- 1Department of Biotechnology, Institute of Applied Sciences & Humanities, GLA University, Mathura, Uttar Pradesh, India
- 2Department of Physiology and Pharmacology Vittorio Erspamer, Sapienza University of Rome, Rome, Italy
Advances in nanotechnology have attracted a lot of potential medical applications, such as therapeutic agents, diagnostics, and theranostics for complex diseases. The intersection of nanotechnologies, molecular and cell biology, and medicine can function to improve human health and quality of life, making healthcare a primary target of nanotechnology research. However, this seems like a promising future, ethical, health, and safety concerns must be considered before a reasoned evaluation of the situation can be made. Most nanostructures, however, typically fail to reach their intended targets because they get trapped inside innate immune cells. Since little is known about how nanomaterials and nanotechnologies change their identity inside the biological system, there is a wide-ranging discussion on possible concerns. In this regard, we present a perspective on how biological systems may interact with nanoscale materials and how that interaction might affect cellular recognition of nanostructures. We will also discuss dynamic modifications of the nanomaterials inside biological systems and, in particular, inflammation responses.
Introduction
Nano-bio interfaces are where nanoscale surfaces meet biology to control metabolism, the immune system, the cell cycle, and disease-associated pathways. The term “nanostructures” encompasses various materials with diverse sizes and shapes. Nanostructures of superparamagnetic iron oxide, silver, gold, lipids, carbon-based, protein, and polymers exhibit therapeutic, imaging, and diagnostic applications (Vieira Rocha et al., 2020). Owing to their unique characteristics like high aspect ratio, surface area, loading capacity, and tunable surface chemistry, nanotechnology is a potential platform against immune-related diseases and for targeted and controlled delivery of drugs and vaccines (Li et al., 2019; Liu et al., 2020). Nanotechnology broadly focuses on improving the physicochemical properties of drugs, such as aqueous solubility, pharmacokinetics, therapeutic index, targeted and controlled delivery to the site of interest, the half-life for clearance, and the residence time of the drug in circulation (Patra et al., 2018; Anderson et al., 2019). However, in current scenario, nanotechnology is going through an identity crisis inside the biological system. In all cases, nanostructures are primarily engulfed by tissue-resident macrophages, neutrophils and circulating monocytes (Weissleder et al., 2014; Bilyy et al., 2020). What was first viewed as a disadvantage for nanosystems as medicines quickly evolved into a valuable tool for combating diseases by altering the immune system. Since the immune cells can self-regulate, it is conceivable to overcome the limited effectiveness of nanosystems by modulating immune cells.
Additionally, due to their critical function in all pathogenic and inflammatory circumstances, immune cells play a significant part in the progression of various diseases. Monoclonal antibodies, cancer vaccines, cytokines, and anti-inflammatory small molecules can all be used in immunotherapy. However, uncontrolled off-target activation of immune cells is usually present with these therapies. The use of nanotechnology in conjunction with therapeutic applications can aid in the resolution of these issues. In addition, owing to their small size, nanostructures can assess areas of the body far from their point of entry. According to Kreyling et al. (2013), the translocation of nanoparticles across membranes is also greatly aided by biomolecules’ binding to nanoparticles. Top-down and bottom-up are the two strategies for the synthesis of nanostructures.
This article considers the possibility of how the biological identity of a nanoparticle system could alter dynamically in a biological system through the adsorption and desorption of proteins on the surface of the nanoparticles. To comprehend the mechanism by which nanostructures affect immune cells, we will also discuss how nanomaterial interactions with biological systems could influence immunotherapy. Finally, we will also go through potential barriers to practical implementation and their consequences for the logical design of nanomaterials.
Why do we need the tag of “nano”?
To create the legal jargon for patents and commercialize products using nanotechnology, precise definitions of terms are crucial. For regulatory purposes, it is also required to define nanotechnology and associated words because, at this scale, specific properties of the materials differ from those of their bulk equivalents. The “1–1000 nm rule” is based on the prefix “nano,” which stands for one billionth of a meter and is most readily understood by users from a wide range of disciplines. The shift to nanotechnology can be characterized as a “step crossing the limit” and entering a domain where new laws, such as the quantum size, are in effect. According to the National Nanotechnology Initiative, nanotechnology is the study of phenomena and materials at the nanoscale and the development of technology at the atomic, molecular, or macromolecular levels, in the length scale of 1–100 nm, in order to create and use structures, devices, and systems with novel properties and functions as a result of their small and/or intermediate size. The critical length scale for novel properties and phenomena may, in some specific cases, be smaller than 100 nm or larger than 1 nm (for example, nanoparticle-reinforced polymers due to the local bridges or bonds between the nanoparticles and the polymer) (Schmid et al., 2003). Because of the influence of quantum effects, the surface properties, and the collective qualities of the structures, nanoscale materials exhibit variable (new) properties as opposed to the bulk material’s properties, which are governed by only classical laws. Despite being a subfield of nanotechnology, nanomedicine nonetheless includes a wide range of tactics for managing healthcare, such as nanotherapeutics, nanodiagnostics, and nanomaterials for pharmaceutical uses. The National Institutes of Health. (2006) roadmap programme defines nanomedicine as a highly specialized molecular intervention for drug delivery or diagnosis. Despite this scientific justification, there are still additional elements that affect how terminology connected to nanotechnology is defined. Science, the general public, and regulation can all be distinguished as three distinct purposes (Balogh, 2010).
Behavior of nanos inside the human body: Immune system perspectives
Influence of protein corona
Although much research is ongoing on the pre-clinical or clinical trials for nanomaterials, many challenges still exist. Nanostructures do not reach the target site in the same form when entering the body. Once inside the body, nanostructures will first come across a dense protein network, thus, producing a protein corona layer on the nanostructure’s surface (Caracciolo et al., 2017) (Figure 1). The protein corona will lead to physiological recognition and thereby could be recognized by the immune system and removed by the macrophages. Owing to the importance of protein coronas in the immune system, many efforts are being made to understand the immune system’s response to nanoparticles (Hussain et al., 2012). The protein corona on the surface of nanoparticles depends on their binding affinities and equilibrium constants (Lynch and Dawson, 2008). Also, the association and dissociation rate of the nanostructures with protein and the content of the diverse protein strongly influence the protein corona features (Nel et al., 2009). It has also been reported that protein corona and aggregation are entirely prevented by the cyclic poly(2-ethyl-2-oxazoline) brushes because they produce NP shells that are denser and more compact than their linear equivalents (Schroffenegger et al., 2020). Another study demonstrates that The polymers polyethyleneimine (PEI), polyvinylpyrrolidone (PVP), and poly(2-vinyl pyridine)-b-poly(ethylene oxide) (PEO-b-P2VP) shells strongly repel model protein adsorption (Batista et al., 2020).
Cai et al. (2020) evaluated how the protein corona of nanostructures affects macrophages’ phagocytosis and immune response toward the nanostructures. Protein corona could reportedly change the internalization of gold nanorods through macrophages via cell membrane receptors. The adsorption mode of the protein corona could also influence the secretion of cytokines from macrophages, showing the increased release of interleukin-1β (IL-1β). Similarly, in a most recent study, silica nanoparticles’ surface structure strongly influences the protein corona’s composition and subsequent inflammatory responses. Silica nanoparticles rich with carboxylic acid surface significantly raised the pro-inflammatory cytokines secretions and subsequently lowered the number of lipoproteins. In contrast, amine-rich silica nanoparticles upregulated expressions of anti-inflammatory markers (González-García et al., 2022). Likewise, introducing a hydroxyl shell in the cationic nanoparticles could effectively reduce the adsorption of a protein corona and also affect the activation of the immune response by regulating the complement proteins (Liu et al., 2021). Thus, the activity of nanoparticles could arise from either themselves as a whole structure or from the soluble ions released from them, or due to both. So, we can conclude that the surface chemistry of the nanoparticles influences the immune system, which should also be reviewed.
Influence of size and shape of nanomaterials
Before cellular interaction, a crucial stage is the efficient contact of nanoparticles with the cell membrane. Some types of nanoparticles have demonstrated significant involvement in cellular uptake, cell proliferation (Hou et al., 2013), apoptosis (Wang et al., 2012), and other biological pathways depending on where they are located - on the inner or outer membrane surface or outside of the cell membrane. Phagocytosis, diffusion, and fluid phase endocytosis are the three main methods by which cells absorb nanoparticles (He et al., 2009). Reticuloendothelial systems readily absorb microparticles, but macrophages frequently fail to recognize nanoparticles as foreign substances even though they can enter macrophage cells through membrane gaps (Choi et al., 2009). Smaller particles have more surface area, and larger surfaces make it easier for particles to diffuse into cells (Behzadi et al., 2017). For instance, 30 nm single-walled carbon nanotubes (SWNT) are internalized more readily than 50 nm ones in cells and nuclei (Donkor and Tang, 2014). Clathrin-coated pits control microspheres with a diameter of less than 200 nm; however, when the size increases to 500 nm, the caveolae-mediated mechanism becomes the dominating phenomenon in the internalization of cells (Rejman et al., 2004). In addition, clathrin-targeted nanoparticles with a diameter of 50 nm effectively kill human mesenchymal stem cells without relying on endocytosis (Lu et al., 2007). Gold nanoparticles (AuNPs) of 10 nm and 50 nm were reportedly internalized via a clathrin-dependent mechanism by dendritic cells. However, the results also showed that the uptake of 10 nm-sized particles was higher than 50 nm particles per cell. In both the MCF-7 and MDA-MB-231 tumor cell lines, the cellular absorption of AuNPs was shown to be size dependent, with the 20-nm-sized AuNPs having lower cellular uptake than the 50-nm-sized AuNPs. Smaller GNPs have a reduced surface area to engage with cell membrane receptors, which causes cells to need less energy to engulf NPs. Another study found that due to their enhanced passive transport, single AuNPs between 4 and 17 nm in size were taken up by cells at higher rates.
Similarly, the shape of the nanoparticles, such as spherical, rod-like, discoid, wires, tubes, and nano-needles, also significantly impacted the internalization (Truong et al., 2014). Moreover, a shape-dependent effect was also observed on receptor-mediated endocytosis of nanocarriers (Cho et al., 2010). Compared to spherical nanoparticles, the elongated nanoparticles are more effective at adhering to the cells due to their increased surface area, which facilitates their multivalent interaction with the surface of cells (Agarwal et al., 2013). Hela and caco-2 cells absorbed rod-like nanoparticles with higher aspect ratios far more effectively than smaller particles and nanoparticles with lower aspect ratios, which had minimal effect on cellular absorption (Gratton et al., 2008). In addition, in vitro and in vivo tests with rod-shaped nanoparticles on endothelial cells revealed greater affinities and efficacy (Kolhar et al., 2013). Comparing the cellular uptake of spherical nanoparticles with various aspect ratios revealed that the extent and rate of absorption of the nanoparticles with higher aspect ratios were, respectively, greater and quicker (Augustine et al., 2020). According to recent studies, ellipsoidal nanoparticles are more difficult for cells to absorb than spherical nanoparticles (Desai et al., 2018). In the following sequence, the exterior morphology of AuNPs may directly affect absorption into cells: rods/spheres, cylinders, and cubes (Chithrani et al., 2006; Gratton et al., 2008; Qiu et al., 2010).
Similarly, the size and shape of the nanostructures also greatly influence the biological safety and inflammatory response (Figure 2). Small NPs are more likely to interact with nearby biomolecules and, as a result, cause adverse reactions because of their large surface area. Reports suggested that larger, 15-nm AuNPs exhibit minimal toxicity, whereas smaller, 1.4-nm AuNPs were extremely toxic and mostly caused fast cell death by necrosis within 12 h (Pan et al., 2007). Similarly, 4-nm AgNPs were observed to significantly increase ROS generation and interleukin-8 secretion compared to 20- and 70-nm AgNPs. A size dependent toxicity was also identified for SiO2 and polymer NPs (Ariano et al., 2011; Bhattacharjee et al., 2012). However, as indicated by the production of the pro-inflammatory gene products IL-1, IL-6, and TNFα, macrophage immunological responses to AgNPs in the 3–25 nm size range were not substantially different (Yen et al., 2009). Although 20 nm spherical AuNPs did not significantly harm any organs or cells in mice, they were linked to a considerable reduction in body fat and a suppression of inflammatory effects (Chen et al., 2013). Oh et al. (2010) found that the 50-nm silica-titania hollow nanoparticles had the highest toxicity on macrophages when studied with uniform diameters of 25 nm, 50 nm, 75 nm, 100 nm, and 125 nm.
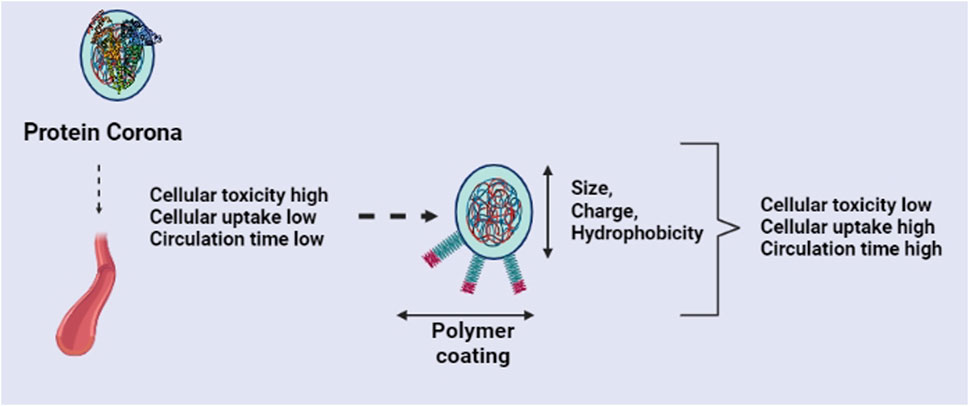
FIGURE 2. Effects of protein corona and strategies to improve the nanomaterials behavior inside the living system.
The shape of different nanostructures may also impact the immune response in biological systems (Mostaghaci et al., 2015; Moyano et al., 2016). Bartneck et al. (2010) revealed that the nanorod uptake by macrophages was higher than that of nanospheres. Aluminum oxyhydroxide nanomaterials in different shapes, including rods, plates, and polyhedra, nanorods showed to activate NLRP3 inflammasome and trigger IL-1β release in THP-1 cells and BMDCs (Sun et al., 2013). Here, nanorods reportedly reduced phagocytosis and elicited cytokine secretion (IL-6 and IFN-γ) (Sun et al., 2013). Similarly, another study compared spherical, rods, and disks-shaped polystyrene nanoparticles for complement activation. All the shapes of nanoparticles induced complement activation. However, rods and disks showed higher effects than spheres (Wibroe et al., 2017). Another study reported effects of AuNPs of different shapes coated with West Nile virus envelope (E) protein on cytokine release (Niikura et al., 2013). They reported that the rod-shaped AuNPs enhanced the secretion of the inflammasome-related cytokines interleukin 1β and IL-18, however, spherical or cubic AuNPs induce the secretion of the pro-inflammatory cytokines (Niikura et al., 2013). More recently, it has been shown that short rod-shaped thiolated poly(methacrylic acid) capsules provoked TNF-α and IL-8 secretion when compared with spherical and long rod-shape capsules (Chen et al., 2016). Another study showed that spherical AuNPs were much more internalized than cylindrical AuNPs in RAW 264.7 macrophages (Li et al., 2016). Compared to spherical or short cylindrical AuNPs, long cylindrical AuNPs provoked a higher release of IL-6 (Li et al., 2016). Sun et al. (2016) studied the role of the plasma membrane and shedding of cells due to graphene oxide (GO) in RBL cells, MDA-MB-231, and NIH-3T3 cells. The results showed peripheral membrane fragment generation. The study with AuNPs showed that smaller particles discourage LPS-induced dendritic cell maturation (Tomić et al., 2014). Similarly, silica-titania nanoparticles with a size range between 50 nm and 125 nm leads to higher ROS generation and release of inflammatory cytokines (IL-1, IL-6, and TNF-α) in J774.1 cells (Oh et al., 2010).
Reactive oxygen species (ROS) is the hallmark of the inflammatory response by macrophages to nanomaterials (Liu et al., 2017). In this regard, Kim et al. (2011) synthesized polypyrrole (PPy) nanoparticles of different size ranges. PPy nanoparticles of nearly 60 nm in size elicited the highest ROS generation and upregulated the expression CD40 and CD80 in macrophages. Introducing a hydrophobic zwitterionic group in the nanoparticle surface will raise the LPS-induced inflammatory outcomes. However, in contrast, hydrophilic groups generate a minimal inflammatory response in vivo (Moyano et al., 2016). Yoshida et al. (2012) showed that particle size was a significant factor in determining the intracellular distribution of amorphous silica and its induction of ROS production, which resulted in DNA damage in human skin HaCaT cells. Additionally, when the size of nanoparticles decreases, bactericidal or hazardous effects become more pronounced (Auffan et al., 2009). According to Li et al. (2010), ROS and oxidative stress were responsible for the wire-shaped nanomaterial’s ability to cause cytotoxicity, DNA oxidative damage, and death in HeLa cells. In cultured fibroblasts, long nanowires result in defective cell division, DNA damage, and a rise in ROS; in contrast, vertical nanowire arrays promote cell motility and proliferation rate (Persson et al., 2013). According to Jiang and others, an S-shaped curve dependency for ROS production per unit surface area occurred as a function of particle size (4–195 nm) for a fixed total surface area (Braydich-Stolle et al., 2009). The photocytotoxicity of nano-TiO2 in human skin keratinocytes was investigated by Yin et al. (2012) using nano-TiO2 of four different sizes (25 nm, 31 nm, 100 nm, and 325 nm) and two distinct crystal forms (anatase and rutile). Smaller-particle nano-TiO2 caused more cell damage than larger-particle versions. Compared to the rutile form, nano-TiO2’s anatase form caused more photocytotoxicity.
Influence of surface properties of nanomaterials
Basically, surface properties such as surface charge, hydrophilicity, and nanostructure modifications like PEGylation, aliphatic chains, peptide moiety, or coating of other entities could minimize the side effects and affect future clinical translation (Yin et al., 2019) (Figure 2). The alteration of nanoparticle surface charge greatly impacts the immune response. Positively charged nanoparticles are known to trigger the immune system’s inflammatory response, whereas neutral or negatively charged nanoparticles cause greatly less inflammation (Yue et al., 2011; Hwang et al., 2015). Likewise, the positive surface charge on the nanostructures may interact with the nucleic acids and anionic proteins, thereby leading to an inflammatory response. Kedmi et al. (2010) modified the surface of the nanomaterials with a positive charge, and they observed that these could activate interferon type I response. Compared with negatively-charged nanostructures, the mRNA levels of interferon-responsive genes were increased. They conclude that the positive charge nanomaterials induce an inflammatory response through TLR4 activation. However, one study reported that instead of the absolute magnitude of a cationic carbon NP’s -potential, the surface charge density might be a more useful descriptor for determining lung toxicity (Weiss et al., 2021). Moreover, the electrostatic interaction between cationic nanoparticles and the anionic cell membrane increased their uptake in macrophages and DCs (Xu et al., 2016; Fytianos et al., 2017). Interestingly, another work showed that the cationic iron oxide nanoparticles promoted penetration into DCs, however, anionic nanoparticles undergo rapid autophagy (Mou et al., 2017).
The strongest long-range noncovalent interaction in biological systems is said to be hydrophobic interaction, which is advantageous for biomolecule adsorption, improving interaction/adhesion with cellular membranes, enhancing the cellular uptake, as well as customizing drug release rates (Nel et al., 2009). Rough mesoporous hollow silica nanoparticles exhibit remarkable hydrophobicity compared to a smooth surface with the same hydrophilic composition (Ahmad Nor et al., 2015). This leads to increased adsorption of various hydrophobic compounds and regulated release of hydrophilic molecules. Another study conducted in vitro and in vivo reported that the treatment of hydrophilic nanogels reduced the immunological reactions brought on by LPS (Li et al., 2018). Comparatively to the cationic and NPs, a negative surface charge resulted in more accumulation in inflamed skin hair follicles (Abdel-Mottaleb et al., 2012). On a therapeutic level, negatively and positively charged particles outperformed noncharged carriers. Another report suggested an increase in IL-8 expression when positively or negatively surface-charged SiO2 NPs were introduced to differentiated Caco-2 cells (Tada-Oikawa et al., 2020). A linear relationship between hydrophobicity and immune system activation has been reported (Moyano et al., 2012). In the case of AuNPs, increasing hydrophobicity causes an immunological response to be more pronounced at lower log P levels. However, at higher levels of hydrophobicity, the dependency is less obvious, and a maximal immunological response is shown.
The surface modification of nanoparticles with the PC-COOH group showed more internalization in macrophages than THP-1 cells, whereas the PS-NH2 surface showed more uptake inside THP-1 cells (Lunov et al., 2011). Further research revealed that macrophages uptake depends on the interactions with the CD63, however, in the case of THP-1 cells, it depends on dynamin-dependent endocytosis. Also, the same study observed the accumulation of PS-NH2-modified nanoparticles in tumor xenografts. The surface modification of nanostructures with CD47 significantly reduced phagocytosis of nanoparticles via binding to signal regulatory protein a (SIRPa) (Liu et al., 2015). Similarly, the surface modification of nanoparticles with TLR agonists, elevated cytokine secretion, expression of activation markers (CD40, CD80, and CD86), and upregulation of immunoregulatory genes (CXCL1, CCL4, and CD14) (Siefert et al., 2016). Ag, TiO2, and ZnO nanoparticles elicit pro-inflammatory responses via NF-κB at extraordinarily low concentrations (Giovanni et al., 2015). It was observed that the titanium sulfonate ligand (TiL4) on the surface of black phosphorus nanosheets decreased inflammation reaction and conferred biocompatibility (Qu et al., 2017).
Many research groups focused on PEGylation, which reduces the protein-corona effect, increasing the circulation time of nanostructures and stability in the biological system. In this context, computational and experimental investigation of MoS2 nanoflakes showed that PEGylation inhibits the penetration into the macrophage membrane and enhances cytokine secretion and ROS generation (Gu et al., 2019). In a similar study, PEGylated GO nanosheets induced cytokine secretion irrespective of their internalization. Thus, surface modification did not always inhibit immunological toxicity to nanostructures (Luo et al., 2017).
Wang et al. (2017) synthesized MnO2-CpG-silver nanoclusters conjugated with doxorubicin and observed antitumor efficiency due to immune modulation. Toll-like 9 receptors recognized CpG and therefore, has been used for targeted delivery. Similarly, silica nanosheets alone and coated with carbon were evaluated for biocompatibility in peripheral blood mononuclear cells (PBMCs) (Al Soubaihi et al., 2018). The results revealed that carbon coating enhanced biocompatibility and less hemoglobin release, cytotoxicity, and no direct acute immune response.
Addressing challenges for sustainable nanomedicines
Nanotechnology is one of the most promising innovations that can offer breakthroughs in almost every field, like healthcare, energy, environment, engineering, etc. However, there are several technical challenges that we need to address. The road from lab studies to clinical translation requires understanding how we can control the material to dictate its role in the body and how the body will react to this material. For this, we need to study nanomaterials at the molecular level for their effectivity, then at the size and structure level for product designing, and finally at the bulk processing level for manufacturing. Many molecules come daily for their pharmacological activity; among them, only a small fraction reaches the clinic for patients. In this regard, nanoformulations play a big picture by improving their properties.
Owing to their smaller size, nanostructures-based drug delivery systems can be routed by both invasive and non-invasive modes of administration, however, the bioavailability and tissue distribution would be different for different modes, which influences the therapeutic outcomes. So far, the focus is mainly dedicated to the invasive administration of the therapeutics, however, still the major concerns exist, such as limited vascular permeability and phagocytic uptake (Chrastina et al., 2011). The choice of administration via the oral route lies in its compliance due to the availability of a larger surface area for absorption. Apart from the considerations in the routes of administration, the stability of the nanosystems is also a key factor for the efficient delivery of therapeutic agents.
Biodegradability and biocompatibility are the two crucial considerations for nanomaterial chemistry for practical applications. The chemical structure of the nanomaterial predominantly influences both factors. The crystallinity and hydrophobicity also affect the properties. The aspect ratio of the nanostructures influences the in vitro and in vivo stability. Also, a very high surface area can also make them thermodynamically unstable, resulting in agglomeration. Agglomeration could lead to settling, crystal growth, and dose differences (Wu et al., 2011).
With regard to the uptake of nanostructures by the biological systems, studies should consider aggregation and agglomeration, which might co-occur in the media (Limbach et al., 2005). The concentration and the size of the nanoparticles also influence the rate of agglomeration and could be avoided by electrostatic or steric stabilizers (Tam et al., 2010). Another critical question in the use of nanoparticles is their safety, however, if the benefits offered by the nanosystem outweigh the associated risk factors, they should be accepted. Apart from this, efforts are also needed for bio persistence and environmental impacts while using nanostructures. Also, real-time imaging is needed to track the fate and location of injected nanoparticles in vivo (Yin et al., 2023).
Recently, studies focused on modifying the flocs to increase the stability of the nanosuspensions (Engstrom et al., 2009). Surface curvature or geometry of the nanostructures is also an important factor that controls phagocytosis by macrophages. The curvature of the nanoparticles at the initial contact with the cells influences macrophage uptake (Guo et al., 2023). The question is how much of this research will translate the nanoparticles to the clinic. For internalization, nanostructures have to cross cellular barriers to reach the target site, therefore, research is also needed in this direction in different cell lines with nanotherapeutics destined to reach their target site.
Equal efforts are also needed to bring nano-based therapeutics, sensors, and other products to the lab scale. The industrialization of nanotechnologies at the industrial level is currently lacking for successful clinical usage. Therefore, successful industrial scaling for large-scale production of the nanoparticles is needed to reach the market for these systems. Also, it is challenging to develop animal models to investigate the environmental hazards of nanomaterials where a proper consideration of the dose, time span, and route of administration is crucial.
The crucial challenges encountered by nanotechnology are not just confined to science, but instead, there are also concerns regarding communication, regulations, ethics, patenting, and marketing strategy (Balogh, 2011). There is an urgent need to revise the patenting laws to restrict the claims to research data only rather than including a whole range of applications. This could save time and resources for practical applications of existing inventions already. At this junction, we should concentrate on the underexplored essential first- and second-generation nano-based systems before we get to the more complicated third and fourth-generation systems. Therefore, we promote more applied research that is problem-driven and user-informed, as well as closer integration of problem-driven and curiosity-motivated basic research.
Conclusion
Regarding how nanomaterials interact with biotic (cells, tissues, and organisms) and abiotic (pH, ionic strength, size, shape, etc.) environments, it is evident that fate at the nano-bio-eco interface is far more intricate than the predictions. It is necessary to have a thorough understanding of the biological and synthetic “identity” of manufactured nanomaterials. Without considering the biological effects, synthetic nanostructures dominated the early stages of nanomedicine. However, as knowledge of the biocompatibility, biodegradability, and toxicity of nanomaterials has grown, safer delivery methods are now becoming more common. In this article, we explore how a nanoparticle’s (NP) “biological identity"—defined by the protein corona and other biomolecules on the NP’s surface—affects its cellular absorption and dynamic changes in a living system, which is hypothesized to correlate with toxicity, in particular to inflammation. We have not to look at problems for solutions from pure nanotechnology perspectives but also need to think for sustainable solutions for overall societal benefits. The “technology push” approach can be avoided if bodies of knowledge about market needs, commercial imperatives, stakeholders’ perspectives on the most urgent risks to human health and the environment, and the social acceptability of potential technological solutions are all brought into the lab early and translated in ways that shape research designs.
Data availability statement
The original contributions presented in the study are included in the article/supplementary material, further inquiries can be directed to the corresponding authors.
Author contributions
SS: Conceptualization, Writing–original draft, Writing–review and editing. LS: Conceptualization, Writing–review and editing.
Funding
The author(s) declare that no financial support was received for the research, authorship, and/or publication of this article.
Acknowledgments
Authors acknowledge their respective department and institutions for providing support and facilities.
Conflict of interest
The authors declare that the research was conducted in the absence of any commercial or financial relationships that could be construed as a potential conflict of interest.
The author(s) declared that they were an editorial board member of Frontiers, at the time of submission. This had no impact on the peer review process and the final decision.
Publisher’s note
All claims expressed in this article are solely those of the authors and do not necessarily represent those of their affiliated organizations, or those of the publisher, the editors and the reviewers. Any product that may be evaluated in this article, or claim that may be made by its manufacturer, is not guaranteed or endorsed by the publisher.
References
Abdel-Mottaleb, M. M., Moulari, B., Beduneau, A., Pellequer, Y., and Lamprecht, A. (2012). Surface-charge-dependent nanoparticles accumulation in inflamed skin. J. Pharm. Sci. 101 (11), 4231–4239. doi:10.1002/jps.23282
Agarwal, R., Singh, V., Jurney, P., Shi, L., Sreenivasan, S., and Roy, K. (2013). Mammalian cells preferentially internalize hydrogel nanodiscs over nanorods and use shape-specific uptake mechanisms. Proc. Natl. Acad. Sci. 110, 17247–17252. doi:10.1073/pnas.1305000110
Ahmad Nor, Y., Niu, Y., Karmakar, S., Zhou, L., Xu, C., Zhang, J., et al. (2015). Shaping nanoparticles with hydrophilic compositions and hydrophobic properties as nanocarriers for antibiotic delivery. ACS Cent. Sci. 1 (6), 328–334. doi:10.1021/acscentsci.5b00199
Al Soubaihi, R. M., Furesi, G., Saoud, K. M., Al-Muhtaseb, S. A., Khatat, A. E., Delogu, L. G., et al. (2018). Silica and carbon decorated silica nanosheet impact on primary human immune cells. Colloids Surfaces B Biointerfaces 172, 779–789. doi:10.1016/j.colsurfb.2018.09.022
Anderson, S. D., Gwenin, V. V., and Gwenin, C. D. (2019). Magnetic functionalized nanoparticles for biomedical, drug delivery and imaging applications. Nanoscale Res. Lett. 14, 188. doi:10.1186/s11671-019-3019-6
Ariano, P., Zamburlin, P., Gilardino, A., Mortera, R., Onida, B., Tomatis, M., et al. (2011). Interaction of spherical silica nanoparticles with neuronal cells: size-dependent toxicity and perturbation of calcium homeostasis. Small 7, 766–774. doi:10.1002/smll.201002287
Auffan, M., Rose, J., Wiesner, M. R., and Bottero, J. Y. (2009). Chemical stability of metallic nanoparticles: a parameter controlling their potential cellular toxicity in vitro. Environ. Pollut. 157 (4), 1127–1133. doi:10.1016/j.envpol.2008.10.002
Augustine, R., Hasan, A., Primavera, R., Wilson, R. J., Thakor, A. S., and Kevadiya, B. D. (2020). Cellular uptake and retention of nanoparticles: insights on particle properties and interaction with cellular components. Mat. Today Comm. 25, 101692. doi:10.1016/j.mtcomm.2020.101692
Balogh, L. P. (2010). Why do we have so many definitions for nanoscience and nanotechnology? Nanomedicine 6 (3), 397–398. doi:10.1016/j.nano.2010.04.001
Bartneck, M., Keul, H. A., Singh, S., Czaja, K., Bornemann, J., Bockstaller, M., et al. (2010). Rapid uptake of gold nanorods by primary human blood phagocytes and immunomodulatory effects of surface chemistry. ACS Nano 4, 3073–3086. doi:10.1021/nn100262h
Batista, C. C. S., Albuquerque, L. J. C., Jäger, A., Stepánek, P., and Giacomelli, F. C. (2020). Probing protein adsorption onto polymer-stabilized silver nanocolloids towards a better understanding on the evolution and consequences of biomolecular coronas. Mat. Sci. Eng. C Mat. Biol. Appl. 111, 110850. doi:10.1016/j.msec.2020.110850
Behzadi, S., Serpooshan, V., Tao, W., Hamaly, M. A., Alkawareek, M. Y., Dreaden, E. C., et al. (2017). Cellular uptake of nanoparticles: journey inside the cell. Chem. Soc. Rev. 46 (14), 4218–4244. doi:10.1039/c6cs00636a
Bhattacharjee, S., Ershov, D., Fytianos, K., van der Gucht, J., Alink, G. M., Rietjens, I. M. C. M., et al. (2012). Cytotoxicity and cellular uptake of triblock copolymer nanoparticles with different size and surface characteristics. Part. Fibre. Toxicol. 9, 11. doi:10.1186/1743-8977-9-11
Bilyy, R., Bila, G., Vishchur, O., Vovk, V., and Herrmann, M. (2020). Neutrophils as main players of immune response towards nondegradable nanoparticles. Nanomater. (Basel) 10 (7), 1273. doi:10.3390/nano10071273
Braydich-Stolle, L. K., Schaeublin, N. M., Murdock, R. C., Jiang, J., Biswas, P., Schlager, J. J., et al. (2009). Crystal structure mediates mode of cell death in TiO2 nanotoxicity. J. Nanopart. Res. 11, 1361–1374. doi:10.1007/s11051-008-9523-8
Cai, R., Ren, J., Ji, Y., Wang, Y., Liu, Y., Chen, Z., et al. (2020). Corona of thorns: the surface chemistry-mediated protein corona perturbs the recognition and immune response of macrophages. ACS Appl. Mat. Interfaces. 12, 1997–2008. doi:10.1021/acsami.9b15910
Caracciolo, G., Farokhzad, O. C., and Mahmoudi, M. (2017). Biological identity of nanoparticles in vivo: clinical implications of the protein corona. Trends Biotechnol. 35, 257–264. doi:10.1016/j.tibtech.2016.08.011
Chen, H., Dorrigan, A., Saad, S., Hare, D. J., Cortie, M. B., and Valenzuela, S. M. (2013). In vivo study of spherical gold nanoparticles: inflammatory effects and distribution in mice. PLoS ONE 8 (2), e58208. doi:10.1371/journal.pone.0058208
Chen, X., Yan, Y., Müllner, M., Ping, Y., Cui, J., Kempe, K., et al. (2016). Shape-dependent activation of cytokine secretion by polymer capsules in human monocyte-derived macrophages. Biomacromol 17, 1205–1212. doi:10.1021/acs.biomac.6b00027
Chithrani, B. D., Ghazani, A. A., and Chan, W. C. (2006). Determining the size and shape dependence of gold nanoparticle uptake into mammalian cells. Nano Lett. 6 (4), 662–668. doi:10.1021/nl052396o
Cho, E. C., Au, L., Zhang, Q., and Xia, Y. (2010). The Effects of size, shape, and surface functional group of gold nanostructures on their adsorption and internalization by cells. Small 6, 517–522. doi:10.1002/smll.200901622
Choi, J., Zhang, Q., Reipa, V., Wang, N. S., Stratmeyer, M. E., Hitchins, V. M., et al. (2009). Comparison of cytotoxic and inflammatory responses of photoluminescent silicon nanoparticles with silicon micron-sized particles in RAW 264.7 macrophages. J. Appl. Toxicol. 29, 52–60. doi:10.1002/jat.1382
Chrastina, A., Massey, K. A., and Schnitzer, J. E. (2011). Overcoming in vivo barriers to targeted nanodelivery WIREs Nanomed. Nanobiotechnol 3, 421–437. doi:10.1002/wnan.143
Desai, P., Venkataramanan, A., Schneider, R., Jaiswal, M. K., Carrow, J. K., Purwada, A., et al. (2018). Self-assembled, ellipsoidal polymeric nanoparticles for intracellular delivery of therapeutics. J. Biomed. Mat. Res. A 106 (7), 2048–2058. doi:10.1002/jbm.a.36400
Donkor, D. A., and Tang, X. S. (2014). Tube length and cell type-dependent cellular responses to ultra-short single-walled carbon nanotube. Biomaterials 35, 3121–3131. doi:10.1016/j.biomaterials.2013.12.075
Engstrom, J. D., Tam, J. M., Miller, M. A., Williams, R. O., and Johnston, K. P. (2009). Templated open flocs of nanorods for enhanced pulmonary delivery with pressurized metered dose inhalers. Pharm. Res. 26, 101–117. doi:10.1007/s11095-008-9707-z
Fytianos, K., Chortarea, S., Rodriguez-Lorenzo, L., Blank, F., Von Garnier, C., Petri-Fink, A., et al. (2017). Aerosol delivery of functionalized gold nanoparticles target and activate dendritic cells in a 3D lung cellular model. ACS Nano 11, 375–383. doi:10.1021/acsnano.6b06061
Giovanni, M., Yue, J., Zhang, L., Xie, J., Ong, C. N., and Leong, D. T. (2015). Pro-inflammatory responses of RAW264.7 macrophages when treated with ultralow concentrations of silver, titanium dioxide and zinc oxide nanoparticles. J. Hazard Mat. 297, 146–152. doi:10.1016/j.jhazmat.2015.04.081
González-García, L. E., MacGregor, M. N., Visalakshan, R. M., Lazarian, A., Cavallaro, A. A., Morsbach, S., et al. (2022). Nanoparticles surface chemistry influence on protein corona composition and inflammatory responses. Nanomater. (Basel). 12, 682. doi:10.3390/nano12040682
Gratton, S. E., Ropp, P. A., Pohlhaus, P. D., Luft, J. C., Madden, V. J., Napier, M. E., et al. (2008). The effect of particle design on cellular internalization pathways. Proc. Natl. Acad. Sci. 105, 11613–11618. doi:10.1073/pnas.0801763105
Gu, Z., Chen, S. H., Ding, Z., Song, W., Wei, W., Liu, S., et al. (2019). The molecular mechanism of robust macrophage immune responses induced by PEGylated molybdenum disulfide. Nanoscale 11, 22293–22304. doi:10.1039/c9nr04358f
Guo, Y., Ao, Y., Ye, C., Xia, R., Mi, J., Shan, Z., et al. (2023). Nanotopographic micro-nano forces finely tune the conformation of macrophage mechanosensitive membrane protein integrin β2 to manipulate inflammatory responses. Nano Res. 16, 9715–9729. doi:10.1007/s12274-023-5550-0
He, Q., Zhang, Z., Gao, Y., Shi, J., and Li, Y. (2009). Intracellular localization and cytotoxicity of spherical mesoporous silica nano-and microparticles. Small 5, 2722–2729. doi:10.1002/smll.200900923
Hou, Y., Cai, K., Li, J., Chen, X., Lai, M., Hu, Y., et al. (2013). Effects of titanium nanoparticles on adhesion, migration, proliferation, and differentiation of mesenchymal stem cells. Int. J. Nanomed. 8, 3619–3630. doi:10.2147/ijn.s38992
Hussain, S., Vanoirbeek, J. A., and Hoet, P. H. (2012). Interactions of nanomaterials with the immune system. Wiley Interdiscip. Rev. Nanomed. Nanobiotechnol. 4, 169–183. doi:10.1002/wnan.166
Hwang, T. L., Aljuffali, I. A., Lin, C. F., Chang, Y. T., and Fang, J. Y. (2015). Cationic additives in nanosystems activate cytotoxicity and inflammatory response of human neutrophils: lipid nanoparticles versus polymeric nanoparticles. Int. J. Nanomed. 10, 371–385. doi:10.2147/ijn.s73017
Kedmi, R., Ben-Arie, N., and Peer, D. (2010). The systemic toxicity of positively charged lipid nanoparticles and the role of Toll-like receptor 4 in immune activation. Biomaterials 31, 6867–6875. doi:10.1016/j.biomaterials.2010.05.027
Kim, S., Oh, W. K., Jeong, Y. S., Hong, J. Y., Cho, B. R., Hahn, J. S., et al. (2011). Cytotoxicity of, and innate immune response to, size-controlled polypyrrole nanoparticles in mammalian cells. Biomaterials 32, 2342–2350. doi:10.1016/j.biomaterials.2010.11.080
Kolhar, P., Anselmo, A. C., Gupta, V., Pant, K., Prabhakarpandian, B., Ruoslahti, E., et al. (2013). Using shape effects to target antibody-coated nanoparticles to lung and brain endothelium. Proc. Natl. Acad. Sci. 110, 10753–10758. doi:10.1073/pnas.1308345110
Kreyling, W. G., Semmler-Behnke, M., Takenaka, S., and Möller, W. (2013). Differences in the biokinetics of inhaled nano-versus micrometer-sized particles. Acc. Chem. Res. 46, 714–722. doi:10.1021/ar300043r
Li, B. L., Wang, J., Gao, Z. F., Shi, H., Zou, H. L., Ariga, K., et al. (2019). Ratiometric immunoassays built from synergistic photonic absorption of size diverse semiconducting MoS2 nanostructures. Mat. Horiz. 6, 563–570. doi:10.1039/c8mh01232f
Li, B., Xie, J., Yuan, Z., Jain, P., Lin, X., Wu, K., et al. (2018). Mitigation of inflammatory immune responses with hydrophilic nanoparticles. Angew. Chem. Int. Ed. Engl. 57 (17), 4617–4621. doi:10.1002/ange.201710068
Li, Y., Tian, X., Lu, Z., Yang, C., Yang, G., Zhou, X., et al. (2010). Mechanism for alpha-MnO2 nanowire-induced cytotoxicity in Hela cells. J. Nanosci. Nanotechnol. 10 (1), 397–404. doi:10.1166/jnn.2010.1719
Li, Z., Sun, L., Zhang, Y., Dove, A. P., O'Reilly, R. K., and Chen, G. (2016). Shape effect of glyco-nanoparticles on macrophage cellular uptake and immune response. ACS Macro. Lett. 5 (9), 1059–1064. doi:10.1021/acsmacrolett.6b00419
Limbach, L. K., Li, Y., Grass, R. N., Brunner, T. J., Hintermann, M. A., Muller, M., et al. (2005). Oxide nanoparticle uptake in human lung fibroblasts: effects of particle size, agglomeration, and diffusion at low concentrations. Environ. Sci. Technol. 39, 9370–9376. doi:10.1021/es051043o
Liu, S., Pan, X., and Liu, H. (2020). Two-dimensional nanomaterials for photothermal therapy. Angew. Chem. Int. Ed. 132, 5943–5953. doi:10.1002/ange.201911477
Liu, W., Hu, T., Zhou, L., Wu, D., Huang, X., Ren, X., et al. (2017). Nrf2 protects against oxidative stress induced by SiO2 nanoparticles. Nanomedicine (Lond). 12, 2303–2318. doi:10.2217/nnm-2017-0046
Liu, X., Liang, H., Yan, Y., Wu, J., Bottini, M., Liu, L., et al. (2021). The protein corona modulates the inflammation inhibition by cationic nanoparticles via cell-free DNA scavenging. Bioact. Mat. 13, 249–259. doi:10.1016/j.bioactmat.2021.10.044
Liu, X., Pu, Y., Cron, K., Deng, L., Kline, J., Frazier, W. A., et al. (2015). CD47 blockade triggers t cell-mediated destruction of immunogenic tumors. Nat. Med. 21, 1209–1215. doi:10.1038/nm.3931
Lu, C. W., Hung, Y., Hsiao, J. K., Yao, M., Chung, T. H., Lin, Y. S., et al. (2007). Bifunctional magnetic silica nanoparticles for highly efficient human stem cell labeling. Nano. Lett. 7, 149–154. doi:10.1021/nl0624263
Lunov, O., Syrovets, T., Loos, C., Beil, J., Delacher, M., Tron, K., et al. (2011). Differential uptake of functionalized polystyrene nanoparticles by human macrophages and a monocytic cell line. ACS Nano 5, 1657–1669. doi:10.1021/nn2000756
Luo, N., Weber, J. K., Wang, S., Luan, B., Yue, H., Xi, X., et al. (2017). PEGylated graphene oxide elicits strong immunological responses despite surface passivation. Nat. Commun. 8, 14537. doi:10.1038/ncomms14537
Lynch, I., and Dawson, K. A. (2008). Protein-nanoparticle interactions. Nano Today 3, 40–47. doi:10.1016/s1748-0132(08)70014-8
Mostaghaci, B., Susewind, J., Kickelbick, G., Lehr, C. M., and Loretz, B. (2015). Transfection system of amino-functionalized calcium phosphate nanoparticles: in vitro efficacy, biodegradability and immunogenicity study. ACS Appl. Mat. Interfaces 7, 5124–5133. doi:10.1021/am507193a
Mou, Y., Xing, Y., Ren, H., Cui, Z., Zhang, Y., Yu, G., et al. (2017). The effect of superparamagnetic iron oxide nanoparticle surface charge on antigen cross-presentation. Nanoscale Res. Lett. 12, 52. doi:10.1186/s11671-017-1828-z
Moyano, D. F., Goldsmith, M., Solfiell, D. J., Landesman-Milo, D., Miranda, O. R., Peer, D., et al. (2012). Nanoparticle hydrophobicity dictates immune response. J. Am. Chem. Soc. 134 (9), 3965–3967. doi:10.1021/ja2108905
Moyano, D. F., Liu, Y., Peer, D., and Rotello, V. M. (2016). Modulation of immune response using engineered nanoparticle surfaces. Small 12, 76–82. doi:10.1002/smll.201502273
National Institutes of Health (NIH) (2006). NIH roadmap for medical research: nanomedicine. Avaliable At: https://commonfund.nih.gov/nanomedicine/.
Nel, A. E., Mädler, L., Velegol, D., Xia, T., Hoek, E. M. V., Somasundaran, P., et al. (2009). Understanding biophysicochemical interactions at the nano–bio interface. Nat. Mat. 8, 543–557. doi:10.1038/nmat2442
Niikura, K., Matsunaga, T., Suzuki, T., Kobayashi, S., Yamaguchi, H., Orba, Y., et al. (2013). Gold nanoparticles as a vaccine platform: influence of size and shape on immunological responses in vitro and in vivo. ACS Nano 7, 3926–3938. doi:10.1021/nn3057005
Oh, W. K., Kim, S., Choi, M., Kim, C., Jeong, Y. S., Cho, B. R., et al. (2010). Cellular uptake, cytotoxicity, and innate immune response of silica-titania hollow nanoparticles based on size and surface functionality. ACS Nano 4 (9), 5301–5313. doi:10.1021/nn100561e
Pan, Y., Neuss, S., Leifert, A., Fischler, M., Wen, F., Simon, U., et al. (2007). Size-dependent cytotoxicity of gold nanoparticles. Small 3, 1941–1949. doi:10.1002/smll.200700378
Patra, J. K., Das, G., Fraceto, L. F., Campos, E. V. R., Rodriguez- Torres, M. D. P., Acosta-Torres, L. S., et al. (2018). Nano based drug delivery systems: recent developments and future prospects. J. Nanobiotechnol. 16, 71. doi:10.1186/s12951-018-0392-8
Persson, H., Købler, C., Mølhave, K., Samuelson, L., Tegenfeldt, J. O., Oredsson, S., et al. (2013). Fibroblasts cultured on nanowires exhibit low motility, impaired cell division, and DNA damage. Small 9 (23), 4006–4016. doi:10.1002/smll.201300644
Qiu, Y., Liu, Y., Wang, L., Xu, L., Bai, R., Ji, Y., et al. (2010). Surface chemistry and aspect ratio mediated cellular uptake of Au nanorods. Biomat 31 (30), 7606–7619. doi:10.1016/j.biomaterials.2010.06.051
Qu, G., Liu, W., Zhao, Y., Gao, J., Xia, T., Shi, J., et al. (2017). Improved biocompatibility of black phosphorus nanosheets by chemical modification. Angew. Chem. Int. Ed. 56, 14680–14685. doi:10.1002/ange.201706228
Rejman, J., Oberle, V., Zuhorn, I., and Hoekstra, D. (2004). Size-dependent internalization of particles via the pathways of clathrin-and caveolae-mediated endocytosis. Biochem. J. 377, 159–169. doi:10.1042/bj20031253
Schmid, G., Decker, M., Ernst, H., Fuchs, H., Grunwald, W., Grunwald, A., et al. (2003). “Small Dimensions and Material Properties. A Definition of Nanotechnology. Graue Reihe Edited by the Europäische Akademie zur Erforschung von Folgen wissenschaftlich-technischer Entwicklungen: bad Neuenahr-Ahrweiler,” in Boeing N: Nano? Die Technik des 21. Jahrhunderts. Berlin: Rohwolt; 2004. Jopp K: Nanotechnologie – aufbruch ins Reich der Zwerge (Wiesbaden: Gabler).
Schroffenegger, M., Leitner, N. S., Morgese, G., Ramakrishna, S. N., Willinger, M., Benetti, E. M., et al. (2020). Polymer topology determines the formation of protein corona on core-shell nanoparticles. ACS Nano 14 (10), 12708–12718. doi:10.1021/acsnano.0c02358
Siefert, A. L., Caplan, M. J., and Fahmy, T. M. (2016). Artificial bacterial biomimetic nanoparticles synergize pathogen-associated molecular patterns for vaccine efficacy. Biomaterials 97, 85–96. doi:10.1016/j.biomaterials.2016.03.039
Sun, B., Ji, Z., Liao, Y. P., Wang, M., Wang, X., Dong, J., et al. (2013). Engineering an effective immune adjuvant by designed control of shape and crystallinity of aluminum oxyhydroxide nanoparticles. ACS Nano 7, 10834–10849. doi:10.1021/nn404211j
Sun, C., Wakefield, D. L., Han, Y., Muller, D. A., Holowka, D. A., Baird, B. A., et al. (2016). Graphene oxide nanosheets stimulate ruffling and shedding of mammalian cell plasma membranes. Chem 1, 273–286. doi:10.1016/j.chempr.2016.06.019
Tada-Oikawa, S., Eguchi, M., Yasuda, M., Izuoka, K., Ikegami, A., Vranic, S., et al. (2020). Functionalized surface-charged SiO2 nanoparticles induce pro-inflammatory responses, but are not lethal to Caco-2 cells. Chem. Res. Toxicol. 33 (5), 1226–1236. doi:10.1021/acs.chemrestox.9b00478
Tam, J. M., Engstrom, J. D., Ferrer, D., and Johnston, K. P. (2010). Templated open flocs of anisotropic particles for pulmonary delivery with pressurized metered dose inhalers. J. Pharm. Sci. 99, 3150–3165. doi:10.1002/jps.22091
Tomić, S., Dokić, J., Vasilijić, S., Ogrinc, N., Rudolf, R., Pelicon, P., et al. (2014). Size-dependent effects of gold nanoparticles uptake on maturation and antitumor functions of human dendritic cells in vitro. PLoS One 9, 965844–e96613. doi:10.1371/journal.pone.0096584
Truong, N. P., Whittaker, M. R., Mak, C. W., and Davis, T. P. (2014). The importance of nanoparticle shape in cancer drug delivery. Expert. Opin. Drug. del. 2, 129–142. doi:10.1517/17425247.2014.950564
Vieira Rocha, C., Costa da Silva, M., Banobre-Lopez, M., and Gallo, J. (2020). Para)magnetic hybrid nanocomposites for dual MRI detection and treatment of solid tumours. Chem. Commun. 56, 8695–8698. doi:10.1039/d0cc03020a
Wang, L., Zhang, H., Chen, B., Xia, G., Wang, S., Cheng, J., et al. (2012). Effect of magnetic nanoparticles on apoptosis and cell cycle induced by wogonin in Raji cells. Int. J. Nanomed. 7, 789–798. doi:10.2147/ijn.s28089
Wang, Z., Zhang, Y., Liu, Z., Dong, K., Liu, C., Ran, X., et al. (2017). A bifunctional nanomodulator for boosting CpG-mediated cancer immunotherapy. Nanoscale 9, 14236–14247. doi:10.1039/c7nr04396a
Weiss, M., Fan, J., Claudel, M., Sonntag, T., Didier, P., Ronzani, C., et al. (2021). Density of surface charge is a more predictive factor of the toxicity of cationic carbon nanoparticles than zeta potential. J. Nanobiotechnol 19, 5. doi:10.1186/s12951-020-00747-7
Weissleder, R., Nahrendorf, M., and Pittet, M. J. (2014). Imaging macrophages with nanoparticles. Nat. Mat. 13, 125–138. doi:10.1038/nmat3780
Wibroe, P. P., Anselmo, A. C., Nilsson, P. H., Sarode, A., Gupta, V., Urbanics, R., et al. (2017). Bypassing adverse injection reactions to nanoparticles through shape modification and attachment to erythrocytes. Nat. Nanotechnol. 12, 589–594. doi:10.1038/nnano.2017.47
Wu, L., Zhang, J., and Watanabe, W. (2011). Physical and chemical stability of drug nanoparticles. Adv. Drug Deliv. Rev. 63, 456–469. doi:10.1016/j.addr.2011.02.001
Xu, Y., Sherwood, J. A., Lackey, K. H., Qin, Y., and Bao, Y. (2016). The responses of immune cells to iron oxide nanoparticles. J. Appl. Toxicol. 36, 543–553. doi:10.1002/jat.3282
Yen, H. J., Hsu, S. H., and Tsai, C. L. (2009). Cytotoxicity and immunological response of gold and silver nanoparticles of different sizes. Small 5, 1553–1561. doi:10.1002/smll.200900126
Yin, B., Chan, C. K. W., Liu, S., Hong, H., Wong, S. H. D., Lee, L. K. C., et al. (2019). Intrapulmonary cellular-level distribution of inhaled nanoparticles with defined functional groups and its correlations with protein corona and inflammatory response. ACS Nano 13 (12), 14048–14069. doi:10.1021/acsnano.9b06424
Yin, B., Ho, W. K. H., Xia, X., Chan, C. K. W., Zhang, Q., Ng, Y. M., et al. (2023). A multilayered mesoporous gold nanoarchitecture for ultraeffective near-infrared light-controlled chemo/photothermal therapy for cancer guided by SERS imaging. Small 19, e2206762. doi:10.1002/smll.202206762
Yin, J. J., Liu, J., Ehrenshaft, M., Roberts, J. E., Fu, P. P., Mason, R. P., et al. (2012). Phototoxicity of nano titanium dioxides in HaCaT keratinocytes--generation of reactive oxygen species and cell damage. Toxicol. Appl. Pharmacol. 263 (1), 81–88. doi:10.1016/j.taap.2012.06.001
Yoshida, T., Yoshikawa, T., Nabeshi, H., and Tsutsumi, Y. (2012). Relation analysis between intracellular distribution of nanomateriarls, ROS generation and DNA damage. Yakugaku Zasshi 132 (3), 295–300. doi:10.1248/yakushi.132.295
Keywords: nanotechnology, protein corona, inflammation, sustainability, cellular uptake
Citation: Saha S and Saso L (2023) Identity crisis of nanostructures inside the human body: a perspective on inflammation. Front. Nanotechnol. 5:1256952. doi: 10.3389/fnano.2023.1256952
Received: 11 July 2023; Accepted: 09 August 2023;
Published: 22 August 2023.
Edited by:
Małgorzata Kujawska, Poznan University of Medical Sciences, PolandReviewed by:
Siu Hong Dexter Wong, Hong Kong Polytechnic University, Hong Kong SAR, ChinaCopyright © 2023 Saha and Saso. This is an open-access article distributed under the terms of the Creative Commons Attribution License (CC BY). The use, distribution or reproduction in other forums is permitted, provided the original author(s) and the copyright owner(s) are credited and that the original publication in this journal is cited, in accordance with accepted academic practice. No use, distribution or reproduction is permitted which does not comply with these terms.
*Correspondence: Sarmistha Saha, c2FybWlzdGhhX3BoYXJtYWNvbEB5YWhvby5jb20=; Luciano Saso, bHVjaWFuby5zYXNvQHVuaXJvbWExLml0