- 1Dipartimento di Scienze Farmaceutiche, Universitá Degli Studi di Milano, Milan, Italy
- 2Department of Molecular Chemistry and Pharmacology, Istituto di Ricerche Farmacologiche Mario Negri IRCCS, Milan, Italy
There are many challenges in delivering active pharmaceutical ingredients from biomaterials, including retention of payload activity, accurate temporal release, and precise spatial administration, to name only a few. With our constantly increasing knowledge of biology and physiology, pathologies that require therapeutic interventions are becoming more understood. While the desired temporal and spatial administration of a therapy might be theorized, the ability to deliver an active therapeutic in a precise location during a specific time frame is often challenging. This has led researchers to develop hybrid biomaterials containing inorganic nanoparticles in order to combine the advantages of both inorganics and organics in payload delivery applications. Organic materials have many beneficial properties, including the ability to form networks and matrices to create three-dimensional structures from the nanometer to centimeter scale, biodegradability, the versatility to use both synthetic and natural precursors, and ease of chemical modifications, while inorganic materials offer highly controllable nanoscale features, can entrap and protect therapeutics, and have degradation properties that can be tightly regulated. Here in, we discuss the current state-of-the-art in active pharmaceutical ingredient delivery from biomaterial hybrids, demonstrate the added levels of control that these hybrid biomaterials offer, and give our perspective on future innovations in the field.
Introduction
Innovations in biomaterial design have accelerated greatly over the last decades, expanding from materials that have limited interactions with the body to current designs where natural, synthetic, composite, living, stimuli responsive and/or actuable biomaterials not only interact with cells and tissues but can also instruct specific outcomes post implantation (Dos Santos et al., 2020; Lavrador et al., 2021). Implanted organic biomaterials are most commonly in the form of hydrogels or polymer scaffolds, and can either be injected into tissues or preformed structures can be surgically placed into an exposed area (Pawelec et al., 2018; Babu et al., 2021). One important property engineered into biomaterials is the capability to release active pharmaceutical ingredients (APIs) for applications ranging from cancer therapeutics to nervous system repair (Fadia et al., 2020; Yang et al., 2020; Ciciriello et al., 2021). Releasing APIs from implantable biomaterials has several advantages, including on site release with tunable kinetics, administration of multiple APIs from the same biomaterial, and actuable payload release (Dumont et al., 2015; Rambhia and Ma, 2015; Gayet et al., 2020). As a way to more precisely regulate API release kinetics from biomaterials, inorganic nanoparticles have begun to be incorporated into their structure to form nanoparticle-laden composites. These composites allow for the advantages of organic biomaterials, formation of 3D architectures, biodegradability, cellular adhesion, and diverse material selections, to be combined with the advantages of inorganic nanoparticles, including designed degradability, tunable nanoscale sizes, the capability to include mesopores into the nanoparticles, and, importantly, entrapment and protection of APIs.
Inorganic nanoparticles used in biomedical applications are derived from many different materials, including gold, silver, iron oxide, clay, silicon, and silica (Park et al., 2009; Mousa et al., 2018; Dutz et al., 2020; Kankala et al., 2020; Mitchell et al., 2021; Morillas-Becerril et al., 2021). Gold nanoparticles are inert and nontoxic, can be synthesized with diameters ranging from 1–150 nm, and offer photophysical properties as well as the ability for drugs to be incorporated onto their surface (Ghosh et al., 2008). Silver nanoparticles are readily fabricated below 100 nm, and show the most promise in biomedical applications as anti-bacterial and anti-fungals (Nene et al., 2021). Super paramagnetic iron oxide nanoparticles can be fabricated in a range of sizes from >10 nm to more than 200 nm and their magnetic properties allow them to be contrasting agents in biomedical imaging (Dadfar et al., 2019). It is difficult to make these three classes of nanoparticles porous, limiting the potential to trap APIs within these particles. Clay nanoparticles are layered silicate structures that crystalize into nanoparticles, and their interactions with biologics make them intriguing carriers for drug delivery, but their variability and solubility may be problematic (Dawson and Oreffo, 2013). Silicon nanoparticles, especially porous silicon nanoparticles (pSiNPs), are most commonly created through electrochemical etching of crystalline silicon in sizes ranging from ∼70 nm to multiple micrometers, and their large pore volume, biodegradability, and ease of surface functionalization make them excellent API carriers (Canham, 1995; Li et al., 2018). Fabrication of these particles predominately requires hydrofluoric acid and there is variability in their size distribution. Mesoporous silica nanoparticles (MSNs) are most commonly synthesized through the surfactant templating approach, and their large pore volume, tunable sizes and shapes, and ease of surface functionalization give them exceptional properties for API delivery (Manzano and Vallet-Regi, 2020). However, controlling their biodegradation properties can be difficult. While the main research thrust in particle development for applications in health has been towards nanomedicine, the unique properties of inorganic nanoparticles have begun to lead researchers to incorporate them into biomaterials to create multifunctional hybrid materials with a greater level of control of API release.
Inorganic nanoparticles incorporated into three-dimensional biomaterials
There are many different strategies that have been used to incorporate inorganic nanoparticles into biomaterials, and these procedures are selected based on several factors, including the desired material properties, the fabrication processes of both the inorganic nanoparticles and the biomaterial, and the desired application. Hydrogels, polymer networks that have the ability to retain water and form a swollen gel, are used extensively as biomaterials due to their biocompatibility, resemblance to native tissues in mechanical properties and water content, ease of fabrication, injectability, and their versatility to be readily modified to induce cell adhesion and growth (Kim et al., 1992; Mandal et al., 2020). Inorganic nanoparticles have been incorporated into hydrogels through three general design principles: 1) micro or nano-gels that stabilize nanoparticles, 2) nanoparticles non-covalently incorporated into the hydrogel network, and 3) nanoparticles covalently crosslinked in a hydrogel matrix (Thoniyot et al., 2015). One straightforward fabrication approach is the formation of a hydrogel in a nanoparticle suspension that non-covalently incorporates nanoparticles into the hydrogel structure (Sershen et al., 2002). Nanoparticles have also been incorporated into hydrogels after gelation, either through addition of nanoparticles during hydrogel swelling or through various centrifugation steps with a preformed hydrogel (Pardo-Yissar et al., 2001; Jones and Lyon, 2003). Another approach loads nanoparticle precursors into crosslinked hydrogels with the addition of reducing agents forming nanoparticles throughout the hydrogel network (Wang et al., 2004). Nanoparticles themselves have been used as crosslinkers for hydrogels, incorporating them directly into the hydrogel network to impact the hydrogel mechanical properties and gelation rate (Skelton et al., 2013; Rose et al., 2014; Fiorini et al., 2016). By applying these techniques, inorganic nanoparticles can be incorporated into hydrogels in order to capitalize on the advantages of both material types. This has allowed researchers to create hydrogels with unique characteristics, including self-healing (Wu et al., 2020), photothermal activity (Sershen et al., 2005), magnetically induced hydrogel disruption (Qin et al., 2009), anti-bacterial effects (Urzedo et al., 2020), and electrical conductivity (Koppes A.N et al., 2016), demonstrating the various functions that these materials may possess.
Biodegradable polymers represent a class of biomaterial scaffolds that are used extensively in tissue engineering (Zhang and King, 2020). They can be fabricated into a variety of structures, including films (Lyu et al., 2019), tissue engineering scaffolds (Daly et al., 2012; Shahriari et al., 2017; Pawelec et al., 2018), microneedles (Sullivan et al., 2010), and micro- and nano-fibers (Venugopal and Ramakrishna, 2005). Polymer films are readily fabricated via casting or spin coating, and porosity can be incorporated into scaffolds through particulate leaching from the polymer films (Suntornnond et al., 2015). Microneedles are commonly fabricated through creation of a master mold followed by filling molds with polymers of interest (Park et al., 2005). Micro- and nano-fibers are made predominately via electrospinning, but other techniques, including blow spinning, centrifugal or force spinning, or thermal drawing, have also been applied to make these structures (Coffer et al., 2005; Padron et al., 2013; Behrens et al., 2014; Canales et al., 2015; Koppes R.A et al., 2016; Dos Santos et al., 2020; Rihova et al., 2021). Because most of these polymer structures are made from a polymer melt or from polymers dissolved in an organic solvent, inorganic nanoparticles can be readily mixed into the melt or polymer solution prior to creation of the 3D structure and incorporated throughout the formed architectures (Fan et al., 2009; Kashanian et al., 2010; Kim et al., 2010; Johnson et al., 2019; Yadid et al., 2019). Inorganic nanoparticles can also be coupled onto the surface of these materials, creating polymer structures that have surfaces composed of inorganic nanoparticles (Demir et al., 2018; Funnell et al., 2021). One other approach is pressing inorganic particles into polymer structures via heating of the polymers near their melt temperatures, pressing dried particles onto the structures, and then cooling the polymers back below their melt temperature (Irani et al., 2015; Bodiford et al., 2018). 3D printing of polymer solutions and melts containing inorganic nanoparticles is another more recent advance in this field, allowing for tightly controlled three-dimensional architectures with nanoparticles embedded throughout (dos Santos et al., 2021). Using these approaches, inorganic nanoparticles are incorporated into biomaterials to capitalize on the advantageous properties of both materials.
Drug delivery from inorganic nanoparticles
Inorganic nanoparticles are being extensively explored in nanomedicine due to their capability to load APIs and target them to tissues of interest. There are several strategies that can be used to incorporate payloads within metal nanoparticles, including covalent attachment of the API to the surface of the nanoparticles (Gibson et al., 2007) (Figure 1A). There are also non-covalent interactions that have been applied by modifying the surface of gold nanoparticles to create electrostatic affinity towards nucleic acids and anionic proteins (Sandhu et al., 2002). Modifying iron oxide NP surfaces (Hola et al., 2015) allows drugs to be loaded into the interspace of nanoparticle clusters (Bakandritsos et al., 2012) or linked to an activated nanoparticle surface (Magro et al., 2014). Porous inorganic nanoparticles are especially attractive for applications in API delivery because of the large pore volume they offer to load APIs inside the structure of the nanoparticle. Pores allow for an added control of drug release kinetics, where pore size, surface chemistry, and pore capping or filling strategies can be used to tailor API release from these structures (Figure 1A). pSiNPs and MSNs have been studied extensively as API carriers because of these properties (Anglin et al., 2008; Slowing et al., 2008). APIs can be loaded using a variety of techniques, including covalent attachment to the surface (Secret et al., 2013; Zhang et al., 2019), affinity adsorption (Liu et al., 2013; Kaasalainen et al., 2015; Kwon et al., 2017; Zilony et al., 2017), oxidation trapping (Fry et al., 2014; Kim et al., 2016), calcium and magnesium silicate trapping (Kang et al., 2016; Wang et al., 2018), during synthesis (Prasetyanto et al., 2016), post-synthesis adsorption (Wang et al., 2014), adsorption and subsequent rapid solvent evaporation (Mellaerts et al., 2008), and pore-capping following drug incorporation (Lai et al., 2003; Giri et al., 2005; Aznar et al., 2009; Zhao et al., 2009).
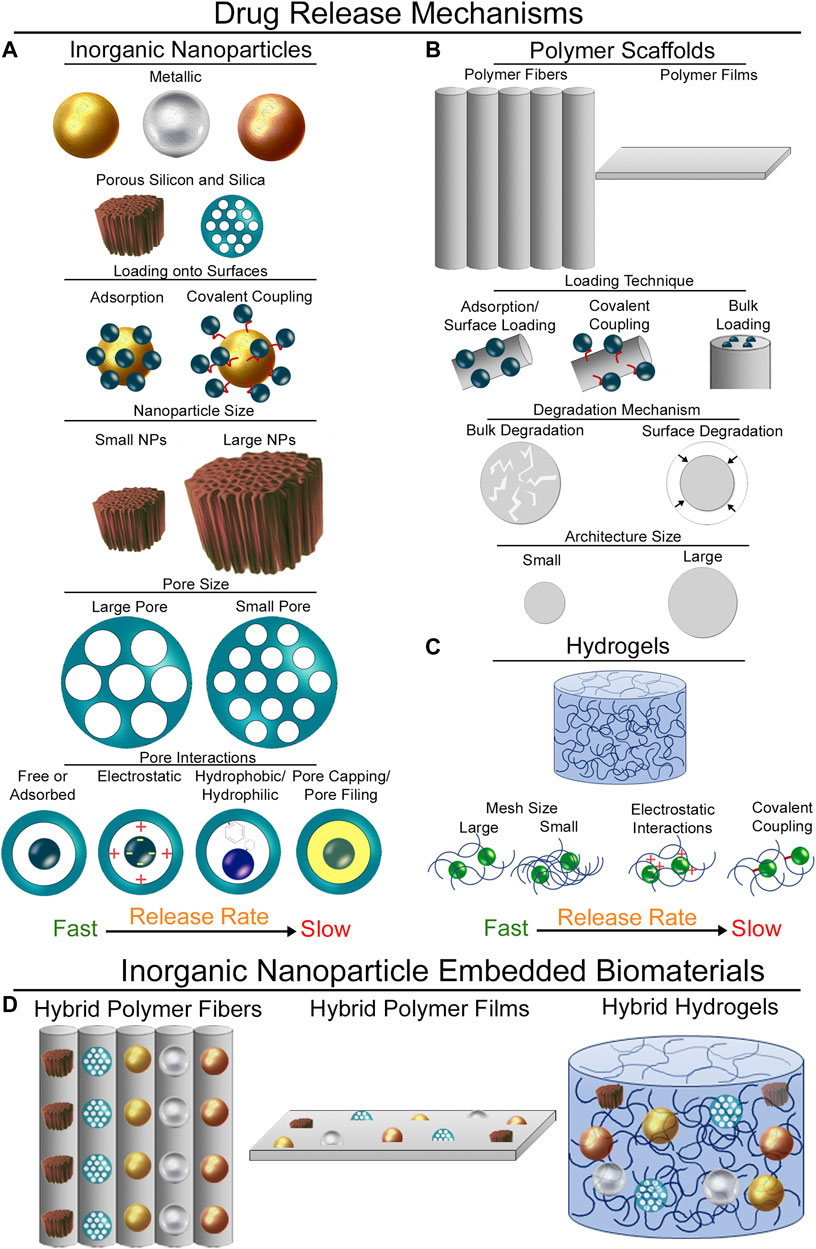
FIGURE 1. Mechanisms of payload release. (A) Mechanisms of drug release from different inorganic nanoparticles. Surface loading techniques, nanoparticle size, pore size, and pore interactions all impact the release rate of APIs from inorganic nanoparticles. (B) API release from polymer scaffolds. Loading techniques, degradation mechanisms of the polymer, and architecture size of the polymer structures impact payload release rate. (C) Release of APIs from hydrogels is impacted by hydrogel mesh size, electrostatic and other affinity-based interactions, or the ability to covalently couple APIs into the hydrogel network. (D) Inorganic nanoparticles incorporated into biomaterials. These drug release mechanisms can all be combined by incorporating inorganic nanoparticles into biomaterial scaffolds.
Polymer scaffolds in drug delivery
Polymer scaffolds, especially those composed of polycaprolactone, polylactic acid, polyglycolide, and copolymers such as poly (lactic-co-glycolic acid), have been used extensively in tissue engineering due to their biocompatibility, biodegradability, and ease of manufacturing (Liu and Ma, 2004; Place et al., 2009). These advantageous properties have led researchers to develop techniques to release APIs from polymers. The most commonly employed strategy is to blend the polymer and drug together either in solution or in a polymer melt prior to formation of the scaffold. Drug release is governed by several factors, including polymer degradation (bulk vs. surface) and the scale of the polymer architecture (Figure 1B) (Xu et al., 2017). While this method works well for hydrophobic APIs with commonly employed synthetic polymers, blending does not work well for hydrophilic APIs. Soak loading is one technique that can be used to overcome this limitation (Cho et al., 2015), but this technique can lead to burst release of the drug from the surface. In order to extend the release timeframe, APIs have been covalently linked to the polymer surface (Seifu and Nath, 2019). Fabrication specific techniques have also been employed to incorporate APIs into polymer scaffolds. Specifically, electrospinning allows for the formation of core-sheath fibers, where the inner core can be hydrophilic and the outer polymer sheath can be formed to be hydrophobic (Sill and von Recum, 2008; Hu et al., 2014), and centrifugal spinning has been used to incorporate water soluble vitamins into fibers (Rihova et al., 2022). However, hydrophilic APIs may not be homogenously distributed throughout the polymer structures and fabrication techniques need to be optimized for each API type.
Hydrogels in drug delivery
Hydrogels offer many beneficial properties in API delivery, providing spatial control of API release in their site of implantation, temporal control through polymer network interactions, and protection of APIs from the native tissue environment (Li and Mooney, 2016). The most straight-forward approach for incorporation of APIs is through mixing the payload with the hydrogel precursors prior to gel formation (Leach and Schmidt, 2005). The mesh size plays an important role in API release rates with this technique (Figure 1C) (Amsden, 1998). Hydrogels have also been designed to electrostatically interact with charged APIs to modulate their release (Tabata and Ikada, 1998). Other affinity-based mechanisms have been exploited to control API release (Delplace et al., 2016) including covalent attachment of aptamers into hydrogel networks (Soontornworajit et al., 2010), extracellular matrix-protein interactions (Sakiyama-Elbert and Hubbell, 2000), protein-protein interactions (Pakulska et al., 2013), and co-polymers containing hydrophobic domains (Thatiparti et al., 2010). Covalently binding of desired payloads to the hydrogel backbone represents a highly stable incorporation strategy that can greatly extend API release (Mann et al., 2001). The difficulty in loading hydrophobic drugs, the relatively quick release of hydrophilic APIs, and the problems associated with covalently crosslinking APIs into hydrogel networks leave areas for further innovation in the design of drug eluting hydrogels.
Payload delivery from hybrid biomaterials
Hybrid biomaterials offer diverse mechanisms to control API release kinetics. These biomaterials create complex drug interactions with the implanted scaffolds, first through the API loading strategy employed, then the choice of material and architecture, and finally through affinities to the biomaterial network. Polymeric nano-and micro-fibers have been created with drug loaded inorganic nanoparticles embedded throughout (Figure 1D) (Elsherbini and Sabra, 2022). API loaded MSNs have been used extensively to create these hybrid materials using electrospinning techniques (Qiu et al., 2013; Zhou et al., 2015; Lim et al., 2016; Yuan et al., 2016; Chen et al., 2019; Lian et al., 2019; Wang et al., 2019; Sun et al., 2020; Mohebian et al., 2021; Xu et al., 2021; Batista et al., 2022). This approach can be used to extend release of loaded APIs since the nanoparticles act to protect their payloads. They also provide a versatile platform, where different classes of APIs can be loaded into the MSNs while the fabrication procedure of the hybrid fibers remains largely unchanged. Importantly, APIs can be incorporated both throughout the polymer network and within loaded nanoparticles to have two different drug release profiles (Yuan et al., 2016; Samadzadeh et al., 2021; Xu et al., 2021). This allows for quick release of the incorporated API followed by a long-term sustained release due to loading into the nanoparticles. Other nanoparticles have also been used in these strategies, including amorphous calcium phosphate (Fu et al., 2016), graphene oxide (Rezaei et al., 2021), and zinc oxide (Fazli et al., 2016). Further control of drug release from these scaffolds can be added by incorporating magnetic nanoparticles into scaffolds (Kim et al., 2013). Through application of an alternating magnetic field, magnetic nanoparticles in the scaffolds generate heat to induce swelling of the polymers and release incorporated payloads. Similar strategies can be used to incorporate pSiNPs into fibrous scaffolds. Spray nebulization techniques have been used to create nanofibrous scaffolds containing API loaded porous silicon nanoparticles (Zuidema et al., 2018; Zuidema et al., 2020a; Zuidema et al., 2020b). These platforms are highly versatile, and by simply changing the approach to load APIs into nanoparticles, the same fabrication technique was used for prolonged release of active proteins, nucleic acid-based devices and aptamers, and hydrophilic APIs. API loaded porous silicon particles have also been incorporated into poly (ε-caprolactone) films to slow release kinetics (Irani et al., 2015; Bodiford et al., 2018).
There are several mechanisms with which to control drug release from hybrid hydrogels, and the most elementary is through passive diffusion out of the nanoparticle and through the hydrogel matrix. Silica nanoparticles loaded with ibuprofen (Zhao et al., 2014), antibiotics (Alvarez et al., 2014), and proteins (Lee et al., 2013; Fiorini et al., 2016) have been embedded into hydrogels to prolong their release compared to loading the APIs directly into a hydrogel. We demonstrate this concept here to provide an obvious visual to show the power of these hybrid materials. Rhodamine 6G was loaded into poly (ethylene glycol) conjugated, cage-like organosilica nanoparticles (OSCs) following a previously reported two-step bottom-up protocol (Supplementary Material) (Talamini et al., 2021). The OSCs have a relatively small diameter (44 ± 4 nm, Supplementary Figure S1A), that allows them to be uniformly distributed throughout the precursor solution without forming aggregates. Their cage-like structure provides space for API loading while protecting the payload inside the cage. While these properties are beneficial for applications in nanomedicine, they can also be employed to control payload release from hybrid materials. OSCs were then non-covalently incorporated into a polyacrylamide hydrogel (Figure 2A). As a control, free rhodamine 6G was dispersed in the hydrogel precursor solution prior to gelation. Qualitative release of rhodamine 6G was then visualized over time from the hydrogels (Figure 2B). Rhodamine 6G hydrogels were placed in the center of a hydrogel mold so visualization of the release could be monitored. After only 3 h of incubation in PBS, it is apparent that the control hydrogels have begun to release rhodamine 6G from the hydrogel network, while only a small amount of the rhodamine can be seen outside the original area of gelation in the OSC hydrogels. The rhodamine could freely diffuse into the solution when no nanoparticles were present. Even after 48 h, rhodamine 6G can be visualized in the nanoparticle containing gels where there is only a small amount of rhodamine 6G in the surrounding hydrogel from the controls at this time frame. This experiment demonstrates how inorganic nanoparticles can be used to control the release of small molecules out of hydrogel networks. While this model system provides a visual of the advantages of these hybrids, more advanced designs are easily envisioned. Engineering the particle degradation timeline can be used to precisely release payloads instead of the elementary release kinetics demonstrated here, while incorporation of API-loaded nanoparticles into hydrogels that biodegrade on-demand may provide a means to release a nanoparticle from hours, to days, to even weeks after a hydrogel is implanted. Beyond nanoparticle loading, there are several other mechanisms with which to control drug release from hybrid hydrogels which include electro-responsive, magnetically responsive, and light responsive mechanisms (Liu et al., 2006; Giri et al., 2011; Yan et al., 2012; Merino et al., 2015). As an example, pSiNPs combined with gold nanorods have been incorporated into alginate hydrogels, and near infrared light can be used to trigger release from these materials (Zhang et al., 2018).
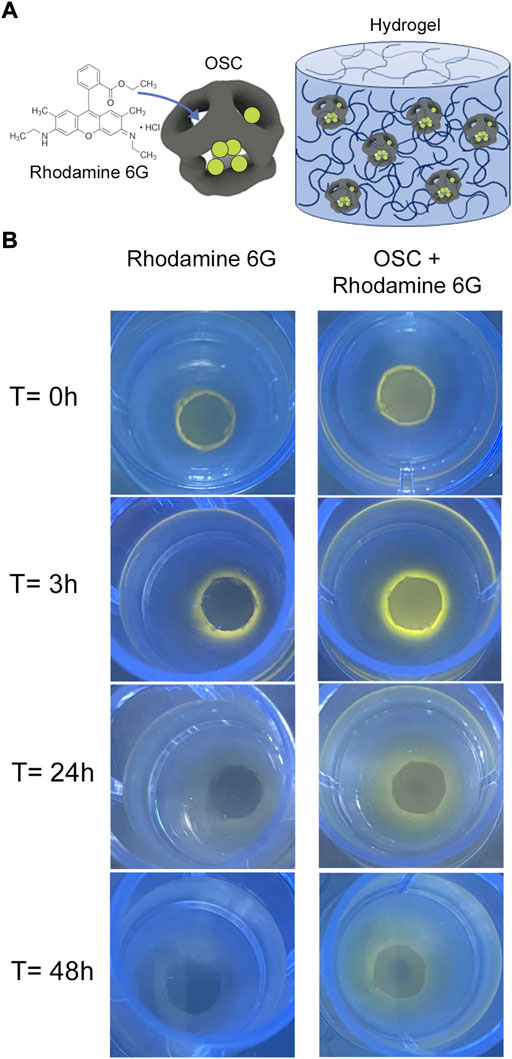
FIGURE 2. Rhodamine 6G release from OSC incorporated polyacrylamide hydrogels. (A) Schematic depicting hydrogel design. Rhodamine 6G was loaded into OSCs, following by physical entrapment in UV crosslinked polyacrylamide hydrogels. (B) Release of rhodamine 6G from hydrogels. Rhodamine 6G control hydrogels are compared to rhodamine 6G-loaded OSC incorporated hydrogels. At t = 0, rhodamine 6G control and OSC hydrogels are crosslinked into the center of the molds. At t = 3 h, the release of rhodamine 6G from control hydrogels is obvious as the dye can diffuse out of the gel through the network mesh and as the gel swells. In the OSC-rhodamine hydrogels, very little diffusion is evident. 24 h after crosslinking control hydrogels have very low amounts of rhodamine visible, while the OSC-rhodamine hydrogels still have rhodamine in the center and around the crosslinked hydrogel. At 48 h, there is nearly no rhodamine visible in the control hydrogels, while rhodamine 6G is still visible in the center the OSC-rhodamine crosslinked hydrogels and throughout the hydrogel mold.
Discussion
There are many unexplored research avenues that may elicit even further control of API release from inorganic nanoparticle biomaterial hybrids. In hybrid hydrogels, many different drug interactions can be foreseen that will improve control of API release timelines. API release first can be governed by loading into inorganic nanoparticles, and the rate can be controlled through applying adsorption loading, adding electrostatic interactions, adjusting the pore size, covalent attachment, or capping/filling the pores after loading. Once an API leaves the nanoparticle, release can be further controlled by interactions with the hydrogel network. This may be through affinity with the hydrogel itself, either through controlling the mesh size of the hydrogel or electrostatic interactions. More advanced affinities can be envisioned, such as releasing an API from an inorganic nanoparticle with affinity to an aptamer coupled to the hydrogel, as previous studies have demonstrated the ability of aptamers to sequester chemokines inside a hydrogel (Enam et al., 2017). Recent advances in hydrogel design explore injectable microparticle hydrogels that can assemble to form granular hydrogels (Daly et al., 2020; Darling et al., 2020; Muir et al., 2022). Incorporating API-loaded inorganic nanoparticles into hydrogel microparticles could be used to further control the release characteristics from these biomaterials. 3D printing strategies can also be used, where API-loaded inorganic nanoparticles are printed into hydrogels during fabrication. Within polymeric scaffolds, new pathways to control API release can also be envisioned. The ability to load and protect different categories of APIs into pSiNPS and fabricate nanofibers from solutions of polymers in organic solvents can be exploited in many different ways. One clear advancement would be to incorporate multiple therapeutics into the same scaffold. This could be done by loading different APIs into inorganic nanoparticles, and incorporating all the nanoparticles together into polymer solutions prior to fabrication. Through this process, there are possibilities to release proteins, nucleic acids, and small molecule drugs all from the same scaffold. Release rates can be controlled by selection of the polymer used to create the scaffolds and the API loading technique (Zuidema et al., 2020a).
The field of API delivery from inorganic nanoparticle incorporated biomaterials is still relatively young, and as advances to both inorganic nanoparticles and biomaterial scaffolds are realized, further complexities can be engineered into these hybrids. Currently, one of the major limitations in the field is incorporating nanoparticles only into specific regions of biomaterials. Nanoparticle location impacts API release over time, and can be important at tissue interfaces and for creating API gradients. If a pathology can be improved by releasing two APIs, location of the nanoparticles also plays a key role in their release profiles. 3D printing will play a role in selective placement of different NPs throughout biomaterial regions, however, this becomes more complicated for applications that require injectable materials. Other limitations are in optimizing biomaterial mechanical properties, since most inorganic nanoparticles will create stiff nanoregions throughout biomaterials that may impact cellular growth. Nonetheless, as nanotechnology fabrication techniques become more ubiquitous and costs decrease, combining nanoparticles with biomaterials will be a common research thrust for control of API release from biomaterials. It can be envisioned that precise API release paradigms can be achieved using biomaterial hybrids that traditional biomaterials cannot realize. With applications foreseen in diverse areas- ranging from cancer therapy, wound healing, bone repair, and nervous system regeneration- research into biomaterials that exploit the advantages of nanotechnology will continue to evolve towards applications in the clinic.
Data availability statement
The raw data supporting the conclusions of this article will be made available by the authors, without undue reservation.
Author contributions
LM-B and JZ conceived the experiments. LD provided laboratory support. LM-B synthesized nanoparticles, performed the experiments, and collected the data. JZ and LM-B wrote the manuscript. LM-B, LD, and JZ discussed the results and commented on the manuscript. This project was coordinated by JZ.
Funding
This project received funding through the European Union’s Horizon 2021 research and innovation program under the Marie Skłodowska-Curie grant agreement No. 1016770 (“PACMAN”). JZ is a Marie Skłodowska-Curie fellow.
Acknowledgments
The authors acknowledge the support of the APC central fund of the University of Milan.
Conflict of interest
The authors declare that the research was conducted in the absence of any commercial or financial relationships that could be construed as a potential conflict of interest.
Publisher’s note
All claims expressed in this article are solely those of the authors and do not necessarily represent those of their affiliated organizations, or those of the publisher, the editors and the reviewers. Any product that may be evaluated in this article, or claim that may be made by its manufacturer, is not guaranteed or endorsed by the publisher.
Supplementary material
The Supplementary Material for this article can be found online at: https://www.frontiersin.org/articles/10.3389/fnano.2022.999923/full#supplementary-material
References
Alvarez, G. S., Helary, C., Mebert, A. M., Wang, X. L., Coradin, T., and Desimone, M. F. (2014). Antibiotic-loaded silica nanoparticle-collagen composite hydrogels with prolonged antimicrobial activity for wound infection prevention. J. Mat. Chem. B 2 (29), 4660–4670. doi:10.1039/c4tb00327f
Amsden, B. (1998). Solute diffusion within hydrogels. Mechanisms and models. Macromolecules 31 (23), 8382–8395. doi:10.1021/ma980765f
Anglin, E. J., Cheng, L., Freeman, W. R., and Sailor, M. J. (2008). Porous silicon in drug delivery devices and materials. Adv. Drug Deliv. Rev. 60 (11), 1266–1277. doi:10.1016/j.addr.2008.03.017
Aznar, E., Marcos, M. D., Martinez-Manez, R., Sancenon, F., Soto, J., Amoros, P., et al. (2009). pH- and photo-switched release of guest molecules from mesoporous silica supports. J. Am. Chem. Soc. 131 (19), 6833–6843. doi:10.1021/ja810011p
Babu, S., Albertino, F., Omidinia Anarkoli, A., and De Laporte, L. (2021). Controlling structure with injectable biomaterials to better mimic tissue heterogeneity and anisotropy. Adv. Healthc. Mat. 10 (11), e2002221. doi:10.1002/adhm.202002221
Bakandritsos, A., Papagiannopoulos, A., Anagnostou, E. N., Avgoustakis, K., Zboril, R., Pispas, S., et al. (2012). Merging high doxorubicin loading with pronounced magnetic response and bio-repellent properties in hybrid drug nanocarriers. Small 8 (15), 2381–2393. doi:10.1002/smll.201102525
Batista, H., Freitas, J. P., Abrunheiro, A., Goncalves, T., Gil, M. H., Figueiredo, M., et al. (2022). Electrospun composite fibers of PLA/PLGA blends and mesoporous silica nanoparticles for the controlled release of gentamicin sulfate. Int. J. Polym. Mater. Polym. Biomaterials 71 (9), 635–646. doi:10.1080/00914037.2021.1876053
Behrens, A. M., Casey, B. J., Sikorski, M. J., Wu, K. L., Tutak, W., Sandler, A. D., et al. (2014). In situ deposition of PLGA nanofibers via solution blow spinning. ACS Macro Lett. 3 (3), 249–254. doi:10.1021/mz500049x
Bodiford, N. K., McInnes, S. J. P., Voelcker, N. H., and Coffer, J. L. (2018). Porous silicon-poly(ε-caprolactone) film composites: evaluation of drug release and degradation behavior. Biomed. Microdevices 20 (3), 71. doi:10.1007/s10544-018-0313-5
Canales, A., Jia, X. T., Froriep, U. P., Koppes, R. A., Tringides, C. M., Selvidge, J., et al. (2015). Multifunctional fibers for simultaneous optical, electrical and chemical interrogation of neural circuits in vivo. Nat. Biotechnol. 33 (3), 277–284. doi:10.1038/nbt.3093
Canham, L. T. (1995). Bioactive silicon structure fabrication through nanoetching techniques. Adv. Mat. 7 (12), 1033–1037. doi:10.1002/adma.19950071215
Chen, X. B., Xu, C., and He, H. (2019). Electrospinning of silica nanoparticles-entrapped nanofibers for sustained gentamicin release. Biochem. Biophysical Res. Commun. 516 (4), 1085–1089. doi:10.1016/j.bbrc.2019.06.163
Cho, S. J., Jung, S. M., Kang, M., Shin, H. S., and Youk, J. H. (2015). Preparation of hydrophilic PCL nanofiber scaffolds via electrospinning of PCL/PVP-b-PCL block copolymers for enhanced cell biocompatibility. Polymer 69, 95–102. doi:10.1016/j.polymer.2015.05.037
Ciciriello, A. J., Smith, D. R., Munsell, M. K., Boyd, S. J., Shea, L. D., and Dumont, C. M. (2021). IL-10 lentivirus-laden hydrogel tubes increase spinal progenitor survival and neuronal differentiation after spinal cord injury. Biotechnol. Bioeng. 118 (7), 2609–2625. doi:10.1002/bit.27781
Coffer, J. L., Whitehead, M. A., Nagesha, D. K., Mukherjee, P., Akkaraju, G., Totolici, M., et al. (2005). Porous silicon-based scaffolds for tissue engineering and other biomedical applications. Phys. Stat. Sol. 202 (8), 1451–1455. doi:10.1002/pssa.200461134
Dadfar, S. M., Roemhild, K., Drude, N. I., von Stillfried, S., Knuchel, R., Kiessling, F., et al. (2019). Iron oxide nanoparticles: Diagnostic, therapeutic and theranostic applications. Adv. Drug Deliv. Rev. 138, 302–325. doi:10.1016/j.addr.2019.01.005
Daly, A. C., Riley, L., Segura, T., and Burdick, J. A. (2020). Hydrogel microparticles for biomedical applications. Nat. Rev. Mat. 5 (1), 20–43. doi:10.1038/s41578-019-0148-6
Daly, W., Yao, L., Zeugolis, D., Windebank, A., and Pandit, A. (2012). A biomaterials approach to peripheral nerve regeneration: bridging the peripheral nerve gap and enhancing functional recovery. J. R. Soc. Interface 9 (67), 202–221. doi:10.1098/rsif.2011.0438
Darling, N. J., Xi, W. X., Sideris, E., Anderson, A. R., Pong, C., Carmichael, S. T., et al. (2020). Click by click microporous annealed particle (MAP) scaffolds. Adv. Healthc. Mat. 9 (10), 1901391. doi:10.1002/adhm.201901391
Dawson, J. I., and Oreffo, R. O. C. (2013). Clay: New opportunities for tissue regeneration and biomaterial design. Adv. Mat. 25 (30), 4069–4086. doi:10.1002/adma.201301034
Delplace, V., Obermeyer, J., and Shoichet, M. S. (2016). Local affinity release. Acs Nano 10 (7), 6433–6436. doi:10.1021/acsnano.6b04308
Demir, U. S., Shahbazi, R., Calamak, S., Ozturk, S., Gultekinoglu, M., and Ulubayram, K. (2018). Gold nano-decorated aligned polyurethane nanofibers for enhancement of neurite outgrowth and elongation. J. Biomed. Mat. Res. A 106 (6), 1604–1613. doi:10.1002/jbm.a.36365
Dos Santos, D. M., Correa, D. S., Medeiros, E. S., Oliveira, J. E., and Mattoso, L. H. C. (2020). Advances in functional polymer nanofibers: From spinning fabrication techniques to recent biomedical applications. ACS Appl. Mat. Interfaces 12 (41), 45673–45701. doi:10.1021/acsami.0c12410
dos Santos, J., de Oliveira, R. S., de Oliveira, T. V., Velho, M. C., Konrad, M. V., da Silva, G. S., et al. (2021). 3D printing and nanotechnology: A multiscale Alliance in personalized medicine. Adv. Funct. Mat. 31 (16), 2009691. doi:10.1002/adfm.202009691
Dumont, C. M., Park, J., and Shea, L. D. (2015). Controlled release strategies for modulating immune responses to promote tissue regeneration. J. Control. Release 219, 155–166. doi:10.1016/j.jconrel.2015.08.014
Dutz, S., Buske, N., Landers, J., Grafe, C., Wende, H., and Clement, J. H. (2020). Biocompatible magnetic fluids of Co-doped iron oxide nanoparticles with tunable magnetic properties. Nanomaterials 10 (6), 1019. doi:10.3390/nano10061019
Elsherbini, A. M., and Sabra, S. A. (2022). Nanoparticles-in-nanofibers composites: Emphasis on some recent biomedical applications. J. Control. Release 348, 57–83. doi:10.1016/j.jconrel.2022.05.037
Enam, S. F., Krieger, J. R., Saxena, T., Watts, B. E., Olingy, C. E., Botchwey, E. A., et al. (2017). Enrichment of endogenous fractalkine and anti-inflammatory cells via aptamer-functionalized hydrogels. Biomaterials 142, 52–61. doi:10.1016/j.biomaterials.2017.07.013
Fadia, N. B., Bliley, J. M., DiBernardo, G. A., Crammond, D. J., Schilling, B. K., Sivak, W. N., et al. (2020). Long-gap peripheral nerve repair through sustained release of a neurotrophic factor in nonhuman primates. Sci. Transl. Med. 12 (527), eaav7753. doi:10.1126/scitranslmed.aav7753
Fan, D. M., Loni, A., Canham, L. T., and Coffer, J. L. (2009). Location-dependent controlled release kinetics of model hydrophobic compounds from mesoporous silicon/biopolymer composite fibers. Phys. Status Solidi 206 (6), 1322–1325. doi:10.1002/pssa.200881118
Fazli, Y., Shariatinia, Z., Kohsari, I., Azadmehr, A., and Pourmortazavi, S. M. (2016). A novel chitosan-polyethylene oxide nanofibrous mat designed for controlled co-release of hydrocortisone and imipenem/cilastatin drugs. Int. J. Pharm. 513 (1-2), 636–647. doi:10.1016/j.ijpharm.2016.09.078
Fiorini, F., Prasetyanto, E. A., Taraballi, F., Pandolfi, L., Monroy, F., Lopez-Montero, I., et al. (2016). Nanocomposite hydrogels as platform for cells growth, proliferation, and chemotaxis. Small 12 (35), 4881–4893. doi:10.1002/smll.201601017
Fry, N. L., Boss, G. R., and Sailor, M. J. (2014). Oxidation-induced trapping of drugs in porous silicon microparticles. Chem. Mat. 26, 2758–2764. doi:10.1021/cm500797b
Fu, Q. W., Zi, Y. P., Xu, W., Zhou, R., Cai, Z. Y., Zheng, W. J., et al. (2016). Electrospinning of calcium phosphate-poly(D, L-lactic acid) nanofibers for sustained release of water-soluble drug and fast mineralization. Int. J. Nanomedicine 11, 5087–5097. doi:10.2147/Ijn.S114224
Funnell, J. L., Ziemba, A. M., Nowak, J. F., Awada, H., Prokopiou, N., Samuel, J., et al. (2021). Assessing the combination of magnetic field stimulation, iron oxide nanoparticles, and aligned electrospun fibers for promoting neurite outgrowth from dorsal root ganglia in vitro. Acta Biomater. 131, 302–313. doi:10.1016/j.actbio.2021.06.049
Gayet, R. V., de Puig, H., English, M. A., Soenksen, L. R., Nguyen, P. Q., Mao, A. S., et al. (2020). Creating CRISPR-responsive smart materials for diagnostics and programmable cargo release. Nat. Protoc. 15 (9), 3030–3063. doi:10.1038/s41596-020-0367-8
Ghosh, P., Han, G., De, M., Kim, C. K., and Rotello, V. M. (2008). Gold nanoparticles in delivery applications. Adv. Drug Deliv. Rev. 60 (11), 1307–1315. doi:10.1016/j.addr.2008.03.016
Gibson, J. D., Khanal, B. P., and Zubarev, E. R. (2007). Paclitaxel-functionalized gold nanoparticles. J. Am. Chem. Soc. 129 (37), 11653–11661. doi:10.1021/ja075181k
Giri, A., Bhowmick, M., Pal, S., and Bandyopadhyaya, A. (2011). Polymer hydrogel from carboxymethyl guar gum and carbon nanotube for sustained trans-dermal release of diclofenac sodium. Int. J. Biol. Macromol. 49 (5), 885–893. doi:10.1016/j.ijbiomac.2011.08.003
Giri, S., Trewyn, B. G., Stellmaker, M. P., and Lin, V. S. Y. (2005). Stimuli-responsive controlled-release delivery system based on mesoporous silica nanorods capped with magnetic nanoparticles. Angew. Chem. Int. Ed. 44 (32), 5038–5044. doi:10.1002/anie.200501819
Hola, K., Markova, Z., Zoppellaro, G., Tucek, J., and Zboril, R. (2015). Tailored functionalization of iron oxide nanoparticles for MRI, drug delivery, magnetic separation and immobilization of biosubstances. Biotechnol. Adv. 33 (6), 1162–1176. doi:10.1016/j.biotechadv.2015.02.003
Hu, X. L., Liu, S., Zhou, G. Y., Huang, Y. B., Xie, Z. G., and Jing, X. B. (2014). Electrospinning of polymeric nanofibers for drug delivery applications. J. Control. Release 185, 12–21. doi:10.1016/j.jconrel.2014.04.018
Irani, Y. D., Tian, Y., Wang, M. J., Klebe, S., McInnes, S. J., Voelcker, N. H., et al. (2015). A novel pressed porous silicon-polycaprolactone composite as a dual-purpose implant for the delivery of cells and drugs to the eye. Exp. Eye Res. 139, 123–131. doi:10.1016/j.exer.2015.08.007
Johnson, C. D. L., Ganguly, D., Zuidema, J. M., Cardina, T. J., Ziemba, A. M., Kearns, K. R., et al. (2019). Injectable, magnetically orienting electrospun fiber conduits for neuron guidance. ACS Appl. Mat. Interfaces 11 (1), 356–372. doi:10.1021/acsami.8b18344
Jones, C. D., and Lyon, L. A. (2003). Photothermal patterning of microgel/gold nanoparticle composite colloidal crystals. J. Am. Chem. Soc. 125 (2), 460–465. doi:10.1021/ja027431x
Kaasalainen, M., Rytkonen, J., Makila, E., Narvanen, A., and Salonen, J. (2015). Electrostatic interaction on loading of therapeutic peptide GLP-1 into porous silicon nanoparticles. Langmuir 31 (5), 1722–1729. doi:10.1021/la5047047
Kang, J., Joo, J., Kwon, E. J., Skalak, M., Hussain, S., She, Z. G., et al. (2016). Self-sealing porous silicon-calcium silicate core-shell nanoparticles for targeted siRNA delivery to the injured brain. Adv. Mat. 28, 7962–7969. doi:10.1002/adma.201600634
Kankala, R. K., Han, Y. H., Na, J., Lee, C. H., Sun, Z. Q., Wang, S. B., et al. (2020). Nanoarchitectured structure and surface biofunctionality of mesoporous silica nanoparticles. Adv. Mat. 32 (23), 1907035. doi:10.1002/adma.201907035
Kashanian, S., Harding, F., Irani, Y., Klebe, S., Marshall, K., Loni, A., et al. (2010). Evaluation of mesoporous silicon/polycaprolactone composites as ophthalmic implants. Acta Biomater. 6 (9), 3566–3572. doi:10.1016/j.actbio.2010.03.031
Kim, D., Zuidema, J. M., Kang, J., Pan, Y., Wu, L., Warther, D., et al. (2016). Facile surface modification of hydroxylated silicon nanostructures using heterocyclic silanes. J. Am. Chem. Soc. 138 (46), 15106–15109. doi:10.1021/jacs.6b08614
Kim, E. S., Kim, S. H., and Lee, C. H. (2010). Electrospinning of polylactide fibers containing silver nanoparticles. Macromol. Res. 18 (3), 215–221. doi:10.1007/s13233-010-0316-4
Kim, S. W., Bae, Y. H., and Okano, T. (1992). Hydrogels - swelling, drug loading, and release. Pharm. Res. 9 (3), 283–290. doi:10.1023/A:1015887213431
Kim, Y. J., Ebara, M., and Aoyagi, T. (2013). A smart hyperthermia nanofiber with switchable drug release for inducing cancer apoptosis. Adv. Funct. Mat. 23 (46), 5753–5761. doi:10.1002/adfm.201300746
Koppes, A. N., Keating, K. W., McGregor, A. L., Koppes, R. A., Kearns, K. R., Ziemba, A. M., et al. (2016). Robust neurite extension following exogenous electrical stimulation within single walled carbon nanotube-composite hydrogels. Acta Biomater. 39, 34–43. doi:10.1016/j.actbio.2016.05.014
Koppes, R. A., Park, S., Hood, T., Jia, X. T., Poorheravi, N. A., Achyuta, A. H., et al. (2016). Thermally drawn fibers as nerve guidance scaffolds. Biomaterials 81, 27–35. doi:10.1016/j.biomaterials.2015.11.063
Kwon, E. J., Skalak, M., Bertucci, A., Braun, G., Ricci, F., Ruoslahti, E., et al. (2017). Porous silicon nanoparticle delivery of tandem peptide anti-infectives for the treatment of Pseudomonas aeruginosa lung infections. Adv. Mater. 29 (35), 1701527. doi:10.1002/adma.201701527
Lai, C. Y., Trewyn, B. G., Jeftinija, D. M., Jeftinija, K., Xu, S., Jeftinija, S., et al. (2003). A mesoporous silica nanosphere-based carrier system with chemically removable CdS nanoparticle caps for stimuli-responsive controlled release of neurotransmitters and drug molecules. J. Am. Chem. Soc. 125 (15), 4451–4459. doi:10.1021/ja028650l
Lavrador, P., Esteves, M. R., Gaspar, V. M., and Mano, J. F. (2021). Stimuli-responsive nanocomposite hydrogels for biomedical applications. Adv. Funct. Mat. 31 (8), 2005941. doi:10.1002/adfm.202005941
Leach, J. B., and Schmidt, C. E. (2005). Characterization of protein release from photocrosslinkable hyaluronic acid-polyethylene glycol hydrogel tissue engineering scaffolds. Biomaterials 26 (2), 125–135. doi:10.1016/j.biomaterials.2004.02.018
Lee, J. H., Park, J. H., Eltohamy, M., Perez, R., Lee, E. J., and Kim, H. W. (2013). Collagen gel combined with mesoporous nanoparticles loading nerve growth factor as a feasible therapeutic three-dimensional depot for neural tissue engineering. RSC Adv. 3 (46), 24202–24214. doi:10.1039/c3ra43534b
Li, J., and Mooney, D. J. (2016). Designing hydrogels for controlled drug delivery. Nat. Rev. Mat. 1 (12), 16071. doi:10.1038/natrevmats.2016.71
Li, W., Liu, Z. H., Fontana, F., Ding, Y. P., Liu, D. F., Hirvonen, J. T., et al. (2018). Tailoring porous silicon for biomedical applications: From drug delivery to cancer immunotherapy. Adv. Mater. 30 (24), 1703740. doi:10.1002/adma.201703740
Lian, M. F., Sun, B. B., Qiao, Z. G., Zhao, K., Zhou, X. J., Zhang, Q. Q., et al. (2019). Bi-layered electrospun nanofibrous membrane with osteogenic and antibacterial properties for guided bone regeneration. Colloids Surfaces B Biointerfaces 176, 219–229. doi:10.1016/j.colsurfb.2018.12.071
Lim, H. C., Nam, O. H., Kim, M. J., El-Fiqi, A., Yun, H. M., Lee, Y. M., et al. (2016). Delivery of dexamethasone from bioactive nanofiber matrices stimulates odontogenesis of human dental pulp cells through integrin/BMP/mTOR signaling pathways. Int. J. Nanomedicine 11, 2557–2567. doi:10.2147/Ijn.S97846
Liu, D. F., Bimbo, L. M., Makila, E., Villanova, F., Kaasalainen, M., Herranz-Blanco, B., et al. (2013). Co-delivery of a hydrophobic small molecule and a hydrophilic peptide by porous silicon nanoparticles. J. Control. Release 170 (2), 268–278. doi:10.1016/j.jconrel.2013.05.036
Liu, T. Y., Hu, S. H., Liu, T. Y., Liu, D. M., and Chen, S. Y. (2006). Magnetic-sensitive behavior of intelligent ferrogels for controlled release of drug. Langmuir 22 (14), 5974–5978. doi:10.1021/la060371e
Liu, X. H., and Ma, P. X. (2004). Polymeric scaffolds for bone tissue engineering. Ann. Biomed. Eng. 32 (3), 477–486. doi:10.1023/B:ABME.0000017544.36001.8e
Lyu, J. S., Lee, J. S., and Han, J. (2019). Development of a biodegradable polycaprolactone film incorporated with an antimicrobial agent via an extrusion process. Sci. Rep. 9, 20236. doi:10.1038/s41598-019-56757-5
Magro, M., Campos, R., Baratella, D., Lima, G., Hola, K., Divoky, C., et al. (2014). A magnetically drivable nanovehicle for curcumin with antioxidant capacity and MRI relaxation properties. Chem. Eur. J. 20 (37), 11913–11920. doi:10.1002/chem.201402820
Mandal, A., Clegg, J. R., Anselmo, A. C., and Mitragotri, S. (2020). Hydrogels in the clinic. Bioeng. Transl. Med. 5 (2), e10158. doi:10.1002/btm2.10158
Mann, B. K., Schmedlen, R. H., and West, J. L. (2001). Tethered-TGF-beta increases extracellular matrix production of vascular smooth muscle cells. Biomaterials 22 (5), 439–444. doi:10.1016/S0142-9612(00)00196-4
Manzano, M., and Vallet-Regi, M. (2020). Mesoporous silica nanoparticles for drug delivery. Adv. Funct. Mat. 30 (2), 1902634. doi:10.1002/adfm.201902634
Mellaerts, R., Mols, R., Jammaer, J. A. G., Aerts, C. A., Annaert, P., Van Humbeeck, J., et al. (2008). Increasing the oral bioavailability of the poorly water soluble drug itraconazole with ordered mesoporous silica. Eur. J. Pharm. Biopharm. 69 (1), 223–230. doi:10.1016/j.ejpb.2007.11.006
Merino, S., Martin, C., Kostarelos, K., Prato, M., and Vazquez, E. (2015). Nanocomposite hydrogels: 3D polymer-nanoparticle synergies for on-demand drug delivery. Acs Nano 9 (5), 4686–4697. doi:10.1021/acsnano.5b01433
Mitchell, M. J., Billingsley, M. M., Haley, R. M., Wechsler, M. E., Peppas, N. A., and Langer, R. (2021). Engineering precision nanoparticles for drug delivery. Nat. Rev. Drug Discov. 20 (2), 101–124. doi:10.1038/s41573-020-0090-8
Mohebian, Z., Babazadeh, M., Zarghami, N., and Mousazadeh, H. (2021). Anticancer efficiency of curcumin-loaded mesoporous silica nanoparticles/nanofiber composites for potential postsurgical breast cancer treatment. J. Drug Deliv. Sci. Technol. 61, 102170. doi:10.1016/j.jddst.2020.102170
Morillas-Becerril, L., Franco-Ulloa, S., Fortunati, I., Marotta, R., Sun, X. H., Zanoni, G., et al. (2021). Specific and nondisruptive interaction of guanidium-functionalized gold nanoparticles with neutral phospholipid bilayers. Commun. Chem. 4 (1), 93. doi:10.1038/s42004-021-00526-x
Mousa, M., Evans, N. D., Oreffo, R. O. C., and Dawson, J. I. (2018). Clay nanoparticles for regenerative medicine and biomaterial design: A review of clay bioactivity. Biomaterials 159, 204–214. doi:10.1016/j.biomaterials.2017.12.024
Muir, V. G., Qazi, T. H., Weintraub, S., Maldonado, B. O. T., Arratia, P. E., and Burdick, J. A. (2022). Sticking together: Injectable granular hydrogels with increased functionality via dynamic covalent inter-particle crosslinking. Small 18, 2201115. doi:10.1002/smll.202201115
Nene, A., Galluzzi, M., Hongrong, L., Somani, P., Ramakrishna, S., and Yu, X. F. (2021). Synthetic preparations and atomic scale engineering of silver nanoparticles for biomedical applications. Nanoscale 13 (33), 13923–13942. doi:10.1039/d1nr01851e
Padron, S., Fuentes, A., Caruntu, D., and Lozano, K. (2013). Experimental study of nanofiber production through forcespinning. J. Appl. Phys. 113 (2), 024318. doi:10.1063/1.4769886
Pakulska, M. M., Vulic, K., and Shoichet, M. S. (2013). Affinity-based release of chondroitinase ABC from a modified methylcellulose hydrogel. J. Control. Release 171 (1), 11–16. doi:10.1016/j.jconrel.2013.06.029
Pardo-Yissar, V., Gabai, R., Shipway, A. N., Bourenko, T., and Willner, I. (2001). Gold nanoparticle/hydrogel composites with solvent-switchable electronic properties. Adv. Mat. 13 (17), 1320–1323. doi:10.1002/1521-4095(200109)13:17<1320::aid-adma1320>3.0.co;2-8
Park, J.-H., Gu, L., Maltzahn, G. v., Ruoslahti, E., Bhatia, S. N., and Sailor, M. J. (2009). Biodegradable luminescent porous silicon nanoparticles for in vivo applications. Nat. Mat. 8, 331–336. doi:10.1038/NMAT2398
Park, J. H., Allen, M. G., and Prausnitz, M. R. (2005). Biodegradable polymer microneedles: Fabrication, mechanics and transdermal drug delivery. J. Control. Release 104 (1), 51–66. doi:10.1016/j.jconrel.2005.02.002
Pawelec, K. M., Koffler, J., Shahriari, D., Galvan, A., Tuszynski, M. H., and Sakamoto, J. (2018). Microstructure and in vivo characterization of multi-channel nerve guidance scaffolds. Biomed. Mat. 13 (4), 044104. doi:10.1088/1748-605X/aaad85
Place, E. S., George, J. H., Williams, C. K., and Stevens, M. M. (2009). Synthetic polymer scaffolds for tissue engineering. Chem. Soc. Rev. 38 (4), 1139–1151. doi:10.1039/b811392k
Prasetyanto, E. A., Bertucci, A., Septiadi, D., Corradini, R., Castro-Hartmann, P., and De Cola, L. (2016). Breakable hybrid organosilica nanocapsules for protein delivery. Angew. Chem. Int. Ed. 55 (10), 3323–3327. doi:10.1002/anie.201508288
Qin, J., Asempah, I., Laurent, S., Fornara, A., Muller, R. N., and Muhammed, M. (2009). Injectable superparamagnetic ferrogels for controlled release of hydrophobic drugs. Adv. Mat. 21 (13), 1354–1357. doi:10.1002/adma.200800764
Qiu, K. X., He, C. L., Feng, W., Wang, W. Z., Zhou, X. J., Yin, Z. Q., et al. (2013). Doxorubicin-loaded electrospun poly(L-lactic acid)/mesoporous silica nanoparticles composite nanofibers for potential postsurgical cancer treatment. J. Mat. Chem. B 1 (36), 4601–4611. doi:10.1039/c3tb20636j
Rambhia, K. J., and Ma, P. X. (2015). Controlled drug release for tissue engineering. J. Control. Release 219, 119–128. doi:10.1016/j.jconrel.2015.08.049
Rezaei, H., Shahrezaee, M., Monfared, M. J., Karkan, S. F., and Ghafelehbashi, R. (2021). Simvastatin-loaded graphene oxide embedded in polycaprolactone-polyurethane nanofibers for bone tissue engineering applications. J. Polym. Eng. 41 (5), 375–386. doi:10.1515/polyeng-2020-0301
Rihova, M., Ince, A. E., Cicmancova, V., Hromadko, L., Castkova, K., Pavlinak, D., et al. (2021). Water‐born 3D nanofiber mats using cost‐effective centrifugal spinning: comparison with electrospinning process: A complex study. J. Appl. Polym. Sci. 138 (5), 49975. doi:10.1002/app.49975
Rihova, M., Lepcio, P., Cicmancova, V., Frumarova, B., Hromadko, L., Bures, F., et al. (2022). The centrifugal spinning of vitamin doped natural gum fibers for skin regeneration. Carbohydr. Polym. 294, 119792. doi:10.1016/j.carbpol.2022.119792
Rose, S., Prevoteau, A., Elziere, P., Hourdet, D., Marcellan, A., and Leibler, L. (2014). Nanoparticle solutions as adhesives for gels and biological tissues. Nature 505 (7483), 382–385. doi:10.1038/nature12806
Sakiyama-Elbert, S. E., and Hubbell, J. A. (2000). Development of fibrin derivatives for controlled release of heparin-binding growth factors. J. Control. Release 65 (3), 389–402. doi:10.1016/S0168-3659(99)00221-7
Samadzadeh, S., Babazadeh, M., Zarghami, N., Pilehvar-Soltanahmadi, Y., and Mousazadeh, H. (2021). An implantable smart hyperthermia nanofiber with switchable, controlled and sustained drug release: Possible application in prevention of cancer local recurrence. Mater. Sci. Eng. C 118, 111384. doi:10.1016/j.msec.2020.111384
Sandhu, K. K., McIntosh, C. M., Simard, J. M., Smith, S. W., and Rotello, V. M. (2002). Gold nanoparticle-mediated Transfection of mammalian cells. Bioconjug. Chem. 13 (1), 3–6. doi:10.1021/bc015545c
Secret, E., Maynadier, M., Gallud, A., Gary-Bobo, M., Chaix, A., Maillard, P., et al. (2013). Anionic porphyrin-grafted porous silicon nanoparticles for photodynamic therapy. Chem. Commun. 49, 4202–4204. doi:10.1039/C3CC38837A
Seifu, M. F., and Nath, L. K. (2019). Polymer-drug conjugates: Novel carriers for cancer chemotherapy. Polymer-Plastics Technol. Mater. 58 (2), 158–171. doi:10.1080/03602559.2018.1466172
Sershen, S. R., Mensing, G. A., Ng, M., Halas, N. J., Beebe, D. J., and West, J. L. (2005). Independent optical control of microfluidic valves formed from optomechanically responsive nanocomposite hydrogels. Adv. Mat. 17 (11), 1366–1368. doi:10.1002/adma.200401239
Sershen, S. R., Westcott, S. L., Halas, N. J., and West, J. L. (2002). Independent optically addressable nanoparticle-polymer optomechanical composites. Appl. Phys. Lett. 80 (24), 4609–4611. doi:10.1063/1.1481536
Shahriari, D., Koffler, J. Y., Tuszynski, M. H., Campana, W. M., and Sakamoto, J. S. (2017). Hierarchically ordered porous and high-volume polycaprolactone microchannel scaffolds enhanced axon growth in transected spinal cords. Tissue Eng. Part A 23 (9-10), 415–425. doi:10.1089/ten.TEA.2016.0378
Sill, T. J., and von Recum, H. A. (2008). Electro spinning: Applications in drug delivery and tissue engineering. Biomaterials 29 (13), 1989–2006. doi:10.1016/j.biomaterials.2008.01.011
Skelton, S., Bostwick, M., O'Connor, K., Konst, S., Casey, S., and Lee, B. P. (2013). Biomimetic adhesive containing nanocomposite hydrogel with enhanced materials properties. Soft Matter 9 (14), 3825–3833. doi:10.1039/c3sm27352k
Slowing, I. I., Vivero-Escoto, J. L., Wu, C. W., and Lin, V. S. Y. (2008). Mesoporous silica nanoparticles as controlled release drug delivery and gene transfection carriers. Adv. Drug Deliv. Rev. 60 (11), 1278–1288. doi:10.1016/j.addr.2008.03.012
Soontornworajit, B., Zhou, J., Zhang, Z. Y., and Wang, Y. (2010). Aptamer-Functionalized in situ injectable hydrogel for controlled protein release. Biomacromolecules 11 (10), 2724–2730. doi:10.1021/bm100774t
Sullivan, S. P., Koutsonanos, D. G., Martin, M. D., Lee, J. W., Zarnitsyn, V., Choi, S. O., et al. (2010). Dissolving polymer microneedle patches for influenza vaccination. Nat. Med. 16 (8), 915–920. doi:10.1038/nm.2182
Sun, J., Fan, Y., Zhang, P., Zhang, X., Zhou, Q., Zhao, J., et al. (2020). Self-enriched mesoporous silica nanoparticle composite membrane with remarkable photodynamic antimicrobial performances. J. Colloid Interface Sci. 559, 197–205. doi:10.1016/j.jcis.2019.10.021
Suntornnond, R., An, J., Yeong, W. Y., and Chua, C. K. (2015). Biodegradable polymeric films and membranes processing and forming for tissue engineering. Macromol. Mat. Eng. 300 (9), 858–877. doi:10.1002/mame.201500028
Tabata, Y., and Ikada, Y. (1998). Protein release from gelatin matrices. Adv. Drug Deliv. Rev. 31 (3), 287–301. doi:10.1016/S0169-409x(97)00125-7
Talamini, L., Picchetti, P., Ferreira, L. M., Sitia, G., Russo, L., Violatto, M. B., et al. (2021). Organosilica cages target hepatic sinusoidal endothelial cells avoiding macrophage filtering. Acs Nano 15 (6), 9701–9716. doi:10.1021/acsnano.1c00316
Thatiparti, T. R., Shoffstall, A. J., and von Recum, H. A. (2010). Cyclodextrin-based device coatings for affinity-based release of antibiotics. Biomaterials 31 (8), 2335–2347. doi:10.1016/j.biomaterials.2009.11.087
Thoniyot, P., Tan, M. J., Karim, A. A., Young, D. J., and Loh, X. J. (2015). Nanoparticle-hydrogel composites: Concept, design, and applications of these promising, multi-functional materials. Adv. Sci. (Weinh). 2 (1-2), 1400010. doi:10.1002/advs.201400010
Urzedo, A. L., Goncalves, M. C., Nascimento, M. H. M., Lombello, C. B., Nakazato, G., and Seabra, A. B. (2020). Cytotoxicity and antibacterial activity of alginate hydrogel containing nitric oxide donor and silver nanoparticles for topical applications. ACS Biomater. Sci. Eng. 6 (4), 2117–2134. doi:10.1021/acsbiomaterials.9b01685
Venugopal, J., and Ramakrishna, S. (2005). Applications of polymer nanofibers in biomedicine and biotechnology. Appl. Biochem. Biotechnol. 125 (3), 147–158. doi:10.1385/Abab:125:3:147
Wang, C., Flynn, N. T., and Langer, R. (2004). Controlled structure and properties of thermoresponsive nanoparticle-hydrogel composites. Adv. Mat. 16 (13), 1074–1079. doi:10.1002/adma.200306516
Wang, J., Kumeria, T., Bezem, M. T., Wang, J., and Sailor, M. J. (2018). Self-reporting photoluminescent porous silicon microparticles for drug delivery. ACS Appl. Mat. Interfaces 10 (4), 3200–3209. doi:10.1021/acsami.7b09071
Wang, Y., Cui, W. G., Zhao, X., Wen, S. Z., Sun, Y. L., Han, J. M., et al. (2019). Bone remodeling-inspired dual delivery electrospun nanofibers for promoting bone regeneration. Nanoscale 11 (1), 60–71. doi:10.1039/c8nr07329e
Wang, Y. Z., Sun, L. Z., Jiang, T. Y., Zhang, J. H., Zhang, C., Sun, C. S., et al. (2014). The investigation of MCM-48-type and MCM-41-type mesoporous silica as oral solid dispersion carriers for water insoluble cilostazol. Drug Dev. Industrial Pharm. 40 (6), 819–828. doi:10.3109/03639045.2013.788013
Wu, M., Chen, J. S., Huang, W. J., Yan, B., Peng, Q. Y., Liu, J. F., et al. (2020). Injectable and self-healing nanocomposite hydrogels with ultrasensitive pH-responsiveness and tunable mechanical properties: Implications for controlled drug delivery. Biomacromolecules 21 (6), 2409–2420. doi:10.1021/acs.biomac.0c00347
Xu, L. G., Li, W., Sadeghi-Soureh, S., Amirsaadat, S., Pourpirali, R., and Alijani, S. (2021). Dual drug release mechanisms through mesoporous silica nanoparticle/electrospun nanofiber for enhanced anticancer efficiency of curcumin. J. Biomed. Mat. Res. A 110, 316–330. doi:10.1002/jbm.a.37288
Xu, Y. H., Kim, C. S., Saylor, D. M., and Koo, D. (2017). Polymer degradation and drug delivery in PLGA-based drug-polymer applications: A review of experiments and theories. J. Biomed. Mat. Res. 105 (6), 1692–1716. doi:10.1002/jbm.b.33648
Yadid, M., Feiner, R., and Dvir, T. (2019). Gold nanoparticle-integrated scaffolds for tissue engineering and regenerative medicine. Nano Lett. 19 (4), 2198–2206. doi:10.1021/acs.nanolett.9b00472
Yan, B., Boyer, J. C., Habault, D., Branda, N. R., and Zhao, Y. (2012). Near infrared light triggered release of biomacromolecules from hydrogels loaded with upconversion nanoparticles. J. Am. Chem. Soc. 134 (40), 16558–16561. doi:10.1021/ja308876j
Yang, M., Lee, S. Y., Kim, S., Koo, J. S., Seo, J. H., Jeong, D. I., et al. (2020). Selenium and dopamine-crosslinked hyaluronic acid hydrogel for chemophotothermal cancer therapy. J. Control. Release 324, 750–764. doi:10.1016/j.jconrel.2020.04.024
Yuan, Z. M., Pan, Y., Cheng, R. Y., Sheng, L. L., Wu, W., Pan, G. Q., et al. (2016). Doxorubicin-loaded mesoporous silica nanoparticle composite nanofibers for long-term adjustments of tumor apoptosis. Nanotechnology 27 (24), 245101. doi:10.1088/0957-4484/27/24/245101
Zhang, D. X., Yoshikawa, C., Welch, N. G., Pasic, P., Thissen, H., and Voelcker, N. H. (2019). Spatially controlled surface modification of porous silicon for sustained drug delivery applications. Sci. Rep. 9, 1367. doi:10.1038/s41598-018-37750-w
Zhang, F., and King, M. W. (2020). Biodegradable polymers as the pivotal player in the design of tissue engineering scaffolds. Adv. Healthc. Mat. 9 (13), 1901358. doi:10.1002/adhm.201901358
Zhang, H. B., Zhu, Y. Q., Qu, L. L., Wu, H. Y., Kong, H. X., Yang, Z., et al. (2018). Gold nanorods conjugated porous silicon nanoparticles encapsulated in calcium alginate nano hydrogels using microemulsion templates. Nano Lett. 18 (2), 1448–1453. doi:10.1021/acs.nanolett.7b05210
Zhao, P. K., Liu, H. Y., Deng, H. B., Xiao, L., Qin, C. Q., Du, Y. M., et al. (2014). A study of chitosan hydrogel with embedded mesoporous silica nanoparticles loaded by ibuprofen as a dual stimuli-responsive drug release system for surface coating of titanium implants. Colloids Surfaces B Biointerfaces 123, 657–663. doi:10.1016/j.colsurfb.2014.10.013
Zhao, Y. N., Trewyn, B. G., Slowing, I. I., and Lin, V. S. Y. (2009). Mesoporous silica nanoparticle-based double drug delivery system for glucose-responsive controlled release of insulin and cyclic AMP. J. Am. Chem. Soc. 131 (24), 8398–8400. doi:10.1021/ja901831u
Zhou, X. J., Chen, L., Wang, W. Z., Jia, Y. T., Chang, A. N., Mo, X. M., et al. (2015). Electrospun nanofibers incorporating self-decomposable silica nanoparticles as carriers for controlled delivery of anticancer drug. RSC Adv. 5 (81), 65897–65904. doi:10.1039/c5ra11830a
Zilony, N., Rosenberg, M., Holtzman, L., Schori, H., Shefi, O., and Segal, E. (2017). Prolonged controlled delivery of nerve growth factor using porous silicon nanostructures. J. Control. Release 257, 51–59. doi:10.1016/j.jconrel.2016.12.008
Zuidema, J. M., Bertucci, A., Kang, J., Sailor, M. J., and Ricci, F. (2020a). Hybrid polymer/porous silicon nanofibers for loading and sustained release of synthetic DNA-based responsive devices. Nanoscale 12 (4), 2333–2339. doi:10.1039/c9nr08474f
Zuidema, J. M., Dumont, C. M., Wang, J., Batchelor, W. M., Lu, Y. S., Kang, J., et al. (2020b). Porous silicon nanoparticles embedded in poly(lactic-co-glycolic acid) nanofiber scaffolds deliver neurotrophic payloads to enhance neuronal growth. Adv. Funct. Mat. 30 (25), 2002560. doi:10.1002/adfm.202002560
Keywords: hybrid biomaterials, inorganic nanoparticles, drug delivery, hydrogels, polymer scaffolds, silica nanoparticles, tissue engineering
Citation: Morillas-Becerill L, De Cola L and Zuidema JM (2022) Inorganic nanoparticle empowered biomaterial hybrids: Engineered payload release. Front. Nanotechnol. 4:999923. doi: 10.3389/fnano.2022.999923
Received: 21 July 2022; Accepted: 15 September 2022;
Published: 04 October 2022.
Edited by:
Jan M. Macak, University of Pardubice, CzechiaReviewed by:
Nathan Ryan Bruce Boase, Queensland University of Technology, AustraliaCopyright © 2022 Morillas-Becerill, De Cola and Zuidema. This is an open-access article distributed under the terms of the Creative Commons Attribution License (CC BY). The use, distribution or reproduction in other forums is permitted, provided the original author(s) and the copyright owner(s) are credited and that the original publication in this journal is cited, in accordance with accepted academic practice. No use, distribution or reproduction is permitted which does not comply with these terms.
*Correspondence: Jonathan M. Zuidema, am9uYXRoYW4uenVpZGVtYUBtYXJpb25lZ3JpLml0