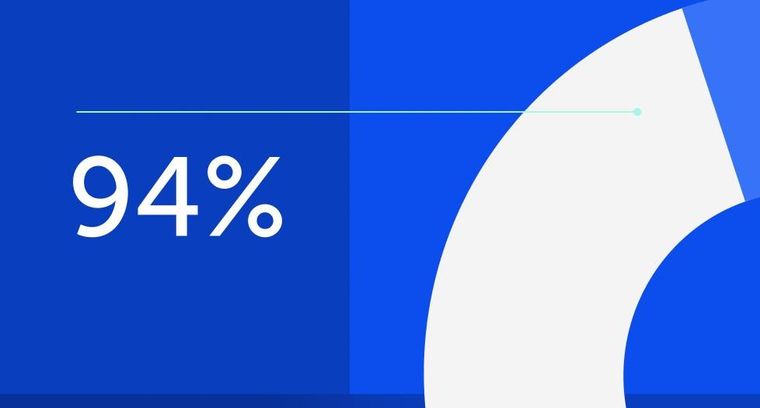
94% of researchers rate our articles as excellent or good
Learn more about the work of our research integrity team to safeguard the quality of each article we publish.
Find out more
REVIEW article
Front. Nanotechnol., 27 March 2024
Sec. Nanomaterials
Volume 6 - 2024 | https://doi.org/10.3389/fnano.2024.1310165
This article is part of the Research TopicOpportunities and Challenges for Nanotechnology in Sustainable Agri-Food ProductionView all 5 articles
The increase in global population has had a tremendous impact on sustainable agri-food practices. With the growth in world population, various modern technologies are being utilized that more often result in the opening of tremendous opportunities in the agriculture and food sectors. Nanotechnology is used in agri-food sectors for a variety of purposes, including enhancing flavor, pest/pathogen diagnosis, production, processing, storage, packaging, and transportation of agricultural products. Plant pathogenic microorganisms including bacteria, viruses, fungi, and nematodes have a significant impact on the global economy. In particular, advances in nanotechnology, including nanobiosensors, have been used in the detection of plant diseases and pathogens, the evaluation and examination of infections caused by microorganisms, the management of diseases and, thus, the promotion of food security. Apart from the management of plant diseases, nanobiosensors offer better opportunities for sustainable agri-food production by controlling physical, chemical, and biological processes, thus improving food safety and the agricultural economy. This review outlines the application of nano-integrated nanobiosensors for better agricultural and food practices.
The world’s economic strength largely depends upon the agricultural and food sectors. The global demand for food production is expected to double by 2050. It is thus important to note that the majority of crop losses are due to biotic and abiotic stresses. It is thought that 20%–40% of crop losses are due to biotic factors including pest attack, pathogen invasion, herbicides, and insect attack (Dutta et al., 2022; Munir et al., 2023). Therefore, a major challenge is the management of plant diseases against biotic factors. Previously, conventional methods have managed to control pathogens such as bacteria, viruses, and fungi, including spectroscopy, chromatography, enzymatic methods, proteomics, and metabolomics (Schieber, 2018). However, these conventional methods have detrimental effects on plant ecosystems, human health, and the environment (Tanwar and Sushil, 2019; Sarkar et al., 2022). Nanotechnology has recently been applied as a wide range of technical processes, including the characterization and synthesis of nanoparticles and the fabrication and regulation of new materials with wide applications in pharmaceuticals, industry, agriculture, and medicine (Ansari, 2023). Nanobiosensors are composed of nanocomposites of organic, inorganic, or combined materials, synthesized using novel nanotechnological approaches (Romanovskii and Periakaruppan, 2023). Nanobiosensors involve various nanomaterials such as metals, carbon nanotubes (CNTs), magnetic nanoparticles, nanowires, nano-probes, and quantum dots (QDs) (Beeguma and Dasb, 2022). Applications of nanobiosensors in agriculture include the real-time monitoring of crop conditions with the detection of soil moisture content, nutrients, pH, and temperature using piezoelectric nanosensors (Dimri et al., 2020). The wide acceptance of nanocomposites can replace the use of pesticides, fungicides, and synthetic chemicals in agricultural crops to inhibit plant pests. Small-scale nanocomposites overcome biological barriers to ensure the efficient transfer of nutrients through foliar or root applications (Izaz et al., 2023). Recently, Li et al. (2023) reported the use of nanocomposites with horseradish peroxidase to detect mycotoxin AFB and evaluate the quality of agri-food products. Moreover, nanoparticles can ameliorate plant genetic health and make plants tolerant to drought, heat, and salt stress which might be due to the height-surface-to-volume ratio of nanoparticles that increases their reactivity and possible biochemical activity (Khan and Rizvi, 2014; El Moneim et al., 2021). Nanomaterials are useful for researchers and scientists because of their ability as carriers, and their small size, ease of transport, and active surface area (Hazarika et al., 2021; Dutta et al., 2022). Ligand-assisted nanoclusters can be used to detect contaminants in agricultural products and offer a range of applications that leverage their unique properties. Ligand-protected nanoclusters can serve as carriers for pesticide and fertilizer delivery, plant growth promotion, soil remediation, detection of pathogens in crops, detection of environmental parameters, photocatalysis of water to generate hydrogen for a strong irrigation system, and can enhance stress tolerance in plants (Aparna et al., 2022).
Microbial contamination is a major cause of waterborne and foodborne diseases and crop failure in the environment. The only way to prevent these diseases is the highly accurate rapid detection of bacterial contamination at an early stage. Given the limitations of traditional methods in terms of time-consuming procedures, complex instrumentation, and laborious sample preparation by trained personnel, researchers are adopting sensing technology to explore nanomaterials for the detection of microbial infection. Nanocomposites offer great benefits in terms of flexible morphology, high surface area, and enhanced optical, electrical, thermal, and mechanical properties. Foodborne pathogenic microorganisms have long been monitored using nanomaterial-based optical biosensors, including fluorescence, colorimetric, surface-enhanced Raman scattering (SERS), and surface plasmon resonance (SPR) sensors. Nanomaterials offer a significant enhancement in the optical abilities of sensors. Integrated optical sensing technologies can support functions by multiplexed detection, higher accuracy and sensitivity, and the availability of signal readout and electrical control options on a single chip, making them suitable for point-of-care (POC) detection (Kumari et al., 2023). As demand for food production increases, nanoparticles such as gold, silver, and copper play a critical role in mitigating new challenges in disease management, fertilizer production, and antimicrobial action. Recent studies have shown promising results for nanoparticles such as B, Cu, Mn, Si, and Zn that appear to function as nanofertilizers in host defense mechanism (Elmer et al., 2018). Recently, Abdelhamied et al. (2023) reported the development of a novel bacteriophage-based nanobiosensor for the quantitative detection of Escherichia coli O157:H7 in food samples via nanocomposites consisting of gold nanoparticles and multiwalled CNTs and tungsten oxide nanostructures.
Recent advances in the field of nanotechnology include nanoparticle-integrated biosensors, also known as nanosensors. Being small, easily portable, efficient, specific, and sensitive makes them indispensable for the agri-food sector. Researchers in clinical diagnostics and research institutions can utilize nanoparticle-based nanobiosensors for smaller delivery systems, plant protection, promotion of better oil health, increased fruit shelf life, and sustainable agriculture (Dar et al., 2020). The availability of nanobiosensor-detection systems allows agriculturists to identify plant pathogens at an early stage, thus avoiding significant economic losses (Figure 1).
The present review discusses types of nanobiosensors and the application of nanoparticles for pest management, pesticides, and toxin detection in detail, along with advancements in nanobiosensors in the agricultural and food sectors and their future prospects.
There is a wide variety of nanomaterials that are used for nanobiosensors. Nanobiosensors are classified based on the kind of material under analysis and how the signal is transmitted. There is a growing need for sensor devices with features such as enhanced sensitivity, cost-effectiveness, rapid response, robustness, carrier recovery, a small footprint, in situ analysis, and ease of operation (Aghaei et al., 2018; Bezzon et al., 2019). Nanostructured materials are used in the construction of nanosensor devices such as nanoscale wires (high detection sensitivity), CNTs (high surface area and high electron conductance), and nano-sized thin films, polymers, and biomaterials (Kokate et al., 2013; Ayesh, 2016; Abdel-Karim et al., 2020).
Due to their physical and chemical characteristics, metal nanoparticles (mNPs) are highly suitable for the development of new and advanced sensing devices, such as electrochemical sensors (ESS) and biosensors (BAS) (Jin et al., 2017). mNPs can act as analytical sensors in a wide range of sensing principles and signal amplifiers (Su et al., 2019). Combining high-throughput sensing principles with detection elements has led to bioassays that provide rapid reactions and visual results that are suitable for use in low-resource environments. The basic properties of mNPs have been leveraged to create colorimetric sensing arrays with a potential for fast analysis that are cost-effective and easy to implement due to their naked-eye observation capability (Sun et al., 2020). Biosensors based on predictable color changes include but are not limited to biosensors for detecting alpha-1-feto protein in human serum anti-hepatitis-B virus antibodies in human serum breast cancer biomarkers Mycobacterium tuberculosis complex, human immunodeficiency virus type-1, DNA toxic metal pollutants (Priyadarshini et al., 2017), and organochlorine endosulfan pesticide (ESP) (Arora, 2018).
Silver nanoparticles have also been incorporated into polymers to be used in sensors; Kariuki et al. (2016) incorporated silver nanoparticles into poly(amic acid) (PAA) polymer matrix nanoparticle-based sensors for nitrobenzene detection. The detection limit of a PAA-Ag nanoparticle-based sensor was 1.68 mM, with a linear range of 10–600 mM and 7.88 M A (M−1) sensitivity with a low interference against structurally related nitro-aromatic compounds. Silver nanoparticles allow the detection of different analytes by tagging them with silver nanoparticles. Sepunaru et al. (2016) used silver nanoparticles to tag influenza virus, resulting in its effective electrochemical detection because the size and frequency increased linearly as the virus concentration increased, along with the surface coverage of silver nanoparticles. The catalytic properties of platinum nanoparticles are typically well-characterized and have been incorporated into sensors or into various analytes such as hydrogen peroxide, cholesterol, mercury ions, and hydrogen sensors (Song et al., 2018; Alias et al., 2016; Jung et al., 2018).
A biomaterial exists when a single piece of equipment uses a certain biomolecule that is sensitive to certain bioreactors and biomolecules, such as analytes, to create high-quality analytical data. In this case, the sensors are either physical or chemical, and they use recognition elements to detect biological products (Huang et al., 2021). Organic coatings associate along analytics, For example, transformations in the organic coatings associated with the analytics can be easily detectable with the help of these sensors. The sensors and recognition elements can be used to classify the biosensor types. Biosensors based on transducers are electromagnetic, impedimetric, pulsemetric, calorimetric quartz crystal account wave, piezoelectric, reflection absorption transmission, fluorescence surface, and plasmon waveguide. Depending on the recognition element, biosensors may be aptamer-based, enzyme-based, nucleic acid based, oligonucleotide-based, or immunosensor-based (antigen–antibody reciprocation or advanced biomarker sensors). With whole cell-based organelles, there are some paper-based biosensors that are quite versatile, such as microfluidic biosensors, LFA bioassays, and dipstick tests. These sensors look for biomarkers using optical detection, but they can also detect them through electrochemical, chemical, or electro-chemiluminescence discernment (Campuzano et al., 2011) (Table 1).
The development of biosensors using nanoparticles has the potential to overcome the limitations of traditional methods. Some advantages of polymer particle-based biological detectors over traditional methods include high sensitivity and specificity, rapid and efficient response time, and real-time output (Chakroborty et al., 2023). In addition, the methods can detect very small levels of analytes that could pose a risk to vegetation and domestic animals, in addition to transportable equipment. Since the nanoparticles can be used in multiple combinations, they can be used in multiplexing appliance and amperometric biosensors. Biosensing research is currently focused on when biological probes are used in combination with nanoparticles such as metallurgic, coefficient dot, and captivating nanoparticles, and graphite oxide nanoparticles, and coal nanotubes (Ahmed et al., 2022). Fullerenes, titanium nanoparticles, and silicon oxide nanoparticles are sometimes used in this area. However, polymerases such as polyacrylic acids, zeolites, and chitosans can also be used for encapsulation (Malhotra and Ali, 2018). Nanobiosensors can be made from a variety of mNPs, usually gold and silver. Among the noble metals, well-known anion nanoparticles (AuNPs) occur widely, followed by cinnabar nanoparticles (CuNPs) and captivating nanoparticles (Fe3O4). Hybrid nanomaterials commonly used in studies include multiwall carbon nanoparticles (MNCs)/gold nanoparticles (AuNPs), number dots, nano-sized magnetic beads, cadmium tetrachloride QDs, and magnetic nanoparticles. observe confirmable agriculture practices within terms about nourishment security and capricious application regarding high microbicides have being highly efficient. Nanobiosensors can detect pollutants at nanomolar to picomolar levels, including heavy metals, pesticides, endocrine disruptors, dioxin, biological oxygen demand and microbial pathogens (Verma and Rani, 2021).
Carbon and zinc oxide nanomaterials are multifunctional nanomaterials used in the agricultural industry with high automatic optics together with synthetic properties, short virulence, and good biocompatibility (Table 2). Nanomaterials with good metal properties, such as gold and platinum nanomaterials, are widely used and have unique sensing properties (Yu et al., 2021). Nano-selenium has been utilized in biosensors, and nanoparticle composites such as chitosan/gold nanoparticles and graphene nanosheets have considerable potential in agriculture (Bao et al., 2015).
Graphene has a biocompatibility profile that allows for the chemical functionalization of graphene into metal composites; graphene-based biosensors have these unique characteristics, making them excellent candidates for biosensors (Verma et al., 2022). Some applications of nanofabrication include loamy standard sensing, vegetation pathogen detection, growth regulators, pesticide detection, and substantial metal detection (Ali et al., 2021). The development of sensitive nanobiosensors necessitates better understanding of the biological interface and use of optimal bio-conjugation approaches (Verma, 2017).
New cutting-edge technologies are needed to sustain sustainable agriculture, including nano-based sensors. These provide cost-effective detection with higher sensitivity in the agro-food sector. Incorporating nanoparticles and nanostructures into sensors can significantly improve device performance in terms of length of time toward sensor reactivity and precision and multi-photon observation, including flexibility (Butnariu and Butu, 2019).
Nanobiosensors have several uses in the creation of cutting-edge techniques for organic farming that depends on intelligent sensing and precise reactions based on the nanobionic methods. Nanobiosensors may be based on a broad response of a crop in a whole field. Nanobionics is a mathematical model for sensors that track the flow of different phytohormones and signaling molecules (Sharma et al., 2021). Due to their capacity to feel, analyze, and detect changes, nanobiosensors generally enhance precision farming that maximizes production while using fewer chemical fertilizers and pesticides; decisive farm management provides a correct account of the information gathered from field or soil conditions.
The main focus of plant pathology research is reducing the risk of catastrophic crop loss through direct or indirect control of plant diseases (Li et al., 2020). Foliar and soil application of fungicides, pesticides, and insecticides can control plant diseases. However, overexposure to these toxic chemicals can lead to ecological problems. The long-term application of these chemicals may also lead to resistant characteristics among the pathogens. For this reason, simple and fast sensing platforms or tools have been essential for plant disease management research. In modern agriculture, biosensors serve as a basis or nanomaterials can be recycled, while various fast-recognition biosensing strategies have been developed by researchers, including electrochemical sensing (ESS), immune-sensing (IOS), and apta-sensing. Recently, label free electrochemical biosensor has been developed to detect the LOD of several drugs resistant strains (PV) Lachryamanial achymania (PS1) (LOD337CFU/Ml) in assay. The biosensor is based on a 4- aminothiophenol, glutaraldehyde, and an anti-PSI antibody-modified gold electrode.
Plant pathogens play a major role in declining crop productivity which could lead to food shortages for humans and animals alike. Food production is projected to grow 35%–50% between 2012 and 2050 to meet the needs of the world’s population—approximately 10 billion people by 2050 (nutriment and husbandry concern toward the limited sovereign state, FAO 2019). Approximately 16% of the global harvest damage is attributed to plant microbes (Ficke et al., 2018). The Irish Potato Famine (1845–1849), was caused by the late blight infection of potatoes (Goss et al., 2014) caused by Phytophthora fungus infestation. Other pathogens include Acidovorax sp., Pseudomonas syringae, Ralstonia solanacearum, and Leptosphaeria maculans. The scientific field of phytopathology searches for pathogens. Early diagnosis of infections was mostly based on their visual manifestation, which often required several days (Dyussembayev et al., 2021). However, the use of cutting-edge biosensing techniques such as nanosensors allows infestations to be managed earlier, resulting in fewer production losses. The development of plant treatment of infections can benefit from their accurate identification (Ali et al., 2021). “Nano-diagnostics” is another term for these cutting-edge diagnostic techniques. Pathogens that cause contamination have been found using nanobiosensors. Nanobiosensor-based gold nanoparticles have been used to diagnose agro-terrorism chemicals, and gold-based nanoparticle sensors have been created to detect plant diseases (Acharya and Pal, 2020).
Detection procedures are contingent upon the oscillatory generation of DNA segments (deciding the disease-causing agent) along RPA (recombinant DNA misrepresentation) at electrodeposition analysis. Another colorimetric detection for P. syringae has been proposed by Vaseghi et al. (2013) involving the use of thiol-linking DNA gold nano-probes. Colorimetric detection is based on the color red to violet during the operation which indicates the worthwhile reaction. A similar colorimetric detection method was developed for the soil bacterium Ralstonia solanacearum (the pathogen causing potato wilt disease); no prior DNA amplification was necessary in this assay, the microbe’s existence was identified via a direct route amongst the topsoil representative (Ha et al., 2018). There are also biotic stress markers such as germination block for Arabidopsis seed, root-pathogenicity for Arabidopsis root, and sweet basil pathogenicity. Pseudomonas aeruginosa versatile pathogen is one of the most well-characterized human opportunistic pathogens. It can be easily identified by a fast, on-the-spot detection method like aptamer AuNPs to establish ingenious drift examination (Soundy and Day, 2017).
The continuous application of pesticides in agriculture leads to their contaminating the natural habitat and food chain and other associated hazards (Figure 2). Pesticides are the chemicals or biological agents which target pests, weeds, and plant pathogens (Mfarrej and Rara, 2019). Biosensing approaches with nanomaterials detect plant pathogens at an early stage and help in disease management (Thakur et al., 2022). With the aid of novel diagnostic approaches, nanobiosensors appear a more suitable analytical method than conventional methods such as standard chromatographic techniques (Figure 3). Recently, gas nanosensors were used for the in vivo detection of pheromones and associated compounds in Euschistus heros (F.) stink bug insects (Martinazzo et al., 2022). Nanoparticles based on PANI.Ag gas have been developed to detect the volatiles secreted from insects. Gas nanosensors are efficient in distinguishing the sex hormones emitted by male stink bugs. Moreover, they can be a promising alternative approach to pest management in agricultural crops. Hence, pesticide detection in agricultural crops is one of the most important factors for farmers during agri-food production. Carbaryls are the most widely used insecticides for pest control in agricultural fields. In addition to their rapid action and short environmental persistence, carbaryls can also potentially contaminate agricultural land and thus enter the food chain. These residues have short-term cumulative effects on aquatic animals such as Lymnaea acuminata (water fish), Danio rerio (zebra fish), plankton, and other fish (Sunaryani and Rosmalina, 2021). Carbaryl residues have detrimental effects on the environment as well as on human health. Amino-modified AuNPs, blue-emitting silicon quantum dots (SiQDs) and red-emitting cadmium telluride quantum dots (CdTe QDs) have been successfully developed to detect carbaryl residues. Fahimi-Kashani and Hormozi-Nezhad (2016) and Zhang et al. (2023) have detected organophosphate pesticides with citrate-capped 13-mm AuNPs, including by hierarchical cluster analysis (HCA) and linear discriminant analysis (LDA).
Organophosphates have been categorized in toxicology class I by the U.S. Environmental Protection Agency (EPA). Organophosphates are widely used to control pests but, due to their high toxicity, they can enter the food chain, and their toxicological effects can lead to irreversible phosphorylation which is later responsible for the inactivation of acetylcholinesterase (AChE) in the central and peripheral nervous system. Gold nanoparticle-based biosensors have been successfully developed to detect five toxic organophosphates (pesticides including, azinphos-methyl (AM), chlorpyrifos (CP), fenamiphos (FP), pirimiphos-methyl (PM), and phosalone (PS) at concentrations of 120–400 ng.mL−1. Various nanomaterials can be used in the fabrication of biosensors as herbicides, pesticides, and insecticides, including gold, silver (Jiang et al., 2015; Eskandari et al., 2022), CNTs (Ivanov et al., 2012), silica-coated carbon dot-assisted ratiometric fluorescent nanosensors (Ma et al., 2023), and aptasensors (Verdian, 2018).
Conventional approaches including high-performance liquid chromatography (HPLC), gas chromatography–mass spectrophotometry (GCMS), liquid chromatography (LC), and polymerase chain reaction (PCR); these are effective techniques for identifying and detecting biological and chemical constituents. The major drawbacks with these methods are the time consumed and their expense. Therefore, novel diagnostic techniques require sensors and transducer elements to ensure efficient detection and signal binding vents (Anh et al., 2022).
A ratiometric fluorescent nanobiosensor has been developed by coupling mesoporous silica sphere-coated nitrogen-doped carbon dots (N-CDs@SiO2) with bovine serum albumin-stabilized gold nanoclusters (BSA-AuNCs) to detect glyphosates in malt samples (Ma et al., 2023). This nanosensor detects glyphosates from most samples with broad detectability in concentrations of 5–100 ng/mL and high sensitivity with a low detection limit of 3.4 ng/mL. The versatility of BSA-AuNCs can be applied in the environmental and food safety fields. A similar non-enzymatic nanosensor has been developed using carbon-shell silver (Ag@C) nonmaterials for the colorimetric detection of organophosphorus pesticides, including CP in agricultural products (Bilal et al., 2022). Cellulose non-enzymatic nanosensors detect CP with a detection limit of 0.097 ng/mL and quantification limit of 0.293 ng/mL. CP is a toxic organophosphate pesticide that, when applied to agricultural land, results in a loss of diversity in beneficial microflora. CP is resistant to degradation from conventional approaches and must be quantified for better biodegradation (Johhn and Shaike, 2015). Another organophosphate pesticide that can be environmentally harmful is dimethoate, which inhibits the enzymatic activity of AChE, leading to death in organisms. It is therefore important to develop highly sensitive and reliable techniques that monitor pesticide residues in the environment (Liu et al., 2022). Another example of enzyme-based nanobiosensors is based on the peroxidase-like activity of silver nanoparticle-modified oxidized multiwalled carbon nanotubes (AgNPs/OXMWCNTS). The nanobiosensor has been used to detect dimethoate organophosphate pesticide. This nanosensor has exhibited excellent peroxidase-like enzymatic activity in combination with silver nanoparticles (AgNPs) with a detection limit of 0.003 μg/mL. This method relies on the easy detection of dimethoate from water and fruit samples (Hsu et al., 2017).
Early detection of toxins in food products can be achieved using conventional methods. However, due to their time-consuming methods, higher cost and low sensitivity, conventional techniques have been replaced by designing various kinds of biosensors to overcome barriers of lower sensitivity (Girigoswami et al., 2021). A large number of synthetic dyes are generally used in food industries to color food particles. Metanil yellow and fast green are two widely used food dyes which are added to food commodities including chili powder, sauces, ice cream, soft drinks, and fruit juices. Metanil yellow is categorized as a type II toxin by the WHO. The overuse of synthetic food dyes can lead to cancer development, respiratory tract irritation, skin disease, and the inhibited release of neurotransmitters. A specially designed glassy carbon electrode nanosensor (calix8/AuNPs/GCE) can be employed to detect food toxin dyes. Due to the presence of gold nanoparticles associated with nanosensors, calix8/AuNPs/GCE activity was found significantly enhanced. Moreover, the detection limits for metanil yellow and fast green were found to be 9.8 and 19.7 nM, respectively. (Shah, 2020). Thus far, disposable colorimetric sensors based on AuNPs and plasma resonance have been applied to detect the presence of bacteria and their toxins in milk products, meat, and other foods (Marin et al., 2021). Lisa et al. (2009) developed a gold nanoparticle-based dipstick competitive immunoassay for detecting organochlorine pesticides such as DDT (dichloro-diphenyl-trichloro-ethane) in food samples. This biosensor can be effectively used for the on-site detection of DDT in food and environmental samples. Toxins produced by fungal and bacterial sources during food fermentation can lead to a multitude of foodborne illnesses, adverse gastrointestinal disorders, and cardiovascular aberration. Toxins produced by improper handling and processing conditions include biogenic amines such as cadaverine, mycotoxins such as fumonisins, aflatoxins, ochratoxin A, and zearalenone, and bacterial toxins including endotoxins, enterotoxins, and emetic toxins (Fayyaz et al., 2022).
Jia et al. (2022) developed a biosensor which can detect ricin, staphylococcal enterotoxin B (SEB), and botulinum neurotoxin type A (BONT/A). SiO2-based gold nanoparticles were used to develop surface-enhanced Raman-scattering lateral flow immunoassay (SERS-2FIA) biosensor strips. The accuracies for the detection of ricin, SEB, and BONT/A were 0.1, 0.05, and 0.2 ng/mL. Moreover, this nanobiosensor can also be used to detect toxins and prevent bioterrorism attacks.
Okadaic acid (OA) is a marine toxin produced from the toxicogenic algae of the Prorocentrum and Dinophysis genera. Eating OA-contaminated shellfish may lead to diarrhea, vomiting, and DSP (diarrheic shellfish poisoning). OA may be monitored by single-stranded DNA-binding proteins coupled with aptasensors. Nanosensors coupled with carbon–gold nanoparticles along with aptamers can detect OA of 2.5–80 ppb and even at the lower limit of 0.68 ppb (Kong et al., 2023).
Algal toxins contaminate drinking water and lead to growing effects on human health and the ecology. Algal toxins such as microcystins, OA, tetrodotoxin, brevetoxin, and lipopolysaccharides have been detected with aptamer-functionalized hybrid nanomaterials (Bilibana et al., 2022). In recent years, biosensor strategies based on graphene and its derivatives such as graphene oxide, grapheme QDs, and graphene-based nanocomposites have been used to detect microorganisms including prions, viroids, bacteria, fungi, protozoa, microbial toxins, and microbial sources of antibiotics (Pourmadadi et al., 2021). Aflatoxin B1 is mycotoxin that has mutagenic and carcinogenic properties produced from Aspergillus sp. (Jallow and Govender, 2021). Aflatoxin B1 contaminates food and agricultural products and can be monitored by aptamers. However, the aggregation of gold nanoparticles with aptamers facilitates the binding of aflatoxin B1 and detects mycotoxin within a detection range of 7 nM (Hosseini et al., 2015). A novel FRET-based nanobiosensor has been fabricated to detect aflatoxin B1 in rice and peanut samples using aptamer-conjugated QDs with gold nanoparticles and a toxin detection limit of 0.34 nM with a linear range of 10–400 nM (Sabet et al., 2017).
Nanosensors have recently been fabricated to detect water pollution with the ultra-sensitized detection of toxins using plasmonic nanosensors functionalized with silver or gold nanoparticles, which can help detect water toxicants even at very low concentrations (Brahmkhatri et al., 2021). Salmonella typhimurium, E. coli O157:H7, and Staphylococcus aureus are foodborne pathogens responsible for major foodborne outbreaks. High-affinity gold nanoparticle-conjugated aptamers and streptavidin-conjugated aptamers have been designed to detect S. typhimurium, E. coli O157:H7, and S. aureus within 10 min (Lu et al., 2020). E. coli O157:H7 is one of the most widespread pathogens for food contamination and the spoilage of dairy products (Yang et al., 2021). Single-strand DNA-aptamers synthesized with gold nanoparticles have been designed to detect E. coli O157:H7 from milk samples. Highly sensitized aptamer-exonuclease III-assisted with lateral flow assay (LFA) has high sensitivity to E. coli O157:H7 with a detection limit of 8.35 × 102 cfu/mL in milk samples (Ren et al., 2021).
Recent advance in nanosensor technology are vitamin-based sensors, catechol sensors, and hydrogen peroxide sensors. Vitamin-based sensors are innovative devices that use vitamin molecules to detect and monitor specific substances at nanoscale. These nanosensors leverage the unique properties of vitamins for targeted detection, offering potential applications in fields such as diagnostics, environmental monitoring, and healthcare. These are electrochemical sensors which detect both water- and fat-soluble vitamins via electrochemical oxidation and the reduction reaction of vitamins in electrolytes (Huang et al., 2021). With changing lifestyles, vitamin D deficiency is becoming more common, and sensors for this vitamin are currently needed. Various techniques have been employed for the early detection of vitamins, including fluorescence spectroscopy, surface-enhanced Raman spectroscopy, SPR, capillary electrophoresis, ultraviolet-visible spectroscopy, and ultra-performance liquid chromatography electrospray ionization multiple reaction monitoring/mass spectrometry (UPLC-ESIMRM/MS) (Yang et al., 2022). Being highly discerning and resolute, these methods possess some disadvantages such as high time requirements, higher costs, and highly complex processes. A novel antibody-free, CNT-based, highly responsive vitamin D3 sensor has been developed by Bora et al. (2022). Biogenic metals/metal oxide-based nanoparticles have wide utility in agriculture with antimicrobial action, monitoring plant stress, and detecting pesticides. Singh (2021) synthesized bio-inspired triangular zinc oxide nanoclusters from the leaf extract of Argyreia nervosa to electrochemically determine vitamin C. Electrochemical sensor-based ZnO NCs have been highly beneficial in combating bacterial diseases in rice crops, including both Gram-positive and -negative bacteria. The electrochemical deposition of bT-ZnO NCs into indium tin oxide (ITO) glass substrate has analyzed the electrochemical oxidation of vitamin C with a very high electrochemical sensitivity of 29.88 µAcm−2 and low limit detection of 0.5321 mM. Since plants can tolerate a high range of pH levels, it is more challenging to develop sensors for the in vivo monitoring of bioactive chemicals in plants. Liu et al. (2019) reported the in situ detection of vitamin B6 and melatonin in plants using a highly sensitive and sustainable plant sensor. An electrochemical sensor constituted with a CuO-poly(L-lysine)/graphene-sensing electrode recognized melatonin and vitamin B6 levels in plants with an average sensitivity of 0.076 μAμM−1 cm−2 for melatonin and 0.230 µAµM−1 cm−2 for vitamin B6. CuO-poly(L-lysine) has significant practical effectiveness for tracking plant growth processes and examining the constituents of agricultural products to determine melatonin and vitamin B6 in a variety of fruits.
A catechol-based sensor can detect changes in plant health by measuring various parameters such as pH, conductivity, or specific ion concentrations in the plant’s environment. The sensor reacts to biochemical changes associated with plant stress, providing valuable insights for monitoring and maintaining plant health. Catechol-based sensors often rely on the interaction of catechol compounds with metal ions. In the presence of stress-induced changes in plant physiology, these interactions can be altered, leading to measurable variations in the sensor’s output. This allows for the real-time monitoring of stressors that affect plant health, aiding in timely intervention and care (Wang et al., 2023). Catechol-based biosensors have a number of potential applications in agriculture. For example, they can be used to help to ensure food safety, protect consumers from exposure to harmful chemicals, and monitor fertilizer levels in soil and water, which can help prevent over-fertilization, pollution of waterways, and crop damage. This can help prevent the spread of disease and protect crops from damage. Li et al. (2023) demonstrated the potential of catechol-based biosensors to detect a wide range of important targets in agriculture. These biosensors are sensitive, selective, and stable, making them suitable for field applications. A catechol-based biosensor for detecting glyphosate, a widely used herbicide, was constructed by immobilizing a glyphosate-specific aptamer onto a gold electrode modified with catechol. The biosensor was shown to be highly sensitive and specific for glyphosate and could be used to detect glyphosates in a variety of samples, including food, water, and soil. The detection limit of the biosensor was 0.1 ng/mL, and the linear range was 0.1–100 ng/mL. This biosensor could be used to improve food safety and protect consumers from exposure to glyphosate (Wang et al., 2022). One of the most promising applications of catechol-based biosensors is in detecting pesticides. Catechol-based biosensors can be used to detect a wide range of pesticides, including organophosphates, carbamates, and pyrethroids. These biosensors are typically based on the use of enzymes that are inhibited by pesticides. For example, one study developed a catechol-based biosensor to detect the organophosphate pesticide malathion. The biosensor was based on the use of the enzyme AChE, which is inhibited by malathion. The biosensor could detect malathion at concentrations as low as 10 nM (Tsong and Khor, 2022). It was based on the use of the enzyme glyphosate dehydrogenase, which converts glyphosate into a fluorescent product. The biosensor was able to detect glyphosate at concentrations as low as 10 nM (Liu et al., 2019). Catechol-based biosensors can be used to detect a variety of fertilizers, including nitrogen, phosphorus, and potassium. Zhang et al. (2023) developed a catechol-based biosensor for detecting nitrogen fertilizer nitrate using the enzyme nitrate reductase, which converts nitrate to a fluorescent product. The biosensor could detect nitrate at concentrations as low as 10 nM.
Hydrogen peroxide (H2O2) is a very important compound involved in many oxidative physiological processes (Smirnoff and Arnaud, 2019). This molecule, the superoxide anion, and the hydroxyl radical (OH) are commonly known as free radicals and are from a class of compounds called reactive oxygen species (ROS) (Sies, 2017). H2O2 is also a contaminant in many foods, pharmaceuticals, and environment processes. The human body cannot efficiently process it, so H2O2 excess can lead to a pathological condition known as oxidative stress which can damage cellular lipids, proteins, or DNA. H2O2 is a versatile chemical that has a wide range of applications in agriculture. It is a safe and effective way to control pests and diseases, promote plant growth, and improve soil health. One of the most common uses of H2O2 in agriculture is pest control. According to Daughtrey and Buitenhuis (2020), H2O2 can be used to control a variety of pests, including aphids, spider mites, whiteflies, and fungus gnats. It can be applied as a foliar spray or soil drench and can be used to control a variety of plant diseases, including powdery mildew, downy mildew, and black spot. H2O2 can be applied as a foliar spray or soil drench. It can be used to promote plant growth by increasing root development and nutrient uptake and can be applied as a foliar spray or soil drench. H2O2 can be used to improve soil health by killing harmful bacteria and fungi and increasing the activity of beneficial microorganisms. In plants, H2O2 acts as a signaling molecule that facilitates various biochemical and physiological processes under both stressful and stress-free conditions (Yan et al. 2022). H2O2 is also pivotal in improved growth, photosynthetic capacity, and antioxidant protection. However, H2O2 can have many harmful effects on plants and food, so its detection is an important goal of clinical and industrial research. This highlights the critical need of precise H2O2 detection in healthcare and food safety, where the use of electrochemical sensors can enhance detection limits. Nonetheless, there are as yet few commercial biosensors that are utilized for biological and food detection (Erkmen et al., 2022). These are the primary constraints that electrochemical H2O2-detecting sensors currently face. Ag nanomaterials have attracted increasing attention due to their quantum characteristics including large specific surface area, easy preparation, and small granule diameter (Shafa et al. 2023). Furthermore, Ag nanomaterials exhibit the highest thermal and electrical conductivity and are widely used in electronics, photonics, biological labeling, and sensor fabrications. Thus, Ag nanoparticles are also frequently employed to construct H2O2 biosensors based on heme proteins, modifying the platinum electrode with the matrix of polyvinyl butyral, Ag colloid, and HRP. Using Co (bpy)33+ as the mediator, the resulting biosensor displayed an excellent electrocatalytic response to H2O2. The presence of Ag nanoparticles provided a biocompatible microenvironment for HRP, and the resulting biosensor exhibited obvious electrocatalytic behavior toward the H2O2 reduction. Carbone et al. (2019) have proposed a simple method for the synthesis of AgNP−PMMA and also studied the formation of nanoparticles using a UV-vis spectrometer, measured nanoparticle size and their size distribution using the TEM technique, and confirmed the crystalline nature of formed nanoparticles using SAED patterns. Au nanoparticles are used in enzyme-based biosensors and non-enzymatic H2O2 sensors due to their ability to provide a friendly microenvironment for immobilized enzymes and act as a conducting tunnel. Different Au nanostructures such as nanocrystals, nanospheres, nanorods, and nanoporous have been used for sensitive and selective H2O2 detection (Liu et al., 2020). Optical nanosensors monitor plant signaling molecules that communicate and trigger plant stress responses through epifluorescence microscopy of H2O2 and nitric oxide (NO) in leaf sections and remotely detect glucose in algae and whole plants (Lew et al., 2020). Under the highest stresses, including light, heat, salinity, wounding, and accumulating H2O2 in plants (Fichman et al., 2019), H2O2 nanotechnology-based sensors can detect H2O2 within the plant’s physiological range of 10–100 µm (Giraldo et al., 2019).
Micro- and nanotechnologies have attracted recent attention and assumed a leading role in the struggle against bacterial infections. Nano-mechanical, CMOS, wearable sensors, and photonic crystals are recent innovations that show the tremendous potential for addressing the primary shortcomings of current approaches. Recent reviews of analytical and developing methods for bacteria identification and antibiotic susceptibility testing (AST) have been published. Due to its high integration, multiplexing capabilities, exceptional sensitivity, and quick reaction compared to other technologies, nano-mechanical sensors have generated much attention among developing technologies (Pujol-Vila et al., 2020). Nanobiosensors, a novel class of sensors, have exceptional abilities in detecting microbial contamination by tracking a wide range of variables, including temperature, humidity, gas composition, and microbial agents (Willner and Vikesland, 2018; Wlodkowic and Karpiński, 2021). By seamless integration into food packaging materials, nanomaterials can enable the continuous and real-time monitoring of food quality and safety, which can transform food safety (Wlodkowic and Karpiński, 2021). Nano-mechanical sensors have successfully detected numerous pollutants in water, including bacteria, in practical applications (Salouti and Khadivi Derakhshan, 2020). The particular type of sensor used determines how nanobiosensors for microbial contamination detection work. The fundamental idea, however, continues to be the same: the sensors detect changes in their surroundings brought on by the presence of microbial organisms. Some of these sensors use bioactive chemicals or antibodies that have a specific affinity for certain microbes, enabling targeted detection. Others rely on nanoparticles that, in reacting to the presence of microbial organisms, experience changes in their electrical or optical characteristics, so generating a detectable signal (Nile et al., 2020).
Electrochemical biosensors can be divided into two categories for their biorecognition components: biocatalytic or biocomplexing. Enzymes are commonly used in biochemical sensing applications as biorecognition components, but they are typically employed as labels in the detection of pathogens through a secondary binding stage (Choi et al., 2018). This is how analytes interact with macromolecules or how ordered molecular assemblies trigger a response in a biosensor—influenced by various factors such as assay design, biosensor performance (e.g., sensitivity and LOD), volume, material qualities, the composition of the pathogen-containing sample, and the specific application. All these factors help determine the optimal measurement format (Jeerapan et al., 2020). Functionalized electrodes can be employed in a range of electrochemical techniques for detecting pathogens. These techniques include amperometric, conductimetric, potentiometric, ion charge/field effect, and impedimetric methods. The measured signal is often used to classify the type of electrochemical technique used for pathogen detection, with signals being applied either continuously or intermittently (Saxena et al., 2018). The relationship between the recognition of virus-related elements and proteins is linear up to 50 viral elements per 100 mL−1(R2 = 0.93) and 53 pgml−1(R2 = 0.99) at pH 7, with extremely low detection limits of approximately two viral particles per 100 mL and 4 pgml−1—similar to the sensitivity of PCR methods. The relative standard deviation (RSD) for viral RNA detection in whole serum samples was found to be 6.9%. Wan et al. (2011) developed reduced graphene sheets with impedimetric immuno-sensor technology for bacteria detection (Chen et al., 2016). The most common types of optical biosensors developed for detecting plant pathogens include calorimetric biosensors, SPR-based biosensors, and fluorescence-based assays (Khasili et al., 2018; Umapathi et al., 2022). Colorimetric biosensors can quickly detect harmful bacteria in 10–15 min by observing a color change in the samples. The assay can be conducted in a flat- or solution-based format. A machine-learning approach was developed by Wahabzada et al. (2016) to characterize hyperspectral reflectance signals across net blotch, powdery mildew, and brown rust pathogenesis in barley (also Lei et al., 2022). This presents the potential for creating a new type of spectral library that outlines the health and illnesses of plants based on their spectral properties. Collecting hyperspectral signatures of plants would have wide-ranging advantages and create opportunities for non-experts to benefit from this field (Table 1).
The interaction between plants, soil, and microbes is very complex and varies from symbiosis to mutualism, depending on the type of association microbes have with plants and soil. Understanding the mechanisms of these interactions is highly desired because, with increasing world global demands for food, agriculture must undergo some radical changes in order to remain stable, sustainable, and economically friendly. The aim of sustainable agriculture is to refrain from using chemical pesticides and fertilizers which worsen the health of humans, animals, and plants (Chodak et al., 2016). Plants have complex interactions both below and above ground which serve for their mutual benefit. Microbial colonies present on plants above ground can be categorized into epiphytes, endophytes located inside the plant, phyllospheric microbes growing on leaf surfaces, and rhizospheric microbes of the roots. Among these, rhizospheric association is considered the most dynamic association of plant and microbes that significantly impacts the nutritional status and growth of the plant (Bakker et al., 2013; Lakshmanan et al., 2014). Plant growth-promoting rhizobacteria (PGPR) and other pant growth-promoting microbes (PGPMs) promote plant growth by producing siderophores, antioxidants, stress resistance response, and nutrient acquisition for plants from the soil (Kumar and Verma, 2018). Plants and soil benefit directly or indirectly through associated microbes: not only do soil microbes improve plant health but plant-associated microbes improve soil health.
During plant and rhizospheric colonization, many microbes and microbial communities regulate plant development and greatly influence root systems. The complex interaction among rhizospheric microbes is required for plant growth (Bonfante and Anca, 2009). Microbial diversity in soil is measured by traditional methods and conventional sensors.
Microbes on their own also produce various types of sophisticated nanomachines, such as bacterial flagellar nanomotors, which are composed of numbers of innate proteins (Chalmeau et al., 2009). These are used as the building blocks for the construction of nano-devices including sensors and drug-delivery vehicles (Petrov and Audette, 2012). Peptide nanotubes are used as casting modulates or biotemplates for the synthesis of metal nanowires (Scanlon and Aggeli, 2008). Extracellular electrically conductive protein nanofilaments or microbial nanowires (MNWs) discovered in numerous anaerobic and aerobic microorganisms are used in the sensing and detoxification of metals. MNWs can facilitate electron transfer between microbial cells, such as metal-reducing bacteria like Geobacter sulfurreducens. These MNWs help transfer the electrons to metals which act as electron acceptors, even though they are at distance, without the need of direct cell attachment (Reguera et al., 2005). With the metal-oxidizing bacteria Acidithiobacillus ferrooxidans, these MNWs possess the ability to transfer electrons to the cell surface, allowing the accessibility of electron donors at a distance (Li and Li, 2014).
Several nanoparticles have been exploited in the construction of biosensors as probes to interact with the target analyte or have been used as transducers to measure the binding event and for the quantification of signals. Nanomaterials that have been investigated for the detection of microbes include CNTs, mNPs, QDs, silica nanoparticles, and up-conversion nanoparticles (UNCPs). These materials are easily malleable to nano-sized structures, conferring superior properties, specifically for biosensing applications (El-Ansary and Faddah, 2010).
To begin with mNPs, gold nanoparticle-based optical sensors have been developed for detecting Karnal bunt disease in wheat (Singh et al., 2010). These gold nanoparticles have also been used in the construction of probes to identify pathogenic bacteria using DNA-microarray technology (Taton et al., 2000). Magnetic nanoparticles have been used as contrasting agents in magnetic resonance imaging and as immunomagnetic separators for nucleic acids, proteins, viruses, and bacterial cells (Abd-Elsalam et al., 2019). The use of magnetic nanoparticles ensured specific binding to Mycobacterium spp. (Kaittanis et al., 2007). UNCP-based biosensors have been used to detect a consortium of three microbes present in food and the environment (Wu et al., 2014).
Nanotechnology has provided solutions to mitigate the challenges of existing agriculture practices. The fundamental components of nanotechnology are nano-tools and nano-devices. Nano-tools are different types of nanomaterials—carbon-based nanomaterials, mineral nanoparticles, and metal and metal oxide nanoparticles—which have very high surface areas (Lowry et al., 2019). Nano-based devices have improved existing plant breeding and genetic transformation technologies. It has also been reported that engineered nanomaterial (ENM)-based agricultural practices can acclimatize to fluctuating environmental and ENM-based climatic conditions (Mittal et al., 2020). ENMs coated with molecular recognition elements such as aptamers or antibodies help the targeted delivery of nutrients. This increases crop yield by minimizing adverse impacts on the environment and also leads to the sustainable use of nutrients. By enhancing targeted delivery, these ENMs improve agricultural practices and overcome issues with conventional and modern agricultural practices of agrochemicals and their efficient utilization and management. ENMs have shown promising sustainable applications in agricultural practices in the design of nanoscaled agrochemicals (Gilbertson et al., 2020). ENMs can improve agricultural sustainability by (i) improving soil texture by restricting the use of nitrogen fertilizers; (ii) coating seedlings with Zn and Cu nanoparticles, thus augmented seed germination rates in comparison to conventional practices with potassium chloride, potassium nitrate, and polyethylene glycol (PEG), which have high embodied energy; and (iii) foliar spray of ENMs, including nano-Zn, ZnO, and TiO2, which have also increased crop productivity by the efficient targeted uptake of agrichemicals. ENM-based foliar treatment increases crop productivity by augmenting the photosynthetic efficiency of crops by preventing the effect of abiotic stresses on the photosynthesis rate, elevating chlorophyll quantity, increasing light absorption efficiency, scavenging reactive oxygen species, and supporting effective electron transport system (Lowry et al., 2019). High photosynthetic rates have sometimes been found to lower the nutritional value of food. Therefore, spraying iron, zinc, and silica micronutrients with ENMs maintains high nutrient content in foods. Moreover, modifying the surface chemistry of ENMs improves their adherence to leaves and thus increases nutrient uptake efficiency by cuticle and mesophyll cells. Moreover, these ENMs have been used in the fabrication of sensing devices to evaluate the health of crops in high spatial and temporal resolution (Giraldo et al., 2019). Bartolucci et al. (2020) have reported the tremendous applications of distinct types of green nanoparticles in agricultural systems. Various plant sources have been employed to synthesize green nanoparticles, which have been documented to have diverse agricultural applications such as nanofertilizers, nanopesticides, plant development processes, and the regulation of plant hormones.
With advancements in nanomaterial utilization, nanoparticles can be coupled with a variety of micro- and macromolecules for a better detection system. Nanomaterials have shown great promise in applications including medical diagnostics, food safety, detecting contamination, and environmental monitoring. Despite their potential to detect nutrient deficiency and diseases in plants, there are some major limitations and challenges that should be addressed. One of the major limitations of nanomaterial-based sensing systems is high cost and product sensitivity. Nanomaterials and components used to fabricate nanosensors such as CNTs, graphene, or nanoparticles are much more expensive. High material costs contribute significantly to the overall cost of manufacturing nanosensors. Another limitation is associated with the toxic nature of nanomaterials, and their size, structure, composition, and surface-to-volume ratio. The large-scale fabrication of nanocomposites is quite challenging. Therefore, investment and infrastructure will be required to ensure their uniform coverage across sizeable agricultural landscapes. The synthesis of nanomaterials embraces the sensitivity and stability of the raw materials used. Nanosensors frequently experience difficulties in reducing the interference generated from environmental factors such as electromagnetic sensors. This sensitization could produce false readings and undermine the veracity of the information collected. Apart from scalability and sensitization, challenging with existing sensors are power consumption, biocompatibility, durability, and integration with the existing systems. Some nanosensors, including nanoscale gas sensors, biological sensors, nano-mechanical sensors, semiconductor nanostructure nanosensors, and electrochemical nanosensors often require considerable power consumption for long-term applications. Therefore, the development of low-powered energy-efficient sensors is required. Another challenge associated with nanosensors is related to their durability in harsh environmental conditions, including high temperature and humidity. Increased temperature, humidity, and soil chemicals may affect the performance and longevity of nanosensors. These challenges can be mitigated by a combination of careful design, protective measures, and appropriate materials such as encapsulating nanosensors, robust design, remote monitoring, and vacuum packaging. Another major challenge is that the deployment of these sensors can raise ethical and privacy concerns; the collection of data can be regulated by standardized guidelines and regulations.
Although nanobiosensors are a promising tool to ensure agricultural sustainability and viability, they still face many challenges. One is that use of nanomaterials is associated with ecotoxicity due to unique properties such as structure, size, surface-to-volume ratio, and composition. Their impact on the environment should thus be assessed accurately in terms of dose accumulation, immune responses, retention time, and size (Prasad et al., 2017). Considering their potential environmental impact, it is necessary to examine the manufacturing utilization and end-of-life disposal of nanomaterials used to produce nanobiosensors. This type of risk assessment is considered a life cycle assessment (LCA) and includes the production of nanomaterial-containing products, their use, disposal, and such end-of-life stages as reuse, recycling, recovery, and final disposal. To overcome toxicity concerns associated with nanomaterial exposure in occupational settings, greater standardization of regulations is needed relating to exposure limits and safety controls. The potential impact of nanobiosensors on the environment is also associated with the intentional and unintentional release of nanoparticles in waste streams. Nanomaterial waste from nanobiosensors can also originate from the disposal of spent devices in landfills and leachates associated with such disposal. In order to mitigate possible risks associated with the disposal of nanobiosensors, reuse and recycling methods should be explored. Traditional recycling techniques include separation by centrifugation and solvent evaporation. Alternative methods include the use of molecular antisolvents, pH or thermal responsive materials, and magnetic fields. Strategies for reducing and controlling the toxicity of nanoparticles are also needed; current strategies include coating, encapsulation, loading, grafting, and doping of nanomaterials used to produce nanobiosensors (Kelli et al., 2022). Another challenge to overcome is the fabrication of miniature forms that resolves their portability. For instance, if nanobiosensors can be successfully associated with information technology, farmers in the remote areas will also benefit from lower costs, improved productivity, and better understanding about disease outbreaks. Farmers will also best be able to utilize natural resources like soil, water, and other climatic factors. Deriving novel nanomaterials from waste biomass can provide a cost-effective alternative solution. Moreover, the commercialization of nanobiosensors is also linked with risk factors such as (a) initial applications that could act as substitutes for agricultural commodities which might be disastrous for the economies of developing countries; (b) variable import regulations in different nations that could obstruct nano-product expansion; and (c) nanotechnology could have a negative effect on the poor by increasing productivity in developed countries, leading to decreased commodity prices in developing countries. A solution could be properly labeling nano-products in certain developed countries which might lead to technology consumption and regulations in developing countries (Vineeta and Sachin, 2013). A major challenge yet to be resolved is transforming nanobiosensors from prototypes to commercial products. For this reason, field-scale trials need to be assessed and nanobiosensors perform in real applications (Kaushal and Wani, 2017).
Nanobiosensors have potential in the food and agriculture sectors in remote locations when they are effectively connected to information technology, resulting in reduced expenses, increased output, enhanced awareness of disease outbreaks, and better utilization of natural resources such as soil, water, and climatic conditions. The costs associated with the fabrication of nanobiosensors are still a challenge and they need to be lowered. For this reason, other less expensive biological components may be used, such as cells or enzymes, novel matrices for stabilization, and the use of chitosan for the immobilization of nanomaterials to improve the stability and reusability of nanobiosensors (Alvarado et al., 2019). The efficient utilization associated with validamycin of nano-silica is for the regulated pesticides dissolved in water. Nanoscale silica combined with methyl methoxysilane can act as a nanofungicide to fight mildew and as an inhibitor of plant growth division (Huang et al., 2015). Biosensor nanotechnology technology has made recent advances in addressing challenges in agriculture. Reduced stress, efficient use of resources, and crop loss due to environmental and pathogenic stresses are some of the challenges faced by plant farmers. Smart plant sensors increase plant yield by maximizing the use of agrochemicals and water distribution. Real-time monitoring of the physiological and developmental responses of plants requires new technologies. Plant chemical signals are converted into digital information by means of nanomaterials in such new technologies that can be observed via electronic tools such as biosensors (Giraldo et al., 2019).
The food and agricultural industries make extensive use of nanotechnology. However, due to their extremely small sizes and their ability to enter the body, nanomaterials present a number of safety concerns (Rajput, 2022). The main reason is the small size and larger surface area of nanoparticles, which are easily dispersible and can penetrate cells to reach any part of the body and be potentially toxic (Dasgupta et al., 2015). According to Moustafa et al. (2019), both environmental factors and the release of inserted nanomaterials from polymeric textures into the environment can cause nanocomposites to degrade. Certain types of food packaging, such as low-density polyethylene, lose their strength when exposed to humidity, UV light, or other environmental factors (Han et al., 2018). Low-density polyethylene samples oxidize when exposed to UV light or their thermal, physical, and structural properties are significantly modified. High health risks for farmers result from the use of pesticides and nano-fertilizers in the agriculture sector, which can end up in the soil, water, or atmosphere (Roohinejad and Greiner, 2017). Rajput et al. (2020) expect that the accumulation of nanoparticles in the soil will impede plant growth and potentially accumulate in edible plant tissues. Nanoparticles can cause oxidative harm and provocative reactions, and wasted nanoparticles could be toxic (Han et al., 2018). The main mechanism through which nanotoxicity is mitigated is the massive generation of free radicals, which leads to oxidative anxiety and ultimately impairs cells’ ability to perform routine biological redox-regulated functions (Pathakoti et al., 2017). Several studies have explored the antibacterial features of silver nanoparticles. Silver, although a heavy metal, can cause toxicity when present in high concentrations by denaturing the body’s proteins and enzymes; its migration threat was estimated by Li et al. (2017).
Recent advancements in nanotechnology may greatly revolutionize disease protection, prevention, and management. Although some advances are still in the initial phases of research and development, the following disciplines may see future revolutionary developments.
Nanotechnology offers potential advancements in the agri-food sector, including the monitoring of soil conditions, nutrient levels, and microbial activity. Nanomaterials can enhance the effectiveness of pesticide delivery via nanoemulsions, polymer-based nanopesticides, and metal/metal oxide nanoparticle-based pesticides, thereby reducing the quantity required for pesticides to be effective (Zhang et al., 2023). Nanosensor-enabled drones can provide valuable data on crop health and pest infestations (Vaseashta, 2020). Soil and water remediation can be accomplished with nanoparticles, including carbon metal, metal oxide, and other nanocomposites (Rahman et al., 2023). Apart from the above advances, nano-coatings on seeds before sowing can protect crops from pest attack and pathogenic microorganisms. Furthermore, metal-phenolic network (MPN)-coated microbes help improve seed germination, build resilience against environmental stressors, and promote beneficial soil microflora under delicate conditions (Burke et al., 2023). Another possible advance in nanotechnology is in food packaging, where nanocomposites can enhance the barrier potential and extent the shelf life of packed foods.
Research on nanoscale devices is revolutionizing agriculture in monitoring animal health. Similarly, drug-delivery technologies that include nanoparticles are experiencing rapid growth in delivering nutrients to plants and providing controlled targeted release of drugs (Patel and Geed, 2024). The use of nanofertilizer is an important step in allowing the delivery of nutrients directly to plant cells, improving the efficiency of nutrient uptake, and reducing the environmental impact of traditional fertilizers (Singh et al., 2024). Similarly, nanoencapsulation is another subfield of nanotechnology that has broad-range application. Plants benefit from nanoencapsulation and gradually release nutrients that utilize nanoparticles, supplying them with ample nutrients for long-term use.
In order to reduce food waste, the world’s enormous annual food output must be appropriately managed, stored, or processed into value-added goods. Advanced packaging technology has now progressed to include a controlled gaseous microenvironment. Food is packaged intelligently to detect changes in its interior components across time and space, in addition to extending its shelf life (Yang et al., 2021). Biosensors for food packaging can function in particular physical–chemical conditions in the packaged microenvironment. Their widespread application in this sector has some limits. Such devices need to work in wide temperature ranges, humidity, exposure to light, and gaseous concentrations. Fast responses and high throughput are required for the real-time and online identification of improperly sealed packets. Many types of biosensors integrated for intelligent packaging have been realized but not commercialized due to limitations of high sensitivity and reversibility. When integrated with direct biosensing, intelligent tags and stickers can be placed inside packaging to reflect the deterioration of the packed material over time. This allows any smart packaging to communicate directly with the consumer through electronic devices that offer visually appealing and easily comprehensible indicative information (Belgacem and Bras, 2016). Biosensor developments have had several phases. In previous generations, the biocatalyst and transducers were initially separated; later, they were integrated so closely that the removal of one had an impact on the operation of the other. With today’s biosensors, a mediator is not necessary. The enzyme is directly decreased on their electrode surface (Zeinhom et al., 2018). Biosensors have created a new entry point into smart and precision agriculture for the whole agricultural community, including farmers, researchers, and end users. Agricultural biosensors can be categorized according to the kind of biorecognition system utilized. Biorecognition systems that are often employed include complementary sequences in nucleic acids, enzyme–coenzyme–substrate, and antibody–antigen. Furthermore, tissue, plant, animal, and microbe cells can all be utilized as biorecognition components. Multiple fungicides are sprayed in liquid or powder form on horticultural crops (Silins, Korhonen, and Stenius, 2014), cereals (Dork and Anastassiades, 2017), oil seed crops, floriculture produce, and even fodder crops (Kotinagu and Krishnaiah, 2015) like cotton and grass during post-harvest operations. However, because these substances block AChE, they also pose a risk to human health because they can result in intellectual and cognitive problems and may result in death (Chen et al., 2016). The future of nanobiosensor development for agriculture holds immense potential, and computational biology, including machine learning and deep learning, will play a crucial role in unlocking its full potential (Magarelli et al., 2023). Computational modeling can help predict and optimize these interactions, leading to more effective sensors (Swierczewska et al., 2012). Machine learning algorithms can analyze the vast amount of data generated by nanobiosensor networks deployed in the field. This can enable the real-time monitoring of soil health, plant stress, and pest outbreaks, thus facilitating proactive interventions (Buja et al., 2021). Integrating sensor data with weather and environmental data can enable deep learning models to predict crop yields, optimize irrigation and fertilization, and provide early warning of disease outbreaks (Elbasi et al., 2023). Smarter sensor systems using ML in real-world sensor applications may effectively implement ML “smart” models with proven data processing power and the analysis of massive amounts of data points, intelligent systems, and chemical and biological sensing for detecting physical and chemical properties of target items or the surrounding surroundings. By combining nanobiosensors with IoT and robotics by connecting the former to wireless networks and integrating them with robotic systems, automated precision agriculture can be rapidly realized; applications of smart IoT-based technologies have opened up many new possible technical developments in all areas of life. IoT technologies are widely regarded as one of the fundamental pillars of the fourth industrial revolution due to their great potential for innovation and societal benefits. The IoT is a new internet-based technology that promises to connect physical devices like industrial equipment and home appliances or “things” (Haseeb et al., 2019). It allows the targeted application of pesticides and fertilizers, thus minimizing waste and environmental impact. Machine learning algorithms can analyze data from nanobiosensors and other sources to generate targeted recommendations for farmers on irrigation, pest control, and nutrient management, empowering farmers to make data-driven decisions and improve their productivity (Ha et al., 2020). Automatic robotic harvesters work on the control unit design of image space where the controller guides the harvester on the sloping ground. It is already commercially feasible to harvest tomatoes, cherry tomatoes, cucumbers, strawberries, watermelons, and grapes with robots (Fountas et al., 2020).
Microbial sensors have the potential to offer a wide range of biomarker sensing applications in vitro and in vivo; for example, creating a novel synthetic receptor platform called Engineered Modularized Receptors Activated by Ligand-induced Dimerization (EMeRALD), which allows various ligand-binding domains to be fused to an E. coli-derived synthetic receptor scaffold. Within 2 h, the developed sensors can identify abnormal bile salts as biomarkers in human serum samples with excellent sensitivity and specificity using zinc-responsive transcription elements and isopropyl ß-D-1-thiogalactopyranoside-inducible elements in E. coli produce two-input zinc-sensing WCSs that could synthesize colorful pigments in response to zinc concentrations ranging from 0.1 to 1.0 mm (Chang et al., 2021).
Nanobiosensors have revolutionized the development of biosensors. These sensors have become more usable due to their high accuracy, stability, and reactivity. Advanced nanobiosensors enhance detection through their sensitivity and are used as a diagnostic tool for soil quality, for detecting pathogens, and in disease assessment, and have thus become agents for promoting sustainable agriculture. The disciplined use of these advanced nanobiosensors helps promote sustainable agriculture, the detection of pathogens, and thus improve plant and soil health and eventually enhance crop productivity. Nanobiosensors are being increasing utilized in agri-food industries.
The future of nanobiosensors in agriculture holds great potential for enhancing crop productivity and sustainability. Nanobiosensors can be applied to detect pathogens and pesticides in plants, soil, and water. By integrating nanotechnology with biosensing capabilities, nanobiosensors can provide real-time monitoring of nutrient levels, pesticide residues, and the presence of diseases in agricultural crops. However, the application of nanobiosensors in agriculture faces many challenges, such as cost-effectiveness, scalability, and regulatory considerations. Therefore, continuous progress is needed to face such challenges and ensure the successful integration of nanobiosensors in the agri-food sector by enhanced sensitivity, portability, longevity, and the stability of nanomaterials. These advances in nanobiosensors will assist agriculturists by enabling precision farming, reducing resource wastage, and promoting sustainable practices. Future work will eventually involve the development of competent and advanced nanobiosensors to contribute to the complex microbe–plant interaction mechanism, providing previously inaccessible information and microbial responses in soil microbiome under variable environmental conditions.
VV: conceptualization, Formal analysis, Project administration, and Writing–review and editing. HD: Writing–original draft and Writing–review and editing. KT: writing–original draft, writing–review and editing. RS: writing–original draft. SG: writing–original draft.
The author(s) declare that no financial support was received for the research, authorship, and/or publication of this article.
The authors are thankful to Kanya Gurukul Campus, Gurukula Kangari (Deemed to be University), Haridwar, for providing all laboratory facilities for contributing this review work.
The authors declare that the research was conducted in the absence of any commercial or financial relationships that could be construed as a potential conflict of interest.
All claims expressed in this article are solely those of the authors and do not necessarily represent those of their affiliated organizations, or those of the publisher, the editors, and the reviewers. Any product that may be evaluated in this article or claim that may be made by its manufacturer, is not guaranteed or endorsed by the publisher.
Abdelhamied, N., Abdelrahman, F., El-Shibiny, A., and Hassan, R. Y. (2023). Bacteriophage-based nano-biosensors for the fast impedimetric determination of pathogens in food samples. Sci. Rep. 13 (1), 3498. doi:10.1038/s41598-023-30520-3
Abdel-Karim, R., Reda, Y., and Abdel-Fattah, A. (2020). Review—nanostructured materials-based nanosensors. J. Electrochem. Soc. 167 (3), 037554. doi:10.1149/1945-7111/ab67aa
Abd-Elsalam, K. A., Mohamed, M. A., and Prasad, R. (2019). Magnetic nanostructures. Cham, Germany: Springer.
Acharya, A., and Pal, P. K. (2020). Agriculture nanotechnology: translating research outcome to field applications by influencing environmental sustainability. NanoImpact 19, 100232. doi:10.1016/j.impact.2020.100232
Aghaei, A., Khorasanizadeh, H., and Sheikhzadeh, G. A. (2018). Measurement of the dynamic viscosity of hybrid engine oil-Cuo-MWCNT nanofluid, development of a practical viscosity correlation and utilizing the artificial neural network. Heat Mass Transf. 54, 151–161. doi:10.1007/s00231-017-2112-6
Ahmad, T., Iqbal, A., Halim, S. A., Uddin, J., Khan, A., El Deeb, S., et al. (2022). Recent advances in electrochemical sensing of hydrogen peroxide (H2O2) released from cancer cells. Nanomaterials 12 (9), 1475. doi:10.3390/nano12091475
Ali, H. S., Fakhri, M. A., and Khalifa, Z. (2021). “Optical and structural properties of the gold nanoparticles ablated by laser ablation in ethanol for biosensors,” in Journal of physics: conference series 1795 (1), 012065. doi:10.1088/1742-6596/1795/1/012065 (IOP Publishing).
Alias, N., Hanan, S. A., Ali, A., and Dollah, Z. (2016). Numerical analysis on mathematical model for drug delivery system on blood flow in external magnetic fields by magnetic nanoparticles. J. Teknol. 78 (4-4), 31–37. doi:10.11113/jt.v78.8290
Alvarado, K., Bolaños, M., Camacho, C., Quesada, E., and Vega-Baudrit, J. (2019). Nanobiotechnology in agricultural sector: overview and novel applications. J. Biomaterials Nanobiotechnology 10 (02), 120–141. doi:10.4236/jbnb.2019.102007
Anh, N. H., Doan, M. Q., Dinh, N. X., Huy, T. Q., Tri, D. Q., Van Hao, B., et al. (2022). Gold nanoparticle-based optical nanosensors for food and health safety monitoring: recent advances and future perspectives. RSC Adv. 12 (18), 10950–10988. doi:10.1039/d1ra08311b
Ansari, M. A. (2023). Nanotechnology in food and plant science: challenges and future prospects. Plants 12 (13), 2565. doi:10.3390/plants12132565
Antonacci, A., Arduini, F., Moscone, D., Palleschi, G., and Scognamiglio, V. (2017). Nanostructured (Bio) sensors for smart agriculture. TrAC Trends Anal. Chem. 98, 95–103. doi:10.1016/j.trac.2017.10.022
Aparna, A., Sreehari, H., Chandran, A., Anjali, K. P., Alex, A. M., Anuvinda, P., et al. (2022). Ligand-protected nanoclusters and their role in agriculture, sensing and allied applications. Talanta 239, 123134. doi:10.1016/j.talanta.2021.123134
Arora, K. (2018). “Advances in nano-based biosensors for food and agriculture,” in Nanotechnology, food security and water treatment, 1–52.
Ayesh, A. I. (2016). Metal/metal-oxide nanoclusters for gas sensor applications. J. Nanomater. 2016, 1–17. doi:10.1155/2016/2359019
Bakker, P. A., Berendsen, R. L., Doornbos, R. F., Wintermans, P. C., and Pieterse, C. M. (2013). The rhizosphere revisited: root microbiomics. Front. plant Sci. 4, 165. doi:10.3389/fpls.2013.00165
Bao, J., Huang, T., Wang, Z., Yang, H., Geng, X., Xu, G., et al. (2019). 3D graphene/copper oxide nano-flowers based acetylcholinesterase biosensor for sensitive detection of organophosphate pesticides. Sensors actuators B Chem. 279, 95–101. doi:10.1016/j.snb.2018.09.118
Bao, Y., De Keersmaecker, H., Corneillie, S., Yu, F., Mizuno, H., Zhang, G., et al. (2015). Tunable ratiometric fluorescence sensing of intracellular pH by aggregation-induced emission-active hyperbranched polymer nanoparticles. Chem. Mater. 27 (9), 3450–3455. doi:10.1021/acs.chemmater.5b00858
Bartolucci, C., Antonacci, A., Arduini, F., Moscone, D., Fraceto, L., Campos, E., et al. (2020). Green nanomaterials fostering agrifood sustainability. TrAC Trends Anal. Chem. 125, 115840. doi:10.1016/j.trac.2020.115840
Beeguma, S., and Dasb, S. (2022). Nanosensors in agriculture 17. in Agricultural nanobiotechnology: biogenic nanoparticles, nanofertilizers and nanoscale biocontrol agents, 465.
Belgacem, M. N., Bras, J., and Naceur Belgacem, M. (2016). Nisin anchored cellulose nanofibers for long term antimicrobial active food packaging. RSC Adv. 6 (15), 12422–12430. doi:10.1039/c5ra22748h
Bezzon, V. D., Montanheiro, T. L., de Menezes, B. R., Ribas, R. G., Righetti, V. A., Rodrigues, K. F., et al. (2019). Carbon nanostructure-based sensors: a brief review on recent advances. Adv. Mater. Sci. Eng. 2019, 1–21. doi:10.1155/2019/4293073
Bilal, S., Saleem, S., Akhtar, N., Sami, A. J., Gokce, G., and Hayat, A. (2022). A novel cellulosic non-enzymatic nanosensor based on carbon shell silver (Ag@ C) nanoparticles for colorimetric detection of Chlorpyrifos in agricultural products. JSFA Rep. 2 (10), 511–523. doi:10.1002/jsf2.85
Bilibana, M. P., Citartan, M., Fuku, X., Jijana, A. N., Mathumba, P., and Iwuoha, E. (2022). Aptamers functionalized hybrid nanomaterials for algal toxins detection and decontamination in aquatic system: current progress, opportunities, and challenges. Ecotoxicol. Environ. Saf. 232, 113249. doi:10.1016/j.ecoenv.2022.113249
Bonfante, P., and Anca, I. A. (2009). Plants, mycorrhizal fungi, and bacteria: a network of interactions. Annu. Rev. Microbiol. 63, 363–383. doi:10.1146/annurev.micro.091208.073504
Bora, S., Pooja, D., and Kulhari, H. (2022). “Applications of surface modified carbon nanotubes in drug delivery,” in Surface modified carbon nanotubes: industrial applications (American Chemical Society), 19–46.
Brahmkhatri, V., Pandit, P., Rananaware, P., D’Souza, A., and Kurkuri, M. D. (2021). Recent progress in detection of chemical and biological toxins in Water using plasmonicnanosensors. Trends Environ. Anal. Chem. 30, e00117. doi:10.1016/j.teac.2021.e00117
Buja, I., Sabella, E., Monteduro, A. G., Chiriacò, M. S., De Bellis, L., Luvisi, A., et al. (2021). Advances in plant disease detection and monitoring: from traditional assays to in-field diagnostics. Sensors 21 (6), 2129. doi:10.3390/s21062129
Burke, B., Fan, G., Wasuwanich, P., Moore, E. B., and Furst, A. L. (2023). Self-Assembled nanocoatings protect microbial fertilizers for climate-resilient agriculture. JACS Au 3 (11), 2973–2980. doi:10.1021/jacsau.3c00426
Butnariu, M., and Butu, A. (2019). “Plant nanobionics: application of nanobiosensors in plant biology,” in Plant Nanobionics: Approaches in Nanoparticles, Biosynthesis, and Toxicity, 337–376. doi:10.1007/978-3-030-16379-2_12
Campuzano, S., Kagan, D., Orozco, J., and Wang, J. (2011). Motion-driven sensing and biosensing using electrochemically propelled nanomotors. Analyst 136 (22), 4621–4630. doi:10.1039/c1an15599g
Carbone, G. G., Serra, A., Buccolieri, A., and Manno, D. (2019). A silver nanoparticle-poly (methyl methacrylate) based colorimetric sensor for the detection of hydrogen peroxide. Heliyon 5 (11), e02887. doi:10.1016/j.heliyon.2019.e02887
Chakroborty, S., Pal, K., Nath, N., Singh, V., Barik, A., Soren, S., et al. (2023). Sustainable synthesis of multifunctional nanomaterials from rice wastes: a comprehensive review. Environ. Sci. Pollut. Res. 30 (42), 95039–95053. doi:10.1007/s11356-023-29235-9
Chalmeau, J., Dagkessamanskaia, A., Le Grimellec, C., Francois, J. M., Sternick, J., and Vieu, C. (2009). Contribution to the elucidation of the structure of the bacterial flagellum nano-motor through AFM imaging of the M-Ring. Ultramicroscopy 109 (8), 845–853. doi:10.1016/j.ultramic.2009.03.035
Chang, H. J., Zúñiga, A., Conejero, I., Voyvodic, P. L., Gracy, J., Fajardo-Ruiz, E., et al. (2021). Programmable receptors enable bacterial biosensors to detect pathological biomarkers in clinical samples. Nat. Commun. 12 (1), 5216. doi:10.1038/s41467-021-25538-y
Chen, M., Hou, C., Huo, D., Bao, J., Fa, H., and Shen, C. (2016). An electrochemical DNA biosensor based on nitrogen-doped graphene/Au nanoparticles for human multidrug resistance gene detection. Biosens. Bioelectron. 85, 684–691. doi:10.1016/j.bios.2016.05.051
Chesterfield, R. J., Whitfield, J. H., Pouvreau, B., Cao, D., Alexandrov, K., Beveridge, C. A., et al. (2020). Rational design of novel fluorescent enzyme biosensors for direct detection of strigolactones. ACS Synth. Biol. 9 (8), 2107–2118. doi:10.1021/acssynbio.0c00192
Chodak, M., Klimek, B., and Niklińska, M. (2016). Composition and activity of soil microbial communities in different types of temperate forests. Biol. Fertil. Soils 52, 1093–1104. doi:10.1007/s00374-016-1144-2
Choi, S., Han, S. I., Jung, D., Hwang, H. J., Lim, C., Bae, S., et al. (2018). Highly conductive, stretchable and biocompatible Ag–Au core–sheath nanowire composite for wearable and implantable bioelectronics. Nat. Nanotechnol. 13 (11), 1048–1056. doi:10.1038/s41565-018-0226-8
Cui, H. F., Wu, W. W., Li, M. M., Song, X., Lv, Y., and Zhang, T. T. (2018). A highly stable acetylcholinesterase biosensor based on chitosan-TiO2-graphene nanocomposites for detection of organophosphate pesticides. Biosens. Bioelectron. 99, 223–229. doi:10.1016/j.bios.2017.07.068
Dar, F. A., Qazi, G., and Pirzadah, T. B. (2020). “Nano-biosensors: NextGen diagnostic tools in agriculture,” in Nanobiotechnology in agriculture: an approach towards sustainability, 129–144.
Dasgupta, N., Ranjan, S., Mundekkad, D., Ramalingam, C., Shanker, R., and Kumar, A. (2015). Nanotechnology in agro-food: from field to plate. Food Res. Int. 69, 381–400. doi:10.1016/j.foodres.2015.01.005
Daughtrey, M., and Buitenhuis, R. (2020). “Integrated pest and disease management in greenhouse ornamentals,” in Integrated pest and disease management in greenhouse crops, 625–679.
Dimri, A., Pathak, N., and Sharma, S. (2020). “Nanosensors for root zone parameters influencing plant growth,” in Nanomaterials for agriculture and forestry applications (Elsevier), 387–406.
Dork, D., Anastassiades, M., Spitzke, M., and Dork, D. (2017). Development of a QuEChERS-based method for the simultaneous determination of acidic pesticides, their esters, and conjugates following alkaline hydrolysis. J. Agric. food Chem. 65 (6), 1296–1305. doi:10.1021/acs.jafc.6b05407
Dutta, P., Sharma, V., Bhardwaj, H., Agrawal, V. V., Rajesh, , and Sumana, G. (2022). Fabrication of electrochemical biosensor using zinc oxide nanoflowers for the detection of uric acid. MAPAN 37 (3), 585–595. doi:10.1007/s12647-022-00598-7
Dyussembayev, K., Sambasivam, P., Bar, I., Brownlie, J. C., Shiddiky, M. J., and Ford, R. (2021). Biosensor technologies for early detection and quantification of plant pathogens. Front. Chem. 9, 636245. doi:10.3389/fchem.2021.636245
El-Ansary, A., and Faddah, L. M. (2010). Nanoparticles as biochemical sensors. Nanotechnol. Sci. Appl. 3, 65–76. doi:10.2147/nsa.s8199
Elbasi, E., Zaki, C., Topcu, A. E., Abdelbaki, W., Zreikat, A. I., Cina, E., et al. (2023). Crop prediction model using machine learning algorithms. Appl. Sci. 13 (16), 9288. doi:10.3390/app13169288
Elmer, W., Ma, C., and White, J. (2018). Nanoparticles for plant disease management. Curr. Opin. Environ. Sci. Health 6, 66–70. doi:10.1016/j.coesh.2018.08.002
El-Moneim, D. A., Dawood, M. F., Moursi, Y. S., Farghaly, A. A., Afifi, M., and Sallam, A. (2021). Positive and negative effects of nanoparticles on agricultural crops. Nanotechnol. Environ. Eng. 6 (2), 21. doi:10.1007/s41204-021-00117-0
Erkmen, C., Unal, D. N., Kurbanoglu, S., and Uslu, B. (2022). “Basics of electrochemical sensors,” in Organic electrodes: fundamental to advanced emerging applications (Cham: Springer International Publishing), 81–99.
Eskandari, V., Sahbafar, H., Zeinalizad, L., Mahmoudi, R., Karimpour, F., Hadi, A., et al. (2022). Coating of silver nanoparticles (AgNPs) on glass fibers by a chemical method as plasmonic surface-enhanced Raman spectroscopy (SERS) sensors to detect molecular vibrations of Doxorubicin (DOX) drug in blood plasma. Arabian J. Chem. 15 (8), 104005. doi:10.1016/j.arabjc.2022.104005
Esker, P. D., Sparks, A., Amorim, L., Bergamin Filho, A., Caffi, T., Castilla, N., et al. (2018). Concepts, approaches, and avenues for modelling crop health and crop losses. Eur. J. Agron. 100, 4–18. doi:10.1016/j.eja.2018.04.003
Fahimi-Kashani, N., and Hormozi-Nezhad, M. R. (2016). Gold-nanoparticle-based colorimetric sensor array for discrimination of organophosphate pesticides. Anal. Chem. 88 (16), 8099–8106. doi:10.1021/acs.analchem.6b01616
Fayyaz, K., Nawaz, A., Olaimat, A. N., Akram, K., Farooq, U., Fatima, M., et al. (2022). Microbial toxins in fermented foods: health implications and analytical techniques for detection. J. Food Drug Analysis 30 (4), 523–537. doi:10.38212/2224-6614.3431
Fichman, Y., Miller, G., and Mittler, R. (2019). Whole-plant live imaging of reactive oxygen species. Mol. Plant 12 (9), 1203–1210. doi:10.1016/j.molp.2019.06.003
Ficke, A., Cowger, C., Bergstrom, G., and Brodal, G. (2018). Understanding yield loss and pathogen biology to improve disease management: septoria nodorum blotch-a case study in wheat. Plant Dis. 102 (4), 696–707. doi:10.1094/pdis-09-17-1375-fe
Fountas, S., Mylonas, N., Malounas, I., Rodias, E., Hellmann Santos, C., and Pekkeriet, E. (2020). Agricultural robotics for field operations. Sensors 20 (9), 2672. doi:10.3390/s20092672
Fu, D. J., Li, P., Song, J., Zhang, S. Y., and Xie, H. Z. (2019). Mechanisms of synergistic neurotoxicity induced by two high risk pesticide residues–Chlorpyrifos and Carbofuran via oxidative stress. Toxicol. Vitro 54, 338–344. doi:10.1016/j.tiv.2018.10.016
Ge, Y., Liu, P., Xu, L., Qu, M., Hao, W., Liang, H., et al. (2022). A portable wireless intelligent electrochemical sensor based on layer-by-layer sandwiched nanohybrid for terbutaline in meat products. Food Chem. 371, 131140. doi:10.1016/j.foodchem.2021.131140
Giraldo, J. P., Wu, H., Newkirk, G. M., and Kruss, S. (2019). Nanobiotechnology approaches for engineering smart plant sensors. Nat. Nanotechnol. 14 (6), 541–553. doi:10.1038/s41565-019-0470-6
Girigoswami, A., Ghosh, M. M., Pallavi, P., Ramesh, S., and Girigoswami, K. (2021). Nanotechnology in detection of food toxins–focus on the dairy products. Biointerface Res. Appl. Chem. 11 (6), 14155–14172. doi:10.33263/BRIAC116.1415514172
Goss, E. M., Tabima, J. F., Cooke, D. E., Restrepo, S., Fry, W. E., Forbes, G. A., et al. (2014). The Irish potato famine pathogen Phytophthora infestans originated in central Mexico rather than the Andes. Proc. Natl. Acad. Sci. 111 (24), 8791–8796. doi:10.1073/pnas.1401884111
Ha, N., Xu, K., Ren, G., Mitchell, A., and Ou, J. Z. (2020). Machine learning-enabled smart sensor systems. Adv. Intell. Syst. 2 (9), 2000063. doi:10.1002/aisy.202000063
Ha, N. T., Minh, T. Q., Hoi, P. X., Thuy, N. T. T., Furuya, N., and Long, H. H. (2018). Biological control of potato tuber soft rot using N-acyl-L-homoserine lactone-degrading endophytic bacteria. Curr. Sci. 115 (10), 1921–1927. doi:10.18520/cs/v115/i10/1921-1927
Hamza, R. S. A., and &Habeeb, M. A. (2023). Reinforcement of morphological, structural, optical, and antibacterial characteristics of PVA/CMC bioblend filled with SiO2/Cr2O3 hybrid nanoparticles for optical nanodevices and food packing industries. Polym. Bull., 1–22. doi:10.1007/s00289-023-04913-3
Han, L., Zhao, Y., Cui, S., and Liang, B. (2018). Redesigning of microbial cell surface and its application to whole-cell biocatalysis and biosensors. Appl. Biochem. Biotechnol. 185, 396–418. doi:10.1007/s12010-017-2662-6
Haseeb, K., Almogren, A., Islam, N., Ud Din, I., and Jan, Z. (2019). An energy-efficient and secure routing protocol for intrusion avoidance in IoT-based WSN. Energies 12 (21), 4174. doi:10.3390/en12214174
Hazarika, S., Ahmed, S., and Chamkha, A. J. (2021). Investigation of nanoparticles Cu, Ag and Fe3O4 on thermophoresis and viscous dissipation of MHD nanofluid over a stretching sheet in a porous regime: a numerical modeling. Math. Comput. Simul. 182, 819–837. doi:10.1016/j.matcom.2020.12.005
He, C., Ledezma, U. H., Gurnani, P., Albelha, T., Thurecht, K. J., Correia, R., et al. (2020). Surface polymer imprinted optical fibre sensor for dose detection of dabrafenib. Analyst 145 (13), 4504–4511. doi:10.1039/d0an00434k
Hosseini, M., Khabbaz, H., Dadmehr, M., Ganjali, M. R., and Mohamadnejad, J. (2015). Aptamer-based colorimetric and chemiluminescence detection of aflatoxin B1 in foods samples. ActaChimicaSlovenica 62 (3), 721–728. doi:10.17344/acsi.2015.1358
Hou, C., Zhang, X., Wang, L., Zhang, F., Huang, X., and Wang, Z. (2020). A buckypaper decorated with CoP/Co for nonenzymatic amperometric sensing of glucose. Microchim. Acta 187, 101–108. doi:10.1007/s00604-019-4076-3
Hou, W., Zhang, Q., Dong, H., Li, F., Zhang, Y., Guo, Y., et al. (2019). Acetylcholinesterase biosensor modified with ATO/OMC for detecting organophosphorus pesticides. New J. Chem. 43 (2), 946–952. doi:10.1039/c8nj03429j
Hsu, C. W., Lin, Z. Y., Chan, T. Y., Chiu, T. C., and Hu, C. C. (2017). Oxidized multiwalled carbon nanotubes decorated with silver nanoparticles for fluorometric detection of dimethoate. Food Chem. 224, 353–358. doi:10.1016/j.foodchem.2016.12.095
Huang, H., Bai, W., Dong, C., Guo, R., and Liu, Z. (2015). An ultrasensitive electrochemical DNA biosensor based on graphene/Au nanorod/polythionine for human papillomavirus DNA detection. Biosens. Bioelectron. 68, 442–446. doi:10.1016/j.bios.2015.01.039
Huang, H., Wang, D., Zhou, Y., Wu, D., Liao, X., Xiong, W., et al. (2021a). Multiwalled carbon nanotubes modified two dimensional MXene with high antifouling property for sensitive detection of ochratoxin A. Nanotechnology 32 (45), 455501. doi:10.1088/1361-6528/ac1a42
Huang, X., Zhu, Y., and Kianfar, E. (2021b). Nano biosensors: properties, applications and electrochemical techniques. J. Mater. Res. Technol. 12, 1649–1672. doi:10.1016/j.jmrt.2021.03.048
Hwa, K. Y., Santhan, A., and Sharma, T. S. K. (2022). One-dimensional self-assembled Co2SnO4 nanosphere to nanocubes intertwined in two-dimensional reduced graphene oxide: an intriguing electrocatalytic sensor toward mesalamine detection. Mater. Today Chem. 23, 100739. doi:10.1016/j.mtchem.2021.100739
Ijaz, U., Ahmed, T., Rizwan, M., Noman, M., Shah, A. A., Azeem, F., et al. (2023). Rice straw based silicon nanoparticles improve morphological and nutrient profile of rice plants under salinity stress by triggering physiological and genetic repair mechanisms. Plant Physiology Biochem. 201, 107788. doi:10.1016/j.plaphy.2023.107788
Ivanov, S., Zhuravsky, S., Yukina, G., Tomson, V., Korolev, D., and Galagudza, M. (2012). In vivo toxicity of intravenously administered silica and silicon nanoparticles. Materials 5 (10), 1873–1889. doi:10.3390/ma5101873
Jallow, S., and Govender, N. P. (2021). Ibrexafungerp: a first-in-class oral triterpenoid glucan synthase inhibitor. J. Fungi 7 (3), 163. doi:10.3390/jof7030163
James, A., Franco, F., Merca, E. M., Rodriguez, S., Johny, F., Balidon-Veronica, P., et al. (2019). DNA-based electrochemical nanobiosensor for the detection of Phytophthora palmivora (Butler) Butler, causing black pod rot in cacao (Theobroma cacao L.) pods. Physiol. Mol. Plant Pathol. 107, 14–20. doi:10.1016/j.pmpp.2019.04.004
Jeerapan, I., Sonsa-Ard, T., and &Nacapricha, D. (2020). Applying nanomaterials to modern biomedical electrochemical detection of metabolites, electrolytes, and pathogens. Chemosensors 8 (3), 71. doi:10.3390/chemosensors8030071
Jia, X., Wang, K., Li, X., Liu, Z., Liu, Y., Xiao, R., et al. (2022). Highly sensitive detection of three protein toxins via SERS-lateral flow immunoassay based on SiO2@ Au nanoparticles. Nanomedicine Nanotechnol. Biol. Med. 41, 102522. doi:10.1016/j.nano.2022.102522
Jiang, Y., Huo, S., Mizuhara, T., Das, R., Lee, Y. W., Hou, S., et al. (2015). The interplay of size and surface functionality on the cellular uptake of sub-10 nm gold nanoparticles. ACS Nano 9 (10), 9986–9993. doi:10.1021/acsnano.5b03521
Jin, Z. H., Liu, Y. L., Chen, J. J., Cai, S. L., Xu, J. Q., and Huang, W. H. (2017). Conductive polymer-coated carbon nanotubes to construct stretchable and transparent electrochemical sensors. Anal. Chem. 89 (3), 2032–2038. doi:10.1021/acs.analchem.6b04616
John, E. M., and Shaike, J. M. (2015). Chlorpyrifos: pollution and remediation. Environ. Chem. Lett. 13, 269–291. doi:10.1007/s10311-015-0513-7
Jung, E., Kang, C., Lee, J., Yoo, D., Hwang, D. W., Kim, D., et al. (2018). Molecularly engineered theranostic nanoparticles for thrombosed vessels: H2O2-activatable contrast-enhanced photoacoustic imaging and antithrombotic therapy. Acs Nano 12 (1), 392–401. doi:10.1021/acsnano.7b06560
Kaittanis, C., Naser, S. A., and Perez, J. M. (2007). One-step, nanoparticle-mediated bacterial detection with magnetic relaxation. Nano Lett. 7 (2), 380–383. doi:10.1021/nl062553z
Kariuki, V. M., Fasih-Ahmad, S. A., Osonga, F. J., and Sadik, O. A. (2016). An electrochemical sensor for nitrobenzene using π-conjugated polymer-embedded nanosilver. Analyst 141 (7), 2259–2269. doi:10.1039/c6an00029k
Kaushal, M., and Wani, S. P. (2017). Nanosensors: frontiers in precision agriculture. Nanotechnol. Agric. paradigm, 279–291. doi:10.1007/978-981-10-4573-8_13
Kelli, V., Radoglou-Grammatikis, P., Lagkas, T., Markakis, E. K., and Sarigiannidis, P. (2022). “Risk analysis of DNP3 attacks,” in 2022 IEEE international conference on cyber security and resilience (CSR) (IEEE), 351–356.
Khalili, H., Brocchini, S., Khaw, P. T., and Filippov, S. K. (2018). Comparative thermodynamic analysis in solution of a next generation antibody mimetic to VEGF. RSC Adv. 8 (62), 35787–35793. doi:10.1039/c8ra07059h
Khan, M. R., and Rizvi, T. F. (2014). Nanotechnology: scope and application in plant disease management. Plant Pathol. J. 13 (3), 214–231. doi:10.3923/ppj.2014.214.231
Kokate, M., Garadkar, K., and Gole, A. (2013). One pot synthesis of magnetite–silica nanocomposites: applications as tags, entrapment matrix and in water purification. J. Mater. Chem. A 1 (6), 2022–2029. doi:10.1039/c2ta00951j
Kong, L., Gan, Y., Wang, T., Sun, X., Ma, C., Wang, X., et al. (2023). Single-stranded DNA binding protein coupled aptasensor with carbon-gold nanoparticle amplification for marine toxins detection assisted by a miniaturized absorbance reader. J. Hazard. Mater. 450, 131023. doi:10.1016/j.jhazmat.2023.131023
Kotinagu, K., and Krishnaiah, N. (2015). Organochlorine and organophosphorus pesticide residues in fodder and milk samples along Musi river belt, India. Veterinary world 8 (4), 544–550. doi:10.14202/vetworld.2015.544-550
Kumar, A., and Verma, J. P. (2018). Does plant—microbe interaction confer stress tolerance in plants: a review? Microbiol. Res. 207, 41–52. doi:10.1016/j.micres.2017.11.004
Kumari, M., Pandey, S., Giri, V. P., Nautiyal, C. S., and Mishra, A. (2023). A critical review on green approaches in shape and size evolution of metal nanoparticles and their environmental applications. in Environmental nanotechnology, Monitoring and Management, 100895. doi:10.1016/j.enmm.2023.100895
Lakshmanan, V., Selvaraj, G., and Bais, H. P. (2014). Functional soil microbiome: belowground solutions to an aboveground problem. Plant physiol. 166 (2), 689–700. doi:10.1104/pp.114.245811
Lei, Y., Wang, M., Wan, A., Xia, C., See, D. R., Zhang, M., et al. (2017). Virulence and molecular characterization of experimental isolates of the stripe rust pathogen (Puccinia striiformis) indicate somatic recombination. Phytopathology 107 (3), 329–344. doi:10.1094/phyto-07-16-0261-r
Lew, T. T. S., Koman, V. B., Silmore, K. S., Seo, J. S., Gordiichuk, P., Kwak, S. Y., et al. (2020). Real-time detection of wound-induced H2O2 signalling waves in plants with optical nanosensors. Nat. plants 6 (4), 404–415. doi:10.1038/s41477-020-0632-4
Li, H., Lin, H., Lv, W., Gai, P., and Li, F. (2020). Equipment-free and visual detection of multiple biomarkers via an aggregation induced emission luminogen-based paper biosensor. Biosens. Bioelectron. 165, 112336. doi:10.1016/j.bios.2020.112336
Li, H., Qi, H., Chang, J., Gai, P., and Li, F. (2022). Recent progress in homogeneous electrochemical sensors and their designs and applications. TrAC Trends Anal. Chem. 156, 116712. doi:10.1016/j.trac.2022.116712
Li, L., Chen, D., Chen, J., Yang, C., Zeng, Y., Jin, T., et al. (2023). Gelatin and catechol-modified quaternary chitosan cotton dressings with rapid hemostasis and high-efficiency antimicrobial capacity to manage severe bleeding wounds. Mater. Des. 229, 111927. doi:10.1016/j.matdes.2023.111927
Li, L., Ding, Y., Xu, L., Chen, S., Dai, G., Han, P., et al. (2024). Visualized time-temperature monitoring by triplet-sensitized ratiometric fluorescent nanosensors. Sensors Actuators B Chem. 400, 134900. doi:10.1016/j.snb.2023.134900
Li, L., Zhao, C., Zhang, Y., Yao, J., Yang, W., Hu, Q., et al. (2017). Effect of stable antimicrobial nano-silver packaging on inhibiting mildew and in storage of rice. Food Chem. 215, 477–482. doi:10.1016/j.foodchem.2016.08.013
Li, M., Zeng, Y., Huang, Z., Zhang, L., and Liu, Y. (2023). Vertical graphene-based printed electrochemical biosensor for simultaneous detection of four Alzheimer’s disease blood biomarkers. Biosensors 13 (8), 758. doi:10.3390/bios13080758
Li, Y., and Li, H. (2014). Type IV pili of Acidithiobacillus ferrooxidans can transfer electrons from extracellular electron donors. J. Basic Microbiol. 54 (3), 226–231. doi:10.1002/jobm.201200300
Li, Z., Li, F., Xing, Y., Liu, Z., You, M., Li, Y., et al. (2017). Pen-on-paper strategy for point-of-care testing: rapid prototyping of fully written microfluidic biosensor. Biosens. Bioelectron. 98, 478–485. doi:10.1016/j.bios.2017.06.061
Lisa, M., Chouhan, R. S., Vinayaka, A. C., Manonmani, H. K., and Thakur, M. S. (2009). Gold nanoparticles based dipstick immunoassay for the rapid detection of dichlorodiphenyltrichloroethane: an organochlorine pesticide. Biosens. Bioelectron. 25 (1), 224–227. doi:10.1016/j.bios.2009.05.006
Liu, H., Chen, P., Liu, Z., Liu, J., Yi, J., Xia, F., et al. (2020). Electrochemical luminescence sensor based on double suppression for highly sensitive detection of glyphosate. Sensors Actuators B Chem. 304, 127364. doi:10.1016/j.snb.2019.127364
Liu, H., Chen, Q., Cheng, X., Wang, Y., Zhang, Y., and Fan, G. (2020). Sustainable and scalable in-situ fabrication of Au nanoparticles and Fe3O4 hybrids as highly efficient electrocatalysts for the enzyme-free sensing of H2O2 in neutral and basic solutions. Sensors Actuators B Chem. 314, 128067. doi:10.1016/j.snb.2020.128067
Liu, P., Zhao, M., Zhu, H., Zhang, M., Li, X., Wang, M., et al. (2022). Dual-mode fluorescence and colorimetric detection of pesticides realized by integrating stimulus-responsive luminescence with oxidase-mimetic activity into cerium-based coordination polymer nanoparticles. J. Hazard. Mater. 423, 127077. doi:10.1016/j.jhazmat.2021.127077
Liu, Z., Zhang, Y., Bian, C., Xia, T., Gao, Y., Zhang, X., et al. (2019). Highly sensitive microbial biosensor based on recombinant Escherichia coli overexpressing catechol 2, 3-dioxygenase for reliable detection of catechol. Biosens. Bioelectron. 126, 51–58. doi:10.1016/j.bios.2018.10.050
Lowry, G. V., Avellan, A., and Gilbertson, L. M. (2019). Opportunities and challenges for nanotechnology in the agri-tech revolution. Nat. Nanotechnol. 14 (6), 517–522. doi:10.1038/s41565-019-0461-7
Lu, C., Gao, X., Chen, Y., Ren, J., and Liu, C. (2020). Aptamer-based lateral flow test strip for the simultaneous detection of Salmonella typhimurium, Escherichia coli O157: H7 and Staphylococcus aureus. Anal. Lett. 53 (4), 646–659. doi:10.1080/00032719.2019.1663528
Ma, J., Yu, J., Chen, G., Bai, Y., Liu, S., Hu, Y., et al. (2023). Rational design of N-doped carbon-coated cobalt nanoparticles for highly efficient and durable photothermal CO2 conversion. Adv. Mater. 35 (42), 2302537. doi:10.1002/adma.202302537
Magarelli, G., Freire, A. M., and Silva, L. P. (2023). “Electrochemical sensors coupled with machine learning for food safety and quality inspection,” in Food quality analysis (Academic Press), 171–200.
Mahmoudi, E., Fakhri, H., Hajian, A., Afkhami, A., and Bagheri, H. (2019). High-performance electrochemical enzyme sensor for organophosphate pesticide detection using modified metal-organic framework sensing platforms. Bioelectrochemistry 130, 107348. doi:10.1016/j.bioelechem.2019.107348
Malhotra, B. D., and Ali, M. A. (2018). “Nanomaterials in biosensors: fundamentals and applications,” in Nanomaterials for biosensors, 1.
Marin, M., Nikolic, M. V., and Vidic, J. (2021). Rapid point-of-need detection of bacteria and their toxins in food using gold nanoparticles. Compr. Rev. Food Sci. Food Saf. 20 (6), 5880–5900. doi:10.1111/1541-4337.12839
Martinazzo., J., Moraes, M. C. B., Steffens, J., and Steffens, C. (2022). Application of gas nanosensor for detection pheromone and its interferents compounds in vivo Euschistus heros (F.) stink bugs insects. Sensors Actuators A Phys. 345, 113804. doi:10.1016/j.sna.2022.113804
Mfarrej, M. F. B., and Rara, F. M. (2019). Competitive, sustainable natural pesticides. ActaEcologicaSinica 39 (2), 145–151. doi:10.1016/j.chnaes.2018.08.005
Mishra, A., Kumar, J., Melo, J. S., and Sandaka, B. P. (2021). Progressive development in biosensors for detection of dichlorvos pesticide: a review. J. Environ. Chem. Eng. 9 (2), 105067. doi:10.1016/j.jece.2021.105067
Mittal, D., Kaur, G., Singh, P., Yadav, K., and Ali, S. A. (2020). Nanoparticle-based sustainable agriculture and food science: recent advances and future outlook. Front. Nanotechnol. 2, 579954. doi:10.3389/fnano.2020.579954
Munir, N., Gulzar, W., Abideen, Z., Hancook, J. T., El-Keblawy, A., and Radicetti, E. (2023). Nanotechnology improves disease resistance in plants for food security: applications and challenges. Biocatal. Agric. Biotechnol. 51, 102781. doi:10.1016/j.bcab.2023.102781
Mustafa, F., and Andreescu, S. (2020). Nanotechnology-based approaches for food sensing and packaging applications. RSC Adv. 10 (33), 19309–19336. doi:10.1039/d0ra01084g
Negrete, J. C. (2020). Nanotechnology an option in Mexican agriculture. J. Biotechnol. Bioinforma. Res. 2 (2), 1–3. doi:10.47363/jbbr/2020(2)106
Nile, S. H., Baskar, V., Selvaraj, D., Nile, A., Xiao, J., and Kai, G. (2020). Nanotechnologies in food science: applications, recent trends, and future perspectives. Nano-micro Lett. 12, 45–34. doi:10.1007/s40820-020-0383-9
Oymen, B., Jalilzadeh, M., Yılmaz, F., Aşır, S., Türkmen, D., and &Denizli, A. (2023). Simple and fast pesticide nanosensors: example of surface plasmon resonance CoumaphosNanosensor. Micromachines 14 (4), 707. doi:10.3390/mi14040707
Patel, P., and Geed, S. R. (2024). “Recent advancements in the application of nanomaterial in modern drug delivery and future perspective,” in Biogenic nanomaterials for environmental sustainability: principles, practices, and opportunities (Cham: Springer International Publishing), 319–351.
Pathakoti, K., Manubolu, M., and Hwang, H. M. (2017). Nanostructures: current uses and future applications in food science. J. food drug analysis 25 (2), 245–253. doi:10.1016/j.jfda.2017.02.004
Petrov, A., and Audette, G. F. (2012). Peptide and protein-based nanotubes for nanobiotechnology. Wiley Interdiscip. Rev. Nanomedicine Nanobiotechnology 4 (5), 575–585. doi:10.1002/wnan.1180
Prasad, R., Bhattacharyya, A., and Nguyen, Q. D. (2017). Nanotechnology in sustainable agriculture: recent developments, challenges, and perspectives. Front. Microbiol. 8, 1014. doi:10.3389/fmicb.2017.01014
Priyadarshini, P., Tiwari, K., Das, A., Kumar, D., Mishra, M. N., Desikan, P., et al. (2017). Evaluation of highly conserved hsp65-specific nested PCR primers for diagnosing Mycobacterium tuberculosis. Int. J. Tuberc. Lung Dis. 21 (2), 214–217. doi:10.5588/ijtld.16.0343
Pujol-Vila, F., Villa, R., and Alvarez, M. (2020). Nanomechanical sensors as a tool for bacteria detection and antibiotic susceptibility testing. Front. Mech. Eng. 6, 44. doi:10.3389/fmech.2020.00044
Qin, Y., He, K., Chen, B., Ji, Z., and Wang, J. (2023). Ultrathin 2D/2D nanohybrid for efficient electrochemical detection of nitrite. Applied Surface Science, 157776. doi:10.1016/j.apsusc.2023.157776
Rahman, M. M., Ahmed, L., Anika, F., Riya, A. A., Kali, S. K., Rauf, A., et al. (2023). Bioinorganic nanoparticles for the remediation of environmental pollution: critical appraisal and potential avenues. Bioinorganic Chemistry and Applications 202. doi:10.1155/2023/2409642
Rajput, J. K. (2022). Nanomaterial-based sensors as potential remedy for detection of biotoxins. Food control. 135, 108686. doi:10.1016/j.foodcont.2021.108686
Reguera, G., McCarthy, K. D., Mehta, T., Nicoll, J. S., Tuominen, M. T., and Lovley, D. R. (2005). Extracellular electron transfer via microbial nanowires. Nature 435 (7045), 1098–1101. doi:10.1038/nature03661
Ren, Y., Gao, P., Song, Y., Yang, X., Yang, T., Chen, S., et al. (2021). An aptamer-exonuclease III (Exo III)–assisted amplification-based lateral flow assay for sensitive detection of Escherichia coli O157: H7 in milk. J. Dairy Sci. 104 (8), 8517–8529. doi:10.3168/jds.2020-19939
Romanovski, V., and Periakaruppan, R. (2023). “Why metal oxide nanoparticles are superior to other nanomaterials for agricultural application?,” in Nanometal oxides in horticulture and agronomy (Academic Press), 7–18.
Sabet, F. S., Hosseini, M., Khabbaz, H., Dadmehr, M., and Ganjali, M. R. (2017). FRET-based aptamer biosensor for selective and sensitive detection of aflatoxin B1 in peanut and rice. Food Chem. 220, 527–532. doi:10.1016/j.foodchem.2016.10.004
Salouti, M., and KhadiviDerakhshan, F. (2020). “Biosensors and nanobiosensors in environmental applications,” in Biogenic nano-particles and their use in agro-ecosystems, 515–591.
Sarkar, R., Farghaly, A. A., and Arachchige, I. U. (2022). Oxidative self-assembly of Au/Ag/Pt alloy nanoparticles into high-surface area, mesoporous, and conductive aerogels for methanol electro-oxidation. Chem. Mater. 34 (13), 5874–5887. doi:10.1021/acs.chemmater.2c00717
Saxena, K. K., Qian, J., and Reynaerts, D. (2018). A review on process capabilities of electrochemical micromachining and its hybrid variants. Int. J. Mach. tools Manuf. 127, 28–56. doi:10.1016/j.ijmachtools.2018.01.004
Scanlon, S., and Aggeli, A. (2008). Self-assembling peptide nanotubes. Nano Today 3 (3-4), 22–30. doi:10.1016/s1748-0132(08)70041-0
Schieber, A. (2018). “Introduction to food authentication,” in Modern techniques for food authentication (Academic Press), 1–21.
Sepunaru, L., Plowman, B. J., Sokolov, S. V., Young, N. P., and Compton, R. G. (2016). Rapid electrochemical detection of single influenza viruses tagged with silver nanoparticles. Chem. Sci. 7 (6), 3892–3899. doi:10.1039/c6sc00412a
Shafa, M., Ahmad, I., Hussain, S., Asif, M., Pan, Y., Zairov, R., et al. (2023). Ag-Cu nanoalloys: an electrochemical sensor for H2O2 detection. Surfaces Interfaces 36, 102616. doi:10.1016/j.surfin.2022.102616
Shah, A. (2020). A novel electrochemical nanosensor for the simultaneous sensing of two toxic food dyes. ACS omega 5 (11), 6187–6193. doi:10.1021/acsomega.0c00354
Sharma, A., Mishra, R. K., Goud, K. Y., Mohamed, M. A., Kummari, S., Tiwari, S., et al. (2021). Optical biosensors for diagnostics of infectious viral disease: a recent update. Diagnostics 11 (11), 2083. doi:10.3390/diagnostics11112083
Sies, H. (2017). Hydrogen peroxide as a central redox signaling molecule in physiological oxidative stress: oxidative eustress. Redox Biol. 11, 613–619. doi:10.1016/j.redox.2016.12.035
Silins, I., Korhonen, A., and Stenius, U. (2014). Evaluation of carcinogenic modes of action for pesticides in fruit on the Swedish market using a text-mining tool. Front. Pharmacol. 5, 145. doi:10.3389/fphar.2014.00145
Singh, P., Khan, D., and Kumar, A. (2024). “Introduction to nanopesticides, nanoherbicides, and nanofertilizers,” in Nanopesticides, nanoherbicides, and nanofertilizers (CRC Press), 1–25. doi:10.1201/9781003364429-1
Singh, R. P. (2021). “Recent trends, prospects, and challenges of nanobiosensors in agriculture,” in Biosensors in agriculture: recent trends and future perspectives, 3–13.
Singh, S., Singh, M., Agrawal, V. V., and Kumar, A. (2010). An attempt to develop surface plasmon resonance based immunosensor for Karnal bunt (Tilletia indica) diagnosis based on the experience of nano-gold based lateral flow immuno-dipstick test. Thin Solid Films 519 (3), 1156–1159. doi:10.1016/j.tsf.2010.08.061
Smirnoff, N., and Arnaud, D. (2019). Hydrogen peroxide metabolism and functions in plants. New phytol. 221 (3), 1197–1214. doi:10.1111/nph.15488
Soundy, J., and Day, D. (2017). Selection of DNA aptamers specific for live Pseudomonas aeruginosa. PLoS One 12 (9), e0185385. doi:10.1371/journal.pone.0185385
Su, L. J., Zhang, J. H., Gomez, H., Murugan, R., Hong, X., Xu, D., et al. (2019). “Reactive oxygen species-induced lipid peroxidation in apoptosis, autophagy, and ferroptosis,” in Oxidative medicine and cellular longevity.
Sun, Y., Shi, L., Mi, L., Guo, R., and Li, T. (2020). Recent progress of SERS optical nanosensors for miRNA analysis. J. Mater. Chem. B 8 (24), 5178–5183. doi:10.1039/d0tb00280a
Sunaryani, A., and Rosmalina, R. T. (2021). Persistence of carbaryl pesticide in environment using system dynamics model IOP Conference Series: earth and Environmental Science. IOP Publ. 623 (No. 1), 012048. doi:10.1088/1755-1315/623/1/012048
Swierczewska, M., Liu, G., Lee, S., and Chen, X. (2012). High-sensitivity nanosensors for biomarker detection. Chem. Soc. Rev. 41 (7), 2641–2655. doi:10.1039/c1cs15238f
Tanwar, A., and Sushil, S. (2019). “Role and effects of nanotechnology used in pesticides and agriculture field,” in AIP conference proceedings (AIP Publishing), 2142. doi:10.1063/1.5122581
Taton, T. A., Mirkin, C. A., and Letsinger, R. L. (2000). Scanometric DNA array detection with nanoparticle probes. science 289 (5485), 1757–1760. doi:10.1126/science.289.5485.1757
Thakur, M., Wang, B., and Verma, M. L. (2022). Development and applications of nanobiosensors for sustainable agricultural and food industries: recent developments, challenges and perspectives. Environmental Technology and Innovation 26, 102371. doi:10.1016/j.eti.2022.102371
Tsong, J. L., and Khor, S. M. (2023). Modern analytical and bioanalytical technologies and concepts for smart and precision farming. Anal. Methods 15 (26), 3125–3148. doi:10.1039/d3ay00647f
Umapathi, R., Ghoreishian, S. M., Sonwal, S., Rani, G. M., and Huh, Y. S. (2022). Portable electrochemical sensing methodologies for on-site detection of pesticide residues in fruits and vegetables. Coord. Chem. Rev. 453, 214305. doi:10.1016/j.ccr.2021.214305
Vaseashta, A. (2020). “Cyber-physical systems—nanomaterial sensors based unmanned aerial platforms for real-time monitoring and analysis,” in 4th international conference on nanotechnologies and biomedical engineering: proceedings of ICNBME-2019, september 18-21, 2019, chisinau, moldova (Springer International Publishing), 685–689.
Vaseghi, A., Safaie, N., Bakhshinejad, B., Mohsenifar, A., and Sadeghizadeh, M. (2013). Detection of Pseudomonas syringae pathovars by thiol-linked DNA–Gold nanoparticle probes. Sensors Actuators B Chem. 181, 644–651. doi:10.1016/j.snb.2013.02.018
Verdian, A. (2018). Apta-nanosensors for detection and quantitative determination of acetamiprid–A pesticide residue in food and environment. Talanta 176, 456–464. doi:10.1016/j.talanta.2017.08.070
Verma, D., Ranjan, K. R., Mukherjee, M. D., and Solanki, P. R. (2022). Bioinspired synthesis of hematite nanoparticles-reduced graphene oxide composite for application in bisphenol a detection: a new in-sight. Biosens. Bioelectron. 11, 100217. doi:10.1016/j.biosx.2022.100217
Verma, M. L. (2017). Nanobiotechnology advances in enzymatic biosensors for the agri-food industry. Environ. Chem. Lett. 15 (4), 555–560. doi:10.1007/s10311-017-0640-4
Verma, M. L., and Rani, V. (2021). Biosensors for toxic metals, polychlorinated biphenyls, biological oxygen demand, endocrine disruptors, hormones, dioxin, phenolic and organophosphorus compounds: a review. Environ. Chem. Lett. 19 (2), 1657–1666. doi:10.1007/s10311-020-01116-4
Vineeta, M., and Sachin, K. (2013). Prevalence of inducible clindamycin resistance among clinical isolates of Staphylococcus aureus detected by phenotypic method: a preliminary report. J. Infect. Dis. Immun. 5 (1), 10–12. doi:10.5897/jidi12.005
Wahabzada, M., Mahlein, A. K., Bauckhage, C., Steiner, U., Oerke, E. C., and Kersting, K. (2016). Plant phenotyping using probabilistic topic models: uncovering the hyperspectral language of plants. Sci. Rep. 6 (1), 22482. doi:10.1038/srep22482
Wan, Y., Lin, Z., Zhang, D., Wang, Y., and &Hou, B. (2011). Impedimetricimmunosensor doped with reduced graphene sheets fabricated by controllable electrodeposition for the non-labelled detection of bacteria. Biosens. Bioelectron. 26 (5), 1959–1964. doi:10.1016/j.bios.2010.08.008
Wang, H., Gu, M., Yan, M., Wu, X., Dong, Y., and Wang, G. L. (2023). Catechol exerts an in situ surface oxygen vacancy effect on BiOI: an innovative signal transduction mode for cathodic photoelectrochemistry. J. Mater. Chem. B 11 (23), 5123–5130. doi:10.1039/d3tb00566f
Wang, M., Xu, Y., Yang, Y., Mu, B., Nikitina, M. A., and Xiao, X. (2022). Vis/NIR optical biosensors applications for fruit monitoring. Biosens. Bioelectron. 11, 100197. doi:10.1016/j.biosx.2022.100197
Willner, M. R., and Vikesland, P. J. (2018). Nanomaterial enabled sensors for environmental contaminants. J. nanobiotechnology 16 (1), 95–16. doi:10.1186/s12951-018-0419-1
Wlodkowic, D., and Karpiński, T. M. (2021). Live-cell systems in real-time biomonitoring of water pollution: practical considerations and future perspectives. Sensors 21 (21), 7028. doi:10.3390/s21217028
Wu, H., Nißler, R., Morris, V., Herrmann, N., Hu, P., Jeon, S. J., et al. (2020). Monitoring plant health with near-infrared fluorescent H2O2 nanosensors. Nano Lett. 20 (4), 2432–2442. doi:10.1021/acs.nanolett.9b05159
Wu, S., Duan, N., Shi, Z., Fang, C., and Wang, Z. (2014). Simultaneous aptasensor for multiplex pathogenic bacteria detection based on multicolor upconversion nanoparticles labels. Anal. Chem. 86 (6), 3100–3107. doi:10.1021/ac404205c
Xu, L., Shoaie, N., Jahanpeyma, F., Zhao, J., Azimzadeh, M., and Al, K. T. (2020). Optical, electrochemical and electrical (nano) biosensors for detection of exosomes: a comprehensive overview. Biosens. Bioelectron. 161, 112222. doi:10.1016/j.bios.2020.112222
Yan, Y., Chen, X., Huang, J., Huan, C., and Li, C. (2022). H2O2-induced oxidative s impairs meat quality by inducing apoptosis and autophagy via ROS/NF-κB signaling pathway in broiler thigh muscle. Poult. Sci. 101 (4), 101759. doi:10.1016/j.psj.2022.101759
Yang, J., Shen, M., Luo, Y., Wu, T., Chen, X., Wang, Y., et al. (2021). Advanced applications of chitosan-based hydrogels: from biosensors to intelligent food packaging system. Trends Food Sci. Technol. 110, 822–832. doi:10.1016/j.tifs.2021.02.032
Yang, Q., Rosati, G., Abarintos, V., Aroca, M. A., Osma, J. F., and Merkoçi, A. (2022). Wearable and fully printed microfluidic nanosensor for sweat rate, conductivity, and copper detection with healthcare applications. Biosens. Bioelectron. 202, 114005. doi:10.1016/j.bios.2022.114005
Yang, T., Wang, Z., Song, Y., Yang, X., Chen, S., Fu, S., et al. (2021). A novel smartphone-based colorimetric aptasensor for on-site detection of Escherichia coli O157: H7 in milk. J. Dairy Sci. 104 (8), 8506–8516. doi:10.3168/jds.2020-19905
Yılmaz, F., Saylan, Y., Akgonullu, S., Çimen, D., Derazshamshir, A., Bereli, N., et al. (2017). “Surface plasmon resonance based nanosensors for detection of triazinic pesticides in agricultural foods,” in New pesticides and soil sensors (Academic Press), 679–718.
Yu, J., Wang, D., Geetha, N., Khawar, K. M., Jogaiah, S., and Mujtaba, M. (2021). Current trends and challenges in the synthesis and applications of chitosan-based nanocomposites for plants: a review. Carbohydr. Polym. 261, 117904. doi:10.1016/j.carbpol.2021.117904
Zeinhom, M. M. A., Wang, Y., Song, Y., Zhu, M. J., Lin, Y., and Du, D. (2018). A portable smart-phone device for rapid and sensitive detection of E. coli O157: H7 in Yoghurt and Egg. Biosens. Bioelectron. 99, 479–485. doi:10.1016/j.bios.2017.08.002
Zhang, J., Ma, X., and Wang, Z. (2019). Surface-enhanced Raman scattering-fluorescence dual-mode nanosensors for quantitative detection of cytochrome c in living cells. Anal. Chem. 91 (10), 6600–6607. doi:10.1021/acs.analchem.9b00480
Zhang, X., Zhang, W., and Zhao, H. (2022). Ultrasound-assisted fabrication of Ti3C2Tx MXene toward enhanced energy storage performance. Ultrason. Sonochemistry 86, 106024. doi:10.1016/j.ultsonch.2022.106024
Zhang, Y., Cai, N., and Chan, V. (2023). Recent advances in silicon quantum dot-based fluorescent biosensors. Biosensors 13 (3), 311. doi:10.3390/bios13030311
Keywords: nanotechnology, nanobiosensors, diagnosis, food sector, agriculture
Citation: Virk V, Deepak H, Taneja K, Srivastava R and Giri S (2024) Amelioration in nanobiosensors for the control of plant diseases: current status and future challenges. Front. Nanotechnol. 6:1310165. doi: 10.3389/fnano.2024.1310165
Received: 09 October 2023; Accepted: 30 January 2024;
Published: 27 March 2024.
Edited by:
Ramesh Namdeo Pudake, Amity University, IndiaReviewed by:
Kshitij R. B. Singh, Kyushu Institute of Technology, JapanCopyright © 2024 Virk, Deepak, Taneja, Srivastava and Giri. This is an open-access article distributed under the terms of the Creative Commons Attribution License (CC BY). The use, distribution or reproduction in other forums is permitted, provided the original author(s) and the copyright owner(s) are credited and that the original publication in this journal is cited, in accordance with accepted academic practice. No use, distribution or reproduction is permitted which does not comply with these terms.
*Correspondence: Verinder Virk, dmlyZW5kZXIud2FobGFAZ2t2LmFjLmlu
Disclaimer: All claims expressed in this article are solely those of the authors and do not necessarily represent those of their affiliated organizations, or those of the publisher, the editors and the reviewers. Any product that may be evaluated in this article or claim that may be made by its manufacturer is not guaranteed or endorsed by the publisher.
Research integrity at Frontiers
Learn more about the work of our research integrity team to safeguard the quality of each article we publish.