- Cancer Biology, CSIR-Centre for Cellular and Molecular Biology (CCMB), Hyderabad, India
Over the past two decades, unique and comprehensive cancer treatment has ushered new hope in the holistic management of the disease. Cancer immunotherapy, which harnesses the immune system of the patient to attack the cancer cells in a targeted manner, scores over others by being less debilitating compared to the existing treatment strategies. Significant advancements in the knowledge of immune surveillance in the last few decades have led to the development of several types of immune therapy like monoclonal antibodies, cancer vaccines, immune checkpoint inhibitors, T-cell transfer therapy or adoptive cell therapy (ACT) and immune system modulators. Intensive research has established cancer immunotherapy to be a safe and effective method for improving survival and the quality of a patient’s life. However, numerous issues with respect to site-specific delivery, resistance to immunotherapy, and escape of cancer cells from immune responses, need to be addressed for expanding and utilizing this therapy as a regular mode in the clinical treatment. Development in the field of nanotechnology has augmented the therapeutic efficiency of treatment modalities of immunotherapy. Nanocarriers could be used as vehicles because of their advantages such as increased surface areas, targeted delivery, controlled surface and release chemistry, enhanced permeation and retention effect, etc. They could enhance the function of immune cells by incorporating immunomodulatory agents that influence the tumor microenvironment, thus enabling antitumor immunity. Robust validation of the combined effect of nanotechnology and immunotherapy techniques in the clinics has paved the way for a better treatment option for cancer than the already existing procedures such as chemotherapy and radiotherapy. In this review, we discuss the current applications of nanoparticles in the development of ‘smart’ cancer immunotherapeutic agents like ACT, cancer vaccines, monoclonal antibodies, their site-specific delivery, and modulation of other endogenous immune cells. We also highlight the immense possibilities of using nanotechnology to accomplish leveraging the coordinated and adaptive immune system of a patient to tackle the complexity of treating unique disease conditions and provide future prospects in the field of cancer immunotherapy.
1 Introduction
The very idea of harnessing the immune system to develop efficacious treatment and prevention strategies has long been adopted. Although chemotherapeutic and radiation-based therapies have significant tumoricidal properties, the fact that they end up disturbing the healthy cells makes these techniques widely unacceptable. Considered to be a medical breakthrough, immunotherapy technique of exploiting immune cells to fight and even kill cancer cells, known as cancer immunotherapy, has completely transformed the field of oncology. Compared to traditional therapies, immunotherapy in combination with other targeted therapies could bring about a more specific response that lasts longer in targeted cancer cells. While active immunotherapy depends on the production of a tumor-specific immune response by the host’s immune system, passive immunotherapy is basically dependent on the administration of large quantities of cancer antigen-specific antibodies. Different approaches in cancer immunotherapies like cancer vaccines, oncolytic viral therapy (OVT), chimeric antigen receptor (CAR) T-cell therapy, and immune checkpoint blockade (ICB) therapy have been developed that have demonstrated encouraging clinical responses. ICBs developed using antibodies against cytotoxic T-lymphocyte antigen 4 (CTLA-4), programmed cell death-1 (PD-1), and programmed cell death ligand-1 (PD-L1) have been approved for various cancers like melanoma, non–small cell lung cancer, renal cancer, bladder cancer, head and neck cancer, and Hodgkin’s lymphoma (Tsai et al., 2014; Rotte, 2019). CAR T-cell therapy, on the other hand, combined with co-stimulatory molecules and signaling chains of the T-cell receptor (TCR) after modification of its antigen-targeting regions has achieved significant progress in clinics, particularly in the treatment of non-Hodgkin lymphoma and B-cell acute lymphoblastic leukemia (Vitale and Strati, 2020; Sheykhhasan et al., 2022). Another promising method involves regulating dendritic cells (DCs) using cancer vaccines. DCs are loaded with tumor-associated antigens (TAAs) and presented to cytotoxic CD8+ T lymphocytes in situ, leading to their proliferation. These cells then differentiate into specific T cell subsets that have the capability to kill cancer cells (Timmerman and Levy, 1999).
Usually, it is the therapeutic index that compares the amount of a drug that produces the therapeutic effect with that of the amount that causes toxicity, which in turn determines the response rate of any therapeutic technique. Oncologists usually assess the tumor regression with respect to its size or volume and that these might occur quite early with respect to chemotherapy and radiotherapy. However, that does not reflect on the overall survival rate of the cancer patients due to acquired resistance, etc. On the contrary, immunotherapy effects may not be perceived as a reduction in the tumor volume, but exhibit prolonged tumor control leading to a better survival rate in the long run. The fact that most of the tumors exhibit adaptive and acquired mechanisms of resistance, keeps these objective responses to therapies reasonably low. Also, immunotherapy is not well pronounced in the case of solid tumors when compared to lymphomas due to the interference of the immunosuppressive tumor microenvironment (TME) as well as the presence of abnormal extracellular matrix (ECM) surrounding cancer cells (Marvel and Gabrilovich, 2015; Munn and Bronte, 2016). Like any other cancer treatment technique, cancer immunotherapy also creates outright changes to the patient’s body in the form of side effects. Sometimes these side effects can induce destructive autoimmunity (Phan et al., 2003). It is predicted that the patient outcomes can be changed by focusing on breaking local immune tolerance without disturbing systemic immune tolerance, making it imperative to improve the chances of cancer antigens reaching the immune cells rather than other cells. The use of combination therapies has traditionally been in use in the field of oncology. In this era of molecular targeted therapies, the development of combinatorial therapies (Kourani et al., 2022; Kumar et al., 2022) is proving to be beneficial with respect to both responses to therapy and combating the drug resistance. Under such circumstances, where immunodrugs are combined with molecular or chemo drugs, it is important to assess the possible interactions between them. Combinatorial therapies along with molecular targeting are the ideal way to ensure that responses are converted towards translational benefits.
The advancements in the area of nanotechnology and bioengineering have proffered new approaches that can drastically enhance the targeting and efficacy of cancer immunotherapy. Nanoparticles (NPs) are basically materials in nanoscale sizes that are usually polymeric, liposomal, or metallic in nature (Min et al., 2015). An ideal NP for therapeutic application should be less toxic, biodegradable, highly specific, cost-effective, and can deliver the cancer antigens to target sites through either passive, active, or physical targeting. Passive targeting enables selective targeting of cancer cells through the enhanced permeation and retention (EPR) effect, while active targeting couples the NPs with ligands that can get attached to overexpressed entities on the tumor cells. On the other hand, physical targeting employs optical, thermal, and magnetic properties of NPs to guide them in targeting specific cells (Bertrand et al., 2014; Selvaraja and Gudipudi, 2020). The use of NPs in cancer immunotherapy has widened the therapeutic window and has led to effective drug delivery. The abnormality of the cancer vasculature and ineffective clearance of lymphatic vessels enable the accumulation of NPs within the tumor tissues helping the drug accumulate to the desired pharmaceutical levels; at the same time, the size of the NP enables the needed renal clearance. Moreover, the delivery of sensitive antigens or proteins using NPs protects their enzymatic degradation or inactivation in a complex physiological environment. The ability to modify its own surface is another prominent characteristic feature of NPs that are taken into consideration while targeting cancer cells. This review narrates the current trends in the field of nanotechnology-based cancer immunotherapy and comprehensively evaluates the need to improve the existing methods for the betterment of patient outcomes.
2 Immunotherapy: The Need of the Hour in Cancer Treatment
The ineludible role of the immune system in the control of neoplastic growth and development has been known to humankind for centuries. Multiple accounts have been documented from ancient Egypt to the early 18th century about the regression of tumors after an infection or high febrile episode. However, researchers started attempting to modulate the immune system to treat cancer only in the second half of the 18th century after the histological validation of malignancies (Oiseth and Aziz, 2017). The first successful attempt to harness the power of the immune system was carried out by an orthopedic surgeon named William B Coley. His observation that some patients with major post-operative wound infections underwent spontaneous regression of their unresected tumors compelled him to inject them with mixtures of live and inactivated bacteria such as Streptococcus pyogenes and Serratia marcescens intending to create sepsis and thus antitumor responses. This is considered the first documented active cancer immunotherapy intervention, and the bacterial suspension was termed Coley’s toxins (McCarthy, 2006). Nevertheless, how this sepsis was mediated remained a mystery until the discovery of the cytokine family of proteins like Interleukins (ILs), interferons (IFNs), and chemokines that were utilized to induce clinical remission. Injection of IL-2 in renal cell carcinoma models induced remission of cancer, but the same done for stages III and IV of melanoma depicted debatable responses (Di Trolio et al., 2015). Most of the time, adverse effects due to unpredictable immune responses were observed in patients who underwent these therapies, suggesting that only a few of them could benefit from them.
The fact that cancer cells are particularly efficient in suppressing immune surveillance was first put forward as a hypothesis by Burnet and Thomas (Thomas, 1959; Burnet, 1970). This theory is now the basis of a technique known as cancer immunoediting, wherein the body’s surveillance system detects the immunogenicity of tumor cells that escaped the initial elimination. The cells that elude elimination usually express fewer antigens on their surfaces or lose their MHC class I expression (Tsukahara et al., 2006). The T-cell escape occurs by expressing immune checkpoint molecules like CTLA-4 and PD-1 on their surfaces like normal cells. Studies that gave a better understanding of this immune surveillance system created a revolutionary wave in the developing era of cancer immunotherapy. This has even led to a Nobel prize in physiology and medicine for Drs. Allison and Honjo in 2018. Blocking the T- cell receptors was found to enhance autoimmunity and thus immune responses against tumor cells. Similarly, various biological modifiers were used to develop numerous treatment techniques like OVT, adoptive therapy, cancer vaccines, and ICB-based drugs to target the immune system in cancer patients. Several combinations of these therapies have been experimented with and underwent clinical trials both pre-clinically and clinically, which we will be discussing further in this review.
2.1 Cancer Vaccines
The successful use of preventative vaccines against viruses like hepatitis B and human papillomavirus, which can lead to cancer-causing infectious diseases, prompted the development of cancer vaccination as a key approach to stimulate host T cells. This was further underpinned by the knowledge that CD8+ and CD4+ T-cells, capable of identifying tumor-expressed antigens, may be present in cancer patients (Pardoll and Topalian, 1998; Mellman et al., 2011). Basically, cancer vaccination involves exogenous administration of selected tumor antigens combined with adjuvants that has the ability to activate DCs (dendritic cells) or even DCs themselves. DCs being the most effective antigen-presenting cell (APC) and its important role in coordinating innate and adaptive immune cells make them a reliable target (Palucka and Banchereau, 2012). Thus, a successful administration of cancer vaccine involves delivering large amounts of a high-quality antigen to DCs, leading to its activation. This further leads to the induction of sustained cytotoxic T lymphocyte (CTL) and CD4+ T helper cell responses, thus maintaining the robustness of the obtained responses (Saxena et al., 2021). Some DC-based vaccines are created by isolating DCs from the patient’s peripheral blood mononuclear cells (PBMC) and loading it with tumor antigens ex vivo, and then by reinfusing them into the patient after activation. Patients with advanced cancers presented encouraging responses after administration of the same. Another vaccine that has caught attention recently was the in-situ vaccines (ISV). Traditional vaccines are generated by choosing particular antigens and administering them to patients; however, in situ approaches utilize antigens obtained from dead tumor cells present within the TME. These vaccines are currently increasing the scope of techniques that modulate immune responses to destroy tumors and are now evolving as approaches to improve survival and response rates (Saxena et al., 2021).
However, there are numerous obstacles on the path of developing cancer vaccines as a single agent against cancer. One of the major hurdles is the lack of specificity in the tumor antigen chosen to be used as a vaccine. An ideal tumor antigen is the one that is overexpressed in tumor cells and not in healthy cells. Not many antigens fulfill this criterion and thus do not ensure the activation of protective T cell response (Fenoglio et al., 2013). Moreover, the heterogeneous nature of most of the tumors suggests that they have varied mutational status and antigen expression. Though this problem was addressed by using bioinformatics along with mass spectroscopy of peptides eluted from MHC-I molecules, the therapeutic technique was unable to elicit required T-cell responses (Abelin et al., 2017).
2.2 Oncolytic Viral Therapy
Oncolytic viral therapy (OVT), which is active at the intermediate level of biological treatment and immunotherapy, has recently emerged as a novel approach to cure cancer. This cancer therapy makes use of native and engineered viruses which will replicate only within the tumor cells and kill them (Kaufman et al., 2015). Major features required by these viruses to be considered for OVT are that they must be non-pathogenic and should have the capacity to be transformed to express tumor-killing factors through genetic engineering methods (Maroun et al., 2017). RIGVIR, Oncorine, and Talimogene laherparepvec (T-VEC) are three of the oncolytic viruses currently approved for use in a clinical setting and have shown satisfactory therapeutic potential. Other than these, herpesvirus, adenovirus, and vaccinia virus are currently under preclinical studies and are showing promising results. In spite of the approval all around the world for OVT, there are only a few publications on the use of RIGVIR for cancer therapy. It is usually more sensitive while treating low-grade melanomas that have undergone surgical resection rather than high grade (Babiker et al., 2017). Oncorine, on the other hand, is the only adenovirus that has been approved for use as a cancer treatment option. These adenoviral vectors, though in clinical trials, often are accustomed to seroprevalence, limiting the ability of the same to be delivered intravenously to treat highly metastatic diseases (Nwanegbo et al., 2004). T-VEC has shown efficacy as a single agent and is hence approved for the treatment of cutaneous high-grade melanoma lesions by intratumoral injection. Single-agent efficacy was also being applied for evaluating patients with liver, pancreatic, and advanced nervous system solid tumors, as well as checking the safety and efficacy of T-VEC alone or in combination with checkpoint inhibitors, chemotherapy, or radiation therapy in melanoma. This was approved by US FDA in 2015 for the treatment of non-resectable metastatic melanoma and in Europe for locally advanced or metastatic cutaneous melanomas (Cao et al., 2020). Although promising, several limitations associated with OVT exist. Immuno-compromised patients are not usually good candidates for oncolytic virus-based treatments, since antitumor immunity could be compromised in these patients. There are limitations regarding the levels of efficiency observed in patients with more advanced stages of the disease while undergoing OVT. Also, OVT is observed to be more effective if combined with other cancer immune-therapeutics.
2.3 Adoptive Cell Therapy
Adoptive cell therapy (ACT) is a type of immunotherapy where the endogenous T cells from the patients are extracted and made to undergo expansion in vitro after which it is reinfused into the body. It basically exploits the antitumor properties of lymphocytes to eradicate primary and metastatic tumor cells (Shi et al., 2015). This strategy has the power to enable the body to tolerate tumor antigens and produce high avidity effector T cells. This ability of ACT has thus made it a viable therapeutic option with a high potential to induce dramatic clinical responses. Currently, ACT can be classified into three different types with each of their own mechanism of action, namely ACT with tumor-infiltrating lymphocytes (TILs), ACT using TCR (T-cell receptor) gene therapy, and ACT with chimeric antigen receptor (CAR) modified T cells (June et al., 2015). ACT with TILs is done by harvesting and multiplying CD8+ and CD4+ T cells grown from resected metastatic tumor deposits ex vivo prior to transfer. In TCR gene therapy, tumor antigen recognition is achieved by introducing a novel TCR into T cells leading to easy detection of the antigens (Sterner and Sterner, 2021). On the other hand, CARs used mainly to fight against hematological malignancies are engineered synthetic receptors which are introduced to T cells to recognize and eliminate cells expressing a specific target antigen. These receptors are usually independent of being recognized by the MHC molecules thereby causing significant antitumor responses due to high T cell activation (Rohaan et al., 2019).
Clinical trials done on 93 patients with metastatic melanoma showed that infusion of autologous TILs in conjunction with IL-2 administration resulted in an increase in the objective response rate to 72% and a complete regression of the tumor in 22% of the subjects (Rosenberg et al., 2011). High response rates and long-lasting tumor regression were also observed during large-scale experiments done at MD Anderson Cancer Centre and the Sheba Medical Centre (Yee, 2013). Advances in T cell culturing methods and genetic T cell engineering techniques ensure the production and timely delivery of antigen-specific T cells, further ensuring the development of these therapies to battle malignancies. Still, there exist numerous challenges that prevent ACT from becoming the ultimate personalized cellular product solely reactive to the tumor. In TCR therapy, it is mandatory that the targetable antigens are present only on the tumor, and not on the healthy tissues. This can decrease the off-tumor toxicity and enables the effectiveness of the therapy. Targeted cell trafficking is another hurdle in the path of these therapies, especially for CAR T-cell therapy where the major challenges include new target discovery, reduction of toxicity, and improvement of cell trafficking. However, significant improvements have already been seen with the use of TIL treatment in melanoma and CAR T-cell therapy in hematologic malignancies. Further, optimization of this promising therapeutic modality will ensure a fair fight against cancer with the requisite antitumor effect and reduced toxicity (Rohaan et al., 2019).
2.4 Immune Checkpoint Blockade
Significant success in the treatment of a wide variety of malignancies has been demonstrated with the therapies directed against negative immunologic regulators (checkpoints). This method, termed immune checkpoint blockade therapy targets the immune inhibitory pathway activated by the cancer cells and has now become a well-known approach to ensure an antitumor response. Cytotoxic T-lymphocyte antigen 4 (CTLA-4) was the first immune checkpoint receptor to be targeted. CTLA-4 is upregulated in activated T cells and acts via a negative feedback mechanism to control T cell function (Linsley et al., 1991). Two antibodies targeting CTLA-4 were used, namely, ipilimumab and tremelimumab that entered clinical trials and showed durable clinical responses in patients. Advanced melanoma patients undergoing phase III clinical trials have also responded to ipilimumab through enhanced survival rate. Another receptor, PD-1 (programmed cell death-1) interacts with its ligand (PD-L1 and PD-L2) and negatively regulates T cell function. Antibody-based drugs such as Nivolumab, Pembrolizumab, and Pidilizumab can target PD-1 whereas Atezolizumab, Durvalumab, BMS-936559, and Avelumab target PD-L1. Since PD-1 is expressed on various other immunological cells like B-cells and natural killer (NK) cells, the usage of drugs that target PD-1 can have an unwanted effect on the function of these cells (Postow et al., 2015).
In spite of the significant antitumor benefits induced by the use of ICB, the fact that it does not just enhance tumor-specific immune responses leads to various nonspecific immunologic activation and ultimately, adverse effects. To overcome these, various combination therapies have been proposed that combine CTLA-4 and PD-1 blockers with anticancer agents such as chemotherapy, targeted therapy, radiotherapy, and other immunotherapy. With proper dosage and schedule of drug delivery, the combination of chemotherapy with ipilimumab was generally safe during clinical studies on non–small-cell lung cancer and melanoma patients (Lynch et al., 2012; Weber et al., 2013). Preclinical studies that depict the possibility of enhancing the CTLA-4 and PD-1 blockade efficacy by combining it with radiotherapy have led to prospective trials (Demaria et al., 2005). Other than these, the combination of CTLA-4 along with PD-1 has shown promising results. A particular phase I study of ipilimumab and nivolumab in patients with melanoma resulted in a high durable response rate and impressive overall survival compared to already existing data (Wolchok et al., 2013). Despite the promise of this approach of using ICB, many hindrances such as the immune-related adverse events and the problem of arriving at the best combination approach to determine whether they increase the efficacy of the technique, need to be considered to convert this into a propitious immunotherapy model.
3 Widening the Window of Cancer Immunotherapy Using Nanoparticles
Nanomedicine, where nanoparticles (NPs) are used as drug delivery vehicles, is a decade-old concept that has gained immense interest and has been developing rapidly in recent years. Due to their wide range of biological properties and composition, NPs have been extensively studied in the use of drug delivery, where the substances in the nanoscale range were employed to transport bioactive drug or therapeutic agents towards a major intracellular target site in a regulated way (Swaminathan et al., 2021). Being sub-micron in size, NPs have the advantage that they can penetrate into the targeted epithelial lining or tissue, subsequently penetrating the cell in order to achieve desired therapeutic effects. Understanding the interplay between the nanobody and surface receptors of target cells, therapeutic agent’s stability and release, cell environment, etc. are some of the key factors involved in the development of an efficient drug delivery mechanism (Vinogradov et al., 2002; Suri et al., 2007). Chemotherapy, the mainstream treatment regime for cancer, has many disadvantages including its non-specificity. Even though many biological drugs have been discovered, targeted delivery is a major issue scientist are facing globally. Therefore, as a consequence of these shortcomings in the current cancer treatments, researchers have devised techniques with more precise tumor site targeting with fewer side effects. Different chemotherapeutic, immunotherapeutic and biological agents were conjugated with different nano-vehicular coatings to treat diseases, especially cancer (Patra et al., 2018). Several studies have shown that chronic human diseases could be treated by nanotechnology-mediated therapy due to their multiple actions, including target-specific delivery of therapeutic drugs.
On the other hand, immunotherapy is considered one of the most successful cancer treatment options, providing a precise target to both the immune system and the tumor environment (Farkona et al., 2016; Kokate, 2017). Multiple studies on active biomaterials have been conducted that specifically target immune reactions and immune cells (Sun, 2017; McCune, 2018). The use of nanotechnology as a combination of biomaterials and drugs has yielded many intriguing performances in terms of effective therapies (Chen and Mellman, 2013; Martin-Liberal et al., 2017; Oiseth and Aziz, 2017; Yu and Cui, 2018). Currently, PLGA (polylactic-co-glycolic acid) NPs, liposomes, dendrimers, and gold NPs (AuNPs) are broadly studied and used in cancer immunotherapy. According to the substrate component, NP-based platforms for cancer immunotherapy may be split into two groups—naturally derived NPs and synthetic NPs. Exosomes and lipid-based NPs are examples of naturally derived NPs that include biological components or cell-derived vesicles, while inorganic NPs and polymeric NPs come under synthetic NPs. In this section, we will deal with each of these classes of NPs with examples and the advancements witnessed in recent times (Figure 1).
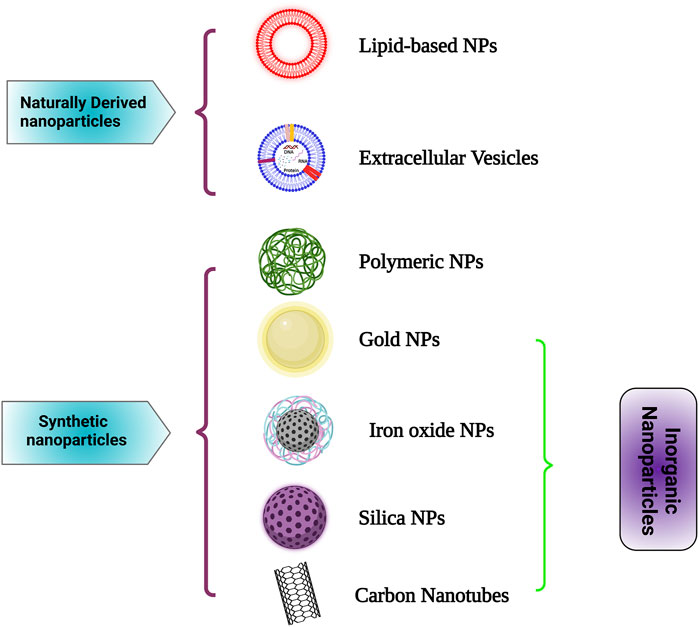
FIGURE 1. Different nanocarriers used in cancer immunotherapy such as Lipid-based NPs, Extracellular Vesicles, Polymeric NPs, Gold NPs, Iron oxide NPs, Silica NPs and Carbon Nanotubes for cancer immunotherapy are depicted here. Created with Biorender.com.
3.1 Naturally Derived Nanoparticles for Cancer Immunotherapy
3.1.1 Lipid-Based Nanoparticles
Lipids are micro-biomolecules that are known to be the basic building blocks of biological membranes, made up of fatty acids (Ibarguren et al., 2014). Lipid-based NPs (LNPs), particularly liposomal NPs and solid lipid NPs, have been widely employed in FDA-approved clinical applications such as cancer biomarker diagnostics and relevant therapeutics. LNPs are being studied widely as immunoadjuvants and transporters for nucleic acids, antigenic peptides/proteins, and other immunostimulating compounds used in cancer immunotherapeutics. LNP systems are considered to have low toxicity, specific cellular uptakes, and high loading capacity as immuno-modulating agents. Due to their versatile component, biomimetic multilayer structure (mainly liposomes), and flexible functionalization, they act as excellent immunoadjuvants and carriers for immuno-modulating drugs. The use of pH-sensitive liposomes to transport a co-delivery system consisting of ovalbumin (OVA) and IFN-γ -encoding pDNA (plasmid-DNA) to dendritic cells of cancer-bearing mice boosted CTL infiltration and led to substantial anticancer activity (Yuba et al., 2015).
Since nucleic acids and most immuno-stimulating molecules have an intrinsic molecular charge, integrating cationic/ionizable lipids into LNP formulations is thought to be an effective strategy for promoting stable and efficient encapsulation as well as neutralization of negatively charged payloads of interest. These cationic groups have been studied extensively in preclinical and clinical studies of human gene and drug deliveries (Simberg et al., 2004). Yuba et al. reported that pH-sensitive dextran liposomes modified with TGF-β 1 receptors (transforming growth factor) as well as PEG (polyethylene glycol) showed strong immunotherapeutic responses due to CD8+ T-cells arriving at the tumor sites (Yuba et al., 2017a). They also reported that modification of liposomes with pH-sensitive bioactive polysaccharides such as mannan and curdlan, releases their payloads in mildly acidic pH thus delivering the drug into the cytosol of DCs. These liposomes, when compared to liposomes modified with dextran derivatives, elicited higher antigen-specific immune responses and antitumor effects when injected subcutaneously (Yuba et al., 2017b). To overcome the immunosuppressive tumor microenvironment (TME), the efficacy of cancer immunotherapy needs to be boosted. A cytosolic immunological adaptor protein (STING), when administered into TME, was found to show substantial anticancer action. Since STING agonists like cGAMP penetrate the cell membrane poorly due to their anionic properties, cationic liposomes with varied surface polyethylene glycol (PEG) levels could be utilized to encapsulate and promote delivery at the cytosol. Administration of liposomes boosts STING agonist action and creates immunological memory, allowing tumor cells to be challenged again (Koshy et al., 2017).
3.1.2 Extracellular Vesicles
Extracellular vesicles (EVs) are bilayer phospholipid vesicles, secreted from various living cells that differ in size (50–1,000 nm), origin, and content (György et al., 2011). These vesicles can be found in many body fluids (e.g., saliva, urine, serum etc.) and have been extensively researched for their ability to stop the initiation and progression of various diseases (Zhang et al., 2019). EVs are long-distance transportation vehicles that contain specific proteins, peptides and transmembrane markers apart from DNA and RNA (Zhang et al., 2018). EVs of immune cells include distinct proteins and endosome-associated peptides, while those of tumor cells contain specific tumor antigens. EVs have recently been identified as interesting immunotherapeutic platforms due to their endogenous capabilities. EV-based cancer immunotherapy can be classified into two subtypes on the basis of excreting cells. The subtypes include Immune cell-derived EVs (ICEVs) and Tumor-derived EVs (TEVs). In ICEVs, DC-derived exosomes (DEXs) have been shown to express MHC complexes (MHC- I and II), adhesion molecules (integrin and ICAM-1) and T cell co-stimulatory molecules (eg. CD86, CD80). This suppresses tumor cells through T cell activation by stimulating antigen-specific CD4+ and CD8+ immune responses (Wolfers et al., 2001; Li et al., 2015). TEVs are significantly more productive than ICEVs during therapy due to the extrinsic factors (thermal stress and hypoxic conditions) as well as their specific TME (tumor microenvironment) (Eng et al., 2015; Patel and Sant, 2016). Furthermore, the incorporation of cytokines and nucleic acids into EVs resulted in the development of TEV-based vaccines with improved immunogenic activation and efficacy (Koyama et al., 2016). Hadla et al. (2016) employed exosome-laden doxorubicin (exo-DOX) to treat human breast cancer cells in a study to assess cardio-toxicity. Compared to free DOX, they found that exo-DOX exhibited better doxorubicin cytotoxicity and reduced drug build-up inside the heart. Although many studies have been done, more extensive investigations involving biological characteristics and safety analysis are required to determine the therapeutic effect of EVs in cancer immunotherapy.
3.2 Synthetic Nanoparticles for Cancer Immunotherapy
3.2.1 Polymeric Nanoparticles
Polymeric NPs (PNPs) are colloidal macromolecules that act as drug carriers, transporting chemical drugs to malignant areas and ensuring their continuous release (Masood, 2016). A nanosphere or a nanocapsule is formulated where the drug or chemicals of interest are linked to the surface of NPs or encapsulated inside it. The evolution of NPs from non-biodegradable polymers like polyacrylates, polystyrene, polymethyl methacrylate (PMMA), etc. (Shastri, 2003; Vijayan et al., 2013) to biodegradable forms solved the major issues of toxicities, at the same time enhancing the biocompatibility, biodistribution and pharmacokinetic properties of the drug. Biodegradable polymers such as poly (ε- caprolactone) (PCL), polylactic acid (PLA), poly (D, L-lactide-co-glycolic acid) (PLGA) (Elsabahy and Wooley, 2012), and some of the naturally occurring polymers like gelatin, chitosan, albumin and alginate are being widely used now for cancer immunotherapy. Further advancements have brought in modifications in their structures and properties, wherein PNPs could provide a variety of administration modalities, like oral and intravenous routes with different loading capacities (Tangudu et al., 2015). They also contributed to the stability of the drug by reducing the volatilization properties and preventing its degradation. These properties of PNPs were observed by Martín- Saldaña et al. (2017) when they coupled PNPs with cisplatin (e.g., α-tocopheryl succinate or dexamethasone) in chemotherapy and opined that they reduced cisplatin-induced ototoxicity. These PNPs can also serve as adjuvants and delivery vehicles for immunostimulating drugs during the activation of an immune response to suppress tumor development. PNPs are widely acknowledged as adjuvants in cancer immunotherapy due to their exceptionally strong payload capacity, high solubility in water as well as biocompatibility for immune-associated components. However, several PNPs have shown a remarkable trigger of the immune system against tumor cells. Kumar et al. (2017) devised a pathogen-mimicking vaccine delivery system (PMVDS) using PLGA conjugated with the bioactive polymer- inulin acetate (InAc). In these PMVDS systems, enhanced and consistent antigen delivery was found, along with toll-like receptor (TLR) 4 activation in APCs (antigen-presenting cells), resulting in the generation of cell-mediated immunity in vaccinated mice, leading to the prevention of tumorigenesis and delayed tumor growth. PLGA is a class of biodegradable NPs that has low systemic toxicity and great biodegradability compared to other PNPs and is known to be one of the effective immunotherapy components. In cancer immunotherapy-based studies, it was shown that the majority of PLGA NPs were targeted to the dendritic cells (DCs), where DCs take up the PLGA NPs without recognizing the specific character of NPs (Ghotbi, 2011; Colzani et al., 2018). So, for the delivery of therapeutic moieties to DCs, PLGA is preferred over other PNPs and is employed extensively for immunotherapy.
In a comparative in vitro study done with PLGA microparticles v/s nanoparticles to check the efficacy of targeting humanized antibody (hD1) to DCs, NPs showed 10 to 100-fold greater efficacy compared to microparticles (Cruz et al., 2010). Also, the surface of PLGA NPs was modified to enhance the delivery of antigens to DCs. DC immunizations with stronger IL-10 production were achieved by modifying the surface of the NPs with maximal concentrations of monoclonal antibody (mAb) (Bandyopadhyay et al., 2011). In addition to PLGA NP’s mode of delivery and actions, cancer immunotherapy reportedly also utilizes polymeric micelles composed mainly of amphiphilic polymers. Here, the hydrophobic moieties of the amphiphilic polymers created the inner core, and the hydrophilic residues formed the outer shell that could be engineered for functionalities like pH-sensitive responses or cell targeting (Jones and Leroux, 1999). The polymeric micelles were designed exclusively for enveloping hydrophobic adjuvants and antigens, which is a key drawback for this class of molecules as well as PLGA NPs. Since a majority of effective immune-stimulating drugs are protein-based, the development of new nanocarrier formulations is needed for effective and targeted immunotherapy.
3.2.2 Inorganic Nanoparticles
Metallic NPs (MNPs) have fascinated scientists in cancer immunotherapy because of their enormous potential as nanocarriers. MNPs have precise controlled and delivery action, which can be achieved by modifying the charge, size, and surface (Sperling and Parak, 2010; Evans et al., 2018). Various functional groups were synthesized with the NPs, enabling them to be linked with numerous drugs, different ligands and antibodies, thus creating a wide range of applications in cancer diagnosis. MNPs, such as magnetic NPs (Iron oxides), silver and AuNPs (gold NPs), nanocages and nanoshells have been developed and utilized for the past several years for various applications in cancer diagnosis and therapeutics. Various metal NPs have been studied for several decades for their improved therapeutic impact, although few noble metal NPs (Au, Hg, Cu, Ag, and Pt) have attracted researchers’ interest (Ramalingam et al., 2014).
AuNPs are considered to be one of the most stable, non-reactive and efficient NPs among others due to their multiple medical applications, unique functional properties and ease of synthesis. They can be synthesized into a variety of structures and forms like nanowires, nanocages, nanocubes, nanorods, nanobranches, nanopyramids, nanoflowers, nanoshells, etc. The efficacy of these NPs for specific targeting and accumulation in different immune cells can be enhanced by modifying their shape, charge, size, and functional groups. Different molecules such as enzymes, nucleic acids, proteins, antibodies, fluorescent dyes, etc. can easily be conjugated with AuNPs, which can be applied to a wide range of medical applications like PTT (photothermal therapy), PDT (photodynamic therapy), RT (Radiation therapy), CT (computer tomography), X-ray imaging, etc. for efficient diagnosis and therapy.
Iron oxide NPs (IONPs) like other NPs vary from 1 to 100 nm and have mainly two forms, Fe2O3 in an oxidized form, also called maghemite and Fe3O4 is known as magnetite. The superparamagnetic features of these NPs expanded their applications in a variety of fields. As an effective carrier for drug delivery, Zhao et al. (2018) employed superparamagnetic Fe3O4 alone as well as in conjugation with ovalbumin to check the immunological effect in colon adenocarcinoma CT26-tumor-bearing animal model. They reported that the administration of IONPs alone showed a decent cytokine production along with good immune cell activation with slight tumor retardation, while the conjugated form inhibited the tumor significantly. In cancer immunotherapy another working principle of IONPs is the polarization of the immune cells, which leads to stronger immune responses against cancer cells. Zanganeh et al. (2016) reported an increased caspase-3 activity along with polarization of macrophages in a mammary cancer-bearing model when administered with ferumoxytol (FDA- approved) iron supplements. Branched polyethylenimine–super magnetic IONPs have been used to study DC polarization by other groups as well as to check the therapeutic potentials in treating cancers (Hoang et al., 2015).
Carbon nanotubes (CNTs) are allotropes of carbon, mainly graphene, which are elongated and hollow cylindrical open or closed-ended nanostructures with a diameter as low as 1 nm but with a variable length of several micrometers. Based on their structures, these nanotubes were categorized into two types: single-walled nanotubes (SWNTs) and multi-walled nanotubes (MWNTs). Due to their relaxed surface modification and adaptability with physicochemical properties, CNTs have been widely researched as effective drug delivery systems for the intracellular transfer of genes, proteins, and medicines (Ji et al., 2012; Li et al., 2014). Several adjuvants or antigens can be chemically attached to the surface or encapsulated inside the nanotubes’ inner core. Excitingly, cargo-loading CNTs can penetrate APCs quickly via a variety of channels, including energy-dependent or passive paths. This results in increased internalization of CNTs, leading to a greater effective immune response than other NPs. Hassan’s group discovered the combinatorial therapeutic approach by successfully utilizing MWNTs in co-delivering antigens and different types of immunoadjuvants to APCs that triggered antigen-specific T cell response in vitro and thereby tumor regression in vivo (Hassan et al., 2016). CNTs are known to be non-biodegradable NPs that may induce harmful consequences such as interstitial fibrosis, mesothelioma, granuloma and alteration of DNA by releasing reactive oxygen species and oxidative stress. Despite its potential as an effective immunotherapy delivery system, CNTs are limited in clinical use due to safety and biocompatibility concerns compared to other MNPs (Kostarelos, 2008; Almeida et al., 2014).
NPs made of silica have been demonstrated to be excellent drug carriers in cancer immunotherapy due to their biocompatibility, small size, and simplicity of synthesis, which allows modification on the surface. In recent years, mesoporous silica NPs (MSNPs) have been considered to be one of the attractive classes of drug delivery systems due to their unique properties, which can be utilized to treat several diseases including cancer. MSNPs have a large amount of available open pores, in which a considerable amount of active moiety can be enclosed. Silica NPs are a suitable option for the regulated release of medicinal drugs because of their large surface area, pore capacity, and greater stability. MSNPs-based chemotherapy showed a high therapeutic effect when combined with other cancer therapies (Gao et al., 2020). When employed as vaccine carriers, MSNPs can moderate the APC intracellular microenvironment with diverse antigens and significantly enhance immunity and anticancer activity. Kong et al. synthesized a NP for combinational chemo-immunotherapy using LNPs-coated MSNPs encapsulating IL-2, DOX (doxorubicin), and ATRA (all-trans retinoic acid). In vivo administrations of these accumulated in the liver tissue, resulting in the increased levels of IL-12 and IFN-γ, which further increased the activity of NK cells and CD8+ T-cells that eventually inhibited the tumor development (Kong et al., 2017).
4 Application of Nanotechnology in Cancer Immunotherapy
The use of nanotechnology in cancer immunotherapy can create the anticipated robust immune response by targeting not only tumor cells but also lymphocytes and APCs that are under circulation within the patients’ bodies. Basically, it can be used in the delivery of neoantigens, for developing vaccines against cancer, enhancing immunogenicity, modulating TME, improving immune recognition, inhibiting immune checkpoints, in adoptive immunotherapy and establishing efficient image-guided immunotherapy, the details of which are discussed below (Figure 2).
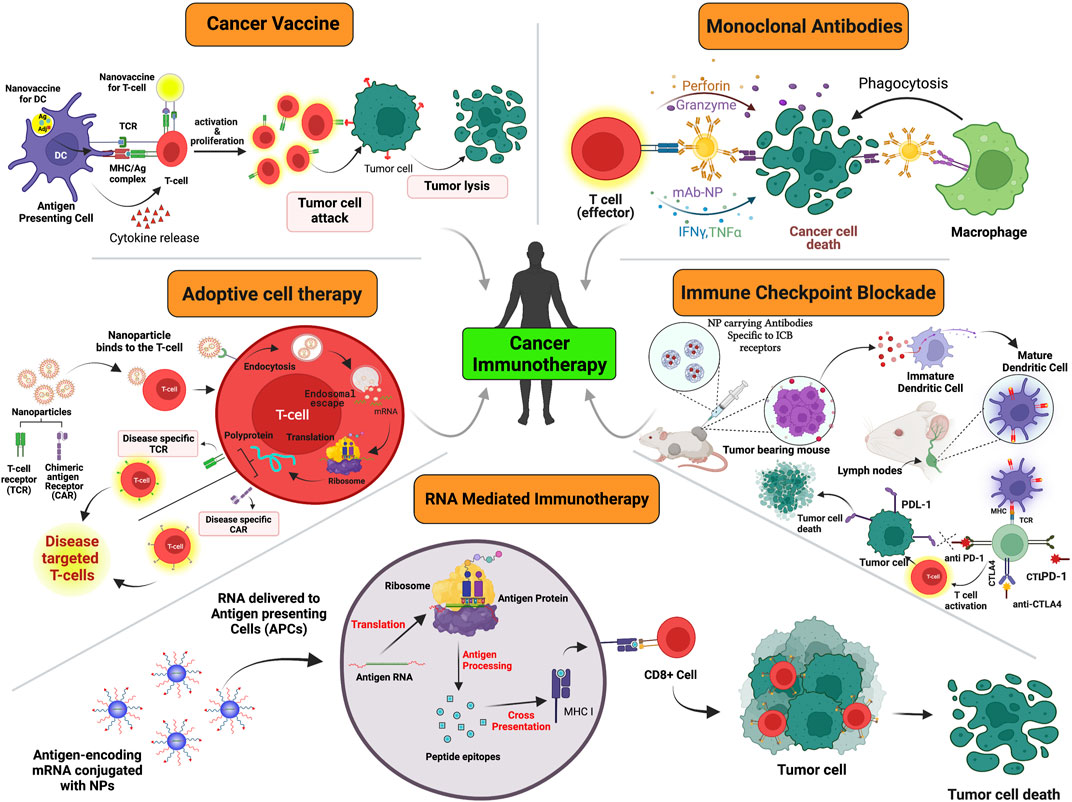
FIGURE 2. A schematic representation of various modules in which cancer immunotherapy plays a role which includes but is not limited to adoptive cell therapy, monoclonal antibodies, cancer vaccines, ICBs, oncolytic viral therapy and RNA- mediated silencing. Created with Biorender.com.
4.1 Nanotechnology Based Vaccines for Cancer Immunotherapy
In cancer immunotherapy, one of the approaches is the delivery of tumor vaccines, which focus on training the immune cells to recognize and boost their responses towards cancer cells, thereby preventing their further growth. Cancer vaccines, in general, target TAAs (tumor- associated antigens), which are expressed at higher levels on tumor cells compared to normal tissues (Ahmad et al., 2020). The primary concept behind this approach is modulation of the immune system for identification and elimination of tumor cells with some associated benefits, such as systemic stimulation of antitumor response. This ensures negligible damage to non-cancerous cells while inducing specific killing of cancer cells, by generating an immunological memory, thus protecting against remission (Krishnamachari et al., 2011). Additionally, DC-based vaccines have been shown to be potential cell-based modulators for treating patients suffering from cancer by delivering tumor-specific antigens (TSAs) to lymphatic organs and boosting cytotoxic T lymphocytes (CTL) mediated immune response. However, there are some drawbacks of tumor vaccines, such as reduced uptake of soluble materials, intrinsic instability of soluble macromolecules, and inadequate cross-presentation to CTLs. Recently vaccine delivery mediated by NPs has addressed these limitations (Park et al., 2013). Many nano-sized pharmaceutical carriers have been successfully created to enhance the efficacy of pharmaceutical drugs (Sakhalkar et al., 2003; Salem et al., 2003; Pearce et al., 2007; Intra and Salem, 2008; Pearce et al., 2008). Additional advantages such as biocompatibility, biodegradability, minimal toxicity and immunogenicity make NPs a promising clinical option in cancer immunotherapy. A promising strategy to generate nano-vaccines is via encapsulating antigens within the NPs. This prevents the degradation of antigens instantaneously upon administration and helps to elicit an enhanced immunological response. Alternatively, the vaccine antigens can be conjugated on the surface of NPs, thereby facilitating immunogen manifestation to immune cells similar to induction of Th1-type immune responses elicited by intracellular pathogens (Park et al., 2013).
Whole-cell cancer vaccines have been reported to be effective in treating cancers. Accumulating evidence show their capability to trigger multivalent immunological reactions in patients through improving recognition of epitopes and subsequently aid in the development of customized immunotherapy. There are two ways to obtain whole-cell antigens, the first being from entire tumor cells with apoptotic characteristics that have been inactivated and another from lysates of tumor cells exhibiting necrotic characteristics (Keenan and Jaffee, 2012). Subunit vaccines also play a key role in patients developing protective immunity by administration of virus particles. Based on a principle similar to the subunit vaccine under a particulate delivery system using PLGA NPs and liposomes, TLR agonists and cancer cell lysates can also be encapsulated in the same (Wang et al., 2012; Gross et al., 2014). Alternatively, a biodegradable PLGA matrix that mimics an infection has been employed for delivering a whole-cell cancer vaccine. Here, tumor lysate acts as a tumor antigen reservoir while granulocyte macrophage-colony stimulating factor (GM-CSF) promotes in situ recruitment of DCs, and CpG motifs stimulate them (Ali et al., 2009). Compared to a traditional whole-cell vaccine GVAX made up of radiated GM-CSF-secreting tumor cells, the new PLGA matrix-based whole-cell cancer vaccine significantly evoked antigen-specific CD8+ T cells as well as enhanced both preventive and therapeutic antitumor activity (Fan and Moon, 2015).
4.2 Nanotechnology Based Adoptive Immunotherapy
Adoptive immunotherapy or ACT, a type of cell-based immunotherapy, aims to destroy cancerous cells by using the inherent anti-tumoral properties of the immune system. This approach has opened up new avenues for cancer treatment. ACT involves the isolation and ex vivo manipulation of autologous or allogeneic lymphocytes, which are stimulated for the expansion of these cells ex vivo, and then re-infused into patients to generate a robust anti-neoplastic response. The application of ACT as a potential treatment option was initially demonstrated in 1953 through experiments on murine models (Mitchison, 1955). The first successful ACT performed in a clinical setting with allogeneic hematopoietic stem cell transplants was in leukemia patients (Weiden et al., 1979). A key approach is to use TILs (tumor-infiltrating lymphocytes) derived from patients’ own tumors (Ngo Trong et al., 2020). Early clinical studies showed that TILs were a viable treatment option to cure patients suffering from metastatic melanoma with an objective response (OR) rate of approximately 50% (Rosenberg et al., 2008). Other approach includes genetically-engineered T cells either with antitumor T-cell receptors (TCRs) or chimeric antigen receptors (CARs) (Rosenberg and Restifo, 2015). Recently, the first CAR T-cell products, Tisagenlecleucel (Kymriah®) (O'Leary et al., 2019) and axicabtagene ciloleucel (Yescarta®) (Bouchkouj et al., 2019), were approved by the US FDA to treat relapsed or refractory large B cell lymphoma. Other than T cells, ACT using natural killer (NK) cells also has excellent potential to treat cancer (Oh et al., 2019). Adoptive transfer of NK cells involves using either allogeneic NK cells activated ex vivo or NK cells expressing CARs (CAR-NK) (Hu et al., 2019). However, there are limitations in effective responses to ACT due to inadequacy in T cell proliferation ex vivo, lack of trafficking of lymphocytes to the tumor site, and decreased persistence of T cell activation due to immunosuppressive TME (Wallace et al., 2008; Mardiana et al., 2019).
Additionally, CAR T-cell therapy can cause severe toxicities, leading to organ damage and serious neurological problems (Brudno and Kochenderfer, 2016). Therefore, the application of NPs in ACT may be a better treatment option. The conventional strategy is to improve the efficacy of ACT via transporting immunosuppression inhibitors using nano-based delivery system to the surfaces of lymphocytes. The administration of TGF-β inhibitor conjugated nanoliposomes, before adoptive therapy, to target T cells has been shown to trigger the proliferation of ACT T-cell ex vivo and elicited enhanced tumor regression when infused back into recipients (Zheng et al., 2017). The use of liposomes conjugated with antibodies and cytokines have also been explored to target specific surface antigens and trigger T- cell activation signals respectively to improve T cell expansion. To activate and monitor adoptively transferred T cells, IL-2-Fc-liposomes and anti-Thy1.1-liposomes were generated that have consecutively been shown to target T cells efficiently in vivo. These NPs enhanced tumor clearance by facilitating sustained pseudo-autocrine activation of ACT T-cells (Stephan et al., 2010; Zheng Y. et al., 2013).
Another strategy to improve in vivo T cell expansion is conjugating drug-loaded nanomaterials (‘backpacks’) to the surfaces of T cells instead of delivering both of them separately. This approach facilitated the contents to be released in a pseudo-autocrine manner, resulting in increased delivery specificity and reduced in vivo toxicity (Berger et al., 2009; Xie et al., 2019). Tang et al. designed the IL-15 Superagonist (IL-15Sa) nanogels backpacked onto CAR T-cells. In melanoma mouse model, this backpack system was injected, where it bound to tumor cells via antigen recognition resulting in the change of redox activity at the surface of CAR T-cell. This led to the cleavage of disulfide bonds, thereby resulting in the release of IL-15Sa, which increased the expansion of T-cells in a TCR activation-responsive way. This approach has led to an improvement in therapeutic efficacy, increased survival, and limited systemic toxicity in vivo compared to systemic administration of free cytokines (Berger et al., 2009; Robinson and Schluns, 2017; Tang et al., 2018). Recently, this backpacking strategy entered a Phase 1 clinical trial (NCT03815682) for patients with selected solid tumors and lymphomas (US National Library of Medicine, 2019). In the context of immunotherapy-loaded backpacking nanomaterials, many studies have reported tumor growth suppression and better mouse survival (Huang et al., 2015; Jones et al., 2017; Siegler et al., 2017; Siriwon et al., 2018). In addition to the backpacking strategy, tumor-specific T-cells can also be enriched and expanded from rare naïve precursors using nanoscale artificial antigen-presenting cells (aAPCs). Nanoplatforms such as iron-dextran-derived nano-aAPCs were utilized for the enrichment of antigen-specific T-cells in a magnetic column. The interaction between aAPCs and enriched T cells induced the expansion of tumor-specific T cells (Perica et al., 2015; Mi et al., 2019).
Genome-editing tools like CRISPR/ Cas9 can be transported to perform in situ CAR-T therapy via a NP-mediated delivery system (Liu et al., 2017; Wang et al., 2017a). Safety issues arising in engineering CAR T-cells can be circumvented with targeted delivery of leukemia-specific CAR genes into T cells in vivo. Smith et al. (2017) developed polymer-based NPs conjugated with anti-CD3e f(ab′)2 fragments-polyglutamic acid (PGA) on the surface. These polymer NPs facilitate in situ reprogramming of T cells by delivering plasmid DNA encoding leukemia-specific CAR. This method has been shown to provide an appropriate way for tumor regression in B-cell acute lymphoblastic leukemia mouse model. An alternative strategy involved the use of nanomaterials to increase T cell penetration and alter the immunosuppressive TME in solid tumors thus enhancing the efficacy of ACT (Gong et al., 2021). In melanoma mouse model, PLGA nanomaterial containing photothermal agent indocyanine green (ICG) was injected intratumorally in combination with near-infrared light irradiation, prior to administration of CAR T-cell, to promote tumor cells killing and removing the extracellular matrix (ECM) barrier (Chen et al., 2019). In contrast to CAR T-cells alone, the use of nanomaterials has been shown to enhance T cell penetration in solid tumors and thus improve the antitumor activity of CAR T-cells. Some studies have reported a nano-mediated delivery of antifibrotic drugs to disrupt the fibrotic ECM of solid tumors (Chen et al., 2019; Huang et al., 2020). To improve the efficacy of CAR T-cell therapy, Coon et al. (2020) locally delivered antigen-specific T cells using micropatterned nickel-titanium (Nitinol) thin films (TFN). These micromesh implants, loaded with CAR T-cells, served as an efficient method to systematically deliver T cells directly to the tumor and promote the expansion of T cell populations in an ovarian cancer mouse model. Thin-film-deployed T cells were able to inhibit tumor growth and enhance survival in various mouse models. Hence, targeting adaptive immune cells using CAR T-cells is an effective anticancer strategy.
The potential of NPs to improve the efficacy of NK cell-based immunotherapy has been explored. Nanomaterials have been designed to expand and/or activate NK cells and target NK cells to the tumor sites (Di Trolio et al., 2015). Plasma membrane (PM15) particles enhanced the rate of NK cell expansion from peripheral blood mononuclear cells (PBMCs) in a PM-particle concentration-dependent manner. In various leukaemia cell lines and also against patient AML blasts, cytotoxicity of PM-NK cells was higher than freshly isolated NK cells (Oyer et al., 2015). Artificial necroptotic cancer cells were shown to promote the expansion of IFN-γ-expressing CD8+ T cells and NK cell subsets. These cells act as flexible platforms for co-delivering cancer membrane proteins, danger-associated molecular patterns (DAMPs), signal-augmenting element α-helix HSP70, and CpG to NK cells and antigen-presenting cells (APCs). In a mouse model, αHSP70p-CM-CaP nanovaccine resulted in almost complete tumor regression combined with anti-PD-1 antibody treatment (Kang et al., 2018). Another approach involved targeting NK cells to tumor sites using magnetic NPs under the influence of an external magnetic field. A fluorophore Cy5.5 - magnetic iron oxide NP coated with silica (Fe3O4/SiO2) was also used to target NK cells. These NPs were able to control the movement of NK-92MI cells using an external magnetic field. This magnetic field-induced strategy enhanced NK-92MI cell penetration into the target tumor site (Jang et al., 2012). Lipid-based immuno-NPs coated with polyethylene glycol (PEG) encapsulated with cyclic diguanylate monophosphate (cdGMP), and monophosphoryl lipid A, a TLR4 agonist were also designed for tumor targeting. The systemic administration of these NPs promoted the accumulation of NK cells into tumor sites and mediated the production of IFN-β, resulting in a sturdy antitumor response (Atukorale et al., 2019).
4.3 Modulation of Innate Immune Cells Using Nanoparticles
The tumor microenvironment (TME) consists of tumor cells, non-cancerous cells, soluble signaling molecules, extracellular matrix (ECM), and vasculature. Non-cancerous cells comprise fibroblasts, immune cells (including innate and adaptive cells), and stromal cells. The innate cells such as macrophages, DCs, and neutrophils interact with various chemokines and within themselves, resulting in either inhibiting or promoting tumor growth. The adaptive immune cells can identify and destroy the tumor cells known as ‘Tumor immune surveillance’ (Quail and Joyce, 2013; Hirata and Sahai, 2017; Le et al., 2019). However, immune cells have been shown to play a critical role in tumor progression to advanced stages in cancer patients. The cells of the immune system, both innate and adaptive, interact with the tumor cells resulting in angiogenesis which in turn promotes tumor development and metastasis. In addition, tumor cells may evade immune recognition by secreting molecules responsible for the inactivation of immune cells. The influence of the immune system in promoting tumor progression makes them a potent target for treating cancer. There have also been efforts to modulate various innate immune cells for cancer immunotherapy (Le et al., 2019).
4.3.1 Dendritic Cells
Dendritic cells (DCs), also known as professional APCs, are considered the center of the immune system. They act as a bridge between innate and adaptive immunity and can recognize antigens and present them on the cell surface to activate naïve T cells, thereby generating a robust immune response (Constantino et al., 2017). The various components found in TME disrupt or block DC-mediated immune responses. Tumor-induced DC tolerance plays a crucial role in immune evasion and tumor development (Li R. et al., 2017). As a therapeutic strategy, immunomodulatory molecules are used for enhancing the functions of DC. However, there are limitations with stability and adverse effects with these immunomodulators (Le et al., 2019). Thus, NPs conjugated with immunomodulators were developed in combination with photothermal therapy (Chen et al., 2016) or with cancer vaccines (Kuai et al., 2017; Wilson et al., 2018).
Various studies have explored the use of nano-mediated delivery of peptide molecules for efficient activation and maturation of DCs. Lipid-calcium-phosphate (LCP) NPs were used as carriers to deliver a tumor-specific antigen, to DCs. LCP NPs function as an efficient encapsulation to prevent cargo drugs from enzymatic degradation. The results showed that NPs led to DC maturation for antigen presentation by alternating intracellular calcium dynamics. This facilitated the production of IFN-γ, thereby inducing an antigen-specific CTL (cytotoxic T lymphocyte) immune response (Liu Q. et al., 2018). Oligonucleotides containing unmethylated CpG motif (CpG-ODN) have been shown to facilitate activation of DCs via TLR9 mediated binding. This strategy has been employed in numerous clinical trials for the treatment of non-small cell lung cancer (NCT00070629) and skin cancer (NCT01149343), in combination with chemotherapy and cancer vaccines, respectively. CpG-ODN wrapped in nanocarriers has been shown to achieve highly efficient co-delivery of antigens and CpG adjuvants (Le et al., 2019). Kuai et al. (2017) designed a high-density lipoprotein (HDL) nanodisc conjugated with neoantigen-derived peptides and cholesterol-modified CpG-ODN. This nanodisc administration to the melanoma mouse model was shown to induce a robust CTL-mediated immune response compared to free CpG-ODN resulting in improved tumor protection and decreased lung metastatic lesions. This nanovaccine enhanced delivery of CpG adjuvants to DCs at draining lymph nodes (dLNs) and sustained antigen presentation on DCs.
In addition to working as a delivery system, some NPs have been reported that act as potent immunotherapeutic molecules. Luo et al. (2019) demonstrated for the first time that ultra-small iron oxide (Fe3O4) NPs combined with ovalbumin (OVA) could function as nano-immunopotentiators. These Fe3O4-OVA nanocomposites promote in vitro activation of mature bone marrow-derived DCs (BMDCs) and macrophages. It has also been shown as an effective therapeutic and prophylactic agent for lung metastasis of melanoma. A chimeric crossed-linked polymersomes formed as a result of self-assembly of triblock copolymers called pyropheophobide-a has enabled the MC38-colon adenocarcinoma cells to accelerate immunogenic cell death and has facilitated photodynamic therapy when encapsulated with doxorubicin and photosensitizer, respectively (Mehata et al., 2020). A nano-based approach for combinatorial photothermal therapy and immunotherapy has also been explored to treat cancer. An endogenous vaccine composed of a TLR7/8 agonist, and indocyanine green, a photothermal agent enclosed in PLGA NP was designed. After administration of this complex to an orthotopic murine breast cancer model, near-infrared light is utilized to induce photothermal ablation at the tumor site. This resulted in the release of tumor-associated antigens to promote maturation and antigen presentation of DCs. When combined with anti-CTLA-4 therapy, this strategy prevented tumor relapse by generating a strong immune-memory effect (Chen et al., 2016).
4.3.2 Tumor-Associated Macrophages
Macrophages are phagocytes that help remove pathogens and cellular debris. Other function of macrophages includes antigen presentation and secretion of several inflammatory mediators. Macrophages consist of two subtypes: M1 (classically activated) and M2 (alternatively activated) phenotypes. The plasticity of these cells depends on the type of cytokine they are exposed to. The TME is mainly composed of tumor-associated macrophages (TAMs); for instance, in the case of breast carcinomas the TME comprises up to 70% of TAMs. They are key regulators which contribute to the immunosuppressive TME. TAMs promote the recruitment of myeloid-derived suppressor cells (MDSCs) and regulatory T cells (Tregs) at tumor sites while inhibiting the antitumor activity of CTLs. TAMs function as a double-edged sword in tumors by expressing M1-type cells with antitumor effects and M2-type cells with protumor effects. Generally, the M2-type is a dominant phenotype in the TME. As the tumor progresses, the polarization state of TAMs shifts from M1 to M2 phenotype depending on stimuli from the microenvironment. The M2-type cells play an essential role in tumor invasion and metastasis and correlate with poor clinical outcomes (Webb et al., 2007; Yang Y. et al., 2020; Zhu et al., 2020). Thus, therapeutic strategies targeting TAMs have great potential in cancer immunotherapy. The heterogeneous population of macrophages coexisting within the TME poses an obstacle to the specificity of TAM-targeting drugs. Recently, NPs have been explored as a drug-delivery system to facilitate the modulation of TAMs (Yang Y et al., 2020).
The application of NPs has been studied to reprogram the TAM phenotype (Figure 3). Iron oxide (Fe3O4) NPs such as FDA-approved Ferumoxytol are commonly used for treating iron deficiency. In a study, Zanganeh et al. (2016) demonstrated the anticancer effect of ferumoxytol NPs, which is attributed to its ability to modulate the polarization of TAMs to the M1 phenotype. The phenotypic transition promotes the generation of reactive oxygen species (ROS) via Fenton reaction, which kills cancer cells. The apoptotic cancer cells trigger M1 polarization, creating an autocrine feedback loop that resulted in the continuous generation of tumor necrosis factor-alpha (TNF-α) and nitric oxide. This study confirmed that the administration of ferumoxytol suppresses tumor growth and metastasis in mouse models. Macrophages are usually present in large numbers within the hypoxic region of solid tumors. Tumor hypoxia shifts the polarization of TAMs from M1 towards M2 phenotype, which then contributed to tumor growth and metastasis. Song et al. (2016) utilized mannan-coated manganese-dioxide (MnO2) NPs conjugated with hyaluronic acid (HA) to form Man-HA-MnO2. After treatment, Man-HA-MnO2 NPs were taken up by TAMs via mannose receptor binding and reacted with hydrogen peroxide to lessen tumor hypoxia. The HA layer further improved hypoxia and chemotherapy response by facilitating the phenotypic transition of TAMs towards M1-type. In another study IL-12 conjugated polymeric NPs were developed by the emulsion water-in-oil method. This nanostructure dissociated in a slightly acidic pH of 6–7, resulting in the prolonged release of IL-12. The released IL-12 promoted the transition of macrophages towards tumor-suppressive M1-type to generate an antitumor immune response. The B16F10 cell-bearing tumor mice model treated with IL-12 loaded-cationic NPs showed an improved immunotherapeutic effect than those treated with free IL-12. However, this study showed that pH-responsive nanomaterials could locally modulate the phenotype of TAMs (Wang et al., 2017b).
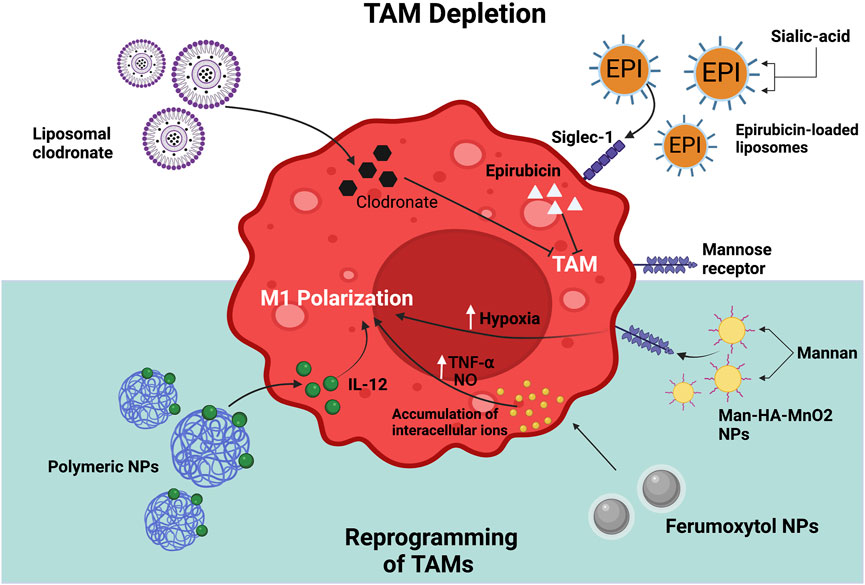
FIGURE 3. Strategies for targeting TAMs using NPs. The main approaches involve depletion of TAMs in TME; and reprogramming of TAMs to a M1 phenotype. The figure illustrates different types of NPs that have been used to target TAMs and their mechanism of action in macrophages. Created with Biorender.com.
Another promising strategy involves the targeted delivery of drugs that can remove TAMs in the tumor tissue. For this, the use of bisphosphonate has been widely explored owing to its ability to cause apoptosis of TAMs. The combination therapy of these drugs with NPs aimed towards overcoming the issues with bisphosphonates, such as short half-life and increased accumulation in the bone (Zhu et al., 2020). For example, Zhan et al. (2014) synthesized a bisphosphonate-glucomannan nanoconjugate to develop a macrophage-targeting carrier. The glucomannan polysaccharide from Bletilla striata (BSP), which has a high affinity for mannose receptors on macrophages, was conjugated with alendronate (ALN), a bisphosphonate compound with the ability to eliminate TAMs. The nanoconjugate was observed to target and induce apoptosis of TAMs in vivo, compared to free ALN. This eventually resulted in inhibition of angiogenesis and thereby tumor progression in the subcutaneous S180 tumor-bearing mice model. Thus, the study suggested that ALN-BSP nanoconjugate can be a safe and efficient therapeutic strategy. The systemic injection of clodronate, (a bisphosphonate compound) conjugated with liposomes decreased the number of macrophages in a genetic mouse model of pancreatic cancer. This led to the prevention of metastasis in liver and lungs, which was related to diminished angiogenesis and altered infiltration of immune cells (Griesmann et al., 2017). Sialoadhesin, also called Siglec-1, is a sialic acid (SA) binding receptor expressed on the surface of TAMs. Hence, targeted delivery of drugs using liposomes decorated with sialic acid could be a potential therapeutic strategy for depleting TAMs in the TME (She et al., 2014; Zhou et al., 2017). For example, Zhou et al. (2017) designed epirubicin (EPI) loaded liposomes modified with SA-cholesterol conjugate. The use of SA conjugated liposomes increased the accumulation of epirubicin in TAMs, which led to their depletion in tumor-bearing mice. The elimination of TAMs and the shedding of tumor tissues contributed to the improved antitumor efficacy of these NPs. In addition, this study reported improved mice survival and reduced systemic toxicity.
4.3.3 Myeloid-Derived Suppressor Cells
Myeloid-derived suppressor cells (MDSCs) are a heterogeneous population of immune cells of myeloid origin, which are immunosuppressive in nature. These cells are generated during inflammation and low-level exposure to molecules associated with the typical maturation of myeloid cells. There are two major subsets of MDSCs, namely mononuclear-MDSCs (Mo-MDSC) and polymorphonuclear-MDSCs (PMN-MDSCs). These cells are responsible for immunologically “cold” TME as they suppress innate and adaptive immunity. Hence, the accumulation of MDSCs is associated with cancer progression and poor clinical outcomes. Therapeutic strategies aimed at modulating MDSCs to overcome immunosuppressive TME have unlocked a new avenue for immunotherapy (Rameshbabu et al., 2021).
The application of nanomaterials for the modulation of MDSCs has been explored to restore antitumor immune responses. One way is to deplete MDSCs in the tumor tissues and this strategy has been utilized in a study where Gemcitabine, a nucleotide analog, was encapsulated in anti-Flt1 antibody-conjugated PEG-cored poly(amidoamine) (PAMAM) dendrimers. This resulted in a significant reduction in the number of myeloid cells and alternation in levels of immune infiltration. In addition, the dendrimeric delivery platform led to improvement in the anticancer efficacy of Gemcitabine against pancreatic cancer (Yoyen-Ermis et al., 2018). Liu H. et al. (2018) utilized T1 aptamer conjugated to liposomes encapsulating doxorubicin (DOX) to kill tumor cells via MDSC depletion. T1 DNA aptamer effectively bound to PMN-MDSCs and tumor cells which was validated in several mouse models of breast cancer. Here, the improved antitumor immune response was also identified by an increase in the number of tumor-infiltrating CD8+ T cells.
Apart from the depletion of MDSCs, another strategy is to differentiate MDSCs into antitumor cell types. Kong et al. (2017) designed MSNPs to co-deliver IL-2, doxorubicin (DOX), and all-trans retinoic acid (ATRA) for chemo-immunotherapy. ATRA enhanced anticancer immune response by inducing differentiation of MDSCs into mature DCs, macrophages, and granulocytes. In B16F10 melanoma model, these NPs have demonstrated inhibition of tumor growth and metastasis. Another effective treatment involved the manipulation of MDSCs by suppressing the expansion and tumor trafficking of MDSCs. For example, neutrophil plasma membrane-coated NPs neutralized MDSC-related cytokines, as the coat of the neutrophil plasma membrane acquired most membrane receptors from the “parental” neutrophils. This pseudocell nanoplatform decreased the proliferation and accumulation of MDSCs in tumors and peripheral lymphoid organs. In addition, there was an increase in the numbers of TILs (tumor-infiltrating lymphocytes), resulting in the reversal of immune tolerance in the TME (Li et al., 2020). Alternatively, Huo et al. (2017) developed a potent mannose-modified lipid-calcium-phosphate (LCP) NP-based Tyrosinase-related protein 2 (Trp2) vaccine that inhibited tumor progression in the early stages of melanoma. Sunitinib base, a multitarget receptor tyrosine kinase inhibitor, was delivered to the tumor using anisamide-modified PLGA-PEG polymeric micelles (SUNb-PM) to treat advanced melanoma models. Within the TME, they observed a reduction in levels of MDSC and T regulator cells as well as an increase in the number of tumor-infiltrating cytotoxic T cells. The SUNb-PM nanoplatform working in synergy with vaccine therapy remodels the TME and impedes the growth of late-stage tumors. The modulation of the various components of TME such as DCs, macrophages using NPs has shown to enhance the therapeutic efficacy of conventional strategies such as radiotherapy, chemotherapy as well as counter several challenges such as drug resistance, etc. However, little is known about the effect of these variations in the innate and adaptive immune cell dynamics on the whole system. Hence, with further research in the field of cancer immunology and nanomedicine, NPs have the potential as a promising tool in cancer immunotherapy (Yang M et al., 2020).
4.4 Development of Monoclonal Antibodies
Recently, monoclonal antibodies (mAbs) have become a successful and important strategy for treating cancer. Each mAb is generated from a single B cell clone, and each binds to a specific epitope. In 1973, Jerrold Schwaber identified a method of producing mAbs using human-mouse hybrid cells. Later, George Köhler and Cesar Milstein successfully produced human-derived hybridomas capable of producing antibodies, for which they were awarded the Nobel Prize in 1984. This discovery has inspired the development of mAb-based therapy for treating cancers (Zahavi and Weiner, 2020). Rituximab became the first FDA-approved mAb for treating patients suffering from relapsed B-cell non-Hodgkin’s lymphoma. Since then, numerous mAbs have been approved for clinical use against various cancers (Adler and Dimitrov, 2012). The mechanism of action by which antibodies induce their antitumor response includes targeting tumor cells and enhancing host immune responses for long-term effects. One strategy for inducing tumor cell death is via directly targeting surface antigens overexpressed in cancer cells. There is a subclass of mAbs which works indirectly by activating the immune system by inducing either complement-dependent cytotoxicity (CDC), antibody-dependent cellular cytotoxicity (ADCC), or antibody-dependent cellular phagocytosis (ADCP). In recent years, mAb-based therapy has achieved notable success with clinical applications in cancer immunotherapy. Trastuzumab (anti-HER2 mAb) and Ipilimumab (anti-CTLA-4) were approved by the US FDA for treating patients with HER2-positive breast cancer and melanoma, respectively (Zahavi and Weiner, 2020). Despite the success of mAbs, some immune-related side effects such as fever, headache, muscle pain, and fatigue were observed in patients during clinical trials. These adverse effects are associated with the half-life and dosage of antibodies. This limitation in the therapeutic efficacy of mAbs is due to its structural instability and vulnerability to chemical degradation. Hence, NPs have been developed as carrier systems for releasing mAbs in a precise manner to protect them from degradation and enhance their biological activity (Wang et al., 2007; Wang et al., 2021).
Nanoplatforms have been employed for transporting antibodies directly to the tumor site. In a study, Rahimian et al. (2015) utilized biodegradable poly (d, l lactic-co-hydroxymethylglycolic acid (pLHMGA) microparticles for co-delivering immunomodulatory antibodies (antiCTLA-4 and antiCD40). These microparticles demonstrated high loading efficiency of >85% and prolonged release of the antibodies for over 30 days. In colon carcinoma tumor model (MC-38), microparticles exhibited antitumor efficacy similar to incomplete Freund’s adjuvant (IFA). In addition, the cured mice showed complete resorption of microparticles and no local side effects. Polymeric microparticles have shown potential as an antibody-delivery system for cancer immunotherapy. Chen et al. (2014) synthesized PLGA NPs through double emulsion method for efficiently delivering anti-OX40 mAb to the target site. This NP conjugated mAbs was able to enhance CTL proliferation as well as tumor antigen-specific cytotoxicity compared to free anti-OX40 mAb. This robust immune response is also associated with increased production of cytokines such as IL-2 and IFN-γ.
Antibodies are used in conjugation with cytotoxic molecules, also known as antibody-drug conjugates (ADC), to target tumor cells directly. These conjugates exhibited improved clinical efficacy and reduced toxicity than anticancer drugs alone (Sievers and Senter, 2013). Various studies have demonstrated the benefits of antibody-NP conjugates, such as preventing the inactivation of drugs and maximizing the administered dose to the tumor site (Fay and Scott, 2011). Furthermore, NPs loaded with mAbs are commonly used as immuno-nanocarriers in cancer treatment. Cirstoiu-Hapca et al. (2010) were able to efficiently deliver paclitaxel (Tx) with the help of trastuzumab (anti-HER2)-coated immuno-nanocarriers. The NPs-Tx-HER showed enhanced anticancer efficacy compared to paclitaxel alone. These results were validated using bioluminescence imaging and measuring the survival rate in ovarian xenograft cancer model. The anti-HER2 coating improved the biodistribution of paclitaxel at tumor sites and prolonged the survival of mice. Thus, the combination of ADC (antibody-drug conjugates) and NPs has been shown to enhance drug efficacy. Various studies have demonstrated the usage of anti-EGFR conjugated NPs for cancer treatment. EGFR, a tyrosine kinase receptor, is expressed more in various cancers including breast and ovarian cancer. Hence, PLGA NPs coated with EGFR antibodies were designed for targeted delivery of rapamycin, an anticancer drug, in breast carcinoma MCF7 cell line. This nanoconjugate has shown increased antigrowth activity than unconjugated NPs or free rapamycin, which is linked to their improved cellular uptake. In addition, injected NPs promoted the expression of proteins involved in cell death pathways, resulting in enhanced cellular cytotoxicity (Acharya et al., 2009). The use of AuNPs tethered to VEGF antibody (AbVF) has been explored as a treatment option for B-cell chronic lymphocytic leukemia (CLL). The co-culture of CLL B cells with these antibody-nanoconjugates was observed to promote apoptosis more than non-coated AuNPs or AbVF alone. This improvement in the therapeutic effectiveness of VEGF antibody as well as a reduction in the amount administered can be incorporated into the treatment of chronic lymphocytic leukemia (Mukherjee et al., 2007). Currently, numerous antibody-nanoconjugates have undertaken preclinical research whereas clinical trials have begun for only some of them. Nanoconjugates such as BIND-014 have successfully completed Phase II clinical trials and showed therapeutic effects in several cancers along with minimal side effects (Cruz and Kayser, 2019).
4.5 RNA Mediated Cancer Immunotherapy
In 1986, IFN-α2 was first utilized for immunotherapeutic purposes, marking the beginning of immunotherapy. Since then, mAbs, other recombinant proteins, as well as cell therapy and ex vivo gene therapy, have received substantial attention as immunotherapy candidates. In recent years, RNA therapeutics has emerged as a novel class of drugs with a wide range of potential applications. Unlike the conventional small molecular drugs (primarily antibodies), RNA-based therapies could perform remarkable regulatory activities in targeted cells by modulating the expression of various proteins or knocking down targeted genes to differing levels. Some of the major capabilities of RNA-based therapeutics that are utilized in immunomodulation and cancer immunotherapy include silencing immunological checkpoint genes (ICB), acting as a tumor antigen vaccine, and activating the innate or adaptive immune system by altering cytokine expressions (Aagaard and Rossi, 2007; Li and Rana, 2014). RNA-based agents, such as mRNA, microRNA (miRNA), small interfering RNA (siRNA), and shRNAs (short hairpin RNAs), have been recognized as extremely appealing therapeutic options for different types of diseases such as cancers, genetic disorders, inflammatory and neurodegenerative diseases (Adams et al., 2017; Chakraborty et al., 2017; Kaczmarek et al., 2017; Rupaimoole and Slack 2017; Zhao et al., 2017; Mirzaei et al., 2018). Over the past decade, many clinical trials have been conducted using these genetic elements, proving them to be promising for cancer treatment. However, certain factors such as their stability, innate immune responses and delivery to the tumor site pose significant technical challenges. The presence of various biological barriers and the instability of RNAs themselves prevent their delivery and transfection hindering their clinical application in cancer treatment (Iyer et al., 2014; Chen et al., 2017). Furthermore, the intrinsic defense systems such as the innate immune system, enzymes (RNases and exonucleases), and tissues or organs like liver and kidney can eliminate foreign RNA in various ways (Iyer et al., 2014; Kaczmarek et al., 2017). The application of NP-based delivery systems in vitro and in vivo could overcome these immunological barriers, thereby aiding the safe transport of the RNA therapeutics to their targeted sites (Chen et al., 2017; Hajj and Whitehead, 2017). Also, naked RNA molecules have very short half-lives, low chemical stability, and are degraded by nucleases in in vivo applications; therefore, nanotechnology offers a diverse and controlled mechanism for their safe delivery to the targeted system (Mura et al., 2013).
The advancements in drug delivery through the use of diverse NP-based technologies, such as liposomes (Allen and Cullis, 2013), inorganic NPs (Guo and Huang, 2012), PNPs (Zhang et al., 2007; Lin et al., 2015; Wang et al., 2015; Lin et al., 2016; Loh et al., 2016; Shen et al., 2018), and bio-inspired nanovehicles (Yoo et al., 2011; Li X. et al., 2017), have provided effective delivery of RNAs in cancer immunotherapy. Several crucial obstacles, particularly the rapid degradation of exogenous RNA by RNases found abundantly in the environment and host tissues had to be overcome in the development of RNA therapies. NPs, along with RNase resistance strategies, are being developed to enhance the stability of RNA (Pastor et al., 2018). Thus, the development of NP-based delivery systems Helps shield RNA molecules from enzymatic degradation and immunological challenges and enables the accumulation of RNA at the tumor site (Pecot et al., 2011; Xiong et al., 2018).
In immunomodulatory approaches too, RNA-based treatments have various benefits such as high specificity and selectivity for silencing (e.g., siRNA, shRNA, and microRNA) or enhancing the expression of specific targets. Numerous studies have validated that the antitumor immune response can be efficiently induced by silencing some key tumor development factors, particularly the knocking down of the immunosuppressive genes in cancer cells and cancer-associated immune cells (Aleku et al., 2008; Ghafouri-Fard and Ghafouri-Fard, 2012; Anfossi et al., 2018). Nanomaterials can serve as a shield for delivering siRNAs to cancer or immune cells to regulate the immunological responses (Freeley and Long, 2013; Conde et al., 2016). Utilizing RNA therapeutics in conjugation with nanomaterials is a promising strategy to boost cancer immunomodulation efficacy. Here, we summarize some possible siRNA/shRNA-mediated immunotherapy with a nanostructure delivery system.
The usage of siRNA nanotherapeutics in targeting tumors has gained significant attention in recent years for their ability to induce antitumor immune responses by downregulating anti-inflammatory cytokines, “don’t eat me” signals, immune checkpoint proteins, and so on. For example, Wang et al. (2013) devised a systemic delivery model for CD47 siRNA administration to melanoma cancer cells using hyaluronic acid-coated lipid NPs, which resulted in an effective CD47 knockdown in cancer cells. This CD47 silencing significantly reduced tumor development in the melanoma mouse model. Similarly, an improved antitumor response was achieved by employing siRNA-based nanotherapeutics, that knocked down immune checkpoints and anti-inflammatory cytokines on tumor cells, causing tumor suppression in vivo. The silencing of TGF-β siRNA with liposome-protamine-hyaluronic acid (LPH) NPs in the TME enhanced the efficacy of LCP-based nano vaccine and significantly regressed tumor growth in an advanced melanoma model (Xu et al., 2014). Thus, the combinatorial therapy involving two NPs was seen to elicit a robust anticancer effect and act as a viable option for treatment for cancer. Based on these, several recent studies have reported a variety of techniques that combine chemical or photodynamic drugs with RNA-based nanoplatforms (Wang et al., 2016; Dai et al., 2018). In one such study, a pH-responsive nano-system was used by combining photodynamic therapy and immunotherapy. PD-L1 (programmed cell death ligand-1) specific siRNA and a mitochondrion-targeting photosensitizer were combined and targeted to the tumor sites, resulting in a combinatorial anticancer effect (Dai et al., 2018). The nanocarrier generated an effective immunological response by photodynamic treatment and induced siRNA-mediated Immune checkpoint blockade, which was confirmed by both in vitro and in vivo experiments. A similar experiment was also done by Qiao et al. (2018), where they developed a reactive oxygen species (ROS)-responsive nanotheranostic system coupled with temozolomide (TMZ) and siTGF-β. This downregulated TGF-β expression in tumor cells dramatically improved TMZ-mediated chemotherapy efficiency, thus considerably increasing the survival time of glioblastoma tumor-bearing mice.
Cancer cells have been shown to overexpress inhibitory molecules such as PD-L1 (programmed cell death ligand-1), known as immune checkpoints. Likewise, certain activated T cells also overexpress corresponding inhibitory molecules, like TIM-3 (mucin-containing protein 3), PD-1 and CTLA-4, that can downregulate T cell activities, thus preventing tumor cells from being eliminated by effector T cells (Byun et al., 2017; Tanaka and Sakaguchi, 2017). As a consequence, the antitumor effect could be significantly boosted by targeting the immune checkpoints of these T-cells. For this, Li et al., administered CTLA-4 siRNA to T cells using PEG–PLA-based NPs with cationic lipids (Li et al., 2016). Although only 4–6% of T cells consumed the NPs in vivo, it resulted in a 2-fold increase in effector CD8+ T cells thus drastically impairing tumor development, thereby increasing the survival time of mice with melanoma. A bio-NP-mediated delivery system was developed using EVs to deliver β-catenin siRNA to hepatocellular carcinoma (HCC) via intrahepatic injection (Matsuda et al., 2019). These EVs were systemically delivered along with anti-PD-1-based treatment. In vivo studies revealed that the anti-PD-1 with EVs enhanced CD8+ T cell infiltration and priming, thereby boosting the antitumor effect.
Another approach in cancer immunotherapy involves lowering survival and recruitment of TAMs (tumor-associated macrophages) in the tumor region, or executing targeted drug administration to the protumor M2-type TAMs, for decreasing their numbers in tumors or repolarizing them to antitumor M1-type TAMs (Wang et al., 2017b). Also, several studies have shown that certain cytokines such as vascular endothelial growth factor (VEGF), colony-stimulating factor 1 (CSF-1), etc., facilitate the recruitment of monocytes which are known to differentiate into TAMs (Guo et al., 2016). For this, Qian et al. (2017) employed dual-targeting NPs (M2NPs) which transported the siRNA of CSF-1 Receptor to the M2-like TAMs. This resulted in inhibiting the survival of these TAMs and thereby reducing their numbers within the tumor region. In addition, they have also been shown to increase the expression of immunostimulatory cytokines as well as CD8+ T cells infiltration into the tumor sites. The authors also reported that reduction in the expression of exhaustion markers (PD-1 and TIM-3) and increase in IFN-γ production resulted in enhancing the antitumor activity of T cells significantly. In vivo data showed that administration of M2NPs reduced M2-like TAMS by 52%, thus dramatically suppressing the tumor development, thereby extending the survival of mice. Additionally, Conde et al. (2015) designed peptide-functionalized AuNPs which specifically transported VEGF (vascular endothelial growth factor) siRNA to M2-like TAMs. VEGF, a major angiogenic component, is strongly expressed on M2-like TAMs and also helps to promote tumor growth and metastasis. Thus, the silencing of VEGF resulted in significant regression of tumor growth, thereby increasing the survival of the lung cancer orthotopic mouse model. The use of siRNA-mediated silencing was able to immunomodulate the population of TAMs in the TME (tumor microenvironment). A lower accumulation of TAMs was achieved by targeting the VEGF pathway, which implied that TAMs modulation would generate an antitumor immune response and could be a viable cancer therapeutic target.
DCs (dendritic cells) express various co-inhibitory molecules such as signal transducers and activators of transcription-3 (STAT3), suppressors of cytokine signaling (SOCS) 1 and indoleamine 2,3-dioxygenase (IDO), which may hamper the antigen presentation process of DCs. Hence, knocking down these molecules with RNAi is demonstrated to be a viable approach for DC-based immunotherapy (Conroy et al., 2012; Zheng X. et al., 2013). Heo et al. (2014) developed PLGA polymeric NPs encapsulating siRNA of immunosuppressive protein SOCS1 and certain tumor antigens such as ovalbumin (OVA). SOCSI acts as a negative regulator of Janus kinases (JAKS), which induce immunological resistance, as well as plays a key role in hindering antigen presentation of DCs to T cells. The authors observed that the PLGA NPs were readily absorbed by bone marrow-derived DCs (BMDCs). This uptake resulted in a significant knockdown of SOCS1 expression leading to an increase in the production of cytokines like IL-6 and IL-12, thus generating a robust antitumor immune response.
5 Application of Nanotechnology in Cancer Theranostics
As an essential component in the development of personalised medical treatment, cancer theranostics represents combining diagnosis and therapeutic approaches to ease patient care. The idea developed from the general belief that the treatment procedure could be eased if the growth could be reduced by the process of diagnosis itself (Lim et al., 2015). Though numerous approaches have been under trial to improve the efficacy of cancer immunotherapy, the development of a distinct formulation of NPs in combination with antigens, cytokines, nucleotides, and chemokines has shown promising results under various preclinical studies. The development of artificial antigen-presenting cells (APCs) helps to seize foreign particles and pathogens and enable the activation of T cells. Such synthetic APCs often possess MHC-epitope or anti-CD28 agonist that are conjugated (Goldberg, 2015). Similarly, in association with nano-vaccines perishable poly(dilactide-co-glycolide) NP carrying anti-OX40 antibody was used to induce tumor-specific toxicity and cytotoxic lymph proliferation (Chen et al., 2014). A hundred percent survival was observed in the TC1 model when PC7A NPs were co-delivered with anti-PD1 and antigens, depicting extraordinary synergy (Luo et al., 2017). Research has shown that polymeric NPs can imitate biological interactions between APCs and T cells, encouraging antitumor immunity (Gao et al., 2015). Accumulation of NPs in the resection cavity after tumor surgery has also, in many cases, enabled the reduction of local and distant recurrence risk (Miller et al., 2017; Wang et al., 2017). NPs are also employed to mimic the function of innate immune cell subsets, increasing the efficacy of T cell activation. Surface-developed NPs were used to kill TAMs present in the TME. Active or passive transport of immunomodulatory materials with the help of NPs to the TME has been achieved and, when combined with existing therapeutic approaches, has been used to tackle the immunosuppressive nature of the same. Other than these, combining polymeric nanocarriers like PNPs with inorganic ones like Au, SPIONs, and Ag and their assembly into nano-sized clusters give rise to the emergence of new possibilities in tumor targeting, imaging, therapy, and drug delivery (Villela Zumaya et al., 2022)
6 Nanotechnology Based Cancer Immunotherapeutic Strategies in Clinical Trials
The studies on the feasibility of the use of NPs in cancer immunotherapy in animal models have led to the next big question of it being a felicitous agent to be taken up for clinical trials. Clinically approved nanomedicines that exploit the advantages of nanoscale entities like targeted liposomes, and even combination therapies using them have trickled in over the subsequent years and are recently being approved for next-generation platforms. Other than these, there are at least 80 clinical trials that involve cancer nanomedicines which are either in the active or recruiting phase according to clinicaltrials.gov (clinicaltrials.gov). A study that evaluated the safety and tolerability of paclitaxel using albumin-based NP in patients with triple-negative breast cancer and gynecological malignancies was completed by Arcus Biosciences, Inc. and Infinity Pharmaceuticals, Inc. (NCT03719326). Atomic nanogenerators constituting Actinium-225 labeled humanized anti-CD33 monoclonal antibody (HuM195) have completed clinical trials in patients with advanced myeloid malignancies (NCT00672165). PRECIOUS-01 is an immunomodulating agent composed of threitolceramide-6, a natural killer T-cell activator that is in the recruiting phase for patients with NY-ESO-1-positive cancers (NCT04751786). Various combination therapies that combine the tumoricidal property of radiation and chemotherapy with the specificity of action of immune therapy are also being exploited and have entered clinical trials. Hafnium oxide-containing NPs NBTXR3, have been used in a combination between radiation therapy and pembrolizumab against metastatic head and neck squamous carcinoma. This has been tested in patients who have already undergone one radiation therapy (NCT04834349) and those who have not (NCT04862455). This was also studied in anti-PD1 therapy in combination with radiotherapy (NCT03589339). Another clinical trial in the recruiting phase analyzed the response and relapse in participants with diffuse large B-cell lymphoma using nanochip technology (immuno-tethered lipoplex NP biochip) (NCT03656835). BCMA-nano antibody-based CAR T-cell therapies are also recruiting patients with multiple myeloma under both refractory and relapsed category (NCT03661554). MM-398, a nano liposomal irinotecan (a medication used to treat colon and small cell lung cancers), was used to infer the feasibility of using a tumor imaging agent called Ferumoxytol (NCT01770353). Ferumoxytol is widely used in non-invasive diagnostic assays to visualize TAMs (Daldrup-Link et al., 2011). A cationic liposomal DNA-adjuvant complex, known to have been in use with H5N1 vaccine (Liu et al., 2016), has undergone clinical trial as an immunostimulant in the treatment of relapsed and refractory leukaemia (NCT00860522). Advanced triple-negative breast cancer, a high chemosensitive disease, has been treated at the first line using an anti-EGFR immunoliposome loaded with doxorubicin (anti-EGFR-IL-dox). Anti-EGFR-IL-dox was administrated to the patients every 28 days until progression or inadmissible toxicity (NCT02833766). Also, the efficiency of nano-luteolin was examined under clinical trials by employing its apoptotic properties on squamous cell carcinoma cell lines. The primary outcome though is apoptosis; the trial is expected to create a secondary outcome of cell viability. The results are not yet updated (NCT03288298).
Nanotechnology has also been applied in general and emergency surgeries. Bucky paper, a prosthetic material used in the treatment of hernia, is now under clinical trial to be used as an implant against solid and hematopoietic tumors (NCT02328352). Digital subtraction angiography-based nano-drug interventional therapy against lung cancer is being documented clinically to analyze the short and long-term efficacy (NCT02449122, NCT02449109). The safety and efficacy of AGuIX, a gadolinium-based NP, when in conjugation with MR-guided stereotactic body radiation therapy (SBRT) is also being determined in pancreatic and lung tumors (NCT04789486). Dose escalation of ceramide-based nanoliposome was analyzed clinically through intravenous administration in patients with advanced solid tumors (NCT02834611). Though there are several clinical trials that documented a lack of updates about their results and recruitment phase on the website, which makes these cases difficult for follow up.
Despite the successes of the research done to date, the utility of this field can only be determined by the impact it has on patient care outcomes. The fact that several successful therapies, even though they took more than two decades from their initial research discovery to their approval, implementation into standard practice is a silver lining. Thus, there is an immense need to keep enduring these efforts to bring the treatment methods to their clinical application. Studies that improve the biosafety of these drug carriers are the need of the hour. Moreover, a detailed analysis of the pharmacokinetic behavior of nanomaterials in animal models and the evaluation of host immune responses by various components need to be examined (Yan et al., 2019) (Table 1).
7 Conclusion and Future Prospects
To summarize, cancer immunotherapy, a method of artificially stimulating the immune system of a cancer patient to recognize and attack their cancer cells, has transformed the field of oncology both practically as well as philosophically. Be it the molecular targeted therapy or cytotoxic chemotherapy, the technique of exploiting the role of immune cells to target cancer cells has conferred better outcomes when compared to the previous regimen of treatment and care. Various clinical trials that have led to impressive improvement in the patient outcomes are proof of the same and have led to the development of novel immunotherapeutics. Despite this glorification, major hurdles like the complexity of tumors, TME, off-target side effects, and the low immunogenicity elicited are the challenges in the path of developing an ideal cancer immunotherapy technique. Acknowledging the fact that reawakening the immune response within this complexity can be arduous, and various modifications have been proposed in the existing techniques to enhance the permeation and retention of the molecules that are expected to act as anticancer drugs. The development of nanotechnology and nanomaterials can be exploited owing to their unique advantages. Nanotechnology offers to overcome the shortcomings such as targeted delivery, off-target effects and enhance the efficacy of the traditional immunotherapy techniques. Through this review, we have discussed how different NPs have been used to induce an immune response and target the immune-suppressive nature of the TME. Higher cellular uptake of immune-stimulatory agents with the use of NPs, and co-delivery of antigens and adjuvants have enhanced the efficacy of the existing techniques. However, the targeting ability of NPs depends on the characteristic of the material used and how advanced we are in developing these molecules to suit the experimental needs.
Biosafety of the nanocarriers is also an issue when considering the success of a preclinical trial further for its clinical interpretations, indicating an immense need to analyze the pharmacokinetic behavior of these NPs in animal models and a detailed understanding of the host immune response towards these molecules. Only a few studies have focused on ensuing immunogenic effects through the systemic interaction between the incorporated agents and the NPs (Kranz et al., 2016; Sayour et al., 2016). An ideal NP would be the one that does not induce hypersensitivity, the release of ROS or cause allergic reactions. Also, such NPs should be easily excreted from the body and should not interact with serum proteins, generating autoimmunity against itself. Nature-derived NPs are being formulated and exploited more these days due to their biocompatibility, but a detailed investigation is still required to analyze their immunostimulatory nature. Advancement in designing and fabricating nanomaterials and incisive and conclusive clinical research are the need of the hour to aid the development of safer and more efficient cancer immune therapeutics. With the proper characterization of these molecules, nano-pharmaceutical administration, and control of adverse after-effects, the combination of NPs with cancer immunotherapy can act as a pillar for cancer treatment, especially in the process of developing personalized therapy.
Author Contributions
LDK conceptualized, decided on the contents, critically reviewed and edited the manuscript. AN contributed to 50% of the manuscript. EG and AS have written rest of 50% and Tables and Figures respectively.
Conflict of Interest
The authors declare that the research was conducted in the absence of any commercial or financial relationships that could be construed as a potential conflict of interest.
Publisher’s Note
All claims expressed in this article are solely those of the authors and do not necessarily represent those of their affiliated organizations, or those of the publisher, the editors and the reviewers. Any product that may be evaluated in this article, or claim that may be made by its manufacturer, is not guaranteed or endorsed by the publisher.
Acknowledgments
The authors are extremely grateful to V. Dinesh Kumar for his critical evaluation, suggestions, and help in editing the manuscript. Authors also thank Aviral Kumar for his valuable help with Biorender. EG was supported by INSPIRE scholarship for higher education from Department of Science and Technology (DST), Government of India.
References
Aagaard, L., and Rossi, J. J. (2007). RNAi Therapeutics: Principles, Prospects and Challenges. Adv. drug Deliv. Rev. 59 (2-3), 75–86. doi:10.1016/j.addr.2007.03.005
Abelin, J. G., Keskin, D. B., Sarkizova, S., Hartigan, C. R., Zhang, W., Sidney, J., et al. (2017). Mass Spectrometry Profiling of HLA-Associated Peptidomes in Mono-allelic Cells Enables More Accurate Epitope Prediction. Immunity 46 (2), 315–326. doi:10.1016/j.immuni.2017.02.007
Acharya, S., Dilnawaz, F., and Sahoo, S. K. (2009). Targeted Epidermal Growth Factor Receptor Nanoparticle Bioconjugates for Breast Cancer Therapy. Biomaterials 30 (29), 5737–5750. doi:10.1016/j.biomaterials.2009.07.008
Adams, B. D., Parsons, C., Walker, L., Zhang, W. C., and Slack, F. J. (2017). Targeting Noncoding RNAs in Disease. J. Clin. Invest. 127 (3), 761–771. doi:10.1172/JCI84424
Adler, M. J., and Dimitrov, D. S. (2012). Therapeutic Antibodies against Cancer. Hematol. Oncol. Clin. N. Am. 26 (3), 447–481. doi:10.1016/j.hoc.2012.02.013
Ahmad, M. Z., Ahmad, J., Haque, A., Alasmary, M. Y., Abdel-Wahab, B. A., and Akhter, S. (2020). Emerging Advances in Synthetic Cancer Nano-Vaccines: Opportunities and Challenges. Expert Rev. Vaccines 19 (11), 1053–1071. doi:10.1080/14760584.2020.1858058
Aleku, M., Schulz, P., Keil, O., Santel, A., Schaeper, U., Dieckhoff, B., et al. (2008). Atu027, a Liposomal Small Interfering RNA Formulation Targeting Protein Kinase N3, Inhibits Cancer Progression. Cancer Res. 68 (23), 9788–9798. doi:10.1158/0008-5472.CAN-08-2428
Ali, O. A., Huebsch, N., Cao, L., Dranoff, G., and Mooney, D. J. (2009). Infection-mimicking Materials to Program Dendritic Cells In Situ. Nat. Mater. 8 (2), 151–158. doi:10.1038/nmat2357
Allen, T. M., and Cullis, P. R. (2013). Liposomal Drug Delivery Systems: from Concept to Clinical Applications. Adv. Drug Deliv. Rev. 65 (1), 36–48. doi:10.1016/j.addr.2012.09.037
Almeida, J. P. M., Figueroa, E. R., and Drezek, R. A. (2014). Gold Nanoparticle Mediated Cancer Immunotherapy. Nanomedicine 10 (3), 503–514. doi:10.1016/j.nano.2013.09.011
Anfossi, S., Babayan, A., Pantel, K., and Calin, G. A. (2018). Clinical Utility of Circulating Non-coding RNAs - an Update. Nat. Rev. Clin. Oncol. 15 (9), 541–563. doi:10.1038/s41571-018-0035-x
Atukorale, P. U., Raghunathan, S. P., Raguveer, V., Moon, T. J., Zheng, C., Bielecki, P. A., et al. (2019). Nanoparticle Encapsulation of Synergistic Immune Agonists Enables Systemic Codelivery to Tumor Sites and IFNβ-Driven Antitumor Immunity. Cancer Res. 79 (20), 5394–5406. doi:10.1158/0008-5472.CAN-19-0381
Babiker, H. M., Riaz, I. B., Husnain, M., and Borad, M. J. (2017). Oncolytic Virotherapy Including Rigvir and Standard Therapies in Malignant Melanoma. Oncolytic virotherapy 6, 11. doi:10.2147/OV.S100072
Bandyopadhyay, A., Fine, R. L., Demento, S., Bockenstedt, L. K., and Fahmy, T. M. (2011). The Impact of Nanoparticle Ligand Density on Dendritic-Cell Targeted Vaccines. Biomaterials 32 (11), 3094–3105. doi:10.1016/j.biomaterials.2010.12.054
Berger, C., Berger, M., Hackman, R. C., Gough, M., Elliott, C., Jensen, M. C., et al. (2009). Safety and Immunologic Effects of IL-15 Administration in Nonhuman Primates. Blood 114 (12), 2417–2426. doi:10.1182/blood-2008-12-189266
Bertrand, N., Wu, J., Xu, X., Kamaly, N., and Farokhzad, O. C. (2014). Cancer Nanotechnology: the Impact of Passive and Active Targeting in the Era of Modern Cancer Biology. Adv. drug Deliv. Rev. 66, 2–25. doi:10.1016/j.addr.2013.11.009
Bouchkouj, N., Kasamon, Y. L., de Claro, R. A., George, B., Lin, X., Lee, S., et al. (2019). FDA Approval Summary: Axicabtagene Ciloleucel for Relapsed or Refractory Large B-Cell Lymphoma. Clin. Cancer Res. 25 (6), 1702–1708. doi:10.1158/1078-0432.ccr-18-2743
Brudno, J. N., and Kochenderfer, J. N. (2016). Toxicities of Chimeric Antigen Receptor T Cells: Recognition and Management. Blood 127 (26), 3321–3330. doi:10.1182/blood-2016-04-703751
Burnet, F. M. (1970). The Concept of Immunological Surveillance. Prog. Exp. Tumor. Res. 13, 1–27. doi:10.1159/000386035
Byun, D. J., Wolchok, J. D., Rosenberg, L. M., and Girotra, M. (2017). Cancer Immunotherapy - Immune Checkpoint Blockade and Associated Endocrinopathies. Nat. Rev. 13 (4), 195–207. doi:10.1038/nrendo.2016.205
Cao, G. D., He, X. B., Sun, Q., Chen, S., Wan, K., Xu, X., et al. (2020). The Oncolytic Virus in Cancer Diagnosis and Treatment. Front. Oncol. 10, 1786. doi:10.3389/fonc.2020.01786
Chakraborty, C., Sharma, A. R., Sharma, G., Doss, C., and Lee, S. S. (2017). Therapeutic miRNA and siRNA: Moving from Bench to Clinic as Next Generation Medicine. Mol. Ther. Nucleic acids 8, 132–143. doi:10.1016/j.omtn.2017.06.005
Chen, B., Dai, W., He, B., Zhang, H., Wang, X., Wang, Y., et al. (2017). Current Multistage Drug Delivery Systems Based on the Tumor Microenvironment. Theranostics 7 (3), 538–558. doi:10.7150/thno.16684
Chen, D. S., and Mellman, I. (2013). Oncology Meets Immunology: the Cancer-Immunity Cycle. Immunity 39 (1), 1–10. doi:10.1016/j.immuni.2013.07.012
Chen, M., Ouyang, H., Zhou, S., Li, J., and Ye, Y. (2014). PLGA-nanoparticle Mediated Delivery of Anti-OX40 Monoclonal Antibody Enhances Anti-tumor Cytotoxic T Cell Responses. Cell. Immunol. 287 (2), 91–99. doi:10.1016/j.cellimm.2014.01.003
Chen, Q., Hu, Q., Dukhovlinova, E., Chen, G., Ahn, S., Wang, C., et al. (2019). Photothermal Therapy Promotes Tumor Infiltration and Antitumor Activity of CAR T Cells. Adv. Mater 31, 1900192. doi:10.1002/adma.201900192
Chen, Q., Xu, L., Liang, C., Wang, C., Peng, R., and Liu, Z. (2016). Photothermal Therapy with Immune-Adjuvant Nanoparticles Together with Checkpoint Blockade for Effective Cancer Immunotherapy. Nat. Commun. 7, 13193. doi:10.1038/ncomms13193
Cirstoiu-Hapca, A., Buchegger, F., Lange, N., Bossy, L., Gurny, R., and Delie, F. (2010). Benefit of Anti-HER2-coated Paclitaxel-Loaded Immuno-Nanoparticles in the Treatment of Disseminated Ovarian Cancer: Therapeutic Efficacy and Biodistribution in Mice. J. Control. Release 144 (3), 324–331. doi:10.1016/j.jconrel.2010.02.026
Colzani, B., Pandolfi, L., Hoti, A., Iovene, P. A., Natalello, A., Avvakumova, S., et al. (2018). Investigation of Antitumor Activities of Trastuzumab Delivered by PLGA Nanoparticles. Int. J. Nanomed. 13, 957–973. doi:10.2147/IJN.S152742
Conde, J., Arnold, C. E., Tian, F. R., and Artzi, N. (2016). RNAi Nanomaterials Targeting Immune Cells as an Anti-tumor Therapy: the Missing Link in Cancer Treatment? Mater Today 19, 29–43. doi:10.1016/j.mattod.2015.07.005
Conde, J., Bao, C., Tan, Y., Cui, D., Edelman, E. R., Azevedo, H. S., et al. (2015). Dual Targeted Immunotherapy via In Vivo Delivery of Biohybrid RNAi-Peptide Nanoparticles to Tumour-Associated Macrophages and Cancer Cells. Adv. Funct. Mater. 25 (27), 4183–4194. doi:10.1002/adfm.201501283
Conroy, H., Galvin, K. C., Higgins, S. C., and Mills, K. H. (2012). Gene Silencing of TGF-Β1 Enhances Antitumor Immunity Induced with a Dendritic Cell Vaccine by Reducing Tumor-Associated Regulatory T Cells. Cancer Immunol. Immunother. 61 (3), 425–431. doi:10.1007/s00262-011-1188-y
Constantino, J., Gomes, C., Falcão, A., Neves, B. M., and Cruz, M. T. (2017). Dendritic Cell-Based Immunotherapy: a Basic Review and Recent Advances. Immunol. Res. 65 (4), 798–810. doi:10.1007/s12026-017-8931-1
Coon, M. E., Stephan, S. B., Gupta, V., Kealey, C. P., and Stephan, M. T. (2020). Nitinol Thin Films Functionalized with CAR-T Cells for the Treatment of Solid Tumours. Nat. Biomed. Eng. 4 (2), 195–206. doi:10.1038/s41551-019-0486-0
Cruz, E., and Kayser, V. (2019). Monoclonal Antibody Therapy of Solid Tumors: Clinical Limitations and Novel Strategies to Enhance Treatment Efficacy. Biol. targets Ther. 13, 33–51. doi:10.2147/BTT.S166310
Cruz, L. J., Tacken, P. J., Fokkink, R., Joosten, B., Stuart, M. C., Albericio, F., et al. (2010). Targeted PLGA Nano- but Not Microparticles Specifically Deliver Antigen to Human Dendritic Cells via DC-SIGN In Vitro. J. Control. Release 144 (2), 118–126. doi:10.1016/j.jconrel.2010.02.013
Dai, L. L., Li, K., Li, M. H., Zhao, X. J., Luo, Z., Lu, L., et al. (2018). Size/charge Changeable Acidity-Responsive Micelleplex for Photodynamic-Improved PD-L Immunotherapy with Enhanced Tumor Penetration. Adv. Funct. Mater 28, 1707249. doi:10.1002/adfm.201707249
Daldrup-Link, H. E., Golovko, D., Ruffell, B., DeNardo, D. G., Castaneda, R., Ansari, C., et al. (2011). MRI of Tumor-Associated Macrophages with Clinically Applicable Iron Oxide Nanoparticles. Clin. Cancer Res. 17 (17), 5695–5704. doi:10.1158/1078-0432.ccr-10-3420
Demaria, S., Kawashima, N., Yang, A. M., Devitt, M. L., Babb, J. S., Allison, J. P., et al. (2005). Immune-mediated Inhibition of Metastases after Treatment with Local Radiation and CTLA-4 Blockade in a Mouse Model of Breast Cancer. Clin. Cancer Res. 11 (2 Pt 1), 728–734.
Di Trolio, R., Simeone, E., Di Lorenzo, G., Buonerba, C., and Ascierto, P. A. (2015). The Use of Interferon in Melanoma Patients: a Systematic Review. Cytokine Growth Factor Rev. 26 (2), 203–212. doi:10.1016/j.cytogfr.2014.11.008
Elsabahy, M., and Wooley, K. L. (2012). Design of Polymeric Nanoparticles for Biomedical Delivery Applications. Chem. Soc. Rev. 41 (7), 2545–2561. doi:10.1039/c2cs15327k
Eng, J. W., Reed, C. B., Kokolus, K. M., Pitoniak, R., Utley, A., Bucsek, M. J., et al. (2015). Housing Temperature-Induced Stress Drives Therapeutic Resistance in Murine Tumour Models through β2-adrenergic Receptor Activation. Nat. Commun. 6, 6426. doi:10.1038/ncomms7426
Evans, E. R., Bugga, P., Asthana, V., and Drezek, R. (2018). Metallic Nanoparticles for Cancer Immunotherapy. Mater. Today 21 (6), 673–685. doi:10.1016/j.mattod.2017.11.022
Fan, Y., and Moon, J. J. (2015). Nanoparticle Drug Delivery Systems Designed to Improve Cancer Vaccines and Immunotherapy. Vaccines 3 (3), 662–685. doi:10.3390/vaccines3030662
Farkona, S., Diamandis, E. P., and Blasutig, I. M. (2016). Cancer Immunotherapy: the Beginning of the End of Cancer? BMC Med. 14, 73. doi:10.1186/s12916-016-0623-5
Fay, F., and Scott, C. J. (2011). Antibody-targeted Nanoparticles for Cancer Therapy. Immunotherapy 3 (3), 381–394. doi:10.2217/imt.11.5
Fenoglio, D., Traverso, P., Parodi, A., Kalli, F., Zanetti, M., and Filaci, G. (2013). Generation of More Effective Cancer Vaccines. Hum. vaccines Immunother. 9 (12), 2543–2547. doi:10.1002/cncr.2442910.4161/hv.26147
Freeley, M., and Long, A. (2013). Advances in siRNA Delivery to T-Cells: Potential Clinical Applications for Inflammatory Disease, Cancer and Infection. Biochem. J. 455 (2), 133–147. doi:10.1042/BJ20130950
Gao, W., Fang, R. H., Thamphiwatana, S., Luk, B. T., Li, J., Angsantikul, P., et al. (2015). Modulating Antibacterial Immunity via Bacterial Membrane-Coated Nanoparticles. Nano Lett. 15 (2), 1403–1409. doi:10.1021/nl504798g
Gao, Y., Gao, D., Shen, J., and Wang, Q. (2020). A Review of Mesoporous Silica Nanoparticle Delivery Systems in Chemo-Based Combination Cancer Therapies. Front. Chem. 8, 598722. doi:10.3389/fchem.2020.598722
Ghafouri-Fard, S., and Ghafouri-Fard, S. (2012). siRNA and Cancer Immunotherapy. Immunotherapy 4 (9), 907–917. doi:10.2217/imt.12.87
Ghotbi, Z. (2011). PLGA-Based Nanoparticles in Cancer Immunotherapy and Immunomonitoring: A Versatile Vehicle for Targeting of Dendritic Cells, Master of Science Thesis. Edmonton: University of Alberta. Available at: https://era.library.ualberta.ca/items/0e3b489f-de97-49a4-bc45-bc09684ed568.
Goldberg, M. S. (2015). Immunoengineering: How Nanotechnology Can Enhance Cancer Immunotherapy. Cell 161 (2), 201–204. doi:10.1016/j.cell.2015.03.037
Gong, N., Sheppard, N. C., Billingsley, M. M., June, C. H., and Mitchell, M. J. (2021). Nanomaterials for T-Cell Cancer Immunotherapy. Nat. Nanotechnol. 16 (1), 25–36. doi:10.1038/s41565-020-00822-y
Griesmann, H., Drexel, C., Milosevic, N., Sipos, B., Rosendahl, J., Gress, T. M., et al. (2017). Pharmacological Macrophage Inhibition Decreases Metastasis Formation in a Genetic Model of Pancreatic Cancer. Gut 66 (7), 1278–1285. doi:10.1136/gutjnl-2015-310049
Gross, B. P., Wongrakpanich, A., Francis, M. B., Salem, A. K., and Norian, L. A. (2014). A Therapeutic Microparticle-Based Tumor Lysate Vaccine Reduces Spontaneous Metastases in Murine Breast Cancer. AAPS J. 16 (6), 1194–1203. doi:10.1208/s12248-014-9662-z
Guo, Q., Jin, Z., Yuan, Y., Liu, R., Xu, T., Wei, H., et al. (2016). New Mechanisms of Tumor-Associated Macrophages on Promoting Tumor Progression: Recent Research Advances and Potential Targets for Tumor Immunotherapy. J. Immunol. Res. 2016, 9720912. doi:10.1155/2016/9720912
Guo, X., and Huang, L. (2012). Recent Advances in Nonviral Vectors for Gene Delivery. Accounts Chem. Res. 45 (7), 971–979. doi:10.1021/ar200151m
György, B., Szabó, T. G., Pásztói, M., Pál, Z., Misják, P., Aradi, B., et al. (2011). Membrane Vesicles, Current State-Of-The-Art: Emerging Role of Extracellular Vesicles. Cell. Mol. life Sci. 68 (16), 2667–2688. doi:10.1007/s00018-011-0689-3
Hadla, M., Palazzolo, S., Corona, G., Caligiuri, I., Canzonieri, V., Toffoli, G., et al. (2016). Exosomes Increase the Therapeutic Index of Doxorubicin in Breast and Ovarian Cancer Mouse Models. Nanomedicine Lond. Engl. 11 (18), 2431–2441. doi:10.2217/nnm-2016-0154
Hajj, K., and Whitehead, K. (2017). Tools for Translation: Non-viral Materials for Therapeutic mRNA Delivery. Nat. Rev. Mater 2, 17056. doi:10.1038/natrevmats.2017.56
Hassan, H., Smyth, L., Wang, J. T. W., Costa, P. M., Ratnasothy, K., Diebold, S. S., et al. (2016). Dual Stimulation of Antigen Presenting Cells Using Carbon Nanotube-Based Vaccine Delivery System for Cancer Immunotherapy. Biomaterials 104, 310–322. doi:10.1016/j.biomaterials.2016.07.005
Heo, M. B., Cho, M. Y., and Lim, Y. T. (2014). Polymer Nanoparticles for Enhanced Immune Response: Combined Delivery of Tumor Antigen and Small Interference RNA for Immunosuppressive Gene to Dendritic Cells. Acta biomater. 10 (5), 2169–2176. doi:10.1016/j.actbio.2013.12.050
Hirata, E., and Sahai, E. (2017). Tumor Microenvironment and Differential Responses to Therapy. Cold Spring Harb. Perspect. Med. 7 (7), a026781. doi:10.1101/cshperspect.a026781
Hoang, M. D., Lee, H. J., Lee, H. J., Jung, S. H., Choi, N. R., Vo, M. C., et al. (2015). Branched Polyethylenimine-Superparamagnetic Iron Oxide Nanoparticles (bPEI-SPIONs) Improve the Immunogenicity of Tumor Antigens and Enhance Th1 Polarization of Dendritic Cells. J. Immunol. Res. 2015, 706379. doi:10.1155/2015/706379
Hu, W., Wang, G., Huang, D., Sui, M., and Xu, Y. (2019). Cancer Immunotherapy Based on Natural Killer Cells: Current Progress and New Opportunities. Front. Immunol. 10, 1205. doi:10.3389/fimmu.2019.01205
Huang, B., Abraham, W. D., Zheng, Y., Bustamante López, S. C., Luo, S. S., and Irvine, D. J. (2015). Active Targeting of Chemotherapy to Disseminated Tumors Using Nanoparticle-Carrying T Cells. Sci. Transl. Med. 7 (291), 291ra94. doi:10.1126/scitranslmed.aaa5447
Huang, Y., Chen, Y., Zhou, S., Chen, L., Wang, J., Pei, Y., et al. (2020). Dual-mechanism Based CTLs Infiltration Enhancement Initiated by Nano-Sapper Potentiates Immunotherapy against Immune-Excluded Tumors. Nat. Commun. 11 (1), 622. doi:10.1038/s41467-020-14425-7
Huo, M., Zhao, Y., Satterlee, A. B., Wang, Y., Xu, Y., and Huang, L. (2017). Tumor-targeted Delivery of Sunitinib Base Enhances Vaccine Therapy for Advanced Melanoma by Remodeling the Tumor Microenvironment. J. Control. Release 245, 81–94. doi:10.1016/j.jconrel.2016.11.013
Ibarguren, M., López, D. J., and Escribá, P. V. (2014). The Effect of Natural and Synthetic Fatty Acids on Membrane Structure, Microdomain Organization, Cellular Functions and Human Health. Biochimica Biophys. Acta 1838 (6), 1518–1528. doi:10.1016/j.bbamem.2013.12.021
Intra, J., and Salem, A. K. (2008). Characterization of the Transgene Expression Generated by Branched and Linear Polyethylenimine-Plasmid DNA Nanoparticles In Vitro and after Intraperitoneal Injection In Vivo. J. Control. Release 130 (2), 129–138. doi:10.1016/j.jconrel.2008.04.014
Iyer, A. K., Duan, Z., and Amiji, M. M. (2014). Nanodelivery Systems for Nucleic Acid Therapeutics in Drug Resistant Tumors. Mol. Pharm. 11 (8), 2511–2526. doi:10.1021/mp500024p
Jang, E. S., Shin, J. H., Ren, G., Park, M. J., Cheng, K., Chen, X., et al. (2012). The Manipulation of Natural Killer Cells to Target Tumor Sites Using Magnetic Nanoparticles. Biomaterials 33 (22), 5584–5592. doi:10.1016/j.biomaterials.2012.04.041
Ji, Z., Lin, G., Lu, Q., Meng, L., Shen, X., Dong, L., et al. (2012). Targeted Therapy of SMMC-7721 Liver Cancer In Vitro and In Vivo with Carbon Nanotubes Based Drug Delivery System. J. colloid interface Sci. 365 (1), 143–149. doi:10.1016/j.jcis.2011.09.013
Jones, M. C., and Leroux, J. C. (1999). Polymeric Micelles - a New Generation of Colloidal Drug Carriers. Eur. J. Pharm. Biopharm. 48 (2), 101–111. doi:10.1016/s0939-6411(99)00039-9
Jones, R. B., Mueller, S., Kumari, S., Vrbanac, V., Genel, S., Tager, A. M., et al. (2017). Antigen Recognition-Triggered Drug Delivery Mediated by Nanocapsule-Functionalized Cytotoxic T-Cells. Biomaterials 117, 44–53. doi:10.1016/j.biomaterials.2016.11.048
June, C. H., Riddell, S. R., and Schumacher, T. N. (2015). Adoptive Cellular Therapy: a Race to the Finish Line. Sci. Transl. Med. 7 (280), 280ps7. doi:10.1126/scitranslmed.aaa3643
Kaczmarek, J. C., Kowalski, P. S., and Anderson, D. G. (2017). Advances in the Delivery of RNA Therapeutics: from Concept to Clinical Reality. Genome Med. 9, 60. doi:10.1186/s13073-017-0450-0
Kang, T., Huang, Y., Zhu, Q., Cheng, H., Pei, Y., Feng, J., et al. (2018). Necroptotic Cancer Cells-Mimicry Nanovaccine Boosts Anti-tumor Immunity with Tailored Immune-Stimulatory Modality. Biomaterials 164, 80–97. doi:10.1016/j.biomaterials.2018.02.033
Kaufman, H. L., Kohlhapp, F. J., and Zloza, A. (2015). Oncolytic Viruses: a New Class of Immunotherapy Drugs. Nat. Rev. Drug Discov. 14 (9), 642–662. doi:10.1038/nrd4663
Keenan, B. P., and Jaffee, E. M. (2012). Whole Cell Vaccines-Ppast Progress and Future Strategies. Seminars Oncol. 39 (3), 276–286. doi:10.1053/j.seminoncol.2012.02.007
Kokate, R. (2017). A Systematic Overview of Cancer Immunotherapy: an Emerging Therapy. Pharm. Pharmacol. Int. J. 5 (2), 31–35. doi:10.15406/ppij.2017.05.00112
Kong, M., Tang, J., Qiao, Q., Wu, T., Qi, Y., Tan, S., et al. (2017). Biodegradable Hollow Mesoporous Silica Nanoparticles for Regulating Tumor Microenvironment and Enhancing Antitumor Efficiency. Theranostics 7 (13), 3276–3292. doi:10.7150/thno.19987
Koshy, S. T., Cheung, A. S., Gu, L., Graveline, A. R., and Mooney, D. J. (2017). Liposomal Delivery Enhances Immune Activation by STING Agonists for Cancer Immunotherapy. Adv. Biosyst. 1 (1–2), 1600013. doi:10.1002/adbi.201600013
Kostarelos, K. (2008). The Long and Short of Carbon Nanotube Toxicity. Nat. Biotechnol. 26 (7), 774–776. doi:10.1038/nbt0708-774
Kourani, K., Jain, P., Kumar, A., Jangid, A. K., Swaminathan, G., Durgempudi, V. R., et al. (2022). Inulin Coated MN3O4 Nanocuboids Coupled with RNA Interference Reverse Intestinal Tumorigenesis in APC Knockout Murine Colon Cancer Models. Nanomedicine Nanotechnol. Biol. Med. 40, 102504. doi:10.1016/j.nano.2021.102504
Koyama, Y., Ito, T., Hasegawa, A., Eriguchi, M., Inaba, T., Ushigusa, T., et al. (2016). Exosomes Derived from Tumor Cells Genetically Modified to Express Mycobacterium tuberculosis Antigen: A Novel Vaccine for Cancer Therapy. Biotechnol. Lett. 38 (11), 1857–1866. doi:10.1007/s10529-016-2185-1
Kranz, L. M., Diken, M., Haas, H., Kreiter, S., Loquai, C., Reuter, K. C., et al. (2016). Systemic RNA Delivery to Dendritic Cells Exploits Antiviral Defence for Cancer Immunotherapy. Nature 534 (7607), 396–401. doi:10.1038/nature18300
Krishnamachari, Y., Geary, S. M., Lemke, C. D., and Salem, A. K. (2011). Nanoparticle Delivery Systems in Cancer Vaccines. Pharm. Res. 28 (2), 215–236. doi:10.1007/s11095-010-0241-4
Kuai, R., Ochyl, L. J., Bahjat, K. S., Schwendeman, A., and Moon, J. J. (2017). Designer Vaccine Nanodiscs for Personalized Cancer Immunotherapy. Nat. Mater. 16 (4), 489–496. doi:10.1038/nmat4822
Kumar, A., Singam, A., Swaminathan, G., Killi, N., Tangudu, N. K., Jose, J., et al. (2022). Combinatorial Therapy Using RNAi and Curcumin Nano-Architectures Regresses Tumors in Breast and Colon Cancer Models. Nanoscale 14 (2), 492–505. doi:10.1039/d1nr04411g
Kumar, S., Kesharwani, S. S., Kuppast, B., Bakkari, M. A., and Tummala, H. (2017). Pathogen-Mimicking Vaccine Delivery System Designed with a Bioactive Polymer (Inulin Acetate) for Robust Humoral and Cellular Immune Responses. J. Control. Release 261, 263–274. doi:10.1016/j.jconrel.2017.06.026
Le, Q. V., Yang, G., Wu, Y., Jang, H. W., Shokouhimehr, M., and Oh, Y. K. (2019). Nanomaterials for Modulating Innate Immune Cells in Cancer Immunotherapy. Asian J. Pharm. Sci. 14 (1), 16–29. doi:10.1016/j.ajps.2018.07.003
Li, J., Li, X., Wang, Q., Hu, S., Wang, Y., Masoudi, F. A., et al. (2015). ST-segment Elevation Myocardial Infarction in China from 2001 to 2011 (The China PEACE-Retrospective Acute Myocardial Infarction Study): A Retrospective Analysis of Hospital Data. Lancet 385 (9966), 441–451. doi:10.1016/S0140-6736(14)60921-1
Li, R., Fang, F., Jiang, M., Wang, C., Ma, J., Kang, W., et al. (2017). STAT3 and NF-κB are Simultaneously Suppressed in Dendritic Cells in Lung Cancer. Sci. Rep. 7, 45395. doi:10.1038/srep45395
Li, S., Wang, Q., Shen, Y., Hassan, M., Shen, J., Jiang, W., et al. (2020). Pseudoneutrophil Cytokine Sponges Disrupt Myeloid Expansion and Tumor Trafficking to Improve Cancer Immunotherapy. Nano Lett. 20 (1), 242–251. doi:10.1021/acs.nanolett.9b03753
Li, S. Y., Liu, Y., Xu, C. F., Shen, S., Sun, R., Du, X. J., et al. (2016). Restoring Anti-tumor Functions of T Cells via Nanoparticle-Mediated Immune Checkpoint Modulation. J. Control. Release 231, 17–28. doi:10.1016/j.jconrel.2016.01.044
Li, W., Joshi, M. D., Singhania, S., Ramsey, K. H., and Murthy, A. K. (2014). Peptide Vaccine: Progress and Challenges. Vaccines 2 (3), 515–536. doi:10.3390/vaccines2030515
Li, X., Liang, Q., Zhang, W., Li, Y., Ye, J., Zhao, F., et al. (2017). Bio-inspired Bioactive Glasses for Efficient microRNA and Drug Delivery. J. Mater. Chem. B 5 (31), 6376–6384. doi:10.1039/c7tb01021d
Li, Z., and Rana, T. M. (2014). Therapeutic Targeting of microRNAs: Current Status and Future Challenges. Nat. Rev. Drug Discov. 13 (8), 622–638. doi:10.1038/nrd4359
Lim, E. K., Kim, T., Paik, S., Haam, S., Huh, Y. M., and Lee, K. (2015). Nanomaterials for Theranostics: Recent Advances and Future Challenges. Chem. Rev. 115 (1), 327–394. doi:10.1021/cr300213b
Lin, Y. X., Gao, Y. J., Wang, Y., Qiao, Z. Y., Fan, G., Qiao, S. L., et al. (2015). pH-Sensitive Polymeric Nanoparticles with Gold(I) Compound Payloads Synergistically Induce Cancer Cell Death through Modulation of Autophagy. Mol. Pharm. 12 (8), 2869–2878. doi:10.1021/acs.molpharmaceut.5b00060
Lin, Y. X., Wang, Y., Qiao, S. L., An, H. W., Zhang, R. X., Qiao, Z. Y., et al. (2016). pH-Sensitive Polymeric Nanoparticles Modulate Autophagic Effect via Lysosome Impairment. Small 12 (21), 2921–2931. doi:10.1002/smll.201503709
Linsley, P. S., Brady, W., Urnes, M., Grosmaire, L. S., Damle, N. K., and Ledbetter, J. A. (1991). CTLA-4 is a Second Receptor for the B Cell Activation Antigen B7. J. Exp. Med. 174 (3), 561–569. doi:10.1084/jem.174.3.561
Liu, C., Zhang, L., Liu, H., and Cheng, K. (2017). Delivery Strategies of the CRISPR-Cas9 Gene-Editing System for Therapeutic Applications. J. Control Release 266, 17–26. doi:10.1016/j.jconrel.2017.09.012
Liu, F., Sun, X., Fairman, J., Lewis, D. B., Katz, J. M., Levine, M., et al. (2016). A Cationic Liposome–DNA Complexes Adjuvant (JVRS-100) Enhances the Immunogenicity and Cross-Protective Efficacy of Pre-pandemic Influenza A (H5N1) Vaccine in Ferrets. Virology 492, 197–203. doi:10.1016/j.virol.2016.02.024
Liu, H., Mai, J., Shen, J., Wolfram, J., Li, Z., Zhang, G., et al. (2018). A Novel DNA Aptamer for Dual Targeting of Polymorphonuclear Myeloid-Derived Suppressor Cells and Tumor Cells. Theranostics 8 (1), 31–44. doi:10.7150/thno.21342
Liu, Q., Zhu, H., Liu, Y., Musetti, S., and Huang, L. (2018). BRAF Peptide Vaccine Facilitates Therapy of Murine BRAF-Mutant Melanoma. Cancer Immunol. Immunother. 67 (2), 299–310. doi:10.1007/s00262-017-2079-7
Loh, X. J., Lee, T. C., Dou, Q., and Deen, G. R. (2016). Utilising Inorganic Nanocarriers for Gene Delivery. Biomaterials Sci. 4 (1), 70–86. doi:10.1039/c5bm00277j
Luo, L., Iqbal, M. Z., Liu, C., Xing, J., Akakuru, O. U., Fang, Q., et al. (2019). Engineered Nano-Immunopotentiators Efficiently Promote Cancer Immunotherapy for Inhibiting and Preventing Lung Metastasis of Melanoma. Biomaterials 223, 119464. doi:10.1016/j.biomaterials.2019.119464
Luo, M., Wang, H., Wang, Z., Cai, H., Lu, Z., Li, Y., et al. (2017). A STING-Activating Nanovaccine for Cancer Immunotherapy. Nat. Nanotechnol. 12 (7), 648–654. doi:10.1038/nnano.2017.52
Lynch, T. J., Bondarenko, I., Luft, A., Serwatowski, P., Barlesi, F., Chacko, R., et al. (2012). Ipilimumab in Combination with Paclitaxel and Carboplatin as First-Line Treatment in Stage IIIB/IV Non-Small-Cell Lung Cancer: Results from a Randomized, Double-Blind, Multicenter Phase II Study. J. Clin. Oncol. 30 (17), 2046–2054. doi:10.1200/JCO.2011.38.4032
Mardiana, S., Solomon, B. J., Darcy, P. K., and Beavis, P. A. (2019). Supercharging Adoptive T Cell Therapy to Overcome Solid Tumor-Induced Immunosuppression. Sci. Transl. Med. 11 (495), eaaw2293. doi:10.1126/scitranslmed.aaw2293
Maroun, J., Munoz-Alia, M., Ammayappan, A., Schulze, A., Peng, K. W., and Russell, S. (2017). Designing and Building Oncolytic Viruses. Fut. Virol. 12 (4), 193–213. doi:10.2217/fvl-2016-0129
Martin‐Liberal, J., Ochoa de Olza, M., Hierro, C., Gros, A., Rodon, J., and Tabernero, J. (2017). The Expanding Role of Immunotherapy. Cancer Treat. Rev. 54, 74–86. doi:10.1016/j.ctrv.2017.01.008
Martín-Saldaña, S., Palao-Suay, R., Aguilar, M. R., Ramírez-Camacho, R., and San Román, J. (2017). Polymeric Nanoparticles Loaded with Dexamethasone or α-tocopheryl Succinate to Prevent Cisplatin-Induced Ototoxicity. Acta biomater. 53, 199–210. doi:10.1016/j.actbio.2017.02.019
Marvel, D., and Gabrilovich, D. I. (2015). Myeloid-Derived Suppressor Cells in the Tumor Microenvironment: Expect the Unexpected. J. Clin. Invest. 125 (9), 3356–3364. doi:10.1172/jci80005
Masood, F. (2016). Polymeric Nanoparticles for Targeted Drug Delivery System for Cancer Therapy. Mater. Sci. Eng. Mater. Biol. Appl. 60, 569–578. doi:10.1016/j.msec.2015.11.067
Matsuda, A., Ishiguro, K., Yan, I. K., and Patel, T. (2019). Extracellular Vesicle-Based Therapeutic Targeting of β-Catenin to Modulate Anticancer Immune Responses in Hepatocellular Cancer. Hepatol. Commun. 3 (4), 525–541. doi:10.1002/hep4.1311
McCarthy, E. F. (2006). The Toxins of William B. Coley and the Treatment of Bone and Soft-Tissue Sarcomas. Iowa Orthop. J. 26, 154.
McCune, J. S. (2018). Rapid Advances in Immunotherapy to Treat Cancer. Clin. Pharmacol. Ther. 103 (4), 540–544. doi:10.1002/cpt.985
Mehata, A. K., Viswanadh, M. K., Priya, V., Vikas, , and Muthu, M. S. (2020). Dendritic Cell-Targeted Theranostic Nanomedicine: Advanced Cancer Nanotechnology for Diagnosis and Therapy. Nanomedicine 15 (10), 947–949. doi:10.2217/nnm-2020-0032
Mellman, I., Coukos, G., and Dranoff, G. (2011). Cancer Immunotherapy Comes of Age. Nature 480 (7378), 480–489. doi:10.1038/nature10673
Mi, Y., Hagan, C. T., 4thVincent, B. G., and Wang, A. Z. (2019). Emerging Nano-/Microapproaches for Cancer Immunotherapy. Adv. Sci. 6 (6), 1801847. doi:10.1002/advs.201801847
Miller, M. A., Chandra, R., Cuccarese, M. F., Pfirschke, C., Engblom, C., Stapleton, S., et al. (2017). Radiation Therapy Primes Tumors for Nanotherapeutic Delivery via Macrophage-Mediated Vascular Bursts. Sci. Transl. Med. 9 (392), eaal0225. doi:10.1126/scitranslmed.aal0225
Min, Y., Caster, J. M., Eblan, M. J., and Wang, A. Z. (2015). Clinical Translation of Nanomedicine. Chem. Rev. 115 (19), 11147–11190. doi:10.1021/acs.chemrev.5b00116
Mirzaei, H., Masoudifar, A., Sahebkar, A., Zare, N., Sadri Nahand, J., Rashidi, B., et al. (2018). MicroRNA: A Novel Target of Curcumin in Cancer Therapy. J. Cell. Physiol. 233 (4), 3004–3015. doi:10.1002/jcp.26055
Mitchison, N. A. (1955). Studies on the Immunological Response to Foreign Tumor Transplants in the Mouse : I. The Role of Lymph Node Cells in Conferring Immunity by Adoptive Transfer. J. Exp. Med. 102 (2), 157–177. doi:10.1084/jem.102.2.157
Mukherjee, P., Bhattacharya, R., Bone, N., Lee, Y. K., Patra, C. R., Wang, S., et al. (2007). Potential Therapeutic Application of Gold Nanoparticles in B-Chronic Lymphocytic Leukemia (BCLL): Enhancing Apoptosis. J. Nanobiotechnol. 5, 4. doi:10.1186/1477-3155-5-4
Munn, D. H., and Bronte, V. (2016). Immune Suppressive Mechanisms in the Tumor Microenvironment. Curr. Opin. Immunol. 39, 1–6. doi:10.1016/j.coi.2015.10.009
Mura, S., Nicolas, J., and Couvreur, P. (2013). Stimuli-responsive Nanocarriers for Drug Delivery. Nat. Mater. 12 (11), 991–1003. doi:10.1038/nmat3776
Ngo Trong, H., Le Van Manh, H., Thanh Dang, V., Ho-Thao Nguyen, N., Vu Thanh, B., and Pham, P. (2020). Current Strategies for Adoptive Immunotherapy for Cancer: “Off-The-Shelf” Immune Cells. Biomed. Res. Ther. 7 (12), 4170–4189. doi:10.15419/bmrat.v7i12.655
Nwanegbo, E., Vardas, E., Gao, W., Whittle, H., Sun, H., Rowe, D., et al. (2004). Prevalence of Neutralizing Antibodies to Adenoviral Serotypes 5 and 35 in the Adult Populations of the Gambia, South Africa, and the United States. Clin. Diagn. Lab. Immunol. 11 (2), 351–357. doi:10.1128/CDLI.11.2.351-357.2004
O'Leary, M. C., Lu, X., Huang, Y., Lin, X., Mahmood, I., Przepiorka, D., et al. (2019). FDA Approval Summary: Tisagenlecleucel for Treatment of Patients with Relapsed or Refractory B-Cell Precursor Acute Lymphoblastic Leukemia. Clin. Cancer Res. 25 (4), 1142–1146. doi:10.1158/1078-0432.CCR-18-2035
Oh, S., Lee, J-H., Kwack, K., and Choi, S-W. (2019). Natural Killer Cell Therapy: A New Treatment Paradigm for Solid Tumors. Cancers 11 (10), 1534. doi:10.3390/cancers11101534
Oiseth, S. J., and Aziz, M. S. (2017). Cancer Immunotherapy: a Brief Review of the History, Possibilities, and Challenges Ahead. J. cancer metastasis Treat. 3, 250–261. doi:10.20517/2394-4722.2017.41
Oyer, J. L., Igarashi, R. Y., Kulikowski, A. R., Colosimo, D. A., Solh, M. M., Zakari, A., et al. (2015). Generation of Highly Cytotoxic Natural Killer Cells for Treatment of Acute Myelogenous Leukemia Using a Feeder-free, Particle-Based Approach. Biol. Blood Marrow Transplant. 21 (4), 632–639. doi:10.1016/j.bbmt.2014.12.037
Palucka, K., and Banchereau, J. (2012). Cancer Immunotherapy via Dendritic Cells. Nat. Rev. Cancer 12 (4), 265–277. doi:10.1038/nrc3258
Pardoll, D. M., and Topalian, S. L. (1998). The Role of CD4+ T Cell Responses in Antitumor Immunity. Curr. Opin. Immunol. 10 (5), 588–594. doi:10.1016/s0952-7915(98)80228-8
Park, Y. M., Lee, S. J., Kim, Y. S., Lee, M. H., Cha, G. S., Jung, I. D., et al. (2013). Nanoparticle-based Vaccine Delivery for Cancer Immunotherapy. Immune Netw. 13 (5), 177–183. doi:10.4110/in.2013.13.5.177
Pastor, F., Berraondo, P., Etxeberria, I., Frederick, J., Sahin, U., Gilboa, E., et al. (2018). An RNA Toolbox for Cancer Immunotherapy. Nat. Rev. Discov. 17 (10), 751–767. doi:10.1038/nrd.2018.132
Patel, A., and Sant, S. (2016). Hypoxic Tumor Microenvironment: Opportunities to Develop Targeted Therapies. Biotechnol. Adv. 34 (5), 803–812. doi:10.1016/j.biotechadv.2016.04.005
Patra, J. K., Das, G., Fraceto, L. F., Campos, E., Rodriguez-Torres, M., Acosta-Torres, L. S., et al. (2018). Nano Based Drug Delivery Systems: Recent Developments and Future Prospects. J. Nanobiotechnol. 16 (1), 71. doi:10.1186/s12951-018-0392-8
Pearce, M. E., Mai, H. Q., Lee, N., Larsen, S. C., and Salem, A. K. (2008). Silicalite Nanoparticles that Promote Transgene Expression. Nanotechnology 19 (17), 175103. doi:10.1088/0957-4484/19/17/175103
Pearce, M. E., Melanko, J. B., and Salem, A. K. (2007). Multifunctional Nanorods for Biomedical Applications. Pharm. Res. 24 (12), 2335–2352. doi:10.1007/s11095-007-9380-7
Pecot, C. V., Calin, G. A., Coleman, R. L., Lopez-Berestein, G., and Sood, A. K. (2011). RNA Interference in the Clinic: Challenges and Future Directions. Nat. Rev. Cancer 11 (1), 59–67. doi:10.1038/nrc2966
Perica, K., Bieler, J. G., Schütz, C., Varela, J. C., Douglass, J., Skora, A., et al. (2015). Enrichment and Expansion with Nanoscale Artificial Antigen Presenting Cells for Adoptive Immunotherapy. ACS Nano 9 (7), 6861–6871. doi:10.1021/acsnano.5b02829
Phan, G. Q., Yang, J. C., Sherry, R. M., Hwu, P., Topalian, S. L., Schwartzentruber, D. J., et al. (2003). Cancer Regression and Autoimmunity Induced by Cytotoxic T Lymphocyte-Associated Antigen 4 Blockade in Patients with Metastatic Melanoma. Proc. Natl. Acad. Sci. U. S. A. 100 (14), 8372–8377. doi:10.1073/pnas.1533209100
Postow, M. A., Callahan, M. K., and Wolchok, J. D. (2015). Immune Checkpoint Blockade in Cancer Therapy. J. Clin. Oncol. 33 (17), 1974–1982. doi:10.1200/JCO.2014.59.4358
Qian, Y., Qiao, S., Dai, Y., Xu, G., Dai, B., Lu, L., et al. (2017). Molecular-Targeted Immunotherapeutic Strategy for Melanoma via Dual-Targeting Nanoparticles Delivering Small Interfering RNA to Tumor-Associated Macrophages. ACS Nano 11 (9), 9536–9549. doi:10.1021/acsnano.7b05465
Qiao, C., Yang, J., Shen, Q., Liu, R., Li, Y., Shi, Y., et al. (2018). Traceable Nanoparticles with Dual Targeting and ROS Response for RNAi-Based Immunochemotherapy of Intracranial Glioblastoma Treatment. Adv. Mater. 30 (18), e1705054. doi:10.1002/adma.201705054
Quail, D. F., and Joyce, J. A. (2013). Microenvironmental Regulation of Tumor Progression and Metastasis. Nat. Med. 19 (11), 1423–1437. doi:10.1038/nm.3394
Rahimian, S., Fransen, M. F., Kleinovink, J. W., Amidi, M., Ossendorp, F., and Hennink, W. E. (2015). Polymeric Microparticles for Sustained and Local Delivery of antiCD40 and antiCTLA-4 in Immunotherapy of Cancer. Biomaterials 61, 33–40. doi:10.1016/j.biomaterials.2015.04.043
Ramalingam, V., Rajaram, R., Premkumar, C., Santhanam, P., Dhinesh, P., Vinothkumar, S., et al. (2014). Biosynthesis of Silver Nanoparticles from Deep Sea Bacterium Pseudomonas aeruginosa JQ989348 for Antimicrobial, Antibiofilm, and Cytotoxic Activity. J. Basic Microbiol. 54, 928–936. doi:10.1002/jobm.201300514
Rameshbabu, S., Labadie, B. W., Argulian, A., and Patnaik, A. (2021). Targeting Innate Immunity in Cancer Therapy. Vaccines 9 (2), 138. doi:10.3390/vaccines9020138
Robinson, T. O., and Schluns, K. S. (2017). The Potential and Promise of IL-15 in Immuno-Oncogenic Therapies. Immunol. Lett. 190, 159–168. doi:10.1016/j.imlet.2017.08.010
Rohaan, M. W., Wilgenhof, S., and Haanen, J. B. (2019). Adoptive Cellular Therapies: the Current Landscape. Virchows Arch. 474 (4), 449–461. doi:10.1007/s00428-018-2484-0
Rosenberg, S. A., and Restifo, N. P. (2015). Adoptive Cell Transfer as Personalized Immunotherapy for Human Cancer. Science 348 (6230), 62–68. doi:10.1126/science.aaa4967
Rosenberg, S. A., Restifo, N. P., Yang, J. C., Morgan, R. A., and Dudley, M. E. (2008). Adoptive Cell Transfer: a Clinical Path to Effective Cancer Immunotherapy. Nat. Rev. Cancer 8 (4), 299–308. doi:10.1038/nrc2355
Rosenberg, S. A., Yang, J. C., Sherry, R. M., Kammula, U. S., Hughes, M. S., Phan, G. Q., et al. (2011). Durable Complete Responses in Heavily Pretreated Patients with Metastatic Melanoma Using T-Cell Transfer Immunotherapy. Clin. Cancer Res. 17 (13), 4550–4557. doi:10.1158/1078-0432.CCR-11-0116
Rotte, A. (2019). Combination of CTLA-4 and PD-1 Blockers for Treatment of Cancer. Jurnal Exp. Clin. Cancer Res. 38 (1), 1–12. doi:10.1186/s13046-019-1259-z
Rupaimoole, R., and Slack, F. J. (2017). MicroRNA Therapeutics: towards a New Era for the Management of Cancer and Other Diseases. Nat. Rev. Drug Discov. 16 (3), 203–222. doi:10.1038/nrd.2016.246
Sakhalkar, H. S., Dalal, M. K., Salem, A. K., Ansari, R., Fu, J., Kiani, M. F., et al. (2003). Leukocyte-Inspired Biodegradable Particles that Selectively and Avidly Adhere to Inflamed Endothelium In Vitro and In Vivo. Proc. Natl. Acad. Sci. U. S. A. 100 (26), 15895–15900. doi:10.1073/pnas.2631433100
Salem, A. K., Searson, P. C., and Leong, K. W. (2003). Multifunctional Nanorods for Gene Delivery. Nat. Mater. 2 (10), 668–671. doi:10.1038/nmat974
Saxena, M., van der Burg, S. H., Melief, C. J., and Bhardwaj, N. (2021). Therapeutic Cancer Vaccines. Nat. Rev. Cancer 21 (6), 360–378. doi:10.1038/s41568-021-00346-0
Sayour, E. J., De Leon, G., Pham, C., Grippin, A., Kemeny, H., Chua, J., et al. (2016). Systemic Activation of Antigen-Presenting Cells via RNA-Loaded Nanoparticles. Oncoimmunology 6 (1), e1256527. doi:10.1080/2162402X.2016.1256527
Selvaraja, V. K., and Gudipudi, D. K. (2020). Fundamentals to Clinical Application of Nanoparticles in Cancer Immunotherapy and Radiotherapy. ecancermedicalscience 14. doi:10.3332/ecancer.2020.1095
Shastri, V. P. (2003). Non-degradable Biocompatible Polymers in Medicine: Past, Present and Future. Curr. Pharm. Biotechnol. 4 (5), 331–337. doi:10.2174/1389201033489694
She, Z., Zhang, T., Wang, X., Li, X., Song, Y., Cheng, X., et al. (2014). The Anticancer Efficacy of Pixantrone-Loaded Liposomes Decorated with Sialic Acid-Octadecylamine Conjugate. Biomaterials 35 (19), 5216–5225. doi:10.1016/j.biomaterials.2014.03.022
Shen, J., Zhang, W., Qi, R., Mao, Z. W., and Shen, H. (2018). Engineering Functional Inorganic-Organic Hybrid Systems: Advances in siRNA Therapeutics. Chem. Soc. Rev. 47 (6), 1969–1995. doi:10.1039/c7cs00479f
Sheykhhasan, M., Manoochehri, H., and Dama, P. (2022). Use of CAR T-Cell for Acute Lymphoblastic Leukemia (ALL) Treatment: A Review Study. Cancer Gene Ther. [Epub ahead of print]. doi:10.1038/s41417-021-00418-1
Shi, H., Qi, X., Ma, B., Cao, Y., Wang, L., Sun, L., et al. (2015). The Status, Limitation and Improvement of Adoptive Cellular Immunotherapy in Advanced Urologic Malignancies. Chin. J. Cancer Res. 27 (2), 128. doi:10.3978/j.issn.1000-9604.2014.12.15
Siegler, E. L., Kim, Y. J., Chen, X., Siriwon, N., Mac, J., Rohrs, J. A., et al. (2017). Combination Cancer Therapy Using Chimeric Antigen Receptor-Engineered Natural Killer Cells as Drug Carriers. Mol. Ther. 25 (12), 2607–2619. doi:10.1016/j.ymthe.2017.08.010
Sievers, E. L., and Senter, P. D. (2013). Antibody-drug Conjugates in Cancer Therapy. Annu. Rev. Med. 64, 15–29. doi:10.1146/annurev-med-050311-201823
Simberg, D., Weisman, S., Talmon, Y., and Barenholz, Y. (2004). DOTAP (And Other Cationic Lipids): Chemistry, Biophysics, and Transfection. Crit. Rev. Ther. Drug Carrier Syst. 21 (4), 257–317. doi:10.1615/critrevtherdrugcarriersyst.v21.i4.10
Siriwon, N., Kim, Y. J., Siegler, E., Chen, X., Rohrs, J. A., Liu, Y., et al. (2018). CAR-T Cells Surface-Engineered with Drug-Encapsulated Nanoparticles Can Ameliorate Intratumoral T-Cell Hypofunction. Cancer Immunol. Res. 6 (7), 812–824. doi:10.1158/2326-6066.CIR-17-0502
Smith, T. T., Stephan, S. B., Moffett, H. F., McKnight, L. E., Ji, W., Reiman, D., et al. (2017). In Situ programming of Leukaemia-specific T Cells Using Synthetic DNA Nanocarriers. Nat. Nanotechnol. 12 (8), 813–820. doi:10.1038/nnano.2017.57
Song, M., Liu, T., Shi, C., Zhang, X., and Chen, X. (2016). Bioconjugated Manganese Dioxide Nanoparticles Enhance Chemotherapy Response by Priming Tumor-Associated Macrophages toward M1-like Phenotype and Attenuating Tumor Hypoxia. ACS Nano 10 (1), 633–647. doi:10.1021/acsnano.5b06779
Sperling, R. A., and Parak, W. J. (2010). Surface Modification, Functionalization and Bioconjugation of Colloidal Inorganic Nanoparticles. Philos. Trans. A Math. Phys. Eng. Sci. 368, 1333–1383. doi:10.1098/rsta.2009.0273
Stephan, M. T., Moon, J. J., Um, S. H., Bershteyn, A., and Irvine, D. J. (2010). Therapeutic Cell Engineering with Surface-Conjugated Synthetic Nanoparticles. Nat. Med. 16 (9), 1035–1041. doi:10.1038/nm.2198
Sterner, R. C., and Sterner, R. M. (2021). CAR-T Cell Therapy: Current Limitations and Potential Strategies. Blood Cancer J. 11 (4), 1–11. doi:10.1038/s41408-021-00459-7
Sun, W. (2017). Recent Advances in Cancer Immunotherapy. J. Hematol. Oncol. 10, 96. doi:10.1186/s13045-017-0460-9
Suri, S. S., Fenniri, H., and Singh, B. (2007). Nanotechnology-based Drug Delivery Systems. J. Occup. Med. Toxicol. 2, 16. doi:10.1186/1745-6673-2-16
Swaminathan, G., Shigna, A., Kumar, A., Byroju, V. V., Durgempudi, V. R., and Dinesh Kumar, L. (2021). RNA Interference and Nanotechnology: A Promising Alliance for Next Generation Cancer Therapeutics. Front. Nanotechnol. 3, 42. doi:10.3389/fnano.2021.694838
Tanaka, A., and Sakaguchi, S. (2017). Regulatory T Cells in Cancer Immunotherapy. Cell Res. 27 (1), 109–118. doi:10.1038/cr.2016.151
Tang, L., Zheng, Y., Melo, M. B., Mabardi, L., Castaño, A. P., Xie, Y. Q., et al. (2018). Enhancing T Cell Therapy Through TCR-Signaling-Responsive Nanoparticle Drug Delivery. Nat. Biotechnol. 36 (8), 707–716. doi:10.1038/nbt.4181
Tangudu, N. K., Verma, V. K., Clemons, T. D., Beevi, S. S., Hay, T., Mahidhara, G., et al. (2015). RNA Interference Using c-Myc–Conjugated Nanoparticles Suppresses Breast and Colorectal Cancer Models. Mol. Cancer Ther. 14 (5), 1259–1269. doi:10.1158/1535-7163.MCT-14-0970
Thomas, L. (1959). Discussion, Cellular and Humoral Aspects of the Hypersensitive State. Editor H. S. Lawrence (John Wiley & Sons). doi:10.1002/path.2865
Timmerman, J. M., and Levy, R. (1999). Dendritic Cell Vaccines for Cancer Immunotherapy. Annu. Rev. Med. 50, 507–529. doi:10.1146/annurev.med.50.1.507
Tsai, K. K., Zarzoso, I., and Daud, A. I. (2014). PD-1 and PD-L1 Antibodies for Melanoma. Hum. vaccines Immunother. 10 (11), 3111–3116. doi:10.4161/21645515.2014.983409
Tsukahara, T., Kawaguchi, S., Torigoe, T., Asanuma, H., Nakazawa, E., Shimozawa, K., et al. (2006). Prognostic Significance of HLA Class I Expression in Osteosarcoma Defined by Anti-pan HLA Class I Monoclonal Antibody, EMR8-5. Cancer Sci. 97 (12), 1374–1380. doi:10.1111/j.1349-7006.2006.00317.x
US National Library of Medicine (2019). ClinicalTrials.gov. Available at: https://clinicaltrials.gov/ct2/show/NCT03815682.
Vijayan, V., Reddy, K. R., Sakthivel, S., and Swetha, C. (2013). Optimization and Charaterization of Repaglinide Biodegradable Polymeric Nanoparticle Loaded Transdermal Patchs: In Vitro and In Vivo Studies. Colloids Surf. B Biointerfaces 111, 150–155. doi:10.1016/j.colsurfb.2013.05.020
Villela Zumaya, A. L., Mincheva, R., Raquez, J. M., and Hassouna, F. (2022). Nanocluster-Based Drug Delivery and Theranostic Systems: Towards Cancer Therapy. Polymers 14 (6), 1188. doi:10.3390/polym14061188
Vinogradov, S. V., Bronich, T. K., and Kabanov, A. V. (2002). Nanosized Cationic Hydrogels for Drug Delivery: Preparation, Properties and Interactions with Cells. Adv. Drug Deliv. Rev. 54, 135–147. doi:10.1016/s0169-409x(01)00245-9
Vitale, C., and Strati, P. (2020). CAR T-Cell Therapy for B-Cell Non-hodgkin Lymphoma and Chronic Lymphocytic Leukemia: Clinical Trials and Real-World Experiences. Front. Oncol. 10, 849. doi:10.3389/fonc.2020.00849
Wallace, A., Kapoor, V., Sun, J., Mrass, P., Weninger, W., Heitjan, D. F., et al. (2008). Transforming Growth Factor-Beta Receptor Blockade Augments the Effectiveness of Adoptive T-Cell Therapy of Established Solid Cancers. Clin. Cancer Res. 14 (12), 3966–3974. doi:10.1158/1078-0432.CCR-08-0356
Wang, C., Sun, W., Ye, Y., Hu, Q., Bomba, H. N., and Gu, Z. (2017). In Situ activation of Platelets with Checkpoint Inhibitors for Post-surgical Cancer Immunotherapy. Nat. Biomed. Eng. 1 (2), 1–10. doi:10.1038/s41551-016-0011
Wang, C., Zhuang, Y., Zhang, Y., Luo, Z., Gao, N., Li, P., et al. (2012). Toll-like Receptor 3 Agonist Complexed with Cationic Liposome Augments Vaccine-Elicited Antitumor Immunity by Enhancing TLR3-IRF3 Signaling and Type I Interferons in Dendritic Cells. Vaccine 30 (32), 4790–4799. doi:10.1016/j.vaccine.2012.05.027
Wang, D. G., Wang, T. T., Liu, J. P., Yu, H. J., Jiao, S., Feng, B., et al. (2016). Acid-activatable Versatile Micelleplexes for PD-L1 Blockade-Enhanced Cancer Photodynamic Immunotherapy. Nano Lett. 16 (9), 5503–5513. doi:10.1021/acs.nanolett.6b01994
Wang, H. X., Li, M., Lee, C. M., Chakraborty, S., Kim, H. W., Bao, G., et al. (2017a). CRISPR/Cas9-Based Genome Editing for Disease Modeling and Therapy: Challenges and Opportunities for Nonviral Delivery. Chem. Rev. 117 (15), 9874–9906. doi:10.1021/acs.chemrev.6b00799
Wang, S., Sun, Z., and Hou, Y. (2021). Engineering Nanoparticles toward the Modulation of Emerging Cancer Immunotherapy. Adv. Healthc. Mater. 10 (5), e2000845. doi:10.1002/adhm.202000845
Wang, W., Singh, S., Zeng, D. L., King, K., and Nema, S. (2007). Antibody Structure, Instability, and Formulation. J. Pharm. Sci. 96 (1), 1–26. doi:10.1002/jps.20727
Wang, Y., Lin, Y. X., Qiao, S. L., An, H. W., Ma, Y., Qiao, Z. Y., et al. (2017b). Polymeric Nanoparticles Promote Macrophage Reversal from M2 to M1 Phenotypes in the Tumor Microenvironment. Biomaterials 112, 153–163. doi:10.1016/j.biomaterials.2016.09.034
Wang, Y., Lin, Y. X., Qiao, Z. Y., An, H. W., Qiao, S. L., Wang, L., et al. (2015). Self-assembled Autophagy-Inducing Polymeric Nanoparticles for Breast Cancer Interference In-Vivo. Adv. Mater. Deerf. Beach, Fla.) 27 (16), 2627–2634. doi:10.1002/adma.201405926
Wang, Y., Xu, Z., Guo, S., Zhang, L., Sharma, A., Robertson, G. P., et al. (2013). Intravenous Delivery of siRNA Targeting CD47 Effectively Inhibits Melanoma Tumor Growth and Lung Metastasis. Mol. Ther. 21 (10), 1919–1929. doi:10.1038/mt.2013.135
Webb, S. D., Owen, M. R., Byrne, H. M., Murdoch, C., and Lewis, C. E. (2007). Macrophage-Based Anti-Cancer Therapy: Modelling Different Modes of Tumour Targeting. Bull. Math. Biol. 69 (5), 1747–1776. doi:10.1007/s11538-006-9189-2
Weber, J., Hamid, O., Amin, A., O'Day, S., Masson, E., Goldberg, S. M., et al. (2013). Randomized Phase I Pharmacokinetic Study of Ipilimumab with or without One of Two Different Chemotherapy Regimens in Patients with Untreated Advanced Melanoma. Cancer Immun. 13, 7.
Weiden, P. L., Flournoy, N., Thomas, E. D., Prentice, R., Fefer, A., Buckner, C. D., et al. (1979). Antileukemic Effect of Graft-Versus-Host Disease in Human Recipients of Allogeneic-Marrow Grafts. N. Engl. J. Med. 300 (19), 1068–1073. doi:10.1056/NEJM197905103001902
Wilson, D. R., Sen, R., Sunshine, J. C., Pardoll, D. M., Green, J. J., and Kim, Y. J. (2018). Biodegradable STING Agonist Nanoparticles for Enhanced Cancer Immunotherapy. Nanomedicine 14 (2), 237–246. doi:10.1016/j.nano.2017.10.013
Wolchok, J. D., Kluger, H., Callahan, M. K., Postow, M. A., Rizvi, N. A., Lesokhin, A. M., et al. (2013). Nivolumab Plus Ipilimumab in Advanced Melanoma. N. Engl. J. Med. 369 (2), 122–133. doi:10.1056/NEJMoa1302369
Wolfers, J., Lozier, A., Raposo, G., Regnault, A., Théry, C., Masurier, C., et al. (2001). Tumor-Derived Exosomes are a Source of Shared Tumor Rejection Antigens for CTL Cross-Priming. Nat. Med. 7 (3), 297–303. doi:10.1038/85438
Xie, Y. Q., Arik, H., Wei, L., Zheng, Y., Suh, H., Irvine, D. J., et al. (2019). Redox-Responsive Interleukin-2 Nanogel Specifically and Safely Promotes the Proliferation and Memory Precursor Differentiation of Tumor-Reactive T-Cells. Biomaterials Sci. 7 (4), 1345–1357. doi:10.1039/c8bm01556b
Xiong, Q., Lee, G. Y., Ding, J., Li, W., and Shi, J. (2018). Biomedical Applications of mRNA Nanomedicine. Nano Res. 11 (10), 5281–5309. doi:10.1007/s12274-018-2146-1
Xu, Z., Wang, Y., Zhang, L., and Huang, L. (2014). Nanoparticle-Delivered Transforming Growth Factor-β siRNA Enhances Vaccination Against Advanced Melanoma by Modifying Tumor Microenvironment. ACS Nano 8 (4), 3636–3645. doi:10.1021/nn500216y
Yan, S., Zhao, P., Yu, T., and Gu, N. (2019). Current Applications and Future Prospects of Nanotechnology in Cancer Immunotherapy. Cancer Biol. Med. 16 (3), 486–497. doi:10.20892/j.issn.2095-3941.2018.0493
Yang M, M., Li, J., Gu, P., and Fan, X. (2020). The Application of Nanoparticles in Cancer Immunotherapy: Targeting Tumor Microenvironment. Bioact. Mater. 6 (7), 1973–1987. doi:10.1016/j.bioactmat.2020.12.010
Yang, Y., Guo, J., and Huang, L. (2020). Tackling TAMs for Cancer Immunotherapy: It's Nano Time. Trends Pharmacol. Sci. 41 (10), 701–714. doi:10.1016/j.tips.2020.08.003
Yee, C. (2013). Adoptive T-Cell Therapy for Cancer: Boutique Therapy or Treatment Modality? Clin. Cancer Res. 19 (17), 4550–4552. doi:10.1158/1078-0432.CCR-13-1367
Yoo, J. W., Irvine, D. J., Discher, D. E., and Mitragotri, S. (2011). Bio-inspired, Bioengineered and Biomimetic Drug Delivery Carriers. Nat. Rev. Drug Discov. 10 (7), 521–535. doi:10.1038/nrd3499
Yoyen-Ermis, D., Ozturk, K., Kursunel, M. A., Aydin, C., Ozkazanc, D., Gurbuz, M. U., et al. (2018). Tumor-Induced Myeloid Cells are Reduced by Gemcitabine-Loaded PAMAM Dendrimers Decorated with Anti-Flt1 Antibody. Mol. Pharm. 15 (4), 1526–1533. doi:10.1021/acs.molpharmaceut.7b01075
Yu, Y., and Cui, J. (2018). Present and Future of Cancer Immunotherapy: A Tumor Microenvironmental Perspective. Oncol. Lett. 16 (4), 4105–4113. doi:10.3892/ol.2018.9219
Yuba, E., Kanda, Y., Yoshizaki, Y., Teranishi, R., Harada, A., Sugiura, K., et al. (2015). PH‐Sensitive Polymer‐liposome‐based Antigen Delivery Systems Potentiated with Interferon‐γ Gene Lipoplex for Efficient Cancer Immunotherapy. Biomaterials 67, 214–224. doi:10.1016/j.biomaterials.2015.07.031
Yuba, E., Uesugi, S., Yoshizaki, Y., Harada, A., and Kono, K. (2017a). Potentiation of Cancer Immunity-Inducing Effect by pH-Sensitive Polysaccharide-Modified Liposomes with Combination of TGF-β Type I Receptor Inhibitor-Embedded Liposomes. Med. Res. Archives 5 (5). doi:10.18103/mra.v5i5.1243
Yuba, E., Yamaguchi, A., Yoshizaki, Y., Harada, A., and Kono, K. (2017b). Bioactive Polysaccharide-Based pH-Sensitive Polymers for Cytoplasmic Delivery of Antigen and Activation of Antigen-specific Immunity. Biomaterials 120, 32–45. doi:10.1016/j.biomaterials.2016.12.021
Zahavi, D., and Weiner, L. (2020). Monoclonal Antibodies in Cancer Therapy. Antibodies 9 (3), 34. doi:10.3390/antib9030034
Zanganeh, S., Hutter, G., Spitler, R., Lenkov, O., Mahmoudi, M., Shaw, A., et al. (2016). Iron Oxide Nanoparticles Inhibit Tumour Growth by Inducing Pro-inflammatory Macrophage Polarization in Tumour Tissues. Nat. Nanotechnol. 11 (11), 986–994. doi:10.1038/nnano.2016.168
Zhan, X., Jia, L., Niu, Y., Qi, H., Chen, X., Zhang, Q., et al. (2014). Targeted Depletion of Tumour-Associated Macrophages by an Alendronate-Glucomannan Conjugate for Cancer Immunotherapy. Biomaterials 35 (38), 10046–10057. doi:10.1016/j.biomaterials.2014.09.007
Zhang, S., Zhao, B., Jiang, H., Wang, B., and Ma, B. (2007). Cationic Lipids and Polymers Mediated Vectors for Delivery of siRNA. J. Control. Release 123 (1), 1–10. doi:10.1016/j.jconrel.2007.07.016
Zhang, W. W., Li, L., Li, D., Liu, J., Li, X., Li, W., et al. (2018). The First Approved Gene Therapy Product for Cancer Ad-p53 (Gendicine): 12 Years in the Clinic. Hum. gene Ther. 29 (2), 160–179. doi:10.1089/hum.2017.218
Zhang, Y., Lin, S., Wang, X. Y., and Zhu, G. (2019). Nanovaccines for Cancer Immunotherapy. Wiley Interdisciplinary Reviews. Nanomedicine nanobiotechnology 11 (5), e1559. doi:10.1002/wnan.1559
Zhao, B. S., Roundtree, I. A., and He, C. (2017). Post-transcriptional Gene Regulation by mRNA Modifications. Nat. Rev. Mol. Cell Biol. 18 (1), 31–42. doi:10.1038/nrm.2016.132
Zhao, Y., Zhao, X., Cheng, Y., Guo, X., and Yuan, W. (2018). Iron Oxide Nanoparticles-Based Vaccine Delivery for Cancer Treatment. Mol. Pharm. 15 (5), 1791–1799. doi:10.1021/acs.molpharmaceut.7b01103
Zheng, X., Koropatnick, J., Chen, D., Velenosi, T., Ling, H., Zhang, X., et al. (2013). Silencing Ido in Dendritic Cells: a Novel Approach to Enhance Cancer Immunotherapy in a Murine Breast Cancer Model. Int. J. Cancer 132 (4), 967–977. doi:10.1002/ijc.27710
Zheng, Y., Stephan, M. T., Gai, S. A., Abraham, W., Shearer, A., and Irvine, D. J. (2013). In Vivo Targeting of Adoptively Transferred T-Cells with Antibody- and Cytokine-Conjugated Liposomes. J. Control. Release 172 (2), 426–435. doi:10.1016/j.jconrel.2013.05.037
Zheng, Y., Tang, L., Mabardi, L., Kumari, S., and Irvine, D. J. (2017). Enhancing Adoptive Cell Therapy of Cancer through Targeted Delivery of Small-Molecule Immunomodulators to Internalizing or Noninternalizing Receptors. ACS Nano 11 (3), 3089–3100. doi:10.1021/acsnano.7b00078
Zhou, S., Zhang, T., Peng, B., Luo, X., Liu, X., Hu, L., et al. (2017). Targeted Delivery of Epirubicin to Tumor-Associated Macrophages by Sialic Acid-Cholesterol Conjugate Modified Liposomes with Improved Antitumor Activity. Int. J. Pharm. 523 (1), 203–216. doi:10.1016/j.ijpharm.2017.03.034
Keywords: cancer, immunotherapy, cancer vaccine, oncolytic virotherapy, immune checkpoint blockade, CAR T cell therapy, nanotechnology/nanomaterials
Citation: Nadukkandy AS, Ganjoo E, Singh A and Dinesh Kumar L (2022) Tracing New Landscapes in the Arena of Nanoparticle-Based Cancer Immunotherapy. Front. Nanotechnol. 4:911063. doi: 10.3389/fnano.2022.911063
Received: 01 April 2022; Accepted: 10 May 2022;
Published: 08 June 2022.
Edited by:
Saba Tufail, Aligarh Muslim University, IndiaReviewed by:
Atif Zafar, Case Western Reserve University, United StatesAbhishesh Kumar Mehata, Indian Institute of Technology (BHU), India
Copyright © 2022 Nadukkandy, Ganjoo, Singh and Dinesh Kumar. This is an open-access article distributed under the terms of the Creative Commons Attribution License (CC BY). The use, distribution or reproduction in other forums is permitted, provided the original author(s) and the copyright owner(s) are credited and that the original publication in this journal is cited, in accordance with accepted academic practice. No use, distribution or reproduction is permitted which does not comply with these terms.
*Correspondence: Lekha Dinesh Kumar, bGVraGFAY2NtYi5yZXMuaW4=