- 1Department of Allied and Public Health, School of Health, Sport and Bioscience, University of East London, London, United Kingdom
- 2Department of Public Health, York St John University, London, United Kingdom
- 3Department of Research and Innovation, Medway NHS Foundation Trust, Gillingham, United Kingdom
- 4Division of Sustainable Development, College of Science and Engineering, Hamad Bin Khalifa University, Qatar Foundation, Doha, Qatar
- 5Department of Chemistry, Virginia Tech University, Blacksburg, VA, United States
- 6Department of Chemistry and Biochemistry, University of Arizona, Tucson, United States
- 7Department of Chemistry, Faculty of Science, University of Ibadan, Ibadan, Nigeria
- 8Systems and Industrial Engineering Department, University of Arizona, Tucson, United States
- 9Department of Comparative Biomedical Science, College of Veterinary Medicine, Mississippi State University, Starkville, United States
Heavy metal contamination in water sources poses a significant threat to environmental and public health, necessitating effective remediation strategies. Nanomaterial-based approaches have emerged as promising solutions for heavy metal removal, offering enhanced selectivity, efficiency, and sustainability compared to traditional methods. This comprehensive review explores novel nanomaterial-based approaches for heavy metal remediation, focusing on factors such as selectivity, regeneration, scalability, and practical considerations. A systematic literature search was conducted using multiple academic databases, including PubMed, Web of Science, and Scopus, to identify relevant articles published between 2013 and 2024. The review identifies several promising nanomaterials, such as graphene oxide, carbon nanotubes, and metal-organic frameworks, which exhibit high surface areas, tunable surface chemistries, and excellent adsorption capacities. Surface functionalization with specific functional groups (e.g., carboxyl, amino, thiol) significantly enhances the selectivity for target heavy metal ions. Advances in regeneration strategies, including chemical desorption, electrochemical regeneration, and photocatalytic regeneration, have improved the reusability and cost-effectiveness of these materials. Scalability remains a critical challenge, but recent developments in synthesis methods, such as green synthesis and continuous-flow synthesis, offer promising solutions for large-scale production. The stability and longevity of nanomaterials have been improved through surface modification and the development of hybrid nanocomposites. Integrating nanomaterials with existing water treatment infrastructure and combining them with other remediation techniques, such as membrane filtration and electrochemical methods, can enhance overall treatment efficiency and feasibility. In conclusion, nanomaterial-based approaches hold immense promise for revolutionizing heavy metal remediation and advancing sustainable water management practices. As future research is geared towards retrofitting existing treatment plants, it is equally critical to mitigate unintended environmental and public health consequences associated with the widespread production and use of nanomaterials, such as their leachability into water systems and environmental persistence.
1 Introduction
The contamination of fresh and marine water sources with heavy metals is a pressing environmental and public health concern with profound implications for ecosystems and human health. This is attributed to the exponential increase in urbanization and industrial and human activities (Qasem et al., 2021; Hama Aziz et al., 2023). These toxic pollutants, including lead (Pb), mercury (Hg), cadmium (Cd), arsenic (As), and chromium (Cr), infiltrate water bodies through various pathways such as industrial discharges, mining activities, agricultural runoff, and natural processes like rock weathering (Qasem et al., 2021). The WHO reported that about 1 million people lost their lives in 2019 due to lead exposure and about 140 million people from 70 countries have been drinking water contaminated with arsenic (WHO, 2022; WHO 2023).
The non-biodegradable nature of heavy metals facilitates their persistence and accumulation in the environment. The repercussions of heavy metal pollution are extensive, affecting both the human and aquatic ecosystems (Roy et al., 2024). Aquatic ecosystems suffer from bioaccumulation and biomagnification of heavy metals, leading to ecological imbalances and long-term risks (Sharma et al., 2024). In humans, exposure to heavy metals via contaminated water or food can result in severe health problems ranging from neurological disorders to cancer, with vulnerable groups like children and pregnant women facing heightened risks (Edo et al., 2024). For instance, heavy metal contamination of surface water sources from persistent industrial discharge results in the bioaccumulation of these metals in aquatic organisms like fish, leading to increased morbidity and mortality among aquatic life. Moreover, consuming these contaminated fish can pose serious health risks to humans. Thus, evaluating water sources for metal pollution is critical due to the environmental persistence of heavy metals and their detrimental impact on flora and fauna, even at minute concentration levels (Edo et al., 2024). Table 1 below highlights some heavy metals of public health concern, their permissible limits, implications, and sources.
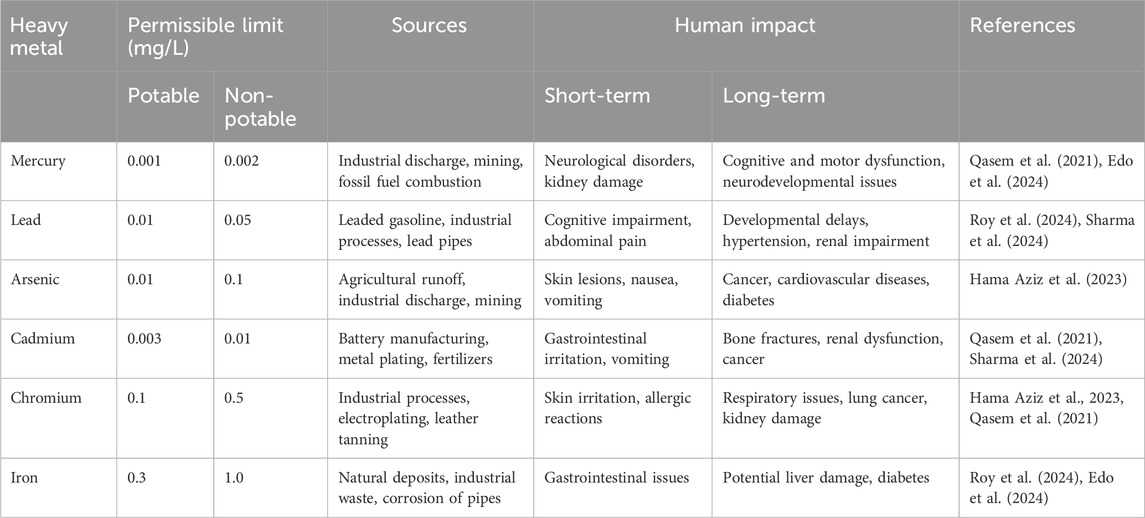
Table 1. Heavy metals of public health concern, their permissible limits, implications, and sources.
Traditional methods for heavy metal remediation, such as chemical precipitation, coagulation-flocculation, ion exchange, and membrane filtration, have limitations that impede their effectiveness (See Table 2). These methods often lack selectivity, removing essential ions along with heavy metals and generating toxic byproducts like sludge (Zamora-Ledezma et al., 2021). For instance, coagulation-flocculation processes result in the production of significant quantities of chemical sludge and can leave residual coagulant metals in the treated water (Zinicovscaia, 2016). Moreover, residual coagulant metals like aluminium are associated with several issues, including increased turbidity, reduced disinfection efficiency, decreased hydraulic capacity, and potential adverse health effects such as Alzheimer’s disease (Zinicovscaia, 2016). Additionally, traditional heavy metal remediation methods can be cost-prohibitive and challenging to scale up for large-scale water treatment applications (Qasem et al., 2021; Zamora-Ledezma et al., 2021). For example, carbon-based adsorbents such as activated carbon are prepared following high heat and pressure requirements, which are energy and cost-intensive (Qasem et al., 2021). In response to these challenges, nanomaterial-based approaches have emerged as promising solutions due to their unique properties and versatile applications in heavy metal remediation (Solomon NkoOkina et al., 2024). Table 2 summarizes traditional methods of treating heavy metals in wastewater and their potential drawbacks.
Nanomaterials offer several advantages for addressing heavy metal contamination in water sources. Their high surface area-to-volume ratio, tunable surface chemistry, and enhanced reactivity make them highly efficient adsorbents and catalysts (Ali et al., 2023). Recent trends in nanomaterial-based approaches focus on achieving selective adsorption, regeneration capability, scalability, and multifunctionality (Yang et al., 2019; Kolluru et al., 2021). Functionalized nanomaterials with tailored surface properties exhibit selective adsorption of specific heavy metal ions, minimizing interference from other ions and enhancing treatment efficiency (Mensah et al., 2021). Some nanomaterials are capable of removing toxic metal ions and tiny pollutants smaller than 300 nm (Baby et al., 2022). Moreover, some nanomaterials possess inherent regenerative properties or can be easily regenerated through desorption processes, enabling multiple cycles of use and reducing operational costs (Mensah et al., 2021). Some of the commonly explored nanomaterials for heavy metal remediation in wastewater are zero-valent metals, carbon-based materials, polymer-based materials, zeolite, magnetic materials, nanocomposites, and metal oxides (Yang et al., 2019; Ethaib et al., 2022). In one study, composite hydrogel consisting of gum tragacanth and graphene oxide (GO) was effectively used to adsorb 65 ppm of the following heavy metal contaminants: Cd (II), Pb (II), and Ag (I) at 89%, 96.4%, and 85.4% removal efficiency respectively (Sahraei and Ghaemy, 2017). The GO nanosheets, rich in hydrophilic hydroxyl and carboxyl functional groups, not only increased the hydrogel’s water absorption capacity but also provided multiple active sites for metal ion binding. This incorporation of GO expanded the hydrogel network structure, enhancing its ability to swell in aqueous environments and improving metal ion uptake. In another study, sulfide nanoscale zero-valent iron (S-NZVI) treated with nitro-functionalized UiO-66 was a reliable adsorbent for radioactive uranium (VI) and had a high removal rate (895 mg/g) as opposed to S-NZVI (434 mg/g) and UiO66-NO2 (267 mg/g) (Zhang et al., 2023). The study highlights that the removal mechanisms for U(VI) include physical adsorption, electrostatic attraction, and complexation by UiO-66-NO2, while S-NZVI contributes to uranium reduction and further complexation. The improved reactivity and smaller particle size of S-NZVI/UiO-66 result in greater contact with uranium ions, allowing for more efficient removal across a wide pH range.
While nanomaterials are emerging as a game-changer in the treatment of heavy metals in wastewater, current research is also focusing on sustainable production processes and the integration of these materials into existing treatment facilities (Solomon N. O. et al., 2024; Poonia et al., 2024; Shingare et al., 2024). Advances in nanomaterial synthesis techniques and reactor design are facilitating scalable production and seamless integration into conventional systems, enabling the efficient removal of heavy metals from large volumes of water (Khan et al., 2024; Nupur and Nipun, 2024). Moreover, researchers are developing multifunctional nanomaterials that can simultaneously target multiple contaminants, including heavy metals, organic pollutants, and pathogens, thereby addressing the complex nature of water pollution more comprehensively (Zhang et al., 2019).
Thus, given the persistent challenges associated with heavy metal contamination, the limitations of traditional remediation methods, and the emergence of nanomaterials as a potentially more effective treatment method, this review is essential. It responds to the urgent need for innovative and sustainable solutions to combat heavy metal pollution and safeguard water quality and public health. This review is novel in its comprehensive examination of recent advances in nanomaterial-based approaches for heavy metal remediation, specifically focusing on selectivity, regeneration capabilities, and scalability. The primary objectives are to provide a thorough overview of the current state-of-the-art in nanomaterial-based heavy metal remediation, identify key challenges and opportunities in the field, and propose future directions for research and development aimed at advancing sustainable water treatment technologies.
2 Nanomaterials for heavy metal remediation
Nanotechnology has revolutionized the field of environmental remediation, offering innovative solutions to tackle heavy metal pollution in water sources. Nanomaterials, with their unique properties and high surface area-to-volume ratio, have shown great promise in efficiently removing heavy metals from contaminated water. Table 3 provides a comprehensive comparison of the key nanomaterials used for heavy metal remediation, focusing on their adsorption capacity, selectivity, regeneration efficiency, and unique characteristics.
Figure 1 below shows various types of nanomaterials utilized and mechanisms for heavy metal remediation, including nanoparticles, nanocomposites, and nanostructured materials.
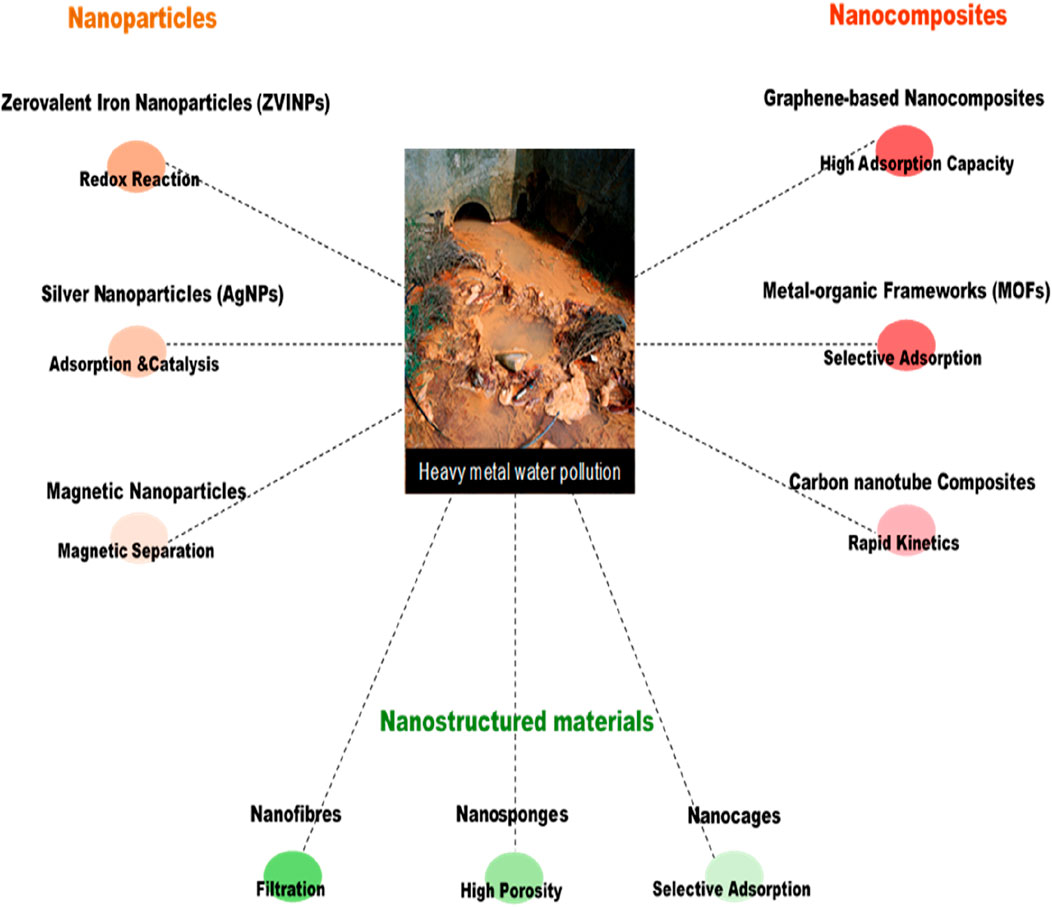
Figure 1. Overview of nanomaterials and their mechanisms for heavy metal remediation in water sources.
2.1 Nanoparticles
Nanoparticles, owing to their small size and large surface area, exhibit enhanced reactivity and adsorption capacities, making them excellent candidates for heavy metal removal (Sarma et al., 2019; Yu et al., 2021). Several studies have demonstrated that nanomaterials also possess significant redox and catalytic properties. The high surface area provides numerous active sites for interaction with heavy metal ions, facilitating efficient adsorption and transformation processes. Additionally, the tunable surface chemistry of nanomaterials allows for the modification of their properties to target specific contaminants (Garcia-Segura et al., 2020). This adaptability enhances their versatility in various environmental conditions. The potential for functionalization with specific ligands or coatings further improves their selectivity and effectiveness in complex environmental matrices. Nanoparticles are increasingly explored for environmental cleanup and sustainable water treatment technologies. Based on their magnetic properties, nanoparticles can be categorized into magnetic and non-magnetic nanoparticles.
2.1.1 Non-magnetic nanoparticles
Non-magnetic nanoparticles, despite lacking magnetic properties, play a crucial role in heavy metal remediation due to their exceptional adsorption and catalytic capabilities. These nanoparticles often exhibit high surface areas, which provide numerous active sites for metal ion binding and reduction processes. Additionally, their tunable surface chemistries allow for the modification of their surfaces with functional groups or coatings, enhancing their selectivity for specific contaminants. Non-magnetic nanoparticles, such as zerovalent iron nanoparticles (ZVINPs) and silver nanoparticles (AgNPs), are widely studied for their ability to reduce and adsorb heavy metals from water. While they may not offer the same ease of separation as magnetic nanoparticles, non-magnetic nanoparticles make up for this limitation through their efficient remediation performance and the ease with which they can be synthesized and functionalized for specific environmental conditions. Their relatively low cost and the potential for regeneration also make them highly attractive for large-scale environmental applications.
2.1.1.1 Zerovalent iron nanoparticles (ZVINPs)
Zerovalent iron nanoparticles (ZVINPs) have garnered significant attention for their ability to efficiently reduce a wide range of heavy metal ions through redox reactions. Recent studies have demonstrated that ZVINPs efficiently adsorb metal ions onto their surface, followed by electron transfer, resulting in the formation of insoluble metal oxides or hydroxides (Rodríguez-Rasero et al., 2024; Moond et al., 2024). The adsorption process is significantly favored by an increase in temperature (Tang et al., 2023; Bazarin et al., 2024). ZVINPs can be regenerated through simple treatments such as pH adjustment or chemical reduction, which enhances their reusability and cost-effectiveness (Thomas et al., 2017). Due to their relatively low toxicity compared to other nanomaterials, ZVINPs present a safer option for environmental applications. However, ongoing research is addressing their environmental and health impacts while optimizing their performance and scalability for large-scale remediation projects (Naveed et al., 2023; Xu et al., 2020).
2.1.1.2 Silver nanoparticles (AgNPs)
Silver nanoparticles (AgNPs) possess inherent antimicrobial properties and have emerged as effective adsorbents for heavy metal ions (Egbewole et al., 2022). Recent research has shown that AgNPs efficiently remove toxic metals such as mercury, cadmium, and copper from contaminated water sources (Sudarman et al., 2023). AgNPs also catalyze the reduction of heavy metal ions into less toxic forms, further enhancing remediation efficiency. However, concerns regarding the potential environmental impact of AgNPs, particularly their ecotoxicity and long-term stability, have been raised (Noga et al., 2023). Further investigations are required to ensure the safe and sustainable use of AgNPs in environmental applications.
2.1.2 Magnetic nanoparticles
Magnetic nanoparticles, such as magnetite (Fe3O4) and maghemite (γ-Fe2O3), offer distinct advantages due to their magnetic properties, which enable easy separation and recovery from water using external magnetic fields (de Oliveira et al., 2020; Zeng et al., 2020; Borji et al., 2020). Functionalized magnetic nanoparticles have been explored for the selective removal of heavy metal ions through surface modification with chelating agents or ligands. These modifications improve the specificity and binding affinity toward target heavy metals (Shao et al., 2020; Pardo et al., 2021), making magnetic nanoparticles suitable for large-scale water treatment applications. Their magnetic properties facilitate rapid and efficient contaminant removal, and their regeneration and reuse reduce operational costs. Moreover, integrating magnetic nanoparticles with other nanomaterials enhances adsorption capacity and recyclability, further improving their performance in heavy metal remediation.
2.2 Nanocomposites
Nanocomposites, composed of two or more distinct components at the nanoscale, offer synergistic properties for enhanced heavy metal removal. Compared to single-component systems, two distinct components at the nanoscale level offer synergistic properties that significantly enhance heavy metal removal (Singh and Bhateria, 2021). By incorporating the unique properties of each constituent nanomaterials, superior stability, adsorption capacities (Baig et al., 2021), and improved selectivity towards specific contaminants can be reached. This combination of properties allows for more efficient and effective remediation processes compared to single-component systems. Moreover, the possibility of tunability in the design of nanocomposites can be optimally repurposed for various environmental conditions. (Darwish et al., 2022; Hassan et al., 2021). This tunability is a versatile tool in the fight against heavy metal pollution. As research in this field progresses, the development of novel nanocomposite materials will provide highly extensive sustainable, and high-performance water treatment technologies. In this study, we will delve a little into the recent study of nanocomposites and their applications to remediate heavy metals from the environment. Some such nanocomposites include graphene nanocomposites, metal-organic frameworks (MOFs), and carbon nanotube composites.
2.2.1 Graphene-based nanocomposites
Due to their low cost, well-defined pore-forming mechanisms, and excellent magnetic properties that facilitate magnetic separation, graphene-based nanocomposites have emerged as a viable option for heavy metal remediation (Donga et al., 2021; Goyat et al., 2022). These materials have attracted significant interest because of their high surface area, exceptional mechanical strength, and chemical stability. Recent advancements in graphene-based materials have also demonstrated their potential as antifouling agents (Su and Hu, 2021), thanks to their excellent antibacterial properties, leading to the development of novel adsorbents for heavy metal removal. Graphene oxide (GO) and reduced graphene oxide (rGO) composites functionalized with various functional groups exhibit excellent adsorption capacities for heavy metal ions (Jahan et al., 2022), including cadmium, lead, and nickel. The unique structure of graphene-based nanocomposites enables rapid and selective removal of contaminants from aqueous solutions (Malhotra and Jain, 2021; Pena-Pereira et al., 2021), offering potential solutions to mitigate heavy metal pollution.
2.2.2 Metal-organic frameworks (MOFs)
Metal-organic frameworks (MOFs) are porous materials composed of metal ions or clusters coordinated with organic ligands, offering high surface areas and tunable pore structures. Recent research has demonstrated the applicability of MOFs as efficient adsorbents for heavy metal removal due to their photocatalytic reduction properties (Li et al., 2021). The modular nature of MOFs allows for precise control over their pore size and surface chemistry, enabling selective adsorption of specific heavy metal ions. MOFs can be functionalized with various active sites to enhance their adsorption capacities and catalytic activities (Hou et al., 2022). This adaptability makes them promising candidates for tailored environmental remediation strategies, addressing diverse and complex contamination scenarios. Furthermore, the incorporation of functional groups or post-synthetic modifications enhances the adsorption capacity and stability of MOFs, making them promising candidates for water purification.
2.2.3 Carbon nanotube composites
Carbon nanotubes (CNTs) possess extraordinary mechanical strength, electrical conductivity, and large surface area (Nurazzi et al., 2021; Zhang H. et al., 2020), making them ideal candidates for heavy metal remediation applications. Recent studies have investigated the use of CNT-based composites for the removal of heavy metal ions from aqueous solutions (Akhter et al., 2023; Aslam et al., 2022). Functionalization of CNTs with various organic or inorganic materials enhances their adsorption capacity and selectivity towards specific heavy metal contaminants. Through coprecipitation with certain metal oxides such as iron and zirconium, they are modified for excellent removal of chromium, mercury, lead, arsenic, etc. From water (by CBN et al., 2020). Additionally, the unique one-dimensional structure of CNTs facilitates the diffusion of metal ions into the internal pore spaces (Dey et al., 2024; Luo et al., 2022), leading to rapid adsorption kinetics and high removal efficiency.
2.3 Nanostructured materials
Nanostructured materials, including nanofibers, nanosponges, and nanocages, offer unique architectures for efficient heavy metal removal. These structures facilitate greater interaction with molecules of the pollutants. Their porous and adaptable designs allow for the incorporation of functional groups that improve selectivity and adsorption capacity. Additionally, the adaptability of these nanostructures enables their application in various environmental conditions, making them highly effective for diverse heavy metal remediation efforts. Some of these nanostructured materials are classified as nanofibers, nano-sponges, and nanocages.
2.3.1 Nanofibers
Nanofibers, characterized by their high aspect ratio and interconnected porous structure, provide large surface areas for the adsorption and filtration of heavy metal ions. Recent advancements in electrospinning techniques have enabled the fabrication of nanofiber-based membranes with precise control of the membrane characteristics tailored for water purification (Liao et al., 2018). Functionalization of nanofibers with specific ligands or nanoparticles enhances their adsorption capacity and selectivity (El-Aswar et al., 2022), making them effective adsorbents for heavy metal removal from aqueous solutions. These functionalized nanofibers exhibit improved mechanical strength and stability, ensuring long-term durability in filtration systems. The ability to integrate multiple functional groups within nanofibers further broadens their application (Zamel and Khan, 2021), providing the avenue for the concurrent removal of various contaminants. This makes nanofiber-based membranes a promising solution for advanced water treatment technologies.
2.3.2 Nanosponges
Nanosponges are porous materials composed of interconnected networks of nanoparticles or nanofibers, offering high surface area and porosity for adsorption applications. Recent research has demonstrated the feasibility of nanosponge-based materials for heavy metal remediation (Iravani and Varma, 2022). The sponge-like structure of nanosponges enables rapid diffusion of heavy metal ions into the interior pores, where they are effectively sequestered through chemical interactions or physical adsorption. Furthermore, the flexibility in the design and synthesis of nanosponges allows for the customization of their properties to meet specific application requirements (Goyal et al., 2023).
2.3.3 Nanocages
Nanocages are hollow nanostructures with well-defined cavities and porous walls, offering unique confinement effects and high surface areas for the adsorption of heavy metal ions. Recent studies have explored the use of nanocages derived from various materials, including metal oxides, carbon-based materials, and polymers, for heavy metal removal. The tunable pore size and surface chemistry of nanocages enable selective adsorption of specific heavy metal contaminants, making them promising candidates for water purification applications (Ahmed et al., 2023).
3 Mechanisms of heavy metal removal
Heavy metal removal from water sources involves various mechanisms, each with its advantages and limitations. These mechanisms include adsorption, precipitation, ion exchange, membrane filtration, and electrochemical methods (Figure 2), each suited to specific types of contaminants and environmental conditions (Li et al., 2022; Peng and Guo, 2020; Singh et al., 2021). The mechanisms of heavy metal removal continue to evolve with advancements in materials science, process engineering, and environmental biotechnology. Innovations such as nanomaterials, bio-based adsorbents, and hybrid systems are pushing the boundaries of efficiency and selectivity (Rajendran et al., 2022). By harnessing the synergistic effects of advanced materials, innovative process technologies, and sustainable practices, researchers and engineers can develop efficient, cost-effective, and environmentally friendly solutions for mitigating heavy metal pollution and safeguarding water quality for future generations. These integrated approaches are crucial for safeguarding water quality for future generations and ensuring compliance with stringent environmental regulations. Table 4 provides a comprehensive comparison of the key nanomaterials used for heavy metal remediation, focusing on their adsorption capacity, selectivity, regeneration efficiency, and unique characteristics.
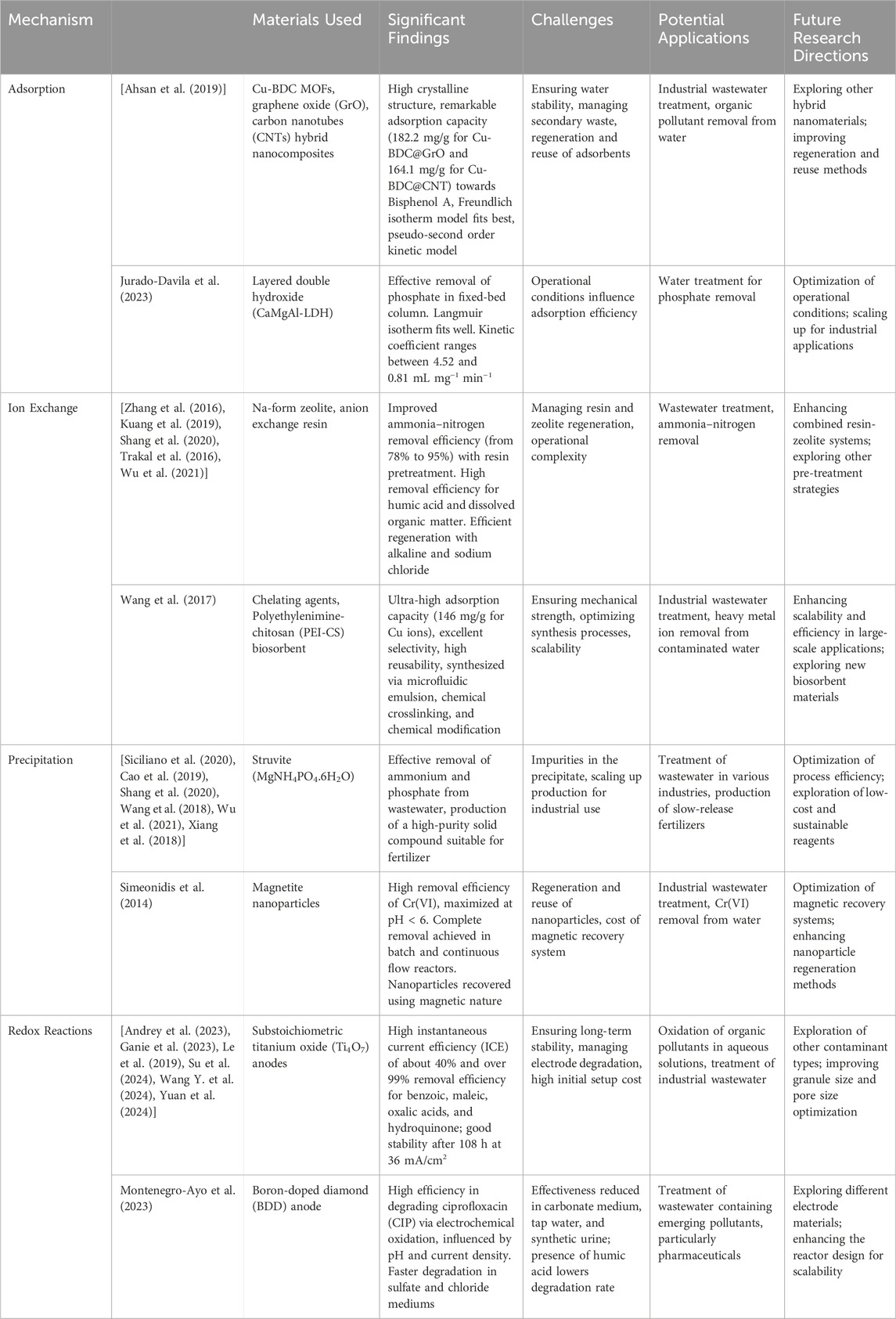
Table 4. Innovative mechanisms and materials for heavy metal and emerging pollutant removal from water.
3.1 Adsorption
Adsorption involves the attachment of heavy metal ions onto the surface of solid adsorbents, forming a monolayer or multilayer adsorption layer (Khulbe and Matsuura, 2018). The process relies on the affinity between the surface functional groups of the adsorbent and the heavy metal ions in the solution. Recent studies have focused on enhancing the adsorption capacity and selectivity of adsorbents through the use of novel materials and surface modifications. Nanomaterials, such as graphene oxide, carbon nanotubes, and metal-organic frameworks (MOFs), exhibit high surface area and tunable surface chemistry, making them effective adsorbents for heavy metal removal (Ahsan et al., 2020; Das et al., 2021). Surface functionalization with specific functional groups, such as carboxyl, amino, or thiol groups, enhances the adsorption affinity towards target heavy metal ions (Ahmad et al., 2020; Zeng et al., 2022). Additionally, hybrid materials combining different types of nanomaterials or incorporating metal nanoparticles demonstrate synergistic effects, leading to improved adsorption capacities and kinetics. Advancements in adsorption mechanisms also include the development of continuous-flow adsorption systems such as fixed-bed columns and membrane adsorbers, for practical applications (Aydına et al., 2021; Taka et al., 2021). These systems enable efficient removal of heavy metals from large volumes of water and offer advantages in terms of scalability and cost-effectiveness.
3.2 Ion exchange
Ion exchange involves the replacement of ions in solution with ions attached to a solid phase, typically an ion exchange resin or zeolite (Rathi et al., 2021; Ray, 2023). The process relies on the affinity between the ions in the solution and the functional groups on the surface of the ion exchange material. Recent trends in ion exchange mechanisms focus on improving the selectivity and regeneration capabilities of ion exchange materials. Functionalization of ion exchange resins with specific ligands or chelating agents enables selective removal of target heavy metal ions based on their charge and coordination chemistry (Quintas et al., 2021). Additionally, the regeneration of spent ion exchange materials through elution or desorption processes enables their reuse and reduces operational costs. Integration of ion exchange processes with other treatment methods, such as membrane filtration and adsorption (Peng and Guo, 2020; Vieira et al., 2020), enhances treatment efficiency, and offers synergistic effects. Moreover, advancements in process engineering, including continuous-flow ion exchange reactors and in-line monitoring techniques, enable precise control over ion exchange reactions and facilitate scale-up for industrial applications.
3.3 Precipitation
Precipitation involves the chemical reaction between heavy metal ions and precipitating agents to form insoluble precipitates, which can then be separated from the solution (Pillai and Thombre, 2024; Zhang et al., 2023). The process relies on the solubility product of the metal precipitate and the pH and temperature conditions of the solution. Recent trends in precipitation mechanisms focus on enhancing the efficiency and selectivity of precipitation reactions while minimizing the generation of secondary wastes. Advanced precipitation methods, such as co-precipitation and hydrothermal synthesis (Gholamrezaei et al., 2019), enable the synthesis of highly crystalline and uniform precipitates with controlled morphologies and compositions. The use of novel precipitating agents, including natural polymers, biodegradable chelating agents, and green chemistry reagents, reduces the environmental impact of precipitation processes and enhances the sustainability of heavy metal removal systems. Moreover, recent advancements in process engineering, such as continuous-flow precipitation reactors and in-line monitoring techniques, enable precise control over precipitation reactions and facilitate scale-up for industrial applications.
3.4 Redox reactions
Redox reactions involve the transfer of electrons between chemical species, leading to the transformation of heavy metal ions into less toxic or insoluble forms (Xu et al., 2022). The process relies on the redox potential of the metal ions and the reducing or oxidizing agents present in the solution (Gulcin and Alwasel, 2022). Recent trends in redox reactions for heavy metal removal focus on developing sustainable and energy-efficient processes with minimal environmental impact. Electrochemical methods, such as electrocoagulation, electrooxidation, and electrochemical reduction, offer selective and precise control over redox reactions, enabling the removal of specific heavy metal contaminants from complex water matrices (Feng et al., 2023; Martínez-Huitle et al., 2018; Yasri and Gunasekaran, 2017). Advanced electrode materials, including carbon-based electrodes, metal oxide nanoparticles, and conductive polymers, exhibit enhanced electrochemical activity and stability, leading to improved treatment efficiency and durability of electrochemical reactors. Integration of renewable energy sources, such as solar and wind power, into electrochemical systems reduces the carbon footprint and energy consumption of heavy metal removal processes (Ganiyu and Martinez-Huitle, 2020; Klemeš et al., 2019; Zahmatkesh et al., 2022).
4 Potential synergistic effect of nanomaterials combined with other remediation techniques
Emerging research on the environmental applications of nanomaterials has highlighted their potential to inspire the field of contaminant removal through synergy with existing remediation techniques. Traditionally, remediation methods such as adsorption, filtration, and electrochemical processes operate independently, each with its own limitations regarding efficiency, selectivity, and operational cost. However, the unique properties of nanomaterials, including tunable surface chemistry, high reactivity, and nanoscale porosity, provide a new dimension for enhancing these conventional techniques. In this section we will highlight the concepts—nanomaterial-catalyzed cascade remediation, a hybrid strategy that leverages the cooperative interaction between nanomaterials and existing techniques, forming a chain of processes that cumulatively magnify the overall contaminant removal efficiency.
4.1 Nanomaterial-driven cascade adsorption-electrochemical treatment
Unlike traditional adsorption techniques, which are limited by saturation and slow kinetic rates, nanomaterial-driven cascade systems propose a new mechanism where adsorption is not an endpoint but a trigger for secondary electrochemical reactions (Y. Zhang et al., 2023). For instance, zero-valent iron nanoparticles (ZVINPs) serve as a starting point by reducing heavy metal ions, such as Pb2⁺ and As³⁺, and simultaneously generating electrons that catalyze subsequent redox reactions in an electrochemical process (Silva-Calpa et al., 2020; Tarekegn et al., 2021; Yang et al., 2021). This cascading system ensures that once the adsorptive sites of the nanomaterials are saturated, electrochemical reduction and oxidation cycles are initiated, allowing continuous heavy metal transformation and immobilization, thus enhancing overall efficiency.
4.2 Photocatalytic-electromagnetic hybrid remediation using functional nanoparticles
The concept of coupling photocatalytic nanomaterials, such as TiO₂ nanoparticles, with electromagnetic fields opens up new avenues for intensifying contaminant degradation. In this hybrid approach, UV-activated TiO₂ nanoparticles generate reactive oxygen species (ROS) capable of breaking down organic pollutants (Park et al., 2021; Sun et al., 2020). However, instead of limiting the process to the photocatalytic activity alone, the addition of a low-frequency electromagnetic field enhances nanoparticle dispersion and accelerates the electron transfer processes involved in pollutant degradation (Cai et al., 2019; Rawat et al., 2021). This strategy increases the reaction rate and extends the lifetime of ROS, effectively expanding the scope of contaminants that can be addressed, including both heavy metals and organic pollutants.
4.3 Nanomaterial-enhanced phytomining: Harnessing biological synergy
Another concept emerging in environmental remediation is nanomaterial-enhanced phytomining, where engineered nanomaterials are combined with hyperaccumulating plants to extract valuable metals from contaminated environments (Li et al., 2020; Tognacchini et al., 2019). Traditional phytoremediation is often hindered by the slow uptake of metals and limited bioavailability (Khalid et al., 2021). By introducing metal-seeking nanoparticles, such as functionalized carbon nanotubes or metal-organic frameworks (MOFs), to the root zone of these plants, the uptake of metals such as nickel, cadmium, and zinc is greatly amplified (Doria-Manzur et al., 2022; Rossi et al., 2019). These nanoparticles enhance the bioavailability of metals by chelating them into soluble forms that are more easily transported through the plant’s vascular system, ultimately resulting in higher metal yields. This synergistic approach not only cleanses contaminated soils but also offers a novel method for recovering valuable metals, providing a sustainable alternative to traditional mining processes.
4.4 Dynamic self-regenerating systems with nanocomposites and microbial consortia
A pioneering concept is the design of dynamic self-regenerating systems, in which nanomaterials and microbial consortia work together to sustain long-term contaminant degradation without requiring external inputs (Fu et al., 2021; Xia et al., 2023). For example, magnetic nanoparticles (MNPs) can be combined with microbial biofilms capable of biodegrading organic pollutants (Liu et al., 2022; Zhu et al., 2020). The MNPs serve two primary functions: first, they adsorb heavy metals, preventing them from inhibiting microbial activity; second, they act as catalysts that regenerate the microbial degradation capacity by facilitating electron transfer between the bacteria and the contaminants. This interaction sets up a dynamic feedback loop where contaminants are continuously broken down and the system regenerates itself, maintaining efficiency over extended operational periods.
4.5 Nanoparticle-enhanced thermal remediation for heavy metal recovery
Thermal remediation methods, such as soil heating, have traditionally been used for organic pollutant removal but are less effective for heavy metal immobilization. A novel approach involves using thermally activated nanoparticles, such as aluminum oxide (Al₂O₃) or zirconium oxide (ZrO₂), which, when heated, change their surface chemistry to selectively bind and sequester heavy metals like lead and mercury (Allwin Mabes Raj et al., 2022; Fan et al., 2020; Guo et al., 2021; Huang et al., 2021). This nanoparticle-enhanced thermal remediation technique leverages the temperature-induced phase transformation in the nanoparticles, enhancing their adsorptive capacities and providing an innovative method for metal recovery during remediation processes (Al-Najar et al., 2021; Williams and Peterson, 2021).
4.6 Nanomaterial-enabled electrohydrodynamic remediation
Another concept is the nanomaterial-enabled electrohydrodynamic remediation, which combines electric fields with fluid dynamics to direct the flow of contaminants toward reactive nanomaterials embedded in porous media (Ji et al., 2023; Sprocati and Rolle, 2019). In this system, nanomaterials like functionalized graphene oxide are integrated within a hydrodynamic framework that is manipulated through external electric fields. This enables selective capture of contaminants by steering them towards nanomaterials with high affinity for specific heavy metals, ensuring high capture rates and fast remediation times (Ahmad et al., 2020; Nisola et al., 2020). Additionally, the fluid flow generated by electrohydrodynamics can be fine-tuned to optimize the contact time between the contaminants and the nanomaterials, leading to a highly efficient and targeted removal process.
5 Selectivity of nanomaterials
The selectivity of nanomaterials for heavy metal removal is crucial for ensuring efficient and targeted remediation of contaminated water sources (Tahoon et al., 2020). Surface functionalization, ligand design, and molecular imprinting represent versatile approaches for enhancing the selectivity of nanomaterials in heavy metal remediation (Rafeeq et al., 2022). By tailoring the surface chemistry, incorporating specific ligands, and imprinting molecular recognition sites, researchers can design highly selective adsorbents for targeted removal of heavy metal contaminants from water sources (Fei and Hu, 2022). Continued advancements in materials synthesis, molecular design, and characterization techniques will further accelerate the development of selective nanomaterials for sustainable water treatment applications (Saravanan et al., 2022).
5.1 Surface functionalization
Surface functionalization plays a pivotal role in tailoring the selectivity of nanomaterials by modifying their surface chemistry to preferentially adsorb specific heavy metal ions (Sarma et al., 2019). Recent research has demonstrated the efficacy of surface functionalization strategies for enhancing the selectivity of nanomaterials in heavy metal removal applications (Jawed et al., 2020). Functional groups such as carboxyl (-COOH), amino (-NH2), thiol (-SH), and hydroxyl (-OH) are commonly introduced onto the surface of nanomaterials to impart specific binding affinity towards target heavy metal ions (Rai et al., 2022). For example, graphene oxide (GO) and carbon nanotubes (CNTs) functionalized with carboxyl groups exhibit enhanced selectivity for heavy metal ions such as lead (Pb), cadmium (Cd), and mercury (Hg) due to the formation of strong metal-carboxylate complexes (Mohan et al., 2023). Similarly, metal-organic frameworks (MOFs) can be functionalized with tailored ligands to selectively adsorb specific heavy metal ions based on their size, charge, and coordination chemistry (Wen et al., 2018).
Furthermore, the development of multifunctional nanomaterials through the simultaneous incorporation of multiple functional groups enables synergistic effects and enhanced selectivity toward target heavy metal contaminants. For instance, hybrid nanocomposites composed of graphene oxide and magnetic nanoparticles functionalized with both carboxyl and amino groups demonstrate superior selectivity and adsorption capacity for heavy metal ions compared to individual components (Natarajan et al., 2023). Advancements in surface functionalization techniques, including covalent bonding, electrostatic interactions, and chemical modification, enable precise control over the surface chemistry of nanomaterials, thereby facilitating the design of highly selective adsorbents for heavy metal removal (Liu et al., 2019).
5.2 Ligand design
Ligand design represents a versatile approach for enhancing the selectivity of nanomaterials by incorporating specific chelating agents or receptors that exhibit high affinity towards target heavy metal ions (Dzhardimalieva and Uflyand, 2018). Recent studies have focused on the rational design and synthesis of ligands with tailored properties to selectively form complexes with specific heavy metal contaminants. Ligands such as crown ethers, cyclodextrins, and calixarenes offer unique binding cavities and coordination sites for selective recognition and capture of heavy metal ions (Liang et al., 2022). Functionalization of nanomaterials with ligands featuring complementary functional groups enables the formation of stable metal-ligand complexes, thereby enhancing the selectivity and affinity of nanomaterials for target heavy metal ions (de Oliveira et al., 2021).
Moreover, the integration of molecular modeling techniques, such as density functional theory (DFT) calculations and molecular dynamics simulations, aids in the rational design and optimization of ligands for selective heavy metal removal (Ebenezer and Solomon, 2024). By elucidating the underlying interactions between ligands and heavy metal ions at the molecular level, researchers can tailor the structure and properties of ligands to maximize selectivity and binding affinity (Li et al., 2023a). Furthermore, the development of stimuli-responsive ligands that undergo conformational changes or structural transformations in response to specific environmental cues offers dynamic control over the selectivity of nanomaterials (Grzelczak et al., 2019). Stimuli-responsive ligands can selectively bind heavy metal ions under certain conditions, such as pH, temperature, or redox potential, enabling on-demand capture and release of target contaminants (Mamidi et al., 2021; Sharma et al., 2024).
5.3 Molecular imprinting
Molecular imprinting is an emerging technique for imprinting specific binding sites or cavities within the structure of nanomaterials to selectively recognize and capture target heavy metal ions (Tchekwagep et al., 2022). Recent advancements in molecular imprinting technology have led to the development of highly selective nanomaterials for heavy metal removal (Ali et al., 2024). Molecularly imprinted polymers (MIPs) represent a promising class of materials that mimic the molecular recognition properties of natural receptors or antibodies (Parisi et al., 2022). By imprinting the desired heavy metal ions within a polymer matrix through template-assisted polymerization or sol-gel methods, MIPs can selectively bind and remove target contaminants from aqueous solutions (ul Gani Mir et al., 2022). The precise control over the size, shape, and functionality of imprinted cavities enables high selectivity and affinity towards specific heavy metal ions (El Ouardi et al., 2021).
Moreover, the integration of molecular imprinting technology with nanomaterials, such as graphene-based nanocomposites, metal oxide nanoparticles, and carbon nanotubes, enhances the stability, reusability, and performance of imprinted materials for heavy metal removal applications (Yahaya et al., 2021). Functionalization of nanomaterials with template molecules and cross-linking agents facilitates the formation of well-defined imprinted cavities with tailored recognition properties (Parisi et al., 2020). Furthermore, the development of smart and stimuli-responsive molecularly imprinted nanomaterials offers dynamic control over the selectivity and release of captured heavy metal ions (Li et al., 2023b). Stimuli-responsive MIPs can undergo reversible changes in their structure or properties in response to external stimuli, enabling the on-demand release of adsorbed contaminants under specific conditions (Mintz Hemed et al., 2023).
6 Regeneration strategies
Regeneration of adsorbents and other materials used in heavy metal removal processes is essential for enhancing the sustainability and cost-effectiveness of water treatment systems. Regeneration strategies play a crucial role in enhancing the sustainability and cost-effectiveness of heavy metal removal processes. Recent advancements in desorption techniques, electrochemical regeneration, and photocatalytic regeneration offer promising solutions for the efficient recovery and reuse of adsorbents and electrodes in water treatment systems.
6.1 Desorption techniques
Desorption techniques involve the removal of adsorbed heavy metal ions from the surface of adsorbent materials, allowing for the regeneration and reuse of the adsorbents. Various desorption methods have been developed to efficiently recover heavy metal ions while minimizing the generation of secondary wastes (Lata et al., 2015). Recent trends in desorption techniques focus on enhancing the efficiency and selectivity of desorption processes while reducing energy consumption and environmental impact (Kopac, 2021). Thermal desorption, which involves heating the adsorbent material to release adsorbed contaminants, remains a commonly used method due to its simplicity and effectiveness. However, advancements in alternative desorption methods, such as chemical desorption, solvent extraction, and microwave-assisted desorption, offer advantages in terms of selectivity, speed, and energy efficiency (Jalili et al., 2020).
Chemical desorption techniques involve the use of desorbing agents, such as acids, bases, or complexing agents, to break the bonds between the adsorbent and the heavy metal ions (Chatterjee and Abraham, 2019). Recent studies have investigated the use of environmentally friendly desorbing agents, such as citric acid, EDTA, and hydrochloric acid, to minimize the generation of hazardous wastes and reduce environmental impact (Golmaei et al., 2018; Yaashikaa et al., 2021). Furthermore, the development of innovative desorption techniques, such as ultrasonic-assisted desorption and supercritical fluid extraction, enables rapid and efficient recovery of heavy metal ions from adsorbent materials (Wang J. et al., 2024). These techniques offer advantages in terms of selectivity, speed, and energy efficiency, making them promising candidates for the regeneration of adsorbents in heavy metal removal systems (Shrestha et al., 2021).
6.2 Electrochemical regeneration
Electrochemical regeneration involves the application of an electric current to regenerate adsorbent materials or electrodes used in heavy metal removal processes (Ganzoury et al., 2020). The process relies on electrochemical reactions to desorb and recover heavy metal ions from the surface of the electrodes or adsorbents. Recent advancements in electrochemical regeneration techniques focus on optimizing electrode materials, operating conditions, and regeneration protocols to enhance efficiency and reduce energy consumption (Romano et al., 2020). Electrochemical regeneration methods, such as electrochemical desorption and electrodialysis, offer advantages in terms of selectivity, scalability, and environmental sustainability (Sedighi et al., 2023).
Advanced electrode materials, including carbon-based electrodes, metal oxide nanoparticles, and conductive polymers, exhibit enhanced electrochemical activity and stability, leading to improved regeneration efficiency and durability of electrochemical regeneration systems (Pan et al., 2019). Moreover, the integration of renewable energy sources, such as solar and wind power, into electrochemical regeneration systems reduces the carbon footprint and energy consumption of heavy metal removal processes (Zahmatkesh et al., 2022). Additionally, the development of smart and adaptive electrochemical regeneration systems enables real-time monitoring and control of regeneration processes, leading to enhanced efficiency and reliability (Lv et al., 2023). These systems offer advantages in terms of process optimization, resource utilization, and environmental sustainability, making them promising candidates for the regeneration of adsorbents and electrodes in heavy metal removal systems (Shrestha et al., 2021; Younas et al., 2021).
6.3 Photocatalytic regeneration
Photocatalytic regeneration involves the use of photocatalysts to facilitate the degradation or transformation of adsorbed contaminants under irradiation with light, typically ultraviolet (UV) or visible light (Liao et al., 2022). The process relies on the generation of reactive oxygen species (ROS) or photoinduced electrons and holes to oxidize or reduce the adsorbed contaminants (Afreen et al., 2020). Recent trends in photocatalytic regeneration focus on developing efficient photocatalysts with enhanced activity and stability for the degradation of heavy metal ions (Pang et al., 2024). Advanced photocatalytic materials, such as metal oxides, semiconductor nanoparticles, and carbon-based nanomaterials, exhibit high photocatalytic activity and selectivity towards specific heavy metal contaminants (Farhan et al., 2023).
Moreover, the integration of advanced reactor designs, such as photocatalytic membranes, immobilized photocatalysts, and flow-through photocatalytic reactors, enables efficient utilization of light energy and enhancement of mass transfer kinetics (Molinari et al., 2021; Wang Z. et al., 2021). These reactor designs offer advantages in terms of scalability, continuous operation, and process integration, making them suitable for practical applications in heavy metal removal systems (Qasem et al., 2021). Hence, the development of hybrid photocatalytic systems, such as photocatalytic-adsorption and photocatalytic-electrochemical systems, enables synergistic effects and enhanced regeneration efficiency. These hybrid systems offer advantages in terms of selectivity, efficiency, and versatility, making them promising candidates for the regeneration of adsorbents and electrodes in heavy metal removal systems.
7 Scalability and practical considerations
Scalability and practical considerations are critical factors in the development and implementation of heavy metal removal nanotechnologies (Ateia et al., 2024). Recent advancements in synthesis methods, stability, longevity, cost-effectiveness, and integration with existing water treatment infrastructure offer promising solutions for addressing heavy metal contamination in water sources and ensuring access to clean and safe drinking water for all (Hussain et al., 2024; Jadhao et al., 2024; Maji and Dutta, 2024).
7.1 Synthesis methods
Synthesis methods are crucial in determining the scalability, reproducibility, and properties of nanomaterials used in heavy metal removal technologies (Wawata and Fabiyi, 2024). Recent advancements in synthesis techniques focus on enhancing the scalability and cost-effectiveness of nanomaterial production while maintaining control over the properties and performance of the materials (Gohar et al., 2024; Saleh, 2024). Traditional synthesis methods, such as chemical precipitation, sol-gel, and hydrothermal synthesis, offer advantages in terms of simplicity, versatility, and scalability (Zohrabi, 2024). However, these methods may suffer from limitations in terms of particle size control, uniformity, and reproducibility (Li et al., 2023a; Lin et al., 2023). Recent trends in synthesis methods include the development of scalable and environmentally friendly approaches, such as green synthesis, microwave-assisted synthesis, and continuous-flow synthesis, which offer advantages in terms of energy efficiency, reaction control, and waste reduction (Adeola et al., 2023; Ahmed S. F. et al., 2022; Kaur et al., 2023). Moreover, advancements in bottom-up and top-down fabrication techniques enable precise control over the size, shape, and surface properties of nanomaterials, leading to improved performance and selectivity in heavy metal removal applications. Integration of advanced characterization techniques, such as electron microscopy, X-ray diffraction, and spectroscopy, facilitates real-time monitoring and optimization of synthesis processes, enabling rapid scale-up and commercialization of nanomaterial-based technologies (Abid et al., 2022; Baig et al., 2021; Chen et al., 2022; El-Khawaga et al., 2023).
7.2 Stability and longevity
Stability and longevity are critical factors in the performance and sustainability of nanomaterials used for heavy metal removal, particularly in long-term and continuous water treatment applications (Gul Zaman et al., 2021; Yu et al., 2022). While significant advancements have been made in improving the stability and durability of nanomaterials, challenges remain. Nanomaterials are prone to aggregation due to weaker intermolecular interactions, which can reduce their surface area and subsequently diminish their removal efficiency (Ahmed S. F. et al., 2022). In addition, nanomaterials, particularly nanomembranes, often suffer from mechanical instability, which results in performance degradation over time, limiting their effectiveness in long-term applications. One major issue is the environmental fate of nanomaterials, especially when they interact with microorganisms or are released into aquatic environments. Nanoparticles, such as carbon nanotubes and graphene-based materials, are prone to settling in sediments where they can harm benthic organisms. For instance, graphene oxide can produce reactive oxygen species that damage cell membranes in marine life (Saleem and Zaidi, 2020). These interactions with environmental microorganisms could lead to unintended ecological consequences, complicating the large-scale deployment of nanomaterials in water treatment systems.
The aggregation of nanomaterials in wastewater treatment plants is another significant concern. Studies have shown that a substantial proportion of nanoparticles are removed during the activated sludge process via bioadsorption onto the sludge surface. For example, nanoparticles like WO₃ and TiO₂ were found to accumulate in sludge (Simelane and Dlamini, 2019). The aggregation of nanoparticles in sludge complicates the management of waste sludge, as it can lead to potential environmental risks if not properly managed. To address these challenges, surface modification techniques have been developed to enhance the stability and dispersibility of nanomaterials. Functionalization with stabilizing agents, encapsulation within protective coatings, and immobilization on support matrices can extend the operational lifespan of nanomaterials by preventing aggregation and degradation in harsh water treatment environments (Alipour Atmianlu et al., 2021). For instance, by reducing aggregation and enhancing dispersibility, these modifications can significantly extend the operational lifespan of nanomaterials. However, long-term environmental assessments are still necessary, as exposure to various environmental factors can alter the size, composition, and stability of these materials, potentially changing their behaviour and environmental impact (Simeonidis et al., 2019). Nanoparticles such as Ag and ZnO have been reported to negatively affect phytoplankton and diatoms, demonstrating the need for more research on the environmental impact of spent nanomaterials.
In addition to these stability issues, proper disposal and recyclability of spent nanomaterials are crucial for ensuring sustainable applications. Recycling methods, such as using spent nanoparticles in brick manufacturing or disposing them in controlled landfills, have been suggested, but long-term evaluation is required, as many approaches have only been tested at the laboratory scale (Prathna et al., 2018). Clear guidelines on nanomaterial disposal are also necessary to avoid unintended environmental contamination when scaling up their use in industrial applications. Despite these challenges, advancements in nanocomposite and hybrid materials show promise for enhancing stability and longevity. For example, combining different nanomaterials can create synergistic effects, improving their structural integrity and resistance to environmental degradation (Hassan et al., 2021). Integrating nanomaterials with biodegradable or natural matrices also enhances their environmental compatibility while maintaining high removal efficiency.
7.3 Cost-effectiveness
Cost-effectiveness is a critical consideration in developing and implementing heavy metal removal technologies, particularly for large-scale and decentralized water treatment systems (Ayach et al., 2024; Neisan et al., 2023). Several economic factors can mitigate against investing in nanomaterial-based remediation technologies. The running cost of the technologies can be huge. This may be due to the raw materials used, the synthesis method adopted, specialized equipment and facilities, energy consumption cost, labour, and environmental and health risk assessment costs (Asghar et al., 2024).
However, recent advancements in materials synthesis, process engineering, and system design focus on reducing costs while maintaining performance and reliability (Chai et al., 2021; Gupta et al., 2021). The cost of nanomaterials, energy consumption, and operational maintenance are primary factors influencing the overall cost-effectiveness of heavy metal removal technologies. Recent trends in materials synthesis emphasize scalable and low-cost approaches, such as template-assisted synthesis, self-assembly, and waste-derived materials, to reduce production costs and enhance affordability (Abdullahi et al., 2024; Luo et al., 2021).
Furthermore, advancements in process engineering, such as optimization of reactor design, flow rate control, and automation, enable efficient utilization of resources and energy, leading to reduced operational costs and improved cost-effectiveness (Constance Obiuto et al., 2024). Integration of renewable energy sources, such as solar and wind power, into water treatment systems further enhances sustainability and reduces operating expenses (Shokri and Sanavi Fard, 2022). Moreover, life cycle cost analysis and techno-economic assessments provide valuable insights into the cost-effectiveness and feasibility of heavy metal removal technologies, guiding decision-making and investment strategies for water treatment infrastructure (Ćetković et al., 2022; Ilyas et al., 2021; Kehrein et al., 2021).
Specifically, to address scalability, cost-effectiveness, and environmental sustainability challenges associated with the use of nanoparticles for heavy metal remediation, there is a need to improve surface functionalization. When nanoparticle surfaces are optimally functionalized, the tendency for aggregation is reduced. This will ultimately improve its stability. Also, using green chemistry and biological methods of synthesis would tremendously reduce the cost associated with the use of nano-based heavy metal remediation. Furthermore, optimizing the magnetic properties of nanoparticles to improve their separation from the environment will increase recovery and reusability (Asghar et al., 2024).
7.4 Integration with existing water treatment infrastructure
Integrating nanomaterials with existing water treatment technologies, such as membrane filtration, electrochemical treatment, and biological remediation, presents an opportunity to enhance the efficiency of heavy metal removal (Elgarahy et al., 2021; Khan et al., 2021). Recent efforts focus on hybrid systems that leverage the unique properties of nanomaterials alongside traditional remediation techniques, offering synergistic effects that can improve selectivity, efficiency, and fouling resistance (Adeola and Forbes, 2021; Pérez et al., 2023; Thakur and Kumar, 2022). For instance, membrane-based nanocomposites embedded with functionalized nanoparticles have demonstrated superior performance in selectively removing heavy metals from water while also improving membrane durability and reducing fouling.
The successful incorporation of nanomaterials into current water treatment systems is exemplified by ceramic disk filters coated with nano-ZnO. These filters, which utilize the photocatalytic antibacterial properties of ZnO to reduce Escherichia coli in drinking water, offer a cost-effective solution, particularly beneficial for remote and rural communities (Huang et al., 2018). This hybrid approach, combining traditional filtration methods with nanomaterials, provides a low-cost upgrade to existing systems, enhancing water safety without requiring substantial modifications. However, integrating nanomaterials into large-scale WWTPs presents unique challenges, as demonstrated by studies on the behaviour of AgNPs in wastewater treatment processes. Research indicates that during wastewater treatment, AgNPs undergo sulfidation, particularly in anaerobic zones, converting them to Ag₂S. This transformation reduces their effectiveness in contaminant removal (Kent et al., 2014). This transformation complicates the integration of nanomaterials in large-scale systems, due to the altered adsorption properties of the nanoparticles.
Further challenges include the handling of nanomaterials in the sludge generated by wastewater treatment processes. A recent study (Cervantes-Avilés and Keller, 2021) demonstrated that while WWTPs can remove between 84% and 99% of metal-based nanoparticles from influent wastewater, substantial concentrations of nanoparticles, especially Mg, Ni, and Cd, still accumulate in the waste sludge. The accumulation of nanoparticles in waste sludge requires careful consideration during disposal, as the potential release of nanomaterials into the environment could pose additional risks. This was affirmed by another study that researched integrating WO₃ and TiO₂ mixtures into a wastewater treatment plant (Simelane and Dlamini, 2019). The nanoparticles were mainly adsorbed onto the sludge and removed from the wastewater, with the activated sludge process proving effective in their elimination. However, the long-term fate of these nanoparticles, including the stability of their polymorphs like monoclinic WO₃ and anatase TiO₂, remains a concern for sludge management strategies. Moreover, a study on silver nanoparticles in municipal WWTPs demonstrated that both mechanical and biological treatments are effective in reducing nanoscale silver particles from wastewater, with a 95% reduction achieved by combining these processes (Li et al., 2013). However, despite this high reduction rate, residual concentrations of n-Ag-Ps in the effluent still pose a challenge for WWTPs, particularly when scaling up the technology for larger plants.
System optimization and advancements in process integration have facilitated the incorporation of nanomaterials into conventional water treatment infrastructure. For instance, modular approaches allow for easier retrofitting of remediation units, enabling the deployment of nanomaterials without extensive changes to existing systems (Ruíz-Baltazar, 2024; Vasoya, 2023). However, the transformation, stability, and long-term behaviour of nanomaterials within these systems, particularly under varying environmental conditions, remain areas of concern. Collaborative efforts between nanotechnology experts, environmental engineers, and materials scientists are essential for overcoming these challenges and realizing the full potential of nanomaterials in integrated water treatment solutions.
Overall, integrating nanomaterial with existing water treatment infrastructure is essential for the practical implementation and adoption of heavy metal removal technologies in real-world settings (Singh et al., 2023). Recent advancements in system design, modularization, and process optimization focus on compatibility and interoperability with existing treatment processes and infrastructure (Brad et al., 2021). Heavy metal removal technologies should be designed to complement and integrate seamlessly with conventional water treatment processes, such as coagulation-flocculation, sedimentation, filtration, and disinfection (Khan Khanzada et al., 2023; Vidu et al., 2020). Modular and scalable designs enable flexible deployment and retrofitting of treatment units within existing infrastructure, facilitating gradual upgrades and expansions according to specific water quality and capacity requirements (Brears, 2021; Daigger et al., 2020; Leigh and Lee, 2019). Moreover, advancements in sensor technology, remote monitoring, and automation enable real-time data acquisition, process control, and performance optimization, enhancing the operational efficiency and reliability of integrated water treatment systems (Martínez et al., 2020; Park et al., 2020; Zainurin et al., 2022; Zhang W. et al., 2020). Integration of smart sensors and IoT-enabled devices facilitates remote monitoring and control of treatment processes, enabling predictive maintenance and early detection of system failures.
8 Challenges and future directions
Addressing heavy metal contamination in water sources poses significant challenges, ranging from environmental impact assessment to regulatory compliance and scaling up production. Addressing the challenges of heavy metal contamination in water sources requires multidisciplinary approaches, collaboration, and innovation (Chernov et al., 2024; Ding, 2024; Kupa et al., 2024).
8.1 Environmental impact assessment
Nanoparticles could be optimized to become highly reusable. For instance, platinum magnesium and copper oxide nanoparticles have been reused in literature. However, when nanoparticles are not properly managed, they could accumulate in the environment due to their long-term stability. They can react with organic matter in the environment to cause environmental damage, and health issues such as apoptosis, oxidative stress, etc (Fu et al., 2020; Imran et al., 2021; Wahl et al., 2021; Zhang X. et al., 2020).
One of the primary challenges in heavy metal remediation is conducting comprehensive environmental impact assessments to evaluate the potential risks and benefits associated with remediation technologies (Khatun et al., 2024; Rashid et al., 2023). While nanomaterials offer promising solutions for heavy metal removal, concerns remain regarding their environmental fate, toxicity, and long-term effects on ecosystems. Recent studies have focused on assessing the environmental implications of nanomaterial-based remediation technologies through rigorous toxicity testing, fate and transport modelling, and ecological risk assessments (Isibor, 2024; Isibor et al., 2024; Prasad and Gupta, 2024; Shanker, 2024). These efforts aim to identify potential environmental hotspots, evaluate exposure pathways, and mitigate adverse impacts on aquatic organisms, soil microbiota, and human health. Moreover, advancements in life cycle assessment (LCA) and environmental footprint analysis enable holistic evaluation of the environmental impacts of heavy metal remediation technologies, considering factors such as energy consumption, resource utilization, and waste generation (Ding et al., 2024; Pandit et al., 2024; Rothee et al., 2024). Integration of environmental sustainability criteria into the design and implementation of remediation strategies ensures responsible stewardship of natural resources and minimizes unintended consequences.
8.2 Regulatory compliance
Regulatory compliance is another significant challenge in the development and deployment of heavy metal remediation technologies, as stringent regulations govern the use, disposal, and discharge of contaminants in water sources. Recent trends in regulatory compliance focus on harmonizing standards and guidelines for heavy metal concentrations in drinking water, surface water, and wastewater effluents to ensure the protection of human health and the environment (Kumar and Samadder, 2023; Shaikh and Birajdar, 2024). Regulatory agencies, such as the Environmental Protection Agency (EPA) in the United States and the European Chemicals Agency (ECHA) in Europe, play a crucial role in setting and enforcing regulatory requirements for heavy metal remediation technologies (Nwokediegwu et al., 2024). Moreover, advancements in risk-based approaches and adaptive management strategies enable flexible and pragmatic regulatory frameworks that balance environmental protection with technological innovation (Gikay, 2024; Dada et al., 2024). Collaborative efforts between government agencies, industry stakeholders, and research institutions facilitate knowledge exchange, capacity building, and continuous improvement in regulatory compliance.
8.3 Scaling up production
Scaling up the production of nanomaterial-based remediation technologies from laboratory-scale prototypes to commercially viable systems poses practical challenges, including cost considerations, process optimization, and supply chain management. Recent advancements in scaling up production focus on optimizing synthesis methods, improving materials efficiency, and streamlining manufacturing processes to reduce production costs and enhance scalability (Ganguly et al., 2024; Palit and Ranjit, 2024; Tyagi et al., 2024). Automation, robotics, and process intensification techniques enable high-throughput production of nanomaterials with consistent quality and performance (Aithal and Aithal, 2024; Darwish et al., 2024). Furthermore, partnerships between academia, industry, and government agencies facilitate technology transfer, knowledge dissemination, and capacity building to accelerate the commercialization of nanomaterial-based remediation technologies (Kumar et al., 2023). Investment in research infrastructure, pilot-scale testing facilities, and demonstration projects enables validation of scalability and performance under real-world conditions.
8.4 Multifunctional nanomaterials
Multifunctional nanomaterials offer exciting opportunities for addressing multiple challenges in heavy metal remediation simultaneously, including selectivity, stability, and regeneration (Feisal et al., 2024; Nikić et al., 2024). Recent trends in multifunctional nanomaterials focus on integrating multiple functionalities, such as adsorption, catalysis, and sensing, into a single platform to enhance performance and versatility in heavy metal removal applications (Asghar et al., 2024; Godja and Munteanu, 2024; Shanmugavel et al., 2024). For example, nanocomposites composed of graphene oxide, metal nanoparticles, and molecularly imprinted polymers exhibit synergistic effects and enhanced selectivity for specific heavy metal contaminants. Moreover, advancements in responsive and stimuli-triggered nanomaterials enable dynamic control over adsorption, desorption, and regeneration processes, enhancing efficiency and sustainability (Makaev et al., 2023; Shahrokhinia et al., 2024; Zhou et al., 2021).
However, designing multifunctional nanomaterials also presents several challenges. The complexity of synthesizing materials that combine multiple functionalities while maintaining structural integrity and long-term stability is a significant barrier. For instance, some multifunctional materials, such as those used for both adsorption and catalysis, must balance these functions without compromising their effectiveness in any particular role (Li and Liu, 2023). Additionally, the recovery and recyclability of these materials are critical for large-scale applications. Magnetic nanomaterials, such as ZnO-Fe3O4 composites, have shown promise in this regard due to their ability to be easily separated from treated water using external magnets, thus enhancing the regeneration process and overall cost-effectiveness (Goyal et al., 2018). Furthermore, multifunctional nanomaterials must maintain high selectivity in complex water matrices containing competing ions, a factor that often limits their performance (Baby et al., 2022).
Despite these challenges, advancements in stimuli-responsive and smart nanomaterials have opened new avenues for overcoming these limitations. Materials that can dynamically alter their adsorption properties based on environmental conditions, such as pH-responsive poly 4-hydroxyphenyl methacrylate single-walled carbon nanotube (PHPMA-SWCNT) nanocomposites, have demonstrated the ability to selectively target heavy metals like Pb2⁺ and Cd2⁺, even in the presence of multiple interfering ions (Mamidi et al., 2021). This adaptability enhances the material’s performance in real-world applications, where water compositions can vary significantly. A practical example of multifunctionality can be seen in the work by Goyal et al. (2018), where ZnO-Fe3O4 (ZF) nanocomposites were successfully used for simultaneous adsorption of heavy metals, degradation of organic dyes, and antibacterial activity. This multifunctional performance is crucial for dealing with complex contamination scenarios, such as those found in industrial effluents, where pollutants often coexist. Moreover, the ZF nanocomposite could be easily recovered using magnetic separation and reused multiple times, offering a sustainable solution for water treatment.
Further practical examples of multifunctional nanomaterials include the multifunctional nanomaterials is the biomaterial-functionalized graphene-magnetite (Bio-GM) nanocomposite, developed by Ramalingam et al., 2018, which addresses the challenges of colloidal stability and recyclability in graphene-based materials. By incorporating Shewanella oneidensis cells into the nanocomposite, Bio-GM efficiently adsorbed both dyes and Cr⁶⁺, with removal capacities of 189.63 mg/g for dyes and 222.2 mg/g for Cr⁶⁺. Additionally, the nanocomposite facilitated the biocatalytic reduction of Cr⁶⁺ to Cr³⁺, demonstrating its multifunctionality. Bio-GM could be regenerated and reused without releasing harmful components, making it a sustainable option for water treatment. In another example, El Mouden et al., 2023 synthesized NC@Co₃O₄ nanocomposites by co-precipitating natural clay with cobalt oxide nanoparticles for heavy metal removal. These nanocomposites exhibited high adsorption efficiencies for Pb2⁺ and Cd2⁺, with rates of 86.89% and 82.06%, respectively.
9 Conclusion
Heavy metal contamination poses a significant threat to environmental and public health, necessitating effective remediation strategies. Nanomaterial-based approaches offer promising solutions for heavy metal removal from water sources, leveraging the unique properties of nanomaterials to enhance selectivity, efficiency, and sustainability. Through advancements in synthesis methods, surface functionalization, and integration with existing water treatment infrastructure, nanomaterials have demonstrated remarkable potential in addressing the challenges of heavy metal pollution. However, several critical aspects must be considered to ensure the successful implementation of nanomaterial-based remediation technologies. Environmental impact assessment and regulatory compliance are paramount to mitigating potential risks and ensuring the responsible use of nanomaterials in water treatment applications. Additionally, scalability, cost-effectiveness, and integration with other remediation techniques are essential considerations for practical implementation and widespread adoption of nanomaterial-based solutions. Furthermore, ongoing research efforts should focus on addressing key challenges such as the stability, longevity, and multifunctionality of nanomaterials to enhance their performance and reliability in real-world settings. Collaboration between key stakeholders in academia, industry, government agencies, and communities is essential to drive innovation, foster technology transfer, and accelerate the translation of research findings into actionable solutions.
Author contributions
DBO: Conceptualization, Methodology, Project administration, Resources, Supervision, Validation, Writing–original draft, Writing–review and editing. OZW: Conceptualization, Formal Analysis, Methodology, Writing–original draft, Writing–review and editing. BIE: Data curation, Writing–original draft, Writing–review and editing. OF: Writing–original draft, Writing–review and editing. AOI: Data curation, Software, Visualization, Writing–original draft, Writing–review and editing. SOU: Writing–original draft, Writing–review and editing. OA: Writing–original draft, Writing–review and editing.
Funding
The author(s) declare that no financial support was received for the research, authorship, and/or publication of this article.
Conflict of interest
The authors declare that the research was conducted in the absence of any commercial or financial relationships that could be construed as a potential conflict of interest.
Publisher’s note
All claims expressed in this article are solely those of the authors and do not necessarily represent those of their affiliated organizations, or those of the publisher, the editors and the reviewers. Any product that may be evaluated in this article, or claim that may be made by its manufacturer, is not guaranteed or endorsed by the publisher.
References
Abdel Salam, M., Owija, N. Y., and Kosa, S. A. (2020). Removal of the toxic cadmium ions from aqueous solutions by zero-valent iron nanoparticles. Int. J. Environ. Sci. Technol. 18, 2391–2404. doi:10.1007/s13762-020-02990-9
Abdullahi, S. S., Mohammad, R. E. A., Jagaba, A. H., Musa, H., and Birniwa, A. H. (2024). Natural, synthetic, and composite materials for industrial effluents treatment: a mini review on current practices, cost-effectiveness, and sustainability. Case Stud. Chem. Environ. Eng. 9, 100570. doi:10.1016/j.cscee.2023.100570
Abid, N., Khan, A. M., Shujait, S., Chaudhary, K., Ikram, M., Imran, M., et al. (2022). Synthesis of nanomaterials using various top-down and bottom-up approaches, influencing factors, advantages, and disadvantages: a review. Adv. Colloid Interface Sci. 300, 102597. doi:10.1016/j.cis.2021.102597
Adeola, A. O., Duarte, M. P., and Naccache, R. (2023). Microwave-assisted synthesis of carbon-based nanomaterials from biobased resources for water treatment applications: emerging trends and prospects. Front. Carbon 2, 1220021. doi:10.3389/frcrb.2023.1220021
Adeola, A. O., and Forbes, P. B. C. (2021). Advances in water treatment technologies for removal of polycyclic aromatic hydrocarbons: existing concepts, emerging trends, and future prospects. Water Environ. Res. 93, 343–359. doi:10.1002/wer.1420
Afreen, G., Shoeb, M., and Upadhyayula, S. (2020). Effectiveness of reactive oxygen species generated from rGO/CdS QD heterostructure for photodegradation and disinfection of pollutants in waste water. Mater. Sci. Eng. C 108, 110372. doi:10.1016/j.msec.2019.110372
Ahmad, S. Z. N., Salleh, W. N. W., Ismail, A. F., Yusof, N., Yusop, M. Z. M., and Aziz, F. (2020). Adsorptive removal of heavy metal ions using graphene-based nanomaterials: toxicity, roles of functional groups and mechanisms. Chemosphere 248, 126008. doi:10.1016/j.chemosphere.2020.126008
Ahmed, M. T., Roy, D., Al Roman, A., Islam, S., and Ahmed, F. (2023). A first-principles investigation of Cr adsorption on C 8 and B 4 N 4 nanocages in aqueous mediums. Phys. Chem. Chem. Phys. 25, 32261–32272. doi:10.1039/d3cp04225a
Ahmed, S. F., Mofijur, M., Ahmed, B., Mehnaz, T., Mehejabin, F., Maliat, D., et al. (2022a). Nanomaterials as a sustainable choice for treating wastewater. Environ. Res. 214, 113807. doi:10.1016/j.envres.2022.113807
Ahmed, S. F., Mofijur, M., Rafa, N., Chowdhury, A. T., Chowdhury, S., Nahrin, M., et al. (2022b). Green approaches in synthesising nanomaterials for environmental nanobioremediation: technological advancements, applications, benefits and challenges. Environ. Res. 204, 111967. doi:10.1016/j.envres.2021.111967
Ahsan, A., Jabbari, V., Islam, T., Turley, R. S., Dominguez, N., Kim, H., et al. (2019). Sustainable synthesis and remarkable adsorption capacity of MOF/graphene oxide and MOF/CNT based hybrid nanocomposites for the removal of Bisphenol A from water. Sci. Total Environ. 673, 306–317. doi:10.1016/j.scitotenv.2019.03.219
Ahsan, M. A., Jabbari, V., Imam, M. A., Castro, E., Kim, H., Curry, M. L., et al. (2020). Nanoscale nickel metal organic framework decorated over graphene oxide and carbon nanotubes for water remediation. Sci. Total Environ. 698, 134214. doi:10.1016/j.scitotenv.2019.134214
Aithal, S., and Aithal, P. S. (2024). Predictive analysis of use of AI-driven GPTs in nanomaterials research breakthroughs in the 21st century. IJAEML, 131–144. doi:10.47992/IJAEML.2581.7000.0226
Akhter, F., Zoppas, F. M., Soomro, M., Jatoi, A. S., Noureen, F., Akhtar, M. N., et al. (2023). Carbon-based sorbets for heavy metal removal from aqueous solution, discrepancies, and future prospects: a state-of-the-art review. Biomass Convers. Biorefinery 13, 10343–10359. doi:10.1007/s13399-021-01866-3
Ali, Q., Zia, M. A., Kamran, M., Shabaan, M., Zulfiqar, U., Ahmad, M., et al. (2023). Nanoremediation for heavy metal contamination: a review. Hybrid. Adv. 4, 100091. doi:10.1016/j.hybadv.2023.100091
Ali, Y. A., Azzouz, A., Ahrouch, M., Lamaoui, A., Raza, N., and Lahcen, A. A. (2024). Molecular imprinting technology for next-generation water treatment via photocatalysis and selective pollutant adsorption. J. Environ. Chem. Eng. 12 (3), 112768. doi:10.1016/j.jece.2024.112768
Alipour Atmianlu, P., Badpa, R., Aghabalaei, V., and Baghdadi, M. (2021). A review on the various beds used for immobilization of nanoparticles: overcoming the barrier to nanoparticle applications in water and wastewater treatment. J. Environ. Chem. Eng. 9, 106514. doi:10.1016/j.jece.2021.106514
Allwin Mabes Raj, A. F. P., Bauman, M., Lakić, M., Dimitrušev, N., Lobnik, A., and Košak, A. (2022). Removal of Pb2+, CrT, and Hg2+ ions from aqueous solutions using amino-functionalized magnetic nanoparticles. Int. J. Mol. Sci. 23, 16186. doi:10.3390/ijms232416186
Al-Najar, B., Younis, A., Hazeem, L., Sehar, S., Rashdan, S., Shaikh, M. N., et al. (2021). Thermally induced oxygen related defects in eco-friendly ZnFe2O4 nanoparticles for enhanced wastewater treatment efficiencies. Chemosphere 288, 132525. doi:10.1016/j.chemosphere.2021.132525
Andrey, K., Moroz, I., Guliaeva, V., Prokhorov, Y., Klevtsova, A., and Mareev, S. (2023). Electrochemical oxidation of organic pollutants in aqueous solution using a Ti4O7 particle anode. Membranes 13 (5), 521. doi:10.3390/membranes13050521
Asghar, N., Hussain, A., Nguyen, D. A., Ali, S., Hussain, I., Junejo, A., et al. (2024). Advancement in nanomaterials for environmental pollutants remediation: a systematic review on bibliometrics analysis, material types, synthesis pathways, and related mechanisms. J. Nanobiotechnology 22 (1), 26. doi:10.1186/s12951-023-02151-3
Aslam, M. M. A., Kuo, H.-W., Den, W., Sultan, M., Rasool, K., and Bilal, M. (2022). “Recent trends of carbon nanotubes and chitosan composites for hexavalent chromium removal from aqueous samples,” in Separation science and technology. Elsevier.
Ateia, M., Wei, H., and Andreescu, S. (2024). Sensors for emerging water contaminants: overcoming roadblocks to innovation. Environ. Sci. Technol. 58, 2636–2651. doi:10.1021/acs.est.3c09889
Ayach, J., El Malti, W., Duma, L., Lalevée, J., Al Ajami, M., Hamad, H., et al. (2024). Comparing conventional and advanced approaches for heavy metal removal in wastewater treatment: an in-depth review emphasizing filter-based strategies. Polymers 16, 1959. doi:10.3390/polym16141959
Aydına, S., Nura, H. M., Traorea, A. M., Yıldırımb, E., and Emikb, S. (2021). Fixed bed column adsorption of vanadium from water using amino-functional polymeric adsorbent. Desalination Water Treat. 209, 280–288. doi:10.5004/dwt.2021.26493
Baby, R., Hussein, M. Z., Abdullah, A. H., and Zainal, Z. (2022). Nanomaterials for the treatment of heavy metal contaminated water. Polym. (Basel) 14, 583. doi:10.3390/polym14030583
Baig, N., Kammakakam, I., and Falath, W. (2021). Nanomaterials: a review of synthesis methods, properties, recent progress, and challenges. Mater. Adv. 2, 1821–1871. doi:10.1039/D0MA00807A
Bazarin, G., Módenes, A. N., Espinoza-Quiñones, F. R., Borba, C. E., Trigueros, D. E. G., and Dall’Oglio, I. C. (2024). High removal performance of reactive blue 5G dye from industrial dyeing wastewater using biochar in a fixed-bed adsorption system: approaches and insights based on modeling, isotherms, and thermodynamics study. J. Environ. Chem. Eng. 12, 111761. doi:10.1016/j.jece.2023.111761
Borji, H., Ayoub, G. M., Bilbeisi, R., Nassar, N., and Malaeb, L. (2020). How effective are nanomaterials for the removal of heavy metals from water and wastewater? Water, Air, and Soil Pollut. 231, 330–335. doi:10.1007/s11270-020-04681-0
Brad, S., Murar, M., Vlad, G., Brad, E., and Popanton, M. (2021). Lifecycle design of disruptive SCADA systems for waste-water treatment installations. Sustainability 13, 4950. doi:10.3390/su13094950
Brears, R. C. (2021). Water resources management: innovative and green solutions. De Gruyter. doi:10.1515/9783110685640
Cai, J., Huang, J., Wang, S., Iocozzia, J., Sun, Z., Sun, J., et al. (2019). Crafting mussel-inspired metal nanoparticle-decorated ultrathin graphitic carbon nitride for the degradation of chemical pollutants and production of chemical resources. Adv. Mater. 31, e1806314. doi:10.1002/adma.201806314
Cao, Y., Xiao, W., Shen, G., Ji, G., Zhang, Y., Gao, C., et al. (2019). Carbonization and ball milling on the enhancement of Pb(II) adsorption by wheat straw: competitive effects of ion exchange and precipitation. Bioresour. Technol. 273, 70–76. doi:10.1016/j.biortech.2018.10.065
Cbn, H. M. A., Madhavi, V., Ahmad, A., and Madhavi, G. (2020). Heavy metals removal using carbon based nanocomposites. Environ. Remediat. through Carbon Based Nano Compos. 249, 249–274. doi:10.1007/978-981-15-6699-8_12
Cervantes-Avilés, P., and Keller, A. A. (2021). Incidence of metal-based nanoparticles in the conventional wastewater treatment process. Water Res. 189, 116603. doi:10.1016/j.watres.2020.116603
Ćetković, J., Knežević, M., Lakić, S., Žarković, M., Vujadinović, R., Živković, A., et al. (2022). Financial and economic investment evaluation of wastewater treatment plant. Water 14, 122. doi:10.3390/w14010122
Chai, W. S., Cheun, J. Y., Kumar, P. S., Mubashir, M., Majeed, Z., Banat, F., et al. (2021). A review on conventional and novel materials towards heavy metal adsorption in wastewater treatment application. J. Clean. Prod. 296, 126589. doi:10.1016/j.jclepro.2021.126589
Chandrashekhar Nayak, M., Isloor, A. M., Lakshmi, B., Marwani, H. M., and Khan, I. (2020). Polyphenylsulfone/multiwalled carbon nanotubes mixed ultrafiltration membranes: fabrication, characterization and removal of heavy metals Pb2+, Hg2+, and Cd2+ from aqueous solutions. Arabian J. Chem. 13, 4661–4672. doi:10.1016/j.arabjc.2019.10.007
Chatterjee, A., and Abraham, J. (2019). Desorption of heavy metals from metal loaded sorbents and e-wastes: a review. Biotechnol. Lett. 41, 319–333. doi:10.1007/s10529-019-02650-0
Chen, F., Yan, T.-H., Bashir, S., and Liu, J. L. (2022). “Synthesis of nanomaterials using top-down methods,” in Advanced nanomaterials and their applications in renewable energy (Elsevier), 37–60. doi:10.1016/B978-0-323-99877-2.00007-2
Chernov, I., Elsler, M., Maillart, T., Cacciatori, C., Tavazzi, S., Gawlik, B. M., et al. (2024). Innovative solutions for global water quality challenges: insights from a collaborative hackathon event. Front. Water 6, 1363116. doi:10.3389/frwa.2024.1363116
Constance Obiuto, N., Ninduwezuor-Ehiobu, N., Chigozie Ani, E., Olu-lawal, K. A., and Ugwuanyi, E. D. (2024). Simulation-driven strategies for enhancing water treatment processes in chemical engineering: addressing environmental challenges. Eng. Sci. Technol. J. 5, 854–872. doi:10.51594/estj.v5i3.942
Dada, M. A., Oliha, J. S., Majemite, M. T., Obaigbena, A., and Biu, P. W. (2024). A review of predictive analytics in the exploration and management of u.s. geological resources. Eng. Sci. Technol. J. 5, 313–337. doi:10.51594/estj.v5i2.763
Daigger, G. T., Voutchkov, N., Lall, U., and Sarni, W. (2020). A collection of essays on “disruptive” technologies that may transform the water sector in the next 10 years. The Future of Water. doi:10.18235/0001666
Darwish, M. A., Abd-Elaziem, W., Elsheikh, A., and Zayed, A. A. (2024). Advancements in nanomaterials for nanosensors: a comprehensive review. Nanoscale Adv. 10, 4015–4046. doi:10.1039/D4NA00214H
Darwish, M. S., Mostafa, M. H., and Al-Harbi, L. M. (2022). Polymeric nanocomposites for environmental and industrial applications. Int. J. Mol. Sci. 23 (3), 1023. doi:10.3390/ijms23031023
Das, P. N., Jithesh, K., and Raj, K. G. (2021). Recent developments in the adsorptive removal of heavy metal ions using metal-organic frameworks and graphene-based adsorbents. J. Indian Chem. Soc. 98 (11), 100188. doi:10.1016/j.jics.2021.100188
De Oliveira, H. A. L., Campos, A. F. C., Gomide, G., Zhang, Y., and Ghoshal, S. (2020). Elaboration of a core@ shell bimagnetic nanoadsorbent (CoFe2O4@ γ-Fe2O3) for the removal of as (V) from water. Colloids Surfaces A Physicochem. Eng. Aspects 600, 125002. doi:10.1016/j.colsurfa.2020.125002
De Oliveira, R. F., Montes-García, V., Ciesielski, A., and Samorì, P. (2021). Harnessing selectivity in chemical sensing via supramolecular interactions: from functionalization of nanomaterials to device applications. Mater. Horizons 8 (10), 2685–2708. doi:10.1039/d1mh01117k
Dey, K., Koner, K., Mukhopadhyay, R. D., Shetty, D., and Banerjee, R. (2024). Porous organic nanotubes: chemistry of one-dimensional space. Accounts Chem. Res. 57, 1839–1850. doi:10.1021/acs.accounts.4c00224
Ding, N., Meng, X., Zhang, Z., Ma, J., Shan, Y., Zhong, Z., et al. (2024). A review of life cycle assessment of soil remediation technology: method applications and technological characteristics. Rev. Env.Contamination formerly:Residue Rev. 262, 4. doi:10.1007/s44169-023-00051-z
Ding, Y. (2024). A bibliographic exploration: tackling heavy metal contamination. doi:10.20944/preprints202401.0625.v1
Donga, C., Mishra, S. B., Abd-El-Aziz, A. S., and Mishra, A. K. (2021). Advances in graphene-based magnetic and graphene-based/TiO2 nanoparticles in the removal of heavy metals and organic pollutants from industrial wastewater. J. Inorg. Organomet. Polym. Mater. 31 (2), 463–480. doi:10.1007/s10904-020-01679-3
Doria-Manzur, A., Sharifan, H., and Tejeda-Benítez, L. P. (2022). Application of zinc oxide nanoparticles to promote remediation of nickel by Sorghum bicolor: metal ecotoxic potency and plant response. Int. J. Phytoremediation 25, 98–105. doi:10.1080/15226514.2022.2060934
Dzhardimalieva, G. I., and Uflyand, I. E. (2018). Design strategies of metal complexes based on chelating polymer ligands and their application in nanomaterials science. J. Inorg. Organomet. Polym. Mater. 28, 1305–1393. doi:10.1007/s10904-018-0841-8
Ebenezer, C., and Solomon, R. V. (2024). Computational tools and techniques in designing ligands for the selective separation of actinide and lanthanide: a review. Comments Inorg. Chem. 10, 385–459. doi:10.1080/02603594.2024.2305884
Edo, G. I., Samuel, P. O., Oloni, G. O., Ezekiel, G. O., Ikpekoro, V. O., Obasohan, P., et al. (2024). Environmental persistence, bioaccumulation, and ecotoxicology of heavy metals. Chem. Ecol. 40, 322–349. doi:10.1080/02757540.2024.2306839
Egbewole, B. I., Ogunsile, B. O., Adeola, A. O., Olawade, D. B., Adekunle, Y. A., and Nomngongo, P. N. (2022). Mallotus oppositifolius-mediated biosynthesis of bimetallic nanoparticles of silver and nickel: antimicrobial activity and plausible mechanism (s) of action. Biomass Convers. Biorefinery 14, 14083–14094. doi:10.1007/s13399-022-03463-4
El-Aswar, E. I., Ramadan, H., Elkik, H., and Taha, A. G. (2022). A comprehensive review on preparation, functionalization and recent applications of nanofiber membranes in wastewater treatment. J. Environ. Manag. 301, 113908. doi:10.1016/j.jenvman.2021.113908
Elgarahy, A. M., Elwakeel, K. Z., Akhdhar, A., and Hamza, M. F. (2021). Recent advances in greenly synthesized nanoengineered materials for water/wastewater remediation: an overview. Nanotechnol. Environ. Eng. 6, 9. doi:10.1007/s41204-021-00104-5
El-Khawaga, A. M., Zidan, A., and El-Mageed, A. I. A. A. (2023). Preparation methods of different nanomaterials for various potential applications: a review. J. Mol. Struct. 1281, 135148. doi:10.1016/j.molstruc.2023.135148
El Mouden, A., El Messaoudi, N., El Guerraf, A., Bouich, A., Mehmeti, V., Lacherai, A., et al. (2023). Multifunctional cobalt oxide nanocomposites for efficient removal of heavy metals from aqueous solutions. Chemosphere 317, 137922. doi:10.1016/j.chemosphere.2023.137922
El Ouardi, Y., Giove, A., Laatikainen, M., Branger, C., and Laatikainen, K. (2021). Benefit of ion imprinting technique in solid-phase extraction of heavy metals, special focus on the last decade. J. Environ. Chem. Eng. 9 (6), 106548. doi:10.1016/j.jece.2021.106548
Esrafili, L., Firuzabadi, F. D., Morsali, A., and Hu, M. L. (2021). Reuse of predesigned dual-functional metal organic frameworks (DF-MOFs) after heavy metal removal. J. Hazard. Mater. 403, 123696. doi:10.1016/j.jhazmat.2020.123696
Ethaib, S., Al-Qutaifia, S., Al-Ansari, N., and Zubaidi, S. L. (2022). Function of nanomaterials in removing heavy metals for water and wastewater remediation: a review. Environments 9, 123. doi:10.3390/environments9100123
Fan, Y. Z., Han, L., Yang, Y. Z., Sun, Z., Li, N., Li, B. L., et al. (2020). Multifunctional binding strategy on non-conjugated polymer nanoparticles for ratiometric detection and effective removal of mercury ions. Environ. Sci. and Technol. 54, 10270–10278. doi:10.1021/acs.est.0c00702
Farhan, A., Zulfiqar, M., Samiah, R. E. U., Nawaz, S., Iqbal, H. M., Jesionowski, T., et al. (2023). Removal of toxic metals from water by nanocomposites through advanced remediation processes and photocatalytic oxidation. Curr. Pollut. Rep. 9 (3), 338–358. doi:10.1007/s40726-023-00253-y
Fei, Y., and Hu, Y. H. (2022). Design, synthesis, and performance of adsorbents for heavy metal removal from wastewater: a review. J. Mater. Chem. A 10 (3), 1047–1085. doi:10.1039/d1ta06612a
Feisal, N. A. S., Kamaludin, N. H., Ahmad, M. A., and Tengku Ibrahim, T. N. B. (2024). A comprehensive review of nanomaterials for efficient heavy metal ions removal in water treatment. J. Water Process Eng. 64, 105566. doi:10.1016/j.jwpe.2024.105566
Feng, A., Feng, J., Xing, W., Jiang, K., and Tang, W. (2023). Versatile applications of electrochemical flow-through systems in water treatment processes. Chem. Eng. J. 145400. doi:10.1016/j.cej.2023.145400
Fu, W., Min, J., Jiang, W., Li, Y., and Zhang, W. (2020). Separation, characterization and identification of microplastics and nanoplastics in the environment. Sci. Total Environ. 721, 137561. doi:10.1016/j.scitotenv.2020.137561
Fu, X.-Z., Wu, J., Cui, S., Wang, X.-M., Liu, H., He, R.-L., et al. (2021). Self-regenerable bio-hybrid with biogenic ferrous sulfide nanoparticles for treating high-concentration chromium-containing wastewater. Water Res. 206, 117731. doi:10.1016/j.watres.2021.117731
Ganguly, P., Mandal, J., Paramsivam, M., and Patra, S. (2024). Environmental contaminants: impact, assessment, and remediation (Apple Academic Press). Palm Bay, FL.
Ganie, Z. A., Khandelwal, N., Choudhary, A., and Darbha, G. K. (2023). Clean water production from plastic and heavy metal contaminated waters using redox-sensitive iron nanoparticle-loaded biochar. Environ. Res. 235, 116605. doi:10.1016/j.envres.2023.116605
Ganiyu, S. O., and Martinez-Huitle, C. A. (2020). The use of renewable energies driving electrochemical technologies for environmental applications. Curr. Opin. Electrochem. 22, 211–220. doi:10.1016/j.coelec.2020.07.007
Ganzoury, M. A., Chidiac, C., Kurtz, J., and de Lannoy, C. F. (2020). CNT-sorbents for heavy metals: electrochemical regeneration and closed-loop recycling. J. Hazard. Mater. 393, 122432. doi:10.1016/j.jhazmat.2020.122432
Garcia-Segura, S., Qu, X., Alvarez, P. J., Chaplin, B. P., Chen, W., Crittenden, J. C., et al. (2020). Opportunities for nanotechnology to enhance electrochemical treatment of pollutants in potable water and industrial wastewater–a perspective. Environ. Sci. Nano 7, 2178–2194. doi:10.1039/d0en00194e
Gholamrezaei, S., Ghiyasiyan-Arani, M., Salavati-Niasari, M., and Moayedi, H. (2019). Multidisciplinary methods (co-precipitation, ultrasonic, microwave, reflux and hydrothermal) for synthesis and characterization of CaMn3O6 nanostructures and its photocatalytic water splitting performance. Int. J. Hydrogen Energy 44 (48), 26373–26386. doi:10.1016/j.ijhydene.2019.08.141
Gikay, A. A. (2024). Risks, innovation, and adaptability in the UK’s incrementalism versus the European Union’s comprehensive artificial intelligence regulation. Int. J. Law Inf. Technol. 32, eaae013. doi:10.1093/ijlit/eaae013
Godja, N.-C., and Munteanu, F.-D. (2024). Hybrid nanomaterials: a brief overview of versatile solutions for sensor technology in healthcare and environmental applications. Biosensors 14, 67. doi:10.3390/bios14020067
Gohar, O., Zubair Khan, M., Bibi, I., Bashir, N., Tariq, U., Bakhtiar, M., et al. (2024). Nanomaterials for advanced energy applications: recent advancements and future trends. Mater. and Des. 241, 112930. doi:10.1016/j.matdes.2024.112930
Golmaei, M., Kinnarinen, T., Jernström, E., and Häkkinen, A. (2018). Extraction of hazardous metals from green liquor dregs by ethylenediaminetetraacetic acid. J. Environ. Manag. 212, 219–227. doi:10.1016/j.jenvman.2018.01.078
Goyal, N., Amar, A., Gulati, S., and Varma, R. S. (2023). Cyclodextrin-based nanosponges as an environmentally sustainable solution for water treatment: a review. ACS Appl. Nano Mater. 6 (15), 13766–13791. doi:10.1021/acsanm.3c02026
Goyal, P., Chakraborty, S., and Misra, S. K. (2018). Multifunctional Fe3O4-ZnO nanocomposites for environmental remediation applications. Environ. Nanotechnol. Monit. Manag. 10, 28–35. doi:10.1016/j.enmm.2018.03.003
Goyat, R., Saharan, Y., Singh, J., Umar, A., and Akbar, S. (2022). Synthesis of graphene-based nanocomposites for environmental remediation applications: a review. Molecules 27 (19), 6433. doi:10.3390/molecules27196433
Grzelczak, M., Liz-Marzán, L. M., and Klajn, R. (2019). Stimuli-responsive self-assembly of nanoparticles. Chem. Soc. Rev. 48 (5), 1342–1361. doi:10.1039/c8cs00787j
Gulcin, İ., and Alwasel, S. H. (2022). Metal ions, metal chelators and metal chelating assay as antioxidant method. Processes 10 (1), 132. doi:10.3390/pr10010132
Gul Zaman, H., Baloo, L., Pendyala, R., Singa, P., Ilyas, S., and Kutty, S. (2021). Produced water treatment with conventional adsorbents and mof as an alternative: a review. Materials 14, 7607. doi:10.3390/ma14247607
Guo, J., Tian, H., and He, J. (2021). Integration of CuS nanoparticles and cellulose fibers towards fast, selective and efficient capture and separation of mercury ions. Chem. Eng. J. 408, 127336. doi:10.1016/j.cej.2020.127336
Gupta, A., Sharma, V., Sharma, K., Kumar, V., Choudhary, S., Mankotia, P., et al. (2021). A review of adsorbents for heavy metal decontamination: growing approach to wastewater treatment. Materials 14, 4702. doi:10.3390/ma14164702
Hama Aziz, K. H., Mustafa, F. S., Omer, K. M., Hama, S., Hamarawf, R. F., and Rahman, K. O. (2023). Heavy metal pollution in the aquatic environment: efficient and low-cost removal approaches to eliminate their toxicity: a review. RSC Adv. 13, 17595–17610. doi:10.1039/D3RA00723E
Hassan, T., Salam, A., Khan, A., Khan, S. U., Khanzada, H., Wasim, M., et al. (2021). Functional nanocomposites and their potential applications: a review. J. Polym. Res. 28 (2), 36. doi:10.1007/s10965-021-02408-1
Hou, X., Wang, J., Mousavi, B., Klomkliang, N., and Chaemchuen, S. (2022). Strategies for induced defects in metal–organic frameworks for enhancing adsorption and catalytic performance. Dalton Trans. 51 (21), 8133–8159. doi:10.1039/d2dt01030e
Huang, J., Huang, G., An, C., He, Y., Yao, Y., Zhang, P., et al. (2018). Performance of ceramic disk filter coated with nano ZnO for removing Escherichia coli from water in small rural and remote communities of developing regions. Environ. Pollut. 238, 52–62. doi:10.1016/j.envpol.2018.03.008
Huang, Y., Zheng, H., Hu, X.-b., Wu, Y., Tang, X., He, Q., et al. (2021). Enhanced selective adsorption of lead(II) from complex wastewater by DTPA functionalized chitosan-coated magnetic silica nanoparticles based on anion-synergism. J. Hazard. Mater. 422, 126856. doi:10.1016/j.jhazmat.2021.126856
Hussain, M. K., Khatoon, S., Nizami, G., Fatma, U. K., Ali, M., Singh, B., et al. (2024). Unleashing the power of bio-adsorbents: efficient heavy metal removal for sustainable water purification. J. Water Process Eng. 64, 105705. doi:10.1016/j.jwpe.2024.105705
Ilyas, M., Kassa, F. M., and Darun, M. R. (2021). Life cycle cost analysis of wastewater treatment: a systematic review of literature. J. Clean. Prod. 310, 127549. doi:10.1016/j.jclepro.2021.127549
Imran, M., Iqbal, M. M., Iqbal, J., Shah, N. S., Khan, Z. U. H., Murtaza, B., et al. (2021). Synthesis, characterization and application of novel MnO and CuO impregnated biochar composites to sequester arsenic (As) from water: modeling, thermodynamics and reusability. J. Hazard. Mater. 401, 123338. doi:10.1016/j.jhazmat.2020.123338
Iravani, S., and Varma, R. S. (2022). Nanosponges for water treatment: progress and challenges. Appl. Sci. 12 (9), 4182. doi:10.3390/app12094182
Irshad, M. A., Shakoor, M. B., Nawaz, R., Yasmeen, T., Arif, M. S., Rizwan, M., et al. (2022). Green and eco-friendly synthesis of TiO2 nanoparticles and their application for removal of cadmium from wastewater: reaction kinetics study. Z. fur Phys. Chem. 236, 637–657. doi:10.1515/zpch-2021-3171
Isawi, H. (2020). Using Zeolite/Polyvinyl alcohol/sodium alginate nanocomposite beads for removal of some heavy metals from wastewater. Arabian J. Chem. 13, 5691–5716. doi:10.1016/j.arabjc.2020.04.009
Isibor, P. O. (2024). “Regulations and policy considerations for nanoparticle safety,” in Environmental nanotoxicology. Editors P. O. Isibor, G. Devi, and A. A. Enuneku (Nature Switzerland, Cham: Springer), 295–316. doi:10.1007/978-3-031-54154-4_14
Isibor, P. O., Kayode-Edwards, I. I., and Taiwo, O. S. (2024). “Emerging technology and future directions in environmental nanotoxicology,” in Environmental nanotoxicology. Editors P. O. Isibor, G. Devi, and A. A. Enuneku (Nature Switzerland, Cham: Springer), 325–346. doi:10.1007/978-3-031-54154-4_16
Jadhao, R. B., Jayant, V., Halyal, U. A., Yusuf, M., and Sharma, B. (2024). Water treatment using nanofiltration technology: a sustainable way towards contaminant removal from wastewater. Jabirian J. Biointerface Res. Pharm. Appl. Chem. 1, 06–10. doi:10.55559/jjbrpac.v1i2.242
Jahan, N., Roy, H., Reaz, A. H., Arshi, S., Rahman, E., Firoz, S. H., et al. (2022). A comparative study on sorption behavior of graphene oxide and reduced graphene oxide towards methylene blue. Case Stud. Chem. Environ. Eng. 6, 100239. doi:10.1016/j.cscee.2022.100239
Jalili, V., Barkhordari, A., and Ghiasvand, A. (2020). New extraction media in microextraction techniques. A review of reviews. Microchem. J. 153, 104386. doi:10.1016/j.microc.2019.104386
Jawed, A., Saxena, V., and Pandey, L. M. (2020). Engineered nanomaterials and their surface functionalization for the removal of heavy metals: a review. J. Water Process Eng. 33, 101009. doi:10.1016/j.jwpe.2019.101009
Ji, Y., Choi, Y. J., Fang, Y., Pham, H. S., Nou, A. T., Lee, L. S., et al. (2023). Electric field-assisted nanofiltration for PFOA removal with exceptional flux, selectivity, and destruction. Environ. Sci. and Technol. 57, 18519–18528. doi:10.1021/acs.est.2c04874
Jurado-Davila, V., Toffoli, J., Estumano, D., and Féris, L. A. (2023). Fixed-bed column for phosphate adsorption combining experimental observation, mathematical simulation, and statistics: classical and Bayesian. Sep. Purif. Technol. 317, 123914. doi:10.1016/j.seppur.2023.123914
Kaur, J., Kaur, K., Kaur, K., Matharu, A. S., and Mehta, S. K. (2023). Greener aspects of nanoparticle synthesis for water remediation: challenges and future perspective. Adv. Environ. Eng. Res. 4, 1–56. doi:10.21926/aeer.2302027
Kehrein, P., Jafari, M., Slagt, M., Cornelissen, E., Osseweijer, P., Posada, J., et al. (2021). A techno-economic analysis of membrane-based advanced treatment processes for the reuse of municipal wastewater. J. Water Reuse Desalination 11, 705–725. doi:10.2166/wrd.2021.016
Kent, R. D., Oser, J. G., and Vikesland, P. J. (2014). Controlled evaluation of silver nanoparticle sulfidation in a full-scale wastewater treatment plant. Environ. Sci. Technol. 48, 8564–8572. doi:10.1021/es404989t
Khalid, M., Ur-Rahman, S., Hassani, D., Hayat, K., Zhou, P., and Hui, N. (2021). Advances in fungal-assisted phytoremediation of heavy metals: a review. Pedosphere 31, 475–495. doi:10.1016/s1002-0160(20)60091-1
Khan, M., Wibowo, A., Karim, Z., Posoknistakul, P., Matsagar, B., Wu, K., et al. (2024). Wastewater treatment using membrane bioreactor technologies: removal of phenolic contaminants from oil and coal refineries and pharmaceutical industries. Polym. (Basel) 16, 443. doi:10.3390/polym16030443
Khan, S., Naushad, Mu., Al-Gheethi, A., and Iqbal, J. (2021). Engineered nanoparticles for removal of pollutants from wastewater: current status and future prospects of nanotechnology for remediation strategies. J. Environ. Chem. Eng. 9, 106160. doi:10.1016/j.jece.2021.106160
Khan Khanzada, A., Al-Hazmi, H. E., Śniatała, B., Muringayil Joseph, T., Majtacz, J., Abdulrahman, S. A. M., et al. (2023). Hydrochar-nanoparticle integration for arsenic removal from wastewater: challenges, possible solutions, and future horizon. Environ. Res. 238, 117164. doi:10.1016/j.envres.2023.117164
Khatun, M. H., Kobir, Md.M., Miah, Md.A. R., Sarkar, A. K., and Alam, Md.A. (2024). Technologies for remediation of heavy metals in environment and ecosystem: a critical overview of comparison study. AJEE 23, 61–80. doi:10.9734/ajee/2024/v23i4540
Khulbe, K., and Matsuura, T. (2018). Removal of heavy metals and pollutants by membrane adsorption techniques. Appl. water Sci. 8, 19–30. doi:10.1007/s13201-018-0661-6
Klemeš, J. J., Varbanov, P. S., Ocłoń, P., and Chin, H. H. (2019). Towards efficient and clean process integration: utilisation of renewable resources and energy-saving technologies. Energies 12 (21), 4092. doi:10.3390/en12214092
Kolluru, S. S., Agarwal, S., Sireesha, S., Sreedhar, I., and Kale, S. R. (2021). Heavy metal removal from wastewater using nanomaterials-process and engineering aspects. Process Saf. Environ. Prot. 150, 323–355. doi:10.1016/j.psep.2021.04.025
Kong, Q., Preis, S., Li, L., Luo, P., Hu, Y., and Wei, C. (2020). Graphene oxide-terminated hyperbranched amino polymer-carboxymethyl cellulose ternary nanocomposite for efficient removal of heavy metals from aqueous solutions. Int. J. Biol. Macromol. 149, 581–592. doi:10.1016/j.ijbiomac.2020.01.185
Kopac, T. (2021). Emerging applications of process intensification for enhanced separation and energy efficiency, environmentally friendly sustainable adsorptive separations: a review. Int. J. Energy Res. 45 (11), 15839–15856. doi:10.1002/er.6944
Kuang, M., Shang, Y., Yang, G., Liu, B., and Yang, B. (2019). Facile synthesis of hollow mesoporous MgO spheres via spray-drying with improved adsorption capacity for Pb(II) and Cd(II). Environ. Sci. Pollut. Res. 26 (18), 18825–18833. doi:10.1007/s11356-019-05277-w
Kumar, A., and Samadder, S. R. (2023). Development of lower heating value prediction models and estimation of energy recovery potential of municipal solid waste and RDF incineration. Energy 274, 127273. doi:10.1016/j.energy.2023.127273
Kumar, V., Singh, E., Singh, S., Pandey, A., and Bhargava, P. C. (2023). Micro- and nano-plastics (MNPs) as emerging pollutant in ground water: environmental impact, potential risks, limitations and way forward towards sustainable management. Chem. Eng. J. 459, 141568. doi:10.1016/j.cej.2023.141568
Kumari, V., and Tripathi, A. K. (2020). Remediation of heavy metals in pharmaceutical effluent with the help of Bacillus cereus-based green-synthesized silver nanoparticles supported on alumina. Appl. Nanosci. 10, 1709–1719. doi:10.1007/s13204-020-01351-9
Kupa, E., Adanma, U. M., Ogunbiyi, E. O., and Solomon, N. O. (2024). Groundwater quality and agricultural contamination: a multidisciplinary assessment of risk and mitigation strategies. World J. Adv. Res. Rev. 22, 1772–1784. doi:10.30574/wjarr.2024.22.2.1607
Lata, S., Singh, P. K., and Samadder, S. R. (2015). Regeneration of adsorbents and recovery of heavy metals: a review. Int. J. Environ. Sci. Technol. 12, 1461–1478. doi:10.1007/s13762-014-0714-9
Le, A. T., Pung, S.-Y., Sreekantan, S., Matsuda, A., and Huynh, D. P. (2019). Mechanisms of removal of heavy metal ions by ZnO particles. Heliyon 5 (4), e01440. doi:10.1016/j.heliyon.2019.e01440
Leigh, N. G., and Lee, H. (2019). Sustainable and resilient urban water systems: the role of decentralization and planning. Sustainability 11, 918. doi:10.3390/su11030918
Li, C., Ji, X.-h., and Luo, X. (2020). Visualizing hotspots and future trends in phytomining research through scientometrics. Sustainability 12, 4593. doi:10.3390/su12114593
Li, L., Hartmann, G., Doblinger, M., and Schuster, M. (2013). Quantification of nanoscale silver particles removal and release from municipal wastewater treatment plants in Germany. Environ. Sci. Technol. 47, 7317–7323. doi:10.1021/es3041658
Li, L., and Liu, Y. (2023). “Chemical methods of heavy metal management—multifunctional nanomaterials,” in Heavy metals in environment: management strategies for global pollution, (ACSSymposiumSeries), 261–295. doi:10.1021/bk-2023-1456.ch014
Li, M., Shi, Q., Song, N., Xiao, Y., Wang, L., Chen, Z., et al. (2023b). Current trends in the detection and removal of heavy metal ions using functional materials. Chem. Soc. Rev. 52, 5827–5860. doi:10.1039/D2CS00683A
Li, N., Hou, J., Ou, R., Yeo, L., Choudhury, N. R., and Zhang, H. (2023a). Stimuli-responsive ion adsorbents for sustainable separation applications. ACS nano 17 (18), 17699–17720. doi:10.1021/acsnano.3c04942
Li, Y., Yang, Z., Yang, K., Wei, J., Li, Z., Ma, C., et al. (2022). Removal of chloride from water and wastewater: removal mechanisms and recent trends. Sci. Total Environ. 821, 153174. doi:10.1016/j.scitotenv.2022.153174
Li, Z., Wang, L., Qin, L., Lai, C., Wang, Z., Zhou, M., et al. (2021). Recent advances in the application of water-stable metal-organic frameworks: adsorption and photocatalytic reduction of heavy metal in water. Chemosphere 285, 131432. doi:10.1016/j.chemosphere.2021.131432
Liang, Y., Li, E., Wang, K., Guan, Z. J., He, H. H., Zhang, L., et al. (2022). Organo-macrocycle-containing hierarchical metal–organic frameworks and cages: design, structures, and applications. Chem. Soc. Rev. 51 (19), 8378–8405. doi:10.1039/d2cs00232a
Liao, W., Zhao, M., Rong, H., Jiang, P., Liao, Q., Zhang, C., et al. (2022). Photocatalyst immobilized by hydrogel, efficient degradation and self regeneration: a review. Mater. Sci. Semicond. Process. 150, 106929. doi:10.1016/j.mssp.2022.106929
Liao, Y., Loh, C.-H., Tian, M., Wang, R., and Fane, A. G. (2018). Progress in electrospun polymeric nanofibrous membranes for water treatment: fabrication, modification and applications. Prog. Polym. Sci. 77, 69–94. doi:10.1016/j.progpolymsci.2017.10.003
Lin, G., Zeng, B., Li, J., Wang, Z., Wang, S., Hu, T., et al. (2023). A systematic review of metal organic frameworks materials for heavy metal removal: synthesis, applications and mechanism. Chem. Eng. J. 460, 141710. doi:10.1016/j.cej.2023.141710
Liu, L., Luo, X. B., Ding, L., and Luo, S. L. (2019). “Application of nanotechnology in the removal of heavy metal from water,” in In nanomaterials for the removal of pollutants and resource reutilization. Elsevier, 83–147.
Liu, W., Pei, W., Moradi, M., Zhao, D., Li, Z., Zhang, M., et al. (2022). Polyethyleneimine functionalized mesoporous magnetic nanoparticles with enhanced antibacterial and antibiofilm activity in an alternating magnetic field. ACS Appl. Mater. and interfaces 14, 18794–18805. doi:10.1021/acsami.1c24148
Luo, J., Fu, K., Yu, D., Hristovski, K. D., Westerhoff, P., and Crittenden, J. C. (2021). Review of advances in engineering nanomaterial adsorbents for metal removal and recovery from water: synthesis and microstructure impacts. ACS Est. Eng. 1, 623–661. doi:10.1021/acsestengg.0c00174
Luo, J., Guan, K., Lei, W., Zhang, S., Jia, Q., and Zhang, H. (2022). One dimensional carbon-based composites as cathodes for lithium-sulfur battery. J. Mater. Sci. and Technol. 122, 101–120. doi:10.1016/j.jmst.2021.12.048
Lv, Z., Li, W., Wei, J., Ho, F., Cao, J., and Chen, X. (2023). Autonomous chemistry enabling environment-adaptive electrochemical energy storage devices. CCS Chem. 5 (1), 11–29. doi:10.31635/ccschem.022.202202153
Maji, D., and Dutta, S. (2024). “Strategies to safeguard drinking water from hazardous chemical contaminants,” in Development in wastewater treatment research and processes (Elsevier), 419–441. doi:10.1016/B978-0-443-13884-3.00024-X
Makaev, S., Badenhorst, R., Reukov, V., and Minko, S. (2023). “Stimuli-responsive interfaces,” in ACS symposium series. Editor R. Nagarajan (Washington, DC: American Chemical Society), 149–194. doi:10.1021/bk-2023-1457.ch008
Malhotra, P., and Jain, A. (2021). “Graphene oxide-based nanocomposites for adsorptive removal of water pollutants,” in Contamination of water (Elsevier), 431–448.
Mamidi, N., Delgadillo, R. M. V., and Castrejón, J. V. (2021). Unconventional and facile production of a stimuli-responsive multifunctional system for simultaneous drug delivery and environmental remediation. Environ. Sci. Nano 8, 2081–2097. doi:10.1039/D1EN00354B
Martínez, R., Vela, N., el Aatik, A., Murray, E., Roche, P., and Navarro, J. M. (2020). On the use of an IoT integrated system for water quality monitoring and management in wastewater treatment plants. Water 12, 1096. doi:10.3390/w12041096
Martínez-Huitle, C. A., Rodrigo, M. A., and Scialdone, O. (2018). Electrochemical water and wastewater treatment. Butterworth-Heinemann.
Mensah, M. B., Lewis, D. J., Boadi, N. O., and Awudza, J. A. M. (2021). Heavy metal pollution and the role of inorganic nanomaterials in environmental remediation. R. Soc. Open Sci. 8, 201485. doi:10.1098/rsos.201485
Mintz Hemed, N., Leal-Ortiz, S., Zhao, E. T., and Melosh, N. A. (2023). On-demand, reversible, ultrasensitive polymer membrane based on molecular imprinting polymer. ACS nano 17 (6), 5632–5643. doi:10.1021/acsnano.2c11618
Modi, A., and Bellare, J. R. (2020). Zeolitic imidazolate framework-67/carboxylated graphene oxide nanosheets incorporated polyethersulfone hollow fiber membranes for removal of toxic heavy metals from contaminated water. Sep. Purif. Technol. 249, 117160. doi:10.1016/j.seppur.2020.117160
Mohan, B., Kadiyan, R., Singh, K., Singh, G., Kumar, K., Sharma, H. K., et al. (2023). MOFs composite materials for Pb2+ ions detection in water: recent trends and advances. Microchem. J. 190, 108585. doi:10.1016/j.microc.2023.108585
Molinari, R., Lavorato, C., and Argurio, P. (2021). The evolution of photocatalytic membrane reactors over the last 20 years: a state of the art perspective. Catalysts 11 (7), 775. doi:10.3390/catal11070775
Montenegro-Ayo, R., Pérez, T., Lanza, M. R. V., Brillas, E., Garcia-Segura, S., and Santos, A. J. (2023). New electrochemical reactor design for emergent pollutants removal by electrochemical oxidation. Electrochimica acta 458, 142551. doi:10.1016/j.electacta.2023.142551
Moond, M., Singh, S., Sangwan, S., Rani, J., Devi, R., and Devi, P. (2024). 1 role removal of nanoparticles of heavy metals for and dyes from wastewater. Environmental nexus approach: management of water. Waste, Soil 3.
Natarajan, B., Kannan, P., Rather, J. A., and Sheikh, R. A. (2023). Recent developments in metal nanoparticles functionalized nanocomposite adsorbents for heavy metals removal from wastewaters. J. Taiwan Inst. Chem. Eng. 147, 104942. doi:10.1016/j.jtice.2023.104942
Naveed, M., Makhdoom, S. I., Rehman, S. u., Aziz, T., Bashir, F., Ali, U., et al. (2023). Biosynthesis and mathematical interpretation of zero-valent iron NPs using Nigella sativa seed tincture for indemnification of carcinogenic metals present in industrial effluents. Molecules 28 (8), 3299. doi:10.3390/molecules28083299
Neisan, R. S., Saady, N. M. C., Bazan, C., Zendehboudi, S., Al-nayili, A., Abbassi, B., et al. (2023). Arsenic removal by adsorbents from water for small communities’ decentralized systems: performance, characterization, and effective parameters. Clean. Technol. 5, 352–402. doi:10.3390/cleantechnol5010019
Nikić, J., Watson, M., Tubić, A., Šolić, M., and Agbaba, J. (2024). Recent trends in the application of magnetic nanocomposites for heavy metals removal from water: a review. Sep. Sci. Technol. 59, 293–331. doi:10.1080/01496395.2024.2315626
Nisola, G. M., Parohinog, K. J., Cho, M. K., Burnea, F. K., Lee, J. Y., Seo, J. G., et al. (2020). Covalently decorated crown ethers on magnetic graphene oxides as bi-functional adsorbents with tailorable ion recognition properties for selective metal ion capture in water. Chem. Eng. J. 389, 123421. doi:10.1016/j.cej.2019.123421
Noga, M., Milan, J., Frydrych, A., and Jurowski, K. (2023). Toxicological aspects, safety assessment, and green toxicology of silver nanoparticles (AgNPs)—critical review: state of the art. Int. J. Mol. Sci. 24, 5133. doi:10.3390/ijms24065133
Nthwane, Y. B., Fouda-Mbanga, B. G., Thwala, M., and Pillay, K. (2023). Synthesis and characterization of MC/TiO2 NPs nanocomposite for removal of Pb2+ and reuse of spent adsorbent for blood fingerprint detection. ACS Omega 8, 26725–26738. doi:10.1021/acsomega.2c05765
Nupur, B., and Nipun, B. (2024). Integration of tadox® technology to achieve net zero in textile wastewater treatment: policy recommendations based on pilot study in a CETP. Available at: https://nmcgtericoe-wr.in/assets/pdf/Policy_Brief_Integration_of_TADOX_Technology.pdf (Accessed July 17, 2024).
Nurazzi, N., Sabaruddin, F., Harussani, M., Kamarudin, S., Rayung, M., Asyraf, M., et al. (2021). Mechanical performance and applications of cnts reinforced polymer composites—a review. Nanomaterials 11 (9), 2186. doi:10.3390/nano11092186
Nwokediegwu, Z. Q. S., Daraojimba, O. H., Oliha, J. S., Obaigbena, A., Dada, M. A., and Majemite, M. T. (2024). Review of emerging contaminants in water: USA and African perspectives. Int. J. Sci. Res. Arch. 11, 350–360. doi:10.30574/ijsra.2024.11.1.0073
Palit, S., and Ranjit, P. S. (2024). “Large scale applications of nanomaterials for water treatment: challenges, future prospects, and the visionary future,” in Sustainable production innovations. Editors A. K. Patel, and A. K. Sharma (Wiley), 137–162. doi:10.1002/9781119792888.ch4
Pan, Y., Xu, K., and Wu, C. (2019). Recent progress in supercapacitors based on the advanced carbon electrodes. Nanotechnol. Rev. 8 (1), 299–314. doi:10.1515/ntrev-2019-0029
Pandit, S., Yadav, N., Sharma, P., Prakash, A., and Kuila, A. (2024). Life cycle assessment and techno-economic analysis of nanotechnology-based wastewater treatment: status, challenges and future prospectives. J. Taiwan Inst. Chem. Eng. 105567, 105567. doi:10.1016/j.jtice.2024.105567
Pang, A. L., Arsad, A., Ahmad Zaini, M. A., Garg, R., Saqlain Iqbal, M., Pal, U., et al. (2024). A comprehensive review on photocatalytic removal of heavy metal ions by polyaniline-based nanocomposites. Chem. Eng. Commun. 211 (2), 275–299. doi:10.1080/00986445.2023.2227568
Pardo, A., Pelaz, B., del Pino, P., Al-Modlej, A., Cambon, A., Velasco, B., et al. (2021). Monodisperse superparamagnetic nanoparticles separation adsorbents for high-yield removal of arsenic and/or mercury metals in aqueous media. J. Mol. Liq. 335, 116485. doi:10.1016/j.molliq.2021.116485
Parisi, O. I., Francomano, F., Dattilo, M., Patitucci, F., Prete, S., Amone, F., et al. (2022). The evolution of molecular recognition: from antibodies to molecularly imprinted polymers (MIPs) as artificial counterpart. J. Funct. Biomaterials 13 (1), 12. doi:10.3390/jfb13010012
Parisi, O. I., Ruffo, M., and Puoci, F. (2020). Molecularly imprinted polymers for selective recognition in regenerative medicine. nanostructured biomaterials Regen. Med. 1, 141–163. doi:10.1016/b978-0-08-102594-9.00005-x
Park, J., Kim, K. T., and Lee, W. H. (2020). Recent advances in information and communications technology (ICT) and sensor technology for monitoring water quality. Water 12, 510. doi:10.3390/w12020510
Park, S.-A., Keum, Y., and Park, J. (2021). Ti-Based porous materials for reactive oxygen species-mediated photocatalytic reactions. Chem. Commun. 58, 607–618. doi:10.1039/d1cc04858a
Pena-Pereira, F., Romero, V., de la Calle, I., Lavilla, I., and Bendicho, C. (2021). Graphene-based nanocomposites in analytical extraction processes. TRAC Trends Anal. Chem. 142, 116303. doi:10.1016/j.trac.2021.116303
Peng, H., and Guo, J. (2020). Removal of chromium from wastewater by membrane filtration, chemical precipitation, ion exchange, adsorption electrocoagulation, electrochemical reduction, electrodialysis, electrodeionization, photocatalysis and nanotechnology: a review. Environ. Chem. Lett. 18 (6), 2055–2068. doi:10.1007/s10311-020-01058-x
Peng, L., Zhang, X., Sun, Y., Xing, Y., and Li, C. (2020). Heavy metal elimination based on metal organic framework highly loaded on flexible nanofibers. Environ. Res. 188, 109742. doi:10.1016/j.envres.2020.109742
Pérez, H., Quintero García, O. J., Amezcua-Allieri, M. A., and Rodríguez Vázquez, R. (2023). Nanotechnology as an efficient and effective alternative for wastewater treatment: an overview. Water Sci. and Technol. 87, 2971–3001. doi:10.2166/wst.2023.179
Pillai, S. B., and Thombre, N. V. (2024). “Coagulation, flocculation, and precipitation in water and used water purification,” in Handbook of water and used water purification (Springer), 3–27.
Poonia, K., Singh, P., Ahamad, T., Le, Q. V., Phan Quang, H. H., Thakur, S., et al. (2024). Sustainability, performance, and production perspectives of waste-derived functional carbon nanomaterials towards a sustainable environment: a review. Chemosphere 352, 141419. doi:10.1016/j.chemosphere.2024.141419
Pradhan, S. K., Pareek, V., Panwar, J., and Gupta, S. (2019). Synthesis and characterization of ecofriendly silver nanoparticles combined with yttrium oxide (Ag-Y2O3) nanocomposite with assorted adsorption capacity for Cu(II) and Cr(VI) removal: a mechanism perspective. J. water process Eng., 32, 100917. doi:10.1016/j.jwpe.2019.100917
Prasad, R. T., and Gupta, J. (2024). “Recent advances in the application of nanomaterials for environmental sustainability,” in Hybrid composite materials. Editors A. Verma, H. S. Gupta, and S. K. Sethi (Singapore: Springer Nature Singapore), 333–348. doi:10.1007/978-981-97-2104-7_14
Prathna, T. C., Sharma, S. K., and Kennedy, M. (2018). Nanoparticles in household level water treatment: an overview. Sep. Purif. Technol. 199, 260–270. doi:10.1016/j.seppur.2018.01.061
Qasem, N. A. A., Mohammed, R. H., and Lawal, D. U. (2021). Removal of heavy metal ions from wastewater: a comprehensive and critical review. NPJ Clean. Water 4, 36. doi:10.1038/s41545-021-00127-0
Quintas, P. Y., Fiorentini, E. F., and Escudero, L. B. (2021). Chelating materials for the removal of heavy metals from water. Remediat. Heavy Metals, 379–417. doi:10.1007/978-3-030-80334-6_16
Rafeeq, H., Hussain, A., Ambreen, A., Waqas, M., Bilal, M., Iqbal, H. M., et al. (2022). Functionalized nanoparticles and their environmental remediation potential: a review. J. Nanostructure Chem. 12 (6), 1007–1031. doi:10.1007/s40097-021-00468-9
Rai, S., Guin, M., De, A., and Singh, N. B. (2022). Functionalized nanomaterials: basics, properties and applications. InFunctionalized nanomaterials for corrosion mitigation: synthesis, characterization, and applications. Am. Chem. Soc., 27–66. doi:10.1021/bk-2022-1418.ch002
Rajendran, S., Priya, A., Kumar, P. S., Hoang, T. K., Sekar, K., Chong, K. Y., et al. (2022). A critical and recent developments on adsorption technique for removal of heavy metals from wastewater-A review. Chemosphere 303, 135146. doi:10.1016/j.chemosphere.2022.135146
Ramalingam, B., Parandhaman, T., Choudhary, P., and Das, S. K. (2018). Biomaterial functionalized graphene-magnetite nanocomposite: a novel approach for simultaneous removal of anionic dyes and heavy-metal ions. ACS Sustain Chem. Eng. 6, 6328–6341. doi:10.1021/acssuschemeng.8b00139
Rashid, A., Schutte, B. J., Ulery, A., Deyholos, M. K., Sanogo, S., Lehnhoff, E. A., et al. (2023). Heavy metal contamination in agricultural soil: environmental pollutants affecting crop health. Agronomy 13, 1521. doi:10.3390/agronomy13061521
Rathi, B. S., Kumar, P. S., Ponprasath, R., Rohan, K., and Jahnavi, N. (2021). An effective separation of toxic arsenic from aquatic environment using electrochemical ion exchange process. J. Hazard. Mater. 412, 125240. doi:10.1016/j.jhazmat.2021.125240
Rawat, S., Singh, J., and Koduru, J. R. (2021). Effect of ultrasonic waves on degradation of phenol and para-nitrophenol by iron nanoparticles synthesized from Jatropha leaf extract. Environ. Technol. Innovation 24, 101857. doi:10.1016/j.eti.2021.101857
Ray, A. K. (2023). “Ion exchange,” in Coulson and richardson's chemical engineering (Elsevier), 631–656.
Rodríguez-Rasero, C., Montes-Jimenez, V., Alexandre-Franco, M. F., Fernández-González, C., Píriz-Tercero, J., and Cuerda-Correa, E. M. (2024). Use of zero-valent iron nanoparticles (nZVIs) from environmentally friendly synthesis for the removal of dyes from water—a review. Water 16 (11), 1607. doi:10.3390/w16111607
Romano, A., Urtiaga, A. M., and Ortiz, I. (2020). Optimized energy consumption in electrochemical-based regeneration of RAS water. Sep. Purif. Technol. 240, 116638. doi:10.1016/j.seppur.2020.116638
Rossi, L., Bagheri, M., Zhang, W., Chen, Z., Burken, J. G., and Ma, X. (2019). Using artificial neural network to investigate physiological changes and cerium oxide nanoparticles and cadmium uptake by Brassica napus plants. Environ. Pollut. 246, 381–389. doi:10.1016/j.envpol.2018.12.029
Rothee, S. R., Heidari, H., Fortier, M.-O., and Khan, E. (2024). Applications of ionic liquids in soil remediation: mechanisms, efficiency and life cycle assessment. Soil and Environ. Health 2, 100097. doi:10.1016/j.seh.2024.100097
Roy, B., Bhunia, B., Bandyopadhyay, T. K., Khan, S. A., Nandi, N. B., and Nath, P. C. (2024). “Water pollution by heavy metals and their impact on human health,” in Handbook of water pollution (Wiley), 333–352. doi:10.1002/9781119904991.ch10
Ruíz-Baltazar, Á. de J. (2024). Advancements in nanoparticle-modified zeolites for sustainable water treatment: an interdisciplinary review. Sci. Total Environ. 946, 174373. doi:10.1016/j.scitotenv.2024.174373
Sahraei, R., and Ghaemy, M. (2017). Synthesis of modified gum tragacanth/graphene oxide composite hydrogel for heavy metal ions removal and preparation of silver nanocomposite for antibacterial activity. Carbohydr. Polym. 157, 823–833. doi:10.1016/j.carbpol.2016.10.059
Saleem, H., and Zaidi, S. J. (2020). Developments in the application of nanomaterials for water treatment and their impact on the environment. Nanomaterials 10, 1764. doi:10.3390/nano10091764
Saleh, T. A. (2024). Trends in nanomaterial types, synthesis methods, properties and uses: toxicity, environmental concerns and economic viability. Nano-Structures and Nano-Objects 37, 101109. doi:10.1016/j.nanoso.2024.101109
Saravanan, A., Kumar, P. S., Hemavathy, R. V., Jeevanantham, S., Jawahar, M. J., Neshaanthini, J. P., et al. (2022). A review on synthesis methods and recent applications of nanomaterial in wastewater treatment: challenges and future perspectives. Chemosphere 307, 135713. doi:10.1016/j.chemosphere.2022.135713
Sarma, G. K., Sen Gupta, S., and Bhattacharyya, K. G. (2019). Nanomaterials as versatile adsorbents for heavy metal ions in water: a review. Environ. Sci. Pollut. Res. 26, 6245–6278. doi:10.1007/s11356-018-04093-y
Sedighi, M., Usefi, M. M., Ismail, A. F., and Ghasemi, M. (2023). Environmental sustainability and ions removal through electrodialysis desalination: operating conditions and process parameters. Desalination 549, 116319. doi:10.1016/j.desal.2022.116319
Shahrokhinia, A., Tafazoli, S., Rijal, S., Shuster, D. B., Scanga, R. A., Morefield, D. J., et al. (2024). Dynamic worm-gel materials as tunable, regenerable adsorbents for water treatment. Macromolecules 57, 628–639. doi:10.1021/acs.macromol.3c02090
Shaikh, M., and Birajdar, F., (2024). Groundwater and public health: exploring the connections and challenges. doi:10.5281/ZENODO.10730864
Shang, H., Li, Y., Liu, J., Wan, Y., Feng, Y., and Yu, Y. (2020). Preparation of nitrogen doped magnesium oxide modified biochar and its sorption efficiency of lead ions in aqueous solution. Bioresour. Technol. 314, 123708. doi:10.1016/j.biortech.2020.123708
Shanker, U. (2024). “Occurrence,” in Distribution and toxic effects of emerging contaminantsx. First edition. CRC Press. Place of publication not identified.
Shanmugavel, A., Rene, E. R., Balakrishnan, S. P., Krishnakumar, N., and Jose, S. P. (2024). Heavy metal ion sensing strategies using fluorophores for environmental remediation. Environ. Res. 119544, 119544. doi:10.1016/j.envres.2024.119544
Shao, P., Liang, D., Yang, L., Shi, H., Xiong, Z., Ding, L., et al. (2020). Evaluating the adsorptivity of organo-functionalized silica nanoparticles towards heavy metals: quantitative comparison and mechanistic insight. J. Hazard. Mater. 387, 121676. doi:10.1016/j.jhazmat.2019.121676
Sharma, M., Kant, R., Sharma, A. K., and Sharma, A. K. (2024). Exploring the impact of heavy metals toxicity in the aquatic ecosystem. Int. J. Energy Water Resour. doi:10.1007/s42108-024-00284-1
Shingare, S. P., Kanfade, L. B., Mane, V. B., Kumbhar, G. B., and Suryawanshi, M. A. (2024). Sun-powered wastewater treatment with a hydrogen bonus: unveiling the potential of zno-tio2 nanoparticles. Waste Biomass Valorization. doi:10.1007/s12649-024-02570-9
Shokri, A., and Sanavi Fard, M. (2022). A sustainable approach in water desalination with the integration of renewable energy sources: environmental engineering challenges and perspectives. Environ. Adv. 9, 100281. doi:10.1016/j.envadv.2022.100281
Shrestha, R., Ban, S., Devkota, S., Sharma, S., Joshi, R., Tiwari, A. P., et al. (2021). Technological trends in heavy metals removal from industrial wastewater: a review. J. Environ. Chem. Eng. 9 (4), 105688. doi:10.1016/j.jece.2021.105688
Siciliano, A., Limonti, C., Curcio, G. M., and Molinari, R. (2020). Advances in struvite precipitation technologies for nutrients removal and recovery from aqueous waste and wastewater. Sustainability 12 (18), 7538. doi:10.3390/su12187538
Silva-Calpa, L. d. R., Correia, T. O. F., Netto-Ferreira, J. C., Kuriyama, S. N., Letichevsky, S., and Avillez, R. (2020). Stable and highly active zero-valent iron-nickel nanofilaments/silica for the hexavalent chromium reduction. Environ. Nanotechnol. Monit. Manag. 14, 100332. doi:10.1016/j.enmm.2020.100332
Simelane, S., and Dlamini, L. N. (2019). An investigation of the fate and behaviour of a mixture of WO3 and TiO2 nanoparticles in a wastewater treatment plant. J. Environ. Sci. (China) 76, 37–47. doi:10.1016/j.jes.2018.03.018
Simeonidis, K., Andritsos, N., Kaprara, E., Mourdikoudis, S., and Mitrakas, M. (2014). Adapting the use of Fe3O4 nanoparticles in large-scale water treatment facilities. MRS proceedings/Materials Res. Soc. symposia Proc. 1708. doi:10.1557/opl.2014.487
Simeonidis, K., Martinez-Boubeta, C., Zamora-Pérez, P., Rivera-Gil, P., Kaprara, E., Kokkinos, E., et al. (2019). Implementing nanoparticles for competitive drinking water purification. Environ. Chem. Lett. 17, 705–719. doi:10.1007/s10311-018-00821-5
Singh, A., Chaudhary, S., and Dehiya, B. S. (2020). Fast removal of heavy metals from water and soil samples using magnetic Fe3O4 nanoparticles. Environ. Sci. Pollut. Res. 28, 3942–3952. doi:10.1007/s11356-020-10737-9
Singh, B. J., Chakraborty, A., and Sehgal, R. (2023). A systematic review of industrial wastewater management: evaluating challenges and enablers. J. Environ. Manag. 348, 119230. doi:10.1016/j.jenvman.2023.119230
Singh, R., and Bhateria, R. (2021). Core–shell nanostructures: a simplest two-component system with enhanced properties and multiple applications. Environ. Geochem. Health 43, 2459–2482. doi:10.1007/s10653-020-00766-1
Singh, S., Kapoor, D., Khasnabis, S., Singh, J., and Ramamurthy, P. C. (2021). Mechanism and kinetics of adsorption and removal of heavy metals from wastewater using nanomaterials. Environ. Chem. Lett. 19 (3), 2351–2381. doi:10.1007/s10311-021-01196-w
Solomon, N. O., Kanchan, S., and Kesheri, M. (2024b). Nanoparticles as detoxifiers for industrial wastewater. Water Air Soil Pollut. 235, 214. doi:10.1007/s11270-024-07016-5
Solomon, N. O., Simpa, P., Adenekan, O. A., and Obasi, S. C. (2024a). Sustainable nanomaterials’ role in green supply chains and environmental sustainability. Eng. Sci. and Technol. J. 5, 1678–1694. doi:10.51594/estj.v5i5.1136
Sprocati, R., and Rolle, M. (2019). Charge interactions, reaction kinetics and dimensionality effects on electrokinetic remediation: a model-based analysis. J. Contam. Hydrology 229, 103567. doi:10.1016/j.jconhyd.2019.103567
Su, H., and Hu, Y. H. (2021). Recent advances in graphene-based materials for fuel cell applications. Energy Sci. and Eng. 9 (7), 958–983. doi:10.1002/ese3.833
Su, T., Mao, X., Wang, Z., Pan, Y., Xu, B., Yang, W., et al. (2024). Cellulose nanocrystal-infused polymer hydrogel imbued with ferric-manganese oxide nanoparticles for efficient antinomy removal. J. Hazard. Mater. 476, 135097. doi:10.1016/j.jhazmat.2024.135097
Sudarman, F., Shiddiq, M., Armynah, B., and Tahir, D. (2023). Silver nanoparticles (AgNPs) synthesis methods as heavy-metal sensors: a review. Int. J. Environ. Sci. Technol. 20 (8), 9351–9368. doi:10.1007/s13762-022-04745-0
Sun, H., He, F.-F., and Choi, W. (2020). Production of reactive oxygen species by the reaction of periodate and hydroxylamine for rapid removal of organic pollutants and waterborne bacteria. Environ. Sci. and Technol. 54, 6427–6437. doi:10.1021/acs.est.0c00817
Tahoon, M. A., Siddeeg, S. M., Salem Alsaiari, N., Mnif, W., and Ben Rebah, F. (2020). Effective heavy metals removal from water using nanomaterials: a review. Processes 8 (6), 645. doi:10.3390/pr8060645
Taka, A. L., Klink, M. J., Mbianda, X. Y., and Naidoo, E. B. (2021). Chitosan nanocomposites for water treatment by fixed-bed continuous flow column adsorption: a review. Carbohydr. Polym. 255, 117398. doi:10.1016/j.carbpol.2020.117398
Tang, Q., Yin, Z., Wang, R., Zhu, W., Zhang, Z., Wang, Y., et al. (2023). New strategy to remove phosphate from low concentration solution by MOFs-modified resin: high affinity and thermal desorption. Chem. Eng. J. 465, 142864. doi:10.1016/j.cej.2023.142864
Tarekegn, M. M., Hiruy, A. M., and Dekebo, A. H. (2021). Nano zero valent iron (nZVI) particles for the removal of heavy metals (Cd2+, Cu2+ and Pb2+) from aqueous solutions. RSC Adv. 11, 18539–18551. doi:10.1039/d1ra01427g
Tchekwagep, P. M., Crapnell, R. D., Banks, C. E., Betlem, K., Rinner, U., Canfarotta, F., et al. (2022). A critical review on the use of molecular imprinting for trace heavy metal and micropollutant detection. Chemosensors 10 (8), 296. doi:10.3390/chemosensors10080296
Thakur, A., and Kumar, A. (2022). Recent advances on rapid detection and remediation of environmental pollutants utilizing nanomaterials-based (bio)sensors. Sci. Total Environ. 834, 155219. doi:10.1016/j.scitotenv.2022.155219
Thomas, S., Abraham, S. V., Aravind, U. K., and Aravindakumar, C. T. (2017). Enhanced degradation of acid red 1 dye using a coupled system of zero valent iron nanoparticles and sonolysis. Environ. Sci. Pollut. Res. 24, 24533–24544. doi:10.1007/s11356-017-0080-5
Tognacchini, A., Rosenkranz, T., van der Ent, A., Machinet, G. E., Echevarria, G., and Puschenreiter, M. (2019). Nickel phytomining from industrial wastes: growing nickel hyperaccumulator plants on galvanic sludges. J. Environ. Manag. 254, 109798. doi:10.1016/j.jenvman.2019.109798
Trakal, L., Veselská, V., Šafařík, I., Vítková, M., Číhalová, S., and Komárek, M. (2016). Lead and cadmium sorption mechanisms on magnetically modified biochars. Bioresour. Technol. 203, 318–324. doi:10.1016/j.biortech.2015.12.056
Tyagi, S., Kapoor, R. T., Solanki, S., Goyal, A., and Singh, R. (2024). Nanomaterial mediated wastewater treatment: a new frontier in environmental remediation. Microbiome-Based Decontam. Environ. Pollut., 31–49. doi:10.1016/B978-0-443-21781-4.00009-8
ul Gani Mir, T., Malik, A. Q., Singh, J., Shukla, S., and Kumar, D. (2022). An overview of molecularly imprinted polymers embedded with quantum dots and their implementation as an alternative approach for extraction and detection of crocin. ChemistrySelect 7 (21), e202200829. doi:10.1002/slct.202200829
Vasoya, Dr.N. (2023). Revolutionizing nano materials processing through IoT-AI integration: opportunities and challenges, 6, 294–328.
Vidu, R., Matei, E., Predescu, A. M., Alhalaili, B., Pantilimon, C., Tarcea, C., et al. (2020). Removal of heavy metals from wastewaters: a challenge from current treatment methods to nanotechnology applications. Toxics 8, 101. doi:10.3390/toxics8040101
Vieira, W. T., de Farias, M. B., Spaolonzi, M. P., da Silva, M. G. C., and Vieira, M. G. A. (2020). Removal of endocrine disruptors in waters by adsorption, membrane filtration and biodegradation. A review. Environ. Chem. Lett. 18 (4), 1113–1143. doi:10.1007/s10311-020-01000-1
Wahl, A., Le Juge, C., Davranche, M., El Hadri, H., Grassl, B., Reynaud, S., et al. (2021). Nanoplastic occurrence in a soil amended with plastic debris. Chemosphere 262, 127784. doi:10.1016/j.chemosphere.2020.127784
Wang, B., Zhu, Y., Bai, Z., Luque, R., and Xuan, J. (2017). Functionalized chitosan biosorbents with ultra-high performance, mechanical strength and tunable selectivity for heavy metals in wastewater treatment. Chem. Eng. J. 325, 350–359. doi:10.1016/j.cej.2017.05.065
Wang, D., Mueses, M. A., Márquez, J. A., Machuca-Martínez, F., Grčić, I., Moreira, R. P., et al. (2021). Engineering and modeling perspectives on photocatalytic reactors for water treatment. Water Res. 202, 117421. doi:10.1016/j.watres.2021.117421
Wang, J., Lai, Y., Wang, X., and Ji, H. (2024). Advances in ultrasonic treatment of oily sludge: mechanisms, industrial applications, and integration with combined treatment technologies. Environ. Sci. Pollut. Res. 31 (10), 14466–14483. doi:10.1007/s11356-024-32089-4
Wang, R.-Z., Huang, D.-L., Liu, Y.-G., Zhang, C., Lai, C., Zeng, G.-M., et al. (2018). Investigating the adsorption behavior and the relative distribution of Cd2+ sorption mechanisms on biochars by different feedstock. Bioresour. Technol. 261, 265–271. doi:10.1016/j.biortech.2018.04.032
Wang, Y., Yang, Y., Shi, J., An, W., Lyu, T., and Zhang, P. (2024). Processes and mechanisms in remediation of aqueous chromium contamination by sulfidated nano-scale zerovalent iron (S-nZVI): experimental and computational investigations. J. Hazard. Mater. 469, 134031. doi:10.1016/j.jhazmat.2024.134031
Wang, Z., Qin, K., Wang, Z., Shen, D., and Wu, C. (2021). Carbon nanotubes/Al2O3 composite derived from catalytic reforming of the pyrolysis volatiles of the mixture of polyethylene and lignin for highly-efficient removal of Pb(ii). RSC Adv. 11, 37851–37865. doi:10.1039/d1ra06762a
Wawata, I. G., and Fabiyi, O. A. (2024). “Sustainable application of nanomaterials in the removal of heavy metals from water,” in Sustainable nanomaterials, sustainable materials and technology. Editor I. Uddin (Singapore: Springer Nature Singapore), 21–44. doi:10.1007/978-981-97-2761-2_2
Wen, J., Fang, Y., and Zeng, G. (2018). Progress and prospect of adsorptive removal of heavy metal ions from aqueous solution using metal–organic frameworks: a review of studies from the last decade. Chemosphere 201, 627–643. doi:10.1016/j.chemosphere.2018.03.047
WHO (2022). Arsenic. Available at: https://www.who.int/news-room/fact-sheets/detail/arsenic.
WHO (2023). Lead poisoning. Available at: https://www.who.int/news-room/fact-sheets/detail/lead-poisoning-and-health.
Williams, J. D., and Peterson, G. P. (2021). A review of thermal property enhancements of low-temperature nano-enhanced phase change materials. Nanomaterials 11, 2578. doi:10.3390/nano11102578
Wu, J., Wang, T., Wang, J., Zhang, Y., and Pan, W.-P. (2021). A novel modified method for the efficient removal of Pb and Cd from wastewater by biochar: enhanced the ion exchange and precipitation capacity. Sci. Total Environ. 754, 142150. doi:10.1016/j.scitotenv.2020.142150
Xia, Y., Xia, L., and Lin, X. (2023). Laccase-based self-amplifying catalytic system enables efficient antibiotic degradation for sustainable environmental remediation. Adv. Sci. 10, e2300210. doi:10.1002/advs.202300210
Xiang, J., Lin, Q., Cheng, S., Guo, J., Yao, X., Liu, Q., et al. (2018). Enhanced adsorption of Cd(II) from aqueous solution by a magnesium oxide–rice husk biochar composite. Environ. Sci. Pollut. Res. 25 (14), 14032–14042. doi:10.1007/s11356-018-1594-1
Xu, W., Hu, X., Lou, Y., Jiang, X., Shi, K., Tong, Y., et al. (2020). Effects of environmental factors on the removal of heavy metals by sulfide-modified nanoscale zerovalent iron. Environ. Res. 187, 109662. doi:10.1016/j.envres.2020.109662
Xu, Z., Zhang, Q., Li, X., and Huang, X. (2022). A critical review on chemical analysis of heavy metal complexes in water/wastewater and the mechanism of treatment methods. Chem. Eng. J. 429, 131688. doi:10.1016/j.cej.2021.131688
Yaashikaa, P. R., Kumar, P. S., Saravanan, A., and Vo, D. V. (2021). Advances in biosorbents for removal of environmental pollutants: a review on pretreatment, removal mechanism and future outlook. J. Hazard. Mater. 420, 126596. doi:10.1016/j.jhazmat.2021.126596
Yahaya, N., Zain, N. N., Miskam, M., and Kamaruzaman, S. (2021). “Molecularly imprinted polymer composites in wastewater treatment,” in Molecularly imprinted polymer composites, 381–413.
Yang, J., Hou, B., Wang, J., Tian, B., Bi, J., Wang, N., et al. (2019). Nanomaterials for the removal of heavy metals from wastewater. Nanomaterials 9, 424. doi:10.3390/nano9030424
Yang, M., Xie, F., Li, S.-S., Lin, C.-H., Huang, X., and Liu, W. (2021). Zero-valent iron nanomaterial Fe0@Fe2MnO4 for ultrasensitive electroanalysis of As(III): Fe0 influenced surficial redox potential. Chem. Commun. 57, 1324–1327. doi:10.1039/d0cc07256g
Yasri, N. G., and Gunasekaran, S. (2017). Electrochemical technologies for environmental remediation. Enhancing Cleanup Environ. Pollut. Volume 2 Non-Biological Approaches, 5–73. doi:10.1007/978-3-319-55423-5_2
Younas, F., Mustafa, A., Farooqi, Z. U., Wang, X., Younas, S., Mohy-Ud-Din, W., et al. (2021). Current and emerging adsorbent technologies for wastewater treatment: trends, limitations, and environmental implications. Water 13 (2), 215. doi:10.3390/w13020215
Yu, G., Wang, G., Chi, T., Du, C., Wang, J., Li, P., et al. (2022). Enhanced removal of heavy metals and metalloids by constructed wetlands: a review of approaches and mechanisms. Sci. Total Environ. 821, 153516. doi:10.1016/j.scitotenv.2022.153516
Yu, G., Wang, X., Liu, J., Jiang, P., You, S., Ding, N., et al. (2021). Applications of nanomaterials for heavy metal removal from water and soil: a review. Sustainability 13, 713. doi:10.3390/su13020713
Yuan, Y., Wei, X., Zhu, M., Cai, Y., Wang, Y., Dang, Z., et al. (2024). Unravelling the removal mechanisms of trivalent arsenic by sulfidated nanoscale zero-valent iron: the crucial role of reactive oxygen species and the multiple effects of citric acid. Sci. Total Environ. 916, 170275. doi:10.1016/j.scitotenv.2024.170275
Zahmatkesh, S., Amesho, K. T., Sillanpaa, M., and Wang, C. (2022). Integration of renewable energy in wastewater treatment during COVID-19 pandemic: challenges, opportunities, and progressive research trends. Clean. Chem. Eng. 3, 100036. doi:10.1016/j.clce.2022.100036
Zainurin, S. N., Wan Ismail, W. Z., Mahamud, S. N. I., Ismail, I., Jamaludin, J., Ariffin, K. N. Z., et al. (2022). Advancements in monitoring water quality based on various sensing methods: a systematic review. IJERPH 19, 14080. doi:10.3390/ijerph192114080
Zamel, D., and Khan, A. U. (2021). New trends in nanofibers functionalization and recent applications in wastewater treatment. Polym. Adv. Technol. 32 (12), 4587–4597. doi:10.1002/pat.5471
Zamora-Ledezma, C., Negrete-Bolagay, D., Figueroa, F., Zamora-Ledezma, E., Ni, M., Alexis, F., et al. (2021). Heavy metal water pollution: a fresh look about hazards, novel and conventional remediation methods. Environ. Technol. Innov. 22, 101504. doi:10.1016/j.eti.2021.101504
Zeng, H., Zhai, L., Qiao, T., Yu, Y., Zhang, J., and Li, D. (2020). Efficient removal of as (V) from aqueous media by magnetic nanoparticles prepared with Iron-containing water treatment residuals. Sci. Rep. 10 (1), 9335. doi:10.1038/s41598-020-65840-1
Zeng, X., Zhang, G., Zhu, J., and Wu, Z. (2022). Adsorption of heavy metal ions in water by surface functionalized magnetic composites: a review. Environ. Sci. Water Res. and Technol. 8 (5), 907–925. doi:10.1039/d1ew00868d
Zhang, D., Tang, H., Zhao, B., Liu, L., Pang, H., Wang, X., et al. (2023). Immobilization of uranium by S-NZVI and UiO-66-NO2 composite through combined adsorption and reduction. J. Clean. Prod. 390, 136149. doi:10.1016/j.jclepro.2023.136149
Zhang, H., Huang, M., Zhang, W., Gardea-Torresdey, J. L., White, J. C., Ji, R., et al. (2020). Silver nanoparticles alter soil microbial community compositions and metabolite profiles in unplanted and cucumber-planted soils. Environ. Sci. and Technol. 54 (6), 3334–3342. doi:10.1021/acs.est.9b07562
Zhang, H., Li, A., Zhang, W., and Shuang, C. (2016). Combination of Na-modified zeolite and anion exchange resin for advanced treatment of a high ammonia–nitrogen content municipal effluent. J. Colloid Interface Sci. 468, 128–135. doi:10.1016/j.jcis.2015.10.006
Zhang, T., Lowry, G. V., Capiro, N. L., Chen, J., Chen, W., Chen, Y., et al. (2019). In situ remediation of subsurface contamination: opportunities and challenges for nanotechnology and advanced materials. Environ. Sci. Nano 6, 1283–1302. doi:10.1039/C9EN00143C
Zhang, W., Tooker, N. B., and Mueller, A. V. (2020). Enabling wastewater treatment process automation: leveraging innovations in real-time sensing, data analysis, and online controls. Environ. Sci. Water Res. Technol. 6, 2973–2992. doi:10.1039/D0EW00394H
Zhang, X., Lu, W., Zhou, G., and Li, Q. (2020). Understanding the mechanical and conductive properties of carbon nanotube fibers for smart electronics. Adv. Mater. 32 (5), 1902028. doi:10.1002/adma.201902028
Zhou, Z., Vázquez-González, M., and Willner, I. (2021). Stimuli-responsive metal–organic framework nanoparticles for controlled drug delivery and medical applications. Chem. Soc. Rev. 50, 4541–4563. doi:10.1039/D0CS01030H
Zhu, G., Bian, Y., Hursthouse, A. S., Xu, S., Xiong, N., and Wan, P. (2020). The role of magnetic MOFs nanoparticles in enhanced iron coagulation of aquatic dissolved organic matter. Chemosphere 247, 125921. doi:10.1016/j.chemosphere.2020.125921
Zhuang, P., Zhang, P., Li, K., Kumari, B., Li, D., and Mei, X. (2019). Silver nanoclusters encapsulated into metal–organic frameworks for rapid removal of heavy metal ions from water. Molecules 24, 2442. doi:10.3390/molecules24132442
Zinicovscaia, I. (2016). “Conventional methods of wastewater treatment,” in Cyanobacteria for bioremediation of wastewaters (Cham: Springer International Publishing), 17–25. doi:10.1007/978-3-319-26751-7_3
Keywords: nanoparticles, heavy metals, wastewater treatment, adsorption mechanisms, membrane filtration
Citation: Olawade DB, Wada OZ, Egbewole BI, Fapohunda O, Ige AO, Usman SO and Ajisafe O (2024) Metal and metal oxide nanomaterials for heavy metal remediation: novel approaches for selective, regenerative, and scalable water treatment. Front. Nanotechnol. 6:1466721. doi: 10.3389/fnano.2024.1466721
Received: 18 July 2024; Accepted: 02 October 2024;
Published: 16 October 2024.
Edited by:
Emmanuel Emeka Okoro, University of Port Harcourt, NigeriaReviewed by:
Priya Banerjee, Rabindra Bharati University, IndiaSahil Tahiliani, Applied Materials, United States
Copyright © 2024 Olawade, Wada, Egbewole, Fapohunda, Ige, Usman and Ajisafe. This is an open-access article distributed under the terms of the Creative Commons Attribution License (CC BY). The use, distribution or reproduction in other forums is permitted, provided the original author(s) and the copyright owner(s) are credited and that the original publication in this journal is cited, in accordance with accepted academic practice. No use, distribution or reproduction is permitted which does not comply with these terms.
*Correspondence: David B. Olawade, ZC5vbGF3YWRlQHVlbC5hYy51aw==