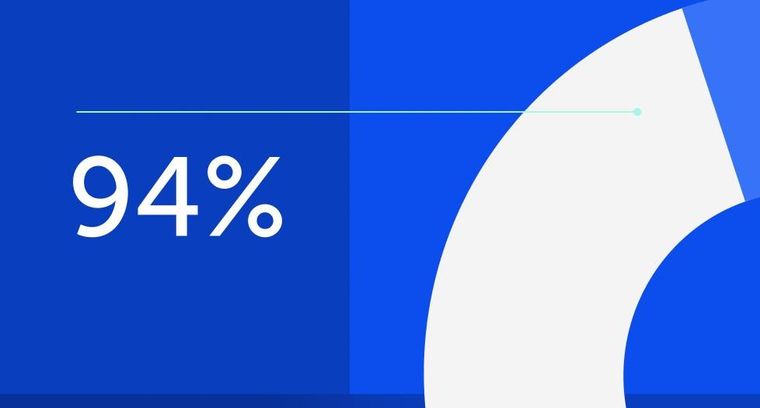
94% of researchers rate our articles as excellent or good
Learn more about the work of our research integrity team to safeguard the quality of each article we publish.
Find out more
MINI REVIEW article
Front. Nanotechnol., 09 February 2024
Sec. Biomedical Nanotechnology
Volume 6 - 2024 | https://doi.org/10.3389/fnano.2024.1348308
A nanobiosensor is a tool that converts a biological stimulus into an electrical output via nanosized transducer elements. Nanobiosensors are promising instruments, especially in biomedical applications in the literature and industry. To develop a nanobiosensor from idea to product, a life-cycle approach that comprises various processes ranging from conception through commercialization is required. Developers and potential investors should examine market requirements, design possibilities, feasibility, financial return, and risk assessments when developing a nanobiosensor development concept. It is critical to establish a well-defined regulatory pathway for bringing innovation to market at a low cost and in a short period. R&D should conduct thorough examinations of nanomaterial toxicity and health effects, involving marketing, advertising, and financial analysis. Stakeholders should discuss technology transfer office protocols for faster, healthier operations.
Public and personal health issues are among the biggest risks to humankind. The World Health Organisation (2023) claims that millions of individuals suffer from illnesses each year, with over half of those cases resulting in serious disorders(World health statistics, 2023). In traditional settings, the diagnosis of sick cases is carried out using very complex equipment often found in centralized laboratories, mostly using molecular and microbiology-based methods. Thus, in present settings, by the time clinical diagnostics are finished, the patient has developed a severe condition. These tactics are incredibly accurate and potent, but they typically require a lengthy procedure, which renders them useless in a variety of situations, such as emergency, ambulatory, and remote contexts. Extensive attempts have been made to provide alternative methodologies for the diagnosis to alleviate such limits (Chandra, 2023; Gupta et al., 2023). Moreover, identifying the metabolic shift of the symptomless transition period is a critical step in the fight against any illness or condition since, at this moment, a little clinical immune booster can reverse the disease, which would otherwise be extremely difficult to treat in its advanced phases. Biomarkers are often detected at much lower quantities in the early phases, which limits the use of important traditional procedures and diagnostic tools while simultaneously providing opportunities for a variety of ultra-sensitive modules. Biosensors have emerged as a key player in the detection of ultralow levels among all available choices (Mahato et al., 2018; Qiu et al., 2023; Rahman and Schellhorn, 2023).
Because smart technologies allow these biosensors to gather data remotely and may be used without the assistance of a specialist, they may change the production costs associated with them. Technological benefits will increase the application domains for the biosensors, and several nations have begun using these trend techniques for newly produced biosensors. Wearable, wireless, multiplex, smartphone-integrated, artificial intelligence-based sensor devices are becoming more noticeable in the COVID-19 (Pateraki et al., 2020; Verma et al., 2022; Parihar et al., 2023; Smith et al., 2023). Due to the drastically rising number of patients worldwide during the pandemic crisis, illness diagnosis, and monitoring have become more crucial than ever. These smart gadgets and telemedicine concepts facilitate remote disease control and communication between medical personnel and patients (Haleem et al., 2021; Omboni et al., 2022; Krishna et al., 2023).
Over the past few decades, there has been a significant increase in the study and creation of biosensors and related signal-processing systems. Some of the biosensors described may never be commercialized. For a nation’s instrumentation business to remain competitive in international markets, biosensor development is essential (Chadha et al., 2022; Geballa-Koukoula et al., 2023; Shoaib et al., 2023). In addition to the overall technical improvement of conventional sensors, many new fields focused on novel sensing methods and materials have emerged. Commercial forces often change, increasing the attractiveness of formerly unprofitable items. New materials or production techniques may also be discovered, which might lead to this (Jackson, 2019; Trigona et al., 2020; Ramesh et al., 2022).
Nanotechnology intervention has transformed several basic and applied scientific domains in the previous few decades. Additionally, the development of nanomedicine and nano-diagnostics has led to significant improvements in the healthcare field in recent times. Because of their superior performance over traditional methodologies, these advancements have garnered increased interest from scientists, engineers, physicians, and communities in both the research and development as well as the commercial domains (Bardhan, 2022; Dash and Kundu, 2023). Due to their widespread domination over lab-based approaches, low sample requirements, speedy analysis, onsite detection, and sturdy nature, these nano-diagnostics are more appealing for commercial acclaim. The advancement of nanotechnology has opened up new possibilities for the development of submicron-sized nanobiosensors that are suitable for intracellular use. Various distinctive phenomena, including dimension, quantum size, and surface effect, which are unique to the production of nanostructured materials and are fundamentally their most appealing characteristic, should be investigated. Furthermore, to demonstrate even superior capabilities for biosensing applications, new nanomaterials must be found (Vo-Dinh et al., 2001; Jianrong et al., 2004; Kubik et al., 2005; Kashyap et al., 2016; Mohammadi Aloucheh et al., 2018; Dedeoglu et al., 2020). Nanotechnology-based biosensors ought to be completely integrated with miniature microfluidic devices, featuring on-chip controllers, electronics, sample processing, and analysis. Providing tools that are easy to use, inexpensive, lightweight, disposable, environmentally friendly, and highly adaptable, would significantly improve their operations (Noah and Ndangili, 2019; Mejía-Salazar et al., 2020; Berlanda et al., 2021; Kulkarni et al., 2022; Radhakrishnan et al., 2022).
Nanobiosensor research has been increasing over the years, according to Pubmed data while searching with the “nano biosensor” keyword. The article numbers of nanobiosensors are folding 7 between 2006–2022. For 2023, 588 articles have been published, and for 2024, 21 articles were already accepted (Pubmed research-nanobiosensors, 2023). The worldwide nanosensors market is estimated to earn $536.6 million in revenue in 2019 and $1,321.3 million by 2026, growing at an 11.0% CAGR during the forecast period (Research, 2019). The nanobiosensor market is expected to reach USD 798.41 Million by 2030 with a 10.60% CAGR(Gotadki, 2023).
Commercialization of nanobiosensor technology has been attempted for more than 10 years, with many of the businesses involved being university spin-outs. Numerous companies have focused on the healthcare sector with goods designed to make laboratory analytical and diagnostic operations easier, faster, or less expensive (Bogue, 2012; Tzouvadaki and Prodromakis, 2023). Even though nanobiosensor research studies are increasing, the number of patents, articles, and commercial products is not consistent. Most research remains at the article level or cannot be commercialized even if a patent is obtained. The insufficient number of commercialized products causes the need in the market to not be fully met (Juanola-Feliu et al., 2012; Farjadian et al., 2019; Rambaran and Schirhagl, 2022a). More institutional and political pressure is being placed on university academics to prioritize commercializing research and to quickly bring their findings to market. There is constant pressure to commercialize, which is frequently portrayed as an unequivocal social benefit deserving of special attention and backing from institutions and the government. Increasing data points to possible hazards arising from or linked to the drive toward commercialization, yet policy declarations and conversations seldom take these concerns into account. Although more investigation is required to validate the presence of these possible hazards, they ought to, at the very least, be included in the public and political discourse on the influence of commercialization on the scope and course of academic research (Caulfield and Ogbogu, 2015).
Because the importance of liaison offices, or technology transfer offices, has been underlined in studies on the relationship between universities and industry, some policymakers have turned to funding technology transfer initiatives at universities. The causes and implications of academic involvement are identified for individuals, organizations, and institutions. These results are then juxtaposed with those of commercialization. Academic engagement is different from commercialization, besides being more common, in that it is strongly related to conventional academic research activities and is sought by academics to get resources that support their research goals (Hulten, 2010; Perkmann et al., 2013).
The inclusion of the sustainability factor in research initiatives from the outset is becoming more and more necessary. As novel materials are being created in lab settings, a common job is to demonstrate their “sustainability” in comparison to pre-existing materials at an extremely early stage of development. Life cycle assessment (LCA) is regarded as the best instrument to measure stated dimensions since it is a commonly used and standardized technique to examine the environmental effect of a process, product, or service. However, a lot of research studies only give data at the lab size, which often leads to a considerably bigger effect when compared to products that are commercially accessible. Advantageous material and energy efficiencies are the consequence of the latter’s scaling effects and optimized process. As such, this comparison does not accurately capture the potential of the created material or technique. Therefore, a scale-up that enables a commercial-scale comparison with rival technology can greatly enhance the environmental evaluation (Piccinno et al., 2016; Kuru et al., 2022). The major phases for effective and safe sensor device usage are defined by the nanobiosensor development life cycle. The stages of the life cycle are research and development, testing, calibration, and assessment (Nanotechnology and Office, 2014). When converting these sensors from an opportunity to a common practical use, it is important to assess their manufacturing potential and include them in the product development cycle. The crucial elements in this case are the metrics of accuracy, stability, repeatability, and reproducibility. All of these characteristics are the end product of the sensor development life cycle, which aims to meet the objectives of the created sensors (Fadel et al., 2016).
Nanotechnology research often requires specialized facilities and costly equipment, which can be costly for research institutions. There may be a disconnect between industry demands and scholarly research, and partnerships may be challenging. Intellectual property ownership and protection can be challenging, and navigating regulatory environments can stall commercialization. Scalable procedures are crucial for preserving nanomaterials’ desired features Early-stage collaboration between industry partners and research institutions can bridge the gap between nanotech research and commercial feasibility. Technology transfer initiatives, government funding, and entrepreneurial skills can accelerate the development of marketable products. Partnerships between research institutes and private businesses are also crucial (Thursby and Thursby, 2011; Rambaran and Schirhagl, 2022b; Malik et al., 2023).
The application of intellectual property and technology to a new product or concept to make a profit is known as commercialization. An excellent foundation for ongoing technological study and development precedes every technology-based production. Stated differently, a newly created technology will improve upon the old technology and bring a new product to market based on demand by using existing technology to a novel concept with a new need (Scheller et al., 1985; Lin, 2005). It is well known that canvas business model implementation is necessary to maintain sustainability and convert concepts into intellectual property (Hernández-Chea et al., 2020). When someone has an innovative concept for a product, business models can help clarify how this enterprise will develop, oversee, and produce (Ucler and Kristensen, 2016). The most popular business model idea for product realization is CANVAS, which is appropriate for value cost, manufacturing strategy, and competitiveness. (Chavarría-barrientos et al., 2017). It is referred to be a visual representation of several factors that illustrate an organization’s ideals and enable decision-making. Combining the triangle of consumers, goods, and market (Mičieta et al., 2020). Marketing is frequently used as a technique to meet needs and increase market share for efficient and competitive business management. Simple good product development, pricing setting, giving to customers, and product enhancement based on consumer feedback are examples of modern marketing methods(Kireev et al., 2016; Tokarski et al., 2017).
An innovative idea with commercial potential requires a well-thought-out framework, definitions, conceptualizations, and procedures. The typical commercialization process includes the following steps: concept, design, prototype design, data analysis and validation, approval, and commercialization. Research and commercial manufacturing follow identical procedures until the approval stage(PELLIKKA and MALINEN, 2014; Datta et al., 2015; Nieto Cubero et al., 2021; Rambaran and Schirhagl, 2022a).
The TRL scale, developed and used by NASA in the 1970s, is used to measure technological maturity and scale for a translational research lab to industry. From 1 to 9, this scale is used to assess the level of technical development. The scale begins at the bottom with TRL 1, which comprises a very basic theoretical type of research and progresses with greater technological improvements. The TRL scale is also known as the technological readiness level. TRL evaluations can be valuable for project management, risk management, and tracking the development of a new technology. TRL may be highly useful in deciding between numerous different technologies that provide the same job(Héder, 2017; Olechowski et al., 2020; BHATTACHARYA et al., 2022). To define the sensitivity of developed nanobiosensors, testing is often utilized to calibrate these tools (Zhou et al., 2015; Gauglitz, 2018; Basicairdata, 2023). TRL 5 to TRL 8 levels of testing procedures would be connected, with different environments and methods for the assessment of sensor performance (Waldmann et al., 2010; Achterberg et al., 2020). Validation of the sensors should also be done because of the development process. Numerous kinds of sensor errors may have an impressive impact on the data’s accuracy and dependability. A three-step process may be used to identify, isolate, and rebuild the aforementioned sensor faults: i) sensor fault detection, ii) sensor fault isolation Reconstructing the sensor defect (iii). Numerous mathematical models, equations, and statistical analyses are used in each of these steps. Remember that to prevent false alarms or missed detections, sensor validation should be carried out before deploying a produced sensor in the field (Pires et al., 2016; Yi et al., 2017).
The commercialization roadmap can be seen in Figure 1. Following these protocols, some concepts need to be pertained to the manufacturing of commercial sensors. i) determining the market needs for a particular analyte, application area, or location. ii) outlining the benefits of the newly designed sensor over the currently used techniques for the same study. iii)verifying and testing the new sensor’s functionality both during operation and after storage. The sensor’s reaction should be at the very least after 6 months of storage for any kind of viable commercial use. iv) Calculating the production costs and manufacturing ease of each component of the sensor (Bhalla et al., 2016). The primary obstacles to the commercialization of sensors may include subpar or improper manufacturing processes, high detection limits, low specificity, low repeatability, inefficient sample flow, low stability, the need for multiplexing, and subjective data interpretation (Kuswandi and Ensafi, 2020).
Determining the needs of the client, scoping, product development, production, sale, and distribution are all steps in the final product realization process. The most fundamental ideas throughout the entire process are decisions. The finest choices and traceability to the product quality management system are necessary for a successful transition to commercialization (International Standard Organization, 2008; Elgh et al., 2018). SWOT analysis, which is regarded as a quicker and easier method of adaptation to identify influencing aspects and discover the optimum solution, may be used to improve quality management systems. It comprises an organization’s or product’s strengths (S), weaknesses (W), opportunities (O), and threats (T) (Paveliuc Olariu, 2009; Dana, 2012). In Figure 2. SWOT analysis of nanobiosensors is listed.
International standards that guide goods in terms of their respective fields, such as ISO 9001:2000, ISO 13407 (human-centered design), and ISO 13485 (medical devices), can also be used to govern the realization of products (ISO, 2000; Jokela et al., 2003; Abuhav, 2011). ISO 14040:2006 defines life cycle assessment (LCA), outlining its purpose, extent, reporting, critical evaluation, limitations, relationships, and requirements for value choices and optional elements (ISO, 2006). A seven-step, systematic approach to methodology has been proposed in the literature to undertake sustainable product realization. It offers a connection between the information and how it is applied to products and market demands. The following are the most important factors: i. Process and control that are appropriate for the desired goals; ii. Customer feedback and critical analysis to enhance the finished product. iii. Selecting the best corrective measures. Any of these may be used to create a new product or make the ones that already exist better. The aforementioned methodology’s phases begin with parameter selection and proceed through process identification, planning, evaluation, and revision (Rihar and Kušar, 2021; OECD, 2023). Nanobiosensors should also encounter these regulations and demands in terms of using them in the industry, hospitals, or onsite applications.
Technological advancements and population growth have led to a surge in the need for new sensors, impacting industrial output, food and water supplies, and health services. Smart sensors have improved people’s quality of life, with future sensors being more sophisticated, sensitive, and accurate. These sensors will be available in various forms, including implantable, wearable, and digestible options. Commercialization is the first step towards their realization. Research on nanotechnology products in industrial and residential applications is growing globally, but their development often outweighs commercialization initiatives, necessitating early commercialization strategies.
Technology transfer protocols can significantly improve the efficiency of the commercialization process for nanotechnology. Labs can adopt these protocols by creating a well-defined Intellectual Property (IP) plan, collaborating with a Technology Transfer Office (TTO), participating in market research, keeping detailed records, forming partnerships with industry partners, formulating a licensing plan, training researchers in technology transfer, obtaining funding for product commercialization, complying with laws and regulations, creating a comprehensive technology transfer plan, and encouraging the commercialization of culture. These actions can help laboratories prepare for technology transfer and increase the likelihood of a seamless transition from the laboratory to the commercial domain. By implementing these protocols, labs can enhance their preparedness for technology transfer and increase the likelihood of a seamless transition from the laboratory to the commercial domain (Palmberg, 2008; National Academies of Sciences, 2016; Europa, 2019).
Comprehensive risk assessments are essential for identifying potential dangers related to nanomaterials in goods. Toxicology studies are crucial for understanding health effects. Exposure situations should be evaluated at every stage of the product’s lifecycle. Risk management techniques like engineering controls, PPE, and containment measures are necessary. Clear communication is also crucial. Regulatory compliance with nanomaterials laws, standardization protocols, clear labeling, monitoring systems, emergency response strategies, and continuous improvement are crucial for safe handling, storage, disposal, environmental impact assessment, and safety procedures (Demirbaş and Çevik, 2020; Kumari et al., 2023).
Researchers are working to address the knowledge gap in understanding the harmful effects of nanotechnology products. Companies using nanotechnology innovations must have risk management protocols, address regulatory barriers, establish technology transfer policies, encourage business incubators, and improve public understanding. Coordination among social actors is essential for ensuring the benefits of nanotechnology outputs align with society’s needs and expectations. To ensure nanotechnology benefits meet society’s needs and expectations, coordinated actions among stakeholders are crucial. This includes sharing resources and expertise, prioritizing ethical considerations, involving the public in decision-making, creating flexible regulatory frameworks, and conducting comprehensive risk assessments. Sustainable technologies should be promoted, considering long-term environmental and socioeconomic implications. By implementing these techniques, stakeholders can ensure research and commercialization align with societal expectations, resulting in beneficial and responsible outcomes.
FU-K: Conceptualization, Data curation, Formal Analysis, Investigation, Methodology, Resources, Software, Validation, Visualization, Writing–original draft, Writing–review and editing. CK: Conceptualization, Data curation, Formal Analysis, Investigation, Methodology, Validation, Writing–original draft. SA: Conceptualization, Funding acquisition, Project administration, Supervision, Writing–review and editing.
The author(s) declare that no financial support was received for the research, authorship, and/or publication of this article.
The authors declare that the research was conducted in the absence of any commercial or financial relationships that could be construed as a potential conflict of interest.
All claims expressed in this article are solely those of the authors and do not necessarily represent those of their affiliated organizations, or those of the publisher, the editors and the reviewers. Any product that may be evaluated in this article, or claim that may be made by its manufacturer, is not guaranteed or endorsed by the publisher.
Abuhav, I., 2011. Iso 13485 A Complete Guide to Quality Management in the Medical Device Industry. Taylor & Francis, Boca Raton, Florida, United States
Bardhan, N. (2022). Nanomaterials in diagnostics, imaging and delivery: applications from COVID-19 to cancer. MRS Commun. 12, 1119–1139. doi:10.1557/s43579-022-00257-7
Basicairdata (2023). Testing sensors. URL https://www.basicairdata.eu/introduction-to-sensor-testing/.
Berlanda, S. F., Breitfeld, M., Dietsche, C. L., and Dittrich, P. S. (2021). Recent advances in microfluidic technology for bioanalysis and diagnostics. Anal. Chem. 93, 311–331. doi:10.1021/acs.analchem.0c04366
Bhalla, N., Jolly, P., Formisano, N., and Estrela, P. (2016). Introduction to biosensors. Essays Biochem. 60, 1–8. doi:10.1042/ebc20150001
Bhattacharya, S., Kumar, V., and Narayan Nishad, S. (2022). Technology readiness level: an assessment of the usefulness of this scale for translational research. Productivity 62, 106–118. doi:10.32381/prod.2021.62.02.2
Caulfield, T., and Ogbogu, U. (2015). The commercialization of university-based research: balancing risks and benefits. BMC Med. Ethics 16, 70. doi:10.1186/s12910-015-0064-2
Chadha, U., Bhardwaj, P., Agarwal, R., Rawat, P., Agarwal, R., Gupta, I., et al. (2022). Recent progress and growth in biosensors technology: a critical review. J. Industrial Eng. Chem. 109, 21–51. doi:10.1016/j.jiec.2022.02.010
Chandra, P. (2023). Personalized biosensors for point-of-care diagnostics: from bench to bedside applications. Nanotheranostics 7, 210–215. doi:10.7150/ntno.81485
Chavarría-barrientos, D., Batres, R., Perez, R., Wright, P., Chavarría-barrientos, D., Batres, R., et al. 2017.
Dana, B. G. (2012). SWOT analysis to improve quality management production. Procedia Soc. Behav. Sci. 62, 319–324. doi:10.1016/j.sbspro.2012.09.052
Dash, S. R., and Kundu, C. N. (2023). Advances in nanomedicine for the treatment of infectious diseases caused by viruses. Biomater. Sci. 11, 3431–3449. doi:10.1039/d2bm02066a
Datta, A., Mukherjee, D., and Jessup, L. (2015). Understanding commercialization of technological innovation: taking stock and moving forward. R&D Manag. 45, 215–249. doi:10.1111/radm.12068
Dedeoglu, A., Kaya, S. I., Bakirhan, N. K., and Ozkan, S. A. (2020). “Nanotechnological approaches and materials in commercial biosensors,” in Commercial biosensors and their applications (Amsterdam, Netherlands: Elsevier), 301–353.
Demirbaş, K., and Çevik, S. (2020). Regulatory policies for safety of nanomaterials. Open J. Nano, 5–6.
Elgh, F. P. W., Johansson, J., Pookrkiany, M., Stolt, R., and Raudberget, D. (2018). Proc. Int. Des. Conf. Des. 1, 249–260.
Europa (2019). Technology transfer in nanotechnology - publications office of the EU. URL https://op.europa.eu/en/publication-detail/-/publication/2ad0aaaf-5ccd-11e9-9c52-01aa75ed71a1/language-en (Accessed December 1, 2024).
Fadel, T. R., Farrell, D. F., Friedersdorf, L. E., Griep, M. H., Hoover, M. D., Meador, M. A., et al. (2016). Toward the responsible development and commercialization of sensor nanotechnologies. ACS Sens. 1, 207–216. doi:10.1021/acssensors.5b00279
Farjadian, F., Ghasemi, A., Gohari, O., Roointan, A., Karimi, M., and Hamblin, M. R. (2019). Nanopharmaceuticals and nanomedicines currently on the market: challenges and opportunities. Nanomedicine 14, 93–126. doi:10.2217/nnm-2018-0120
Gauglitz, G. (2018). Analytical evaluation of sensor measurements. Anal. Bioanal. Chem. 410, 5–13. doi:10.1007/s00216-017-0624-z
Geballa-Koukoula, A., Ross, G. M. S., Bosman, A. J., Zhao, Y., Zhou, H., Nielen, M. W. F., et al. (2023). Best practices and current implementation of emerging smartphone-based (bio)sensors - Part 2: development, validation, and social impact. TrAC Trends Anal. Chem. 161, 116986. doi:10.1016/j.trac.2023.116986
Gotadki, R. (2023). Nanobiosensors in healthcare market scenario. URL https://www.marketresearchfuture.com/reports/nanobiosensors-in-healthcare-market-7993.
Gupta, E., Saxena, J., Kumar, S., Sharma, U., Rastogi, S., Srivastava, V. K., et al. (2023). Fast track diagnostic tools for clinical management of sepsis: paradigm shift from conventional to advanced methods. Diagnostics 13, 277. doi:10.3390/diagnostics13020277
Haleem, A., Javaid, M., Singh, R. P., and Suman, R. (2021). Telemedicine for healthcare: capabilities, features, barriers, and applications. Sensors Int. 2, 100117. doi:10.1016/j.sintl.2021.100117
Héder, M. (2017). From NASA to EU: the evolution of the TRL scale in public sector innovation. Innovation J. 22, 1–23.
Hernández-Chea, R., Vimalnath, P., Bocken, N., Tietze, F., and Eppinger, E. (2020). Integrating intellectual property and sustainable business models: the SBM-IP canvas. Sustainability 12, 8871. doi:10.3390/su12218871
Hulten, D. G. (2010). University-industry technology transfer: who needs TTOs? Int. J. Technol. Transf. Commer. 9, 40. doi:10.1504/ijttc.2010.029424
International Standard Organization (2008). ISO 9000 introduction and support package:guidance on the concept and use of the process approach for management systems. https://www.iso.org/files/live/sites/isoorg/files/archive/pdf/en/04_concept_and_use_of_the_process_approach_for_management_systems.pdf.
ISO (2000). Implementation guide for ISO 9001. https://www.iso.org/files/live/sites/isoorg/files/archive/pdf/en/iso9001implementation_guidance.pdf.
ISO (2006). ISO 14040:2006 - environmental management — life cycle assessment — principles and framework. URL https://www.iso.org/standard/37456.html (Accessed December 1, 2024).
Jianrong, C., Yuqing, M., Nongyue, H., Xiaohua, W., and Sijiao, L. (2004). Nanotechnology and biosensors. Biotechnol. Adv. 22, 505–518. doi:10.1016/j.biotechadv.2004.03.004
Jokela, T., Iivari, N., Matero, J., and Karukka, M. (2003). ACM Int. Conf. Proceeding Ser. 46, 53–60. doi:10.1145/944519.944525
Juanola-Feliu, E., Colomer-Farrarons, J., Miribel-Català, P., Samitier, J., and Valls-Pasola, J. (2012). Market challenges facing academic research in commercializing nano-enabled implantable devices for in-vivo biomedical analysis. Technovation 32, 193–204. doi:10.1016/j.technovation.2011.09.007
Kashyap, P. L., Rai, P., Sharma, S., Chakdar, H., Kumar, S., Pandiyan, K., et al. (2016). Nanotechnology for the detection and diagnosis of plant pathogens. Nanosci. Food Agric. 2, 253–276. doi:10.1007/978-3-319-39306-3_8
Kireev, V. S., Nekrasova, M. L., Shevchenko, E. V., Alpatskaya, I. E., Makushkin, S. A., and Povorina, E. V. (2016). Int. Rev. Manag. Mark. 6, 228–234.
Krishna, C., Kumar, D., and Kushwaha, D. S. (2023). A comprehensive survey on pandemic patient monitoring system: enabling technologies, opportunities, and research challenges. Wirel. Pers. Commun. 131, 2125–2172. doi:10.1007/s11277-023-10535-9
Kubik, T., Bogunia-Kubik, K., and Sugisaka, M. (2005). Nanotechnology on duty in medical applications. Curr. Pharm. Biotechnol. 6, 17–33. doi:10.2174/1389201053167248
Kulkarni, M. B., Ayachit, N. H., and Aminabhavi, T. M. (2022). Recent advancements in nanobiosensors: current trends, challenges, applications, and future scope. Biosens. (Basel) 12, 892. doi:10.3390/bios12100892
Kumari, R., Suman, K., Karmakar, S., Mishra, V., Lakra, S. G., Saurav, G. K., et al. (2023). Regulation and safety measures for nanotechnology-based agri-products. Front. Genome Ed. 5, 1200987. doi:10.3389/fgeed.2023.1200987
Kuru, C. İ., Ulucan-Karnak, F., and Yilmaz-Sercinoglu, Z. (2022). “Nanobiosensors for environmental risk assessment and management,” in Nanobiosensors for environmental monitoring (Cham, Germany: Springer International Publishing), 93–111.
Kuswandi, B., and Ensafi, A. A. (2020). Perspective—paper-based biosensors: trending topic in clinical diagnostics developments and commercialization. J. Electrochem Soc. 167, 037509. doi:10.1149/2.0092003jes
Lin, C.-T. (2005). Biosensor commercialization strategy - a theoretical approach. Front. Biosci. 10, 99. doi:10.2741/1512
Mahato, K., Maurya, P. K., and Chandra, P. (2018). Fundamentals and commercial aspects of nanobiosensors in point-of-care clinical diagnostics. Biotech 8, 149. doi:10.1007/s13205-018-1148-8
Malik, S., Muhammad, K., and Waheed, Y. (2023). Nanotechnology: a revolution in modern industry. Molecules 28, 661. doi:10.3390/molecules28020661
Mejía-Salazar, J. R., Rodrigues Cruz, K., Materón Vásques, E. M., and Novais de Oliveira, O. (2020). Microfluidic point-of-care devices: new trends and future prospects for eHealth diagnostics. Sensors 20, 1951. doi:10.3390/s20071951
Mičieta, B., Biňasová, V., Kasajová, M., and Howaniec, H. (2020). Business model canvas as a tool of manager 4.0. Zesz. Nauk. Wyższej Szk. Humanit. Zarządzanie 21, 51–64. doi:10.5604/01.3001.0014.1235
Mohammadi Aloucheh, R., Alaee Mollabashi, Y., Asadi, A., Baris, O., and Gholamzadeh, S. (2018). Application of nanoscience in self-cleaning properties of concrete facade for development of sustainable environment. Anthropog. Pollut. 2, 16–25. doi:10.22034/AP.2020.1893251.1061
National Academies of Sciences (2016). Triennial review of the national nanotechnology initiative. Washington, D.C., USA: National Academy of Sciences.
Nieto Cubero, J., Gbadegeshin, S. A., and Consolación, C. (2021). Commercialization of disruptive innovations: literature review and proposal for a process framework. Int. J. Innovation Stud. 5, 127–144. doi:10.1016/j.ijis.2021.07.001
Noah, N. M., and Ndangili, P. M. (2019). Current trends of nanobiosensors for point-of-care diagnostics. J. Anal. Methods Chem. 2019, 1–16. doi:10.1155/2019/2179718
OECD (2023). Action steps for sustainable manufacturing. URL https://www.oecd.org/innovation/green/toolkit/actionstepsforsustainablemanufacturing.htm.
Olechowski, A. L., Eppinger, S. D., Joglekar, N., and Tomaschek, K. (2020). Technology readiness levels: shortcomings and improvement opportunities. Syst. Eng. 23, 395–408. doi:10.1002/sys.21533
Omboni, S., Padwal, R. S., Alessa, T., Benczúr, B., Green, B. B., Hubbard, I., et al. (2022). Connected health.
Palmberg, C. (2008). The transfer and commercialisation of nanotechnology: a comparative analysis of university and company researchers. J. Technol. Transf. 33, 631–652. doi:10.1007/s10961-007-9059-6
Parihar, A., Yadav, S., Sadique, M. A., Ranjan, P., Kumar, N., Singhal, A., et al. (2023). Internet-of-medical-things integrated point-of-care biosensing devices for infectious diseases: toward better preparedness for futuristic pandemics. Bioeng. Transl. Med. 8, e10481. doi:10.1002/btm2.10481
Pateraki, M., Fysarakis, K., Sakkalis, V., Spanoudakis, G., Varlamis, I., Maniadakis, M., et al. (2020). “Biosensors and Internet of Things in smart healthcare applications: challenges and opportunities,” in Wearable and implantable medical devices (Amsterdam, Netherlands: Elsevier), 25–53.
Pellikka, J. T., and Malinen, P. (2014). Business models in the commercialization processes of innovation among small high-technology firms. Int. J. Innovation Technol. Manag. 11, 1450007. doi:10.1142/s0219877014500072
Perkmann, M., Tartari, V., McKelvey, M., Autio, E., Broström, A., D’Este, P., et al. (2013). Academic engagement and commercialisation: a review of the literature on university–industry relations. Res. Policy 42, 423–442. doi:10.1016/j.respol.2012.09.007
Piccinno, F., Hischier, R., Seeger, S., and Som, C. (2016). From laboratory to industrial scale: a scale-up framework for chemical processes in life cycle assessment studies. J. Clean. Prod. 135, 1085–1097. doi:10.1016/j.jclepro.2016.06.164
Pires, I. M., Garcia, N. M., Pombo, N., Flórez-Revuelta, F., and Rodríguez, N. D. (2016). From data acquisition to data fusion: a comprehensive review and a roadmap for the identification of activities of daily living using mobile devices. J. Sens. 16, 1–9. doi:10.3390/s16020184
Pubmed research- nanobiosensors (2023). Pubmed research-nanobiosensors. URL https://pubmed.ncbi.nlm.nih.gov/?term=nano+biosensor.
Qiu, S., Cai, Y., Yao, H., Lin, C., Xie, Y., Tang, S., et al. (2023). Small molecule metabolites: discovery of biomarkers and therapeutic targets. Signal Transduct. Target Ther. 8, 132. doi:10.1038/s41392-023-01399-3
Radhakrishnan, S., Mathew, M., and Rout, C. S. (2022). Microfluidic sensors based on two-dimensional materials for chemical and biological assessments. Mater Adv. 3, 1874–1904. doi:10.1039/d1ma00929j
Rahman, M., and Schellhorn, H. E. (2023). Metabolomics of infectious diseases in the era of personalized medicine. Front. Mol. Biosci. 10, 1120376. doi:10.3389/fmolb.2023.1120376
Rambaran, T., and Schirhagl, R. (2022a). Nanotechnology from lab to industry – a look at current trends. Nanoscale Adv. 4, 3664–3675. doi:10.1039/d2na00439a
Rambaran, T., and Schirhagl, R. (2022b). Nanotechnology from lab to industry – a look at current trends. Nanoscale Adv. 4, 3664–3675. doi:10.1039/d2na00439a
Ramesh, M., Janani, R., Deepa, C., and Rajeshkumar, L. (2022). Nanotechnology-Enabled biosensors: a review of fundamentals, design principles, materials, and applications. Biosens. (Basel) 13, 40. doi:10.3390/bios13010040
Research, A. M. (2019). Nanosensors market outlook 2026. URL https://www.alliedmarketresearch.com/nanosensors-market.
Rihar, L., and Kušar, J. (2021). Implementing concurrent engineering and QFD method to achieve realization of sustainable project. Sustainability 13, 1091. doi:10.3390/su13031091
Scheller, F. W., Schubert, F., Renneberg, R., Müller, H.-G., Jänchen, M., and Weise, H. (1985). Biosensors: trends and commercialization. Biosens. (Basel) 1, 135–160. doi:10.1016/0265-928x(85)80001-8
Shoaib, A., Darraj, A., Khan, M. E., Azmi, L., Alalwan, A., Alamri, O., et al. (2023). A nanotechnology-based approach to biosensor application in current diabetes management practices. Nanomaterials 13, 867. doi:10.3390/nano13050867
Smith, A. A., Li, R., and Tse, Z. T. H. (2023). Reshaping healthcare with wearable biosensors. Sci. Rep. 13, 4998. doi:10.1038/s41598-022-26951-z
Thursby, J., and Thursby, M. (2011). University-industry linkages in nanotechnology and biotechnology: evidence on collaborative patterns for new methods of inventing. J. Technol. Transf. 36, 605–623. doi:10.1007/s10961-011-9213-z
Tokarski, A., Tokarski, M., and Wójcik, J. (2017). The possibility of using the business model canvas in the establishment of an operator’ S business plan. Torun. Bus. Rev. 16, 17–31. doi:10.19197/tbr.v16i4.117
Trigona, C., Graziani, S., and Baglio, S. (2020). Changes in sensors technologies during the last ten years: evolution or revolution? IEEE Instrum. Meas. Mag. 23, 18–22. doi:10.1109/mim.2020.9200876
Tzouvadaki, I., and Prodromakis, T. (2023). Large-scale nano-biosensing technologies. Front. Nanotechnol. 5. doi:10.3389/fnano.2023.1127363
Ucler, C., and Kristensen, K. (2016). “CONTEXT-A novel approach for collaborative virtual product realization and its disclosure using the business model canvas,” in 2016 International Conference on Engineering, Technology and Innovation/IEEE Lnternational Technology Management Conference (ICE/ITMC), Trondheim, Norway, June, 2016, 1–8.
Verma, D., Singh, K. R., Yadav, A. K., Nayak, V., Singh, J., Solanki, P. R., et al. (2022). Internet of things (IoT) in nano-integrated wearable biosensor devices for healthcare applications. Biosens. Bioelectron. X 11, 100153. doi:10.1016/j.biosx.2022.100153
Vo-Dinh, T., Cullum, B. M., and Stokes, D. L. (2001). Nanosensors and biochips: frontiers in biomolecular diagnostics. Sens. Actuators B Chem. 74, 2–11. doi:10.1016/s0925-4005(00)00705-x
Waldmann, C., Tamburri, M., Prien, R. D., and Fietzek, P. (2010). Assessment of sensor performance. Ocean Sci. 6, 235–245. doi:10.5194/os-6-235-2010
World health statistics (2023). Monitoring health for the SDGs. Geneva, Switzerland: Sustainable Development Goals.
Yi, T.-H., Huang, H.-B., and Li, H.-N. (2017). Development of sensor validation methodologies for structural health monitoring: a comprehensive review. Measurement 109, 200–214. doi:10.1016/j.measurement.2017.05.064
Keywords: commercialization, nanobiosensors, life-cycle assessment, risk assessment, design
Citation: Ulucan-Karnak F, Kuru Cİ and Akgöl S (2024) Commercial roadmap of nanobiosensor development. Front. Nanotechnol. 6:1348308. doi: 10.3389/fnano.2024.1348308
Received: 02 December 2023; Accepted: 30 January 2024;
Published: 09 February 2024.
Edited by:
Sathish Kumar Kamaraj, Unidad Altamira, MexicoReviewed by:
Preeti Gupta, Leibniz Institute for Solid State and Materials Research Dresden (IFW Dresden), GermanyCopyright © 2024 Ulucan-Karnak, Kuru and Akgöl. This is an open-access article distributed under the terms of the Creative Commons Attribution License (CC BY). The use, distribution or reproduction in other forums is permitted, provided the original author(s) and the copyright owner(s) are credited and that the original publication in this journal is cited, in accordance with accepted academic practice. No use, distribution or reproduction is permitted which does not comply with these terms.
*Correspondence: Fulden Ulucan-Karnak, dWx1Y2FuZnVsZGVuQGdtYWlsLmNvbQ==
Disclaimer: All claims expressed in this article are solely those of the authors and do not necessarily represent those of their affiliated organizations, or those of the publisher, the editors and the reviewers. Any product that may be evaluated in this article or claim that may be made by its manufacturer is not guaranteed or endorsed by the publisher.
Research integrity at Frontiers
Learn more about the work of our research integrity team to safeguard the quality of each article we publish.