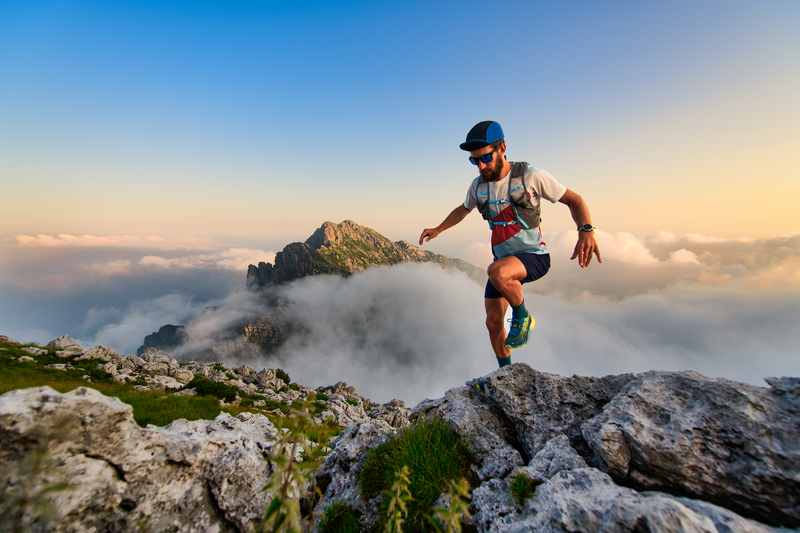
95% of researchers rate our articles as excellent or good
Learn more about the work of our research integrity team to safeguard the quality of each article we publish.
Find out more
BRIEF RESEARCH REPORT article
Front. Nanotechnol. , 16 May 2024
Sec. Nanotechnology for Energy Applications
Volume 6 - 2024 | https://doi.org/10.3389/fnano.2024.1406344
Biogas production via anaerobic digestion is an established and robust technology that produces energy and recycles nutrients. Several biotechnological attempts have been applied to this process to increase biogas production, including adding nanoparticles, but several discrepancies have been reported. To elucidate the contradictory results, we performed a literature review followed by a meta-analysis to evaluate the effect of adding natural nanoparticles to biogas sludge. Our results showed that adding nanoparticles can increase biogas production by up to two orders of magnitude. Considering that, we attribute these results to variability in the nanoparticles applied, leading to less reliable, consistent, and even contradictory results. We observed that the magnetite nanoparticles are the most tested ones with the most promising positive effects. In addition, we observed that concentrations of nanoparticles higher than 100 mg/L can have adverse effects, with an overall decrease in biogas production. The findings in this study highlight the need for a proper characterization of the nanomaterials type and concentration applied to the process to understand the interactions and effects on the microbial communities and dynamics that lead to an overall increase or decrease in biogas yield.
Investment in renewable energy sources is a reality nowadays (Atelge et al., 2020). Among the different renewable energy sources, the production of biogas from organic waste, generated in the complex microbial process of anaerobic digestion (AD), stands out due to its many advantages (Scarlat et al., 2018; Abanades et al., 2022; Wang et al., 2023). AD is an integrated biological process of the decomposition of organic matter in an oxygen-free environment that produces biogas and a digestate. Biogas is mainly composed of methane (CH4), carbon dioxide (CO2) and other minor molecules (H2, H2S, N2, H2O) (Madsen et al., 2011; Xu et al., 2018). Although it is a promising strategy, biogas production from AD still faces challenges in its process stability and efficiency (Appels et al., 2008; Xu et al., 2018). This process also deals with different groups of microorganisms that play crucial roles in AD (Deng et al., 2020) and the syntrophic relationship between these microorganisms, which have different growth requirements (Schnürer and Jarvis, 2018; Deng et al., 2020), is mandatory to achieve maximum biogas production (He et al., 2019). Thus, many strategies have been developed to improve microbial metabolic activities and biogas production in AD, such as co-digestion, pre-treatments and upgrades approaches (Patinvoh et al., 2017; Paritosh et al., 2018; Adnan et al., 2019; Abdelwahab et al., 2020; Anacleto et al., 2022).
The strategy of nanoparticles (NPs) addition to the AD process has shown results in improving the stability of the microbial community and the electric exchange between organic reductive bacteria and methanogenic archaea by enhancing direct interspecies electron transfer (DIET) efficiency (Rotaru et al., 2014; Park et al., 2018). Conductive NPs such as magnetite and nickel would be related to the increase in electron transfer efficiency between methane-producing microorganisms and other species that inhabit biogas reactors, a process also known as “electrical syntrophy” (Kato et al., 2012; Jin et al., 2019). It has also been suggested that the benefit of NPs addition may be related microbial growth requirements for trace elements, such as Fe, Ni, and Cu, for the enzymatic activity of the different microorganisms involved in AD.
NPs with different chemical compositions were studied for such purpose, like composed of silver (Grosser et al., 2021), zero valent iron (Abdelsalam et al., 2017b), cobalt (Abdelsalam et al., 2017a), nickel (Abdelsalam et al., 2017a; Hassanein et al., 2019), carbon (Baniamerian et al., 2019) and magnetite (Fe3O4) (Jin et al., 2019; Uysal and Mut, 2020; Amo-Duodu et al., 2021; Yu et al., 2022), among others (Abdelwahab et al., 2020). In 2019, Jin and others were successful in increasing 3–10 times the production of biogas by using chemically synthesized magnetite NPs. The increased yield was attributed to the conductive property of magnetite to increase the efficiency of DIET (Jin et al., 2019).
The benefit of the addition of NPs can also be a consequence of other NPs interactions such as the reduction of short-chain fatty acids accumulation, which in high amounts inhibits the AD process, organic matter overload, and the mitigation of H2S and ammonia, which ends up directly increasing CH4 production (Hernández et al., 2011; Abdelwahab et al., 2020; Farghali et al., 2020; Cui et al., 2022; Ziganshina et al., 2022). Thus, the NPs could be potentially used to accelerate microbial activities in AD and optimize CH4 production. However, the outcome reported for NPs effect on biogas production is not consistent. Farghali et al. (2019) observed an increase in biogas and CH4 production after adding iron oxide NPs that was lower than those previously observed by Abdelsalam et al. (2016). In their discussion, Farghali et al. (2019) suggested that this difference is due to different parameters in the methods between the studies, despite using NPs of similar composition. These differences include different specific composition and concentrations of NPs (Farghali used Fe2O3 at a concentration of 100 mg/L while Abdelsalam used Fe3O4 at 20 mg/L) and the substrates (Farghali tested cattle manure as substrate, while Abdelsalam used raw manure) (Abdelsalam et al., 2016; Farghali et al., 2019). This type of discrepancy in results emphasizes the importance of studies that allow comparison and systematizing the parameters and respective impacts of adding NPs to increase biogas production by AD.
Performing a systematic analysis is a suitable technique to integrate, in a reliable way, results from different studies to summarize its findings in more clear and robust conclusions (Van Houwelingen et al., 2002). In addition, conducting a meta-analysis contributes to resolving conflicting results from the literature and finding new patterns that bench experiments cannot achieve. Here, we performed a systematic review followed by a meta-analysis according to PRISMA 2020 statement (Page et al., 2021). Data collected was submitted to a series of statistical analysis to clarify NPs addition in the AD process and its implications for methane production improvement.
An advanced search code with the keywords “anaerobic digestion”, “biogas”, “methane” and “nanoparticles” and synonyms combined with Boolean operators (AND, OR and NOT) and wildcards (*, $) was entered with filters for English language and type of article file using the Web of Science (https://www.webofknowledge.com) database. Filters for English language, with document type for articles and time from 1997 to 2022 were used as well. Then, a preliminary screening was carried out with the following eligibility criteria in which studies should: 1) deal with a process of methane production by AD that provides methane yield data of the control and NPs treatments; 2) intentionally add and analyze NPs impact in methane production in AD; 3) provide information on the characteristics of NPs (at least composition, size and concentration added); 4) present experimental data and analysis (excluding modeling, simulations, bibliographic and systematic reviews).
After this screening step, selected articles were analyzed and data about the AD substrates type, NPs features and methane yield in the experiments (control and treatments) was collected. Information about the studies such as year of publication, author names, operational and experimental conditions were also collected. The main data collected from the selected primary publications were converted to a CSV file (Supplementary Table S1).
Data collected was submitted to the ROUT method for identifying outliers (Motulsky and Brown, 2006), followed by One-way ANOVA tests of variance, followed by Fisher’s multiple test, when significant, in GraphPad Prism software version 8.02 (GraphPad, United States of America). p-value <0.05 was considered statistically significant.
Data of different treatments in which NPs were added to the AD process were grouped according to NPs composition. Their effect in CH4 yield was compared to the control (without NPs addition) was given by calculating the natural log response ratio (ratio between the mean of the experimental group and that in the control group), expressed below (Eq. 1) (Hedges et al., 1999). This effect size calculation was chosen due to the high number of articles that did not clearly present a measure of dispersion and which information would have been lost if only those with this information were considered.
Eq. 1 Response ratio between CH4 production in treatment groups (XT) and control groups (XC).
Using the Past software (Hammer, 2001) the Kernel Density Estimation (KDE–Kernel’s density estimator) was calculated in order to provide the coordinates used for elaboration of density graphs in order to analyze the profile of the data obtained (Chen, 2017). The KDE method allows the observation of the density profile of data distribution against two variables. In other words, it allows observing at which points regarding the two variables the data obtained are more concentrated. Pearson’s correlation tests were also performed between the variables “size (nm)” and “concentration (mg/L)”, and the improvement in CH4 production, also expressed by Hedges’ RR.
The advanced search performed on the Web of Science database resulted in 877 articles. After the initial screening, 377 articles were selected for further data collection. Finally, after analysis and data collection according to the criteria described above, 290 data from 64 articles.
Different experimental conditions were used in the selected papers; for example, the volumes of the experiments varied from 25 mL flasks to 9 L reactors. Reactor models and the selected AD temperature varied. However, most of the experiments analyzed were carried out on laboratory scale, in mesophilic batch systems. Circa 68% of the tests were performed in flasks or bottles, 27% in a reactor and 5% in automatic methane potential test systems. The NPs concentrations used were displayed in different units and only those that could be converted to mg/L were kept in the analysis, resulting in these 64 articles remaining. This unit was chosen because it allowed the conversion of the largest number of studies. A great variation was also observed in expressing information related to the size of the NPs used and their origins or production methods, and the improvement of the production of CH4—which was unified by the Natural Logarithmic Response Ratio (RR CH4) described above. The main data used in the analysis of this work are displayed in Supplementary Table S1.
Effect sizes were calculated using Natural Log Response Ratio between the CH4 production in the treatment group (with NPs) and the control (without) of every study selected. The results were grouped according to NPs composition. Different effects of the addition of each NPs composition on CH4 production were observed (Figure 1). The effect size (expressed by the RR) demonstrates how much the treatment (NPs addition) has a positive or negative effect compared to when no NPs are added. When the 95% CI lines overcross the 0 point, it means that the NPs may cause inhibitory effects. When the middle circle (representing the mean RR CH4 of each group) undercrosses the 0 line, it represents a major probability in causing inhibitory rather than stimulatory effects in AD. Thus, certain NPs are more related to increased methane production, while others demonstrate more impacts that are negative on AD (Figure 1). The NPs with the best effects for increasing CH4 production are those composed of cobalt, zero valent iron and magnetite. Zinc oxide as well as copper oxide showed more inhibitory than beneficial effects.
Figure 1. (A) Natural log response ratio of CH4 yield of each NPs composition analyzed. The black lines of each NPs type represent the 95% CI and the circles the mean RR. When the black lines overcome the red line on zero X-axes it means that the addition of this NPs composition may cause an inhibitory effect. Different letters indicate significant differences between the NPs groups. (B–D) Scatter plot of the effect of each NPs composition in different AD substrates. (B) Cobalt; (C) Zero Valent iron; (D) Magnetite. Different letters in C indicate significant differences between the treatment groups.
Some NPs were tested in different types of substrates, such as magnetite and zero valent iron, but a wide range of other compositions was restricted to a few specific substrates. Only magnetite had its effect evaluated for all categories of substrates. For all of them this NPs composition presents, on average, better results (Figures 1A, D). As magnetite was the only tested on all studied substrates, it allowed us to make a specific comparison of those NPs on all substrates (Figure 1D). Here, we compared magnetite NPs results with those of cobalt (Figure 1B) and zero valent iron NPs (Figure 1C). Every color point represents one size effect of NPs impact in CH4 production by DA (Figure 1). On average, magnetite had a positive effect stimulating the biogas production when added to all substrates (Figure 1D). NPs composed by zero valent iron also stimulated biogas production, except when added to synthetic medium (Figure 1C). These results highlight the need for proper testing the effects of different NPs applied to different substrates. It is also relevant to evaluate each AD process in a wide point of view, comparing NPs characteristics, microbial community and biochemical changes to achieve a more complex understanding of its effects.
The surface analysis generated by the Kernel’s density estimator (KDE–Kernel’s density estimator) indicated that the increase in the size or concentration of the NPs used in the AD process did not result in greater beneficial effects in the production of CH4, that is, at a higher RR (Figure 2). Most experiments use concentrations below 400 mg/L. However, those experiments with higher concentrations did not result in better responses in terms of CH4 production (Figure 2).
Figure 2. 3D surface plot (A and C) and contour plot (B and D) from the Kernel Density Estimator. Data distribution of CH4 response (RR CH4) according to the variation of NPs concentration (mg/L) (A and B). Data distribution of CH4 response (RR CH4) according to the variation of NPs size (nm) (C and D). The surface elevation is proportional to the data density with the values referring to the coordinates of the horizontal axes.
Collecting data covering a wide range of parameters (i.e., substrate types and NPs sizes), allows more details to be compared beyond the composition of NPs. Magnetite NPs were the only composition that had enough data for a more robust evaluation of its effect on different tested parameters.
Magnetite NPs data was divided into 3 size ranges: <50 nm, between 50 and 100 nm and >100 nm (Figure 3A). The impact of magnetite NPs on CH4 production was compared by size range. NPs up to 50 nm and between 50 and 100 had better results. NPs greater than 100 nm showed worse and even inhibitory effects in CH4 production (Figure 3A). The effect of the NPs from 50 to 100 nm on CH4 production was significantly higher than the >100 nm group (Kruskal-Wallis, p = 0.0422). There was no significant difference between NPs <50 nm and between 50 and 100 nm. However, even though <50 nm had the higher results on CH4 responses (RR), NPs between 50 and 100 nm had a greater amount of data with greater increases in CH4 production than the other ranges, suggesting a more consistent effect in CH4 production (Figure 3A).
Figure 3. Size distribution of magnetite NPs and their impact on AD. Impacts between three size ranges on CH4 production (A); Scatter plot of impacts on CH4 production (RR) of NPs smaller than 50 nm as a function of added concentration (B); Scatter plot of impacts on CH4 production (RR) of NPs between 50 and 100 nm as a function of added concentration (C).
The impact of magnetite NPs on CH4 production was also evaluated as a function of the added concentration for each of the size ranges. Only NPs with dimensions up to 50 nm demonstrated a significant correlation (p < 0.05), with CH4 production, despite a relatively lower fit (r2 = 0.06324), clearly indicating the larger benefits of addition of less than 100 mg/L (Figure 3B). In other words, adding more NPs does not generate better results in CH4 production, it can actually cause the inverse effect. However, the lower line fit corroborates that it is not just the concentration of NPs that defines the positive effect in CH4 production. For NPs with dimensions between 50 and 100 nm, similar impacts on CH4 production were observed for concentrations of approximately 50 mg/L (Figure 3C).
Considering that pre-treatments can increase CH4 production (Anacleto et al., 2024), we also evaluated the magnetite response when applied associated with and without a pre-treatment. It was possible to observe that when magnetite NPs were added with some kind of pre-treatment it had only beneficial effects (Figure 4).
Figure 4. Scatter plots of response ratio of methane yield of magnetite NPs analyzed separated by experiments performed only in mesophilic batch without (A) and with (B) some kind of pre-treatment strategy linked. Forest plot of response ratio of experiments performed with magnetite NPs addition linked with some pre-treatment (C). Bars represent the 95% CI and the icons the mean RR. Different letters in C indicate significant differences between the treatment groups.
It is important to highlight that the addition of NPs was not considered as a pre-treatment. Evaluating the role of NPs as an AD pre-treatment has been observed by some studies (Su et al., 2019; Tedesco et al., 2020) and may be worth future investigation, but it was not the goal of this study.
The main compositions of NPs with better average effects on CH4 production were those of cobalt, zero valent iron and magnetite. It is worth noting that the number of experiments using magnetite NPs is much higher than for iron and cobalt. Magnetite NPs popularity and performance may be related to the diversity of effects reported for this composition, especially its driving role in DIET (Cruz Viggi et al., 2019; Jin et al., 2019). Magnetite is a metal oxide obtained relatively easily, most often by the process of co-precipitation or hydrothermal decomposition (Reddy et al., 2012). Despite being the most studied NPs, the majority of the studies that presented enough information about NPs did not get much more depth discussions about the microbiology perspective, being limited just to observations about chemical parameters (i.e., VFAs, pH, H2S variations).
Analyzing both structural and functional changes in the community as well as the chemical characteristics is essential to a better understanding of magnetite effects in AD. This highlights the importance of interdisciplinary collaborations in this kind of studies. However, few studies did connect their NPs results with the microbiologic analysis. Huang et al. (2020) used 8 mM of magnetite in the AD of rice plantation residues and observed that there was a modulation of the microbial community with the enrichment of the Geobacter and Methanosaeta genera, which also perform DIET, and increase of substrate degradation in addition to increasing the methanogenic activity (Huang et al., 2020). Jin et al. (2019) applied 20 mM of magnetite into artificial wastewater with high sulfate concentration and increased CH4 production and the number of bacteria genera related to sulfate reduction and methanogenic archaea.
These results indicate the stimulation of DIET among these microorganisms and therefore add a potential tool for “H2S removal” to the possible advantages of using magnetite NPs in AD (Jin et al., 2019). In addition to the modulation of the microbial community by stimulating DIET, the release of Fe+2 ions can favor the formation of iron sulfide salts, helping to mitigate the formation of H2S, which is toxic to a series of microorganisms. Increase in H2S concentrations may result in pH decrease in the system and also in high corrosion of pipes and other facilities, in addition to presenting significant risks to human health when inhaled (Truong et al., 2006; Appels et al., 2008).
Even fewer studies explored both the relation between the NPs and the microbial community. Casals et al. (2014), reported changes in the magnetite structure throughout the municipal waste AD process. Authors observed that not only a NPs size decrease, but also a loss of Fe+2 NPs content. The sustained release of iron from magnetite NPs stimulated the activity of methanogens without causing toxicity to the system, leading to an increase in the production of biogas by 180% and even the total CH4 percentage (Casals et al., 2014). Fe+2 ions are of great importance in methanogenic metabolism, but it is noteworthy that iron ions can also be released by iron oxides such as hematite, maghemite and magnetite, depending on their crystallographic characteristics, which are determinant in the dissolution of these minerals (Allen et al., 1988; Lagoeiro, 1998). In studies involving species of the genus Methanothermobacter, the importance of iron supply for the synthesis of a series of enzymes such as hydrogenases, dehydrogenases and reductases, in addition to ferredoxins, was observed (Kaster et al., 2011).
It is worth noting that merging different amounts of more than one composition of NPs might also display good improvements in CH4 yields. Hassanein et al. (2019) tested different concentrations of Ni, Co, Fe and magnetite and then combinations between these compositions in the AD of poultry waste. These authors observed that the concentrations of 4.5 mg/L of Co, 100 mg/L of Fe and 15 mg/L of magnetite increased by circa 30% the CH4 production, and significantly decreased H2S production (Hassanein et al., 2019). Similar mixtures also promoted an increase of 23.7% in the production of biogas and a significant depletion in the production of H2S in large scale digesters (30 L) (Hassanein et al., 2021). The benefits of NPs such as Co and Ni have been attributed to a possible supplementation of these elements as important components for some enzymatic processes for the microorganisms’ metabolism. For the Co NPs, for example, as it is necessary for synthesizing cobalamin and coenzyme B12, important parts of enzymatic complexes are involved in methanogenesis. Thus, supplementing this and other trace elements through NPs benefits metabolic improvements in AD (Zhang et al., 2009).
Depending on their concentration, smaller magnetite NPs seem to achieve a more effective result in the production of biogas (Figures 3A, B). The NPs’ surface area is inversely proportional to their average size, especially considering that most NPs usually have spherical shape (Juntupally et al., 2017; Liu et al., 2021; Zaidi et al., 2021; Yu et al., 2022). Thus, the addition of NPs with smaller dimensions would have comparably more advantages over larger ones, in addition to potentially presenting better dispersion with less material expense in the sludge present in the reactors.
However, it was observed that, even though <50 nm NPs have the highest individual effect on CH4 production, NPs between 50 and 100 nm promote a mean larger increase in CH4 production (Figure 3A), suggesting that intermediate NPs may be more predictable and less variable impacts on biogas production. The increase of CH4 production of this size range does not have a significant correlation with its concentration, suggesting that more factors are involved in its effect on AD.
The findings that NPs concentrations below 100 mg/L with <50 nm sizes (Figure 3), suggest a possible “limit” in the concentration for the use of magnetite, that could be attributed to their probable dissolution over time, releasing Fe+2 and Fe+3 ions. This could be potentially beneficial, especially considering that Fe is of great importance in the formation of enzymes important in the metabolism of most microorganisms involved in AD. However, it should always be remembered that iron becomes toxic to most microorganisms at higher concentrations (Goswami et al., 2016).
As NPs between 50 and 100 nm seem to be more resistant to dissolution and, therefore, less likely to cause overdose stress, they should be preferably used in AD. The lack of statistical correlation between concentration variables and the CH4 production response for NPs between 50 and 100 nm not only suggests that there are more variables influencing this impact, but that their effect must go beyond the release of iron ions, since the high concentration could easily cause stress. Thus, it is likely that NPs in this size range have a driving influence as a stimulus to DIET rather than iron release. However, such deductions can only be confirmed with certainty from the use of analyzes of the microbial community linked to the conductivity profile of the digestate (Lovley, 2017; Fu et al., 2019).
An increase of studies in crystallographic characterization of the NPs applied to AD could be very enlightening to elucidate their effect, especially because of the existence of irregular faces in the crystals (e.g., the non-typical regular expected geometric morphologies like spherical, cuboid, etc.) that provide electronic instability in the molecular structure of the crystal. Apparently, it seems that NPs with shapes with inclusions that result in the largest contact surface would be of greater interest if the purpose of their addition were strictly the release of iron ions. However, stating that the impact of NPs on the system is solely caused by its iron release is only possible with refined characterization techniques of the nanomaterials added (i.e., analytical microscopy techniques). The crystallographic characteristics of the NPs materials used have an important hole in the resistance and hardness of these NPs. It means that depending on the crystallography of the NPs, their degradation inside the AD system may differ. It can be advantageous if the NPs benefits are related to the sustained release of some elements (i.e., Fe and Co), but it also means that some NPs compositions may persist for longer periods inside the AD than others. Magnetite, for example, has three crystallographic faces: [110], [111] and [100]. According to studies by Allen et al. (1988) and Yamazaki (2020), the [111] face is twice as prone to dissolution due to the compact molecular arrangement of oxygen atoms, which would be more easily protonated in acidified environments. This kind of characterization was rarely observed in the studies involving NPs addition in AD (Casals et al., 2014).
Hu et al. (2010) described that magnetite NPs with distinct surfaces, dissolution and aggregation potential presented different stability and aggregation/disaggregation behaviors according to organic matter concentrations and pH and that this also had a larger impact on particle surface charge status. This means that, depending on where and how NPs are released, their impact can vary from just being suspended particles, as an ions source, to potentially contaminants absorption. This result highlights the need for studies involving the nanotechnology application to AD. Even though a large amount of data based on the addition of magnetite NPs allowed the establishment of clear patterns, more experiments about their structure and other compositions could contribute to reach a deeper impact on all potential roles of NPs to AD.
The limited number of studies suggests a knowledge gap associated with the characterization of the changes promoted directly on the AD microbial community, but also the crystalline and chemical profiles of the NPs employed. Therefore, it is important to standardize the minimum information required in these types of research to allow the comparison, validation and reproducibility of the results described in the literature.
Here, we observed that some NPs have a direct and stimulatory effect on CH4 production, especially magnetite NPs, while other chemical compositions had inhibitory and/or opposite effects depending on the substrate, chemical composition and concentration used in the AD process. Magnetite NPs showed greater benefits with <100 nm sizes and <100 mg/L concentrations; thus these are potentially the future of Nanotechnology in AD for enhancing biogas production. In addition, the fact that the increase in the concentration and size of the NPs used does not directly result in better results in the production of CH4 has a significant impact on the cost of NPs addition in AD. The development of methods to increase CH4 production in AD by the addition of NPs is a promising strategy. Future studies must include proper characterization of the NPs studied as well as microbial community analysis for better understanding of its effects. For a more in-depth discussion on the NPs crystallographic characteristics, it would be necessary to carry analytical electron microscopy techniques like high-resolution transmission electron microscopy (HRTEM), electron energy loss spectroscopy (EELS) analysis, X-ray diffraction (XRD), and X-ray absorption spectroscopy (XANES). These techniques would allow the understanding of the size, shape and structural behavior of the NPs, as well as its possible ion releases and chemical bondings during AD.
The original contributions presented in the study are included in the article/Supplementary Material, further inquiries can be directed to the corresponding authors.
JCC: Conceptualization, Data curation, Investigation, Methodology, Writing–original draft. ER: Data curation, Writing–original draft. IT: Writing–original draft, Methodology, Formal analysis. AE-P: Conceptualization, Funding acquisition, Writing–review and editing, Project administration, Supervision. FA: Conceptualization, Funding acquisition, Project administration, Supervision, Writing–review and editing.
The author(s) declare that financial support was received for the research, authorship, and/or publication of this article. Financial support was provided by the Brazilian agencies CNPq, CAPES, and FAPERJ. This study was financed in part by the Coordenação de Aperfeiçoamento de Pessoal de Nível Superior–Brasil (CAPES)—Finance Code 001. AE-P gratefully acknowledges financial support from the Swedish Energy Agency (project number P2023-00827) and the funding agency Formas (Grant number: 2021-02429). AE-P received funds from the Swedish Energy Agency (Grant number: 35624-2) at the Biogas Research Solution Center hosted by Linköping University, Sweden.
The authors acknowledge the Brazilian agencies CNPq, CAPES, FAPERJ.
The authors declare that the research was conducted in the absence of any commercial or financial relationships that could be construed as a potential conflict of interest.
All claims expressed in this article are solely those of the authors and do not necessarily represent those of their affiliated organizations, or those of the publisher, the editors and the reviewers. Any product that may be evaluated in this article, or claim that may be made by its manufacturer, is not guaranteed or endorsed by the publisher.
The Supplementary Material for this article can be found online at: https://www.frontiersin.org/articles/10.3389/fnano.2024.1406344/full#supplementary-material
Abanades, S., Abbaspour, H., Ahmadi, A., Das, B., Ehyaei, M. A., Esmaeilion, F., et al. (2022). A critical review of biogas production and usage with legislations framework across the globe. Int. J. Environ. Sci. Technol. 19 (4), 3377–3400. doi:10.1007/s13762-021-03301-6
Abdelsalam, E., Samer, M., Attia, Y., Abdel-Hadi, M., Hassan, H., and Badr, Y. (2016). Comparison of nanoparticles effects on biogas and methane production from anaerobic digestion of cattle dung slurry. Renew. Energy 87, 592–598. doi:10.1016/j.renene.2015.10.053
Abdelsalam, E., Samer, M., Attia, Y., Abdel-Hadi, M., Hassan, H., and Badr, Y. (2017a). Effects of Co and Ni nanoparticles on biogas and methane production from anaerobic digestion of slurry. Energy Convers. Manag. 141, 108–119. doi:10.1016/j.enconman.2016.05.051
Abdelsalam, E., Samer, M., Attia, Y., Abdel-Hadi, M., Hassan, H., and Badr, Y. (2017b). Influence of zero valent iron nanoparticles and magnetic iron oxide nanoparticles on biogas and methane production from anaerobic digestion of manure. Energy 120, 842–853. doi:10.1016/j.energy.2016.11.137
Abdelwahab, T. A. M., Mohanty, M. K., Sahoo, P. K., and Behera, D. (2020). Application of nanoparticles for biogas production: current status and perspectives. Energy Sources, Part A Recovery, Util. Environ. Eff., 1–13. doi:10.1080/15567036.2020.1767730
Adnan, I., Ong, M., Nomanbhay, S., Chew, K., and Show, P. (2019). Technologies for biogas upgrading to biomethane: a review. Bioengineering 6 (4), 92. doi:10.3390/bioengineering6040092
Allen, G. C., Kirby, C., and Sellers, R. M. (1988). The effect of the low-oxidation-state metal ion reagent tris-picolinatovanadium(II) formate on the surface morphology and composition of crystalline iron oxides. J. Chem. Soc. Faraday Trans. 1 Phys. Chem. Condens. Phases 84 (1), 355–364. doi:10.1039/F19888400355
Amo-Duodu, G., Tetteh, E. K., Rathilal, S., Armah, E. K., Adedeji, J., Chollom, M. N., et al. (2021). Effect of engineered biomaterials and magnetite on wastewater treatment: biogas and kinetic evaluation. Polymers 13 (24), 4323. doi:10.3390/polym13244323
Anacleto, T. M., Kozlowsky-Suzuki, B., Björn, A., Yekta, S. S., Masuda, L. S. M., de Oliveira, V. P., et al. (2024). Methane yield response to pretreatment is dependent on substrate chemical composition: a meta-analysis on anaerobic digestion systems. Sci. Rep. 14 (1), 1240–1312. doi:10.1038/s41598-024-51603-9
Anacleto, T. M., Kozlowsky-Suzuki, B., Wilson, A. E., and Enrich-Prast, A. (2022). Comprehensive meta-analysis of pathways to increase biogas production in the textile industry. Energies 15 (15), 5574. doi:10.3390/en15155574
Appels, L., Baeyens, J., Degrève, J., and Dewil, R. (2008). Principles and potential of the anaerobic digestion of waste-activated sludge. Prog. Energy Combust. Sci. 34 (6), 755–781. doi:10.1016/j.pecs.2008.06.002
Atelge, M. R., Krisa, D., Kumar, G., Eskicioglu, C., Nguyen, D. D., Chang, S. W., et al. (2020). Biogas production from organic waste: recent progress and perspectives. Waste Biomass Valorization 11 (3), 1019–1040. doi:10.1007/s12649-018-00546-0
Baniamerian, H., Isfahani, P. G., Tsapekos, P., Alvarado-Morales, M., Shahrokhi, M., Vossoughi, M., et al. (2019). Application of nano-structured materials in anaerobic digestion: current status and perspectives. Chemosphere 229, 188–199. doi:10.1016/j.chemosphere.2019.04.193
Casals, E., Barrena, R., García, A., González, E., Delgado, L., Busquets-Fité, M., et al. (2014). Programmed iron oxide nanoparticles disintegration in anaerobic digesters boosts biogas production. Small 10 (14), 2801–2808. doi:10.1002/smll.201303703
Chen, Y. C. (2017). A tutorial on kernel density estimation and recent advances. Biostat. Epidemiol. 1 (1), 161–187. doi:10.1080/24709360.2017.1396742
Cruz Viggi, C., Casale, S., Chouchane, H., Askri, R., Fazi, S., Cherif, A., et al. (2019). Magnetite nanoparticles enhance the bioelectrochemical treatment of municipal sewage by facilitating the syntrophic oxidation of volatile fatty acids. J. Chem. Technol. Biotechnol. 94 (10), 3134–3146. doi:10.1002/jctb.6120
Cui, P., Ge, J., Chen, Y., Zhao, Y., Wang, S., and Su, H. (2022). The Fe3O4 nanoparticles-modified mycelium pellet-based anaerobic granular sludge enhanced anaerobic digestion of food waste with high salinity and organic load. Renew. Energy 185, 376–385. doi:10.1016/j.renene.2021.12.050
Deng, L., Liu, Y., and Wang, W. (2020) Biogas technology, biogas technology. Singapore: Springer Singapore. doi:10.1007/978-981-15-4940-3
Farghali, M., Andriamanohiarisoamanana, F. J., Ahmed, M. M., Kotb, S., Yamamoto, Y., Iwasaki, M., et al. (2020). Prospects for biogas production and H2S control from the anaerobic digestion of cattle manure: the influence of microscale waste iron powder and iron oxide nanoparticles. Waste Manag. 101, 141–149. doi:10.1016/j.wasman.2019.10.003
Farghali, M., Andriamanohiarisoamanana, F. J., Ahmed, M. M., Kotb, S., Yamashiro, T., Iwasaki, M., et al. (2019). Impacts of iron oxide and titanium dioxide nanoparticles on biogas production: hydrogen sulfide mitigation, process stability, and prospective challenges. J. Environ. Manag. 240, 160–167. doi:10.1016/j.jenvman.2019.03.089
Fu, L., Zhou, T., Wang, J., You, L., Lu, Y., Yu, L., et al. (2019). NanoFe3O4 as solid electron shuttles to accelerate acetotrophic methanogenesis by methanosarcina barkeri. Front. Microbiol. 10, 1–13. doi:10.3389/fmicb.2019.00388
Goswami, R., Chattopadhyay, P., Shome, A., Banerjee, S. N., Chakraborty, A. K., Mathew, A. K., et al. (2016). An overview of physico-chemical mechanisms of biogas production by microbial communities: a step towards sustainable waste management. 3 Biotech. 6 (1), 72–12. doi:10.1007/s13205-016-0395-9
Grosser, A., Grobelak, A., Rorat, A., Courtois, P., Vandenbulcke, F., Lemière, S., et al. (2021). Effects of silver nanoparticles on performance of anaerobic digestion of sewage sludge and associated microbial communities. Renew. Energy 171, 1014–1025. doi:10.1016/j.renene.2021.02.127
Hammer, Ø., and Spocova, J. (2001). PAST: paleontological statistics software package for education and data analysis. Palaeontol. Electron. 4 (1). doi:10.26879/384
Hassanein, A., Keller, E., and Lansing, S. (2021). Effect of metal nanoparticles in anaerobic digestion production and plant uptake from effluent fertilizer. Bioresour. Technol. 321, 124455. doi:10.1016/j.biortech.2020.124455
Hassanein, A., Lansing, S., and Tikekar, R. (2019). Impact of metal nanoparticles on biogas production from poultry litter. Bioresour. Technol. 275, 200–206. doi:10.1016/j.biortech.2018.12.048
He, S., Deng, Q., Xian, P., Li, Z., Tan, S., and Liu, Q. (2019). Volatile fatty acid (VFA) and methane generation from sewage sludge and banana straw: influence of pH and two-phase anaerobic fermentation. Desalination Water Treat. 152, 208–213. doi:10.5004/dwt.2019.23872
Hedges, L. V., Gurevitch, J., and Curtis, P. S. (1999). The meta-analysis of response ratios in experimental ecology. Ecology 80 (4), 1150–1156. doi:10.1890/0012-9658(1999)080[1150:TMAORR]2.0.CO;2
Hernández, S. P., Chiappero, M., Russo, N., and Fino, D. (2011). A novel ZnO-based adsorbent for biogas purification in H2 production systems. Chem. Eng. J. 176–177, 272–279. doi:10.1016/j.cej.2011.06.085
Hu, J. D., Zevi, Y., Kou, X. M., Xiao, J., Wang, X. J., and Jin, Y. (2010). Effect of dissolved organic matter on the stability of magnetite nanoparticles under different pH and ionic strength conditions. Sci. Total Environ. 408 (16), 3477–3489. doi:10.1016/j.scitotenv.2010.03.033
Huang, J., Ma, K., Xia, X., Gao, K., and Lu, Y. (2020). Biochar and magnetite promote methanogenesis during anaerobic decomposition of rice straw. Soil Biol. Biochem. 143 (5), 107740–107744. doi:10.1016/j.soilbio.2020.107740
Jin, Z., Zhao, Z., and Zhang, Y. (2019). Potential of direct interspecies electron transfer in synergetic enhancement of methanogenesis and sulfate removal in an up-flow anaerobic sludge blanket reactor with magnetite. Sci. Total Environ. 677, 299–306. doi:10.1016/j.scitotenv.2019.04.372
Juntupally, S., Begum, S., Allu, S. K., Nakkasunchi, S., Madugula, M., and Anupoju, G. R. (2017). Relative evaluation of micronutrients (MN) and its respective nanoparticles (NPs) as additives for the enhanced methane generation. Bioresour. Technol. 238, 290–295. doi:10.1016/j.biortech.2017.04.049
Kaster, A. K., Goenrich, M., Seedorf, H., Liesegang, H., Wollherr, A., Gottschalk, G., et al. (2011). More than 200 genes required for methane formation from H2 and CO2 and energy conservation are present in methanothermobacter marburgensis and methanothermobacter thermautotrophicus. Archaea 2011 (1), 1–23. doi:10.1155/2011/973848
Kato, S., Hashimoto, K., and Watanabe, K. (2012). Methanogenesis facilitated by electric syntrophy via (semi)conductive iron-oxide minerals. Environ. Microbiol. 14 (7), 1646–1654. doi:10.1111/j.1462-2920.2011.02611.x
Lagoeiro, L. E. (1998). Transformation of magnetite to hematite and its influence on the dissolution of iron oxide minerals. J. Metamorph. Geol. 16 (3), 415–423. doi:10.1111/j.1525-1314.1998.00144.x
Liu, X., Du, M., Lu, Q., He, D., Song, K., Yang, Q., et al. (2021). How does chitosan affect methane production in anaerobic digestion? Environ. Sci. Technol. 55 (23), 15843–15852. doi:10.1021/acs.est.1c04693
Lovley, D. R. (2017). Syntrophy goes electric: direct interspecies electron transfer. Annu. Rev. Microbiol. 71, 643–664. doi:10.1146/annurev-micro-030117-020420
Madsen, M., Holm-Nielsen, J. B., and Esbensen, K. H. (2011). Monitoring of anaerobic digestion processes: a review perspective. Renew. Sustain. Energy Rev. 15 (6), 3141–3155. doi:10.1016/j.rser.2011.04.026
Motulsky, H. J., and Brown, R. E. (2006). Detecting outliers when fitting data with nonlinear regression - a new method based on robust nonlinear regression and the false discovery rate. BMC Bioinforma. 7, 123–220. doi:10.1186/1471-2105-7-123
Page, M. J., Moher, D., Bossuyt, P. M., Boutron, I., Hoffmann, T. C., Mulrow, C. D., et al. (2021). PRISMA 2020 explanation and elaboration: updated guidance and exemplars for reporting systematic reviews. BMJ 372, n160. doi:10.1136/bmj.n160
Park, J. H., Kang, H. J., Park, K. H., and Park, H. D. (2018). Direct interspecies electron transfer via conductive materials: a perspective for anaerobic digestion applications. Bioresour. Technol. 254, 300–311. doi:10.1016/j.biortech.2018.01.095
Patinvoh, R. J., Osadolor, O. A., Chandolias, K., Sárvári Horváth, I., and Taherzadeh, M. J. (2017). Innovative pretreatment strategies for biogas production. Bioresour. Technol. 224, 13–24. doi:10.1016/j.biortech.2016.11.083
Reddy, L. H., Arias, J. L., Nicolas, J., and Couvreur, P. (2012). Magnetic nanoparticles: design and characterization, toxicity and biocompatibility, pharmaceutical and biomedical applications. Chem. Rev. 112 (11), 5818–5878. doi:10.1021/cr300068p
Rotaru, A. E., Shrestha, P. M., Liu, F., Shrestha, M., Shrestha, D., Embree, M., et al. (2014). A new model for electron flow during anaerobic digestion: direct interspecies electron transfer to Methanosaeta for the reduction of carbon dioxide to methane. Energy Environ. Sci. 7 (1), 408–415. doi:10.1039/c3ee42189a
Scarlat, N., Dallemand, J. F., and Fahl, F. (2018). Biogas: developments and perspectives in europe. Renew. Energy 129, 457–472. doi:10.1016/j.renene.2018.03.006
Schnürer, A., and Jarvis, Å. (2018) Microbiology of the biogas process. SLU. Uppsala, Sweden: Swedish University of Agricultural Sciences.
Su, C., Zheng, P., Lin, X., Chen, W., Li, X., Chen, Q., et al. (2019). Influence of amoxicillin after pre-treatment on the extracellular polymeric substances and microbial community of anaerobic granular sludge. Bioresour. Technol. 276, 81–90. doi:10.1016/j.biortech.2018.12.104
Tedesco, S., Hurst, G., Imtiaz, A., Ratova, M., Tosheva, L., and Kelly, P. (2020). TiO2 supported natural zeolites as biogas enhancers through photocatalytic pre-treatment of Miscanthus x giganteous crops. Energy 205, 117954. doi:10.1016/j.energy.2020.117954
Truong, D. H., Eghbal, M. A., Hindmarsh, W., Roth, S. H., and O'Brien, P. J. (2006). Molecular mechanisms of hydrogen sulfide toxicity. Drug Metab. Rev. 38 (4), 733–744. doi:10.1080/03602530600959607
Uysal, Y., and Mut, B. (2020). Biogas and methane production efficiency of sewage sludge supplemented with conductive materials. Int. J. Glob. Warming 20 (4), 353–373. doi:10.1504/IJGW.2020.107153
Van Houwelingen, H. C., Arends, L. R., and Stijnen, T. (2002). Advanced methods in meta-analysis: multivariate approach and meta-regression. Statistics Med. 21 (4), 589–624. doi:10.1002/sim.1040
Wang, Q., Xia, C., Alagumalai, K., Thanh Nhi Le, T., Yuan, Y., Khademi, T., et al. (2023). Biogas generation from biomass as a cleaner alternative towards a circular bioeconomy: artificial intelligence, challenges, and future insights. Fuel 333 (P2), 126456. doi:10.1016/j.fuel.2022.126456
Xu, F., Li, Y., Ge, X., Yang, L., and Li, Y. (2018). Anaerobic digestion of food waste – challenges and opportunities. Bioresour. Technol. 247, 1047–1058. doi:10.1016/j.biortech.2017.09.020
Yamazaki, T. (2020). Reductive dissolution of biogenic magnetite. Earth, Planets Space 72 (1), 150. doi:10.1186/s40623-020-01290-3
Yu, J., Liu, J., Senthil Kumar, P., Wei, Y., Zhou, M., Vo, D. V. N., et al. (2022). Promotion of methane production by magnetite via increasing acetogenesis revealed by metagenome-assembled genomes. Bioresour. Technol. 345, 126521. doi:10.1016/j.biortech.2021.126521
Zaidi, A. A., Khan, S. Z., Almohamadi, H., Mahmoud, E. R. I., and Naseer, M. N. (2021). Nanoparticles synergistic effect with various substrate pretreatment and their comparison on biogas production from algae waste. Bull. Chem. React. Eng. Catal. 16 (2), 374–382. doi:10.9767/bcrec.16.2.10637.374-382
Zhang, Y., Rodionov, D. A., Gelfand, M. S., and Gladyshev, V. N. (2009). Comparative genomic analyses of nickel, cobalt and vitamin B12 utilization. BMC Genomics 10, 78. doi:10.1186/1471-2164-10-78
Keywords: biogas, nanoparticles, meta-analysis, biotechnology, methane
Citation: Castro JCd, Resende E, Taveira I, Enrich-Prast A and Abreu F (2024) Nanotechnology boosts the production of clean energy via nanoparticle addition in anaerobic digestion. Front. Nanotechnol. 6:1406344. doi: 10.3389/fnano.2024.1406344
Received: 24 March 2024; Accepted: 22 April 2024;
Published: 16 May 2024.
Edited by:
Sasanka Deka, University of Delhi, IndiaReviewed by:
Avishek Banik, Colorado State University, United StatesCopyright © 2024 Castro, Resende, Taveira, Enrich-Prast and Abreu. This is an open-access article distributed under the terms of the Creative Commons Attribution License (CC BY). The use, distribution or reproduction in other forums is permitted, provided the original author(s) and the copyright owner(s) are credited and that the original publication in this journal is cited, in accordance with accepted academic practice. No use, distribution or reproduction is permitted which does not comply with these terms.
*Correspondence: F. Abreu, ZmVybmFuZGFhYWJyZXVAbWljcm8udWZyai5icg==; A. Enrich-Prast, YWxleC5lbnJpY2gucHJhc3RAbGl1LnNl
Disclaimer: All claims expressed in this article are solely those of the authors and do not necessarily represent those of their affiliated organizations, or those of the publisher, the editors and the reviewers. Any product that may be evaluated in this article or claim that may be made by its manufacturer is not guaranteed or endorsed by the publisher.
Research integrity at Frontiers
Learn more about the work of our research integrity team to safeguard the quality of each article we publish.