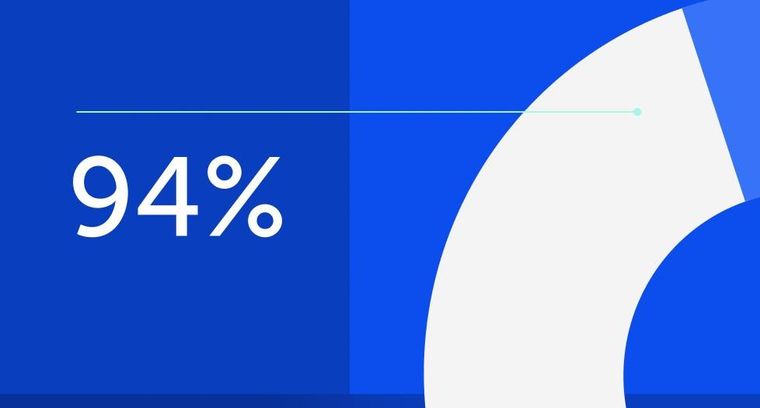
94% of researchers rate our articles as excellent or good
Learn more about the work of our research integrity team to safeguard the quality of each article we publish.
Find out more
REVIEW article
Front. Nanotechnol., 16 February 2024
Sec. Nanomaterials
Volume 6 - 2024 | https://doi.org/10.3389/fnano.2024.1346069
This article is part of the Research TopicOpportunities and Challenges for Nanotechnology in Sustainable Agri-Food ProductionView all 5 articles
Traditional agriculture from cropping to harvesting is contributing to climate change by increasing global greenhouse emissions. Circular economy approaches and biorefinery technologies based on the reuse, recycling, and remanufacturing might result in the valorization of wastes that consequently would avoid environmental pollution. Nanoparticles synthesis using bio-waste such as stems, leaves, seeds, pulp, stubble, and bagasse is considered a green approach with low energy consumption, and low-cost production. Characteristics of raw materials influence the final application of nanoparticles in edible coatings, and films. Therefore, the preparation of nanoparticles based on cellulose, pectin, metal (titanium oxide, silver, zinc oxide), or silica are considered organic, inorganic, or hybrid nanocomposites, are resulted in several benefits including shelf-life extension and broad-spectrum antimicrobial properties by its capacity to encapsulate active compounds that greatly improve food preservation. For considering agro-waste-based nanoparticles in food, challenges in homogenization and synthesis, yield, and toxicity are mainly described. Therefore, this review examines the employment of agro-industrial waste for the development of sustainable nanoparticles and their synthesis methods (top-down and bottom-up). Moreover, it discusses their incorporation and role in active edible coatings and films by highlighting their bioactive properties, mechanisms of action, and applications in food group preservation.
Agricultural production has expanded more than three times in the last 50 years, due to the rising demand for food caused by population growth. The agri-food system is at the center of global challenges including economic, environmental, and social ones; the effects of resource scarcity, climate change, ecosystem degradation, and biodiversity loss are inextricably linked to food production, distribution, consumption, and management policies, as well as the generation of large amounts of waste (Sadh et al., 2018; Duque-Acevedo et al., 2020; Singh et al., 2021; El-Ramady et al., 2022). Currently, around 30% of worldwide agricultural production is classified as agro-industrial waste, which is equivalent to more than 2 billion tons of waste. Agro-industrial waste comes from residues from the production, distribution, and commercialization chain of fruits, vegetables, meat products, and dairy products among many others (Sadh et al., 2018; Duque-Acevedo et al., 2020; Singh et al., 2021; El-Ramady et al., 2022). The intensive search to improve crops usually results in environmental problems such as the excessive use of fertilizers, pesticides, and other additives. In addition, the excessive production of food ends up in the accumulation of large quantities of waste or residues including seeds, pulp, peels, lignocellulosic residues, and so on. All these contaminants are dispersed in superficial water, groundwater, and soils via the natural water cycle; the long-term effects on health and ecosystems are currently impossible to measure (Sadh et al., 2018; Duque-Acevedo et al., 2020; Singh et al., 2021; El-Ramady et al., 2022; Coronado-Apodaca et al., 2023).
The inadequate disposal of agro-industrial waste generates greenhouse gases, which in turn promotes climate change and alterations in the ecosystem (Duque-Acevedo et al., 2020; Ortega et al., 2021). To mitigate its effects, some strategies have been proposed, for example, circular economy approaches and biorefinery technologies based on the reuse, recycling, and remanufacturing might result in the cleaner and ecological management of wastes that consequently would avoid environmental pollution (Barragán-Ocaña et al., 2023). This type of technology allows the transformation of biowaste into value-added products through hydrothermal liquefaction, carbonization, anaerobic digestion, aerobic fermentation, or gasification (Duan et al., 2022). The main value-added products obtained are biofuels, biogas, bioplastics, antibiotics, biofertilizers, compounds for the cosmetic industry, and additives or coatings for the food industry. This wide range of value-added products involves lower production costs and more accessibility, in comparison to the conventional production methods to obtain materials like fuels and plastics (Duque-Acevedo et al., 2020; Singh et al., 2021).
In the food industry, the use of agro-industrial waste offers great opportunities for the development of eco-friendly packaging or coatings able to replace plastic-based packaging derived from petroleum (Ortega et al., 2021). Nanotechnology is playing a critical role in the design of enhanced food packaging. Nanomaterials, defined as materials with at least one dimension in the range of 1–100 nm, have demonstrated beneficial features for food products such as protection from pathogenic microorganisms that might affect human health (Ashfaq et al., 2022). Moreover, the synthesis of nanoparticles using bio-waste is considered a green approach, since the carbon footprint is reduced, and the energy consumption is lower than that required by physical or chemical synthesis methods (Zamare et al., 2016; Lopes and Ligabue-Braun, 2021). The great advantage of agro-industrial waste-based nanoparticles is the low production costs, in addition to their great heterogeneity, which allows their combination with different compounds to obtain different types of nanoparticles based on cellulose, pectin, metal (titanium oxide, silver, zinc oxide), or silica (Aswathi et al., 2022).
Nanoparticles have been recently explored for the preparation of food protection systems since they can extend the food shelf-life through protection against UV irradiation, oxygen uptake, and antimicrobial activity (Figure 1) (Ramesh and Radhakrishnan, 2019; Ashfaq et al., 2022). Materials in the nanoscale possess unique and improved properties in comparison to their larger forms, which are advantageous for food protection systems (Zambrano-Zaragoza et al., 2018). Typically, nanoparticles are introduced or embedded in edible coatings like cellulose, chitosan, and polyvinyl alcohol, which alters the physical and mechanical properties such as flexibility, strength, thermal stability, gas permeability, and antimicrobial activity, while maintaining the quality of food characteristics like flavor and color (Kritchenkov et al., 2020; Kritchenkov et al., 2021; Ashfaq et al., 2022; Mehmood et al., 2022).
FIGURE 1. Schematic illustration of the production of nanoparticles from agro-industrial waste and their incorporation into edible coatings to protect food from gases (carbon dioxide or oxygen), UV rays, and pathogenic microorganisms. Created with BioRender.com and extracted under premium membership.
Nanoparticles applied for food protection systems require certain properties. For instance, antimicrobial activity is of main relevance to guarantee food safety; according to the Food and Agriculture Organization of the United Nations, it is estimated that around 1.3 million tons of food are discarded annually due to spoilage mainly caused by microorganisms (Fao, 2019; Tropea, 2022). Food can be contaminated by microorganisms at any stage of its production, causing alterations in its properties in terms of smell, taste, and appearance, among others (Kamala and Kumar, 2018). More importantly, contaminated food is a serious problem for public health, since it is a source of transmission of diseases that affect around two billion people worldwide (Draeger et al., 2018; Tropea, 2022). In this sense, the nanoparticles in biopolymer-based coatings or films can address microbial contamination providing prolonged antimicrobial activity against a broad spectrum of pathogens such as fungi, viruses, and bacteria, through the diffusion of nanoparticles incorporated into coatings or films, improve the food quality and safety (Adebayo et al., 2019). In food technology, the design of materials to overcome pathogenic growth even microorganism resistant has taken on great relevance. Metal and metal oxide nanoparticles have been recognized as beneficial approaches to manage microbial resistance, promoting the death of these contaminating agents through various mechanisms that damage the structures (DNA, proteins, organelles) of the cells (Deepa et al., 2015). In addition, hybrid nanocomposite materials are innovative platforms with advanced properties for the development of coatings or edible films and are being created to reduce oxidation and spoilage of food products, even to reduce vapor and gas transfer. These nanocomposites can properly align as supporters of antimicrobials, antioxidants, colors, and flavors resulting in excellent performance against pathogens and reinforcing characteristics for successful in market applications (Kumar Trivedi et al., 2023).
Thus, this review is an additional promotion of the reuse of materials derived from agro-industrial waste as a sustainable source of nanoparticles (organic, inorganic, hybrid) that can be produced through green methods making a positive environmental footprint. Furthermore, we focus on the incorporation of nanoparticles in edible coatings or films that are capable of inhibiting or slowing the growth of pathogens and improving mechanical, physical, and biological properties (Deepa et al., 2015; Adebayo et al., 2019; Periakaruppan et al., 2022; Kumar Trivedi et al., 2023). Finally, the current challenges that need to be overcome to achieve applicability in the market have been covered and discussed for improving the preservation of food.
Agro-industrial waste is generated from multiple materials including vegetable organic matter (stems, leaves, seeds, pulp, stubble, bagasse) or animal organic matter (manure, and feathers) (Lopes and Ligabue-Braun, 2021; El-Ramady et al., 2022). Agro-industrial residues need to be adequately processed through biological, chemical, physical pretreatments, or a combination of those processes (Bala et al., 2023). The pretreatments allow the transformation of agro-industrial waste into simpler structures by causing alterations in the physical-chemical properties of the raw material (Ravindran et al., 2018; Judith and Vasudevan, 2022). Different parameters are considered for the selection of the pretreatment such as the composition of the waste material and the desired nanoparticles to be obtained [28,30]. As an additional benefit, pretreatments can be useful to remove contaminants that may affect the development of nanoparticles (Ravindran et al., 2018; Judith and Vasudevan, 2022). Pretreatments are usually considered as a pre-requisite for the subsequent synthesis of nanoparticles which is typically performed through top-down and bottom-up methods (Figure 2) (Zamare et al., 2016; Ashfaq et al., 2022; Aswathi et al., 2022).
FIGURE 2. Methodologies used to produce nanoparticles from agro-industrial waste. Typically, before the nano-synthesis is performed a (Sadh et al., 2018) pretreatment which can be physical, chemical, or biological methods is carried out (Duque-Acevedo et al., 2020). Subsequently, conventional strategies can be using top-down or bottom-up technologies for the synthesis of nanoparticles. Top-down consists of using raw material blocks that are destroyed to produce nanoparticles, while the bottom-up approach uses atoms or molecules for the construction of nanoparticles. Finally (Singh et al., 2021), nanoparticle characterization techniques are carried out to determine their size (microcopy and Dynamic Light Scattering), shape (microscopy), optical properties (UV-Vis), crystallinity (microscopy), and internal structure (FTIR and NMR). Created with BioRender.com and extracted under premium membership.
Top-down technologies consist of breaking down or destroying the bulk matter to reduce it into nanoscale pieces; the transformation can be caused by chemical and mechanical energy forces (González-González et al., 2022a). This approach includes techniques such as electrospinning, laser ablation, sputtering, nanolithography, and ball milling (Ashfaq et al., 2022; Judith and Vasudevan, 2022). The electrospinning technique consists of the synthesis of fibers using polymers and composites derived from agro-industrial bio-waste, which are subjected to electrostatic forces by the electrification of a liquid drop by applying a high electrical voltage (Balzer et al., 2018; Xue et al., 2019; Sharma et al., 2022). The continuous increase in voltage induces the elongation of the drop in a conical shape (Taylor cone shape), avoiding agglomeration due to high shear force and repulsion (Balzer et al., 2018; Xue et al., 2019; Sharma et al., 2022). This technique produces fibers in a size range from nano to micro-scale and with multiple structures and different properties (Balzer et al., 2018; Radacsi et al., 2018). On the other hand, laser ablation uses an intense pulsed laser beam to remove regions of matter by vaporizing atoms of the target (Shojaei et al., 2022). This technique allows the development of nanoparticles and nano-systems without the use of chemical compounds and provides advantages such as a low number of impurities and the agglomeration of nanoparticles during synthesis (Kugarajah et al., 2022).
Another interesting technique is sputtering, which involves using high-energy plasma or gas to produce ion irradiation on a target surface. This causes a series of elastic and inelastic collisions that release a wide variety of particles (positively or negatively charged secondary ions), neutral atoms, and clusters of atoms (Ashfaq et al., 2022). Nanolithography involves the use of light, electron beams, or charged ions to transfer architectural patterns at the nanoscale from a prefabricated photomask to a photoresist layer that covers the target material (Yang, 2015; Ashfaq et al., 2022). On the other hand, ball milling is a mechanical approach for reducing the size of waste materials by using rolling balls that apply kinetic energy to wear down the material at a nanometric scale (Yadav et al., 2012; Ashfaq et al., 2022). There are additional varieties of ball milling, such as mechanochemical milling, which–based on the same principle–employs reagents to produce a chemical reaction and thus promote the synthesis of nanomaterials (Chaudhuri et al., 2021).
Bottom-up techniques enable the formation of nanoparticles from atoms or molecules, by manipulating parameters such as pressure, temperature, or the use of chemical substances (González-González et al., 2022b). This category includes methods such as pyrolysis, co-precipitation, hydrothermal, microwave irradiation, and sol-gel (Ashfaq et al., 2022; Aswathi et al., 2022; Judith and Vasudevan, 2022). Pyrolysis is a thermochemical method that degrades raw materials by increasing the temperature in an environment free of oxygen; this technique has been effectively employed for the synthesis of nanoparticles (Zahid et al., 2018; González-González et al., 2020). The co-precipitation method, on the other hand, is mainly used to prepare metallic nanoparticles using acid substances such as HNO3, HCl, and H2SO4; in this manner, metals can be leached from agro-industrial waste by precipitating them with a basic substance such as MgO or NaOH. Parameters like pH value, reaction temperature, and stirring rate are key factors for the obtention of nanoparticles with specific sizes and shapes (Bajaj and Joshi, 2021; Judith and Vasudevan, 2022).
Another method belonging to this approach is the hydrothermal technique, in which nanoparticles are synthesized in autoclaves subjected to certain conditions of temperature (120°C–550°C) and pressure (20–150 bar) (Judith and Vasudevan, 2022; Cárdenas-Alcaide et al., 2023; Wen et al., 2023). On the other hand, microwave-assisted synthesis involves the generation of electromagnetic waves that interact with reactants and solvents, resulting in efficient heat transfer caused by a chemical reaction. Microwaves have the advantage of accelerating chemical reactions and reducing nanoparticle manufacturing times (Ravindran et al., 2018; Dutta, 2021; Judith and Vasudevan, 2022). Nanoparticles can also be synthesized using the sol-gel technique, which produces a sol as a result of hydrolysis and condensation reactions; subsequently, it is subjected to a set of chemical reactions and/or thermal treatments to form a gel, which is then calcined to yield the final nano-scaled product (Judith and Vasudevan, 2022; D'Arienzo et al., 2017).
Once the nanoparticles have been synthesized, it is highly relevant to analyze their physicochemical properties including shape, crystallinity, internal structure, and size (Mourdikoudis et al., 2018). Microscopy techniques, primarily Transmission Electron Microscope (TEM) or Atomic Force Microscope (AFM), are used to collect information about the morphology, size, and crystalline structure (Mourdikoudis et al., 2018). Dynamic Light Scattering (DLS) is a simple technique for determining the diameter size of nanoparticles distributed in a liquid media (Ramos, 2017). The Fourier-transform spectroscopy (FTIR) technique can be used to determine the composition and structure of nanoparticles by measuring the absorption and emission in the mid-infrared region (4,000–400 cm−1) (Mourdikoudis et al., 2018). On the other hand, UV-Vis can be used to analyze the optical properties, state of agglomeration, and shape of nanoparticles (Mourdikoudis et al., 2018). Molecular analysis such as atomic composition, the influence of ligands on nanoparticles, and electronic core structure, can be obtained by Nuclear Magnetic Resonance (NMR) method (Mourdikoudis et al., 2018).
Nanotechnology has offered great possibilities to innovate in the food industry such as the production of nanoparticles for their incorporation in active edible coatings, and films. In these applications, the antimicrobial potential of the proposed nanomaterials is of main relevance; thus, researchers have recently explored simple synthetic methods, natural and cheap precursors, antimicrobial mechanisms, and factors influencing the antimicrobial capacity, among other aspects (Yallappa et al., 2017; Jadhav et al., 2023; Kumar Trivedi et al., 2023). Thus, different nanomaterials have been prepared by using agro-industrial waste with a clear impact on food preservation (Table 1).
TABLE 1. Sources, synthesis methods, and applications of nanomaterials prepared from agro-industrial wastes.
Organic nanoparticles provide food coating characteristics such as thermal stability, barrier activity against water vapor, increased mechanical properties, tensile strength, longer shelf life, and antimicrobial properties (Ashfaq et al., 2022). Currently, agro-industrial residues have been employed as sustainable sources for the development of nanoparticles because they are cheap, and abundant natural precursors (Nuilek et al., 2018). For instance, Yallappa et al. (2017) prepared carbon-based nanoparticles through a simple pyrolysis process using groundnut shells as a natural precursor (Yallappa et al., 2017). The synthesis was performed under a nitrogen atmosphere at different temperatures (550°C, 750°C, and 950°C). Interestingly, it was observed that increasing the pyrolysis temperature led to carbon nanoparticles with smaller particle sizes, which in turn caused better antibacterial activity. Some characteristics of the particles at the nanoscale such as the high surface area-to-volume ratios are associated with the antibacterial mechanisms. In this manner, the synthesized nanoparticles with reduced size were able to easily interact with bacteria, which in turn improved their activity (Yallappa et al., 2017). On the other hand, Roy et al. (2021) developed porous carbon nanospheres from the biowaste of garlic peels via pyrolysis under a nitrogen atmosphere at 500°C. These nanospheres demonstrated antimicrobial activity against E. coli, which could be attributed to membrane deformations caused by the interaction with the nanospheres (Roy et al., 2021). Both studies used a simple one-pot pyrolysis which has the advantage of being relatively fast, simple, and scalable (Roy et al., 2021).
Nanocellulose, defined as nano-structured cellulose obtained from cellulosic sources, exhibits excellent characteristics such as large surface area, low density, biodegradability, non-toxicity, high tensile strength, high stiffness, and low thermal expansion enabling the production of sustainable and high-value nano-based products (Deepa et al., 2015; Kumar Trivedi et al., 2023). The characteristics and performances of nanocellulose are highly affected by two main factors: the extraction method and the source (Kumar Trivedi et al., 2023). The extraction method is usually a combination of techniques either belonging to chemical or mechanical treatments. The most common pretreatments to eliminate lignin and assist the formation of nanocellulose are acid hydrolysis, alkaline treatment, liquid hot water, and bleaching. Acid hydrolysis and mechanical pretreatments are the most common methods for breaking down cellulose, although these methods demand energy and water. Enzymatic hydrolysis is an eco-friendlier option although much more expensive. The alkaline treatment is also frequently used as it is less dangerous. It can occur under gentler conditions and without persistent or bio accumulative chemicals. Liquid hot water and ionic liquids are more environmentally friendly options and have a high efficiency but work under high pressures. Bleaching commonly uses harsh chemicals as it often involves adding chlorine to the cellulose in the presence of an acid (Mateo et al., 2021). There is a vast variety of cellulosic sources including plants, bacteria, and animals. Examples of plant-based sources include wood, leaves, and cotton; bacteria cellulosic sources include different Gram-negative bacteria (e.g., Pseudomonas, Agrobacterium, Salmonella) and Gram-positive bacteria (Sarcina ventriculi); while animals able to act as cellulosic sources are the urochordates due to their ability to synthesize cellulose (Nakashima et al., 2004; Wang et al., 2019).
Thus, different types of agro-industrial wastes have been employed as sources to produce nanocellulose with specific characteristics. For example, Ramesh and Radhakrishnan (2019) obtained cellulose nanoparticles from potato peel; nanoparticles were extracted using different processes such as alkaline treatment, bleaching, and acid hydrolysis (Ramesh and Radhakrishnan, 2019) (Table 1). Homogeneous spherical nanoparticles with a diameter in the range of 50–100 nm were reported with an extraction yield of 39.8%, which was significantly higher than those reported by other authors. The particle size and crystallinity index, in addition to the results obtained from morphological and topographical analyses, demonstrated the high quality of the nanocellulose for active packaging; the extracted cellulose nanoparticles incorporated in the packaging films caused an enhancement in the elongation properties and tensile strength (Ramesh and Radhakrishnan, 2019). Similarly, Trivedi et al. (2023) extracted nanocellulose with a diameter below 100 nm using wheat straw as the cellulosic source (Kumar Trivedi et al., 2023). They employed an effective combination of extraction methods involving chemical treatments and mechanical grinding. Cellulose nanoparticles exhibited good properties during the characterization techniques suggesting potential applications as a reinforcing material for nanocomposites (Kumar Trivedi et al., 2023).
A wide range of sources has been employed for the preparation of nanocellulose, such as banana rachis, kapok, coir, pineapple leaf, sisal, wheat straw, and palm waste, among others (Deepa et al., 2015; Kumar Trivedi et al., 2023; Yahya et al., 2023). Cellulose nanomaterials have shown excellent properties; however, there is an increasing research interest in enhancing their properties. Nanocellulose with enhanced properties will have a positive impact on the performance of targeted materials since the overall chemical, mechanical, biological, and physical characteristics will be affected (Zaki et al., 2022). In this context, Zaki et al. (2022) explored the addition of a microbial pretreatment aimed at increasing the quality of cellulose nanoparticles (Zaki et al., 2022). Nanocellulose, extracted from sawn timber, showed noticeable differences depending on whether the microbial pretreatment with Echinodontium taxodii was performed. In this manner, the thermal, morphological, and mechanical properties were significantly enhanced on the microbial-treated nanocellulose. This can be attributed to the fact that during the microbial pretreatment, the material turned more fragile and vulnerable to pulping, bleaching, and hydrolysis processes, which in turn improved the extraction of nanocellulose and its quality. Furthermore, microbial-treated nanocellulose and untreated nanocellulose were used for the fabrication of membranes; similarly, the membranes with microbial-treated nanocellulose exhibited superior thermal and mechanical stability, lower water absorption capacity, and higher water contact angle. Thus, the authors concluded that the proposed method produced enhanced nanocellulose with great potential in packaging applications (Zaki et al., 2022).
Other types of nanostructured materials can be obtained from agro-industrial wastes, such as pectin, which is a biopolymer safe for human consumption. Moreover, different studies have reported its successful application in food and pharmaceutical products. For instance, pectin can be used as a stabilizer, or pharmaceutical excipient, a component in the design of drug delivery systems, and a reservoir of molecules with biological activity. For example, Arias et al. (2021) prepared pectin nanoparticles using Musa paradisiaca banana peels as the precursor and evaluated different parameters such as yield, dynamic viscosity, and solubility depending on experimental variables; their research objectives were directed to enhance the nanoparticle’s formation under environmental conditions (Arias et al., 2021).
Palm oil, coconut husk, wheat husk, and fruit peels and seeds, among other agro-waste materials, have been employed to produce different types of nanomaterials. A large fraction of agro-waste is biomass with a diverse composition including carbohydrates, proteins, and chemicals that enable the synthesis of nanoparticles while acting as stabilizing agents at the same time (Nakashima et al., 2004). The production of metallic nanoparticles commonly requires a reducing agent. Recently there has been growing literature on the use of agro-waste as a stabilizing or reducing agent, which is a more environmentally friendly option. For example, Adebayo et al. (2019) report the synthesis of silver nanoparticles, gold nanoparticles, and bimetallic alloy (Ag-Au) nanoparticles using Persea americana (avocado peel) as a reducing agent. Their process consisted of the preparation of the avocado peel extract by suspending milled avocado peels in distilled water; then, the container was placed in dark conditions for 24 h for the subsequent filtration and centrifugation. The obtained extract was added into the reaction vessel containing silver nitrate, chloroauric acid, and a mixture of both reagents to synthesize silver, gold, and bimetallic alloy nanoparticles, respectively. The reaction conditions were kept constant for the three syntheses: room temperature and static conditions until the observation of a color change indicating the formation of nanoparticles (Adebayo et al., 2019). The antioxidant, antimicrobial, and antibacterial properties of the synthesized nanoparticles were evaluated, showing outstanding results. Thus, it was concluded that the avocado peel extract is suitable for the synthesis of metallic nanoparticles for antimicrobial and antioxidant applications (Adebayo et al., 2019). Similarly, Alomar et al. (2020) reported the synthesis of silver nanoparticles using leaf extract of Peganum harmala, as a reducing agent and stabilizer. The synthesized nanoparticles exhibited a remarkable antibacterial potential against E. coli, S. aureus, and B. cereus (Alomar et al., 2020).
It has been demonstrated that plants–and their different parts–have great potential as stabilizing agents in the synthesis of nanomaterials, especially for food-safety applications. Carbohydrates, proteins, and polyphenolic compounds play a key role in the reduction of silver ions and facilitate the synthesis of nanoparticles (Kumar et al., 2021). Moreover, nanoparticles with different characteristics such as shape, morphology, and size can be obtained by varying the pH value, metal ion concentration, reaction time, reaction temperature, or the plant’s composition. For example, the size and morphology of gold nanoparticles obtained from plants are conditioned by the pH, temperature, and incubation time, causing an acidic pH of approximately 2 the production of larger rod-shaped nanoparticles, pH of 3-4 the development of smaller rod-shaped nanoparticles, while pH 9 produces spherical nanoparticles, and pH 11 nanowires. It has also been observed that the increase in salt concentration and temperature accelerates the obtaining of gold nanoparticles (Hammami et al., 2021).
The role of agricultural waste materials during the synthesis of nanomaterials is not limited to acting as a reducing agent, but also, can act as precursors for the extraction and synthesis of nanomaterials such as silica nanoparticles (Snehal et al., 2018; Yadav et al., 2022). Silica nanoparticles extracted from agro-waste provide an innovative approach to the green synthesis of nanomaterial. Moreover, nanosized silica exhibits advantageous features in comparison to their bulk counterparts, such as antibacterial capabilities, high surface area, and photoluminescence that allow their successful application in different fields including catalysis, bioimaging, biomedicine, and the food industry.
As a representative example, Shahi et al. (2021) reported an integrated system for the fractionation of different agro-wastes (sugar cane bagasse, rice husk, and peanut shell) into biopolymers and silica nanoparticles. The most frequently used method for silica nanoparticle synthesis is chemical vapor deposition which requires a hydrocarbon source of carbon, often methane (Ha et al., 2022). This study proposed the use of liquid natural gas which is less expensive than pure methane (Ha et al., 2022). Nanoparticles presented different characteristics according to the source material; sugar cane bagasse and peanut shell formed crystalline nanoparticles, while nanoparticles obtained from rice husk were amorphous. Differences in morphology, size, and shape were also reported. This study demonstrated the feasibility of utilizing both lignocellulosic components and silica from diverse agro-waste materials to produce various by-products using the same source, thus, showing huge environmental and economic advantages (Shahi et al., 2021).
Other silica sources have been reported, for example, the leaves of C. dactylon are an exceptional source of silica nanoparticles due to the silica stored in the form of phytoliths in their epidermal parts. Babu et al. (2018) synthesized spherical silica nanoparticles from C. dactylon with an average size of 60 nm and an amorphous nature (Babu et al., 2018). Furthermore, the synthesized silica nanoparticles showed potential antimicrobial activity against different bacteria such as Escherichia coli, Pseudomonas aeruginosa, Bacillus subtilis, and Staphylococcus aureus. This study highlights the feasibility of synthesizing silica nanoparticles with remarkable antimicrobial activity through an eco-friendly approach; the utilization of parts of plants considered agro-industrial waste as reducing agents is a more environmentally friendly and cost-effective approach in comparison to the chemical and physical synthesis methods (Babu et al., 2018). Similarly, Periakaruppan et al. (2022) prepared silica nanoparticles using Punica granatum leaf extract through a simple and cost-effective process. Nanoparticles exhibited a spherical shape, an average size of 12 nm, and remarkable antibacterial activity against E. coli and Salmonella sp (Periakaruppan et al., 2022). Amorphous silica nanoparticles are frequently used as food additives, for example, as thickeners, anticaking agents, or carriers for flavors in concentrations ranging <0.1–6.9 mg/g of the product and particle sizes ranging from 30 to 200 nm. Further studies to clarify the safety and toxicity of these nanomaterials need to be considered (Sen and Sen, 2020).
Combinations of two or more materials through any method, in which one or more of the components is on the nanometer scale are referred to as nanocomposites (Sen and Sen, 2020). These nanocomposites make advanced use of the features of the contributing materials to display new properties for improved applications, including the production of high-strength and lightweight materials for automotive applications, agricultural use, smart food packaging, biomedical applications, drug delivery systems, wastewater treatment, improved batteries and electronic devices, gas/liquid barriers, sensors, UV protective coatings, consumer goods, among others (Bikiaris, 2022). Several combinations of nanocomposites can be produced, including cellulose-metal-oxide-polymer, metal-metal oxides, metal-polymer, metal-oxide-polymer, metal-carbon dots, metal-graphene oxide-polymer, metal oxide-graphene oxide polymer, and metal-metal oxides. They can be designed to improve food quality and safety. Potential applications have been identified according to their characteristics as active food films, adding antimicrobial and antioxidant activities and enhancing their efficiency.
Food packaging can benefit greatly from the photocatalytic and antibacterial properties of titanium oxide (TiO₂) nanoparticles (Anaya-Esparza et al., 2020). Multiple sources can be used for the green synthesis of TiO₂ nanoparticles, including parts of plants such as roots and leaves from mint and aloe, however, the relevance of fruit peels like plum, peach, and kiwi is highlighted for topics around reuse (Ajmal et al., 2019). Bio-nanocomposites based on TiO₂ have been employed as effective packaging materials for a variety of food goods that are oxygen-sensitive (Zhang and Rhim, 2022). For instance, Salama and Aziz (2020) presented a study on active packaging based on TiO₂ for the preservation of green bell peppers. This coating was synthesized first as a carboxymethyl cellulose and guanidinylated chitosan film, into which TiO₂ nanoparticles were incorporated in different concentrations. It was demonstrated that increasing the percentage of TiO₂ nanoparticles augmented the thermal stability and the water vapor permeability was reduced. Particularly at concentrations of 3% and 5% TiO₂ concentrations, the nanocomposites demonstrated notable UV-barrier characteristics and improved antibacterial activity. During shelf-life testing, green bell peppers coated with nanocomposite films displayed excellent resistance to mass loss and deterioration throughout storage (Salama and Abdel Aziz, 2020a).
Due to their strong antibacterial activity, silver nanoparticles (AgNPs) have attracted a lot of interest in the food packaging industry (Bruna et al., 2021). However, the use of chemically manufactured AgNPs has been constrained because of their possible toxicity on humans at high dosages and the uncertainty associated with their mechanisms of action and their potential effects (Ferdous and Nemmar, 2020). In earlier research, AgNPs were chemically synthesized and used in packaging; however, it was noted that this type of nanoparticle does not present antioxidant effects, which is crucial for packaging applications. To overcome this barrier, several reports demonstrated the importance of using natural sources as stabilizers and reducing agents for the synthesis of AgNPs. The formation of nanoparticles using natural sources like plants has several advantages. For instance, plant-derived nanoparticles might possess extra antioxidant and antibacterial characteristics by phytocompounds from plants that exhibit antioxidant and antibacterial activity (Kumar et al., 2019). In the study conducted by Zhao et al. (2022), two different types of AgNPs were synthesized: GSE-AgNPs that used fruit waste grape seed extract as a stabilizer and PVP-AgNPs in which Polyvinylpyrrolidone was employed. Both types of AgNPs were added to a polymeric matrix of chitosan (CS) to prepare two different coatings for their evaluation in maintaining the quality of grapes. The outcomes demonstrated that in comparison to untreated fruit and fruit treated with CS-PVP-AgNPs, grapes treated with CS-GSE-AgNPs had much lower decay percentages, weight loss, higher antimicrobial, enhanced antioxidant activities, and maintained titratable acidity at high levels. In addition, CS-GSE-AgNPs greatly reduced the overall yeast and mold counts during storage (Zhao et al., 2022).
Similarly, zinc oxide (ZnO) nanoparticles have gained increasing attention in the food industry because of their strong catalytic and photocatalytic properties and relatively high chemical reactivity. ZnO nanoparticles show great resistance to heat, UV light, and infrared radiation, which is helpful for active packaging applications. In addition, zinc serves as a crucial micronutrient in the body’s production of proteins and nucleic acids. Therefore, ZnO nanoparticles are appropriate for the food industry, as long as they are employed in the proper dosage (Subhan et al., 2022). Several forms of ZnO nanoparticles have been manufactured and applied to active packaging. As a representative example, Hu et al. (2022) prepared a multifunctional food packaging material made of biodegradable polyvinyl alcohol/starch (PVA/ST) film added with rod-like ZnO nanofillers. The study evaluates the effect of adding rod-like ZnO nanofillers in the PVA/ST composite films in terms of UV-shielding, antibacterial, mechanical, thermal, and water barrier capabilities. The rod-like ZnO nanofillers were evenly distributed throughout the PVA/ST matrix, producing compact and dense nanocomposite films. In comparison to PVA/ST film without ZnO, the nanocomposite films exhibited enhanced mechanical and water vapor barrier characteristics. Moreover, ZnO nanofillers provided superior antibacterial activity against E. coli and S. aureus, as well as exceptional UV-shielding capabilities while maintaining a high level of optical transparency (approximately 90%). The PVA/ST/ZnO films were tested for the active packaging of fresh-cut carrot slices, which presented an extended shelf-life by preventing microbiological contamination (Hu et al., 2022).
On the other hand, silica nanoparticles (SiO2 NPs) have been widely utilized in different industries including food, microelectronics, pharmaceutical, and cosmetic industries. SiO2 nanoparticles are especially interesting due to their small particle size, large specific surface area, high surface energy, and large proportion of surface atoms (Akhayere et al., 2022). Recent research has demonstrated that the mechanical and barrier characteristics of biopolymer-based films have been improved by the addition of SiO₂ NPs (Lin et al., 2020). However, SiO₂ NPs suffer from the same shortcomings as AgNPs, they do not work as antioxidants. The antioxidant activity is crucial in food packaging applications; thus, SiO₂ NPs must be coupled with other agents to form nanocomposites for active packaging. For example, Cheng et al. (2021) utilized hop extract (HE) to provide the antioxidant effect to a chitosan (CS) and SiO₂ NPs film (Cheng et al., 2021). The tensile strength and elongation at the break of the film were both enhanced by the addition of SiO₂ NPs. In addition, the CS-SiO₂ film presented a significant enhancement in the barrier characteristics against UV irradiation and water vapor permeability. Both CS-HE and CS-SiO₂-HE films demonstrated strong antibacterial and antioxidant properties; but, out of all the combinations, CS-SiO₂-HE had the best tensile strength, biological activity, lower moisture content, water solubility, and water vapor permeability (Cheng et al., 2021).
In summary, Table 2 describes a compilation of the wide diversity of agro-industrial waste as sources have been used for the synthesis of nanomaterials to establish hybrid coatings for food applications.
TABLE 2. Nanocomposites prepared from agro-industrial waste sources and their application in food preservation.
The use of nanoparticles derived from agro-industrial waste in the preparation of edible coatings results in several benefits including shelf-life extension and broad-spectrum antimicrobial properties, which have a positive effect in terms of food safety. Therefore, nanoparticles play a relevant role in preventing the growth and reproduction of microorganisms that are dangerous to the health of humans and animals. Different mechanisms have been attributed to the inhibition of microorganisms by nanoparticles; cell damage can be caused by the production of reactive oxygen species (ROS), which in turn inhibit DNA replication and ATP generation; metabolic pathways can be altered through the inactivation of key enzymes or inhibition of protein synthesis; or cell membranes and walls can be damaged (depolarization and destabilization) resulting in the growth inhibition of microorganism (Figure 3) (Foster, 2017; Reygaert and Reygaert, 2018; Presentato et al., 2020; Zhang et al., 2021; Ashfaq et al., 2022). Antimicrobial nanomaterials derived from agro-industrial waste are offering great opportunities for sustainable manufacturing in the food industry (Table 3).
FIGURE 3. Schematic illustration of the molecular mechanisms by which nanoparticles inhibit the growth of microorganisms. Created with BioRender.com and extracted under premium membership.
The main organic compounds from agro-industrial waste used for the development of nanoparticles are pectin and cellulose. Pectin is a heteropolysaccharide rich in hydroxyl and carboxyl groups in its backbone, which facilitates the interaction with different compounds; its physical properties are determined by the degree of methyl esterification, which results in different levels of gelation. The main disadvantage of pectin is its weak antimicrobial activity; therefore, there is insufficient information on the antimicrobial activity of pectin-based nanomaterials. Some reports have documented that pectin at the nanoscale possesses certain physical properties like chemical stability and structural flexibility, in addition to antimicrobial activity when they are combined with compounds such as metals, metal oxides, essential oils, or phenols; thus, pectin-based nanoparticles can protect food against fungi, Gram-positive bacteria, and Gram-negative bacteria (Ciriminna et al., 2020; Kumar et al., 2020; Huang et al., 2021). These compounds share a molecular mechanism for antimicrobial activity in which they damage the cell membrane of pathogenic microorganisms, altering its permeability and resulting in the loss of cell contents such as proteins, nucleic acids, sugars, and electrolytes (Chouhan et al., 2017; Bouarab Chibane et al., 2019; Stanić and Tanasković, 2020). As an example, Jiang et al. documented the use of pectin as a nanomaterial; nanoparticles derived from citrus pectin and zein exhibited antifungal activity against Alternaria alternata and Botrytis cinerea (Jiang et al., 2020). Similarly, nanoparticles of pectin (low methoxyl) and other polysaccharides such as debranched starch, and k-carrageenan, containing epigallocatechin-3-gallate (polyphenol) have been developed; nanoparticles showed excellent antimicrobial activity against E. coli and Staphylococcus aureus (Liu et al., 2019).
Cellulose is a homopolysaccharide with hydroxyl groups that allow the formation of covalent bonds with different compounds providing different physicochemical properties such as hydrophobicity, polarity, and selectivity to target compounds (Malis et al., 2019; Nemeş et al., 2022). Like pectin, cellulose does not present antimicrobial properties and must be conjugated with antimicrobial agents to display activity against pathogens (Malis et al., 2019). Some compounds usually combined with cellulose to provide antimicrobial activity are essential oils, metals, and peptides; they affect the cell membrane’s stability, which promotes the loss of intracellular material. For example, Ramesh et al., synthesized cellulose nanoparticles from potato peel, which were later incorporated into a polyvinyl alcohol film together with fennel seed oil; their development showed excellent antimicrobial activity against E. coli, Shigella flexneri, Staphylococcus aureus, and Salmonella typhi, demonstrating great potential for food packaging (Ramesh and Radhakrishnan, 2019). Similarly, Ogidi et al. proposed the use of cellulose and silver to obtain a nanomaterial with antimicrobial capacity against microorganisms isolated from fish and meat; thus, the shelf-life of food can be extended by their incorporation into packaging systems (Ogidi et al., 2023).
On the other hand, cellulose nanocrystals have shown antimicrobial activity by protecting different foods against pathogenic microorganisms. Although the precise molecular mechanism by which these nanostructures operate is currently unknown, it has been proposed that the rigid and narrow structure of such nanocrystals might break the cell membrane and release cellular contents (Noronha et al., 2021). In accordance with this, Sousa et al., developed cellulose nanocrystals from wood pulp, which were incorporated into a matrix of polyvinyl alcohol and natamycin–an antimicrobial peptide with antifungal activity–, to inhibit the growth of fungi and yeasts. Experiments were performed to analyze the preservation of cheese and the microorganisms inhibited include Alternaria alternata, Aspergillus niger, Rhizopus stolonifer, Fusarium semitectum, Saccharomyces cerevisiae and Kluyveromyces lactis (de Sousa et al., 2023).
Among the large variety of inorganic nanomaterials, metallic nanoparticles are the most widely used because of their antimicrobial activity. Their antimicrobial activity has been associated with some characteristics such as their morphology–shape, size, roughness, surface energy, electrical properties, and physicochemical properties. Silver, gold, copper oxide, zinc oxide, and titanium oxide are the most common metallic nanoparticles used for this purpose. Interestingly, metallic nanoparticles possess a great advantage associated with their action in preventing antimicrobial resistance since their mechanisms of antimicrobial activity are not specific (Sánchez-López et al., 2020).
The antimicrobial mechanism of action of metallic nanoparticles in bacteria consists of electrostatic interactions between the negatively charged bacteria cell wall and the positively charged surfaces of the nanoparticles, which result in membrane damage and increased cell permeability. Also, the metallic nanoparticles release ions that damage cell functions by interacting with the different cell structures of Gram-positive and Gram-negative bacteria such as DNA, proteins, organelles, and proteins of the respiratory chain (Slavin et al., 2017; Sánchez-López et al., 2020; Stanić and Tanasković, 2020). The antimicrobial molecular mechanisms in fungi are less clarified, but it has been stated that nanoparticles interact with the cell membrane and release metal ions, causing DNA, protein, and cell structure damage (organelle degeneration), affecting sporulation, spore germination, and fungal growth. However, metallic nanoparticles in fungi have shown lower toxicity effects than in bacteria (Brandelli et al., 2017; Correa et al., 2020).
Some research groups have prepared metallic nanoparticles from agro-industrial waste. For instance, Lomelí-Rosales et al. obtained gold and silver nanoparticles from Capsicum Chinese plant leaf extracts. Silver nanoparticles showed excellent antimicrobial activity against Gram-positive and Gram-negative bacteria including E. coli, S. marcescens, and E. faecalis; however, gold nanoparticles derived from agro-industrial waste did not exhibit significant antimicrobial activity (Lomelí-Rosales et al., 2022). Similarly, fruit peels like plum, kiwi, and peach have been used to obtain titanium dioxide (TiO2), showing good antimicrobial activity against E. coli, P. aerugenosa, and Bacillus subtilis (Ajmal et al., 2019). On the other hand, Dawwam et al. created bio-nanocomposites from palm pods that inhibit the growth of E. coli, Salmonella, Listeria monocytogenes, and Staphylococcus aureus; the bio-nanocomposite was prepared by obtaining cellulose nanocrystals, which were used for the synthesis of zinc oxide. These nanoparticles also affected the expression of genes related to virulence by inhibiting the synthesis of exotoxins and inhibiting microbial growth (Dawwam et al., 2022).
Silica is another inorganic material commonly exploited to produce nanoparticles because of its configuration, porosity, crystallinity, and highly reactive and hydrophilic surface, which allows compatibility with various compounds (Peerzada and Chidambaram, 2021). However, silica-based nanoparticles possess some disadvantages such as poor antimicrobial capacity. Some studies have reported that silica interacts with bacterial cell membrane proteins, altering their function and creating channels for the entry of antimicrobial agents, which are typically combined with silica nanoparticles (Tian and Liu, 2021). For example, Unglaube et al. developed carbon-based and silver-containing silica composites from rice husk waste: the nanomaterial showed outstanding activity against K. pneumoniae, A. baumannii, E. faecium, S. aureus, P. aeruginosa, E. coli, and the pathogenic yeast C. albicans (Unglaube et al., 2021). Similarly, Al-Asmar et al., employed mesoporous silica nanoparticles in pectin films to extend the shelf-life of strawberries and control the presence of microorganisms (Al-Asmar et al., 2020). These developments have demonstrated the evident potential of agro-industrial waste for the development of inorganic nanomaterials to form novel active coatings, in which different mechanisms can be promoted to inhibit the growth of pathogenic microorganisms.
Interestingly, agro-waste–in combination with nanotechnology–has demonstrated potential applications in the food industry. Recently, nanoparticles have been developed aimed to be incorporated into edible coatings to improve properties like appearance, smell, texture, and shelf-life by promoting antimicrobial activity against pathogens; thus, ensuring food safety at the same time (Ashfaq et al., 2022).
However, agro-industrial waste materials possess some drawbacks during their employment as precursors for nanomaterials. For instance, the great variability in their chemical composition caused by the vast diversity of agro-industrial wastes causes some issues in obtaining nanomaterials with homogeneous properties. In addition, the standardization of synthesis methods is difficult because of the heterogeneity of samples. Similarly, the synthesis methods and their parameters significantly affect the resulting properties of the nanomaterials. Therefore, it is important to select the appropriate nano-synthesis technique according to the agro-industrial waste and the desired properties of the nanomaterial, considering it, nanoparticles can be obtained in higher yields at lower production costs (Aswathi et al., 2022).
On the other hand, the use of nanoparticles in food products has raised toxicity and health risks concerns (Dimitrijevic et al., 2015). Nanoparticles, either organic or inorganic, possess different properties than their bulk form; thus, further toxicology investigations are required to analyze the toxic potential and evaluate the capacity of nanoparticles to migrate from food coatings or films into the human body (Ovais et al., 2018).
Nanoparticles made from organic, inorganic, and hybrid materials provide excellent protection to food against microorganisms, promoting an increase in their shelf life, but the great concern currently faced is the effect they may have on human health (Teleanu et al., 2018a; Ameta et al., 2020; Zhou and McClements, 2022). Due to their small size, they can easily move to other tissues and organs causing tissue inflammation and sometimes cell death. Thus, it is important to determine the optimal concentration, in which nanoparticles do not cause toxic effects and their bioavailability in vivo and in vitro (Sharaby et al., 2022). Since nanomaterials have unique properties that make them more active at a chemical level, unlike the bulk materials from which they are obtained (Teleanu et al., 2018a; Ameta et al., 2020; Zhou and McClements, 2022). Therefore, it is important to know that properties such as its size, morphology, composition, surface area, and solubility, as well as the concentration to which humans are exposed (Teleanu et al., 2018a; Ameta et al., 2020; Zhou and McClements, 2022). In order to determine the effects of nanoparticles in different organs, upon entering the body, the via of exposition needs to be considered; they can be ingestion, absorption through the skin, and inhalation are the mainly (Teleanu et al., 2018a; Ameta et al., 2020; Zhou and McClements, 2022).
Concerning ingestion, it is considered relevant for the food industry, since nanoparticles pass through different environments where pH, ionic composition, and enzymatic activity have variable parameters (Teleanu et al., 2018a; Ameta et al., 2020; Zhou and McClements, 2022). These diverse environments could change the structure of the nanoparticles, such as size, charge, and aggregation state (Teleanu et al., 2018a; Zhou and McClements, 2022). For example, regarding the structure, it has been reported that 40 nm diameter spherical ZnO nanoparticles are toxic compared to their counterparts nanorods, and it has been observed that the latter are even more cytotoxic (Medici et al., 2021).
The size of the nanoparticle is another factor that plays a key role, since if the size of the nanoparticle is very small, it can pass through the spaces between cells or bind to specific receptors (Ameta et al., 2020), which results in the nanoparticles passing from the gastrointestinal tract into the circulatory system reaching other organs and alter different physicochemical and biochemical processes since the nanoparticles dissolve in the tissue and affect the functioning of cells (Ameta et al., 2020; Medici et al., 2021). In this case, silver nanoparticles have been reported to accumulate in the brain, kidneys, liver, or testicles; despite that low concentrations of silver nanoparticles are typically used (Ahmad et al., 2021) (Figure 4).
FIGURE 4. Representative scheme of the human body exposition to nanoparticles respiratory tract, inhalation, ingestion, and skin absorption.
The charge of the nanoparticles can lead to more severe cytotoxic effects when they have a positive charge, concerning nanoparticles with a neutral or negative charge, by interacting with different compounds in cells, altering homeostatic processes (Ameta et al., 2020). It is also important to highlight that the charge of the nanoparticles promotes the aggregation of digestive enzymes, altering their activity due to electrostatic forces and the pH of the stomach (Ault et al., 2016) as in the case of pepsin, which interacts with silver nanoparticles surface (Stebounova et al., 2011). It has also been observed that organic nanoparticles are aggregated when they are found in environments with high salt concentrations due to electrostatic forces (Lozsan, 2012). These aggregates formed in the stomach can be degraded upon passage to the small intestine, due to the change in pH, allowing the absorption of different compounds (Zhou and McClements, 2022). Organic (pectin and cellulose) and inorganic nanoparticles have also been observed to alter lipid digestion (Espinal-Ruiz et al., 2014). In the case of organic nanoparticles, it promotes the flocculation of lipids. In inorganic nanoparticles, it is due to the absorption of lipids by the surface of the nanoparticles (Deloid et al., 2018).
Regarding the exposure of nanoparticles through inhalation, this is usually accidental, with the highest risk nanoparticles being those that have a size of 5 to 0.1 µm, which can accumulate in different regions of the respiratory tract, altering an exceptional response of the immune system, causing lung inflammation (Teleanu et al., 2018b; Ameta et al., 2020). A clear example is ZnO nanoparticles that at concentrations above 5 μg/mL in lung tissue have been observed to trigger cytokine IL-8 secretion bringing neutrophils, eosinophils, and T-lymphocytes, resulting in the development of an inflammatory response (Medici et al., 2021). In addition, nanoparticles smaller than 0.5 µm can reach blood capillaries, damaging other organs such as the brain and triggering diseases such as Alzheimer’s (Ameta et al., 2020).
On the other hand, the exposure to nanoparticles through the skin is due to their absorption by corneocytes, or by dermal structures, such as hair follicles or sweat glands, leading to the development of autoimmune diseases, urticaria, vasculitis, and dermatitis. Furthermore, studies indicate that ZnO nanoparticles lead to genotoxicity in human epidermal cells, although this toxicity varies with the diameter of the nanoparticle (Dimitrijevic et al., 2015).
Furthermore, most of the studies on the effects of nanoparticles on human health are carried out in animal models and these models do not always reflect real exposure dynamics which can also affect the accuracy of the results. Each nanoparticle must be assessed individually through necessary biological studies in a formal sequence in vitro and in vivo that allow the determination of positive and negative effects that they cause in the human body. (Medici et al., 2021). In addition, it is important to study their permanence in the human body and their impact on the microbiota, the epigenome (altering gene expression), and cellular components (lipid membranes or organelles).
Nanotechnology has positively impacted the food industry by developing nanostructures that are capable of protecting against microorganisms that compromise food safety (Ijaz et al., 2020). In addition to providing the food industry with novel technologies that increase the storage time of food and maintain its nutritional properties, by possessing antimicrobial activity against a broad spectrum of pathogenic microorganisms (Aswathi et al., 2022; Kumar et al., 2022). Nanosystems have developed to inhibit microbial growth and increase the shelf life of foods such as fruits, vegetables, dairy products, bread, and meats. Nanoparticles, regardless of their origin (organic, inorganic, or nanocomposites) can be embedded in coatings or films made from plastic matrices based on chitosan, pectin, and PVA, among others, adding several properties for food safety (Chawla et al., 2021). Other case reports, coatings that use emulsions made up of nanoparticles that absorb an oil-water interface (Pickering emulsion) to form a tight film that protects food against microbial action. Both strategies have a similar purpose to keep and extend the food shelf.
The consumption of fruits and vegetables is of vital importance for a balanced diet in humans, due to their high nutrient content such as carbohydrates, fiber, vitamins, and minerals. Fruits have an inner possible risk of pathogens infections triggered by their fresh, and high content of sugars and water. (Heaton and Jones, 2008; Balali et al., 2020). Developments in this field are used to protect fruits from microorganism’s actions. For example, Dey et al. (2021), developed a PVA matrix integrated with cellulose nanocrystals and chitosan nanoparticles to increase the shelf life of mangoes for up to 20 days, which allowed them to be free of postharvest fungi such as Colletotrichum gloeosporioides and Lasiodiplodia theobromae during storage. Furthermore, these coatings showed thermal stability at 450°C, and biodegradability of 88% in dry soil (Dey et al., 2021). Sunita et al. (2020) used silver oxide nanoparticles to cover fruits (apple, orange, watermelon, and banana) and vegetables (tomato, cucumber, brinjal, lady’s finger, and capsicum) stored at room temperature for 7 days. These nanoparticles showed antimicrobial activity (against Pseudomonas spp., Staphylococcus spp., and Enterobacteriaceae), protected from oxidation, and decreased oxygen spread (Sunita and Yegoti, 2020). For their part, Sharaby et al. (2022), used the Pickering emulsion system to stabilize cinnamon oil into pectin-zein nanoparticles. This nano system showed antimicrobial activity against Alternaria alternata and Botrytis cinerea, in apple pieces for 6 days (Jiang et al., 2020).
Cheese is a food that is consumed worldwide for having natural probiotics and its flavor. Natural issues with this food, it is an important source for the development of microorganisms because of its content in proteins and complex carbohydrates (Choi et al., 2016; Khezerlou et al., 2021). Among some examples of systems used to inhibit the growth of pathogens is the work carried out by de Sousa et al. (2023), in this investigation they manufactured nanocomposites made up of a mixture of cellulose/poly (vinyl alcohol) embedded with natamycin and cellulose nanocrystals, showing antimicrobial activity against S. cerevisiae for up to 12 days of storage (de Sousa et al., 2023). Other work developed pectin-based matrices accompanied by nanocellulose crystals and zinc oxide nanoparticles, which act as a barrier to water vapor, and inhibit the growth of Staphylococcus aureus and Salmonella enterica in contaminated cheese samples at 7 °C for 5 days (Sharaby et al., 2022).
Meat provides proteins, essential fatty acids, vitamins, and minerals, among other compounds (Leroy et al., 2023). Similar to other food groups, meat is also a target for the growth of microorganisms. Investigation into nanomaterials has gained relevance in recent years because of the market cost of meat products. A biodegradable nanosystem for meat protection was manufactured by Mathew et al. (2019), it was made of PVA-montmorillonite K10 clay with silver nanoparticles, to increase the shelf life of chicken meat products, demonstrating that these coatings are water resistant, protect against light and have antimicrobial activity against S. Typhimurium and S. aureus in contaminated samples, incubated at 4°C for 4 days. At the end of the experiments, they validated the shelf life of 110 days, when the coating was buried in the soil. (Mathew et al., 2019). Tapioca starch nanocomposite films have also been used, with cellulose nanocrystals and grape pomace extracts, presenting an increase in tensile strength and a decrease in water vapor permeability and elongation at break. Furthermore, these films exhibited antimicrobial activity against L. monocytogenes in chicken samples at 4°C for a storage time of 10 days (Xu et al., 2018). Meanwhile, Li et al. (2023) used a system based on Pickering emulsion made up of nanoparticles based on Zeina-pectin loaded with thymol, to inhibit the growth of Escherichia coli and Staphylococcus displaying much better results than the control by nearly 7 logs colony-forming unit/g at 36 h (Li et al., 2023).
Bread is a universal food, which can be found in various shapes and sizes, and provides proteins, antioxidants, and minerals (Carcea, 2020). For protection against microorganism growth, some nanosystems have been developed to protect bread from pathogens and increase shelf life. Gelatin-based biocomposite films were fabricated integrating cellulose microcrystals as well as clove (Syzygium aromaticum), nutmeg (Myristica fragrans), and black pepper (Piper nigrum) resins that were used to add the antimicrobial properties. The film developed using black pepper showed a good performance in the microbiology test against Staphylococcus aureus and Escherichia coli and physical and chemical properties and sensorial quality was maintained during 9 days in storage conditions (Figueroa-Lopez et al., 2018). Gelatin films have also integrated zinc oxide nanoparticles, positively impacting bread samples by reducing weight loss and decreasing bacterial growth compared to the control, for 9 days of storage (Yasmeen et al., 2023). Bakouei et al. (2023) used chitosan films with ZnO nanoparticles, decreasing water vapor permeability, and inhibiting the growth of mold and yeast at a temperature of 25°C for 60 days (Bakouei et al., 2023).
Despite the impact that nanotechnology has on the food industry, more research is still needed for the application of nanoparticles and to determine their effects on human health. Furthermore, measurements of indicators in the market because of their implementation can provide improvements for a long time and constitute a safe food supply chain.
The utilization of agro-industrial waste in the fabrication of functional nanoparticles is resulting in sustainable and innovative materials for improving quality and safety parameters in food preservation. After physicochemical pretreatments, the waste from vegetable and fruit peels, husks, shells, or seeds is transformed by top-down and bottom-up technologies into nanomaterials exhibiting enhanced properties against microbial contamination and advanced strategies for use in edible coatings and films. The development of organic and inorganic nanoparticles has shown environmental, and economic advantages and remarkable biological activities such as antioxidant and antimicrobial. Recently, hybrid nanocomposites contributed to and improved food preservation by providing thermostable materials and good performance in the water vapor permeability maintaining the quality of food. Different mechanisms have been attributed to the inhibition of microorganisms by nanoparticles, for example, production of reactive oxygen species (ROS), inhibition of DNA replication, and inactivation of metabolic pathways or cell membranes and walls damaged. Although there are extensive benefits of nanoparticles in food preservation, challenges in agro-waste valorization, methods of synthesis, yields, and costs are being addressed. However, the safety of nanoparticles has been questioned for human consumption derived from food processing by being incorporated into edible matrices. Further studies would determine the effects of nanoparticles for a long time in their final application which can be fruits, meat, and cheeses among others. Finally, is clear that the incorporation of nanoparticles in active coatings, and films using different matrices enhances the quality and increases the shelf-life of food groups either due to the properties of the nanomaterial itself or due to the encapsulation of active compounds.
EF-C: Conceptualization, Investigation, Writing–original draft. RG-G: Conceptualization, Investigation, Writing–original draft. JP: Investigation, Writing–review and editing. LP-A: Investigation, Writing–review and editing. AR-A: Investigation, Writing–review and editing. MI-M: Investigation, Writing–review and editing. GG-M: Investigation, Writing–review and editing. RA: Investigation, Writing–review and editing. DR-G: Investigation, Writing–review and editing. RP-S: Funding acquisition, Supervision, Writing–review and editing. EM-M: Funding acquisition, Supervision, Writing–review and editing.
The author(s) declare financial support was received for the research, authorship, and/or publication of this article. This work received the financial support of the project: Development of smart edible coating for the preservation of berries I025-IAMSM005-C3-T1-T, of the Challenge-Based Research Funding program 2022 of the Tecnologico de Monterrey.
This work was supported by Consejo Nacional de Humanidades Ciencias y tecnologías (CONAHCYT) and Tecnológico de Monterrey under Sistema Nacional de Investigadores (SNI) program to EF-C (CVU: 631205), RG-G (CVU: 661766), GG-M (CVU: 490688), RA (CVU: 714118), MI-M (CVU: 513991), EM-M (CVU: 230784), and RP-S (CVU: 35753). Figures and GA were created by Biorender through the premium membership.
The authors declare that the research was conducted in the absence of any commercial or financial relationships that could be construed as a potential conflict of interest.
All claims expressed in this article are solely those of the authors and do not necessarily represent those of their affiliated organizations, or those of the publisher, the editors and the reviewers. Any product that may be evaluated in this article, or claim that may be made by its manufacturer, is not guaranteed or endorsed by the publisher.
Adebayo, A. E., Oke, A. M., Lateef, A., Oyatokun, A. A., Abisoye, O. D., Adiji, I. P., et al. (2019). Biosynthesis of silver, gold and silver–gold alloy nanoparticles using Persea americana fruit peel aqueous extract for their biomedical properties. Nanotechnol. Environ. Eng. 4 (1), 13–15. doi:10.1007/s41204-019-0060-8
Ahmad, S. S., Yousuf, O., Islam, R. U., and Younis, K. (2021). Silver nanoparticles as an active packaging ingredient and its toxicity. Packag Technol. Sci. 34 (11–12), 653–663. doi:10.1002/pts.2603
Ajmal, N., Saraswat, K., Bakht, M. A., Riadi, Y., Ahsan, M. J., and Noushad, M. (2019). Cost-effective and eco-friendly synthesis of titanium dioxide (TiO2) nanoparticles using fruit’s peel agro-waste extracts: characterization, in vitro antibacterial, antioxidant activities. Green Chem. Lett. Rev. 12 (3), 244–254. doi:10.1080/17518253.2019.1629641
Akhayere, E., Kavaz, D., and Vaseashta, A. (2022). Efficacy studies of silica nanoparticles synthesized using agricultural waste for mitigating waterborne contaminants. Appl. Sci. 12 (18), 9279. doi:10.3390/app12189279
Al-Asmar, A., Giosafatto, C. V. L., Sabbah, M., Sanchez, A., Santana, R. V., and Mariniello, L. (2020). Effect of mesoporous silica nanoparticles on the physicochemical properties of pectin packaging material for strawberry wrapping. Nanomaterials 10 (1), 52. doi:10.3390/nano10010052
Alizadeh-Sani, M., Mohammadian, E., and McClements, D. J. (2020). Eco-friendly active packaging consisting of nanostructured biopolymer matrix reinforced with TiO2 and essential oil: application for preservation of refrigerated meat. Food Chem. 322, 126782. doi:10.1016/j.foodchem.2020.126782
Alomar, T. S., AlMasoud, N., Awad, M. A., El-Tohamy, M. F., and Soliman, D. A. (2020). An eco-friendly plant-mediated synthesis of silver nanoparticles: characterization, pharmaceutical and biomedical applications. Mater Chem. Phys. 249, 123007. doi:10.1016/j.matchemphys.2020.123007
Ameta, S. K., Rai, A. K., Hiran, D., Ameta, R., and Ameta, S. C. (2020). Use of nanomaterials in food science. Biog. Nano-Particles their Use Agro-ecosystems 457. doi:10.1007/978-981-15-2985-6_24
Anaya-Esparza, L. M., Mora, Z. V. de la, Ruvalcaba-Gómez, J. M., Romero-Toledo, R., Sandoval-Contreras, T., Aguilera-Aguirre, S., et al. (2020). Use of titanium dioxide (TiO2) nanoparticles as reinforcement agent of polysaccharide-based materials. Process 8(11):1395. doi:10.3390/pr8111395
Arias, D., Rodríguez, J., López, B., and Méndez, P. (2021). Evaluation of the physicochemical properties of pectin extracted from Musa paradisiaca banana peels at different pH conditions in the formation of nanoparticles. Heliyon 7 (1), e06059. doi:10.1016/j.heliyon.2021.e06059
Ashfaq, A., Khursheed, N., Fatima, S., Anjum, Z., and Younis, K. (2022). Application of nanotechnology in food packaging: pros and Cons. J. Agric. Food Res. 7, 100270. doi:10.1016/j.jafr.2022.100270
Aswathi, V. P., Meera, S., Maria, C. G. A., and Nidhin, M. (2022). Green synthesis of nanoparticles from biodegradable waste extracts and their applications: a critical review. Nanotechnol. Environ. Eng. 1, 377–397. doi:10.1007/s41204-022-00276-8
Ault, A. P., Stark, D. I., Axson, J. L., Keeney, J. N., Maynard, A. D., Bergin, I. L., et al. (2016). Protein corona-induced modification of silver nanoparticle aggregation in simulated gastric fluid. Environ. Sci. Nano 3 (6), 1510–1520. doi:10.1039/c6en00278a
Babu, R. H., Yugandhar, P., and Savithramma, N. (2018). Synthesis, characterization and antimicrobial studies of bio silica nanoparticles prepared from Cynodon dactylon L.: a green approach. Bull. Mater Sci. 41 (3), 65–68. doi:10.1007/s12034-018-1584-4
Bajaj, N. S., and Joshi, R. A. (2021). Energy materials: synthesis and characterization techniques. Energy Mater Fundam Appl, 61–82. doi:10.1016/B978-0-12-823710-6.00019-4
Bakouei, M., Sadeghizadeh Yazdi, J., Ehrampoush, M. H., Salari, M., and Madadizadeh, F. (2023). The effect of active chitosan films containing bacterial cellulose nanofiber and ZnO nanoparticles on the shelf life of loaf bread. J. Food Qual. 2023. doi:10.1155/2023/7470296
Bala, S., Garg, D., Sridhar, K., Inbaraj, B. S., Singh, R., Kamma, S., et al. (2023). Transformation of agro-waste into value-added bioproducts and bioactive compounds: micro/nano formulations and application in the agri-food-pharma sector. Bioengineering 10 (2), 152. doi:10.3390/bioengineering10020152
Balali, G. I., Yar, D. D., Afua Dela, V. G., and Adjei-Kusi, P. (2020). Microbial contamination, an increasing threat to the consumption of fresh fruits and vegetables in today’s world. Int. J. Microbiol. 2020, 1–13. doi:10.1155/2020/3029295
Balzer, C., Armstrong, M., Shan, B., Huang, Y., Liu, J., and Mu, B. (2018). Modeling nanoparticle dispersion in electrospun nanofibers. Langmuir 34 (4), 1340–1346. doi:10.1021/acs.langmuir.7b03726
Barragán-Ocaña, A., Merritt, H., Sánchez-Estrada, O. E., Méndez-Becerril, J. L., and del Pilar Longar-Blanco, M. (2023). Biorefinery and sustainability for the production of biofuels and value-added products: a trends analysis based on network and patent analysis. PLoS One 18 (1), e0279659. doi:10.1371/journal.pone.0279659
Bikiaris, D. N. (2022). Nanocomposites with different types of nanofillers and advanced properties for several applications. Appl. Nano 3 (3), 160–162. doi:10.3390/applnano3030012
Boro, U., and Moholkar, V. S. (2022). Antimicrobial bionanocomposites of poly(lactic acid)/ZnO deposited halloysite nanotubes for potential food packaging applications. Mater Today Commun. 33, 104787. doi:10.1016/j.mtcomm.2022.104787
Bouarab Chibane, L., Degraeve, P., Ferhout, H., Bouajila, J., and Oulahal, N. (2019). Plant antimicrobial polyphenols as potential natural food preservatives. J. Sci. Food Agric. 99 (4), 1457–1474. doi:10.1002/jsfa.9357
Brandelli, A., Ritter, A. C., and Veras, F. F. (2017). Antimicrobial activities of metal nanoparticles. Met. Nanoparticles Pharma, 337–363. doi:10.1007/978-3-319-63790-7_15
Bruna, T., Maldonado-Bravo, F., Jara, P., and Caro, N. (2021). Silver nanoparticles and their antibacterial applications. Int. J. Mol. Sci. 22 (13), 7202. doi:10.3390/ijms22137202
Carcea, M. (2020). Nutritional value of grain-based foods. Foods 9 (4), 504. doi:10.3390/foods9040504
Cárdenas-Alcaide, M. F., González-González, R. B., Villalba-Rodríguez, A. M., López-Pacheco, I. Y., Parra-Saldívar, R., and Iqbal, H. M. N. (2023). Nanofabrication and characterization of green-emitting N-doped carbon dots derived from pulp-free lemon juice extract. Nanofabrication 8. doi:10.37819/nanofab.008.299
Chaudhuri, S., Ghosh, A., and Chattopadhyay, S. K. (2021). Green synthetic approaches for medium ring–sized heterocycles of biological and pharmaceutical interest. Green Synth. Approaches Biol. Relev. Heterocycles Vol. 2 Green Catal. Syst. Solvents, 617–653. doi:10.1016/B978-0-12-820792-5.00004-4
Chawla, R., Sivakumar, S., and Kaur, H. (2021). Antimicrobial edible films in food packaging: current scenario and recent nanotechnological advancements-a review. Carbohydr. Polym. Technol. Appl. 2, 100024. doi:10.1016/j.carpta.2020.100024
Cheng, J., Tian, B., Wang, J., Wang, Z., and Liu, Y. (2021). Development of multifunctional films based on chitosan, nano silica and hops extracts. Eur. Polym. J. 161, 110816. doi:10.1016/j.eurpolymj.2021.110816
Choi, K. H., Lee, H., Lee, S., Kim, S., and Yoon, Y. (2016). Cheese microbial risk assessments — a review. Asian-Australasian J. Anim. Sci. 29 (3), 307–314. doi:10.5713/ajas.15.0332
Chouhan, S., Sharma, K., and Guleria, S. (2017). Antimicrobial activity of some essential oils—present status and future perspectives. Medicines 4 (3), 58. doi:10.3390/medicines4030058
Ciriminna, R., Fidalgo, A., Meneguzzo, F., Presentato, A., Scurria, A., Nuzzo, D., et al. (2020). Pectin: a long-neglected broad-spectrum antibacterial. ChemMedChem 15 (23), 2228–2235. doi:10.1002/cmdc.202000518
Coronado-Apodaca, K. G., Rodríguez-De Luna, S. E., Araújo, R. G., Oyervides-Muñoz, M. A., González-Meza, G. M., Parra-Arroyo, L., et al. (2023). Occurrence, transport, and detection techniques of emerging pollutants in groundwater. MethodsX 10, 102160. doi:10.1016/j.mex.2023.102160
Correa, M. G., Martínez, F. B., Vidal, C. P., Streitt, C., Escrig, J., and de Dicastillo, C. L. (2020). Antimicrobial metal-based nanoparticles: a review on their synthesis, types and antimicrobial action. Beilstein J. Nanotechnol. 11 (1), 1450–1469. doi:10.3762/bjnano.11.129
D’Arienzo, M., Scotti, R., Di Credico, B., and Redaelli, M. (2017). Synthesis and characterization of morphology-controlled TiO2 nanocrystals: opportunities and challenges for their application in photocatalytic materials. Stud. Surf. Sci. Catal. 177, 477–540. doi:10.1016/B978-0-12-805090-3.00013-9
Dawwam, G. E., Al-Shemy, M. T., and El-Demerdash, A. S. (2022). Green synthesis of cellulose nanocrystal/ZnO bio-nanocomposites exerting antibacterial activity and downregulating virulence toxigenic genes of food-poisoning bacteria. Sci. Rep. 12 (1), 16848. doi:10.1038/s41598-022-21087-6
Deepa, B., Abraham, E., Cordeiro, N., Mozetic, M., Mathew, A. P., Oksman, K., et al. (2015). Utilization of various lignocellulosic biomass for the production of nanocellulose: a comparative study. Cellulose 22 (2), 1075–1090. doi:10.1007/s10570-015-0554-x
Deloid, G. M., Sohal, I. S., Lorente, L. R., Molina, R. M., Pyrgiotakis, G., Stevanovic, A., et al. (2018). Reducing intestinal digestion and absorption of fat using a nature-derived biopolymer: interference of triglyceride hydrolysis by nanocellulose. ACS Nano 12 (7), 6469–6479. doi:10.1021/acsnano.8b03074
de Sousa, M. M., Clemente, V. M. C., Iordanskii, A., Maria De Sousa, M., Miria, V., Clemente, C., et al. (2023). Development and characterization of sustainable antimicrobial films incorporated with natamycin and cellulose nanocrystals for cheese preservation. Polysaccharides 4 (1), 53–64. doi:10.3390/polysaccharides4010004
Dey, D., Dharini, V., Selvam, S. P., Sadiku, E. R., Kumar, M. M., Jayaramudu, J., et al. (2021). Physical, antifungal, and biodegradable properties of cellulose nanocrystals and chitosan nanoparticles for food packaging application. Mater Today Proc. 38, 860–869. doi:10.1016/j.matpr.2020.04.885
Dimitrijevic, M., Karabasil, N., Boskovic, M., Teodorovic, V., Vasilev, D., Djordjevic, V., et al. (2015). Safety aspects of nanotechnology applications in food packaging. Procedia Food Sci. 5, 57–60. doi:10.1016/j.profoo.2015.09.015
Draeger, C. L., Akutsu, R. de C. C. de A., Araújo, W. M. C., da Silva, I. C. R., Botelho, R. B. A., and Zandonadi, R. P. (2018). Epidemiological surveillance system on foodborne diseases in Brazil after 10-years of its implementation: completeness evaluation. Int. J. Environ. Res. Public Health 15 (10), 2284. doi:10.3390/ijerph15102284
Duan, Y., Tarafdar, A., Kumar, V., Ganeshan, P., Rajendran, K., Shekhar Giri, B., et al. (2022). Sustainable biorefinery approaches towards circular economy for conversion of biowaste to value added materials and future perspectives. Fuel 325, 124846. doi:10.1016/j.fuel.2022.124846
Duque-Acevedo, M., Belmonte-Ureña, L. J., Cortés-García, F. J., and Camacho-Ferre, F. (2020). Agricultural waste: review of the evolution, approaches and perspectives on alternative uses. Glob. Ecol. Conserv. 22, e00902. doi:10.1016/j.gecco.2020.e00902
Dutta, D. P. (2021). Microwave-assisted synthesis of inorganic nanomaterials. Handb. Synthesis Strategies Adv. Mater., 79–107. Available at: https://link.springer.com/chapter/10.1007/978-981-16-1807-9_3. doi:10.1016/B978-0-12-821887-7.00011-2
El-Ramady, H., Brevik, E. C., Bayoumi, Y., Shalaby, T. A., El-Mahrouk, M. E., Taha, N., et al. (2022). An overview of agro-waste management in light of the water-energy-waste nexus. Sustain 14 (23), 15717. doi:10.3390/su142315717
Espinal-Ruiz, M., Parada-Alfonso, F., Restrepo-Sánchez, L. P., Narváez-Cuenca, C. E., and McClements, D. J. (2014). Impact of dietary fibers [methyl cellulose, chitosan, and pectin] on digestion of lipids under simulated gastrointestinal conditions. Food Funct. 5 (12), 3083–3095. doi:10.1039/c4fo00615a
Fao (2019). MOVING FORWARD ON FOOD LOSS AND WASTE REDUCTION FOOD AND AGRICULTURE. Available at www.fao.org/publications.
Ferdous, Z., and Nemmar, A. (2020). Health impact of silver nanoparticles: a review of the biodistribution and toxicity following various routes of exposure. Int. J. Mol. Sci. 21 (7), 2375. doi:10.3390/ijms21072375
Figueroa-Lopez, K. J., Andrade-Mahecha, M. M., and Torres-Vargas, O. L. (2018). Development of antimicrobial biocomposite films to preserve the quality of bread. Mol 23 (1), 212. doi:10.3390/molecules23010212
Foster, T. J. (2017). Antibiotic resistance in Staphylococcus aureus. Current status and future prospects. FEMS Microbiol. Rev. 41 (3), 430–449. doi:10.1093/femsre/fux007
González-González, R. B., González, L. T., Iglesias-González, S., González-González, E., Martinez-Chapa, S. O., Madou, M., et al. (2020). Characterization of chemically activated pyrolytic carbon black derived from waste tires as a candidate for nanomaterial precursor. Nanomater 10 (11), 2213. doi:10.3390/nano10112213
González-González, R. B., González, L. T., Madou, M., Leyva-Porras, C., Martinez-Chapa, S. O., and Synthesis, M. A. (2022b). Synthesis, purification, and characterization of carbon dots from non-activated and activated pyrolytic carbon black. Nanomater 12 (3), 298. doi:10.3390/nano12030298
González-González, R. B., Morales-Murillo, M. B., Martínez-Prado, M. A., Melchor-Martínez, E. M., Ahmed, I., Bilal, M., et al. (2022a). Carbon dots-based nanomaterials for fluorescent sensing of toxic elements in environmental samples: strategies for enhanced performance. Chemosphere 300, 134515. doi:10.1016/j.chemosphere.2022.134515
Gumber, S., Kanwar, S., and Mazumder, K. (2023). Properties and antimicrobial activity of wheat-straw nanocellulose-arabinoxylan acetate composite films incorporated with silver nanoparticles. Int. J. Biol. Macromol. 246, 125480. doi:10.1016/j.ijbiomac.2023.125480
Ha, J., Park, H., Kim, M., Kim, Y.-T., and Choi, J. (2022). Liquefied-natural-gas-derived vertical carbon layer deposited on SiO as cost-effective anode for Li-ion batteries. J. Electrochem Soc. 169 (2), 020528. doi:10.1149/1945-7111/ac4bf1
Hammami, I., Alabdallah, N. M., jomaa, A. A., and Kamoun, M. (2021). Gold nanoparticles: synthesis properties and applications. J. King Saud. Univ. - Sci. 33 (7), 101560. doi:10.1016/j.jksus.2021.101560
Heaton, J. C., and Jones, K. (2008). Microbial contamination of fruit and vegetables and the behaviour of enteropathogens in the phyllosphere: a review. J. Appl. Microbiol. 104 (3), 613–626. doi:10.1111/j.1365-2672.2007.03587.x
Hu, W., Zou, Z., Li, H., Zhang, Z., Yu, J., and Tang, Q. (2022). Fabrication of highly transparent and multifunctional polyvinyl alcohol/starch based nanocomposite films using zinc oxide nanoparticles as compatibilizers. Int. J. Biol. Macromol. 204, 284–292. doi:10.1016/j.ijbiomac.2022.02.020
Huang, J., Hu, Z., Hu, L., Li, G., Yao, Q., and Hu, Y. (2021). Pectin-based active packaging: a critical review on preparation, physical properties and novel application in food preservation. Trends Food Sci. Technol. 118, 167–178. doi:10.1016/j.tifs.2021.09.026
Ijaz, M., Zafar, M., Afsheen, S., and Iqbal, T. (2020). A review on Ag-nanostructures for enhancement in shelf time of fruits. J. Inorg. Organomet. Polym. Mater 30 (5), 1475–1482. doi:10.1007/s10904-020-01504-x
Jadhav, R., Pawar, P., Choudhari, V., Topare, N., Raut-Jadhav, S., Bokil, S., et al. (2023). An overview of antimicrobial nanoparticles for food preservation. Mater Today Proc. 72, 204–216. doi:10.1016/j.matpr.2022.07.045
Jiang, Y., Wang, D., Li, F., Li, D., and Huang, Q. (2020). Cinnamon essential oil Pickering emulsion stabilized by zein-pectin composite nanoparticles: characterization, antimicrobial effect and advantages in storage application. Int. J. Biol. Macromol. 148, 1280–1289. doi:10.1016/j.ijbiomac.2019.10.103
Judith, J. V., and Vasudevan, N. (2022). Synthesis of nanomaterial from industrial waste and its application in environmental pollutant remediation. Environ. Eng. Res. 27 (2), 200672–200680. doi:10.4491/eer.2020.672
Kamala, K., and Kumar, V. P. (2018). Food products and food contamination. Microb. Contam. Food Degrad., 1–19. doi:10.1016/B978-0-12-811515-2.00001-9
Khezerlou, A., Tavassoli, M., Sani, M. A., Mohammadi, K., Ehsani, A., and McClements, D. J. (2021). Application of nanotechnology to improve the performance of biodegradable biopolymer-based packaging materials. Polym 13 (24), 4399. doi:10.3390/polym13244399
Kritchenkov, A. S., Egorov, A. R., Volkova, O. V., Artemjev, A. A., Kurliuk, A. V., Anh Le, T., et al. (2021). Novel biopolymer-based nanocomposite food coatings that exhibit active and smart properties due to a single type of nanoparticles. Food Chem. 343, 128676. doi:10.1016/j.foodchem.2020.128676
Kritchenkov, A. S., Egorov, A. R., Yagafarov, N. Z., Volkova, O. V., Zabodalova, L. A., Suchkova, E. P., et al. (2020). Efficient reinforcement of chitosan-based coatings for Ricotta cheese with non-toxic, active, and smart nanoparticles. Prog. Org. Coatings 145, 105707. doi:10.1016/j.porgcoat.2020.105707
Kugarajah, V., Dharmalingam, S., Hadem, H., Ojha, A. K., Ranjan, S., Dasgupta, N., et al. (2022). Fabrication of nanomaterials. Food, medical. Environ. Appl. Nanomater, 1–39. doi:10.1016/B978-0-12-822858-6.00001-7
Kumar, A., Kumar, A., Vats, C., Sangwan, P., Kumar, V., Abhineet, S., et al. (2022). Recent insights into metallic nanoparticles in shelf-life extension of agrifoods: properties, green synthesis, and major applications. Front. Sustain Food Syst. 6, 1025342. doi:10.3389/fsufs.2022.1025342
Kumar, M., Tomar, M., Saurabh, V., Mahajan, T., Punia, S., Contreras, M. del M., et al. (2020). Emerging trends in pectin extraction and its anti-microbial functionalization using natural bioactives for application in food packaging. Trends Food Sci. Technol. 105, 223–237. doi:10.1016/j.tifs.2020.09.009
Kumar, S., Basumatary, I. B., Sudhani, H. P. K., Bajpai, V. K., Chen, L., Shukla, S., et al. (2021). Plant extract mediated silver nanoparticles and their applications as antimicrobials and in sustainable food packaging: a state-of-the-art review. Trends Food Sci. Technol. 112, 651–666. doi:10.1016/j.tifs.2021.04.031
Kumar, V., Singh, S., Srivastava, B., Bhadouria, R., and Singh, R. (2019). Green synthesis of silver nanoparticles using leaf extract of Holoptelea integrifolia and preliminary investigation of its antioxidant, anti-inflammatory, antidiabetic and antibacterial activities. J. Environ. Chem. Eng. 7 (3), 103094. doi:10.1016/j.jece.2019.103094
Kumar Trivedi, A., Kumar, A., and Gupta, M. K. (2023). Extraction of nanocellulose from wheat straw and its characterization. Mater Today Proc. 78, 48–54. doi:10.1016/j.matpr.2022.11.038
Leroy, F., Smith, N. W., Adesogan, A. T., Beal, T., Iannotti, L., Moughan, P. J., et al. (2023). The role of meat in the human diet: evolutionary aspects and nutritional value. Anim. Front. Rev. Mag. Anim. Agric. 13 (2), 11–18. doi:10.1093/af/vfac093
Li, W., Li, W., Wan, Y., Zhou, T., and Wang, L. (2023). Thymol-loaded Zein-pectin composite nanoparticles as stabilizer to fabricate Pickering emulsion of star anise essential oil for improved stability and antimicrobial activity. J. Food Sci. 88 (9), 3807–3819. doi:10.1111/1750-3841.16700
Lima, A. R., Cristofoli, N. L., Rosa da Costa, A. M., Saraiva, J. A., and Vieira, M. C. (2023). Comparative study of the production of cellulose nanofibers from agro-industrial waste streams of Salicornia ramosissima by acid and enzymatic treatment. Food Bioprod. Process 137, 214–225. doi:10.1016/j.fbp.2022.11.012
Lin, D., Zheng, Y., Huang, Y., Ni, L., Zhao, J., Huang, C., et al. (2020). Investigation of the structural, physical properties, antioxidant, and antimicrobial activity of chitosan-nano-silicon aerogel composite edible films incorporated with okara powder. Carbohydr. Polym. 250, 116842. doi:10.1016/j.carbpol.2020.116842
Liu, J., Ma, Z., Liu, Y., Zheng, X., Pei, Y., and Tang, K. (2022). Soluble soybean polysaccharide films containing in-situ generated silver nanoparticles for antibacterial food packaging applications. Food Packag Shelf Life 31, 100800. doi:10.1016/j.fpsl.2021.100800
Liu, Q., Li, M., Xiong, L., Qiu, L., Bian, X., Sun, C., et al. (2019). Characterization of cationic modified debranched starch and formation of complex nanoparticles with κ-carrageenan and low methoxyl pectin. J. Agric. Food Chem. 67 (10), 2906–2915. doi:10.1021/acs.jafc.8b05045
Liu, Z., Du, M., Liu, H., Zhang, K., Xu, X., Liu, K., et al. (2021). Chitosan films incorporating litchi peel extract and titanium dioxide nanoparticles and their application as coatings on watercored apples. Prog. Org. Coatings 151, 106103. doi:10.1016/j.porgcoat.2020.106103
Lomelí-Rosales, D. A., Zamudio-Ojeda, A., Reyes-Maldonado, O. K., López-Reyes, M. E., Basulto-Padilla, G. C., Lopez-Naranjo, E. J., et al. (2022). Green synthesis of gold and silver nanoparticles using leaf extract of capsicum chinense plant. Molecules 27 (5), 1692. doi:10.3390/molecules27051692
Lopes, F. C., and Ligabue-Braun, R. (2021). Agro-industrial residues: eco-friendly and inexpensive substrates for microbial pigments production. Front. Sustain Food Syst. 5, 65. doi:10.3389/fsufs.2021.589414
Lozsan, A. (2012). Salt-induced fast aggregation of nano-emulsions: structural and kinetic scaling. Colloid Polym. Sci. 290 (15), 1561–1566. doi:10.1007/s00396-012-2680-4
Malis, D., Jeršek, B., Tomšič, B., Štular, D., Golja, B., Kapun, G., et al. (2019). Antibacterial activity and biodegradation of cellulose fiber blends with incorporated ZnO. Mater. (Basel) 12 (20), 3399. doi:10.3390/ma12203399
Mateo, S., Peinado, S., Morillas-Gutiérrez, F., La Rubia, M. D., and Moya, A. J. (2021). Nanocellulose from agricultural wastes: products and applications—a review. Process 9 (9), 1594. doi:10.3390/pr9091594
Mathew, S., Mathew, J., and Radhakrishnan, E. K. (2019). Biodegradable and active nanocomposite pouches reinforced with silver nanoparticles for improved packaging of chicken sausages. Food Packag Shelf Life 19, 155–166. doi:10.1016/j.fpsl.2018.12.009
Medici, S., Peana, M., Pelucelli, A., and Zoroddu, M. A. (2021). An updated overview on metal nanoparticles toxicity. Semin. Cancer Biol. 76, 17–26. doi:10.1016/j.semcancer.2021.06.020
Mehmood, S., Kausar Janjua, N., Tabassum, S., Faizi, S., and Fenniri, H. (2022). Cost effective synthesis approach for green food packaging coating by gallic acid conjugated gold nanoparticles from Caesalpinia pulcherrima extract. Results Chem. 4, 100437. doi:10.1016/j.rechem.2022.100437
Mourdikoudis, S., Pallares, R. M., and Thanh, N. T. K. (2018). Characterization techniques for nanoparticles: comparison and complementarity upon studying nanoparticle properties. Nanoscale 10 (27), 12871–12934. doi:10.1039/c8nr02278j
Nakashima, K., Yamada, L., Satou, Y., Azuma, J. I., and Satoh, N. (2004). The evolutionary origin of animal cellulose synthase. Dev. Genes Evol. 214 (2), 81–88. doi:10.1007/s00427-003-0379-8
Nemeş, N. S., Ardean, C., Davidescu, C. M., Negrea, A., Ciopec, M., Duţeanu, N., et al. (2022). Antimicrobial activity of cellulose based materials. Polym 14 (4), 735. doi:10.3390/polym14040735
Nguyen, S. V., and Lee, B. K. (2022). PVA/CNC/TiO2 nanocomposite for food-packaging: improved mechanical, UV/water vapor barrier, and antimicrobial properties. Carbohydr. Polym. 298, 120064. doi:10.1016/j.carbpol.2022.120064
Noronha, V. T., Camargos, C. H. M., Jackson, J. C., Souza Filho, A. G., Paula, A. J., Rezende, C. A., et al. (2021). Physical membrane-stress-mediated antimicrobial properties of cellulose nanocrystals. ACS Sustain Chem. Eng. 9 (8), 3203–3212. doi:10.1021/acssuschemeng.0c08317
Nuilek, K., Simon, A., and Baumli, P. (2018). Influence of KOH on the carbon nanostructure of peanut shell. Resolut. Discov. 3 (2), 29–32. doi:10.1556/2051.2018.00060
Ogidi, C. O., Emmanuel, O. P., Daramola, O. O., Bamigboye, O., and Malomo, O. (2023). Synthesis of silver nanoparticles using cellulose and starch extracted from brewer spent grain: assessment of their antimicrobial and preservatives activities. Turk. J. Agric. - Food Sci. Technol. 11 (2), 227–238. doi:10.24925/turjaf.v11i2.227-238.5253
Ortega, F., Versino, F., López, O. V., and García, M. A. (2021). Biobased composites from agro-industrial wastes and by-products. Emergent Mater 5 (3), 873–921. doi:10.1007/s42247-021-00319-x
Ovais, S. Q., Kaiser, Y., Gaurav, S., and Abhaya, K. S. (2018). Nanotechnology in packaging of fresh fruits and vegetables. Emerg. Postharvest Treat. Fruits Veg., 147–166.
Peerzada, J. G., and Chidambaram, R. (2021). A statistical approach for biogenic synthesis of nano-silica from different agro-wastes. Silicon 13 (7), 2089–2101. doi:10.1007/s12633-020-00629-5
Periakaruppan, R., Manju Praveena, S., Priya, C., Ranjitha, P., Gokul Raj, S., and Danaraj, J. (2022). Biosynthesis of silica nanoparticles using the leaf extract of Punica granatum and assessment of its antibacterial activities against human pathogens. Appl. Biochem. Biotechnol. 194 (11), 5594–5605. doi:10.1007/s12010-022-03994-6
Presentato, A., Scurria, A., Albanese, L., Lino, C., Sciortino, M., Pagliaro, M., et al. (2020). Superior antibacterial activity of integral lemon pectin extracted via hydrodynamic cavitation. ChemistryOpen 9 (5), 628–630. doi:10.1002/open.202000076
Radacsi, N., Campos, F. D., Chisholm, C. R. I., and Giapis, K. P. (2018). Spontaneous formation of nanoparticles on electrospun nanofibres. Nat. Commun. 9(1):4740–4748. doi:10.1038/s41467-018-07243-5
Ramesh, S., and Radhakrishnan, P. (2019). Cellulose nanoparticles from agro-industrial waste for the development of active packaging. Appl. Surf. Sci. 484, 1274–1281. doi:10.1016/j.apsusc.2019.04.003
Ramos, A. P. (2017). Dynamic light scattering applied to nanoparticle characterization. Nanocharacterization Tech., 99–110. doi:10.1016/B978-0-323-49778-7.00004-7
Ravindran, R., Hassan, S. S., Williams, G. A., and Jaiswal, A. K. (2018). A review on bioconversion of agro-industrial wastes to industrially important enzymes. Bioengineering 5 (4), 93. doi:10.3390/bioengineering5040093
Reygaert, W. C., and Reygaert, W. C. (2018). An overview of the antimicrobial resistance mechanisms of bacteria. AIMS Microbiol. 4(3):482–501. doi:10.3934/microbiol.2018.3.482
Roy, P., Bhat, V. S., Saha, S., Sengupta, D., Das, S., Datta, S., et al. (2021). Mesoporous carbon nanospheres derived from agro-waste as novel antimicrobial agents against gram-negative bacteria. Environ. Sci. Pollut. Res. Int. 28 (11), 13552–13561. doi:10.1007/s11356-020-11587-1
Sadh, P. K., Duhan, S., and Duhan, J. S. (2018). Agro-industrial wastes and their utilization using solid state fermentation: a review. Bioresour. Bioprocess 5 (1), 1–15. doi:10.1186/s40643-017-0187-z
Salama, H. E., and Abdel Aziz, M. S. (2020a). Optimized carboxymethyl cellulose and guanidinylated chitosan enriched with titanium oxide nanoparticles of improved UV-barrier properties for the active packaging of green bell pepper. Int. J. Biol. Macromol. 165 (Pt A), 1187–1197. doi:10.1016/j.ijbiomac.2020.09.254
Salama, H. E., and Abdel Aziz, M. S. (2020b). Optimized alginate and Aloe vera gel edible coating reinforced with nTiO2 for the shelf-life extension of tomatoes. Int. J. Biol. Macromol. 165, 2693–2701. doi:10.1016/j.ijbiomac.2020.10.108
Sánchez-López, E., Gomes, D., Esteruelas, G., Bonilla, L., Lopez-Machado, A. L., Galindo, R., et al. (2020). Metal-based nanoparticles as antimicrobial agents: an overview. Nanomaterials 10 (2), 292. doi:10.3390/nano10020292
Sen, M., and Sen, M. (2020). Nanocomposite materials. Nanotechnol. Environ. doi:10.5772/intechopen.93047
Shahi, N., Wang, P., Adhikari, S., Min, B., and Rangari, V. K. (2021). Biopolymers fractionation and synthesis of nanocellulose/silica nanoparticles from agricultural byproducts. ACS Sustain Chem. Eng. 9 (18), 6284–6295. doi:10.1021/acssuschemeng.0c09342
Sharaby, M. R., Soliman, E. A., Abdel-Rahman, A. B., Osman, A., and Khalil, R. (2022). Novel pectin-based nanocomposite film for active food packaging applications. Sci. Rep. 12 (1), 20673–20714. doi:10.1038/s41598-022-25192-4
Sharifi, K. A., and Pirsa, S. (2021). Biodegradable film of black mulberry pulp pectin/chlorophyll of black mulberry leaf encapsulated with carboxymethylcellulose/silica nanoparticles: investigation of physicochemical and antimicrobial properties. Mater Chem. Phys. 267, 124580. doi:10.1016/j.matchemphys.2021.124580
Sharma, G. K., James, N. R., Sharma, G. K., and James, N. R. (2022). Electrospinning: the technique and applications. Recent Dev. Nanofibers Res. Work. Title. doi:10.5772/intechopen.105804
Shojaei, T. R., Soltani, S., and Derakhshani, M. (2022). Synthesis, properties, and biomedical applications of inorganic bionanomaterials. Fundam. Bionanomaterials, 139–174. doi:10.1016/B978-0-12-824147-9.00006-6
Singh, R., Das, R., Sangwan, S., Rohatgi, B., Khanam, R., Peera, SKPG, et al. (2021). Utilisation of agro-industrial waste for sustainable green production: a review. Environ. Sustain 4 (4), 619–636. doi:10.1007/s42398-021-00200-x
Slavin, Y. N., Asnis, J., Häfeli, U. O., and Bach, H. (2017). Metal nanoparticles: understanding the mechanisms behind antibacterial activity. J. Nanobiotechnology 15 (1), 65–20. doi:10.1186/s12951-017-0308-z
Snehal, S., Sc, M. A., Lohani, P., Correspondence, S., Snehal, M., and Sc, A. (2018). Silica nanoparticles: its green synthesis and importance in agriculture. ∼ 3383 ∼ J. Pharmacogn. Phytochem. 7(5):3383–3393.
Stanić, V., and Tanasković, S. B. (2020). Antibacterial activity of metal oxide nanoparticles. Nanotoxicity Prev. Antibact. Appl. Nanomater, 241–274. doi:10.1016/B978-0-12-819943-5.00011-7
Stebounova, L. V., Guio, E., and Grassian, V. H. (2011). Silver nanoparticles in simulated biological media: a study of aggregation, sedimentation, and dissolution. J. Nanoparticle Res. 13 (1), 233–244. doi:10.1007/s11051-010-0022-3
Subhan, M. A., Neogi, N., and Choudhury, K. P. (2022). Industrial manufacturing applications of zinc oxide nanomaterials: a comprehensive study. Nanomanufacturing 2 (4), 265–291. doi:10.3390/nanomanufacturing2040016
Sunita, D., and Yegoti, J. (2020). Effect of biosynthesized silver oxide nano particles in increasing the shelf life of different foods. J. Sci. Res. 64 (03), 07–12. doi:10.37398/jsr.2020.640302
Teleanu, D. M., Chircov, C., Grumezescu, A. M., Volceanov, A., and Teleanu, R. I. (2018a). Impact of nanoparticles on brain health: an up to date overview. J. Clin. Med. 7 (12), 490. doi:10.3390/jcm7120490
Teleanu, D. M., Chircov, C., Grumezescu, A. M., Volceanov, A., and Teleanu, R. I. (2018b). Impact of nanoparticles on brain health: an up to date overview. J. Clin. Med. 7 (12), 490. doi:10.3390/jcm7120490
Tian, B., and Liu, Y. (2021). Antibacterial applications and safety issues of silica-based materials: a review. Int. J. Appl. Ceram. Technol. 18 (2), 289–301. doi:10.1111/ijac.13641
Tropea, A. (2022). Microbial contamination and public health: an overview. Int. J. Environ. Res. Public Health 19 (12), 7441. doi:10.3390/ijerph19127441
Unglaube, F., Lammers, A., Kreyenschulte, C. R., Lalk, M., and Mejía, E. (2021). Preparation, characterization and antimicrobial properties of nanosized silver-containing carbon/silica composites from rice husk waste. ChemistryOpen 10 (12), 1244–1250. doi:10.1002/open.202100239
Wang, J., Tavakoli, J., and Tang, Y. (2019). Bacterial cellulose production, properties and applications with different culture methods – a review. Carbohydr. Polym. 219, 63–76. doi:10.1016/j.carbpol.2019.05.008
Wen, S., Bao, G., and Jin, D. (2023). Advanced optical properties of upconversion nanoparticles. Encycl. Nanomater, 613–648. doi:10.1016/b978-0-12-822425-0.00084-1
Xu, Y., Rehmani, N., Alsubaie, L., Kim, C., Sismour, E., and Scales, A. (2018). Tapioca starch active nanocomposite films and their antimicrobial effectiveness on ready-to-eat chicken meat. Food Packag Shelf Life 16, 86–91. doi:10.1016/j.fpsl.2018.02.006
Xue, J., Wu, T., Dai, Y., and Xia, Y. (2019). Electrospinning and electrospun nanofibers: methods, materials, and applications. Chem. Rev. 119 (8), 5298–5415. doi:10.1021/acs.chemrev.8b00593
Yadav, M., Dwibedi, V., Sharma, S., and George, N. (2022). Biogenic silica nanoparticles from agro-waste: properties, mechanism of extraction and applications in environmental sustainability. J. Environ. Chem. Eng. 10 (6), 108550. doi:10.1016/j.jece.2022.108550
Yadav, T. P., Yadav, R. M., and Singh, D. P. (2012). Mechanical milling: a top down approach for the synthesis of nanomaterials and nanocomposites. Nanosci. Nanotechnol. 2 (3), 22–48. doi:10.5923/j.nn.20120203.01
Yahya, E. B., Abdul Khalil, H. P. S., Ahmad, M. I., Rizal, S., and Muhammad, S. (2023). Cleaner approach of preparing antibacterial bioaerogel scaffolds using oil palm waste nanocellulose. Ind. Crops Prod. 191, 115897. doi:10.1016/j.indcrop.2022.115897
Yallappa, S., Deepthi, D. R., Yashaswini, S., Hamsanandini, R., Chandraprasad, M., Ashok Kumar, S., et al. (2017). Natural biowaste of Groundnut shell derived nano carbons: synthesis, characterization and its in vitro antibacterial activity. Nano-Structures Nano-Objects 12, 84–90. doi:10.1016/j.nanoso.2017.09.009
Yang, D., Liu, Q., Gao, Y., Wan, S., Meng, F., Weng, W., et al. (2023). Characterization of silver nanoparticles loaded chitosan/polyvinyl alcohol antibacterial films for food packaging. Food Hydrocoll. 136, 108305. doi:10.1016/j.foodhyd.2022.108305
Yang, L. (2015). Fundamentals of nanotechnology and orthopedic materials. Nanotechnology-Enhanced Orthop. Mat., 1–25. doi:10.1016/B978-0-85709-844-3.00001-X
Yasmeen, F., Karamat, H., Rehman, R., Akram, M., Ghfar, A. A., Abdelghani, H. T. M., et al. (2023). Fabrication and testing of edible films incorporated with ZnO nanoparticles to enhance the shelf life of bread. Food Biosci. 56, 103111. doi:10.1016/j.fbio.2023.103111
Zabidi, N. A., Fuad, M. S. A., Tawakkal, ISMA, Basri, M. S. M., and Othman, S. H. (2023). Mechanical, thermal and antimicrobial properties of PLA/Jackfruit skin composites containing thymol and nanocellulose. Mater Today Proc. doi:10.1016/j.matpr.2023.01.397
Zahid, M. U., Pervaiz, E., Hussain, A., Shahzad, M. I., and Niazi, M. B. K. (2018). Synthesis of carbon nanomaterials from different pyrolysis techniques: a review. Mater Res. Express 5 (5), 052002. doi:10.1088/2053-1591/aac05b
Zaki, M., Atiqah, M. S. N., Khalil, HPSA, Ikram, H., Alfatah, T., Mistar, E. M., et al. (2022). Microbial enhancement of nanocellulose isolation from sawn timber industrial wastes and fabrication of biocomposite membranes. Bioresour. Technol. Rep. 20, 101242. doi:10.1016/j.biteb.2022.101242
Zamare, M., Professor, D., and Babu Associate Professor, R. (2016). BIOSYNTHESIS OF NANOPARTICLES FROM AGRO-WASTE: A SUSTAINABLE APPROACH. Int. J. Eng. Appl. Sci. Technol. 1, 85–92.
Zambrano-Zaragoza, M. L., González-Reza, R., Mendoza-Muñoz, N., Miranda-Linares, V., Bernal-Couoh, T. F., Mendoza-Elvira, S., et al. (2018). Nanosystems in edible coatings: a novel strategy for food preservation. Int. J. Mol. Sci. 19 (3), 705. doi:10.3390/ijms19030705
Zehra, A., Wani, S. M., Bhat, T. A., Jan, N., Hussain, S. Z., and Naik, H. R. (2022). Preparation of a biodegradable chitosan packaging film based on zinc oxide, calcium chloride, nano clay and poly ethylene glycol incorporated with thyme oil for shelf-life prolongation of sweet cherry. Int. J. Biol. Macromol. 217, 572–582. doi:10.1016/j.ijbiomac.2022.07.013
Zhai, X., Zhou, S., Zhang, R., Wang, W., and Hou, H. (2022). Antimicrobial starch/poly(butylene adipate-co-terephthalate) nanocomposite films loaded with a combination of silver and zinc oxide nanoparticles for food packaging. Int. J. Biol. Macromol. 206, 298–305. doi:10.1016/j.ijbiomac.2022.02.158
Zhang, T., Wu, Y., Yang, D., Wu, C., and Li, H. (2021). Preparation, characterization, and in vitro tumor-suppressive effect of anti-miR-21-equipped RNA nanoparticles. Biochem. Biophys. Res. Commun. 558, 107–113. doi:10.1016/j.bbrc.2021.04.040
Zhang, W., and Rhim, J. W. (2022). Titanium dioxide (TiO2) for the manufacture of multifunctional active food packaging films. Food Packag Shelf Life 31, 100806. doi:10.1016/j.fpsl.2021.100806
Zhao, X., Tian, R., Zhou, J., and Liu, Y. (2022). Multifunctional chitosan/grape seed extract/silver nanoparticle composite for food packaging application. Int. J. Biol. Macromol. 207, 152–160. doi:10.1016/j.ijbiomac.2022.02.180
Keywords: agro-waste, nanoparticles, antimicrobial properties, nanoparticle synthesis, food preservation
Citation: Flores-Contreras EA, González-González RB, Pablo Pizaña-Aranda JJ, Parra-Arroyo L, Rodríguez-Aguayo AA, Iñiguez-Moreno M, González-Meza GM, Araújo RG, Ramírez-Gamboa D, Parra-Saldívar R and Melchor-Martínez EM (2024) Agricultural waste as a sustainable source for nanoparticle synthesis and their antimicrobial properties for food preservation. Front. Nanotechnol. 6:1346069. doi: 10.3389/fnano.2024.1346069
Received: 28 November 2023; Accepted: 30 January 2024;
Published: 16 February 2024.
Edited by:
Swarup Roy, Lovely Professional University, IndiaReviewed by:
Anka Trajkovska Petkoska, University St. Clement of Ohrid, North MacedoniaCopyright © 2024 Flores-Contreras, González-González, Pablo Pizaña-Aranda, Parra-Arroyo, Rodríguez-Aguayo, Iñiguez-Moreno, González-Meza, Araújo, Ramírez-Gamboa, Parra-Saldívar and Melchor-Martínez. This is an open-access article distributed under the terms of the Creative Commons Attribution License (CC BY). The use, distribution or reproduction in other forums is permitted, provided the original author(s) and the copyright owner(s) are credited and that the original publication in this journal is cited, in accordance with accepted academic practice. No use, distribution or reproduction is permitted which does not comply with these terms.
*Correspondence: Elda M. Melchor-Martínez, ZWxkYS5tZWxjaG9yQHRlYy5teA==
†These authors have contributed equally to this work
Disclaimer: All claims expressed in this article are solely those of the authors and do not necessarily represent those of their affiliated organizations, or those of the publisher, the editors and the reviewers. Any product that may be evaluated in this article or claim that may be made by its manufacturer is not guaranteed or endorsed by the publisher.
Research integrity at Frontiers
Learn more about the work of our research integrity team to safeguard the quality of each article we publish.