- 1Laboratório de Bioquímica e Produtos Naturais LABINAT, Departamento de Zootecnia e Desenvolvimento Rural, Universidade Federal de Santa Catarina, Florianópolis, Brazil
- 2Departamento de Farmacologia, Centro de Ciências Biológicas, Universidade Federal de Santa Catarina, Florianópolis, Brazil
Nanoformulations with herbal actives for treating bovine mastitis present an alternative for controlling bacterial infections in the emerging scenario of antimicrobial resistance. In this study, we investigated macela (Achyrocline satureioides) nanoemulsion (NE-ML), a formulation developed for the treatment of bovine mastitis (registered under Brazilian patent application BR 10 2021 008630 0), in the context of its bactericidal mechanism(s) of action and potential synergism with commercial antimicrobials. The effect of NE-ML on the integrity and cell permeability of Staphylococcus aureus was evaluated by measuring the electrical conductivity of bacterial suspensions exposed to different concentrations of NE-ML and by assessing the release of cellular constituents. Damage to bacterial ultrastructures was analyzed by transmission electron micrographs. The synergism of NE-ML with beta-lactam antibiotics and aminoglycosides was evaluated by the checkerboard test method against S. aureus (n = 6). The relative electrical conductivity of the bacterial solution gradually increased over time, reaching high values after exposure to 1xMIC (52.3%) and 2xMIC (75.34%) of NE-ML. Total proteins were detected in the bacterial suspensions exposed to NE-ML, increasing in concentration over exposure time (p < 0.05). Through bacterial micrographs, we observed that exposure to NE-ML (1xMIC) affected the integrity of the plasma membrane with invaginations in the cytosolic region and alterations in the cell wall. The increase in NE-ML concentration resulted in greater damage to the ultrastructure of S. aureus with changes in bacterial cell division patterns. When NE-ML was combined with the beta-lactam antimicrobials, the interaction was indifferent, indicating no modulation of antimicrobial resistance. In contrast, when combined with the aminoglycoside, a synergistic interaction did occur. These general findings suggest that the bactericidal action of NE-ML begins in the plasma membrane, causing alterations in its permeability and integrity, and extends to the cell wall, cytoplasm, and cell division. Although synergy was restricted to the aminoglycoside by destabilizing the bacterial cell membrane, this suggests that NE-ML can induce the entry of other actives, potentially reducing their therapeutic doses. Understanding the mechanism of action of this new nanoformulation is certain to drive pharmacological advances, broaden the perspective of its in vivo use, and improve the treatment of bovine mastitis.
1 Introduction
Current agricultural production models and food systems are considered inadequate and unsustainable to meet societal demands in the face of the emerging global climate crisis (Rosati et al., 2021). The widespread use of antibiotics, which is considered a major contributor to the development of antimicrobial resistance, is another significant point observed in conventional production systems (Bezner Kerr et al., 2023). It is estimated that global sales of antimicrobials for intensive animal production will increase by approximately 11.5% by 2030 (Tiseo et al., 2020). Agroecological and organic production systems, as alternatives to the hegemonic model, are practices that seek to strengthen the long-term resilience of food systems and can thus be considered a counterpoint to the alarming situation (Gamage et al., 2023; Bezner Kerr et al., 2023). However, the use of drugs and other inputs to treat animals in organic and agroecological production systems is limited. In Brazilian organic production systems (MAPA, 2021), only a small number of substances are approved for the treatment of various animal diseases, including bovine mastitis. These limitations for the treatment of bovine mastitis in these livestock systems represent a significant challenge for maintaining animal welfare and certification of organic livestock products in the country and globally.
Bovine mastitis is a multifactorial disease affecting dairy cattle, characterized by inflammation of the bovine mammary gland (Ezzat Alnakip et al., 2014) and responsible for significant economic losses in the production sector (Hogeveen et al., 2011; Javed et al., 2022). Staphylococcus aureus is the main pathogen associated with cases of chronic and subclinical mastitis and is considered a contagious etiological agent (Fitzgerald, 2012), highly adaptive, opportunistic, and easily spread among animals during milking (Campos et al., 2022). It is also a facultative intracellular pathogen (Johns et al., 2015) that protects bacteria from defenses of the host immune system and from the action of low-penetration antibiotics in eukaryotic cells (Algharib et al., 2020). It can form biofilms (Vestby et al., 2020) that limit susceptibility to antimicrobial therapy due to low antibiotic diffusion (Amorena et al., 1999).
Antibiotic therapy is the main therapeutic approach in the management of intramammary infections in cattle (El-Sayed and Kamel, 2021). The cure rate for cases of subclinical mastitis during lactation can vary significantly, from 5% to more than 80% (Salat et al., 2008). Due to this variability, new treatment modalities, such as phage therapy (Varela-Ortiz et al., 2018), phage endolysins (Fenton et al., 2013), vaccines (Rainard et al., 2021), probiotic approaches (K. Wallis et al., 2018), stem cells (Singh et al., 2020), herbal medicines (Fiordalisi et al., 2018; Chen et al., 2019) and nanoparticles (Pinheiro Machado et al., 2019) have already been investigated and described as potential tools for the control of staphylococcal bovine mastitis.
The use of phytochemicals as therapeutic molecules via the intramammary route has become a promising biomimetic approach for bovine mastitis management, serving as a potential alternative to the use of antibiotics (Touza-Otero et al., 2024). The development of new antimicrobial agents that incorporate nanosizing the composition of therapeutic actives allows these formulations to become more efficient based on their ability to cross different barriers of the organism, their high stability and bioavailability, as well as their sustained release and lower toxicity (Harwansh et al., 2019; Youssef et al., 2019). These properties can alleviate some of the problems associated with low bacteriological cure rates of bovine mastitis, which result from low penetration and intracellular retention of antibiotics and non-diffusion of acidic antibiotics across the lysosomal membrane at extracellular or neutral cytoplasmic pH (Gruet et al., 2001).
In this sense, a nanoemulsion containing macela extract (Achyrocline satureioides) was developed (Machado et al., 2020; Machado, 2021) as an alternative product for controlling bovine mastitis in organic and agroecological production systems (registered under Brazilian patent application BR 10 2021 008630 0). Macela (A. satureioides Lam. DC.), family Asteraceae (Compositae), is a medicinal herb native to South America (Lorenzo et al., 2000; Ruffa et al., 2002), and its biological activity is related to the presence of flavonoids (Simões et al., 1988; Petrovick et al., 2010; Joray et al., 2013; Moresco et al., 2017; Souza et al., 2018; Bianchi et al., 2019; Martínez-Busi et al., 2019).
Previous studies on the development of the macela-nanoemulsion showed an average particle size of around 200 nm, monodisperse distribution (narrow size distribution) (PdI<0.2), encapsulation efficiency of the extract compounds in nanoparticles greater than 94%, and physical stability over 160 days at room temperature (Machado et al., 2020). It is characterized as a sustained release system for bioactive compounds with antimicrobial activity. Macela-nanoemulsion showed high activity against S. aureus with a minimum inhibitory concentration (MIC) of 125 μg/mL of crude extract, corresponding to 5% of the nanoemulsion (v/v). By showing a cytoprotective effect for bovine mammary epithelial cells (MAC-T) and high penetration capacity into the mammary gland in a Franz cell model (Pinheiro Machado et al., 2022), NE-ML was incorporated into nanocomposites based on natural polymers (flaxseed mucilage and k-carrageenan) for the development of an antimicrobial hydrogel aimed at intramammary therapy of bovine mastitis. During the process of developing and characterization of the nanoemulsion loaded with macela extract (A. satureioides) (Machado et al., 2020; Machado, 2021; Machado et al., 2023), different formulations were tested to identify the most efficient composition in terms of physicochemical stability and antimicrobial activity. Detailed information on the characterization of this nanoformulation can be found in Supplementary Material.
In this article, we investigate the nanoemulsion loaded with macela extract (A. satureioides) (NE-ML) in the context of elucidating the mechanism of bactericidal action against S. aureus and its potential for modulating resistance to beta-lactam and aminoglycoside antimicrobials. Within the permitted pharmacological options and the requirements of organic and agroecological production systems, this formulation is considered a promising candidate bactericide against bovine mastitis.
2 Material and methods
2.1 Bacterial strains and antibiotics
The bacterial strains were the standard strain S. aureus ATCC 25923 and S. aureus isolated from milk of animals with subclinical bovine mastitis (Kuhnen et al., 2021) abbreviated O1, P1, P2, G1, and G2. Antimicrobial susceptibility of the strains was tested against oxacillin (oxacillin sodium - Novafarma Indústria Farmacêutica Ltda), benzetacil (benzathine benzylpenicillin - Eurofarma Laboratórios S.A.) and Gentatec® Mastite (gentamicin - Chemitec).
2.2 Preparation of macela-nanoemulsion
The macela extract was obtained from macerating the plant inflorescences in 80% ethanol (1:60, w/v), and the product was vacuum-filtered. The solvent was then removed in a rotary evaporator according to Machado et al. (2020). The aqueous phase of the crude extract was used to prepare a pre-emulsion containing golden flaxseed oil (Linum usitatissimum L.) and polysorbate 80 (Tween 80). The nanoemulsion was prepared using a high-pressure homogenizer (Homolab A10, Alitec, Brazil) following protocols developed by Machado (2021).
2.3 Antimicrobial activity of macela-nanoemulsion
The antimicrobial activity of NE-ML against S. aureus ATCC 25923 was evaluated using the broth microdilution method (CLSI, 2018) with modifications. This method allowed establishing the minimum inhibitory concentration (MIC), i.e., the lowest concentration of the product capable of reducing bacterial growth by 80% or more. The concentration range tested for NE-ML ranged from 40% to 0.31% (v/v), equivalent to 1,000 to 7.8 μg/mL of nanoencapsulated macela extract. The final bacterial inoculum density corresponded to 105 CFU/mL−1. Positive controls for bacterial growth and sterility of NE-ML, and Mueller Hinton Broth (MHB) were included. The microplate was incubated at a temperature of 37°C for 18–24 h in an incubator (SL-100 Solab). The MIC was visually confirmed by adding resazurin dye (100 μg/mL−1) (Sarker et al., 2007). The assays were performed in triplicate.
2.4 Electrical conductivity of bacterial suspensions
Alteration of bacterial membrane permeability by NE-ML was evaluated through the electrical conductivity of the bacterial membrane (Diao et al., 2014). A bacterial suspension of S. aureus ATCC 25923 of approximately 1.8–2.5 × 107 CFU/mL−1 was centrifuged (High-Speed Centrifuge 5430 R, Eppendorf). The bacterial pellet was washed with 5% glucose until its electrical conductivity was equivalent to that of a pure 5% glucose solution, becoming isotonic. Isotonic bacteria were exposed to NE-ML concentrations of 1xMIC and 2xMIC (hereinafter referred to as L1 and L2, respectively), and the control group of isotonic bacteria without NE-ML (L0) was treated with boiling water for 5 min. 5% glucose solutions were treated with the addition of NE-ML at concentrations of 1xMIC and 2xMIC. The L0, L1, and L2 treatments were incubated at a temperature of 37°C, and their electrical conductivities were measured with a digital conductivity meter (Gehaka CG 1800) every 30 min over 3 h. Bacterial membrane permeability was expressed as Relative Electrical Conductivity (%) and calculated as (%) = 100 × (L2 − L1)/L0 (Diao et al., 2014). The assays were performed in triplicate.
2.5 Integrity of cell membrane
The integrity of the bacterial cell membrane was assessed by UV-VIS spectrophotometry. An initial screening allowed the detection of macromolecules, such as nucleic acids and proteins, released in S. aureus ATCC 25923 suspensions treated with NE-ML (adapted from Diao et al., 2014). Bacterial suspensions of approximately 1.8–2.5 × 107 CFU/mL−1 were centrifuged (High-Speed Centrifuge 5430 R, Eppendorf), the supernatant discarded, and the pellet resuspended in 0.1 M phosphate-buffered saline (PBS buffer). The 1xMIC and 2xMIC concentrations of NE-ML were added to these suspensions. A bacterial solution in 0.1 M PBS buffer without NE-ML was used as control (C). The treatments were incubated at a temperature of 37°C, and after 2 and 4 h, aliquots were collected and centrifuged, followed by evaluation of the supernatant in a UV-VIS spectrophotometer (Bel UV-M51) at 260 and 280 nm. After finding the absorbent material, to determine the concentration of total proteins in the suspensions, new bacterial suspensions were prepared under the same incubation conditions in 0.1 M PBS buffer, with incubation times of 2 and 4 h and a temperature of 37°C both for the control suspensions without addition of NE-ML and for the treatments with the addition of 1xMIC and 2xMIC concentrations of NE-ML. Using both Bradford assay (Bradford, 1976) by incubation in a microplate reader and Coomassie Brilliant Blue BG-250 dye at 595 nm in a UV-VIS spectrophotometer, the concentration of total proteins was determined. The standard curve was determined from a bovine serum albumin (BSA) solution (1 mg/mL−1). The assay was performed in triplicate.
2.6 Effect on bacterial ultrastructure
Alterations in the ultrastructure of S. aureus ATCC 25923 after exposure to NE-ML were confirmed through transmission electron microscopy (TEM). Concentrations of 1xMIC and 2xMIC of NE-ML were added to bacterial suspensions (1.8–2.5 × 107 CFU/mL−1) (adapted from Diao et al., 2014), and a bacterial suspension without NE-ML addition was used as a control group. The treatments were incubated at 37°C, and after 2 and 4 h, aliquots were centrifuged (High-Speed Centrifuge 5430 R, Eppendorf), followed by washing the bacterial pellet with 0.1 M PBS buffer. The pellets were fixed with Karnovsky solution and then washed with 0.1 M Sodium Cacodylate Buffer (SCB). Post-fixation was carried out with a solution composed of osmium tetroxide (1%), potassium ferrocyanide (8%), and calcium chloride (5 mM) in 0.1 M Sodium Cacodylate Buffer (SCB), followed by another wash with 0.1 M SCB. Dehydration of the samples was carried out with increasing concentrations of acetone (30%, 50%, 70%, 90%, and 100%), and infiltration into epoxy resin occurred slowly (Embed-812, Electron Microscopy Sciences). The complete polymerization of the samples occurred at a temperature of 60°C for 48–72 h. The samples were cut using an ultramicrotome and contrasted with 2% uranyl acetate and lead citrate for subsequent analysis in a transmission electron microscope (TEM 100 kV - JEM-1011) (de Souza, 2011; Pergo et al., 2019). The assays were performed in triplicate.
2.7 Modulation of antimicrobial resistance
The checkerboard test allowed the evaluation of the modulatory resistance capacity of NE-ML associated with commercial antibiotics (Humphries, 2016). Initially, the MIC of NE-ML and beta-lactam antimicrobials (oxacillin and penicillin) and aminoglycosides (gentamicin) was determined using the broth microdilution method (CLSI, 2018). Staphylococcus aureus ATCC 25923 and strains O1, P1, P2, G1, AND G2 were selected based on their antimicrobial resistance profile (Kuhnen et al., 2021). Following the determination of the MIC of NE-ML and commercial antimicrobials for S. aureus strains, their concentration ranges were determined for the assay (Table 1).
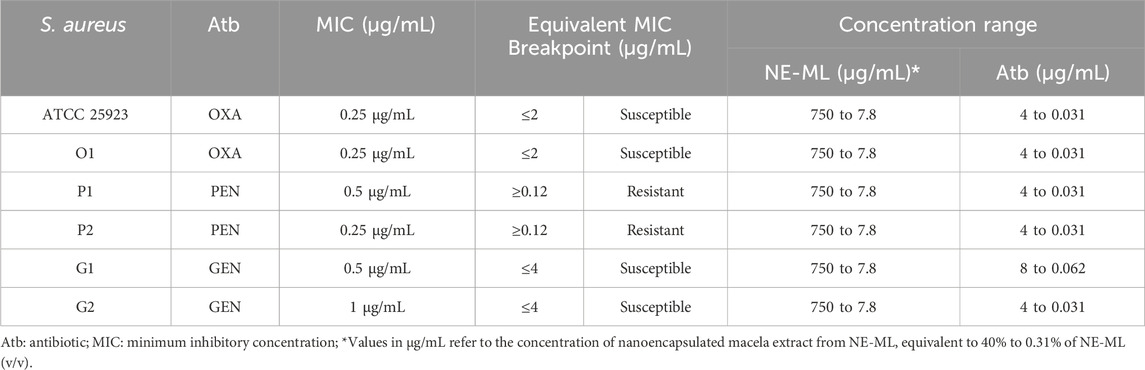
Table 1. MIC, antimicrobial sensitivity profile (CLSI, 2019), and concentration range of antimicrobials used in the checkerboard test against Staphylococcus aureus strains.
For the checkerboard test (Humphries, 2016), a 96-well microplate was used, allowing different dilutions of two antimicrobials simultaneously (Bonapace et al., 2002; Orhan et al., 2005). The highest concentrations of each product were positioned in opposite corners of the microplate. The final bacterial inoculum corresponded to a bacterial density of 105 CFU/mL−1. The microplate was incubated at 37°C for 18–24 h (Bonapace et al., 2002; Orhan et al., 2005). The addition of resazurin dye visually confirmed bacterial growth, allowing for result interpretation based on the bacterial growth and growth inhibition interface (Sarker et al., 2007). The assay was performed in duplicate.
The checkerboard assays resulted in a ΣFIC index (fractional inhibitory concentration index) (Humphries, 2016) per well tested on the microplate and calculated by comparing the MICs of the agents used in combination with the MICs of these agents alone. Therefore, ΣFIC = FICA + FICB, where FICA = MIC of agent A in combination/MIC of agent A alone, and FICB = MIC of agent B in combination/MIC of agent B alone. Each well tested in the microplate resulted in a ΣFIC index. After determining the bacterial growth and non-growth interface, the well with the lowest ΣFIC index among all wells without bacterial growth at the interface was used to determine the modulatory result as synergistic (≤0.5), indifferent (>0.5 ≤ 4) or antagonistic (>4) (Humphries, 2016). This methodology is called Lowest FIC Index (Lowest Fractional Inhibitory Concentration Index) (Humphries, 2016).
3 Statistical analysis
One-way analysis of variance (ANOVA) and Tukey tests were carried out to determine significant differences (p < 0.05) between the means using Microsoft EXCEL.
4 Results and discussion
4.1 Cell membrane permeability
An alteration in the electrical conductivity of the bacterial solution treated with 1xMIC and 2xMIC was detected (Figure 1).
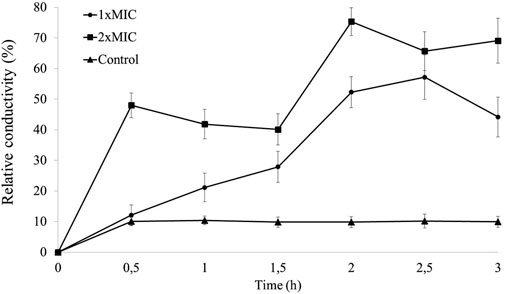
Figure 1. Determination of the relative electrical conductivity (%) of Staphylococcus aureus ATCC 25923 suspensions treated with NE-ML over 3 h of exposure.
The 1xMIC of NE-ML against S. aureus ATCC 25923 was confirmed to be 5% (v/v), equivalent to 125 μg/mL of encapsulated macela extract, as described by Machado et al. (2020). Therefore, the MIC of 2xMIC was 10% (v/v), equivalent to 250 μg/mL of encapsulated macela extract.
While the control group (isotonic S. aureus without NE-ML treated with boiling water) maintained stable relative conductivity over time (between 9.8% and 10.4%), the NE-ML-treated groups showed a considerable increase in relative electrical conductivity in the initial minutes of exposure. The 1xMIC group exhibited a gradual increase in relative electrical conductivity, peaking at 2.5 h of exposure (57.19%). The 2xMIC group showed a steep initial increase at 0.5 h (48.02%) and higher relative conductivity at 2 h of exposure (75.34%). These results suggest that the permeability of the S. aureus cell membrane was altered after treatment with NE-ML, causing the leakage of intracellular electrolytes into the culture medium, as expressed by an increase in the conductivity values of the bacterial suspensions.
The change in membrane permeability observed seems to be dependent on the concentration of the nanoformulation because we found that an increase in the concentration of NE-ML was correlated with an increase in relative electrical conductivity from 12.18% to 48.02%, an increase of about four times. Similar results were found by Wei et al. (2023) when evaluating the activity of madecassic acid isolated from Centella asiatica on the cell membrane of S. aureus. This increase in relative electrical conductivity over time is considered an indicator of changes in the permeability of the plasma membrane. Relatively small changes can negatively affect metabolism and lead to cell death. If plasma membrane permeability is affected, intracellular electrolytes will leak into the culture medium, and the conductivity of the supernatant will reflect it (Wei et al., 2023).
The viscosity of the plasma membrane directly influences its associated functions, including passive permeability of hydrophobic molecules, active solute transport, and protein-protein interactions (Zhang and Rock, 2008). Therefore, it is essential that the membrane remain intact for the performance of these functions without causing metabolic dysfunction (Hartmann et al., 2010) and subsequent loss of cellular functionality (Pérez-Peinado et al., 2018).
The plasma membrane delimits the cytoplasm and controls the diffusion of small molecules and secreted proteins between intracellular and extracellular spaces (Strahl and Errington, 2017), acting as a regulatory barrier to the passage of small intracellular ions that influence bacterial membrane potential. Intracellular potassium contributes to the maintenance of cell turgor and membrane potential, as well as pH homeostasis, in addition to influencing enzymatic activity (Stautz et al., 2021). An increase in conductivity indicates an increase in bacterial membrane permeability, leading to the leakage of intracellular constituents, such as the electrolytes K+, Ca2+, and Na+ (Li and Yu, 2015).
4.2 Cell membrane integrity
Mean values of optical densities were obtained from screening for the detection of absorbing material which at 260 and 280 nm is indicative of the loss of macromolecules, such as nucleic acids (Lucena-Aguilar et al., 2016) and proteins (Stoscheck, 1990), resulting from the alteration of plasma membrane integrity of S. aureus ATCC 25923 treated for 2 and 4 h with NE-ML (Table 2).
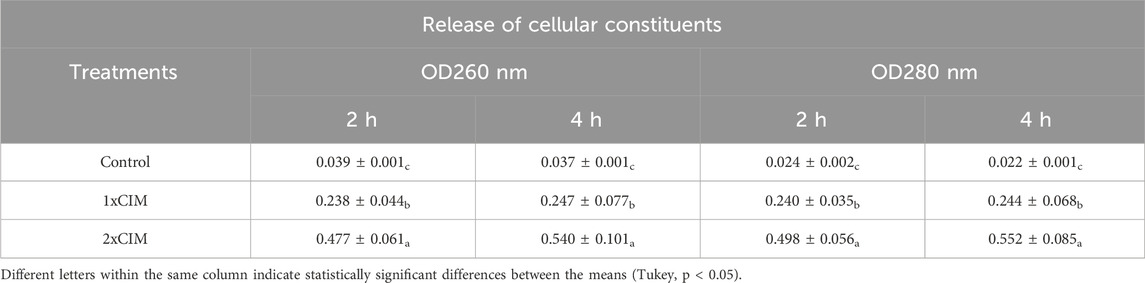
Table 2. Detection of absorbing material at 260 and 280 nm in suspensions of Staphylococcus aureus ATCC 25923 treated with NE-ML for 2 and 4 h.
Low optical density values at 260 nm and 280 nm were found in the control group, suggesting that the bacterial cell was intact in the culture conditions. However, in the groups treated with NE-ML (1xMIC and 2xMIC), a significant increase in the optical density of the bacterial suspensions was observed. By increasing the concentration of NE-ML from 1xMIC to 2xMIC, higher optical density values occurred in bacterial suspensions, in turn indicating a greater release of macromolecules with the increase in product concentration. Following the detection of absorbent material at 260 and 280 nm, total protein was performed in the bacterial suspension.
In this assay, the bacterial suspension treated with 1xMIC resulted in an increase of total protein content from 97.15 μg/mL at 2 h of exposure to 138.63 μg/mL after 4 h (Figure 2). In the group treated with 2xMIC, the content increased from 196.41 μg/mL to 231.96 μg/mL over the same period of exposure to NE-ML. Compared to the control group, 1xMIC and 2xMIC groups at both time points (2 and 4 h) showed a significant difference in total protein concentration (μg/mL) (p < 0.05). These results indicate damage to the plasma membrane integrity of the S. aureus ATCC 25923 in proportion to the concentration of NE-ML in the culture medium.
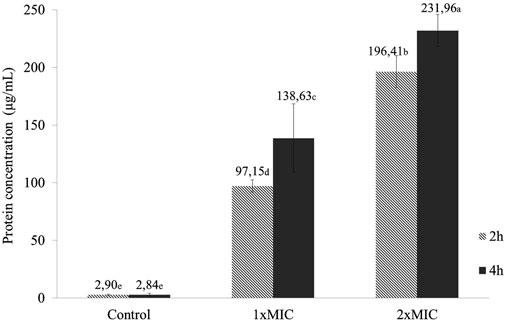
Figure 2. Total protein content (μg/mL) of Staphylococcus aureus ATCC 25923 suspensions exposed for 2 and 4 h to NE-ML and control group. Different letters indicate statistically significant differences between the means (Tukey, p < 0.05).
Similar studies that evaluated the antimicrobial activity of cinnamon essential oil against S. aureus also detected the release of total proteins in the culture medium. The authors observed that the protein concentration increased over time and in response to the increase in cinnamon essential oil concentration. The presence of these cellular constituents in the culture medium possibly indicates damage to and alteration in plasma membrane integrity (Zhang et al., 2016).
Some bioactive compounds present in the macela extract of the nanoemulsion under study, e.g., the flavonoids quercetin and luteolin, have been associated with structural damage responsible for the loss of bacterial membrane function. Similarly, the flavonoids epicatechin, quercetin and its glycosides extracted from white guava leaves also exhibited bacteriostatic activity against S. aureus through mechanisms that disrupt the structure and function of the bacterial cell membrane (Zhang et al., 2020).
4.3 Effect of macela-nanoemulsion on the ultrastructures of Staphylococcus aureus
Transmission electron micrographs revealed alterations in the ultrastructure of S. aureus ATTC 25923 owing to the action of NE-ML. Bacteria from the control group not exposed to NE-ML (Figure 3) exhibit uniform characteristics with intact and well-defined cell walls and plasma membranes. The relief of the cell wall showed a rough to smooth characteristic. The plasma membrane appeared as an electron-dense layer, just below the cell wall. It was also possible to observe the cytosol containing bacterial genetic material clustered in the central region of the cells with electron-dense and electron-transparent areas. Cells undergoing division displayed standard septation characteristics in different phases with the presence of initial septa up to the formation of the complete transverse wall. These ultrastructure characteristics are consistent with the description of control S. aureus in other investigations (Santhana Raj et al., 2007; Grigor’eva et al., 2020).
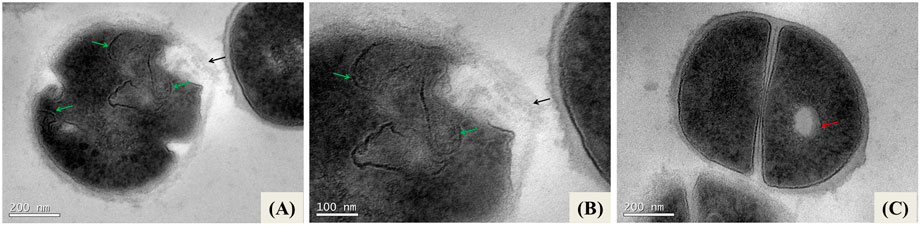
Figure 3. Ultrathin sections of Staphylococcus aureus ATCC 25923 control cells not exposed to NE-ML and incubated for 4 h in 0.1 MPBS buffer at 37°C. Black arrow indicates the cell wall (A); white arrow indicates the plasma membrane (A); green arrow indicates the nuclear/cytosolic region (A); orange arrow indicates the beginning of cell division septum formation (A); blue arrow indicates formation of the transverse wall in dividing cells (C). Bacterial cell with intact morphological features (B).
However, bacteria exposed to NE-ML concentrations for 2 h in 0.1 M PBS buffer at 37°C show structural alterations which are intensified with the increase in NE-ML concentration from 1xMIC to 2xMIC (125 and 250 μg/mL of nanoencapsulated macela extract, equivalent to 5% and 10% of NE-ML v/v, respectively). From ultrathin sections of S. aureus ATCC 25923 exposed to 1xMIC of NE-ML for 2 h (Figure 4), we observed changes in the uniformity of cytosolic density with areas of electron-dense and electron-transparent areas. Cells exhibit expression of cell wall regions, and many areas of the plasma membrane lose their conformity. It is also possible to observe well-defined and pronounced electron-transparent regions (hereinafter referred to as electron-transparent vacuoles).
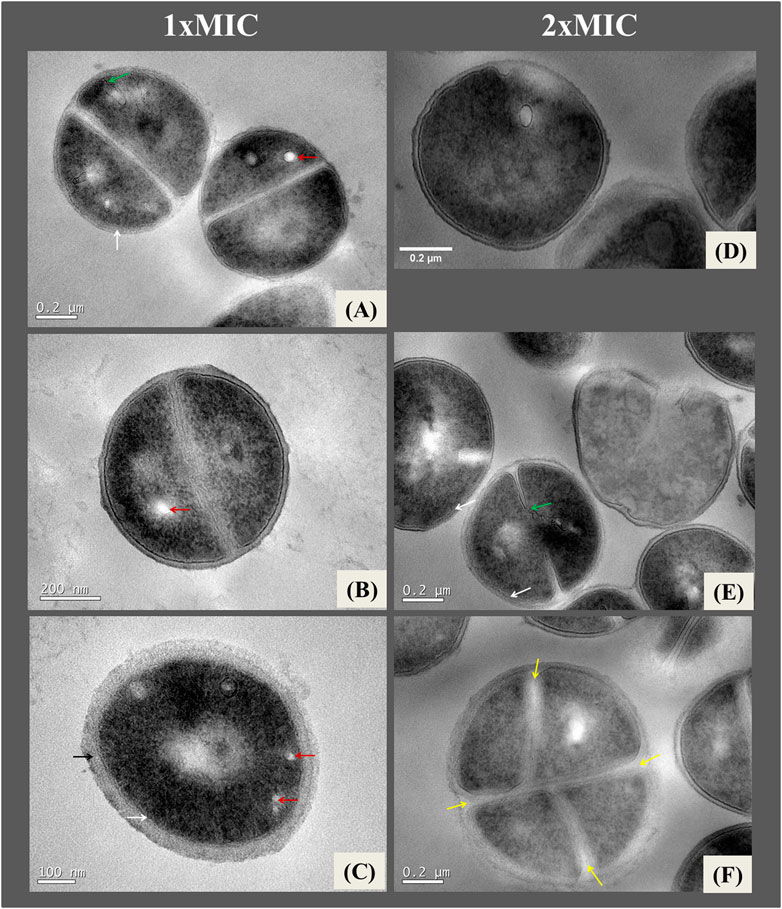
Figure 4. Ultrathin sections of Staphylococcus aureus ATCC 25923 exposed to 1xMIC (A–C) and 2xMIC (D–F) of NE-ML and incubated for 2 h in 0.1 M PBS buffer at 37°C. Black arrows indicate thickening of the cell wall; white arrows indicate loss of definition of the plasma membrane; red arrows indicate electron-transparent regions (vacuoles); green arrows indicate invaginations of the plasma membrane into the bacterial intracellular region; yellow arrows indicate changes in the pattern of cell division.
When increasing exposure of the bacteria to 2xMIC of NE-ML for 2 h (Figure 4), it is possible to observe a greater presence of electro-transparent vacuoles in the bacterial cells. Changes in the plasma membrane intensify and show invaginations towards the intracellular region. It is also possible to observe alterations in the bacterial cell division pattern. The cell wall continues to thicken, and the cell membrane loses structure and definition, denoting significant cell deformation compatible with lysis.
Figure 5 illustrate changes in bacteria exposed to the nanoformulation for 4 h at concentrations of 1xMIC and 2xMIC of NE-ML in 0.1 M PBS buffer solution at 37°C. In cells treated with 1xMIC of NE-ML after 4 h (Figures 5A–C), invaginations of the plasma membrane and some areas of the cell wall with loss of structure and definition were found (Figures 5A, B). The cells began to show a very pronounced electron-transparent area in the central region of the cytosol (Figure 5C). Increasing the concentration of NE-ML to 2xMIC for 4 h (Figures 5D–I) led to an increase in cell density, along with darkening of the cytosol region. The cells continued to show invaginations of the cell membrane (Figures 5D, E). The cells began to show damage and disorganization in the cytoplasm with several amorphous and electron-dense clusters within the entire intracellular structure much more pronounced when compared to cytoplasmic damage and disorganization of bacteria exposed for 2 h (Figure 5I).
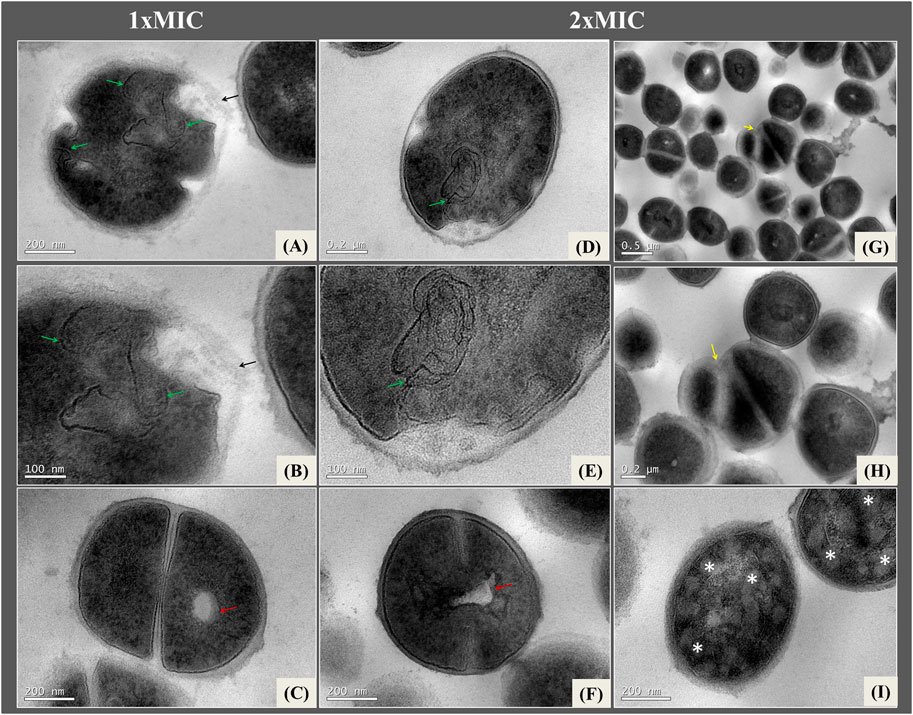
Figure 5. Ultrathin sections of Staphylococcus aureus ATCC 25923 exposed to 1Xmic (A–C) and 2xMIC (D–I) of NE-ML and incubated for 4 h in 0.1 M PBS buffer at a temperature of 37°C. Red arrows indicate electron-transparent vacuoles in the cytoplasm; green arrows indicate plasma membrane invaginations; black arrows indicate loss of cell wall definition; yellow arrows indicate changes in cell division pattern that are misaligned with eletron-transparent material; * indicate cells with disorganization in the intracellular region and electron-transpartent areas in the cytoplasm.
We can also observe that the electron-transparent vacuole in the central region of the cell appears more pronounced after 4 h of exposure to NE-ML (Figure 5F). Some areas of the plasma membrane lose definition. In the group treated with 2xMIC of NE-ML, more pronounced changes and disorganization in the cell division pattern were also noticeable. Some daughter cells lose their structure, and the cell division septa are misaligned and have low-density material (Figures 5G, H), indicating that the areas of septal formation are affected by NE-ML.
Micrographs of S. aureus exposed to the nanoformulation revealed significant structural alterations which became more pronounced with increasing concentration and exposure time, as indicated by the thickening of the cell wall and pronounced deformities in regions of the plasma membrane. Results of the release of cellular constituents and electrical conductivity of bacterial solutions exposed to different concentrations of NE-ML may be recalled. When combined, these findings suggest that the mechanism of action of the macela nanoformulation involves altering the integrity of the plasma membrane and increasing its permeability. These structural changes are responsible for the leakage of electrolytes and intracellular constituents, compromising essential bacterial components.
Other investigations have shown that such products as antimicrobial peptides like gramicidin S (GS) and gramicidin S (GS) and PGLa (peptidyl-glycylleucine-carboxyamide) interact with the bacterial membranes of S. aureus, increasing their permeability and destabilizing them with a decrease in the ability of bacteria to regulate turgor pressure in low ionic strength media (Hartmann et al., 2010). The formation of mesomeric structures surrounded by intracellular membranes was also observed, suggesting that this alteration occurs as an adaptation to the increased cell membrane area after binding to the antimicrobial peptide. These effects are similar to those observed in bacteria treated with gentamicin antibiotics which can bind to ribosomes and produce defective proteins, leading to bacterial death. Gentamicin affects the function of the cell membrane, resulting in the leakage of sodium, potassium, amino acids, and other cellular constituents (Anandabaskar, 2021).
Exposure to NE-ML may have also disrupted crucial cellular processes, such as DNA replication, chromosomal segregation, and enzymatic activity involved in peptidoglycan synthesis, all of which are essential for bacterial cell formation and division. It is not yet fully understood how the effects of many antibiotics can inhibit molecular processes that result in cell death. A growing body of evidence suggests that bacterial death occurs through highly complex and regulated mechanisms, very similar to the apoptosis mechanism in eukaryotic cells (Bayles, 2007). Some studies indicate that autolysis of S. aureus may occur in biofilm environments, possibly regulated by the cidABC and lrgAB operons, but the mechanisms are still poorly understood (Sadykov and Bayles, 2012).
We cannot assert that exposing bacteria to NE-ML directly stimulates the bacterial autolysis process. However, this hypothesis requires further investigation, especially through chromosomal analyses that assess the impact of NE-ML on these structures. Therefore, it is possible to indicate potential damage caused by NE-ML. This is relevant because, in a previous assessment of NE-ML against S. aureus MRSA biofilms in vitro (Machado et al., 2020), it was possible to reduce over 64% of the cellular mass, disrupting the integrity of the biofilm structure. Additionally, NE-ML demonstrated the ability to remove previously formed biofilm over 48 h.
We also observed that increasing the concentration of NE-ML extended cell damage to the cytosol which was marked by density alteration and the presence of electron-dense vacuoles, as well as altered bacterial division process, as observed by the presence of disorganized daughter cells and altered septation patterns. For bacteria to proliferate and ensure cell division, they need to go through consecutive cycles of growth and division, coordinating DNA replication, segregation of new chromosomes, and synthesis of new cell walls until completing cell division. In S. aureus, DNA replication, and peptidoglycan synthesis involve proteins and protein complexes that play a crucial role in regulating these processes (Barbuti et al., 2023). The initial phases of bacterial cell growth and division are marked by septation, a process that involves a coordinated invagination of the cytoplasmic membrane, peptidoglycan, and cell wall into the cytoplasm in the centripetal region of the bacterial cell (Paul et al., 1995; Touhami et al., 2004). In normal bacterial cells, the cell wall with the peptidoglycan of the mother cell remains external to the cell, while a new peptidoglycan wall grows in the septal region to the daughter cells. The lipid membrane defines the edges of the septal plate, while the peptidoglycan material of daughter cells forms the external cell wall, merging with the peptidoglycan of the mother cell’s external wall during septum formation (Erickson, 2017).
4.4 Modulation of the antimicrobial activity of beta-lactam antibiotics and aminoglycosides in association with macela-nanoemulsion
According to the MIC breakpoint (µg/mL) (CLSI, 2019), we obtained bacterial profiles sensitive to oxacillin, resistant to penicillin, and sensitive to gentamicin. The sensitive category refers to an infection that can be treated with the recommended antibiotic dose for the type of infection and infecting species, while strains considered resistant are not inhibited by achievable systemic concentrations of antimicrobials in normal therapeutic regimens (CLSI, 2018). Penicillin-resistant bacterial strains were selected to investigate the modulatory capacity of antibiotics in combination therapy with NE-ML as a potential way to reverse bacterial resistance. The individual and combined MIC results of the tested antimicrobials, which are needed to calculate the ΣFIC index, are presented in Table 3.
Analysis of these data allowed us to determine whether NE-ML combined with oxacillin, penicillin, and gentamicin against S. aureus resulted in synergy, indifference, or antagonism (Table 4).
Most of the combinations tested between NE-ML and the different antibiotics resulted in interactions categorized as indifferent (ΣFIC >0.5 and ≤4). These interactions are so named because the effect of the tested combination is equal to the effect of the most active antimicrobial (EUCAST, 2000). The wild-type S. aureus strains studied (O1, P1, P2, G1, and G2) may exhibit genomic diversity, responding differently to antimicrobial action. Although synergistic interactions are most desired in the clinical context for combined therapy application (Yang et al., 2017), with a significant reduction in used concentrations, indifferent interactions can be valuable for preserving the effectiveness of existing antibiotics. Some antibiotics may present undesirable toxic effects, but when included in a combined therapy system, the degree of side effects could be reduced, while, at the same time, maintaining good antimicrobial activity (Wang et al., 2022). Although the mechanisms of resistance to combined therapy are complex at a population level (Sullivan et al., 2020), combined antimicrobial therapy can also be considered to minimize or block antimicrobial resistance mechanisms and broaden the spectrum of drug activity (Dhanda et al., 2023).
A synergistic interaction between NE-ML and gentamicin was found (G2, ΣFIC 0.31 ± 0.00). In synergism, the effects of the combination exceed the sum of the effects of the individual compounds (EUCAST, 2000). Here, we observed a 16-fold decrease in the concentration of NE-ML, from 125 μg/mL (MIC alone) to 7.8 μg/mL (MIC in combination), and a 4-fold decrease in the concentration of gentamicin, from 1 μg/mL (MIC alone) to 0.25 μg/mL (MIC in combination). Synergy is less common than other antimicrobial interactions because it may be related to different mechanisms of action affecting different targets in bacterial cellular components. It can also be related to specific genes, gene sets, or regulatory elements (Sullivan et al., 2020).
Synergistic interactions between different antibiotics can occur when they target the same cellular process by inhibiting a metabolic pathway in two subsequent steps, either when antibiotics physically interact by binding to the same target, or when antibiotics target different cellular processes, and one modulates the cellular uptake of the other. This modulation of drug uptake and efflux by bacteria is directly or indirectly conditioned by another drug, acting at different sites, i.e., membrane pores, efflux pumps, membrane potential, proton motive force (PMF), the force that moves protons across membranes against their concentration gradient, from areas of higher concentration to areas of lower concentration, and outer membrane composition (Roemhild et al., 2022). The bioavailability modulation model emerged as a concept in the 1960s, and it holds that the activity of one antimicrobial allowed the increase in the concentration of the other (Sullivan et al., 2020). For example, it has been observed that colistin, when binding to lipid A, causes cracks in the membrane and induces increased intracellular accumulation of other antibiotics, including rifampicin (Brennan-Krohn et al., 2018).
Throughout this investigation, we gathered evidence that led us to identify that the mechanism of action of NE-ML is related to the bacterial plasma membrane and that damage subsequently extends to other cellular structures, i.e., cytosol, cell wall, as well as bacterial cell division. These events result in the disruption of bacterial homeostasis with significant alteration of permeability and cellular integrity, as observed through electrolyte and macromolecular constituent leakage. Thus, the synergistic activity between NE-ML and gentamicin, as characterized by the use of lower doses of both antimicrobials, can be explained by uptake modulation. The action of NE-ML on the plasma membrane allowed gentamicin to increase uptake into the bacterial cytoplasm by altering its integrity and permeability, resulting in its action on intracellular targets with a lower concentration. Aminoglycoside antimicrobials, like gentamicin, irreversibly bind to the 30s ribosomal unit, affect mRNA translation, and lead to the incorporation of incorrect amino acids into the polypeptide chain. This results in the production of defective proteins, which play an important structural role essential for bacterial function and viability (Spinosa et al., 2017).
One of the main challenges in the search for new antimicrobials is related to the low cellular permeability of antibiotics (Murugaiyan et al., 2022). The development of drug administration or delivery systems that aim to improve this aspect, facilitating the entry of the antimicrobial into the bacterial intracellular space, proves to be a promising approach to overcoming this obstacle. Owing to their size, adaptability and stability in physiological fluids, nanostructured systems, e.g., metal-based nanoparticles, polymers, liposomes, or carbon-based, represent an option for transporting antimicrobials to hydrophilic and hydrophobic drug molecules (Pham et al., 2019). NE-ML was physically characterized as having an average particle size around 200 nm, monodisperse distribution (narrow size distribution) (PdI<0.2), encapsulation efficiency of extract compounds in nanoparticles exceeding 94%, and physical stability over 160 days at room temperature (Machado et al., 2020). Furthermore, nanoparticles also exhibit the ability to inhibit biofilm formation (Mu et al., 2016), another major problem associated with controlling infections caused by S. aureus.
It is important to highlight a significant characteristic of nanoparticle application in the therapeutic treatment of subclinical bovine mastitis, often caused by intracellular infections of S. aureus. Some classes of antibiotics have limited efficacy against intracellular pathogens because of low intracellular accumulation in eukaryotic cells, such as beta-lactams and aminoglycosides, or because of weak retention inside cells, like macrolides and fluoroquinolones (Abed et al., 2015). On the other hand, nanoparticles transporting antimicrobial molecules into the interior of eukaryotic cells can be taken up by phagocytic cells, resulting in a decrease in intracellular bacterial count (Abed et al., 2015). NE-ML has demonstrated the ability to inhibit biofilm formation by planktonic bacteria with a mass reduction of over 64% (biofilm MIC of 25% v/v), eradication capacity with a mass reduction of over 73% (minimum biofilm eradication concentration - MBEC, of 80% v/v), as well as the ability to remove previously formed biofilm over 48 h (Machado et al., 2020).
Nanostructured systems, such as the NE-ML, are considered effective nanocarriers, enhancing the stability, protection, and bioavailability of the nanoencapsulated compounds. They also increase penetration, solubility, dissolution, and permeation in the biomembrane (Harwansh et al., 2019). The antimicrobial activity of plant extracts, especially those rich in phenolic compounds like flavonoids and their derivatives, can occur through various mechanisms, including inhibition of function, porins inhibition, inhibition of nucleic acid synthesis, inhibition of energy metabolism, and inhibition of attachment and biofilm formation (Xie et al., 2015; Yuan et al., 2021).
The association of antimicrobial activity with changes in permeability and cell membrane rupture, leading to leakage of intracellular content (Álvarez-Martínez et al., 2021), has been explored and linked to the presence of hydroxyl groups at specific locations on aromatic rings (Xie et al., 2015). This characteristic can significantly alter membrane permeability and subsequent affinity with external and internal binding sites in bacteria.
Flavonoid compounds are the primary class of polyphenols by having over 8,000 described molecules and different biological activities. The major phytochemical compounds isolated from macela inflorescences (A. satureioides) and incorporated into the nanoemulsion under study (NE-ML) are 3-O-methylquercetin (187, 3 ± 0.1 µg/mL−1), achyrobichalcone (ACB) (155.4 ± 11.6 µg/mL−1), quercetin (76.3 ± 0.1 µg/mL−1) and luteolin (30.4 ± 0.0 µg/mL−1) (Machado et al., 2020). The antimicrobial action mechanisms of these compounds are not fully elucidated, but it is known that phenolic compounds are hydrophobic in nature and are therefore considered to be active on the membrane (Álvarez-Martínez et al., 2021). Interaction with the lipid bilayer can modify the function of the plasma membrane, leading to the formation of small pores that alter its permeability and fluidity. This can result in the displacement of lipids and proteins, altering the electrical potential and polarity of the bacterial membrane. These changes may be responsible for significant damage, including membrane and cell wall rupture, disruption of DNA and RNA synthesis and function, and disturbance of normal cell communication, leading to inhibition of biofilm formation (Basavegowda and Baek, 2022).
5 Conclusion
Nanoformulations have emerged as a promising strategy for molecule delivery owing to their nanometric size and physical characteristics. The crude macela extract is a complex matrix, primarily composed of flavonoid compounds, such as 3-O-methylquercetin, quercetin, luteolin and achyrobichalcone, responsible for its antimicrobial activity. We confirmed that the formulation acts on the cellular plasma membrane, resulting in alterations in its permeability and integrity. Its adjacent effects include changes in cytosolic content and cell wall, as well as impact on the bacterial cell division process. Another important point to highlight was its synergism when combined with the antimicrobial gentamicin. Besides demonstrating itself to be an efficient delivery system for phytochemical molecules, this macela nanoformulation offers a promising perspective regarding its potential to enhance the absorption of other drugs within bacterial cells.
Data availability statement
The raw data supporting the conclusions of this article will be made available by the authors, without undue reservation.
Author contributions
RR: Conceptualization, Data curation, Formal Analysis, Investigation, Methodology, Project administration, Validation, Visualization, Writing–original draft, Writing–review and editing. BR: Investigation, Methodology, Validation, Writing–review and editing. LH: Conceptualization, Writing–review and editing. SK: Conceptualization, Funding acquisition, Project administration, Resources, Supervision, Visualization, Writing–review and editing.
Funding
The author(s) declare that financial support was received for the research, authorship, and/or publication of this article. This study was funded by CNPq (Edital 39/2013, 403415/2013-6). The authors would like to thank the UNIEDU/FUMDES/ Postgraduate Scholarship Program (471/SED/2021) for providing a university scholarship.
Acknowledgments
The authors would like to thank the LCME-UFSC for technical assistance during the electron microscopy work; Bruna Marcon and the Confocal and Electron Microscopy Platform at the Instituto Carlos Chagas/Fiocruz-PR, Brazil, for assistance and processing of samples for microscopy; Professor Elenara Lemos Senna of the Department of Pharmaceutical Sciences (CCS/UFSC) for providing the high pressure homogenizer equipment; and NanoScoping - Solutions in Nanotechnology Ltda (Florianópolis, Brazil) for processing the nanoemulsion.
Conflict of interest
The authors declare that the research was conducted in the absence of any commercial or financial relationships that could be construed as a potential conflict of interest.
Publisher’s note
All claims expressed in this article are solely those of the authors and do not necessarily represent those of their affiliated organizations, or those of the publisher, the editors and the reviewers. Any product that may be evaluated in this article, or claim that may be made by its manufacturer, is not guaranteed or endorsed by the publisher.
Supplementary material
The Supplementary Material for this article can be found online at: https://www.frontiersin.org/articles/10.3389/fnano.2024.1466988/full#supplementary-material
References
Abed, N., Saïd-Hassane, F., Zouhiri, F., Mougin, J., Nicolas, V., Desmaële, D., et al. (2015). An efficient system for intracellular delivery of beta-lactam antibiotics to overcome bacterial resistance. Sci. Rep. 5 (1), 13500. doi:10.1038/srep13500
Algharib, S. A., Dawood, A., and Xie, S. (2020). Nanoparticles for treatment of bovine Staphylococcus aureus mastitis. Drug Deliv. 27 (1), 292–308. doi:10.1080/10717544.2020.1724209
Álvarez-Martínez, F. J., Barrajon-Catal, E., Herranz-Lopez, M., and Micol, V. (2021). Antibacterial plant compounds, extracts and essential oils: an updated review on their effects and putative mechanisms of action. Phytomedicine 90, 153626. doi:10.1016/j.phymed.2021.153626
Amorena, B., Gracia, E., Monzón, M., Leiva, J., Oteiza, C., Pérez, M., et al. (1999). Antibiotic susceptibility assay for Staphylococcus aureus in biofilms developed in vitro. J. Antimicrob. Chemother. 44 (1), 43–55. doi:10.1093/jac/44.1.43
Anandabaskar, N. (2021). “Protein synthesis inhibitors,” in Introduction to basics of pharmacology and toxicology. Editors A. Paul, N. Anandabaskar, J. Mathaiyan, and G. M. Raj (Singapore: Springer Nature Singapore), 835–868.
Barbuti, M. D., Myrbråten, I. N., Angeles, D. M., and Kjos, M. (2023). The cell cycle of Staphylococcus aureus: an updated review. MicrobiologyOpen 12 (1), e1338. doi:10.1002/mbo3.1338
Basavegowda, N., and Baek, K.-H. (2022). Combination strategies of different antimicrobials: an efficient and alternative tool for pathogen inactivation. Biomedicines 10 (9), 2219. doi:10.3390/biomedicines10092219
Bayles, K. W. (2007). The biological role of death and lysis in biofilm development. Nat. Rev. Microbiol. 5 (9), 721–726. doi:10.1038/nrmicro1743
Bezner Kerr, R., Postigo, J. C., Smith, P., Cowie, A., Singh, P. K., Rivera-Ferre, M., et al. (2023). Agroecology as a transformative approach to tackle climatic, food, and ecosystemic crises. Curr. Opin. Environ. Sustain. 62, 101275. doi:10.1016/j.cosust.2023.101275
Bianchi, S. E., Kaiser, S., Pittol, V., Doneda, E., De Souza, K. C. B., and Bassani, V. L. (2019). Semi-preparative isolation and purification of phenolic compounds from Achyrocline satureioides (Lam) D.C. by high-performance counter-current chromatography. Phytochem. Anal. 30 (2), 182–192. doi:10.1002/pca.2803
Bonapace, C. R., Bosso, J. A., Friedrich, L. V., and White, R. L. (2002). Comparison of methods of interpretation of checkerboard synergy testing. Diagnostic Microbiol. Infect. Dis. 44 (4), 363–366. doi:10.1016/S0732-8893(02)00473-X
Bradford, M. M. (1976). A rapid and sensitive method for the quantitation of microgram quantities of protein utilizing the principle of protein-dye binding. Anal. Biochem. 72 (1–2), 248–254. doi:10.1016/0003-2697(76)90527-3
Brennan-Krohn, T., Pironti, A., and Kirby, J. E. (2018). Synergistic activity of colistin-containing combinations against colistin-resistant enterobacteriaceae. Antimicrob. Agents Chemother. 62 (10), e00873. doi:10.1128/AAC.00873-18
Campos, B., Pickering, A. C., Rocha, L. S., Aguilar, A. P., Fabres-Klein, M. H., Mendes, T. A. O., et al. (2022). Diversity and pathogenesis of Staphylococcus aureus from bovine mastitis: current understanding and future perspectives. BMC Veterinary Res. 18 (1), 115. doi:10.1186/s12917-022-03197-5
Chen, Y., Wang, Y., Yang, M., and Guo, M. (2019). Allicin inhibited Staphylococcus aureus -induced mastitis by reducing lipid raft stability via LxRα in mice. J. Agric. Food Chem. 67 (39), 10863–10870. doi:10.1021/acs.jafc.9b04378
CLSI, Clinical and Laboratory Standards Institute (2018). M07: methods for dilution antimicrobial susceptibility tests for bacteria that grow aerobically. Wayne, PA: Clinical and Laboratory Standards Institute.
CLSI, Clinical and Laboratory Standards Institute (2019). M100: performance standards for antimicrobial susceptibility testing. 29th edn. Wayne, PA: Clinical and Laboratory Standards Institute.
de Souza, W. (2011). Técnicas de Microscopia Eletrônica Aplicadas às Ciências Biológicas. Rio de Janeiro: Sociedade Brasileira de Microscopia e Micronálise.
Dhanda, G., Acharya, Y., and Haldar, J. (2023). Antibiotic adjuvants: a versatile approach to combat antibiotic resistance. ACS Omega 8 (12), 10757–10783. doi:10.1021/acsomega.3c00312
Diao, W.-R., Hu, Q.-P., Zhang, H., and Xu, J.-G. (2014). Chemical composition, antibacterial activity and mechanism of action of essential oil from seeds of fennel (Foeniculum vulgare Mill.). Food control 35 (1), 109–116. doi:10.1016/j.foodcont.2013.06.056
El-Sayed, A., and Kamel, M. (2021). Bovine mastitis prevention and control in the post-antibiotic era. Trop. Animal Health Prod. 53 (2), 236. doi:10.1007/s11250-021-02680-9
Erickson, H. P. (2017). How bacterial cell division might cheat turgor pressure – a unified mechanism of septal division in Gram-positive and Gram-negative bacteria. BioEssays 39 (8). doi:10.1002/bies.201700045
EUCAST, European Committee for Antimicrobial Susceptibility Testing (EUCAST) of the European Society of Clinical Microbiology and Infectious Diseases (ESCMID) (2000). EUCAST Definitive Document E.Def 1.2, May 2000: terminology relating to methods for the determination of susceptibility of bacteria to antimicrobial agents. Clin. Microbiol. Infect. 6 (9), 503–508. doi:10.1046/j.1469-0691.2000.00149.x
Ezzat Alnakip, M., Quintela-Baluja, M., Böhme, K., Fernández-No, I., Caamaño-Antelo, S., Calo-Mata, P., et al. (2014). The immunology of mammary gland of dairy ruminants between healthy and inflammatory conditions. J. Veterinary Med. 2014, 659801–659831. doi:10.1155/2014/659801
Fenton, M., Keary, R., McAuliffe, O., Ross, R. P., O’Mahony, J., and Coffey, A. (2013). Bacteriophage-derived peptidase CHAPK eliminates and prevents staphylococcal biofilms. Int. J. Microbiol. 2013, 625341–625348. doi:10.1155/2013/625341
Fiordalisi, S. A. L., Honorato, L. A., and Kuhnen, S. (2018). Aloe barbadensis Miller leaf exudate is a potential treatment for bovine mastitis. F1000Research 7, 1285. doi:10.12688/f1000research.15671.2
Fitzgerald, J. R. (2012). Livestock-associated Staphylococcus aureus: origin, evolution and public health threat. Trends Microbiol. 20 (4), 192–198. doi:10.1016/j.tim.2012.01.006
Gamage, A., Gangahagedara, R., Gamage, J., Jayasinghe, N., Kodikara, N., Suraweera, P., et al. (2023). Role of organic farming for achieving sustainability in agriculture. Farming Syst. 1 (1), 100005. doi:10.1016/j.farsys.2023.100005
Grigor’eva, A., Bardasheva, A., Tupitsyna, A., Amirkhanov, N., Tikunova, N., Pyshnyi, D., et al. (2020). Changes in the ultrastructure of Staphylococcus aureus treated with cationic peptides and chlorhexidine. Microorganisms 8 (12), 1991. doi:10.3390/microorganisms8121991
Gruet, P., Maincent, P., Berthelot, X., and Kaltsatos, V. (2001). Bovine mastitis and intramammary drug delivery: review and perspectives. Adv. Drug Deliv. Rev. 50 (3), 245–259. doi:10.1016/S0169-409X(01)00160-0
Hartmann, M., Berditsch, M., Hawecker, J., Ardakani, M. F., Gerthsen, D., and Ulrich, A. S. (2010). Damage of the bacterial cell envelope by antimicrobial peptides gramicidin S and PGLa as revealed by transmission and scanning electron microscopy. Antimicrob. Agents Chemother. 54 (8), 3132–3142. doi:10.1128/AAC.00124-10
Harwansh, R. K., Deshmukh, R., and Rahman, M. A. (2019). Nanoemulsion: promising nanocarrier system for delivery of herbal bioactives. J. Drug Deliv. Sci. Technol. 51, 224–233. doi:10.1016/j.jddst.2019.03.006
Hogeveen, H., Huijps, K., and Lam, T. J. G. M. (2011). Economic aspects of mastitis: new developments. N. Z. Veterinary J. 59 (1), 16–23. doi:10.1080/00480169.2011.547165
Humphries, R. M. (2016). “Synergism testing: broth microdilution checkerboard and broth macrodilution methods,” in Clinical microbiology procedures handbook. Editor A. L. Leber (Washington, DC, USA: ASM Press), 5.16.1–5.16.23. doi:10.1128/9781555818814.ch5.16
Javed, S., McClure, J., Syed, M. A., Obasuyi, O., Ali, S., Tabassum, S., et al. (2022). Epidemiology and molecular characterization of Staphylococcus aureus causing bovine mastitis in water buffaloes from the Hazara division of Khyber Pakhtunkhwa, Pakistan. PLOS ONE 17 (5), e0268152. doi:10.1371/journal.pone.0268152
Johns, B. E., Purdy, K. J., Tucker, N. P., and Maddocks, S. E. (2015). Phenotypic and genotypic characteristics of small colony variants and their role in chronic infection. Microbiol. Insights 8, 15–23. doi:10.4137/MBI.S25800
Joray, M. B., Palacios, S. M., and Carpinella, M. C. (2013). Understanding the interactions between metabolites isolated from Achyrocline satureioides in relation to its antibacterial activity. Phytomedicine 20 (3–4), 258–261. doi:10.1016/j.phymed.2012.10.015
Kuhnen, S., Mello, D. F. M. D., Honorato, L. A., Piccinin, I. N., Martins, J., Bernardes, P. A., and et al., (2021). Identification and antimicrobial susceptibility of milk pathogen isolated from dairy production systems. Prev. Veterinary Med. 194, 105451. doi:10.1016/j.prevetmed.2021.105451
Li, C.-M., and Yu, J. P. (2015). Chemical composition, antimicrobial activity and mechanism of action of essential oil from the leaves of Macleaya cordata (willd.) R. Br. J. Food Saf. 35 (2), 227–236. doi:10.1111/jfs.12175
Lorenzo, D., Atti-Serafin, L., Santos, A. C., Frizzo, C. D., Paroul, N., Paz, D., et al. (2000). Achyrocline satureioides essential oils from southern Brazil and Uruguay. Planta Medica 66 (5), 476–477. doi:10.1055/s-2000-8590
Lucena-Aguilar, G., Sánchez-López, A. M., Barberán-Aceituno, C., Carrillo-Ávila, J. A., López-Guerrero, J. A., and Aguilar-Quesada, R. (2016). DNA source selection for downstream applications based on DNA quality indicators analysis. Biopreservation Biobanking 14 (4), 264–270. doi:10.1089/bio.2015.0064
Machado, G. T. B. P. (2021). Desenvolvimento de formas farmacêuticas sustentáveis com nanoemulsão de macela (Achyrocline satureioides) visando à terapêutica da mastite bovina. dissertação de mestrado. Florianópolis (BR): Universidade Federal de Santa Catarina.
Machado, G. T. P., Ferreira, R. G., Veleirinho, M. B., Honorato, L. A., Ramos, R. O., Silva, M. A. S., et al. (2023). In vitro evaluation of nanocomposites of linseed mucilage and k-carrageenan loaded with Achyrocline satureioides nanoemulsion: a gradual-release candidate of antimicrobials for the treatment of bovine mastitis. J. Dairy Res. 90 (4), 376–381. doi:10.1017/S002202992300064X
Machado, G. T. P., Veleirinho, M. B., Honorato, L. A., and Kuhnen, S. (2020). Formulation and evaluation of anti-MRSA nanoemulsion loaded with Achyrocline satureioides: a new sustainable strategy for the bovine mastitis. Nano Express 1 (3), 030004. doi:10.1088/2632-959X/abbcac
MAPA Ministério da Agricultura Pecuária e Abastecimento (2021). Portaria No 52, Regulamento Técnico para os Sistemas Orgânicos de Produção e as listas de substâncias e práticas para o uso nos Sistemas Orgânicos de Produção. Brasília, DF.
Martínez-Busi, M., Arredondo, F., González, D., Echeverry, C., Vega-Teijido, M. A., Carvalho, D., et al. (2019). Purification, structural elucidation, antioxidant capacity and neuroprotective potential of the main polyphenolic compounds contained in Achyrocline satureioides (Lam) D.C. (Compositae). Bioorg. and Med. Chem. 27 (12), 2579–2591. doi:10.1016/j.bmc.2019.03.047
Moresco, K. S., Silveira, A. K., Zeidán-Chuliá, F., Correa, A. P. F., Oliveria, R. R., Borges, A. G., et al. (2017). Effects of Achyrocline satureioides inflorescence extracts against pathogenic intestinal bacteria: chemical characterization, in vitro tests, and in vivo evaluation. Evidence-Based Complementary Altern. Med. 2017, 4874865. doi:10.1155/2017/4874865
Mu, H., Tang, J., Liu, Q., Sun, C., Wang, T., and Duan, J. (2016). Potent antibacterial nanoparticles against biofilm and intracellular bacteria. Sci. Rep. 6 (1), 18877. doi:10.1038/srep18877
Murugaiyan, J., Kumar, P. A., Rao, G. S., Iskandar, K., Hawser, S., Hays, J. P., et al. (2022). Progress in alternative strategies to combat antimicrobial resistance: focus on antibiotics. Antibiotics 11 (2), 200. doi:10.3390/antibiotics11020200
Orhan, G., Bayram, A., Zer, Y., and Balci, I. (2005). Synergy tests by E test and checkerboard methods of antimicrobial combinations against Brucella melitensis. J. Clin. Microbiol. 43 (1), 140–143. doi:10.1128/JCM.43.1.140-143.2005
Paul, T. R., Venter, A., Blaszczak, L. C., Parr, T. R., Labischinski, H., and Beveridge, T. J. (1995). Localization of penicillin-binding proteins to the splitting system of Staphylococcus aureus septa by using a mercury-penicillin V derivative. J. Bacteriol. 177 (13), 3631–3640. doi:10.1128/jb.177.13.3631-3640.1995
Pérez-Peinado, C., Dias, S. A., Domingues, M. M., Benfield, A. H., Freire, J. M., Rádis-Baptista, G., et al. (2018). Mechanisms of bacterial membrane permeabilization by crotalicidin (Ctn) and its fragment Ctn(15–34), antimicrobial peptides from rattlesnake venom. J. Biol. Chem. 293 (5), 1536–1549. doi:10.1074/jbc.RA117.000125
Pergo, É. M., Britta, E. A., and Galletti, P. A. (2019). Metodologia de microscopia eletrônica de transmissão aplicada ao estudo de raízes de plântulas. Rev. Bras. Multidiscip. 22 (1), 175–186. doi:10.25061/2527-2675/ReBraM/2019.v22i1.505
Petrovick, G. F., Petrovick, P. R., and Bassani, V. L. (2010). Achyrocline satureioides (Lam.) DC., Asteraceae: development of granules from spray dried powder. Rev. Bras. Farmacogn. 20 (5), 796–803. doi:10.1590/S0102-695X2010005000020
Pham, T. N., Loupias, P., Dassonville-Klimpt, A., and Sonnet, K. P. (2019). Drug delivery systems designed to overcome antimicrobial resistance. Med. Res. Rev. 39 (6), 2343–2396. doi:10.1002/med.21588
Pinheiro Machado, G. T., Veleirinho, M. B., Ferreira, R. G., Zuglianello, C., Lemos-Senna, E., and Kuhnen, S. (2022). Protection of bovine mammary epithelial cells by a nanoemulsion of the medicinal herb Achyrocline satureioides (Lam.) DC and its capacity of permeation through mammary epithelium. J. Dairy Res. 89 (1), 80–85. doi:10.1017/S0022029922000139
Pinheiro Machado, G. T., Veleirinho, M. B., Mazzarino, L., Pinheiro Machado Filho, L. C., Maraschin, M., Cerri, R. L. A., et al. (2019). Development of propolis nanoparticles for the treatment of bovine mastitis: in vitro studies on antimicrobial and cytotoxic activities. Can. J. Animal Sci. 99 (4), 713–723. doi:10.1139/cjas-2018-0173
Rainard, P., Gilbert, F. B., Germon, P., and Foucras, G. (2021). Invited review: a critical appraisal of mastitis vaccines for dairy cows. J. Dairy Sci. 104 (10), 10427–10448. doi:10.3168/jds.2021-20434
Roemhild, R., Bollenbach, T., and Andersson, D. I. (2022). The physiology and genetics of bacterial responses to antibiotic combinations. Nat. Rev. Microbiol. 20 (8), 478–490. doi:10.1038/s41579-022-00700-5
Rosati, A., Borek, R., and Canali, S. (2021). Agroforestry and organic agriculture. Agrofor. Syst. 95 (5), 805–821. doi:10.1007/s10457-020-00559-6
Ruffa, M. J., Ferraro, G., Wagner, M. L., Calcagno, M. L., Campos, R. H., and Cavallaro, L. (2002). Cytotoxic effect of Argentine medicinal plant extracts on human hepatocellular carcinoma cell line. J. Ethnopharmacol. 79 (3), 335–339. doi:10.1016/S0378-8741(01)00400-7
Sadykov, M. R., and Bayles, K. W. (2012). The control of death and lysis in staphylococcal biofilms: a coordination of physiological signals. Curr. Opin. Microbiol. 15 (2), 211–215. doi:10.1016/j.mib.2011.12.010
Salat, O., Se´rieys, F., Poutrel, B., Durel, L., and Goby, L. (2008). Systemic treatment of subclinical mastitis in lactating cows with penethamate hydriodide. J. Dairy Sci. 91 (2), 632–640. doi:10.3168/jds.2007-0174
Santhana Raj, L., Hing, H. L., Omar, B., Teh Hamidah, Z., Aida Suhana, R., Nor Asiha, C. P., et al. (2007). Mesosomes are a definite event in antibiotic-treated Staphylococcus aureus ATCC 25923. Trop. Biomed. 24 (1), 105–109.
Sarker, S. D., Nahar, L., and Kumarasamy, Y. (2007). Microtitre plate-based antibacterial assay incorporating resazurin as an indicator of cell growth, and its application in the in vitro antibacterial screening of phytochemicals. Methods 42 (4), 321–324. doi:10.1016/j.ymeth.2007.01.006
Simões, C. M. O., Schenke, E. P., Bauer, L., and Langeloh, A. (1988). Pharmacological investigations on Achyrocline satureioides (Lam.) DC., compositae. J. Ethnopharmacol. 22 (3), 281–293. doi:10.1016/0378-8741(88)90239-5
Singh, R., Saini, S., Ansari, S., Jamwal, S., and Malaka, D. (2020). 220 Exploring the use of mesenchymal stem cells for treatment of mastitis and metritis in cattle. Reproduction, Fertil. Dev. 32 (2), 238. doi:10.1071/RDv32n2Ab220
Souza, P. O. D., Bianchi, S. E., Figueiró, F., Heimfartha, L., Moresco, K. S., Gonçalves, R. M., et al. (2018). Anticancer activity of flavonoids isolated from Achyrocline satureioides in gliomas cell lines. Toxicol. Vitro 51, 23–33. doi:10.1016/j.tiv.2018.04.013
Spinosa, H. S., Gorniak, S. L., and Bernardi, M. M. (2017). Farmacologia aplicada à medicina veterinária. Rio de Janeiro: Guanabara Koogan.
Stautz, J., Hellmich, Y., Fuss, M. F., Silberberg, J. M., Devlin, J. R., Stockbridge, R. B., et al. (2021). Molecular mechanisms for bacterial potassium homeostasis. J. Mol. Biol. 433 (16), 166968. doi:10.1016/j.jmb.2021.166968
Stoscheck, C. M. (1990). Quantitation of protein. Methods Enzym. 182, 50–68. doi:10.1016/0076-6879(90)82008-P
Strahl, H., and Errington, J. (2017). Bacterial membranes: structure, domains, and function. Annu. Rev. Microbiol. 71 (1), 519–538. doi:10.1146/annurev-micro-102215-095630
Sullivan, G. J., Delgado, N. N., Maharjan, R., and Cain, A. K. (2020). How antibiotics work together: molecular mechanisms behind combination therapy. Curr. Opin. Microbiol. 57, 31–40. doi:10.1016/j.mib.2020.05.012
Tiseo, K., Huber, L., Gilbert, M., Robinson, T. P., and Van Boeckel, T. P. (2020). Global trends in antimicrobial use in food animals from 2017 to 2030. Antibiotics 9 (12), 918. doi:10.3390/antibiotics9120918
Touhami, A., Jericho, M. H., and Beveridge, T. J. (2004). Atomic force microscopy of cell growth and division in Staphylococcus aureus. J. Bacteriol. 186 (11), 3286–3295. doi:10.1128/JB.186.11.3286-3295.2004
Touza-Otero, L., Landin, M., and Diaz-Rodriguez, P. (2024). Fighting antibiotic resistance in the local management of bovine mastitis. Biomed. and Pharmacother. 170, 115967. doi:10.1016/j.biopha.2023.115967
Varela-Ortiz, D. F., Barboza-Corona, J. E., González-Marrero, J., León-Galván, M. F., Valencia-Posadas, M., Lechuga-Arana, A. A., et al. (2018). Antibiotic susceptibility of Staphylococcus aureus isolated from subclinical bovine mastitis cases and in vitro efficacy of bacteriophage. Veterinary Res. Commun. 42 (3), 243–250. doi:10.1007/s11259-018-9730-4
Vestby, L. K., Grønseth, T., Simm, R., and ande Nesse, L. L. (2020). Bacterial biofilm and its role in the pathogenesis of disease. Antibiotics 9 (2), 59. doi:10.3390/antibiotics9020059
Wallis, J. K., Krömker, V., and Paduch, J.-H. (2018). Biofilm formation and adhesion to bovine udder epithelium of potentially probiotic lactic acid bacteria. AIMS Microbiol. 4 (2), 209–224. doi:10.3934/microbiol.2018.2.209
Wang, N., Luo, J., Deng, F., Huang, Y., and Zhou, H. (2022). Antibiotic combination therapy: a strategy to overcome bacterial resistance to aminoglycoside antibiotics. Front. Pharmacol. 13, 839808. doi:10.3389/fphar.2022.839808
Wei, C., Cui, P., and Liu, X. (2023). Antibacterial activity and mechanism of madecassic acid against Staphylococcus aureus. Molecules 28 (4), 1895. doi:10.3390/molecules28041895
Xie, Y., Yang, W., Tang, F., Xiaoqing, C., and Ren, L. (2015). Antibacterial activities of flavonoids: structure-activity relationship and mechanism. Curr. Med. Chem. 22 (1), 132–149. doi:10.2174/0929867321666140916113443
Yang, S.-K., Yusoff, K., Mai, C.-W., Lim, W.-M., Yap, W.-S., Lim, S.-H. E., et al. (2017). Additivity vs synergism: investigation of the additive interaction of cinnamon bark oil and meropenem in combinatory therapy. Molecules 22 (11), 1733. doi:10.3390/molecules22111733
Youssef, F. S., El-Banna, H. A., Elzorba, H. Y., and Galal, A. M. (2019). Application of some nanoparticles in the field of veterinary medicine. Int. J. Veterinary Sci. Med. 7 (1), 78–93. doi:10.1080/23144599.2019.1691379
Yuan, G., Guan, Y., Yi, H., Lai, S., Sun, Y., and Cao, S. (2021). Antibacterial activity and mechanism of plant flavonoids to gram-positive bacteria predicted from their lipophilicities. Sci. Rep. 11 (1), 10471. doi:10.1038/s41598-021-90035-7
Zhang, W., Wang, J., Chen, Y., Zheng, H., Xie, B., and Sun, Z. (2020). Flavonoid compounds and antibacterial mechanisms of different parts of white guava (Psidium guajava L. cv. Pearl). Nat. Prod. Res. 34 (11), 1621–1625. doi:10.1080/14786419.2018.1522313
Zhang, Y., Liu, X., Wang, Y., Jiang, P., and Quek, S. Y. (2016). Antibacterial activity and mechanism of cinnamon essential oil against Escherichia coli and Staphylococcus aureus. Food control 59, 282–289. doi:10.1016/j.foodcont.2015.05.032
Keywords: macela-nanoemulsion, drug delivery systems, herbal actives, antimicrobial, synergy, ultrastructural damage, bovine mastitis, sustainable dairy systems
Citation: Ribeiro R, Rentes BR, Honorato LA and Kuhnen S (2024) Effect of nanoemulsion loaded with macela (Achyrocline satureioides) on the ultrastructure of Staphylococcus aureus and the modulating activity of antibiotics. Front. Nanotechnol. 6:1466988. doi: 10.3389/fnano.2024.1466988
Received: 18 July 2024; Accepted: 28 October 2024;
Published: 08 November 2024.
Edited by:
Chris A. Kieslich, Auburn University, United StatesReviewed by:
Sandipan Mukherjee, University of Washington, United StatesCelso SantAnna, Inmetro, Brazil
Copyright © 2024 Ribeiro, Rentes, Honorato and Kuhnen. This is an open-access article distributed under the terms of the Creative Commons Attribution License (CC BY). The use, distribution or reproduction in other forums is permitted, provided the original author(s) and the copyright owner(s) are credited and that the original publication in this journal is cited, in accordance with accepted academic practice. No use, distribution or reproduction is permitted which does not comply with these terms.
*Correspondence: Rayanne Ribeiro, cmF5YXJpYkBnbWFpbC5jb20=