and internalized within the plant cells, achieving short-term but highly effective gene silencing, with 97.2% efficiency observed 24 h post-application (Li et al., 2022). Furthermore, Cai et al. (2024) developed a novel spray-based approach using MSNs for siRNA delivery into intact plant leaves. This spray technique achieved up to 98% gene knockdown efficiency at the molecular level without the need for mechanical forces. The method proved to be non-toxic to plant leaves, allowing for repeated siRNA applications for sustained gene silencing. Similarly, several studies have employed spray based technique to deliver nanocarrier complexes into intact leaves (Table 2). Nanoparticle-mediated cargo delivery via the spray method has emerged as one of the most efficient techniques for applying nanocarrier complexes, particularly in achieving gene regulation. However, the efficiency of this spray-based genetic transformation varies depending on the plant species, tissue type, and the formulation of the genetic material used (Sembada and Lenggoro, 2024). While this method is predominantly used to deliver dsRNA and siRNA using nanoparticles as carriers for gene regulation, there remains a need for future research to explore the delivery of CRISPR constructs through nanoparticle-based spray applications.
Figure 6. (A) Schematic representation of nanoparticle-mediated siRNA/dsRNA delivery against different pests using the spray method. (B) Schematic representation of nanoparticle mediated gene delivery into plant tissues using passive diffusion/cellular uptake mechanism.
This approach leverages the natural mechanisms to introduce the exogenous nucleic acid into plant tissue without the aid of external forces. In contrast to external force-assisted gene delivery, passive gene delivery typically involves plant system routes such as leaves and roots for the introduction of nucleic acids (Sembada and Lenggoro, 2024). To carry exogenous nucleic acids, nanoparticles must be compatible with the pore sizes of stomata and root hairs (Figure 6). The ideal size of the nanoparticles used in plant passive transformations ranges from 10 to 40 nm in diameter. The passive gene transformation takes the symplastic routes for the uptake and further translocation of nanoparticles in plant cells. Nanoparticle particles such as oxides of Zn, Au, Ce, Ti, and exclusive carbon are widely employed in passive gene transformation. Due to their microscopic size, nanoparticles are readily absorbed by roots or leaves and taken into the plant vascular route to deliver the exogenous DNA into plant cells without damaging the cell. Generally the passive gene transformation works efficiently where plants require delivering exogenous nucleic acid into plant cells non-invasively (Pal et al., 2024). The study found that plant cell walls can block the entry of particles larger than 5–20 nm without needing any external force (Cunningham et al., 2018; Wang Z.-P. et al., 2020), tested whether 5 nm carbon dots could penetrate walled plant cells and carry a DNA molecule without external aids like a gene gun or ultrasound. Rice Nipponbare plant root tips were soaked in a mixture of carbon dots and plasmid to assess cellular uptake. After 12 h, confocal microscopy showed strong mCherry fluorescence in nearly all root tip cells, indicating that the nanoparticle-plasmid DNA complex can easily pass through the plant cell wall without external assistance, validating the passive diffusion mechanism. Currently, protoplasts are utilized to accelerate plant genetic screening and to facilitate the production of recombinant proteins. This necessitates a transformation platform that is user-friendly and an efficient delivery method that 1can be adapted to various species (Schaumberg et al., 2016). For this purpose, (Demirer et al., 2019) utilized intact, healthy protoplasts derived from arugula leaves to deliver plasmid DNA encoding a nuclear localization signal (UBQ10-GFP), which directs the expressed GFP from the cytosol to the nucleus. They employed carbon nanotubes as carriers to facilitate DNA delivery through a passive diffusion mechanism without the use of any external force. The study demonstrated a protoplast transformation efficiency of 76%. They demonstrated that nanoparticles like carbon nanotubes can swiftly enter plant plastids, with their penetration through the lipid layer happening within seconds of exposure. This nanoparticle-based approach facilitates the rapid and passive delivery of plasmid DNA into protoplasts, achieving high transgene expression efficiency without any noticeable adverse effects on the viability of the protoplasts. Moreover, the use of nanoparticles as carriers has been successfully applied in plants for the delivery of RNA molecules aimed at specific gene knockdown. For instance, (Pal et al., 2024) assessed the effectiveness of dsRNA-induced gene silencing in rice, targeting the phytoene desaturase (PDS) gene using a seedling dip-inoculation method. Three-day-old seedlings were exposed to either naked pds-dsRNA or pds-dsRNA combined with a cationic poly-aspartic acid-derived polymer (CPP6), allowing absorption through the roots. Ten days post-treatment, seedlings exposed to pds-dsRNA showed reduced growth and appeared pale or white. The height of seedlings treated with pds-dsRNA-CPP6 was significantly reduced compared to those treated with CPP6 alone and the control group. This highlights the effectiveness of the cellular uptake method in silencing the targeted gene. This method is used effectively to deliver dsRNA to various plant species, such as Arabidopsis and Cucumber (Pal et al., 2024; Delgado-Martín et al., 2022). Similarly, several studies have employed this technique to deliver nanocarrier complexes into plant tissues (Table 2). Overall, the nanoparticle mediated gene delivery into plant tissues through passive diffusion or cellular uptake mechanism is cost effective and straightforward approach (Sembada and Lenggoro, 2024). However, this method also encounters several challenges, including the size variation in the nanoparticle depending upon pores, channels, or structures within the plant tissue.
Recent progress in nanoparticle-mediated gene delivery techniques has led to remarkable plant genetic engineering advancements. Conventional methods like Agrobacterium-mediated transformation, biolistic particle bombardment, and PEG-mediated delivery have played a pivotal role in shaping the landscape of genetic engineering in plants. However, these techniques often face limitations such as species dependency, potential tissue damage, and varying levels of efficiency. These constraints highlight the need for more versatile and efficient delivery systems, especially as the demand for more precise genetic modifications continues to grow. Nanoparticle-mediated delivery is a promising alternative and addresses many limitations of the conventional methods. The nanoparticle-mediated gene delivery demonstrates the potential of nanoparticles in overcoming barriers such as the plant cell wall and enhancing the uptake of genetic material. The versatility of nanoparticles allows for enhanced compatibility with a wide range of plant species, facilitating both transient and stable genetic transformations. The various delivery methods explored in this review - syringe infiltration, vacuum infiltration, biolistic delivery, magnetofection, ultrasound-based delivery, and spray techniques possess unique benefits. Among these techniques, the spray method has emerged as a highly effective, non-invasive approach, particularly suited for large-scale applications. It efficiently delivers dsRNA and siRNA using nanoparticles as carriers, demonstrating its promise in gene regulation. However, the efficiency of spray-based transformation varies depending on factors such as plant species and tissue type, highlighting the need for further optimization, as most studies have been conducted on Nicotiana benthamiana. Despite the successful application of other gene delivery methods for delivering plasmid DNA into various plant tissues, the delivery of dsRNA and siRNA through these techniques remains underexplored. In the case of biolistic gene delivery systems, amine-coated nanoparticles could potentially replace spermidine or other amine-coated molecules as binding agents between DNA and microcarriers. This would further promote the use of nanoparticles in biolistic DNA delivery, where a binding agent plays a crucial role. Notably, the delivery of CRISPR constructs into plant tissues has been scarcely reported across current delivery methods. Therefore, optimizing the discussed techniques is essential for the efficient delivery of CRISPR constructs for gene knockout applications in plants. Furthermore, the combination of multiple delivery methods, such as sonication and vacuum infiltration previously successful in Agrobacterium-mediated transformation holds promise for enhancing the efficiency and versatility of nanoparticle-mediated gene delivery (Amal et al., 2020). This approach can potentially improve the penetration of the nanoparticle-cargo complex into the plant genome by utilizing sonic waves, while the vacuum infiltration can increase the efficiency of gene transfer. The application of vacuum infiltration following sonication may create additional access points for the nanoparticle complex, facilitating better integration of the genetic material into plant tissues. This combined strategy could offer a significant improvement in the precision and effectiveness of plant genetic transformations. Additionally, despite the phototoxic effects of nanomaterials posing a significant challenge to the advancement of agricultural applications, materials that have already demonstrated efficacy in delivering various biomolecules into plant tissues have proven to be safe and capable of protecting nucleic acids from nuclease activity (Kim et al., 2020). Concurrently, they exhibit significant biocompatibility, thermal stability, and colloidal stability without eliciting a protective immunological response in target plant tissues and a wide range of plant species (Zhan et al., 2020). However, negatively charged nanoparticles necessitate functionalization to render them positively charged, which facilitates efficient conjugation with negatively charged biomolecules. Polyethyleneimine is predominantly employed in numerous studies for this purpose; however, many investigations have demonstrated that high molecular weight polyethyleneimine induces cytotoxicity in animal cells (Lungwitz et al., 2005). Considering this issue, it is advisable to utilize less toxic compounds such as low molecular weight polyethyleneimine, beta-alanine, arginine, and chitosan, which have been shown to be safe for nanoparticle functionalization without significant cytotoxic effects (Wang et al., 2020a; kwak et al., 2019). The utilization of nanoparticles as carriers for delivering biomolecules into diverse plant species raises potential ethical and regulatory concerns, as nanoparticles may inadvertently pose environmental risks through accumulation in soil and water, as well as in edible plant tissues; therefore, comprehensive risk and safety assessments are necessary. Moreover, an in-depth investigation is essential to properly elucidate the interaction between nanoparticles and the ecosystem. Furthermore, various nanoparticles have demonstrated antibacterial properties against bacterial species; consequently, the accumulation of these nanoparticles in soil or water will significantly affect the bacteria essential for the normal growth and development of plants, particularly during the extensive application of the nanocargo complex via the spray method.
KS: Writing–original draft, Writing–review and editing, Methodology. SM: Writing–original draft, Writing–review and editing. KD: Investigation, Writing–original draft, Writing–review and editing. AT: Funding acquisition, Investigation, Supervision, Writing–original draft, Writing–review and editing. ZY: Investigation, Supervision, Writing–original draft, Writing–review and editing.
The author(s) declare financial support was received for the research, authorship, and/or publication of this article. This research was supported in part by the intramural research program of the U.S. Department of Agriculture, National Institute of Food and Agriculture, Evans-Allen: 7000916, USDA 1890 Capacity Building Grant: 2023-38821-39803 and the College of Agriculture, TSU.
The authors declare that the research was conducted in the absence of any commercial or financial relationships that could be construed as a potential conflict of interest.
The author(s) declare that no Generative AI was used in the creation of this manuscript.
All claims expressed in this article are solely those of the authors and do not necessarily represent those of their affiliated organizations, or those of the publisher, the editors and the reviewers. Any product that may be evaluated in this article, or claim that may be made by its manufacturer, is not guaranteed or endorsed by the publisher.
The Supplementary Material for this article can be found online at: https://www.frontiersin.org/articles/10.3389/fnano.2025.1516180/full#supplementary-material
SUPPLEMENTARY FIGURE S1 | Graphical representation of reference distribution by year of publication included in this review article.
Abdallah, N. A., Shah, D., Abbas, D., and Madkour, M. (2010). Stable integration and expression of a plant defensin in tomato confers resistance to fusarium wilt. Gm. Crops 1, 344–350. doi:10.4161/gmcr.1.5.15091
Ali, Z., Serag, M. F., Demirer, G. S., Torre, B., Di Fabrizio, E., Landry, M. P., et al. (2022). DNA–Carbon nanotube binding mode determines the efficiency of carbon nanotube-mediated DNA delivery to intact plants. ACS Appl. Nano Mater. 5, 4663–4676. doi:10.1021/acsanm.1c03482
Alonso, A., Davies, G.-L., Satti, A., Macanás, J., Gun’ko, Y. K., Muñoz, M., et al. (2013). “Intermatrix synthesis and characterization of polymer-stabilized functional metal and metal oxide nanoparticles,” in Nanocomposites. Editors L. Nicolais, and G. Carotenuto (Wiley), 165–194. doi:10.1002/9781118742655.ch8
Altpeter, F., Springer, N. M., Bartley, L. E., Blechl, A., Brutnell, T. P., Citovsky, V., et al. (2016). Advancing crop transformation in the Era of genome editing. Plant Cell 28, 1510–1520. doi:10.1105/tpc.16.00196
Amal, T. C., Karthika, P., Dhandapani, G., Selvakumar, S., and Vasanth, K. (2020). A simple and efficient Agrobacterium-mediated in planta transformation protocol for horse gram (Macrotyloma uniflorum Lam. Verdc.). J. Genet. Eng. Biotechnol. 18, 9. doi:10.1186/s43141-020-00023-z
Amani, A., Zare, N., Asadi, A., and Asghari-Zakaria, R. (2018). Ultrasound-enhanced gene delivery to alfalfa cells by hPAMAM dendrimer nanoparticles. Turk J. Biol. 42, 63–75. doi:10.3906/biy-1706-6
Ameen Rashid, A. H., and Dhahir Lateef, D. (2016). Novel techniques for gene delivery into plants and its applications for disease resistance in crops. AJPS 07 07, 181–193. doi:10.4236/ajps.2016.71019
Anami, S., Njuguna, E., Coussens, G., Aesaert, S., and Van Lijsebettens, M. (2013). Higher plant transformation: principles and molecular tools. Int. J. Dev. Biol. 57, 483–494. doi:10.1387/ijdb.130232mv
Azeem, I., Adeel, M., Ahmad, M. A., Shakoor, N., Jiangcuo, G. D., Azeem, K., et al. (2021). Uptake and accumulation of nano/microplastics in plants: a critical review. Nanomaterials 11, 2935. doi:10.3390/nano11112935
Ben-Haim, A. E., Feldbaum, R. A., Belausov, E., Zelinger, E., Maria, R., Nativ-Roth, E., et al. (2024). DNA delivery to intact plant cells by casein nanoparticles with confirmed gene expression. Adv. Funct. Mater. 34. doi:10.1002/adfm.202314756
Bhaskar, P. B., Venkateshwaran, M., Wu, L., Ané, J.-M., and Jiang, J. (2009). Agrobacterium-mediated transient gene expression and silencing: a rapid tool for functional gene assay in potato. PLoS ONE 4, e5812. doi:10.1371/journal.pone.0005812
Bian, Y., Zhang, X., Chen, C., Wang, G., Zhang, P., Liu, G., et al. (2024). Establish an efficient inoculation system of tomato yellow leaf curl virus to assist tomato resistance breeding. Sci. Hortic. 324, 112567. doi:10.1016/j.scienta.2023.112567
Burris, K. P., Dlugosz, E. M., Collins, A. G., Stewart, C. N., and Lenaghan, S. C. (2016). Development of a rapid, low-cost protoplast transfection system for switchgrass (Panicum virgatum L.). Plant Cell Rep. 35, 693–704. doi:10.1007/s00299-015-1913-7
Cai, Y., Liu, Z., Wang, H., Meng, H., and Cao, Y. (2024). Mesoporous silica nanoparticles mediate SiRNA delivery for long-term multi-gene silencing in intact plants. Adv. Sci. 11, e2301358. doi:10.1002/advs.202301358
Campos, G., Chialva, C., Miras, S., and Lijavetzky, D. (2021). New technologies and strategies for grapevine breeding through genetic transformation. Front. Plant Sci. 12, 767522. doi:10.3389/fpls.2021.767522
Canto, T. (2016). “Transient expression systems in plants: potentialities and constraints,” in Advanced technologies for protein complex production and characterization. Editor M. C. Vega (Cham: Springer International Publishing), 287–301. doi:10.1007/978-3-319-27216-0_18
Chen, J., Wang, Y., Wang, F., Yang, J., Gao, M., Li, C., et al. (2015). The rice CK2 kinase regulates trafficking of phosphate transporters in response to phosphate levels. Plant Cell 27, 711–723. doi:10.1105/tpc.114.135335
Cho, J.-Y., Bhowmik, P., Polowick, P. L., Dodard, S. G., El-Bakkari, M., Nowak, G., et al. (2020). Cellular delivery of plasmid DNA into wheat microspores using rosette nanotubes. ACS Omega 5, 24422–24433. doi:10.1021/acsomega.0c02830
Cho, M.-J., Yano, H., Okamoto, D., Kim, H.-K., Jung, H.-R., Newcomb, K., et al. (2004). Stable transformation of rice (Oryza sativa L.) via microprojectile bombardment of highly regenerative, green tissues derived from mature seed. Plant Cell Rep. 22, 483–489. doi:10.1007/s00299-003-0713-7
Choudhary, M. L., and Chin, C. K. (1995). Ultrasound mediated delivery of compounds into petunia protoplasts and cells. J. Plant Biochem. Biotechnol. 4, 37–39. doi:10.1007/BF03262948
Chung, Y. H., Church, D., Koellhoffer, E. C., Osota, E., Shukla, S., Rybicki, E. P., et al. (2021). Integrating plant molecular farming and materials research for next-generation vaccines. Nat. Rev. Mater 7, 372–388. doi:10.1038/s41578-021-00399-5
Coy, M. R., Abbitt, S. E., and Frank, M. J. (2022). “Protoplast isolation and transfection in maize,” in Methods in molecular biology (New York, NY: Springer US), 91–104. Available at: https://link.springer.com/10.1007/978-1-0716-2164-6_7 (Accessed September 16, 2024).
Cunningham, F. J., Demirer, G. S., Goh, N. S., Zhang, H., and Landry, M. P. (2020). “Nanobiolistics: an emerging genetic transformation approach,” in Biolistic DNA delivery in plants. Editors S. Rustgi, and H. Luo (New York, NY: Springer US), 141–159. doi:10.1007/978-1-0716-0356-7_7
Cunningham, F. J., Goh, N. S., Demirer, G. S., Matos, J. L., and Landry, M. P. (2018). Nanoparticle-mediated delivery towards advancing plant genetic engineering. Trends Biotechnol. 36, 882–897. doi:10.1016/j.tibtech.2018.03.009
Davies, K. M., Deroles, S. C., Boase, M. R., Hunter, D. A., and Schwinn, K. E. (2013). “Biolistics-based gene silencing in plants using a modified particle inflow gun,” in Biolistic DNA delivery. Editors S. Sudowe, and A. B. Reske-Kunz (Totowa, NJ: Humana Press), 63–74. doi:10.1007/978-1-62703-110-3_6
Delgado-Martín, J., Delgado-Olidén, A., and Velasco, L. (2022). Carbon dots boost dsRNA delivery in plants and increase local and systemic siRNA production. IJMS 23, 5338. doi:10.3390/ijms23105338
Demirer, G. S., Silva, T. N., Jackson, C. T., Thomas, J. B., W. Ehrhardt, D., Rhee, S. Y., et al. (2021). Nanotechnology to advance CRISPR–Cas genetic engineering of plants. Nat. Nanotechnol. 16, 243–250. doi:10.1038/s41565-021-00854-y
Demirer, G. S., Zhang, H., Goh, N. S., Pinals, R. L., Chang, R., and Landry, M. P. (2020). Carbon nanocarriers deliver siRNA to intact plant cells for efficient gene knockdown. Sci. Adv. 6, eaaz0495. doi:10.1126/sciadv.aaz0495
Demirer, G. S., Zhang, H., Matos, J. L., Goh, N. S., Cunningham, F. J., Sung, Y., et al. (2019). High aspect ratio nanomaterials enable delivery of functional genetic material without DNA integration in mature plants. Nat. Nanotechnol. 14, 456–464. doi:10.1038/s41565-019-0382-5
Deng, X., Cao, M., Zhang, J., Hu, K., Yin, Z., Zhou, Z., et al. (2014). Hyaluronic acid-chitosan nanoparticles for co-delivery of MiR-34a and doxorubicin in therapy against triple negative breast cancer. Biomaterials 35, 4333–4344. doi:10.1016/j.biomaterials.2014.02.006
Doudna, J. A., and Charpentier, E. (2014). Genome editing. The new frontier of genome engineering with CRISPR-Cas9. Science 346, 1258096. doi:10.1126/science.1258096
Doyle, C., Higginbottom, K., Swift, T. A., Winfield, M., Bellas, C., Benito-Alifonso, D., et al. (2019). A simple method for spray-on gene editing in planta. doi:10.1101/805036
Du, N., and Pijut, P. M. (2009). Agrobacterium-mediated transformation of Fraxinus pennsylvanica hypocotyls and plant regeneration. Plant Cell Rep. 28, 915–923. doi:10.1007/s00299-009-0697-z
Dubrovina, A. S., Aleynova, O. A., Kalachev, A. V., Suprun, A. R., Ogneva, Z. V., and Kiselev, K. V. (2019). Induction of transgene suppression in plants via external application of synthetic dsRNA. IJMS 20, 1585. doi:10.3390/ijms20071585
Duclercq, J., Sangwan-Norreel, B., Catterou, M., and Sangwan, R. S. (2011). De novo shoot organogenesis: from art to science. Trends Plant Sci. 16, 597–606. doi:10.1016/j.tplants.2011.08.004
Dunbar, T., Tsakirpaloglou, N., Septiningsih, E. M., and Thomson, M. J. (2022). Carbon nanotube-mediated plasmid DNA delivery in rice leaves and seeds. IJMS 23, 4081. doi:10.3390/ijms23084081
Fadeev, V., Blinkova, O., and Gaponenko, A. (2006). Optimization of biological and physical parameters for biolistic genetic transformation of common wheat (Triticum aestivum L.) using a particle inflow gun. Russ. J. Genet. 42, 402–411. doi:10.1134/S1022795406040077
Farooq, N., Ather, L., Shafiq, M., Nawaz-ul-Rehman, M. S., Haseeb, M., Anjum, T., et al. (2022). Magnetofection approach for the transformation of okra using green iron nanoparticles. Sci. Rep. 12, 16568. doi:10.1038/s41598-022-20569-x
George, A. C. S. (2018). Magnetofection of tobacco protoplasts: a novel mechanism for plant transformation. Doctoral dissertation, Murdoch University. Available at: https://www.semanticscholar.org/paper/Magnetofection-of-tobacco-protoplasts%3A-a-novel-for-George/76bcc1d5d6291a8596fbbcd9031d76bdb1b1a47b
Golestanipour, A., Nikkhah, M., Aalami, A., and Hosseinkhani, S. (2018). Gene delivery to tobacco root cells with single-walled carbon nanotubes and cell-penetrating fusogenic peptides. Mol. Biotechnol. 60, 863–878. doi:10.1007/s12033-018-0120-5
Gong, J., Tian, Z., Qu, X., Meng, Q., Guan, Y., Liu, P., et al. (2021). Illuminating the cells: transient transformation of citrus to study gene functions and organelle activities related to fruit quality. Hortic. Res. 8, 175. doi:10.1038/s41438-021-00611-1
Guidarelli, M., and Baraldi, E. (2015). Transient transformation meets gene function discovery: the strawberry fruit case. Front. Plant Sci. 6, 444. doi:10.3389/fpls.2015.00444
Gustafson, V., Mallubhotla, S., MacDonnell, J., Sanyal-Bagchi, M., Chakravarty, B., Wang-Pruski, G., et al. (2006). Transformation and plant regeneration from leaf explants of Solanum tuberosum L. Cv. ‘shepody. Plant Cell Tiss. Organ Cult. 85, 361–366. doi:10.1007/s11240-006-9085-3
Gyawali, B., Rahimi, R., Alizadeh, H., and Mohammadi, M. (2024). Graphene quantum dots (GQD)-Mediated dsRNA delivery for the control of Fusarium head blight disease in wheat. ACS Appl. Bio Mater. 7, 1526–1535. doi:10.1021/acsabm.3c00972
Hajiahmadi, Z., Shirzadian-Khorramabad, R., Kazemzad, M., and Sohani, M. M. (2019). Enhancement of tomato resistance to Tuta absoluta using a new efficient mesoporous silica nanoparticle-mediated plant transient gene expression approach. Sci. Hortic. 243, 367–375. doi:10.1016/j.scienta.2018.08.040
Hamada, H., Linghu, Q., Nagira, Y., Miki, R., Taoka, N., and Imai, R. (2017). An in planta biolistic method for stable wheat transformation. Sci. Rep. 7, 11443. doi:10.1038/s41598-017-11936-0
Hamers, R. J. (2017). Nanomaterials and global sustainability. Acc. Chem. Res. 50, 633–637. doi:10.1021/acs.accounts.6b00634
Hernandez, E. S. (2021). Layered double hydroxide (LDH)-mediated topical delivery of dsRNA for protection against Tomato yellow leaf curl virus (TYLCV) in Nicotiana benthamiana. KAUST Res. Repos. doi:10.25781/KAUST-S36CI
Huang, C., Zhang, Y., Yuan, H., Gao, H., and Zhang, S. (2013). Role of nanoparticle geometry in endocytosis: laying down to stand up. Nano Lett. 13, 4546–4550. doi:10.1021/nl402628n
Islam, M. R., Youngblood, M., Kim, H.-I., González-Gamboa, I., Monroy-Borrego, A. G., Caparco, A. A., et al. (2024). DNA delivery by virus-like nanocarriers in plant cells. Nano Lett. 24, 7833–7842. doi:10.1021/acs.nanolett.3c04735
Izuegbunam, C. L., Wijewantha, N., Wone, B., Ariyarathne, M. A., Sereda, G., and Wone, B. W. M. (2021). A nano-biomimetic transformation system enables in planta expression of a reporter gene in mature plants and seeds. Nanoscale Adv. 3, 3240–3250. doi:10.1039/d1na00107h
Kant, R., and Dasgupta, I. (2017). Phenotyping of VIGS-mediated gene silencing in rice using a vector derived from a DNA virus. Plant Cell Rep. 36, 1159–1170. doi:10.1007/s00299-017-2156-6
Kar, S., Loganathan, M., Dey, K., Shinde, P., Chang, H.-Y., Nagai, M., et al. (2018). Single-cell electroporation: current trends, applications and future prospects. J. Micromech. Microeng. 28, 123002. doi:10.1088/1361-6439/aae5ae
Kaur, M., Manchanda, P., Kalia, A., Ahmed, F. K., Nepovimova, E., Kuca, K., et al. (2021). Agroinfiltration mediated scalable transient gene expression in genome edited crop plants. IJMS 22, 10882. doi:10.3390/ijms221910882
Keum, C., Hirschbiegel, C.-M., Chakraborty, S., Jin, S., Jeong, Y., and Rotello, V. M. (2023). Biomimetic and bioorthogonal nanozymes for biomedical applications. Nano Converg. 10, 42. doi:10.1186/s40580-023-00390-6
Kikkert, J. R., Vidal, J. R., and Reisch, B. I. (2004). “Stable transformation of plant cells by particle bombardment/biolistics,” in Transgenic plants (New Jersey: Humana Press), 061–078. doi:10.1385/1-59259-827-7:061
Kim, C.-J., Park, J., Hu, X., Albert, S. K., and Park, S.-J. (2020). Peptide-Driven shape control of low-dimensional DNA nanostructures. ACS Nano 14, 2276–2284. doi:10.1021/acsnano.9b09312
Klein, T. M., Wolf, E. D., Wu, R., and Sanford, J. C. (1987). High-velocity microprojectiles for delivering nucleic acids into living cells. Nature 327, 70–73. doi:10.1038/327070a0
Kondo, T., Hasegawa, H., and Suzuki, M. (2000). Transformation and regeneration of garlic (Allium sativum L.) by Agrobacterium -mediated gene transfer. Plant Cell Rep. 19, 989–993. doi:10.1007/s002990000222
Kotnik, T., Kramar, P., Pucihar, G., Miklavcic, D., and Tarek, M. (2012). Cell membrane electroporation- Part 1: the phenomenon. IEEE Electr. Insul. Mag. 28, 14–23. doi:10.1109/MEI.2012.6268438
Krens, F. A., Molendijk, L., Wullems, G. J., and Schilperoort, R. A. (1982). In vitro transformation of plant protoplasts with Ti-plasmid DNA. Nature 296, 72–74. doi:10.1038/296072a0
Kumar, P., Nagarajan, A., and Uchil, P. D. (2019). Electroporation. Cold Spring Harb. Protoc. 2019, pdb.top096271. doi:10.1101/pdb.top096271
Kumar, S., Raj, S. K., Sharma, A. K., and Varma, H. N. (2012). Genetic transformation and development of Cucumber mosaic virus resistant transgenic plants of Chrysanthemum morifolium cv. Kundan. Sci. Hortic. 134, 40–45. doi:10.1016/j.scienta.2011.10.019
Kwak, S.-Y., Lew, T. T. S., Sweeney, C. J., Koman, V. B., Wong, M. H., Bohmert-Tatarev, K., et al. (2019). Chloroplast-selective gene delivery and expression in planta using chitosan-complexed single-walled carbon nanotube carriers. Nat. Nanotechnol. 14, 447–455. doi:10.1038/s41565-019-0375-4
Lacroix, B., and Citovsky, V. (2020). “Biolistic approach for transient gene expression studies in plants,” in Biolistic DNA delivery in plants. Editors S. Rustgi, and H. Luo (New York, NY: Springer US), 125–139. doi:10.1007/978-1-0716-0356-7_6
Law, S. S. Y., Liou, G., Nagai, Y., Giménez-Dejoz, J., Tateishi, A., Tsuchiya, K., et al. (2022). Polymer-coated carbon nanotube hybrids with functional peptides for gene delivery into plant mitochondria. Nat. Commun. 13, 2417. doi:10.1038/s41467-022-30185-y
Law, S. S. Y., Miyamoto, T., and Numata, K. (2023). Organelle-targeted gene delivery in plants by nanomaterials. Chem. Commun. 59, 7166–7181. doi:10.1039/d3cc00962a
Lei, W.-X., An, Z.-S., Zhang, B.-H., Wu, Q., Gong, W.-J., Li, J.-M., et al. (2020). Construction of gold-siRNANPR1 nanoparticles for effective and quick silencing of NPR1 in Arabidopsis thaliana. RSC Adv. 10, 19300–19308. doi:10.1039/d0ra02156c
Li, S., Li, J., Du, M., Deng, G., Song, Z., and Han, H. (2022). Efficient gene silencing in intact plant cells using siRNA delivered by functional graphene oxide nanoparticles. Angew. Chem. 134, e202210014. doi:10.1002/anie.202210014
Li, Z. (2002). Multiple thiol-anchor capped DNA-gold nanoparticle conjugates. Nucleic Acids Res. 30, 1558–1562. doi:10.1093/nar/30.7.1558
Li, Z., Du, X., Cui, X., and Wang, Z. (2019). Ultrasonic-assisted fabrication and release kinetics of two model redox-responsive magnetic microcapsules for hydrophobic drug delivery. Ultrason. Sonochemistry 57, 223–232. doi:10.1016/j.ultsonch.2019.04.037
Lin, Z., Ali, M. M., Yi, X., Zhang, L., Wang, S., and Chen, F. (2023). Unlocking the potential of carbon quantum dots for cell imaging, intracellular localization, and gene expression control in Arabidopsis thaliana (L.) Heynh. IJMS 24, 15700. doi:10.3390/ijms242115700
Liu, J., Nannas, N. J., Fu, F., Shi, J., Aspinwall, B., Parrott, W. A., et al. (2019). Genome-scale sequence disruption following biolistic transformation in rice and maize. Plant Cell 31, 368–383. doi:10.1105/tpc.18.00613
Liu, Q., Li, Y., Xu, K., Li, D., Hu, H., Zhou, F., et al. (2020b). Clay nanosheet-mediated delivery of recombinant plasmids expressing artificial miRNAs via leaf spray to prevent infection by plant DNA viruses. Hortic. Res. 7, 179. doi:10.1038/s41438-020-00400-2
Liu, W., Rudis, M. R., Cheplick, M. H., Millwood, R. J., Yang, J.-P., Ondzighi-Assoume, C. A., et al. (2020a). Lipofection-mediated genome editing using DNA-free delivery of the Cas9/gRNA ribonucleoprotein into plant cells. Plant Cell Rep. 39, 245–257. doi:10.1007/s00299-019-02488-w
Liu, Y., Yang, F., Jing, X., Liu, X., Wang, G., Jian-Ping, A., et al. (2023). A biomimetic nanoparticle for pDNA delivery and expression in plant cells in a pH-dependent manner. ACS Agric. Sci. Technol. 3, 631–641. doi:10.1021/acsagscitech.3c00068
Liu, Y., Yang, H., and Sakanishi, A. (2006). Ultrasound: mechanical gene transfer into plant cells by sonoporation. Biotechnol. Adv. 24, 1–16. doi:10.1016/j.biotechadv.2005.04.002
Lo, C.-W., Desjouy, C., Chen, S.-R., Lee, J.-L., Inserra, C., Béra, J.-C., et al. (2014). Stabilizing in vitro ultrasound-mediated gene transfection by regulating cavitation. Ultrason. Sonochemistry 21, 833–839. doi:10.1016/j.ultsonch.2013.10.017
Lopes, R. C. F. G., Rocha, B. G. M., Maçôas, E. M. S., Marques, E. F., and Martinho, J. M. G. (2022). Combining metal nanoclusters and carbon nanomaterials: opportunities and challenges in advanced nanohybrids. Adv. Colloid Interface Sci. 304, 102667. doi:10.1016/j.cis.2022.102667
Ludwig, F., Balceris, C., Viereck, T., Posth, O., Steinhoff, U., Gavilan, H., et al. (2017). Size analysis of single-core magnetic nanoparticles. J. Magnetism Magnetic Mater. 427, 19–24. doi:10.1016/j.jmmm.2016.11.113
Lungwitz, U., Breunig, M., Blunk, T., and Göpferich, A. (2005). Polyethylenimine-based non-viral gene delivery systems. Eur. J. Pharm. Biopharm. 60, 247–266. doi:10.1016/j.ejpb.2004.11.011
Lv, Z., Jiang, R., Chen, J., and Chen, W. (2020). Nanoparticle-mediated gene transformation strategies for plant genetic engineering. Plant J. 104, 880–891. doi:10.1111/tpj.14973
Macklin, M. D., Drape, R. J., and Swain, W. F. (1999). “Preparations for particle-mediated gene transfer using the Accell® gene gun,” in DNA vaccines (New Jersey: Humana Press), 297–304. doi:10.1385/1-59259-688-6:297
Mahmoud, L. M., Kaur, P., Stanton, D., Grosser, J. W., and Dutt, M. (2022). A cationic lipid mediated CRISPR/Cas9 technique for the production of stable genome edited citrus plants. Plant Methods 18, 33. doi:10.1186/s13007-022-00870-6
Maliga, P., and Tungsuchat-Huang, T. (2014). “Plastid transformation in Nicotiana tabacum and Nicotiana sylvestris by biolistic DNA delivery to leaves,” in Chloroplast biotechnology. Editor P. Maliga (Totowa, NJ: Humana Press), 147–163. doi:10.1007/978-1-62703-995-6_8
Martin-Ortigosa, S., Peterson, D. J., Valenstein, J. S., Lin, V. S.-Y., Trewyn, B. G., Lyznik, L. A., et al. (2014). Mesoporous silica nanoparticle-mediated intracellular cre protein delivery for maize genome editing via loxP site excision. Plant Physiol. 164, 537–547. doi:10.1104/pp.113.233650
Matsuo, K., Fukuzawa, N., and Matsumura, T. (2016). A simple agroinfiltration method for transient gene expression in plant leaf discs. J. Biosci. Bioeng. 122, 351–356. doi:10.1016/j.jbiosc.2016.02.001
Men, S., Ming, X., Wang, Y., Liu, R., Wei, C., and Li, Y. (2003). Genetic transformation of two species of orchid by biolistic bombardment. Plant Cell Rep. 21, 592–598. doi:10.1007/s00299-002-0559-4
Miki, D., and Shimamoto, K. (2004). Simple RNAi vectors for stable and transient suppression of gene function in rice. Plant Cell Physiology 45, 490–495. doi:10.1093/pcp/pch048
Miller, K., Eggenberger, A. L., Lee, K., Liu, F., Kang, M., Drent, M., et al. (2021). An improved biolistic delivery and analysis method for evaluation of DNA and CRISPR-Cas delivery efficacy in plant tissue. Sci. Rep. 11, 7695. doi:10.1038/s41598-021-86549-9
Mitter, N., Worrall, E. A., Robinson, K. E., Li, P., Jain, R. G., Taochy, C., et al. (2017). Clay nanosheets for topical delivery of RNAi for sustained protection against plant viruses. Nat. Plants 3, 16207. doi:10.1038/nplants.2016.207
Moher, D., Liberati, A., Tetzlaff, J., and Altman, D. G. (2009). Preferred reporting items for systematic reviews and meta-analyses: the PRISMA statement. PLoS Med. 6, e1000097. doi:10.1371/journal.pmed.1000097
Molesini, B., Pennisi, F., Cressoni, C., Vitulo, N., Dusi, V., Speghini, A., et al. (2022). Nanovector-mediated exogenous delivery of dsRNA induces silencing of target genes in very young tomato flower buds. Nanoscale Adv. 4, 4542–4553. doi:10.1039/D2NA00478J
Molla, K. A., Sretenovic, S., Bansal, K. C., and Qi, Y. (2021). Precise plant genome editing using base editors and prime editors. Nat. Plants 7, 1166–1187. doi:10.1038/s41477-021-00991-1
Moore, N. M., Sheppard, C. L., and Sakiyama-Elbert, S. E. (2009). Characterization of a multifunctional PEG-based gene delivery system containing nuclear localization signals and endosomal escape peptides. Acta Biomater. 5, 854–864. doi:10.1016/j.actbio.2008.09.009
Mora, C., McKenzie, T., Gaw, I. M., Dean, J. M., Von Hammerstein, H., Knudson, T. A., et al. (2022). Over half of known human pathogenic diseases can be aggravated by climate change. Nat. Clim. Chang. 12, 869–875. doi:10.1038/s41558-022-01426-1
Narusaka, Y., Narusaka, M., Yamasaki, S., and Iwabuchi, M. (2012). “Methods to transfer foreign genes to plants,” in Transgenic plants - advances and limitations (InTech). doi:10.5772/32773
Nayak, S., and Das, P. (2021). Covalent conjugation of carbon dots with plasmid and DNA condensation thereafter: realistic insights into the condensate morphology, energetics, and photophysics. ACS Omega 6, 21425–21435. doi:10.1021/acsomega.1c02247
O’Leary, B. M., Rico, A., McCraw, S., Fones, H. N., and Preston, G. M. (2014). The infiltration-centrifugation technique for extraction of apoplastic fluid from plant leaves using Phaseolus vulgaris as an example. JoVE, 52113. doi:10.3791/52113
Orzaez, D., Mirabel, S., Wieland, W. H., and Granell, A. (2006). Agroinjection of tomato fruits. A tool for rapid functional analysis of transgenes directly in fruit. Plant Physiol. 140, 3–11. doi:10.1104/pp.105.068221
Ozyigit, I. I. (2020). Gene transfer to plants by electroporation: methods and applications. Mol. Biol. Rep. 47, 3195–3210. doi:10.1007/s11033-020-05343-4
Pal, G., Ingole, K. D., Yavvari, P. S., Verma, P., Kumari, A., Chauhan, C., et al. (2024). Exogenous application of nanocarrier-mediated double-stranded RNA manipulates physiological traits and defence response against bacterial diseases. Mol. Plant Pathol. 25, e13417. doi:10.1111/mpp.13417
Park, C.-W., Choi, J.-Y., Son, Y.-J., Kim, D.-H., Li, H., Liang, W., et al. (2024a). Magnetofected pollen gene delivery system could generate genetically modified Cucumis sativus. Hortic. Res. 11, uhae179. doi:10.1093/hr/uhae179
Park, C.-W., Choi, J.-Y., Son, Y.-J., Kim, D.-H., Li, H., Liang, W., et al. (2024b). Magnetofected pollen gene delivery system could generate genetically modified Cucumis sativus. Hortic. Res. 11, uhae179. doi:10.1093/hr/uhae179
Parra-Torrejón, B., Cáceres, A., Sánchez, M., Sainz, L., Guzmán, M., Bermúdez-Perez, F. J., et al. (2023). Multifunctional nanomaterials for biofortification and protection of tomato plants. Environ. Sci. Technol. 57, 14950–14960. doi:10.1021/acs.est.3c02559
Pasupathy, K., Lin, S., Hu, Q., Luo, H., and Ke, P. C. (2008). Direct plant gene delivery with a poly(amidoamine) dendrimer. Biotechnol. J. 3, 1078–1082. doi:10.1002/biot.200800021
Přibylová, A., Fischer, L., Pyott, D. E., Bassett, A., and Molnar, A. (2022). DNA methylation can alter CRISPR/Cas9 editing frequency and DNA repair outcome in a target-specific manner. New Phytol. 235, 2285–2299. doi:10.1111/nph.18212
Qi, J., Li, Y., Yao, X., Li, G., Xu, W., Chen, L., et al. (2024). Rational design of ROS scavenging and fluorescent gold nanoparticles to deliver siRNA to improve plant resistance to Pseudomonas syringae. J. Nanobiotechnol 22, 446. doi:10.1186/s12951-024-02733-9
Rajkumari, N., Alex, S., Soni, K. B., Anith, K. N., Viji, M. M., and Kiran, A. G. (2021). Silver nanoparticles for biolistic transformation in Nicotiana tabacum L. 3 Biotech. 11, 497. doi:10.1007/s13205-021-03043-9
Ranjbaran, M., and Datta, A. K. (2019). Pressure-driven infiltration of water and bacteria into plant leaves during vacuum cooling: a mechanistic model. J. Food Eng. 246, 209–223. doi:10.1016/j.jfoodeng.2018.10.032
Rank, A. P., and Koch, A. (2021). Lab-to-Field transition of RNA spray applications – how far are we? Front. Plant Sci. 12, 755203. doi:10.3389/fpls.2021.755203
Sabbadini, S., Capriotti, L., Molesini, B., Pandolfini, T., Navacchi, O., Limera, C., et al. (2019). Comparison of regeneration capacity and Agrobacterium-mediated cell transformation efficiency of different cultivars and rootstocks of Vitis spp. via organogenesis. Sci. Rep. 9, 582. doi:10.1038/s41598-018-37335-7
Sangwan, A., Gupta, D., Singh, O. W., Roy, A., Mukherjee, S. K., Mandal, B., et al. (2023). Size variations of mesoporous silica nanoparticle control uptake efficiency and delivery of AC2-derived dsRNA for protection against tomato leaf curl New Delhi virus. Plant Cell Rep. 42, 1571–1587. doi:10.1007/s00299-023-03048-z
Santana, I., Wu, H., Hu, P., and Giraldo, J. P. (2020). Targeted delivery of nanomaterials with chemical cargoes in plants enabled by a biorecognition motif. Nat. Commun. 11, 2045. doi:10.1038/s41467-020-15731-w
Santi, L., Batchelor, L., Huang, Z., Hjelm, B., Kilbourne, J., Arntzen, C. J., et al. (2008). An efficient plant viral expression system generating orally immunogenic Norwalk virus-like particles. Vaccine 26, 1846–1854. doi:10.1016/j.vaccine.2008.01.053
Sanzari, I., Leone, A., and Ambrosone, A. (2019). Nanotechnology in plant science: to make a long story short. Front. Bioeng. Biotechnol. 7, 120. doi:10.3389/fbioe.2019.00120
Schaumberg, K. A., Antunes, M. S., Kassaw, T. K., Xu, W., Zalewski, C. S., Medford, J. I., et al. (2016). Quantitative characterization of genetic parts and circuits for plant synthetic biology. Nat. Methods 13, 94–100. doi:10.1038/nmeth.3659
Schöb, H., Kunz, C., and Meins, F. (1997). Silencing of transgenes introduced into leaves by agroinfiltration: a simple, rapid method for investigating sequence requirements for gene silencing. Mol. Gen. Genet. 256, 581–585. doi:10.1007/s004380050604
Schwartz, S. H., Hendrix, B., Hoffer, P., Sanders, R. A., and Zheng, W. (2020). Carbon dots for efficient small interfering RNA delivery and gene silencing in plants. Plant Physiol. 184, 647–657. doi:10.1104/pp.20.00733
Sembada, A. A., and Lenggoro, I. W. (2024). Transport of nanoparticles into plants and their detection methods. Nanomaterials 14, 131. doi:10.3390/nano14020131
Sharma, P., Holliger, N., Pfromm, P. H., Liu, B., and Chikan, V. (2020). Size-controlled synthesis of iron and iron oxide nanoparticles by the rapid inductive heating method. ACS Omega 5, 19853–19860. doi:10.1021/acsomega.0c02793
Shou, H., Frame, B. R., Whitham, S. A., and Wang, K. (2004). Assessment of transgenic maize events produced by particle bombardment or Agrobacterium-mediated transformation. Mol. Breed. 13, 201–208. doi:10.1023/B:MOLB.0000018767.64586.53
Simmons, C. W., Nitin, N., and VanderGheynst, J. S. (2012). Rapid, in situ detection of Agrobacterium tumefaciens attachment to leaf tissue. Biotechnol. Prog. 28, 1321–1328. doi:10.1002/btpr.1608
Simmons, C. W., VanderGheynst, J. S., and Upadhyaya, S. K. (2009). A model of Agrobacterium tumefaciens vacuum infiltration into harvested leaf tissue and subsequent in planta transgene transient expression. Biotech and Bioeng. 102, 965–970. doi:10.1002/bit.22118
Song, J., Lu, S., Chen, Z.-Z., Lourenco, R., and Chiang, V. L. (2006). Genetic transformation of Populus trichocarpa genotype nisqually-1: a functional genomic tool for woody plants. Plant Cell Physiology 47, 1582–1589. doi:10.1093/pcp/pcl018
Sparkes, I. A., Runions, J., Kearns, A., and Hawes, C. (2006). Rapid, transient expression of fluorescent fusion proteins in tobacco plants and generation of stably transformed plants. Nat. Protoc. 1, 2019–2025. doi:10.1038/nprot.2006.286
Squire, H. J., Tomatz, S., Voke, E., González-Grandío, E., and Landry, M. (2023). The emerging role of nanotechnology in plant genetic engineering. Nat. Rev. Bioeng. 1, 314–328. doi:10.1038/s44222-023-00037-5
Su, W., Xu, M., Radani, Y., and Yang, L. (2023). Technological development and application of plant genetic transformation. IJMS 24, 10646. doi:10.3390/ijms241310646
Sun, X., Hu, Z., Chen, R., Jiang, Q., Song, G., Zhang, H., et al. (2015). Targeted mutagenesis in soybean using the CRISPR-Cas9 system. Sci. Rep. 5, 10342. doi:10.1038/srep10342
Thagun, C., Horii, Y., Mori, M., Fujita, S., Ohtani, M., Tsuchiya, K., et al. (2022). Non-transgenic gene modulation via spray delivery of nucleic acid/peptide complexes into plant nuclei and chloroplasts. ACS Nano 16, 3506–3521. doi:10.1021/acsnano.1c07723
Tian, L., and Seguin, A. (2004). Microprojectile particle effect on stable transformation of black spruce via bombardment. Plant Mol. Biol. Rep. 22, 199. doi:10.1007/BF02772729
Toldi, O., Tuba, Z., and Scott, P. (2009). Vegetative desiccation tolerance: is it a goldmine for bioengineering crops? Plant Sci. 176, 187–199. doi:10.1016/j.plantsci.2008.10.002
Torney, F., Trewyn, B. G., Lin, V. S.-Y., and Wang, K. (2007). Mesoporous silica nanoparticles deliver DNA and chemicals into plants. Nat. Nanotech 2, 295–300. doi:10.1038/nnano.2007.108
Ueki, S., Lacroix, B., Krichevsky, A., Lazarowitz, S. G., and Citovsky, V. (2009). Functional transient genetic transformation of Arabidopsis leaves by biolistic bombardment. Nat. Protoc. 4, 71–77. doi:10.1038/nprot.2008.217
Vaghchhipawala, Z., Rojas, C. M., Senthil-Kumar, M., and Mysore, K. S. (2011). “Agroinoculation and agroinfiltration: simple tools for complex gene function analyses (2011),” in Methods in molecular biology (Totowa, NJ: Humana Press), 65–76. doi:10.1007/978-1-60761-682-5_6
Vanitharani, R., Chellappan, P., and Fauquet, C. M. (2003). Short interfering RNA-mediated interference of gene expression and viral DNA accumulation in cultured plant cells. Proc. Natl. Acad. Sci. U.S.A. 100, 9632–9636. doi:10.1073/pnas.1733874100
Venugopal, P. D., and Dively, G. P. (2017). Climate change, transgenic corn adoption and field-evolved resistance in corn earworm. R. Soc. open Sci. 4, 170210. doi:10.1098/rsos.170210
Vyacheslavova, A. O., Berdichevets, I. N., Tyurin, A. A., Shimshilashvili, Kh. R., Mustafaev, O. N., and Goldenkova-Pavlova, I. V. (2012). Expression of heterologous genes in plant systems: new possibilities. Russ. J. Genet. 48, 1067–1079. doi:10.1134/S1022795412110130
Wang, B., Huang, J., Zhang, M., Wang, Y., Wang, H., Ma, Y., et al. (2020b). Carbon dots enable efficient delivery of functional DNA in plants. ACS Appl. Bio Mater. 3, 8857–8864. doi:10.1021/acsabm.0c01170
Wang, H., Mukherjee, S., Yi, J., Banerjee, P., Chen, Q., and Zhou, S. (2017). Biocompatible chitosan–carbon dot hybrid nanogels for NIR-imaging-guided synergistic photothermal–chemo therapy. ACS Appl. Mater. Interfaces 9, 18639–18649. doi:10.1021/acsami.7b06062
Wang, H., Wang, W., Zhan, J., Huang, W., and Xu, H. (2015). An efficient PEG-mediated transient gene expression system in grape protoplasts and its application in subcellular localization studies of flavonoids biosynthesis enzymes. Sci. Hortic. 191, 82–89. doi:10.1016/j.scienta.2015.04.039
Wang, K., and Frame, B. (2009). “Biolistic gun-mediated maize genetic transformation (2009),” in Methods in molecular biology (Totowa, NJ: Humana Press), 29–45. doi:10.1007/978-1-59745-494-0_3
Wang, M., and Jin, H. (2017a). Spray-induced gene silencing: a powerful innovative strategy for crop protection. Trends Microbiol. 25, 4–6. doi:10.1016/j.tim.2016.11.011
Wang, M., and Jin, H. (2017b). Spray-induced gene silencing: a powerful innovative strategy for crop protection. Trends Microbiol. 25, 4–6. doi:10.1016/j.tim.2016.11.011
Wang, Z., Li, Y., Zhang, B., Gao, X., Shi, M., Zhang, S., et al. (2023). Functionalized carbon dot-delivered RNA nano fungicides as superior tools to control Phytophthora pathogens through plant RdRP1 mediated spray-induced gene silencing. Adv. Funct. Mater. 33. doi:10.1002/adfm.202213143
Wang, Z., Zhang, Z., Zheng, D., Zhang, T., Li, X., Zhang, C., et al. (2022). Efficient and genotype independent maize transformation using pollen transfected by DNA-coated magnetic nanoparticles. JIPB 64, 1145–1156. doi:10.1111/jipb.13263
Wang, Z. P., Zhang, Z. B., Li, X. L., Zhang, C., Yin, L. F., Yu, R., et al. (2020a). Efficient and genotype independent maize pollen transfection mediated by magnetic nanoparticles. doi:10.21203/rs.3.rs-97984/v1
Wong, M. H., Misra, R. P., Giraldo, J. P., Kwak, S.-Y., Son, Y., Landry, M. P., et al. (2016). Lipid exchange envelope penetration (LEEP) of nanoparticles for plant engineering: a universal localization mechanism. Nano Lett. 16, 1161–1172. doi:10.1021/acs.nanolett.5b04467
Woo, J. W., Kim, J., Kwon, S. I., Corvalán, C., Cho, S. W., Kim, H., et al. (2015). DNA-free genome editing in plants with preassembled CRISPR-Cas9 ribonucleoproteins. Nat. Biotechnol. 33, 1162–1164. doi:10.1038/nbt.3389
Wroblewski, T., Tomczak, A., and Michelmore, R. (2005). Optimization of Agrobacterium -mediated transient assays of gene expression in lettuce, tomato and Arabidopsis. Plant Biotechnol. J. 3, 259–273. doi:10.1111/j.1467-7652.2005.00123.x
Wu, H., Acanda, Y., Canton, M., and Zale, J. (2019). Efficient biolistic transformation of immature citrus rootstocks using phosphomannose-isomerase selection. Plants 8, 390. doi:10.3390/plants8100390
Xu, K., Huang, X., Wu, M., Wang, Y., Chang, Y., Liu, K., et al. (2014). A rapid, highly efficient and economical method of agrobacterium-mediated in planta transient transformation in living onion epidermis. PLoS ONE 9, e83556. doi:10.1371/journal.pone.0083556
Xu, X., Yu, T., Zhang, D., Song, H., Huang, K., Wang, Y., et al. (2023). Evaluation of the anti-viral efficacy of three different dsRNA nanoparticles against potato virus Y using various delivery methods. Ecotoxicol. Environ. Saf. 255, 114775. doi:10.1016/j.ecoenv.2023.114775
Yan, H., Fu, D., Zhu, B., Liu, H., Shen, X., and Luo, Y. (2012). Sprout vacuum-infiltration: a simple and efficient agroinoculation method for virus-induced gene silencing in diverse solanaceous species. Plant Cell Rep. 31, 1713–1722. doi:10.1007/s00299-012-1285-1
Yan, T., Hou, Q., Wei, X., Qi, Y., Pu, A., Wu, S., et al. (2023). Promoting genotype-independent plant transformation by manipulating developmental regulatory genes and/or using nanoparticles. Plant Cell Rep. 42, 1395–1417. doi:10.1007/s00299-023-03037-2
Yin, K., Gao, C., and Qiu, J.-L. (2017). Progress and prospects in plant genome editing. Nat. Plants 3, 17107. doi:10.1038/nplants.2017.107
Yong, J., Wu, M., Carroll, B. J., Xu, Z. P., and Zhang, R. (2024). Enhancing plant biotechnology by nanoparticle delivery of nucleic acids. Trends Genet. 40, 352–363. doi:10.1016/j.tig.2024.01.005
Yong, J., Wu, M., Zhang, R., Bi, S., Mann, C. W. G., Mitter, N., et al. (2022). Clay nanoparticles efficiently deliver small interfering RNA to intact plant leaf cells. Plant Physiol. 190, 2187–2202. doi:10.1093/plphys/kiac430
Yong, J., Zhang, R., Bi, S., Li, P., Sun, L., Mitter, N., et al. (2021). Sheet-like clay nanoparticles deliver RNA into developing pollen to efficiently silence a target gene. Plant Physiol. 187, 886–899. doi:10.1093/plphys/kiab303
Yu, D., Wang, A., Huang, H., and Chen, Y. (2008). PEG-PBLG nanoparticle-mediated HSV-TK/GCV gene therapy for oral squamous cell carcinoma. Nanomedicine 3, 813–821. doi:10.2217/17435889.3.6.813
Yu, P., Zheng, X., Alimi, L. O., Al-Babili, S., and Khashab, N. M. (2024). Metal–organic framework-mediated delivery of nucleic acid across intact plant cells. ACS Appl. Mater. Interfaces 16, 18245–18251. doi:10.1021/acsami.3c19571
Zhan, Y., Ma, W., Zhang, Y., Mao, C., Shao, X., Xie, X., et al. (2020). Diversity of DNA nanostructures and applications in oncotherapy. Biotechnol. J. 15, 1900094. doi:10.1002/biot.201900094
Zhang, H., Cao, Y., Xu, D., Goh, N. S., Demirer, G. S., Cestellos-Blanco, S., et al. (2021). Gold-nanocluster-mediated delivery of siRNA to intact plant cells for efficient gene knockdown. Nano Lett. 21, 5859–5866. doi:10.1021/acs.nanolett.1c01792
Zhang, H., Goh, N. S., Wang, J. W., Pinals, R. L., González-Grandío, E., Demirer, G. S., et al. (2022). Nanoparticle cellular internalization is not required for RNA delivery to mature plant leaves. Nat. Nanotechnol. 17, 197–205. doi:10.1038/s41565-021-01018-8
Zhang, K., Liu, J., Zhang, Y., Yang, Z., and Gao, C. (2015). Biolistic genetic transformation of a wide range of Chinese elite wheat (Triticum aestivum L.) varieties. J. Genet. Genomics 42, 39–42. doi:10.1016/j.jgg.2014.11.005
Zhang, M., Ma, X., Jin, G., Han, D., Xue, J., Du, Y., et al. (2023). A modified method for transient transformation via pollen magnetofection in Lilium germplasm. IJMS 24, 15304. doi:10.3390/ijms242015304
Zhang, R., Meng, Z., Abid, M. A., and Zhao, X. (2019). “Novel pollen magnetofection system for transformation of cotton plant with magnetic nanoparticles as gene carriers,” in Transgenic cotton. Editor B. Zhang (New York, NY: Springer), 47–54. doi:10.1007/978-1-4939-8952-2_4
Zhao, X., Meng, Z., Wang, Y., Chen, W., Sun, C., Cui, B., et al. (2017). Pollen magnetofection for genetic modification with magnetic nanoparticles as gene carriers. Nat. Plants 3, 956–964. doi:10.1038/s41477-017-0063-z
Zheng, L., Yang, J., Chen, Y., Ding, L., Wei, J., and Wang, H. (2021). An improved and efficient method of Agrobacterium syringe infiltration for transient transformation and its application in the elucidation of gene function in poplar. BMC Plant Biol. 21, 54. doi:10.1186/s12870-021-02833-w
Zhi, H., Zhou, S., Pan, W., Shang, Y., Zeng, Z., and Zhang, H. (2022). The promising nanovectors for gene delivery in plant genome engineering. IJMS 23, 8501. doi:10.3390/ijms23158501
Keywords: nanoparticles, plants, gene delivery, insertion methods, transformation
Citation: Shivashakarappa K, Marriboina S, Dumenyo K, Taheri A and Yadegari Z (2025) Nanoparticle-mediated gene delivery techniques in plant systems. Front. Nanotechnol. 7:1516180. doi: 10.3389/fnano.2025.1516180
Received: 24 October 2024; Accepted: 21 January 2025;
Published: 06 February 2025.
Edited by:
Ilika Ghosh, Max Planck Florida Institute for Neuroscience (MPFI), United StatesReviewed by:
Amit K. Roy, Northeastern University, United StatesCopyright © 2025 Shivashakarappa, Marriboina, Dumenyo, Taheri and Yadegari. This is an open-access article distributed under the terms of the Creative Commons Attribution License (CC BY). The use, distribution or reproduction in other forums is permitted, provided the original author(s) and the copyright owner(s) are credited and that the original publication in this journal is cited, in accordance with accepted academic practice. No use, distribution or reproduction is permitted which does not comply with these terms.
*Correspondence: Zeinab Yadegari, enlhZGVnYXJpQGZpc2suZWR1; Ali Taheri, YWxpLnRhaGVyaUB0bnN0YXRlLmVkdQ==
Disclaimer: All claims expressed in this article are solely those of the authors and do not necessarily represent those of their affiliated organizations, or those of the publisher, the editors and the reviewers. Any product that may be evaluated in this article or claim that may be made by its manufacturer is not guaranteed or endorsed by the publisher.
Research integrity at Frontiers
Learn more about the work of our research integrity team to safeguard the quality of each article we publish.