- Department of Materials and The Environment, Bundesanstalt für Materialforschung und –prüfung (BAM), Berlin, Germany
Microbiologically influenced corrosion (MIC) is a crucial issue for industry and infrastructure. Biofilms are known to form on different kinds of surfaces such as metal, concrete, and medical equipment. However, in some cases the effect of microorganisms on the material can be negative for the consistency and integrity of the material. Thus, to overcome the issues raised by MIC on a system, different physical, chemical, and biological strategies have been considered; all having their own advantages, limitations, and sometimes even unwanted disadvantages. Among all the methods, biocide treatments and antifouling coatings are more common for controlling MIC, though they face some challenges. They lack specificity for MIC microorganisms, leading to cross-resistance and requiring higher concentrations. Moreover, they pose environmental risks and harm non-target organisms. Hence, the demand for eco-friendly, long-term solutions is increasing as regulations tighten. Recently, attentions have been directed to the application of nanomaterials to mitigate or control MIC due to their significant antimicrobial efficiency and their potential for lower environmental risk compared to the conventional biocides or coatings. Use of nanomaterials to inhibit MIC is very new and there is a lack of literature review on this topic. To address this issue, we present a review of the nanomaterials examined as a biocide or in a form of a coating on a surface to mitigate MIC. This review will help consolidate the existing knowledge and research on the use of nanomaterials for MIC mitigation. It will further contribute to a better understanding of the potential applications and challenges associated with using nanomaterials for MIC prevention and control.
1 Introduction
Metal corrosion has been addressed as an important challenge for different sectors such as industry or public infrastructure, which inflicts economic and environmental problems. It affects the performance of facilities and operations, as well as leads to contamination and wasting of products, causing harmful effects on workers and the surrounding environments (Kruger, 2011). It is estimated that the overall corrosion cost is approximately 3.4% of the global GNP, and around 20% of corrosion damage in aquatic environments is microbiologically influenced corrosion (MIC) (Little et al., 2020a). MIC is identified as a phenomenon in which corrosion process is influenced by the activity of microorganisms (directly) and/or their metabolites (indirectly) (Lou et al., 2021). For a long time, sulfate reducing bacteria (SRB) were considered as a main culprit of MIC, and thus most of the studies in this field have focused on SRB. However, nowadays it is known that other microorganisms such as acid producing bacteria (APB) (Gu and Galicia, 2012), iron oxidizing/reducing bacteria (Enning and Garrelfs, 2014), fungi (Bento et al., 2005; Binkauskien and Lugauskas, 2017), archaea (Larsen and Lundgaard, 2010), etc. are also equally important. Particularly severe corrosion in the field scale often happens in the presence of a mixed population of microorganisms where each MIC-causing species can contribute to the material degradation in a different manner. MIC can compromise the integrity of critical components in industries such as oil and gas, marine, and water treatment, posing safety risks and environmental hazards. Effective mitigation measures help prevent accidents and protect the environment (Little et al., 2020a). For instance, MIC has prompted the release of more than 100,000 tons of methane due to the leakage of a storage field in United States, with considerable negative impacts for the climate and consequently for humanity/nature. The leakage was traced back to the activity of methanogen archaea in the ground water, which resulted external corrosion (Islam et al., 2016). Microorganisms’ activity can significantly accelerate corrosion process, leading to structural damage and potentially catastrophic failures in various industrial systems, pipelines, and infrastructure. By mitigating MIC, companies can extend the lifespan of their assets and reduce maintenance costs. Furthermore, as clean energy continues to expand, it becomes imperative to explore the MIC potential within the clean energy sector, such as offshore wind structures, hydrogen storage, and the infrastructure for the transportation of novel energy sources (Larsenon, 2020). The importance of implementing effective and efficient mitigation strategies for MIC cannot be overstated, as it is vital for safeguarding assets, ensuring safety and environmental protection, optimizing operations, and maintaining compliance with regulations. Thus, these strategies are crucial in minimizing the detrimental effects and production disruptions associated with MIC. Various approaches, encompassing physical, chemical, and biological treatments exist to combat MIC. Depending on the specific system and environmental factors, a combination of these methods may be employed to effectively manage and control MIC (Little et al., 2020a; Knisz et al., 2023). The discussions of various methods for MIC mitigating, exploring their distinctions, as well as their respective advantages and disadvantages are provided in Section 3.
With the increasing application of nanomaterials in various sectors such as medicine, wastewater treatment, and more, attention has turned to utilizing them to mitigate or control MIC in industries (e.g., biocides) (Rasheed et al., 2020a). The use of nanomaterials to inhibit MIC is a new field with sparse literature available. To address this gap, this review aims to consolidate and summarize the existing knowledge and research on the application of nanomaterials in MIC mitigation. By undertaking this review, we aspire to achieve several objectives. Firstly, it will serve as a valuable resource for researchers and professionals seeking to understand the current state of research and development in the field of nanomaterials for MIC prevention and control. Secondly, it will facilitate the identification of potential applications and the recognition of emerging trends in this innovative domain. Lastly, the review will also shed light on the challenges and obstacles inherent in the use of nanomaterials for MIC mitigation, thus contributing to a more holistic comprehension of this promising area of research.
2 Biofilms and MIC mechanisms
A biofilm is an actively induced accumulation of microorganisms wherein cells adhere to a surface embedded in a self-produced matrix of extracellular polymeric substances (EPS). EPS is diverse and consists of, e.g., proteins, sugars, DNA, lipids, and offers the cell community better chances of survival; thus, biofilm formation is the preferred life style of microorganisms, as it is associated with significant advantages. EPS serves as an anchor, firmly attaching microorganisms to the surface. Additionally, it acts as a nutrient trap, capturing essential nutrients from the surrounding environment. It also facilitates the release of nutrients to the microbes, contributing to their sustenance. Furthermore, it offers protection in case of harsh conditions (e.g., increased resistance to biocides, toxic substances, temperature, etc.) (Lewandowski and Beyenal, 2008).
Regarding MIC, biofilm plays a vital role in the degradation process. When a biofilm forms on a surface (such as metal), microorganisms have the capability to modify the environment at the surface/biofilm interface. These modifications may involve changes in the chemical composition (such as dissolved oxygen, pH, organic and inorganic species, etc. compared to the bulk environment), mass transfer rate near the metal surface, and hydrodynamics (Lewandowski and Beyenal, 2013). It has been demonstrated that microorganisms can accelerate the corrosion of metals by modifying the chemical environment near the metal surface, which depends on the properties of the corroding metal and structure of microbial community of the formed biofilm on the metal surface. Also, it has recently been illustrated that some of the microorganisms can uptake electron from metal surface (Lewandowski and Beyenal, 2008; Knisz et al., 2023). Note that there is no universal mechanism to explain the influence of microorganisms on the corrosion rate of different metals/materials. It is accepted in the literature that there are many MIC mechanisms, rather than one. MIC mechanisms are commonly categorized according to the presence and availability of oxygen in a particular environment. However, it should be borne in mind, in real-world scenarios, oxygen availability can fluctuate, and microorganisms capable of directly interacting with metal surfaces may intermittently consume oxygen. Consequently, rather than a purely aerobic or anaerobic setting, there is often an oxygen gradient present which fluctuates over time. This article focuses on MIC mechanisms that have been extensively studied (aerobic and anaerobic), and the key reactions are briefly mentioned.
2.1 MIC mechanisms under aerobic conditions
Metal corrosion can occur through the creation of differential aeration cells, which arise from varying concentrations of oxygen at different locations on the metal surface. This disparity in oxygen levels can be attributed to two main factors: 1) active consumption of oxygen by microorganisms within the biofilm, leading to nonuniform distribution of oxygen on the metal surface; 2) passive mechanisms where certain areas are physically obstructed, hindering oxygen access. Heterogeneous biofilm formation presents a non-uniform coverage on the metal surface. Different studies have shown that the oxygen concentration varies in different locations of biofilm. A lower oxygen concentration can be spotted in the covered regions and become anodic, where uncovered regions demonstrate higher cathodic oxygen concentrations. This can yield a potential difference within the covered and uncovered regions on the metal surface (Lewandowski and Beyenal, 2008; Chilingar et al., 2013; Ziadi et al., 2020). Surface deposits, such as metal oxides can also form oxygen concentration cells, leading to under deposit corrosion or oxygen gradient corrosion. Among the microbial groups associated with these processes, iron-oxidizing bacteria (FeOB) and manganese-oxidizing bacteria (MnOB) have been extensively studied. FeOB, for instance, oxidize Fe2+ to Fe3+ in oxygen-rich environments, creating a potential difference between oxygen-depleted areas underneath iron oxides and oxygen-saturated regions, potentially causing oxygen concentration cells or galvanic corrosion. This corrosion can result in pitting corrosion, characterized by cavities extending from the metal surface. These pits, along with complex metal deposits, can shield areas from the surrounding fluid, leading to self-sustaining pitting. If this occurs in crevices, it is called crevice corrosion (Table 1). Certain materials, such as corrosion-resistant steels, are designed to resist oxygen corrosion through passivation, forming a protective metal-oxide layer. However, some biofilms can disrupt this layer, leading to pitting corrosion. Additionally, aerobic bacteria within biofilms can deplete oxygen levels, creating anaerobic conditions favorable for the growth of anaerobes such as SRB and nitrate-reducing bacteria (NRB) (Knisz et al., 2023; Xu et al., 2023).
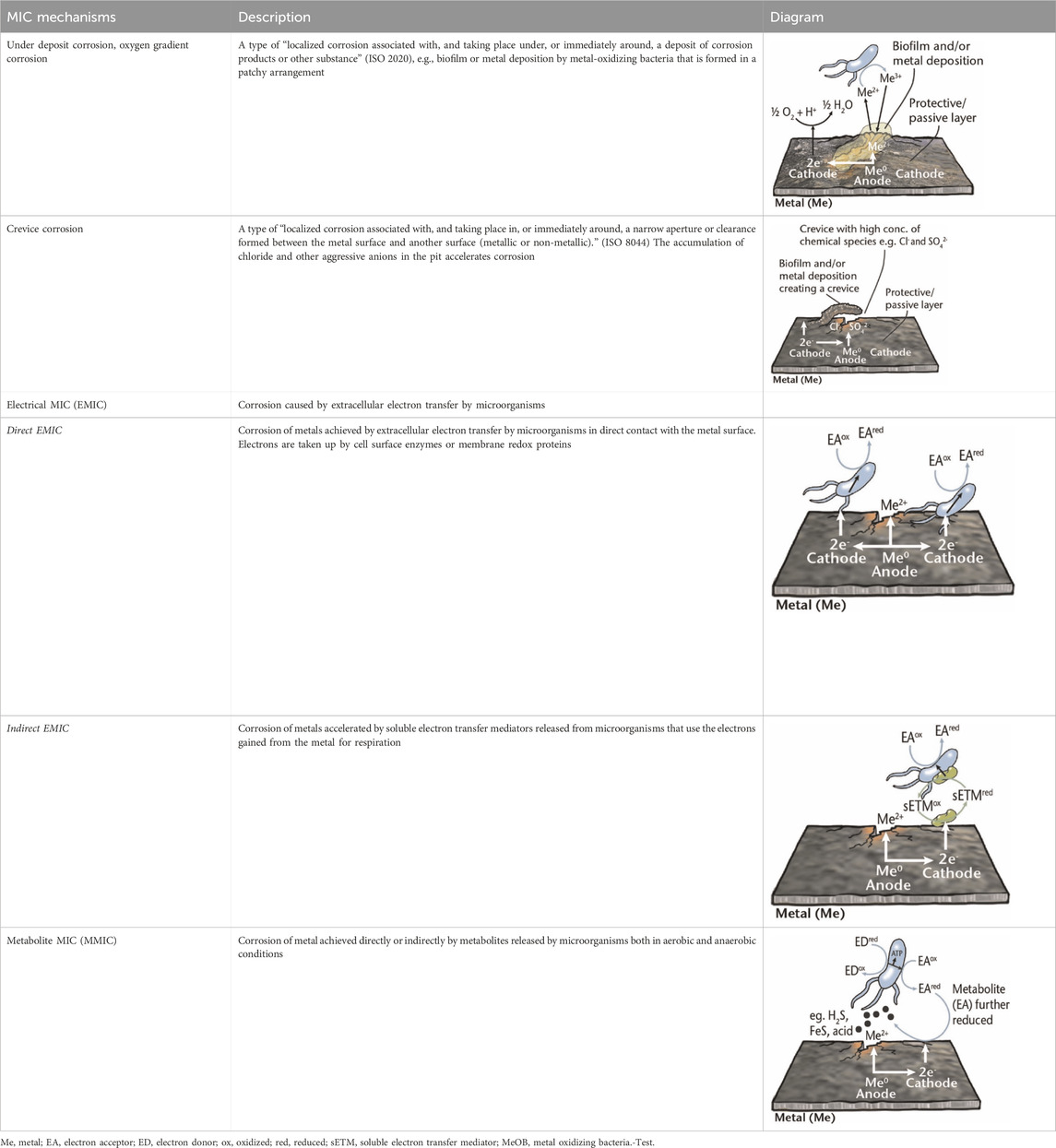
Table 1. Brief description of the main mechanisms associated with MIC of metals (Knisz et al., 2023).
Fungi are often associated with aerobic corrosion, colonizing metal surfaces and creating oxygen-free environments conducive to anaerobic bacteria, particularly SRB. EPS produced by fungi contain high concentrations of organic acids, which can accelerate metal deterioration. Research demonstrated that EPS presence can disrupt the passive film on aluminum, accelerating corrosion, especially pitting corrosion. This occurs as EPS enhances both the anodic dissolution of aluminum and the cathodic reduction of oxygen, resulting in the formation of large cathodic areas and small anodic areas, characteristic of pitting corrosion in the presence of EPS (Okorie and Nwokorie, 2021; He et al., 2022).
2.2 MIC mechanisms under anaerobic conditions
Historically, anaerobic MIC focused on SRB, as it was considered the main culprit of MIC. However, over the last decades it has become increasingly evident that other classes of microorganisms are also involved and can have a substantial contribution to corrosion of critical infrastructure. Recently, two distinct mechanisms including metabolite MIC (MMIC) and electrical MIC (EMIC) have been proposed which explain the principal mechanism behind MIC caused by different species.
MMIC occurs due to substances produced by microorganisms as site product of their metabolism (e.g., H2S). In other words, biofilms can provide locally concentrated corrosive metabolites underneath them causing MMIC. Examples are MIC of steel via acids secreted by acid producing bacteria (APB) in biofilms, and copper via H2S excreted by SRB (Xu et al., 2023). On the other hand, EMIC is induced by the uptake/consumption of electrons from the metal surface. In EMIC, microorganisms possess a unique metabolic process known as extracellular electron transfer (EET), through which they transfer electrons from solid compounds outside their cells to acquire energy, leading to the corrosion of metal structures (Table 1) (Knisz et al., 2023). More information about understanding microbial EET and recent progresses in EMIC is provided by Gu et al. (2021). Up until now, researchers have identified microorganisms from four distinct metabolic groups (SRB, methanogenic archaea, acetogenic bacteria, and NRB) as EMIC inducers. EMIC is caused by microorganisms that can efficiently uptake electrons from metallic iron either through direct or indirect EET (with the assistance of electron mediator molecules) (Knisz et al., 2023). Certain corrosive SRB and methanogenic archaea have been found to directly uptake electrons from metallic iron. Additionally, some microorganisms, including Shewanella spp., secrete electron mediator molecules such as riboflavin and flavin adenine dinucleotide (FAD) to facilitate EMIC (Knisz et al., 2023; Xu et al., 2023). Inorganic chemicals such as manganese and iodide have also been implicated as electron mediators (Kato, 2016). Hydrogen gas may serve as an electron mediator in some cases, but its role in corrosion remains unclear. The mechanisms of EET for EMIC are not fully understood, and it is likely that different EMIC-inducing microorganisms utilize distinct strategies for electron uptake. Further research, combining microbial physiology, genomics, molecular biology, and electrochemistry, is required to gain a comprehensive understanding of how EMIC-inducers efficiently uptake electrons from metallic iron. In the next section, different microbial species and their mechanisms to contribute to MIC are discussed.
2.2.1 SRB
For a long time, cathodic depolarization (CDP) theory was used to explain the observation that the open circuit potential (OCP) of iron during corrosion by SRB showed a positive shift, leading to a severe corrosion linked to SRB activity. This hypothesis primarily revolves around alterations in the cathodic reaction mechanism. Initially, this depolarization was attributed to the removal of hydrogen (H2) at the cathode due to the presence of hydrogenase+ SRB. The theory assumes that the biological oxidation of H2 accelerates the cathodic reaction, causing a depolarization of the OCP, resulting in a positive shift, and hence an increase in the corrosion rate (Little et al., 2020b). However, research has shown that the consumption of cathodic hydrogen by SRB does not significantly increase iron corrosion when iron is the sole electron donor (Venzlaff et al., 2013). While it does not entirely rule out the possibility that microorganisms might utilize hydrogen in MIC, it challenges the original concept proposed by the CDP theory. The current understanding of MIC mechanisms suggests that the role of hydrogen in corrosion by microorganisms may be more complex and different from what was initially proposed by the CDP theory (Knisz et al., 2023).
SRB grow in anaerobic environments, where they oxidize organic matter and reduce sulfate to sulfide. This metabolic activity is pivotal for the subsequent precipitation of metal sulfides. The H2S generated through bacterial processes can react with metal ions to create water-insoluble metal sulfides which may precipitate on the metal surface (Rao et al., 2022). The undissociated protons found in H2S resulting from the respiratory reduction of natural sulfate (for example, in seawater) in the presence of organic nutrients exhibit a higher reactivity with electrons derived from iron compared to protons from, or within, circumneutral H2O (Reaction 1). In this manner, SRB exert their influence indirectly by producing a corrosive metabolite (MMIC).
Recent research has shown that some SRB strains can uptake electron directly from metal surface via protein filament (nanowire) or indirect electron transfer mediated by a redox-active mediator. Through a carbon energy source starvation experiment, the bioenergetic implications of this process were revealed. Despite a decline in the number of sessile cells due to starvation, the experiment showed that SRB became more aggressive in corroding carbon steel, indicating a shift from utilizing organic carbon to Fe0 as the electron donor (Gu et al., 2021). When faced with electron acceptor or donor deficiency, nanowires are generated as a stress response, facilitating the transfer of electrons at an accelerated rate over considerable distances, akin to metal, to fulfill bacterial survival requirements. Although some studies have noted the presence of nanowires in SRB, the understanding of their mechanism, the potential genes implicated, and their regulatory processes have remained largely unexplored. Raya et al. (2023) has presented a comprehensive review of mechanism for nanowire formation.
Further, Desulfovibrio vulgaris has been a model microbe in foundational studies shaping current models of iron-containing metal corrosion under sulfate-reducing conditions. The proposed corrosion mechanisms by D. vulgaris include H2 consumption accelerating Fe0 oxidation, sulfide production forming FeS layer, cell hydrogenase release catalyzing Fe0 oxidation, direct electron transfer from Fe0 to cells, and flavins acting as electron shuttles. However, most of them need further investigations to take or leave the proposed mechanisms (Ueki and Lovley, 2022; Woodard et al., 2023). Two SRB strains named Desulfopila corrodens strain IS4 and Desulfovibrio ferrophilus strain IS5 were isolated from marine sediment, and they were found to quickly reduce sulfate while oxidizing metallic iron (as the sole electron donor) (Enning et al., 2012). Interestingly, other closely related SRB strains with hydrogen-utilization abilities did not exhibit such corrosive activities (Dinh et al., 2004). This ability to induce corrosion seems to be a special feature only specific to some SRB belonging to the Desulfovibrionaceae and Desulfobulbaceae families (Enning and Garrelfs, 2014). This suggests that the molecular mechanism for electron uptake from metallic iron is different for these EMIC-inducing SRBs/hydrogenases and is not a universal trait across all SRBs.
2.2.2 Methanogenic archaea
Methanogenic archaea are the main contributors to methane production in oxygen-depleted environments, often in higher abundance in areas with low sulfate levels. Methanogenic archaea play a significant role in MIC observed in various environments. In some cases, methanogens are considered corrosion-protective by generating non-conductive carbonate-rich depositions. Highly corrosive methanogens have been isolated from environments such as marine sediments and crude oil storage tanks. Methanosarcina strains, capable of growing with Fe0 as their sole energy source, are frequently associated with corroded steel structures, especially in low-sulfide marine environments. Regarding long-term infrastructure integrity, MIC caused by methanogenic archaea may pose concerns for underground storage facilities. Unlike SRB, which produces corrosive H2S, methanogens do not generate corrosive byproducts. Instead, the extraction of electrons from metals in anoxic conditions may be the primary mechanism which accelerates corrosion (Tamisier et al., 2022). The main evidence for direct electron uptake by methanogens was first reported in 2004 by Dinh et al. (2004). They isolated a methanogenic strain, Methanobacterium sp. strain IM1, from marine sediment, which grew and produced methane with metallic iron as the sole electron source, leading to iron corrosion. A couple of years later, another research group isolated EMIC-inducing methanogenic strains, Methanococcus maripaludis strain KA1 and Mic1c10, from crude oil reservoir tanks. Interestingly, both closely related hydrogenotrophic methanogens did not exhibit the same corrosive ability in the presence of metallic iron (Mori et al., 2010; Uchiyama et al., 2010), indicating that more complex mechanisms are involved in these methanogenic archaea strains.
2.2.3 Acetogenic bacteria
Acetogenic bacteria can generate energy through the reductive acetyl-CoA pathway, converting carbon dioxide into acetate. They typically use hydrogen gas or specific organic compounds as sources of reducing equivalents. Researchers have recently demonstrated acetate production in microbial cultures related to known acetogenic bacteria with metallic iron as the sole electron source (Acetobacterium sp.) (Mand et al., 2014). Further investigations by other research groups led to the enrichment of acetogenic microbial communities using metallic iron as the sole energy source, resulting in the identification of acetogenic bacteria that can grow on metallic iron and promote iron corrosion (Kato et al., 2015). In addition to these direct effects (EMIC), acetogenic bacteria might indirectly contribute to iron corrosion by producing acetate, which could stimulate the growth of corrosion-inducing SRB that depend on organic compounds such as acetate for carbon sources (Little et al., 2020b). Moreover, APB can generate both organic and inorganic acids, which can substantially lower the pH levels within the biofilm, pushing them into the acidic range (Gu and Galicia, 2012). In such conditions, a type of corrosion driven by acidity can take place, resulting in the dissolution of metals and the deterioration of concrete structures (MMIC).
2.2.4 NRB
Nitrate reduction is a significant microbial metabolism in anoxic environments, and recent studies have revealed that some NRB can cause EMIC. For example, Bacillus licheniformis ATCC 14580, a facultative NRB strain, was found to induce corrosion of carbon steel under nitrate-reducing conditions (Xu et al., 2013). Additionally, Prolixibacter sp. strain MIC1-1, belonging to the phylum Bacteroidetes, was identified as the first corrosive representative among NRB. Apart from using cathodic electrons from metallic iron as an electron donor, the metabolic end-product, nitrite, known to be a corrosive compound, appears to contribute to severe corrosion (Iino et al., 2015). Nitrate injection in oil and gas reservoirs has been used to mitigate souring and MIC caused by SRB by promoting NRB growth, which suppresses SRB. However, the discovery of EMIC-inducing NRB raised concerns about the risk of NRB assisted corrosion with nitrate supplementation (Yuk et al., 2020).
2.2.5 Key reactions in MIC
Xu et al. (2023) provided a comprehensive review of the fundamental reactions involved in MIC. Under aerobic conditions, Fe0 undergoes abiotic oxidation with oxygen to form Fe2O3, commonly known as rust (Reaction 2). In aqueous environments, heterogeneous biofilms composed of diverse microorganisms develop on metal surfaces, limiting the availability of oxygen for Fe0 oxidation. Under anaerobic conditions, potential Fe0 oxidants include protons (H+), nitrate, Fe3+, sulfate, CO2, and H2S, with only H+ (Reaction 3) and H2S (Reaction 4) reacting abiotically with Fe0 to produce H2. Other electron acceptors require microbial catalysis, where various microorganisms accept electrons from Fe0 to support anaerobic respiration. The resulting Fe2+ can then react with sulfide, carbonate, phosphate, or additional iron ions to form minerals (Reactions 5–10), which accumulate on corroding iron and are indicative of intense corrosion. Understanding the specific reactions occurring within corrosive biofilms is crucial for determining the rate and extent of iron corrosion in different environments.
3 MIC mitigation strategies
Four main approaches, including physical, chemical, electrochemical, and biological treatments have been developed/developing to combat MIC. This section discusses how different approaches are employed to mitigate MIC and their advantages and limitations.
3.1 Physical methods
Pigging, ultraviolet (UV) radiation, and ultrasonic are considered under this category. Pigging is a mechanical cleaning process which removes corrosion products and tubercles from the inner surface of pipelines. Use of UV radiation prevents the microorganism’s growth by altering their DNA, which has a high proficiency in the bacterial reduction (Skovhus and Lee, 2017).
3.2 Chemical treatments
Biocides refer to a broad range of chemical substances employed to prevent and manage the adverse effects caused by various pathogenic microorganisms. Usage of biocides is the most common method to mitigate MIC especially in steel pipes and closed systems. Selecting a biocide depends on the type of the microorganisms involved, the operational history, as well as environmental limitations and restrictions (Little et al., 2020a).
Iron alloys, such as stainless-steel containing chromium, are resistant to microbial corrosion due to the formation of a protective chromium oxide layer. Carbon steel lacks this protection and is more susceptible to corrosion. Adding copper to steel can inhibit corrosive microbes, but higher concentrations reduce steel toughness and may pose toxicity risks. Rare earth elements such as cerium offer antimicrobial properties with minimal impact on cost, but practical assessments are lacking. High-entropy alloys, composed of multiple metals, have shown to be promising in laboratory studies for microbial corrosion resistance, but their high cost and potential health risks limit large-scale applications. Non-ferrous metals such as titanium offer superior corrosion resistance but are costly, restricting their use to small-scale devices (Wang et al., 2023).
3.3 Electrochemical methods
Cathodic protection (CP) and coating are considered as electrochemical methods due to creating an electrochemical cell. CP involves the use of a direct current, either through a galvanic system or an impressed current system, to reduce and maintain the metal’s potential at a sufficiently negative level compared to the surrounding environment (Rasheed et al., 2020a; Knisz et al., 2023).
Coating is supposed to provide a physical barrier which prevents direct contact between microorganisms and the metal surface. For example, internal coatings and linings for carbon steel equipment offer an alternative to prevent contact between the environment and the metal, at least for a certain period. However, potential issues with internal coatings and linings include susceptibility to damage from heat or pigging, as well as mechanical damage during operation or maintenance. Some coatings create an inhospitable surface for biofilm attachment, making it more difficult for microorganisms to colonize and establish themselves on the metal. Regular inspection and maintenance of coatings are essential to ensure they continue to provide effective protection against MIC. Additionally, a multi-faceted approach that combines coatings with other MIC mitigation strategies may be necessary for comprehensive corrosion control in environments prone to biocorrosion (Knisz et al., 2023).
3.4 Biological treatments/using natural products
Recently, the feasibility of a new MIC mitigation method has been examined, where certain microorganisms are used to control the population of the others. The potential pathways by which microorganisms can inhibit corrosion include: 1) eliminating corrosive cathodic agents via bacterial processes, such as oxygen respiration in aerobic environments; 2) suppressing the growth of corrosion-inducing bacteria through the release of antimicrobial substances by other bacteria; and 3) establishing a protective coating on the metal surface, such as passive metal oxides or biofilms that exude adhesive corrosion inhibitors (Zuo, 2007). Nitrate and denitrifying bacteria have reportedly an inhibitory effect on MIC induced by SRB; however, the discovery of EMIC-inducing NRB has raised concerns about the risk of NRB-assisted corrosion (Yuk et al., 2020). Yet, more research is required for the biological treatment of MIC, and there is a lack of evidence for utilization under real-world conditions. There is a potential of using natural compounds derived from microbes or plants as alternatives to synthetic chemicals for preventing corrosion. These compounds offer several advantages, including being more sustainable and potentially less toxic to humans. Examples of such compounds include D-limonene, D-amino acids, peptides, biosurfactants, and quorum sensing inhibitors. Quorum sensing inhibitors interfere with the communication mechanisms of microbes, thereby disrupting biofilm formation and corrosion processes. While methods such as biological approaches and the use of natural compounds show promise in laboratory studies, their effectiveness and practicality in real-world scenarios require further evaluation through large-scale trials. Furthermore, optimizing their production and conducting techno-economic analyses are crucial steps that must be undertaken before these methods can be feasibly employed for microbial corrosion prevention (Wang et al., 2023).
3.5 Advantages and disadvantages of MIC mitigation methods
It is important to note that there is no single perfect method for MIC mitigation. Typically, a combination of two or three methods is employed to effectively control MIC. Each method comes with its own set of advantages and limitations. The selection of a method depends on several factors including the industry type, environmental considerations, cost, availability of the method, skilled professionals, compliance with regulations, the history of MIC and corrosion management within the system, etc. Indeed, physical and chemical treatments remain the two main approaches for controlling MIC. Physical methods such as pigging can remove biofilms from surfaces but do not prevent their recurrence, leading to ongoing maintenance costs. This method is feasible to keep the system clean and manage the possibility of MIC, but it can be applied only on the facilities designed for pigging such as inside the pipelines (Skovhus and Lee, 2017). Also, it falls short in preventing further biofilm formation, which necessitates continual and costly maintenance and retrofitting measures (Knisz et al., 2023). Application of UV as a physical method in a large scale is not possible, and if there are non-living particles shielding the microorganisms from UV, the efficiency will be reduced (Mand et al., 2019; Little et al., 2020a).
The predominant chemical treatment for controlling MIC is the utilization of biocides; however, its effectiveness is often limited. Biocides primarily target planktonic bacteria, but MIC typically occurs beneath adherent biofilms, which demonstrate substantially greater resistance to antimicrobial agents compared to planktonic microorganisms. This reduced susceptibility within biofilms can hinder the efficacy of biocide treatments in combating MIC effectively (Zuo, 2007). Additionally, prolonged use of biocides can lead to the development of resistance over time. To counteract the increased resistance of biofilm cells to biocides, higher concentrations of biocides are frequently needed. However, this strategy can present challenges. For example, research by Sharma et al. (2017) suggests that elevated concentrations of bronopol, particularly when repeatedly applied as is typical in shale gas operations, can result in heightened corrosion levels. Furthermore, biocides can induce cross-resistance, where microorganisms develop resistance to toxic substances, aggravating the challenge of MIC control. Furthermore, biocides pose environmental risks due to their toxicity, potentially harming both human health and ecosystems. When used in large quantities, they can contaminate aquatic environments and harm non-target organisms, entering the food chain (Michalak and Chojnacka, 2014). Glutaraldehyde, a common biocide used in industry and hospital sanitation, has been reported to exhibit acute toxicity to aquatic organisms (Leung, 2001).
In addition to biocide usage, integrating various methods such as CP and protective coatings is often necessary for effective control of MIC. CP is a widely used method against abiotic corrosion. According to the standard EN12495 (Leheta, 2023), biological activity affects its effectiveness, suggesting the need for more negative potentials in anaerobic environments where MIC is active. However, debates persist on whether lowering protective potentials beyond those for abiotic corrosion can effectively combat MIC (Romero et al., 2021). Further research is needed to understand the mechanisms and to optimize CP for MIC prevention or reduction. Antifouling coatings containing active agents, such as biocides and corrosion inhibitors, have been widely used as preventive measures. Nevertheless, a significant drawback is the continuous release of toxic and persistent chemicals, which results in shorter protection periods and potential ecological concerns. These coatings are now subject to strict regulations, prompting the search for effective and environmentally friendly long-term solutions (Knisz et al., 2023).
While biocide treatments and antifouling coatings have the potential to be effective in controlling MIC, they face notable challenges. They are not specifically targeted to MIC microorganisms, leading to cross-resistance issues and often necessitating higher concentrations, which can be ineffective or even counterproductive. Additionally, these treatments pose environmental risks and may harm non-target organisms. Thus, there is a growing need for environmentally friendly long-term solutions as stricter regulations emerge to govern chemical treatments (Ferreira et al., 2019). Greener and less toxic alternatives with enhanced effectiveness have also emerged. These alternatives range from innovative polymer structures to nature-inspired biomimetic approaches, including the incorporation of bioactive nanoparticles and the utilization of natural bioactive compounds or extracts. It can also be changing the chemical structure of a common toxic biocide to a greener one, such as bifunctional antibacterial and anticorrosive broad-spectrum rosin thiourea iminazole quaternary ammonium, which utilizes a chiral environment for effective metal surface adsorption through physical and chemical interactions, offering a simple, cost-effective, and efficient solution for corrosion mitigation (Wang et al., 2024). However, the full adoption of these greener solutions is hindered by challenges such as lengthy synthesis processes, limited availability of some natural sources, the lack of real-world proof of concept, environmental impact assessments, regulatory approvals, and the need for substantial funding and time (Knisz et al., 2023).
Lately, nanomaterials have gained attention for their potential to produce biocides or coatings with enhanced antimicrobial properties against various waterborne microorganisms, while minimizing environmental impact. The reasons they are considered to have great potential for development as a greener strategy to control MIC include: 1) nanomaterial-based biocides or coatings can be engineered to specifically target microorganisms involved in MIC, minimizing parallel damage to non-target organisms and reducing overall toxicity; 2) the small size and high surface area-to-volume ratio of nanoparticles enable better penetration into biofilms and microbial cells, enhancing the biocidal effect and improving overall efficacy in MIC control; 3) they can be designed for controlled and sustained release, ensuring long-lasting protection against microbial colonization without the need for frequent reapplication, thus reducing environmental exposure and toxicity; 4) nanomaterials used in nanobiocides and nanocoatings can be biodegradable and less harmful to the environment compared to traditional biocides, leading to reduced contamination of ecosystems and lower risks to human health; 5) nanocoatings can provide more uniform and complete coverage on surfaces, creating a physical barrier that prevents microbial attachment and biofilm formation, thereby lowering the risk of corrosion; 6) they can be engineered to possess additional functionalities such as self-healing properties, corrosion inhibition, and surface modification, offering comprehensive protection against corrosion and microbial degradation.
Overall, nanobiocides and nanocoatings offer promising alternatives to conventional biocides for MIC mitigation, providing enhanced efficiency and environmental sustainability. However, as with other methods, they also have limitations. Moreover, this field is relatively new and requires further investigation both in laboratory and industrial settings. There is still a long way to go before they can be considered verified and practical solutions. This review discusses the progress made in this field, outlines existing limitations, and offers suggestions for further research.
4 Application of nanomaterials to mitigate MIC
Nanomaterials have been selected as ideal candidates for various applications, due to the properties such as larger surface to volume ratio of nano-sized materials compared to their bulk counterpart. Hence, they have also been applied in different sectors of industries to control corrosion and biofouling. Nanomaterials have been extensively used in the oil and gas sector for different applications such as drilling and hydraulic fracturing fluids, corrosion inhibitors, enhanced oil recovery, viscosity reduction, methane release from gas hydrates, etc. (Fakoya and Shah, 2017). Note that nanomaterial-based biocides or coating materials must have effective antimicrobial properties against different waterborne microorganisms while posing least extent of environmental impacts. Farag (2020) prepared a comprehensive review of all types of nanomaterials applied as corrosion resistance coating and inhibitors (Farag, 2020). Also, various applications of nano coating such as self-healing surfaces (Do and Lin, 2016), improvement of the tribocorrosion performance of materials (Haruna and Haruna, 2018), and nanoparticle-based corrosion inhibitors (Bera et al., 2018) were reported by Li (2006). Further, Omran and Abdel-Salam (2020) addressed the problems of the existing and the new trends applied to control corrosion and biofouling with nanotechnology as a new solution (Omran and Abdel-salam, 2020). However, general corrosion has gained the most momentum among these reviews with biofouling and MIC excluded or mentioned briefly. Rasheed et al. (2019a) briefly reviewed the nanomaterials employed to inhibit SRB (Rasheed et al., 2019a). Considering MIC mitigation, nanomaterials have been predominantly used in either a form of nano biocide or nanocoatings. Therefore, we would like to present a comprehensive review of the nanomaterials with antimicrobial properties investigated as a biocide or in a form of coating on a surface to specifically mitigate MIC. In the following sections, various groups of nanomaterials and their biocidal mechanisms are discussed as along with different nanocoatings on metal surfaces and their mechanisms for controlling MIC.
4.1 Nanomaterials as a biocide
Recently, different nanomaterials have been introduced as an alternative to the conventional biocides (Rasheed et al., 2019b; Kalajahi et al., 2020a; Kalajahi et al., 2020b). An ideal nano biocide must show potent antibacterial properties against waterborne microorganisms with fewer environmental impacts. Different nanomaterials have been frequently investigated as antimicrobial agents, especially for medical application and drug delivery purposes. However, only a few studies have applied nanomaterials to mitigate MIC, as presented in Table 2.
Nanomaterials are commonly classified into four main categories based on their structural configurations (Figure 1): inorganic-based, carbon-based, organic-based, and composite-based nanomaterials (Mekuye and Abera, 2023). In general, the antimicrobial activity of the nanomaterials is influenced by their surface modification, composition, intrinsic properties, and the type of microorganism.
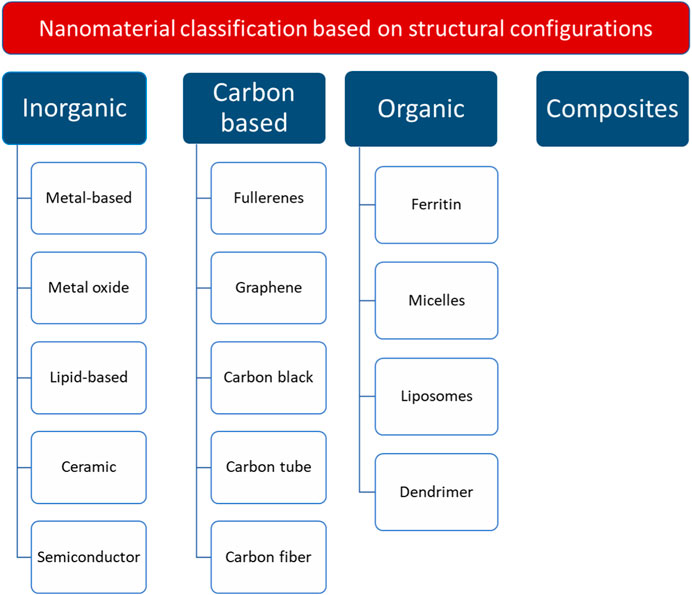
Figure 1. Nanomaterial classification, based on structural configurations. The figure is reconstructed based on (Mekuye and Abera, 2023).
4.1.1 Inorganic-based nanomaterials
Inorganic based nanomaterials consist of various metal and metal oxide nanoparticles (M&MONPs), which have been noticed due to their high potential antimicrobial activity. There are different methods for the synthesis of inorganic nanoparticles, with biological methods offering environmentally friendly, nontoxic, and cheap replacements compared to chemical and physical methods. One of the promising biofactories to synthesize metal nanoparticles is microbial cells (Narenkumar et al., 2018; Busi and Rajkumari, 2019). Inorganic nanoparticles such as silver, iron, and lead have been synthesized either extra or intracellularly using different kinds of microorganisms such as bacteria, yeast, fungi, algae, and cyanobacteria. Bacteria are the highly employed microorganisms to synthesize M&MONPs due to their abundance in varied environments, significant adaptability, and easy-to-control growth conditions (Swaminathan, 2019).
Mechanism: Several in vitro studies have reported the inhibition of microbial species in contact with M&MONPs, also antimicrobial effectiveness of them depends on the type of material and particle size. Reportedly, the positive charge of the M&MONPs surface improves their binding to the negative surface charge of bacteria, which can improve the bactericidal effects (Bhattacharya and Neogi, 2019). Researchers have already established that M&MONPs can prevent biofilm formation by imparting toxicity in different microorganisms through several independent of codependent pathways such as causing targeted damage to the mitochondria or the nucleus, damage to the cell membrane or even disruption of DNA and protein synthesis. Depending on the functionality and the mode of synthesis, nanoparticles can also release toxic metal ions to cause direct cytotoxicity. Reactive oxygen species (ROS) generation and the resulting cellular oxidative stress can also be another mode of action. Functional nanomaterials can also disrupt the electron transport chain of a living cell, cause quorum sense quenching or inhibit the essential enzymes imperative for their survival (Ejileugha et al., 2021). Microorganisms secrete diffusible signal molecules which regulate mutualistic growth and coordination among species. Microorganisms in biofilms interact with each other through quorum sensing via the secretion and recognition of some micro molecules by both Gram-positive and Gram-negative bacteria. M&MONPs-based biocides can prevent and block this interaction thereby controlling the biofilm formation. Nanoparticles of Ag, Au, TiO2, ZnO, SiO2, and chitosan can act as quorum sensing jammers in biofilms. Figure 2 illustrates different pathways through which nanoparticles can harm microorganisms (Hayat et al., 2019).
Silver nanoparticles (AgNps) have been widely used as popular inorganic nanoparticles. AgNps displayed antimicrobial activity against the drug-resistance bacteria even at low concentrations, and caused cell death due to the interaction with disulfide bonds of the enzymes disrupting the metabolic activity. Skiba et al. (2020) reported the formation of a thinner biofilm of Desulfomicrobium genera and reduction of the corrosion rate of mild steel due to the application of AgNps. However, the result revealed that bacteria of Desulfovibrio genus did not show any obvious change to AgNPs. In a different notable endeavor, researchers generated bioengineered AgNPs surface-functionalized with proteinaceous plant extract material in vitro in a test tube by treating ionic AgNO3 with leaf extract of Azadirachta indica which acted both as a reducing and stabilizing agent. The anti-corrosion test of mild steel (MS1010) by the corrosion-inducing bacterium Bacillus thuringiensis EN2 isolated from cooling towers was evaluated via electrochemical impedance spectroscopy (EIS), weight loss, and surface analysis. The studies indicated that AgNPs robustly inhibited the biofilm-formation on the surface of MS1010 and lowered the corrosion rate with a corrosion rate from 2.2 mm/y to 0.5 mm/year and boosted the inhibition efficiency to 77% compared to 52% offered by the plant extract only. Surface analysis using infrared spectroscopy indicated the formation of a self-assembled protective layer by the AgNPs on the surface of MS1010. Also, AgNPs actively hindered both pitting and biofilm formation (Narenkumar et al., 2018). San Keskin et al. (2021) investigated the antibacterial and anticorrosive properties of green AgNPs. The corrosion of copper in artificial seawater (ASW) was studied under the influence of Halomonas variabilis and AgNPs. Electrochemical measurements were employed to monitor corrosion behaviors. The results revealed that AgNPs exhibited a notable antibacterial activity against pathogenic microorganisms. Electrochemical studies indicated a significant reduction in current density in ASW containing H. variabilis + AgNPs compared to ASW and ASW inoculated with the bacterium alone. This reduction confirmed a decline in the corrosion rate of copper, highlighting the potential of AgNPs as effective agents for both antibacterial and anticorrosive applications in seawater environments.
Zinc oxide nanoparticles (ZnONPs) have an antimicrobial activity against a wide range of bacteria. However, compared to AgNps, the antibacterial efficiency of ZnONPs relies on the particle size and concentration. Thus, they possessed greater effectiveness with higher concentration and lower particle size. A myco-synthesis of ZnONPs was investigated as a potential antibiofouling material against aerobic corrosion-causing bacteria, which showed a significant reduction in the corrosion rate of copper coupon (Sujatha et al., 2019).
Copper nanoparticles (CuNPs) have specific biological, physical, and chemical properties, and requires fewer financial resources for preparation and are a great choice for synthesizing (Singh et al., 2019). As a biologically fabricated NPs, the peel extract of Citrus reticulata (tangerine) was used in the synthesis of CuNPs. The product was characterized and applied as an inhibitor for steel corrosion induced by 1 M HCl and Desulfovibrio sp. Round, monodisperse, non-aggregated, zero-valent Cu crystals with size range between 54 and 72 nm were obtained. CuNPs caused a significant reduction in Desulfovibio sp population at 1.96 mg/L minimum inhibitory concentration with biocorrosion inhibition efficiency of 79.8% and 68.4% at 303 K and 333 K, respectively. At these temperatures, 1.0 g/L CuNPs also inhibited acid corrosion with efficiency of 95.3% and 84.6%, respectively. Due to their small size, the nanoparticles more easily permeate through bacteria cell wall to alter their metabolic activities and growth than the extract (Ituen et al., 2020).
Nickel nanoparticles (NiNPs) were synthesized through the aqueous extract of Citrus reticulata peels (CRE), along with the characterization and application of the resulting composite as an inhibitor against microbial and acid corrosion. The CRE-NiNPs exhibited stability, non-agglomeration, monodispersity, and a round shape, measuring within 40–55 nm. Possessing surfaces abundant in O and N sites, CRE-NiNPs demonstrated efficient adsorption and inhibition of X80 steel corrosion in a 1 M HCl solution, with effectiveness rates of 87.3% and 80.6% at 30°C and 60°C, respectively. In addition, the nanoparticles achieved a 3-log reduction in Desulfovibrio sp. population and a 73.2% inhibition efficiency at a minimum inhibitory concentration of 3.21 mg/L. The inhibition of microbial corrosion is attributed to the nanoparticles’ ability to penetrate bacteria cells, suppressing their metabolism through physical and chemical adsorption mechanisms. Compared to the crude form, CRE-NiNPs demonstrated superior thermal stability, increased efficiency, and provided enhanced surface protection at elevated temperatures (Singh and Yuanhua, 2020).
High Entropy Alloy Nanoparticles (HEA-NPs) with photothermal heating properties are also very effective in achieving antibacterial and antibiofilm effects. Li et al. (2023) reported the synthesis of one such HEA-NP FeNiTiCrMnCux with a high photothermal conversion performance via arc-discharge method. They observed that solar energy irradiation activates the synergistic effect of copper ions release and photothermal damage, causing cell membrane rupture, ROS generation, denaturation of proteins as well as enzymes, etc. Also, positively charged nanoparticles can get accumulated on the surface of negatively charged bacteria, boosting the overall antibacterial capacity.
4.1.2 Carbon-based nanomaterials
Carbon-based nanomaterials have been widely used as antimicrobial agent, including the following:
Fullerene: The antimicrobial activity of fullerene-caged particles has been reported, especially when they are exposed to light, for which several mechanisms were proposed. Fullerenes can trigger oxidative stress through the production of ROS, creating suitable conditions for the destruction of microorganisms. The antibacterial properties of C60 fullerene are likely related to interactions of C60 with membrane proteins and other molecules (Lyon and Alvarez, 2008).
Carbon nanotubes: This group consists of single-walled, double-walled, and multi-walled particles. Various studies indicated that the single-walled and multi-walled particles have significant inhibitory effects against microorganisms. In particular, the size of carbon nanotubes plays an important role in the deactivation of microorganisms. As the size of the carbon nanomaterial shrinks, their surface-to-volume ratio grows, leading to a stronger binding to the cell wall or cell membrane of the microorganisms and thus to a more powerful effect. The operation mechanism is based on the connection of CNT nanotubes with microorganisms and rupture of the cell membrane, metabolic processes, and morphology (Khan et al., 2016). The review of existing literature uncovered no documented instances of employing fullerene and carbon nanotubes to mitigate MIC in the industrial context.
Graphene: Graphene-associated nanomaterials such as graphene nanosheets, graphite, and graphene oxide (GO) have been widely investigated. Divers properties of graphene nanomaterials complicates studying their precise antimicrobial mechanisms (Al-jumaili et al., 2017). Ouyang et al. (2013) reported a new nanocomposite with rGO, CuO, and poly-L-lysine (PLL), showing exceptional bactericidal properties against E. coli and S. aureus, with a lethality rate of 99.9% and prolonged antibacterial effect. The nanocomposite restricts the cell membrane permeability and cause destruction of the microorganisms via altering the ionic concentration of the intracellular fluid. Selim et al. (2023) fabricated a novel nanocomposite of RGO decorated with α-Mn2O3 nanorods (NRs) for mitigating MIC. These nanocomposites displayed controlled morphology, geometry, surface exposure, and orientation. The field emission SEM images indicated that the blade-like RGO/α-Mn2O3 nanocomposites viciously attached themselves into the microbial cell walls resulting in morphology disruption, cell wrapping, and microbial killing. It was observed to be highly effective against the anaerobic SRB strain D. halophilus SL 890, proving to be a potent candidate for microbial growth inhibition.
4.1.3 Organic-based nanomaterials
Examples of these nanomaterials include dendrimers, cyclodextrin, liposomes, and micelles. Berkl et al. (2022) reported that the concentration- and time-dependent quorum quenching ability of different cyclodextrins can impair the mechanisms of biofilm formation of P. aeruginosa PAO1. The cavity size and the chemical environment of cavity entrances of cyclodextrin were found to alter bacterial communication through formation of an inclusion complex with bacterial quorum sensing signaling molecules thus influencing the virulence of the microorganism. The review of the literature revealed no reports on the utilization of organic-based nanoparticles to mitigate MIC.
4.1.4 Composite nanomaterials
These types of materials are combinations of metal-based, metal oxide-based, carbon-based, and/or organic-based nanomaterials. These materials often exhibit complex structures, such as metal-organic frameworks. Morsi et al. (2016) employed core-shell nanocomposites consisting of functional core of ZnONPs and surrounding polyacrylamide shell to inhibit the corrosion and examined the antibacterial activity of the nanocomposite against different types of bacteria. They found a lower corrosion rate in the presence of the nanocomposite (up to 10 wt. % of ZnONPs). Another study reported successful application of a chitosan-ZnO nanocomposite with 10% ZnO content (CZNC-10) as a biocide for the control of biofilm and reduction of biocorrosion on carbon steel. The study indicated that a concentration of 250 μg/mL of CZNC-10 proved highly effective in inhibiting the biofilm formation by SRB on carbon steel. Compared to the control, the charge transfer resistance (Rct) values after 21 and 28 days of incubation in the presence of CZNC-10 were approximately 3.2 and 2.8 times higher, respectively. This substantial rise in Rct values indicates a robust corrosion inhibition, reaching a maximum efficiency of 74% (Rasheed et al., 2019c). In addition, ZnONPs and CuONPs were synthesized via brown algae (Sargassum muticum) to inhibit the corrosion and biofilm formation on the surface of water pipelines, showing 92.31% protection efficiency (Farrag et al., 2019).
Doping is an extremely approved method to improve the antibacterial properties of nanomaterials by affecting the ROS production. It has been reported that the dopant’s type, technique of synthetization, and the experimental factors significantly alter the entire electronic structure of nanomaterials, resulting higher antibacterial efficiency. Ag is one of the widely used dopants, as it can be toxic even in the dosage under the certified level. Kalajahi et al. (2020) inspected the efficiency of graphene oxide/silver nanoparticles nanocomposite (GO-Ag) to inhibit MIC on the surface of steel X60 via electrochemical measurements and surface analyses. The result illustrated that GO sheets have antibacterial activity against SRB, but the antibacterial efficiency of GO was improved by loading AgNPs on the sheets (Figure 3B). Tafel polarization results indicated that the corrosion rate of the steel X60 was reduced in the presence of GO-Ag (Figure 3C), which is attributed to the SRB growth inhibition and creating a resistance film on the metal surface due to the GO precipitation (Kalajahi et al., 2020a). The corrosion inhibition mechanism of GO-Ag was explored through quantum chemical calculations and molecular dynamics (MD) simulations. The findings highlighted the formation of a dense film on the Fe surface by GO-Ag, effectively impeding the movement of corrosive particles towards the Fe surface. Figures 3D, E exhibits the ultimate adsorption configuration of GO-Ag on the Fe (1 1 0) surface. The simulation revealed a gradual movement of inhibitor molecules toward the Fe surface, indicating a robust affinity between the inhibitor and Fe. Assuming that physical interactions play a predominant role in inhibitor-iron interactions, it can be deduced that GO-Ag is likely to function as an effective corrosion inhibitor. Ultimately, a comprehensive understanding of the corrosion inhibition mechanism of multifunctional nanostructured inhibitors was proposed, drawing insights from both experimental and theoretical studies (Figure 3F) (Taghavi Kalajahi et al., 2023).
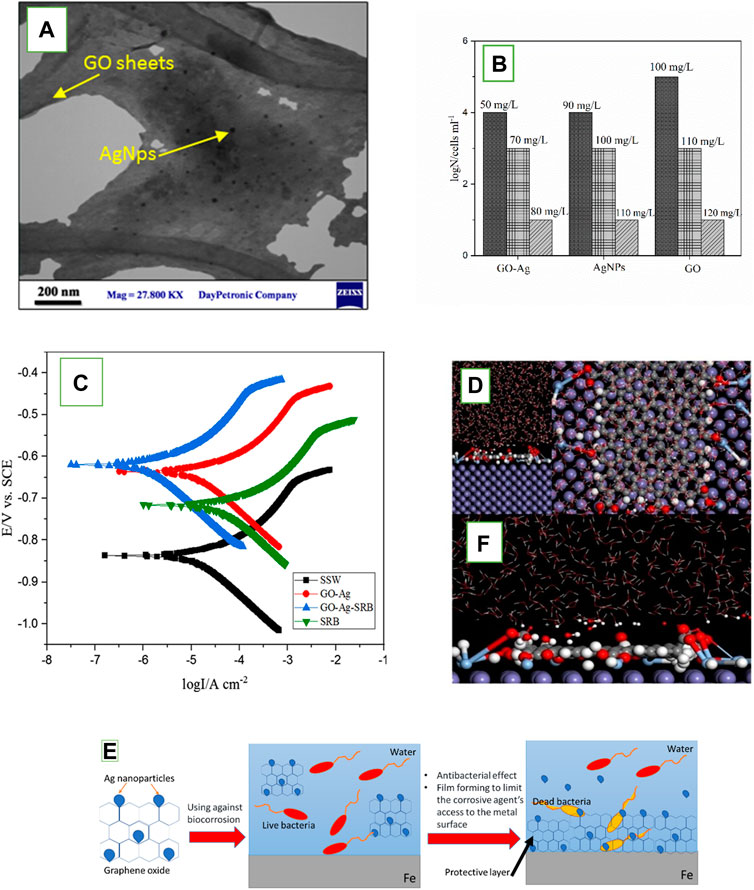
Figure 3. (A) Transmission electron microscopy (TEM) image of the GO-Ag, which shows the distribution of AgNps on the GO sheets (Kalajahi et al., 2020a); (B) SRB growth in the presence of different concentration of GO-Ag, AgNPs, and GO nano sheets; (C) Tafel polarization curves of X60 steel in four cells with different treatments, at 30°C, in 15th day of incubation; (D,E) Equilibrium adsorption configurations of GO-Ag on Fe (1 1 0) surface obtained by MD simulations. (D) top view, (E) side view; (F) Suggested inhibition mechanism for GO-Ag (Taghavi Kalajahi et al., 2023).
4.2 Nano coating
The use of coatings has a long history as a strategy to protect both abiotic and biotic corrosion. Coatings are supposed to form a barrier against the corrosive agents on the surface of interest to prevent or reduce negative effects a material might experience. Attempts have been made to discover and develop more potent anticorrosion formulations to protect the facilities, equipment, and structures from corrosive environments/conditions. The results suggested that achieving a substantial protective effect relies on four key features in the coating. These features are associated with heterogenicity, electrical properties, efficacy of corrosion and reduced adhesion capacity, as well as their ability to release corrosion inhibitors. Powder coating and water-based coating are two methods to coat a material. The advantages of powder coatings include the ability to create a thick film, not creating volatile organics, and high efficiency in corrosion protection, making it customer-friendly. However, powder coating still shows limitations, such as production problems due to not containing volatile components and being inapplicable on large surfaces since 200-degree heating need to be applied after coating. Thus, to overcome some of these problems of coating, nanomaterials were introduced to develop the mechanical, chemical, and optical properties of coating (Farag, 2020). Nanocoating is defined as a nanoscale thickness of the coating, nanosized grains/phases of coating, or the dispersion of the second-phase particles into the nanosized matrix (Alasvand, 2016). The harmful impacts of the corrosive environments on different surfaces were significantly mitigated by applying nanocoating, because of multiple preferences such as adhesive quality, hardness of surface, and surface tribological properties improvement. Furthermore, nanocoating provides smoother and slender thickness allowing greater compatibility in designing the equipment and lowering the maintenance expenses (Farag, 2020).
Manipulation of materials on the nanoscale can impart some unique chemical, mechanical, photophysical, thermal and surface properties which can be deployed to amplify functions necessary for urban built environments. Owing to their clear advantages over thicker coatings (higher surface to volume ratio, strength, toughness, wear resistance and thermal barrier, environmental adaptability, etc.) functional nanocoatings have found diverse applications as hydrophilic and photocatalytic coating, solar reduction coating, phase change materials (PCM) coating, hydrophobic coating amongst many others depending on their targeted modification and function-based fabrication (Zhu et al., 2022).
Smart coating is the most noteworthy approach among the nanotechnology-related coatings. These active coatings can react to external drivers such as humidity variation and pH. The size and dimension of the nanoparticles coupled with the intense layering provide strong adhesion and immensely favorable physical coverage of the protective coating. The barrier property of nanocoatings should also be taken into account during fabrication as suitable functionalization can alter the surface layer sorption and result in more effective corrosion inhibition. For instance, integration of different loading of TiO2 in epoxy polymer matrices within epoxy-TiO2 nanocomposite coatings significantly enhanced the effectiveness of the modified nanocoatings (Thakur et al., 2023). They also showed the ability of further corrosion prevention by releasing the corrosion inhibitors or biocides, which are loaded in dispersed nanocarriers or nanoreservoirs in the matrix (Saji, 2012). Bao et al. (2019) presented the challenges related to the nanocoating using different nanoparticles, and the perspective of nano coating system.
Regarding MIC prevention, nanocoating needs to indicate reduction of cell adhesion and biofilm formation. An effective coating needs to interlink a direct and on-contact biocide, which can provide constant antimicrobial activity. It should prevent the primary surface contamination of a wide range of corrosive microorganisms and inhibit the colonization over time (Swaminathan, 2019). Nanomaterials that show dual functionality of anticorrosion and antimicrobial are ideal coating candidates to inhibit both corrosion and bacterial adhesion. There are some studies on employing nanomaterials for coating different metals to mitigate MIC, as summarized in Table 3.
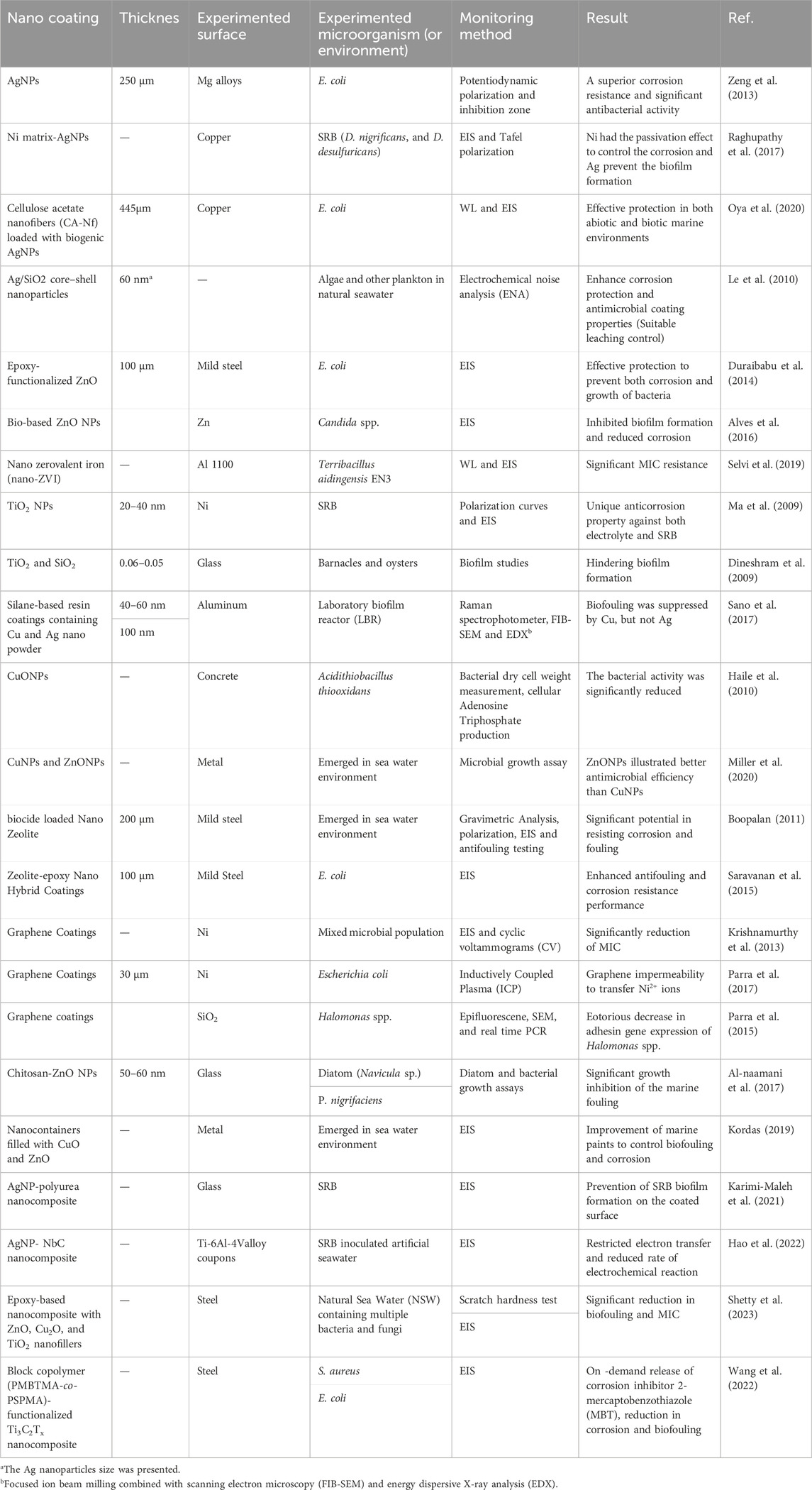
Table 3. Some major studies that applied nanomaterials for coating different metals to mitigate MIC.
4.2.1 M&MONPs nanocomposite coatings
Recently, M&MONPs such as Ag, TiO2, ZnO, and Cu have found applications in the coatings due to their high efficiency to inhibit the growth of a broad range of bacteria. It has been reported that immobilizing AgNPs onto the Mg surface can protect against microbial adhesion and lead to improved corrosion resistance (Zeng et al., 2013). Raghupathy et al. (2017) embedded AgNPs into the Ni matrix, which influenced the coating microstructure and made it less susceptible to corrosion as well as biofilm formation in comparison to the pure Ni coating. Further, Hao et al. (2022) utilized an inventive coating comprising AgNPs and NbC nanocrystals embedded in an amorphous carbon (a-C) matrix to mitigate MIC in a marine environment. The incorporation of Ag into the NbC coating played a crucial role in influencing the grain size, crystallographic texture of the NbC phase, bonding state of carbon species, and surface morphology of the coating. Subsequent immersion in SRB-inoculated artificial seawater revealed a significantly lower planktonic SRB cell count for the AgNPs/NbC nanocomposite coating (103 cells/mL) compared to both the Ag-free NbC coating (106 cells/mL) and bare Ti-6Al-4V (106 cells/mL), with differences of two and three orders of magnitude, respectively (Figures 4A–E). The electrochemical corrosion behavior of the AgNPs/NbC nanocomposite coating was assessed in SRB-inoculated system, where comparisons were made with the Ag-free NbC coating and bare Ti-6Al-4V using Tafel polarization and EIS. According to the Tafel polarization result, the current density diminished in the presence of AgNPs/NbC nanocomposite coating (2.50 × 10–7) compared to the other two treatments (Figures 4F, G). The results indicated that the introduction of Ag enhanced the MIC resistance of the NbC coating, causing a transformation in the electronic structure of the oxide film from an n-type semiconductor to a p–n heterojunction structure. Figure 4H illustrates the mechanism suggested for AgNPs/NbC nanocomposite coating to mitigate MIC (Hao et al., 2022).
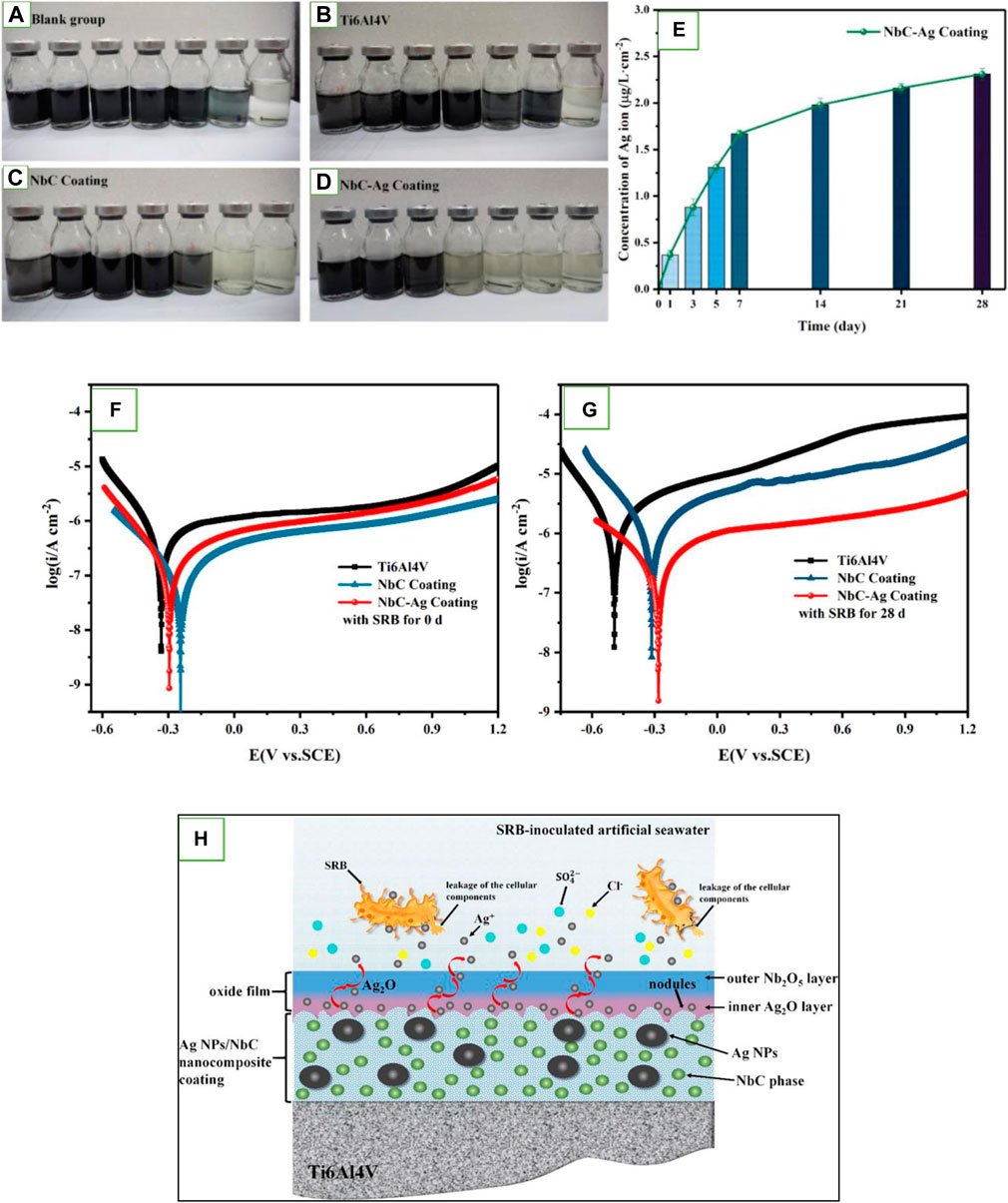
Figure 4. Synthetic culture media utilized to quantify the bacterial growth rate of different treatments (A) blank, (B) bare Ti-6Al-4V, (C) NbC coating, and (D) AgNPs/NbC nanocomposite coating. (E) Concentration of Ag+ ions released from the AgNPs/NbC nanocomposite coating following immersion in artificial seawater at 30°C for different times. (F, G) Tafel polarization curves of the tested coupons before (F) and after 28 days (G) of exposure in SRB-inoculated artificial seawater at 30°C. (H) Schematic representation illustrating the potential antibacterial mechanisms and the electronic structure of the oxide film developed on the AgNPs/NbC nanocomposite coating (Hao et al., 2022).
4.2.2 Graphene as an antibiofouling coating
Four main corrosion protection mechanisms offered by graphene coatings include barrier properties, chemical plus fouling resistance, and antibacterial properties.
Barrier properties: Metals can be protected from corrosive agents using a graphene coating. It has been indicated that graphene coating agitates dissimilatory sulfate reduction metabolites, the formation and growth of biofilm, and corrosion effect of SRB (Berry, 2013). The molecular structure of graphene lattice establishes a geometric pore (r = 64 pm) which is smaller than ionic radii of SRB metabolites such as HS− (r = 207 pm) (Li et al., 2014). Also, graphene coating provides a tortuous diffusion path, which is not easily accessible by corrosive agents and water molecules (Hernandez et al., 2008; Viyannalage, 2013).
Chemical resistance: Sulphur compounds, organic acids, and salinity are the main corrosive agents in biological environments. Graphene coating can withstand aggressive effect of oxidizing, acidic, saline, and thermal environments, as well as SRB metabolites and fermenters. An interesting work by Sarac et al. (2022) reported the formation of Ti-Zr-Ge metallic glass nanofilms free from toxic and corrosive elements (e.g., Cu, Al and Ni) fabricated by DC magnetron sputtering. These nanofilms act as effective nanocoatings for biofouling prevention via chemical and electrochemical resistance. However, this work addressed biofouling in biomedical surfaces, not industrial cases. An interesting study by Islam et al. (2022) explored the use of pristine graphene nanolayers as the thinnest, noninvasive coating for catalyst-free methanol dehydrogenation on copper electrodes in microbial methanol fuel cells. These graphene coatings helped in addressing material degradation and copper toxicity, while retaining the intrinsic work function behavior of the underlying Cu surfaces toward methanol conversion into DC electricity. Such techniques can expand the prevalence of metal electrodes in a number of bioelectrochemically driven batteries and supercapacitors. Another insightful study by Chilkoor et al. (2020a) dealt with the application of maleic-anhydride-functionalized-graphene (MAG) nanofillers in order to achieve higher corrosion resistance of polymer coatings on mild steel under abiotic and aggressive biotic conditions. In this study, graphite was mechanochemically exfoliated into graphene particles with the edges functionalized with maleic anhydride. Electrochemical tests, TEM, and nanoindentation experiments suggested that the carbonyl and hydroxyl groups in the MAG adducts facilitate their dispersion in epoxy matrix, overcoming dispersion challenges, which is common with unfunctionalized graphene platelets. The MAG nanofillers enhance the tensile strength of epoxy coatings; the imide groups in MAGE offer excellent barrier properties against intercalating corrosive species into epoxy coating.
Fouling resistance: Fouling refers to the accumulation of living and non-living organisms on a solid surface. The interaction of foulants with the solid surface contributes to bacterial attachment, biofilm formation, and microbial corrosion. Problems related to fouling are usually diminished by improving the wettability of the surface in the case of metal. The wettability of a metal surface is controlled by its surface free energy and geometrical structure, which shows the adhesion properties of the metal surface. Graphene coating imparts hydrophobic properties to metal, which repels the microorganism’s attachment and biofilm formation. Yu et al. (2023) reports the formation of a graphene nanofluid prepared by dispersing modified graphene in a common lubricant. Porous surfaces on Q235 carbon steel have been generated and then infused with the graphene nanofluid. The coating has high stability, reduced oil-phase leaching, and a self-healing function. Further, the slippery coating exhibited remarkable anti-corrosion performance and improved anti-biofouling properties. The developed graphene nanofluid coating presents great potential for applications in the protection of surfaces exposed to the marine environment.
Antibacterial properties: Various studies have investigated the antibacterial properties of graphene coatings and proposed different theories for the death of bacterial cells due to the contact with graphene surface. Pham et al. (2015) stated that the orientation angle of graphene sheets and density of graphene edges affect the bactericidal efficiency. There have been plenty of research to explore the antibacterial properties of graphene-based materials. However, a few studies have investigated the microbial corrosion resistance of graphene coating against corrosion causing bacteria. Krishnamurthy et al. (2015) reported the utilization of an ultra-thin graphene skin as a highly effective anti-MIC coating, surpassing two commercially available polymeric coatings, Parylene-C (PA), and Polyurethane (PU). That study revealed that the dissolution of Nickel (Ni) in a corrosion cell with graphene-coated Ni is an order of magnitude lower than that observed with PA and PU-coated electrodes. Electrochemical analysis demonstrated a remarkable ∼10 and ∼100 fold enhancement in MIC resistance with the graphene skin coating compared to PU and PA coatings, respectively. Unlike conventional polymer coatings that are non-conformal during deposition or prone to degradation under microbial processes, the electrochemically inert graphene coating exhibited resistance to microbial attacks, being exceptionally conformal and defect-free. Also, they found that while as-grown graphene films lack major defects, the wet transfer process introduces large-scale defects, making it less suitable for the current application. Chilkoor et al. (2020a) examined the protection efficiency of graphene atomic layers to metals against MIC-relevant SRB. It was observed that whereas single-layer graphene (SLG) promotes the SRB attack by five times compared to the bare Cu metal, multilayered graphene (MLG) on Cu reduced the attack by a factor of 10 and a factor of 1.4 respectively compared to SLG-Cu or bare Cu. Combining experimental and computational results, the effect of vacancy defects and grain boundaries on the shielding capacity of graphene atomic layers was rationalized.
Antibacterial coatings can inhibit bacteria in several ways. The coatings can be passive or active based on whether antibacterial agents are locally delivered. The passive antibacterial coatings prevent the adhesion of bacteria to the surface or kill them upon contact. The chemical and physical properties of the coating, such as wettability, surface roughness, and conductivity presented potent influences on the bacterial behavior (Vasilev, 2019). Passive coatings do not develop bacterial resistance but have limited antibacterial efficiency, which even differs for various bacterial strains. Active coatings can release antibacterial agents to the surrounding microorganisms (Li and Aik Khor, 2019).
4.2.3 Hexagonal boron nitride (hBN) nanocoating
Recently, hexagonal boron nitride (hBN)- based nanomaterials have gained attention as effective coating thanks to their exceptional stability, and unique properties relevant to adhesion, impermeability, etc. Chilkoor et al. (2018) reported the ability of single-layered hBN to work as the thinnest insulating barrier against microbially induced corrosion. 2D hBN nanocoating successfully combats the corroding effects of the aggressive metabolites generated by the sulfate reduction process. The MIC resistance results from the combined effects of its impermeability helping in creating an effective barrier for electron acceptors, redox species, and biogenic chemicals, as well as insulating features that suppress cathodic reduction and galvanic effects. Another compelling study (Chilkoor et al., 2020b) from the same research group unfolds how atomic hBN layers lower copper corrosion by a minimum of 12 times under different biotic or abiotic sulfur environments. More number of atomic layers did not ensure an enhanced performance of hBN coatings against abiotic sulfur corrosion. Nevertheless, the differences in the number of atomic layers of hBN influenced nanoscale surface properties, causing the overexpression of adhesion genes responsible for cell attachment, biofilm growth, and biogenic sulfide attack. The nanoscale defects including grain boundaries and point defects influenced the corrosion protection performance of atomic hBN layers in both biotic and abiotic environments.
4.3 Ecotoxicological and adverse effects of nanomaterials
Outstandingly, even the Romans unknowingly used metal-nanoparticles to color windowpanes with remarkable color effects (British Museum). The potential nanoparticles could provide was first mentioned in 1959 by the physicist Richard P. Feynman (Nayfeh, 2018). However, the term nanotechnology was introduced years later, by the Japanese professor Norio Taniguchi (1974) (Bayda et al., 2019). The first visualization methods also took almost 10 more years (Binnig and Rohrer, 1983). Although nanoparticles seem to have been used for a long time, the impact they can have on humans and nature was not of interest for a long time and should not be underestimated, especially nowadays. Nanoparticles have been used in medicine, cosmetics, and the food industry for decades. Surprisingly, in the EU there has been a labeling obligation for products of the cosmetics or food industry containing nanoparticles only since 2013 or respectively since 2014 (Böhmert et al., 2017). Currently, nanoparticles are increasingly developed, show high reactivity, biocidal effects, etc., and used, for example, to protect materials from their degradation. Especially, anti-microbial nanoparticles should be used with great caution, as their far-reaching consequences cannot yet be assessed at present. Although nanoparticles can bring a great advantage and show high potential when used, for example, in various coatings, the long-term and complex interactions with environmental aspects are, on the one hand, not yet fully understood and, on the other, difficult to investigate. Nanoparticles can enter the environment through a variety of pathways and, in some cases, at high levels. As an example, how much nanoparticles are present in the environment and how difficult it can be to determine their amount and the particles themselves is summarized in the review of (Nguyen et al., 2020). One of the best-known sources for the contamination and the uncontrolled released can be observed in the wastewater sector. Although certain precautions (e.g., special filters) are taken to avoid the uncontrolled release of, for example, nanoparticles, studies have shown that these approaches only minimize but do not prevent the exposure. In addition, recent studies also show partially synergistic, additive, or antagonistic effects when certain nanoparticles occur in combination in the environment. In addition to the promising possibilities that the use of nanoparticles can deliver, more consideration should be given to far-reaching consequences. What is problematic about the studies conducted so far is that they are very one-sided. For example, only a certain class of nanoparticles or potential host organisms is investigated, whereas (fatally) the uptake in plants seems to be rather secondary. Also, potentially emerging degradation products and their altered reactivity, as well as the interactions of different nanoparticles/degradation products with each other and thus on the environment are insufficiently investigated. The use of nanoparticles, especially of novel extremely reactive or biocide-active ones, should be done with great caution and care (Schwinn and Völker, 2020; Zhang et al., 2022). One of the major challenges in determining the impact of nanoparticles in the environment is that incredibly sensitive measurement methods are required to detect and identify the minimal traces in the environment and judge how this might impact the environment but also how environmental factors can influence the stability of nanoparticles. Those issues have already been described in several studies (Tortella et al., 2020). “For example, some methods only detect very pure materials at high concentrations. In real environmental samples, however, mixtures of several different particle types are to be expected, which are typically present in very low concentrations within the range of a few nanograms per liter. This corresponds to a “pea”, or even less, in an Olympic swimming pool” (Upadhyayula et al., 2017). Although the concentrations found in the environment are (currently and depending on the location) low, they have a major impact on the “beneficial” ecosystem. Also, this has been discussed in the latest studies. Strategies should therefore be developed to identify and minimize this influence, for example, through better filtering options. To avoid that novel, more effective nanoparticles might further impact the environment, for each nanoparticle already during the development-process, effective removal strategies should also be developed before the product can be used in real applications (Buchman et al., 2019).
Upadhyayula et al. (2017) reported a life cycle assessment (LCA) of anticorrosive graphene poly (ether imide) coatings on unalloyed steel. They investigated global warming potential, acidification potential, carcinogenic potential against human health, fossil fuel depletion potential, etc. It was observed that such impacts are sensitive to the service life and maintenance of the coating and further studies are required to fully comprehend the long-term environmental impact of such coatings. Another thorough investigation on the LCA of anticorrosive graphene oxide-epoxy (GOE) liners in biodiesel storage steel tanks (Chilkoor et al., 2017) revealed great refined environmental performance for the GOE liner compared to the incumbent system, where the GOE liner showed 91% reduced impacts in ozone depletion potential, 59% reduction in respiratory effect potential, 62% reduction in acidification potential, 67%–69% reduction in global warming and carcinogenic potential, 72%–76% reduction in eutrophication, smog forming and fossil fuel depletion potential, etc. Saadi et al. (2022) developed nanocomposites by dispersing asphaltene-derived flash graphene (AFG, formed by flash joule heating process) into a polymer effectively to achieve upgraded mechanical, thermal, and corrosion-resistant properties compared to the bare polymer. The life cycle and technoeconomic analysis demonstrated that the flash joule heating process leads to reduced environmental impact compared to the traditional processing of asphaltene and lower production cost compared to other flash joule heating precursors. Bheemasetti et al. (2023) indicated effective upcycling of municipal solid waste as precursors for graphene-based nanomaterial synthesis which might lead to the further viable nano-manufacturing techniques for upcycling and recycling of low-value wastes into high-value functional nanomaterials. An extremely insightful read from Upadhyayula et al. (2018) elucidates how sustainability framework comprises of four principal steps: 1) screening level LCA on the outcome of a game changing innovation (e.g., a novel upcycled product such as wood-derived biofuel, etc.), 2) comparison of the screening LCA result with a commercially available product(s) that could be replaced by the newly fabricated product; 3) LCA results to be fitted into methodologies to estimate the financial performance of the target fabricated product; and 4) LCA results to develop parameters that allude at sustainability aspects of a fabricated product from a multidisciplinary point of view. A similar approach could be suggested for MIC-resistant nanocoatings or nano-biocides in order to effectively bridge the gap between fundamental state-of-the-art MIC mitigation research and industry-relevant strategies.
5 Conclusion
Use of nanomaterials has the potential to yield more effective and less toxic compounds compared to conventional biocides. They can also facilitate the development of more efficient antifouling coatings for controlling MIC. Nanomaterials can be designed to target specific microorganisms, exhibit higher microbiocidal effects at lower concentrations, be synthesized using environmentally friendly methods, etc. Additionally, designing multifunctional nanobiocides or coatings is feasible. However, for these designed nanobiocides and coatings to be used in practice, several steps should be addressed. They need to demonstrate efficacy in controlling MIC, be producible on an industrial scale, be compatible with existing systems, and comply with environmental regulations for official commercial use in the industry. Throughout these steps, various considerations should be taken into account, which it is summarized in three different steps.
5.1 Design
The nanobiocides and coatings should be specifically designed to address the MIC mitigation. MIC mitigation is not merely limited tp killing planktonic bacteria. However, in the literature, many studies tend to focus more on the biocidal effects of various nanomaterials against MIC related microorganisms, particularly SRB. These studies often overlook factors beyond killing planktonic bacteria in their design considerations; factors such as targeting MIC microorganisms, biofilm disruption ability, changing the electrochemistry in the biofilm/metal surface, etc. For example, in nanobiocide design, EMIC relies on sessile cells, while MMIC is driven by corrosive metabolites. Thus, eliminating electroactive microbes in the bottom layer of biofilms (where microorganisms have direct access to the metal surface) is essential for addressing EMIC, whereas removing corrosive metabolites is key for managing MMIC. However, distinguishing between EMIC and MMIC is challenging, especially in mixed-culture biofilms. MMIC and EMIC may occur simultaneously on corroding metal surfaces, but their rates and relative contributions to the overall corrosion process can differ. Additionally, the type of metal can influence whether a microbe causes EMIC or MMIC, which should be considered in the design step (Enning and Garrelfs, 2014).
Another example is bifunctional nanomaterials which can function simultaneously as a biocide and a corrosion inhibitor owing to their unique properties. The key factors to consider during design and fabrication of bifunctional nanomaterials for MIC mitigation include the adhesion properties and topology of the target surfaces; thermal and mechanical resistance and hydrophobicity of the nanomaterial and the biocidal efficiency against several MIC relevant microorganisms; and most importantly, toxicological and environmental leaching possibilities. In a more practical context, these types of bifunctional nanomaterials should also be designed in a user-friendly manner (like a spray or deposition coating), have low maintenance cost, be cost effective as well as hydrodynamically stable enough to maintain high performance over long periods of time, especially when deployed against marine biofouling or even in industry (Romeu and Mergulhão, 2023).
One suggestion for improving the design process is to leverage artificial intelligence (AI). AI can assist in designing nanobiocides and coatings by analyzing vast amounts of data from previous studies to identify optimal characteristics and configurations for targeting MIC. AI can use studies that show important factors, which can affect MIC and subsequently the efficiency of nanomaterial. Additionally, computational simulation studies can be highly beneficial in assessing the properties of different nanomaterials before they are produced in the laboratory. These simulations can provide insights into how nanomaterials interact with microorganisms and surfaces, helping researchers make informed decisions about which materials to pursue for further experimentation (Taghavi Kalajahi et al., 2023). By integrating AI and computational simulations into the design process, researchers can potentially reduce the cost of laboratory work, increase the likelihood of designing effective nanobiocides and coatings, and shorten the overall design and testing timeline. This approach holds great promise for advancing the development of tailored solutions to combat MIC effectively.
5.2 Laboratory work
The results of corrosion rate assessments in laboratory experiments for biocide efficiency (even the conventional biocides) often differ from real-world applications, typically requiring higher concentrations in industrial settings. One issue is the possibility of microbial resistance developing over time with frequent exposure to biocides, which can significantly increase costs. Additionally, excessive biocide usage can have adverse environmental impacts. The complexity of real-world conditions significantly contributes to this difference. Therefore, it is crucial to account for various environmental factors and conditions during laboratory work to achieve results (corrosion rates) that align more closely with those observed in the actual environment (Knisz et al., 2023). However, in laboratory research on biocides and antifouling coatings, there exists a significant gap in assessing their actual effectiveness against MIC. This also applied to investigations on the efficiency of nanobiocides and coatings as well. Many studies often overlook important factors and questions critical to understanding the effectiveness of nanomaterials. For instance, while some studies demonstrate biocidal effects using well-defined strains, it is essential to test these materials against mixed microbial cultures representative of real-world environments or industries to evaluate their efficacy in hindering complex biofilms. It should also be investigated how effective these materials are once biofilm has formed. Can they penetrate the biofilm and effectively kill microorganisms? How does their efficiency change during the time and different stage of biofilm formation? etc.
Additionally, these materials should undergo testing under various environmental conditions such as different ranges of temperature, pH, electrical conductivity (EC), flow rates, etc., to assess their efficiency across different settings and over extended exposure periods, beyond the commonly used 14-day timeframe in lab experiments. Considering the intended application in industry is also important. Different industrial settings may require tailored approaches for MIC mitigation.
Furthermore, studying corrosion involves employing diverse methods such as electrochemistry, surface analyses, biofilm characterization, etc. Techniques such as EIS allow for non-destructive monitoring of corrosion development over time. Corrosion is an electrochemical phenomenon; therefore, to understand the influence of microorganisms on corrosion, these methods are highly beneficial. Additionally, Tafel polarization method is invaluable for investigating pitting corrosion, a significant concern related to MIC. Recent studies increasingly leverage these advanced methods to gain deeper insights into the influence of microorganisms on corrosion and metal surfaces.
5.3 Real-world applications and environmental regulations
Once a nanomaterial has shown promise in laboratory testing for commercial use in industries to control MIC, it is crucial to ensure that it complies with environmental regulations before moving forward. One approach is to integrate considerations of environmental regulations into the design process, possibly through AI design tools. Studies on the toxicology of different nanoparticles can provide insights into the toxicity of nanomaterials and their behavior in the environment. Collaboration between research groups focusing on nanomaterials for MIC mitigation and those studying nanomaterial toxicology could facilitate comprehensive assessments.
Regarding real-world applications, products must first pass environmental regulations, such as those in the EU, before being tested or used in industries. This regulatory process can take up to 10 years, making it challenging for new nanomaterials to enter the market quickly. Additionally, not all scientists may be willing to navigate this process. However, if a nanomaterial has been proven effective against MIC in the laboratory and is deemed more environmentally friendly, it may be possible to conduct tests in semi-real-world conditions. Collaboration with consultant companies or industries willing to provide support can facilitate this process. Overall, while there are challenges in transitioning from laboratory to real-world applications, collaborations and careful consideration of environmental regulations can help bring promising nanomaterials to market.
In summary, until a nanobiocide or coating has been thoroughly studied and proven effective under lab conditions, it cannot progress to environmental assessments, industrial testing, and usage. While the high cost and energy demand of nanomaterial production on an industrial scale are significant considerations (which should be considered in the design phase), if the benefits of nanomaterials outweigh these costs compared to conventional methods, there is potential for economic feasibility and mitigation of environmental pollution expenses. Currently, nanomaterials are already under use in the oil and gas sector for different applications such as drilling and hydraulic fracturing fluids, corrosion inhibitors, etc., suggesting it is possible to scale up the usage of nanomaterials. Therefore, addressing deeper questions and aspects of nanomaterial usage to mitigate MIC, as mentioned earlier, in laboratory investigations can be a significant step toward advancing them as a more effective and environmentally friendly solution for MIC.
Author contributions
ST: Writing–original draft. AM: Writing–review and editing. AK: Writing–review and editing.
Funding
The authors declare that no financial support was received for the research, authorship, and/or publication of this article.
Conflict of interest
The authors declare that the research was conducted in the absence of any commercial or financial relationships that could be construed as a potential conflict of interest.
Publisher’s note
All claims expressed in this article are solely those of the authors and do not necessarily represent those of their affiliated organizations, or those of the publisher, the editors and the reviewers. Any product that may be evaluated in this article, or claim that may be made by its manufacturer, is not guaranteed or endorsed by the publisher.
References
Abd El-Salam, H. M., Azzam, E. M. S., and Aboad, R. S. (2018). Synthesis and characterization of poly (2-aminothiophenol-co-2-methylaniline)/silver nanoparticles as antisulfate-reducing bacteria. Int. J. Polym. Mater. Polym. Biomaterials 67 (8), 501–508. doi:10.1080/00914037.2017.1354196
Alasvand, Z. V. R. (2016). Inhibition of a sulfate reducing bacterium, Desulfovibrio marinisediminis GSR3, by biosynthesized copper oxide nanoparticles. 3 Biotech. 6, 1–7. doi:10.1007/s13205-016-0403-0
Al-jumaili, A., Alancherry, S., Bazaka, K., and V Jacob, M. (2017). Review on the antimicrobial properties of carbon nanostructures. Materials 10, 1066–1126. doi:10.3390/ma10091066
Al-naamani, S. D., Dutta, J., and Burgess, J. G. (2017). Chitosan-zinc oxide nanocomposite coatings for the prevention of marine biofouling. Chemosphere 168, 408–417. doi:10.1016/j.chemosphere.2016.10.033
Alves, M. M., Cunha, D. V., Santos, C. F., Mira, N. P., and Montemor, M. F. (2016). In vitro corrosion behaviour and anti-Candida spp. activity of Zn coated with ZnO-nanostructured ‘Anastacia’flowers. J. Mater. Chem. B 4 (27), 4754–4761. doi:10.1039/C6TB00797J
Azzam, E. M. S., Sami, R. M., and Kandile, N. G. (2012). Activity inhibition of sulfate reducing bacteria using some cationic thiol surfactants and their nanostructures. Am. J. Biochem. 2 (3), 29–35. doi:10.5923/j.ajb.20120203.03
Bao, Z. D., Zhang, S., Ji, Z., and Zhang, H. (2019). Next-generation composite coating system: nanocoating. Front. Mat. 6, 1–6. doi:10.3389/fmats.2019.00072
Bayda, S., Adeel, M., Tuccinardi, T., Cordani, M., and Rizzolio, F. (2019). The history of nanoscience and nanotechnology: from chemical–physical applications to nanomedicine. Molecules 25 (1), 112. doi:10.3390/molecules25010112
Bento, I. B. B., Gaylarde, C. C., Englert, G. E., and Muller, I. L. (2005). Degradation and corrosive activities of fungi in a diesel – mild steel – aqueous system. World J. Microbiol. Biotechnol. 21, 135–142. doi:10.1007/s11274-004-3042-2
Bera, M., Gupta, P., and Maji, P. K. (2018). Facile one-pot synthesis of graphene oxide by sonication assisted mechanochemical approach and its surface Chemistry. J. Nanosci. Nanotechnol. 18, 902–912. doi:10.1166/jnn.2018.14306
Berkl, Z., Fekete-Kertész, I., Buda, K., Vaszita, E., Fenyvesi, É., Szente, L., et al. (2022). Effect of cyclodextrins on the biofilm formation capacity of Pseudomonas aeruginosa PAO1. Molecules 27 (11), 3603. doi:10.3390/molecules27113603
Berry, (2013). Impermeability of graphene and its applications. Carbon N. Y. 62, 1–10. doi:10.1016/j.carbon.2013.05.052
Bhattacharya, P., and Neogi, S. (2019). Antibacterial properties of doped nanoparticles. Rev. Chem. Eng. 35 (7), 861–876. doi:10.1515/revce-2017-0116
Bheemasetti, S., Upadhyayula, V. K., and Gadhamshetty, V. (2023). “Upcycling the solid wastes as precursors for graphene production,” in Graphene extraction from waste (Sawston, United Kingdom: Woodhead Publishing), 1–21.
Binkauskien, D.Bu, and Lugauskas, A. (2017). Long-time corrosion of metals and profiles of fungi on their surface in outdoor environments in Lithuania. Berlin, Germany: Springer, 105–117.
Binnig, G., and Rohrer, H. (1983). Scanning tunneling microscopy. Surf. Sci. 126 (1-3), 236–244. doi:10.1016/0039-6028(83)90716-1
Böhmert, L., Laux, P., Luch, A., Braeuning, A., and Lampen, A. (2017). Nanomaterials in food – toxicological properties and risk assessment. Fed. Health Gazette Health Res. Health Prot. 7, 722–727. doi:10.1007/s00103-017-2559-0
Boopalan, A. S. (2011). Studies on biocide free and biocide loaded zeolite hybrid polymer coatings on zinc phosphated mild steel for the protection of ships hulls from biofouling and corrosion. Silicon 250, 207–214. doi:10.1007/s12633-011-9100-0
British Museum The British museum drinking-cup. London, United Kingdom: British Museum. Available at: https://www.britishmuseum.org/collection/object/H_1958-1202-1 (accessed on December 20, 2022).
Buchman, J. T., Hudson-Smith, N. V., Landy, K. M., and Haynes, C. L. (2019). Understanding nanoparticle toxicity mechanisms to inform redesign strategies to reduce environmental impact. Accounts Chem. Res. 52 (6), 1632–1642. doi:10.1021/acs.accounts.9b00053
Busi, S., and Rajkumari, J. (2019). “Microbially synthesized nanoparticles as next generation antimicrobials: scope and applications,” in Nanoparticles in pharmacotherapy (Norwich, NY, USA: William Andrew Publishing), 485–524.
Chilingar, G. V., Mourhatch, R., and Al-Qahtani, G. D. (2013). The fundamentals of corrosion and scaling for petroleum & environmental engineers. Amsterdam, Netherlands: Elsevier.
Chilkoor, G., Jawaharraj, K., Vemuri, B., Kutana, A., Tripathi, M., Kota, D., et al. (2020b). Hexagonal boron nitride for sulfur corrosion inhibition. ACS Nano 14 (11), 14809–14819. doi:10.1021/acsnano.0c03625
Chilkoor, G., Karanam, S. P., Star, S., Shrestha, N., Sani, R. K., Upadhyayula, V. K., et al. (2018). Hexagonal boron nitride: the thinnest insulating barrier to microbial corrosion. ACS Nano 12 (3), 2242–2252. doi:10.1021/acsnano.7b06211
Chilkoor, G., Shrestha, N., Kutana, A., Tripathi, M., Robles Hernández, F. C., Yakobson, B. I., et al. (2020a). Atomic layers of graphene for microbial corrosion prevention. ACS Nano 15 (1), 447–454. doi:10.1021/acsnano.0c03987
Chilkoor, G., Upadhyayula, V. K., Gadhamshetty, V., Koratkar, N., and Tysklind, M. (2017). Sustainability of renewable fuel infrastructure: a screening LCA case study of anticorrosive graphene oxide epoxy liners in steel tanks for the storage of biodiesel and its blends. Environ. Sci. Process. Impacts 19 (2), 141–153. doi:10.1039/c6em00552g
Deng, X., Dohmae, N., Kaksonen, A. H., and Okamoto, A. (2020). Biogenic iron sulfide nanoparticles to enable extracellular electron uptake in sulfate-reducing bacteria. Angew. Chem. 132 (15), 6051–6055. doi:10.1002/ange.201915196
Dineshram, R. S., Somaraju, K. R. C., Jayaraj, K., Vedaprakash, L., Ratnam, K., V Joshi, S., et al. (2009). Biofouling studies on nanoparticle-based metal oxide coatings on glass coupons exposed to marine environment. Colloids Surfaces B Biointerfaces 74, 75–83. doi:10.1016/j.colsurfb.2009.06.028
Dinh, H. T., Kuever, J., Mußmann, M., Hassel, A. W., Stratmann, M., and Widdel, F. (2004). Iron corrosion by novel anaerobic microorganisms. Nature 427 (6977), 829–832. doi:10.1038/nature02321
Do, J., and Lin, K. (2016). Kinetics of urease inhibition-based amperometric biosensors for mercury and lead ions detection. J. Taiwan Inst. Chem. Eng. 63, 25–32. doi:10.1016/j.jtice.2016.03.011
Duraibabu, T. G., Manjumeena, R., Ananda, S., and Dasan, P. (2014). Unique coating formulation for corrosion and microbial prevention of mild steel. Prog. Org. Coatings. 77, 657–664. doi:10.1016/j.porgcoat.2013.12.002
Ejileugha, C., Ezealisiji, K. M., Ezejiofor, A. N., and Orisakwe, O. E. (2021). Microbiologically influenced corrosion: uncovering mechanisms and discovering inhibitor—metal and metal oxide nanoparticles as promising biocorrosion inhibitors. J. Bio-and Tribo-Corrosion 7 (3), 109. doi:10.1007/s40735-021-00545-0
Enning, D., and Garrelfs, J. (2014). Corrosion of iron by sulfate-reducing bacteria: new views of an old problem. Appl. Environ. Microbiol. 80 (4), 1226–1236. doi:10.1128/aem.02848-13
Enning, D., Venzlaff, H., Garrelfs, J., Dinh, H. T., Meyer, V., Mayrhofer, K., et al. (2012). Marine sulfate-reducing bacteria cause serious corrosion of iron under electroconductive biogenic mineral crust. Environ. Microbiol. 14 (7), 1772–1787. doi:10.1111/j.1462-2920.2012.02778.x
Fakoya, M. F., and Shah, S. N. (2017). Emergence of nanotechnology in the oil and gas industry: emphasis on the application of silica nanoparticles. Petroleum 3 (4), 391–405. doi:10.1016/j.petlm.2017.03.001
Farag, A. (2020). Applications of nanomaterials in corrosion protection coatings and inhibitors. J. Corros. Rev. 38, 67–86. doi:10.1515/corrrev-2019-0011
Farrag, H. A., Abdelsalam, S. M., Keiralla, Z. M. H., Raafat, A. I., and Araby, E. (2019). A powerful nanocomposite polymer prepared from metal oxide nanoparticles synthesized via Brown algae as anti-corrosion and anti-biofilm. Front. Mat. 6, 1–17. doi:10.3389/fmats.2019.00140
Ferreira, O., Rijo, P., Gomes, J. F., Santos, R., Monteiro, S., Vilas-Boas, C., et al. (2019). Biofouling inhibition with grafted Econea biocide: toward a nonreleasing eco-friendly multiresistant antifouling coating. ACS Sustain. Chem. Eng. 8 (1), 12–17. doi:10.1021/acssuschemeng.9b04550
Gu, T., and Galicia, B. (2012). “Can acid producing bacteria be responsible for very fast MIC pitting?,” in Nace corrosion (Pennsylvania, United States: NACE).
Gu, T., Wang, D., Lekbach, Y., and Xu, D. (2021). Extracellular electron transfer in microbial biocorrosion. Curr. Opin. Electrochem. 29, 100763. doi:10.1016/j.coelec.2021.100763
Gutner-hoch, R. M.Id, Oliveira, T., Maia, F., Soares, A. M. V. M., Id, S. L., Piller, C., et al. (2018). Antimacrofouling efficacy of innovative inorganic nanomaterials loaded with booster biocides. J. Mar. Sci. Eng. 6, 1–12. doi:10.3390/jmse6010006
Haile, G. N., Allouche, E., and Vaidya, S. (2010). Evaluation of the bactericidal characteristics of nano-copper oxide or functionalized zeolite coating for bio-corrosion control in concrete sewer pipes. Corros. Sci. 52, 45–53. doi:10.1016/j.corsci.2009.08.046
Hao, J., Xu, J., Peng, S., Jiang, S., Xie, Z., and Munroe, P. R. (2022). Dissecting anticorrosion and antimicrobial potency of an Ag nanoparticle/NbC nanocomposite coating in a marine environment containing sulfate-reducing bacteria. ACS ES&T Eng. 2 (8), 1386–1402. doi:10.1021/acsestengg.1c00485
Haruna, T. A. S., and Haruna, K. (2018). Atomistic simulation: a unique and powerful computational tool for corrosion inhibition research. Arab. J. Sci. Eng. 44, 1–32. doi:10.1007/s13369-018-3605-4
Hayat, S., Muzammil, S., Shabana, N., Aslam, B., Siddique, M. H., Saqalein, M., et al. (2019). Quorum quenching: role of nanoparticles as signal jammers in Gram-negative bacteria. Future Microbiol. 14 (1), 61–72. doi:10.2217/fmb-2018-0257
He, J., Tan, Y., Liu, H., Jin, Z., Zhang, Y., He, F., et al. (2022). Extracellular polymeric substances secreted by marine fungus Aspergillus terreus: full characterization and detailed effects on aluminum alloy corrosion. Corros. Sci. 209, 110703. doi:10.1016/j.corsci.2022.110703
Hernandez, Y., Nocolosi, V., Lotya, M., Blighe, F. M., Sun, Z., De, S., et al. (2008). High yield production of graphene by liquid phase exfoliation of graphite. Nat. Nanotechnol. 3, 563–568. doi:10.1038/nnano.2008.215
Idrees, S. B., Kalsoom, T., Raina, S., Sharif, M. A., and Yasmeen, S. (2018). Biosynthesis of silver nanoparticles using Sida acuta extract for antimicrobial actions and corrosion inhibition potential. Environ. Technol. 40, 1–8. doi:10.1080/09593330.2018.1435738
Iino, T., Ito, K., Wakai, S., Tsurumaru, H., Ohkuma, M., and Harayama, S. (2015). Iron corrosion induced by nonhydrogenotrophic nitrate-reducing Prolixibacter sp. strain MIC1-1. Appl. Environ. Microbiol. 81 (5), 1839–1846. doi:10.1128/aem.03741-14
Islam, J., Obulisamy, P. K., Upadhyayula, V. K., Dalton, A. B., Ajayan, P. M., Rahman, M. M., et al. (2022). Graphene as thinnest coating on copper electrodes in microbial methanol fuel cells. ACS Nano 17 (1), 137–145. doi:10.1021/acsnano.2c05512
Islam, M. S., Takeda, Y., Oda, Y., Ikeda, H., Yamamoto, O., Milova, G., et al. (2016). Methane emissions from the 2015 aliso canyon blowout in Los Angeles, CA. Science 351, 1317–1320. doi:10.1126/science.aaf2348
Ituen, E. E., Yuanhua, L., Li, R., and Singh, A. (2020). Mitigation of microbial biodeterioration and acid corrosion of pipework steel using Citrus reticulata peels extract mediated copper nanoparticles composite. Int. Biodeterior. Biodegr. 149, 104935. doi:10.1016/j.ibiod.2020.104935
Kalajahi, S. T., Rasekh, B., Yazdian, F., Neshati, J., and Taghavi, L. (2020b). Green mitigation of microbial corrosion by copper nanoparticles doped carbon quantum dots nanohybrid. Environ. Sci. Pollut. Res. 27, 40537–40551. doi:10.1007/s11356-020-10043-4
Kalajahi, S. T., Rasekh, B., Yazdian, F., Neshati, J., and Taghavi, L. (2020a). Graphene oxide/silver nanostructure as a green anti - biofouling composite toward controlling the microbial corrosion. Int. J. Environ. Sci. Technol. 18, 195–210. doi:10.1007/s13762-020-02846-2
Karimi-Maleh, H., Mousavi, S. J., Mahdavian, M., Khaleghi, M., Bordbar, S., Yola, M. L., et al. (2021). Effects of silver nanoparticles added into polyurea coating on sulfate-reducing bacteria activity and electrochemical properties; an environmental nano-biotechnology investigation. Environ. Res. 198, 111251. doi:10.1016/j.envres.2021.111251
Kato, S. (2016). Microbial extracellular electron transfer and its relevance to iron corrosion. Microb. Biotechnol. 9 (2), 141–148. doi:10.1111/1751-7915.12340
Kato, S., Yumoto, I., and Kamagata, Y. (2015). Isolation of acetogenic bacteria that induce biocorrosion by utilizing metallic iron as the sole electron donor. Appl. Environ. Microbiol. 81 (1), 67–73. doi:10.1128/aem.02767-14
Khan, A. A. P., Khan, A., Rahman, M. M., Asiri, A. M., and Oves, M. (2016). Lead sensors development and antimicrobial activities based on graphene oxide/carbon nanotube/poly (O-toluidine) nanocomposite. Int. J. Biol. Macromol. 89, 198–205. doi:10.1016/j.ijbiomac.2016.04.064
Knisz, J., Eckert, R., Gieg, L. M., Koerdt, A., Lee, J. S., Silva, E. R., et al. (2023). Microbiologically influenced corrosion—more than just microorganisms. FEMS Microbiol. Rev. 47 (5), fuad041. doi:10.1093/femsre/fuad041
Kordas, G. (2019). Nanotechnology to improve the biofouling and corrosion performance of marine paints: from lab experiments to real tests in sea. Int. J. Phys. Res. Appl. 2, 033–037. doi:10.29328/journal.ijpra.1001012
Krishnamurthy, A., Gadhamshetty, V., Mukherjee, R., Natarajan, B., Eksik, O., Ali Shojaee, S., et al. (2015). Superiority of graphene over polymer coatings for prevention of microbially induced corrosion. Sci. Rep. 5 (1), 13858. doi:10.1038/srep13858
Krishnamurthy, A., Gadhamshetty, V., Mukherjee, R., Chen, Z., Ren, W., Cheng, H. -M., et al. (2013). Passivation of microbial corrosion using a graphene coating. Carbon N. Y. 56, 45–49. doi:10.1016/j.carbon.2012.12.060
Kruger, J. (2011). Cost of metallic corrosion. Uhlig's Corros. Handb. 3, 15–20. doi:10.1002/9780470872864.ch2
Larsen, K. R., and Lundgaard, T. (2010). “Consortia of MIC bacteria and archaea causing pitting corrosion in top side oil production facilities,” in CORROSION 2010, San Antonio, Texas, March 2010, 1–12.
Larsenon, K. R. (2020). “Corrosion risks and mitigation strategies for offshore wind turbine foundations,” in Offshore Engineering Society and The Welding Institute, London, April, 2020.
Le, P. H., Wang, J., and Chen, J. (2010). Controlled release active antimicrobial corrosion coatings with Ag/SiO 2 core – shell nanoparticles. Mat. Chem. Phys. 120, 351–355. doi:10.1016/j.matchemphys.2009.11.020
Leheta, H. (2023). “Cathodic protection for fixed steel offshore structures,” in Maritime industry, ocean engineering and coastal resources (Boca Raton, Florida, United States: Taylor & Francis).
Leung, H. W. (2001). Ecotoxicology of glutaraldehyde: review of environmental fate and effects studies. Ecotoxicol. Environ. Saf. 49 (1), 26–39. doi:10.1006/eesa.2000.2031
Lewandowski, Z., and Beyenal, H. (2008). Mechanisms of microbially influenced corrosion. Berlin, Germany: Springer.
Lewandowski, Z., and Beyenal, H. (2013). Fundamentals of biofilm research. Boca Raton, Florida, United States: CRC Press.
Li, J., Wang, G., Zhu, H., Zhang, M., Zheng, X., Di, Z., et al. (2014). Antibacterial activity of large-area monolayer graphene film manipulated by charge transfer. Sci. Rep. 4, 4359. doi:10.1038/srep04359
Li, K. A. K., and Aik Khor, K. (2019). Preparation and properties of coatings and thin films on metal implants. Encycl. Biomed. Eng., 203–212. doi:10.1016/B978-0-12-801238-3.11025-6
Li, Y., Yang, L., Liao, Y., Zhao, R., Ji, L., Su, R., et al. (2023). Photothermal heating-assisted superior antibacterial and antibiofilm activity of high-entropy-alloy nanoparticles. Adv. Funct. Mater. 33, 2302712. doi:10.1002/adfm.202302712
Li, Y. H. (2006). Chitosan bicomponent nanofibers and nanoporous fibers. Carbohydr. Res. 341, 374–381. doi:10.1016/j.carres.2005.11.028
Little, B. J., Hinks, J., and Blackwood, D. J. (2020b). Microbially influenced corrosion: towards an interdisciplinary perspective on mechanisms. Int. Biodeterior. Biodegrad. 154, 105062. doi:10.1016/j.ibiod.2020.105062
Little, D. J. B., Hinks, J., Lauro, F. M., Marsili, E., Okamoto, A., Rice, S. A., et al. (2020a). Microbially influenced corrosion — any progress ? Corros. Sci. 170, 108641. doi:10.1016/j.corsci.2020.108641
Lou, Y., Chang, W., Cui, T., Wang, J., Qian, H., Ma, L., et al. (2021). Microbiologically influenced corrosion inhibition mechanisms in corrosion protection: a review. Bioelectrochemistry 141, 107883. doi:10.1016/j.bioelechem.2021.107883
Lyon, D. Y., and Alvarez, P. J. (2008). Fullerene water suspension (nC60) exerts antibacterial effects via ROS-independent protein oxidation. Environ. Sci. Technol. 42 (21), 8127–8132. doi:10.1021/es801869m
Ma, Y. S., Di, J., Yao, Z., and Liu, H. (2009). Effect of TiO 2 nanoparticles on anticorrosion property in amorphous Ni-P-Cr composite coating in artificial seawater and microbial environment. Polym. (Basel) 13, 274–279. doi:10.1002/maco.200805066
Mand, J., Longwell, J., and Enning, D. (2019). “Observations on the effect of simulated pigging and corrosion inhibitor exposure on microbiologically influenced corrosion of carbon steel,” in Nace corrosion (Pennsylvania, United States: NACE).
Mand, J., Park, H. S., Jack, T. R., and Voordouw, G. (2014). The role of acetogens in microbially influenced corrosion of steel. Front. Microbiol. 5, 268. doi:10.3389/fmicb.2014.00268
Mekuye, B., and Abera, B. (2023). Nanomaterials: an overview of synthesis, classification, characterization, and applications. NanoSelect 4, 486–501. doi:10.1002/nano.202300038
Michalak, I., and Chojnacka, K. (2014). Biocides. Encycl. Toxicol. Third Ed. 1, 461–463. doi:10.1016/B978-0-12-386454-3.00472-3
Miller, R., Adeleye, A. S., Henry, M., Kui, L., Lenihan, H. S., and Keller, A. A. (2020). Nano and traditional copper and zinc antifouling coatings: metal release and impact on marine sessile invertebrate communities. J. Nanoparticle Res. 22, 129. doi:10.1007/s11051-020-04875-x
Mori, K., Tsurumaru, H., and Harayama, S. (2010). Iron corrosion activity of anaerobic hydrogen-consuming microorganisms isolated from oil facilities. J. Biosci. Bioeng. 110 (4), 426–430. doi:10.1016/j.jbiosc.2010.04.012
Morsi, R. E., Labena, A., and Khamis, E. A. (2016). Core/shell (ZnO/polyacrylamide) nanocomposite: in-situ emulsion polymerization, corrosion inhibition, anti-microbial and anti-biofilm characteristics. J. Taiwan Inst. Chem. Eng. 63, 512–522. doi:10.1016/j.jtice.2016.03.037
Narenkumar, J., Parthipan, P., Madhavan, J., Murugan, K., Marpu, S. B., Suresh, A. K., et al. (2018). Bioengineered silver nanoparticles as potent anti-corrosive inhibitor for mild steel in cooling towers. Environ. Sci. Pollut. Res. 25, 5412–5420. doi:10.1007/s11356-017-0768-6
Nayfeh, M. H. (2018). Fundamentals and applications of nano silicon in plasmonics and fullerines: current and future trends. Amsterdam, Netherlands: Elsevier Science. doi:10.1016/B978-0-323-48057-4.00001-3
Nguyen, M. K., Moon, J. Y., and Lee, Y. C. (2020). Microalgal ecotoxicity of nanoparticles: an updated review. Ecotoxicol. Environ. Saf. 201, 110781. doi:10.1016/j.ecoenv.2020.110781
Okeniyi, A. P., Popoola, I., Ojewumi, M. E., Okeniyi, E. T., and Ikotun, J. O. (2018). Tectona grandis capped silver-nanoparticle material effects on microbial strains inducing microbiologically influenced corrosion. Int. J. Chem. Eng. 2018, 1–6. doi:10.1155/2018/7161537
Okeniyi, O., John, G. S., Owoeye, T. F., Okeniyi, E. T., Akinlabu, D. K., Taiwo, O. S., et al. (2017). Effects of dialium guineense based zinc nanoparticle material on the inhibition of microbes inducing microbiologically in fl uenced corrosion. Berlin, Germany: Springer, 21–31. doi:10.1007/978-3-319-52132-9
Okorie, I. E., and Nwokorie, R. C. (2021). A review of fungal influenced corrosion of metals. Zaštita Mater. 62 (4), 333–339. doi:10.5937/zasmat2104333o
Omran, B., and Abdel-salam, M. O. (2020). Advances in material research and Technology A new era for microbial corrosion mitigation using nanotechnology biocorrosion and nanotechnology. Berlin, Germany: Springer.
Omran, B. A., Nassar, H. N., Younis, S. A., Fatthallah, N. A., Hamdy, A., El-Shatoury, E. H., et al. (2019). Physiochemical properties of Trichoderma longibrachiatum DSMZ 16517-synthesized silver nanoparticles for the mitigation of halotolerant sulphate-reducing bacteria. J. Appl. Microbiol. 126, 138–154. doi:10.1111/jam.14102
Ouyang, Y., Cai, X., Shi, Q., Liu, L., Wan, D., Tan, S., et al. (2013). Poly-l-lysine-modified reduced graphene oxide stabilizes the copper nanoparticles with higher water-solubility and long-term additively antibacterial activity. Colloids surfaces B Biointerfaces 107, 107–114. doi:10.1016/j.colsurfb.2013.01.073
Oya, S. K., Deniz, F., and Nazır, H. (2020). Anti microbial corrosion properties of electrospun cellulose acetate nano fi bers containing biogenic silver nanoparticles for copper coatings †. RSC Adv. 10, 39901–39908. doi:10.1039/d0ra07641d
Parra, C., Montero-silva, F., Gentil, D., Campo, V., Henrique, T., Henríquez, R., et al. (2017). The many faces of graphene as protection barrier. Performance under microbial corrosion and Ni allergy conditions, Mater. (Basel) 10, 1406–1415. doi:10.3390/ma10121406
Parra, C., Dorta, F., Jimenez, E., Henríquez, R., Ramírez, C., Rojas, R., et al. (2015). A nanomolecular approach to decrease adhesion of biofouling - producing bacteria to graphene - coated material. J. Nanobiotechnology. 13, 82. doi:10.1186/s12951-015-0137-x
Pham, V. T., Truong, V. K., Quinn, M. D., Notley, S. M., Guo, Y., Baulin, V. A., et al. (2015). Graphene induces formation of pores that kill spherical and rod-shaped bacteria. ACS Nano 9, 8458–8467. doi:10.1021/acsnano.5b03368
Raghupathy, , Natarajan, K. A., and Srivastava, C. (2017). PT SC. Amsterdam, Netherlands: Elsevier B.V. doi:10.1016/j.surfcoat.2017.08.068
Rao, T. S., Panigrahi, S., and Velraj, P. (2022). “Transport and disposal of radioactive wastes in nuclear industry,” in Microbial biodegradation and bioremediation (Amsterdam, Netherlands: Elsevier), 419–440.
Rasheed, A., Jabbar, K. A., Mackey, H. R., and Mahmoud, K. A. (2019a). Recent advancements of nanomaterials as coatings and biocides for the inhibition of sulfate reducing bacteria induced corrosion. Curr. Opin. Chem. Eng. 25, 35–42. doi:10.1016/j.coche.2019.06.003
Rasheed, P. A., Jabbar, K. A., Rasool, K., Pandey, R. P., Sliem, M. H., Helal, M., et al. (2019b). Controlling the biocorrosion of sulfate-reducing bacteria (SRB) on carbon steel using ZnO/chitosan nanocomposite as an eco-friendly biocide. Corros. Sci. 148, 397–406. doi:10.1016/j.corsci.2018.12.028
Rasheed, P. A., Jabbar, K. A., Rasool, K., Pandey, R. P., Sliem, M. H., Helal, M., et al. (2019c). Controlling the biocorrosion of sulfate-reducing bacteria (SRB) on carbon steel using ZnO/chitosan nanocomposite as an eco-friendly biocide. Corros. Sci. 148, 397–406. doi:10.1016/j.corsci.2018.12.028
Rasheed, P. A., Pandey, R. P., Jabbar, K. A., Samara, A., Abdullah, A. M., and Mahmoud, K. A. (2020a). Chitosan/lignosulfonate nanospheres as “green” biocide for controlling the microbiologically influenced corrosion of carbon steel. Materials 13 (11), 2484. doi:10.3390/ma13112484
Rasheed, , Pandey, R. P., Jabbar, K. A., Samara, A., Abdullah, A. M., and Mahmoud, K. A. (2020b). Biocide for controlling the microbiologically influenced corrosion of carbon steel.
Raya, D., Militello, K., Gadhamshetty, V., and Dhiman, S. (2023). “Nanowire Formation in sulfate-reducing bacteria under stress conditions,” in Microbial stress response: mechanisms and data science (Washington, D.C, USA: American Chemical Society), 59–73.
Romero, De, Ocando, L., De Rincón, O., Parra, J., Ruiz, R., and Bracho, M. (2021). “Cathodic polarization effect on sessile SRB growth and iron protection,” in CORROSION 2021, San Diego, California, March 2021, 1–7.
Romeu, M. J., and Mergulhão, F. (2023). Development of antifouling strategies for marine applications. Microorganisms 11 (6), 1568. doi:10.3390/microorganisms11061568
Saadi, M. A. S. R., Advincula, P. A., Thakur, M. S. H., Khater, A. Z., Saad, S., Shayesteh Zeraati, A., et al. (2022). Sustainable valorization of asphaltenes via flash joule heating. Sci. Adv. 8 (46), eadd3555. doi:10.1126/sciadv.add3555
Saji, (2012). The impact of nanotechnology on reducing corrosion cost. Sawston, United Kingdom: Woodhead Publishing Limited. doi:10.1533/9780857095800.1.3
San Keskin, N. O., Yaylaci, E., Durgun, S. G., Deniz, F., and Nazır, H. (2021). Anticorrosive properties of green silver nanoparticles to prevent microbiologically influenced corrosion on copper in the marine environment. J. Mar. Sci. Appl. 20, 10–20. doi:10.1007/s11804-020-00188-6
Sano, H. K., Hirai, N., Ogawa, A., Kougo, T., and Tanaka, T. (2017). The development of the anti-biofouling coating agent using metal nanoparticles and analysis by Raman spectroscopy and FIB system. Surf. Coat. Technol. 325, 715–721. doi:10.1016/j.surfcoat.2017.04.015
Sarac, B., Micusik, M., Putz, B., Wurster, S., Sharifikolouei, E., Xi, L., et al. (2022). Magnetron sputtered non-toxic and precious element-free Ti- Zr- Ge metallic glass nanofilms with enhanced biocorrosion resistance. Adv. Mater. Interfaces 9 (26), 2201223. doi:10.1002/admi.202201223
Saravanan, S. A., Kumar, S. A., and Duraibabu, D. (2015). Studies on biocide encapsulated zeolite-epoxy nano hybrid coatings on mild steel. Curr. Bionanotechnol. 1, 37–50. doi:10.2174/2213529401666150304234007
Schwinn, K., and Völker, D. (2020). Nanomaterials in the environment: current state of science and regulations on chemical safety. Dessau-Roßlau: German Environment Agency.
Selim, M. S., Fatthallah, N. A., Higazy, S. A., Chen, X., and Hao, Z. (2023). Novel blade-like structure of reduced graphene oxide/α-Mn2O3 nanocomposite as an antimicrobial active agent against aerobic and anaerobic bacteria. Mater. Chem. Phys. 298, 127436. doi:10.1016/j.matchemphys.2023.127436
Selvi, A. A., Narenkumar, J., and Arul, A. (2019). Effect of nano - zerovalent iron incorporated polyvinyl - alginate hybrid hydrogel matrix on inhibition of corrosive bacteria in a cooling tower water environment. SN Appl. Sci. 1, 1–9. doi:10.1007/s42452-019-0443-2
Sharma, M., An, D., Liu, T., Pinnock, T., Cheng, F., and Voordouw, G. (2017). Biocide-mediated corrosion of coiled tubing. PLoS One 12 (7), e0181934. doi:10.1371/journal.pone.0181934
Shetty, P., Arya, S. B., and Kodialbail, V. S. (2023). Biocorrosion behavior of epoxy-based multilayer nanocomposite coatings. J. Bio- Tribo-Corrosion 9 (3), 45. doi:10.1007/s40735-023-00763-8
Singh, A., and Yuanhua, L. (2020). Synthesis of bio-based nickel nanoparticles composite, characterization and corrosion in hibition in simulated oilfield microbial and acidizing environments. J. Adhes. Sci. Technol., 1–20. doi:10.1080/01694243.2020.1785992
Singh, M., Thapa, M., Dutta, M., Mukherjee, A., and Ghosh, C. K. (2019). Size-dependent antibacterial activity of copper nanoparticles against Xanthomonas oryzae pv. oryzae – a synthetic and mechanistic approach, Colloid Interface Sci. Commun. 32, 100190. doi:10.1016/j.colcom.2019.100190
Skiba, M., Kurmakova, I., Bondar, O., Demchenko, N., and Vorobyova, V. (2020). The production of silver nanoparticles and their effect on sulfate reducing bacteria under steel microbial corrosion. Chem. Chem. Technol. 14, 70–75. doi:10.23939/chcht14.01.070
Skovhus, D. E., and Lee, J. S. (2017). Microbiologically influenced corrosion in the upstream oil and gas industry. Boca Raton, Florida, United States: CRC Press.
Sujatha, P. J., Arumugam, N., Prakash, A., Abilaji, S., Prakash, C., Rajasekar, A., et al. (2019). Myco-synthesis of zinc oxide nanoparticles as potent anti-corrosion of copper in cooling towers. J. Clust. Sci. 0, 1583–1590. doi:10.1007/s10876-019-01600-0
Swaminathan, N. K. S. (2019). Antimicrobial activity of the engineered nanoparticles used as coating agents. Berlin, Germany: Springer, 549–563.
Taghavi Kalajahi, S., Rezazadeh Mofradnia, S., Yazdian, F., Rasekh, B., Neshati, J., and Taghavi, L. (2023). Computational evaluation of multifunctional nanostructured inhibitors to control microbiologically influenced corrosion: DFT calculations and MD simulations. J. Appl. Electrochem. 53, 2359–2373. doi:10.1007/s10800-023-01934-8
Tamisier, M., Schmidt, M., Vogt, C., Kümmel, S., Stryhanyuk, H., Musat, N., et al. (2022). Iron corrosion by methanogenic archaea characterized by stable isotope effects and crust mineralogy. Environ. Microbiol. 24 (2), 583–595. doi:10.1111/1462-2920.15658
Thakur, A., Kaya, S., and Kumar, A. (2023). Recent trends in the characterization and application progress of nano-modified coatings in corrosion mitigation of metals and alloys. Appl. Sci. 13 (2), 730. doi:10.3390/app13020730
Tortella, G. R., Rubilar, O., Durán, N., Diez, M. C., Martínez, M., Parada, J., et al. (2020). Silver nanoparticles: toxicity in model organisms as an overview of its hazard for human health and the environment. J. Hazard. Mater. 390, 121974. doi:10.1016/j.jhazmat.2019.121974
Uchiyama, T., Ito, K., Mori, K., Tsurumaru, H., and Harayama, S. (2010). Iron-corroding methanogen isolated from a crude-oil storage tank. Appl. Environ. Microbiol. 76 (6), 1783–1788. doi:10.1128/aem.00668-09
Ueki, T., and Lovley, D. R. (2022). Desulfovibrio vulgaris as a model microbe for the study of corrosion under sulfate-reducing conditions. mLife 1 (1), 13–20. doi:10.1002/mlf2.12018
Upadhyayula, V. K., Gadhamshetty, V., Shanmugam, K., Souihi, N., and Tysklind, M. (2018). Advancing game changing academic research concepts to commercialization: a Life Cycle Assessment (LCA) based sustainability framework for making informed decisions in Technology Valley of Death (TVD). Resour. Conservation Recycl. 133, 404–416. doi:10.1016/j.resconrec.2017.12.029
Upadhyayula, V. K., Meyer, D. E., Gadhamshetty, V., and Koratkar, N. (2017). Screening-level life cycle assessment of graphene-poly (ether imide) coatings protecting unalloyed steel from severe atmospheric corrosion. ACS Sustain. Chem. Eng. 5 (3), 2656–2667. doi:10.1021/acssuschemeng.6b03005
Vasilev, K. (2019). Nanoengineered antibacterial coatings and materials: a perspective. Coatings 9, 654. doi:10.3390/coatings9100654
Venzlaff, H., Enning, D., Srinivasan, J., Mayrhofer, K. J., Hassel, A. W., Widdel, F., et al. (2013). Accelerated cathodic reaction in microbial corrosion of iron due to direct electron uptake by sulfate-reducing bacteria. Corros. Sci. 66, 88–96. doi:10.1016/j.corsci.2012.09.006
Viyannalage, (2013). Graphene nanocomposite coatings for protecting lowalloy steels from corrosion. Ohio, United States: American Ceramic Society Bulletin.
Wang, D., Wang, Y., Wu, H., Li, Z., Wu, Y., Liu, B., et al. (2024). Eco-friendly bifunctional antibacterial and anticorrosive broad-spectrum rosin thiourea iminazole quaternary ammonium salt against microbiologically influenced corrosion. Corros. Sci. 229, 111847. doi:10.1016/j.corsci.2024.111847
Wang, D., Zhou, E., Xu, D., and Lovley, D. R. (2023). Burning question: are there sustainable strategies to prevent microbial metal corrosion? Microb. Biotechnol. 16 (11), 2026–2035. doi:10.1111/1751-7915.14347
Wang, P., He, B., Du, Y., Wang, B., Gao, J., Liu, S., et al. (2022). Functionalized Ti3C2Tx-based nanocomposite coatings for anticorrosion and antifouling applications. Chem. Eng. J. 448, 137668. doi:10.1016/j.cej.2022.137668
Woodard, T. L., Ueki, T., and Lovley, D. R. (2023). H2 is a major intermediate in Desulfovibrio vulgaris corrosion of iron. Mbio 14 (2), e0007623–23. doi:10.1128/mbio.00076-23
Xu, D., Gu, T., and Lovley, D. R. (2023). Microbially mediated metal corrosion. Nat. Rev. Microbiol. 21, 705–718. doi:10.1038/s41579-023-00920-3
Xu, D., Li, Y., Song, F., and Gu, T. (2013). Laboratory investigation of microbiologically influenced corrosion of C1018 carbon steel by nitrate reducing bacterium Bacillus licheniformis. Corros. Sci. 77, 385–390. doi:10.1016/j.corsci.2013.07.044
Yu, H., Song, S. K., Xu, Z. Y., Ge, Y., Geng, W., and Yang, X. Y. (2023). A stable graphene nanofluid for creating slippery, corrosion-and biofouling-resistant surfaces. J. Phys. Chem. Solids 182, 111611. doi:10.1016/j.jpcs.2023.111611
Yuk, S., Azam, A. H., Miyanaga, K., and Tanji, Y. (2020). The contribution of nitrate-reducing bacterium Marinobacter YB03 to biological souring and microbiologically influenced corrosion of carbon steel. Biochem. Eng. J. 156, 107520. doi:10.1016/j.bej.2020.107520
Zeng, R., Liu, L., Li, S., Zou, Y., Zhang, F., Yang, Y., et al. (2013). Self-assembled silane film and silver nanoparticles coating on magnesium alloys for corrosion resistance and antibacterial applications. Acta Metall. Sin. Engl. Lett. 26, 681–686. doi:10.1007/S40195-013-0397-0
Zhang, F., Wang, Z., Peijnenburg, W. J., and Vijver, M. G. (2022). Review and prospects on the ecotoxicity of mixtures of nanoparticles and hybrid nanomaterials. Environ. Sci. Technol. 56 (22), 15238–15250. doi:10.1021/acs.est.2c03333
Zhu, Q., Chua, M. H., Ong, P. J., Lee, J. J. C., Chin, K. L. O., Wang, S., et al. (2022). Recent advances in nanotechnology-based functional coatings for the built environment. Mater. Today Adv. 15, 100270. doi:10.1016/j.mtadv.2022.100270
Ziadi, I., Alves, M. M., Taryba, M., El-Bassi, L., Hassairi, H., Bousselmi, L., et al. (2020). Microbiologically influenced corrosion mechanism of 304L stainless steel in treated urban wastewater and protective effect of silane-TiO2 coating. Bioelectrochemistry 132, 107413. doi:10.1016/j.bioelechem.2019.107413
Keywords: microbiologically influenced corrosion (MIC), nano biocide, nano coating, biofilm, biofouling
Citation: Kalajahi ST, Misra A and Koerdt A (2024) Nanotechnology to mitigate microbiologically influenced corrosion (MIC). Front. Nanotechnol. 6:1340352. doi: 10.3389/fnano.2024.1340352
Received: 17 November 2023; Accepted: 02 April 2024;
Published: 24 April 2024.
Edited by:
Satoshi Wakai, Japan Agency for Marine-Earth Science and Technology (JAMSTEC), JapanReviewed by:
Sharanabasava V. Ganachari, KLE Technological University, IndiaDi Wang, Northeastern University, China
Venkataramana Gadhamshetty, South Dakota School of Mines and Technology, United States
Copyright © 2024 Kalajahi, Misra and Koerdt. This is an open-access article distributed under the terms of the Creative Commons Attribution License (CC BY). The use, distribution or reproduction in other forums is permitted, provided the original author(s) and the copyright owner(s) are credited and that the original publication in this journal is cited, in accordance with accepted academic practice. No use, distribution or reproduction is permitted which does not comply with these terms.
*Correspondence: Sara Taghavi Kalajahi, c2FyYS50YWdoYXZpLWthbGFqYWhpQGJhbS5kZQ==