- Department of Pharmacy, The First Affiliated Hospital of Xi’an Jiaotong University, Xi’an, China
Hypoxia-inducible factor (HIF), which plays a crucial role in oxygen homeostasis, contributes to immunosuppression, tumor angiogenesis, multidrug resistance, photodynamic therapy resistance, and metastasis. HIF as a therapeutic target has attracted scientists’ strong academic research interests. Short interfering RNA (siRNA) and antisense oligonucleotide (ASO) are the more promising and broadly utilized methods for oligonucleotide-based therapy. Their physicochemical characteristics such as hydrophilicity, negative charge, and high molecular weight make them impossible to cross the cell membrane. Moreover, siRNA and ASO are subjected to a rapid deterioration in circulation and cannot translocate into nuclear. Delivery of siRNA and ASO to specific gene targets should be realized without off-target gene silencing and affecting the healthy cells. Nanoparticles as vectors for delivery of siRNA and ASO possess great advantages and flourish in academic research. In this review, we summarized and analyzed regulation mechanisms of HIF under hypoxia, the significant role of HIF in promoting tumor progression, and recent academic research on nanoparticle-based delivery of HIF siRNA and ASO for cancer immunotherapy, antiangiogenesis, reversal of multidrug resistance and radioresistance, potentiating photodynamic therapy, inhibiting tumor metastasis and proliferation, and enhancing apoptosis are reviewed in this thesis. Furthermore, we hope to provide some rewarding suggestions and enlightenments for targeting HIF gene therapy.
Introduction
Hypoxia-inducible factor (HIF), which plays a crucial role in oxygen homeostasis, is composed of an inducible alpha subunit in the cytosol and a beta subunit constitutively expressed in the nucleus, and HIF responds to a low oxygen environment in metazoan species (Luo and Wang, 2018). The alpha subunit exists in 1 alpha, 2 alpha, and 3 alpha three forms, and the beta subunit includes HIF-1 beta, also known as aryl hydrocarbon receptor nuclear translocator (ARNT) and paralogue ARNT2 isoform (Albanese et al., 2021). Although HIF-1 alpha and HIF-2 alpha subunits share approximately forty-eight percentages total amino acid sequence identity and own the identical functional zones (Luo and Wang, 2018), HIF-1 alpha is ubiquitously expressed and HIF-2 alpha is only present in certain cell types such as hepatocytes and only in vertebrate species (Ma et al., 2014; Zhao et al., 2014). HIF-3 alpha is also produced in certain cells, and its functions are less well clarified. Human HIF-3 alpha isoforms one and 9 are demonstrated to activate gene transcription (Zhang et al., 2014); however, HIF-3 alpha isoforms 4, which are deficient in the transactivation zone and leucine zipper zone, inhibit both HIF-1 and HIF-2 transcription (Maynard et al., 2007). Whether the HIF-3 variants are of biological significance is still unclear (Gonzalez et al., 2018). HIF-1 beta exists ubiquitously, while ARNT2 isoform is highly expressed in the kidney and brain with low or absent levels in other tissues of mammalian species (Rowatt et al., 2003; Bahrami et al., 2018; Zheng et al., 2018). Hence, HIF-1 and HIF-2 isoforms have a vital effect on development, physiology, and illness, for example, tumor, organ transplant rejection, colitis, and pulmonary arterial hypertension (Semenza, 2012).
Under normoxic circumstances, HIF alpha as the O2 responsive subunit is hydroxylated on proline residues via a family of prolyl hydroxylase enzymes with O2 as a substrate (Epstein et al., 2001; Ivan et al., 2001). In the hydroxylation, one oxygen atom is plugged into the prolyl residue, and the other atom is inserted into the cofactor α-ketoglutatrate, degrading the cofactor into succinate and CO2 (Semenza, 2019). After prolyl hydroxylation, HIF alpha is bound to the von Hippel–Lindau protein, followed by the recruitment of the Cullin-2 and Elongin-B/C as a ubiquitin ligase (E3) for degradation of HIF alpha in the 26S proteasome (Semenza, 2019; Losman et al., 2020). Under hypoxia conditions, the prolyl hydroxylation for HIF alpha is blocked, resulting in impairment of its degradation. Translocation of the stabilized HIF alpha into the nucleus occurs, and HIF alpha dimerizes with HIF-1 beta. Upon dimerization, HIF connects to E-box-like hypoxia-responsive elements [5′-(A/G)CGTG-3′] within the promoter region (Choudhry and Harris, 2018) and activates genes that promote adaptation to hypoxia. HIF is responsible for a protective role in myocardial ischemia, limb ischemia, chronic rejection of organ transplants, pressure overload, heart failure, and wound healing (Semenza, 2014). In contrast, HIF contributes to various diseases, including tumor immune escape, tumor angiogenesis, resistance to chemotherapy and radiation, metastasis, and cancer progression (Bousquet et al., 2016; Mahdi et al., 2019). HIF as a therapeutic target has attracted scientists’ strong academic research interests.
Up to the present, there is still a lack of potent chemical inhibitors which possess the capability to inactivate HIFs (Yang et al., 2020). Oligonucleotide-based therapy, including short interfering RNA (siRNA), an antisense oligonucleotide (ASO), aptamer, and microRNA inhibitor and mimic, utilizes specific nucleic acids for treatment of various gene-related diseases or physical disorders. siRNA and ASO are the more promising and broadly utilized methods for oligonucleotide-based therapy, and both of them often act against similar targets (Chi et al., 2017). siRNA and ASO incorporate with the target RNA through Watson–Crick base paring, yet the mechanisms of gene silencing for them are different. siRNA, a 12–22 bp double-strand RNA duplexes, acts gene silencing effect by integrating its guide strand with the corresponding RNA-induced silencing complex (Osipova et al., 2020). RNA-induced silencing complex then identifies and combines with the targeted mRNA through the complementarity of RNA sequence, and the complementary domain of the targeted mRNA is subsequently broken between the tenth and eleventh nucleotide at the 5′end of the guide strand, leading to the destruction of the targeted mRNA (Dong et al., 2019; Charbe et al., 2020). ASO, usually with a length of 15–20 bp, is a single strand deoxynucleotide analog, and its antisense sequence (3′–5′) and the sense sequence of the targeted mRNA are complementary. The mechanisms of silencing mRNA by ASO include enhancing the potency of RNase H endonuclease that splits the mRNA-ASO heteroduplex, followed by the destruction of the targeted mRNA and intactness of ASO, and translational hindrance through steric effect, exon skipping, instability of pre-mRNA and targeted degradation of miRNA (Chi et al., 2017; Sasaki and Guo, 2018). The physiochemical characteristics of siRNA and ASO, such as hydrophilicity, negative charge, and high molecular weight, make them impossible to cross the cell membrane. Moreover, siRNA and ASO are subjected to a rapid deterioration in blood circulation by nucleases endosome or lysosome trafficking and could not translocate into nuclear (Arnold et al., 2017; Charbe et al., 2020). Delivery of siRNA and ASO to specific gene targets should be realized without off-target gene silencing and affecting the healthy cells. Owing to the unique qualities of nanoparticles, such as low toxicity, long circulation time, protecting nucleic acids from degradation, preventing immune response, and targeted delivery, the application of nanoparticles as vectors for delivery of siRNA and ASO in treatment proceeds to flourish in academic research.
In the review, the topic will be centered on the significant effects of HIF on promoting tumor progress and the recent academic research on the nanoparticles-based delivery system of siRNA and ASO to target the silence of HIF.
Role of Hypoxia-Inducible Factor on Cancer Progression
The tumor microenvironment is prominently featured by hypoxia, and hypoxia can be attributed to the imbalance between overwhelming demand for oxygen and nutrition of rapid proliferating neoplastic cells and insufficiency of oxygen supply by the damaged vessels and abnormal angiogenesis (Cheng et al., 2020; Mohapatra et al., 2021). The oxygen partial pressure (pO2) decreased from the blood vessel to the central section of the cancer and in contrast to in healthy tissues pO2 of 46–76 mmHg hypoxic region with pO2 of less than 10 mmHg is formed (Yao et al., 2018). HIF-1 alpha and HIF-2 alpha that promotes tumor progression are overexpressed transcription factors in carcinoma cells (Talks et al., 2000). HIF-1 is closely related to tumor immune escape, tumor angiogenesis, resistance to chemotherapy, radiotherapy and photodynamic therapy, tumor metastasis, cell proliferation and antiapoptosis (Figure 1) (Zhang et al., 2017; Li et al., 2018; Zeng et al., 2021). HIF-1 overexpression is involved in poor clinical results in gastric, cervical, ovarian, endometrial, nasopharyngeal, osteosarcoma, breast, endometrial, bladder, colorectal, glioblastoma, head and neck, and pancreatic carcinomas (Mahdi et al., 2019).
Role of Hypoxia-Inducible Factor on Tumor Immunotherapy
Hypoxia is a significant stressor in the tumor microenvironment that drives adaptations of cancer cells to escape immune surveillance (Ray and Mukherjee, 2022). HIF-1 promotes the expression of programmed cell death-ligand 1 (PD-L1), which binds to the programmed death receptor (PD-1) of T cells to protect neoplastic cells from T cells mediated death via integrating with the hypoxia response element in the proximal promoter (Sun et al., 2021). Hypoxia inhibits proliferation and activation of autologous T cells as well as the cytotoxic potency of CD8+ T cells or sustains T cells exhaustion, resulting in caner resistance to immune checkpoints blockade (DePeaux and Delgoffe, 2021; Sun et al., 2021). Alleviating hypoxia by hypoxia-activated prodrug evofosfamide or metformin reduced tumor cells’ mitochondrial activity and potentiated T cells’ response to anti-PD1 therapy (Scharping et al., 2017; Jayaprakash et al., 2018). Hypoxia also impairs natural killer cells functions, mediates recruitment of immunosuppressive cells, including tumor-associated macrophages (TAMs) and myeloid-derived suppressor cells, and inhibits mutation of dendritic cells as antigen-presenting cells (Yuan et al., 2021; Kheshtchin and Hadjati, 2022). Targeting the hypoxia microenvironment presents a novel strategy for cancer immunotherapy, and novel combinations of HIF inhibitor and immune checkpoint suppressor are promising approaches to enhance immunotherapeutic sensitivity.
Role of Hypoxia-Inducible Factor on Tumor Angiogenesis
Angiogenesis is essential for solid carcinomas initiation and progression due to the inadequate supply of oxygen and nutrients for cancer cells. To compensate for hypoxic conditions, HIF straightly activates the expression of a series of proangiogenic factors to increase oxygen delivery. The upregulated HIF-1 in hypoxic cells can recognize and bind to the hypoxia response elements in the promoter region and activate the transcription of vascular endothelial growth factor (VEGF) that is crucial in the activation and proliferation of endothelial cells (Jadidi-Niaragh et al., 2017; Yang M. et al., 2021). The other HIF-1-induced proangiogenic factors include VEGF receptor-1, fetal liver kinase-1, which belongs to VEGF receptors, angiopoietins 1 and 2, plasminogen activator inhibitor-1, matrix metalloproteinase 2 and 9, platelet-derived growth factor B, tyrosine kinase with immunoglobulin-like and epidermal growth factor-like domains receptor and platelet-derived growth factor-beta (Kelly et al., 2003; Yang M. et al., 2021; Zepeda et al., 2013).
Role of Hypoxia-Inducible Factor on Tumor Multidrug Resistance and Radioresistance
Acquired multidrug resistance is mainly responsible for therapeutic failure in patients with cancer. The hypoxic tumor microenvironment and multidrug resistance are closely related (Chen et al., 2017; Mi, 2020). The mechanism for HIF-1 alpha of mediating chemoresistance is stimulating genetic transcription of multidrug resistance 1 (MDR1) that translates a P-glycoprotein pertaining to ATP-binding cassette (ABC) transporters family and functioning as an efflux pump for multidrugs (Wigerup et al., 2016; Chen et al., 2017). Therefore, HIF and MDR1 abate the intracellular concentrations for multiple chemotherapeutic agents, leading to hypoxia-induced multidrug resistance. Under oxygen-deprived circumstances, the stabilized HIF-1 alpha also binds to ATP-binding cassette transporter G2 (ABCG2) promoter and activates transcription of another ABC transporter ABCG2 (Chen et al., 2019). Hypoxia-induced chemoresistance is also associated with HIF-1-dependent mechanisms conferring protection against chemotherapy-induced DNA damage (Jing et al., 2019), increased expression of antiapoptotic proteins (Piret et al., 2005) and/or decreased expression of proapoptotic proteins (Erler et al., 2004).
Radiotherapy is one of the most applied approaches for treating various kinds of cancers. However, the clinical efficacy of radiotherapy is seriously undermined by the radioresistance of carcinoma cells. HIF-1 signaling pathway is also responsible for the protective reaction of carcinoma cells to irradiation via repair of DNA double strands breaks, vessel protection after radiotherapy, the boost of glucose uptake and utilization, and enhancement of oxidation resistance (Li et al., 2018; Huang and Zhou, 2020; Wozny et al., 2021). HIF-1 is considered a potential target to enhance radiotherapy sensitivity for tumor cells.
Role of Hypoxia-Inducible Factor on Tumor Photodynamic Therapy Resistance
Photodynamic therapy (PDT), a minimally invasive therapeutic scheme for a variety of carcinomatous disorders, exploits reactive oxygen species produced by the bioreaction between photosensitizer and oxygen existing within the tumor microenvironment under irradiation of the laser at a specific wavelength to kill tumor cells (Liu Y. et al., 2019). Compared to conventional therapeutics, PDT possesses the advantages of minimal invasion, high spatial and temporal controllability, and specific lesion destruction (Fan et al., 2016; Song et al., 2016). However, oxygen consumption during PDT results in hypoxia which activates HIF, and a major challenge for PDT is circumventing PDT-induced hypoxic microenvironment that results in activation of a variety of proangiogenic factors, multidrug resistance, and dramatically deteriorates clinical outcomes (Dang et al., 2017; Zhang et al., 2017; Sun et al., 2020). HIF-1 alpha can also downregulate cytochrome P450 through competition with aryl hydrocarbon receptors to bind with HIF-1 beta (Fradette and du Souich, 2004), and it may act as an inhibitor for prodrug activation under hypoxia.
Role of Hypoxia-Inducible Factor on Tumor Metastasis
Tumor metastasis proposes a great challenge for cancer treatment because surgery, radiation, and chemotherapy focus on eradicating or blocking the progression of primary tumor lesion, and metastatic dissemination is difficult to treat via traditional methods. And more than 90% of carcinoma deaths can be ascribed to tumor metastasis (Ma et al., 2021). Tumor metastasis involves many pathologic steps and is a highly complicated and dynamic procedure. HIF can mediate the expression of genes that are responsible for many processes for tumor metastasis involving epithelial to mesenchymal transition, invasion of extracellular matrix components, intravasation into vessels, extravasation, and generation of micrometastasis in metastatic lesions (Saxena et al., 2020; Tao et al., 2021). Matrix metalloproteinase 2 (MMP 2) and MMP 9 that possess the capability for degrading the components of the extracellular matrix and facilitating the epithelial–mesenchymal transition process closely correlate with metastasis (Scheau et al., 2019; Gonzalez-Avila et al., 2020; Liu L. et al., 2019). HIF-1 alpha rapidly transactivates MMP-2 and MMP-9 expression (Shan et al., 2018; Zhang et al., 2018), and the expression of HIF-1 alpha is positively related to lymphatic metastasis and reduced survival rate (Song et al., 2019).
Role of Hypoxia-Inducible Factor on Cancer Cells Proliferation and Apoptosis
Hypoxia also exerts as a modulator of cell proliferation and apoptosis. In most cells, hypoxia suppresses cell proliferation since the increased demand for O2 for a growing number of cells exacerbates hypoxic stress (Hubbi and Semenza, 2015). However, even in the presence of upregulated HIF-1 alpha expression, many tumor cells maintain proliferation, and HIF-1 alpha promotes cancer cell proliferation and survival under hypoxia (Daniel et al., 2019; Chen et al., 2020). HIF-1 alpha upregulates SUMO-specific protease one expression under hypoxic conditions, and SUMO-specific protease one promotes proliferation and inhibits apoptosis of cancer cells (Wang et al., 2018). Nuclear factor kappa-B (NF-κB), a regulator of proliferation and apoptosis, is rapidly and persistently activated under hypoxia in an inhibitor of NF-κB kinase-dependent manner (D'Ignazio et al., 2017). Downregulation of HIF-1 alpha by siRNA inhibits proliferation and induces apoptosis of human hepatoma cells (Yang et al., 2011).
Recent Advances in Hypoxia-Inducible Factor Short Interfering RNA and Antisense Oligonucleotide Delivery for Cancer Treatment
siRNA and ASO have emerged as promising therapeutic strategies to inhibit HIF-1 alpha expression; however, their unique physiochemical characteristics and insufficient blood supply for hypoxic areas in tumors make it difficult for delivery of siRNA and ASO to hypoxic cells. Scientists utilize nanoparticles as vectors for targeting the delivery of HIF-1 alpha siRNA and ASO to hypoxic cells, and the nanosystems delivering hypoxia HIF-1 siRNA and ASO for cancer treatment were summarized in Table 1.
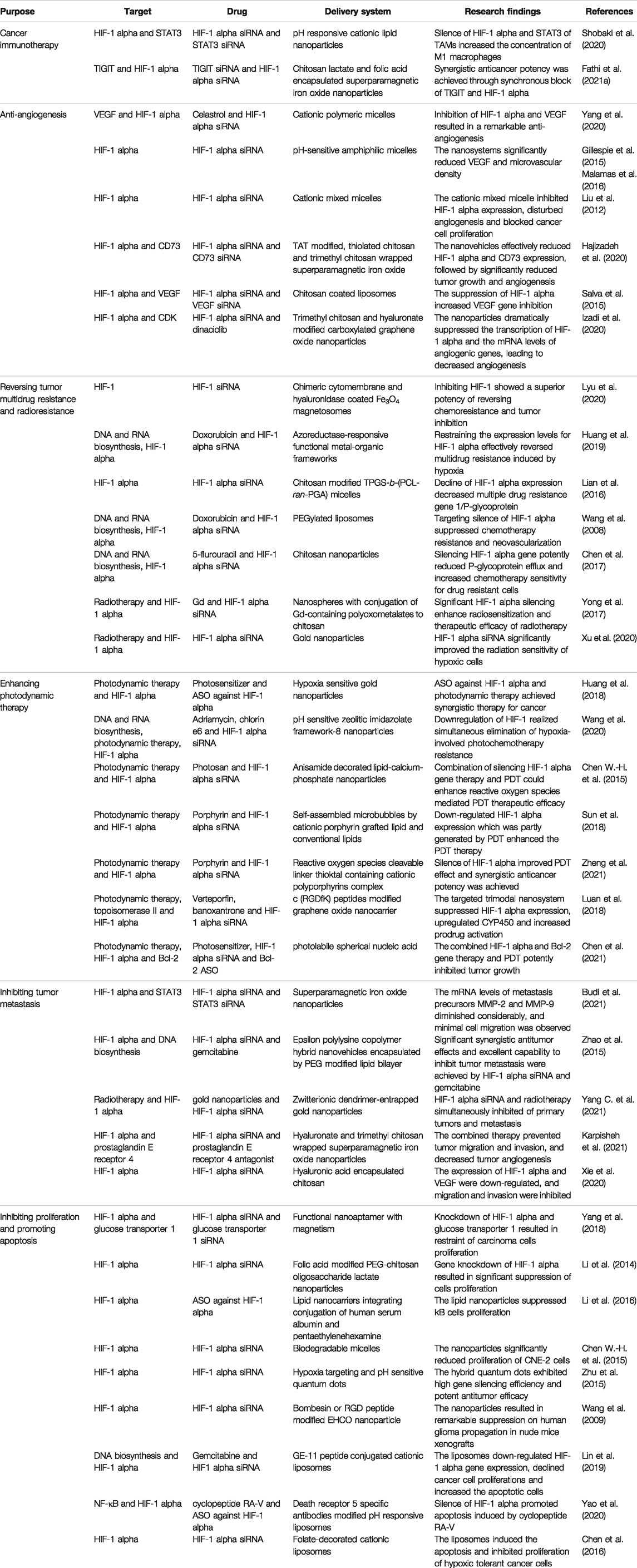
TABLE 1. Summary of nanosystems delivering hypoxia-inducible factor-1 alpha short interfering RNA and antisense oligonucleotide for cancer treatment.
Nanodrugs for Cancer Immunotherapy
TAMs, which are mostly M2 phenotype, account for the main population of immune cells infiltrated in solid tumor microenviroment (Lecoultre et al., 2020). Macrophages are highly plastic and can be polarized into classical M1 phenotype with antitumorous functions and alternative M2 phenotype with protumorous functions under different stimuli (Nowak and Klink, 2020). Hypoxic microenviroment selectively facilitates M2 polarization for macrophages via inducing expression of M2 phenotype-related genes and accumulation of lactic acid (Kheshtchin and Hadjati, 2022). TAMs play pivotal roles in tumor angiogenesis, neovascularization, metastasis, immunosuppression, and resistance to chemotherapy and immunotherapy (Cheng et al., 2021; Hou et al., 2022). However, TAMs can be repolarized to M1 phenotype, and their protumorous capability were reversed to antitumor power (Cheng et al., 2021). A pH-responsive cationic lipid nanoparticle composed of 7-[4-(dipropylamino)butyl]-7-hydroxytridecane-1,13-diyl dioleate, cholesterol, and PEG lipid was applied to encapsulate HIF-1 alpha siRNA and signal transducer and activator of transcription 3 (STAT3) siRNA for silencing the two genes in TAMs for TAMs based cancer immunotherapy (Shobaki et al., 2020). The cationic lipid nanoparticles were stable in blood circulation, highly and selectively taken up by TAMs, and strong in HIF-1 alpha and STAT3 gene silence of TAMs. The lipid nanoparticles increased the concentration of M1 macrophages and reversed the protumorous functions of angiogenesis and tumor cell activation for TAMs. However, the safety of the cationic lipid nanoparticles in vivo should be carefully evaluated.
T-cell immunoglobulin and ITIM domain (TIGIT), an immune checkpoint highly expressed by various immune cells and neoplastic cells, is considered a promising target for cancer immunotherapy (Fathi et al., 2021b). Upon hypoxia, HIF-1 alpha can promote the expression of many different kinds of immune checkpoints. Chitosan lactate and folic acid encapsulated superparamagnetic iron oxide nanoparticles were developed for carrying TIGIT siRNA and HIF-1 alpha siRNA for cancer immunotherapy (Fathi et al., 2021a). The nanosystem strongly arrested the TIGIT and HIF-1 alpha protein expression, angiogenesis and proliferation of colorectal and breast carcinoma cells, and synergistic anticancer potency was achieved through a synchronous block of TIGIT and HIF-1 alpha. Further research is required to realize the complete role of TIGIT and HIF-1 alpha in the tumor microenvironment before translation of combined targeting TIGIT and HIF-1 alpha therapy to humans.
Nanovehicles for Antiangiogenesis
Cationic polymeric micelles as a potential nanocarrier to deliver siRNA to cancer cells have attracted remarkable attention. Yang et al. recruited a cationic multipolymer poly ethylene glycol-poly epsilon-caprolactone-g-poly ethyleneimine to codeliver celastrol belonging to quinone methide triterpene ingredients purified from Tripterygium wilfordii’s root cortex and HIF-1 alpha siRNA for combinatory treatment for retinoblastoma (Yang et al., 2020). The nanomicelles inhibited HIF-1 alpha expression, further downregulated secretion of VEGF, and suppressed proliferation of vascular endothelial cells, resulting in a remarkable antiangiogenesis and antitumor potency. HIF-1 alpha siRNA was delivered by a pH-sensitive amphiphilic 1-(aminoethyl) iminobis [N-(oleicylcysteinylhistinyl-1-aminoethyl)propionamide] (Gillespie et al., 2015) and targeting peptides decorated (1-aminoethyl)iminobis [N-oleicylsteinyl-1-(aminoethyl)propionamide] (Malamas et al., 2016) that owned a distinct advantage of amphiphilic perturbation of cytomembrane under endosomal-lysosomal pH, and targeted endosomal-lysosomal release of siRNA into the cytoplasm was realized. The nanosystems significantly reduced HIF-1 alpha, VEGF, and microvascular density. Cationic mixed micelles formed by poly (epsilon-caprolactone)-block-poly (2-aminoethylethylene phosphate) and poly (epsilon-caprolactone)-block-PEG copolymers containing amphiphilic block were utilized to deliver HIF-1 alpha siRNA (Liu et al., 2012). The cationic mixed micelle inhibited HIF-1 alpha expression, disturbed angiogenesis, and blocked cancer cell proliferation. Safety and biocompatibility of the utilized cationic polymeric are undefined.
Chitosan and its derivatives, which possess a potent affinity to oligonucleotides attributed to cationically charged primary amino groups, offer a high entrapment efficiency for siRNA into nanoparticles (Iacob et al., 2021), and they specifically interact with a negatively charged cellular membrane (Bernkop-Schnurch, 2018). Chitosan and its derivatives were also used to coat nanoparticles for siRNA delivery. Thiolated chitosan and trimethyl chitosan-wrapped superparamagnetic iron oxide for enhancing stability and potential for loading of siRNA was fabricated to carry HIF-1 alpha siRNA and CD73 siRNA, and the nanoparticles were further modified with cell-penetrating peptide TAT to increase cellular transfection (Hajizadeh et al., 2020). The nanovehicles effectively reduced HIF-1 alpha and CD73 expression, followed by significantly reduced tumor growth and angiogenesis. Chitosan-coated liposomes for simultaneous delivery of HIF-1 alpha siRNA and VEGF siRNA demonstrated high stability and protected siRNA from deterioration post coincubation with fetal bovine serum for 24 h, and this nanoformulation significantly inhibited HIF-1 alpha and VEGF protein expression (Salva et al., 2015). Trimethyl chitosan and hyaluronate-modified carboxylated graphene oxide nanoparticles for loading of HIF-1 alpha siRNA and a cyclin-dependent kinase (CDK) inhibitor dinaciclib were developed for silencing HIF-1 alpha and inhibiting CDK in CD44 expressing carcinoma cells (Figure 2) (Izadi et al., 2020). The nanoparticles dramatically suppressed the transcription of HIF-1 alpha and the mRNA levels of angiogenic genes, for instance, VEGF, transforming growth factor-beta and fibroblast growth factor, leading to decreased angiogenesis. Chitosan shows little or no toxicity in an animal model and no major adverse effects in healthy volunteers, but clinical data are lacking. The toxicological profiles of chitosan derivatives are still under investigation.
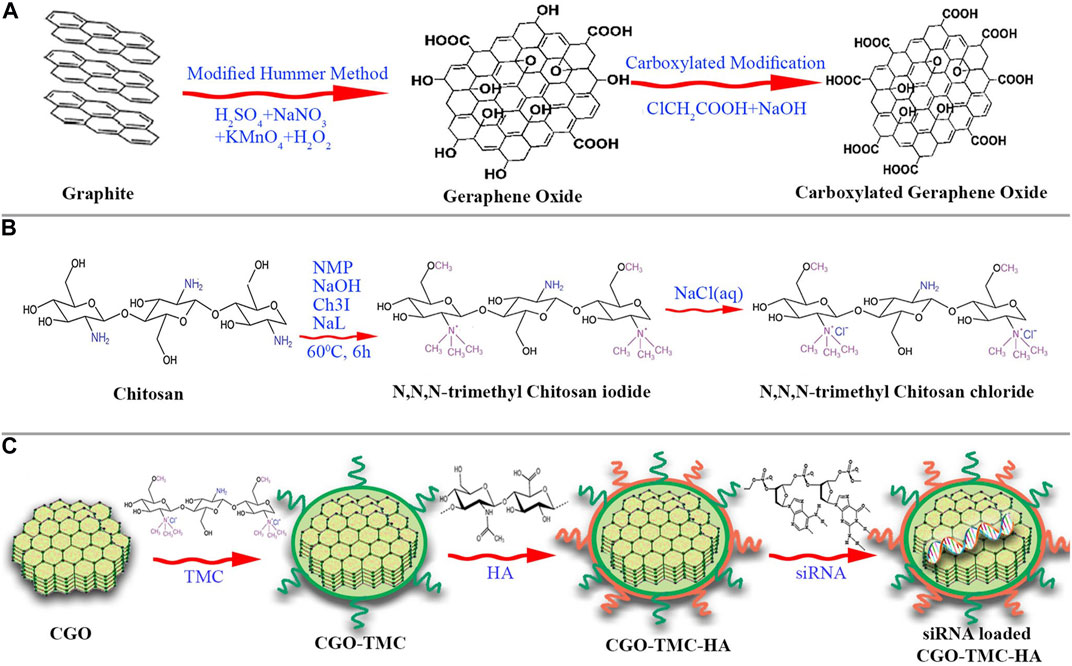
FIGURE 2. The synthesis scheme of carboxylated graphene oxide (CGO) and trimethyl chitosan (TMC) (A,B). Generation of siRNA loaded CGO-TMC-HA NPs, carboxylated graphene oxide conjugated with trimethyl chitosan and hyaluronate nanoparticles (C) (reproduced with permission from Izadi et al. (2020). Copyright 2020 Springer Nature).
Nanoformulations for Reversing Tumor Multidrug Resistance and Radioresistance
Superparamagnetic Fe3O4 magnetosomes coated by a pre-engineered macrophage–cancer chimeric cytomembrane and dibenzocyclooctyne-modified hyaluronidase were used to deliver HIF-1 siRNA into deepened hypoxia region of tumor (Figure 3) (Lyu et al., 2020). The anchored hyaluronidase degraded hyaluronan in the tumor microenvironment and promoted deep penetration into the hypoxia region, wherein HIF-1 and P-glycoprotein were of high content. The results revealed that inhibiting HIF-1 with magnetic nanoparticles showed a superior potency in reversing chemoresistance and tumor inhibition. The chimeric membrane can be prepared by combining other cell types, which will integrate more diverse functions to realize multiple requirements in drug delivery. Huang et al. developed functional metal-organic frameworks (AMOFs), which were responsive to azoreductase to codeliver chemotherapy agents and HIF-1 alpha siRNA (Figure 4) (Huang et al., 2019). The azoreductase-sensitive liberation of HIF-1 alpha siRNA suppressed the active excretion of chemotherapy agents by cancer cells by restraining the expression levels for HIF-1 alpha, multidrug resistance gene 1, and P-glycoprotein and therefore effectively reversed multidrug resistance induced by hypoxia and led to highly efficient therapy for hypoxic carcinoma. The AMOFs achieved an excellent response to the hypoxic tumor microenvironment, and the degradation of AMOFs could greatly facilitate the release of chemotherapeutic drugs and siRNA. Lian et al. prepared chitosan decorated D-alpha-tocopheryl PEG 1000 succinate-b-poly (3-caprolactone-ran-glycolide) (TPGS-b-(PCL-ran-PGA)) nanovehicles to load HIF-1 alpha siRNA (Lian et al., 2016). Chitosan decorated TPGS-b-(PCL-ran-PGA) nanoparticles can efficiently carry HIF-1 alpha siRNA into nasopharyngeal carcinoma cells, leading to a decline of HIF-1 alpha expression and multiple drug resistance gene 1/P-glycoprotein. Wang et al. developed an original composite liposomal drug delivery nanosystem consisting of liposomes for loading therapeutic agents, doxorubicin of anthracycline antibiotics for inducing cell death, and antisense oligonucleotides for targeting silence of HIF-1 alpha mRNA for suppressing chemotherapy resistance and neovascularization (Wang et al., 2008). The nanosystem significantly inhibited the chemoresistance of carcinoma cells by downregulation of HIF-1 alpha, hence increasing the anticancer potency for combined treatment to the degree that cannot be reached by each agent administered separately. Chitosan nanoparticles for codelivery of siRNA against HIF-1 alpha and 5-flurouracil were fabricated by ionic gel method to reverse the multidrug resistance of gastric carcinoma cells, and the codelivery nanosystem demonstrated potent reduction of P-glycoprotein efflux and increase of chemotherapy sensitivity for drug-resistant cells by silencing HIF-1 alpha gene (Chen et al., 2017). However, the efficacy of chitosan nanoparticles was only investigated in vitro, and toxicity was evaluated after treatment for 5 days.
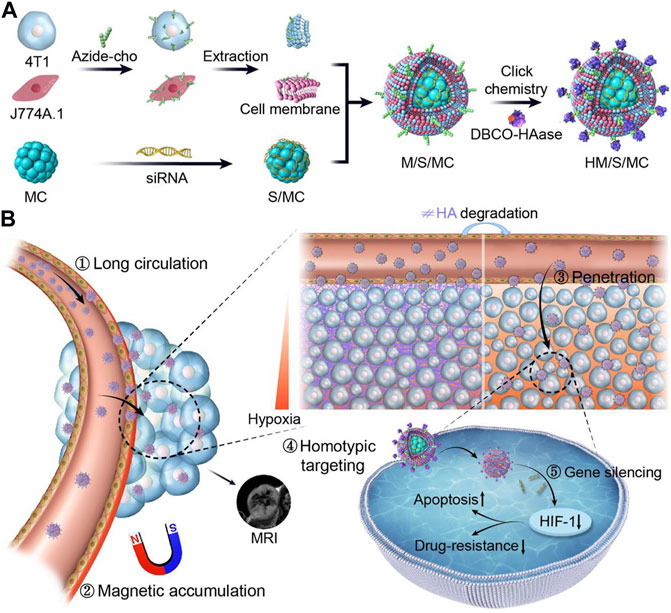
FIGURE 3. Schematic illustration of short interfering RNA (siRNA) loaded, HAase conjugated, and macrophage-cancer chimeric cytomembrane cloaked magnetic nanocluster (denoted as HM/S/MC) for delivery of HIF-1 siRNA to treat cancer. (A) Manufacturing procedures for HM/S/MC. (B) Programmed delivery performance and anticancer mechanisms in vivo for HM/S/MC (reproduced with permission from Lyu et al. (2020). Copyright 2020 Elsevier).
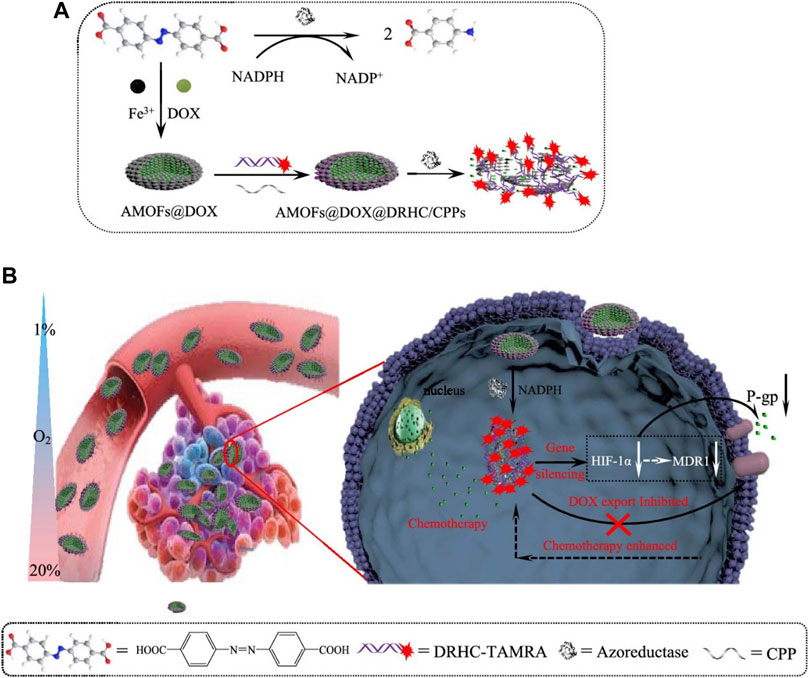
FIGURE 4. (A) Synthesis process and azoreductase responsive release under hypoxia for DOX, doxorubicin encapsulated, double chain DRHC, DNA/RNA hybridized complex consisted of HIF-1 alpha siRNA absorbed, and CPPs, cell-penetrating peptides decorated AMOFs, functional metal-organic frameworks based nanoparticles (DOX@AMOFs@DRHC/CPPs) (B) Cellular function scheme for hypoxia-responsive release of DOX and DRHC, downregulation of HIF-1 alpha, multidrug resistance 1 and P-gp, and reverse of chemoresistance for hypoxic tumor (reproduced with permission from Huang, et al. (2019). Copyright 2019 ACS Publications).
Yong et al. fabricated nanospheres with conjugation of Gd-containing polyoxometalates to chitosan, aiming at enhancing radiotherapy sensitivity, and the highly compressed and positively charged ion on the chitosan shell facilitates the transportation of HIF-1 alpha siRNA into the tumor (Yong et al., 2017). The nanospheres could reverse the radioresistance of hypoxic cancer cells via producing superabundant reactive oxygen species under X-ray radiation and lowering HIF-1 alpha expression to disturb self-restoration of impaired double-stranded DNA. Gold nanocomposites delivering HIF-1 alpha siRNA significantly improved the radiation sensitivity of hypoxic nasopharyngeal carcinoma cells (Xu et al., 2020). Nanoparticles codelivering radiosensitizer and HIF-1 alpha siRNA provide a versatile approach to solve the critical radioresistance issue for hypoxic tumors.
Nanoparticles for Photodynamic Therapy
Huang et al. constructed a smart nanovehicle that combined PDT and hypoxia-regulated gene therapy to achieve synergistic cancer therapy (Huang et al., 2018). Gold nanoparticles modified with beta cyclodextrins were chosen as nanocarriers, and a hypoxia-sensitive azobenzene labeled and hybridized double-stranded DNA/RNA conjugation consisting of ASO against HIF-1 alpha was bound to the surface of the gold nanoparticles through host-guest interaction between beta-cyclodextrin and azobenzene. The hypoxia-sensitive azobenzene linker was degraded under hypoxic conditions during PDT, generating hypoxia-stimulated release of ASO against HIF-1 alpha and synergistic effect. A pH-sensitive zeolitic imidazolate framework-8 nanoparticles were fabricated to convey chlorin e6, adriamycin and HIF-1 alpha siRNA to achieve simultaneous multiple therapeutic objectives (Wang et al., 2020). Specific knockdown of HIF-1 alpha simultaneously eliminates hypoxia-involved PDT resistance through inhibiting DNA repair and immobilization of DNA damage. The calcium phosphate core with a high affinity of nucleic acids was stabilized with dioleoylphosphatydic acid and coated with a cationic lipid to form lipid–calcium–phosphate nanoparticles, and the nanoparticles were decorated with anisamide for the delivery of HIF-1 alpha siRNA to sigma receptor-expressing cells which were simultaneously subjected to PDT (Chen W.-H. et al., 2015). The results demonstrated that knockdown of HIF-1 alpha reduced microvascular density during PDT, and a combination of silencing HIF-1 alpha gene therapy and PDT could enhance reactive oxygen species mediated PDT therapeutic efficacy. A new multifunctional cationic porphyrin grafted lipid that can self-assemble into microbubbles with conventional lipids was developed to load HIF-1 alpha siRNA through electrostatic adsorption of siRNA onto the amino group and exert PDT via porphyrin group as a photosensitizer (Sun et al., 2018). With the assistance of ultrasound-targeted microbubble destruction, the microbubbles were effectively converted into nanoparticles in situ. Downregulated HIF-1 alpha expression, which was partly generated by PDT, enhanced the PDT therapy. A2B2-type porphyrins were polymerized by a reactive oxygen species cleavable linker thioktal to form cationic polyporphyrins, which acted as both delivery carriers for HIF-1 alpha siRNA and photosensitive agent for PDT (Zheng et al., 2021). The polyphotosensitizer based gene nanocarriers, which can simultaneously impart photosensitizer with gene delivery capability and improve PDT effect through the silence of HIF-1 alpha exhibited efficient gene-silencing efficiency and synergistic anticancer potency for the combined PDT and gene therapy. The study provides a promising paradigm for the design of both the gene delivery vehicle and photosensitizer, as well as for broad utilities in combined treatment for cancer.
A trimodal graphene oxide nanocarrier for codelivery of a photosensitizer verteporfin, a hypoxia-activated prodrug banoxantrone dihydrochloride and HIF-1 alpha siRNA was fabricated (Figure 5) (Luan et al., 2018). c (RGDfK) peptides which targeted αvβ3 integrin overexpressed on the surface of vascular endothelial cells and cancer cells, were decorated on the graphene oxide nanovehicle. The targeted trimodal nanosystem suppressed HIF-1 alpha expression, upregulated CYP450, and increased prodrug activation. This study exploited vascular-targeted photodynamic therapy approved for treating solid tumors to induce hypoxia for successfully enhanced hypoxia-activated prodrug activation.
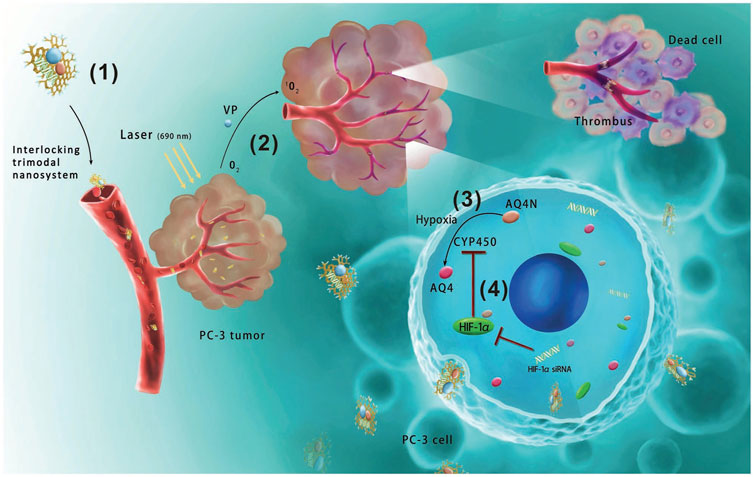
FIGURE 5. Schematic illustration of anticancer mechanisms for the interlocking trimodal c (RGDfK) modified graphene-oxide-based nanosystem for codelivery of VP, verteporfin, banoxantrone dihydrochloride (AQ4N). (1) The trimodal nanosystem targeted prostate cancer after intravenous injection. (2) VP-based photodynamic therapy aggravated hypoxia. (3) Hypoxia effectively transformed AQ4N into cytotoxic metabolite AQ4. (4) However, the upregulated HIF-1 alpha upon photodynamic therapy inhibited CYP450 reductases to prevent AQ4N transformation under hypoxia, hence coloaded HIF-1 alpha siRNA could upregulate CYP450 and reinforce AQ4N activation (reproduced with permission from Luan et al. (2018). Copyright 2018 WILEY-VCH Verlag GmbH and Co. KGaA). The targeted trimodal nanosystem decreased HIF-1 alpha expression in vivo, and the doubling time of tumor volume for the nanosystem was 17.7 days which was 2.4 folds longer than that of nontargeted drugs.
Hydrophilic HIF-1 alpha siRNA tethered to hydrophobic peptide nucleic acid-based B-cell lymphoma 2 (Bcl-2) ASO via 4,4-dimethyl-3,5-dithiaheptanedioic acid as singlet oxygen (1O2) sensitive linker self-assembled to generate photolabile spherical nucleic acid, and near-infrared photosensitizer was loaded in the hydrophobic core (Chen et al., 2021). Upon near-infrared light irradiation, the produced 1O2 cleaved the linker between siRNA and ASO and triggered the disassembly of spherical nucleic acid. The nanovehicle knockdown HIF-1 alpha and Bcl-2 expression and the combined gene therapy and PDT potently inhibited tumor growth in vitro and in vivo. In view of its carrier-free and biocompatibility, photolabile spherical nucleic acid represents a promising self-delivery nanoplatform to integrate various oligonucleotide drugs with a small molecule for cancer therapy.
Nanoformulations for Inhibiting Tumor Metastasis
Superparamagnetic iron oxide nanoparticles coated with thiolated chitosan and tirmethyl chitosan and further functionalized with hyaluronate and TAT peptide were adopted to deliver HIF-1 alpha siRNA and STAT3 siRNA (Figure 6) (Budi et al., 2021). Due to the agglomeration of superparamagnetic iron oxide colloidal suspensions, their application in the clinic is restricted. In this study, the superparamagnetic iron oxide surface was decorated with thiolated chitosan and trimethyl chitosan to suppress the agglomeration and enhance solubility. The polymers could also enhance cellular uptake by increasing the compression of nucleic acids. The mRNA levels of metastasis precursors MMP-2 and MMP-9 diminished considerably after the nanoparticles treatment, and minimal cell migration was observed. Zhao employed positively charged epsilon polylysine copolymer hybrid nanovehicles encapsulated by PEG-modified lipid bilayer for codelivering HIF-1 alpha siRNA and gemcitabine to treat pancreatic cancer (Zhao et al., 2015). The lipid–polymer hybrid nanoparticles, with bilayer lipid shell coated a polymeric core, and combined the merits of polymers and liposomes. The cationic polymeric core could absorb siRNA, and the lipid shell presented a protective effect. The nanosystem potently downregulated expression of HIF-1 alpha in vitro and in vivo and exhibited outstanding power to suppress cancer spreading in the orthotopic transplantation cancer model. The fifth-generation polyamidoamine dendritic polymers that were partially decorated by 1,3-propanesultone were employed for template fabrication of gold nanoparticles and obtained zwitterionic dendrimer-entrapped gold nanoparticles were applied to deliver HIF-1 alpha siRNA (Yang C. et al., 2021). Decoration of amine-terminated dendrimers with 1,3-propanesultone rendered the vector of antifouling property for enhanced serum transfection of HIF-1 alpha. The hybrid nanoparticles exhibited in effective silence of HIF-1 alpha, resulting in downregulation of a series of genes related to tumor metastasis and potent block of pulmonary metastasis. Hyaluronate and trimethyl chitosan-wrapped superparamagnetic iron oxide nanoparticles for loading HIF-1 alpha siRNA and prostaglandin E receptor 4 antagonist were developed for cancer treatment, and affinity of hyaluronate with CD44 overexpressed on carcinoma cells realized targeted delivery of siRNA to malignant cells and dramatically downregulated HIF-1 alpha (Karpisheh et al., 2021). Decoration of the nanoparticles with trimethyl chitosan increased their gene loading capacity owing to the positive charge, and hyaluronate on the nanoparticles increased cellular uptake of the nanoparticles. The combined therapy of inhibiting HIF-1 alpha and prostaglandin E receptor 4 axis by the drug delivery nanosystem prevented tumor migration and invasion by downregulation of MMP-2 and MMP-9 genes and decreased mRNA expression levels of factors involved in angiogenesis. Hyaluronic acid encapsulated chitosan carrying HIF-1 alpha siRNA complexes was developed as an innovative therapeutic strategy targeting HIF-1 alpha signal pathway to treat uveal melanoma (Xie et al., 2020). Coating chitosan with anionic hyaluronic acid shielded the cationic charge of the chitosan/siRNA nanocomplex and facilitated cellular uptake and lysosomal escape ability. The HIF-1 alpha siRNA delivering complexes is low toxic and demonstrated outstanding cellular uptake and lysosome escape capability. The expression of HIF-1 alpha and VEGF were downregulated in uveal melanoma cells, and migration and invasion were successfully inhibited.
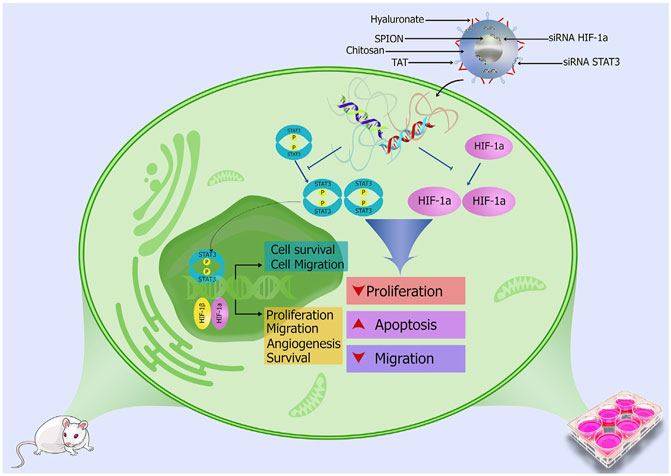
FIGURE 6. HIF-1 alpha siRNA and STAT3 siRNA loaded superparamagnetic iron oxide nanoparticles coated with thiolated chitosan and tirmethyl chitosan and further functionalized with hyaluronate and TAT peptide suppresses cancer development and metastasis (reproduced with permission from Budi et al. (2021). Copyright 2021 Elsevier).
Nanoformulations for Inhibiting Proliferation and Promoting Apoptosis
A DNA strand with high content of GAG was linked to a DNA chain of enriched GC to synthesize functional nanoaptamer with magnetism (DNA-Fe3O4) and to maintain the intrinsic feature for targeting aptamer (Figure 7) (Yang et al., 2018). Hydrophobic fluorescent dye was loaded into DNA-Fe3O4 by the enriched GC sequences, and the nanoaptamers loading fluorescent dye self-assembled with target HIF and glucose transporter 1 aptamers. In general, aptamer conjugates to the surface of nanoparticles by C-S bond, S-S bond, or amide bond. However, it is difficult to keep the innate characteristics for targeting aptamer after chemical modification. Integrating aptamer with magnetic nanoparticles by the self-assembly of aptamers and metals keeps the innate properties of aptamers. The functional DNA-Fe3O4 transported HIF siRNA and glucose transporter 1 siRNA to attenuate HIF-1 alpha and ATP, resulting in the restraint of breast carcinoma cells proliferation in vitro and in vivo. Folic acid-modified PEG-chitosan oligosaccharide lactate nanoparticles were fabricated to deliver HIF-1 alpha siRNA to folic acid acceptors expressing human ovarian endometrioid carcinoma cells, and gene knockdown of HIF-1 alpha resulted in significant suppression of the cells proliferation in vitro (Li et al., 2014). Low molecular chitosan oligosaccharide lactate was chosen in this study over traditional chitosan due to its high solubility in water with low viscosity and a high degree of deacetylation, which presents more amino groups and enables a stronger interaction with siRNA. Li et al. designed lipid nanocarriers that integrated positively charged conjugation of human serum albumin and pentaethylenehexamine to form electrostatic complexes with ASO against HIF-1 alpha, and the lipid nanoparticles suppressed KB cell proliferation in vitro and in vivo (Li et al., 2016). The application of lipid nanoparticles for siRNA delivery is limited because of low delivery efficiency and systemic toxicity. Cationic albumin− pentaethylenehexamine conjugate incorporated with lipid nanoparticles overcame this problem and facilitated the delivery of siRNA. An original biodegradable D-alpha-tocopheryl PEG 1000 succinate-b-poly (epsilon-caprolactone-ran-glycolide) nanoparticles were fabricated to deliver HIF-1 alpha siRNA for the nasopharyngeal neoplasm gene therapeutics. The nanoparticles significantly reduced the proliferation of CNE-2 cells in vitro and in vivo (Chen Y. et al., 2015). D-alpha-tocopheryl PEG 1000 succinate as an excipient can induce cancer cell apoptosis, and it has been applied in combination with other anticancer agents for synergistic effects. pH-sensitive hybrid quantum dots consisted of cadmium tellurium quantum dots as a core and 2-deoxyglucose decorated PEG which was further tethered to lipoic acid, lysine, and 9-poly-d-arginine with a linker hydrazone bond as a functional shell were developed for targeting delivery of HIF-1 alpha siRNA to hypoxic cancer cells (Zhu et al., 2015). 2-deoxyglucose as a glucose analog conferred recognition and transportation of nanodrugs into hypoxic cells by glucose transporters one on the cell membrane, and poly-d-arginine enhanced transfection efficiency. 2-deoxyglucose and hydrazone conferred hypoxic cancer cell targeting and pH-sensitive profiles of the quantum dots, and the hybrid quantum dots exhibited high HIF-1 alpha gene-silencing efficiency and potent antitumor efficacy in vivo. The hybrid quantum dots combined the efficacy of hypoxic tumor targeting and pH-responsive properties, and reduced toxicity to normal tissues. (1-aminoethyl)iminobis [N-(oleicylcysteinylhistinyl-1-aminoethyl)propionamide] (EHCO) formed stable nanoparticles with HIF-1 alpha siRNA, and bombesin or RGD peptide was tethered to nanoparticles surfaces via a PEG spacer (Wang et al., 2009), the peptide-targeted delivery systems resulted in remarkable suppression on human glioma propagation in nude mice xenografts. This targeted delivery system demonstrated advantages of easy modification in formulations, pH-sensitive amphiphilic endosomal-lysosomal escape, low toxicity, and high siRNA delivery efficiency.
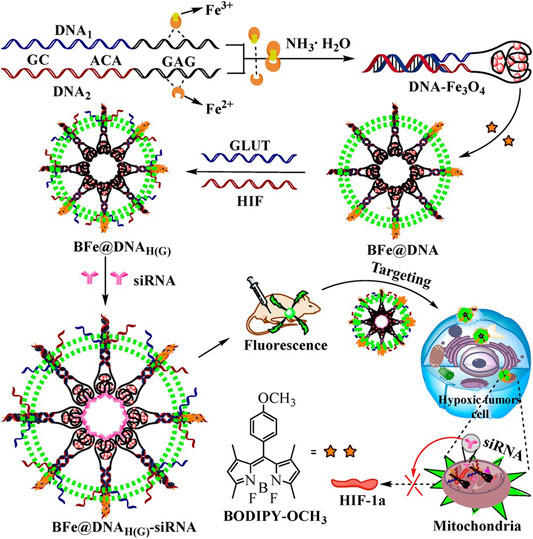
FIGURE 7. An enriched GAG DNA was linked to a GC-rich DNA to synthesize a functional nanoaptamer with magnetism (DNA-Fe3O4), and the obtained DNA-Fe3O4 delivered BODIPY-OCH3 dye by the enriched GC sequences was denoted as BFe@DNA. The fluorescent nanoaptamers self-assembled with target HIF-1 alpha and glucose transporter one aptamers to form BFe@DNAH(G), and HIF-1 alpha siRNA and glucose transporter one siRNA loaded nanoaptamer denoted as BFe@DNAH(G)-siRNA (reproduced with permission from Yang, et al. (2018). Copyright 2018 ACS Publications).
GE-11 peptide conjugated cationic liposomes were prepared to load gemcitabine, and HIF-1 alpha siRNA by Lin et al., and the liposomes effectively downregulated HIF-1 alpha gene expression in pancreatic carcinoma cells, declined cancer cell proliferations, and increased the apoptotic cells number with higher percentage of terminal apoptosis (Lin et al., 2019). The liposome is one of the widely applied nanocarriers for drug delivery owing to its excellent characteristics, including easy decoration on the surface. In this research, GE-11 peptide, which showed specific affinity toward epidermal growth factor receptor, was conjugated to liposome to realize targeting delivery. Yao et al. developed a death receptor 5 specific antibodies modified pH-responsive liposomes for simultaneous delivery of cyclopeptide RA-V and ASO against HIF-1 alpha to attenuate the tumor hypoxia microenvironment and to enhance chemotherapy effects in tumors (Yao et al., 2020). The liposomes achieved significant HIF-1 alpha silence, and silence of HIF-1 alpha promoted apoptosis induced by cyclopeptide RA-V. The pH-responsive liposomes also achieved therapeutic self-monitoring using dynamic visualization of the caspase-8 activation in situ. Folate-decorated cationic liposomes for HIF-1 alpha siRNA delivery induced apoptosis and inhibited the proliferation of hypoxic tolerant melanoma cells in vitro (Chen et al., 2016). However, the transfection efficacy and anticancer potency for the cationic liposomes were not evaluated in vivo.
Conclusion and Perspectives
In recent decades, prominent advances have been achieved in nanomedicine strategies for the delivery of HIF-1 alpha siRNA and ASO in tumor therapy. Recent advances in the fields of nanoparticles loading HIF-1 alpha siRNA and ASO for cancer immunotherapy, antiangiogenesis, reversal of multidrug resistance and radioresistance, potentiating photodynamic therapy, inhibiting tumor metastasis and proliferation, and enhancing apoptosis are reviewed in this thesis. However, academic investigation and particularly clinical translation remain huge challenges.
First, the traits for tumor hypoxia are the heterogeneity of tumor species and clinical stages, and also sterically heterogeneous within a tumor (Rickard et al., 2019; Ron et al., 2019), and hypoxia and the HIF pathway in the tumor microenvironment are far from clearly understood. Therefore, the design of nanovehicles for HIF-1 alpha siRNA and ASO delivery and integration with diverse therapeutic tactics should be on the basis of further fundamental theoretical study on oncology biology, pathology, and clinical stage. Second, although great advances are achieved in siRNA and ASO delivery, there are still formidable challenges for targeting and effective delivery of siRNA in therapeutics. The manufacturing techniques of reported nanoparticles for delivering HIF siRNA and ASO are complicated and difficult to scale up from laboratory scale to industrial scale. The biocompatibility, biodegradability, safety, targeting ability, and efficacy in vivo, especially in humans, should be carefully evaluated. Simple, safe, and efficient nanovehicles with biocompatibility and biodegradability are needed to be developed in the future for targeting HIF therapy. Last but equally important, druggability is supposed to be highly concerned in the academic investigation of nanodrugs for HIF-1 alpha siRNA and ASO delivery. Although a large number of nanoparticles carrying HIF-1 alpha siRNA and ASO have been developed in animal models; however, these nanoparticles are far from a clinical candidate for cancer treatment owing to complex manufacturing techniques and undefined safety. In 2022, Clinicaltrials.gov exhibits no trial for translation of HIF-1 alpha siRNA or HIF-1 alpha ASO to the clinic in progress.
In conclusion, HIF plays a significant role in promoting tumors, and nanotechnologies for delivery of HIF-1 alpha siRNA and ASO for cancer treatment have achieved prosperous accomplishments in fields of fundamental research. We expect that more simple, safe, and potent nanovehicles loading HIF-1 alpha siRNA and ASO for effectively silencing HIF-1 alpha on the basis of further fundamental study progress on oncology biology, pathology, and stage are fabricated and translated into clinical therapy in a short period, and more oncology patients obtain the benefits of gene therapy and nanotechnologies.
Author Contributions
XC directed the review topic and revised the manuscript, YY, HL, and HY searched the literature and wrote the manuscript, and HY revised the manuscript.
Funding
This work was supported by the National Natural Science Foundation of China (81503010) and the scientific and technological foundation of Shaanxi Province (2021SF-300).
Conflict of Interest
The authors declare that the research was conducted in the absence of any commercial or financial relationships that could be construed as a potential conflict of interest.
Publisher’s Note
All claims expressed in this article are solely those of the authors and do not necessarily represent those of their affiliated organizations or those of the publisher, the editors, and the reviewers. Any product that may be evaluated in this article, or claim that may be made by its manufacturer, is not guaranteed or endorsed by the publisher.
References
Albanese, A., Daly, L. A., Mennerich, D., Kietzmann, T., and Sée, V. (2021). The Role of Hypoxia-Inducible Factor Post-Translational Modifications in Regulating its Localisation, Stability, and Activity. Ijms 22, 268. doi:10.3390/ijms22010268
Arnold, A. E., Czupiel, P., and Shoichet, M. (2017). Engineered Polymeric Nanoparticles to Guide the Cellular Internalization and Trafficking of Small Interfering Ribonucleic Acids. J. Control. Release 259, 3–15. doi:10.1016/j.jconrel.2017.02.019
Bahrami, A., Atkin, S. L., Majeed, M., and Sahebkar, A. (2018). Effects of Curcumin on Hypoxia-Inducible Factor as a New Therapeutic Target. Pharmacol. Res. 137, 159–169. doi:10.1016/j.phrs.2018.10.009
Bernkop-Schnürch, A. (2018). Strategies to Overcome the Polycation Dilemma in Drug Delivery. Adv. Drug Deliv. Rev. 136-137, 62–72. doi:10.1016/j.addr.2018.07.017
Bousquet, M. S., Ma, J. J., Ratnayake, R., Havre, P. A., Yao, J., Dang, N. H., et al. (2016). Multidimensional Screening Platform for Simultaneously Targeting Oncogenic KRAS and Hypoxia-Inducible Factors Pathways in Colorectal Cancer. ACS Chem. Biol. 11, 1322–1331. doi:10.1021/acschembio.5b00860
Budi, H. S., Izadi, S., Timoshin, A., Asl, S. H., Beyzai, B., Ghaderpour, A., et al. (2021). Blockade of HIF-1α and STAT3 by Hyaluronate-Conjugated TAT-Chitosan-SPION Nanoparticles Loaded with siRNA Molecules Prevents Tumor Growth. Nanomedicine Nanotechnol. Biol. Med. 34, 102373. doi:10.1016/j.nano.2021.102373
Charbe, N. B., Amnerkar, N. D., Ramesh, B., Tambuwala, M. M., Bakshi, H. A., Aljabali, A. A. A., et al. (2020). Small Interfering RNA for Cancer Treatment: Overcoming Hurdles in Delivery. Acta Pharm. Sin. B 10, 2075–2109. doi:10.1016/j.apsb.2020.10.005
Chen, D., Wu, Y.-X., Qiu, Y.-b., Wan, B.-b., Liu, G., Chen, J.-l., et al. (2020). Hyperoside Suppresses Hypoxia-Induced A549 Survival and Proliferation through Ferrous Accumulation via AMPK/HO-1 axis. Phytomedicine 67, 153138. doi:10.1016/j.phymed.2019.153138
Chen, L., Li, G., Wang, X., Li, J., and Zhang, Y. (2021). Spherical Nucleic Acids for Near-Infrared Light-Responsive Self-Delivery of Small-Interfering RNA and Antisense Oligonucleotide. ACS Nano 15, 11929–11939. doi:10.1021/acsnano.1c03072
Chen, L., Manautou, J. E., Rasmussen, T. P., and Zhong, X.-b. (2019). Development of Precision Medicine Approaches Based on Inter-individual Variability of BCRP/ABCG2. Acta Pharm. Sin. B 9, 659–674. doi:10.1016/j.apsb.2019.01.007
Chen, W.-H., Lecaros, R. L. G., Tseng, Y.-C., Huang, L., and Hsu, Y.-C. (2015). Nanoparticle Delivery of HIF1α siRNA Combined with Photodynamic Therapy as a Potential Treatment Strategy for Head-And-Neck Cancer. Cancer Lett. 359, 65–74. doi:10.1016/j.canlet.2014.12.052
Chen, Y., Sun, L., Guo, D., Wu, Z., and Chen, W. (2017). Co-delivery of Hypoxia Inducible Factor-1α Small Interfering RNA and 5-fluorouracil to Overcome Drug Resistance in Gastric Cancer SGC-7901 Cells. J. Gene Med. 19, e2998. doi:10.1002/jgm.2998
Chen, Y., Xu, G., Zheng, Y., Yan, M., Li, Z., Zhou, Y., et al. (2015). Nanoformulation of D-α-Tocopheryl Polyethylene Glycol 1000 Succinate-B-Poly(ε-Caprolactone-Ran-Glycolide) Diblock Copolymer for siRNA Targeting HIF-1α for Nasopharyngeal Carcinoma Therapy. Int. J. Nanomedicine 10, 1375–1386. doi:10.2147/IJN.S76092
Chen, Z., Zhang, T., Wu, B., and Zhang, X. (2016). Insights into the Therapeutic Potential of Hypoxia-Inducible Factor-1 alpha; Small Interfering RNA in Malignant Melanoma Delivered via Folate-Decorated Cationic Liposomes. Int. J. Nanomedicine 11, 991–1002. doi:10.2147/IJN.S101872
Cheng, N., Bai, X., Shu, Y., Ahmad, O., and Shen, P. (2021). Targeting Tumor-Associated Macrophages as an Antitumor Strategy. Biochem. Pharmacol. 183, 114354. doi:10.1016/j.bcp.2020.114354
Cheng, X., Li, H., Ge, X., Chen, L., Liu, Y., Mao, W., et al. (2020). Tumor-Microenvironment- Responsive Size-Shrinkable Drug-Delivery Nanosystems for Deepened Penetration into Tumors. Front. Mol. Biosci. 7, 576420. doi:10.3389/fmolb.2020.576420
Chi, X., Gatti, P., and Papoian, T. (2017). Safety of Antisense Oligonucleotide and siRNA-Based Therapeutics. Drug Discov. Today 22, 823–833. doi:10.1016/j.drudis.2017.01.013
Choudhry, H., and Harris, A. L. (2018). Advances in Hypoxia-Inducible Factor Biology. Cell Metab. 27, 281–298. doi:10.1016/j.cmet.2017.10.005
Dang, J., He, H., Chen, D., and Yin, L. (2017). Manipulating Tumor Hypoxia toward Enhanced Photodynamic Therapy (PDT). Biomater. Sci. 5, 1500–1511. doi:10.1039/c7bm00392g
Daniel, S. K., Sullivan, K. M., Labadie, K. P., and Pillarisetty, V. G. (2019). Hypoxia as a Barrier to Immunotherapy in Pancreatic Adenocarcinoma. Clin. Transl. Med. 8, 10. doi:10.1186/s40169-019-0226-9
DePeaux, K., and Delgoffe, G. M. (2021). Metabolic Barriers to Cancer Immunotherapy. Nat. Rev. Immunol. 21, 785–797. doi:10.1038/s41577-021-00541-y
D’Ignazio, L., Batie, M., and Rocha, S. (2017). Hypoxia and Inflammation in Cancer, Focus on HIF and NF-κB. Biomedicines 5, 21. doi:10.3390/biomedicines5020021
Dong, Y., Siegwart, D. J., and Anderson, D. G. (2019). Strategies, Design, and Chemistry in siRNA Delivery Systems. Adv. Drug Deliv. Rev. 144, 133–147. doi:10.1016/j.addr.2019.05.004
Epstein, A. C. R., Gleadle, J. M., McNeill, L. A., Hewitson, K. S., O'Rourke, J., Mole, D. R., et al. (2001). C. elegans EGL-9 and Mammalian Homologs Define a Family of Dioxygenases that Regulate HIF by Prolyl Hydroxylation. Cell 107, 43–54. doi:10.1016/s0092-8674(01)00507-4
Erler, J. T., Cawthorne, C. J., Williams, K. J., Koritzinsky, M., Wouters, B. G., Wilson, C., et al. (2004). Hypoxia-mediated Down-Regulation of Bid and Bax in Tumors Occurs via Hypoxia-Inducible Factor 1-dependent and -independent Mechanisms and Contributes to Drug Resistance. Mol. Cell. Biol. 24, 2875–2889. doi:10.1128/mcb.24.7.2875-2889.2004
Fan, H., Yan, G., Zhao, Z., Hu, X., Zhang, W., Liu, H., et al. (2016). A Smart Photosensitizer-Manganese Dioxide Nanosystem for Enhanced Photodynamic Therapy by Reducing Glutathione Levels in Cancer Cells. Angew. Chem. Int. Ed. 55, 5477–5482. doi:10.1002/anie.201510748
Fathi, M., Bahmanpour, S., Barshidi, A., Rasouli, H., Karoon Kiani, F., Mahmoud Salehi Khesht, A., et al. (2021a). Simultaneous Blockade of TIGIT and HIF-1α Induces Synergistic Anti-tumor Effect and Decreases the Growth and Development of Cancer Cells. Int. Immunopharmacol. 101, 108288. doi:10.1016/j.intimp.2021.108288
Fathi, M., Pustokhina, I., Kuznetsov, S. V., Khayrullin, M., Hojjat‐Farsangi, M., Karpisheh, V., et al. (2021b). T‐cell Immunoglobulin and ITIM Domain, as a Potential Immune Checkpoint Target for Immunotherapy of Colorectal Cancer. IUBMB Life 73, 726–738. doi:10.1002/iub.2461
Fradette, C., and Souich, P. (2004). Effect of Hypoxia on Cytochrome P450 Activity and Expression. Cdm 5, 257–271. doi:10.2174/1389200043335577
Gillespie, D. L., Aguirre, M. T., Ravichandran, S., Leishman, L. L., Berrondo, C., Gamboa, J. T., et al. (2015). RNA Interference Targeting Hypoxia-Inducible Factor 1α via a Novel Multifunctional Surfactant Attenuates Glioma Growth in an Intracranial Mouse Model. Jns 122, 331–341. doi:10.3171/2014.10.JNS132363
Gonzalez, F. J., Xie, C., and Jiang, C. (2018). The Role of Hypoxia-Inducible Factors in Metabolic Diseases. Nat. Rev. Endocrinol. 15, 21–32. doi:10.1038/s41574-018-0096-z
Gonzalez-Avila, G., Sommer, B., García-Hernández, A. A., and Ramos, C. (2020). Matrix Metalloproteinases' Role in Tumor Microenvironment. Adv. Exp. Med. Biol. 1245, 97–131. doi:10.1007/978-3-030-40146-7_5
Hajizadeh, F., Moghadaszadeh Ardebili, S., Baghi Moornani, M., Masjedi, A., Atyabi, F., Kiani, M., et al. (2020). Silencing of HIF-1α/CD73 axis by siRNA-Loaded TAT-Chitosan-Spion Nanoparticles Robustly Blocks Cancer Cell Progression. Eur. J. Pharmacol. 882, 173235. doi:10.1016/j.ejphar.2020.173235
Hou, L., Gong, X., Yang, J., Zhang, H., Yang, W., and Chen, X. (2022). Hybrid‐Membrane‐Decorated Prussian Blue for Effective Cancer Immunotherapy via Tumor‐Associated Macrophages Polarization and Hypoxia Relief. Adv. Mater. 34, 2200389. doi:10.1002/adma.202200389
Huang, C., Tan, W., Zheng, J., Zhu, C., Huo, J., and Yang, R. (2019). Azoreductase-Responsive Metal-Organic Framework-Based Nanodrug for Enhanced Cancer Therapy via Breaking Hypoxia-Induced Chemoresistance. ACS Appl. Mat. Interfaces 11, 25740–25749. doi:10.1021/acsami.9b08115
Huang, C., Zheng, J., Ma, D., Liu, N., Zhu, C., Li, J., et al. (2018). Hypoxia-triggered Gene Therapy: a New Drug Delivery System to Utilize Photodynamic-Induced Hypoxia for Synergistic Cancer Therapy. J. Mat. Chem. B 6, 6424–6430. doi:10.1039/c8tb01805g
Huang, R.-X., and Zhou, P.-K. (2020). DNA Damage Response Signaling Pathways and Targets for Radiotherapy Sensitization in Cancer. Sig Transduct. Target Ther. 5, 60. doi:10.1038/s41392-020-0150-x
Hubbi, M. E., and Semenza, G. L. (2015). Regulation of Cell Proliferation by Hypoxia-Inducible Factors. Am. J. Physiology-Cell Physiology 309, C775–C782. doi:10.1152/ajpcell.0027910.1152/ajpcell.00279.2015
Iacob, A. T., Lupascu, F. G., Apotrosoaei, M., Vasincu, I. M., Tauser, R. G., Lupascu, D., et al. (2021). Recent Biomedical Approaches for Chitosan Based Materials as Drug Delivery Nanocarriers. Pharmaceutics 13, 587. doi:10.3390/pharmaceutics13040587
Ivan, M., Kondo, K., Yang, H., Kim, W., Valiando, J., Ohh, M., et al. (2001). HIFα Targeted for VHL-Mediated Destruction by Proline Hydroxylation: Implications for O2 Sensing. Science 292, 464–468. doi:10.1126/science.1059817
Izadi, S., Moslehi, A., Kheiry, H., Karoon Kiani, F., Ahmadi, A., Masjedi, A., et al. (2020). Codelivery of HIF-1α siRNA and Dinaciclib by Carboxylated Graphene Oxide-Trimethyl Chitosan-Hyaluronate Nanoparticles Significantly Suppresses Cancer Cell Progression. Pharm. Res. 37, 196. doi:10.1007/s11095-020-02892-y
Jadidi-Niaragh, F., Atyabi, F., Rastegari, A., Kheshtchin, N., Arab, S., Hassannia, H., et al. (2017). CD73 Specific siRNA Loaded Chitosan Lactate Nanoparticles Potentiate the Antitumor Effect of a Dendritic Cell Vaccine in 4T1 Breast Cancer Bearing Mice. J. Control. Release 246, 46–59. doi:10.1016/j.jconrel.2016.12.012
Jayaprakash, P., Ai, M., Liu, A., Budhani, P., Bartkowiak, T., Sheng, J., et al. (2018). Targeted Hypoxia Reduction Restores T Cell Infiltration and Sensitizes Prostate Cancer to Immunotherapy. J. Clin. Invest. 128, 5137–5149. doi:10.1172/JCI96268
Jing, X., Yang, F., Shao, C., Wei, K., Xie, M., Shen, H., et al. (2019). Role of Hypoxia in Cancer Therapy by Regulating the Tumor Microenvironment. Mol. Cancer 18, 157. doi:10.1186/s12943-019-1089-9
Karpisheh, V., Fakkari Afjadi, J., Nabi Afjadi, M., Haeri, M. S., Abdpoor Sough, T. S., Heydarzadeh Asl, S., et al. (2021). Inhibition of HIF-1α/EP4 axis by Hyaluronate-Trimethyl Chitosan-SPION Nanoparticles Markedly Suppresses the Growth and Development of Cancer Cells. Int. J. Biol. Macromol. 167, 1006–1019. doi:10.1016/j.ijbiomac.2020.11.056
Kelly, B. D., Hackett, S. F., Hirota, K., Oshima, Y., Cai, Z., Berg-Dixon, S., et al. (2003). Cell Type-specific Regulation of Angiogenic Growth Factor Gene Expression and Induction of Angiogenesis in Nonischemic Tissue by a Constitutively Active Form of Hypoxia-Inducible Factor 1. Circulation Res. 93, 1074–1081. doi:10.1161/01.RES.0000102937.50486.1B
Kheshtchin, N., and Hadjati, J. (2022). Targeting Hypoxia and Hypoxia‐inducible Factor‐1 in the Tumor Microenvironment for Optimal Cancer Immunotherapy. J. Cell. Physiology 237, 1285–1298. doi:10.1002/jcp.30643
Lecoultre, M., Dutoit, V., and Walker, P. R. (2020). Phagocytic Function of Tumor-Associated Macrophages as a Key Determinant of Tumor Progression Control: a Review. J. Immunother. Cancer 8, e001408. doi:10.1136/jitc-2020-001408
Li, H., Quan, J., Zhang, M., Yung, B. C., Cheng, X., Liu, Y., et al. (2016). Lipid-Albumin Nanoparticles (LAN) for Therapeutic Delivery of Antisense Oligonucleotide against HIF-1α. Mol. Pharm. 13, 2555–2562. doi:10.1021/acs.molpharmaceut.6b00363
Li, J., Shang, W., Li, Y., Fu, S., Tian, J., and Lu, L. (2018). Advanced Nanomaterials Targeting Hypoxia to Enhance Radiotherapy. Int. J. Nanomedicine 13, 5925–5936. doi:10.2147/IJN.S173914
Li, T. S. C., Yawata, T., and Honke, K. (2014). Efficient siRNA Delivery and Tumor Accumulation Mediated by Ionically Cross-Linked Folic Acid-Poly(ethylene Glycol)-Chitosan Oligosaccharide Lactate Nanoparticles: For the Potential Targeted Ovarian Cancer Gene Therapy. Eur. J. Pharm. Sci. 52, 48–61. doi:10.1016/j.ejps.2013.10.011
Lian, D., Chen, Y., Xu, G., Zeng, X., Li, Z., Li, Z., et al. (2016). Delivery of siRNA Targeting HIF-1α Loaded Chitosan Modifiedd-α-Tocopheryl Polyethylene Glycol 1000 Succinate-B-Poly(ε-Caprolactone-Ran-Glycolide) Nanoparticles into Nasopharyngeal Carcinoma Cell to Improve the Therapeutic Efficacy of Cisplatin. RSC Adv. 6, 37740–37749. doi:10.1039/C6RA03440C
Lin, C., Hu, Z., Yuan, G., Su, H., Zeng, Y., Guo, Z., et al. (2019). HIF1α-siRNA and Gemcitabine Combination-Based GE-11 Peptide Antibody-Targeted Nanomedicine for Enhanced Therapeutic Efficacy in Pancreatic Cancers. J. Drug Target. 27, 797–805. doi:10.1080/1061186X.2018.1552276
Liu, L., Ye, Y., and Zhu, X. (2019). MMP-9 Secreted by Tumor Associated Macrophages Promoted Gastric Cancer Metastasis through a PI3K/AKT/Snail Pathway. Biomed. Pharmacother. 117, 109096. doi:10.1016/j.biopha.2019.109096
Liu, X.-Q., Xiong, M.-H., Shu, X.-T., Tang, R.-Z., and Wang, J. (2012). Therapeutic Delivery of siRNA Silencing HIF-1 Alpha with Micellar Nanoparticles Inhibits Hypoxic Tumor Growth. Mol. Pharm. 9, 2863–2874. doi:10.1021/mp300193f
Liu, Y., Pan, Y., Cao, W., Xia, F., Liu, B., Niu, J., et al. (2019). A Tumor Microenvironment Responsive Biodegradable CaCO3/MnO2- Based Nanoplatform for the Enhanced Photodynamic Therapy and Improved PD-L1 Immunotherapy. Theranostics 9, 6867–6884. doi:10.7150/thno.37586
Losman, J.-A., Koivunen, P., and Kaelin, W. G. (2020). 2-Oxoglutarate-dependent Dioxygenases in Cancer. Nat. Rev. Cancer 20, 710–726. doi:10.1038/s41568-020-00303-3
Luan, X., Guan, Y.-Y., Liu, H.-J., Lu, Q., Zhao, M., Sun, D., et al. (2018). A Tumor Vascular-Targeted Interlocking Trimodal Nanosystem that Induces and Exploits Hypoxia. Adv. Sci. 5, 1800034. doi:10.1002/advs.201800034
Luo, W., and Wang, Y. (2018). Epigenetic Regulators: Multifunctional Proteins Modulating Hypoxia-Inducible Factor-α Protein Stability and Activity. Cell. Mol. Life Sci. 75, 1043–1056. doi:10.1007/s00018-017-2684-9
Lyu, C., Lu, G., Bao, W., Li, F., Wang, S., Zhang, F., et al. (2020). Engineering Magnetosomes with Chimeric Membrane and Hyaluronidase for Efficient Delivery of HIF-1 siRNA into Deep Hypoxic Tumors. Chem. Eng. J. 398, 125453. doi:10.1016/j.cej.2020.125453
Ma, L., Li, G., Zhu, H., Dong, X., Zhao, D., Jiang, X., et al. (2014). 2-Methoxyestradiol Synergizes with Sorafenib to Suppress Hepatocellular Carcinoma by Simultaneously Dysregulating Hypoxia-Inducible Factor-1 and -2. Cancer Lett. 355, 96–105. doi:10.1016/j.canlet.2014.09.011
Ma, Z., Wang, L. Z., Cheng, J.-T., Lam, W. S. T., Ma, X., Xiang, X., et al. (2021). Targeting Hypoxia-Inducible Factor-1-Mediated Metastasis for Cancer Therapy. Antioxidants Redox Signal. 34, 1484–1497. doi:10.1089/ars.2019.7935
Mahdi, A., Darvishi, B., Majidzadeh-A, K., Salehi, M., and Farahmand, L. (2019). Challenges Facing Antiangiogenesis Therapy: The Significant Role of Hypoxia-Inducible Factor and MET in Development of Resistance to Anti-vascular Endothelial Growth Factor-Targeted Therapies. J. Cell. Physiol. 234, 5655–5663. doi:10.1002/jcp.27414
Malamas, A. S., Jin, E., Gujrati, M., and Lu, Z.-R. (2016). Dynamic Contrast Enhanced MRI Assessing the Antiangiogenic Effect of Silencing HIF-1α with Targeted Multifunctional ECO/siRNA Nanoparticles. Mol. Pharm. 13, 2497–2506. doi:10.1021/acs.molpharmaceut.6b00227
Maynard, M. A., Evans, A. J., Shi, W., Kim, W. Y., Liu, F.-F., and Ohh, M. (2007). Dominant-Negative HIF-3 alpha 4 Suppresses VHL-Null Renal Cell Carcinoma Progression. Cell Cycle 6, 2810–2816. doi:10.4161/cc.6.22.4947
Mi, P. (2020). Stimuli-responsive Nanocarriers for Drug Delivery, Tumor Imaging, Therapy and Theranostics. Theranostics 10, 4557–4588. doi:10.7150/thno.38069
Mohapatra, A., Sathiyamoorthy, P., and Park, I.-K. (2021). Metallic Nanoparticle-Mediated Immune Cell Regulation and Advanced Cancer Immunotherapy. Pharmaceutics 13, 1867. doi:10.3390/pharmaceutics13111867
Nowak, M., and Klink, M. (2020). The Role of Tumor-Associated Macrophages in the Progression and Chemoresistance of Ovarian Cancer. Cells 9, 1299. doi:10.3390/cells9051299
Osipova, O., Sharoyko, V., Zashikhina, N., Zakharova, N., Tennikova, T., Urtti, A., et al. (2020). Amphiphilic Polypeptides for VEGF siRNA Delivery into Retinal Epithelial Cells. Pharmaceutics 12, 39. doi:10.3390/pharmaceutics12010039
Piret, J. P., Minet, E., Cosse, J. P., Ninane, N., Debacq, C., Raes, M., et al. (2005). Hypoxia-inducible Factor-1-dependent Overexpression of Myeloid Cell Factor-1 Protects Hypoxic Cells against Tert-Butyl Hydroperoxide-Induced Apoptosis. J. Biol. Chem. 280, 9336–9344. doi:10.1074/jbc.M411858200
Ray, S. K., and Mukherjee, S. (2022). Directing Hypoxic Tumor Microenvironment and HIF to Illuminate Cancer Immunotherapy's Existing Prospects and Challenges in Drug Targets. Cdt 23, 471–485. doi:10.2174/1389450123666220111114649
Rickard, A. G., Palmer, G. M., and Dewhirst, M. W. (2019). Clinical and Pre-clinical Methods for Quantifying Tumor Hypoxia. Adv. Exp. Med. Biol. 1136, 19–41. doi:10.1007/978-3-030-12734-3_2
Ron, A., Deán-Ben, X. L., Gottschalk, S., and Razansky, D. (2019). Volumetric Optoacoustic Imaging Unveils High-Resolution Patterns of Acute and Cyclic Hypoxia in a Murine Model of Breast Cancer. Cancer Res. 79, 4767–4775. doi:10.1158/0008-5472.CAN-18-3769
Rowatt, A. J., Depowell, J. J., and Powell, W. H. (2003). ARNT Gene Multiplicity in Amphibians: Characterization of ARNT2 from the Frog Xenopus laevis. J. Exp. Zool. B Mol. Dev. Evol. 300, 48–57. doi:10.1002/jez.b.45
Şalva, E., Turan, S. Ö., Eren, F., and Akbuğa, J. (2015). The Enhancement of Gene Silencing Efficiency with Chitosan-Coated Liposome Formulations of siRNAs Targeting HIF-1α and VEGF. Int. J. Pharm. 478, 147–154. doi:10.1016/j.ijpharm.2014.10.065
Sasaki, S., and Guo, S. (2018). Nucleic Acid Therapies for Cystic Fibrosis. Nucleic Acid. Ther. 28, 1–9. doi:10.1089/nat.2017.0696
Saxena, K., Jolly, M. K., and Balamurugan, K. (2020). Hypoxia, Partial EMT and Collective Migration: Emerging Culprits in Metastasis. Transl. Oncol. 13, 100845. doi:10.1016/j.tranon.2020.100845
Scharping, N. E., Menk, A. V., Whetstone, R. D., Zeng, X., and Delgoffe, G. M. (2017). Efficacy of PD-1 Blockade Is Potentiated by Metformin-Induced Reduction of Tumor Hypoxia. Cancer Immunol. Res. 5, 9–16. doi:10.1158/2326-6066.CIR-16-0103
Scheau, C., Badarau, I. A., Costache, R., Caruntu, C., Mihai, G. L., Didilescu, A. C., et al. (2019). The Role of Matrix Metalloproteinases in the Epithelial-Mesenchymal Transition of Hepatocellular Carcinoma. Anal. Cell. Pathol. 2019, 1–10. doi:10.1155/2019/9423907
Semenza, G. L. (2012). Hypoxia-Inducible Factors in Physiology and Medicine. Cell 148, 399–408. doi:10.1016/j.cell.2012.01.021
Semenza, G. L. (2014). Oxygen Sensing, Hypoxia-Inducible Factors, and Disease Pathophysiology. Annu. Rev. Pathol. Mech. Dis. 9, 47–71. doi:10.1146/annurev-pathol-012513-104720
Semenza, G. L. (2019). Pharmacologic Targeting of Hypoxia-Inducible Factors. Annu. Rev. Pharmacol. Toxicol. 59, 379–403. doi:10.1146/annurev-pharmtox-010818-021637
Shan, Y., You, B., Shi, S., Shi, W., Zhang, Z., Zhang, Q., et al. (2018). Hypoxia-Induced Matrix Metalloproteinase-13 Expression in Exosomes from Nasopharyngeal Carcinoma Enhances Metastases. Cell Death Dis. 9, 382. doi:10.1038/s41419-018-0425-0
Shobaki, N., Sato, Y., Suzuki, Y., Okabe, N., and Harashima, H. (2020). Manipulating the Function of Tumor-Associated Macrophages by siRNA-Loaded Lipid Nanoparticles for Cancer Immunotherapy. J. Control. Release 325, 235–248. doi:10.1016/j.jconrel.2020.07.001
Song, X., Feng, L., Liang, C., Yang, K., and Liu, Z. (2016). Ultrasound Triggered Tumor Oxygenation with Oxygen-Shuttle Nanoperfluorocarbon to Overcome Hypoxia-Associated Resistance in Cancer Therapies. Nano Lett. 16, 6145–6153. doi:10.1021/acs.nanolett.6b02365
Song, Z., Liu, T., Chen, J., Ge, C., Zhao, F., Zhu, M., et al. (2019). HIF-1α-induced RIT1 Promotes Liver Cancer Growth and Metastasis and its Deficiency Increases Sensitivity to Sorafenib. Cancer Lett. 460, 96–107. doi:10.1016/j.canlet.2019.06.016
Sun, S., Xu, Y., Fu, P., Chen, M., Sun, S., Zhao, R., et al. (2018). Ultrasound-targeted Photodynamic and Gene Dual Therapy for Effectively Inhibiting Triple Negative Breast Cancer by Cationic Porphyrin Lipid Microbubbles Loaded with HIF1α-siRNA. Nanoscale 10, 19945–19956. doi:10.1039/c8nr03074j
Sun, X., Zhou, Z., Zhang, Y., Wang, J., Zhao, X., Jin, L., et al. (2021). Identification and Validation of a Hypoxia-Related Prognostic and Immune Microenvironment Signature in Bladder Cancer. Cancer Cell Int. 21, 251. doi:10.1186/s12935-021-01954-4
Sun, Y., Zhao, D., Wang, G., Wang, Y., Cao, L., Sun, J., et al. (2020). Recent Progress of Hypoxia-Modulated Multifunctional Nanomedicines to Enhance Photodynamic Therapy: Opportunities, Challenges, and Future Development. Acta Pharm. Sin. B 10, 1382–1396. doi:10.1016/j.apsb.2020.01.004
Talks, K. L., Turley, H., Gatter, K. C., Maxwell, P. H., Pugh, C. W., Ratcliffe, P. J., et al. (2000). The Expression and Distribution of the Hypoxia-Inducible Factors HIF-1α and HIF-2α in Normal Human Tissues, Cancers, and Tumor-Associated Macrophages. Am. J. Pathology 157, 411–421. doi:10.1016/s0002-9440(10)64554-3
Tao, J., Yang, G., Zhou, W., Qiu, J., Chen, G., Luo, W., et al. (2021). Targeting Hypoxic Tumor Microenvironment in Pancreatic Cancer. J. Hematol. Oncol. 14, 14. doi:10.1186/s13045-020-01030-w
Wang, H., Chen, Y., Shang, J., Wang, H., Pan, M., Liu, X., et al. (2020). Multifunctional Hypoxia-Involved Gene Silencing Nanoplatform for Sensitizing Photochemotherapy. ACS Appl. Mat. Interfaces 12, 34588–34598. doi:10.1021/acsami.0c08315
Wang, X.-L., Xu, R., Wu, X., Gillespie, D., Jensen, R., and Lu, Z.-R. (2009). Targeted Systemic Delivery of a Therapeutic siRNA with a Multifunctional Carrier Controls Tumor Proliferation in Mice. Mol. Pharm. 6, 738–746. doi:10.1021/mp800192d
Wang, X., Liang, X., Liang, H., and Wang, B. (2018). SENP1/HIF‐1α Feedback Loop Modulates Hypoxia‐induced Cell Proliferation, Invasion, and EMT in Human Osteosarcoma Cells. J Cell. Biochem. 119, 1819–1826. doi:10.1002/jcb.26342
Wang, Y., Saad, M., Pakunlu, R. I., Khandare, J. J., Garbuzenko, O. B., Vetcher, A. A., et al. (2008). Nonviral Nanoscale-Based Delivery of Antisense Oligonucleotides Targeted to Hypoxia-Inducible Factor 1α Enhances the Efficacy of Chemotherapy in Drug-Resistant Tumor. Clin. Cancer Res. 14, 3607–3616. doi:10.1158/1078-0432.CCR-07-2020
Wigerup, C., Påhlman, S., and Bexell, D. (2016). Therapeutic Targeting of Hypoxia and Hypoxia-Inducible Factors in Cancer. Pharmacol. Ther. 164, 152–169. doi:10.1016/j.pharmthera.2016.04.009
Wozny, A.-S., Gauthier, A., Alphonse, G., Malésys, C., Varoclier, V., Beuve, M., et al. (2021). Involvement of HIF-1α in the Detection, Signaling, and Repair of DNA Double-Strand Breaks after Photon and Carbon-Ion Irradiation. Cancers 13, 3833. doi:10.3390/cancers13153833
Xie, L., Yang, Y., and Shen, J. (2020). Efficient Inhibition of Uveal Melanoma via Ternary siRNA Complexes. Int. J. Pharm. 573, 118894. doi:10.1016/j.ijpharm.2019.118894
Xu, G., Zhang, H., Li, Z., Wu, S., Quan, R., Mao, K., et al. (2020). Effect of HIF-1αsiRNA-Linked AuNRs on Radiotherapy of Nasopharyngeal Carcinoma. Cell Mol. Biol. (Noisy-le-grand) 66, 185–190. doi:10.14715/cmb/2020.66.5.31
Yang, C., Gao, Y., Fan, Y., Cao, L., Li, J., Ge, Y., et al. (2021). Dual-mode Endogenous and Exogenous Sensitization of Tumor Radiotherapy through Antifouling Dendrimer-Entrapped Gold Nanoparticles. Theranostics 11, 1721–1731. doi:10.7150/thno.54930
Yang, F., Guo, Z., Shi, L., Li, Z., Zhang, J., Chai, C., et al. (2020). Antiangiogenic and Antitumor Therapy for Retinoblastoma with Hypoxia-Inducible Factor-1α siRNA and Celastrol Co-delivery Nanomicelles. J. Biomed. Nanotechnol. 16, 1471–1481. doi:10.1166/jbn.2020.2983
Yang, M., Li, J., Gu, P., and Fan, X. (2021). The Application of Nanoparticles in Cancer Immunotherapy: Targeting Tumor Microenvironment. Bioact. Mater. 6, 1973–1987. doi:10.1016/j.bioactmat.2020.12.010
Yang, R., Mu, W.-Y., Chen, Q.-Y., Wang, Q., and Gao, J. (2018). Smart Magnetic Nanoaptamer: Construction, Subcellular Distribution, and Silencing HIF for Cancer Gene Therapy. ACS Biomater. Sci. Eng. 4, 2606–2613. doi:10.1021/acsbiomaterials.8b00204
Yang, W., Sun, T., Cao, J., and Fan, S. (2011). Hypoxia-Inducible Factor-1α Downregulation by Small Interfering RNA Inhibits Proliferation, Induces Apoptosis, and Enhances Radiosensitivity in Chemical Hypoxic Human Hepatoma SMMC-7721 Cells. Cancer Biotherapy Radiopharm. 26, 565–571. doi:10.1089/cbr.2011.0955
Yao, M., Sattler, T., Rabbani, Z. N., Pulliam, T., Walker, G., and Gamcsik, M. P. (2018). Mixing and Delivery of Multiple Controlled Oxygen Environments to a Single Multiwell Culture Plate. Am. J. Physiology-Cell Physiology 315, C766–C775. doi:10.1152/ajpcell.00276.2018
Yao, Y., Feng, L., Wang, Z., Chen, H., and Tan, N. (2020). Programmed Delivery of Cyclopeptide RA-V and Antisense Oligonucleotides for Combination Therapy on Hypoxic Tumors and for Therapeutic Self-Monitoring. Biomater. Sci. 8, 256–265. doi:10.1039/c9bm00905a
Yong, Y., Zhang, C., Gu, Z., Du, J., Guo, Z., Dong, X., et al. (2017). Polyoxometalate-Based Radiosensitization Platform for Treating Hypoxic Tumors by Attenuating Radioresistance and Enhancing Radiation Response. Acs Nano 11, 7164–7176. doi:10.1021/acsnano.7b03037
Yuan, C.-S., Deng, Z.-W., Qin, D., Mu, Y.-Z., Chen, X.-G., and Liu, Y. (2021). Hypoxia-modulatory Nanomaterials to Relieve Tumor Hypoxic Microenvironment and Enhance Immunotherapy: Where Do We Stand? Acta Biomater. 125, 1–28. doi:10.1016/j.actbio.2021.02.030
Zeng, Z., Lu, Q., Liu, Y., Zhao, J., Zhang, Q., Hu, L., et al. (2021). Effect of the Hypoxia Inducible Factor on Sorafenib Resistance of Hepatocellular Carcinoma. Front. Oncol. 11, 641522. doi:10.3389/fonc.2021.641522
Zepeda, A. B., Pessoa, A., Castillo, R. L., Figueroa, C. A., Pulgar, V. M., and Farías, J. G. (2013). Cellular and Molecular Mechanisms in the Hypoxic Tissue: Role of HIF‐1 and ROS. Cell biochem. Funct. 31, 451–459. Epub 2013 Jun 13. doi:10.1002/cbf.2985
Zhang, L., Chen, C., Duanmu, J., Wu, Y., Tao, J., Yang, A., et al. (2018). Cryptotanshinone Inhibits the Growth and Invasion of Colon Cancer by Suppressing Inflammation and Tumor Angiogenesis through Modulating MMP/TIMP System, PI3K/Akt/mTOR Signaling and HIF-1α Nuclear Translocation. Int. Immunopharmacol. 65, 429–437. doi:10.1016/j.intimp.2018.10.035
Zhang, P., Yao, Q., Lu, L., Li, Y., Chen, P.-J., and Duan, C. (2014). Hypoxia-Inducible Factor 3 Is an Oxygen-dependent Transcription Activator and Regulates a Distinct Transcriptional Response to Hypoxia. Cell Rep. 6, 1110–1121. doi:10.1016/j.celrep.2014.02.011
Zhang, X., Tan, X., Zhang, D., Liao, N., Zheng, Y., Zheng, A., et al. (2017). A Cancer Cell Specific Targeting Nanocomplex for Combination of mRNA-Responsive Photodynamic and Chemo-Therapy. Chem. Commun. 53, 9979–9982. doi:10.1039/c7cc05295b
Zhao, D., Zhai, B., He, C., Tan, G., Jiang, X., Pan, S., et al. (2014). Upregulation of HIF-2α Induced by Sorafenib Contributes to the Resistance by Activating the TGF-α/EGFR Pathway in Hepatocellular Carcinoma Cells. Cell. SignallingSignal 26, 1030–1039. doi:10.1016/j.cellsig10.1016/j.cellsig.2014.01.026
Zhao, X., Li, F., Li, Y., Wang, H., Ren, H., Chen, J., et al. (2015). Co-delivery of HIF1α siRNA and Gemcitabine via Biocompatible Lipid-Polymer Hybrid Nanoparticles for Effective Treatment of Pancreatic Cancer. Biomaterials 46, 13–25. doi:10.1016/j.biomaterials.2014.12.028
Zheng, N., Luo, X., Zhang, Z., Wang, A., and Song, W. (2021). Cationic Polyporphyrins as siRNA Delivery Vectors for Photodynamic and Gene Synergistic Anticancer Therapy. ACS Appl. Mat. Interfaces 13, 27513–27521. doi:10.1021/acsami.1c07662
Zheng, X., Ho, C. Q. W., Zheng, X., Lee, K. L., Gradin, K., Pereira, T. S., et al. (2018). Co-immunoprecipitation Assay Using Endogenous Nuclear Proteins from Cells Cultured under Hypoxic Conditions. JoVE 2, 57836. doi:10.3791/57836
Keywords: HIF-1 alpha, siRNA, antisense oligonucleotide, cancer, nanoparticles
Citation: Yan Y, Li H, Yao H and Cheng X (2022) Nanodelivery Systems Delivering Hypoxia-Inducible Factor-1 Alpha Short Interfering RNA and Antisense Oligonucleotide for Cancer Treatment. Front. Nanotechnol. 4:932976. doi: 10.3389/fnano.2022.932976
Received: 30 April 2022; Accepted: 01 June 2022;
Published: 19 July 2022.
Edited by:
Zhi Ping (Gordon) Xu, The University of Queensland, AustraliaReviewed by:
Hao Song, University of Queensland, AustraliaYannan Yang, The University of Queensland, Australia
Copyright © 2022 Yan, Li, Yao and Cheng. This is an open-access article distributed under the terms of the Creative Commons Attribution License (CC BY). The use, distribution or reproduction in other forums is permitted, provided the original author(s) and the copyright owner(s) are credited and that the original publication in this journal is cited, in accordance with accepted academic practice. No use, distribution or reproduction is permitted which does not comply with these terms.
*Correspondence: Hongping Yao, eWFvaHAyMDA1QHhqdHVmaC5lZHUuY24=; Xiaoliang Cheng, Y2hlbmd4aWFvbGlhbmdAbWFpbC54anR1LmVkdS5jbg==