- 1Institute of Life Sciences, Wenzhou University, Wenzhou, China
- 2Guangdong Provincial Key Laboratory of Bioengineering Medicine, Department of Cell Biology, Jinan University, Guangzhou, China
Cancer is a major cause of death worldwide, and nearly 1 in 6 deaths each year is caused by cancer. Traditional cancer treatment strategies cannot completely solve cancer recurrence and metastasis. With the development of nanotechnology, the study of nanoparticles (NPs) has gradually become a hotspot of medical research. NPs have various advantages. NPs exploit the enhanced permeability and retention (EPR) of tumour cells to achieve targeted drug delivery and can be retained in tumours long-term. NPs can be used as a powerful design platform for vaccines as well as immunization enhancers. Liposomes, as organic nanomaterials, are widely used in the preparation of nanodrugs and vaccines. Currently, most of the anticancer drugs that have been approved and entered clinical practice are prepared from lipid materials. However, the current clinical conversion rate of NPs is still extremely low, and the transition of NPs from the laboratory to clinical practice is still a substantial challenge. In this paper, we review the in vivo targeted delivery methods, material characteristics of NPs and the application of NPs in vaccine preparation. The application of nanoliposomes is also emphasized. Furthermore, the challenges and limitations of NPs are briefly discussed.
1 Introduction
1.1 Health Challenges of Tumour Therapy
Cancer is a disease caused by the uncontrolled growth of malignant cells, also known as malignant tumours. Cancer cells are characterized by strong invasiveness. Currently, cancer is a major cause of death worldwide, and nearly 1 in 6 deaths is caused by cancer each year. According to the World Cancer Report 2020, there are approximately 9.96 million cancer deaths worldwide; the common types of cancer associated with death are lung cancer (1.8 million deaths, approximately 18%), colorectal cancer (935,000 deaths, approximately 9.4%), and liver cancer (830,000 deaths, approximately 8.3%) (Liu et al., 2021) (Figure 1).
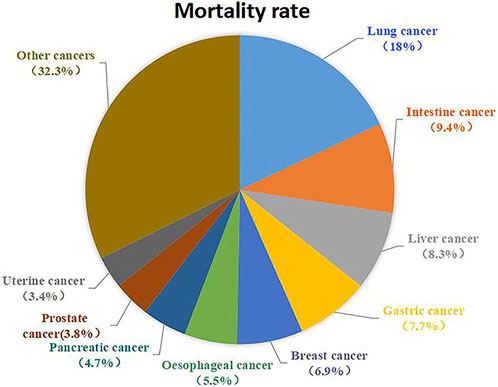
FIGURE 1. 2020 Mortality rates for different tumours (Liu et al., 2021).
Cancer has become a common public health problem worldwide. Traditional cancer treatment strategies include surgery, radiotherapy, chemotherapy, and immunotherapy. However, these methods still cannot completely prevent cancer metastasis and postoperative recurrence, which are substantial challenges in clinical practice. Additionally, common chemotherapy also has its own limitations, such as multidrug resistance (Barker et al., 2015), cardiotoxicity (Chang and Wang, 2018), and infertility (Brigden and McKenzie, 2000), among other complications, as well as poor drug selectivity against cancer. These severe side effects greatly limit the clinical treatment of cancer. Therefore, to overcome these shortcomings, it is urgent to introduce a new drug delivery platform to enhance the ability to target tumours and reduce side effects.
1.2 Application of Nanoparticles in Cancer and Vaccine
In recent years, NP drugs have gradually become a hotspot in medical research. The size of NPs is in the nanometre range (approximately less than 1 μm), and NPs generally have physical interfaces (Sheena et al., 2020). NPs are mainly divided into polymers (Rapoport, 2007), inorganic NPs (Arami et al., 2015; Wang et al., 2016) and liposomes (Tan et al., 2021). Liposomes are composed of phospholipid molecules and are less toxic than are inorganic NPs. In addition, their phospholipid bilayer structure can not only encapsulate hydrophobic drugs and hydrophilic drugs but can also simultaneously deliver 2 drugs, enabling more types of drugs to be encapsulated and greatly improving drug delivery efficiency (Xiang et al., 2012). In addition, liposomes have good biocompatibility and biodegradability.
The emergence of NPs and their development in cancer treatment have had an important impact on clinical chemotherapy. NP drug delivery platforms can address various shortcomings of traditional treatment strategies. First, NPs can improve targeted drug delivery; the structural design of NPs can be modified so that they can more effectively deliver therapeutic drugs to the tumour site, thus minimizing the toxic side effects of the drugs and adverse reactions at sites external to the target. Second, NPs can deliver higher local drug concentrations to tumour site via enhanced permeability and retention (EPR), thereby improving drug availability and drug sensitivity and overcoming the multidrug resistance of tumour cells (Maeda et al., 2000). Third, NP drug delivery platforms can be used in combination with a variety of drugs to reduce the toxic side effects of chemotherapy drugs and improve the tumour microenvironment (TME) (Zhang et al., 2020a). However, due to the heterogeneity of the EPR effect, drug delivery remains very poor (Leroux, 2017). Therefore, in-depth explorations of how NPs enter tumour tissues is the first step to better target nanodrugs to tumours and improve the clinical conversion rate.
Because of the coronavirus disease 2019 (COVID-19) pandemic, the global emphasis on vaccines has increased, and vaccine research and development technologies have rapidly improved. As a powerful development platform for vaccines, nanotechnology can be used in both therapeutic and preventive vaccines. They can be used as transport systems to enhance the function of antigen-presenting cells or as an immune enhancer to activate immune responses. In addition, NPs can play roles in drug targeting, sustained and controlled drug release.
In summary, NPs provide an important strategy and new direction for tumour treatment and vaccination. In this paper, the targeted delivery mechanisms of NPs in cells and in vivo are briefly described, and the material characteristics of NPs and the application of NPs in tumour-targeted therapy and vaccine preparation are reviewed.
2 In vivo Tumour-Targeted Delivery Mechanisms of NPs
The efficacy of antitumour drugs largely depends on whether the drugs are delivered to the correct location (Bae and Park, 2011). To achieve drug-targeted therapy, it is necessary to find effective drugs, appropriate targets, and the right mode of delivery (Danhier et al., 2010). The distribution of traditional chemotherapeutic drugs in the body is nonspecific, and the high toxicity of those drugs can cause excessive damage to normal tissues and cells. Therefore, the long-term goal of cancer treatment is to increase the healthy lifespan and mobility of patients by reducing the systemic toxicity of drugs (Byrne et al., 2008). While the characteristics of NPs meet the needs for antitumour drug delivery, the specific tumour targeting of NPs allows drugs to exhibit better pharmacokinetic characteristics and reduce systemic toxicity while improving drug specificity and increasing intracellular drug delivery (Bae, 2009). Therefore, NP drug delivery platforms have become strategies to overcome the nonspecificity of chemotherapeutic drugs (Jain and Stylianopoulos, 2010). We firstly talk about two common modes of tumour-targeted delivery as shown in Figure 2.
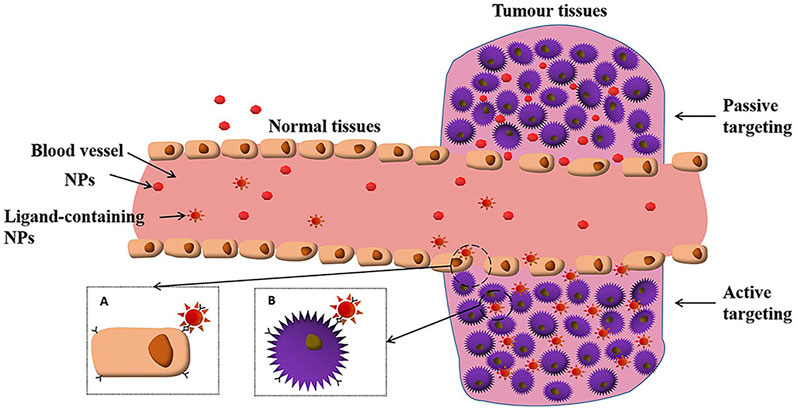
FIGURE 2. Schematic illustration of the EPR effect, Active and Passive targeting. In tumour tissues, endothelial cells are irregular and have large gaps. Therefore, NPs tend to accumulate in tumor tissues much more than in normal tissues. In active targeting, targeting ligand-modified NPs can bind to specific receptors on tumor cells or tumor endothelial cells.
2.1 Passive Targeting
Passive targeting is based on enhanced permeability and retention effect (EPR) (Matsumura and Maeda, 1986; Torchilin, 2011) The rapid growth of tumours results in large gaps in vascular endothelial cells, leading to more drugs entering tumour tissue. The imperfect lymphatic reflux function of tumour tissue results in the long-term retention of drugs at the tumour site. The EPR effect is known as the “royal gate” (Danhier et al., 2010) and is the gold standard for the design of antitumour drugs and the physiological basis for the entry and accumulation of macromolecules and small particles in tumours. The presence of NPs can not only reduce the toxic side effects of chemotherapy drugs but also enhance the EPR effect and improve the targeting ability and efficacy of drugs (Torchilin, 2007a). For example, Doxil (pegylated liposomal doxorubicin) has a drug concentration that is 10 times higher than that of free doxorubicin at the tumour site (Torchilin, 2007b).
Except for tumours with blood vessels, such as prostate cancer or pancreatic cancer, almost all fast-growing tumours exhibit the EPR effect (Maeda et al., 2001; Din et al., 2017; Fang et al., 2020a). NPs must be of a certain size to exploit the EPR effect. The size of the drug in blood circulation must be larger than the renal clearance threshold to ensure long-term circulation. Therefore, the size of the NPs must be greater than 10 nm (Maeda et al., 2009; Maeda et al., 2013). Second, the size of NPs should be smaller than the lumen size of the vasculature at the tumour site; therefore, they need to be smaller than 100 nm (Noguchi et al., 1998; Liu et al., 2018a). In-depth studies have found that NPs that are approximately 50 nm have the highest efficacy on primary and metastatic tumours (Tang et al., 2014a). In addition to size, other NP properties, such as biocompatibility and surface charge, impact the EPR effect (Kobayashi et al., 2014; Ulbrich et al., 2016).
Currently, there are many anticancer drug preparations based on the EPR effect. Among them, the earliest passive targeting drug is a polymer-conjugated drug prepared by Maeda et al., i.e., SMANCS (Maeda, 2001). This anticancer polymer was approved in 1993 by the Japanese government for the treatment of liver cancer (Maeda et al., 1985; Maeda, 1994). Despite continuous in-depth research on the EPR effect, there is still great controversy regarding the EPR effect and clinical treatment. First, Shrey et al. used mathematical models and animal models to study murine and human tumours and showed that endothelial gaps were not the reason for the entry of NPs into solid tumours. There were only 26 intercellular spaces in 313 blood vessels. The overall coverage rate was only 0.048%, and only 7 of these 26 intercellular spaces were interendothelial channels; the remaining 19 were intercellular channels. The gaps on the tumour were 60 times smaller than that required for the observed accumulation of NPs (Sindhwani et al., 2020). Second, based on a literature survey of NP delivery from 2006 to 2016, it was found that only 0.7% (median) of drug-containing NPs entered solid tumours. In addition, more than 95% of targeted NPs cannot reach tumours during intravenous administration; therefore, the current clinical efficacy cannot significantly improve (Wilhelm et al., 2016). Because the EPR effect is a dynamic phenomenon, one possible cause of this situation is the heterogeneity of the EPR effect, that is, tumour vascular occlusion or embolism, which has become one of the main obstacles to the targeting of drug-containing NPs (Gerlinger et al., 2012; Fisher et al., 2013).
2.2 Active Targeting
Active targeting refers to the binding of NPs modified with targeting ligands to specific receptors on tumour cells or the tumour endothelium on the basis of passive targeting, thereby allowing tumour-specific targeting. In the selection of targeting ligands, receptors that are expressed on all types of tumour cells should be selected, and the selected targeting receptors should only be overexpressed on tumour cells (Adams et al., 2001). Commonly used tumour cell receptors include transferrin receptor (TfR), folate receptor (FR), glycoproteins, and epidermal growth factor receptor (EGFR) (Table 1).
Active targeting plays a dominant role in the targeted delivery of NPs. Schleich et al. (Schleich et al., 2014)compared nontargeted and single-targeted NPs and found that the accumulation of NPs in the single-targeted group was more than 2.5 times higher than that in the passively targeted group. In the process of active targeting, two decisive factors can be adjusted. The first factor is the abundance of tumour surface receptors. The long-term targeted delivery of nanodrugs can lead to a reduction in gene expression or to gene mutations, which will lead to a reduction in the efficacy of targeted nanodrugs and the development of multidrug resistance (Li et al., 2017; Hayashi and Konishi, 2021). Chen et al. (Chen et al., 2021)prepared chitosan oligosaccharide (COS)-coated and sialic acid (SA) receptor-targeted nano-micelles and found that these nano-micelles inhibited tumour epithelial mesenchymal metastasis by downregulating the expression of Hypoxia Inducible Factor-1α (HIF-1α), Glutathione (GSH), Multidrug Resistance-associated Protein 2 (MRP2) and Matrix Metalloproteinase 9 (MMP9). The results showed that these nano-micelles significantly enhanced the antitumour effect in vivo and in vitro, providing an effective strategy for the treatment of drug-resistant metastatic tumours (Figures 3A–D). The second factor is the selection of targeting agents for receptors (Zhukov and Tjulandin, 2008; Ma yer, 2009). Wang et al. (Wang et al., 2021b) selected coagulation peptide (A15) as a targeting agent to generate a self-amplified tumour nanotherapy platform with a chain reaction mechanism (Figure 3E). After the administration of drugs to mice, the total CA4 concentration in A15-PLG-CA4-treated C26 tumours was 2.9 times that in the control group at 24 h, the total BLZ945 concentration (24 h) in the C26 tumours treated with A15-PLG-CA4/BLZ945 was 3.8-fold more than that in the tumours treated with A15′-PLG-CA4/BLZ945, with a significant antitumour effect.
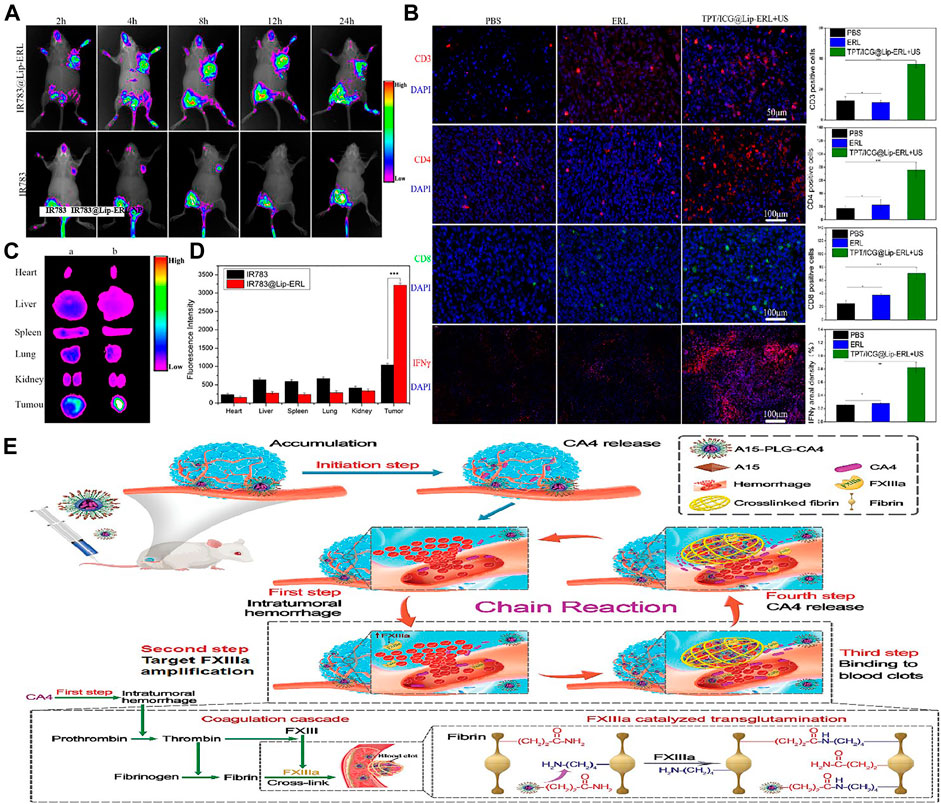
FIGURE 3. (A) Semiquantitative mean fluorescence intensity results of organs and tumours. Data are expressed as mean ± SD (n = 3). (B) In vivo images of 4T1 tumour-bearing mice administrated with free DiR or CTP/CDDP/DiR at different times. (C) Ex vivo images of organs and tumours excised from tumour-bearing nude mice at 12 h after intravenous injection of different drugs. (D) Semiquantitative mean fluorescence intensity results of organs and tumours. Data are expressed as mean ± SD (n = 3). (E) Schematic illustration of the self-amplifying tumour-homing nanotherapeutic platform, A15-PLG-CA4, characterized by chain reactions. (A,B,C,D) is reprinted with permission from reference (Chen et al., 2021). (E) is reprinted with permission from reference (Wang et al., 2021b).
2.3 Other Modes
Besides the above two common modes of tumour-targeted delivery, there are also a variety of transport modes. Transcytosis is an active vesicle-mediated delivery pathway that is often used by macromolecules to cross biological barriers. The EPR effect has long been recognized as the main factor for the entry of NPs into tumour cells (Gerlowski and Jain, 1986). However, the EPR effect has always been controversial with regard to clinical application (Danhier, 2016; Nel et al., 2017). In 2015, Anders proposed that the EPR effect is not a common feature of all solid tumours (Hansen et al., 2015). Recently, Chan et al. found that transcytosis may be the main mode of entry of NPs into tumours. The effect of transcytosis on the enrichment of NPs at tumour sites was investigated. The observation of 3 sizes of gold NPs (AuNPs) via transmission electron microscopy (TEM) provided direct evidence of transcytosis; moreover, after blocking the transcytosis pathway, only 3–25% of the total AuNPs entered tumours via the EPR effect, indicating that transcytosis may play a dominant role. However, the mechanism of transcytosis and the triggering factors remain unclear. Studies have shown that receptor-glycoprotein binding (Nel et al., 2017) and charge inversion (Schulz et al., 2019; Fang et al., 2020b) may trigger transcytosis. Therefore, it is possible that transcytosis can be used to improve the efficiency of targeted drug delivery by NPs.
Targeted drug delivery mechanisms mediated by the TME are also feasible methods. The TME is hypoxic, has a low pH, and generates an inflammatory response and immunosuppression. It contains a large number of interstitial cells and immune cells and is an environment in which tumour cells depend on for survival (Schulz et al., 2019). Smart nanodrug delivery platforms utilize the internal phenomena of the TEM [e.g., low pH (Bener et al., 2020), overexpressed enzymes (Liu et al., 2017), and hyperthermia] or external stimuli (e.g., light, heat, and magnetic fields) to control the release of drugs (Shrestha et al., 2021). pH-responsive nano-formulations use pH changes to cause conformational or solubility changes, charge reversal, and chemical bond cleavage. The control and release of drugs from the tumour environment are achieved through the acidic environment of the tumour mesenchyme combined with acid-sensitive chemical bonding, thereby facilitating the endocytosis and targeting of nano-agents (Bener et al., 2020; Lin et al., 2020). By comparing poly(ethylene glycol)-poly(benzyl-l-aspartate) (PPA) and poly-imino-poly(benzyl-l-aspartate) (PIPA) block copolymers, Pu et al. (Pu et al., 2019)found that PIPAH had a better drug release rate and antitumour effect than that of pH-insensitive PPAH because the imine bond of PIPAH utilizes the acidic condition of the TME to more effectively release the active drug.
3 Biomaterials of NPs
Nanoparticles have unique properties that can improve drug biocompatibility, reduce drug toxicity, and enter tumour sites in a specific manner. These properties are closely related to the prepared materials. The preparation materials of nanoparticles mainly include lipid materials, polymer materials and inorganic materials as show in Table 2. Next, we discuss the lipid and polymer materials.
3.1 Lipid Materials
Lipid materials are biofriendly, highly versatile, biocompatible and have low toxicity. In addition, lipid materials can reduce the adverse reactions of the body to other exogenous biological carriers. Therefore, lipid materials have always been excellent carrier materials for the preparation of drugs. Different types of lipid nanoparticles can be prepared using lipid materials as show in Figure 4 (Feeney et al., 2016).
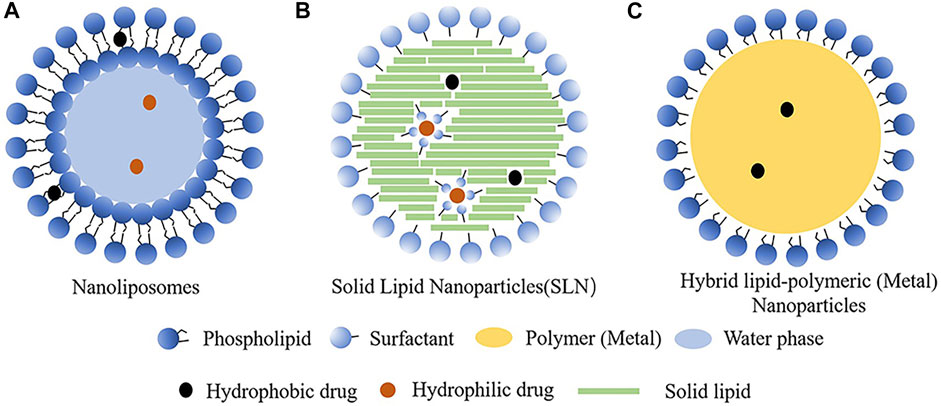
FIGURE 4. Schematic diagram of different types of lipid nanoparticles: (A) Nanoliposomes (B) SLN (C) Hybrid lipid—polymeric (metal) Nanoparticles.
Liposomes are the most common lipid-based carriers prepared from lipid materials (Plaza-Oliver et al., 2021). Currently, a variety of liposome preparations have been approved by the FDA for clinical use (Nguyen et al., 2016). Liposomes have good encapsulation capacity for water-soluble and fat-soluble drugs, crystalline drugs, biological macromolecules and are excellent drug carriers (Bangham et al., 1965). The superior properties of liposomes have led to their extensive application in anticancer treatments. First, liposomes can improve the delivery of chemotherapeutic drugs and improve the therapeutic effect of drugs. Li et al. (Li et al., 2021) prepared curcumin-loaded liposomes (Cur + Lip) that were sequentially coated with chitooligosaccharides (Cur + Lip-Cos) and negative phospholipids (Cur + Lip-Cos-PC) to improve the water solubility and encapsulation rate and thus delay the release of Cur. Second, The Lips were then fixed in an injectable thiolated chitosan hydrogel which enhanced the antitumour effect. Xu et al. (Xu et al., 2019) constructed bifunctional liposomes (DOX-ACF + Lip) that overcome chemotherapy resistance caused by hypoxia. In addition, liposomes can be modified with other ligands and functional components. The range of drug delivery of conventional liposomes has been extended by modifying them to increase their cycle time (e.g., long-cycle liposomes) (Zylberberg and Matosevic, 2016), to increase their local drug delivery concentration (e.g., liposome gel systems) (Zeng et al., 2020) or for gene delivery (e.g., cationic liposomes) (Ahmad et al., 2021; Zhao et al., 2021a). Solid lipid nanoparticles (SLNs) are solid micelle drug delivery systems made of natural or synthetic solid lipid materials as carriers and encapsulated drugs in lipid cores (Araujo et al., 2021). Compared with liposomes, SLNs exhibit better physical stability and higher drug loading capacity and bioavailability. Due to their low molecular mobility, SLNs can more accurately control the release of the drug payload in the cancer microenvironment (Tenchov et al., 2021). Kumar et al. (Pandian et al., 2021) developed and evaluated rutin-loaded SLNs for the treatment of brain tumours. The study found rutin-loaded SLNs have superior characterization for their physicochemical properties. Its biocompatibility and stability have been confirmed in vitro. At 54 h after injection, the distribution of rutin in the brain was 15.23 ± 0.32%, and prepared rutin- loaded SLNs were stable in circulation for up to 5 days. Therefore, rutin-loaded SLNs can be used as carriers for the targeting of tumours across the blood–brain barrier (BBB).
3.2 Polymer Materials
Nanopolymer materials have a wide range of applications in biomedicine. They have adjustable molecular designs, are highly stable, and have structural diversity (Zhang et al., 2019a). Nanopolymer materials are currently the mainstream nanodrug carrier. For example, the nanopolymer micellar paclitaxel (pm-Pac) provides a new option for advanced lung cancer chemotherapy. The objective remission rates (ORRs) for pm-Pac and paclitaxel treatment were shown to be 50 and 26%, respectively, and the safety analysis indicated that the incidence of serious adverse events in the pm-Pac group was lower, only half of that in the paclitaxel group (Shi et al., 2021).
Biodegradable biopolymers are one of the most important biomaterials. In recent years, the development of degradable biopolymers to replace nondegradable polymers has been a trend (Kirillova et al., 2021). NPs composed of early polymer materials exhibited rapid and effective clearance. However, due to their non-degradability, these NPs accumulated in the body, resulting in chronic toxicity and inflammatory responses. Therefore, the development of biodegradable polymer materials has received extensive recognition and attention (Anju et al., 2020). After degradable biopolymers carry drugs to specific target sites, they begin to slowly degrade into smaller nontoxic substances, ultimately being metabolized by the body. Biopolymers can be classified based on the source, i.e., natural (such as polysaccharides and chitosan) and synthetic (such as polyesters and their copolymers). Because these biodegradable biomedical polymer materials have good biosafety, they are often used in biological tissue engineering and 3D scaffolds (Maity and Cha kraborti, 2020). In addition, some biodegradable biopolymers, such as polyglycolic acid (PGA) and poly(lactic-co-glycolic acid) (PLGA), have been approved by the U.S. Food and Drug Administration (FDA) and the European Medicines Agency (EMA) as materials for drug preparation (Palma et al., 2018). For specific applications in the medical field, the functionalization of biodegradable biopolymer materials is one of the development trends in the future (Masood, 2016; Gagliardi et al., 2021).
3.3 Inorganic Materials
Inorganic nanomaterials can be easily prepared into different shapes and sizes in different tumour environments, which are good carriers for preparing tumour vaccines. Therefore, they are widely used in the biomedical field (Liu et al., 2020). However, most inorganic nanomaterials are non-biodegradable, causing direct cytotoxicity, and the aggregation of some potential inorganic nanoparticles can easily lead to vascular occlusion (Fadeel and Garcia-Bennett, 2010; Mukherjee and Patra, 2016). At the same time, inorganic nanomaterials tend to cause non-specific drug leakage, which limits the development of inorganic nanomaterials (Gorbet and Ranjan, 2020). Common inorganic nanomaterials can be mainly divided into two categories: non-metallic and metallic.
There are many kinds of metal nanomaterials. The currently developed nano-metal materials include gold, titanium, thallium, zinc, etc. (Gussone et al., 2014). Photothermal therapy (PTT) is a kind of cancer treatment with high specificity and low toxicity. Qiuhong Zhang (Zhang et al., 2020b) developed a highly efficient near-infrared photothermal agent (NIR-II PTA) based on liquid exfoliated FePS₃ nanosheets. Using the properties of iron, the prepared nanosheets showed an ultra-high specific surface area, improved the catalytic activity of Fenton, and achieved a photothermal conversion efficiency of 43.3%, while realizing cancer chemodynamic therapy (CDT). Iron-based metal nanomaterials have the potential to be a new nanotherapeutic platform.
The biosafety of non-metallic nanomaterials is better than that of metal nanomaterials. Silicon-based nanomaterials are one of the most widely used non-metallic nanomaterials. They are easy to synthesize and manipulate. They also have many unique advantages in in vivo applications, such as excellent biocompatibility, versatile surface chemistry, etc (Wang et al., 2021c). Chen Qi et al. (Chen et al., 2020) developed organic-inorganic hybrid hollow mesoporous silica nanoparticles (HMONs) as vaccine carriers, and used dopamine to modify the surface to improve the controllability of biodegradation and drug release. Molecular disulfide-bonded hybrid backbones enable stepwise degradation in a reducing tumour microenvironment. The results showed that HMONs had an effective slow-release effect, significantly inhibited the proliferation of tumour cells, and achieved anti-tumour effects in vivo through the dual-reaction release of the tumour microenvironment. In general, the nanoparticles have good application prospects in tumour vaccines.
4 Clinical Application of NPs in Tumour Therapy and Vaccines
4.1 Application of NPs in Tumour Therapy
As mentioned above, the emergence of NPs can greatly improve current cancer treatment as show in Figure 5. NPs can be used as carriers to deliver cargo to cancer cells. NPs can improve the efficacy of drugs by improving their safety, tolerability and their targeting (Almanghadim et al., 2021). In photothermal therapy, NPs can be used as carriers of photothermal agents for tumour elimination. Cheng et al. (Cheng et al., 2021) used self-designed hybrid therapeutic NPs loaded with photothermal agents; after the intravenous injection of NPs, laser irradiation was used to achieve excellent photothermal therapeutic outcomes; the results showed that the tumour was completely eliminated, immunogenic cells died, and a large number of tumour-related antigens were generated. Moreover, NPs can also be directly used as photothermal agents for tumour therapy (An et al., 2021).
In addition, NPs also play a substantial role in the early diagnosis and treatment of tumours. Currently, for tumour imaging, the distribution of imaging contrast agents and tracers in the body is not well understood, the clearance rate is fast, the pharmacokinetics are poor, and there are certain adverse reactions. NPs open a new path for cancer imaging (Xu et al., 2018). Sun et al. (Sun et al., 2014) used nanorods as substrates, and the surfaces were modified with PEG and 64Cu to successfully apply them in PET. Wang et al. (Wang et al., 2015b) generated superparamagnetic iron oxide nanoparticles (SPIONs) coated with dSiO2 as core–shell NPs and labelled them with near infrared fluorescence material and an anti-CD146 monoclonal antibody for magnetic resonance imaging. The MKN 45 xenograft tumour model can be clearly identified as early as 30 min after injection.
Paclitaxel (PTX) is currently one of the most effective drugs for the treatment of cancer. However, its availability is limited due to its low solubility and various side effects. In clinical practice the use of PTX as an NP carrier can effectively reduce the toxic effect of PTX on noncancerous cells and significantly reduce the survival rate of all cancer cells (Danışman-Kalındemirtaş et al., 2021). Currently, a variety of NP drugs have been approved for the treatment of tumours (Table 3).
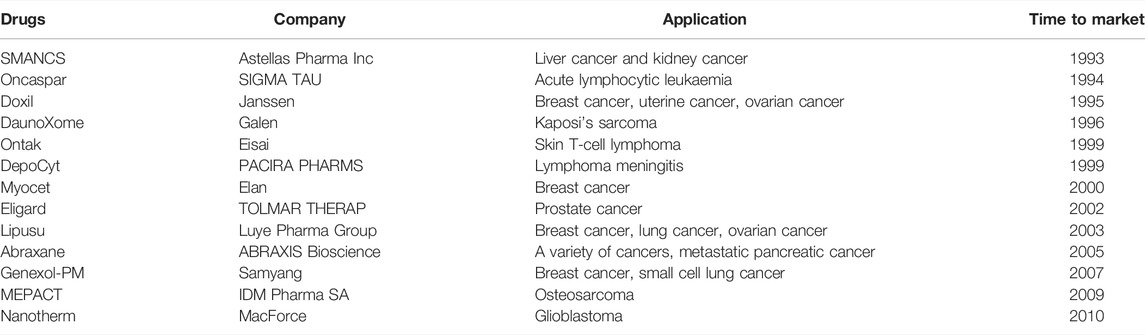
TABLE 3. Antitumour nanodrugs currently approved for marketing (Danhier, 2016; Liu et al., 2018b).
4.2 Application of NPs in Vaccines
NPs are good candidates for the preparation of vaccines. Nano-vaccines are safer and more stable and have better versatility (Mamo and Poland, 2012; Yadav et al., 2018). In the application of tumour vaccines, NPs have greatly improved the efficacy of vaccines and reduced the number of drug administrations (Sulczewski et al., 2018; Wadhwa et al., 2020). Many studies have reported the preparation of synthetic vaccines based on NPs; such vaccines have the potential to enhance the corresponding immune response (lymph node transport efficiency) and improve vaccine delivery (Fu et al., 2018; Kheirollahpour et al., 2020). Zhuang et al. (Zhuang et al., 2016) used lipid-enveloped zinc phosphate hybrid (LZnP) NPs to deliver polypeptides (TRP2180-188 and HGP10025-33) and Toll-like receptor 4 agonists. The results indicated that LZnP NPs increased the secretion of cytokines and the number of CD8+ T cells. Compared with free antigens and single peptide-loaded nano-vaccines, nano-vaccines had significant antitumour effects in the treatment and prevention of melanoma in a mouse model.
The emergence of nanomedicine has accelerated the development of vaccines. As one of the nine adjuvants approved by regulatory authorities, liposomes play an indispensable role in the preparation of vaccines (Delany et al., 2014; Dowling and Levy, 2015). Currently, there are only a few cancer vaccines on the market worldwide (such as HPV vaccines), but many cancer vaccines are already in the clinical stage (Table 4). It is believed that with the development of liposomes, liposomes will be widely used as high-quality materials in vaccine preparation.
4.2.1 Application in Traditional Vaccines
In vaccine applications, nanoliposomes can be used as carriers of immunostimulants to improve the capacity of immunostimulants (Zamani et al., 2018; Huang et al., 2021b). Zhang et al. (Zhang et al., 2019b) synthesized lipid NPs to carry imiquimod (IMQ), a toll-like receptor 7/8 (TLR 7/8) agonist, and monophosphoryl lipid A (MPLA), a TLR4 agonist. The results from the tumour treatment experiments indicated that the lipid NPs were more effective than other preparations at inhibiting tumour growth and that the loading of immune checkpoint blockade agents further enhanced the antitumour effect. In addition, lipid nanoparticles (LNPs) can also be used as carriers for drug delivery. Noh et al. (Kim et al., 2012; Noh et al., 2017) designed and synthesized immunomodulatory liposomes (denoted as “tumosomes”) that can simultaneously deliver cancer antigens and adjuvants. These “tumosomes” have 2 lipid adjuvants, namely, MPLA and DDA, which have risk signals that can be used as pathogen characteristics. Experiments have shown that “tumosomes” effectively improve antitumour immune function, antigenicity and antigen uptake efficiency. This method induces and enhances the antitumour immune response and transforms the tumour into a vaccine. In addition, the vaccine can also be used in combination with other cancer treatment methods to improve the efficacy of cancer treatment.
In addition to acting as carriers, LNPs can also be used as adjuvants to prolong the biological half-life of vaccines and the ability of antigen-presenting cells to take up antigens (Kno tigová et al., 2015). They induce the production of immune regulatory cytokines, activate inflammation, local inflammation and cell recruitment, and induce faster, more extensive and stronger immune response (Park et al., 2018). Kocabas et al. (Kocabas et al., 2020) prepared dual- adjuvant liposomes by co-encapsulating cGAMP and oligodeoxynucleotides (ODN) containing unmethylated CpG motifs (CpGODN) into sterically stabilized cationic liposomes (SSCLs). The SSCLs promoted the formation of type I and type II interferons, the dual-adjuvant liposomes enhanced the immunostimulatory properties of cGAMP and CpGODN, promoted Th1 immunity, and caused melanoma remission by approximately 70%, and the lipid preparations reversed macrophage polarization to an M1 inflammatory phenotype. Farzad et al. (Farzad et al., 2019) coupled the P435 HER2/neu-derived peptide with drugs to establish an effective nanoliposome vaccine, which can be used as an adjuvant. The liposomal P435 preparation induced IL-4 in mice, and the Lip + DOPE + P435 preparation stimulated IFN-c. The authors concluded that Lip + DOPE + P435 is a promising candidate for the development of an effective vaccine for HER2-positive breast cancer.
Cationic liposomes are often used in the development of vaccines because cationic liposomes can better interact with negatively charged ions in the cell membrane than can other types of liposomes (Thi et al., 2021). This storage effect leads to a prolonged antigen release time at the injection site, and the effective transfection of macromolecules (such as pDNA and mRNA) also requires the presence of positively charged cationic lipids (Habrant et al., 2016). Additionally, studies have found that compared with negatively charged NPs, positively charged NPs can be rapidly taken up by cells (Zaki et al., 2011). However, these cationic lipids show higher cytotoxicity than do neutral or anionic lipids; therefore, their application has certain limitations (Wei et al., 2015). To allow more drugs to be prepared into NPs and used in the preparation of vaccines, negatively charged or neutral nanoliposomes must be developed. Naomi Benne et al. (Benne et al., 2018) inoculated atherosclerotic mice with anionic DSPG-Nanoliposomes and found that anionic DSPG-liposomes can serve as a useful delivery vector to induce antigen-specific Tregs, and that empty anionic liposomes reduce plaque size and cellular content to a similar extent as injection of apoptotic cells. The ApoB100-derived peptides were then encapsulated in anionic liposomes for administration. Anionic liposomes were found to significantly reduce plaque formation by about 50% and increase plaque stability. These results indicate that anionic DSPG-Nanoliposomes have potential as a delivery system in vaccination against atherosclerosis (Figures 6A,B6).
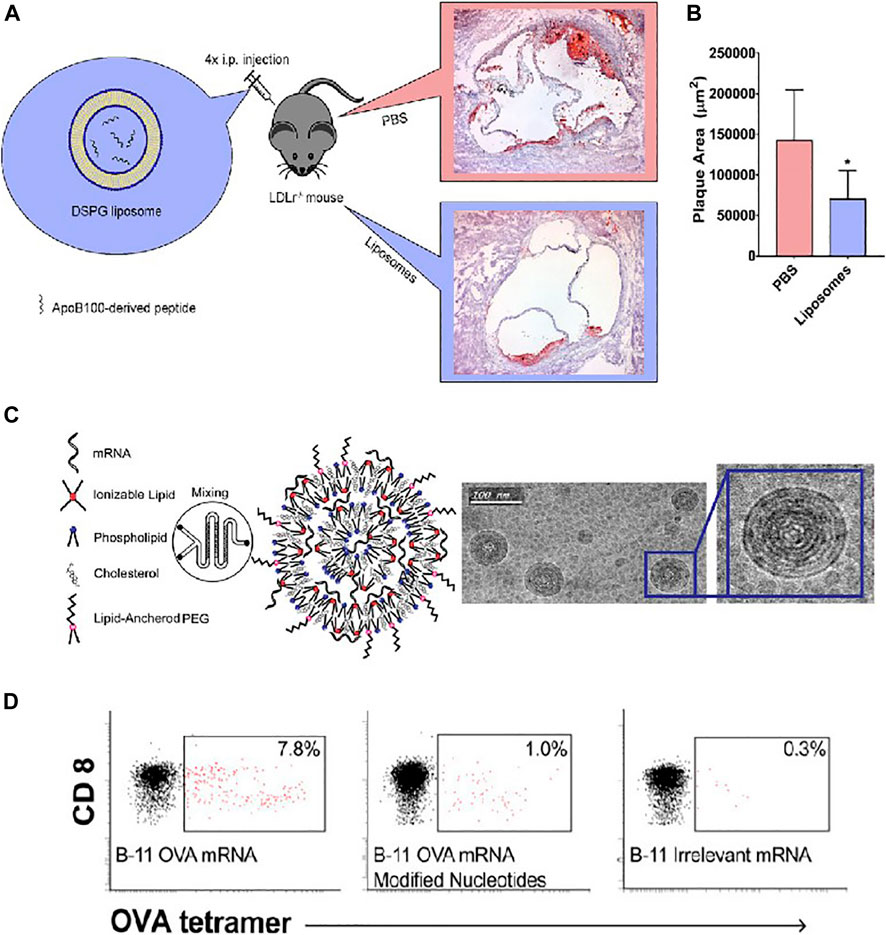
FIGURE 6. (A) Representative images of sections of the aortic valve area in a mouse receiving PBS or DSPG/p3500-liposomes. (B) Lesion area as determined by Oil-Red-O staining in a mouse receiving PBS or DSPG/p3500-liposomes. (C) “Sandwich” structure and Cryogenic transmission electron microscopy image of the LNP solution. (D) Representative FACS profiles of mice treated with the indicated conditions. (A,B) is reprinted with permission from reference. (Benne et al., 2018). (C,D) is reprinted with permission from reference (Oberli et al., 2017).
4.2.2 Application in DNA Vaccines
Currently, liposome-based nanodrug delivery systems are mainly used in the development and application of modern vaccines, of which nucleic acid vaccines are the mainstay (D’Amico et al., 2021; Alfagih et al., 2021). Nucleic acid vaccines have good stability and can be produced rapidly (Iavarone et al., 2017; Rockman et al., 2020). There are currently 2 types: DNA vaccines and RNA vaccines. DNA vaccines prevent diseases by injecting plasmids used for encoding. After the injection of plasmids, cells directly produce antigens, thereby causing a protective immune response (Gary and Weiner, 2020). Won (Youn et al., 2020) designed a DNA vaccine against HPV virus-specific antigens and found that the GX-188E vaccine induced HPV-16 E6- and E7-specific T-cell responses in patients with precancerous lesions, thereby alleviating cervical lesions. A small-scale clinical trial showed that PD-L1 antibody can treat patients with advanced refractory cervical cancer. However, due to the weak immunogenicity and short half-life of DNA vaccines, only a number of DNA vaccines are used in veterinary medicine (e.g., melanoma vaccines for dogs (Gummow et al., 2020)); no DNA vaccines are currently used in humans (Liu, 2019). As an excellent delivery system, LNPs can enhance the humoral and cellular immune responses of DNA vaccines, thereby compensating for the shortcomings of DNA vaccines and enabling their further development (Gary and Weiner, 2020). Zhao et al. (Zhao et al., 2021b) developed a novel liposome-polymer hybrid NP (pSFV-MEG/LNPs) for the delivery of multi-epitope self-replication DNA vaccines. These LNPs induced a strong humoral and cellular immune response compared with that generated with common preparations, with approximately 3.22-fold and 1.6-fold increases, respectively. The research and development (R&D) of DNA vaccines against COVID-19 has received close attention, and more than ten COVID-19 DNA vaccines are used in clinical practice worldwide. Among them, the COVID-19 DNA vaccine ZyCoV-D developed by Zydus Cadila has received emergency use authorization by the Indian drug regulatory agency, becoming the world’s first COVID-19 DNA vaccine (Abdulla et al., 2021).
4.2.3 Application in mRNA Vaccines
mRNA vaccines are based on the mRNA sequences of pathogen antigen proteins. After delivery into the body, antigen proteins are produced through translation to induce specific immune responses in the body and ultimately eliminate cancer cells (Pardi et al., 2018). mRNA vaccines have multiple advantages. First, they reduce the risk of T-cell function failure caused by persistent antigen exposure. Second, as the smallest genetic carrier, mRNA is non-infectious and does not integrate in the genome, playing its role exclusively in the cytoplasm, thereby avoiding genetic risks and increasing safety. In addition, the in vivo delivery of mRNA is fast and effective (Maruggi et al., 2019). Compared with traditional vaccines, mRNA vaccines can simultaneously deliver multiple antigens and immunomodulators, and the manufacturing process is simple, fast and inexpensive (Sahin et al., 2014); Compared with DNA vaccines, mRNA vaccines have faster action and good efficacy (Maruggi et al., 2019; Teo, 2021). However, mRNA vaccines are unstable in the body and have strict transport conditions, which limits their use (Guevara et al., 2019). The encapsulation of mRNA vaccines in LNPs greatly improves their stability and plays a key role in the transport of mRNAs to cells (Guan and Rosenecker, 2017; Hajj and Whitehead, 2017). Oberli et al. (Oberli et al., 2017) reported an LNP for the delivery of mRNA vaccines. They treated B16F10 melanoma with LNPs containing mRNA coding for the tumour-associated antigens gp100 and TRP2, resulting in tumour shrinkage and in an extension of overall survival in treated mice. They concluded that LNPs are a promising mRNA vaccine vector capable of inducing a strong cytotoxic T-cell response (Figure 6C,D).
Due to the COVID-19 pandemic, mRNA vaccines have attracted the attention of many pharmaceutical companies, such as Pfizer and Moderna. The FDA has issued emergency use authorizations for 2 mRNA vaccines. The efficacies of Pfizer/BioNTech’s BNT12b2 and Modern’s mRNA-1273 were 95 and 94.1%, respectively (Teo, 2021). However, the original intention of mRNA vaccine innovation was to develop anticancer vaccines. Moderna has released its new cancer vaccine mRNA-4157, which is a personalized cancer vaccine based on lipid encapsulation. A clinical trial found that in 10 patients with advanced brain cancer, the total remission rate was 50% and the disease control rate (DCR) was up to 90% (Bauman et al., 2020). In addition, the injectable liposome formulated mRNA vaccine BNT111 developed by Pfizer/BioNTech for melanoma tumours has achieved good efficacy and good safety. This vaccine can control cancer by enhancing the immune system and improving immune targeting. This is an effective immunotherapy for patients with melanoma. It is also the world’s first mRNA vaccine for tumours (Sahin et al., 2020).
5 Conclusion and Discussion
In this paper, we reviewed the in vivo targeted delivery mechanisms, application evolution of NPs. NPs provide a novel drug delivery platform for tumour drugs and can improve the efficiency of tumour treatment and reduce the side effects of tumour treatment. However, the mechanisms by which NPs enter tumours are still unclear, and further studies are needed. In the future, the focus of research on NPs should shift from the design of their structures to targeting, so that more NP drugs can be applied in clinical practice.
In recent years, the rapid development of nanotechnology has made NPs not only a hotspot for tumour treatment but also a hotspot for vaccine preparation. The focus of future research on tumour vaccines should be on safety and efficacy in tumour treatment and prevention. In the preparation of tumour vaccines, common preparation issues include the insufficient delivery of antigens and the insufficient release of drugs, limiting the development of vaccines. NPs are safe, can be modified for the controlled release of cargo and for targeting, and have a high antigen uptake rate and strong immunogenicity. Therefore, they are ideal carriers for the preparation of tumour vaccines. Currently, the nanodrugs available on the market are mainly liposomes and polymer micelle preparations. Compared with other NPs, nanoliposomes have better biocompatibility and biodegradability. However, the application of nanoliposomes in vaccines is still at an early stage of development and has certain limitations. The development of noncationic nanoliposomes, the mechanism of nanoliposome delivery systems, and the safety of vaccines all require further in-depth studies. We believe that when these remaining problems are properly addressed, NPs will transition from the laboratory to clinical application, launching a new era in cancer treatment.
Author Contributions
SL and ZS contributed to the conception of this review. YT, ZS, and SL analyzed literatures and wrote the manuscript. YT, ZY, and ST completed figures drawing. YT, WY, YW, SL, and ZS revised the manuscript. All authors have read and agreed to the published version of the manuscript.
Funding
This work was financially supported by Zhejiang Provincial Natural Science Foundation of China under Grant No. LGF21H040001, Wenzhou City Public Welfare Science and Technology Project (ZY2019005), The Graduate Scientific Research Foundation of Wenzhou University.
Conflict of Interest
The authors declare that the research was conducted in the absence of any commercial or financial relationships that could be construed as a potential conflict of interest.
Publisher’s Note
All claims expressed in this article are solely those of the authors and do not necessarily represent those of their affiliated organizations, or those of the publisher, the editors and the reviewers. Any product that may be evaluated in this article, or claim that may be made by its manufacturer, is not guaranteed or endorsed by the publisher.
References
Abdulla, Z. A., Al-Bashir, S. M., Al-Salih, N. S., Aldamen, A. A., and Abdulazeez, M. Z. (2021). A Summary of the SARS-CoV-2 Vaccines and Technologies Available or under Development. Pathogens 10 (7), 788. doi:10.3390/pathogens10070788
Adams, G. P., Schier, R., McCall, A. M., Simmons, H. H., Horak, E. M., Alpaugh, R. K., et al. (2001). High Affinity Restricts the Localization and Tumor Penetration of Single-Chain Fv Antibody Molecules. Cancer Res. 61 (12), 4750–4755.
Ahmad, M. Z., Ahmad, J., Alasmary, M. Y., Abdel-Wahab, B. A., Warsi, M. H., Haque, A., et al. (2021). Emerging Advances in Cationic Liposomal Cancer Nanovaccines: Opportunities and Challenges. Immunotherapy 13 (6), 491–507. doi:10.2217/imt-2020-0258
Alfagih, I. M., Aldosari, B., AlQuadeib, B., Almurshedi, A., and Alfagih, M. M. (2021). Nanoparticles as Adjuvants and Nanodelivery Systems for mRNA-Based Vaccines. Pharmaceutics 13 (1), 45. doi:10.3390/pharmaceutics13010045
Almanghadim, H. G., Nourollahzadeh, Z., Khademi, N. S., Tezerjani, M. D., Sehrig, F. Z., Estelami, N., et al. (2021). Application of Nanoparticles in Cancer Therapy with an Emphasis on Cell Cycle. Cell Biol. Int. 45 (10), 1989–1998. doi:10.1002/cbin.11658
An, D., Fu, J., Zhang, B., Xie, N., Nie, G., Ågren, H., et al. (2021). NIR‐II Responsive Inorganic 2D Nanomaterials for Cancer Photothermal Therapy: Recent Advances and Future Challenges. Adv. Funct. Mater. 31 (32), 2101625. doi:10.1002/adfm.202101625
Anju, S., Prajitha, N., Sukanya, V. S., and Mohanan, P. V. (2020). Complicity of Degradable Polymers in Health-Care Applications. Mater. Today Chem. 16, 100236. doi:10.1016/j.mtchem.2019.100236
Arami, H., Khandhar, A., Liggitt, D., and Krishnan, K. M. (2015). In Vivo delivery, Pharmacokinetics, Biodistribution and Toxicity of Iron Oxide Nanoparticles. Chem. Soc. Rev. 44 (23), 8576–8607. doi:10.1039/C5CS00541H
Araujo, V. H. S., Delello Di Filippo, L., Duarte, J. L., Spósito, L., Camargo, B. A. F. d., da Silva, P. B., et al. (2021). Exploiting Solid Lipid Nanoparticles and Nanostructured Lipid Carriers for Drug Delivery against Cutaneous Fungal Infections. Crit. Rev. Microbiol. 47 (1), 79–90. doi:10.1080/1040841X.2020.1843399
Bae, Y. H. (2009). Drug Targeting and Tumor Heterogeneity. J. Control. Release 133 (1), 2–3. doi:10.1016/j.jconrel.2008.09.074
Bae, Y. H., and Park, K. (2011). Targeted Drug Delivery to Tumors: Myths, Reality and Possibility. J. Control. Release 153 (3), 198–205. doi:10.1016/j.jconrel.2011.06.001
Bangham, A. D., Standish, M. M., and Watkins, J. C. (1965). Diffusion of Univalent Ions across the Lamellae of Swollen Phospholipids. J. Mol. Biol. 13 (1), 238–IN27. doi:10.1016/S0022-2836(65)80093-6
Barker, H. E., Paget, J. T. E., Khan, A. A., and Harrington, K. J. (2015). The Tumour Microenvironment after Radiotherapy: Mechanisms of Resistance and Recurrence. Nat. Rev. Cancer 15 (7), 409–425. doi:10.1038/nrc3958
Bauman, J., Burris, H., Clarke, J., Patel, M., Cho, D., Gutierrez, M., et al. (2020). 798 Safety, Tolerability, and Immunogenicity of mRNA-4157 in Combination with Pembrolizumab in Subjects with Unresectable Solid Tumors (KEYNOTE-603): an Update. J. Immunother. Cancer 8 (Suppl. 3), A477. doi:10.1136/jitc-2020-SITC2020.0798
Bener, S., Puglisi, A., and Yagci, Y. (2020). pH‐Responsive Micelle‐Forming Amphiphilic Triblock Copolymers. Macromol. Chem. Phys. 221 (11), 2000109. doi:10.1002/macp.202000109
Benne, N., van Duijn, J., Lozano Vigario, F., Leboux, R. J. T., van Veelen, P., Kuiper, J., et al. (2018). Anionic 1,2-Distearoyl-Sn-Glycero-3-Phosphoglycerol (DSPG) Liposomes Induce Antigen-specific Regulatory T Cells and Prevent Atherosclerosis in Mice. J. Control. Release 291, 135–146. doi:10.1016/j.jconrel.2018.10.028
Berry, J. S., Trappey, A. F., Sears, A. K., Vreeland, T. J., Clifton, G. T., Hale, D. F., et al. (2013). Biomarker Correlation to Clinical Response in Phase I/II Trials of the Adjuvant Breast Cancer Vaccine Neuvax (Nelipepimut-S or E75). Jco 31 (15_Suppl. l), TPS3126. doi:10.1200/jco.2013.31.15_suppl.tps3126
Bonello, F., D’Agostino, M., Moscvin, M., Cerrato, C., Boccadoro, M., and Gay, F. (2018). CD38 as an Immunotherapeutic Target in Multiple Myeloma. Expert Opin. Biol. Ther. 18 (12), 1209–1221. doi:10.1080/14712598.2018.1544240
Bota, D., Piccioni, D., Duma, C., LaRocca, R., Kesari, S., Abedi, M., et al. (2021). 952 Phase II Trial of AV-GBM-1: Dendritic Cell Vaccine Pulsed with Lysate Enriched for Autologous Tumor-Initiating Cell Antigens in the Treatment of Patients with Newly Diagnosed Glioblastoma. J. Immunother. Cancer 9 (Suppl. 2), A1001. doi:10.1136/jitc-2021-SITC2021.952
Brigden, M., and McKenzie, M. (2000). Treating Cancer Patients. Practical Monitoring and Management of Therapy-Related Complications. Can. Fam. Physician 46, 2258–2268.
Budd, G. T., Johnson, J. M., Esakov Rhoades, E., Moore, H. C. F., Kruse, M. L., Roesch, E. E., et al. (2022). Phase I Trial of an Alpha-Lactalbumin Vaccine in Patients with Moderate- to High-Risk Operable Triple-Negative Breast Cancer (TNBC). Jco 40 (16_Suppl. l), TPS1125. doi:10.1200/JCO.2022.40.16_suppl.TPS1125
Butts, C., Maksymiuk, A., Goss, G., Soulières, D., Marshall, E., Cormier, Y., et al. (2011). Updated Survival Analysis in Patients with Stage IIIB or IV Non-small-cell Lung Cancer Receiving BLP25 Liposome Vaccine (L-BLP25): Phase IIB Randomized, Multicenter, Open-Label Trial. J. Cancer Res. Clin. Oncol. 137 (9), 1337–1342. doi:10.1007/s00432-011-1003-3
Byrne, J. D., Betancourt, T., and Brannon-Peppas, L. (2008). Active Targeting Schemes for Nanoparticle Systems in Cancer Therapeutics. Adv. Drug Deliv. Rev. 60 (15), 1615–1626. doi:10.1016/j.addr.2008.08.005
Cai, J., Fu, J., Li, R., Zhang, F., Ling, G., and Zhang, P. (2019). A Potential Carrier for Anti-tumor Targeted Delivery-Hyaluronic Acid Nanoparticles. Carbohydr. Polym. 208, 356–364. doi:10.1016/j.carbpol.2018.12.074
Cappuzzo, F., Giulia, P., Angelo, D., Lorenza, L., Beatrice, B., Giulio, M., et al. (2022). Combi-TED: A Multicenter, Phase II, Open-Label, Randomized Trial Evaluating Efficacy of OSE2021 Plus Docetaxel or OSE2021 Plus Nivolumab as Second-Line Therapy in Metastatic NSCLC Progressing after First-Line Chemo-Immunotherapy. J. Clin. Oncol. 40 (16_Suppl. l), TPS9140. doi:10.1200/JCO.2022.40.16_suppl.TPS9140
Chang, V. Y., and Wang, J. J. (2018). Pharmacogenetics of Chemotherapy-Induced Cardiotoxicity. Curr. Oncol. Rep. 20 (7), 52. doi:10.1007/s11912-018-0696-8
Chen, Q., Chen, Y., Zhang, W., Huang, Q., Hu, M., Peng, D., et al. (2020). Acidity and Glutathione Dual‐Responsive Polydopamine‐Coated Organic‐Inorganic Hybrid Hollow Mesoporous Silica Nanoparticles for Controlled Drug Delivery. ChemMedChem 15 (20), 1940–1946. doi:10.1002/cmdc.202000263
Chen, Y., Fang, L., Zhou, W., Chang, J., Zhang, X., He, C., et al. (2021). Nitric Oxide-Releasing Micelles with Intelligent Targeting for Enhanced Anti-tumor Effect of Cisplatin in Hypoxia. J. Nanobiotechnol 19 (1), 246. doi:10.1186/s12951-021-00989-z
Cheng, L., Zhang, X., Tang, J., Lv, Q., and Liu, J. (2021). Gene-engineered Exosomes-Thermosensitive Liposomes Hybrid Nanovesicles by the Blockade of CD47 Signal for Combined Photothermal Therapy and Cancer Immunotherapy. Biomaterials 275, 120964. doi:10.1016/j.biomaterials.2021.120964
Cheng, Y., and Tian, H. (2017). Current Development Status of MEK Inhibitors. Molecules 22 (10), 1551. doi:10.3390/molecules22101551
Cini, E., Faltoni, V., Petricci, E., Taddei, M., Salvini, L., Giannini, G., et al. (2018). Antibody Drug Conjugates (ADCs) Charged with HDAC Inhibitor for Targeted Epigenetic Modulation. Chem. Sci. 9 (31), 6490–6496. doi:10.1039/C7SC05266A
Corroyer-Dulmont, A., Valable, S., Falzone, N., Frelin-Labalme, A.-M., Tietz, O., Toutain, J., et al. (2020). VCAM-1 Targeted Alpha-Particle Therapy for Early Brain Metastases. Neuro Oncol. 22 (3), 357–368. doi:10.1093/neuonc/noz169
CowmanCowman, S. J., and Koh, M. Y. (2022). Revisiting the HIF Switch in the Tumor and its Immune Microenvironment. Trends Cancer 8 (1), 28–42. doi:10.1016/j.trecan.2021.10.004
D’Amico, C., Fontana, F., Cheng, R., and Santos, H. A. (2021). Development of Vaccine Formulations: Past, Present, and Future. Drug Deliv. Transl. Res. 11 (2), 353–372. doi:10.1007/s13346-021-00924-7
Danhier, F., Feron, O., and Préat, V. (2010). To Exploit the Tumor Microenvironment: Passive and Active Tumor Targeting of Nanocarriers for Anti-cancer Drug Delivery. J. Control. Release 148 (2), 135–146. doi:10.1016/j.jconrel.2010.08.027
Danhier, F. (2016). To Exploit the Tumor Microenvironment: Since the EPR Effect Fails in the Clinic, what Is the Future of Nanomedicine? J. Control. Release 244, 108–121. doi:10.1016/j.jconrel.2016.11.015
Danışman-Kalındemirtaş, F., Kari̇per, İ. A., Hepokur, C., and Erdem-Kuruca, S. (2021). Selective Cytotoxicity of Paclitaxel Bonded Silver Nanoparticle on Different Cancer Cells. J. Drug Deliv. Sci. Technol. 61, 102265. doi:10.1016/j.jddst.2020.102265
Das, A., and Ali, N. (2021). Nanovaccine: an Emerging Strategy. Expert Rev. Vaccines 20 (10), 1273–1290. doi:10.1080/14760584.2021.1984890
Delany, I., Rappuoli, R., and De Gregorio, E. (2014). Vaccines for the 21st Century. EMBO Mol. Med. 6 (6), 708–720. doi:10.1002/emmm.201403876
Deng, X., Klussmann, S., Wu, G.-M., Akkerman, D., Zhu, Y.-Q., Liu, Y., et al. (2008). Effect of LHRH-PE40 on Target Cells via LHRH Receptors. J. Drug Target. 16 (5), 379–388. doi:10.1080/10611860802102324
Din, F. U., Aman, W., Ullah, I., Qureshi, O. S., Mustapha, O., Shafique, S., et al. (2017). Effective Use of Nanocarriers as Drug Delivery Systems for the Treatment of Selected Tumors. Ijn 12, 7291–7309. doi:10.2147/ijn.S146315
Doñate, F., Raitano, A., Morrison, K., An, Z., Capo, L., Aviña, H., et al. (2016). AGS16F Is a Novel Antibody Drug Conjugate Directed against ENPP3 for the Treatment of Renal Cell Carcinoma. Clin. Cancer Res. 22 (8), 1989–1999. doi:10.1158/1078-0432.Ccr-15-1542
Dowling, D. J., and Levy, O. (2015). Pediatric Vaccine Adjuvants. Pediatr. Infect. Dis. J. 34 (12), 1395–1398. doi:10.1097/inf.0000000000000893
Esnault, C., Leblond, V., Martin, C., Desgranges, A., Baltus, C. B., Aubrey, N., et al. (2022). Adcitmer , a New CD56‐targeting Monomethyl Auristatin E‐conjugated Antibody, Is a Potential Therapeutic Approach in Merkel Cell Carcinoma*. Br. J. Dermatol 186 (2), 295–306. doi:10.1111/bjd.20770
Fadeel, B., and Garcia-Bennett, A. E. (2010). Better Safe Than Sorry: Understanding the Toxicological Properties of Inorganic Nanoparticles Manufactured for Biomedical Applications. Adv. Drug Deliv. Rev. 62 (3), 362–374. doi:10.1016/j.addr.2009.11.008
Fang, J., Islam, W., and Maeda, H. (2020). Exploiting the Dynamics of the EPR Effect and Strategies to Improve the Therapeutic Effects of Nanomedicines by Using EPR Effect Enhancers. Adv. Drug Deliv. Rev. 157, 142–160. doi:10.1016/j.addr.2020.06.005
Fang, Z., Pan, S., Gao, P., Sheng, H., Li, L., Shi, L., et al. (2020). Stimuli-responsive Charge-Reversal Nano Drug Delivery System: The Promising Targeted Carriers for Tumor Therapy. Int. J. Pharm. 575, 118841. doi:10.1016/j.ijpharm.2019.118841
Farzad, N., Barati, N., Momtazi-Borojeni, A. A., Yazdani, M., Arab, A., Razazan, A., et al. (2019). P435 HER2/neu-Derived Peptide Conjugated to Liposomes Containing DOPE as an Effective Prophylactic Vaccine Formulation for Breast Cancer. Artif. Cells, Nanomedicine, Biotechnol. 47 (1), 664–672. doi:10.1080/21691401.2019.1576702
Feeney, O. M., Crum, M. F., McEvoy, C. L., Trevaskis, N. L., Williams, H. D., Pouton, C. W., et al. (2016). 50 Years of Oral Lipid-Based Formulations: Provenance, Progress and Future Perspectives. Adv. Drug Deliv. Rev. 101, 167–194. doi:10.1016/j.addr.2016.04.007
Fisher, R., Pusztai, L., and Swanton, C. (2013). Cancer Heterogeneity: Implications for Targeted Therapeutics. Br. J. Cancer 108 (3), 479–485. doi:10.1038/bjc.2012.581
Fu, B., Huang, X., Deng, J., Gu, D., Mei, Q., Deng, M., et al. (2018). Application of Multifunctional Nanomaterials in Cancer Vaccines (Review). Oncol. Rep. 39 (3), 893–900. doi:10.3892/or.2018.6206
Gagliardi, A., Giuliano, E., Venkateswararao, E., Fresta, M., Bulotta, S., Awasthi, V., et al. (2021). Biodegradable Polymeric Nanoparticles for Drug Delivery to Solid Tumors. Front. Pharmacol. 12. doi:10.3389/fphar.2021.601626
Gary, E. N., and Weiner, D. B. (2020). DNA Vaccines: Prime Time Is Now. Curr. Opin. Immunol. 65, 21–27. doi:10.1016/j.coi.2020.01.006
Gerlinger, M., Rowan, A. J., Horswell, S., Larkin, J., Endesfelder, D., Gronroos, E., et al. (2012). Intratumor Heterogeneity and Branched Evolution Revealed by Multiregion Sequencing. N. Engl. J. Med. 366 (10), 883–892. doi:10.1056/NEJMoa1113205
Gerlowski, L. E., and Jain, R. K. (1986). Microvascular Permeability of Normal and Neoplastic Tissues. Microvasc. Res. 31 (3), 288–305. doi:10.1016/0026-2862(86)90018-X
Gopalakrishnan, K., Venkatesan, S., Low, E. S. H., and Hande, M. P. (2018). Effects of Rapamycin on the Mechanistic Target of Rapamycin (mTOR) Pathway and Telomerase in Breast Cancer Cells. Mutat. Research/Genetic Toxicol. Environ. Mutagen. 836, 103–113. doi:10.1016/j.mrgentox.2018.03.008
Gorbet, M.-J., and Ranjan, A. (2020). Cancer Immunotherapy with Immunoadjuvants, Nanoparticles, and Checkpoint Inhibitors: Recent Progress and Challenges in Treatment and Tracking Response to Immunotherapy. Pharmacol. Ther. 207, 107456. doi:10.1016/j.pharmthera.2019.107456
Gou, W., Li, Z., Xu, X., Shen, J., Guo, M., Zhou, X., et al. (2020). ZX-29, a Novel ALK Inhibitor, Induces Apoptosis via ER Stress in ALK Rearrangement NSCLC Cells and Overcomes Cell Resistance Caused by an ALK Mutation. Biochimica Biophysica Acta (BBA) - Mol. Cell Res. 1867 (7), 118712. doi:10.1016/j.bbamcr.2020.118712
Graziadio, A., Zanda, M., Frau, S., Fleming, I. N., Musolino, M., Dall’Angelo, S., et al. (2016). NGR Tumor-Homing Peptides: Structural Requirements for Effective APN (CD13) Targeting. Bioconjugate Chem. 27 (5), 1332–1340. doi:10.1021/acs.bioconjchem.6b00136
Guan, S., and Rosenecker, J. (2017). Nanotechnologies in Delivery of mRNA Therapeutics Using Nonviral Vector-Based Delivery Systems. Gene Ther. 24 (3), 133–143. doi:10.1038/gt.2017.5
Guevara, M. L., Persano, S., and Persano, F. (2019). Lipid-Based Vectors for Therapeutic mRNA-Based Anti-cancer Vaccines. Cpd 25 (13), 1443–1454. doi:10.2174/1381612825666190619150221
Gummow, J., Masavuli, M. G., Mekonnen, Z. A., Li, Y., Wijesundara, D. K., Shrestha, A. C., et al. (2020). Safety Profile of a Multi-Antigenic DNA Vaccine against Hepatitis C Virus. Vaccines 8 (1), 53. doi:10.3390/vaccines8010053
Gussone, J., Reinhard, C., Kasperovich, G., Gherekhloo, H., Merzouk, T., and Hausmann, J. (2014). In-situ Investigation of Microcrack Formation and Strains in Ag-Cu-Based Multi-Metal Matrix Composites Analysed by Synchrotron Radiation. Mater. Sci. Eng. A 612, 102–114. doi:10.1016/j.msea.2014.06.018
Habrant, D., Peuziat, P., Colombani, T., Dallet, L., Gehin, J., Goudeau, E., et al. (2016). Design of Ionizable Lipids to Overcome the Limiting Step of Endosomal Escape: Application in the Intracellular Delivery of mRNA, DNA, and siRNA. J. Med. Chem. 59 (7), 3046–3062. doi:10.1021/acs.jmedchem.5b01679
Hajj, K. A., and Whitehead, K. A. (2017). Tools for Translation: Non-viral Materials for Therapeutic mRNA Delivery. Nat. Rev. Mater 2 (10), 17056. doi:10.1038/natrevmats.2017.56
Hansen, A. E., Petersen, A. L., Henriksen, J. R., Boerresen, B., Rasmussen, P., Elema, D. R., et al. (2015). Positron Emission Tomography Based Elucidation of the Enhanced Permeability and Retention Effect in Dogs with Cancer Using Copper-64 Liposomes. ACS Nano 9 (7), 6985–6995. doi:10.1021/acsnano.5b01324
Hayashi, T., and Konishi, I. (2021). Correlation of Anti-tumour Drug Resistance with Epigenetic Regulation. Br. J. Cancer 124 (4), 681–682. doi:10.1038/s41416-020-01183-y
Huang, H., Liu, Y., Ouyang, X., Wang, H., and Zhang, Y. (2020). Identification of a Peptide Targeting CD56. Immunobiology 225 (4), 151982. doi:10.1016/j.imbio.2020.151982
Huang, J., Yuen, D., Mintern, J. D., and Johnston, A. P. R. (2021). Opportunities for Innovation: Building on the Success of Lipid Nanoparticle Vaccines. Curr. Opin. Colloid & Interface Sci. 55, 101468. doi:10.1016/j.cocis.2021.101468
Huang, M.-Y., Jiang, X.-M., Wang, B.-L., Sun, Y., and Lu, J.-J. (2021). Combination Therapy with PD-1/pd-L1 Blockade in Non-small Cell Lung Cancer: Strategies and Mechanisms. Pharmacol. Ther. 219, 107694. doi:10.1016/j.pharmthera.2020.107694
Hubbard, J. M., Cremolini, C., Graham, R. P., Moretto, R., Mitchelll, J. L., Wessling, J., et al. (2019). A Phase I Study of PolyPEPI1018 Vaccine Plus Maintenance Therapy in Patients with Metastatic Colorectal Cancer with a Predictive Biomarker (OBERTO). Jco 37 (15_Suppl. l), 3557. doi:10.1200/JCO.2019.37.15_suppl.3557
Iavarone, C., O’hagan, D. T., Yu, D., Delahaye, N. F., and Ulmer, J. B. (2017). Mechanism of Action of mRNA-Based Vaccines. Expert Rev. Vaccines 16 (9), 871–881. doi:10.1080/14760584.2017.1355245
Ikeda, M., Yamaguchi, M., Kato, K., Nakamura, K., Shiina, S., Ichikawa-Ando, T., et al. (2015). Pr1E11, a Novel Anti-TROP-2 Antibody Isolated by Adenovirus-Based Antibody Screening, Recognizes a Unique Epitope. Biochem. Biophysical Res. Commun. 458 (4), 877–882. doi:10.1016/j.bbrc.2015.02.051
Jain, A. K., Kumar, S., Choy, G. S., Hollemon, D., and Mittendorf, E. A. (2015). Analytical Validation of Bond Oracle HER2 IHC System for Identifying Low to Intermediate HER2-Expressing Breast Cancer in NeuVax PRESENT Phase III Clinical Trial. Jco 33 (15_Suppl. l), e11609. doi:10.1200/jco.2015.33.15_suppl.e11609
Jain, R. K., and Stylianopoulos, T. (2010). Delivering Nanomedicine to Solid Tumors. Nat. Rev. Clin. Oncol. 7 (11), 653–664. doi:10.1038/nrclinonc.2010.139
Jiang, L., Yuan, C. M., Hubacheck, J., Janik, J. E., Wilson, W., Morris, J. C., et al. (2009). Variable CD52 Expression in Mature T Cell and NK Cell Malignancies: Implications for Alemtuzumab Therapy. Br. J. Haematol. 145 (2), 173–179. doi:10.1111/j.1365-2141.2009.07606.x
Kaufman, N. E. M., Dhingra, S., Jois, S. D., and Vicente, M. D. G. H. (2021). Molecular Targeting of Epidermal Growth Factor Receptor (EGFR) and Vascular Endothelial Growth Factor Receptor (VEGFR). Molecules 26 (4), 1076. doi:10.3390/molecules26041076
Kheirollahpour, M., Mehrabi, M., Dounighi, N. M., Mohammadi, M., and Masoudi, A. (2020). Nanoparticles and Vaccine Development. Pnt 8 (1), 6–21. doi:10.2174/2211738507666191024162042
Kikuchi, J., Hori, M., Iha, H., Toyama-Sorimachi, N., Hagiwara, S., Kuroda, Y., et al. (2020). Soluble SLAMF7 Promotes the Growth of Myeloma Cells via Homophilic Interaction with Surface SLAMF7. Leukemia 34 (1), 180–195. doi:10.1038/s41375-019-0525-6
Kim, J. H., Noh, Y.-W., Heo, M. B., Cho, M. Y., and Lim, Y. T. (2012). Multifunctional Hybrid Nanoconjugates for Efficient In Vivo Delivery of Immunomodulating Oligonucleotides and Enhanced Antitumor Immunity. Angew. Chem. Int. Ed. 51 (38), 9670–9673. doi:10.1002/anie.201204989
Kirillova, A., Yeazel, T. R., Asheghali, D., Petersen, S. R., Dort, S., Gall, K., et al. (2021). Fabrication of Biomedical Scaffolds Using Biodegradable Polymers. Chem. Rev. 121 (18), 11238–11304. doi:10.1021/acs.chemrev.0c01200
Knotigová, P. T., Zyka, D., Mašek, J., Kovalová, A., Křupka, M., Bartheldyová, E., et al. (2015). Molecular Adjuvants Based on Nonpyrogenic Lipophilic Derivatives of norAbuMDP/GMDP Formulated in Nanoliposomes: Stimulation of Innate and Adaptive Immunity. Pharm. Res. 32 (4), 1186–1199. doi:10.1007/s11095-014-1516-y
Kobayashi, H., Watanabe, R., and Choyke, P. L. (2014). Improving Conventional Enhanced Permeability and Retention (EPR) Effects; what Is the Appropriate Target? Theranostics 4 (1), 81–89. doi:10.7150/thno.7193
Kocabas, B. B., Almacioglu, K., Bulut, E. A., Gucluler, G., Tincer, G., Bayik, D., et al. (2020). Dual-adjuvant Effect of pH-Sensitive Liposomes Loaded with STING and TLR9 Agonists Regress Tumor Development by Enhancing Th1 Immune Response. J. Control. Release 328, 587–595. doi:10.1016/j.jconrel.2020.09.040
Kumar, P., Huo, P., and Liu, B. (2019). Formulation Strategies for Folate-Targeted Liposomes and Their Biomedical Applications. Pharmaceutics 11 (8), 381. doi:10.3390/pharmaceutics11080381
Leroux, J.-C. (2017). Editorial: Drug Delivery: Too Much Complexity, Not Enough Reproducibility? Angew. Chem. Int. Ed. 56 (48), 15170–15171. doi:10.1002/anie.201709002
Li, J., Zhang, J., Zhang, Q., Bai, Z., Zhao, Q., He, D., et al. (2018). Syntheses and Anti-cancer Activity of CO-releasing Molecules with Targeting Galactose Receptors. Org. Biomol. Chem. 16 (43), 8115–8129. doi:10.1039/c8ob01921e
Li, R., Lyu, Y., Luo, S., Wang, H., Zheng, X., Li, L., et al. (2021). Fabrication of a Multi-Level Drug Release Platform with Liposomes, Chitooligosaccharides, Phospholipids and Injectable Chitosan Hydrogel to Enhance Anti-tumor Effectiveness. Carbohydr. Polym. 269, 118322. doi:10.1016/j.carbpol.2021.118322
Li, R., Zheng, K., Yuan, C., Chen, Z., and Huang, M. (2017). Be Active or Not: the Relative Contribution of Active and Passive Tumor Targeting of Nanomaterials. Nanotheranostics 1 (4), 346–357. doi:10.7150/ntno.19380
Li, S.-Y., Cheng, H., Qiu, W.-X., Liu, L.-H., Chen, S., Hu, Y., et al. (2015). Protease-Activable Cell-Penetrating Peptide-Protoporphyrin Conjugate for Targeted Photodynamic Therapy In Vivo. ACS Appl. Mat. Interfaces 7 (51), 28319–28329. doi:10.1021/acsami.5b08637
Li, Y., Chen, R., Li, Z., Cheng, H., Li, X., Li, T., et al. (2019). miR-204 Negatively Regulates Cell Growth and Metastasis by Targeting ROBO4 in Human Bladder Cancer. Onco Targets Ther. 12, 8515–8524. doi:10.2147/OTT.S205023
Lin, J., Peng, C., Ravi, S., Siddiki, A. K. M. N. A., Zheng, J., Balkus, K. J., et al. (2020). Biphenyl Wrinkled Mesoporous Silica Nanoparticles for pH-Responsive Doxorubicin Drug Delivery. Materials 13 (8), 1998. doi:10.3390/ma13081998
Lindskog, M., Laurell, A., Kjellman, A., Melichar, B., Rey, P. M., Zieliński, H., et al. (2022). Ilixadencel, a Cell-Based Immune Primer, Plus Sunitinib versus Sunitinib Alone in Metastatic Renal Cell Carcinoma: A Randomized Phase 2 Study. Eur. Urology Open Sci. 40, 38–45. doi:10.1016/j.euros.2022.03.012
Liu, J., Tang, H., Peng, M., and Yuquan, W. (2018). Research Progress on Clinical Translation of Anti-tumor Nanomedicines. Sci. Technol. Her. 36 (22), 118–126.
Liu, J.-n., Bu, W., and Shi, J. (2017). Chemical Design and Synthesis of Functionalized Probes for Imaging and Treating Tumor Hypoxia. Chem. Rev. 117 (9), 6160–6224. doi:10.1021/acs.chemrev.6b00525
Liu, J., Miao, L., Sui, J., Hao, Y., and Huang, G. (2020). Nanoparticle Cancer Vaccines: Design Considerations and Recent Advances. Asian J. Pharm. Sci. 15 (5), 576–590. doi:10.1016/j.ajps.2019.10.006
Liu, M. A. (2019). A Comparison of Plasmid DNA and mRNA as Vaccine Technologies. Vaccines 7 (2), 37. doi:10.3390/vaccines7020037
Liu, R., Xiao, W., Hu, C., Xie, R., and Gao, H. (2018). Theranostic Size-Reducible and No Donor Conjugated Gold Nanocluster Fabricated Hyaluronic Acid Nanoparticle with Optimal Size for Combinational Treatment of Breast Cancer and Lung Metastasis. J. Control. Release 278, 127–139. doi:10.1016/j.jconrel.2018.04.005
Liu, Z., Li, Z., Zhang, Y., Zhou, T., Zhang, J., You, W., et al. (2021). Interpretation of the 2020 Global Cancer Statistical Report. Electron. J. Compr. Cancer Ther. 7 (02), 1–14.
Lopez, S., Zeybek, B., and Santin, A. D. (2018). Targeting Her2/neu in Uterine Serous Carcinoma: A Paradigm Shift in Management. Oncotarget 9 (94), 36652–36653. doi:10.18632/oncotarget.26413
Loquai, C., Hassel, J., Brück, P., Derhovanessian, E., Cuk, K., Lörks, V., et al. (2021). 549 an RNA-Lipoplex (RNA-LPX) Vaccine Demonstrates Strong Immunogenicity and Promising Clinical Activity in a Phase I Trial in Cutaneous Melanoma Patients with No Evidence of Disease at Trial Inclusion. J. Immunother. Cancer 9 (Suppl. 2), A579. doi:10.1136/jitc-2021-SITC2021.549
Loquai, C., Hassel, J. C., Oehm, P., Derhovanessian, E., Jabulowsky, R. A., Gold, M., et al. (2020). A Shared Tumor-Antigen RNA-Lipoplex Vaccine With/without Anti-PD1 in Patients with Checkpoint-Inhibition Experienced Melanoma. Jco 38 (15_Suppl. l), 3136. doi:10.1200/JCO.2020.38.15_suppl.3136
Mayer, R. J. (2009). Targeted Therapy for Advanced Colorectal Cancer - More Is Not Always Better. N. Engl. J. Med. 360 (6), 623–625. doi:10.1056/NEJMe0809343
Macias-Perez, I. M., and Flinn, I. W. (2013). GS-1101: A Delta-Specific PI3K Inhibitor in Chronic Lymphocytic Leukemia. Curr. Hematol. Malig. Rep. 8 (1), 22–27. doi:10.1007/s11899-012-0142-1
Maeda, H., Sawa, T., and Konno, T. (2001). Mechanism of Tumor-Targeted Delivery of Macromolecular Drugs, Including the EPR Effect in Solid Tumor and Clinical Overview of the Prototype Polymeric Drug SMANCS. J. Control Release 74 (1-3), 47–61. doi:10.1016/s0168-3659(01)00309-1
Maeda, H. (2001). SMANCS and Polymer-Conjugated Macromolecular Drugs: Advantages in Cancer Chemotherapy. Adv. Drug Deliv. Rev. 46 (1), 169–185. doi:10.1016/S0169-409X(00)00134-4
Maeda, H., Wu, J., Sawa, T., Matsumura, Y., and Hori, K. (2000). Tumor Vascular Permeability and the EPR Effect in Macromolecular Therapeutics: a Review. J. Control Release 65 (1-2), 271–284. doi:10.1016/s0168-3659(99)00248-5
Maeda, H., Bharate, G. Y., and Daruwalla, J. (2009). Polymeric Drugs for Efficient Tumor-Targeted Drug Delivery Based on EPR-Effect. Eur. J. Pharm. Biopharm. 71 (3), 409–419. doi:10.1016/j.ejpb.2008.11.010
Maeda, H., Nakamura, H., and Fang, J. (2013). The EPR Effect for Macromolecular Drug Delivery to Solid Tumors: Improvement of Tumor Uptake, Lowering of Systemic Toxicity, and Distinct Tumor Imaging In Vivo. Adv. Drug Deliv. Rev. 65 (1), 71–79. doi:10.1016/j.addr.2012.10.002
Maeda, H., Ueda, M., Morinaga, T., and Matsumoto, T. (1985). Conjugation of Poly(styrene-Co-Maleic Acid) Derivatives to the Antitumor Protein Neocarzinostatin: Pronounced Improvements in Pharmacological Properties. J. Med. Chem. 28 (4), 455–461. doi:10.1021/jm00382a012
Maity, S., and Chakraborti, A. S. (2020). Formulation, Physico-Chemical Characterization and Antidiabetic Potential of Naringenin-Loaded Poly D, L Lactide-Co-Glycolide (N-PLGA) Nanoparticles. Eur. Polym. J. 134, 109818. doi:10.1016/j.eurpolymj.2020.109818
Mamo, T., and Poland, G. A. (2012). Nanovaccinology: The Next Generation of Vaccines Meets 21st Century Materials Science and Engineering. Vaccine 30 (47), 6609–6611. doi:10.1016/j.vaccine.2012.08.023
Maruggi, G., Zhang, C., Li, J., Ulmer, J. B., and Yu, D. (2019). mRNA as a Transformative Technology for Vaccine Development to Control Infectious Diseases. Mol. Ther. 27 (4), 757–772. doi:10.1016/j.ymthe.2019.01.020
Masood, F. (2016). Polymeric Nanoparticles for Targeted Drug Delivery System for Cancer Therapy. Mater. Sci. Eng. C 60, 569–578. doi:10.1016/j.msec.2015.11.067
Matsumura, Y., and Maeda, H. (1986). A New Concept for Macromolecular Therapeutics in Cancer Chemotherapy: Mechanism of Tumoritropic Accumulation of Proteins and the Antitumor Agent Smancs. Cancer Res. 46 (12 Pt 1), 6387–6392.
Merli, M., Ferrario, A., Maffioli, M., Olivares, C., Stasia, A., Arcaini, L., et al. (2016). New Uses for Brentuximab Vedotin and Novel Antibody Drug Conjugates in Lymphoma. Expert Rev. Hematol. 9 (8), 767–780. doi:10.1080/17474086.2016.1205949
Mukherjee, S., and Patra, C. R. (2016). Therapeutic Application of Anti-angiogenic Nanomaterials in Cancers. Nanoscale 8 (25), 12444–12470. doi:10.1039/C5NR07887C
Nazha, B., Inal, C., and Owonikoko, T. K. (2020). Disialoganglioside GD2 Expression in Solid Tumors and Role as a Target for Cancer Therapy. Front. Oncol. 10, 1000. doi:10.3389/fonc.2020.01000
Nel, A., Ruoslahti, E., and Meng, H. (2017). New Insights into “Permeability” as in the Enhanced Permeability and Retention Effect of Cancer Nanotherapeutics. ACS Nano 11 (10), 9567–9569. doi:10.1021/acsnano.7b07214
Nguyen, T. X., Huang, L., Gauthier, M., Yang, G., and Wang, Q. (2016). Recent Advances in Liposome Surface Modification for Oral Drug Delivery. Nanomedicine 11 (9), 1169–1185. doi:10.2217/nnm.16.9
Noguchi, Y., Wu, J., Duncan, R., Strohalm, J., Ulbrich, K., Akaike, T., et al. (1998). Early Phase Tumor Accumulation of Macromolecules: A Great Difference in Clearance Rate between Tumor and Normal Tissues. Jpn. J. Cancer Res. 89 (3), 307–314. doi:10.1111/j.1349-7006.1998.tb00563.x
Nogueira-Librelotto, D. R., Codevilla, C. F., Farooqi, A., and Rolim, C. M. B. (2017). Transferrin-Conjugated Nanocarriers as Active-Targeted Drug Delivery Platforms for Cancer Therapy. Cpd 23 (3), 454–466. doi:10.2174/1381612822666161026162347
Noh, Y.-W., Kim, S.-Y., Kim, J.-E., Kim, S., Ryu, J., Kim, I., et al. (2017). Multifaceted Immunomodulatory Nanoliposomes: Reshaping Tumors into Vaccines for Enhanced Cancer Immunotherapy. Adv. Funct. Mat. 27 (8), 1605398. doi:10.1002/adfm.201605398
O'Leary, B., Finn, R. S., and Turner, N. C. (2016). Treating Cancer with Selective CDK4/6 Inhibitors. Nat. Rev. Clin. Oncol. 13 (7), 417–430. doi:10.1038/nrclinonc.2016.26
Oberli, M. A., Reichmuth Andreas, A. M., Dorkin, J. R., Mitchell, M. J., Fenton, O. S., Jaklenec, A., et al. (2017). Lipid Nanoparticle Assisted mRNA Delivery for Potent Cancer Immunotherapy. Nano Lett. 17 (3), 1326–1335. doi:10.1021/acs.nanolett.6b03329
Palma, E., Pasqua, A., Gagliardi, A., Britti, D., Fresta, M., and Cosco, D. (2018). Antileishmanial Activity of Amphotericin B-Loaded-PLGA Nanoparticles: An Overview. Materials 11 (7), 1167. doi:10.3390/ma11071167
Pandian, S. R. K., Pavadai, P., Vellaisamy, S., Ravishankar, V., Palanisamy, P., Sundar, L. M., et al. (2021). Formulation and Evaluation of Rutin-Loaded Solid Lipid Nanoparticles for the Treatment of Brain Tumor. Schmiedeb. Arch. Pharmacol. 394 (4), 735–749. doi:10.1007/s00210-020-02015-9
Pardi, N., Hogan, M. J., Porter, F. W., and Weissman, D. (2018). mRNA Vaccines - a New Era in Vaccinology. Nat. Rev. Drug Discov. 17 (4), 261–279. doi:10.1038/nrd.2017.243
Park, W., Heo, Y.-J., and Han, D. K. (2018). New Opportunities for Nanoparticles in Cancer Immunotherapy. Biomater. Res. 22 (1), 24. doi:10.1186/s40824-018-0133-y
Patel, M. R., Bauer, T. M., Jimeno, A., Wang, D., LoRusso, P., Do, K. T., et al. (2020). A Phase I Study of mRNA-2752, a Lipid Nanoparticle Encapsulating mRNAs Encoding Human OX40L, IL-23, and IL-36γ, for Intratumoral (iTu) Injection Alone and in Combination with Durvalumab. Jco 38 (15_Suppl. l), 3092. doi:10.1200/JCO.2020.38.15_suppl.3092
Plaza-Oliver, M., Santander-Ortega, M. J., and Lozano, M. V. (2021). Current Approaches in Lipid-Based Nanocarriers for Oral Drug Delivery. Drug Deliv. Transl. Res. 11 (2), 471–497. doi:10.1007/s13346-021-00908-7
Porciuncula, A., Morgado, M., Gupta, R., Syrigos, K., Meehan, R., Zacharek, S. J., et al. (2021). Spatial Mapping and Immunomodulatory Role of the OX40/OX40L Pathway in Human Non-small Cell Lung Cancer. Clin. Cancer Res. 27 (22), 6174–6183. doi:10.1158/1078-0432.CCR-21-0987
Pu, X., Zhao, L., Li, J., Song, R., Wang, Y., Yu, K., et al. (2019). A Polymeric Micelle with an Endosomal pH-Sensitivity for Intracellular Delivery and Enhanced Antitumor Efficacy of Hydroxycamptothecin. Acta Biomater. 88, 357–369. doi:10.1016/j.actbio.2019.02.039
Radionuclide (2021). Radionuclide Reduces PSA in mCRPC. Cancer Discov. 11 (5), 998–999. doi:10.1158/2159-8290.CD-NB2021-0325
Ragelle, H., Danhier, F., Préat, V., Langer, R., and Anderson, D. G. (2017). Nanoparticle-based Drug Delivery Systems: a Commercial and Regulatory Outlook as the Field Matures. Expert Opin. Drug Deliv. 14 (7), 851–864. doi:10.1080/17425247.2016.1244187
Rapoport, N. (2007). Physical Stimuli-Responsive Polymeric Micelles for Anti-cancer Drug Delivery. Prog. Polym. Sci. 32 (8), 962–990. doi:10.1016/j.progpolymsci.2007.05.009
Rockman, S., Laurie, K. L., Parkes, S., Wheatley, A., and Barr, I. G. (2020). New Technologies for Influenza Vaccines. Microorganisms 8 (11), 1745. doi:10.3390/microorganisms8111745
Rowshanravan, B., Halliday, N., and Sansom, D. M. (2018). CTLA-4: a Moving Target in Immunotherapy. Blood 131 (1), 58–67. doi:10.1182/blood-2017-06-741033
Sahin, U., Karikó, K., and Türeci, Ö. (2014). mRNA-based Therapeutics - Developing a New Class of Drugs. Nat. Rev. Drug Discov. 13 (10), 759–780. doi:10.1038/nrd4278
Sahin, U., Oehm, P., Derhovanessian, E., Jabulowsky, R. A., Vormehr, M., Gold, M., et al. (2020). An RNA Vaccine Drives Immunity in Checkpoint-Inhibitor-Treated Melanoma. Nature 585 (7823), 107–112. doi:10.1038/s41586-020-2537-9
Sanchez, L., Dardac, A., Madduri, D., Richard, S., and Richter, J. (2021). B-Cell Maturation Antigen (BCMA) in Multiple Myeloma: the New Frontier of Targeted Therapies. Ther. Adv. Hematol. 12, 204062072198958. doi:10.1177/2040620721989585
Schleich, N., Po, C., Jacobs, D., Ucakar, B., Gallez, B., Danhier, F., et al. (2014). Comparison of Active, Passive and Magnetic Targeting to Tumors of Multifunctional paclitaxel/SPIO-Loaded Nanoparticles for Tumor Imaging and Therapy. J. Control. Release 194, 82–91. doi:10.1016/j.jconrel.2014.07.059
Schulz, M., Salamero-Boix, A., Niesel, K., Alekseeva, T., and Sevenich, L. (2019). Microenvironmental Regulation of Tumor Progression and Therapeutic Response in Brain Metastasis. Front. Immunol. 10. doi:10.3389/fimmu.2019.01713
Sheena, P. A., Hitha, H., Sreedevi, A., and Varghese, T. (2020). Influence of Finite Size and Surface Effects on the Structural, Electrical and Magnetic Properties of Nanostructured Nickel Oxide. J. Mater Sci. Mater Electron 31 (7), 5769–5778. doi:10.1007/s10854-020-03147-7
Shi, M., Gu, A., Tu, H., Huang, C., Wang, H., Yu, Z., et al. (2021). Comparing Nanoparticle Polymeric Micellar Paclitaxel and Solvent-Based Paclitaxel as First-Line Treatment of Advanced Non-small-cell Lung Cancer: an Open-Label, Randomized, Multicenter, Phase III Trial. Ann. Oncol. 32 (1), 85–96. doi:10.1016/j.annonc.2020.10.479
Shrestha, B., Wang, L., Brey, E. M., Uribe, G. R., and Tang, L. (2021). Smart Nanoparticles for Chemo-Based Combinational Therapy. Pharmaceutics 13 (6), 853. doi:10.3390/pharmaceutics13060853
Sindhwani, S., Syed, A. M., Ngai, J., Kingston, B. R., Maiorino, L., Rothschild, J., et al. (2020). The Entry of Nanoparticles into Solid Tumours. Nat. Mat. 19 (5), 566–575. doi:10.1038/s41563-019-0566-2
Subbiah, V., and Cote, G. J. (2020). Advances in Targeting RET-dependent Cancers. Cancer Discov. 10 (4), 498–505. doi:10.1158/2159-8290.Cd-19-1116
Sulczewski, F. B., Liszbinski, R. B., Romão, P. R. T., and Rodrigues Junior, L. C. (2018). Nanoparticle Vaccines against Viral Infections. Arch. Virol. 163 (9), 2313–2325. doi:10.1007/s00705-018-3856-0
Sun, X., Huang, X., Yan, X., Wang, Y., Guo, J., Jacobson, O., et al. (2014). Chelator-Free 64Cu-Integrated Gold Nanomaterials for Positron Emission Tomography Imaging Guided Photothermal Cancer Therapy. ACS Nano 8 (8), 8438–8446. doi:10.1021/nn502950t
Tan, O. J., Loo, H. L., Thiagarajah, G., Palanisamy, U. D., and Sundralingam, U. (2021). Improving Oral Bioavailability of Medicinal Herbal Compounds through Lipid-Based Formulations - A Scoping Review. Phytomedicine 90, 153651. doi:10.1016/j.phymed.2021.153651
Tang, B., Yong, X., Xie, R., Li, Q.-W., and Yang, S.-M. (2014). Vasoactive Intestinal Peptide Receptor-Based Imaging and Treatment of Tumors. Int. J. Oncol. 44 (4), 1023–1031. doi:10.3892/ijo.2014.2276
Tang, L., Yang, X., Yin, Q., Cai, K., Wang, H., Chaudhury, I., et al. (2014). Investigating the Optimal Size of Anticancer Nanomedicine. Proc. Natl. Acad. Sci. U.S.A. 111 (43), 15344–15349. doi:10.1073/pnas.1411499111
Taya, M., and Hammes, S. R. (2018). Glycoprotein Non-metastatic Melanoma Protein B (GPNMB) and Cancer: A Novel Potential Therapeutic Target. Steroids 133, 102–107. doi:10.1016/j.steroids.2017.10.013
Tenchov, R., Bird, R., Curtze, A. E., and Zhou, Q. (2021). Lipid Nanoparticles─From Liposomes to mRNA Vaccine Delivery, a Landscape of Research Diversity and Advancement. ACS Nano 15 (11), 16982–17015. doi:10.1021/acsnano.1c04996
Teo, S. P. (2021). Review of COVID-19 mRNA Vaccines: BNT162b2 and mRNA-1273. J. Pharm. Pract. 0 (0), 089719002110096. doi:10.1177/08971900211009650
Thi, T. T. H., Suys, E. J. A., Lee, J. S., Nguyen, D. H., Park, K. D., and Truong, N. P. (2021). Lipid-Based Nanoparticles in the Clinic and Clinical Trials: From Cancer Nanomedicine to COVID-19 Vaccines. Vaccines 9, 359. doi:10.3390/vaccines9040359
Torchilin, V. P. (2007). Targeted Pharmaceutical Nanocarriers for Cancer Therapy and Imaging. AAPS J. 9 (2), E128–E147. doi:10.1208/aapsj0902015
Torchilin, V. P. (2007). Targeted Pharmaceutical Nanocarriers for Cancer Therapy and Imaging. AAPS J. 9 (2), E128–E147. doi:10.1208/aapsj0902015
Torchilin, V. (2011). Tumor Delivery of Macromolecular Drugs Based on the EPR Effect. Adv. Drug Deliv. Rev. 63 (3), 131–135. doi:10.1016/j.addr.2010.03.011
Trembley, J. H., Chen, Z., Unger, G., Slaton, J., Kren, B. T., Van Waes, C., et al. (2010). Emergence of Protein Kinase CK2 as a Key Target in Cancer Therapy. BioFactors 36 (3), 187–195. doi:10.1002/biof.96
Ulbrich, K., Holá, K., Šubr, V., Bakandritsos, A., Tuček, J., and Zbořil, R. (2016). Targeted Drug Delivery with Polymers and Magnetic Nanoparticles: Covalent and Noncovalent Approaches, Release Control, and Clinical Studies. Chem. Rev. 116 (9), 5338–5431. doi:10.1021/acs.chemrev.5b00589
van de Donkvan de Donk, N. W. C. J. (2018). Immunomodulatory Effects of CD38-Targeting Antibodies. Immunol. Lett. 199, 16–22. doi:10.1016/j.imlet.2018.04.005
Wadhwa, A., Aljabbari, A., Lokras, A., Foged, C., and Thakur, A. (2020). Opportunities and Challenges in the Delivery of mRNA-Based Vaccines. Pharmaceutics 12 (2), 102. doi:10.3390/pharmaceutics12020102
Wajant, H. (2016). Therapeutic Targeting of CD70 and CD27. Expert Opin. Ther. Targets 20 (8), 959–973. doi:10.1517/14728222.2016.1158812
Wang, F., Li, C., Cheng, J., and Yuan, Z. (2016). Recent Advances on Inorganic Nanoparticle-Based Cancer Therapeutic Agents. Ijerph 13 (12), 1182. doi:10.3390/ijerph13121182
Wang, J., Zhu, S., Chen, Q., Sun, J., Xia, Y., Zhang, Y., et al. (2020). Preclinical Evaluation of SIM1803-1A, a Small Molecule Trk/ROS1 Dual Inhibitor for Wild and Mutate NTRK/ROS1 Fusion Solid Malignancies. Jco 38 (15_Suppl. l), e21663. doi:10.1200/JCO.2020.38.15_suppl.e21663
Wang, P., Qu, Y., Li, C., Yin, L., Shen, C., Chen, W., et al. (2015). Bio-functionalized Dense-Silica Nanoparticles for MR/NIRF Imaging of CD146 in Gastric Cancer. Ijn 10, 749–763. doi:10.2147/ijn.S62837
Wang, R.-T., Zhi, X.-Y., Yao, S.-Y., and Zhang, Y. (2015). LFC131 Peptide-Conjugated Polymeric Nanoparticles for the Effective Delivery of Docetaxel in CXCR4 Overexpressed Lung Cancer Cells. Colloids Surfaces B Biointerfaces 133, 43–50. doi:10.1016/j.colsurfb.2015.05.030
Wang, X., Zhong, X., Li, J., Liu, Z., and Cheng, L. (2021). Inorganic Nanomaterials with Rapid Clearance for Biomedical Applications. Chem. Soc. Rev. 50 (15), 8669–8742. doi:10.1039/D0CS00461H
Wang, Y., Liu, D., Zhang, T., and Xia, L. (2021). FGF/FGFR Signaling in Hepatocellular Carcinoma: From Carcinogenesis to Recent Therapeutic Intervention. Cancers 13 (6), 1360. doi:10.3390/cancers13061360
Wang, Y., Shen, N., Wang, Y., Zhang, Y., Tang, Z., and Chen, X. (2021). Self‐Amplifying Nanotherapeutic Drugs Homing to Tumors in a Manner of Chain Reaction. Adv. Mat. 33 (7), 2002094. doi:10.1002/adma.202002094
Wei, J., and Hui, A.-M. (2022). The Paradigm Shift in Treatment from Covid-19 to Oncology with mRNA Vaccines. Cancer Treat. Rev. 107, 102405. doi:10.1016/j.ctrv.2022.102405
Wei, X., Shao, B., He, Z., Ye, T., Luo, M., Sang, Y., et al. (2015). Cationic Nanocarriers Induce Cell Necrosis through Impairment of Na+/K+-ATPase and Cause Subsequent Inflammatory Response. Cell Res. 25 (2), 237–253. doi:10.1038/cr.2015.9
Wiedermann, U., Wiltschke, C., Jasinska, J., Kundi, M., Zurbriggen, R., Garner-Spitzer, E., et al. (2010). A Virosomal Formulated Her-2/neu Multi-Peptide Vaccine Induces Her-2/neu-specific Immune Responses in Patients with Metastatic Breast Cancer: a Phase I Study. Breast Cancer Res. Treat. 119 (3), 673–683. doi:10.1007/s10549-009-0666-9
Wilhelm, S., Tavares, A. J., Dai, Q., Ohta, S., Audet, J., Dvorak, H. F., et al. (2016). Analysis of Nanoparticle Delivery to Tumours. Nat. Rev. Mater 1 (5), 16014. doi:10.1038/natrevmats.2016.14
Williams, M., Li, D., Fuji, R., Fuh, F., Prabhu, S., Zheng, B., et al. (2008). Pharmacodynamics, Pharmacokinetics, and Tolerability of a B-Cell Specific Antibody-Targeted Chemotherapeutic Agent, Anti-CD79b-MCC-DM1, in Cynomolgus Monkeys. Blood 112 (11), 4974. doi:10.1182/blood.V112.11.4974.4974
Wu, P.-H., Opadele, A. E., Onodera, Y., and Nam, J.-M. (2019). Targeting Integrins in Cancer Nanomedicine: Applications in Cancer Diagnosis and Therapy. Cancers 11 (11), 1783. doi:10.3390/cancers11111783
Xiang, L., Du, J., Xiang, X., Tang, T., Hu, X., and Xiang, D. (2012). Research Progress on the Application of Liposomes in Antitumor Drugs. South-Central Pharm. 10 (4), 290–294. doi:10.3969/j.issn.1672-2981.2012.04.016
Xu, J., Liao, K., Jiang, H., and Zhou, W. (2018). Research Progress of Novel Inorganic Nanometre Materials Carriers in Nanomedicine for Cancer Diagnosis and Treatment. Artif. Cells, Nanomedicine, Biotechnol. 46 (Suppl. 3), S492–S502. doi:10.1080/21691401.2018.1499665
Xu, L., Zhang, Z., Ding, Y., Wang, L., Cheng, Y., Meng, L., et al. (2019). Bifunctional Liposomes Reduce the Chemotherapy Resistance of Doxorubicin Induced by Reactive Oxygen Species. Biomater. Sci. 7 (11), 4782–4789. doi:10.1039/C9BM00590K
Yadav, H. K. S., Dibi, M., Mohammad, A., and Srouji, A. E. (2018). Nanovaccines Formulation and Applications-A Review. J. Drug Deliv. Sci. Technol. 44, 380–387. doi:10.1016/j.jddst.2018.01.015
Yao, Y., Yao, X., Zhu, S., Zhu, W., Li, Z., Wang, Q., et al. (2017). Target Cell Killing Effects of CD20 Targeting Chimeric Antigen Receptor T Cells Derived from the Type II Anti-CD20 Antibody. Jco 35 (15_Suppl. l), e14548. doi:10.1200/JCO.2017.35.15_suppl.e14548
Youn, J. W., Woo, J. W., Kim, Y.-M., LimYoon, M. C., Park, S. Y., Seo, S. S., et al. (2020). Pembrolizumab Plus GX-188E Therapeutic DNA Vaccine in Patients with HPV-16-Positive or HPV-18-Positive Advanced Cervical Cancer: Interim Results of a Single-Arm, Phase 2 Trial. Lancet Oncol. 21 (12), 1653–1660. doi:10.1016/S1470-2045(20)30486-1
Youssoufian, H., Amato, R. J., Sweeney, C. J., Chiorean, E. G., Fox, F., Katz, T., et al. (2008). Phase 1 Study of IMC-3G3, an IgG1 Monoclonal Antibody Targeting PDGFRα in Patients with Advanced Solid Malignancies. Jco 26 (15_Suppl. l), 14617. doi:10.1200/jco.2008.26.15_suppl.14617
Zaki, N. M., Nasti, A., and Tirelli, N. (2011). Nanocarriers for Cytoplasmic Delivery: Cellular Uptake and Intracellular Fate of Chitosan and Hyaluronic Acid-Coated Chitosan Nanoparticles in a Phagocytic Cell Model. Macromol. Biosci. 11 (12), 1747–1760. doi:10.1002/mabi.201100156
Zaman, A., Wu, W., and Bivona, G. (2019). Targeting Oncogenic BRAF: Past, Present, and Future. Cancers 11 (8), 1197. doi:10.3390/cancers11081197
Zaman, M. S., Johnson, A. J., Petersingham, G., Muench, G. W., Dong, Q., and Wu, M. J. (2019). Protein Kinase CK2 Is Involved in Zinc Homeostasis in Breast and Prostate Cancer Cells. BioMetals 32 (6), 861–873. doi:10.1007/s10534-019-00218-z
Zamani, P., Momtazi‐Borojeni, A. A., Nik, M. E., Oskuee, R. K., and Sahebkar, A. (2018). Nanoliposomes as the Adjuvant Delivery Systems in Cancer Immunotherapy. J. Cell. Physiology 233 (7), 5189–5199. doi:10.1002/jcp.26361
Zammarchi, F., Corbett, S., Adams, L., Tyrer, P. C., Kiakos, K., Janghra, N., et al. (2018). ADCT-402, a PBD Dimer-Containing Antibody Drug Conjugate Targeting CD19-Expressing Malignancies. Blood 131 (10), 1094–1105. doi:10.1182/blood-2017-10-813493
Zeng, Q., Cai, X., Cao, Y., Zhou, C., Yu, L., and Chen, J. (2020). Preparation, Characterization, and Pharmacodynamic Study on Deep Second Degree Burns of Total Flavonoids Composite Phospholipids Liposome Gel of Oxytropis Falcata Bunge. Drug Dev. Industrial Pharm. 46 (12), 2000–2009. doi:10.1080/03639045.2020.1841787
Zhang, D., Wu, W., and Jiang, X. (2019). Controllable Synthesis and Biological Applications of Single-Molecule Polymer Nanomaterials. Acta Polym. Sin. 50 (3), 199–208. doi:10.11777/j.issn1000-3304.2019.18191
Zhang, L., Wu, S., Qin, Y., Fan, F., Zhang, Z., Huang, C., et al. (2019). Targeted Codelivery of an Antigen and Dual Agonists by Hybrid Nanoparticles for Enhanced Cancer Immunotherapy. Nano Lett. 19 (7), 4237–4249. doi:10.1021/acs.nanolett.9b00030
Zhang, Q., Guo, Q., Chen, Q., Zhao, X., Pennycook, S. J., and Chen, H. (2020). Highly Efficient 2D NIR‐II Photothermal Agent with Fenton Catalytic Activity for Cancer Synergistic Photothermal-Chemodynamic Therapy. Adv. Sci. 7 (7), 1902576. doi:10.1002/advs.201902576
Zhang, Z., Yang, W., Ma, F., Ma, Q., Zhang, B., Zhang, Y., et al. (2020). Enhancing the Chemotherapy Effect of Apatinib on Gastric Cancer by Co-treating with Salidroside to Reprogram the Tumor Hypoxia Micro-environment and Induce Cell Apoptosis. Drug Deliv. 27 (1), 691–702. doi:10.1080/10717544.2020.1754528
Zhao, Y., Xu, J., Le, V. M., Gong, Q., Li, S., Gao, F., et al. (2019). EpCAM Aptamer-Functionalized Cationic Liposome-Based Nanoparticles Loaded with miR-139-5p for Targeted Therapy in Colorectal Cancer. Mol. Pharm. 16 (11), 4696–4710. doi:10.1021/acs.molpharmaceut.9b00867
Zhao, Y., Zheng, H., Wang, X., Zheng, X., Zheng, Y., Chen, Y., et al. (2021). Preparation and Biological Property Evaluation of Novel Cationic Lipid-Based Liposomes for Efficient Gene Delivery. AAPS PharmSciTech 22 (1), 22. doi:10.1208/s12249-020-01868-w
Zhao, Z., Ma, X., Zhang, R., Hu, F., Zhang, T., Liu, Y., et al. (2021). A Novel Liposome-Polymer Hybrid Nanoparticles Delivering a Multi-Epitope Self-Replication DNA Vaccine and its Preliminary Immune Evaluation in Experimental Animals. Nanomedicine Nanotechnol. Biol. Med. 35, 102338. doi:10.1016/j.nano.2020.102338
Zhou, D., Chen, H., Mpoy, C., Afrin, S., Rogers, B. E., Garbow, J. R., et al. (2021). Radiosynthesis and Evaluation of Talazoparib and its Derivatives as PARP-1-Targeting Agents. Biomedicines 9 (5), 565. doi:10.3390/biomedicines9050565
Zhuang, X., Wu, T., Zhao, Y., Hu, X., Bao, Y., Guo, Y., et al. (2016). Lipid-enveloped Zinc Phosphate Hybrid Nanoparticles for Codelivery of H-2Kb and H-2Db-Restricted Antigenic Peptides and Monophosphoryl Lipid A to Induce Antitumor Immunity against Melanoma. J. Control. Release 228, 26–37. doi:10.1016/j.jconrel.2016.02.035
Zhukov, N. V., and Tjulandin, S. A. (2008). Targeted Therapy in the Treatment of Solid Tumors: Practice Contradicts Theory. Biochem. Mosc. 73 (5), 605–618. doi:10.1134/S000629790805012X
Zylberberg, C., and Matosevic, S. (2016). Pharmaceutical Liposomal Drug Delivery: a Review of New Delivery Systems and a Look at the Regulatory Landscape. Drug Deliv. 23 (9), 3319–3329. doi:10.1080/10717544.2016.1177136
Glossary
ALK anaplastic lymphoma kinase
ASGP-R Asi-aloglycoprotein receptor
BTK Bruton’s tyrosine kinase
CA4 combretastatin A4
cGAMP Cyclic GMP-AMP
CTLA-4 cytotoxic T lymphocyte-associated antigen-4
DDA Dimethyldioctadecylammonium bromide
EGF Epidermal Growth Factor
EGFR growth factor receptor
EPR enhanced permeability and retention
EpCAM Epithelial cell adhesion molecule
FGF Fibroblast growth factors
FR folate receptor
GD2 Disialoganglioside
GPNMB glycoprotein non-metastatic melanoma protein B
HDAC histonedeacetylases
HIF hypoxia inducible factor
ICAM-1 intercellular cell adhesion molecule-1
LHRHR Luteinizing hormone releasing hormone receptor
LNPs lipid nanoparticles
MPLA 3-O-desacyl-4′-monophosphoryl lipid A
MEK Mitogen-activated extracellular signal-regulated kinase
MMPs matrix metalloproteinases
mTOR mammalian target of rapamycin
NPs nanoparticles
PARP poly ADP-ribose polymerase
PD-1 Programmed cell death protein 1
PDGFR platelet-derived growth factor receptor
PI-3K phosphatidylinositol 3-kinase
ROBO4 Roundabout homolog 4
ROS1 Reaciveoxygenspecies
SA sialic acid
SLN Solid Lipid Nanoparticle
TEM transmission electron microscopy
TME tumour microenvironment
TfR transferrin receptor
VIPR Vasoacitve intestinal peptide receptor
VEGF vascular endothelial growth factor
Keywords: cancer, nanoparticles, liposomes, targeting mechanism, tumour therapy
Citation: Tu Y, Yao Z, Yang W, Tao S, Li B, Wang Y, Su Z and Li S (2022) Application of Nanoparticles in Tumour Targeted Drug Delivery and Vaccine. Front. Nanotechnol. 4:948705. doi: 10.3389/fnano.2022.948705
Received: 29 May 2022; Accepted: 24 June 2022;
Published: 15 July 2022.
Edited by:
Zhiqing Pang, Fudan University, ChinaReviewed by:
Anupam Dhasmana, The University of Texas Rio Grande Valley, United StatesYa Nan Song, Fudan University, China
Copyright © 2022 Tu, Yao, Yang, Tao, Li, Wang, Su and Li. This is an open-access article distributed under the terms of the Creative Commons Attribution License (CC BY). The use, distribution or reproduction in other forums is permitted, provided the original author(s) and the copyright owner(s) are credited and that the original publication in this journal is cited, in accordance with accepted academic practice. No use, distribution or reproduction is permitted which does not comply with these terms.
*Correspondence: Zhijian Su, dGpudXN6akBqbnUuZWR1LmNu; Shijun Li, Y3NncDAyQDEyNi5jb20=