- Peter the Great St. Petersburg Polytechnic University, Saint Petersburg, Russia
The current state of the art in active corrosion prevention is based on the use of macromolecular containers that can store and release corrosion inhibitors particularly to the surface when corrosion develops. These corrosion inhibitor-containing nano- or microcontainers are subsequently infused into coatings, allowing them to self-heal. Especially, nanocontainers for self-healing coatings with controlled corrosion inhibitors, energy storage, cement fracture repair, and antifouling metal protection have recently been developed. Incorporating these nanocontainers into materials in small amounts (e.g., 5–10 wt% in paints) provided anticorrosion protection that was incomparably better than the current approaches. Furthermore, the materials developed had multifunctional properties, including self-healing, antibacterial, and antimicrobial properties. The primary goal of this review was to compile the different research studies that have been published in a variety of publications so that the reader may better understand the potential of these new types of nanotechnology and the prospects for nanocontainers.
Introduction
Nanocontainers have stimulated our research group to apply them to medicine (Efthimiadou et al., 2011; Lelovas et al., 2018), catalysis (Kartsonakis et al., 2008), implants (Angelopoulou et al., 2015), metal protection (Montemor et al., 2012), energy (Kordas, 2021), biofouling (Kordas, 2020a), and cement (Kordas, 2021). The main motive for developing nanocontainers was the possibility of encapsulation of substances inside them and their release there and when necessary to act on its target, e.g., targeted cancer therapy (Kordas, 2022), and self-healing of corrosion (Kartsonakis et al., 2013a; Kordas et al., 2015). The triggers used to release the substances trapped in the nanocontainers are internal or external, such as temperature (Kordas, 2019a), redox (Kordas, 2019a), pH (Tapeinos et al), rf-field (Chatzipavlidis et al., 2011), and chemical potential on the surface of the corroded metal (Kartsonakis et al., 2013a). Hollow nanocontainers are produced following three steps: core production, coating production, and removal of the core via thermal (polystyrene core) or dilute HF solution (silica core) treatment (Kordas, 2019b).
Other groups followed a different path for incorporating corrosion inhibitors within nanocrystalline layered double hydroxide (LDH)-based nanocontainers. These nanocontainers consist of layered double Mg/Al and Zn/Al hydroxides with vanadium incorporated in the interlayer zones. The LDHs release the inhibitors on demand (Shchukina and Shchukin, 2019). We studied the beneficial effect of self-healing combining the two nanocontainer systems, namely, CeMo and LDH nanocontainers (Montemor et al., 2012). We attributed the observed phenomena to the different release kinetics of the inhibitors from the nanocontainers.
The purpose of this article was to gather the scattered literature on nanocontainers to review the state of the art of the impinging technology in self-healing of coatings and to highlight the opportunities arising from this technology. The discussion will be limited to the autonomous self-healing of materials and the antifouling technology that we developed in the sol-gel laboratory of the National Center for Scientific Research (NCSR) Demokritos. In addition, we developed technologies for self-healing of concrete (Kordas, 2021), energy storage (Belessiotis et al., 2018) and absorption (Kordas, 2020b), and quadrupole stimuli-responsive nanocontainers for targeted cancer therapy in the same laboratory (Tapeinos et al., 2016). We will not discuss these technologies here but refer the reader to the existing literature.
Concept of the Multilevel Protection Approach
Figure 1 summarized the active ingredients incorporated into the protective system that respond to different types of mechanisms of destruction due to the external environment:
1) The first level of protection consists of CuO or ZnO nanocontainers enriched with bromosphaerol or SeaNine©. Next, antimicrobial action occurs via TiO2 nanocontainers.
2) The second level of protection comprises water and chlorine nanotraps.
3) The third level of protection involves the encapsulation of inorganic anticorrosion substances (corrosion inhibitors) in different types of micro/nanocontainers (CeO2 (5-ATDT) or CeMo (8-HQ)) that will, upon release, suppress corrosion.
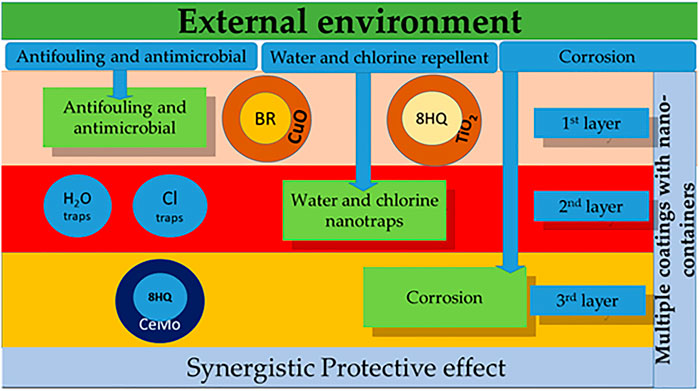
FIGURE 1. Multilevel protection of metals via nano/microcontainers. BR, bromosphaerol; 8-HQ, 8-hydroxyquinoline; CeMo, cerium/molybdenum oxide; CuO, copper oxide.
The technology we developed uses nanocontainers on the coatings of the order of 5–10% with multiple coatings to achieve multifunctionality of the protection system. In addition, some nanocontainers perform multiple functions on the coatings.
In the following, we focus on (I) antifouling protection for metals used in ships and (II) the anticorrosion protection that we can use in the transport industry (cars and aircraft).
Biofouling Protection via Nanocontainers
Biofouling is a process that occurs on the skeletons of ships via the accumulation of organisms or rust on them. Thus, one can divide the development of pollution into two factors. The chemical factor is due to the corrosive processes on the marine structures developing rust and other corrosive products, and the organic factor is due to fouling occurring gradually, which is more evident in a marine environment than in freshwater. A metallic surface exposed to the atmosphere or an electrolyte acts automatically as an ion conductor, where load-carrying reactions (electrons) take place in the interface of the metal and the electrolyte, resulting in corrosion of the metal. Thus, corrosion of a metal is a destructive attack through an electrochemical reaction with the environment. Organisms that cling persistently to the skeleton of a ship slow down the speed of its journey. The result is that its efficiency decreases (Singh and Turner, 2009). Solutions to this problem have been attempted using different technologies with different results. One used natural products such as wax, tar, and asphalt, and wooden inserts with fat, tar, and studs placed next to each other to create a metal shell. Other tests used rubber, vulcanite, and cork, and were soon discarded due to high cost and difficulty of application. Others used copper as a nail attached on zinc, nickel, steel, and other materials but were soon discarded, as well as the bronze exterior with iron pins due to their corrosion. In 1950, studies included the use of organo-metallic dyes with tin, arsenic, and mercury, leading to the discovery of tributyltin compounds (TBT; (C4H9)3Sn), but were discarded due to their toxicity, primarily to oysters, snails, and shellfish (Carlsson et al., 2000). Nowadays, copper oxide used in commercially available antifouling has been found to be very detrimental to marine organisms, although aquatic species differ greatly in their sensitivity to the heavy metal. Some animals, such as mollusks, can tolerate high concentrations of the metal, while others are adversely affected by very low concentrations of copper. In general, copper toxicity mechanisms involve the inhibition of some key respiratory enzymes, the generation of oxygen species (ROS), and changes in gene transcription especially related to oxidative stress (Kiaune and Singhasemanon, 2011). Some Baltic Sea countries limit the use of copper to incorporating them into antifouling paints. Due to environmental concerns, the Swedish authorities placed restrictions on the use of copper antifouling paints for recreational boats in 2000. However, this restriction has been lifted today in Sweden, where biocides are allowed in a limited concentration, including copper. In Denmark, there are restrictions on the use of copper in paints for recreational boats (HELCOM et al., 2010). The requirement (HELCOM et al., 2010) is a limited copper release rate in the color of 200 μg Cu/cm2 in 14 days and 350 μg Cu/cm2 after 30 days (Baaner and Anker, 2015). It is also forbidden to use antifouling paints on boats that sail mainly in freshwater (Kordas, 2019a). Netherlands has proposed to abolish the use of copper in antifouling paints in small boats but changed the policy after the EU’s scientific committee responsible for health and environment concluded in a study that there is no satisfactory evidence of damage to health caused by the use of copper. So, the Dutch government has prevented its use in antifouling (HELCOM et al., 2010). Today, no such restrictions are imposed on large ships, and copper is used in percentages ranging from 26 to 76 wt%, along with zinc and SeaNine©, to act against the growth of organisms (Kordas, 2019a). However, because zinc is a weak biocide, copper is added to the paint, thus increasing the toxicity of the final product. Some organisms are still resistant to inorganic Cu and Zn, so it is possible to enrich the antifouling paint to improve its properties by adding other ingredients that support biocidal products containing Cu, Zn, or others (Magin et al., 2010). Sea-Nine 211© is another example that is known to act against nontarget phytoplankton, bivalves, echinoderms, and ascidians by causing embryotoxicity, larval mortality, and immunotoxicity (Cima et al., 2013). Today, there is a demand to find natural and environmentally friendly antifouling compounds (Callow and Callow, 2002). Much effort has been directed toward identifying such natural compounds that may act as antifoulants (Burgess et al., 2003). In the marine environment, some organisms are equipped with natural physical or chemical defense mechanisms against fouling (Hellio et al., 2000). Several marine metabolites show significant levels of antibacterial, antifungal, antiprotozoal, and antimacrofouling properties and have good potential to be developed as additives for antifouling paints (Ali et al., 2002). There are many demonstrable examples of marine organisms that resist epibiotic biofilms, including several species of algae that contain a diverse spectrum of chemical entities with antifouling activity. Algae seem to be chemically protected by surface-bound or continuously released water-soluble compounds that can deter invertebrate larvae from settling. Algal metabolites can affect some settling organisms’ development and grazing behavior, pointing to chemical antifouling mechanisms. Such bioactive secondary macroalgae metabolites can be developed into new eco-friendly antifouling paints. This research field has been quite fruitful (Turner, 2010), and recently, bromosphaerol was identified as a naturally occurring diterpene from the red algae Sphaerococcus coronopifolius (Hellenic, 2015) and was found to inhibit the settlement of barnacles on painted substrates. The antifouling activity of this compound has been tested in laboratory assays. It is important to test such compounds in commercial paint formulations to determine if they are active when included in an antifouling coating. Testing of compounds after incorporation into paints is extremely important for assessing their potential use as a commercial antifouling agent.
In the epoch of nanotechnology, scientists attempted to solve the problem by using 2 wt% of CeO2−x nanorods in the paints (Herget et al., 2017). The technology was tested in the laboratory and the field. Another team developed vanadium pentoxide nanowires incorporated into ship paints as anti-biofouling agents (Natalio et al., 2012). A large number of scientists tried to mimic the surfaces of sharks, mussels, and crabs that do not exhibit biofouling (Piola et al., 2009; Magin et al., 2010; Xu and Siedlecki, 2012; Chapman et al., 2014). In the field of biocides, a research group isolated bromosphaerol from red algae of the genus Laurencia found in the sea near Corfu (Protopapa et al., 2019). We incorporated bromosphaerol into CuO and ZnO nanocontainers, which exhibited excellent antifouling properties (Kordas, 2020a; Kordas, 2020).
CeMo nanocontainers loaded with 8-hydroxyquinoline (8-HQ) have been synthesized by our sol-gel laboratory as described in recent literature (Kordas, 2020). Additionally, we produced CuO and ZnO nanocontainers filled with bromosphaerol and SeaNine© (Kordas, 2020a). We obtained commercial paints from Wilkens and Re-Turn, containing our nanocontainers but not including any extracts. The nanocontainers mix very well with the commercial paints. We used two commercial paints and not paints of our laboratory because no shipping company will accept to paint its ships with commercially unknown paints that have not passed the approval process. We tested the paints in the seawater of Mikrolimano, a few kilometers from the Piraeus harbor, and other samples in the harbor of Singapore. We constructed a test facility consisting of a tube and a propeller rotating to move the seawater inside the tube at a speed of 14 knots and placed our test samples in the tube. We wanted to observe results corresponding to a ship moving with the mentioned velocity. We took the samples from the tubes after 3 months for further laboratory examinations. We placed the samples on a platform in the Singapore experiment for more than 35 months without significant fouling (Kordas, 2020a).
Figures 2A–F summarize the frequency response analysis (FRA) results of this study and present the SEM micrographs of the nanocontainers (top) and paints (bottom) of the measurements.
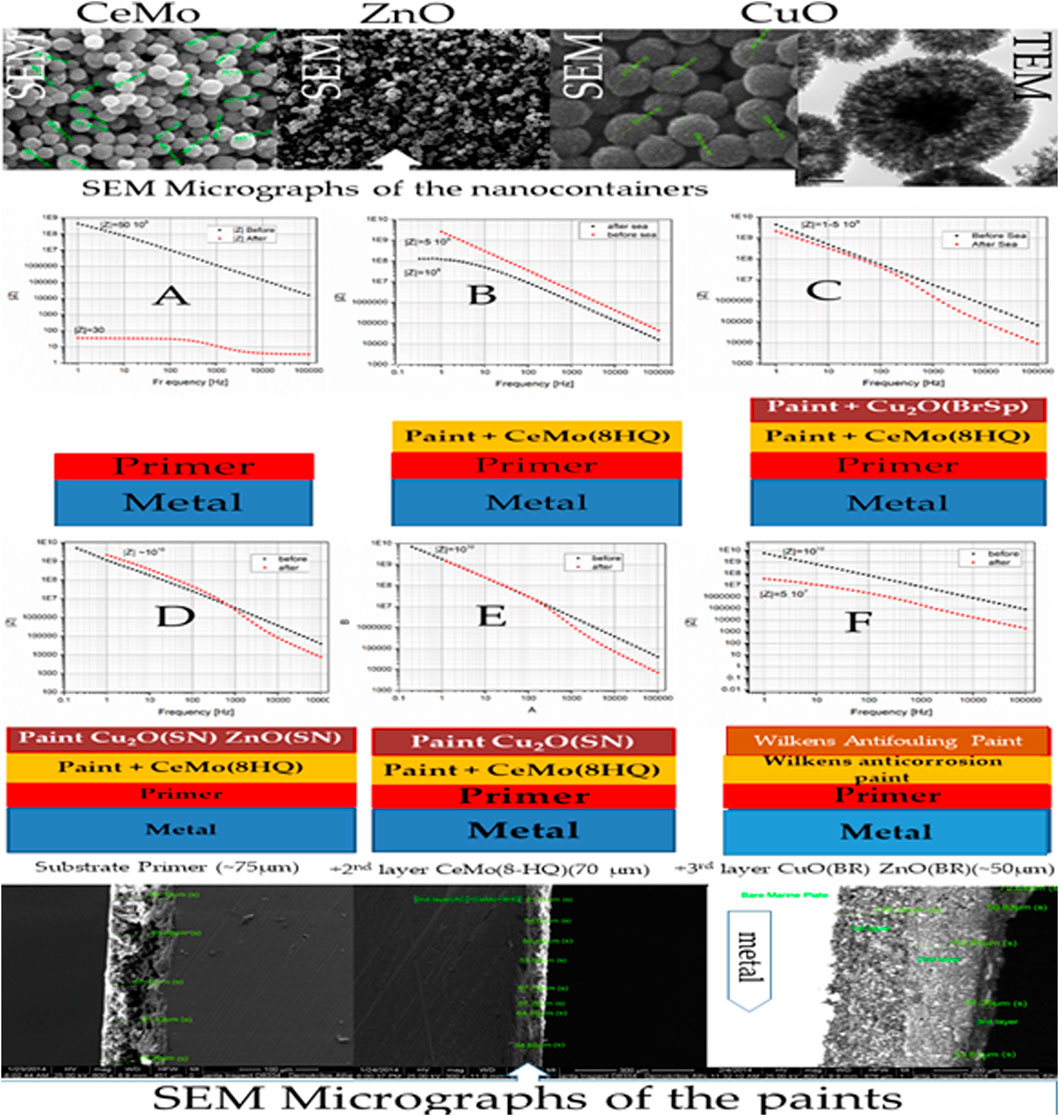
FIGURE 2. (A) FRA of the primer, (B) FRA of the primer and anticorrosion paint with 5 wt% CeMo (8-HQ), (C) FRA of the primer, the anticorrosion layer with 5 wt% CeMo (8-HQ), and the antifouling topcoat with 5 wt% CuO (BrSp), (D) FRA of the primer, anticorrosion paint with 5 wt% CeMo (8-HQ), and the antifouling topcoat with 5 wt% CuO (SN)–ZnO (SN), (E) FRA of the primer, anticorrosion paint with 5 wt% CeMo (8-HQ), and the antifouling topcoat with 5 wt% CuO (SN)–ZnO (SN), (F) FRA of the primer, Wilkens anticorrosion, and antifouling paint. The symbol “after” in the graphs means 3-month exposure to seawater. Top figure: SEM of the CuO, ZnO, and CeMo nanocontainers as produced in the literature (Kordas, 2020a). The top right figure shows the TEM micrograph of CuO nanocontainers. The bottom figures present the SEM micrographs of the paints.
The top part of Figure 2 shows the SEM micrographs of the CeMo (left), ZnO (middle), and CuO (right) nanocontainers incorporated into the paints. One can also observe the TEM picture of the CuO nanocontainers on the right top of this figure, indicating their porous structure allowing the load and release of the active agents. The bottom part of Figure 1 shows the image of the primer (∼90 μm), primer + anticorrosion paint (∼70 μm), and primer + anticorrosion + antifouling (∼100 μm) paint. The identical Figure 2 shows the FRA measurements of paints with compositions indicated below each FRA graph. Figure 2A shows that the FRA measurement of the primer drops considerably after exposure of the sample for 3 months to sea at the port of Mikrolimano. Figure 2B shows poor anticorrosion resistance of the sample containing the primer and the anticorrosion paint starting with a |Z| greater than 5 109 Ω before and |Z| about 108 Ω after exposure to seawater for 3 months. One can perceive from measurements shown in Figures 2C-E a significant improvement of the anticorrosion performance of the samples: |Z| does not change after exposure to seawater after 3 months.
We noted that the |Z| increases significantly after 3 months of exposure to marine water in the sample shown in Figure 2D. This outcome is due to the self-healing phenomenon observed in all our coatings containing nanocontainers loaded with inhibitors (Montemor et al., 2012; Kordas et al., 2013). At this point, we discuss the measurements made on coatings of Wilkens SA shown in Figure 2F. The measurement for this sample shows a significant drop in the |Z| from 1010 Ω to 510 Ω (Kordas, 2020), which proves that the samples in Figures 2C-E passed the test, demonstrating better performance.
Samples produced with the commercial paints from Wilkens and Re-Turn were exposed to a marine environment for many months, and results pertaining to the antifouling protection they offer to the metal were evaluated in the laboratory. Figure 3 shows a typical result of a sample that was exposed for 36 months to the marine environment of Singapore. We observed fouling mostly composed of sediments, slime, and green algae. Very few tubeworms were found. We did not observe other foulers on the substrate.
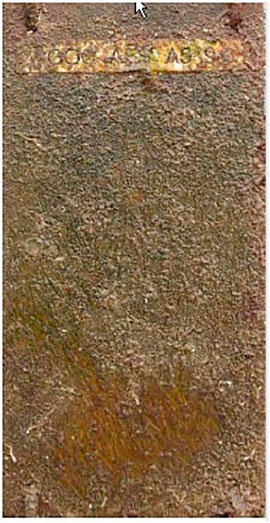
FIGURE 3. Sample with copper oxide nanocontainers loaded with SeaNine© incorporated into Re-Turn paints exposed to the seaport in Singapore.
This laboratory finding will have a commercial application if we prove that paints based on nanotechnology will have better results than commercial paints in the sea environment. This led us to work with Wilkens and Re-Turn, and we painted a small section of two ships. Figure 4 shows the strip we painted in the Sea Anemos passenger ship traveling across the Adriatic Sea between Ancona and Patras for a year. The ship was taken out in a yard after a year (shown in Figure 4), and no fouling contrary to the other part of the ship with commercial paint was found. We observed the same result for the ship painted with the Re-Turn paint (Kordas, 2019a; Kordas, 2020a; Kordas, 2020).
We used 5% CuO nanocontainers in the paints, whereas today’s commercial technology uses 40–76% copper in paints. Copper reduction with improved properties makes this technology very attractive. Moreover, we used bromosphaerol, a compound taken from the sea. In other words, our technology will not require extra costs to approve its use. All these make our technology more applicable and effective.
Other technologies to solve the biofouling problem are available in the literature. One idea is to detach the microorganisms from the epidermis of the metal in the way it is achieved with the shark’s skin, which consists of small triangular surfaces of geometric length ∼350 μm, with gaps between them of the order of 70 μm (Kordas, 2020b). This structure does not allow the deposit of microorganisms permanently on the shark’s skin because the movement rinses away the fouling products. This hypothesis in our case, like others, must prove more convincing, but the result is that this surface prevents the establishment of algae, barnacles, and bacteria.
Modification of polymer surfaces with different molecular structures that determine their length can lead to a new surface with a wide variety of biosystems. For example, addition of a PEG layer with a co-polar connection to a polymer substrate reduces bacterial adhesion (Kordas, 2019a). Another work showed that PEG-based coating inhibits the adherence of proteins, bacteria, and mammalian cells (Stobie et al., 2008; Chapman et al., 2010; Hench and Thompson, 2010; Francolini et al., 2012; Zhao et al., 2018). A sequence poly (L-lysine) (PLL)–PEG creates a coating that prevents cell adhesion, such as that of fibroblast and osteoblast cells (Gillies et al., 2004; Peleshanko and Tsukruk, 2008; Ma et al., 2012). The disadvantage of PEG-based systems is the lack of stability that is often observed over time (Banerjee et al., 2011; Charnley et al., 2011; Qian et al., 2013) and is the main reason it is not used. A successful coating involved attaching vancomycin to aneseline chromosomes via a PEG connector (Cutler and Cutler, 1999; Manahan, 2003; Andreas et al., 2011; Charnley et al., 2011). The aneseline chromophore allows strong connection to TiO2 surfaces and vancomycin provides antimicrobial action. Polyexamethylene biguanides (PHMBs) can also be used as a potential antimicrobial agent (Lubell, 2001; Charnley et al., 2011).
The biocides we use today are small molecules that must be delivered on demand. One must guarantee a life expectancy of about 5 years in the seawater environment. In this study, antifoulant immobilization is achieved by trapping bromosphaerol, SeaNine©, and 8-hydroxyquinoline into nanocontainers, where the actual diffusion coefficient is controlled by the porosity of the nanocontainers and the dissolution kinetic. Diffusion control of molecules incorporated into nanocontainers was proven in previous studies by our group (Kartsonakis et al., 2008). Immobilization of biocides in antifoulant paints was carried out with Cu2+ and Zn2+ nanoparticles, where a different adsorption and release profile was observed depending on the type of metal (Saji, 2019). The biocides incorporated into the nanocontainers in this work may be connected to the inner surface of the metal or stored freely inside the containers. Therefore, different release kinetics of biocides could be achieved depending on the external conditions.
We know from previous studies the behavior of Cu and Zn elements in their antifouling ability (Kordas, 2019a; Kordas, 2020a). We also note that the anticorrosion property is close to 10E10 (Kordas, 2021) in the CeMo (8-HQ) coating measured at 10E8 (Kordas, 2021) after 3 months of exposure to the marine environment. The as-received of the company is 10E10 (Kordas, 2020a) before exposure to the marine environment, and after 3 months of exposure, it is 5*10E7 (Kordas, 2020a), which is less than the value of the unexposed coating to the sea with CeMo (8-HQ) the as received paint of the company. This value increases when CeMo nanocontainers or ZnO charged with bromosphaerol or SeaNine211© is added to the triple paint. We must emphasize the fact that the values of |Z| after 3 months are the same as the best of the original paint without exposure to the sea. This is due to the phenomenon of “self-healing” that we have proved in other coatings with nanocontainers on a different metal but with the use of the scanning vibrating electrode technique (SVET) method (Montemor et al., 2012).
Recently, there has been a great effort in the literature toward the development of supramolecular chemistry, which is one of the exciting branches of chemistry that deals with co-polar interactions between molecules and subsequent super molecular structures for corrosion and biofouling prevention (Saji, 2019). This exciting work was aimed at developing surface coatings and corrosion inhibitors that include supramolecular polymers, organic–inorganic hybrid materials, and supramolecular graphene structures. We note that strategies that are not based on currently used industrial coatings will fail because the ship painting industry is very conservative and penetration of foreign technologies is very difficult. A new technology will be adopted more easily if it is based on current commercial paints, as we did in this study.
Many companies in the market produce corrosion-resistant and antifouling paints for ships. These companies have invested in the advancement of their technologies by developing excellent paints. The question is whether our technology can penetrate the ship painting industry and deliver improved properties. We have shown with our work that nanotechnology is a revolutionary technology with many expectations in this area.
Corrosion Protection Using Nanocontainers
In the recent past, we produced several types of nanocontainers that can protect iron, aluminum, and magnesium metals (Kartsonakis et al., 2012a; Kartsonakis et al., 2013b; Kartsonakis et al., 2014). The results were satisfactory, with many expectations for the automobile, ship, and airplane industry. In addition to nanocontainers filled with corrosion inhibitors, we developed nanosystems called nanotraps and water traps to control the diffusion of water and chlorine in the coatings (Krzak et al., 2012; Kartsonakis et al., 2014). Our research has shown that the coefficient of water and chlorine diffusion depends on the incorporation of these nanostructures in coatings (Krzak et al., 2012). We also made significant progress in the development of organically modified silica (ORMOSIL) coatings (Kartsonakis et al., 2011; Balaskas et al., 2012; Mekeridis et al., 2012; Kartsonakis et al., 2013a). Figure 5 shows the FRA results of samples containing CeMo and water traps of coatings on AA2024-T3 metals incorporating nanocontainers such as water traps, chlorine traps, and CeMo (8-HQ), offering multiple properties to protect metals using the technology presented in Figure 1. We exposed the coatings to NaCl (aq) 0.5 M for 1, 124, and 288 h. High-frequency spectra can be used to understand the development of the protective barrier properties of the coating. We noticed that the protective coatings containing water and chlorine nanotraps together with CeMo (8-HQ) nanocontainers remain unchanged from their original value even when exposed to NaCl (aq) 0.5 M for 288 h. These results of the FRA revealed that the incorporation of nanocontainers and micro-water traps into coatings is of extreme importance for substantial improvement of the corrosion properties of protective coatings without damaging the barrier properties and for preventing corrosion.
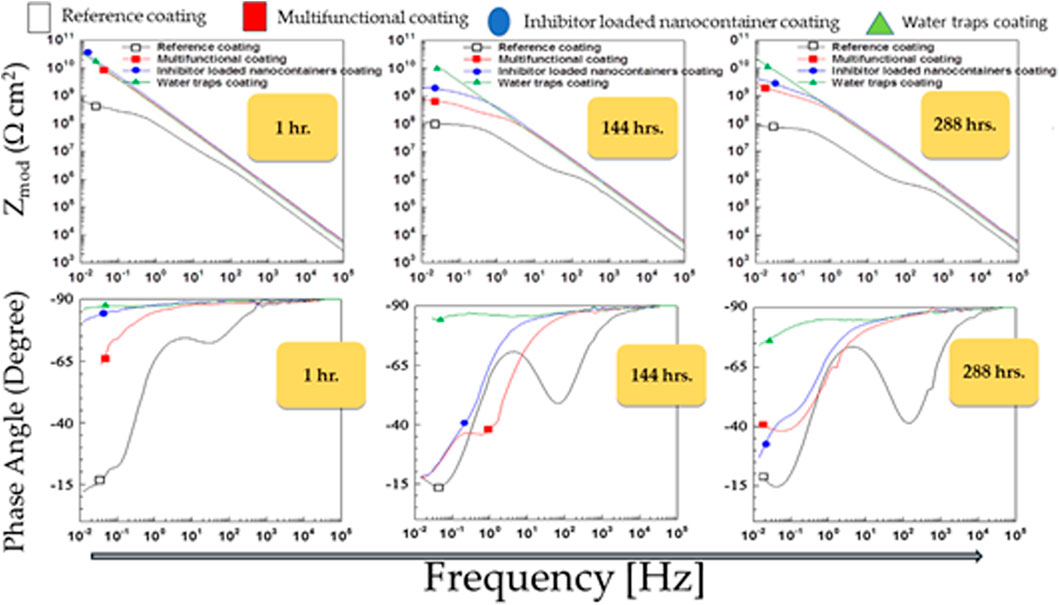
FIGURE 5. FRA of the multifunctional coating on AA2024-T3 metals including inhibitor-loaded nanocontainers and nanotraps of water and chlorine exposed to 0.5 M NaCl (aq) for 1, 144, and 288 h.
In another experiment, we incorporated CeMo (8-HQ) nanocontainers on Mg ZK10 in ORMOSIL coatings to show another application of our technology to another metal. SVET measurements were made in collaboration with the UAVR Aveiro and IST Lisbon Portugal teams (Kartsonakis et al., 2012b; Montemor et al., 2012). Figure 6 shows Rpolarization (Rpol) versus the exposure time to 0.5 M NaCl (aq) of an epoxy coating with CeMo (8-HQ) on Mg ZK10 substrate. One can perceive from this figure that Rpol first decreases with time from time point (a) to (b) and then increases with time from time point (b) to (c) for coatings with CeMo (8-HQ). The increase in Rpol from (b) to (c) results from the self-healing effect due to the action of 8-HQ from the nanocontainers. On the contrary, we noticed a temporary increase from time point (d) to (e), and then we observed a decrease in Rpol from time point (e) to (f) in samples with coatings without CeMo (8-HQ) due to corrosion.
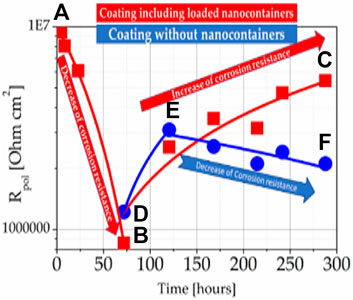
FIGURE 6. Rpol versus the time of exposure to 0.5 M NaCl (aq) in epoxy coating with ((A–C) route) and without ((D–F) route) CeMo (8-HQ) on Mg ZK10 substrate.
Hot-dip galvanized zinc (HDG) steel is used in marine environments such as decks of bridges and various marine structures under adverse climatic conditions to strengthen concrete (Kartsonakis et al., 2008; Efthimiadou et al., 2011; Lelovas et al., 2018). When hardening the concrete to a very high pH, hydrogen and zinc corrosion products are created, resulting in a loss of strength of the bonds between the concrete and galvanized bar (Zhang, 1996; Maldonado et al., 2010). The evolution of hydrogen occurs on the surface of zinc-coated steel incorporated in portland cement where iron and zinc are in contact (Bird, 1962). The corrosion reaction of a part of the pure zinc layer is responsible for the passivity of galvanized steel (Wilson et al., 1987). While zinc tends to dissolve in acidic aqueous solutions, with the evolution of hydrogen, in alkaline solutions with pH values between 8.5 and 10.5, zinc forms a zinc hydroxide film, and erosion occurs only at a low rate (Yeomans, 1994). We produced ORMOSIL coatings in which we incorporated CeO2 nanocontainers loaded with 5-amino-1, 3, 4-thiadiazole-2-thiol (5-ATDT), and we evaluated them with electrochemical impedance spectroscopy (EIS) and determined the value of Rp for the various coatings we developed as a function of immersion time in 0.5 M NaCl for 30, 60, 90, and 180 h (Kordas, 2021). The Rp at 30 h for HDG, HDG + ORMOSIL, HDG + ORMOSIL + 5-ATDT, HDG + ORMOSIL + CeO2 (EMPTY), and HDG + ORMOSIL + CeO2 (5-ATDT) was 1.62 E+03, 1.54 E+04, 4.0 E+04, 21.62 E+04, and 16.78 E+05 Ω cm2, respectively. Rp was smaller for HDG + ORMOSIL+5-ATDT than for HDG + ORMOSIL + CeO2 (5-ATDT) because we assume that 5-ATDT was captured in the coating and thus immobilized, without being able to be released when needed and offer protection like the 5-ATDT in the nanocontainers (Kordas, 2021). Figure 7 presents the Rp for HDG + ORMOSIL + CeO2 (EMPTY) and HDG + ORMOSIL + CeO2 (5-ATDT) after exposure to 0.5 M NaCl for 30, 60, 90, and 180 h. We noticed that the Rp for HDG + ORMOSIL + CeO2 (EMPTY) depends linearly on the time of exposure but has values smaller than the corresponding values of HDG + ORMOSIL + CeO2 (5-ATDT). The extra protection comes from the release of 5-ATDT from the nanocontainers, which creates gelatin on the HDG surface and protects it.
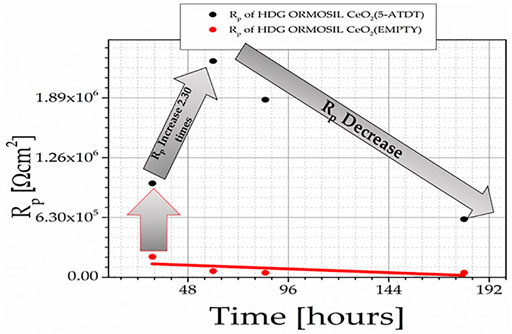
FIGURE 7. Rp of HDG + ORMOSIL + CeO2 (EMPTY) and HDG + ORMOSIL + CeO2 (5-ATDT) exposed to 0.5 M NaCl for 30, 60, 90, and 180 h.
The Rp increase in HDG + ORMOSIL + CeO2 (5-ATDT) coatings up to 60 h is explained by the self-healing phenomenon resulting from the release of corrosion inhibitors from nanocontainers. It seems that a significant fraction was consumed in the initial stage (60 h), and over time, the efficiency was reduced, offering protection against corrosion. Several organic compounds demonstrated an inhibiting effect toward aqueous corrosion in various metals and alloys (Guo et al., 2017). Among them, 5-ATDT forms a membrane on the iron surface, hindering the diffusion of water on the surface (Ahamad et al., 2010). Molecules comprising nitrogen and sulfur are very efficient inhibitors. 5-ATDT performs outstandingly on aggressive media (Ramesh Saliyan and Adhikari, 2008).
ORMOSIL coatings containing TiO2 nanocontainers loaded with 8-hydroxyquinoline (8-HQ) were made on pure copper exposed to 0.05 M NaCl solution for 3, 6, and 50 h (Kordas et al., 2013). Figure 8 shows the impedance spectrum of the coated samples compared with bare Cu substrate. After 3, 6, and 50 h of immersion, the impedance spectra show that the phase angle’s shape, in the medium frequency range, is broader when the epoxy coating is formed compared to that of the bare Cu substrate. This was explained assuming two overlapping processes in the midterm frequency range. The first process is due to an interfacial oxide layer, probably involving a copper oxide layer (Kordas et al., 2013). The second process is due to the formation of an additional interfacial layer generated by inhibitors, namely, the formation of Cu (II)–hydroxyquinoline complexes (Kordas et al., 2013). We observed another time constant in the bare Cu substrate in the low-frequency region attributed to diffusion-controlled pathways in the copper oxide layer for corrosive species such as Cl− and O2- to the Cu substrate (Kordas et al., 2013).
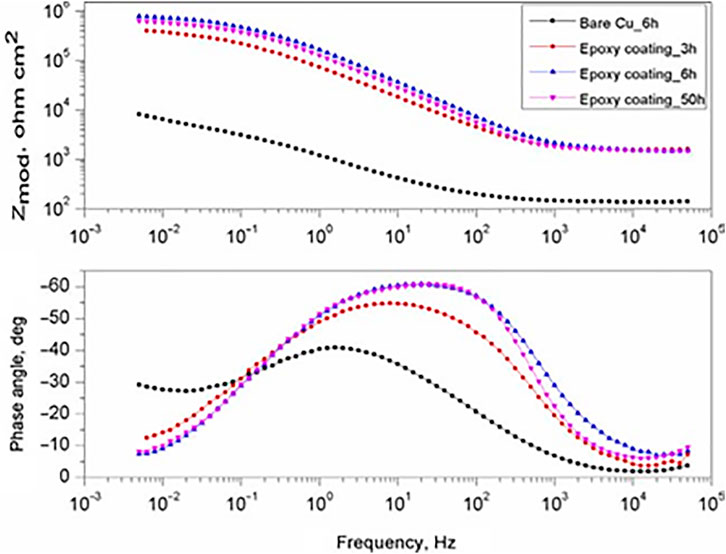
FIGURE 8. EIS Bode plots for ORMOSIL-TiO2 (8-HQ) after 3, 6, and 50 h in 0.05 M NaCl together with bare Cu.
We fitted the EIS spectra using the equivalent circuits (EC) of Figure 7. We observed the two time constants R1oxide/CPE1oxide formed at frequencies of about 120 Hz and the R2oxide/CPE2oxide at slightly lower frequencies (about 2 Hz). Figure 9 shows the results of R1oxide and R2oxide at 3, 6, and 50 h in 0.05 M NaCl solution. From Figure 9, one can perceive an increase in R1oxide and R2oxide from 3 to 6 h and then a decrease from 6 to 50 h of immersion in the salt solution.
We took a sample by engraving a 50-μm scratch to remove the ORMOSIL coating with the TiO2 (8-HQ) nanocontainers. This sample was immersed in a 0.05 M solution of NaCl water for 6 h. Then, Raman spectra of the scratch were recorded (Kordas et al., 2013). Figure 10B shows the Raman spectrum of the compound formed on the scratch. This spectrum can be interpreted possibly as the spectrum of the Cu(8-HQ)2 compound. To prove that, we composed the Cu(8-HQ)2 compound in the laboratory with the procedure described in the bibliography and measured the Raman spectra (Figure 10A) that emerges from this process (Kordas et al., 2013). Figure 10C shows the Cu(8-HQ)2 spectrum calculated using the DFT (B3LYP-6-311G) method. The theoretical spectrum matches very well with the spectrum of the synthetic Cu(8-HQ)2 (Figure 10A) and the Raman spectrum of the compound formed on copper after corrosion-induced diffusion of 8-HQ from the nanocontainers. Therefore, we conclude that Cu(8-HQ)2 formed after the reaction of 8-HQ with the copper surface. The Raman investigation, together with the EIS measurements, suggests the “self-healing” phenomenon occurring in our samples. These experiments have shown that the self-healing mechanism is driven by the reaction of 8-HQ with the copper of the substrate, thus the Gibbs energy of the Cu(8-HQ)2 formation. 8-HQ was released from the CuO nanocontainers.
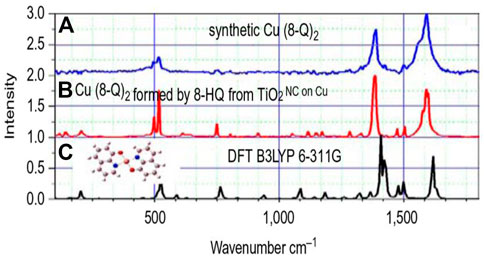
FIGURE 10. Raman spectra of the synthetic Cu(8-HQ)2 (B), Cu(8-HQ)2 formed as a result of the reaction between the released 8-HQ and copper substrate (A), and theoretical Raman spectrum of the Cu(8-HQ)2 compound (C) (Kordas et al., 2013).
Relevant Works for Future Study
Based on the encapsulation of ultrasonically produced zinc molybdate (ZM) nanoparticles, Karekar et al. created a smart coating of corrosion inhibitive nanocontainers. The results of corrosion potential and Bode graphs indicate that ZM nanocontainers can be successfully used in multifunctional anticorrosion coating formulations (Karekar et al., 2018). Mechanized hollow mesoporous silica nanoparticles (HMSNs) as smart nanocontainers implanted into self-assembled nanophase particle (SNAP) coating were used by Fu et al. to create an intelligent anticorrosion coating. According to the scanning vibrating electrode technique, smart nanocontainers with acid and alkaline dual stimuli-responsive properties can suppress corrosion activities on both microanodic and microcathodic regions at the same time, displaying superior self-healing functionality (Fu et al., 2013). Wang et al. employed a sol–gel protection technique to effectively construct morphologically intact hollow mesoporous zirconia nanospheres (HMZSs) with a hollow core mesoporous shell structure as scaffolds for smart nanocontainers (Wang et al., 2015). Feng and Cheng created an intelligent coating based on the encapsulation of benzotriazole (BTA) inhibitors in SiO2 nanoparticle-based polyelectrolyte nanocontainers and self-release of the inhibitors for corrosion inhibition of pipeline steel in a chloride solution (Wang et al., 2015). To preserve mild steel as a substrate, Yeganeh et al. created a composite coating by embedding 1% mesoporous silica loaded with sulfamethazine (MSInh) as a corrosion inhibitor into an epoxy film (Feng and Cheng, 2017). The results showed that epoxy/MSInh had a stronger corrosion resistance, confirming the barrier action of mesoporous silica against corrosive species and the corrosion inhibitory impact of released sulfamethazine molecules (Yeganeh et al., 2019). Xu et al. described a one-step approach for making inhibitor-loaded mesoporous silica nanocontainers without the need for extra processes such as template removal or inhibitor loading. The findings indicate that the one-step synthesis technique was effective, energy-efficient, and devoid of organic solvents, among other things (Xu et al., 2018). As corrosion inhibitor hosts, Saremi and Yeganeh created mesoporous silica nanocontainer powders and disseminated them in a polypyrrole matrix. In a NaCl solution, the release and corrosion resistance of composite coatings were investigated with and without an inhibitor (Saremi and Yeganeh, 2014). Lanzara et al. studied the dynamics of a fluid flowing out of a ruptured single-walled CNT (SWNT), where the fluid resembles an organic healing agent, to see if CNTs may be used as nanocontainers (Lanzara et al., 2009).
The photothermal response of plasmonic titanium nitride nanoparticles (TiN NPs) generated a new nanocomposite coating with dual-action self-healing corrosion protection, according to Ma et al. (TiN NPs) (Ma et al., 2021). In vitro cell cytotoxicity experiments performed by Yang et al. revealed that the loaded doxorubicin’s cell-killing effectiveness against human fibrosarcoma (HT-1080) and human breast adenocarcinoma (MCF-7) cells was pH-dependent (Yang et al., 2010). As a result, these hybrid composites could be used as pH-controlled drug delivery devices (Wang et al., 2015). Balaskas et al. synthesized and studied unique two-shell structured inhibitor-loaded poly (methacrylic acid)@cerium oxide (PMAA@CeO2) nanocontainers. The objective of the nanocontainers was to improve the corrosion resistance of an epoxy coating applied to an aeronautical metal (AA 2024-T3) (Balaskas et al., 2017). By loading a conventional 1-hydroxybenzotriazole (HOBT) inhibitor for corrosion protection of copper alloys in mesoporous silica nanocontainers, Ma et al. created a new form of composite nanoparticles (CNPs) (Ma et al., 2017). Microcapsules containing linseed oil were made by in situ polymerization, and benzotriazole was entrapped between polyelectrolyte layers constructed outside the microcapsules using the layer-by-layer process, according to Leal et al. (2018). As an unique nanocontainer system, Li et al. developed silica/polymer double-walled hybrid nanotubes with a hollow cavity, a porous silica inner wall, and a stimuli-responsive (pH, temperature, and redox) polymeric outer wall (Leal et al., 2018). Qian et al. demonstrated a novel self-healing coating based on nanocontainers. To make these nanocontainers and build a final structure of SiO2/chitosan/alginate/polyaspartic acid/alginate/polyaspartic acid, layer-by-layer deposition was used (Abu-Thabit and Hamdy, 2016). In another work, with the use of tannic acid complexes, Qian et al. demonstrated a simple approach for encapsulating a corrosion inhibitor in mesoporous silica nanoparticles (MSNs), resulting in inhibitor-loaded MSNs with a pH-controlled release function (Qian et al., 2019). Polyaniline capsules showed delayed release under oxidation and quick release under reduction, making them attractive candidates for anticorrosion applications, according to Lv et al. (2013).
The goal of Liu et al. was to create an intelligent coating using pH-responsive attapulgite (ATP) nanocontainers. Layer-by-layer (LBL) self-assembly technology was used to build these nanocontainers containing polyelectrolytes and inhibitors (Liu et al., 2018). For the corrosion inhibition of carbon steel, Yabuk et al. created a polymer covering incorporating cellulose nanofibers and a corrosion inhibitor (Yabuki et al., 2016). Sonawane et al. introduced a unique encapsulation-based method for the manufacture of nanocontainers that may release corrosion inhibitors in a controlled manner (benzotriazole) (Sonawane et al., 2012). Chenan et al. developed hollow mesoporous zirconia (hm-ZrO) nanospheres with a hollow core/porous shell configuration that could be used to load and release corrosion inhibitors. Solid silica nanoparticles serve as templates for hm-ZrO nanocontainers (Chenan et al., 2014). He et al. used a unique and simple chemical approach to successfully manufacture a new type of functional nanoreservoir based on MWCNTs and cyclodextrin (He et al., 2016). The research of Sambyal et al. focused on the creation of composite coatings for mild steel corrosion prevention in marine environments. In an aqueous medium of chitosan, a chemical oxidative polymerization technique was used to create poly (aniline-anisidine)/chitosan/SiO2 composites (Sambyal et al., 2018).
Conclusions and Prospects
Our team started research on nanocontainers around 2000, applying this technology to medicine (Efthimiadou et al., 2017; Kordas, 2019b), concrete (Kordas, 2021), and many other materials. Nanocontainers respond autonomously after excitation and correct damage resulting from the influence of the environment. Depending on the problem, one must choose the nanocontainers and their content. This technology has attracted the scientific and industrial world in the last 20 years because it is a flexible and promising technology. When a damage is caused, the substances trapped in the nanocontainers are released and cause self-healing, resulting in the coating acquiring its original characteristics. In collaboration with many other European teams and companies, our team worked to transfer this technology to the commercial level by subjecting it to strict industrial tests. This study shows that there is no value in producing a new technology if it is not tested in the field operating conditions by commercial users. We concluded from our many years of experience that the capabilities of nanocontainers are endless. This technology will undoubtedly be a part of the fourth industrial revolution with products to improve people’s lives.
Author Contributions
The author confirms being the sole contributor of this work and has approved it for publication.
Funding
Supporting the grant Self-Healing Construction Materials (contract No. 075-15-2021-590 dated 04.06.2021).
Conflict of Interest
The author declares that the research was conducted in the absence of any commercial or financial relationships that could be construed as a potential conflict of interest.
Publisher’s Note
All claims expressed in this article are solely those of the authors and do not necessarily represent those of their affiliated organizations, or those of the publisher, the editors, and the reviewers. Any product that may be evaluated in this article, or claim that may be made by its manufacturer, is not guaranteed or endorsed by the publisher.
Acknowledgments
The author thanks for supporting the grant Self-Healing Construction Materials (Contract Nos. 075-15-2021-590 dated 04.06.2021). The author also thanks Dr. M. R. Sanjay (Natural Composites Research Group Lab, Department of Materials and Production Engineering, The Sirindhorn International Thai-German Graduate School of Engineering (TGGS), King Mongkut’s University of Technology North Bangkok (KMUTNB), Bangkok, Thailand) for his contribution and proof reading.
References
Abu-Thabit, N. Y., and Hamdy, A. S. (2016). Stimuli-responsive Polyelectrolyte Multilayers for Fabrication of Self-Healing Coatings - A Review. Surf. Coat. Technol. 303, 406–424. doi:10.1016/j.surfcoat.2015.11.020
Ahamad, I., Prasad, R., and Quraishi, M. A. (2010). Inhibition of Mild Steel Corrosion in Acid Solution by Pheniramine Drug: Experimental and Theoretical Study. Corrosion Sci. 52 (No. 9), 3033–3041. doi:10.1016/j.corsci.2010.05.022
Ali, M. S., Saleem, M., Yamdagni, R., and Ali, M. A. (2002). Steroid and Antibacterial Steroidal Glycosides from marine green Alga Codium Iyengarii Borgesen. Nat. Prod. Lett. 16 (No. 6), 407–413. doi:10.1080/10575630290034249
Andreas, K., Zehbe, R., Kazubek, M., Grzeschik, K., Sternberg, N., Bäumler, H., et al. (2011). Biodegradable Insulin-Loaded PLGA Microspheres Fabricated by Three Different Emulsification Techniques: Investigation for Cartilage Tissue Engineering. Acta Biomater. 7 (No. 4), 1485–1495. doi:10.1016/j.actbio.2010.12.014
Angelopoulou, A., Efthimiadou, E. K., and Kordas, G. (2015). A New Approach to Fabricate Bioactive Silica Binary and Ternary Hybrid Microspheres. Mater. Sci. Eng. C 53, 76–82. doi:10.1016/j.msec.2015.04.014
Baaner, L., and Anker, H. T. (2015). Antifouling for Leisure Boats in the Baltic Sea. Sweden: Gothenburg School of Economics.
Balaskas, A. C., Hashimoto, T., Curioni, M., and Thompson, G. E. (2017). Two-shell Structured PMAA@CeO2 Nanocontainers Loaded with 2-mercaptobenzothiazole for Corrosion protection of Damaged Epoxy Coated AA 2024-T3. Nanoscale 9 (No. 17), 5499–5508. doi:10.1039/c7nr00858a
Balaskas, A. C., Kartsonakis, I. A., Tziveleka, L. A., and Kordas, G. (2012). Improvement of Anti-corrosive Properties of Epoxy-Coated AA 2024-T3 with TiO2 Nanocontainers Loaded with 8-hydroxyquinoline. Prog. Org. Coat. 74 (No. 3), 418–426. doi:10.1016/j.porgcoat.2012.01.005
Banerjee, I., Pangule, R. C., and Kane, R. S. (2011). Antifouling Coatings: Recent Developments in the Design of Surfaces that Prevent Fouling by Proteins, Bacteria, and Marine Organisms. Adv. Mater. 23, 690–718. doi:10.1002/adma.201001215
Belessiotis, G. V., Papadokostaki, K. G., Favvas, E. P., Efthimiadou, E. K., and Karellas, S. (2018). Preparation and Investigation of Distinct and Shape Stable paraffin/SiO2 Composite PCM Nanospheres. Energ. Convers. Manage. 168 (No. January), 382–394. doi:10.1016/j.enconman.2018.04.059
Bird, C. E. (1962). Bond of Galvanized Steel Reinforcement in Concrete. Nature 194, 798. doi:10.1038/194798a0
Burgess, J. G., Boyd, K. G., Armstrong, E., Jiang, Z., Yan, L., Berggren, M., et al. (2003). The Development of a marine Natural Product-Based Antifouling Paint. Biofouling 19 Suppl (No. Suppl. L), 197–205. doi:10.1080/0892701031000061778
Callow, M. E., and Callow, J. E. (2002). Marine Biofouling: a Sticky Problem. Biologist (London) 49 (No. 1), 10–14.
Carlsson, B., Mo, K., Frei, U., and Köhl, M. (2000). Comparison between Predicted and Actually Observed In-Service Degradation of a Nickel Pigmented Anodized Aluminium Absorber Coating for Solar DHW Systems. Solar Energ. Mater. Solar Cell 61, 223–238. doi:10.1016/s0927-0248(99)00112-9
Chapman, J., Weir, E., and Regan, F. (2010). Period Four Metal Nanoparticles on the Inhibition of Biofouling. Colloids Surf. B Biointerfaces 78 (No. 2), 208–216. doi:10.1016/j.colsurfb.2010.03.002
Chapman, J., Hellio, C., Sullivan, T., Brown, R., Russell, S., Kiterringham, E., et al. (2014). Bioinspired Synthetic Macroalgae: Examples from Nature for Antifouling Applications. Int. Biodeterioration Biodegradation 86, 6–13. doi:10.1016/j.ibiod.2013.03.036
Charnley, M., Textor, M., and Acikgoz, C. (2011). Designed Polymer Structures with Antifouling-Antimicrobial Properties. Reactive Funct. Polym. 71 (No. 3), 329–334. doi:10.1016/j.reactfunctpolym.2010.10.013
Chatzipavlidis, A., Bilalis, P., Efthimiadou, E. K., Boukos, N., and Kordas, G. C. (2011). Sacrificial Template-Directed Fabrication of Superparamagnetic Polymer Microcontainers for pH-Activated Controlled Release of Daunorubicin. Langmuir 27, 8478–8485. doi:10.1021/la201240h
Chenan, A., Ramya, S., George, R. P., and Mudali, U. K. (2014). Hollow Mesoporous Zirconia Nanocontainers for Storing and Controlled Releasing of Corrosion Inhibitors. Ceramics Int. 40 (No. 7), 10457–10463. doi:10.1016/j.ceramint.2014.03.016
Cima, F., Ferrari, G., Ferreira, N. G. C., Rocha, R. J. M., Serôdio, J., Loureiro, S., et al. (2013). Preliminary Evaluation of the Toxic Effects of the Antifouling Biocide Sea-Nine 211 in the Soft Coral Sarcophyton Cf. Glaucum (Octocorallia, Alcyonacea) Based on PAM Fluorometry and Biomarkers. Mar. Environ. Res. 83, 16–22. doi:10.1016/j.marenvres.2012.10.004
Cutler, S. J., and Cutler, H. G. (1999). Biologically Active Natural Products. Boca Raton, Florida, United States: CRC Press, 1–268.
Efthimiadou, E. K., Fragogeorgi, E., Palamaris, L., Karampelas, T., Lelovas, P., Loudos, G., et al. (2017). Versatile Quarto Stimuli Nanostructure Based on Trojan Horse Approach for Cancer Therapy: Synthesis, Characterization, In Vitro and In Vivo Studies. Mater. Sci. Eng. C 79, 605–612. doi:10.1016/j.msec.2017.05.082
Efthimiadou, E. K., Tapeinos, C., Bilalis, P., and Kordas, G. (2011). New Approach in Synthesis, Characterization and Release Study of pH-Sensitive Polymeric Micelles, Based on PLA-Lys-B-PEGm, Conjugated with Doxorubicin. J. Nanoparticle Res. 13 (No. 12), 6725–6736. doi:10.1007/s11051-011-0579-5
Feng, Y., and Cheng, Y. F. (2017). An Intelligent Coating Doped with Inhibitor-Encapsulated Nanocontainers for Corrosion protection of Pipeline Steel. Chem. Eng. J. 315, 537–551. doi:10.1016/j.cej.2017.01.064
Francolini, I., Crisante, F., Martinelli, A., D'Ilario, L., and Piozzi, A. (2012). Synthesis of Biomimetic Segmented Polyurethanes as Antifouling Biomaterials. Acta Biomater. 8 (No. 2), 549–558. doi:10.1016/j.actbio.2011.10.024
Fu, J., Chen, T., Wang, M., Yang, N., Li, S., Wang, Y., et al. (2013). Acid and Alkaline Dual Stimuli-Responsive Mechanized Hollow Mesoporous Silica Nanoparticles as Smart Nanocontainers for Intelligent Anticorrosion Coatings. ACS Nano 7 (No. 12), 11397–11408. doi:10.1021/nn4053233
Gillies, E. R., Jonsson, T. B., and Fréchet, J. M. (2004). Stimuli-responsive Supramolecular Assemblies of Linear-Dendritic Copolymers. J. Am. Chem. Soc. 126 (No. 38), 11936–11943. doi:10.1021/ja0463738
Guo, L., Ren, X., Zhou, Y., Xu, S., Gong, Y., and Zhang, S. (2017). Theoretical Evaluation of the Corrosion Inhibition Performance of 1,3-thiazole and its Amino Derivatives. Arabian J. Chem. 10 (No. 1), 121–130. doi:10.1016/j.arabjc.2015.01.005
He, Y., Zhang, C., Wu, F., and Xu, Z. (2016). Fabrication Study of a New Anticorrosion Coating Based on Supramolecular Nanocontainer. Synth. Met. 212, 186–194. doi:10.1016/j.synthmet.2015.10.022
HELCOM (2010). “Hazardous Substances in the Baltic Sea. An Integrated Thematic Assessment of Hazardous Substances in the Baltic Sea,” in Baltic Sea Environment Proceedings 120B. Editors S. Korpinen,, and M. Laamanen (Helsinki, Finland: HELCOMNo. February), 119.
Hellenic, N. (2015). “Development of New Antifouling Agents Derived from the Red Alga Sphaerococcus Coronopifolius,” in Proceedings of the 11th Panhellenic Symposium on Oceanography & Fisheries, Mytilene, Lesvos, Greece, May, 2015, 397–400.
Hellio, C., Bourgougnon, N., and Gal, Y. L. (2000). Phenoloxidase (E.C. 1.14.18.1) from the Byssus Gland ofMytilus edulis:Purification, Partial Characterization and Application for Screening Products with Potential Antifouling Activities. Biofouling 16, 235–244. Nos. 2–4. doi:10.1080/08927010009378448
Hench, L. L., and Thompson, I. (2010). Twenty-first century Challenges for Biomaterials. J. R. Soc. Interf. 7 Suppl 4 (No. Suppl. 4), S379–S391. doi:10.1098/rsif.2010.0151.focus
Herget, K., Hubach, P., Pusch, S., Deglmann, P., Götz, H., Gorelik, T. E., et al. (2017). Haloperoxidase Mimicry by CeO2-X Nanorods Combats Biofouling. Adv. Mater. 29 (No. 4), 1–8. doi:10.1002/adma.201603823
Hill, G. A., Spellman, D. L., and Stratfull, R. F. (1976). Laboratory Corrosion Tests of Galvanized Steel in Concrete. Sacramento, California, United States: California Department of Transport, 25–37. Transportation Research Record, No. 604.
Karekar, S. E., Bagale, U. D., Sonawane, S. H., Bhanvase, B. A., and Pinjari, D. V. (2018). A Smart Coating Established with Encapsulation of Zinc Molybdate Centred Nanocontainer for Active Corrosion protection of Mild Steel: Release Kinetics of Corrosion Inhibitor. Compos. Inter. 25 (No. 9), 785–808. doi:10.1080/09276440.2018.1439631
Kartsonakis, I. A., Athanasopoulou, E., Snihirova, D., Martins, B., Koklioti, M. A., Montemor, M. F., et al. (2014). Multifunctional Epoxy Coatings Combining a Mixture of Traps and Inhibitor Loaded Nanocontainers for Corrosion protection of AA2024-T3. Corrosion Sci. 85, 147–159. doi:10.1016/j.corsci.2014.04.009
Kartsonakis, I. A., Balaskas, A. C., and Kordas, G. C. (2011). Influence of Cerium Molybdate Containers on the Corrosion Performance of Epoxy Coated Aluminium Alloys 2024-T3. Corrosion Sci. 53 (No. 11), 3771–3779. doi:10.1016/j.corsci.2011.07.026
Kartsonakis, I. A., Balaskas, A. C., Koumoulos, E. P., Charitidis, C. A., and Kordas, G. C. (2012). Incorporation of Ceramic Nanocontainers into Epoxy Coatings for the Corrosion protection of Hot Dip Galvanized Steel. Corrosion Sci. 57, 30–41. doi:10.1016/j.corsci.2011.12.037
Kartsonakis, I. A., Balaskas, A. C., Koumoulos, E. P., Charitidis, C. A., and Kordas, G. (2012). Evaluation of Corrosion Resistance of Magnesium alloy ZK10 Coated with Hybrid Organic-Inorganic Film Including Containers. Corrosion Sci. 65, 481–493. doi:10.1016/j.corsci.2012.08.052
Kartsonakis, I. A., Balaskas, A. C., Koumoulos, E. P., Charitidis, C. A., and Kordas, G. (2013). ORMOSIL-epoxy Coatings with Ceramic Containers for Corrosion protection of Magnesium Alloys ZK10. Prog. Org. Coat. 76 (Nos. 2–3), 459–470. doi:10.1016/j.porgcoat.2012.10.028
Kartsonakis, I. A., Koumoulos, E. P., Charitidis, C. A., and Kordas, G. (2013). Hybrid Organic-Inorganic Coatings Including Nanocontainers for Corrosion protection of Magnesium alloy ZK30 Nanostructured Materials 2012. Special Issue Editors: Juan Manuel Rojo, Vasileios Koutsos. J. Nanoparticle Res. 15 (No. 8). doi:10.1007/s11051-013-1871-3
Kartsonakis, I., Daniilidis, I., and Kordas, G. (2008). Encapsulation of the Corrosion Inhibitor 8-hydroxyquinoline into Ceria Nanocontainers. J. Sol-Gel Sci. Technol. 48 (Nos. 1–2), 24–31. doi:10.1007/s10971-008-1810-4
Kiaune, L., and Singhasemanon, N. (2011). Pesticidal Copper (I) Oxide: Environmental Fate and Aquatic Toxicity. Rev. Environ. Contam. Toxicol. 213 (No. I), 1–26. doi:10.1007/978-1-4419-9860-6_1
Kordas, G. C., Balaskas, A. C., Kartsonakis, I. A., and Efthimiadou, E. K. (2013). A Raman Study of 8-Hydroxyquinoline Release from Loaded TiO2 Nanocontainer. Int. J. Struct. Integrity 4 (No. 1), 121–126. doi:10.1108/17579861311303672
Kordas, G. (2020). CuO (Bromosphaerol) and CeMo (8 Hydroxyquinoline) Microcontainers Incorporated into Commercial marine Paints. J. Am. Ceram. Soc. 103 (No. 4), 2340–2350. doi:10.1111/jace.16917
Kordas, G., and Efthimiadou, E. K. (2015). “Self-Healing Coatings for Corrosion Protection of Metals,” in The Sol-Gel Handbook 3–3. Editors D. Levy,, and M. Zayat (Hoboken, New Jersey, United States: John Wiley & Sons), 1371–1384. No. 2007. doi:10.1002/9783527670819.ch44
Kordas, G. (2020). Incorporation of Spherical Shaped CuO@SiO2 Light Microtraps into CuCoMnOx Spinels to Enhance Solar Absorbance. J. Am. Ceram. Soc. 103 (No. 3), 1536–1541. doi:10.1111/jace.16851
Kordas, G. (2021). Nanocontainers (CeO2): Synthesis, Characterization, Properties, and Anti-corrosive Application. ACS Symp. Ser. 1404, 177–185.
Kordas, G. (2021). “Nanocontainers to Increase the Absorption of Energy and Heat Conversion,” in Advanced Ceramics for Energy and Environmental Applications. Editor A. Kumar (Boca Raton, Florida, United States: CRC Press), 104–115. doi:10.1201/9781003005155-4
Kordas, G. (2019). Nanotechnology in Cancer Treatment as a Trojan Horse: From the Bench to Preclinical Studies. Amsterdam, Netherlands: Elsevier, 323–365. doi:10.1016/b978-0-12-816771-7.00017-x
Kordas, G. (2019). Nanotechnology to Improve the Biofouling and Corrosion Performance of marine Paints: from Lab Experiments to Real Tests in Sea. Int. J. Phys. Res. Appl. 2 (No. 1). 7–33. doi:10.29328/journal.ijpra.1001012
Kordas, G. (2020). “Novel Antifouling and Self-Healing Eco-Friendly Coatings for Marine Applications Enhancing the Performance of Commercial Marine Paints,” in Engineering Failure Analysis. Editor K. Thanapalan (London UK: Intech Open). doi:10.5772/intechopen.89261
Kordas, G. (2022). “Quadrupole Stimuli‐Responsive Targeted Polymeric Nanocontainers for Cancer Therapy: Artificial Intelligence in Drug Delivery Systems,” in Nanoengineering of Biomaterials. Editor S. J. S. Jana (Weinheim, Germany: WILEY‐VCH GmbH), 1, 505–522. doi:10.1002/9783527832095.ch16
Kordas, G. (2021). “Self-Healing of Concrete through Ceramic Nanocontainers Loaded with Corrosion Inhibitors and Microorganisms,” in Advanced Ceramic Materials. Editor M. Mhadhbi (London, UK: Intech Open), 1–28. doi:10.5772/intechopen.93514
Krzak, M., Tabor, Z., Nowak, P., Warszyński, P., Karatzas, A., Kartsonakis, I. A., et al. (2012). Water Diffusion in Polymer Coatings Containing Water-Trapping Particles. Part 2. Experimental Verification of the Mathematical Model. Prog. Org. Coat. 75, 207–214. doi:10.1016/j.porgcoat.2012.05.008
Lanzara, G., Yoon, Y., Liu, H., Peng, S., and Lee, W.-I. (2009). Carbon Nanotube Reservoirs for Self-Healing Materials. Nanotechnology 20 (33), 335704. doi:10.1088/0957-4484/20/33/335704
Leal, D. A., Riegel-Vidotti, I. C., Ferreira, M. G. S., and Marino, C. V. B. (2018). Smart Coating Based on Double Stimuli-Responsive Microcapsules Containing Linseed Oil and Benzotriazole for Active Corrosion protection. Corrosion Sci. 130 (No), 56–63.
Lelovas, P., Efthimiadou, E. K., Mantziaras, G., Siskos, N., Kordas, G., and Kostomitsopoulos, N. (2018). In Vivo toxicity Study of Quatro Stimuli Nanocontainers in Pregnant Rats: Gestation, Parturition and Offspring Evaluation. Regul. Toxicol. Pharmacol. 98, 161–167. doi:10.1016/j.yrtph.2018.07.023
Liu, X., Zhang, D., Hou, P., Pan, J., Zhao, X., and Hou, B. (2018). Preparation and Characterization of Polyelectrolyte-Modified Attapulgite as Nanocontainers for Protection of Carbon Steel. J. Electrochem. Soc. 165 (No. 13), C907–C915. doi:10.1149/2.0871813jes
Lubell, W. D. (2001). Solid-Phase Organic Synthesis Edited by Kevin Burgess (Texas A & M University). Wiley Interscience: New York. 2000. Xiv + 277 Pp. $69.95. ISBN 0-471-31825-6. J. Am. Chem. Soc. 123 (27), 6744–6744283. doi:10.1021/ja004851i
Lv, L. P., Zhao, Y., Vilbrandt, N., Gallei, M., Vimalanandan, A., Rohwerder, M., et al. (2013). Redox Responsive Release of Hydrophobic Self-Healing Agents from Polyaniline Capsules. J. Am. Chem. Soc. 135 (No. 38), 14198–14205. doi:10.1021/ja405279t
Ma, C., Yang, H., Zhou, X., Wu, B., and Zhang, G. (2012). Polymeric Material for Anti-biofouling. Colloids Surf. B: Biointerfaces 100, 31–35. doi:10.1016/j.colsurfb.2012.04.045
Ma, L., Wang, J., Zhang, D., Huang, Y., Huang, L., Wang, P., et al. (2021). Dual-action Self-Healing Protective Coatings with Photothermal Responsive Corrosion Inhibitor Nanocontainers. Chem. Eng. J. 404, 127118. doi:10.1016/j.cej.2020.127118
Ma, X., Xu, L., Wang, W., Lin, Z., and Li, X. (2017). Synthesis and Characterisation of Composite Nanoparticles of Mesoporous Silica Loaded with Inhibitor for Corrosion protection of Cu-Zn alloy. Corrosion Sci. 120, 139–147. doi:10.1016/j.corsci.2017.02.004
Magin, C. M., Cooper, S. P., and Brennan, A. B. (2010). Non-toxic Antifouling Strategies. Mater. Today 13 (No. 4), 36–44. doi:10.1016/s1369-7021(10)70058-4
Maldonado, L., Quiroz-Zavala, O., and Díaz-Ballote, L. (2010). Bond between Galvanized Steel and concrete Prepared with limestone Aggregates. Anti-Corrosion Methods Mater. 57 (No. 6), 305–313. doi:10.1108/00035591011087163
Manahan, S. E. (2003). Toxicological Chemistry and Biochemistry. 3rd Edition. Boca Raton, Florida, United States: CRC Press.
Mekeridis, E. D., Kartsonakis, I. A., and Kordas, G. C. (2012). Multilayer Organic-Inorganic Coating Incorporating TiO 2 Nanocontainers Loaded with Inhibitors for Corrosion protection of AA2024-T3. Prog. Org. Coat. 73 (Nos. 2–3), 142–148. doi:10.1016/j.porgcoat.2011.10.005
Montemor, M. F., Snihirova, D. V., Taryba, M. G., Lamaka, S. V., Kartsonakis, I. A., Balaskas, A. C., et al. (2012). Evaluation of Self-Healing Ability in Protective Coatings Modified with Combinations of Layered Double Hydroxides and Cerium Molibdate Nanocontainers Filled with Corrosion Inhibitors. Electrochimica Acta 60, 31–40. doi:10.1016/j.electacta.2011.10.078
Natalio, F., André, R., Hartog, A. F., Stoll, B., Jochum, K. P., Wever, R., et al. (2012). Vanadium Pentoxide Nanoparticles Mimic Vanadium Haloperoxidases and Thwart Biofilm Formation. Nat. Nanotech 7 (8), 530–535. doi:10.1038/nnano.2012.91
Peleshanko, S., and Tsukruk, V. (2008). The Architectures and Surface Behavior of Highly Branched Molecules. Prog. Polym. Sci. (Oxford) 33 (No. 5), 523–580. doi:10.1016/j.progpolymsci.2008.01.003
Piola, R. F., Dafforn, K. A., and Johnston, E. L. (2009). The Influence of Antifouling Practices on marine Invasions. Biofouling 25 (7), 633–644. No. 912521679. doi:10.1080/08927010903063065
Protopapa, M., Kotsiri, M., Mouratidis, S., Roussis, V., Ioannou, E., and Dedos, S. G. (2019). Evaluation of Antifouling Potential and Ecotoxicity of Secondary Metabolites Derived from Red Algae of the Genus Laurencia. Mar. Drugs 17 (No. 11). doi:10.3390/md17110646
Qian, B., Michailidis, M., Bilton, M., Hobson, T., Zheng, Z., and Shchukin, D. (2019). Tannic Complexes Coated Nanocontainers for Controlled Release of Corrosion Inhibitors in Self-Healing Coatings. Electrochimica Acta 297, 1035–1041. doi:10.1016/j.electacta.2018.12.062
Qian, P. Y., Xu, Y., and Fusetani, N. (2013). Natural Products as Antifouling Compounds: Recent Progress and Future Perspectives. Biofouling 26 (2), 223–234. doi:10.1080/08927010903470815
Ramesh Saliyan, V., and Adhikari, A. V. (2008). Inhibition of Corrosion of Mild Steel in Acid media by N’-benzylidene-3- (Quinolin-4-ylthio)propanohydrazide. Bull. Mater. Sci. 31 (No. 4), 699–711. doi:10.1007/s12034-008-0111-4
Saji, S. (2019). Supramolecular Concepts and Approaches in Corrosion and Biofouling Prevention. Corrosion Rev. 37 (No. 3), 187–230. doi:10.1515/corrrev-2018-0105
Sambyal, P., Ruhi, G., Dhawan, S. K., Bisht, B. M. S., and Gairola, S. P. (2018). Enhanced Anticorrosive Properties of Tailored Poly(aniline-anisidine)/chitosan/SiO2 Composite for protection of Mild Steel in Aggressive marine Conditions. Prog. Org. Coat. 119, 203–213. doi:10.1016/j.porgcoat.2018.02.014
Saremi, M., and Yeganeh, M. (2014). Application of Mesoporous Silica Nanocontainers as Smart Host of Corrosion Inhibitor in Polypyrrole Coatings. Corrosion Sci. 86, 159–170. doi:10.1016/j.corsci.2014.05.007
Shchukina, E., and Shchukin, D. G. (2019). Nanocontainer-Based Active Systems: From Self-Healing Coatings to Thermal Energy Storage. Langmuir 35 (No. 26), 8603–8611. doi:10.1021/acs.langmuir.9b00151
Singh, N., and Turner, A. (2009). Leaching of Copper and Zinc from Spent Antifouling Paint Particles. Environ. Pollut. 157 (No. 2), 371–376. doi:10.1016/j.envpol.2008.10.003
Sonawane, S. H., Bhanvase, B. A., Jamali, A. A., Dubey, S. K., Kale, S. S., Pinjari, D. V., et al. (2012). Improved Active Anticorrosion Coatings Using Layer-By-Layer Assembled ZnO Nanocontainers with Benzotriazole. Chem. Eng. J. 189-190, 464–472. doi:10.1016/j.cej.2012.02.076
Stobie, N., Duffy, B., Mccormack, D. E., Colreavy, J., Hidalgo, M., McHale, P., et al. (2008). Prevention of Staphylococcus Epidermidis Biofilm Formation Using a Low-Temperature Processed Silver-Doped Phenyltriethoxysilane Sol-Gel Coating. Biomaterials 29, 963–969. doi:10.1016/j.biomaterials.2007.10.057
Tapeinos, C., Efthimiadou, E. K., Boukos, N., et al. (). Synthesis of pH Responsive Nanocontainers Using Poly Glycidyl Methacrylate as Template.
Tapeinos, C., Efthimiadou, E. K., Boukos, N., and Kordas, G. (2016). Sustained Release Profile of Quatro Stimuli Nanocontainers as a Multi Sensitive Vehicle Exploiting Cancer Characteristics. Colloids Surf. B: Biointerfaces 148, 95–103. doi:10.1016/j.colsurfb.2016.08.019
Turner, A. (2010). Marine Pollution from Antifouling Paint Particles. Mar. Pollut. Bull. 60 (No. 2), 159–171. doi:10.1016/j.marpolbul.2009.12.004
Wang, M., Liu, M., and Fu, J. (2015). An Intelligent Anticorrosion Coating Based on pH-Responsive Smart Nanocontainers Fabricated via a Facile Method for protection of Carbon Steel. J. Mater. Chem. A 3 (No. 12), 6423–6431. doi:10.1039/c5ta00417a
Wilson, A. D., Nicholson, J. W., and Prosser, H. J. (1987). Surface Coatings-2. New York: Elsevier Science, 1–229.
Xu, J.-B., Cao, Y.-Q., Fang, L., and Hu, J.-M. (2018). A One-step Preparation of Inhibitor-Loaded Silica Nanocontainers for Self-Healing Coatings. Corrosion Sci. 140 (No. February), 349–362. doi:10.1016/j.corsci.2018.05.030
Xu, L. C., and Siedlecki, C. A. (2012). Submicron-textured Biomaterial Surface Reduces Staphylococcal Bacterial Adhesion and Biofilm Formation. Acta Biomater. 8 (No. 1), 72–81. doi:10.1016/j.actbio.2011.08.009
Yabuki, A., Shiraiwa, T., and Fathona, I. W. (2016). pH-controlled Self-Healing Polymer Coatings with Cellulose Nanofibers Providing an Effective Release of Corrosion Inhibitor. Corrosion Sci. 103, 117–123. doi:10.1016/j.corsci.2015.11.015
Yang, Y. J., Tao, X., Hou, Q., Ma, Y., Chen, X. L., and Chen, J. F. (2010). Mesoporous Silica Nanotubes Coated with Multilayered Polyelectrolytes for pH-Controlled Drug Release. Acta Biomater. 6 (No. 8), 3092–3100. doi:10.1016/j.actbio.2010.02.042
Yeganeh, M., Asadi, N., Omidi, M., and Mahdavian, M. (2019). An Investigation on the Corrosion Behavior of the Epoxy Coating Embedded with Mesoporous Silica Nanocontainer Loaded by Sulfamethazine Inhibitor. Prog. Org. Coat. 128 (No. November), 75–81. doi:10.1016/j.porgcoat.2018.12.022
Yeomans, S. R. (1994). Performance of Black, Galvanized, and Epoxy-Coated Reinforcing Steels in Chloride-Contaminated concrete. Corrosion 50 (No. 1), 72–81. doi:10.5006/1.3293496
Zhang, X. G. (1996). Corrosion and Electrochemistry of Zinc. New York: Springer Science+ Business Media, 1–474.
Keywords: antifouling, nanocontainers, corrosion, self-healing, biocides
Citation: Kordas G (2022) Nanocontainers Against Biofouling and Corrosion Degradation of Materials: A Short Review With Prospects. Front. Nanotechnol. 4:813908. doi: 10.3389/fnano.2022.813908
Received: 12 November 2021; Accepted: 21 February 2022;
Published: 23 March 2022.
Edited by:
Jin-Long Yang, Shanghai Ocean University, ChinaReviewed by:
Mahmood Aliofkhazraei, Tarbiat Modares University, IranFei Ye, Royal Institute of Technology, Sweden
Copyright © 2022 Kordas. This is an open-access article distributed under the terms of the Creative Commons Attribution License (CC BY). The use, distribution or reproduction in other forums is permitted, provided the original author(s) and the copyright owner(s) are credited and that the original publication in this journal is cited, in accordance with accepted academic practice. No use, distribution or reproduction is permitted which does not comply with these terms.
*Correspondence: George Kordas, Z2Nrb3JkYXNAZ21haWwuY29t