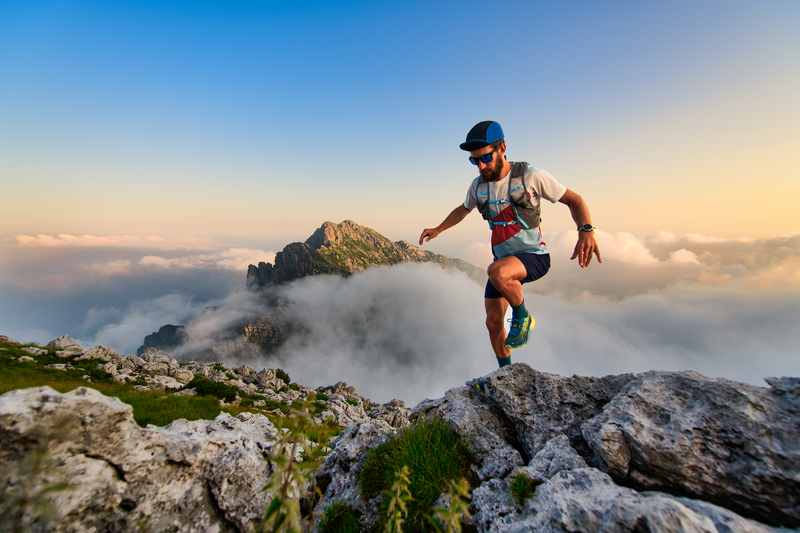
94% of researchers rate our articles as excellent or good
Learn more about the work of our research integrity team to safeguard the quality of each article we publish.
Find out more
ORIGINAL RESEARCH article
Front. Nanotechnol. , 16 January 2023
Sec. Nanomaterials
Volume 4 - 2022 | https://doi.org/10.3389/fnano.2022.1096267
This article is part of the Research Topic The Molecular Underpinnings of Nanoscale Semiconductor Synthesis View all 5 articles
The use of microfluidics in chemical synthesis is topical due to the potential to improve reproducibility and the ability promptly interrogate a wide range of reaction parameters, the latter of which is necessary for the training of artificial intelligence (AI) algorithms. Applying microfluidic techniques to semiconductor nanocrystals, or quantum dots (QDs), is challenging due to the need for a high-temperature nucleation event followed by particle growth at lower temperatures. Such a high-temperature gradient can be realized using complex, segmented microfluidic reactor designs, which represents an engineering approach. Here, an alternative chemical approach is demonstrated using the cluster seed method of nanoparticle synthesis in a simple microfluidic reactor system. This enables quantum dot nucleation at lower temperatures due to the presence of molecular organometallic compounds (NMe4)4[Cd10Se4(SPh)16] and (NMe4)4[Zn10Se4(SPh)16]. This integration of cluster seeding with microfluidics affords a new mechanism to tailor the reaction conditions for optimizing yields and tuning product properties.
The development of semiconductor nanoscience has been driven by the materials’ efficacy in several applications such as photonics (Owen and Brus, 2017), electronics (Koh et al., 2011), and biomedical imaging (Lim et al., 2015). Semiconductor nanoparticles are generally referred to as “quantum dots” (QDs) owing to their size-dependent optical and electronic properties that result from the spatial confinement of excitons (Alivisatos, 1996). The rapid-injection method is the most common protocol used to synthesize a variety of semiconductor QDs (Murray et al., 1993). Although mechanically simple, this procedure separates high-temperature nucleation from the lower temperature growth phase (∼200°C) due to the sudden cooling of the hot coordinating solvent (>300°C) after the rapid introduction of room temperature precursors (Murray et al., 2001; Qu et al., 2004). Disadvantages include reproducibility issues that result from inconsistent reaction conditions as well as oversensitivity to solvent and precursor purities (Wang et al., 2008). Flow chemistry using microfluidics is an alternative technique that may improve reproducibility since greater control of the reaction parameters enables consistency in conditions (Nette et al., 2020). Furthermore, the application of artificial intelligence (AI) in chemical synthesis is best realized using a microfluidic platform due to the ability to vary reaction conditions quickly and accurately (Orimoto et al., 2012; Maceiczyk and deMello, 2014). This facilitates the creation of large datasets that connect parameters to products, which is necessary to “train” AI algorithms. Although most frequently applied in organic synthesis, this technology non-etheless has attracted significant interest for solid-state semiconductor nanoparticles (Chan et al., 2003; Yen et al., 2003; Voznyy et al., 2019), plasmonic materials (Nette et al., 2020), and perovskites (Lignos et al., 2016).
Applying microfluidic technology to QD synthesis is problematic due to the need for a high-temperature nucleation event followed by a cooler growth period. Currently, this is achieved through inserting insulating blocks or separating stages, which introduces greater complexity to the microfluidic device (Pan et al., 2013; Baek et al., 2018). However, our alternative chemistry-based approach is to negate the initial high-temperature nucleation altogether to enable the use of simplified microfluidic reactor designs. To this end, the cluster seed method of nanoparticle synthesis is attractive as it does not require high temperatures (Jawaid et al., 2013) due to the presence of a nucleation catalyst, see Scheme 1. This spurred our examination into integrating cluster-seeded synthesis within a continuous flow reactor system. In this report, CdSe QDs were synthesized with and without (NMe4)4[Cd10Se4(SPh)16] and (NMe4)4[Zn10Se4(SPh)16] nucleating catalysts while varying other conditions such as reaction time, temperature, and catalyst concentrations in the one- or two-stage reactor system shown in Figure 1. The nanocrystals were synthesized at significantly lower temperatures using the cluster seed method; furthermore, the concentration of clusters enables greater flexibility in the reaction parameter set for developing optimal conditions.
FIGURE 1. A scheme of a microfluidic reactor and CdSe QD absorption spectra with and without (NMe4)4[Cd10Se4(SPh)16] cluster seeds. Single-stage temperatures were (A) 100°C and (B) 120°C.
Cadmium nitrate hexahydrate (Cd(NO3)2·6H2O, 99.99%), cadmium acetate dihydrate (Cd(CH3COO)2·2H2O, 98.0+%), anhydrous acetonitrile (CH3CN, 99.8%), anhydrous N,N-dimethylformamide (DMF, 99.8%), chloroform (CHCl3, 99.8%), diethyl ether ((CH3CH2)2O, 99.0+%), technical grade diphenyl ether ((C6H5)2O), tetramethylammonium chloride (C4H12NCl), thiophenol (C6H5SH, 97%) and triethylamine ((C2H5)3N, 98%) and were purchased from Sigma-Aldrich. 2-hexyldecanoic acid (C16H32O2, 98.0+%) was purchased from TCI America. Selenium shot (99.99%) and powder (99.5%), and tri-n-octylphosphine (TOP, 97%) were purchased from Strem Chemicals. Technical grade methanol was purchased from Fischer. Anhydrous and air-sensitive solvents were stored in a glove box. Cadmium acetate was recrystallized from hot acetic acid, while all chemicals were used without further purification.
Precursors were injected through the microfluidic reactor system using a PHD 2000 syringe pump from Harvard Apparatus. The reagents were first mixed through PFA high-pressure tubing (0.020″ ID) that was connected to polyimide tubing (0.024″ ID) wrapped around one (single-stage synthesis) or two (dual-stage synthesis) toroidal aluminum heating block(s). The polyimide reaction tubing includes both the nucleation stage (30″, 240 µL volume) and a growth stage (16″, 80 µL volume). The material was chosen due to its thermal stability (−269°C → 400 °C according to the manufacturer, Nordson Medical, PN# 141–0036). Each metal block was machined in-house; the temperature was set using a controller that regulated power coupling from a variable transformer to a TUTCO cartridge heater. Tubing connectors were PEEK/stainless steel collect-lock system ferrule, ETFE flat-bottom fittings, PEEK Y-connectors, polypropylene union body connectors, and ETFE shut-off valves from IDEX Health and Science and Nordson Medical. Plastic Luer-lock sterile syringes were purchased from Norm-Ject.
The organometallic clusters used in this study have been prepared using literature procedures. Characterization data are provided in the Supplementary Material, Supplementary Figure S1.
The cluster was synthesized according to the procedure of (Dance et al., 1984).
The cluster was synthesized according to the procedure of (Dance et al., 1984).
(NMe4)4[Cd10Se4(SPh)16] clusters (10 mg) were dissolved in 10 mL DMF. Samples were monitored by UV/Vis spectroscopy for ∼7 h to quantify aggregation into magic size (CdSe)32. Another portion was applied into the microfluidic reaction system via PFA (0.03″ ID, 16″ length) tubing at a 3 mL/h flow rate over a 25°C → 100°C before collection. All samples were diluted 4× before optical characterization, see Supplementary Figure S2.
1M TOPSe was prepared in a glove box by mixing selenium shot (0.7896 g, 10 mmol) in 10 mL of TOP until the Se dissolved (∼4 h). Cd(2-hexyldecaonate)2 was prepared by dissolving cadmium acetate (1.0 mmol) and 2-hexyldecanoic acid (5 mmol) in 10 mL diphenyl ether in a three-neck round bottom flask under an inert gas using a Schlenk line. The reaction mixture was stirred under vacuum at 100°C for 2 h. The flask was backfilled with N2 gas and heated to 250°C until the presence of a yellow color was detected. The mixture was cooled slowly to room temperature.
Cadmium hexyldecanoate and TOPSe were added to diphenyl ether to create 0.1 M solutions that were degassed and loaded into individual syringes. Various cluster seeds (0 µmol → 4 µmol) were dissolved in anhydrous DMF (0 µL → 100 µL) and were added to the cadmium precursor solution. The flow rates were set to 2 mL → 20 mL per hour. Nucleation and growth temperatures varied over 100°C → 200°C and 150°C → 220°C, respectively. The products were collected in hexane for characterization.
Absorption spectra (optical and IR) were collected with a Bio 300 Cary spectrophotometer and a Thermo Scientific Nicolet iS 5, respectively. Fluorescence spectra were recorded on a Horiba Jobin Yvon FluoroMax-4 spectrofluorometer using 400 nm excitation, while time resolved PL was characterized with an Edinburgh FLS1000 PL spectrometer. 1H NMR spectra were characterized on a Bruker DPX 400 MHz. PXRD diffractograms were collected using a D8 Advance ECO Bruker XRD diffractometer with Cu Kα (λ = 1.54056 Å) radiation. The sizes and concentrations of CdSe QD products were estimated from previously published regressions (Yu et al., 2003; Jasieniak et al., 2009). The regression of Jasieniak et al. was used for large bandgap QDs (<450 nm) due to greater accuracy when applied to smaller CdSe nanoparticles. Such analyses are only possible if a well-defined first exciton absorption feature is observed.
The rapid injection method for semiconductor QD synthesis produces homogeneous materials due to the ability to separate nucleation from growth stages. This approach is most amenable for batch synthesis and is difficult to reproduce on a simple microfluidic platform, which has instigated the development of complex reactor systems. The use of molecular clusters in the synthesis of nanomaterials represents an alternative approach. There is a growing number of demonstrations of the use of well-defined molecular clusters in nanomaterial synthesis (Friedfeld et al., 2017). Early research demonstrated their use as singular reagents to deliver both the metal and chalcogenide elements of the semiconductor stoichiometry (“single-source precursor”) and minimize the handling of especially toxic chemicals (Trindade and O’Brien, 1996; Trindade et al., 1997; Cumberland et al., 2002). “Magic-sized” clusters have been used as precursors for semiconductor quantum dots (Friedfeld et al., 2019) as well as for nanorods and other shapes (Peng and Peng, 2002; Jiang and Kelley, 2010). A radically different approach was introduced by Pickett, whereby CdSe QDs were prepared in the presence of catalytic quantities of (NMe4)4[Cd10Se4(SPh)16] (Pickett, 2006). In this seeded method, the organometallic clusters were not reagents, rather they served as nucleation sites for QDs that grew from Cd(2-hexyldecanoate)2 and TOPSe precursors. The cluster-seeded method was designed to scale up the production of nanomaterials in a batch process, and later our group and others found that seeding could be exploited to prepare doped nanomaterials. Specifically, several types of guest/host systems were produced using gold or copper-containing organometallic compounds, resulting in stoichiometrically doped nanoparticles (Jawaid et al., 2013; Hassan et al., 2017; Hassan et al., 2018; Santiago-González et al., 2017; Capitani et al., 2019). This process subverts the known issues of dopant Poissonain statistical inhomogeneity (Meulenberg et al., 2004; Chikan, 2011; Zheng and Strouse, 2011; Zheng et al., 2011) since each QD contains the same number of guest ions as the nucleating cluster due to the 1:1 QD product to cluster catalyst relationship.
The cluster seed approach was applied to the synthesis of CdSe QDs in the microfluidic system shown in Figure 1 to evaluate the dependence on product quality (stoichiometry, average size, and homogeneity) on the reaction parameter space (temperature, time, and concentration). QD sizes and concentrations were estimated from absorption spectra using previously published regressions. The main goal of synthesizing QDs at low temperatures (100°C) in a single-stage microfluidic reactor was successful as shown in Figure 1A, where the presence of (NMe4)4[Cd10Se4(SPh)16] resulted in products with typical CdSe nanoparticle absorption spectra. The first absorption feature at 389 nm reveals that the QDs are small, estimated to be between 1.4 nm and 1.5 nm. Larger 1.9 nm diameter products are prepared at 120°C as shown in Figure 1B. Quantum dot features are absent with no catalysts are used, which was expected given the low temperatures employed.
Figure 2 shows the normalized absorption spectra of cluster seeded CdSe QDs as a function of temperature in a single-stage reactor, from 100°C → 200°C. The red shift in absorptions (401 nm → 525 nm) is due to an increase in size from 1.5 nm → 2.8 nm (see also Supplementary Figure S3 and Supplementary Table S1). This indicates that the QDs both nucleate and grow despite the modest residency time in the single-stage reactor. Furthermore, the size distribution appears sub-optimal and does not improve at higher temperatures, suggesting that the QDs are not experiencing focused growth. The emission was also found to be broad; however this is not directly indicative of the size distribution as the photoluminescence mostly originates from deep trap emission as shown in Supplementary Figure S3. An attempt was made to enhance monodispersity by synthesis through a temperature gradient from low to high; note the reversal from convention. CdSe QDs were nucleated at 120°C in a first stage reactor, producing a modest size product (1.6 nm diameter) with a first absorption feature at 419 nm (Supplementary Figure S3). These materials were connected to a second reactor to grow further over a 120°C → 180°C temperature range. New QDs were not nucleated regardless of the temperature as the concentrations of products were invariant. Figure 3 shows that the first absorption features red-shifted from 424 nm → 478 nm due to the increase in QD size from 1.7 nm → 2.2 nm (Supplementary Table S2) as the second stage reactor temperature was increased. While conventional wisdom suggests these are good conditions for focused growth, in fact, there was no noticeable improvement in monodispersity as revealed by the absence of sharp exciton features in Figure 3. Furthermore, adding a growth stage does not provide the same level of size tunability compared to that realized by simply varying the first stage nucleating reactor temperature (Figure 2).
FIGURE 2. The normalized absorption of (NMe4)4[Cd10Se4(SPh)16] cluster seeded CdSe QDs nucleated in a single-stage reactor at various temperatures.
FIGURE 3. The normalized absorption of (NMe4)4[Cd10Se4(SPh)16] cluster seeded CdSe QDs nucleated at 120°C and grown in a second stage reactor at various temperatures.
These results demonstrate that the cluster seed method enables the synthesis of CdSe QDs in a microfluidic system at significantly lower temperatures than in conventional rapid injection and in other examples of microfluidic synthesis. To provide contrast, the synthesis was examined under identical reaction conditions without clusters; characterization data are shown in Supplementary Figures S4, S5 in the Supplementary Material. A minimum reactor temperature of 150°C was necessary to observe some absorption, although definitive excitonic features are not evident from reactor temperatures less than 180°C. When the temperature was high enough for spontaneous nucleation (>150°C), the nanomaterials were larger than that observed from cluster seeded synthesis albeit at a lower concentration. This is likely due to the formation of a smaller number of nuclei compared to the quantity of clusters added, resulting in a higher precursor-to-QD ratio and larger products. Regardless of the temperature, the absorption features are indicative of materials with poor size distributions to the extent that characterization of the bandgap is problematic. To summarize, the presence of cluster seeds has a profound effect on the size, quantity, and quality of products synthesized in a microfluidic system. And although cluster seeded QDs do not have ideal monodispersity, the results are superior to preparations without cluster catalysts.
Seeding was investigated using (NMe4)4[Zn10Se4(SPh)16] to study the flexibility of the method concerning the identity of the cluster. The absorption spectrum of the materials prepared using the zinc selenide cluster is shown in Figure 4. The results reveal successful CdSe QD nucleation; furthermore, the QD size as characterized by the first absorption feature (462 nm) is nearly identical to that realized from the use of (NMe4)4[Cd10Se4(SPh)16] seeds (460 nm, Supplementary Figure S4B). However, the excitonic features are not as distinct, suggesting less homogeneity.
FIGURE 4. CdSe QD absorption spectra with and without (NMe4)4[Zn10Se4(SPh)16] cluster seeds. The single-stage reactor temperature was set to 150°C.
Cluster concentrations were varied over a ∼70 μM → 350 μM range in a single-stage reactor at two temperatures (100°C and 120°C) to study the effect on QD product properties. The effect of the addition of clusters is to produce smaller QDs as evident from product spectra (Figure 5), where it can be seen that the absorptions blue shift with increasing cluster concentration regardless of the temperature. This is consistent with our previous proposal that the application of too many nucleating seeds lowers the precursor activity such that the ability of the products to gain additional mass is impaired (Jawaid et al., 2013). These data also reveal a linear increase in absorptivity and a corresponding rise in the number of products as a function of cluster concentration (Supplementary Figure S6). It is tempting to ascribe the effect as akin to simply adding more reagents; however, the mass range of (NMe4)4[Cd10Se4(SPh)16] added varies from 5 mg → 20 mg in these experiments (Supplementary Table S3). This is too low to account for a near tripling of the product yields as seen in a regression of product vs. cluster seed concentrations shown in Supplementary Figure S6. What is perplexing in these data is the fact that the regression in Supplementary Figure S6 has a non-zero y-intercept, which means that a minimum ∼55 μM catalyst concentration is necessary to observe product seeding. This threshold behavior suggests a loss of catalytic activity that can occur due to a variety of reasons including aggregation of the clusters as discussed in Section 3.3. Poisoning of the catalysts may also play a role, (Norrish and Russell, 1947; Kumar et al., 2014), especially by oxygen as we cannot vigorously prevent O2 diffusion through the plastic components of the microfluidic reactor.
FIGURE 5. The effect of increasing (NMe4)4[Cd10Se4(SPh)16] cluster seed concentration on the absorption spectra of CdSe QDs nucleated and grown in a single-stage reactor at (A) 100°C and (B) 120°C.
While the non-zero y-intercept of the regression of Supplementary Figure S6 can be attributed to sensible factors that may be addressed with additional engineering controls, the fact that the slope is less than 1:1 is problematic. This is because the number of clusters and the number of QDs produced should equate in a seeded mechanism, as was observed in previous studies (Jawaid et al., 2013). An examination of all the data collected in this study reveals that, while the products are responsive to seed concentration, the effect is non-stoichiometric (generally the product concentrations are ∼15% of that of the cluster). This could be the result of the limited synthesis time (<7 min), and to investigate the concentration of QD as a function of flow rate was measured (Supplementary Figure S7). These data reveal that increasing the residency time of precursors in a single-stage reactor enhanced the product population by ∼50%. Other potential contributing factors include Ostwald ripening which consumes the smaller QD populations. That the products are ripening is evident from the absorption spectra that reveal sub-optimal size distributions. While these issues are problematic, they cannot fully account for the discrepancy between catalysts and products. Further confounding the issue is the fact that one dataset was collected that revealed a near quantitative agreement between product and cluster concentration in one of our temperature-dependent studies, see Supplementary Figures S8, S9. The correlations between reagent and product were equal up to a reactor temperature of 200°C, whereupon the number of QDs rose above the line as seen in Supplementary Figure S8. Given the observation of spontaneous nucleation >150°C for unseeded growth, a simple explanation is that both spontaneous and seeded nucleation events occur and the resulting QD concentration at 200°C is a sum of both.
These issues are likely resolved by the ability of (NMe4)4[Cd10Se4(SPh)16] clusters to act as both seeds and reagents given that these species have been used as precursors for CdSe QDs albeit at much higher temperatures (Cumberland et al., 2002). As a result, the time and temperature dependence to the evolution of the neat clusters in solution was investigated, which revealed a rich cache of chemical dynamics as discussed below.
The inconsistent yields on a per catalyst basis prompted an investigation into the mechanism of cluster seeding. Our working hypothesis is that partial decomposition via ligand dissociation of the cluster instigates dynamics that result in nucleation of a single QD; furthermore, this must occur at a temperature lower than that necessary for spontaneous nucleation. This mechanism was proposed earlier to describe the evolution of (NMe4)4[Cd10S4(SPh)16] into higher order clusters and (NMe4)4[Cd10Se4(SPh)16] (acting as reagents) into CdSe QDs by Bendova and Cumberland, respectively (Bendova et al., 2010; Cumberland et al., 2002). To investigate, the changes in the absorption of (NMe4)4[Cd10Se4(SPh)16] clusters in DMF were measured over time with and without thermolysis in the microfluidic system over a 25°C → 140°C temperature range. It was found that a ∼400 nm feature slowly appears at room temperature as shown in Supplementary Figure S2A. This is likely magic sized (CdSe)32 (Landes et al., 2001). This transformation eventually ceases; however, at temperatures >50°C additional conversion is observed as evident from the growth of the ∼400 nm absorption as shown in Supplementary Figure S2B. Past 100°C the absorptions’ red shifting and the loss of less well-defined excitonic features can be attributed to Ostwald ripening.
In most preparations some time would elapse before the clusters were fully dissolved in DMF. As a result we attribute the issues with the observation of a consistent 1:1 QD to catalyst ratio to the cluster’s propensity to aggregate before injection into the flow system. In the future, we hope to minimize this by enhancing the cluster solubility, perhaps through variation of the counterion. And while these results may tempt the employment of clusters with greater stability, this may be counterproductive as we hypothesize that ligand displacement of a ligand, or perhaps via the loss of the apical [Cd(SPh)3]–, is essential to the cluster seeding process; further investigations will examine this potential.
A simple microfluidic device was used to nucleate and grow semiconductor quantum dots at low temperatures using the cluster-seeded method. Multiple catalysts (NMe4)4[Cd10Se4(SPh)16] and (NMe4)4[Zn10Se4(SPh)16] were successfully employed. The nucleation and growth temperatures, cluster content, and reaction times were studied, the results from which were consistent with mechanistic expectations and previous demonstrations although the expected 1:1 relationship between cluster seed and QD concentrations was difficult to reproduce due to issues with cluster aggregation. Future work will examine modification of the cluster properties and the use of more robust engineering controls to minimize these discrepancies. Overall, our chemical approach to address the need for high-temperature nucleation followed by cooler growth was successful, which allowed us to avoid the use of a complex reactor system. It is hoped that this approach can enhance the parameter space via cluster identity and concentration for future research on the microfluidic synthesis of inorganic nanomaterials.
Copyright © 2022 Kim, Tomczak, Chandrasiri, Pálmai, Ghaznavi, Gritsenko, Xu, and Snee. This is an open-access article distributed under the terms of the Creative Commons Attribution License (CC BY). The use, distribution or reproduction in other forums is permitted, provided the original author(s) and the copyright owner(s) are credited and that the original publication in this journal is cited, in accordance with accepted academic practice. No use, distribution or reproduction is permitted which does not comply with these terms.
The original contributions presented in the study are included in the article/Supplementary Materials, further inquiries can be directed to the corresponding author.
EK, AG, DG, and JX designed the microfluidic reactor, EK performed QD synthesis and characterization and prepared the manuscript. HC assisted with characterization and KT synthesized and characterized cluster seeds and MP assisted with cluster seeds synthesis. PS assisted with data analysis and manuscript preparation.
This work was supported by the University of Illinois at Chicago. Acknowledgment is made to the donors of the American Chemical Society Petroleum Research Fund for partial support of this research (60875ND10).
We thank Prof. A. Jean-Luc Ayitou for collecting time resolved emission data.
The authors declare that the research was conducted in the absence of any commercial or financial relationships that could be construed as a potential conflict of interest.
All claims expressed in this article are solely those of the authors and do not necessarily represent those of their affiliated organizations, or those of the publisher, the editors and the reviewers. Any product that may be evaluated in this article, or claim that may be made by its manufacturer, is not guaranteed or endorsed by the publisher.
The Supplementary Material for this article can be found online at: https://www.frontiersin.org/articles/10.3389/fnano.2022.1096267/full#supplementary-material
Alivisatos, A. P. (1996). Perspectives on the physical chemistry of semiconductor nanocrystals. J. Phys. Chem. 100, 13226–13239. doi:10.1021/jp9535506
Baek, J., Shen, Y., Lignos, I., Bawendi, M. G., and Jensen, K. F. (2018). Multistage microfluidic platform for the continuous synthesis of III–V core/shell quantum dots. Angew. Chem. Int. Ed. 57, 10915–10918. doi:10.1002/anie.201805264
Bendova, M., Puchberger, M., Pabisch, S., Peterlik, H., and Schubert, U. (2010). Studies on the formation of CdS nanoparticles from solutions of (NMe4)4[Cd10S4(SPh)16]. Eur. J. Inorg. Chem. 2010, 2266–2275. doi:10.1002/ejic.201000080
Capitani, C., Pinchetti, V., Gariano, G., Santiago-Gonzalez, B., Santambrogio, C., Campione, M., et al. (2019). Quantized electronic doping towards atomically controlled “charge-engineered” semiconductor nanocrystals. Nano Lett. 19, 1307–1317. doi:10.1021/acs.nanolett.8b04904
Chan, E., Mathies, R., and Alivisatos, A. P. (2003). Size-controlled growth of CdSe nanocrystals in microfluidic reactors. Nano Lett. 3, 199–201. doi:10.1021/nl0259481
Chikan, V. (2011). Challenges and prospects of electronic doping of colloidal quantum dots: Case study of CdSe. J. Phys. Chem. Lett. 2, 2783–2789. doi:10.1021/jz2012325
Cumberland, S. L., Hanif, K. M., Javier, A., Khitrov, G. A., Strouse, G. F., Woessner, S. M., et al. (2002). Inorganic clusters as single-source precursors for preparation of CdSe, ZnSe, and CdSe/ZnS nanomaterials. Chem. Mat. 14, 1576–1584. doi:10.1021/cm010709k
Dance, I. G., Choy, A., and Scudder, M. L. (1984). Synthesis, properties, and molecular and crystal-structures of (Me4N)4[E4M10(SPh)16] (E = S, Se; M = Zn, Cd): Molecular supertetrahedral fragments of the cubic metal chalcogenide lattice. J. Am. Chem. Soc. 106, 6285–6295. doi:10.1021/ja00333a030
Friedfeld, M. R., Johnson, D. A., and Cossairt, B. M. (2019). Conversion of InP clusters to quantum dots. Inorg. Chem. 58, 803–810. doi:10.1021/acs.inorgchem.8b02945
Friedfeld, M. R., Stein, J. L., and Cossairt, B. M. (2017). Main-group-semiconductor cluster molecules as synthetic intermediates to nanostructures. Inorg. Chem. 56, 8689–8697. doi:10.1021/acs.inorgchem.7b00291
Hassan, A., Zhang, X., Liu, C., and Snee, P. T. (2018). Electronic structure and dynamics of copper-doped indium phosphide nanocrystals studied with time-resolved X-ray absorption and large-scale DFT calculations. J. Phys. Chem. C 122, 11145–11151. doi:10.1021/acs.jpcc.8b02124
Hassan, A., Zhang, X., Liu, X., Rowland, C. E., Jawaid, A. M., Chattopadhyay, S., et al. (2017). Charge carriers modulate the bonding of semiconductor nanoparticle dopants as revealed by time-resolved X-ray spectroscopy. ACS Nano 11, 10070–10076. doi:10.1021/acsnano.7b04414
Jasieniak, J., Smith, L., van Embden, J., Mulvaney, P., and Califano, M. (2009). Re-Examination of the size-dependent absorption properties of CdSe quantum dots. J. Phys. Chem. C 113, 19468–19474. doi:10.1021/jp906827m
Jawaid, A. M., Chattopadhyay, S., Wink, D. J., Page, L. E., and Snee, P. T. (2013). Cluster-seeded synthesis of doped CdSe:Cu 4 quantum dots. ACS Nano 7, 3190–3197. doi:10.1021/nn305697q
Jiang, Z.-J., and Kelley, D. F. (2010). Role of magic-sized clusters in the synthesis of CdSe nanorods. ACS Nano 4, 1561–1572. doi:10.1021/nn100076f
Koh, W., Saudari, S. R., Fafarman, A. T., Kagan, C. R., and Murray, C. B. (2011). Thiocyanate-capped PbS nanocubes: Ambipolar transport enables quantum dot based circuits on a flexible substrate. Nano Lett. 11, 4764–4767. doi:10.1021/nl202578g
Kumar, M., Hammond, G. B., and Xu, B. (2014). Cationic gold catalyst poisoning and reactivation. Org. Lett. 16, 3452–3455. doi:10.1021/ol501663f
Landes, C., Braun, M., Burda, C., and El-Sayed, M. A. (2001). Observation of large changes in the band gap absorption energy of small CdSe nanoparticles induced by the adsorption of a strong hole acceptor. Nano Lett. 1, 667–670. doi:10.1021/nl015619t
Lignos, I., Stavrakis, S., Nedelcu, G., Protesescu, L., deMello, A. J., and Kovalenko, M. V. (2016). Synthesis of cesium lead halide perovskite nanocrystals in a droplet-based microfluidic platform: Fast parametric space mapping. Nano Lett. 16, 1869–1877. doi:10.1021/acs.nanolett.5b04981
Lim, S. J., Zahid, M. U., Le, P., Ma, L., Entenberg, D., Harney, A. S., et al. (2015). Brightness-equalized quantum dots. Nat. Commun. 6, 8210. doi:10.1038/ncomms9210
Maceiczyk, R. M., and deMello, A. J. (2014). Fast and reliable metamodeling of complex reaction spaces using universal kriging. J. Phys. Chem. C 118, 20026–20033. doi:10.1021/jp506259k
Meulenberg, R. W., van Buuren, T., Hanif, K. M., Willey, T. M., Strouse, G. F., and Terminello, L. J. (2004). Structure and composition of Cu-doped CdSe nanocrystals using soft X-ray absorption spectroscopy. Nano Lett. 4, 2277–2285. doi:10.1021/nl048738s
Murray, C. B., Norris, D. J., and Bawendi, M. G. (1993). Synthesis and characterization of nearly monodisperse CdE (E = sulfur, selenium, tellurium) semiconductor nanocrystallites. J. Am. Chem. Soc. 115, 8706–8715. doi:10.1021/ja00072a025
Murray, C. B., Sun, S. H., Gaschler, W., Doyle, H., Betley, T. A., and Kagan, C. R. (2001). Colloidal synthesis of nanocrystals and nanocrystal superlattices. IBM J. Res. Dev. 45, 47–56. doi:10.1147/rd.451.0047
Nette, J., Howes, P. D., and deMello, A. J. (2020). Microfluidic synthesis of luminescent and plasmonic nanoparticles: Fast, efficient, and data-rich. Adv. Mat. Technol. 5, 2000060. doi:10.1002/admt.202000060
Norrish, R. G. W., and Russell, K. E. (1947). Friedel-crafts catalysts in polymerization: Kinetic measurements and the role of water. Nature 160, 543–544. doi:10.1038/160543a0
Orimoto, Y., Watanabe, K., Yamashita, K., Uehara, M., Nakamura, H., Furuya, T., et al. (2012). Application of artificial neural networks to rapid data analysis in combinatorial nanoparticle syntheses. J. Phys. Chem. C 116, 17885–17896. doi:10.1021/jp3031122
Owen, J., and Brus, L. (2017). Chemical synthesis and luminescence applications of colloidal semiconductor quantum dots. J. Am. Chem. Soc. 139, 10939–10943. doi:10.1021/jacs.7b05267
Pan, J., El-Ballouli, A. O., Rollny, L., Voznyy, O., Burlakov, V. M., Goriely, A., et al. (2013). Automated synthesis of photovoltaic-quality colloidal quantum dots using separate nucleation and growth stages. ACS Nano 7, 10158–10166. doi:10.1021/nn404397d
Peng, Z. A., and Peng, X. (2002). Nearly monodisperse and shape-controlled CdSe nanocrystals via alternative routes: Nucleation and growth. J. Am. Chem. Soc. 124, 3343–3353. doi:10.1021/ja0173167
Pickett, N. (2006). (Nanoco technologies limited, GB) controlled preparation of nanoparticle materials. U.S. Patent 7,867,556.
Qu, L., Yu, W. W., and Peng, X. (2004). In situ observation of the nucleation and growth of CdSe nanocrystals. Nano Lett. 4, 465–469. doi:10.1021/nl035211r
Santiago-González, B., Monguzzi, A., Pinchetti, V., Casu, A., Prato, M., Lorenzi, R., et al. (2017). Quantized” doping of individual colloidal nanocrystals using size-focused metal quantum clusters. ACS Nano 11, 6233–6242. doi:10.1021/acsnano.7b02369
Trindade, T., and O’Brien, P. (1996). A single source approach to the synthesis of CdSe nanocrystallites. Adv. Mat. 8, 161–163. doi:10.1002/adma.19960080214
Trindade, T., O’Brien, P., and Zhang, X. (1997). Synthesis of CdS and CdSe Nanocrystallites Using a Novel Single-Molecule Precursors Approach. Chem. Mat. 9, 523–530. doi:10.1021/cm960363r
Voznyy, O., Levina, L., Fan, J. Z., Askerka, M., Jain, A., Choi, M.-J., et al. (2019). Machine learning accelerates discovery of optimal colloidal quantum dot synthesis. ACS Nano 13, 11122–11128. doi:10.1021/acsnano.9b03864
Wang, F., Tang, R., and Buhro, W. E. (2008). The trouble with TOPO; identification of adventitious impurities beneficial to the growth of cadmium selenide quantum dots, rods, and wires. Nano Lett. 8, 3521–3524. doi:10.1021/nl801692g
Yen, B. K. H., Stott, N. E., Jensen, K. F., and Bawendi, M. G. (2003). A continuous-flow microcapillary reactor for the preparation of a size series of CdSe nanocrystals. Adv. Mat. 15, 1858–1862. doi:10.1002/adma.200305162
Yu, W. W., Qu, L., Guo, W., and Peng, X. (2003). Experimental determination of the extinction coefficient of CdTe, CdSe, and CdS nanocrystals. Chem. Mat. 15, 2854–2860. doi:10.1021/cm034081k
Zheng, W. W., and Strouse, G. F. (2011). Involvement of carriers in the size-dependent magnetic exchange for Mn:CdSe quantum dots. J. Am. Chem. Soc. 133, 7482–7489. doi:10.1021/ja200508e
Keywords: microfluidic, cadmium selenide, quantum dot (QD), nucleation, artificial intelligence (AI)
Citation: Kim EB, Tomczak KM, Chandrasiri HB, Pálmai M, Ghaznavi A, Gritsenko D, Xu J and Snee PT (2023) Nucleation control of quantum dot synthesis in a microfluidic continuous flow reactor. Front. Nanotechnol. 4:1096267. doi: 10.3389/fnano.2022.1096267
Received: 11 November 2022; Accepted: 06 December 2022;
Published: 16 January 2023.
Edited by:
Brandi Cossairt, University of Washington, United StatesReviewed by:
James McBride, Vanderbilt University, United StatesCopyright © 2023 Kim, Tomczak, Chandrasiri, Pálmai, Ghaznavi, Gritsenko, Xu and Snee. This is an open-access article distributed under the terms of the Creative Commons Attribution License (CC BY). The use, distribution or reproduction in other forums is permitted, provided the original author(s) and the copyright owner(s) are credited and that the original publication in this journal is cited, in accordance with accepted academic practice. No use, distribution or reproduction is permitted which does not comply with these terms.
*Correspondence: Preston T. Snee, c25lZXBAdWljLmVkdQ==
Disclaimer: All claims expressed in this article are solely those of the authors and do not necessarily represent those of their affiliated organizations, or those of the publisher, the editors and the reviewers. Any product that may be evaluated in this article or claim that may be made by its manufacturer is not guaranteed or endorsed by the publisher.
Research integrity at Frontiers
Learn more about the work of our research integrity team to safeguard the quality of each article we publish.