- 1Department of Biochemistry, Bharathidasan University, Tiruchirappalli, Tamil Nadu, India
- 2Department of Clinical Sciences, Center of Medical and Bio-allied Health Sciences and Research, College of Dentistry, Ajman University, Ajman, United Arab Emirates
- 3Department of Public Health Dentistry, Thai Moogambigai Dental College and Hospital, Dr. MGR Educational and Research Institute, Chennai, Tamil Nadu, India
- 4Department of Anatomy, Bhaarath Medical College and hospital, Bharath Institute of Higher Education and Research (BIHER), Chennai, Tamil Nadu, India
- 5Faculty of Agricultural Sciences Food Industry and Environmental Protection, Lucian Blaga University of Sibiu, Sibiu, Romania
- 6Department of Chemical Technology, Faculty of Science, Chulalongkorn University, Bangkok, Thailand
Nanoparticles are attractive therapeutic tools due to their distinctive characteristics, including more accurate drug delivery, improved bioavailability, and enhanced targeted therapy. This review offers a comprehensive analysis of the therapeutic potentials of cutting-edge nanoparticles as demonstrated in human clinical trials, based on empirical evidence. Through systematic searches of major scientific databases, relevant studies published up to March 2024 were included, focusing on clinical trials utilizing advanced nanoparticles for therapeutic purposes. The review discusses the diverse applications of nanoparticles in oncology, infectious diseases, neurology, and other medical fields. Additionally, it scrutinizes the safety profiles, efficacy outcomes, and challenges associated with nanoparticle-based therapies. The findings underscore significant progress in translating nanoparticle research into clinical practice and highlight the potential of these innovative platforms to revolutionize medical treatments. This review contributes valuable insights into the growing field of nanoparticle-based therapeutics, fostering a deeper understanding of their clinical applications and implications in medical practice.
1 Introduction
Nanoparticles, those minuscule entities with dimensions less than 100 nm, have emerged as veritable powerhouses in the realm of medical research (Palanisamy et al., 2020). Despite their tiny dimensions, nanoparticles harbor vast potential due to their distinctive physical and chemical characteristics, making them exceptionally versatile and appealing for a wide array of biomedical uses (Pei et al., 2023). From drug delivery to imaging, diagnostics, and even regenerative medicine, nanoparticles have garnered significant attention for their ability to revolutionize medical treatments and improve patient outcomes (Palanisamy et al., 2022). The attraction of nanoparticles lies in their extraordinary characteristics, which set them apart from conventional materials. A distinguishing characteristic of nanoparticles is their exceptionally elevated surface area-to-volume ratio. Their diminutive size enables them to boast a disproportionately large surface area in comparison to their volume, offering abundant prospects for engaging with biological molecules and cellular frameworks (Manimaran et al., 2022). This property is particularly advantageous in drug delivery, as it allows nanoparticles to efficiently encapsulate therapeutic agents and deliver them to target tissues or cells with precision (Lavanya et al., 2023). Furthermore, nanoparticles offer customizable surface chemistry, which can be tailored to suit specific biomedical applications. By functionalizing nanoparticle surfaces with targeting ligands or antibodies, researchers can direct them to specific sites within the body, enabling targeted therapy with minimal off-target effects (Pei et al., 2024a). This capability holds immense promise for personalized medicine, as nanoparticles can be designed to deliver therapeutics to individual patients based on their unique molecular profiles (Pei et al., 2024b). In addition to their surface properties, nanoparticles exhibit unique optical and magnetic characteristics that make them invaluable tools for medical imaging. Nanoparticle-based contrast agents, like iron oxide particles used in magnetic resonance imaging (MRI) or quantum dots for fluorescence imaging, present enhanced imaging capabilities in contrast to traditional agents. These advanced imaging techniques provide clinicians with high-resolution visualization of biological structures and pathological processes, facilitating timely detection, precise diagnosis, and customized treatment strategizing (Rezaei et al., 2024).
The potential of nanoparticles in medicine is further underscored by their ability to surmount physiological obstacles that constrain the effectiveness of traditional treatments. As an illustration, nanoparticles have the capability to circumvent the blood-brain barrier, a formidable obstacle that prevents many drugs from reaching the brain. By encapsulating therapeutic agents within nanoparticles, researchers can navigate around this barrier, enabling the direct delivery of medications to the brain, thus unveiling novel avenues for addressing neurological conditions like Alzheimer’s disease and brain tumors (Huang et al., 2024). Moreover, nanoparticles offer controlled release kinetics, allowing for the sustained release of therapeutic agents over time. This property is particularly advantageous in drug delivery, allows for extended drug presence at the specific site, reducing the risk of systemic toxicity. By modulating the release profile of therapeutic agents, nanoparticles can optimize drug pharmacokinetics, enhance therapeutic efficacy, and improve patient compliance with treatment regimens (Kashkooli et al., 2020). Despite their immense potential, the translation of nanoparticle-based therapies from laboratory bench to bedside necessitates rigorous evaluation through human clinical trials. Clinical trials are regarded as the benchmark for evaluating the safety, effectiveness, and viability of novel therapeutic methods within actual patient populations. By systematically evaluating nanoparticle formulations in clinical settings, researchers can optimize dosage regimens, define therapeutic windows, and identify potential adverse effects or drug interactions (Halwani, 2022).
Furthermore, clinical trials are invaluable for validating preclinical findings, refining experimental protocols, and overcoming translational challenges including scalability, reproducibility, and regulatory compliance (Đorđević et al., 2022). Collaboration among academia, industry, regulatory agencies, and patient advocacy organizations is crucial for designing and conducting well-controlled clinical trials that adhere to ethical standards and regulatory requirements (Wichman et al., 2021). Nanoparticles represent a promising frontier in medicine, with the potential to revolutionize medical treatments across various disease areas. Their distinct physical and chemical attributes render them indispensable assets for drug delivery, imaging, diagnostics, and precise therapeutic interventions (Liu et al., 2023). However, realizing the full potential of nanoparticle-based therapies requires ongoing research, interdisciplinary collaboration, and rigorous evaluation through well-designed clinical trials. By harnessing the insights gained from clinical trials, researchers and clinicians can leverage the transformative power of nanoparticles to improve patient outcomes and propel the practice of medicine into a new era of precision and efficacy (Hu et al., 2020).
2 Types of nanoparticles
Nanoparticles display remarkable diversity and can be classified into various categories based on their composition, size, shape, and characteristics which are; Metallic Nanoparticles: Comprising metals like gold, silver, iron, or platinum, these particles possess distinctive optical, electrical, and catalytic properties. They find utility in catalysis, sensing, imaging, and drug delivery applications (Sharma et al., 2021). Polymeric Nanoparticles: Formed from biodegradable or non-biodegradable polymers like poly (lactic-co-glycolic acid) (PLGA), polyethylene glycol (PEG), or polystyrene, these nanoparticles find widespread use in drug delivery systems due to their compatibility with biological systems, customizable characteristics, and ability to encase various therapeutic substances (Pulingam et al., 2022). Lipid-Based Nanoparticles: This group of nanoparticles comprises liposomes, solid lipid nanoparticles (SLNs), nanostructured lipid carriers (NLCs), and lipid nanoparticles, composed of lipids like phospholipids, cholesterol, or triglycerides. They are commonly utilized for drug delivery, gene therapy, and vaccine delivery purposes (Sheoran et al., 2022). Carbon-Based Nanoparticles: Encompassing fullerenes, carbon nanotubes (CNTs), graphene, and carbon dots, these nanoparticles exhibit exceptional mechanical, electrical, and optical properties. They find applications in electronics, sensors, drug delivery, and biomedical imaging (Sharma et al., 2020). Ceramic Nanoparticles: Ceramic nanoparticles are composed of inorganic materials such as silica, alumina, titania, or zirconia. These nanoparticles have high mechanical strength, thermal stability, and chemical inertness, this renders them valuable in various fields, including catalysis, drug delivery, tissue engineering, and biomedical imaging (Treccani, 2023). Quantum Dots: Quantum dots are semiconductor nanoparticles with unique optical properties, including size-tunable fluorescence and high photostability. Quantum dots are used in biological imaging, biosensing, photovoltaics, and light-emitting devices (Sobhanan et al., 2023). Magnetic Nanoparticles: Magnetic nanoparticles, like iron oxide nanoparticles, display magnetic characteristics and find application in biomedicine, this encompasses magnetic resonance imaging (MRI), targeted drug delivery, and hyperthermia therapy (Montiel Schneider et al., 2022). Composite Nanoparticles: Composite nanoparticles are made up of various components, usually two or more, mixed together, such as metal-polymer composites, lipid-polymer hybrids, or carbon-metal hybrids. These nanoparticles combine the properties of their constituent materials and are employed in a multitude of applications such as drug delivery, imaging, sensing, and catalysis (Liu et al., 2020). These are just a few examples of the diverse range of nanoparticles available, and researchers continue to explore new materials and synthesis methods to develop nanoparticles with specified qualities for use in medicine, electronics, energy, and environmental cleanup.
3 General therapeutic mechanism of nanoparticles
The mechanisms of action of nanoparticles vary depending on circumstances like their composition, size, shape, and surface characteristics, as well as the intended application. Nonetheless, certain overarching mechanisms can be outlined;
Drug Delivery: Nanoparticles are widely used as carriers for therapeutic compounds in medication delivery systems. The mechanism of action involves encapsulating drugs within or attaching them to the surface of nanoparticles. Following administration, nanoparticles have the capacity to circulate within the bloodstream and gather at the desired location through passive targeting mechanisms (such as the increased permeability and retention effect observed in malignancies) or active targeting strategies (including ligand-receptor interactions). Upon reaching the target site, nanoparticles release the encapsulated drugs through diffusion, degradation, or triggered release mechanisms, resulting in localized therapeutic effects while minimizing systemic toxicity (Raj et al., 2021).
Imaging: Nanoparticles are used as contrast agents in a range of imaging modalities, including MRI, CT, PET, and fluorescence imaging. The mechanism of action involves the interaction between nanoparticles and electromagnetic radiation or magnetic fields, leading to changes in signal intensity or emission that can be detected and visualized by imaging instruments. Depending on their composition, nanoparticles can enhance contrast, improve resolution, and provide functional information about biological structures and processes (Kalra et al., 2021).
Theranostics: Nanoparticles can be designed for theranostic applications, integrating both therapeutic and diagnostic capabilities into a unified platform. The mechanism of action involves integrating therapeutic agents and imaging probes into nanoparticles, allowing simultaneous monitoring of treatment response and delivery of therapy. Theranostic nanoparticles facilitate tailored medical interventions through providing real-time feedback on treatment efficacy, optimizing therapeutic regimens, and facilitating early intervention based on individual patient responses (Llop and Lammers, 2021).
Gene Delivery: Nanoparticles are used as carriers for nucleic acids such as DNA, RNA, and small interfering RNA (siRNA) in gene therapy experiments. The process involves compacting nucleic acids into nanoparticles to shield them from degradation and enhance cellular absorption. Once internalized by target cells, nanoparticles release the nucleic acids, allowing them to interact with intracellular machinery and modulate gene expression. Gene delivery nanoparticles hold promise for treating genetic disorders, cancer, and infectious diseases by correcting or suppressing abnormal gene expression (Yadav et al., 2023).
Hyperthermia Therapy: Magnetic nanoparticles have applications in hyperthermia therapy, a treatment approach that involves heating tumor tissues to induce cell death. The mechanism of action involves the administration of magnetic nanoparticles, delivering them specifically to the tumour location, followed by the external administration of an alternating magnetic field (AMF) (Peñate Medina et al., 2020). The magnetic nanoparticles absorb energy from the AMF, converting it into heat, which elevates the temperature of the surrounding tissues. This rise in temperature can lead to apoptosis or necrosis in cancer cells. Hyperthermia therapy using magnetic nanoparticles offers a targeted and minimally invasive approach for cancer treatment (Jose et al., 2020).
Antimicrobial Activity: Nanoparticles possess intrinsic antimicrobial characteristics stemming from their diminutive size and expansive surface area, fostering heightened interactions with microbial cells (Bains et al., 2020). The mechanism of action involves physical disruption of microbial membranes, generation of reactive oxygen species (ROS), inhibition of enzyme activity, or disruption of microbial biofilms. Nanoparticles can be used as antimicrobial agents in wound dressings, coatings for medical devices, and disinfectants for water purification and surface sterilization (Sayed et al., 2022).
These are just a few examples of the diverse mechanisms of action of nanoparticles in biomedical applications. As research in nanotechnology continues to advance, novel nanoparticles with tailored properties and functionalities will likely be developed, expanding the scope of their applications in medicine and beyond.
4 Human clinical trials and its importance
Human clinical trials involve crucial examinations that test the efficacy, security, efficiency, and practicability of innovative medical interventions, therapies, or procedures in human beings (Blivet et al., 2024). These trials are essential steps in the translational research process, bridging the gap between preclinical studies conducted in laboratory settings and the application of medical innovations in real-world clinical practice (Sujitha et al., 2023). The importance of human clinical trials cannot be overstated, and here are several key reasons why they are crucial:
Assessment of Safety: Human clinical trials provide a platform for assessing the safety of new medical interventions in humans. While preclinical studies in animal models can provide valuable insights into potential toxicity and adverse effects, human trials are necessary to evaluate how interventions interact with the complex biological systems of human subjects. By systematically monitoring participants for adverse events and side effects, clinical trials help ensure that new treatments are safe for human use (Subbiah, 2023).
Evaluation of Efficacy: Clinical trials are designed to determine the efficacy of novel medical therapies in achieving their intended therapeutic outcomes. This involves assessing whether the intervention produces the desired effects in human subjects, such as reducing symptoms, improving health outcomes, or prolonging survival. By employing rigorous study designs and statistical analyses, clinical trials provide evidence-based data on the effectiveness of new treatments, helping healthcare professionals and regulatory organizations make educated judgments about its use in clinical settings (Fountzilas et al., 2022).
Validation of Hypotheses: Clinical trials enable researchers to test hypotheses generated from preclinical studies or observational data in real-world human populations. By designing controlled experiments with carefully defined endpoints and inclusion criteria, clinical trials offer impartial evidence to either validate or refute hypotheses regarding the effectiveness and safety of emerging medical interventions. This process of hypothesis testing is essential for advancing scientific knowledge and informing clinical decision-making (Aday et al., 2022).
Optimization of Treatment Protocols: Clinical trials are vital in optimizing treatment methods by identifying the most effective dosages, treatment regimens, and patient selection criteria for new interventions. Through randomized controlled trials (RCTs) and other study designs, researchers can compare different treatment strategies head-to-head and determine which approach yields the best outcomes for patients. This iterative process of refinement helps refine clinical practice guidelines and improve patient care (Pivonello et al., 2020).
Regulatory Approval: Information from clinical trials is frequently necessary for regulatory clearance of novel medical interventions by governmental authorities such as the Food and Drug Administration (FDA) in the United States and the European Medicines Agency (EMA) in Europe. These agencies review clinical trial outcomes to evaluate the safety, effectiveness, and standards of novel medicines before approving their commercialization and adoption in clinical settings. Without robust clinical trial data, regulatory agencies cannot ensure the safety and effectiveness of new medical products, potentially putting patients at risk (Meyer et al., 2020).
Informed Decision-Making: Clinical trials provide patients and healthcare providers with valuable information to make educated choices regarding treatment alternatives. Clinical trials provide patients with access to cutting-edge medical interventions that may offer potential benefits not available through standard treatments. Moreover, findings from clinical trials aid clinicians in balancing the advantages and drawbacks of various treatment choices, allowing them to customize treatment strategies according to each patient’s unique needs, preferences, and values (Walton et al., 2020).
Contribution to Scientific Knowledge: Clinical trial data contribute to the collective body of scientific knowledge and drive advancements in medical research and innovation. By disseminating trial results through peer-reviewed publications and scientific conferences, researchers can share their findings with the broader scientific community, stimulate further research, and inspire new discoveries. This iterative process of knowledge generation and dissemination is fundamental to the progress of medicine and the improvement of patient outcomes over time (Pitzalis et al., 2020).
Human clinical trials are pivotal in the progression of medical science, enhancing patient care, and guaranteeing the safety and effectiveness of novel medical interventions. By systematically evaluating treatments in real-world human populations, clinical trials provide valuable evidence to inform clinical practice, regulatory decisions, and scientific advancements. Their importance cannot be overstated, as they serve as the cornerstone of evidence-based medicine and the foundation upon which medical progress is built (Kelly et al., 2020).
5 Clinical applications of human clinical trials completed advanced nanoparticles
Human clinical trials of advanced nanoparticles are a significant frontier in medical research, providing diverse solutions across various healthcare fields (Figure 1). Engineered at the nanoscale, these particles possess unique characteristics conducive to precise medical interventions. Their small size facilitates efficient systemic circulation and penetration into cellular and subcellular compartments. Additionally, nanoparticles offer versatile platforms for therapeutic interventions, spanning from cancer treatment to regenerative medicine. Their capacity to modulate cellular behavior, deliver therapeutic payloads, and interact at the molecular level highlights their potential in personalized medicine (Pandey et al., 2024). As human clinical trials continue to uncover the breadth of nanoparticle applications, the convergence of nanotechnology and medicine holds promise for groundbreaking advancements in patient care, ushering in an era of precision healthcare (Amjad et al., 2024). This section discusses several clinical applications of advanced nanoparticles explored in human clinical trials.
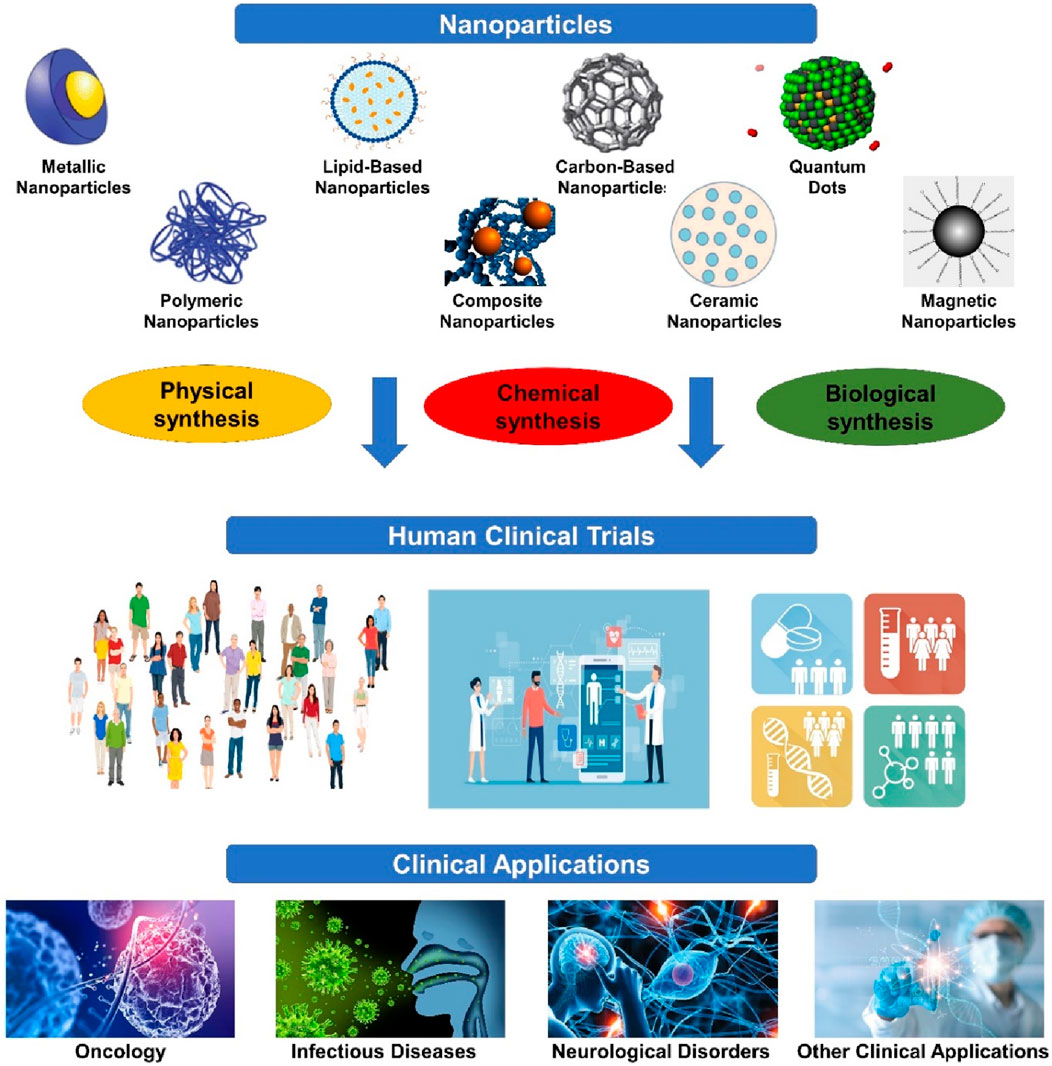
Figure 1. Clinical applications of advanced nanoparticles. It showcases the culmination of human clinical trials employing advanced nanoparticles, highlighting their successful integration into various medical applications. These trials represent significant strides in harnessing nanoparticle technology for improved therapeutic outcomes, diagnostic accuracy, and personalized medicine.
5.1 Oncology
Oncology stands as one of the extensively researched domains in nanoparticle therapeutics. Scientists have tailored nanoparticles to augment the administration of chemotherapy drugs, refine tumor targeting, and surmount multidrug resistance (Wang and Zhang, 2023). Clinical trials have scrutinized different nanoparticle compositions for treating solid tumors, encompassing liposomal doxorubicin for breast cancer, paclitaxel-loaded nanoparticles for lung cancer, and nanoparticle albumin-bound (nab) paclitaxel for pancreatic cancer. These trials have demonstrated significant improvements in drug delivery, tumor response rates, and patient outcomes compared to conventional chemotherapy regimens (Supplementary Table S1). For example, a phase III clinical research found that Liposomal doxorubicin enhanced progression-free survival and reduced cardiotoxicity in individuals who have extensive breast cancer as contrasted with standard doxorubicin (Rashidi et al., 2024). Similarly, nab-paclitaxel demonstrated increased effectiveness and decreased toxicity in patients diagnosed with late-stage pancreatic cancer when compared to typical paclitaxel formulations (Chehelgerdi et al., 2023). In addition to enhancing chemotherapy delivery, nanoparticles have also been explored for targeted therapy and imaging in oncology.
Nanoparticles that are targeted and attached with ligands specific to tumor markers can deliver therapeutic agents exclusively to cancer cells, thus reducing the impact on healthy tissues and minimizing off-target effects. Clinical trials investigating targeted nanoparticles have shown promising results, with improved tumor localization and enhanced therapeutic efficacy observed in patients with various malignancies (Raj et al., 2021).
Furthermore, contrast agents for MRI, CT, and PET have been used with nanoparticles. These nanoparticle-based imaging agents offer superior contrast enhancement, improved tissue penetration, and prolonged circulation time compared to conventional contrast agents (Molkenova et al., 2022). Clinical trials evaluating nanoparticle-based imaging have demonstrated their utility in tumor detection, staging, and monitoring treatment response, facilitating personalized cancer care (Murar et al., 2022). Despite the promising results observed in oncology clinical trials, challenges remain regarding the optimization of nanoparticle formulations, scalability of manufacturing processes, and long-term safety considerations. Further research is needed to address these issues and optimize nanoparticle-based therapies for widespread clinical adoption (Zheng et al., 2021). Expanding on the therapeutic applications of nanoparticles in oncology underscores their versatility in improving cancer diagnosis, treatment, and management across various tumor types. Nanoparticles offer unique advantages in drug delivery, imaging, and combination therapy, enabling targeted and personalized approaches for cancer treatment (Rashidi et al., 2024).
5.2 Drug delivery enhancement
Targeted Drug Delivery: Without causing systemic harm to healthy tissues, nanoparticles can deliver chemotherapeutic drugs, targeted medicines, or nucleic acid-based medications to tumor cells selectively. Surface alterations, like attaching ligands or targeting antibodies, empower nanoparticles to identify and adhere to particular receptors that are overly expressed on cancer cells. This process aids in cellular absorption and the release of drugs within the cells (Ali et al., 2021).
In a phase I trial for bacillus Calmette-Guerin refractory non-muscle invasive bladder cancer, intravenous injection of albumin-bound rapamycin nanoparticles (ABI-009) (ClinicalTrials.gov identifier: NCT02009332) resulted in low local toxicity and no systemic damage. Two patients had a post-therapy cystoscopy with no signs of disease, and thirteen patients completed treatment without the illness advancing. The average drug level in serum was 19.7 ng/mL, indicating tissue penetration. A phase II trial is being planned to learn more about ABI-009s efficacy as a potential targeted therapy for NMIBC (McKiernan et al., 2016).
In a multicenter, single-arm phase II research, the effectiveness and safety of NC-6004, a cisplatin nanoparticle formulation, in combination with gemcitabine were investigated in patients with advanced, incurable bladder, biliary tract, or lung cancer (NCT02240238). Gemcitabine and NC-6004 were administered to 97 patients suffering from diverse cancer types. Depending on the kind of malignancy, median progression-free survival ranged from 3.9 to 6.8 months. Anemia, hyponatremia, nausea, and neutropenia were the most commonly reported Grade 3 Treatment Emergent Adverse Events. Quality of life indicators were generally consistent with what was expected of chemotherapy patients. Overall, the combination therapy had an excellent safety profile and maintained anticancer activity, implying that it might be employed as a therapeutic for a variety of cancers. It is important to look at this combination therapy further (Subbiah et al., 2016).
In a phase 1/2a trial (NCT01380769), CRLX101, a polymer-camptothecin nanopharmaceutical containing cyclodextrin, was evaluated in patients with advanced solid tumors. Weekly doses ranged from 6 to 18 mg/m (2), whereas biweekly doses varied from 12 to 18 mg/m (2). The maximum tolerable dose was 15 mg/m (2) biweekly, with tiredness and neutropenia being major side effects. Myelosuppression was the dose-limiting hazard. The pharmacokinetic analysis demonstrated systemic exposure to both polymer-conjugated and unconjugated CPT. Median progression-free survival was 3.7 months, with stable illness being the most common response. These excellent results support continuing international phase II trials across tumor types (Weiss et al., 2013).
Enhanced Penetration and Retention: Nanoparticles can overcome biological barriers within the tumor microenvironment, including the extracellular matrix (ECM) and tumor vasculature, to penetrate deep into solid tumors (Li J.-X. et al., 2020). Strategies such as size optimization, surface charge modulation, or stimuli-responsive drug release enhance nanoparticle extravasation, accumulation, and retention within the tumor tissue, improving drug efficacy and overcoming multidrug resistance (Xu et al., 2022).
Sande et al. (2018) completed a phase I trial using intraperitoneal aerosolization of albumin-stabilized paclitaxel nanoparticles (Abraxane™) for peritoneal carcinomatosis. The study attempts to improve treatment efficacy in comparison to typical paclitaxel formulations. Patients with a variety of tumors will get pressurized intraperitoneal aerosol chemotherapy (PIPAC) with increasing doses of ABP. The primary focus is on dose-limiting toxicity, with secondary assessments covering surgical complications, pharmacokinetics, quality of life, and biomarkers. This trial, registered under EudraCT: 2017-001688–20 and ClinicalTrials.gov: NCT03304210, intends to discover optimal dosages for future randomized phase II trials, suggesting ABP’s potential efficacy in PIPAC for peritoneal metastases (Sande et al., 2018).
The SWOG S0800 trial (NCT00856492) looked at combining bevacizumab with neoadjuvant nab-paclitaxel, followed by dose-dense doxorubicin and cyclophosphamide in HER2-negative locally progressed or inflammatory breast cancer. Among 215 individuals, bevacizumab significantly raised overall pathologic complete response rates, particularly in triple-negative cases. There were no significant differences in overall or event-free survival, however there was a trend favoring bevacizumab in triple-negative breast cancer event-free survival. Grade 3-4 adverse events were similar between groups. These data highlight the potential benefits of bevacizumab in high-risk triple-negative locally progressed or inflammatory breast cancer, which require further investigation (Nahleh et al., 2016).
5.3 Imaging and diagnosis
Contrast Enhancement: Nanoparticles function as adaptable contrast agents across multiple imaging techniques including MRI, CT, PET, and fluorescence imaging (Hsu et al., 2023). Surface functionalization with imaging probes or targeting ligands enables nanoparticles to selectively accumulate in tumors and provide high-resolution imaging of tumor morphology, vascularity, and metabolic activity (Luo et al., 2021).
Using MRI/ultrasound fusion technology, 3 individuals diagnosed with prostate cancer at low to intermediate risk underwent focal therapy (ClinicalTrials.gov: NCT02680535, NCT04656678). One patient received nanoparticle-directed ablation, while two received cryoablation. The Philips UroNav 4.0 system facilitated precise treatment, reporting 100% ablation of tumors and up to 94% coverage of surrounding margins. The DynaCAD 5.0 Urology system facilitated three-dimensional visualization of vital structures and tumor positions, assisting in preoperative strategizing. This approach shows promise for focal treatment of prostate tumors, offering enhanced precision and minimal invasiveness (Jue et al., 2022).
The Act. In.Sarc study (NCT02379845) evaluated NBTXR3, a radioenhancer, with preoperative radiation therapy (RT) in locally advanced soft tissue sarcoma. NBTXR3 doubled the pathologic complete response rate compared to RT alone (16.1% vs. 7.9%). Safety analysis revealed no significant increase in serious adverse events associated with NBTXR3, and it did not negatively affect health-related quality of life (HRQoL). During follow-up, NBTXR3 plus RT maintained or improved HRQoL scores. Long-term safety results support the favorable benefit-risk ratio of NBTXR3 plus RT, highlighting its potential in locally advanced soft tissue sarcoma treatment (Bonvalot et al., 2022).
The trial (NCT03712423) used PET/CT imaging to assess the tumor absorption of a docetaxel-entrapping polymeric nanoparticle (89Zr-CPC634) in seven solid tumor patients. The patients were given two doses of docetaxel: 60 mg/m2 for therapy and 1–2 mg for diagnostic purposes. The pharmacokinetic analysis revealed that 89Zr-CPC634 had a longer half-life with the on-treatment dose. Tumor accumulation was found in 46% and 41% of lesions, respectively, with comparable median accumulation levels. PET/CT imaging with diagnostic dose accurately reflects on-treatment tumor accumulation, indicating its promise in cancer nanomedicine patient classification (Miedema et al., 2022).
Theranostic Applications: Theranostic nanoparticles combine diagnostic imaging capabilities with therapeutic functionalities, allowing simultaneous visualization of tumors and targeted drug delivery (Hosseini et al., 2023). Multifunctional nanoparticles have the capability to combine imaging agents, therapeutic drugs, and targeting components into one platform, facilitating the real-time monitoring of treatment response and personalized guidance for therapy (Siafaka et al., 2021).
Doswald et al. (2022) conducted a study (NCT04290923) aiming to remove circulating tumor cells (CTCs) using magnetic nanoparticles. Novel carbon-coated cobalt (C/Co) nanoparticles, conjugated with anti-EpCAM antibodies, efficiently eliminated CTCs from healthy blood and achieved ≥68% removal in cancer patients. This suggests potential for a CTC removal device to improve cancer prognosis (Doswald et al., 2022).
In this phase II experiment (NCT00729612), genomic analysis was performed on tumor specimens from patients with non-small cell lung cancer (NSCLC) who underwent carboplatin and nab-paclitaxel. Mutations in DNA repair mechanisms, specifically homologous recombination (HR), were investigated for their prognostic and predictive value. Out of the 63 persons registered, 25 had sufficient DNA samples to sequence. The most frequently modified pathways included DNA repair, JAK-STAT signaling, IGF-1, mTOR, and MAPK-ERK. Patients with HR mutations showed worse progression-free survival (PFS) and overall survival (OS), with hazard ratios of 4.54 (p = 0.026) and 6.3 (p = 0.003), respectively. This suggests that HR pathway mutations may serve as predictors of inferior outcomes in NSCLC patients receiving carboplatin and nab-paclitaxel, underscoring the significance of molecular markers in treatment decision-making. Further validation in larger datasets is recommended, especially in patients receiving combinations of immunotherapy and platinum-based chemotherapy (Owen et al., 2019).
In the Phase II trial (NCT00356811), lapatinib in combination with nab-paclitaxel was tested in HER2-overexpressing metastatic breast cancer (MBC) patients who had previously received one chemotherapy regimen. The primary outcome was the overall response rate (ORR), with secondary endpoints being progression-free survival (PFS), overall survival, duration of response (DoR), time to response (TTR), and time to progression (TTP). The overall response rate was 53%, with partial responses accounting for 47%. The median estimate for PFS, DoR, TTR, and TTP was 39.7, 48.7, 7.8, and 41 weeks, respectively. The combination showed acceptable side effects and a promising ORR, indicating its potential for HER2-positive MBC patients (Yardley et al., 2013).
5.4 Combination therapy
Synergistic Drug Delivery: Nanoparticles enable combination therapy by co-delivering multiple therapeutic agents with complementary mechanisms of action, such as chemotherapy with immunotherapy or targeted therapy. Synergistic interactions between nanoparticle-loaded drugs can enhance cytotoxicity, overcome drug resistance, and minimize off-target effects, improving overall treatment efficacy (Chen et al., 2023).
In the GeparSepto (GBG 69) study, nab-paclitaxel was compared to solvent-based paclitaxel as neoadjuvant chemotherapy for early-stage breast cancer. Patients were given either nab-paclitaxel (150 mg/m2) or solvent-based paclitaxel (80 mg/m2) across four three-week cycles, followed by epirubicin and cyclophosphamide. Pathological complete response (pCR) rates were significantly higher with nab-paclitaxel (38%), compared to solvent-based paclitaxel (29%). Despite a higher incidence of grade 3-4 anemia and peripheral neuropathy, nab-paclitaxel demonstrated higher pCR rates. This shows that nab-paclitaxel may replace solvent-based paclitaxel as the preferred taxane in neoadjuvant therapy for breast cancer (Trials.gov: NCT01583426) (Untch et al., 2016).
In a neoadjuvant phase II experiment (ClinicalTrials.gov ID NCT00110695), 66 women with locally advanced breast cancer were given nanoparticle albumin-bound paclitaxel followed by 5-fluorouracil/epirubicin/cyclophosphamide (FEC). The primary goal was pathological complete response (pCR) in the breast, which was met in 29% of patients, with HER2+ patients having a greater incidence (58%). Both treatments were well tolerated, with grade 2/3 neuropathy (16%) from albumin-bound paclitaxel and grade 3/4 febrile neutropenia (7%) from FEC. Treatment with albumin-bound paclitaxel for 12 weeks was well tolerated, warranting future evaluation in randomized adjuvant and neoadjuvant trials (Robidoux et al., 2010).
In a phase I research (NCT00736619), patients with stage III-IVB head and neck squamous cell carcinoma were given weekly nab-paclitaxel, cetuximab, and intensity-modulated radiation therapy (IMRT). Nab-paclitaxel was studied at four dose levels, with a maximum tolerated dose (MTD) of 60 mg/m (2) weekly. Neuropathy, dehydration, mucositis, and anemia were all considered Grade 3 adverse effects. At 33 months of median follow-up, the 2-year failure-free survival (FFS) and overall survival (OS) rates were 65% and 91%, respectively. This treatment demonstrated effectiveness and an acceptable safety profile, providing a non-platinum option to IMRT with cetuximab alone for advanced HNSCC patients. Further investigation in phase II trials is required (Fury et al., 2014).
In a phase 2 trial (NCT02562716), preoperative chemotherapy for resectable pancreatic ductal adenocarcinoma (PDA) did not enhance overall survival (OS) when compared to adjuvant studies. 102 individuals were given either mFOLFIRINOX or gemcitabine/nab-paclitaxel. Two-year OS rates were 47% and 48%, respectively, lower than the anticipated 40%. The median survival time with mFOLFIRINOX was 23.2 months, and with gemcitabine/nab-paclitaxel was 23.6 months. Despite safety and high resectability rates, maintaining quality control for resectability criteria remains difficult. Additional research is needed to improve outcomes in resectable PDA (Sohal et al., 2021).
Multimodal Approaches: Nanoparticles enable multimodal combination therapy by integrating different treatment modalities, including treatments like chemotherapy combined with photothermal therapy (PTT) or photodynamic therapy (PDT). Combining nanoparticles with outside triggers, including light or magnetic fields, enhances treatment selectivity, spatiotemporal control, and synergistic tumor ablation while sparing surrounding healthy tissues (Kadkhoda et al., 2022).
The phase I clinical trial (ClinicalTrials.gov Identifier: NCT01300533) investigated BIND-014, an innovative PSMA-targeted nanoparticle carrying docetaxel, among patients diagnosed with advanced solid tumors demonstrated good tolerability, predictable toxicity, and unique pharmacokinetics. Administered every 3 weeks or weekly, BIND-014 showed manageable toxicities like neutropenia, fatigue, and diarrhea. Encapsulated docetaxel exhibited prolonged circulation. Out of 52 individuals suitable for examination, one had a complete response and five had partial responses across multiple tumor types, including PSMA-detectable and -undetectable tumors. The suggested phase II dose is 60 mg/m (2) every 3 weeks or 40 mg/m (2) weekly. This study demonstrates BIND-014s potential as a versatile therapy approach (Von Hoff et al., 2016).
In a phase II trial (NCT01620190), nab-paclitaxel monotherapy was tested in EGFR-mutant metastatic non-small cell lung cancer (NSCLC) following tyrosine kinase therapies. Out of 27 patients, 21 were examined for response, resulting in a 35% verified partial response and a 58% disease control rate. The median progression-free survival was 4.0 months. Common progression sites included CNS. Nab-paclitaxel exhibited modest antitumor activity, offering an option for platinum-ineligible patients. The study underscores the importance of CNS activity in systemic therapies for this group. No new safety concerns arose (Baik et al., 2021).
The PEANUT study investigated pembrolizumab and nab-paclitaxel combination therapy in platinum-treated advanced urothelial carcinoma (UC). 70 patients received the combo as a second or third-line treatment. After a median follow-up of 9.8 months, the median progression-free survival (PFS) was 5.9 months, with a 38.6% verified objective response rate. The combination exhibited a favorable safety profile, with alopecia, neutropenia, and peripheral neuropathy as common adverse events. These interim results suggest potential efficacy and tolerability, warranting further investigation in randomized trials (Giannatempo et al., 2020).
A phase III trial (NCT00046527) compared nanoparticle albumin-bound paclitaxel (ABI-007) to conventional paclitaxel in patients with metastatic breast cancer (MBC). ABI-007 had greater response rates (33% vs. 19%) and a longer time to tumor progression (23.0 vs. 16.9 weeks) than standard paclitaxel. Despite a 49% higher paclitaxel dose, ABI-007 exhibited a lower grade 4 neutropenia incidence (9% vs. 22%). ABI-007 was associated with greater cases of sensory neuropathy (10% vs. 2%), however it is curable. ABI-007 did not elicit hypersensitivity reactions, indicating enhanced efficacy and safety in MBC treatment, perhaps eliminating corticosteroid premedication required for solvent-based taxanes (Gradishar et al., 2005).
The Phase I trial (NCT00748553) looked at azacitidine with nanoparticle albumin-bound paclitaxel (nab-paclitaxel) in advanced solid tumors. Azacitidine’s maximum tolerated dose (MTD) is 75 mg/m2. Clinical responses included complete responses (CR) in refractory DLBCL and ovarian cancer, partial responses (PR) in ovarian and endometrial cancer, stable disease (SD) in lung, sarcoma, and pancreatic cancer, as well as additional responses in breast cancer and CLL/SLL. The combination demonstrated promising tolerance and therapeutic efficacy (Cohen et al., 2017).
5.5 Therapeutic resistance overcoming
Multidrug Resistance Reversal: Nanoparticles can circumvent multidrug resistance mechanisms by encapsulating chemotherapeutic agents within nanoparticle carriers, protecting drugs from efflux transporters and enzymatic degradation. Additionally, nanoparticles can bypass drug-resistant cellular pathways or induce alternative cell death mechanisms, restoring sensitivity to conventional therapies in resistant tumor cells (Liu S. et al., 2021).
In a phase I trial (Clinical trial registration: NCT00825201), intraperitoneal (IP) nab-paclitaxel was evaluated in patients with advanced malignancies primarily localized in the peritoneal cavity. Dose escalation demonstrated a maximum tolerated dose (MTD) of 140 mg/m2. Higher doses were associated with dose-limiting toxicities (DLTs), including neutropenia and stomach discomfort. IP nab-paclitaxel demonstrated a low safety profile, significant pharmacokinetic benefits, and promising therapeutic activity. Among the 27 patients included, eight had progression-free survival of ≥6 months, including one full and one partial response. This study underscores the potential of weekly IP nab-paclitaxel as a viable treatment option (Cristea et al., 2019).
The trial (ClinicalTrials.gov NCT03505528) investigated the safety and efficacy of combining nab-paclitaxel with phenelzine, an LSD1 inhibitor, in patients with metastatic breast cancer (mBC). Patients received nab-paclitaxel weekly for 3 weeks, with a 1-week break in a 28-day cycle, as well as escalating doses of phenelzine. The suggested phase 2 dose was 60 mg of phenelzine per day. Adverse effects were mostly mild to severe, with weariness and dizziness being prevalent. Although all patients had disease progression, some showed anticancer action, with a median progression-free survival of 34 weeks. Notably, LSD1 inhibition reduced mesenchymal markers in circulating tumor cells. The study suggests that the combination therapy is well tolerated and shows promise in mBC treatment (Prasanna et al., 2022).
Immune Modulation: Nanoparticles can modulate the tumor immune microenvironment to overcome immunosuppression and enhance antitumor immune responses. Immunomodulatory nanoparticles, such as immune checkpoint inhibitors or adjuvants, activate immune effector cells and promote tumor antigen presentation, augmenting the efficacy of immunotherapy and reducing tumor recurrence (Li J. et al., 2020).
In a phase 2 trial (NCT01812746), 42 chemotherapy-naive metastatic castration-resistant prostate cancer patients were given BIND-014, a docetaxel nanoparticle that targets prostate-specific membrane antigen (PSMA). Intravenous BIND-014 resulted in a PSA response of 30%, a clinical response of 32%, and a 50% conversion of circulating tumor cells (CTCs). The median radiographic progression-free survival was 9.9 months. The majority of adverse events were mild to moderate. A decrease in PSMA-positive CTCs after therapy suggests potential patient selection (Autio et al., 2018).
A phase I trial (NCT01493310) looked examined the combination of mifepristone and nanoparticle albumin-bound paclitaxel (nab-paclitaxel) in patients with advanced breast cancer, with a focus on inhibiting the glucocorticoid receptor. Nine individuals were included, and their serum cortisol levels increased following mifepristone therapy, indicating successful GR inhibition. Neutropenia was controlled with dose modifications. Pharmacokinetic results revealed a possible interaction between nab-paclitaxel and mifepristone in some people. Two patients experienced complete responses, three had partial responses, and one had persistent disease. Immunohistochemical staining revealed GR positivity in six out of nine tumors, all of which were triple-negative upon recurrence. The combination of GR inhibition and chemotherapy demonstrated promising activity alongside manageable toxicity. Based on these results, a randomized phase 2 trial of nab-paclitaxel with or without mifepristone in GR-positive advanced triple-negative breast cancer is scheduled (Nanda et al., 2016).
5.6 Minimally invasive interventions
Image-Guided Therapy: Nanoparticles enable image-guided minimally invasive interventions, such as image-guided surgery or image-guided ablation therapy. Functionalized nanoparticles can accumulate selectively in tumor tissues, providing intraoperative guidance and real-time visualization of tumor margins, residual disease, and metastatic lesions, facilitating precise tumor resection or localized therapy delivery (Bortot et al., 2023).
In 2022, Farag and Hassabou registered a clinical trial (ID: NCT 04907422) examining the potential of a CD24-gold nanocomposite which serves as a biomarker to identify cancer stem cells within salivary gland tumors. Cancer stem cells are essential in tumor initiation, progression, and resistance to therapy. The study included 60 cases, comprising various tumor types and controls. Gold nanoparticles conjugated with CD24 primer formed the nanocomposite. Statistical analyses correlated biomarker expression with clinicopathological features. Diagnostic and prognostic validations were conducted using ROC and Kaplan-Meier curves. Results demonstrated the potential of the CD24-gold nanocomposite as a sensitive biomarker holds significant promise for diagnosing salivary gland tumors and predicting prognosis showcasing the potential of nanotechnology-based biomarkers in cancer management (Farag and Hassabou, 2022).
Nanoparticles offer multifaceted solutions for overcoming challenges in cancer diagnosis, treatment, and management. Using the unique features of nanoparticles for targeted medication administration, imaging, combination therapy, and immune regulation, oncology researchers can develop innovative strategies to improve patient outcomes, enhance therapeutic efficacy, and personalize cancer care. Continued research efforts are needed to optimize nanoparticle formulations, evaluate their safety profiles, and translate these advancements into clinical practice, ultimately advancing the field of oncology and benefiting cancer patients worldwide (Yu et al., 2021).
5.7 Infectious diseases
Nanoparticles have demonstrated promise in combating infectious diseases, including bacterial, viral, and fungal infections. By leveraging their unique properties, nanoparticles can enhance the delivery of antimicrobial agents, overcome microbial resistance mechanisms, and improve therapeutic outcomes. Clinical trials exploring nanoparticle-based treatments for infectious diseases have emphasized notable enhancements in drug efficacy, decreased systemic toxicity, and improved patient outcomes when contrasted with traditional therapies (Table 1). As an illustration, in a phase III clinical trial comparing liposomal doxorubicin to conventional doxorubicin in patients with metastatic breast cancer, the liposomal formulation demonstrated higher response rates and reduced cardiotoxicity. Similarly, nab-paclitaxel demonstrated enhanced survival outcomes and decreased neuropathy in comparison to conventional paclitaxel among patients with metastatic pancreatic cancer (Jangjou et al., 2022). Beyond chemotherapy, nanoparticles have been investigated for alternative modalities in oncology, including photothermal therapy (PTT) and photodynamic therapy (PDT). For instance, gold nanoparticles have been employed as photothermal agents, selectively heating and eliminating tumor cells when exposed to near-infrared (NIR) light. Clinical trials assessing the safety and effectiveness of gold nanoparticle-based PTT in patients with different solid tumors have yielded encouraging outcomes, demonstrating tumor regression and minimal adverse effects (Koc et al., 2024). Despite these advancements, challenges remain in optimizing nanoparticle-based therapies for oncology, including issues related to nanoparticle stability, scalability, and heterogeneity in patient responses. Moreover, the development of resistance mechanisms and the potential for off-target effects necessitate ongoing research efforts to refine nanoparticle formulations and treatment strategies (Mirza and Karim, 2021).
Nanoparticles have also shown promise in combating infectious diseases, including viral infections and bacterial pathogens. In the context of viral infections such as HIV and hepatitis B, nanoparticles are delved as carriers for delivering antiviral agents and as immunomodulatory agents to enhance host immune responses. Clinical trials assessing the efficacy of nanoparticle-based antiviral therapies have demonstrated improvements in viral suppression and immune function, albeit with variable outcomes across different patient populations (Liu H. et al., 2021). Furthermore, nanoparticles have been investigated for delivering antimicrobial agents to address drug-resistant bacterial infections. Formulations of nanoparticles have been developed to improve the stability and availability of antibiotics, enabling precise delivery to infection sites while reducing systemic toxicity. Clinical trials investigating nanoparticle-based antibiotic therapies have shown promising results in terms of improved microbial eradication and reduced antibiotic resistance development (Makabenta et al., 2021). Expanding on the therapeutic applications of nanoparticles in infectious diseases highlights their potential in combating viral, bacterial, and fungal pathogens. Nanoparticles offer unique advantages in drug delivery, immunomodulation, and antimicrobial activity, potential contenders for the creation of innovative antiviral solutions and antibacterial therapies (Chand and Kushawaha, 2023).
5.7.1 Antiviral therapy
Drug Delivery: Nanoparticles have the capacity to act as carriers for antiviral medications, enhancing their stability, bioavailability, and targeted delivery to infected cells or tissues. Functionalized nanoparticles can bypass biological barriers, such as the mucosal epithelium or BBB, and deliver therapeutic payloads directly to viral reservoirs, reducing viral replication and spread (Delshadi et al., 2021).
The study (ClinicalTrials.gov number: NCT01709019) aimed to evaluate the safety and immune response of a new recombinant RSV F nanoparticle vaccine in older adults, four formulations were given simultaneously with a licensed influenza vaccine. The trial involved 220 healthy adults aged ≥60 years. All vaccine formulations exhibited acceptable safety profiles without serious adverse events. The immune responses triggered by the vaccine were swift, reaching peak antibody levels within either 28 or 56 days after vaccination. Significant increases in anti-F protein IgG antibody levels were observed, particularly with adjuvanted formulations, and this immune response endured for 12 months following vaccination. Neutralizing antibody levels increased post-vaccination, and the vaccine did not interfere with influenza vaccine responses. Overall, the RSV F nanoparticle vaccine demonstrated promising immunogenicity and safety, warranting further investigation in older adults (Fries et al., 2017).
Immunomodulation: Nanoparticles can modulate host immune responses to enhance antiviral defense mechanisms. Immunostimulatory nanoparticles, such as virus-like particles (VLPs) or Toll-like receptor (TLR) agonists, can activate innate immune pathways and stimulate antiviral cytokine production, promoting viral clearance and adaptive immune responses (Perciani et al., 2020).
In a phase 1 clinical trial (registered as NCT04935801), a synthetic nanoparticle-based peptide vaccine targeting dengue virus was tested for safety and immunogenicity in Switzerland. Participants received either PepGNP-Dengue or a control (vehicle-GNP) via intradermal microneedle injection. There were no serious adverse events attributed to the vaccine, and only mild adverse events were noted. PepGNP-Dengue led to notable increases in specific CD8+ T cells, particularly in the low-dose group. This study demonstrates the safety and potential efficacy of PepGNP-Dengue in stimulating virus-specific CD8+ T cells, supporting further development of this nanoparticle-based vaccine for dengue (Miauton et al., 2024).
Arunachalam et al. (2021) study a subunit COVID-19 vaccination that employs the SARS-CoV-2 spike protein receptor-binding domain on an I53-50 protein nanoparticle scaffold (RBD-NP). The vaccine, which was improved with numerous compounds including AS03 and CpG1018-alum, generated robust neutralizing antibody responses and provided protection against SARS-CoV-2 infection in rhesus macaques for up to 180 days. While it was efficient against the B.1.1.7 variety, its efficiency against the B.1.351 variant varied according to the adjuvants used. RBD–NP-AS03 demonstrated comparable immunogenicity to HexaPro with AS03. These findings support further clinical trials (NCT04742738 and NCT04750343) for assessing vaccine efficacy in humans (Arunachalam et al., 2021).
In a phase 3 controlled experiment (NCT04120194), 2654 older persons were recruited and divided into two groups: one received a Matrix-M-adjuvanted quadrivalent nanoparticle influenza vaccine (qNIV), while the other received a licensed quadrivalent inactivated influenza vaccine (IIV4). qNIV has been shown to be immunologically comparable to IIV4, with enhanced humoral and cellular immune responses. The geometric mean titers and seroconversion rates for vaccine-homologous influenza strains were comparable amongst the groups. Adverse events were more common in the qNIV group, particularly mild to moderate transitory injection site pain. Overall, qNIV was well tolerated and displayed potential in enhancing seasonal influenza vaccination efficacy in older adults. Subsequent studies to establish clinical efficacy are planned based on these results (Shinde et al., 2022).
Keech et al. (2020) evaluated NVX-CoV2373, a recombinant SARS-CoV-2 nanoparticle vaccine, in a randomized, placebo-controlled phase 1-2 trial with 131 healthy people. The vaccine, administered in 5-μg and 25-μg doses with or without Matrix-M1 adjuvant, is both safe and highly immunogenic. The reactogenicity is modest, with no serious adverse events documented. The addition of adjuvant enhances immune responses, with the 5-μg adjuvanted regimen showing particularly robust IgG and neutralization responses surpassing convalescent serum levels. NVX-CoV2373, deemed safe and effective, holds promise for combating COVID-19. ClinicalTrials.gov identifier: NCT04368988 (Keech et al., 2020).
Viral Entry Inhibition: Nanoparticles can interfere with viral attachment, fusion, or entry into host cells by presenting viral receptor mimics, blocking viral glycoproteins, or sequestering viral particles. Multivalent nanoparticles decorated with viral receptor ligands or glycan moieties can competitively hinder viral binding to host cell receptors, thereby preventing viral entry and subsequent infection (Sarkar et al., 2022).
Shinde et al. (2018) compared adjuvanted recombinant hemagglutinin trivalent nanoparticle influenza vaccine (tNIV) to high-dose Fluzone vaccine (IIV3-HD) in 330 people aged 60 and up. Matrix-M adjuvanted tNIV elicited considerably greater hemagglutination inhibition (HAI) antibody responses to A (H3N2) strains, including antigenically varied variants. Short-term reactogenicity and safety profiles were comparable between tNIV and IIV3-HD. tNIV-induced HAI responses were 47%–64% stronger than IIV3-HD against A (H3N2) strains, including a variety selected for the forthcoming influenza season (Shinde et al., 2018).
In this sub-study of a phase 3 trial (NCT04583995), researchers examined NVX-CoV2373 safety, immunogenicity, and efficacy when given with seasonal influenza vaccines. Participants received NVX-CoV2373 or placebo, with or without influenza vaccination. Co-administration resulted in more reactogenicity events, but adverse events were mild and evenly distributed. It didn't affect influenza vaccine response but reduced NVX-CoV2373 antibody responses. NVX-CoV2373 efficacy was 87.5%, consistent with the main study. Simultaneous COVID-19 and influenza vaccination appears feasible (Toback et al., 2022).
Clinical trial registration: NCT01704365. A randomized, blinded, and controlled trial looked at a respiratory syncytial virus (RSV) recombinant fusion (F) nanoparticle vaccination in healthy women of reproductive age. Three hundred thirty participants received 1 or 2 doses of the vaccine at different doses and with or without an adjuvant, or placebo. Safety was monitored for 180 days, while immunogenicity and RSV infection rates were assessed for 112 days. All variations of the vaccine were well received, showing, there were no significant adverse effects associated to the vaccination. Antibody levels increased significantly, particularly with the 2-dose, adjuvanted regimens. Notably, vaccine recipients showed a reduction in RSV infections compared to placebo recipients. These data demonstrate that the vaccination is safe, immunogenic, and effective in lowering RSV infections, supporting further development for maternal immunization (Glenn et al., 2016).
The trial (NCT02624947) investigated the efficacy as well as the effectiveness of a respiratory syncytial virus (RSV) fusion (F) protein nanoparticle vaccination administered to healthy pregnant women in late pregnancy. Infants born to immunized mothers were monitored for lower respiratory tract infection outcomes for 180 days and safety for 364 days. The primary focus was on RSV-associated, medically important lower respiratory tract infections in infants aged up to 90 days. Vaccine efficacy against this endpoint was 39.4%, with similar trends noted for severe hypoxemia and hospitalizations due to RSV-associated lower respiratory tract infections. The immunization resulted in more local injection site responses, but other adverse events were comparable between groups. Although the vaccine did not meet the predefined efficacy criterion, potential benefits in other RSV-associated respiratory disease outcomes warrant further exploration (Madhi et al., 2020).
RNA Interference (RNAi): Nanoparticles can deliver small interfering RNA (siRNA) or microRNA (miRNA) molecules to silence viral gene expression or disrupt viral replication. RNAi-based nanoparticles can target conserved viral sequences or essential viral genes, providing a broad-spectrum antiviral strategy against RNA and DNA viruses (Kang et al., 2023).
The PREVENT-19 study (registered as NCT04611802) investigated the safety, immune response, and effectiveness of the NVX-CoV2373 vaccine in adolescents aged 12 to under 18 years. The current study was an extension of a phase 3 randomized, observer-blinded, placebo-controlled examination carried out in the United States. Individuals received two doses of NVX-CoV2373 or placebo, 21 days intervals. Among 2,247 enrolled participants, NVX-CoV2373 demonstrated a 1.5-fold increase in neutralizing antibody titers compared to young adults. The effectiveness of the vaccine against laboratory-confirmed COVID-19 was 79.5%, with all identified viral genomes being of the Delta variant. Reactogenicity, predominantly mild-to-moderate, was more common following the subsequent dose but transient. Significant negative consequences were occurred infrequent and evenly distributed across both treatment groups. In conclusion, the study affirms that NVX-CoV2373 was safe, elicited an immune response, and effectively prevented COVID-19, including Delta variant cases, in adolescents (Áñez et al., 2022).
ChulaCov19 vaccine (ClinicalTrials.gov Identifier: NCT04566276), which encodes an mRNA of the prefusion non-stabilized SARS-CoV-2 spike protein, exhibited safety and elicited an immune response in a phase I trial. It can be stored at temperatures ranging from 2°C to 8°C for a duration of up to 3 months, it offers logistical advantages. In a dose-escalation study involving 72 volunteers (ages 18–75), all doses (10, 25, and 50 μg) were well tolerated and induced robust B- and T-cell responses. Transient mild to moderate adverse reactions were observed, with a higher frequency noted after the administration of the second dose. At 50 μg, vaccine showed higher neutralizing antibodies than convalescent sera against wild-type SARS-CoV-2, with cross-neutralization against Alpha, Beta, Gamma, and Delta variants. All doses achieved 100% seroconversion with strong T-cell responses. ChulaCov19 has advanced to phase 2 trials, indicating promising safety and immunogenicity profiles (Gatechompol et al., 2022).
5.7.2 Antibacterial therapy
Targeted Drug Delivery: Nanoparticles can encapsulate antibiotics or antimicrobial agents and deliver them selectively to bacterial infection sites, reducing systemic exposure and minimizing off-target effects. Surface-modified nanoparticles can evade host immune defenses and penetrate bacterial biofilms, enhancing drug accumulation and efficacy against multidrug-resistant bacteria (Makabenta et al., 2021).
Arafa et al., 's 2023 study, registered under Clinical trial ID NCT05475444 on ClinicalTrials.gov, investigated PLGA nanoparticles coated with chitosan in a randomized clinical trial aimed at treating endodontic infections. Incorporated into a thermosensitive gel with Ciprofloxacin hydrochloride (CIP), the formulation displayed favorable rheology and sustained drug release. Clinical efficacy trials demonstrated significant reduction in Enterococcus faecalis infection with CIP-CS-PLGA-NPs gel (F1), surpassing free CIP and Ca(OH)2 pastes. F1 exhibited superior bacterial reduction and biofilm inhibition, indicating effective delivery deep into the root canal, effectively combating antibiotic-resistant endodontic infections. This study underscores the potential of nanoparticle-based therapies in clinical practice (Arafa et al., 2023).
Elabd et al. (2024) conducted a randomized controlled clinical trial, registered under ID NCT06051487 at ClinicalTrials.gov. The project entailed incorporating titanium dioxide nanoparticles onto acrylic baseplates for orthodontic equipment. Twenty-six patients were separated into two groups, with one group receiving equipment with 1% titanium dioxide nanoparticles. Swab tests demonstrated lower bacterial colony numbers in the nanoparticle group compared to controls, especially after four to 6 months. The addition of nanoparticles notably diminished bacterial colonization under the baseplates, indicating the potential to enhance orthodontic appliance hygiene and lower the risk of bacterial infections during treatment (Elabd et al., 2024).
Synergy with Conventional Antibiotics: Nanoparticles can synergize with conventional antibiotics to overcome drug resistance mechanisms and enhance antimicrobial activity. Nanoparticle-mediated delivery of antibiotics can potentiate their intracellular uptake, overcome efflux pump resistance, or disrupt bacterial membrane integrity, increasing bacterial susceptibility to antibiotic treatment (Adeniji et al., 2022).
Elsawy et al. conducted a clinical trial at Assiut University in 2021, following IRB clearance (13,700,424) and registration (NCT04431440). The study aims to determine the efficacy of silver nanoparticles against vancomycin-resistant S. aureus (VRSA) in critically ill patients. Among 150 samples, 55.3% contained methicillin-resistant Staphylococcus aureus (MRSA), and 12.67% contained VRSA. Silver nanoparticles showed bactericidal activity against MRSA and VRSA, indicating their potential as a treatment for VRSA infections (Elsawy et al., 2021).
In 2010, Beyth et al. developed a method approved by the Helsinki Committee for Human Clinical Trials (Identifier: NCT00299598) to insert cross-linked quaternary ammonium polyethylenimine (QPEI) nanoparticles into dental resin composite, demonstrating long-lasting antibacterial characteristics. The incorporation of polyethyleneimine nanoparticles into resin composites causes cell death and biofilm stress in vivo. Volunteers wearing acrylic appliances with control or QPEI-incorporated specimens showed over 50% reduction in bacterial vitality and increased biofilm thickness. Laboratory experiments validated a 70% reduction in viable bacteria. Examination under a scanning electron microscope unveiled distinct morphological changes. Low concentrations of QPEI nanoparticles demonstrated notable antibiofilm effects in live settings and displayed wide-ranging antibacterial effectiveness against salivary bacteria, indicating their potential for dental applications (Beyth et al., 2010).
Photodynamic Therapy (PDT): Nanoparticles can serve as photosensitizers in PDT to generate ROS upon light activation, inducing bacterial cell death and biofilm disruption. PDT using nanoparticles offers a non-invasive and targeted approach for treating localized bacterial infections, with potential applications in wound healing, dental caries, and chronic infections (Xie et al., 2021).
5.7.3 Antifungal therapy
Nanoparticles can encapsulate antifungal drugs and enhance their solubility, stability, and bioavailability, overcoming limitations associated with conventional formulations. Lipid-based nanoparticles, polymeric nanoparticles, and lipid-polymer hybrid nanoparticles have been explored for the delivery of azole drugs, polyenes, and echinocandins, improving their efficacy against fungal pathogens (Nami et al., 2021). Nanoparticles can penetrate fungal biofilms and deliver antifungal agents directly to microbial communities, disrupting biofilm architecture and enhancing drug penetration into deeper layers. Surface-modified nanoparticles can selectively bind to fungal cell wall components or extracellular matrix proteins, improving drug retention and biofilm eradication (Sousa et al., 2020). Nanoparticles can facilitate combination therapy by co-delivering multiple antifungal agents or adjuvants with complementary mechanisms of action. Synergistic interactions between nanoparticles and antifungal drugs can overcome drug resistance, reduce drug dosages, and enhance therapeutic outcomes against drug-resistant fungal infections (Zheng and McClements, 2020).
In 2024, Gamil et al. undertook a research endeavor to assess the efficacy of miconazole and miconazole-loaded chitosan nanoparticles in managing oral candidiasis in individuals with diabetes. The randomized controlled trial included 80 participants treated for 28 days. Clinical evaluations and microbiological analyses were performed throughout. Both treatments exhibited similar efficacy in symptom control and Candida reduction, with significant improvement observed in the nanoparticle group. Reduction in Candida albicans colonies was particularly noteworthy in the nanoparticle-treated group. The trial confirmed the efficacy of both treatments without adverse effects. This research offers valuable insights into alternative therapies for oral candidiasis in diabetic individuals. The trial was registered under NCT06072716 on 10/10/2023 (Gamil et al., 2024).
Overall, nanoparticles hold tremendous potential in the fight against infectious diseases by offering innovative approaches for antiviral, antibacterial, and antifungal therapy. Continued research efforts are needed to optimize nanoparticle formulations, evaluate their safety profiles, and translate these strategies into clinical practice, ultimately improving patient outcomes and combating the global burden of infectious diseases (Kirtane et al., 2021).
5.8 Neurology
Nanoparticles present promising avenues in neurology for addressing diverse conditions like neurodegenerative disorders, brain tumors, and neurological injuries. By traversing the BBB, nanoparticles enable targeted delivery of therapeutic compounds to the central nervous system, surmounting a significant challenge in neurological drug administration. Ongoing clinical investigations are delving into nanoparticle-based treatments for neurodegenerative ailments such as Alzheimer’s and Parkinson’s diseases have demonstrated mixed results, with some showing improvements in disease progression or symptom management, while others have faced challenges related to nanoparticle penetration into target brain regions and potential neurotoxicity. Despite these complexities, ongoing research aims to optimize nanoparticle formulations and delivery strategies for enhanced efficacy and safety in neurology (Mukherjee et al., 2020).
Expanding on the therapeutic applications of nanoparticles in neurology reveals a promising avenue for addressing various neurodegenerative disorders, brain tumors, and neurological injuries. Despite the challenges posed by the blood-brain barrier (BBB), which restricts the delivery of therapeutic agents to the central nervous system (CNS), nanoparticles offer unique opportunities to overcome these barriers and deliver drugs, imaging agents, or therapeutic payloads directly to target sites within the brain (Smith et al., 2019).
5.8.1 Neurodegenerative disorders
Neurodegenerative disorders, such as Alzheimer’s disease (AD), Parkinson’s disease (PD), and amyotrophic lateral sclerosis (ALS), are distinguished by increasing neuronal loss and reduced function. Nanoparticles offer a promising avenue for transporting therapeutic substances, encompassing small molecules, peptides, proteins, and nucleic acids, to address specific pathological features like amyloid-beta plaques and tau tangles in AD, or alpha-synuclein aggregates in PD. Functionalized nanoparticles can bypass the BBB and selectively accumulate in affected brain regions, offering a targeted approach for disease modification or symptom management. Moreover, nanoparticles can serve as carriers for neuroprotective agents, antioxidant compounds, or anti-inflammatory drugs to mitigate neuroinflammation and oxidative stress, which are implicated in the pathogenesis of neurodegenerative disorders (Padmanabhan et al., 2020).
The research conducted by Vucic et al. (2023) explored the therapeutic potential of CNM-Au8, a catalytically-active gold nanocrystal with neuroprotective properties, in the context of ALS. Spanning 36 weeks and involving 45 participants, the trial (registered under NCT04098406 on clinicaltrials.gov) evaluated changes in motor unit number index (MUNIX) and forced vital capacity (FVC) as primary and secondary endpoints, respectively. Although no significant differences were observed in these metrics between the CNM-Au8 and placebo groups, long-term survival analysis revealed a noteworthy 60% decrease in all-cause mortality among those treated with CNM-Au8. Moreover, participants initially assigned to CNM-Au8 showed slower disease progression during the open-label extension phase. Despite the absence of significant changes in primary and secondary outcomes, the study affirmed the safety of CNM-Au8 in combination with riluzole, suggesting further investigation is warranted (Vucic et al., 2023).
5.8.2 Brain tumors
Nanoparticles have surfaced as encouraging instruments for both diagnosing and treating primary and metastatic brain tumors, encompassing gliomas, meningiomas, and brain metastases. Functionalized nanoparticles can selectively target tumor cells while sparing normal brain tissue, enhancing the efficacy of chemotherapy, radiotherapy, or photothermal therapy (PTT). Additionally, nanoparticles can be engineered to deliver imaging contrast agents for non-invasive detection and monitoring of tumor growth, infiltration, and response to therapy. Strategies such as magnetic targeting, ligand-mediated targeting, or stimuli-responsive drug release facilitate accurate transport of therapeutic substances to tumor locations, enhancing treatment effectiveness and reducing unintended impacts (Skandalakis et al., 2020).
In a phase 0 clinical trial (registered under NCT03020017), individuals with recurrent glioblastoma (GBM) received intravenous administration of spherical nucleic acids (SNAs) based on RNA interference, termed NU-0129, targeting the GBM-associated oncogene Bcl2Like12 (Bcl2L12). Safety evaluation revealed no instances of severe (grade 4 or 5) treatment-related adverse events. SNAs successfully reached tumor sites in patients, as indicated by the presence of gold enrichment in cells associated with tumors. The uptake of NU-0129 correlated with a decrease in the expression of Bcl2L12 protein associated with tumors. These results suggest that SNAs hold promise as a potential precision medicine for systemic GBM therapy, highlighting their ability to penetrate the brain and target intracranial tumor sites, overcoming challenges posed by the blood-brain barrier and limited access to therapeutic agents in GBM treatment (Kumthekar et al., 2021).
The Act. In.Sarc trial (NCT02379845) assessed NBTXR3, a nanoparticle composed of hafnium oxide, was investigated in a study comparing its combination with radiotherapy against radiotherapy alone in individuals with locally advanced soft-tissue sarcoma. Among 176 patients, the NBTXR3 group showed a higher rate of pathological complete response (16% vs. 8%) with manageable adverse events, primarily postoperative wound complications. NBTXR3-related adverse events included injection site pain and hypotension. The study validates NBTXR3’s efficacy as a radioenhancer in soft-tissue sarcoma treatment, suggesting potential broader applications in cancer therapy. The safety and effectiveness of NBTXR3 were validated in this phase 2-3 trial, paving the way for further clinical utilization in various cancers (Zhou et al., 2022).
Nanoparticles hold tremendous potential for addressing unmet medical needs in neurology by enabling targeted drug delivery, imaging, and regenerative therapies for neurodegenerative disorders, brain tumors, and neurological injuries. Continued research efforts aimed at optimizing nanoparticle formulations, improving BBB penetration, and enhancing therapeutic efficacy will further advance the field and translate these innovative strategies into clinical practice, ultimately improving patient outcomes and quality of life (Mukherjee et al., 2020).
5.8.3 Neurological injuries and regeneration
Nanoparticles hold promise for promoting tissue repair and regeneration following neurological injuries such as traumatic brain injury (TBI), spinal cord injury (SCI), or stroke. Nanoparticle-based therapies can modulate the inflammatory response, promote neurogenesis and axonal regeneration, and enhance functional recovery through the delivery of growth factors, neurotrophic factors, or stem cells. Encapsulation of therapeutic agents within nanoparticles protects them from enzymatic degradation and enhances their bioavailability at the injury site, facilitating targeted delivery and sustained release of regenerative cues. Furthermore, nanoparticles can serve as carriers for imaging agents to track transplanted cells or monitor tissue repair processes in vivo, enabling real-time assessment of treatment efficacy and patient outcomes (Kumar et al., 2014).
5.9 Other therapeutic applications
Beyond oncology, infectious diseases, and neurology, nanoparticles have been investigated for a wide range of therapeutic applications in areas such as cardiovascular disease, autoimmune disorders, regenerative medicine, and ophthalmology (Yetisgin et al., 2020). Clinical trials have explored nanoparticle-based therapies for drug-eluting stents in coronary artery disease, targeted drug delivery in rheumatoid arthritis, stem cell delivery for tissue regeneration, and intraocular drug delivery for age-related macular degeneration (AMD). These trials have provided valuable insights into the feasibility, safety, and efficacy of nanoparticle-based interventions across diverse medical specialties (Supplementary Table S2). While many trials have shown promising results, challenges persist in scaling up nanoparticle production, ensuring long-term stability, and addressing regulatory hurdles associated with novel therapeutic modalities (Kar et al., 2022).
5.9.1 Cardiovascular disease
Nanoparticles exhibit potential in addressing cardiovascular ailments such as atherosclerosis, myocardial infarction, and restenosis. Utilizing nanoparticle-driven drug delivery systems can enhance the effectiveness of therapeutic medications such as statins, antiplatelet drugs, and anticoagulants by enhancing their stability, targeting specificity, and sustained release kinetics. Moreover, nanoparticles can be customized with targeting ligands to precisely transport medications to atherosclerotic plaques or damaged vascular tissues. This approach reduces off-target effects and systemic toxicity. Clinical trials have investigated the safety and efficacy of nanoparticle-coated stents, drug-eluting nanoparticles, and nanocarriers for imaging and diagnosis of cardiovascular conditions, paving the way for personalized treatment approaches and improved patient outcomes (Pala et al., 2020).
NANOM first-in-man trial (NCT01270139) was conducted by Kharlamov et al., 2017. In the NANOM-FIM trial, plasmonic photothermal therapy using nanoparticles for atherosclerosis showed promising long-term safety outcomes. A 5-year observational analysis compared nano, ferro, and stent groups (n = 180). The nano group exhibited lower mortality, major adverse cardiovascular events, late thrombosis, and target lesion revascularization compared to ferro and stent groups. These discoveries underscore the promise of nanoparticle-based therapy in reducing adverse cardiovascular events and improving long-term outcomes in atherosclerosis treatment. The study suggests nanomedicine as a safe and effective approach for managing atherosclerosis, supporting further exploration in clinical practice (Kharlamov et al., 2015).
In a clinical trial registered under NCT05032937, researchers assessed the effectiveness and safety of ferumoxytol-enhanced coronary magnetic resonance angiography (CMRA) in 30 participants suspected of having coronary artery disease. The study aimed to evaluate diagnostic accuracy and potential adverse effects. Results indicated that ferumoxytol administration produced high-quality images, achieving a segment-based sensitivity of 92.3%, specificity of 96.7%, and accuracy of 96.0% compared to invasive coronary angiography. Notably, no adverse events related to ferumoxytol were reported during the 3-month follow-up period. This study concludes that ferumoxytol-enhanced CMRA exhibits excellent diagnostic performance and safety, offering a non-radiation alternative to coronary computed tomography angiography for detecting significant coronary stenosis in patients suspected of having coronary artery disease (Dong et al., 2023).
5.9.2 Autoimmune disorders
Nanoparticles offer potential in tackling autoimmune conditions such as rheumatoid arthritis, multiple sclerosis, and inflammatory bowel disease by transporting immunomodulatory agents, biologics, or small-molecule inhibitors to target inflammatory pathways or regulate abnormal immune responses. Encapsulating drugs within nanoparticles shields them from enzymatic degradation, extends their circulation time, and enables selective delivery to inflamed tissues or immune cells. Clinical trials have explored nanoparticle-based therapies’ safety and efficacy in modulating immune responses, reducing disease activity, and enhancing clinical outcomes in patients with autoimmune disorders (Yadav et al., 2022).
In the NCT00466960 phase II trial, investigators assessed the effectiveness and immunological reactions of weekly nab-paclitaxel coupled with GM-CSF as an immune modulator in patients with recurrent platinum-resistant ovarian, fallopian tube, or primary peritoneal cancer. Peripheral blood mononuclear cells (PBMC) were tested for immune cell populations and ovarian cancer antigen responses. Twenty-one patients recruited, with a median overall survival time of 16.8 months. The overall response rate was 71%, with 29% complete and 43% incomplete responses. Interferon gamma Elispot responses targeting the insulin-like growth factor 1 receptor (IGF1R) were observed to be associated with progression time. Levels of myeloid-derived suppressor cells (MDSC) at enrollment correlated with achieving a complete response. These findings suggest the regimen’s efficacy and identify potential immune correlates for response, supporting further investigation of immune modulators combined with chemotherapy in platinum-resistant recurrent ovarian cancer (Liao et al., 2015).
Another study, NCT02247726, aimed to assess the safety and immunogenicity of a respiratory syncytial virus (RSV) fusion protein nanoparticle vaccine in healthy third-trimester pregnant women and their newborns. Fifty pregnant women were randomly randomized to have the vaccine or a placebo. The vaccine was well tolerated, with no significant differences observed in pregnancy or neonatal outcomes between the vaccine and placebo groups. RSV-specific antibody levels rose dramatically among vaccine recipients, with some antibodies competing with known neutralizing epitopes. Robust transplacental antibody transfer to infants was noted, and infants of vaccinated women did not exhibit severe RSV disease. These findings support maternal immunization as a strategy to safeguard infants from RSV disease (Muňoz et al., 2019).
Moreover, the study registered as NCT04533399 assessed the safety and immunogenicity of NVX-CoV2373 in both HIV-positive and HIV-negative individuals. This randomized phase 2A/B trial included participants aged 18–84, assigning them to receive NVX-CoV2373 or placebo. Safety analysis encompassed 4164 HIV-negative and 244 HIV-positive participants. While more adverse events occurred in HIV-negative individuals, serious events were unrelated to the vaccine. Among baseline SARS-CoV-2-negative participants, HIV-positive individuals exhibited lower anti-spike IgG levels post-vaccination compared to HIV-negative counterparts. However, those baseline SARS-CoV-2-positive showed robust antibody responses. The vaccine was safe in HIV-positive individuals, but their immune responses were attenuated compared to HIV-negative individuals unless they were baseline SARS-CoV-2-positive (Madhi et al., 2022).
5.9.3 Regenerative medicine
Nanoparticles have emerged as valuable tools in regenerative medicine for tissue engineering, wound healing, and organ transplantation. Nanoparticle-based scaffolds, hydrogels, and matrices can provide structural support, promote cellular adhesion, and deliver bioactive factors to facilitate tissue regeneration and repair. Functionalized nanoparticles can modulate cellular behavior, enhance angiogenesis, and regulate the inflammatory microenvironment to promote tissue healing and regeneration. Clinical trials have investigated the use of nanoparticle-based scaffolds for bone regeneration, cartilage repair, and skin regeneration, demonstrating encouraging results in improving tissue function and promoting wound closure (Fathi-Achachelouei et al., 2019).
The research, registered as NCT03420768, assessed the efficacy and safety of BMS-986263, a lipid nanoparticle carrying short interfering RNA targeting HSP47 mRNA, in patients with severe hepatic fibrosis caused by HCV infection. This Phase 2 trial included HCV-SVR patients with advanced fibrosis who received weekly infusions of either placebo or BMS-986263 (45 or 90 mg doses) for 12 weeks. The primary goal was to advance at least one METAVIR stage by week 12. Secondary outcomes included improved Ishak scores, pharmacokinetics, fibrosis biomarkers, and safety assessments. The results showed improvements in METAVIR and Ishak scores, but no substantial accumulation of BMS-986263 plasma concentrations. Adverse events were primarily mild or moderate infusion-related responses. These findings suggest that BMS-986263 was well-tolerated and improved fibrosis scores in HCV-SVR patients with advanced fibrosis, warranting further investigation in patients with active fibrogenesis (Lawitz et al., 2022).
5.9.4 Ophthalmology
Nanoparticles show great promise in the field of ophthalmology, particularly in the treatment of visual disorders such age-related macular degeneration (AMD), diabetic retinopathy, and glaucoma. Using nanoparticle-driven drug delivery systems can improve the effectiveness and duration of therapeutic substances in ocular tissues, overcoming hurdles such as the blood-retinal barrier and corneal epithelium. These nanoparticles can be engineered into ocular implants, nanoformulations, or suspensions to achieve sustained drug release, precise targeting of specific ocular regions, and improved therapeutic outcomes. Clinical trials have evaluated nanoparticle-based therapies for intraocular drug delivery, gene therapy, and imaging of retinal diseases, demonstrating potential benefits in preserving vision, reducing disease progression, and improving patient quality of life (Cheng et al., 2024).
The research conducted by Jackson and colleagues in 2021, registered under NCT04008771 on ClinicalTrials.gov, delved into the safety assessment of intravitreal quantum dots (QDs) for retinitis pigmentosa (RP). This open-label, fellow eye-controlled trial involved 20 patients who received intravitreal injections of QDs. Notably, no adverse events were linked to QDs. Furthermore, among patients with severe RP, there was an observed improvement in mean visual acuity, shifting from 6/398 to 6/177, suggesting potential therapeutic advantages. However, in end-stage RP, visual acuity remained unchanged. This suggests that intravitreal QDs are safe for RP patients, with promising implications for vision improvement. Additional research is necessary to confirm these observations and fully uncover the therapeutic capabilities of QDs in the treatment of retinitis pigmentosa (Jackson et al., 2021).
Overall, the diverse therapeutic applications of nanoparticles across medical specialties highlight their versatility and potential to address unmet clinical needs. Continued research efforts, multidisciplinary collaborations, and clinical validation are essential for translating nanoparticle-based therapies from preclinical development to clinical practice, ultimately improving patient outcomes and advancing personalized medicine approaches.
6 Current status of nanoparticle-based clinical trials
Nanoparticles have emerged as promising therapeutic platforms in modern medicine, especially for drug delivery, diagnostics, and regenerative medicine. The clinical success of various types of nanoparticles is crucial in determining their practical utility and future development (Table 2) (Huang et al., 2020). Below is a summary of the current clinical trial status, organized by nanoparticle type, based on recent literature and clinical trial databases.
6.1 Lipid-based nanoparticles (LNPs)
Lipid nanoparticles (LNPs), including liposomes and solid lipid nanoparticles, are among the most widely investigated in clinical trials. They have shown considerable success in delivering small molecules and nucleic acids, particularly in cancer therapy and mRNA vaccines. Success Stories: The approval of Comirnaty and Spikevax mRNA vaccines for COVID-19 marks a major milestone for lipid-based nanoparticles. Several cancer treatments based on liposomal formulations like Doxil (liposomal doxorubicin) are FDA-approved and have shown improved efficacy and reduced side effects. Ongoing Trials: ClinicalTrials.gov currently lists over 1,200 active studies related to lipid nanoparticles, primarily focusing on cancer, genetic disorders, and vaccines (Thi et al., 2021).
6.2 Polymeric nanoparticles
Polymeric nanoparticles, made from biodegradable polymers such as PLGA, are versatile carriers for controlled and sustained drug release. Their usage is gaining traction in the delivery of anticancer agents, therapeutic proteins, and genes (Elmowafy et al., 2023). Success Stories: Several polymeric formulations are in late-stage trials. For instance, BIND-014, a PSMA-targeted docetaxel NP, has shown promise in metastatic prostate cancer clinical trials. However, many polymeric nanoparticle formulations are still in early stages of clinical evaluation. Ongoing Trials: Around 300 ongoing clinical trials focus on polymeric nanoparticles. These studies target cancer, neurodegenerative diseases, and diabetes, reflecting their growing application in various therapeutic areas (Oner et al., 2023).
6.3 Metallic nanoparticles (e.g., gold, silver, iron oxide)
Metallic nanoparticles have shown potential in areas like photothermal therapy, diagnostics, and antimicrobial applications. Gold nanoparticles (AuNPs) and iron oxide nanoparticles are under investigation for cancer therapy and diagnostic imaging, respectively (Dediu et al., 2023). Success Stories: Gold-based nanoparticles are currently undergoing clinical trials for treating cancers such as head and neck and prostate cancers. Although promising, most metallic nanoparticle therapies have not yet reached approval. Ongoing Trials: More than 200 ongoing clinical trials focus on metallic nanoparticles, with a majority involving diagnostic imaging and photothermal treatments in cancer (Zhang et al., 2023).
6.4 Silica nanoparticles
Mesoporous silica nanoparticles (MSNs) have shown potential as carriers for targeted drug delivery due to their tunable porosity, high surface area, and biocompatibility. They are being evaluated in cancer therapies, particularly for drug delivery to tumor sites (Rani et al., 2023). Success Stories: Some silica-based systems are in Phase I/II trials for cancer and autoimmune diseases. Despite being a promising technology, they have not yet achieved widespread clinical use. Ongoing Trials: There are currently around 80 ongoing clinical trials evaluating silica nanoparticles, mostly in cancer therapy, demonstrating the early yet promising stage of their development (Tng and Low, 2023).
6.5 Carbon-based nanoparticles
Carbon-based nanoparticles, including carbon nanotubes and graphene oxide, have gained attention for their potential use in drug delivery, tissue engineering, and imaging. Their unique properties, such as large surface area and electrical conductivity, make them attractive for various biomedical applications (Sharker, 2024). Success Stories: Most carbon-based nanoparticle systems are still in preclinical stages, but some have reached early-phase clinical trials, particularly for cancer imaging and drug delivery. Ongoing Trials: Fewer than 30 ongoing clinical trials are evaluating carbon nanoparticles, reflecting the challenges related to toxicity and biocompatibility that need to be overcome (Hristova-Panusheva et al., 2024).
6.6 Hybrid nanoparticles
Hybrid nanoparticles, which combine two or more types of nanomaterials (e.g., polymer-metal hybrids), are under investigation for their enhanced therapeutic efficacy and ability to combine diagnostics with treatment (theranostics) (Singh et al., 2024). Success Stories: Several hybrid nanoparticle platforms are in early clinical trials, targeting applications such as cancer theranostics. Though still in developmental stages, they represent a promising area for future therapies. Ongoing Trials: Only a limited number of clinical trials (around 50) are investigating hybrid nanoparticles, mostly in the fields of cancer and cardiovascular diseases (Yanar et al., 2023).
When mapping the success rates and progression of various types of nanoparticles through clinical trials, lipid-based nanoparticles clearly lead the way, primarily due to their success in mRNA vaccine platforms. Polymeric nanoparticles follow, showing great promise in drug delivery systems but with fewer FDA-approved examples. Metallic nanoparticles, although highly researched, are still facing challenges in clinical approval due to concerns about long-term safety and biocompatibility.
Incorporating this clinical trial data provides a clearer view of the current landscape for nanoparticle-based therapies. Lipid-based nanoparticles, particularly for cancer and vaccine delivery, appear to be the most successful in clinical applications. Polymeric and metallic nanoparticles also hold promise, though they face more hurdles in terms of regulatory approval. Future research and clinical trials will determine which of these nanoparticle platforms will lead in the realm of precision medicine and drug delivery. This information can guide readers in understanding which types of nanoparticles are more likely to succeed based on current clinical progress.
7 Safety considerations
An essential aspect of nanoparticle-based therapies is the evaluation of safety profiles in clinical settings. Although nanoparticles present distinct benefits in targeted drug delivery and imaging, there are lingering concerns regarding their potential toxicity, immunogenicity, and enduring impact on human health. Clinical trials are pivotal in evaluating the safety of nanoparticle formulations through thorough monitoring of adverse events, laboratory analyses, and extended follow-up investigations (Ragelle et al., 2017). Several factors influence the safety of nanoparticle-based therapies, including nanoparticle composition, size, surface charge, route of administration, and biodegradability. Understanding the pharmacokinetics and biodistribution of nanoparticles in vivo is critical for predicting their systemic effects and minimizing potential risks to patients. Furthermore, the development of standardized protocols for nanoparticle characterization and toxicity assessment is essential for ensuring consistency and comparability across different studies (Elumalai et al., 2024). Safety considerations are paramount in the development and clinical translation of nanoparticle-based therapies. Although nanoparticles offer promising benefits in targeted drug delivery and imaging, their potential toxicity and long-term effects on human health necessitate thorough evaluation in clinical trials (Taha et al., 2020).
The composition and physicochemical properties of nanoparticles play a crucial role in determining their safety profiles. Variations in nanoparticle composition, such as different materials (e.g., metal, polymer, lipid), surface modifications (e.g., PEGylation, surface charge), and size distributions, can significantly impact their interactions with biological systems and potential toxicity. For example, certain metal nanoparticles may exhibit cytotoxic effects due to the release of metal ions, while surface modifications can influence nanoparticle stability, biodistribution, and clearance pathways (Yusuf et al., 2023). Analyzing the biodistribution and pharmacokinetics of nanoparticles in living organisms is crucial for evaluating their safety characteristics. Nanoparticles may undergo systemic distribution following administration, with potential accumulation in off-target organs and tissues. Methods like PET, MRI, and fluorescence imaging facilitate the non-invasive monitoring of nanoparticle biodistribution in both preclinical and clinical environments. Longitudinal studies are needed to evaluate the clearance kinetics and tissue retention of nanoparticles over time, informing dosing regimens and minimizing potential adverse effects (Kozics et al., 2021).
Nanoparticles have the potential to elicit immune responses, leading to inflammatory reactions or immune cell activation. Surface modifications and coatings can mitigate immunogenicity and enhance biocompatibility by reducing protein adsorption and shielding nanoparticles from recognition by the immune system. Immunotoxicity assessments in preclinical studies and immunomonitoring in clinical trials are critical for evaluating immune responses to nanoparticle formulations and predicting potential hypersensitivity reactions or immune-mediated adverse events in patients (Muhammad et al., 2020).
Nanoparticles may exhibit organ-specific toxicity, particularly in the liver, kidneys, and lungs, which are primary sites of nanoparticle accumulation and clearance. Hepatotoxicity and nephrotoxicity are common concerns associated with nanoparticles due to their uptake by hepatic Kupffer cells and renal filtration pathways. Preclinical studies using animal models and in vitro assays can elucidate the mechanisms underlying nanoparticle-induced organ toxicity and guide the selection of safe dosage regimens and administration routes in clinical trials (Ferdous and Nemmar, 2020). Assessing the long-term safety and biodegradation of nanoparticles is essential for predicting their potential risks and environmental impacts. While some nanoparticles undergo metabolism and excretion through renal or hepatobiliary pathways, others may persist in biological tissues or undergo gradual degradation over time. Longitudinal follow-up studies in clinical trials are needed to monitor the persistence of nanoparticles in vivo, assess potential accumulation in organs or tissues, and evaluate late-onset adverse effects or carcinogenicity (Singh and Gurjar, 2022).
Overall, a comprehensive understanding of the safety considerations associated with nanoparticle-based therapies is essential for informing regulatory decisions, guiding clinical practice, and ensuring patient safety. Continued interdisciplinary research efforts combining nanotechnology, toxicology, pharmacology, and clinical medicine are crucial for advancing the field and maximizing the therapeutic potential of nanoparticles while minimizing associated risks.
8 Major key challenges
While nanoparticle-based therapies have demonstrated substantial promise, several critical challenges remain that must be addressed to advance their clinical translation. A significant hurdle is manufacturing scalability. Although nanoparticles can be highly effective in preclinical and early-stage clinical trials, scaling up their production for widespread clinical use poses significant difficulties. The intricate synthesis and functionalization processes required for nanoparticles often make large-scale manufacturing complex and costly. For example, maintaining batch-to-batch consistency while preserving the unique properties of nanoparticles, such as size, shape, and surface charge, is difficult. The development of more efficient, scalable production methods will be essential to meeting clinical demands while maintaining product quality and effectiveness.
Another important consideration is cost-effectiveness. While nanoparticles can enhance drug delivery and reduce systemic side effects, the overall cost of nanoparticle-based treatments can be prohibitively high. The manufacturing process, raw materials (e.g., rare or specialized components), and the need for highly controlled production environments all contribute to these costs. For instance, Abraxane™, a widely used nanoparticle formulation of paclitaxel, has been shown to improve therapeutic outcomes in various cancers, but its high cost has limited accessibility for many patients. Ensuring that nanoparticle therapies are not only clinically effective but also economically viable will be essential to their broader adoption in healthcare systems.
In addition to these practical challenges, regulatory approvals remain a significant obstacle. Nanoparticles, due to their unique physicochemical properties, often do not fit neatly into existing regulatory frameworks. This has led to longer approval timelines and increased scrutiny from agencies such as the FDA and EMA. For example, while Doxil, a liposomal formulation of doxorubicin, was one of the first nanoparticle-based drugs to receive FDA approval, the process highlighted the need for more defined regulatory pathways for nanomedicines. Regulatory agencies must develop clear guidelines that address the specificities of nanoparticle-based therapies, such as their biodistribution, long-term safety, and potential environmental impact, to accelerate approval processes while ensuring patient safety.
Moreover, challenges related to the long-term safety of nanoparticles also warrant deeper exploration. Many clinical trials, such as the NCT01380769 trial involving CRLX101, have demonstrated short-term efficacy and manageable side effects, but questions remain about the potential long-term toxicity of nanoparticles, particularly concerning their accumulation in tissues and their eventual clearance from the body. Comprehensive long-term studies will be needed to ensure that the benefits of nanoparticle-based therapies outweigh any potential risks associated with prolonged use.
By addressing these challenges—particularly in the areas of scalability, cost, and regulatory frameworks—future research and development efforts can ensure that nanoparticle-based therapies reach their full potential in clinical practice. These factors are critical not only for the advancement of nanomedicine but also for its accessibility and integration into global healthcare systems.
While nanoparticle-based therapies have shown significant promise in several areas, there are critical areas where further research is needed. For instance, improving nanoparticle targeting remains a challenge, as current methods often result in suboptimal accumulation in target tissues. Some breakthroughs have been made in this area, such as in the NCT01688999 trial, where PEGylated liposomal doxorubicin was used to treat patients with recurrent ovarian cancer. This trial demonstrated that nanoparticle modification could improve drug delivery by enhancing circulation time and reducing off-target effects, yet precise tumor targeting still needs improvement to maximize therapeutic outcomes.
Additionally, the NCT01692079 trial, which evaluated BIND-014, a PSMA-targeted nanoparticle containing docetaxel, marked a significant advancement in targeted nanoparticle therapy for prostate cancer. The trial showcased enhanced targeting to prostate tumor cells, yet off-target toxicity still occurred, underlining the need for more selective and adaptable targeting strategies to minimize adverse effects and improve therapeutic indices.
Beyond targeting, regulatory hurdles present another significant challenge. Nanoparticle formulations, due to their complexity, face difficulties in gaining approval from regulatory agencies like the FDA and EMA. For example, while Abraxane™, an albumin-bound paclitaxel nanoparticle, successfully gained FDA approval for the treatment of metastatic breast cancer, the lengthy regulatory process highlights the complexities associated with the approval of nanoparticle-based therapies. Streamlined regulatory frameworks are necessary to encourage innovation while ensuring patient safety.
The NCT03304210 trial using Abraxane™ for peritoneal carcinomatosis via pressurized intraperitoneal aerosol chemotherapy (PIPAC) also represents a breakthrough, showcasing how nanoparticle formulations can improve drug distribution and efficacy in difficult-to-treat cancers. However, the regulatory pathway for such novel delivery systems needs further clarification, with the establishment of nanomedicine-specific guidelines to aid in faster approvals.
Moreover, long-term safety data remain scarce for many nanoparticle formulations. In the NCT01380769 trial involving CRLX101, a cyclodextrin-based polymer-camptothecin, although positive clinical responses were observed in solid tumors, the long-term impacts on patient health, including nanoparticle clearance from the body and potential toxicity, warrant continued investigation. Addressing these regulatory and safety challenges will be crucial to optimizing the translation of nanoparticle therapies into mainstream clinical practice.
By continuing to explore these avenues—particularly improving targeting specificity and navigating regulatory complexities—future research can push the boundaries of nanoparticle therapeutics, ensuring that their potential is fully realized in clinical settings.
9 Collaborative efforts driving nanoparticle-based therapies
The development of effective nanoparticle-based therapies is not the result of isolated research efforts; rather, it is the product of successful collaborations between researchers, clinicians, and industry professionals. These partnerships have been instrumental in advancing therapeutic nanotechnologies from preclinical development to human clinical trials and, eventually, to clinical practice. Below are examples of how these collaborations have driven innovation in nanoparticle-based therapeutics.
9.1 Abraxane™: A collaborative success story
The development of Abraxane™, an albumin-bound formulation of paclitaxel, is a prime example of how industry-academia partnerships can lead to groundbreaking therapies. Researchers at Abraxis BioScience collaborated with clinicians to design a nanoparticle formulation that could enhance the solubility of paclitaxel and reduce the need for toxic solvents used in traditional formulations. Through extensive preclinical studies and clinical trials, Abraxane™ was found to improve the efficacy and safety of paclitaxel, leading to its FDA approval for metastatic breast cancer in 2005. This collaboration between academic researchers, clinicians, and industry professionals helped transform a promising scientific concept into a clinically viable therapy, now widely used for various cancers, including lung and pancreatic cancer.
9.2 BIND therapeutics: Academia and industry unite
BIND Therapeutics, in collaboration with MIT researchers, developed BIND-014, a PSMA-targeted nanoparticle loaded with docetaxel, specifically designed to treat prostate cancer. The partnership between academic scientists, who brought cutting-edge research on nanoparticle targeting, and industry professionals, who contributed to the scalability and commercialization process, led to successful early-stage clinical trials. The NCT01692079 trial demonstrated that BIND-014 effectively targeted prostate cancer cells, offering a more precise delivery of chemotherapy and reducing systemic side effects. This partnership showcased how targeted nanoparticles could improve therapeutic outcomes, encouraging further research into other cancer types.
9.3 The CRLX101 trial: Industry-clinician synergy
The development of CRLX101, a nanoparticle formulation of camptothecin, exemplifies how collaboration between pharmaceutical companies and clinical researchers can enhance cancer treatment. Cerulean Pharma worked closely with oncologists to design and implement the NCT01380769 clinical trial, which tested CRLX101 in patients with advanced solid tumors. The synergy between clinicians, who provided real-world patient insights, and researchers, who optimized the nanoparticle’s design, led to the successful completion of the trial, demonstrating significant efficacy in drug delivery. This collaboration continues to influence ongoing studies, with potential future applications in various cancers.
9.4 Nanotechnology innovation at the university of California, san diego (UCSD)
A collaborative effort between UCSD researchers, oncologists, and Sorrento Therapeutics resulted in the development of novel liposomal nanoparticles for targeted cancer therapy. By combining academic expertise in nanoparticle engineering with clinical insights on cancer treatment, the team developed lipid-based nanoparticles capable of delivering chemotherapy drugs directly to tumor sites. This collaborative approach facilitated the transition from laboratory research to clinical application, demonstrating that multi-disciplinary partnerships are essential for translating nanomedicine research into practical cancer therapies.
9.5 NIH-industry partnerships for nanomedicine
The National Cancer Institute’s (NCI) Alliance for Nanotechnology in Cancer has been a driving force in promoting collaborations between researchers, clinicians, and industry partners. Through this initiative, numerous grants have been awarded to teams working on nanoparticle-based therapies, with a focus on accelerating the translation of laboratory discoveries into clinical treatments. The partnership between the NCI and industry players has led to advancements in nanoparticle formulations for cancer treatment, as seen with CALAA-01, a siRNA-loaded nanoparticle, which became one of the first RNA interference therapies tested in clinical trials. The NCI’s support has bridged the gap between academia and industry, encouraging the development of innovative cancer treatments.
10 The impact of collaborative efforts
These collaborations exemplify the importance of bringing together diverse expertise to tackle the complexities of nanoparticle-based therapies. Researchers contribute cutting-edge innovations in nanoparticle design, clinicians provide insights into patient needs and clinical trial design, and industry professionals ensure that these innovations are scalable, cost-effective, and meet regulatory standards. Through these partnerships, nanoparticle therapies are moving from theoretical concepts to effective treatments that can improve patient outcomes in oncology, infectious diseases, and beyond.
The continued success of nanoparticle-based therapies will depend on fostering even stronger collaborations between academia, industry, and clinical practitioners. By working together, these groups can overcome the challenges of manufacturing scalability, regulatory approval, and cost-effectiveness, ultimately ensuring that these innovative therapies are widely accessible to patients in need.
11 Future perspectives
The field of nanoparticle-based therapeutics is rapidly evolving due to advances in nanotechnology, materials science, and biomedical engineering. Future research aims to develop multifunctional nanoparticles for integrated diagnostic and therapeutic purposes, personalized medicine approaches, and optimized combination therapies. Collaboration among researchers, clinicians, regulatory bodies, and industry partners is crucial for translating nanoparticle research into clinical applications, improving patient care and treatment outcomes (Sahu et al., 2021). Expanding future directions in nanoparticle-based therapeutics involves exploring emerging trends, innovative technologies, and addressing potential challenges. This includes advancements in nanoparticle design, integrating novel therapeutic modalities, and strategies for overcoming translational barriers (Abdel-Mageed et al., 2021).
Multifunctional nanoparticles are being developed to merge diagnostic, therapeutic, and theranostic capabilities into a unified platform. Precision medicine approaches will utilize patient-specific biomarkers to deliver tailored therapies targeting specific molecular pathways or cellular abnormalities. Targeting the tumor microenvironment using nanoparticles aims to overcome immunosuppression, enhance antitumor immune responses, and improve overall survival in cancer patients (Thorat et al., 2020). Integration of emerging therapeutic modalities such as RNA interference, gene editing, and cell-based therapies into clinical practice will be facilitated by nanoparticles. Nanotechnology offers solutions for addressing global health challenges, including infectious diseases and resource-limited settings, through portable diagnostic devices and enhanced drug delivery systems (Swaminathan et al., 2021). Despite the potential, significant translational barriers and regulatory challenges remain, requiring collaborative efforts to develop standardized protocols and safety standards (Đorđević et al., 2022). In conclusion, future directions in nanoparticle-based therapeutics emphasize multidisciplinary collaboration, technological innovation, and personalized medicine approaches to improve patient outcomes globally.
12 Conclusion
In conclusion, human clinical trials play a crucial role in advancing advanced nanoparticles from lab research to clinical application, showcasing their transformative potential across medical fields like oncology, infectious diseases, and neurology. In oncology, nanoparticle therapies have reshaped treatment approaches, improved drug delivery, imaging, and combination therapies while minimizing toxicity. In infectious diseases, nanoparticles offer innovative solutions, from targeted drug delivery to immunomodulation, addressing challenges like drug resistance. Similarly, in neurology, nanoparticles offer new avenues for treating neurodegenerative disorders and brain tumors by bypassing barriers like the blood-brain barrier. Despite progress, challenges persist in optimizing formulations and ensuring safety, requiring standardized protocols and interdisciplinary collaboration. Continued partnership among stakeholders is crucial for translating nanoparticle research into clinical practice, potentially revolutionizing medical care and paving the way for personalized therapeutics in the future.
Author contributions
RK: Formal Analysis, Investigation, Resources, Validation, Writing–original draft. PN: Formal Analysis, Investigation, Resources, Validation, Writing–review and editing. VU: Data curation, Formal Analysis, Investigation, Resources, Writing–review and editing. Jeane Rebecca Roy: Formal Analysis, Investigation, Resources, Validation, Writing–review and editing. MM: Investigation, Resources, Supervision, Validation, Writing–review and editing. CP: Conceptualization, Formal Analysis, Investigation, Resources, Supervision, Validation, Writing–review and editing.
Funding
The author(s) declare that financial support was received for the research, authorship, and/or publication of this article. Project financed by Lucian Blaga University of Sibiu through the research grant LBUS-IRG-2023. This study also supported by Deanship of Research and Graduate Studies, King Khalid University, Abha, Saudi Arabia, through the Large Research Group Project under Grant no. R.G.P.2/506/45.
Conflict of interest
The authors declare that the research was conducted in the absence of any commercial or financial relationships that could be construed as a potential conflict of interest.
Publisher’s note
All claims expressed in this article are solely those of the authors and do not necessarily represent those of their affiliated organizations, or those of the publisher, the editors and the reviewers. Any product that may be evaluated in this article, or claim that may be made by its manufacturer, is not guaranteed or endorsed by the publisher.
Supplementary material
The Supplementary Material for this article can be found online at: https://www.frontiersin.org/articles/10.3389/fnano.2024.1479993/full#supplementary-material
References
Abdel-Mageed, H. M., Abuelezz, N. Z., Radwan, R. A., and Mohamed, S. A. (2021). Nanoparticles in nanomedicine: a comprehensive updated review on current status, challenges and emerging opportunities. J. Microencapsul. 38, 414–436. doi:10.1080/02652048.2021.1942275
Aday, J. S., Heifets, B. D., Pratscher, S. D., Bradley, E., Rosen, R., and Woolley, J. D. (2022). Great Expectations: recommendations for improving the methodological rigor of psychedelic clinical trials. Psychopharmacology 239, 1989–2010. doi:10.1007/s00213-022-06123-7
Adeniji, O. O., Ojemaye, M. O., and Okoh, A. I. (2022). Antibacterial activity of metallic nanoparticles against multidrug-resistant pathogens isolated from environmental samples: nanoparticles/antibiotic combination therapy and cytotoxicity study. ACS Applied Bio Mater. 5, 4814–4826. doi:10.1021/acsabm.2c00527
Ali, E. S., Sharker, S. M., Islam, M. T., Khan, I. N., Shaw, S., Rahman, M. A., et al. (2021). “Targeting cancer cells with nanotherapeutics and nanodiagnostics: current status and future perspectives,” in Seminars in cancer biology (Elsevier), 52–68.
Amjad, M. S., Fatima, R., Qureshi, H., and Benish, Z. (2024). The application of nanomaterials in biological and biomedical processes: advances and perspectives. Nanomater. Biomed. Bioeng. Appl., 1–25. doi:10.1007/978-981-97-0221-3_1
Áñez, G., Dunkle, L. M., Gay, C. L., Kotloff, K. L., Adelglass, J. M., Essink, B., et al. (2022). Safety, immunogenicity and efficacy of NVX-CoV2373 in adolescents in PREVENT-19: a randomized, phase 3 trial. medRxiv., 22279903. medRxiv. doi:10.1101/2022.09.20.22279903
Annu, , Sartaj, A., Qamar, Z., Md, S., Alhakamy, N. A., Baboota, S., et al. (2022). An insight to brain targeting utilizing polymeric nanoparticles: effective treatment modalities for neurological disorders and brain tumor. Front. Bioeng. Biotechnol. 10, 788128. doi:10.3389/fbioe.2022.788128
Arafa, M. G., Mousa, H. A., Kataia, M. M., Shehabeldin, M., and Afifi, N. N. (2023). Functionalized surface of PLGA nanoparticles in thermosensitive gel to enhance the efficacy of antibiotics against antibiotic resistant infections in endodontics: a randomized clinical trial. Int. J. Pharm. 6, 100219. doi:10.1016/j.ijpx.2023.100219
Arunachalam, P. S., Walls, A. C., Golden, N., Atyeo, C., Fischinger, S., Li, C., et al. (2021). Adjuvanting a subunit COVID-19 vaccine to induce protective immunity. Nature 594, 253–258. doi:10.1038/s41586-021-03530-2
Autio, K. A., Dreicer, R., Anderson, J., Garcia, J. A., Alva, A., Hart, L. L., et al. (2018). Safety and efficacy of BIND-014, a docetaxel nanoparticle targeting prostate-specific membrane antigen for patients with metastatic castration-resistant prostate cancer: a phase 2 clinical trial. JAMA Oncol. 4, 1344–1351. doi:10.1001/jamaoncol.2018.2168
Baik, C., Lee, S., Cook, K., Wallace, S., Wood, R., Santana-Davila, R., et al. (2021). A Phase II study of nab-Paclitaxel (nab-P) in patients with advanced non-small cell lung cancer with EGFR mutations after frontline tyrosine kinase inhibitor therapy. Cancer Treat. Res. Commun. 28, 100416. doi:10.1016/j.ctarc.2021.100416
Bains, A., Narang, D., Chawla, P., and Dhull, S. B. (2020). “Nanoparticles and antibiotic drug composite: a novel approach towards antimicrobial activity,” in Nanotechnological approaches in Food microbiology (Boca Raton, FL: CRC Press), 165–186.
Beyth, N., Yudovin-Farber, I., Perez-Davidi, M., Domb, A. J., and Weiss, E. I. (2010). Polyethyleneimine nanoparticles incorporated into resin composite cause cell death and trigger biofilm stress in vivo. Proc. Natl. Acad. Sci. 107, 22038–22043. doi:10.1073/pnas.1010341107
Blivet, G., Roman, F. J., Delrieu, J., and Touchon, J. (2024). Translation from preclinical research to clinical trials: brain–gut photobiomodulation therapy for Alzheimer's disease. J. Integr. Neurosci. 23, 57. doi:10.31083/j.jin2303057
Bonvalot, S., Rutkowski, P. L., Thariat, J., Carrère, S., Ducassou, A., Sunyach, M.-P., et al. (2022). Final safety and health-related quality of LIfe results of the phase 2/3 act. In. Sarc study with preoperative NBTXR3 plus radiation therapy versus radiation therapy in locally advanced soft-tissue sarcoma. Int. J. Radiat. Oncology* Biology* Phys. 114, 422–432. doi:10.1016/j.ijrobp.2022.07.001
Bortot, B., Mangogna, A., Di Lorenzo, G., Stabile, G., Ricci, G., and Biffi, S. (2023). Image-guided cancer surgery: a narrative review on imaging modalities and emerging nanotechnology strategies. J. Nanobiotechnology 21, 155. doi:10.1186/s12951-023-01926-y
Chand, U., and Kushawaha, P. K. (2023). Nano-immunomodulators: prospective applications to combat drug resistant bacterial infections and related complications. J. Biomaterials Sci. Polym. Ed. 34, 2577–2597. doi:10.1080/09205063.2023.2265619
Chehelgerdi, M., Chehelgerdi, M., Allela, O. Q. B., Pecho, R. D. C., Jayasankar, N., Rao, D. P., et al. (2023). Progressing nanotechnology to improve targeted cancer treatment: overcoming hurdles in its clinical implementation. Mol. cancer 22, 169. doi:10.1186/s12943-023-01865-0
Chen, D., Liu, X., Lu, X., and Tian, J. (2023). Nanoparticle drug delivery systems for synergistic delivery of tumor therapy. Front. Pharmacol. 14, 1111991. doi:10.3389/fphar.2023.1111991
Cheng, Y., Cai, S., Wu, H., Pan, J., Su, M., Wei, X., et al. (2024). Revolutionizing eye care: the game-changing applications of nano-antioxidants in ophthalmology. Nanoscale 16, 7307–7322. doi:10.1039/d4nr00611a
Cohen, A. L., Ray, A., Van Brocklin, M., Burnett, D. M., Bowen, R. C., Dyess, D. L., et al. (2017). A phase I trial of azacitidine and nanoparticle albumin bound paclitaxel in patients with advanced or metastatic solid tumors. Oncotarget 8, 52413–52419. doi:10.18632/oncotarget.14183
Cristea, M. C., Frankel, P., Synold, T., Rivkin, S., Lim, D., Chung, V., et al. (2019). A phase I trial of intraperitoneal nab-paclitaxel in the treatment of advanced malignancies primarily confined to the peritoneal cavity. Cancer Chemother. Pharmacol. 83, 589–598. doi:10.1007/s00280-019-03767-9
Dediu, V., Ghitman, J., Gradisteanu Pircalabioru, G., Chan, K. H., Iliescu, F. S., and Iliescu, C. (2023). Trends in photothermal nanostructures for antimicrobial applications. Int. J. Mol. Sci. 24, 9375. doi:10.3390/ijms24119375
Delshadi, R., Bahrami, A., Mcclements, D. J., Moore, M. D., and Williams, L. (2021). Development of nanoparticle-delivery systems for antiviral agents: a review. J. Control. Release 331, 30–44. doi:10.1016/j.jconrel.2021.01.017
Dong, Z., Si, G., Zhu, X., Li, C., Hua, R., Teng, J., et al. (2023). Diagnostic performance and safety of a novel ferumoxytol-enhanced coronary magnetic resonance angiography. Circ. Cardiovasc. Imaging 16, 580–590. doi:10.1161/circimaging.123.015404
Đorđević, S., Gonzalez, M. M., Conejos-Sánchez, I., Carreira, B., Pozzi, S., Acúrcio, R. C., et al. (2022). Current hurdles to the translation of nanomedicines from bench to the clinic. Drug Deliv. Transl. Res. 12, 500–525. doi:10.1007/s13346-021-01024-2
Doswald, S., Herzog, A. F., Zeltner, M., Zabel, A., Pregernig, A., Schläpfer, M., et al. (2022). Removal of circulating tumor cells from blood samples of cancer patients using highly magnetic nanoparticles: a translational research project. Pharmaceutics 14, 1397. doi:10.3390/pharmaceutics14071397
Elabd, G. M., Eldars, W., Shamaa, M. S., and Tawfik, M. A. (2024). Evaluation of the antibacterial effect of titanium dioxide nanoparticles combined with acrylic laminates for functional orthodontic appliances: a randomized controlled clinical trial. BMC Oral Health 24, 20. doi:10.1186/s12903-023-03805-2
Elmowafy, M., Shalaby, K., Elkomy, M. H., Alsaidan, O. A., Gomaa, H. A., Abdelgawad, M. A., et al. (2023). Polymeric nanoparticles for delivery of natural bioactive agents: recent advances and challenges. Polymers 15, 1123. doi:10.3390/polym15051123
Elsawy, S., Elsherif, W. M., and Hamed, R. (2021). Effect of silver nanoparticles on vancomycin resistant Staphylococcus aureus infection in critically ill patients. Pathogens Glob. Health 115, 315–324. doi:10.1080/20477724.2021.1914412
Elumalai, K., Srinivasan, S., and Shanmugam, A. (2024). Review of the efficacy of nanoparticle-based drug delivery systems for cancer treatment. Biomed. Technol. 5, 109–122. doi:10.1016/j.bmt.2023.09.001
Farag, A. F., and Hassabou, N. F. (2022). CD24-gold nanocomposite as promising and sensitive biomarker for cancer stem cells in salivary gland tumors. Nanomedicine Nanotechnol. Biol. Med. 46, 102598. doi:10.1016/j.nano.2022.102598
Fathi-Achachelouei, M., Knopf-Marques, H., Ribeiro Da Silva, C. E., Barthès, J., Bat, E., Tezcaner, A., et al. (2019). Use of nanoparticles in tissue engineering and regenerative medicine. Front. Bioeng. Biotechnol. 7, 113. doi:10.3389/fbioe.2019.00113
Ferdous, Z., and Nemmar, A. (2020). Health impact of silver nanoparticles: a review of the biodistribution and toxicity following various routes of exposure. Int. J. Mol. Sci. 21, 2375. doi:10.3390/ijms21072375
Fountzilas, E., Tsimberidou, A. M., Vo, H. H., and Kurzrock, R. (2022). Clinical trial design in the era of precision medicine. Genome Med. 14, 101. doi:10.1186/s13073-022-01102-1
Fries, L., Shinde, V., Stoddard, J. J., Thomas, D. N., Kpamegan, E., Lu, H., et al. (2017). Immunogenicity and safety of a respiratory syncytial virus fusion protein (RSV F) nanoparticle vaccine in older adults. Immun. and Ageing 14, 8–14. doi:10.1186/s12979-017-0090-7
Fury, M., Sherman, E., Rao, S., Wolden, S., Smith-Marrone, S., Mueller, B., et al. (2014). Phase I study of weekly nab-paclitaxel+ weekly cetuximab+ intensity-modulated radiation therapy (IMRT) in patients with stage III–IVB head and neck squamous cell carcinoma (HNSCC). Ann. Oncol. 25, 689–694. doi:10.1093/annonc/mdt579
Gamil, Y., Hamed, M. G., Elsayed, M., Essawy, A., Medhat, S., Zayed, S. O., et al. (2024). The anti-fungal effect of miconazole and miconazole-loaded chitosan nanoparticles gels in diabetic patients with Oral candidiasis-randomized control clinical trial and microbiological analysis. BMC Oral Health 24, 196. doi:10.1186/s12903-024-03952-0
Gatechompol, S., Kittanamongkolchai, W., Ketloy, C., Prompetchara, E., Thitithanyanont, A., Jongkaewwattana, A., et al. (2022). Safety and immunogenicity of a prefusion non-stabilized spike protein mRNA COVID-19 vaccine: a phase I trial. Nat. Microbiol. 7, 1987–1995. doi:10.1038/s41564-022-01271-0
Giannatempo, P., Raggi, D., Marandino, L., Bandini, M., Fare, E., Calareso, G., et al. (2020). Pembrolizumab and nab-paclitaxel as salvage therapy for platinum-treated, locally advanced or metastatic urothelial carcinoma: interim results of the open-label, single-arm, phase II PEANUT study. Ann. Oncol. 31, 1764–1772. doi:10.1016/j.annonc.2020.09.012
Glenn, G. M., Fries, L. F., Thomas, D. N., Smith, G., Kpamegan, E., Lu, H., et al. (2016). A randomized, blinded, controlled, dose-ranging study of a respiratory syncytial virus recombinant fusion (F) nanoparticle vaccine in healthy women of childbearing age. J. Infect. Dis. 213, 411–422. doi:10.1093/infdis/jiv406
Gradishar, W. J., Tjulandin, S., Davidson, N., Shaw, H., Desai, N., Bhar, P., et al. (2005). Phase III trial of nanoparticle albumin-bound paclitaxel compared with polyethylated castor oil–based paclitaxel in women with breast cancer. J. Clin. Oncol. 23, 7794–7803. doi:10.1200/jco.2005.04.937
Halwani, A. A. (2022). Development of pharmaceutical nanomedicines: from the bench to the market. Pharmaceutics 14, 106. doi:10.3390/pharmaceutics14010106
Hosseini, S., Mohammadnejad, J., Salamat, S., Zadeh, Z. B., Tanhaei, M., and Ramakrishna, S. (2023). Theranostic polymeric nanoparticles as a new approach in cancer therapy and diagnosis: a review. Mater. Today Chem. 29, 101400. doi:10.1016/j.mtchem.2023.101400
Hristova-Panusheva, K., Xenodochidis, C., Georgieva, M., and Krasteva, N. (2024). Nanoparticle-mediated drug delivery systems for precision targeting in oncology. Pharmaceuticals 17, 677. doi:10.3390/ph17060677
Hsu, J. C., Tang, Z., Eremina, O. E., Sofias, A. M., Lammers, T., Lovell, J. F., et al. (2023). Nanomaterial-based contrast agents. Nat. Rev. Methods Prim. 3, 30. doi:10.1038/s43586-023-00211-4
Hu, X., Zhang, Y., Ding, T., Liu, J., and Zhao, H. (2020). Multifunctional gold nanoparticles: a novel nanomaterial for various medical applications and biological activities. Front. Bioeng. Biotechnol. 8, 990. doi:10.3389/fbioe.2020.00990
Huang, H., Feng, W., Chen, Y., and Shi, J. (2020). Inorganic nanoparticles in clinical trials and translations. Nano today 35, 100972. doi:10.1016/j.nantod.2020.100972
Huang, Q., Chen, Y., Zhang, W., Xia, X., Li, H., Qin, M., et al. (2024). Nanotechnology for enhanced nose-to-brain drug delivery in treating neurological diseases. J. Control. Release 366, 519–534. doi:10.1016/j.jconrel.2023.12.054
Jackson, T. L., Mandava, N., Quiroz-Mercado, H., Benage, M., Garcia-Aguirre, G., Morales-Canton, V., et al. (2021). Intravitreal quantum dots for retinitis pigmentosa: a first-in-human safety study. Nanomedicine 16, 617–626. doi:10.2217/nnm-2020-0471
Jangjou, A., Zareshahrabadi, Z., Abbasi, M., Talaiekhozani, A., Kamyab, H., Chelliapan, S., et al. (2022). Time to conquer fungal infectious diseases: employing nanoparticles as powerful and versatile antifungal nanosystems against a wide variety of fungal species. Sustainability 14, 12942. doi:10.3390/su141912942
Jose, J., Kumar, R., Harilal, S., Mathew, G. E., Parambi, D. G. T., Prabhu, A., et al. (2020). Magnetic nanoparticles for hyperthermia in cancer treatment: an emerging tool. Environ. Sci. Pollut. Res. 27, 19214–19225. doi:10.1007/s11356-019-07231-2
Jue, J. S., Coons, S., Hautvast, G., Thompson, S. F., Geraats, J., Richstone, L., et al. (2022). Novel automated three-dimensional surgical planning tool and magnetic resonance imaging/ultrasound fusion technology to perform nanoparticle ablation and cryoablation of the prostate for focal therapy. J. Endourology 36, 369–372. doi:10.1089/end.2021.0266
Kadkhoda, J., Tarighatnia, A., Barar, J., Aghanejad, A., and Davaran, S. (2022). Recent advances and trends in nanoparticles based photothermal and photodynamic therapy. Photodiagnosis Photodyn. Ther. 37, 102697. doi:10.1016/j.pdpdt.2021.102697
Kalra, J., Krishna, V., Reddy, B. S., Dhar, A., Venuganti, V. V., and Bhat, A. (2021). “Nanoparticles in medical imaging,” in Nanoparticles in analytical and medical devices (Elsevier), 175–210.
Kang, H., Ga, Y. J., Kim, S. H., Cho, Y. H., Kim, J. W., Kim, C., et al. (2023). Small interfering RNA (siRNA)-based therapeutic applications against viruses: principles, potential, and challenges. J. Biomed. Sci. 30, 88. doi:10.1186/s12929-023-00981-9
Kar, A., Ahamad, N., Dewani, M., Awasthi, L., Patil, R., and Banerjee, R. (2022). Wearable and implantable devices for drug delivery: applications and challenges. Biomaterials 283, 121435. doi:10.1016/j.biomaterials.2022.121435
Kashkooli, F. M., Soltani, M., and Souri, M. (2020). Controlled anti-cancer drug release through advanced nano-drug delivery systems: static and dynamic targeting strategies. J. Control. release 327, 316–349. doi:10.1016/j.jconrel.2020.08.012
Keech, C., Albert, G., Cho, I., Robertson, A., Reed, P., Neal, S., et al. (2020). Phase 1–2 trial of a SARS-CoV-2 recombinant spike protein nanoparticle vaccine. N. Engl. J. Med. 383, 2320–2332. doi:10.1056/nejmoa2026920
Kelly, J. T., Campbell, K. L., Gong, E., and Scuffham, P. (2020). The Internet of Things: impact and implications for health care delivery. J. Med. Internet Res. 22, e20135. doi:10.2196/20135
Kharlamov, A. N., Tyurnina, A. E., Veselova, V. S., Kovtun, O. P., Shur, V. Y., and Gabinsky, J. L. (2015). Silica–gold nanoparticles for atheroprotective management of plaques: results of the NANOM-FIM trial. Nanoscale 7, 8003–8015. doi:10.1039/c5nr01050k
Kirtane, A. R., Verma, M., Karandikar, P., Furin, J., Langer, R., and Traverso, G. (2021). Nanotechnology approaches for global infectious diseases. Nat. Nanotechnol. 16, 369–384. doi:10.1038/s41565-021-00866-8
Koc, S. N. T., Benam, S. R., Aral, I. P., Shahbazi, R., and Ulubayram, K. (2024). Gold nanoparticles-mediated photothermal and photodynamic therapies for cancer. Int. J. Pharm. 124057. doi:10.1016/j.ijpharm.2024.124057
Kozics, K., Sramkova, M., Kopecka, K., Begerova, P., Manova, A., Krivosikova, Z., et al. (2021). Pharmacokinetics, biodistribution, and biosafety of PEGylated gold nanoparticles in vivo. Nanomaterials 11, 1702. doi:10.3390/nano11071702
Kumar, P., Choonara, Y. E., Modi, G., Naidoo, D., and Pillay, V. (2014). Nanoparticulate strategies for the five R’s of traumatic spinal cord injury intervention: restriction, repair, regeneration, restoration and reorganization. Nanomedicine 9, 331–348. doi:10.2217/nnm.13.203
Kumthekar, P., Ko, C., Paunesku, T., Dixit, K., Sonabend, A., Bloch, O., et al. (2021). A first-in-human phase 0 clinical study of RNA interference-based spherical nucleic acids in patients with recurrent glioblastoma. Sci. Transl. Med. 13 (584), eabb3945. doi:10.1126/scitranslmed.abb3945
Lavanya, M., Krishnamoorthy, R., Alshuniaber, M. A., Manoharadas, S., Palanisamy, C. P., Veeraraghavan, V. P., et al. (2023). Formulation, characterization and evaluation of gelatin-syringic acid/zinc oxide nanocomposite for its effective anticancer, antioxidant and anti-inflammatory activities. J. King Saud University-Science 35, 102909. doi:10.1016/j.jksus.2023.102909
Lawitz, E. J., Shevell, D. E., Tirucherai, G. S., Du, S., Chen, W., Kavita, U., et al. (2022). BMS-986263 in patients with advanced hepatic fibrosis: 36-week results from a randomized, placebo-controlled phase 2 trial. Hepatology 75, 912–923. doi:10.1002/hep.32181
Li, J., Zhao, M., Sun, M., Wu, S., Zhang, H., Dai, Y., et al. (2020b). Multifunctional nanoparticles boost cancer immunotherapy based on modulating the immunosuppressive tumor microenvironment. ACS Applied Mater. and Interfaces 12, 50734–50747. doi:10.1021/acsami.0c14909
Li, J.-X., Huang, Q.-Y., Zhang, J.-Y., and Du, J.-Z. (2020a). Engineering nanoparticles to tackle tumor barriers. J. Mater. Chem. B 8, 6686–6696. doi:10.1039/d0tb00967a
Liao, J. B., Swensen, R. E., Reichow, J., Ovenell, K. J., Childs, J., Higgins, D., et al. (2015). Phase II trial of weekly nab-paclitaxel with GM-CSF as an immune modulator in recurrent platinum resistant ovarian, fallopian tube, and primary peritoneal cancer: clinical and immune responses, 33. American Society of Clinical Oncology, 5580.
Liu, H., Zhong, W., Zhang, X., Lin, D., and Wu, J. (2021a). Nanomedicine as a promising strategy for the theranostics of infectious diseases. J. Mater. Chem. B 9, 7878–7908. doi:10.1039/d1tb01316e
Liu, Q., Chen, J., Qin, Y., Jiang, B., and Zhang, T. (2020). Zein/fucoidan-based composite nanoparticles for the encapsulation of pterostilbene: preparation, characterization, physicochemical stability, and formation mechanism. Int. J. Biol. Macromol. 158, 461–470. doi:10.1016/j.ijbiomac.2020.04.128
Liu, R., Luo, C., Pang, Z., Zhang, J., Ruan, S., Wu, M., et al. (2023). Advances of nanoparticles as drug delivery systems for disease diagnosis and treatment. Chin. Chem. Lett. 34, 107518. doi:10.1016/j.cclet.2022.05.032
Liu, S., Khan, A. R., Yang, X., Dong, B., Ji, J., and Zhai, G. (2021b). The reversal of chemotherapy-induced multidrug resistance by nanomedicine for cancer therapy. J. Control. Release 335, 1–20. doi:10.1016/j.jconrel.2021.05.012
Llop, J., and Lammers, T. (2021). Nanoparticles for cancer diagnosis, radionuclide therapy and theranostics. ACS nano 15, 16974–16981. doi:10.1021/acsnano.1c09139
Luo, D., Wang, X., Burda, C., and Basilion, J. P. (2021). Recent development of gold nanoparticles as contrast agents for cancer diagnosis. Cancers 13, 1825. doi:10.3390/cancers13081825
Madhi, S. A., Moodley, D., Hanley, S., Archary, M., Hoosain, Z., Lalloo, U., et al. (2022). Immunogenicity and safety of a SARS-CoV-2 recombinant spike protein nanoparticle vaccine in people living with and without HIV-1 infection: a randomised, controlled, phase 2A/2B trial. Lancet HIV 9, e309–e322. doi:10.1016/s2352-3018(22)00041-8
Madhi, S. A., Polack, F. P., Piedra, P. A., Munoz, F. M., Trenholme, A. A., Simões, E. A., et al. (2020). Respiratory syncytial virus vaccination during pregnancy and effects in infants. N. Engl. J. Med. 383, 426–439. doi:10.1056/nejmoa1908380
Makabenta, J. M. V., Nabawy, A., Li, C.-H., Schmidt-Malan, S., Patel, R., and Rotello, V. M. (2021). Nanomaterial-based therapeutics for antibiotic-resistant bacterial infections. Nat. Rev. Microbiol. 19, 23–36. doi:10.1038/s41579-020-0420-1
Manimaran, D., Elangovan, N., Mani, P., Subramanian, K., Ali, D., Alarifi, S., et al. (2022). Isolongifolene-loaded chitosan nanoparticles synthesis and characterization for cancer treatment. Sci. Rep. 12, 19250. doi:10.1038/s41598-022-23386-4
Mckiernan, J. M., Onyeji, I., Lascano, D., Ahn, J., Desai, N., Abate-Shen, C., et al. (2016). A phase I/II study of albumin-bound rapamycin nanoparticles in the treatment of bacillus calmette-guerin refractory non-muscle invasive transitional cell bladder cancer, 34. American Society of Clinical Oncology.e16008
Meyer, E. L., Mesenbrink, P., Dunger-Baldauf, C., Fülle, H.-J., Glimm, E., Li, Y., et al. (2020). The evolution of master protocol clinical trial designs: a systematic literature review. Clin. Ther. 42, 1330–1360. doi:10.1016/j.clinthera.2020.05.010
Miauton, A., Audran, R., Besson, J., Maby-El Hajjami, H., Karlen, M., Warpelin-Decrausaz, L., et al. (2024). Safety and immunogenicity of a synthetic nanoparticle-based, T cell priming peptide vaccine against dengue in healthy adults in Switzerland: a double-blind, randomized, vehicle-controlled, phase 1 study. EBioMedicine 99, 104922. doi:10.1016/j.ebiom.2023.104922
Miedema, I. H., Zwezerijnen, G. J., Huisman, M. C., Doeleman, E., Mathijssen, R. H., Lammers, T., et al. (2022). PET-CT imaging of polymeric nanoparticle tumor accumulation in patients. Adv. Mater. 34, 2201043. doi:10.1002/adma.202201043
Mirza, Z., and Karim, S. (2021). Nanoparticles-based drug delivery and gene therapy for breast cancer: recent advancements and future challenges. Seminars cancer Biol. 69, 226–237. doi:10.1016/j.semcancer.2019.10.020
Molkenova, A., Atabaev, T. S., Hong, S. W., Mao, C., Han, D.-W., and Kim, K. S. (2022). Designing inorganic nanoparticles into computed tomography and magnetic resonance (CT/MR) imaging-guidable photomedicines. Mater. Today Nano 18, 100187. doi:10.1016/j.mtnano.2022.100187
Montiel Schneider, M. G., Martín, M. J., Otarola, J., Vakarelska, E., Simeonov, V., Lassalle, V., et al. (2022). Biomedical applications of iron oxide nanoparticles: current insights progress and perspectives. Pharmaceutics 14, 204. doi:10.3390/pharmaceutics14010204
Muhammad, Q., Jang, Y., Kang, S. H., Moon, J., Kim, W. J., and Park, H. (2020). Modulation of immune responses with nanoparticles and reduction of their immunotoxicity. Biomaterials Sci. 8, 1490–1501. doi:10.1039/c9bm01643k
Mukherjee, S., Madamsetty, V. S., Bhattacharya, D., Roy Chowdhury, S., Paul, M. K., and Mukherjee, A. (2020). Recent advancements of nanomedicine in neurodegenerative disorders theranostics. Adv. Funct. Mater. 30, 2003054. doi:10.1002/adfm.202003054
Muňoz, F. M., Swamy, G. K., Hickman, S. P., Agrawal, S., Piedra, P. A., Glenn, G. M., et al. (2019). Safety and immunogenicity of a respiratory syncytial virus fusion (F) protein nanoparticle vaccine in healthy third-trimester pregnant women and their infants. J. Infect. Dis. 220, 1802–1815. doi:10.1093/infdis/jiz390
Murar, M., Albertazzi, L., and Pujals, S. (2022). Advanced optical imaging-guided nanotheranostics towards personalized cancer drug delivery. Nanomaterials 12, 399. doi:10.3390/nano12030399
Nahleh, Z., Barlow, W., Hayes, D., Schott, A., Gralow, J., Sikov, W., et al. (2016). SWOG S0800 (NCI CDR0000636131): addition of bevacizumab to neoadjuvant nab-paclitaxel with dose-dense doxorubicin and cyclophosphamide improves pathologic complete response (pCR) rates in inflammatory or locally advanced breast cancer. Breast cancer Res. Treat. 158, 485–495. doi:10.1007/s10549-016-3889-6
Nami, S., Aghebati-Maleki, A., and Aghebati-Maleki, L. (2021). Current applications and prospects of nanoparticles for antifungal drug delivery. EXCLI J. 20, 562–584. doi:10.17179/excli2020-3068
Nanda, R., Stringer-Reasor, E. M., Saha, P., Kocherginsky, M., Gibson, J., Libao, B., et al. (2016). A randomized phase I trial of nanoparticle albumin-bound paclitaxel with or without mifepristone for advanced breast cancer. Springerplus 5, 947–949. doi:10.1186/s40064-016-2457-1
Oner, E., Ilhan, M., Gultekin, H. E., and Karpuz, M. (2023). Nanoconjugate formulations for enhanced drug delivery. Adv. Mod. Approaches Drug Deliv., 441–491. doi:10.1016/b978-0-323-91668-4.00023-x
Owen, D. H., Williams, T. M., Bertino, E. M., Mo, X., Webb, A., Schweitzer, C., et al. (2019). Homologous recombination and DNA repair mutations in patients treated with carboplatin and nab-paclitaxel for metastatic non-small cell lung cancer. Lung Cancer 134, 167–173. doi:10.1016/j.lungcan.2019.06.017
Padmanabhan, P., Palanivel, M., Kumar, A., Máthé, D., Radda, G. K., Lim, K.-L., et al. (2020). Nanotheranostic agents for neurodegenerative diseases. Emerg. Top. Life Sci. 4, 645–675. doi:10.1042/etls20190141
Pala, R., Anju, V., Dyavaiah, M., Busi, S., and Nauli, S. M. (2020). Nanoparticle-mediated drug delivery for the treatment of cardiovascular diseases. Int. J. Nanomedicine Vol. 15, 3741–3769. doi:10.2147/ijn.s250872
Palanisamy, C. P., Cui, B., Zhang, H., Gunasekaran, V. P., Ariyo, A. L., Jayaraman, S., et al. (2022). A critical review on starch-based electrospun nanofibrous scaffolds for wound healing application. Int. J. Biol. Macromol. 222, 1852–1860. doi:10.1016/j.ijbiomac.2022.09.274
Palanisamy, C. P., Cui, B., Zhang, H., Jayaraman, S., and Kodiveri Muthukaliannan, G. (2020). A comprehensive review on corn starch-based nanomaterials: properties, simulations, and applications. Polymers 12, 2161. doi:10.3390/polym12092161
Pandey, A., Singh, B. K., Gayathiri, E., Balasubramani, S., Duraisamy, S. M., Kothari, A., et al. (2024). “Nanoparticles in biomedical and clinical research: a current perspective and future implications,” in Nanomaterials for biomedical and bioengineering applications (Springer), 415–457.
Pei, J., Palanisamy, C. P., Alugoju, P., Anthikapalli, N. V. A., Natarajan, P. M., Umapathy, V. R., et al. (2023). A comprehensive review on bio-based materials for chronic diabetic wounds. Molecules 28, 604. doi:10.3390/molecules28020604
Pei, J., Yan, Y., Jayaraman, S., Rajagopal, P., Natarajan, P. M., Umapathy, V. R., et al. (2024a). A review on advancements in the application of starch-based nanomaterials in biomedicine: precision drug delivery and cancer therapy. Int. J. Biol. Macromol. 130746. doi:10.1016/j.ijbiomac.2024.130746
Pei, J., Yan, Y., Palanisamy, C. P., Jayaraman, S., Natarajan, P. M., Umapathy, V. R., et al. (2024b). Materials-based drug delivery approaches: recent advances and future perspectives. Green Process. Synthesis 13, 20230094. doi:10.1515/gps-2023-0094
Peñate Medina, T., Gerle, M., Humbert, J., Chu, H., Köpnick, A.-L., Barkmann, R., et al. (2020). Lipid-iron nanoparticle with a cell stress release mechanism combined with a local alternating magnetic field enables site-activated drug release. Cancers 12, 3767. doi:10.3390/cancers12123767
Perciani, C. T., Liu, L. Y., Wood, L., and Macparland, S. A. (2020). Enhancing immunity with nanomedicine: employing nanoparticles to harness the immune system. ACS nano 15, 7–20. doi:10.1021/acsnano.0c08913
Pitzalis, C., Choy, E. H., and Buch, M. H. (2020). Transforming clinical trials in rheumatology: towards patient-centric precision medicine. Nat. Rev. Rheumatol. 16, 590–599. doi:10.1038/s41584-020-0491-4
Pivonello, R., Ferrigno, R., De Martino, M. C., Simeoli, C., Di Paola, N., Pivonello, C., et al. (2020). Medical treatment of Cushing's disease: an overview of the current and recent clinical trials. Front. Endocrinol. 11, 648. doi:10.3389/fendo.2020.00648
Prasanna, T., Malik, L., Mccuaig, R. D., Tu, W. J., Wu, F., Lim, P. S., et al. (2022). A phase 1 proof of concept study evaluating the addition of an LSD1 inhibitor to nab-paclitaxel in advanced or metastatic breast cancer (EPI-PRIMED). Front. Oncol. 12, 862427. doi:10.3389/fonc.2022.862427
Pulingam, T., Foroozandeh, P., Chuah, J.-A., and Sudesh, K. (2022). Exploring various techniques for the chemical and biological synthesis of polymeric nanoparticles. Nanomaterials 12, 576. doi:10.3390/nano12030576
Ragelle, H., Danhier, F., Préat, V., Langer, R., and Anderson, D. G. (2017). Nanoparticle-based drug delivery systems: a commercial and regulatory outlook as the field matures. Expert Opin. drug Deliv. 14, 851–864. doi:10.1080/17425247.2016.1244187
Raj, S., Khurana, S., Choudhari, R., Kesari, K. K., Kamal, M. A., Garg, N., et al. (2021). “Specific targeting cancer cells with nanoparticles and drug delivery in cancer therapy,” in Seminars in cancer biology (Elsevier), 166–177.
Rani, R., Malik, P., Dhania, S., and Mukherjee, T. K. (2023). Recent advances in mesoporous silica nanoparticle-mediated drug delivery for breast cancer treatment. Pharmaceutics 15, 227. doi:10.3390/pharmaceutics15010227
Rashidi, N., Davidson, M., Apostolopoulos, V., and Nurgali, K. (2024). Nanoparticles in cancer diagnosis and treatment: progress, challenges, and opportunities. J. Drug Deliv. Sci. Technol. 95, 105599. doi:10.1016/j.jddst.2024.105599
Rezaei, B., Yari, P., Sanders, S. M., Wang, H., Chugh, V. K., Liang, S., et al. (2024). Magnetic nanoparticles: a review on synthesis, characterization, functionalization, and biomedical applications. Small 20, 2304848. doi:10.1002/smll.202304848
Robidoux, A., Buzdar, A. U., Quinaux, E., Jacobs, S., Rastogi, P., Fourchotte, V., et al. (2010). A phase II neoadjuvant trial of sequential nanoparticle albumin-bound paclitaxel followed by 5-fluorouracil/epirubicin/cyclophosphamide in locally advanced breast cancer. Clin. breast cancer 10, 81–86. doi:10.3816/cbc.2010.n.011
Sahu, T., Ratre, Y. K., Chauhan, S., Bhaskar, L., Nair, M. P., and Verma, H. K. (2021). Nanotechnology based drug delivery system: current strategies and emerging therapeutic potential for medical science. J. Drug Deliv. Sci. Technol. 63, 102487. doi:10.1016/j.jddst.2021.102487
Sande, L., Graversen, M., Hubner, M., Pocard, M., Reymond, M., Vaira, M., et al. (2018). Intraperitoneal aerosolization of albumin-stabilized paclitaxel nanoparticles (Abraxane™) for peritoneal carcinomatosis - a phase I first-in-human study. Pleura Perit. 3, 20180112. doi:10.1515/pp-2018-0112
Sarkar, J., Das, S., Aich, S., Bhattacharyya, P., and Acharya, K. (2022). Antiviral potential of nanoparticles for the treatment of Coronavirus infections. J. Trace Elem. Med. Biol. 72, 126977. doi:10.1016/j.jtemb.2022.126977
Sayed, F. a.-Z., Eissa, N. G., Shen, Y., Hunstad, D. A., Wooley, K. L., and Elsabahy, M. (2022). Morphologic design of nanostructures for enhanced antimicrobial activity. J. Nanobiotechnology 20, 536. doi:10.1186/s12951-022-01733-x
Sharker, S. M. (2024). “Emerging carbon-based nanomaterials for biomedical and bioengineering applications,” in Nanomaterials for biomedical and bioengineering applications (Springer), 203–219.
Sharma, R. K., Yadav, S., Dutta, S., Kale, H. B., Warkad, I. R., Zbořil, R., et al. (2021). Silver nanomaterials: synthesis and (electro/photo) catalytic applications. Chem. Soc. Rev. 50, 11293–11380. doi:10.1039/d0cs00912a
Sharma, S., Shekhar, S., Gautam, S., Sharma, B., Kumar, A., and Jain, P. (2020). Carbon-based nanomaterials as novel nanosensors. Nanofabrication smart nanosensor Appl., 323–347. doi:10.1016/b978-0-12-820702-4.00014-3
Sheoran, S., Arora, S., Samsonraj, R., Govindaiah, P., and vuree, S. (2022). Lipid-based nanoparticles for treatment of cancer. Heliyon 8, e09403. doi:10.1016/j.heliyon.2022.e09403
Shinde, V., Cho, I., Plested, J. S., Agrawal, S., Fiske, J., Cai, R., et al. (2022). Comparison of the safety and immunogenicity of a novel Matrix-M-adjuvanted nanoparticle influenza vaccine with a quadrivalent seasonal influenza vaccine in older adults: a phase 3 randomised controlled trial. Lancet Infect. Dis. 22, 73–84. doi:10.1016/s1473-3099(21)00192-4
Shinde, V., Fries, L., Wu, Y., Agrawal, S., Cho, I., Thomas, D. N., et al. (2018). Improved titers against influenza drift variants with a nanoparticle vaccine. N. Engl. J. Med. 378, 2346–2348. doi:10.1056/nejmc1803554
Siafaka, P. I., Okur, N. Ü., Karantas, I. D., Okur, M. E., and Gündoğdu, E. A. (2021). Current update on nanoplatforms as therapeutic and diagnostic tools: a review for the materials used as nanotheranostics and imaging modalities. Asian J. Pharm. Sci. 16, 24–46. doi:10.1016/j.ajps.2020.03.003
Singh, D., and Gurjar, B. R. (2022). Nanotechnology for agricultural applications: facts, issues, knowledge gaps, and challenges in environmental risk assessment. J. Environ. Manag. 322, 116033. doi:10.1016/j.jenvman.2022.116033
Singh, S., Banerjee, R., and Pal, K. (2024). Emerging trends in biodegradable polymer-metal nanoconjugates for cancer therapeutics. Eur. Polym. J. 207, 112835. doi:10.1016/j.eurpolymj.2024.112835
Skandalakis, G. P., Rivera, D. R., Rizea, C. D., Bouras, A., Jesu Raj, J. G., Bozec, D., et al. (2020). Hyperthermia treatment advances for brain tumors. Int. J. Hyperth. 37, 3–19. doi:10.1080/02656736.2020.1772512
Smith, E. S., Porterfield, J. E., and Kannan, R. M. (2019). Leveraging the interplay of nanotechnology and neuroscience: designing new avenues for treating central nervous system disorders. Adv. drug Deliv. Rev. 148, 181–203. doi:10.1016/j.addr.2019.02.009
Sobhanan, J., Rival, J. V., Anas, A., Shibu, E. S., Takano, Y., and Biju, V. (2023). Luminescent quantum dots: synthesis, optical properties, bioimaging and toxicity. Adv. Drug Deliv. Rev. 114830. doi:10.1016/j.addr.2023.114830
Sohal, D. P., Duong, M., Ahmad, S. A., Gandhi, N. S., Beg, M. S., Wang-Gillam, A., et al. (2021). Efficacy of perioperative chemotherapy for resectable pancreatic adenocarcinoma: a phase 2 randomized clinical trial. JAMA Oncol. 7, 421–427. doi:10.1001/jamaoncol.2020.7328
Sousa, F., Ferreira, D., Reis, S., and Costa, P. (2020). Current insights on antifungal therapy: novel nanotechnology approaches for drug delivery systems and new drugs from natural sources. Pharmaceuticals 13, 248. doi:10.3390/ph13090248
Subbiah, V. (2023). The next generation of evidence-based medicine. Nat. Med. 29, 49–58. doi:10.1038/s41591-022-02160-z
Subbiah, V., Combest, A., Grilley-Olson, J., Sharma, N., Andrews, E., Bobe, I., et al. (2016). Phase Ib/II trial of NC-6004 (nanoparticle cisplatin) plus gemcitabine (G) in patients (pts) with advanced solid tumors. Eur. J. Cancer 69, S118–S119. doi:10.1016/s0959-8049(16)32952-5
Sujitha, S. D. A., Dhanam, N., Lavanya, M. S., and Ariyamuthu, R. (2023). From lab to clinic: translating biochemical discoveries into medical innovations. J. Popul. Ther. Clin. Pharmacol. 30, 881–891. doi:10.53555/jptcp.v30i17.3909
Swaminathan, G., Shigna, A., Kumar, A., Byroju, V. V., Durgempudi, V. R., and Dinesh Kumar, L. (2021). RNA interference and nanotechnology: a promising alliance for next generation cancer therapeutics. Front. Nanotechnol. 3, 694838. doi:10.3389/fnano.2021.694838
Taha, M. S., Padmakumar, S., Singh, A., and Amiji, M. M. (2020). Critical quality attributes in the development of therapeutic nanomedicines toward clinical translation. Drug Deliv. Transl. Res. 10, 766–790. doi:10.1007/s13346-020-00744-1
Thi, T. T. H., Suys, E. J., Lee, J. S., Nguyen, D. H., Park, K. D., and Truong, N. P. (2021). Lipid-based nanoparticles in the clinic and clinical trials: from cancer nanomedicine to COVID-19 vaccines. Vaccines 9, 359. doi:10.3390/vaccines9040359
Thorat, N. D., Townley, H. E., Patil, R. M., Tofail, S. A., and Bauer, J. (2020). Comprehensive approach of hybrid nanoplatforms in drug delivery and theranostics to combat cancer. Drug Discov. Today 25, 1245–1252. doi:10.1016/j.drudis.2020.04.018
Tng, D. J. H., and Low, J. G. H. (2023). Current status of silica-based nanoparticles as therapeutics and its potential as therapies against viruses. Antivir. Res. 210, 105488. doi:10.1016/j.antiviral.2022.105488
Toback, S., Galiza, E., Cosgrove, C., Galloway, J., Goodman, A. L., Swift, P. A., et al. (2022). Safety, immunogenicity, and efficacy of a COVID-19 vaccine (NVX-CoV2373) co-administered with seasonal influenza vaccines: an exploratory substudy of a randomised, observer-blinded, placebo-controlled, phase 3 trial. Lancet Respir. Med. 10, 167–179. doi:10.1016/s2213-2600(21)00409-4
Treccani, L. (2023). “Introduction to ceramic materials,” in Surface-functionalized ceramics: for biotechnological and environmental applications, 1–46.
Untch, M., Jackisch, C., Schneeweiss, A., Conrad, B., Aktas, B., Denkert, C., et al. (2016). Nab-paclitaxel versus solvent-based paclitaxel in neoadjuvant chemotherapy for early breast cancer (GeparSepto—GBG 69): a randomised, phase 3 trial. lancet Oncol. 17, 345–356. doi:10.1016/s1470-2045(15)00542-2
Von Hoff, D. D., Mita, M. M., Ramanathan, R. K., Weiss, G. J., Mita, A. C., Lorusso, P. M., et al. (2016). Phase I study of PSMA-targeted docetaxel-containing nanoparticle BIND-014 in patients with advanced solid tumors. Clin. Cancer Res. 22, 3157–3163. doi:10.1158/1078-0432.ccr-15-2548
Vucic, S., Menon, P., Huynh, W., Mahoney, C., Ho, K. S., Hartford, A., et al. (2023). Efficacy and safety of CNM-Au8 in amyotrophic lateral sclerosis (RESCUE-ALS study): a phase 2, randomised, double-blind, placebo-controlled trial and open label extension. EClinicalMedicine 60, 102036. doi:10.1016/j.eclinm.2023.102036
Walton, M., Cappelleri, J., Byrom, B., Goldsack, J., Eremenco, S., Harris, D., et al. (2020). Considerations for development of an evidence dossier to support the use of mobile sensor technology for clinical outcome assessments in clinical trials. Contemp. Clin. Trials 91, 105962. doi:10.1016/j.cct.2020.105962
Wang, C., and Zhang, S. (2023). Advantages of nanomedicine in cancer therapy: a review. ACS Applied Nano Mater. 6, 22594–22610. doi:10.1021/acsanm.3c04487
Weiss, G. J., Chao, J., Neidhart, J. D., Ramanathan, R. K., Bassett, D., Neidhart, J. A., et al. (2013). First-in-human phase 1/2a trial of CRLX101, a cyclodextrin-containing polymer-camptothecin nanopharmaceutical in patients with advanced solid tumor malignancies. Investig. new drugs 31, 986–1000. doi:10.1007/s10637-012-9921-8
Wichman, C., Smith, L. M., and Yu, F. (2021). A framework for clinical and translational research in the era of rigor and reproducibility. J. Clin. Transl. Sci. 5, e31. doi:10.1017/cts.2020.523
Xie, W., Zhang, S., Pan, F., Chen, S., Zhong, L., Wang, J., et al. (2021). Nanomaterial-based ROS-mediated strategies for combating bacteria and biofilms. J. Mater. Res. 36, 822–845. doi:10.1557/s43578-021-00134-4
Xu, X., Wu, Y., Qian, X., Wang, Y., Wang, J., Li, J., et al. (2022). Nanomedicine strategies to circumvent intratumor extracellular matrix barriers for cancer therapy. Adv. Healthc. Mater. 11, 2101428. doi:10.1002/adhm.202101428
Yadav, K., Pradhan, M., Singh, D., and Singh, M. R. (2022). “Targeting autoimmune disorders through metal nanoformulation in overcoming the fences of conventional treatment approaches,” in Translational autoimmunity (Elsevier), 361–393.
Yadav, K., Sahu, K. K., Gnanakani, S. P. E., Sure, P., Vijayalakshmi, R., Sundar, V., et al. (2023). Biomedical applications of nanomaterials in the advancement of nucleic acid therapy: mechanistic challenges, delivery strategies, and therapeutic applications. Int. J. Biol. Macromol. 124582. doi:10.1016/j.ijbiomac.2023.124582
Yanar, F., Carugo, D., and Zhang, X. (2023). Hybrid nanoplatforms comprising organic nanocompartments encapsulating inorganic nanoparticles for enhanced drug delivery and bioimaging applications. Molecules 28, 5694. doi:10.3390/molecules28155694
Yardley, D. A., Hart, L., Bosserman, L., Salleh, M. N., Waterhouse, D. M., Hagan, M. K., et al. (2013). Phase II study evaluating lapatinib in combination with nab-paclitaxel in HER2-overexpressing metastatic breast cancer patients who have received no more than one prior chemotherapeutic regimen. Breast cancer Res. Treat. 137, 457–464. doi:10.1007/s10549-012-2341-9
Yetisgin, A. A., Cetinel, S., Zuvin, M., Kosar, A., and Kutlu, O. (2020). Therapeutic nanoparticles and their targeted delivery applications. Molecules 25, 2193. doi:10.3390/molecules25092193
Yu, Z., Gao, L., Chen, K., Zhang, W., Zhang, Q., Li, Q., et al. (2021). Nanoparticles: a new approach to upgrade cancer diagnosis and treatment. Nanoscale Res. Lett. 16, 88. doi:10.1186/s11671-021-03489-z
Yusuf, A., Almotairy, A. R. Z., Henidi, H., Alshehri, O. Y., and Aldughaim, M. S. (2023). Nanoparticles as Drug Delivery Systems: a Review of the implication of nanoparticles’ physicochemical properties on responses in biological systems. Polymers 15, 1596. doi:10.3390/polym15071596
Zhang, R., Kiessling, F., Lammers, T., and Pallares, R. M. (2023). Clinical translation of gold nanoparticles. Drug Deliv. Transl. Res. 13, 378–385. doi:10.1007/s13346-022-01232-4
Zheng, B., and Mcclements, D. J. (2020). Formulation of more efficacious curcumin delivery systems using colloid science: enhanced solubility, stability, and bioavailability. Molecules 25, 2791. doi:10.3390/molecules25122791
Zheng, C., Li, M., and Ding, J. (2021). Challenges and opportunities of nanomedicines in clinical translation. Bio Integr. 2, 57–60. doi:10.15212/bioi-2021-0016
Keywords: nanoparticles, nanomedicine, clinical trials, drug delivery, targeted therapy
Citation: Kumarasamy RV, Natarajan PM, Umapathy VR, Roy JR, Mironescu M and Palanisamy CP (2024) Clinical applications and therapeutic potentials of advanced nanoparticles: a comprehensive review on completed human clinical trials. Front. Nanotechnol. 6:1479993. doi: 10.3389/fnano.2024.1479993
Received: 13 August 2024; Accepted: 20 September 2024;
Published: 21 October 2024.
Edited by:
Mona Khafaji, Sharif University of Technology, IranReviewed by:
Maqsood Ur Rehman, University of Malakand, PakistanKatyayani (Katie) Tatiparti, Sanofi Genzyme, United States
Copyright © 2024 Kumarasamy, Natarajan, Umapathy, Roy, Mironescu and Palanisamy. This is an open-access article distributed under the terms of the Creative Commons Attribution License (CC BY). The use, distribution or reproduction in other forums is permitted, provided the original author(s) and the copyright owner(s) are credited and that the original publication in this journal is cited, in accordance with accepted academic practice. No use, distribution or reproduction is permitted which does not comply with these terms.
*Correspondence: Monica Mironescu, bW9uaWNhLm1pcm9uZXNjdUB1bGJzaWJpdS5ybw==; Chella Perumal Palanisamy, Y2hlbGxhLnBAY2h1bGEuYWMudGg=