- 1Department of Physics, University of Oslo, Oslo, Norway
- 2Department of Biosciences, University of Oslo, Oslo, Norway
Metal nanoparticles have attracted considerable attention due to their astounding potential for a wide range of commercial applications. From targeted drug delivery and antimicrobial agents to electronics, metal nanoparticles seem to have immeasurable prospects in all areas of science. However, modern industrial production frequently involves complex procedures, large amounts of energy, utilizes strong chemical solvents, or produces hazardous waste. Biological synthesis has been proposed as an alternative for simpler, inexpensive, and more eco-friendly metal nanoparticle production. Microorganisms possess multiple mechanisms to transport, regulate and bind metal ions that may result in the biosynthesis of nanoparticles. They can synthesize even complex bimetallic nanoparticles, which are difficult to produce with normal chemical and physical processes. A better understanding of bacteria-metal interactions might thus pave the way for a wide array of industrial applications. This review will summarize the current methods for metal nanoparticle synthesis, with a focus on the microbial (bio) synthesis of nanoparticles. We will describe the general mechanisms of bacteria-metal ion interactions, including cellular uptake and the subsequent reduction into nanoparticles. Protocols for the production of metal-based nanoparticles of relevant elements with different bacterial strains are compiled and the current challenges in bacterial synthesis of metal nanoparticles in the industry are discussed.
1 Introduction
Nanoparticles (NPs) are defined as nano-structures with dimensions of 1–100 nm that can be comprised of a variety of materials such as carbon, metal, or organic substances. At the limits of this dimensions in the range of 1–10 nm, some literature may also referred to them as nanoclusters which are typically composed of up to 100 atoms and possess relevant physicochemical properties (Jimenez-Sandoval et al., 2023). Metal-based nanoparticles (MNPs) contain at least one metallic element and can display diverse shapes. Most of them have different properties compared to bulk metals due to their large surface-to-volume ratio, unique electromagnetic behavior, and high catalytic activity (Gao et al., 2021). In this review, a variety of MNPs is described, which not only comprise pure metals nanoparticles (e.g., Au, Ag, Pd, Pt, Fe), but also minerals or metal oxides NPs (e.g., Fe2O3, Co3O4, TiO2), metal sulfides (e.g., Fe3S4, CdS), doped metal/metal compounds, and metal-organic complexes (Yaqoob et al., 2020).
The unique behavior of nanoparticles and other nanostructured materials is strictly size-dependent and can provide improved functional performance in a variety of technical applications. Examples of industrial applications of MNPs include their use in chemical catalysis, cosmetics, detergents, water remediation, and even medical applications. The particular electromagnetic properties of Fe-based nanoparticles have secured their place as one of the most frequently used contrast agents in medical imaging (Javed et al., 2017; Makela et al., 2022) while the antibacterial properties of Ag have been effectively enhanced when it is used in the nanoparticle form (Nanda and Saravanan, 2009; Jaidev and Narasimha, 2010; Oves et al., 2019; Das et al., 2020). The large active surface of MNPs makes them highly reactive, and therefore, nanoparticles based on Ag, Ni, Pt, Pd, and others, are distinctly effective in heterogeneous catalysis (Bogireddy et al., 2016; Weng et al., 2017; Stephen et al., 2019; Krebsz et al., 2021). Pd nanoparticles are an example of an efficient catalyst of Suzuki-Miyaura reactions, in which carbon-carbon single bonds are formed to produce a complex variety of molecules that are especially important for the pharmaceutical industry (Søbjerg et al., 2009). Other useful potential applications include the degradation of a variety of toxic Azo dyes present in the wastewater produced by the textile industries (Bogireddy et al., 2016; Narasaiah and Mandal, 2020; Krebsz et al., 2021), and their use in anticancer treatments (Patil et al., 2022).
The targeted production of nanoparticles with specific properties such as defined shapes or size distributions requires strict control of the experimental conditions. Small changes in the production process can have a strong effect on the size, crystallinity, porosity, roughness, and the shape of the produced nanoparticles (Vijayakumar et al., 2013; Jamkhande et al., 2019). Current methods for MNP synthesis are based on different chemical, physical, or biological methods, which can be classified into two major categories: (a) top-down and (b) bottom-up approaches (Figure 1). The preferred approach is dependent on the desired particles characteristics, the size of production, cost of operation, and the applications intended for the produced nanoparticles (de Jesus et al., 2021).
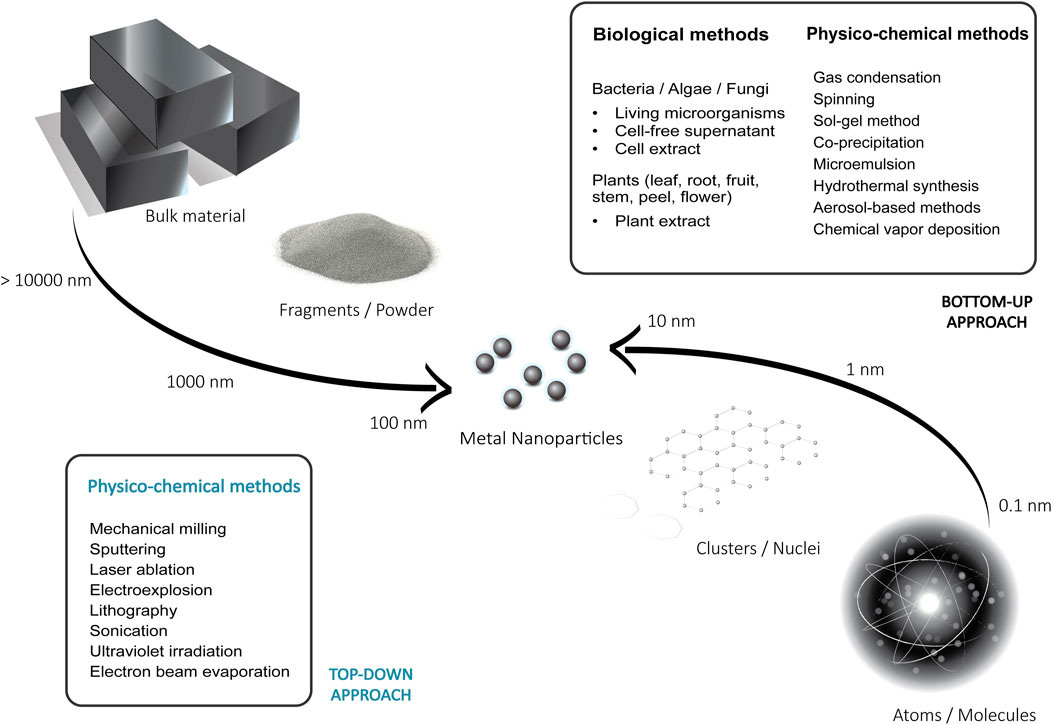
FIGURE 1. Illustration of the top-down and bottom-up approaches for the synthesis of metal nanoparticles.
Top-down methods involve physical or chemical processes that aim to reduce or decompose large substrates or bulk materials. Preparation of MNPs by these methods allows the large-scale production of the high purity nanoparticles, which is crucial for many applications. It includes methods such as mechanical milling (Shojaei et al., 2021), sputtering (Orozco-Montes et al., 2021), chemical etching (Butterfield et al., 2020), laser ablation (Menazea, 2020), electroexplosion (Lozhkomoev et al., 2021), lithography (Fu et al., 2018), sonication (Tang et al., 2019), ultraviolet irradiation (Henglein, 1999), and electron beam evaporation (Nomoev and Bardakhanov, 2012). Traditional top-down methods can produce particles with well-controlled shapes and uniform sizes, however, they usually require specialized fabrication facilities, large amounts of energy, produce hazardous waste and entail high production costs (Fu et al., 2018). Additionally, most of them are not suitable for the preparation of extremely small-sized nanoparticles (<10 nm) (Fu et al., 2018; Jamkhande et al., 2019).
In the bottom-up approaches, dissolved or evaporated substances are used as the basis for growth and synthesis of the particles. Using a variety of chemical reactions and physical processes, the building blocks are assembled by controlled precipitation, crystallization and condensation with a low energy input to result in the nanoparticles (Chan and Kwok, 2011). These bottom-up approaches are widely used, as they tend to be more accessible and cheaper compared to the top-down methods. Among them are gas condensation (Zheng and Branicio, 2020), spinning (Stoller and Ochando-Pulido, 2020), sol-gel method (Parashar et al., 2020), co-precipitation (Andrade Neto et al., 2020), microemulsion technique (Mangaiyarkarasi et al., 2020), hydrothermal synthesis (Vinay et al., 2020), aerosol-based methods (Quintanilla et al., 2010; Yang et al., 2020; Gautam et al., 2021), plasma arcing (Tavares et al., 2008), and chemical vapor deposition (Katsui and Goto, 2021). Frequently, they are referred to as wet methods since most of them involve solvents, stabilizers, reducing agents and other chemicals. Nanomaterials produced by these methods often must be capped to restrict the particle growth and to obtain homogeneous nanoparticle populations. Capping agents are stabilization molecules widely employed to control the material’s particle size, agglomeration, and morphology. They attach to the surface of the nanoparticle and reduce the surface energy, which has a direct effect on the dispersion of the NPs in wet media. Examples include gums, cationic surfactants, polymers, and plant extracts (Restrepo and Villa, 2021). The toxicity of some of the capping agents and other chemicals involved in this process has proven to be an important disadvantage of the techniques, along with the lack of precise control of particle shape, size and dispersity (Fu et al., 2018). As an alternative, bio-based methods have received increasing attention, as they promise a more reliable, non-toxic and eco-friendly synthesis of MNPs (Iravani, 2014).
The production of metal nanoparticles using biological methods belongs to the bottom-up approaches. A great variety of organisms such as plants, bacteria, fungi, and algae have been explored for their potential to synthesize for example silver, gold, iron, palladium, selenium, zinc, and platinum-based nanoparticles (Iravani, 2014; Ahmad et al., 2019; Li et al., 2021; Li et al., 2022). In addition, not only living organisms, but also many chemical substances derived from the metabolism of microbes and plants, such as biopolymers and biological extracts have been proposed as agents for the biological synthesis of MNPs (de Jesus et al., 2021; Soni et al., 2021). The natural reduction and stabilization agents in these reactions result in a potentially more sustainable synthesis compared to current chemical and physical methods. In general, these biological methods are considered to be more inexpensive, non-toxic, and environmentally benign as most reactions occur at room temperature and with a low energy input (Prasad et al., 2021). However, it is pertinent to note that these bio-nanoparticles tend to be polydisperse and difficult to purify. Some of the most significant challenges of nanoparticle biosynthesis include the control of size distribution and dispersity. In addition, it is important to understand the pathways of reduction that may lead to efficient nanoparticle production, and to develop suitable purification protocols (Khalil et al., 2022).
Reports on the synthesis of nanoparticles with bacteria can be categorized into three main methodologies, where NP production is mediated by intact living cells, by bacterial cell-free supernatants, or by cell lysate supernatants (cell extracts). In contrast to NPs formed by intact bacterial cells, supernatant-based synthesis has the advantage of a simpler downstream purification, characterization, and easy visualization of the produced NPs. Most importantly, this biosynthesis is not directly mediated by the metabolic processes of the bacteria, but only by the functional groups of the bacterial proteins or other biomolecules present in the reaction mixture and can be more directly influenced by the experimental conditions. Nanoparticles produced by biological methods are usually capped with non-toxic biomolecule coatings. These bio-coatings can enhance bio-compatibility of the particles e.g., in medical applications, and can also work as stabilizers that promote the formation of homogeneous small-size nanoparticles by preventing their aggregation (Ahmad et al., 2019). An example is the reduced toxicity of iron oxide nanoparticles by the addition of a biocompatible coating of polysaccharides that enables targeted delivery of materials for cancer therapy (Kianpour et al., 2019). The biological synthesis of metal nanoparticles has many potential uses not only in biomedical applications, but also in bioremediation, bioleaching, and biocorrosion (Narayanan and Sakthivel, 2010).
This review provides a comprehensive description of the biological synthesis of metal nanoparticles by bacteria, both in nature and in technical processes. We focus not only on the general interaction of metal ions with bacteria, but also include the findings on the pathways that lead to their reduction and subsequent nanoparticle formation. Examples of recent advances in MNP synthesis using different bacterial strains are presented and their potential applications are reviewed. Finally, the current challenges of the industrial production of metal nanoparticles by bacteria are discussed.
2 Bacteria-metal interactions
Bacteria have ubiquitous interactions with metals. They can be classified into the homeostasis of essential metals, and (heavy) metal resistance to harmful metals. Some metals are important as micronutrients whereas other (heavy) metals (such as Al, Pd, Cd, Au, Hg, Ag) are toxic to living organisms and do not have specific biological roles (Igiri et al., 2018). The essential metals are nutrients and trace elements for key metabolic processes, such as metals in the bacterial respiratory chain. Therefore, microbes have developed a variety of often highly specific mechanisms for metal transport, oxidation/reduction, or detoxification. Nevertheless, the unintentional uptake of high concentrations of metal ions or toxic heavy metals can occur, and microorganisms have developed different strategies to cope with heavy metal stress. Microbial populations can survive in metal-polluted locations by, directly or indirectly, modifying the metal availability through physical or biochemical mechanisms to protect their integrity (Iravani, 2014). The complex mechanisms developed by different species and strains vary and depend both on the native environment and on the heavy metal content. The basic mechanisms of bacteria-metal interactions can be divided into the following four categories:
2.1 Metal sequestration
Many essential biological processes involve metal ions, and in particular, redox active transition metals like manganese, iron, cobalt, copper and magnesium. For instance, divalent ions such as Mn2+ and Fe2+, are indispensable for oxidative stress resistance or as cofactors for the respiratory chain in bacterial core metabolism (Palmer and Skaar, 2016). Hence, microorganisms have evolved pathways of metal sequestration to transport ions and to regulate ion availability. In the simplest case, the sequestration of metal ions occurs by adsorption. In Figure 2, metal ions are depicted as positively charged entities interacting with the bacterial membrane and other biomolecules. During adsorption, deprotonated functional groups (carboxyl, phosphonate, amine, and hydroxyl groups) on the cell surface result in a net negative charge which attracts metal cations and leads to non-specific binding of the metal to the cell surface (Saravanan et al., 2021). This process is independent of the cell metabolism, and it is mainly influenced by factors such as temperature, pH, ionic strength, concentration and by the complex composition of the microorganism’s surface. As an example, Gram-positive bacteria expose a large amounts of carboxyl groups in their peptidoglycan-rich cell walls, making them efficient metal chelators (Pham et al., 2022). As a consequence of this sequestration, these metals are enriched on the cell surface and can be taken up by specific transport processes (discussed below).
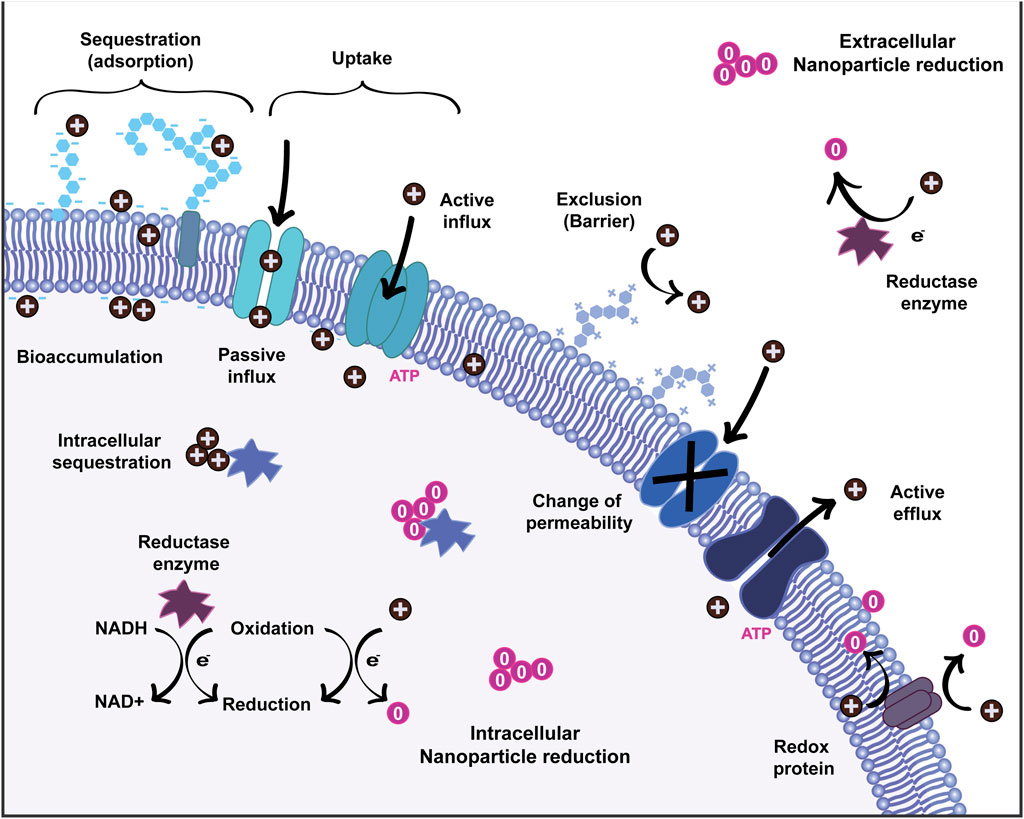
FIGURE 2. Schematics of bacteria interaction with heavy metals and mechanisms of nanoparticle formation.
However, the extracellular sequestration of heavy metals not only allows to accumulate relevant metabolic cofactors, it is also a defense mechanism against toxic metals, by reducing the availability of unwanted metal ions. Polymers secreted by bacteria such as the exopolysaccharide (EPS) coatings of some species, have the ability to adsorb and bind metals extracellularly, thus acting as a protective layer that prevents the uptake of toxic metals (Bruins et al., 2000). Bacteria are likely to sequester non-essential metal ions, such as Au and Pd, by adsorption mechanisms. Compared to other microorganisms, Gram-negative bacteria such as Acinetobacter calcoaceticus, Erwinia herbicola, Pseudomonas maltophilia and Pseudomonas aeruginosa have the highest ability to accumulate metals such as gold (Tsuruta, 2004). This is a result of the surface charge present on the Gram-negative bacterial membrane, which promotes the gold adsorption from aqueous solutions. In the same way, the biosorption of Pd is presumably a result of the chemical affinity of metal complexes such as [PdCl4]2- for protonated groups on the cell surface at an acidic pH (Deplanche et al., 2010).
Free metal ions passively or actively transported from outside of the membrane into the cytoplasm can pose a danger to the cell and must be immobilized or transformed. Bacteria can produce metabolites, like metallothioneins, phytochelatins and glutathione with high affinity for metals for the intracellular sequestration of metal ions. Metallothioneins are small, cysteine-rich proteins that are directly involved in the homeostasis of different metal ions, including Cu+ and Zn2+. These proteins have been reported in bacteria such as Bacillus altitudinis MT422188 and Mycobacterium tuberculosis where they are expressed under Zn2+ and Cu+ induced stress, respectively (Johnstone and Nolan, 2015; Khan et al., 2022). The immobilization of the ions decreases the toxicity; however, the metal sequestration mechanisms are limited by the saturation of the binding sites in the extracellular and intracellular matrix (Bruins et al., 2000).
2.2 Metal uptake
The metal ions in proximity to the extracellular layers can be imported by passive or active influx through the different layers of the cell wall, using non-specific transporters, metal-specific channels or passive diffusion (Figure 2) (Bruins et al., 2000). The indispensable uptake of essential metals is highly regulated and typically mediated by specific, energy-dependent uptake systems. In E. coli, Alcaligenes eutrophus and many other enterobacteria, essential trace elements such as Ni2+, Co2+, and Zn2+ ions are transported into the cell by constitutive Mg2+ transport systems (Nies, 1992; Komeda et al., 1997). These relatively unspecific uptake systems allow the “cost-effective” accumulation of macronutrients such as Mg and of trace metal ions in environments with habitual metal concentrations. In extreme conditions, with deficits in trace metals, bacteria can upregulate genes related to specific, ATP-dependent uptake systems for these elements in order to transport the ions against the concentration gradient. The TonB-dependent transporter, OprC, is repressed in P. aeruginosa by high exogenous Cu ion concentrations and expressed in anaerobic conditions to bind and transport Cu+/2+ (Bhamidimarri et al., 2021). In environments with low availability of essential metals, many bacteria also secrete metal-sequestering proteins or other chelators to bind the biologically relevant ions and to specifically import them (Palmer and Skaar, 2016). An example of this mechanism are iron siderophores, which are low molecular weight compounds known for their high affinity for Fe ions. Siderophores are frequently used by pathogenic bacteria to chelate Fe2+ and Fe3+ in host environments that are otherwise deprived of free Fe ions (Johnstone and Nolan, 2015). The siderophores with bound ions are then recognized by specific outer membrane receptors that guide the chelated ions through energy-dependent ATP-binding cassette (ABC) transporters (Krewulak and Vogel, 2008; Ma et al., 2009). Because of the different chemical properties of Fe3+ and Fe2+, bacteria utilize different specific transport systems (and siderophores) for each ion (Lau et al., 2016; Crespo et al., 2017). Examples of different transport systems involved in bacterial Fe uptake, include MntH, ZupT, YfeABCD, FutABC, EfeUOB and Feo (Lau et al., 2016).
The uptake of precious metals like Au, Ag, Pt, and Pd ions into bacteria is not well understood, as cells do not possess inherent active, specific transport mechanisms to regulate non-essential ion uptake. The absorption of toxic heavy metals is presumably a process carried out by passive mechanisms such as diffusion. Therefore, at elevated concentrations, non-essential metals can interact with the cell, cross into the cytoplasm and accumulate there (Lusa et al., 2016). However, some authors suggest that heavy metals like Pd may also accidentally be absorbed and imported into living cells through systems specific for essential metallic metabolites, such as Fe, Ni, Cu or Zn (Omajali et al., 2015). Metal ions with comparable properties and ion radius, due to their similar conformations, can out-compete essential ions in binding to proteins. As an example, the high affinity of Hg and Cd ions to cysteine-rich sites in Zn-binding proteins results in translocation of these toxic metals. A similar effect is found in Cu-binding proteins with Au and Ag as competing metal ions (Waldron and Robinson, 2009). A special case of non-essential metal uptake is the homeostasis mechanism of specialized metal-resistant bacterial strains for some toxic heavy metal ions. In the case of Cupriavidus metallidurans, genes in the gig (gold-induced genes) operon are strongly upregulated after sequestration of Au ions in the membrane. The proteins encoded by this operon are suggested to be directly involved in the import of Au ions into the cytoplasm to prevent the harmful action of Au3+ in the periplasm. The reduced complexes there are later removed from the cytoplasm (Wiesemann et al., 2013; Zammit and Reith, 2013).
2.3 Metal detoxification
If not regulated, high concentrations of metals would have adverse effects in the metabolic processes of the cell. In metals such as Cu, their redox activity favors the formation of active oxygen species, which explains their toxicity. Excess of essential metals or toxic, non-essential heavy metals also leads to mismetallation of proteins which results in their inactivation (Andrei et al., 2020). The exposure to high concentrations of heavy metals activates a mechanism of defense called metal exclusion, in which the permeability of the cell membrane, the envelope, or the surface layer is modified to prevent or reduce the further influx of metal ions (Figure 2). Bacteria can alter the composition of the cell wall in an attempt to form a barrier for ions. A well-known example of this mechanism is the reduction of the expression of Porin channels in heavy-metal resistant mutants of E. coli. In this case, pore proteins such as OmpF are expressed at a reduced rate, leading to increased Cu and Ag resistance (Lutkenhaus, 1977; Rouch et al., 1995; Li et al., 1997). In a complementary mechanism, the active efflux of metal ions involves the use of highly specific membrane transport systems. This process to decrease the concentration of harmful metal ions inside the cell is wide-spread among bacteria. The transport of cations and anions from the cytoplasm against a concentration gradient requires energy. The involved efflux pumps can be broadly classified by their energy source into ATP-dependent and proton-gradient-based systems. Further classifications are based on sequence similarity, transport function and substrate specificity (Nies and Silver, 1995; Hynninen, 2010; Delmar et al., 2014).
Some examples of well-studied heavy metal efflux pumps are the P-type ATPase transport protein CopA, known to be involved in copper resistance (Rensing et al., 2000) and the resistance-nodulation-cell division (RND) family proteins, CzcA and CusA, which bind and export Cu+ and Ag+ in E. coli (Kim et al., 2011). In partly silver-resistant E. coli bacteria, the detoxification mechanism for silver is based on the expression of the CusCFBA copper/silver efflux systems that actively export the silver ions from the cytoplasm and bind it in the periplasm (Lin et al., 2014). In some bacteria, metal ions transported by efflux pumps may be retained in the periplasm even after the efflux process, along with the metal ions sequestered by EPSs and by other metal-binding proteins (Blindauer, 2011). The ions bioaccumulated in the cytoplasm, periplasm and in the extracellular matrix can later become sites for nucleation and formation of nanoparticles (Figure 2).
2.4 Metal transformation
Some microorganisms have a remarkable ability to transform heavy metal ions in their environment and to produce MNPs. Even when sequestered, the high reactivity of transition metal ions could disrupt metabolic processes or damage DNA molecules. Various metal ions have a high binding affinity for biological macromolecules such as nucleic acids, or thiol-containing proteins (Delmar et al., 2014; Boedicker et al., 2021), and therefore, they need to be transformed into inert or less toxic substances. This is mediated by redox-active metabolic pathways in order to change solubility and toxicity, and often leads to complexation or precipitation of metals extracellularly (Silver, 1996; Bruins et al., 2000; Hood and Skaar, 2012). Alternatively, biomethylation is a metabolic process in which methyl groups are transferred to the toxic metal ions or metalloids to create less toxic, sometimes volatile compounds. Hg, Sn, As, Se, Te, Au, Tl, and Pb have been reported as methyl group acceptors in primary and secondary metabolic processes (Kosolapov et al., 2004). The resulting products are more toxic than the inorganic compounds in many cases, so it is debatable whether methylation is, in fact, a detoxification mechanism. However, it is important to note that the volatility has an important effect on reducing the concentration of the metals in the surrounding environment. Extended descriptions of the general pathways for biomethylation can be found elsewhere (Ridley et al., 1977a; Ridley et al., 1977b; Fatoki, 1997).
Some bacteria can decrease the mobility of metal ions through enzymatically promoted redox reactions that reduce the metals irreversibly, leading to the assembly into metal clusters. An illustration of the general process of reduction of the metal ions to neutral ions by redox proteins intra- and extracellularly can be found in Figure 2. The electron-accepting potential of different metal ions known to be reduced by bacteria are shown in Table 1. The reduction potential is expressed as the E0 value, ranging from very negative E0 values for compounds that are easily oxidized, to large positive E0 values for compounds that readily accept electrons (are easily reduced). “Precious” metals such as Au, Pd or Ag have cations species with very positive potentials and are frequently reduced into nanoparticles by bacteria.
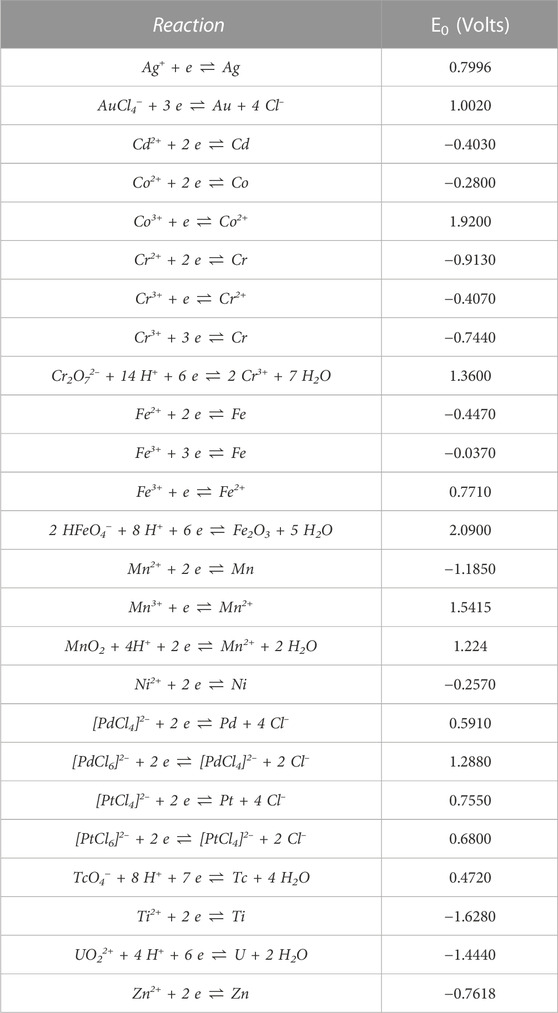
TABLE 1. List of relevant standard reduction potential (E0) in respect to the standard hydrogen electrode at 298.15 K (25°C), 101.325 kPa (1 atm). The list is in alphabetical order, according to the symbol of metal element. Taken from Haynes, (2014).
The cornerstone of every living organism is energy conservation where chemical or light energy is ultimately converted into adenosine triphosphate (ATP). ATP production is facilitated by substrate-level phosphorylation, oxidative phosphorylation, or photophosphorylation. In order to achieve this, electrons need to be transferred in an oxidation-reduction coupled reaction. This electron movement within the cell is facilitated by electron carriers, such as nicotinamide adenine dinucleotide (NAD+/NADH), which promotes a diversity of reduction-oxidation reactions as they allow a variety of electron partners to interact with each other (Muller, 2003; Herrmann et al., 2008; Schuchmann and Müller, 2012). It is these metabolic reactions and electron carriers that are diverted and used for metal ion reduction.
On an extracellular level, bacterial growth is heavily influenced by the oxidation-reduction (redox) potential of the environment, and in turn, bacteria can modify the redox potential of their environment. Surprisingly, this ability is highly species-specific and can be exploited to identify different species by using redox electrodes (Reichart et al., 2007). A change in redox potential indicates the availability of electron acceptors and donors. Since bacteria can inhabit every niche that can support life, their diversity is also manifested in the type of electron acceptors that they can utilize intracellularly. Under aerobic respiration conditions, oxygen is the final electron acceptor while under anaerobic respiration conditions, a variety of electron acceptors can be utilized, such as nitrate (NO3-), trimethylamine oxide/dimethyl sulfoxide (TMAO/DMSO), carbon dioxide (CO2) or organic electron acceptors such as fumarate, depending on the bacterial species studied (Kröger et al., 1992; Vincent et al., 2021). Metal-based respiration is characterized by the utilization of manganic manganese (Mn4+) or ferric iron (Fe3+) as electron acceptors. Bacteria can reduce these metal compounds through mechanisms that involve redox-active proteins like reductases, cytochromes, and metallothioneins (Bloch et al., 2021).
Sulfate- and metal-reducing bacteria are frequently employed for the synthesis of metal nanoparticles, as these organisms are rich in membrane redox-active proteins and therefore easily enzymatically reduce different metal ions. These ‘reducing’ microorganisms can use the metal cations as terminal electron acceptors while performing anaerobic respiration, reducing Fe3+ to Fe2+ and Mn4+ to Mn2+ for energy conservation (Lloyd, 2003). These proteins however, are not necessarily highly specific and multiple enzymes are capable of reducing several redox-active metals ions (Barton and Fauque, 2009). The interaction of some bacteria with non-essential, toxic metal ions such as Au3+, Ag+, Pd2+ and Pt2+ also upregulates the expression of multiple redox-active enzymes located on the cell walls, in periplasmic space and intracellular contents, such as hydrogenases and NADH reductases, which can reduce ions to insoluble complexes that are subsequently transformed into MNPs (Omajali et al., 2015; Chen et al., 2019). One example is nitrate reductase known to be involved in the bioreduction of the bound Ag+ to Ag0 in Bacillus licheniformis as defense from heavy metals exposure (Kalimuthu et al., 2008; Rajora et al., 2016). As suggested by their negative reduction potential (Table 1), the NAD(P)H-dependent reduction of ions such as Cd2+, Zn2+, Co2+ or Ni2+ to metallic form may not be energetically favored, so the reduction of these ions is uncommon in bacteria and the particular cases of reduction must involve different mechanisms (Nies, 1992). In Shewanella strains, EPS-induced reduction and indirect electron transfer using electron shuttles such as flavins, L-cysteine, and quinones are possible alternative pathways that have been thoroughly described elsewhere (Yang et al., 2023). Metal NPs reduced and precipitated in the extracellular and intracellular space may possess different characteristics than their chemically produced counterparts. The biomolecules associated with them create a biological cap that stops nanoparticle growth, minimizes cytotoxicity, and may affect their chemical and physical properties (Bulgarini et al., 2021). The reduced metal particles accumulate without much effect on the cell population. Therefore, the formation of MNPs can be regarded as a by-product of microbial defense mechanisms, of bacterial metabolism, or as a biomineralization process intended to control, immobilize, or decrease the heavy metal bioavailability.
3 Bacterial synthesis of nanoparticles
During the microbial reduction of metals into MNPs, cations are transformed into ions with a lower valence. A special case is the precious metals, including Pd, Pt, Au, and Ag, that are easily reduced into elementary forms (Zhan et al., 2012). The challenge of producing nanoparticles using bacteria, is identifying the exact chemical components and mechanisms involved in nanoparticle formation. The pathways may result in nanoparticles formed only extracellularly, intracellularly, or both, and there is a debate about which method is the most efficient for production of NPs in industrial processes. The intracellular nanoparticles formed in living cells are attached to the biological material, making their purification difficult; however, capping of the nanoparticles provides better control of their size, generating more homogeneous populations of biocompatible nanoparticles with interesting novel physical and chemical properties. In contrast, nanoparticles produced externally are preferred in many cases due to their faster production, facile recovery, and simple purification. These extracellular nanoparticles can be produced using bacterial cell-free supernatants, cell lysate supernatants, and some living bacterial strains. In this section, examples of relevant metal-based nanoparticles produced by bacteria are described.
3.1 Bacterial metal nanoparticles in nature
Even though naturally occurring nanoparticles are widely found in living organisms, the intracellular accumulation of solid metals is usually not a favorable condition for the cells and can lead to cell death. A complete metal particle detoxification would require energy and elaborated mechanisms of particle excretion (Griffin et al., 2017). This is probably the reason why only few bacterial species produce metal-based nanoparticles as part of their normal metabolic processes. The most remarkable example are magnetotactic bacteria, an extraordinary group of Gram-negative organisms that rely on biosynthetic iron-based crystals to orient and migrate along the geomagnetic field lines in a behavior known as “magnetotaxis” (Yan et al., 2012). The highly specialized organelles made of nanometer-sized metal crystals wrapped in a membrane are called magnetosomes. They are composed of magnetic iron oxide magnetite (Fe3O4) or iron sulfide greigite (Fe3S4) of different shapes and sizes depending on the exact bacterial species. The magnetosomes are frequently aligned in chains across the motility axis of the cell. This imparts the bacteria with a permanent magnetic dipole moment, causing them to align passively in a parallel orientation with external magnetic fields (Monteil and Lefevre, 2020). The cells swim along the geomagnetic field lines of the Earth to the oxic-anoxic interface of the aquatic habitat where are the most favorable conditions for growth (Le Nagard et al., 2018).
Despite the abundance of magnetotactic bacteria in the environment, only a few strains have been isolated into pure culture due to their particular growth requirements. Strains from the proteobacterial genus Magnetospirillum are the most common isolates. However, recent publications report up to 16 different lineages that include Proteobacteria, Nitrospirota, and Omnitrophota (Gareev et al., 2021; Goswami et al., 2022). The strains Magnetospirillum gryphiswaldense MSR-1 [first isolated in 1991 by Schleifer et al. (Schleifer et al., 1991)], Magnetospirillum magnetotacticum strain MS-1 and Magnetospirillum magneticum AMB-1, are currently the most studied bacteria for the understanding of magnetosome formation and its complex genetic regulation (Nudelman and Zarivach, 2014). In M. gryphiswaldense MSR-1, the magnetosome island (MAI) comprises most of the genes that control the magnetosome synthesis. These genes encode all the essential processes in the majors steps of the organelle formation and determine the morphology and chemical composition of the particles (Uzun et al., 2020; Dziuba et al., 2021). In the past, different models of magnetosome biomineralization have been proposed (Schuler, 2002; Yan et al., 2012; Nudelman and Zarivach, 2014). Currently, four major steps are considered necessary to achieve the biomineralization. The first step is i) the cytoplasmic membrane invagination into vesicles, followed by ii) the arrangement of specific magnetosome proteins into the organelle membrane. Then, iii) the internalization and accumulation of iron into the vesicles by energy-dependent transport proteins takes place. Here, the cell rapidly chemically transforms the Fe ions and combines them into metal crystals. Specific proteins guide this process in order to avoid the toxic effect of intracellular Fe. Finally, iv) the magnetosomes are assembled into a linear chain along cytoskeletal filaments (Dziuba et al., 2021).
The bacteria tightly controls the size and morphology of the magnetosomes. Magnetosome crystals typically fall in a size distribution of about 30–120 nm. However, some species can form particles up to 250 nm in length (Yan et al., 2012). The spirillum-shaped bacterium M. magneticum AMB-1, originally collected from fresh water sediments of a natural spring in Tokyo, presents magnetite magnetosomes with an average diameter of 50 nm, aligned in chains of around 15 particles per cell (Matsunaga et al., 1991). In contrast, the M. gryphiswaldense MSR-1 bacteria were found to produce chains of up to 60 homogeneous magnetosomes with particle sizes of around 42 nm (Schuler, 2002). Both strains, like other bacteria from the Magnetospirillum genus, produce cubo-octahedral crystalline magnetosomes. In general, three different morphologies of magnetosomes can be found in nature: i) cubic or cubo-octahedral, ii) elongated hexa- or octahedral and iii) even more elongated crystals with large, anisotropic faces, showing tooth, arrowhead, or bullet shapes (Cypriano et al., 2022). These crystal structures are consistently replicated in the magnetosomes of bacteria of the same strain and have a direct influence on the final magnetic behavior of the particles.
The magnetosomes, due to their narrow size distribution, low aggregation, and strong ferromagnetism, have many potential technical applications. The low toxicity of bacterial magnetosomes in comparison with synthetic magnetic nanoparticles makes them especially attractive for biomedical applications. Cytotoxicity of bacterial magnetosomes varies depending on various factors, including cell type, incubation time, and concentration. While many types of magnetite nanoparticles [such as Superparamagnetic iron oxide nanoparticles (SPION)] are considered cytotoxic at exposure levels above 70–100 μg/ml (Alphandéry, 2014; Singh et al., 2010; Toropova et al., 2017), a study by Alphandéry et al. in MDA-MB-231 cells suggested low cytotoxicity due to magnetosome exposure at concentrations below 1,000 μg/ml which can be further improved by removing bacterial endotoxins and using biocoatings (Alphandéry et al., 2011; Gareev et al., 2021). In a recent study, Gwisai et al. evaluated the use of the ferromagnetic properties of AMB-1 bacterial magnetosomes for targeted cancer therapy. Thanks to their susceptibility to external magnetic fields, magnetotactic bacterial cells were magnetically manipulated and studied as a vehicle for tumor infiltration and drug delivery (Gwisai et al., 2022). In a similar approach, Xing et al. successfully demonstrated the colonization and ablation of tumors in mice by means of in vivo magnetically manipulated M. magneticum AMB-1 bacteria, showing their enormous potential for an efficient cancer treatment (Xing et al., 2021). Other potential application of magnetosomes include their use as magnetic resonance contrast agents. In vivo experiments were conducted on mice to examine how magnetosomes were distributed and eliminated after injection as a contrast agent in magnetic resonance imaging (MRI). The researchers concluded that their use in MRI was adequate in terms of spatial resolution and sensitivity (Nan et al., 2021).
Microorganisms play an important role in the process of biomineralization and metal geochemistry. The metals transformation into nanoparticles is also a natural process observed in some astounding microorganisms known as dissimilatory metal-reducing bacteria. In anoxic environments with abundant metal species such as Fe3+, Mn3+/4+, U6+, Cr6+, Co3+ and Tc7+, these metal cations are reduced into nanoparticles by the bacteria (Lovley, 1993; Shi et al., 2016). All these elements have in common that they are good electron acceptors due to their high reduction potential. Then, during anaerobic respiration, the bacteria can replace oxygen as the terminal electron acceptor with the available cations. Fe3+ is the most abundant possible electron acceptor in anoxic environments. Due to its comparatively high reactivity with oxygen, elemental Fe is not frequently found in nature, but instead, is commonly found in the form of iron oxides. In metal- and sulfur-reducing microorganisms like, Geobacter sulfurreducens, Geobacter metallireducens GS15 and Shewanella oneidensis MR1, specialized membrane proteins are suggested to be involved in the Fe3+ reduction. Their outer membranes are rich in c-type cytochromes that transfer electrons coupled to the oxidation of lactate and other carbon sources to soluble Fe3+ oxides resulting in iron oxide NPs (Lovley et al., 1993; Mehta et al., 2005; Shi et al., 2012). Many Fe-based NPs naturally synthesized by bacteria consist of diverse compositions of iron oxides. The metal-based anaerobic mechanism of respiration is considered part of the natural cycle of precipitation of many heavy metals into anoxic sediments (Lovley, 1993). Multiple processes of bioremediation of heavy metals from the environment and contaminated water streams have been engineered based on the reducing ability of these bacteria (Iravani and Varma, 2020). Other transition metals such as gold, silver, and copper have high positive reduction potentials and are exchanged with iron. Bacteria readily precipitate them during remediation, which can then be applied to the biogenic production of these metal nanoparticles.
3.2 Biosynthetic nanoparticles
Bio-inspired NPs of different metals have been successfully synthesized using a large variety of bacterial strains. Under optimal conditions in temperature, pH and redox state, some bacterial species can reduce metal ions and form nanoparticles. Among the metals successfully recovered by bacteria in form of nanoparticles are iron, manganese, chromium, cobalt, palladium, gold, silver, arsenic, selenium, uranium, and polonium (Boedicker et al., 2021). Their resulting size-distribution, morphology and functional properties are highly dependent on the protocols used for the biosynthesis as well as on the type of bacteria and metal involved. In Table 2, details of recent, published protocols for metal nanoparticles fabrication using different bacterial strains are listed.
3.2.1 Gold nanoparticles
Metallic gold (Au) can be used in a wide range of applications due to its high stability, oxidation resistance, and biocompatibility. In nanoparticle form, gold displays an improved potential for chemical catalysis and has antimicrobial effects. Despite these antibacterial properties, some microorganisms have been successfully used to synthesize Au NPs. One of the first bacterial species studied for the reduction of Au was Bacillus subtilis. In their work, Beveridge and Murray exposed bacterial cells to solutions of Au3+ chloride at room temperature and observed the formation of Au NPs of octahedral morphology with sizes of approximately 5–25 nm (Beveridge and Murray, 1980; Iravani, 2014). Since then, many other species and strains have been examined for their capacity to synthesize Au NPs of diverse morphologies and dimensions. Pseudomonas stutzeri cells produce Au NPs in the size range of 10–20 nm with a spherical morphology when incubated at 80°C in a medium with chloroauric acid (HAuCl4) as the precursor (Desai et al., 2021). Escherichia coli also biosynthesizes spherical Au NPs found primarily in the extracellular matrix with dimensions that vary with the pH of the medium from 6 to 60 nm in size. In similar conditions, Au NPs of sizes between 5 and 40 nm can be prepared with Bifidobacterium lactis bacteria, but with hexagonal morphologies and mostly intracellularly (Chen et al., 2021). Thus far, however, the complex mechanisms of Au NPs formation by bacteria are still not completely understood. For Lactobacillus casei bacteria, it was found that high cell numbers and high concentrations of Au salt solutions inhibit the particle formation. The same study suggested a direct participation of unsaturated fatty acids from di- and tri-glycosyldiacylglycerol glycolipids in the membrane in the formation of Au NPs (Kikuchi et al., 2016).
3.2.2 Silver nanoparticles
The production of Ag NPs is of special interest for their uses in the biotechnology industry. Drug delivery, diagnostics, cancer treatment and antibacterial agents are some of their most known applications (Jain et al., 2021). Several bacterial species have been exploited in the biogenic synthesis of intracellular and extracellular Ag NPs, including species from the Pseudomonas (Rajora et al., 2016; Quinteros et al., 2019), Lactobacillus (Riaz Rajoka et al., 2020; Abishad et al., 2022) and Bacillus (Mukherjee et al., 2018; Saravanan et al., 2018; Ibrahim et al., 2021; Esmail et al., 2022) genus. An example is P. stutzeri AG259, which was originally isolated from a silver mine and can bioaccumulate and synthesize silver crystalline nanoparticles when cultured in presence of high concentrations of silver salt (AgNO3). These Ag and Ag2S NPs are usually found in the bacteria plasma membrane and display triangular or hexagonal morphologies with a wide range of sizes from 35 to 200 nm (Klaus et al., 1999). Synthesis of Ag NPs with cell-free supernatants of Stenotrophomonas maltophilia bacteria results in cuboidal NPs of the average size of 93 nm (Oves et al., 2013), while Pseudomonas aeruginosa extracts can be used to synthesize spherical nanoparticles of 25 nm in size (Quinteros et al., 2019).
3.2.3 Platinum nanoparticles
Metals from the Platinum group of elements are notorious for their outstanding catalytic properties, in particular when used in nanoparticle form. However, limited studies have been conducted with bacteriogenic Pt nanoparticles, and their applications are rarely explored (Bloch et al., 2021). Zero-valent Pt NPs produced by the acidophilic Fe3+-Reducing Acidocella aromatica PFBC and Acidiphilium cryptum SJH bacterial strains were tested in a Cr6+ reduction experiment for their catalytic activity. The experiment demonstrated comparable catalytic efficiency to a commercially purchased Pt/C catalyst (Matsumoto et al., 2021). Yet, the potential applications of Pt NPs also include their use in diagnostics (Dash et al., 2021), anticancer treatments (Baskaran et al., 2017), and as antibacterial agents (Hosny et al., 2022).
Live bacterial cells and bacterial lysate supernatants have been studied for Pt NP production. Typical synthesis of intracellular Pt NPs is performed under anaerobic conditions and starts with the incubation of bacteria such as E. coli or Plectonema boryanum in aqueous solutions of H2PtCl6 or Na2PtCl4 (Lengke et al., 2006; Attard et al., 2012; Gaidhani et al., 2014). The cells are then loaded with the metal ions and in some cases sodium formate solutions are added as an external electron donor for accelerating the metal reduction. For the extracellular biosynthesis of nanoparticles, the cells are utrasonicated and centrifuged. Then only the cell lysate supernatant is incubated with the Pt precursor solutions. Most of the Pt nanoparticles crystalize in spherical morphologies. Pseudomonas kunmingensis ADR19, Psychrobacter faecalis FZC6 and Pseudomonas putida KT244 synthesize spherical Pt NPs of the average sizes of 3.95, 2.49 and 8.06 nm (Eramabadi et al., 2020). Other species, such as Acinetobacter calcoaceticus, synthesize small cuboidal Pt NPs of 2–3.5 nm in size (Gaidhani et al., 2014).
3.2.4 Palladium nanoparticles
Palladium is a precious metal with outstanding catalytic properties. Currently it is extensively used in car converters and is crucial in a large variety of industrial-scale chemical reactions; in particular, it is an indispensable tool for modern organic synthesis (Chernyshev and Ananikov, 2022). In nanoparticle form, palladium is utilized for hydrogenation and as a carbon-carbon bond forming catalyst in Heck reactions and in Suzuki-Miyaura coupling. The synthesis of Pd NPs by bacteria has progressed rapidly, and a wide range of bacterial species and strains have been studied for the efficient production of nanoparticles. A recent study by Mikheenko et al. describes the formation of Pd NPs by sulfate-reducing bacteria, Desulfovibrio desulfuricans, and their application for the Heck reaction and hydrogenation. The cells were grown in anaerobic conditions, and a Na2PdCl4 solution in HNO3 has been used with H2 gas as supporting electron donor. The examination of the samples showed Pd NPs on the cell surface and in the different membrane layers with sizes in the range of 3–13 nm (Mikheenko et al., 2022). Some additional examples include the metal-reducing bacterium Shewanella oneidensis MR-1, which can synthesize extracellular 2–12 nm Pd NPs (Zhang et al., 2022a), the metal-resistant species Cupriavidus metallidurans (Tan et al., 2020), and the sulfate-reducing bacterium, Desulfovibrio fructosivorans (Mikheenko et al., 2008). Other strains studied for their capacity for Pd NP synthesis, which are not specialized in metal reduction for detoxification include E. coli (Bachar et al., 2020), Bacillus megaterium (Chen et al., 2019), and Bacillus benzeovorans (Omajali et al., 2015).
3.2.5 Iron-based nanoparticles
Iron is an indispensable element for all living organisms, known mainly as a co-factor in important biological redox reactions. The use of iron in nanoparticle form has attracted considerable interest in applications as contrast agents, as a drug carrier, and in cancer treatment (Samuel et al., 2021). It should be noted that not just the reduced metal but also some iron oxides possess remarkable magnetic properties that are attractive in countless applications, making the development of new methods for the synthesis of Fe nanoparticles an attractive field of research. Bio-production of iron-based nanoparticles is frequently described as a clean, simple, and economic way to obtain Fe NPs. Organisms such as bacteria can utilize various Fe sources to fulfill their nutritional requirements and have elaborate mechanisms to regulate the uptake of Fe ions. Some bacteria, including metal-reducing and magnetotactic bacteria (see above), also have the ability to reduce Fe ions and form crystals naturally.
For Fe-based NP biosynthesis, different sources of iron can be used, such as FeSO4, FeCl3, FeCl2, and iron citrate. The NPs produced by E. coli living cells can be found intracellularly and extracellularly, depending on the iron precursor used, the pH and the metal concentration (Crespo et al., 2017). E. coli and P. aeruginosa cell extracts facilitate the synthesis of spherical magnetic Fe3O4 NPs when incubated on 1 mM FeSO4 at pH 6.5 for 48 h and 37°C (Crespo et al., 2017). Fe3O4 NPs were also produced in cell-free Enterococcus faecalis supernatants incubated with FeCl3 and FeCl2 solutions. In this case, the reported nanoparticles were cubical, hexagonal, and irregular in shape of between 15 and 20 nm in size (Samuel et al., 2021). Bacillus cereus cell-free supernatant can also be used to reduce Fe into spherical magnetic Fe3O4 NPs in the size range of 18–29 nm (Fatemi et al., 2018).
3.2.6 Cobalt-based nanoparticles
Cobalt is a transition metal with interesting magnetic, catalytic, optical, and electrical properties. Cobalt oxide nanoparticles are considered promising in technologies for energy storage (Shim et al., 2011; Hsu et al., 2018), catalysis (Jang et al., 2015), and as antimicrobial agents (Hafeez et al., 2020; Mubraiz et al., 2021). The synthesis of pure Co NPs by microorganisms can be challenging due to the extreme toxicity of high concentrations of Co ions. Similar to Fe, Co is highly reactive in zero-valent state, and when in contact with aqueous solutions, it rapidly oxidizes. Therefore, the NPs produced by bacteria are typically composed of more stable, oxidized forms of cobalt such as Co3O4.
In G. sulfurreducens, the upregulation of c-cytocrome OmcC in Co-rich environments is an indication of Co competition with Fe for binding to the cytochromes, that ultimately promotes the reduction of Co2+ to Co0 and its precipitation on the cell surface (Dulay et al., 2020). In other methods, Co3O4 NPs of 10–31 nm size are produced by chemical alteration in the media when the urealytic Bacillus pasteurii hydrolyzes urea in the presence of high concentrations of Co(NO3)2 (Hsu et al., 2018). Brevibacterium casei bacteria produce quasi-spherical 6-nm Co3O4 NPs when incubated in aqueous solution of cobalt acetate (Kumar et al., 2008). Other strains studied for Co NP synthesis include Micrococcus lylae, Bacilus subtilis, E. coli, and Haloarcula vallismortis (Jang et al., 2015).
3.2.7 Nickel-based nanoparticles
In microorganisms, there are highly specific and efficient systems for the transport and regulation of nickel in the cell to avoid its accumulation and toxicity in the cytoplasm (Navarro et al., 1993). Taking into account that at high concentrations Ni is extremely toxic to bacteria, the interaction between Ni and the cells has to be carefully controlled. In addition, due to its low redox potential the reduction of Ni ions by bacteria is not energetically favorable for NP formation under standard growth conditions (Zhan et al., 2012). However, a recent study with metal-reducing bacteria Pseudomonas alcaliphila described the successful reduction of Ni2+ by incubation at 28°C under aerobic conditions in a solution with 2 mM NiCl2 and sodium citrate as an electron donor. The formed Ni NPs were found intracellularly and in the bacterial periplasm, with irregular shapes (Zhan et al., 2012). Another example is the synthesis of NiO NPs by Microbacterium sp. MRS-1, as a result of the bioremediation of nickel electroplating industrial wastewater. The synthesized Ni NPs had spherical, flower-like structures and displayed particle sizes in the range of 100–500 nm (Sathyavathi et al., 2014).
3.2.8 Other elements, alloys, and bimetallic nanoparticles
Many other types of metal-based nanoparticles have been produced successfully using bacteria. These include nanoparticles composed of elements such as Zn, Cr, Cd, Ti, Cu, and different mixtures of metals. For example, antibacterial nanoparticles made of TiO2 and ZnO can be bio-synthesized with bacteria. The Gram-negative bacterium Halomonas elongata can be used to produce spherical TiO2 NPs and anisotropic ZnO NPs by incubating the cells with various concentrations of TiO(OH)2 and ZnCl2 solutions at different temperatures. The synthesized TiO2 and ZnO NPs were on average of 104.63 ± 27.75 and 18.11 ± 8.93 nm in size, respectively (Taran et al., 2017). Other examples are the nanoparticles produced by the metal-reducing bacterium Shewanella oneidensis MR-1, known for its ability to live in heavy-metals polluted environments and studied for the synthesis of NPs of a wide range of metal ions, including ZnS (Taran et al., 2017), Pd (Zhang et al., 2022a; Zhang et al., 2022b), Cr (Wang et al., 2013) and U (Burgos et al., 2008). Synthesis of Te NPs has also been reported using a methanogenic microbial consortium in 270 ml continuous reactors as an alternative of Te recovery in wastewater treatments (Ramos-Ruiz et al., 2017).
Although microbial-mediated monometallic nanoparticles have been extensively studied, there is limited research on the production of bimetallic nanoparticles by bacteria. The synergistic effect of metal combinations can result in superior or novel catalytic, electronic, and optical properties. Therefore, bimetallic nanoparticles often exhibit improved performance that could be interesting for different applications (Xu et al., 2019). Exposing bacteria to high concentrations of various mixes of heavy metal ions can result in the formation of nanoparticles made of combinations of these elements. Bimetallic nanoparticles can have structures such as core-shell, alloys, crown jewel, cluster-in-cluster, hollow, and porous NPs (Sasireka and Lalitha, 2021) and it is not surprising that the synthesis methodology has a direct effect on the final architecture of the formed bimetallic NP. An example is the synthesis of spherical bimetallic Pd-Pt NPs by S. oneidensis MR-1 prepared by incubation of the cell’s biomass in a Pd2+ and Pt4+ mixed solution. Research showed that the synthesized polycrystalline Pd-Pt NPs possessed an improved catalytic performance in comparison with pure Pd or Pt NPs (Xu et al., 2019). Extracellular biosynthesis of bimetallic FeO-MnO NPs can be performed using Paenibacillus polymyxa. In this case, cell-free extracts were incubated with solutions of FeCl3 and MnSO4 at 45°C in the dark for 5 h and the formed spherical nanoparticles had sizes in the range of 11.28–543.59 nm. These particles are suitable as micronutrient additives to fertilizers for crop production (de França Bettencourt et al., 2020). For core-shell Pd-Ru bimetallic NP production, Gomez-Bolivar et al. incubated E. coli bacteria in a Pd2+ solution for an initial reduction of Pd under H2 on the cell surface. The biomass with Pd0 was then resuspended in Ru3+ solution for Pd-Ru NPs formation. Pd-Ru NPs synthesized mainly at the cell surface, although small MNPs were also present intracellularly. (Gomez-Bolivar et al., 2019a). Table 3 summarize examples of bimetallic nanoparticles produced by different bacteria.
4 Discussion—challenges for potential industrial-scale synthesis
There seems to be an enormous potential for a cost-effective and environmentally friendly synthesis of metal nanoparticles by bacteria. Yet, some special considerations must be assessed before the implementation of these approaches on a large scale and for commercial applications. The most common disadvantages of nanoparticle synthesis using microorganisms is the polydispersity of the particles, low yield and agglomeration (Narayanan and Sakthivel, 2010). Many bacterial strains that enable high yields of NPs are extremophilic organisms that require harsh environmental conditions in terms of temperature, radiation, pH and medium composition (metal concentration, oxygen, salinity, etc.,) in order to thrive (Atalah et al., 2022). The complicated culture conditions that are necessary for their isolation and cultivation, and the fact that small modifications of the environment can immediately result in the disruption of the metabolic routes that promote the metal ion reduction, make them challenging to use in large-scale processes. An example is the effect of dissolved oxygen concentration (DO) on the yield of magnetosomes produced by magnetotactic bacteria. It was suggested that M. gryphiswaldense MSR-1 bacteria form magnetosomes as a response to a low DO that is detrimental for their growth. Thus, only a strict control of the DO during production would guarantee higher yields of magnetosomes (Sun et al., 2008). This illustrates that optimized conditions for bacterial growth and nanoparticle formation must be determined in detail before any large-scale synthesis is considered, and that it is crucial to evaluate how controllable these parameters are on larger scales. Usually, large-scale cultivation requires further refinement of nutrients control and other culture conditions (Liu et al., 2010). In addition, the cost of specific culture media for microbial growth, instrumentation and facilities needs to be considered in potential commercial productions (Capuzzo, 2021).
By tuning the metal concentration and cell density, it is possible to obtain well-defined nanoparticles with narrow size distribution and homogeneous morphology using bacteria (Bing et al., 2018; Yu et al., 2020). The development of efficient mechanisms to supervise and adjust these parameters, in combination with choosing the ideal bacterial species and strains, would be important for an effective large-scale synthesis. Microbial MNP synthesis can occur intracellularly or extracellularly depending on the reduction mechanism used by the selected organism. Most studies favor the extracellular, cell lysate or cell-free production, as the resulting nanoparticles require less complex downstream processing. Intracellular synthesis tends to require a further purification processes to release nanoparticles from the cytosol, and ultrasound treatment to reduce agglomeration (Marooufpour et al., 2019). In addition, the biosynthesized NPs frequently use biological material as nucleation sites and scaffolding. Therefore, many of them feature biocappings that may have desired or undesired effects on the nanoparticle properties (Schröfel et al., 2014). Some of the capping agents ensure the production of small homogeneous nanoparticles by preventing the agglomeration and by decreasing their toxicity (Yahyaei et al., 2016). However, a removal process for these biocoatings from the nanoparticles is sometimes necessary depending on the targeted application. Excess proteins, ligands and impurities hinder the catalytic performance of MNPs like Ag NPs, and protocols such as dialysis, filtration, and sucrose density centrifugation have been introduced as purification methods (Khositanon et al., 2020; Ahmad et al., 2021). A study by Khositanon et al., reported the removal of up to 73.3% of ligands by continuous-flow solvent extraction in a production of Ag NPs coated with surfactants (Khositanon et al., 2020). Yet, most of the methods to clean the bio-nanoparticles have proven to be time consuming, laborious, or not cost-effective, which may prevent the profitable use of the particles for their intended purposes.
Because of these technical hurdles, only few examples have been reported for the successful, large-scale production of NPs, showing that further research is necessary to develop sustainable production processes (Gahlawat and Choudhury, 2019). Successful examples include the productions of ZnS NPs by the thermophilic bacterium Thermoanaerobacter sp. X513 in 100-L and 900-L fermenters (Moon et al., 2016), and CdS NPs in cultures from 10 ml up to 24 L of volume (Moon et al., 2013). Magnetic, Fe-based NPs were synthesized by Thermoanaerobacter sp. TOR-39 in 35 L reactors (Moon et al., 2010).
The key parameters that have a significant effect on NP yield are time of incubation, biomass concentration, and type of precursor, aside from the choice of bacterial species. In principle, these parameters are relatively easy to control, and along with further understanding of the bacterial redox activity and the mechanism of metal transport would greatly benefit the development of strains that naturally have a high tolerance for heavy metals and remarkably produce large populations of nanoparticles. Then, the insights gained in numerous studies give hope that industrial-scale protocols can be implemented for commercial applications in the future (Narayanan and Sakthivel, 2010).
Author contributions
AC and DL contributed to conception of the manuscript. AC wrote the first draft and AS wrote sections of the manuscript. DL, AS, and PM conducted manuscript editing and proofreading. All authors contributed to the article and approved the submitted version.
Funding
This work is part of the Project “BEDPAN—Bio-Engineered Palladium Nanoparticles,” funded by the Research Council of Norway, RCN294605.
Conflict of interest
The authors declare that the research was conducted in the absence of any commercial or financial relationships that could be construed as a potential conflict of interest.
Publisher’s note
All claims expressed in this article are solely those of the authors and do not necessarily represent those of their affiliated organizations, or those of the publisher, the editors and the reviewers. Any product that may be evaluated in this article, or claim that may be made by its manufacturer, is not guaranteed or endorsed by the publisher.
References
Abishad, P., Vergis, J., Unni, V., Ram, V. P., Niveditha, P., Yasur, J., et al. (2022). Green synthesized silver nanoparticles using Lactobacillus acidophilus as an antioxidant, antimicrobial, and antibiofilm agent against multi-drug resistant enteroaggregative Escherichia coli. Probiotics Antimicrob. Proteins 14, 904–914. doi:10.1007/s12602-022-09961-1
Ahmad, B., Shireen, F., Rauf, A., Shariati, M. A., Bashir, S., Patel, S., et al. (2021). Phyto-fabrication, purification, characterisation, optimisation, and biological competence of nano-silver. IET Nanobiotechnology 15, 1–18. doi:10.1049/nbt2.12007
Ahmad, F., Ashraf, N., Ashraf, T., Zhou, R-B., and Yin, D-C. (2019). Biological synthesis of metallic nanoparticles (MNPs) by plants and microbes: Their cellular uptake, biocompatibility, and biomedical applications. Appl. Microbiol. Biotechnol. 103, 2913–2935. doi:10.1007/s00253-019-09675-5
Al-Kordy, H. M. H., Sabry, S. A., and Mabrouk, M. E. M. (2021). Statistical optimization of experimental parameters for extracellular synthesis of zinc oxide nanoparticles by a novel haloalaliphilic Alkalibacillus sp.W7. Sci. Rep. 11, 10924. doi:10.1038/s41598-021-90408-y
Alphandéry, E. (2014). Applications of magnetosomes synthesized by magnetotactic bacteria in medicine. Front. Bioeng. Biotechnol. 2, 5. doi:10.3389/fbioe.2014.00005
Alphandéry, E., Faure, S., Seksek, O., Guyot, F., and Chebbi, I. (2011). Chains of magnetosomes extracted from AMB-1 magnetotactic bacteria for application in alternative magnetic field cancer therapy. ACS Nano 5, 6279–6296. doi:10.1021/nn201290k
Andrade Neto, N. F., Nascimento, L. E., Correa, M., Bohn, F., Bomio, M. R. D., and Motta, F. V. (2020). Characterization and photocatalytic application of Ce4+, Co2+, Mn2+ and Ni2+ doped Fe3O4 magnetic nanoparticles obtained by the co-precipitation method. Mater. Chem. Phys. 242, 122489. doi:10.1016/j.matchemphys.2019.122489
Andrei, A., Öztürk, Y., Khalfaoui-Hassani, B., Rauch, J., Marckmann, D., Trasnea, P-I., et al. (2020). Cu homeostasis in bacteria: The ins and outs. Membranes 10, 242. doi:10.3390/membranes10090242
Atalah, J., Espina, G., Blamey, L., Muñoz-Ibacache, S. A., and Blamey, J. M. (2022). Advantages of using extremophilic bacteria for the biosynthesis of metallic nanoparticles and its potential for rare earth element recovery. Front. Microbiol. 13, 855077. doi:10.3389/fmicb.2022.855077
Attard, G., Casadesús, M., Macaskie, L. E., and Deplanche, K. (2012). Biosynthesis of platinum nanoparticles by Escherichia coli MC4100: Can such nanoparticles exhibit intrinsic surface enantioselectivity? Langmuir 28, 5267–5274. doi:10.1021/la204495z
Bachar, O., Meirovich, M. M., Kurzion, R., and Yehezkeli, O. (2020). In vivo and in vitro protein mediated synthesis of palladium nanoparticles for hydrogenation reactions. Chem. Commun. 56, 11211–11214. doi:10.1039/D0CC04812G
Bachii, S. A., Sahib, W. H. A-A., and Salah, A. A. R. (2021). Preparation and characterization of silver nanoparticles biosynthesis by Pseudomonas stutzeri environmental bacteria isolated from oil fields and their antimicrobial activity. Sci. J. Med. Res. 5.
Baltazar-Encarnación, E., Escárcega-González, C. E., Vasto-Anzaldo, X. G., Cantú-Cárdenas, M. E., and Morones-Ramírez, J. R. (2019). Silver nanoparticles synthesized through green methods using Escherichia coli top 10 (Ec-Ts) growth culture medium exhibit antimicrobial properties against nongrowing bacterial strains. J. Nanomater. 2019, 1–8. doi:10.1155/2019/4637325
Barton, L. L., and Fauque, G. D. (2009). “Chapter 2 biochemistry, physiology and biotechnology of sulfate-reducing bacteria,” in Advances in applied microbiology (United States: Academic Press), 41–98. doi:10.1016/S0065-2164(09)01202-7
Baskaran, B., Muthukumarasamy, A., Chidambaram, S., Sugumaran, A., Ramachandran, K., and Rasu Manimuthu, T. (2017). Cytotoxic potentials of biologically fabricated platinum nanoparticles from Streptomyces sp. on MCF-7 breast cancer cells. IET Nanobiotechnology 11, 241–246. doi:10.1049/iet-nbt.2016.0040
Beveridge, T. J., and Murray, R. G. E. (1980). Sites of metal deposition in the cell wall of Bacillus subtilis. J. Bacteriol. 141, 876–887. doi:10.1128/jb.141.2.876-887.1980
Bhamidimarri, S. P., Young, T. R., Shanmugam, M., Soderholm, S., Baslé, A., Bumann, D., et al. (2021). Acquisition of ionic copper by the bacterial outer membrane protein OprC through a novel binding site. Stock Am. PLOS Biol. 19, e3001446. doi:10.1371/journal.pbio.3001446
Bing, W., Sun, H., Wang, F., Song, Y., and Ren, J. (2018). Hydrogen-producing hyperthermophilic bacteria synthesized size-controllable fine gold nanoparticles with excellence for eradicating biofilm and antibacterial applications. J. Mater. Chem. B 6, 4602–4609. doi:10.1039/C8TB00549D
Blindauer, C. A. (2011). Bacterial metallothioneins: Past, present, and questions for the future. JBIC J. Biol. Inorg. Chem. 16, 1011–1024. doi:10.1007/s00775-011-0790-y
Bloch, K., Pardesi, K., Satriano, C., and Ghosh, S. (2021). Bacteriogenic platinum nanoparticles for application in nanomedicine. Front. Chem. 9, 624344. doi:10.3389/fchem.2021.624344
Boedicker, J. Q., Gangan, M., Naughton, K., Zhao, F., Gralnick, J. A., and El-Naggar, M. Y. (2021). Engineering biological electron transfer and redox pathways for nanoparticle synthesis. Bioelectricity 3, 126–135. doi:10.1089/bioe.2021.0010
Bogireddy, N. K. R., Kiran Kumar, H. A., and Mandal, B. K. (2016). Biofabricated silver nanoparticles as green catalyst in the degradation of different textile dyes. J. Environ. Chem. Eng. 4, 56–64. doi:10.1016/j.jece.2015.11.004
Bruins, M. R., Kapil, S., and Oehme, F. W. (2000). Microbial resistance to metals in the environment. Ecotoxicol. Environ. Saf. 45, 198–207. doi:10.1006/eesa.1999.1860
Bulgarini, A., Lampis, S., Turner, R. J., and Vallini, G. (2021). Biomolecular composition of capping layer and stability of biogenic selenium nanoparticles synthesized by five bacterial species. Microb. Biotechnol. 14, 198–212. doi:10.1111/1751-7915.13666
Burgos, W. D., McDonough, J. T., Senko, J. M., Zhang, G., Dohnalkova, A. C., Kelly, S. D., et al. (2008). Characterization of uraninite nanoparticles produced by Shewanella oneidensis MR-1. Geochimica Cosmochimica Acta 72, 4901–4915. doi:10.1016/j.gca.2008.07.016
Butterfield, A. G., Steimle, B. C., and Schaak, R. E. (2020). Retrosynthetic design of morphologically complex metal sulfide nanoparticles using sequential partial cation exchange and chemical etching. ACS Mater. Lett. 2, 1106–1114. doi:10.1021/acsmaterialslett.0c00287
Capuzzo, A. M. (2021). Bacterial synthesis of nanoparticles: Current trends in biotechnology and biomedical fields. Ann. Adv. Biomed. Sci. 4. doi:10.23880/aabsc-16000161
Carrasco, V., Amarelle, V., Lagos-Moraga, S., Quezada, C. P., Espinoza-González, R., Faccio, R., et al. (2021). Production of cadmium sulfide quantum dots by the lithobiontic Antarctic strain Pedobacter sp. UYP1 and their application as photosensitizer in solar cells. Microb. Cell Factories 20, 41. doi:10.1186/s12934-021-01531-4
Chan, H-K., and Kwok, P. C. L. (2011). Production methods for nanodrug particles using the bottom-up approach. Adv. Drug Deliv. Rev. 63, 406–416. doi:10.1016/j.addr.2011.03.011
Chen, C-Y., Chang, Y-C., Tsai, T-H., Liu, M-H., and Chung, Y-C. (2021). Multifunctional activities of gold nanoparticles biosynthesized using bacteria isolated from mining areas. Appl. Sci. 11, 3670. doi:10.3390/app11083670
Chen, Y., Chen, Y., Wu, J., and Zhang, J. (2018). The effect of biotic and abiotic environmental factors on Pd(II) adsorption and reduction by Bacillus wiedmannii MSM. Ecotoxicol. Environ. Saf. 162, 546–553. doi:10.1016/j.ecoenv.2018.07.043
Chen, Y., Hu, K., and Chen, Y. (2019). The effect of biotic and abiotic environmental factors on Pd(II) adsorption and reduction by Bacillus megaterium Y-4. Chemosphere 220, 1058–1066. doi:10.1016/j.chemosphere.2019.01.011
Chernyshev, V. M., and Ananikov, V. P. (2022). Nickel and palladium catalysis: Stronger demand than ever. ACS Catal. 12, 1180–1200. doi:10.1021/acscatal.1c04705
Crespo, K. A., Baronetti, J. L., Quinteros, M. A., Páez, P. L., and Paraje, M. G. (2017). Intra- and extracellular biosynthesis and characterization of iron nanoparticles from prokaryotic microorganisms with anticoagulant activity. Pharm. Res. 34, 591–598. doi:10.1007/s11095-016-2084-0
Cypriano, J., Castro, J., Taveira, I., Correa, T., Acosta-Avalos, D., Abreu, F., et al. (2022). Magnetosome biomineralization by magnetotactic bacteria. Cham: Springer, 243–281. doi:10.1007/978-3-030-80807-5_7
Das, C. G. A., Kumar, V. G., Dhas, T. S., Karthick, V., Govindaraju, K., Joselin, J. M., et al. (2020). Antibacterial activity of silver nanoparticles (biosynthesis): A short review on recent advances. Biocatal. Agric. Biotechnol. 27, 101593. doi:10.1016/j.bcab.2020.101593
Dash, S. R., Bag, S. S., and Golder, A. K. (2021). Bio-inspired PtNPs/Graphene nanocomposite based electrocatalytic sensing of metabolites of dipyrone. Anal. Chim. Acta 1167, 338562. doi:10.1016/j.aca.2021.338562
De Corte, S., Hennebel, T., Fitts, J. P., Sabbe, T., Bliznuk, V., Verschuere, S., et al. (2011). Biosupported bimetallic Pd–Au nanocatalysts for dechlorination of environmental contaminants. Environ. Sci. Technol. 45, 8506–8513. doi:10.1021/es2019324
de França Bettencourt, G. M., Degenhardt, J., Zevallos Torres, L. A., de Andrade Tanobe, V. O., and Soccol, C. R. (2020). Green biosynthesis of single and bimetallic nanoparticles of iron and manganese using bacterial auxin complex to act as plant bio-fertilizer. Biocatal. Agric. Biotechnol. 30, 101822. doi:10.1016/j.bcab.2020.101822
de Jesus, R. A., de Assis, G. C., de Oliveira, R. J., Costa, J. A. S., da Silva, C. M. P., Bilal, M., et al. (2021). Environmental remediation potentialities of metal and metal oxide nanoparticles: Mechanistic biosynthesis, influencing factors, and application standpoint. Environ. Technol. Innovation 24, 101851. doi:10.1016/j.eti.2021.101851
Delmar, J. A., Su, C-C., and Yu, E. W. (2014). Bacterial multidrug efflux transporters. Annu. Rev. Biophysics 43, 93–117. doi:10.1146/annurev-biophys-051013-022855
Deplanche, K., Caldelari, I., Mikheenko, I. P., Sargent, F., and Macaskie, L. E. (2010). Involvement of hydrogenases in the formation of highly catalytic Pd(0) nanoparticles by bioreduction of Pd(II) using Escherichia coli mutant strains. Microbiology 156, 2630–2640. doi:10.1099/mic.0.036681-0
Deplanche, K., Merroun, M. L., Casadesus, M., Tran, D. T., Mikheenko, I. P., Bennett, J. A., et al. (2012). Microbial synthesis of core/shell gold/palladium nanoparticles for applications in green chemistry. J. R. Soc. Interface 9, 1705–1712. doi:10.1098/rsif.2012.0003
Desai, M. P., Patil, R. V., Harke, S. S., and Pawar, K. D. (2021). Bacterium mediated facile and green method for optimized biosynthesis of gold nanoparticles for simple and visual detection of two metal ions. J. Clust. Sci. 32, 341–350. doi:10.1007/s10876-020-01793-9
Desai, M. P., Patil, R. V., and Pawar, K. D. (2020). Selective and sensitive colorimetric detection of platinum using Pseudomonas stutzeri mediated optimally synthesized antibacterial silver nanoparticles. Biotechnol. Rep. 25, e00404. doi:10.1016/j.btre.2019.e00404
Dong, G., Wang, Y., Gong, L., Wang, M., Wang, H., He, N., et al. (2013). Formation of soluble Cr(III) end-products and nanoparticles during Cr(VI) reduction by Bacillus cereus strain XMCr-6. Biochem. Eng. J. 70, 166–172. doi:10.1016/j.bej.2012.11.002
Dulay, H., Tabares, M., Kashefi, K., and Reguera, G. (2020). Cobalt resistance via detoxification and mineralization in the iron-reducing bacterium geobacter sulfurreducens. Front. Microbiol. 11, 600463. doi:10.3389/fmicb.2020.600463
Dziuba, M., Riese, C. N., Borgert, L., Wittchen, M., Busche, T., Kalinowski, J., et al. (2021). The complex transcriptional landscape of magnetosome gene clusters in Magnetospirillum gryphiswaldense. mSystems 6, e0089321–e0089914. doi:10.1128/mSystems.00893-21
Ebadi, M., Zolfaghari, M. R., Aghaei, S. S., Zargar, M., Shafiei, M., Zahiri, H. S., et al. (2019). A bio-inspired strategy for the synthesis of zinc oxide nanoparticles (ZnO NPs) using the cell extract of cyanobacterium nostoc sp. EA03: From biological function to toxicity evaluation. RSC Adv. 9, 23508–23525. doi:10.1039/C9RA03962G
El-Saadony, M. T., Abd El-Hack, M. E., Taha, A. E., Fouda, M. M. G., Ajarem, J. S., Maodaa S, N., et al. (2020). Ecofriendly synthesis and insecticidal application of copper nanoparticles against the storage pest Tribolium castaneum. Nanomaterials 10, 587. doi:10.3390/nano10030587
Eltarahony, M., Zaki, S., ElKady, M., and Abd-El-Haleem, D. (2018). Biosynthesis, characterization of some combined nanoparticles, and its biocide potency against a broad spectrum of pathogens. J. Nanomater. 2018, 1–16. doi:10.1155/2018/5263814
Eramabadi, P., Masoudi, M., Makhdoumi, A., and Mashreghi, M. (2020). Microbial cell lysate supernatant (CLS) alteration impact on platinum nanoparticles fabrication, characterization, antioxidant and antibacterial activity. Mater. Sci. Eng. C 117, 111292. doi:10.1016/j.msec.2020.111292
Esmail, R., Afshar, A., Morteza, M., Abolfazl, A., and Akhondi, E. (2022). Synthesis of silver nanoparticles with high efficiency and stability by culture supernatant of Bacillus ROM6 isolated from Zarshouran gold mine and evaluating its antibacterial effects. BMC Microbiol. 22, 97. doi:10.1186/s12866-022-02490-5
Fatemi, M., Mollania, N., Momeni-Moghaddam, M., and Sadeghifar, F. (2018). Extracellular biosynthesis of magnetic iron oxide nanoparticles by Bacillus cereus strain HMH1: Characterization and in vitro cytotoxicity analysis on MCF-7 and 3T3 cell lines. J. Biotechnol. 270, 1–11. doi:10.1016/j.jbiotec.2018.01.021
Fatoki, O. S. (1997). Biomethylation in the natural environment: A review. South Afr. J. Sci. 1997, 366–370.
Fu, X., Cai, J., Zhang, X., Li, W-D. D., Ge, H., and Hu, Y. (2018). Top-down fabrication of shape-controlled, monodisperse nanoparticles for biomedical applications. Adv. Drug Deliv. Rev. 132, 169–187. doi:10.1016/j.addr.2018.07.006
Gahlawat, G., and Choudhury, A. R. (2019). A review on the biosynthesis of metal and metal salt nanoparticles by microbes. RSC Adv. 9, 12944–12967. doi:10.1039/C8RA10483B
Gaidhani, S. V., Yeshvekar, R. K., Shedbalkar, U. U., Bellare, J. H., and Chopade, B. A. (2014). Bio-reduction of hexachloroplatinic acid to platinum nanoparticles employing Acinetobacter calcoaceticus. Process Biochem. 49, 2313–2319. doi:10.1016/j.procbio.2014.10.002
Gao, C., Lyu, F., and Yin, Y. (2021). Encapsulated metal nanoparticles for catalysis. Chem. Rev. 121, 834–881. doi:10.1021/acs.chemrev.0c00237
Gareev, K. G., Grouzdev, D. S., Kharitonskii, P. V., Kosterov, A., Koziaeva, V. V., Sergienko, E. S., et al. (2021). Magnetotactic bacteria and magnetosomes: Basic properties and applications. Magnetochemistry 7, 86. doi:10.3390/magnetochemistry7060086
Gautam, M., Kim, J. O., and Yong, C. S. (2021). Fabrication of aerosol-based nanoparticles and their applications in biomedical fields. J. Pharm. Investigation 51, 361–375. doi:10.1007/s40005-021-00523-1
Gomez-Bolivar, J., Mikheenko, I. P., Macaskie, L. E., and Merroun, M. L. (2019). Characterization of palladium nanoparticles produced by healthy and microwave-injured cells of Desulfovibrio desulfuricans and Escherichia coli. Nanomaterials 9, 857. doi:10.3390/nano9060857
Gomez-Bolivar, J., Mikheenko, I. P., Orozco, R. L., Sharma, S., Banerjee, D., Walker, M., et al. (2019). Synthesis of Pd/Ru bimetallic nanoparticles by Escherichia coli and potential as a catalyst for upgrading 5-hydroxymethyl furfural into liquid fuel precursors. Front. Microbiol. 10, 1276–1317. doi:10.3389/fmicb.2019.01276
Gopinath, V., Priyadarshini, S., Loke, M. F., Arunkumar, J., Marsili, E., MubarakAli, D., et al. (2017). Biogenic synthesis, characterization of antibacterial silver nanoparticles and its cell cytotoxicity. Arabian J. Chem. 10, 1107–1117. doi:10.1016/j.arabjc.2015.11.011
Goswami, P., He, K., Li, J., Pan, Y., Roberts, A. P., and Lin, W. (2022). Magnetotactic bacteria and magnetofossils: Ecology, evolution and environmental implications. npj Biofilms Microbiomes 8, 43. doi:10.1038/s41522-022-00304-0
Govindaraju, K., Basha, S. K., Kumar, V. G., and Singaravelu, G. (2008). Silver, gold and bimetallic nanoparticles production using single-cell protein (Spirulina platensis) Geitler. J. Mater. Sci. 43, 5115–5122. doi:10.1007/s10853-008-2745-4
Griffin, S., Masood, M., Nasim, M., Sarfraz, M., Ebokaiwe, A., Schäfer, K-H., et al. (2017). Natural nanoparticles: A particular matter inspired by nature. Antioxidants 7, 3. doi:10.3390/antiox7010003
Gwisai, T., Mirkhani, N., Christiansen, M. G., Nguyen, T. T., Ling, V., and Schuerle, S. (2022). Magnetic torque–driven living microrobots for increased tumor infiltration. Sci. Robotics 7, eabo0665. doi:10.1126/scirobotics.abo0665
Hafeez, M., Shaheen, R., Akram, B., Zain-ul-Abdin, , Haq, S., Mahsud, S., et al. (2020). Green synthesis of cobalt oxide nanoparticles for potential biological applications. Mater. Res. Express 7, 025019. doi:10.1088/2053-1591/ab70dd
W. M. Haynes (Editor) (2014). CRC handbook of chemistry and physics (United States: CRC Press). doi:10.1201/b17118
Henglein, A. (1999). Radiolytic preparation of ultrafine colloidal gold particles in aqueous solution: Optical spectrum, controlled growth, and some chemical reactions. Langmuir 15, 6738–6744. doi:10.1021/la9901579
Herrmann, G., Jayamani, E., Mai, G., and Buckel, W. (2008). Energy conservation via electron-transferring flavoprotein in anaerobic bacteria. J. Bacteriol. 190, 784–791. doi:10.1128/JB.01422-07
Hood, M. I., and Skaar, E. P. (2012). Nutritional immunity: Transition metals at the pathogen–host interface. Nat. Rev. Microbiol. 10, 525–537. doi:10.1038/nrmicro2836
Hosny, M., Fawzy, M., El-Fakharany, E. M., Omer, A. M., El-Monaem, E. M. A., Khalifa, R. E., et al. (2022). Biogenic synthesis, characterization, antimicrobial, antioxidant, antidiabetic, and catalytic applications of platinum nanoparticles synthesized from Polygonum salicifolium leaves. J. Environ. Chem. Eng. 10, 106806. doi:10.1016/j.jece.2021.106806
Hosseinkhani, B., Søbjerg, L. S., Rotaru, A-E., Emtiazi, G., Skrydstrup, T., and Meyer, R. L. (2012). Microbially supported synthesis of catalytically active bimetallic Pd-Au nanoparticles. Biotechnol. Bioeng. 109, 45–52. doi:10.1002/bit.23293
Hsu, C-M., Huang, Y-H., Chen, H-J., Lee, W-C., Chiu, H-W., Maity, J. P., et al. (2018). Green synthesis of nano-Co3O4 by Microbial Induced Precipitation (MIP) process using Bacillus pasteurii and its application as supercapacitor. Mater. Today Commun. 14, 302–311. doi:10.1016/j.mtcomm.2018.02.005
Huq, M. A., and Akter, S. (2021). Bacterial mediated rapid and facile synthesis of silver nanoparticles and their antimicrobial efficacy against pathogenic microorganisms. Materials 14, 2615. doi:10.3390/ma14102615
Hynninen, A. (2010). Zinc, cadmium and lead resistance mechanisms in bacteria and their contribution to biosensing. Finland: University of Helsinki.
Ibrahim, S., Ahmad, Z., Manzoor, M. Z., Mujahid, M., Faheem, Z., and Adnan, A. (2021). Optimization for biogenic microbial synthesis of silver nanoparticles through response surface methodology, characterization, their antimicrobial, antioxidant, and catalytic potential. Sci. Rep. 11, 770. doi:10.1038/s41598-020-80805-0
Igiri, B. E., Okoduwa, S. I. R., Idoko, G. O., Akabuogu, E. P., Adeyi, A. O., and Ejiogu, I. K. (2018). Toxicity and bioremediation of heavy metals contaminated ecosystem from tannery wastewater: A review. J. Toxicol. 2018, 1–16. doi:10.1155/2018/2568038
Iravani, S. (2014). Bacteria in nanoparticle synthesis: Current status and future prospects. Int. Sch. Res. Notices 2014, 1–18. doi:10.1155/2014/359316
Iravani, S., and Varma, R. S. (2020). Bacteria in heavy metal remediation and nanoparticle biosynthesis. ACS Sustain. Chem. Eng. 8, 5395–5409. doi:10.1021/acssuschemeng.0c00292
Jaidev, L. R., and Narasimha, G. (2010). Fungal mediated biosynthesis of silver nanoparticles, characterization and antimicrobial activity. Colloids Surfaces B Biointerfaces. 81, 430–433. doi:10.1016/j.colsurfb.2010.07.033
Jain, A. S., Pawar, P. S., Sarkar, A., Junnuthula, V., and Dyawanapelly, S. (2021). Bionanofactories for green synthesis of silver nanoparticles: Toward antimicrobial applications. Int. J. Mol. Sci. 22, 11993. doi:10.3390/ijms222111993
Jamkhande, P. G., Ghule, N. W., Bamer, A. H., and Kalaskar, M. G. (2019). Metal nanoparticles synthesis: An overview on methods of preparation, advantages and disadvantages, and applications. J. Drug Deliv. Sci. Technol. 53, 101174. doi:10.1016/j.jddst.2019.101174
Jang, E., Shim, H-W., Ryu, B. H., An, D. R., Yoo, W. K., Kim, K. K., et al. (2015). Preparation of cobalt nanoparticles from polymorphic bacterial templates: A novel platform for biocatalysis. Int. J. Biol. Macromol. 81, 747–753. doi:10.1016/j.ijbiomac.2015.09.009
Javed, Y., Akhtar, K., Anwar, H., and Jamil, Y. (2017). MRI based on iron oxide nanoparticles contrast agents: Effect of oxidation state and architecture. J. Nanoparticle Res. 19:366. doi:10.1007/S11051-017-4045-X
Jimenez-Sandoval, R., Pedireddy, S., Katuri, K. P., and Saikaly, P. E. (2023). Facile biological-based synthesis of size-controlled palladium nanoclusters anchored on the surface of geobacter sulfurreducens and their application in electrocatalysis. ACS Sustain. Chem. Eng. 11, 1100–1109. doi:10.1021/acssuschemeng.2c06143
Johnstone, T. C., and Nolan, E. M. (2015). Beyond iron: Non-classical biological functions of bacterial siderophores. Dalton Trans. 44, 6320–6339. doi:10.1039/c4dt03559c
Kalimuthu, K., Suresh Babu, R., Venkataraman, D., Bilal, M., and Gurunathan, S. (2008). Biosynthesis of silver nanocrystals by Bacillus licheniformis. Colloids Surfaces B Biointerfaces. 65, 150–153. doi:10.1016/j.colsurfb.2008.02.018
Kanakalakshmi, A., Janaki, V., Shanthi, K., and Kamala-Kannan, S. (2017). Biosynthesis of Cr(III) nanoparticles from electroplating wastewater using chromium-resistant Bacillus subtilis and its cytotoxicity and antibacterial activity. Artif. Cells, Nanomedicine, Biotechnol. 45, 1304–1309. doi:10.1080/21691401.2016.1228660
Katsui, H., and Goto, T. (2021). Chemical vapor deposition. Multi-Dimensional additive manufacturing. Singapore: Springer Singapore, 75–95. doi:10.1007/978-981-15-7910-3_6
Khalil, A. T., Ovais, M., Iqbal, J., Ali, A., Ayaz, M., Abbas, M., et al. (2022). Microbes-mediated synthesis strategies of metal nanoparticles and their potential role in cancer therapeutics. Seminars Cancer Biol. 86, 693–705. doi:10.1016/j.semcancer.2021.06.006
Khan, M., Ijaz, M., Chotana, G. A., Murtaza, G., Malik, A., and Shamim, S. (2022). Bacillus altitudinis MT422188: A potential agent for zinc bioremediation. Bioremediation J. 26, 228–248. doi:10.1080/10889868.2021.1927973
Khan, S., Akhtar, N., Rehman, S. U., Shujah, S., Rha, E. S., and Jamil, M. (2020). Biosynthesized iron oxide nanoparticles (Fe3O4 NPs) mitigate arsenic toxicity in rice seedlings. Toxics 9, 2. doi:10.3390/toxics9010002
Khositanon, C., Adpakpang, K., Bureekaew, S., and Weeranoppanant, N. (2020). Continuous-flow purification of silver nanoparticles and its integration with flow synthesis. J. Flow Chem. 10, 353–362. doi:10.1007/s41981-020-00084-8
Kianpour, S., Ebrahiminezhad, A., Deyhimi, M., Negahdaripour, M., Raee, M. J., Mohkam, M., et al. (2019). Structural characterization of polysaccharide-coated iron oxide nanoparticles produced by Staphylococcus warneri, isolated from a thermal spring. J. Basic Microbiol. 59, 569–578. doi:10.1002/jobm.201800684
Kikuchi, F., Kato, Y., Furihata, K., Kogure, T., Imura, Y., Yoshimura, E., et al. (2016). Formation of gold nanoparticles by glycolipids of Lactobacillus casei. Sci. Rep. 6, 34626. doi:10.1038/srep34626
Kim, E-H., Nies, D. H., McEvoy, M. M., and Rensing, C. (2011). Switch or funnel: How RND-type transport systems control periplasmic metal homeostasis. J. Bacteriol. 193, 2381–2387. doi:10.1128/JB.01323-10
Kimber, R. L., Parmeggiani, F., Neill, T. S., Merroun, M. L., Goodlet, G., Powell, N. A., et al. (2021). Biotechnological synthesis of Pd/Ag and Pd/Au nanoparticles for enhanced Suzuki–Miyaura cross-coupling activity. Microb. Biotechnol. 14, 2435–2447. doi:10.1111/1751-7915.13762
Klaus, T., Joerger, R., Olsson, E., and Granqvist, C-G. (1999). Silver-based crystalline nanoparticles, microbially fabricated. Proc. Natl. Acad. Sci. 96, 13611–13614. doi:10.1073/pnas.96.24.13611
Komeda, H., Kobayashi, M., and Shimizu, S. (1997). A novel transporter involved in cobalt uptake. Proc. Natl. Acad. Sci. 94, 36–41. doi:10.1073/pnas.94.1.36
Kosolapov, D. B., Kuschk, P., Vainshtein, M. B., Vatsourina, A. V., Wießner, A., Kästner, M., et al. (2004). Microbial processes of heavy metal removal from carbon-deficient effluents in constructed wetlands. Eng. Life Sci. 4, 403–411. doi:10.1002/elsc.200420048
Krebsz, M., Kótai, L., Sajó, I. E., Váczi, T., and Pasinszki, T. (2021). Carbon microsphere-supported metallic nickel nanoparticles as novel heterogeneous catalysts and their application for the reduction of nitrophenol. Molecules 26, 5680. doi:10.3390/molecules26185680
Krewulak, K. D., and Vogel, H. J. (2008). Structural biology of bacterial iron uptake. Biochimica Biophysica Acta (BBA) - Biomembr. 1778, 1781–1804. doi:10.1016/j.bbamem.2007.07.026
Kröger, A., Geisler, V., Lemma, E., Theis, F., and Lenger, R. (1992). Bacterial fumarate respiration. Archives Microbiol. 158, 311–314. doi:10.1007/BF00245358
Kumar, U., Shete, A., Harle, A. S., Kasyutich, O., Schwarzacher, W., Pundle, A., et al. (2008). Extracellular bacterial synthesis of protein-functionalized ferromagnetic Co 3 O 4 nanocrystals and imaging of self-organization of bacterial cells under stress after exposure to metal ions. Chem. Mater. 20, 1484–1491. doi:10.1021/cm702727x
Lau, C. K. Y., Krewulak, K. D., and Vogel, H. J. (2016). Bacterial ferrous iron transport: The Feo system. FEMS Microbiol. Rev. 40, 273–298. doi:10.1093/femsre/fuv049
Le Nagard, L., Morillo-López, V., Fradin, C., and Bazylinski, D. A. (2018). Growing magnetotactic bacteria of the genus Magnetospirillum: Strains MSR-1, AMB-1 and MS-1. J. Vis. Exp. 2018, 58536. doi:10.3791/58536
Lengke, M. F., Fleet, M. E., and Southam, G. (2006). Synthesis of platinum nanoparticles by reaction of filamentous cyanobacteria with platinum(IV)−Chloride complex. Langmuir 22, 7318–7323. doi:10.1021/la060873s
Li, J., Tian, B., Li, T., Dai, S., Weng, Y., Lu, J., et al. (2018). Biosynthesis of Au, Ag and Au&ndash;Ag bimetallic nanoparticles using protein extracts of <em>Deinococcus radiodurans</em> and evaluation of their cytotoxicity. Int. J. Nanomedicine 13, 1411–1424. doi:10.2147/IJN.S149079
Li, Q., Liu, F., Li, M., Chen, C., and Gadd, G. M. (2022). Nanoparticle and nanomineral production by fungi. Fungal Biol. Rev. 41, 31–44. doi:10.1016/j.fbr.2021.07.003
Li, S-N., Wang, R., and Ho, S-H. (2021). Algae-mediated biosystems for metallic nanoparticle production: From synthetic mechanisms to aquatic environmental applications. J. Hazard. Mater. 420, 126625. doi:10.1016/j.jhazmat.2021.126625
Li, X. Z., Nikaido, H., and Williams, K. E. (1997). Silver-resistant mutants of Escherichia coli display active efflux of Ag+ and are deficient in porins. J. Bacteriol. 179, 6127–6132. doi:10.1128/jb.179.19.6127-6132.1997
Lin, I. W-S., Lok, C-N., and Che, C-M. (2014). Biosynthesis of silver nanoparticles from silver(i) reduction by the periplasmic nitrate reductase c-type cytochrome subunit NapC in a silver-resistant E. coli. Chem. Sci. 5, 3144–3150. doi:10.1039/C4SC00138A
Liu, Y., Li, G. R., Guo, F. F., Jiang, W., Li, Y., and Li, L. J. (2010). Large-scale production of magnetosomes by chemostat culture of Magnetospirillum gryphiswaldense at high cell density. Microb. Cell Factories 9, 99. doi:10.1186/1475-2859-9-99
Lloyd, J. R. (2003). Microbial reduction of metals and radionuclides. FEMS Microbiol. Rev. 27, 411–425. doi:10.1016/S0168-6445(03)00044-5
Lovley, D. R. (1993). Dissimilatory metal reduction. Annu. Rev. Microbiol. 47, 263–290. doi:10.1146/annurev.mi.47.100193.001403
Lovley, D. R., Giovannoni, S. J., White, D. C., Champine, J. E., Phillips, E. J. P., Gorby, Y. A., et al. (1993). Geobacter metallireducens gen. nov. sp. nov., a microorganism capable of coupling the complete oxidation of organic compounds to the reduction of iron and other metals. Archives Microbiol. 159, 336–344. doi:10.1007/BF00290916
Lozhkomoev, A. S., Pervikov, A. V., Kazantsev, S. O., Sharipova, A. F., Rodkevich, N. G., Toropkov, N. E., et al. (2021). Synthesis of Fe/Fe3O4 core-shell nanoparticles by electrical explosion of the iron wire in an oxygen-containing atmosphere. J. Nanoparticle Res. 23, 73. doi:10.1007/s11051-021-05180-x
Lusa, M., Lehto, J., and Bomberg, M. (2016). The uptake of Ni<sup>2+</sup> and Ag<sup>+</sup> by bacterial strains isolated from a boreal nutrient-poor bog. AIMS Microbiol. 2, 120–137. doi:10.3934/microbiol.2016.2.120
Lutkenhaus, J. F. (1977). Role of a major outer membrane protein in Escherichia coli. J. Bacteriol. 131, 631–637. doi:10.1128/jb.131.2.631-637.1977
Ma, Z., Jacobsen, F. E., and Giedroc, D. P. (2009). Coordination chemistry of bacterial metal transport and sensing. Chem. Rev. 109, 4644–4681. doi:10.1021/cr900077w
Mahdi, Z. S., Talebnia Roshan, F., Nikzad, M., and Ezoji, H. (2020). Biosynthesis of zinc oxide nanoparticles using bacteria: A study on the characterization and application for electrochemical determination of bisphenol A. Inorg. Nano-Metal Chem. 51, 1–9. doi:10.1080/24701556.2020.1835962
Majeed, S., Danish, M., Mohamad Ibrahim, M. N., Sekeri, S. H., Ansari, M. T., Nanda, A., et al. (2021). Bacteria mediated synthesis of iron oxide nanoparticles and their antibacterial, antioxidant, cytocompatibility properties. J. Clust. Sci. 32, 1083–1094. doi:10.1007/s10876-020-01876-7
Makela, A. V., Schott, M. A., Madsen, C. S., Greeson, E. M., and Contag, C. H. (2022). Magnetic particle imaging of magnetotactic bacteria as living contrast agents is improved by altering magnetosome arrangement. Nano Lett. 22, 4630–4639. doi:10.1021/acs.nanolett.1c05042
Mangaiyarkarasi, R., Priyanga, M., Santhiya, N., and Umadevi, S. (2020). In situ preparation of palladium nanoparticles in ionic liquid crystal microemulsion and their application in Heck reaction. J. Mol. Liq. 310, 113241. doi:10.1016/j.molliq.2020.113241
Marimuthu, S., Rahuman, A. A., Kirthi, A. V., Santhoshkumar, T., Jayaseelan, C., and Rajakumar, G. (2013). Eco-friendly microbial route to synthesize cobalt nanoparticles using Bacillus thuringiensis against malaria and dengue vectors. Parasitol. Res. 112, 4105–4112. doi:10.1007/s00436-013-3601-2
Marooufpour, N., Alizadeh, M., Hatami, M., and Asgari Lajayer, B. (2019). “Biological synthesis of nanoparticles by different groups of bacteria,” in Microbial nanobionics (Berlin, Germany: Springer), 63–85. doi:10.1007/978-3-030-16383-9_3
Matsena, M. T., and Chirwa, E. M. N. (2021). Comparative analysis of biological versus chemical synthesis of palladium nanoparticles for catalysis of chromium (VI) reduction. Sci. Rep. 11, 16674. doi:10.1038/s41598-021-96024-0
Matsena, M. T., Tichapondwa, S. M., and Chirwa, E. M. N. (2020). Synthesis of biogenic palladium nanoparticles using Citrobacter sp. for application as anode electrocatalyst in a microbial fuel cell. Catalysts 10, 838. doi:10.3390/catal10080838
Matsumoto, T., Phann, I., and Okibe, N. (2021). Biogenic platinum nanoparticles’ production by extremely acidophilic Fe(III)-Reducing bacteria. Minerals 11, 1175. doi:10.3390/min11111175
Matsunaga, T., Sakaguchi, T., and Tadakoro, F. (1991). Magnetite formation by a magnetic bacterium capable of growing aerobically. Appl. Microbiol. Biotechnol. 35, 651–655. doi:10.1007/BF00169632
Mehta, T., Coppi, M. V., Childers, S. E., and Lovley, D. R. (2005). Outer membrane c -type cytochromes required for Fe(III) and Mn(IV) oxide reduction in geobacter sulfurreducens. Appl. Environ. Microbiol. 71, 8634–8641. doi:10.1128/AEM.71.12.8634-8641.2005
Menazea, A. A. (2020). Femtosecond laser ablation-assisted synthesis of silver nanoparticles in organic and inorganic liquids medium and their antibacterial efficiency. Radiat. Phys. Chem. 168, 108616. doi:10.1016/j.radphyschem.2019.108616
Mikheenko, I. P., Bennett, J. A., Omajali, J. B., Walker, M., Johnson, D. B., Grail, B. M., et al. (2022). Selective hydrogenation catalyst made via heat-processing of biogenic Pd nanoparticles and novel ‘green’ catalyst for Heck coupling using waste sulfidogenic bacteria. Appl. Catal. B Environ. 306, 121059. doi:10.1016/j.apcatb.2021.121059
Mikheenko, I. P., Rousset, M., Dementin, S., and Macaskie, L. E. (2008). Bioaccumulation of palladium by Desulfovibrio fructosivorans wild-type and hydrogenase-deficient strains. Appl. Environ. Microbiol. 74, 6144–6146. doi:10.1128/AEM.02538-07
Monteil, C. L., and Lefevre, C. T. (2020). Magnetoreception in microorganisms. Trends Microbiol. 28, 266–275. doi:10.1016/j.tim.2019.10.012
Moon, J-W., Ivanov, I. N., Duty, C. E., Love, L. J., Rondinone, A. J., Wang, W., et al. (2013). Scalable economic extracellular synthesis of CdS nanostructured particles by a non-pathogenic thermophile. J. Industrial Microbiol. Biotechnol. 40, 1263–1271. doi:10.1007/s10295-013-1321-3
Moon, J-W., Phelps, T. J., Fitzgerald, C. L., Lind, R. F., Elkins, J. G., Jang, G. G., et al. (2016). Manufacturing demonstration of microbially mediated zinc sulfide nanoparticles in pilot-plant scale reactors. Appl. Microbiol. Biotechnol. 100, 7921–7931. doi:10.1007/s00253-016-7556-y
Moon, J-W., Rawn, C. J., Rondinone, A. J., Love, L. J., Roh, Y., Everett, S. M., et al. (2010). Large-scale production of magnetic nanoparticles using bacterial fermentation. J. Industrial Microbiol. Biotechnol. 37, 1023–1031. doi:10.1007/s10295-010-0749-y
Mubraiz, N., Bano, A., Mahmood, T., and Khan, N. (2021). Microbial and plant assisted synthesis of cobalt oxide nanoparticles and their antimicrobial activities. Agronomy 11, 1607. doi:10.3390/agronomy11081607
Mukherjee, K., Gupta, R., Kumar, G., Kumari, S., Biswas, S., and Padmanabhan, P. (2018). Synthesis of silver nanoparticles by Bacillus clausii and computational profiling of nitrate reductase enzyme involved in production. J. Genet. Eng. Biotechnol. 16, 527–536. doi:10.1016/j.jgeb.2018.04.004
Muller, V. (2003). Energy conservation in acetogenic bacteria. Appl. Environ. Microbiol. 69, 6345–6353. doi:10.1128/AEM.69.11.6345-6353.2003
Nair, B., and Pradeep, T. (2002). Coalescence of nanoclusters and formation of submicron crystallites assisted by Lactobacillus strains. Cryst. Growth & Des. 2, 293–298. doi:10.1021/cg0255164
Nan, X., Lai, W., Li, D., Tian, J., Hu, Z., and Fang, Q. (2021). Biocompatibility of bacterial magnetosomes as MRI contrast agent: A long-term in vivo follow-up study. Nanomaterials 11, 1235. doi:10.3390/nano11051235
Nanda, A., and Saravanan, M. (2009). Biosynthesis of silver nanoparticles from Staphylococcus aureus and its antimicrobial activity against MRSA and MRSE. Nanomedicine Nanotechnol. Biol. Med. 5, 452–456. doi:10.1016/j.nano.2009.01.012
Narasaiah, B. P., and Mandal, B. K. (2020). Remediation of azo-dyes based toxicity by agro-waste cotton boll peels mediated palladium nanoparticles. J. Saudi Chem. Soc. 24, 267–281. doi:10.1016/j.jscs.2019.11.003
Narayanan, K. B., and Sakthivel, N. (2010). Biological synthesis of metal nanoparticles by microbes. Adv. Colloid Interface Sci. 156, 1–13. doi:10.1016/j.cis.2010.02.001
Navarro, C., Wu, L-F., and Mandrand-Berthelot, M-A. (1993). The nik operon of Escherichia coli encodes a periplasmic binding-protein-dependent transport system for nickel. Mol. Microbiol. 9, 1181–1191. doi:10.1111/j.1365-2958.1993.tb01247.x
Nies, D. H. (1992). Resistance to cadmium, cobalt, zinc, and nickel in microbes. Plasmid 27, 17–28. doi:10.1016/0147-619X(92)90003-S
Nies, D. H., and Silver, S. (1995). Ion efflux systems involved in bacterial metal resistances. J. Industrial Microbiol. 14, 186–199. doi:10.1007/BF01569902
Noman, M., Shahid, M., Ahmed, T., Niazi, M. B. K., Hussain, S., Song, F., et al. (2020). Use of biogenic copper nanoparticles synthesized from a native Escherichia sp. as photocatalysts for azo dye degradation and treatment of textile effluents. Environ. Pollut. 257, 113514. doi:10.1016/j.envpol.2019.113514
Nomoev, A. V., and Bardakhanov, S. P. (2012). Synthesis and structure of Ag-Si nanoparticles obtained by the electron-beam evaporation/condensation method. Tech. Phys. Lett. 38, 375–378. doi:10.1134/S1063785012040268
Nudelman, H., and Zarivach, R. (2014). Structure prediction of magnetosome-associated proteins. Front. Microbiol. 5, 9. doi:10.3389/fmicb.2014.00009
Omajali, J. B., Gomez-Bolivar, J., Mikheenko, I. P., Sharma, S., Kayode, B., Al-Duri, B., et al. (2019). Novel catalytically active Pd/Ru bimetallic nanoparticles synthesized by Bacillus benzeovorans. Sci. Rep. 9, 4715. doi:10.1038/s41598-019-40312-3
Omajali, J. B., Mikheenko, I. P., Merroun, M. L., Wood, J., and Macaskie, L. E. (2015). Characterization of intracellular palladium nanoparticles synthesized by Desulfovibrio desulfuricans and Bacillus benzeovorans. J. Nanoparticle Res. 17, 264. doi:10.1007/s11051-015-3067-5
Orozco-Montes, V., Caillard, A., Brault, P., Chamorro-Coral, W., Bigarre, J., Sauldubois, A., et al. (2021). Synthesis of platinum nanoparticles by plasma sputtering onto glycerol: Effect of argon pressure on their physicochemical properties. J. Phys. Chem. C 125, 3169–3179. doi:10.1021/acs.jpcc.0c09746
Oves, M., Khan, M. S., Zaidi, A., Ahmed, A. S., Ahmed, F., Ahmad, E., et al. (2013). Antibacterial and cytotoxic efficacy of extracellular silver nanoparticles biofabricated from chromium reducing novel OS4 strain of Stenotrophomonas maltophilia. PLoS ONE 8, e59140. doi:10.1371/journal.pone.0059140
Oves, M., Rauf, M. A., Hussain, A., Qari, H. A., Khan, A. A. P., Muhammad, P., et al. (2019). Antibacterial silver nanomaterial synthesis from mesoflavibacter zeaxanthinifaciens and targeting biofilm formation. Front. Pharmacol. 10, 801. doi:10.3389/fphar.2019.00801
Palmer, L. D., and Skaar, E. P. (2016). Transition metals and virulence in bacteria. Annu. Rev. Genet. 50, 67–91. doi:10.1146/annurev-genet-120215-035146
Parashar, M., Shukla, V. K., and Singh, R. (2020). Metal oxides nanoparticles via sol–gel method: A review on synthesis, characterization and applications. J. Mater. Sci. Mater. Electron. 31, 3729–3749. doi:10.1007/s10854-020-02994-8
Patil, M. P., Kang, M., Niyonizigiye, I., Singh, A., Kim, J-O., Seo, Y. B., et al. (2019). Extracellular synthesis of gold nanoparticles using the marine bacterium Paracoccus haeundaensis BC74171T and evaluation of their antioxidant activity and antiproliferative effect on normal and cancer cell lines. Colloids Surfaces B Biointerfaces. 183, 110455. doi:10.1016/j.colsurfb.2019.110455
Patil, S., Sastry, M., and Bharde, A. (2022). Size and shape directed novel green synthesis of plasmonic nanoparticles using bacterial metabolites and their anticancer effects. Front. Microbiol. 13, 866849. doi:10.3389/fmicb.2022.866849
Pham, V. H. T., Kim, J., Chang, S., and Chung, W. (2022). Bacterial biosorbents, an efficient heavy metals green clean-up strategy: Prospects, challenges, and opportunities. Microorganisms 10, 610. doi:10.3390/microorganisms10030610
Pourali, P., Badiee, S. H., Manafi, S., Noorani, T., Rezaei, A., and Yahyaei, B. (2017). Biosynthesis of gold nanoparticles by two bacterial and fungal strains, Bacillus cereus and Fusarium oxysporum, and assessment and comparison of their nanotoxicity in vitro by direct and indirect assays. Electron. J. Biotechnol. 29, 86–93. doi:10.1016/j.ejbt.2017.07.005
Prasad, S. R., Teli, S. B., Ghosh, J., Prasad, N. R., Shaikh, V. S., Nazeruddin, G. M., et al. (2021). A review on bio-inspired synthesis of silver nanoparticles: Their antimicrobial efficacy and toxicity. Eng. Sci. 2021. doi:10.30919/es8d479
Quintanilla, A., Valvo, M., Lafont, U., Kelder, E. M., Kreutzer, M. T., and Kapteijn, F. (2010). Synthesis of anisotropic gold nanoparticles by electrospraying into a reductive-surfactant solution. Chem. Mater. 22, 1656–1663. doi:10.1021/cm903712y
Quinteros, M. A., Bonilla, J. O., Alborés, S. V., Villegas, L. B., and Páez, P. L. (2019). Biogenic nanoparticles: Synthesis, stability and biocompatibility mediated by proteins of Pseudomonas aeruginosa. Colloids Surfaces B Biointerfaces 184, 110517. doi:10.1016/j.colsurfb.2019.110517
Rajora, N., Kaushik, S., Jyoti, A., and Kothari, S. L. (2016). Rapid synthesis of silver nanoparticles by Pseudomonas stutzeri isolated from textile soil under optimised conditions and evaluation of their antimicrobial and cytotoxicity properties. IET Nanobiotechnology 10, 367–373. doi:10.1049/iet-nbt.2015.0107
Ramos-Ruiz, A., Sesma-Martin, J., Sierra-Alvarez, R., and Field, J. A. (2017). Continuous reduction of tellurite to recoverable tellurium nanoparticles using an upflow anaerobic sludge bed (UASB) reactor. Water Res. 108, 189–196. doi:10.1016/j.watres.2016.10.074
Reichart, O., Szakmár, K., Jozwiak, Á., Felföldi, J., and Baranyai, L. (2007). Redox potential measurement as a rapid method for microbiological testing and its validation for coliform determination. Int. J. Food Microbiol. 114, 143–148. doi:10.1016/j.ijfoodmicro.2006.08.016
Rensing, C., Fan, B., Sharma, R., Mitra, B., and Rosen, B. P. (2000). CopA: An Escherichia coli Cu(I)-translocating P-type ATPase. Proc. Natl. Acad. Sci. 97, 652–656. doi:10.1073/pnas.97.2.652
Restrepo, C. V., and Villa, C. C. (2021). Synthesis of silver nanoparticles, influence of capping agents, and dependence on size and shape: A review. Environ. Nanotechnol. Monit. Manag. 15, 100428. doi:10.1016/j.enmm.2021.100428
Riaz Rajoka, M. S., Mehwish, H. M., Zhang, H., Ashraf, M., Fang, H., Zeng, X., et al. (2020). Antibacterial and antioxidant activity of exopolysaccharide mediated silver nanoparticle synthesized by Lactobacillus brevis isolated from Chinese koumiss. Colloids Surfaces B Biointerfaces. 186, 110734. doi:10.1016/j.colsurfb.2019.110734
Ridley, W. P., Dizikes, L., Cheh, A., and Wood, J. M. (1977). Recent studies on biomethylation and demethylation of toxic elements. Environ. Health Perspect. 19, 43–46. doi:10.1289/ehp.771943
Ridley, W. P., Dizikes, L. J., and Wood, J. M. (1977). Biomethylation of toxic elements in the environment. Science 197, 329–332. doi:10.1126/science.877556
Rouch, D. A., Lee, B. T. O., and Morby, A. P. (1995). Understanding cellular responses to toxic agents: A model for mechanism-choice in bacterial metal resistance. J. Industrial Microbiol. 14, 132–141. doi:10.1007/BF01569895
Samuel, M. S., Datta, S., Chandrasekar, N., Balaji, R., Selvarajan, E., and Vuppala, S. (2021). Biogenic synthesis of iron oxide nanoparticles using Enterococcus faecalis: Adsorption of hexavalent chromium from aqueous solution and in vitro cytotoxicity analysis. Nanomaterials 11, 3290. doi:10.3390/nano11123290
Saravanan, A., Kumar, P. S., Karishma, S., Vo, D-V. N., Jeevanantham, S., Yaashikaa, P. R., et al. (2021). A review on biosynthesis of metal nanoparticles and its environmental applications. Chemosphere 264, 128580. doi:10.1016/j.chemosphere.2020.128580
Saravanan, M., Barik, S. K., MubarakAli, D., Prakash, P., and Pugazhendhi, A. (2018). Synthesis of silver nanoparticles from Bacillus brevis (NCIM 2533) and their antibacterial activity against pathogenic bacteria. Microb. Pathog. 116, 221–226. doi:10.1016/j.micpath.2018.01.038
Sasireka, K. S., and Lalitha, P. (2021). Biogenic synthesis of bimetallic nanoparticles and their applications. Rev. Inorg. Chem. 41, 223–244. doi:10.1515/revic-2020-0024
Sathyavathi, S., Manjula, A., Rajendhran, J., and Gunasekaran, P. (2014). Extracellular synthesis and characterization of nickel oxide nanoparticles from Microbacterium sp. MRS-1 towards bioremediation of nickel electroplating industrial effluent. Bioresour. Technol. 165, 270–273. doi:10.1016/j.biortech.2014.03.031
Schleifer, K. H., Schüler, D., Spring, S., Weizenegger, M., Amann, R., Ludwig, W., et al. (1991). The Genus Magnetospirillum gen. nov. Description of Magnetospirillum gryphiswaldense sp. nov. and Transfer of Aquaspirillum magnetotacticum to Magnetospirillum magnetotacticum comb. nov. Syst. Appl. Microbiol. 14, 379–385. doi:10.1016/S0723-2020(11)80313-9
Schröfel, A., Kratošová, G., Šafařík, I., Šafaříková, M., Raška, I., and Shor, L. M. (2014). Applications of biosynthesized metallic nanoparticles – a review. Acta Biomater. 10, 4023–4042. doi:10.1016/j.actbio.2014.05.022
Schuchmann, K., and Müller, V. (2012). A bacterial electron-bifurcating hydrogenase. J. Biol. Chem. 287, 31165–31171. doi:10.1074/jbc.M112.395038
Schuler, D. (2002). The biomineralization of magnetosomes in Magnetospirillum gryphiswaldense. Int. Microbiol. 5, 209–214. doi:10.1007/s10123-002-0086-8
Sharma, A., Chaturvedi, R., Islam, A., and Singh, P. K. (2021). Eco-friendly synthesis F Cadmium sulfide nanoparticles using supernatant of Bacillus badius and its characterization. Mater. Today Proc. 45, 3419–3421. doi:10.1016/j.matpr.2020.12.926
Sharma, N., Pinnaka, A. K., Raje, M., Fnu, A., Bhattacharyya, M. S., and Choudhury, A. R. (2012). Exploitation of marine bacteria for production of gold nanoparticles. Microb. Cell Factories 11, 86. doi:10.1186/1475-2859-11-86
Shi, L., Dong, H., Reguera, G., Beyenal, H., Lu, A., Liu, J., et al. (2016). Extracellular electron transfer mechanisms between microorganisms and minerals. Nat. Rev. Microbiol. 14, 651–662. doi:10.1038/nrmicro.2016.93
Shi, L., Rosso, K. M., Clarke, T. A., Richardson, D. J., Zachara, J. M., and Fredrickson, J. K. (2012). Molecular underpinnings of Fe(III) oxide reduction by Shewanella oneidensis MR-1. Front. Microbiol. 3, 50. doi:10.3389/fmicb.2012.00050
Shim, H-W., Jin, Y-H., Seo, S-D., Lee, S-H., and Kim, D-W. (2011). Highly reversible lithium storage in Bacillus subtilis-directed porous Co 3 O 4 nanostructures. ACS Nano 5, 443–449. doi:10.1021/nn1021605
Shim, H-W., Lim, A-H., Kim, J-C., Jang, E., Seo, S-D., Lee, G-H., et al. (2013). Scalable one-pot bacteria-templating synthesis route toward hierarchical, porous-Co3O4 superstructures for supercapacitor electrodes. Sci. Rep. 3, 2325. doi:10.1038/srep02325
Shojaei, M., Shokuhfar, A., and Zolriasatein, A. (2021). Synthesis and characterization of CuAlS2 nanoparticles by mechanical milling. Mater. Today Commun. 27, 102243. doi:10.1016/j.mtcomm.2021.102243
Shunmugam, R., Renukadevi Balusamy, S., Kumar, V., Menon, S., Lakshmi, T., and Perumalsamy, H. (2021). Biosynthesis of gold nanoparticles using marine microbe (Vibrio alginolyticus) and its anticancer and antioxidant analysis. J. King Saud Univ. - Sci. 33, 101260. doi:10.1016/j.jksus.2020.101260
Silver, S. (1996). Bacterial resistances to toxic metal ions - a review. Gene 179, 9–19. doi:10.1016/S0378-1119(96)00323-X
Singh, H., Du, J., and Yi, T-H. (2017). Biosynthesis of silver nanoparticles using Aeromonas sp. THG-FG1.2 and its antibacterial activity against pathogenic microbes. Artif. Cells, Nanomedicine, Biotechnol. 45, 584–590. doi:10.3109/21691401.2016.1163715
Singh, N., Jenkins, G. J. S., Asadi, R., and Doak, S. H. (2010). Potential toxicity of superparamagnetic iron oxide nanoparticles (SPION). Nano Rev. 1, 5358. doi:10.3402/nano.v1i0.5358
Singh, P., Singh, H., Kim, Y. J., Mathiyalagan, R., Wang, C., and Yang, D. C. (2016). Extracellular synthesis of silver and gold nanoparticles by Sporosarcina koreensis DC4 and their biological applications. Enzyme Microb. Technol. 86, 75–83. doi:10.1016/j.enzmictec.2016.02.005
Søbjerg, L. S., Gauthier, D., Lindhardt, A. T., Bunge, M., Finster, K., Meyer, R. L., et al. (2009). Bio-supported palladium nanoparticles as a catalyst for suzuki–miyaura and mizoroki–heck reactions. Green Chem. 11, 2041. doi:10.1039/b918351p
Soni, V., Raizada, P., Singh, P., Cuong, H. N., Rangabhashiyam, S., Saini, A., et al. (2021). Sustainable and green trends in using plant extracts for the synthesis of biogenic metal nanoparticles toward environmental and pharmaceutical advances: A review. Environ. Res. 202, 111622. doi:10.1016/j.envres.2021.111622
Stephen, A. J., Rees, N. V., Mikheenko, I., and Macaskie, L. E. (2019). Platinum and palladium bio-synthesized nanoparticles as sustainable fuel cell catalysts. Front. Energy Res. 7, 66. doi:10.3389/fenrg.2019.00066
Stoller, M., and Ochando-Pulido, J. M. (2020). ZnO nano-particles production intensification by means of a spinning disk reactor. Nanomaterials 10, 1321. doi:10.3390/nano10071321
Sun, J-B., Zhao, F., Tang, T., Jiang, W., Tian, J., Li, Y., et al. (2008). High-yield growth and magnetosome formation by Magnetospirillum gryphiswaldense MSR-1 in an oxygen-controlled fermentor supplied solely with air. Appl. Microbiol. Biotechnol. 79, 389. doi:10.1007/s00253-008-1453-y
Sundararaju, S., Arumugam, M., and Bhuyar, P. (2020). Microbacterium sp. MRS-1, a potential bacterium for cobalt reduction and synthesis of less/non-toxic cobalt oxide nanoparticles (Co3O4). Beni-Suef Univ. J. Basic Appl. Sci. 9, 44. doi:10.1186/s43088-020-00070-y
Tan, L., Ray Jones, T., Poitras, J., Xie, J., Liu, X., and Southam, G. (2020). Biochemical synthesis of palladium nanoparticles: The influence of chemical fixatives used in electron microscopy on nanoparticle formation and catalytic performance. J. Hazard. Mater. 398, 122945. doi:10.1016/j.jhazmat.2020.122945
Tang, S., Qiao, R., Lin, Y., Li, Y., Zhao, Q., Yuan, D., et al. (2019). Functional liquid metal nanoparticles produced by liquid-based nebulization. Adv. Mater. Technol. 4, 1800420. doi:10.1002/admt.201800420
Taran, M., Rad, M., and Alavi, M. (2017). Biosynthesis of TiO2 and ZnO nanoparticles by Halomonas elongata IBRC-M 10214 in different conditions of medium. BioImpacts 8, 81–89. doi:10.15171/bi.2018.10
Tavares, J., Swanson, E. J., and Coulombe, S. (2008). Plasma synthesis of coated metal nanoparticles with surface properties tailored for dispersion. Plasma Process. Polym. 5, 759–769. doi:10.1002/ppap.200800074
Tikariha, S., Banerjee, S., Dev, A., and Singh, S. (2017). Growth phase-dependent synthesis of gold nanoparticles using Bacillus licheniformis. Applications of biotechnology for sustainable development. Singapore: Springer Singapore, 121–128. doi:10.1007/978-981-10-5538-6_15
Toropova, Y., Golovkin, A., Malashicheva, A., Korolev, D., Gorshkov, A., Gareev, K., et al. (2017). In vitro toxicity of Fe<sub>m</sub>O<sub>n</sub>, Fe<sub>m</sub>O<sub>n</sub>-SiO<sub>2</sub> composite, and SiO<sub>2</sub>-Fe<sub>m</sub>O<sub>n</sub> core-shell magnetic nanoparticles. Int. J. Nanomedicine 12, 593–603. doi:10.2147/IJN.S122580
Tsuruta, T. (2004). Biosorption and recycling of gold using various microorganisms. J. General Appl. Microbiol. 50, 221–228. doi:10.2323/jgam.50.221
Tuo, Y., Liu, G., Dong, B., Yu, H., Zhou, J., Wang, J., et al. (2017). Microbial synthesis of bimetallic PdPt nanoparticles for catalytic reduction of 4-nitrophenol. Environ. Sci. Pollut. Res. 24, 5249–5258. doi:10.1007/s11356-016-8276-7
Tuo, Y., Liu, G., Dong, B., Zhou, J., Wang, A., Wang, J., et al. (2015). Microbial synthesis of Pd/Fe3O4, Au/Fe3O4 and PdAu/Fe3O4 nanocomposites for catalytic reduction of nitroaromatic compounds. Sci. Rep. 5, 13515. doi:10.1038/srep13515
Uzun, M., Alekseeva, L., Krutkina, M., Koziaeva, V., and Grouzdev, D. (2020). Unravelling the diversity of magnetotactic bacteria through analysis of open genomic databases. Sci. Data 7, 252. doi:10.1038/s41597-020-00593-0
Vijayakumar, M., Priya, K., Nancy, F. T., Noorlidah, A., and Ahmed, A. B. A. (2013). Biosynthesis, characterisation and anti-bacterial effect of plant-mediated silver nanoparticles using Artemisia nilagirica. Industrial Crops Prod. 41, 235–240. doi:10.1016/j.indcrop.2012.04.017
Vinay, S. P., Udayabhanu, , Nagaraju, G., Chandrappa, C. P., and Chandrasekhar, N. (2020). Hydrothermal synthesis of gold nanoparticles using spider cobweb as novel biomaterial: Application to photocatalytic. Chem. Phys. Lett. 748, 137402. doi:10.1016/j.cplett.2020.137402
Vincent, S. G. T., Jennerjahn, T., and Ramasamy, K. (2021). “Environmental variables and factors regulating microbial structure and functions,” in Microbial communities in coastal sediments (Amsterdam, Netherlands: Elsevier), 79–117. doi:10.1016/B978-0-12-815165-5.00003-0
Waldron, K. J., and Robinson, N. J. (2009). How do bacterial cells ensure that metalloproteins get the correct metal? Nat. Rev. Microbiol. 7, 25–35. doi:10.1038/nrmicro2057
Wang, W., Zhang, B., Liu, Q., Du, P., Liu, W., and He, Z. (2018). Biosynthesis of palladium nanoparticles using Shewanella loihica PV-4 for excellent catalytic reduction of chromium(vi). Environ. Sci. Nano. 5, 730–739. doi:10.1039/C7EN01167A
Wang, Y., Sevinc, P. C., Belchik, S. M., Fredrickson, J., Shi, L., and Lu, H. P. (2013). Single-cell imaging and spectroscopic analyses of Cr(VI) reduction on the surface of bacterial cells. Langmuir 29, 950–956. doi:10.1021/la303779y
Weng, X., Guo, M., Luo, F., and Chen, Z. (2017). One-step green synthesis of bimetallic Fe/Ni nanoparticles by eucalyptus leaf extract: Biomolecules identification, characterization and catalytic activity. Chem. Eng. J. 308, 904–911. doi:10.1016/j.cej.2016.09.134
Weng, Y., Li, J., Ding, X., Wang, B., Dai, S., Zhou, Y., et al. (2020). <p>Functionalized gold and silver bimetallic nanoparticles using <em>Deinococcus radiodurans</em> protein extract mediate degradation of toxic dye malachite green</p>. Int. J. Nanomedicine 15, 1823–1835. doi:10.2147/IJN.S236683
Wiesemann, N., Mohr, J., Grosse, C., Herzberg, M., Hause, G., Reith, F., et al. (2013). Influence of copper resistance determinants on gold transformation by Cupriavidus metallidurans strain CH34. J. Bacteriol. 195, 2298–2308. doi:10.1128/JB.01951-12
Xia, X., Wu, S., Li, N., Wang, D., Zheng, S., and Wang, G. (2018). Novel bacterial selenite reductase CsrF responsible for Se(IV) and Cr(VI) reduction that produces nanoparticles in Alishewanella sp. WH16-1. J. Hazard. Mater. 342, 499–509. doi:10.1016/j.jhazmat.2017.08.051
Xiao, X., Ma, X-B., Yuan, H., Liu, P-C., Lei, Y-B., Xu, H., et al. (2015). Photocatalytic properties of zinc sulfide nanocrystals biofabricated by metal-reducing bacterium Shewanella oneidensis MR-1. J. Hazard. Mater. 288, 134–139. doi:10.1016/j.jhazmat.2015.02.009
Xing, J., Yin, T., Li, S., Xu, T., Ma, A., Chen, Z., et al. (2021). Sequential magneto-actuated and optics-triggered biomicrorobots for targeted cancer therapy. Adv. Funct. Mater. 31, 2008262. doi:10.1002/adfm.202008262
Xu, H., Xiao, Y., Xu, M., Cui, H., Tan, L., Feng, N., et al. (2019). Microbial synthesis of Pd–Pt alloy nanoparticles using Shewanella oneidensis MR-1 with enhanced catalytic activity for nitrophenol and azo dyes reduction. Nanotechnology 30, 065607. doi:10.1088/1361-6528/aaf2a6
Xu, S., Luo, X., Huang, Q., and Chen, W. (2021). Calcium-crosslinked alginate-encapsulated bacteria for remediating of cadmium-polluted water and production of CdS nanoparticles. Appl. Microbiol. Biotechnol. 105, 2171–2179. doi:10.1007/s00253-021-11155-8
Yahyaei, B., Peyvandi, N., Akbari, H., Arabzadeh, S., Afsharnezhad, S., Ajoudanifar, H., et al. (2016). Production, assessment, and impregnation of hyaluronic acid with silver nanoparticles that were produced by Streptococcus pyogenes for tissue engineering applications. Appl. Biol. Chem. 59, 227–237. doi:10.1007/s13765-016-0147-x
Yan, L., Zhang, S., Chen, P., Liu, H., Yin, H., and Li, H. (2012). Magnetotactic bacteria, magnetosomes and their application. Microbiol. Res. 167, 507–519. doi:10.1016/j.micres.2012.04.002
Yang, J., Ju, P., Dong, X., Duan, J., Xiao, H., Tang, X., et al. (2023). Green synthesis of functional metallic nanoparticles by dissimilatory metal-reducing bacteria “Shewanella”: A comprehensive review. J. Mater. Sci. Technol. 158, 63–76. doi:10.1016/j.jmst.2023.01.041
Yang, Y., Song, B., Ke, X., Xu, F., Bozhilov, K. N., Hu, L., et al. (2020). Aerosol synthesis of high entropy alloy nanoparticles. Langmuir 36, 1985–1992. doi:10.1021/acs.langmuir.9b03392
Yaqoob, A. A., Ahmad, H., Parveen, T., Ahmad, A., Oves, M., Ismail, I. M. I., et al. (2020). Recent advances in metal decorated nanomaterials and their various biological applications: A review. Front. Chem. 8, 341. doi:10.3389/fchem.2020.00341
Yu, X., and Jiang, J. (2019). Phosphate microbial mineralization removes nickel ions from electroplating wastewater. J. Environ. Manag. 245, 447–453. doi:10.1016/j.jenvman.2019.05.091
Yu, Y-Y., Cheng, Q-W., Sha, C., Chen, Y-X., Naraginti, S., and Yong, Y-C. (2020). Size-controlled biosynthesis of FeS nanoparticles for efficient removal of aqueous Cr(VI). Chem. Eng. J. 379, 122404. doi:10.1016/j.cej.2019.122404
Zaki, S. A. E-F., Kamal, A., Ashmawy, N. A., and Shoeib, A. A. (2021). Nano-metals forming bacteria in Egypt. I. Synthesis, characterization and effect on some phytopathogenic bacteria in vitro. Sci. Rep. 11, 12876. doi:10.1038/s41598-021-92171-6
Zammit, C. M., and Reith, F. (2013). Gold biomineralization in bacterium Cupriavidus metallidurans. Encyclopedia of metalloproteins. New York, NY: Springer New York, 862–867. doi:10.1007/978-1-4614-1533-6_581
Zhan, G., Li, D., and Zhang, L. (2012). Aerobic bioreduction of nickel(II) to elemental nickel with concomitant biomineralization. Appl. Microbiol. Biotechnol. 96, 273–281. doi:10.1007/s00253-011-3827-9
Zhang, S., Zhou, H., Liao, H., Tan, P., Tian, W., and Pan, J. (2022). Microbial synthesis of efficient palladium electrocatalyst with high loadings for oxygen reduction reaction in acidic medium. J. Colloid Interface Sci. 611, 161–171. doi:10.1016/j.jcis.2021.12.080
Zhang, Y., Zhao, Q., and Chen, B. (2022). Reduction and removal of Cr(VI) in water using biosynthesized palladium nanoparticles loaded Shewanella oneidensis MR-1. Sci. Total Environ. 805, 150336. doi:10.1016/j.scitotenv.2021.150336
Keywords: bacteria, metal nanoparticles, biosynthesis, nanoparticles, bionanotechnology
Citation: Campaña AL, Saragliadis A, Mikheenko P and Linke D (2023) Insights into the bacterial synthesis of metal nanoparticles. Front. Nanotechnol. 5:1216921. doi: 10.3389/fnano.2023.1216921
Received: 04 May 2023; Accepted: 02 August 2023;
Published: 10 August 2023.
Edited by:
Sonu Gandhi, National Institute of Animal Biotechnology (NIAB), IndiaReviewed by:
Meghana Ramani, Wayne State University, United StatesZijiao Zhang, Microsoft, United States
Copyright © 2023 Campaña, Saragliadis, Mikheenko and Linke. This is an open-access article distributed under the terms of the Creative Commons Attribution License (CC BY). The use, distribution or reproduction in other forums is permitted, provided the original author(s) and the copyright owner(s) are credited and that the original publication in this journal is cited, in accordance with accepted academic practice. No use, distribution or reproduction is permitted which does not comply with these terms.
*Correspondence: Ana Lucía Campaña, YS5sLmMucGVyaWxsYUBmeXMudWlvLm5v; Dirk Linke, ZGlyay5saW5rZUBpYnYudWlvLm5v