- 1National Centre of Excellence in Analytical Chemistry, University of Sindh, Jamshoro, Pakistan
- 2Department of Chemical Engineering, University of Engineering and Technology, Peshawar, Pakistan
- 3M.A Kazi Institute of Chemistry, University of Sindh, Jamshoro, Pakistan
Nanoparticles can be used as inhibitory agents against various microorganisms, including bacteria, algae, archaea, fungi, and a huge class of viruses. The mechanism of action includes inhibiting the function of the cell membrane/stopping the synthesis of the cell membrane, disturbing the transduction of energy, producing toxic reactive oxygen species (ROS), and inhibiting or reducing RNA and DNA production. Various nanomaterials, including different metallic, silicon, and carbon-based nanomaterials and nanoarchitectures, have been successfully used against different viruses. Recent research strongly agrees that these nanoarchitecture-based virucidal materials (nano-antivirals) have shown activity in the solid state. Therefore, they are very useful in the development of several products, such as fabric and high-touch surfaces. This review thoroughly and critically identifies recently developed nano-antivirals and their products, nano-antiviral deposition methods on various substrates, and possible mechanisms of action. By considering the commercial viability of nano-antivirals, recommendations are made to develop scalable and sustainable nano-antiviral products with contact-killing properties.
1 Introduction
Viruses are the major causative agents for more than 60% of human infections worldwide. The most common viral diseases are produced by enteric and respiratory viruses. There are several ways of viral transmission from an animal or infected person to a new host. Recent research suggests that contaminated surfaces or fomites play a role in spreading viral diseases in humans. The dispersal of viruses due to contaminated surfaces depends on the potential of the virus to sustain its infectivity in the environment. However, infectivity can be reduced by any single factor or a combination of chemical, biological, and physical factors (Vasickova et al., 2010). Environmental disinfection has started to gain importance recently. Currently, it is included in several national and international infection control recommendations and policies.
Effective biocidal action for viruses, bacteria, and fungi is one of many criteria considered for any antimicrobial treatment. However, it is important to concentrate on using less dangerous chemicals. There is urgent need to develop such materials with no or minimum effects on the skin, environmentally friendly, favorable for textile processing, durability, stability, sustain washing chemicals as well as withstand to hot pressing. Textiles are non-woven or woven products produced by synthetic or natural fibers. Textiles have a wide range of usage in different areas, such as clothing, the military, the food industry, home furnishings, sports equipment, the medical industry, building materials, and the automotive industry. Textile usage defines its properties, such as smoothness, waterproofness, breathability, degradability, elasticity thread count, temperature stability, and antibacterial and antiviral properties (Iyigundogdu et al., 2017). Due to their large surface area and ability to absorb and retain oxygen and water, textiles become a good host for the growth of many microorganisms. Furthermore, other factors facilitating the growth and multiplication of microorganisms are pH, nutrition, and temperature (Morais et al., 2016).
Because of the growing demand, there is good room for research on antiviral textile fabrics. Most of the antiviral fabrics reported in the literature have not been widely prepared at an industrial scale and have not been commercialized yet. Antiviral fabric textiles have various purposes and applications and are considered clean, safe, and hygienic fabrics. The health and public transport sector may be the main consumer market for antiviral textile fabrics. Antiviral fabrics could kill viruses on the surface, reducing the chances of biofilm formation (Von Borowski and Trentin, 2021). Thus, the chances of infection and reinfection are reduced. In general, antiviral fabric textile retains virucidal potential up to numerous wash cycles. Its reusable property can help minimize solid waste generation (Abou Elmaaty et al., 2022).
Viruses can be transferred in different ways, including 1) by other inanimate surface or living organisms, 2) from the accumulation of particulate aerosols, 3) by direct transfer from the contact of an infected person through secretion, and 4) by surface contact (Iyigundogdu et al., 2017). A thesis on norovirus detection and surface-to-surface transfer from the University of Helsinki concluded that HuNoV could be transmitted easily from human hands to eatable items and environmental surfaces. Therefore, to inactivate HuNoV, there is a need for proper hand hygiene and other effective measures, including UV, for virus management. The origin of viruses is normally very difficult to define due to sudden outbreaks, but it is necessary to take control measurements efficiently to minimize the deadly effect. Furthermore, none of the peripheral surfaces are completely “clean.” An organic substance layer is always present, and through contact with the animal or human skin, the surface will be contaminated with sebum components, microorganisms, and different molecules. A surface exposed to air, especially a horizontally exposed surface, will be covered with oily emulsions, powder particles, and aqueous aerosols. Examples of such surfaces are service counters in hospitals, railings of trollies, and patient beds. Furthermore, depending on its uses and surface structure, a structural-layered soil may contain different fungal spores, bacterial cells, spores, oils, and dirt. Evidence of the role of surfaces in the transmission of pathogenic microorganisms causing infections from healthcare is reported in detail in a consensus document by Morais et al. (2016). They have concluded that high-touch surfaces cause the spread or transmission of diseases, and disinfection should be considered a holistic approach.
At present, research on the persistence of veterinary and human coronavirus on inanimate surfaces and strategies for their inactivation with different biocidal agents for chemical disinfection is reviewed in health facilities. Reusable pathogen-contaminated medical devices can be a source of human infections. If these tools have to be reused, essential precautions must be taken before reuse for the next patient to prevent pathogen contamination. These measures are termed reprocessing in healthcare settings and include sterilizing, disinfecting, or cleaning medical devices (Patoo et al., 2022). The scientific literature indicates that the contaminated surfaces and non-critical patient care processes play a significant role in the transmission of various health-related pathogens, including vancomycin-resistant Enterococci, methicillin-resistant Staphylococcus aureus, Norovirus, Clostridium difficile, and Acinetobacter. Therefore, the disinfection of the surfaces of medical devices and non-critical environmental surfaces is one of the strategies to prevent infection-causing pathogens and contamination (Jamunkar et al., 2022).
An analysis comprising twenty-two studies reveals that coronaviruses such as severe acute respiratory syndrome (SARS), Middle East respiratory syndrome (MERS), or human coronavirus (HcoV) can persist up to 9 days on glass, plastics, or metals (Goharshadi et al., 2022). Moreover, 229E, a human coronavirus, will remain infectious in the human lungs’ cell culture model for at least 5 days and persist on the range of some common non-biocidal surfaces, including polyvinyl chloride (PVC), PTFE, polytetrafluoroethylene (Teflon), glass, ceramic tiles, stainless steel, and silicon rubber (Góral and Góral-Kowalczyk, 2022). These contaminated surfaces can be effectively inactivated by surface disinfection procedures with 0.1% sodium hypochlorite, 62%–71% ethanol, or 0.5% hydrogen peroxide in 1 min or using 0.02% chlorhexidine digluconate or 0.05%–0.2% benzalkonium chloride, among others. In addition to manual cleaning with liquids, new technologies are emerging. Novel “non-touch” (self-cleaning) decontamination technologies, including aerosol and evaporating hydrogen peroxide emitting mobile devices, pulsed xenon UV light system, ultraviolet light (UV-C), and high-intensity narrow spectrum using 405 nm light. These “non-touch” automatic technologies can reduce bacterial contamination on different surfaces. The micro-condensation hydrogen peroxide system is related to minimizing colonization or reducing infection in multiple studies. However, there is limited evidence of infection reduction by a pulsed xenon system. A prospective randomized controlled trial has been recently completed, where continuous ultraviolet light (UV-C) is an efficient technology that can minimize healthcare-related infections and contaminations (Sondi and Salopek-Sondi, 2004). In addition, the concept of self-cleaning surfaces is developing, and new concepts are emerging in the literature. In hospital environments, high-contact self-sanitizing surfaces are recommended in place of aluminum or stainless steel. The use of copper instead of aluminum or stainless steel has shown clear advantages in the reduction of viral spread. Hydrophobic polymeric covalently applied to solid surfaces on metals, plastics, glass, polymers and fabrics, gauze, bandages, tissues, and other fibers are coated by brushing, spraying, or dipping to make surfaces virucidal and bactericidal are developed. Numerous material are available including hydrophobic, water-soluble polymers, charged and linear or branched polyethyleneimine. High molecular weight polymers are reported to be more virucidal. The dissolution and coating of these polymers by brush or spray is patented by Baram-Pinto et al. (2009). Nanomaterials are emerging antivirals (Patoo et al., 2022). A review of the antiviral activity of silver nanoparticles was reported by Jamunkar et al. (2022), and the role of nanotechnology as a whole is reviewed by Goharshadi et al. (2022).
Viral transmission has many modes, and many studies (even pre-SARS-CoV-2-pandemic) support the role of surfaces in cross-contamination and the spread of viruses. The field of antiviral surface coatings is evolving rapidly, witnessing a large increase in 2021 (Góral and Góral-Kowalczyk, 2022). By considering the emergence of pandemics and the role of virucidal materials, this review summarizes reports on new groups of viral compounds, nanoparticles, compositions, or surface coatings that can be effectively transformed into self-cleaning products. Furthermore, inconsistencies in the analysis of the viral activity of solid surfaces have recently been reported, so a brief overview of the literature that has arisen in the analysis of viral activities is also provided.
2 Nano-antiviral mechanism of action
Nanoparticles show excellent antiviral activity toward many strains of viruses because of their unique properties. Silver nanoparticles are widely studied because of their incredible activities. The antioxidants, anti-inflammatory, antiangiogenic, anticancer, antimicrobial, antifungal, and antiviral activity of silver nanoparticles has been widely studied (Sondi and Salopek-Sondi, 2004; Kalishwaralal et al., 2009; Al-Shmgani et al., 2017; Deyá and Bellotti, 2017; Singh et al., 2018; Yuan et al., 2018; Nagarajan et al., 2019). The mechanism of action of metal ions or metal nanoparticles can be the attachment or connection of nanoparticles to the surface of the virus, making the viral cell unable to be attached to the host cell; the production of highly reactive oxygen species that could attach to the spike or membrane of virus and inhibit the function of nucleic acid and protein; the enhancement of the immune system of the host by stimulating nucleus; and the destruction of the host cell and the inhibition of virus spread (Figure 1).
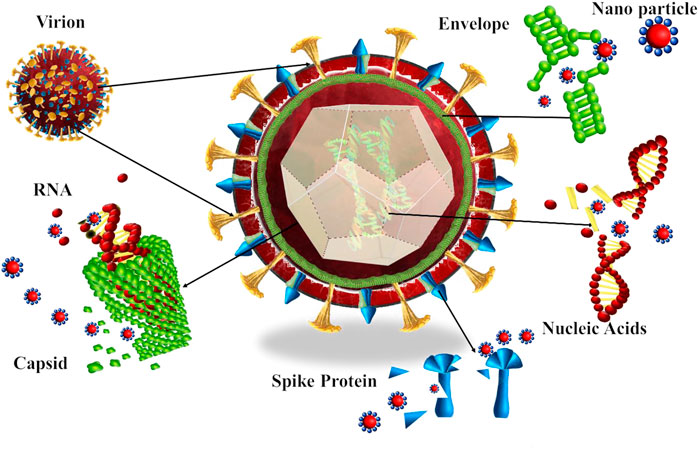
FIGURE 1. Antiviral mechanism of typical metal nanoparticles. Viruses generally infect the host cell through four mechanisms: 1. attachment to the host cell, 2. penetration, 3. viral replication, and 4. budding. The metallic nanoparticle inactivates viruses by attachment to the virus, inhibiting the attachment virus to the host cell, damaging the peptidoglycan bonds, increase levels of H2O2, O2- or OH-, which are reactive oxygen species (ROS), binding to the spike of cell in order to stop attachment, destruction of cell structure, functional and structural proteins, destruction of nucleic acid and promote host immune system to inhibit the spreading and budding of the virus.
Silver nanoparticles are highly effective against viruses including influenza, HIV-1, monkeypox, herpes hepatitis B, respiratory syncytial, simplex, and Tacaribe viruses (Sun et al., 2005; Rogers et al., 2008; Baram-Pinto et al., 2009; Lara et al., 2010a; Papp et al., 2010; Speshock et al., 2010; Lara et al., 2011). In the case of HIV-1, silver nanoparticles get attached to the sulfur of gp120 (a spike protein present on the viral membrane), thereby blocking virus attachment to the host cell membrane (Lara et al., 2010a). The viral entry for SARS-CoV-2 into the host cell depends on proteases that divide angiotensin-converting enzyme 2 (ACE-2), a protease and receptor spike proteins, which are receptors of SARS-CoV-2. The life cycle of SARS-CoV-2 begins when spike protein binds to ACE-2. The ACE-2 has a very important lysine 31 residue recognized as critical in binding with 394 glutamine residues present in SARS-CoV-2 (Bertram et al., 2011; Glowacka et al., 2011; Shereen et al., 2020). Therefore, blocking the 394-glutamine residue in spike protein by silver nanoparticles may play a role in inhibiting the viral entry into the host.
Another report suggested that silver nanoparticles act as an entry inhibitor or virucide toward HIV at the initial stage of the viral cycle. A specific interaction between silver nanoparticles and HIV is formed by binding with gp120. Silver nanoparticles, particularly in the case of HIV, block the entry of the virus by gp120-CD4 interaction through binding with the protein structure of the viral membrane. The interaction of silver nanoparticles with gp120-CD4 is generally electrostatic (Lara et al., 2010a). Additionally, silver nanoparticles may interact with disulfide bonds present in the carboxyl of the HIV-gp120 glycoprotein. As a result, silver ion reduces the disulfide bonds and denatured proteins of the virus. Additionally, silver ions can form a complex with oxygen, sulfur, and nitrogen (electron donor groups), which are generally present as phosphate or thiols on the nucleic acid and amino acid of the virus. These particles can also directly bind to DNA or RNA and reduce the transcription rate (Lekutis et al., 1992; McDonnell, 2007; Varni et al., 2007; Lara et al., 2010a). Another suggested route in the literature for the deactivation of the virus using nanoparticles is the size of nanoparticles, which plays an important role in the antiviral activity. The interaction of silver nanoparticles with glycoprotein present in the spike of virion of HSV-2 shows that the center-to-center space of the spike is about 9–13 nm, and the height of spikes is about 10–25 nm. Hence, small-sized nanoparticles of silver (i.e., 13 nm) have a greater binding affinity compared to the size of 33–46 nm. The shape of nanoparticles is reported to be an important factor in viral inhibition. For instance, cubic, cylindrical, or spherical shapes have less cellular attachment in the case of HeLa cells compared to the rod shape nanoparticles (Orlowski et al., 2014; Banerjee et al., 2016; Alavi et al., 2022).
Besides silver, zinc nanoparticles are also reported as antivirals. Zinc is part of various biological activities, including the expression and activity of different transcription factors and cellular enzymes. Zinc also shows great antiviral potential through various mechanisms. Zinc is a cofactor of different viral proteins and helps in misfolding viral polyproteins by facilitating the proteolytic process through misfolding, which changes the structure of the virus (Te Velthuis et al., 2010). Zinc may inhibit the Semliki Forest virus by inhibiting membrane attachment. Zinc prevents virus entry into the cell by binding with the histidine protein of the Semliki Forest virus at glycoprotein E1 (Kümel et al., 1990; Te Velthuis et al., 2010; Liu and Kielian, 2012; Ishida, 2019; Rani et al., 2021). Another study reported that zinc inactivates the varicella-zoster virus (Shishkov et al., 1996). In many viruses, including HIV, rhinovirus, vaccinia virus HSV, and SARS-CoV, zinc helps kill the virus by stopping its entrance, RNA-dependent RNA polymerase, or polyprotein processes (Te Velthuis et al., 2010). Zinc also plays a role in inhibiting the replication of alphavirus and flavivirus and enhances oxidative stress (Rani et al., 2021).
Copper acts differently on different types of viruses. For example, in the case of the coccolith virus, copper disturbs the lytic cycle by increasing the generation of reactive oxygen species (Haldar et al., 2007). Copper cross-links and binds with the strands of DNA and destroys the viral genome. The contact killing time for copper is suggested as 7–8 logs/h, which means that the virus gets destroyed exponentially 1 × 107 times in an hour (log 3/h reduction is considered 100% as ISO 18184 classification). When copper is contacted with the virus’s surface, copper intervenes in the virus as the nucleic acid of the virus degrades. Copper inactivates the virus by changing its viral genomes through damaging RNA and causing structural changes, which include rupturing of the envelope and surface spike dispersal. Van Doremalen et al., 2020 compared the stability of SARS-CoV-1 with SARS-CoV-2. Results showed that SARS-CoV-2 was more stable on plastic and stainless steel than on copper and cardboard, and a viable virus was detected up to 72 h after application to these surfaces. On copper, no viable SARS-CoV-2 was measured after 4 h and no viable SARS-CoV-1 was measured after 8 h (Van Doremalen et al., 2020). In general, copper nanoparticles create oxidative stress, which can disassemble bacterial or viral membranes. Thus, it can interfere with their viral activity. Furthermore, copper ions facilitate the generation of compounds (e.g., OH radicals and redox actives) that are toxic to microbes. On another side, copper nanoparticles may change the homeostasis of the cell through metal ions. If a cell is exposed to a concentration higher than normal, the system of the cell ultimately collapses (Ermini and Voliani, 2021). However, this mechanism may or may not be applicable for viruses as these infectious agents live in a dynamic equilibrium (homeostasis) with their hosts in which both immune and non-immune pathways contribute to viral homeostasis. However, the disruption of these pathways can have dramatic consequences on pathogenesis (Von Borowski and Trentin, 2021).
Few studies have been conducted on the antiviral activity of quantum dots. Moreover, a few showed that quantum dots exhibited good antiviral activity. Some studies showed that modified quantum dots could inhibit virus entry into most cells by modifying the structure of proteins. The attachment of quantum dots to the membrane reduces the virus quantity and effectively inhibits the multiplication of viral RNA (Du et al., 2016; Du et al., 2018; Gurunathan et al., 2020). In the case of graphene oxide or its derivatives or graphene-based nanoparticles, lateral size plays an important role in the antiviral activity. Sharp edges, adsorption, and desorption are largely affected by the particle size of the material. Studies have revealed that higher lateral size and greater absorption ability attributed to greater surface energies and antimicrobial properties were greater with large-size graphene oxide sheets compared to smaller ones (Cai et al., 2011; Jabbar et al., 2020). Antiviral activities of graphene oxide (large sheets) and reduced graphene oxide (relatively smaller sheets) are significantly different, probably owing to size variations. Other factors include the number of layers present in graphene, which increases its thickness and weakens the nano-knife effect, increases aggregation, decreases dispersion that results in less contact between microbes and materials, and alters the antimicrobial effects (Wang et al., 2013; Zou et al., 2016; áde Leon et al., 2015). Graphene oxide and reduced graphene oxide carry a negative charge, helping them attract viruses with a positive charge, henceforth higher charge attributes more attraction as the graphene oxide has a higher charge compared to the reduced graphene oxide, so it has more affinity to attract virus (Mohammed et al., 2020; Nasrollahzadeh et al., 2020). Fabrication of functional groups, including carboxyl hydroxyl and carbonyl, can amplify the redox reaction between graphene oxide and viruses. The graphene and reduced graphene oxide have an intrinsic capacity to absorb lipids due to their charge, which promotes the destruction of the viral membrane. It has been observed that the feline coronavirus’s lipids bilayer was absorbed on the surface of graphene and the reduced graphene oxide owing to the electrostatic interaction between the negatively charged group in the graphene oxide and the positively charged viral particles, which made it difficult for the virus to bind (Chen et al., 2016). Another important factor that plays in viral inactivation is particle shape. It has been observed that sharp edges of graphene oxide nano-walls and reduced graphene oxide nano-walls inactivate the attachment and inhibit the entrance of viruses before they get into the cell, which is done by destroying the outermost layer. Thus, the morphology of the virus will be changed, and their functions got disturbed (Mohammed et al., 2020). Functionalities of graphene oxide or reduced graphene oxide play a significant role in preventing agglomeration and, hence, their antiviral properties. Antifouling property enhancement can improve the function of antiviral coatings and surfaces (Ayub et al., 2021).
3 Virucidal compositions for solid surfaces
The most common surface coatings for virucidal activity, as reported in the literature, are 1) based on polyethylene-imine or polyethylene-amine type compounds, which are polyelectrolytes, and 2) metal salts and oxides. The supporting materials (substrates) are mostly metallic surfaces, fabric, and glass. Coatings can be accomplished by selecting polyelectrolytes and controlled conditions for the deposition. Multilayers can be deposited on the surface for coating with carefully controlled adhesive properties according to the targeted viruses (Hsu and Wu, 2019). The results obtained from MS2-type bacteriophage (MS2) adhesion using quartz crystal microbalance with the degeneracy monitoring were compared with the predictions by the (XDLVO) Derjaguin–Landau–Verwey–Overbeek theory. Different surfaces show different kinetics and variable capacities for the deposition of MS2, indicating the potential of polyelectrolyte for multilayer deposition, a promising and easy method to apply coating on the surface for adsorption of viruses on the surface, inactivation of the virus by the viricidal properties of cationic polyelectrolytes, and minimization of the exposure of viruses to humans (Dang and Tarabara, 2019). Another ionically crossed-linked polymeric film was introduced using the layer-by-layer (LbL) technology, and silicon was used as a substrate in order to increase contact killing property of surfaces. N,N-dodecyl,methyl-polyethylenimine (polycation) with antimicrobial activity was layered along with poly acrylic acid (polyanion) using the LbL technology to create a film. This surface is highly effective against airborne and waterborne Staphylococcus aureus and Escherichia coli (Gram-positive and Gram-negative bacteria, respectively). It shows great results against A/WSN (H1N1) influenza viruses (Wong et al., 2010).
Another antiviral material was developed using cationic polyallylamine (PA) polymer bonded covalently with glass. This PA film showed excellent antimicrobial activities against Gram-negative and Gram-positive bacteria, including Pseudomonas aeruginosa, Pseudomonas aeruginosa, and Staphylococcus aureus (Iarikov et al., 2014). Moreover, polycation N, N-dodecyl,methyl-polyethylenimine is coated on glass slides along with the solution of polycations butanol. The results showed a 100% reduction in Escherichia coli and Staphylococcus aureus and strains of the influenza virus (Deyá and Bellotti, 2017). Another multistep synthesis of the antimicrobial surface is reported by replacing hydrophobic polycations with an aerosol-assisted plasma deposition procedure, in which HMPEI (N,N-hexyl,methyl-PEI) is coated (plasma-coated) directly on glass surfaces. This coated surface has shown robust results against Escherichia coli and the human influenza virus (Liu et al., 2014). A paint-based application of polycation antibacterial surface has been investigated in detail for its practical applications. The authors claim stability of the coating process over the impregnation process (Mukherjee et al., 2008). N,N-Dodecyl,methyl-polyethylenimines (PEIs) were coated on glass, and their efficacy was tested for H1N1 and H3N2, found as 100% within 30 min and 2 h, receptively (Haldar et al., 2006).
Metallic nanoparticles are deposited directly by adsorption, spray, or dip-coating or using linkers and by sonochemical or magnetron sputtering methods (Góral and Góral-Kowalczyk, 2022; Meister et al., 2022). Zinc citrate was coated on muslin cloth, which showed 99.7% virucidal activity for H3N2 (Nonomura, 2007). The antiviral activity of four compounds, namely, CuCl2 (copper ionic compound), Cu2O and CuO (solid-state copper compounds), AgNO3 (silver ionic compounds), and Ag2S, and Ag2O (a solid-state silver compound) have been investigated against the bacteriophage Qβ and surface protein of influenza A viruses, neuraminidase (NA), and hemagglutinin (HA). Cu2O (solid-state copper) has shown itself to be a promising candidate against non-enveloped and enveloped viruses compared to silver compounds because it has a unique mechanism of inactivation supported by direct contact (Minoshima et al., 2016; Sunada et al., 2012). New and durable platform technologies have been invented to introduce copper into latex, polymeric materials, and cotton fibers. This technology helps produce antiviral filters and gloves that can deactivate viruses, including HIV-1; self-sterilizing antibacterial fabrics that can kill antibiotic-resistant bacteria, including vancomycin-resistant Enterococci, and methicillin-resistant Staphylococcus aureus, anti-dust mite mattress covers to reduce material allergies and antifungal socks, which can help reduce the symptoms of Athlete’s foot (Borkow and Gabbay, 2004). The same researchers have developed copper oxide-impregnated fabric for various uses (Borkow and Gabbay, 2004). Recently, a copper sulfate hybrid with alginate using ceramic as a substrate has been reported for the inactivation of CoV-2 (Bataglioli et al., 2022). Furthermore, the copper oxide-impregnated face mask was approved by Occupational Safety and Health Administration (OSHA) (United States) and is now used in general practice for safety (Borkow et al., 2010).
A new mode of incorporation of virucidal material was reported where copper glass (copper oxide incorporated aluminosilicate) powder was dispersed in latex paint and tested as a durable virucidal surface coating (Gross et al., 2019; Hodek et al., 2016). The coatings were tested using EPA recommended procedure for solid surfaces and found to be comparable with metallic copper.
3.1 Metals as virucides
Metallic nanoparticles have gained the interest of researchers due to their unique properties, including small size and high specific surface areas that can help interact with microorganisms, such as bacteria and viruses (Birkett et al., 2022). Otherwise, nanoparticles can enhance cytokine production and induce a hormonal immune response and have the capacity for cell recruitment, biocompatibility, low toxicity, good biodistribution properties, and chemical inertness (Dykman, 2020; Behzadi et al., 2021; Abate et al., 2022). Several metals and metal oxide nanoparticles, including copper, silver, gold, zinc, and titanium, have been studied as antiviral agents.
3.1.1 Copper and its nanoparticles
Copper is long known for its antimicrobial applications (Haldar et al., 2006; Grass et al., 2011; Steinhauer et al., 2018), whereas some studies have shown equal potential for its alloys. Copper alloys are more active antivirals compared to pure copper. Compared to other metals, such as platinum, gold, silver, and palladium, copper is the cheapest and most easily available (Grass et al., 2011). Another advantage of copper is that it gets oxidized to form copper oxide nanoparticles that can be blended with different macromolecules or polymers and give a stable product in terms of physical and chemical properties (Cioffi et al., 2005). Copper alloys are effective in inactivating murine norovirus by destroying the RNA genome when it is exposed to copper surfaces. A study suggested that copper ions were still indirectly or directly responsible for inactivation, but it has not created reactive oxygen species (ROS) as a toxicity mechanism. Although their mechanism of destruction can vary and can be suggested as multimodal, it is noteworthy that copper alloys are very promising candidates for the destruction of various disease-causing microorganisms (Haldar et al., 2007; Ikner et al., 2020). Besides copper and its alloys, efforts are made to develop coatings for inanimate metallic and non-metallic surfaces to cover the high-touch surfaces, which are prone to soiling (accumulation of dirt, microbes, etc.). Alloys of copper, nickel, and zinc are tested against viruses to replace steel as high-touch surfaces (Bregnocchi et al., 2022). The copper alloy was shown to have a strong virucidal activity under clean and moderate soiling conditions (>four-log reduction) for virus droplets or dried virus onto the surface. Multiple exposures of the surface to viruses indicate that the surface could not inactivate virus droplets (three-log), regardless of no or moderate soiling. Heavy soiling reduced inactivation below an acceptable efficacy threshold. Virucidal tests of copper, nickel, and zinc ions indicate that copper and nickel were significantly virucidal (Konieczny and Rdzawski, 2012). Copper has been known as a biocide since 200 BC and has been extensively used in ancient times (Grass et al., 2011; Konieczny and Rdzawski, 2012). Recently, various compositions of copper have been used for the treatment of anemia, facial neuralgia, chorea, tubercular infections, adenitis, impetigo, scrofulosis, eczema, syphilis, and lupus (Grass et al., 2011). Copper is effective against various types of viruses, including poliovirus, HIV-1, hepatitis C, monkeypox, murine norovirus, herpes simplex, bronchitis, and COVID-19 (Cortes and Zuñiga, 2020; Gauri et al., 2020). The antiviral activity of copper (II) chloride dihydrate solution against the dengue virus type-2 in vivo cell was also reported (Sucipto et al., 2017). Compared to silver, Cu2O has shown a good result against unenveloped and enveloped viruses (Jung et al., 2021). At 2.5–250 µM concentrations over time, Cu2+ can inhibit the H9N2 virus, which infects the MDCK cells. In the 25 µM Cu2+ solution, the virus titer decreased by about three and four logs during 3–6 h. Compared to Cu2+, Zn2+ was much less effective against virus inactivation. At 2.5–250 µM, Cu2+ did not affect the activity of the H9N2 virus hemagglutinin nor the neuraminidase (NA). This shows that copper ions suppress the infectivity of the influenza virus at lower concentrations at which neither NA nor hemagglutination inhibition occurs. Therefore, the mechanism of action requires further studies (Horie et al., 2008; Jana et al., 2021). Copper creates toxicity to microorganisms by various parallel mechanisms that can cause cell death even immediately after minutes of exposure to copper. The first area damaged by copper can be the envelope of microorganisms. Copper-containing steel was found to adhere to the bacterial plasma membrane by exerting the electrostatic forces by Cu2+. Copper may also cause structural changes in protein structure. For example, the oxidation of cysteine in the active region of the protein tyrosine phosphatase associated with the Cu2+ active vaccine H1 resulted in complete inactivation of the protein activity. Copper ions can also damage nucleic acids by cross-linking between and within DNA chains. The mechanism can be considered oxidative damage or like Fenton’s reaction. In general, the redox cycle between Cu2+ and Cu+ may be associated, which can catalyze the production of highly active hydroxyl radicals and then damage proteins, DNA, lipids, and other biomolecules (Papp et al., 2010). Shionoiri et al. (2012) used copper iodide nanoparticles (CuI)NP against feline calicivirus, a non-enveloped virus used as a human norovirus proxy. They found that virus infectivity reduced up to seven times at the dosage of 1,000 μg/ml CuI nanoparticles (Shionoiri et al., 2012). Purniawan et al. (2022) synthesized copper nanoparticles from copper sulfate with a diameter of 254 nm, mixed them with resin-based paint, and used them as a spray for surface coating agents against SARS-CoV-2. The result shows that within 10 min, the antiviral activity of the spray reaches 90%, 97.8%, and 99.99% after 10, 30, and 60 min, respectively. However, paint alone reached 97.8%, after 18 h of exposure time for inactivation of virus. Hutasoit et al. (2020) reported the coating of copper using the cold spray technique to fabricate copper on steel parts against SARS-CoV-2. The results showed that about 96% of SARS-CoV-2 get inactivated within 2 h. In another study, Behzadinasab et al. (2020) fabricated a coating using Cu2O bound with polyurethane and applied it to steel or glass. The virucidal activity was 99.9% compared to the uncoated glass or steel. The coating material remained active for 13 days after various washing cycles with a mixture of 70% ethanol–water mixture. The coating is so strong that it cannot be removed with a razor blade. Bello-Lopez et al. (2021) reported a biocide effect against SARS-CoV-2 and ESKAPE pathogens of a non-cytotoxic silver–copper nanofilm. A nanometric layer of bimetallic AgCu was effectively deposited on polypropylene (PP) fibers. The virucidal results show more than a 95% reduction in viral load after 2 h of contact time (Bello-Lopez et al., 2021). El-Nahhal et al. (2012) reported CuO and CuS nanoparticles coated onto cotton fabric. Accordingly, CuO nanoparticles showed excellent antibacterial activity. However, CuS nanoparticles did not show any activity. Au/CuS based nanoparticles are reported as virucides are reported and recommended generally that inactivation by nanoparticles (NPs) is a universal alternative to other chemical and physical strategies that exhibit variable efficacy (Gurunathan et al., 2020). All the above discussion shows that copper is the antidote for viruses as it has the potency to deactivate viruses in vivo and in vitro. Based on scientific data, a patent entitled “copper-containing dry disinfection apparatus” was registered (Rogers et al., 2008). In addition, copper oxide nanoparticles have been reported as an antibacterial that can be extended to solid surfaces in suspension (Lara et al., 2011).
3.1.2 Silver-based nanoparticles
Silver nanoparticles have been successfully used against different types of respiratory viruses, including coronaviruses, rhinovirus, influenza virus, adenoviruses, hepatitis B virus, human immunodeficiency virus, and herpes simplex virus (Lara et al., 2010a; Galdiero et al., 2011; Demchenko et al., 2022; Parvez et al., 2022; Patoo et al., 2022; Zhang et al., 2022). Before the discovery of penicillin in the 1930s, silver was known as an antimicrobial for centuries. Due to their impressive antimicrobial activity, silver and silver-based nanoparticles still have very high demand. In addition, silver has been used to prevent infection and food spoilage (Hoyme, 1993). Besides the antibacterial and antiviral activity, silver nanoparticles are being extensively studied due to their stability, non-toxic nature, disinfectant capacity, water purification properties, high quantum efficiency, increased conductivity, anticancer biochemical capacity, and easy synthesis process (Jiang et al., 2005; Basheer et al., 2013; Cornelis et al., 2013; Bhosale and Bhanage, 2015; Rasool and Lee, 2016; Pandiarajan and Krishnan, 2017; Loo et al., 2018; Mahdi et al., 2018; Deshmukh et al., 2019; Shrivas et al., 2019; Das et al., 2020a; Ahn and Park, 2020; Das et al., 2020b; Gomathi et al., 2020). In the case of bacteria, silver ions inhibit the growth of bacteria by suppressing electron transport components and respiratory enzymes and interfering with the function of DNA. In addition to the antimicrobial and antibacterial activity, silver nanoparticles have been widely used in coating different medical devices, wound dressing, and textiles to prevent microbial growth and infection. One of the main advantages of using silver nanoparticles is their ability to continuously release silver ions, which increases their antimicrobial ability. The silver nanoparticles have been efficient against more than 650 types of microorganisms, including a wide range of bacteria, fungi, and viruses (Dakal et al., 2016). Generally, silver nanoparticles prevent virus attachment onto the host cell by interacting with the outermost layer of the bacteria. The physiochemical properties, including particle size, surface area, and the shape of nanoparticles, play an important role in antiviral ability. The antiviral activity of silver nanoparticles has been studied against a wide range of viruses including hepatitis B and C virus, influenza virus, poliovirus, dengue virus, herpes simplex virus, chikungunya virus, human immunodeficiency virus, Rift Valley fever virus, respiratory syncytial virus, vaccinia virus, white spot syndrome Virus, African swine fever virus, enterovirus, murine norovirus, porcine reproductive viruses, feline calicivirus, monkeypox virus, respiratory syndrome virus, Tacaribe virus, porcine epidemic diarrhea virus, tobacco mosaic virus, bacteriophage MS2, UZ1, φX17433, bean yellow mosaic, and coronavirus (Elechiguerra et al., 2005; Lu et al., 2008; Rogers et al., 2008; Sun et al., 2008; Speshock et al., 2010; De Gusseme et al., 2011; Gaikwad et al., 2013; Trefry and Wooley, 2013; Xiang et al., 2013; Elbeshehy et al., 2015; Sujitha et al., 2015; Borrego et al., 2016; Li et al., 2017a; Castro-Mayorga et al., 2017; Huy et al., 2017; Park et al., 2018; Ochoa-Meza et al., 2019; Sharma et al., 2019; Ahsan, 2020; Du et al., 2020; Dung et al., 2020; Jeremiah et al., 2020; Shady et al., 2020; Ghosh et al., 2022). Bekele et al. (2016) investigated the antiviral activity of silver nanoparticles with various dosages and sizes to evaluate the antiviral activity of feline calicivirus. The study reveals that the silver nanoparticles with diameters of 10 nm can successfully decrease the virus load because the virus size ranges from 27 to 40 nm, so the smaller size of nanoparticles can easily interact with the virus. Sundararaj Stanleyraj et al. (2021) evaluated the antiviral activity of silver nanoparticles on SARS-CoV-2. The results revealed that silver nanoparticles with a size of 10 nm could successfully inhibit the extracellular SARS-CoV-2. Jeremiah et al. (2020) reported the antiviral activity of silver against SARS-CoV-2. The size of the synthesized nanoparticles is 10 nm, and a dosage of 1–10 ppm prevents viral infiltration (Rahman et al., 2021). In another study, silver nanoparticle-fabricated textiles were tested against the influenza A virus and calicivirus and showed a promising result (Seino et al., 2016). Demchenko et al. (2022) studied the antiviral activity of silver coating polylactic acid chitosan polymer film against adenovirus serotype 2 and herpes virus type 1. The results revealed that 4% of synthesized nanocomposites have high antiviral activity against herpes virus type 1. The inhibition of the cytopathic effect against the virus reached 5.12 log10TCID50/ml, whereas that against adenovirus and influenza virus reached 1.07 and 0.60 log10TCID50/ml. The nanocomposite is ineffective against Hep-2, BHK-21, MDCK, and cell cultures. If the concentration of silver decreases by less than 4%, the antiviral activity also decreases. Nanocomposite contains 1% silver and does not show cytopathic action against adenovirus. In contrast, as the concentration increases to 2%, inhibition starts at 0.18 log10TCID50/ml, whereas the maximum inhibition is achieved at 4% of the silver nanocomposite. Fetouh et al. synthesized silver nanoparticles with activated carbon by photodeposited silver nanoparticles into activated carbon. The composite showed good in vitro antiviral activity against the hepatitis A virus. In another experiment, silver nanoparticles were used to determine the in vitro antiviral effect on adenovirus type 3 (Ad3). The synthesized nanoparticles were fabricated through the redox method using 1% tannic acid in the silver nitrate solution. The results show a remarkable inhibitory effect against adenovirus type 3 (Ad3) due to possible DNA damage and the destruction of the structure of adenovirus type 3 (Chen et al., 2013). AgNPs have been used for the in vitro and in vivo inhibition of fungi, bacteria, and viruses. Particularly, in some reports, researchers used this nanoscale material to inhibit the coronavirus family, such as H1V1 and H3N2 influenzas. However, some reports debated the clinical trial or the clinical use of AgNPs for various treatments. Moreover, some reviews mentioned that the silver element was applied as an effective agent for various treatments in ancient medicine. It is suggested that at least the potential of silver nanoparticles and many non-hazard nano-metals and nano-metal oxides (by special dosages) could be considered (by related scientists) as candidates for the inhibition of 2019-nCoV (Du et al., 2018). Implantation of metal-containing nanoparticles on the surface of the metal oxide cover layer as germicidal has been reported. The plasma method was utilized according to the invention to produce the nanoparticles, which permits the extensive immobilization of the nanoparticles and, therewith, the control of the dosing of the metal ion release and the minimization of the risk of mobility of nanoparticles. The germicidal action of the metal ions without light exposure is of great significance in medicine (Du et al., 2016).
3.1.3 Zinc-based nanoparticles
Zinc is naturally present in a variety of dietary items. Zinc is also known for its antiviral activities against different viruses, including severe acute respiratory syndrome coronavirus (SARS CoV), human immunodeficiency virus (HIV), human papillomavirus (HPV), hepatitis C virus (HCV), hepatitis E virus (HEV) rhinovirus, respiratory syncytial virus (RSV), herpes simplex virus (HSV), and equine arteritis virus (EAV) (Korant et al., 1974; Haraguchi et al., 1999; Suara and Crowe, 2004; Te Velthuis et al., 2010; Kaushik et al., 2017). Gupta et al. (2022) studied the antiviral activity of zinc oxide nanoparticles and tetrapod-shaped zinc oxide nanoparticles against hepatitis C and hepatitis E viruses. The synthesized zinc oxide nanoparticles successfully inhibit viral replication. The study revealed that, at a considerable dosage of zinc oxide, nanoparticles are non-cytotoxic to cells. Ghaffari et al. (2019) used zinc oxide nanoparticles modified with polyethylene glycol and tested them against the H1N1 influenza virus. The size of synthesized nanoparticles was estimated between 16 and 20 nm. The results show viral inhibition up to 94.6%. Melk et al., 2021 synthesized zinc oxide nanoparticles mediated by the extract of Plumbago indica L. The synthesized particle size was 2.56–8.83 nm, applied to an antiviral test against herpes simplex virus type 1(HSV-1). The results show that IC50 and CC50 are equal to 23.17 ± 2.2 μg/ml and 43.96 ± 1.39 μg/ml, respectively (Melk et al., 2021). Zinc basically module the immune response to stop virus replication. It is a mediator of lipopolysaccharides in bacteria (LPS) and toll-like receptor 4- (TLR4-) dependent myeloid differentiation primary response protein 88 (MyD88) that activates nuclear factor-κB (NF-κB). As a result, the production of interleukin-1β (IL-1β), interleukin-6 (IL-6), and tumor necrosis factorα (TNFα) occurs, which controls viral pathogens (Haase et al., 2008; Brieger et al., 2013; Gupta et al., 2022). In another study, El-Megharbel et al. (2021) synthesized zinc oxide nanoparticles, and the antiviral activity of synthesized particles was checked against SARS-CoV-2. The zinc oxide nanoparticles were used as a spray for surface coating, and results revealed that in an in vitro study, at a very low concentration of IC50 = 526 ng/ml, the antiviral activity was CC50/IC50 ≤ 1. The authors recommended these nanoparticles’ spray as a good disinfectant, but it has some cytotoxic effects, CC50 = 292.2 ng/ml to the host cell (El-Megharbel et al., 2021). SARS-CoV-2 has become a matter of concern for scientists during the last 2 years. Many researchers have focused on anti-SARS-CoV-2 activity using different metals. Attia et al. 2021 synthesized zinc oxide nanoparticles using hesperidin (isolated from the orange peel) and checked it against the hepatitis A virus (an example of RNA virus). The results show that the synthesized nanoparticles possess 58.83% activity with minimum cytotoxic concentration.
In addition to metals, metal slats and their nanoparticles reported in the literature are compiled in Table 1. Copper, silver, and zinc are the most common types of metals. Iron also has shown activity, but reports on iron are limited. Copper-based nanoparticles in small doses show faster kinetics in virus deactivation.
3.2 Fullerene and its derivatives
Fullerenes are allotropes of carbon with unique properties. They are used in the preparation of advanced functional materials as a scaffold because of their good biological properties. Fullerenes have gained the attention of researchers due to their water solubility and cyclopentadienyl pattern. They are widely used in self-assembled nanostructures, siRNA AND gene delivery, and DNA binding (Maeda-Mamiya et al., 2010; Minami et al., 2014; Nitta et al., 2015; Castro et al., 2017; Kraevaya et al., 2021; Shi et al., 2021). Dating back to 1993, the antiviral activity of fullerene has been known against HIV. Fullerenes and derivatives showed significant antiviral effects against herpes simplex virus (HSV), Ebola virus, cytomegalovirus (CMV), and HIV influenza virus (Friedman et al., 1993; Mashino et al., 2005; Fedorova et al., 2012; Tollas et al., 2014; Muñoz et al., 2016). Fullerene derivatives are mostly water soluble, broadly characterized into six main types: 1) glycofullerene derivatives; 2) carboxyl derivatives; 3) amino acid, peptide, and primary amine derivatives; 4) hydroxyl derivatives; 5) fullerene complexes; and 6) piperazine and pyrrolidine derivatives (Xu et al., 2022). Fullerene blocks encoded enzymes and fit well on the protease of HIV. In order to block the HIV enzyme, the diamido diacid diphenyl fulleroid was designed first. Then, other groups were synthesized to inhibit the HIV enzyme, DNA polymerase, and HIV-1RT (Sijbesma et al., 1993; Nacsa et al., 1997; Castro et al., 2016; Innocenzi and Stagi, 2020; Xu et al., 2022). Coating of fullerene derivatives on the surface can prevent the possible attachment of SARS-CoV-2 because it produces lipid peroxidation on the lipid layer of SARS-CoV-2 and creates a hydrophobic surface, which minimizes the contact between the surface and the virus due to air entrapped between topographies. These textured surfaces possess anti-biofouling properties (Siddiquie et al., 2020). A visual diagram for probable deactivation is shown in Figure 2. Kraevaya et al. (2021) used fullerene derivative C60Ar5Cl, with thiophenes, and the antiviral activity against influenza A (rimantadine-resistant) Puerto Rico/8/34 H1N1 was evaluated. The synthesized compound K10, with five residues of thien-2-yl acetic acid and hydrocinnamic acid, showed very low cytotoxicity CC50 ∼ 69 μM and good antiviral activity EC50 ∼ 3 μM. In another study, Troshina et al. (2007) synthesized fullerene carboxylic acid derivatives (chlorofullerene C60Cl6) at a pH < 7.5. The water solubility of the reported derivative was about 50–100 mg/ml, with low cytotoxicity greater than 52 μM, and the antiviral activity in vitro and in vivo against HIV-1 was reported with IC50 of 1.20 ± 0.4 µM.
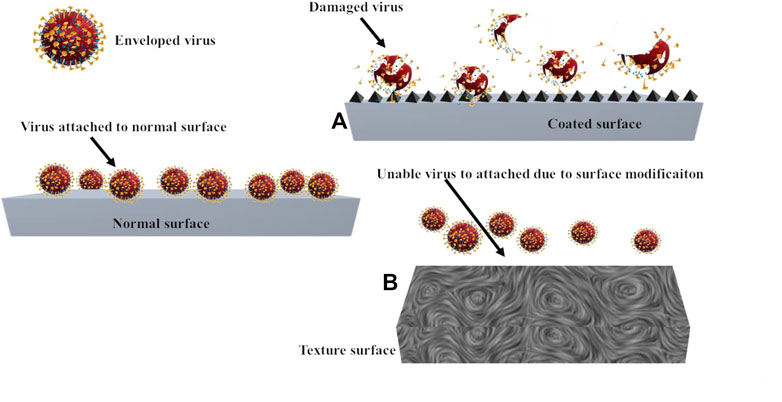
FIGURE 2. Texturing of the surface with fullerene derivatives for virus inactivation. By surface modification (A). Fullerene-textured surface (B).
3.3 Graphene and its derivatives
Graphene has been used in the inactivation of different kinds of viruses and microbes due to its higher surface area, electric conductivity, movement, and mechanical and piezoelectric characteristics (Yang et al., 2014; Zhang et al., 2015; Quan et al., 2017; Zhang et al., 2019; Lu et al., 2020). At first, the viral envelope is damaged when it interacts with graphene oxide, which leads to the production of ROS and virus deactivation (Gurunathan et al., 2012; Díez-Pascual, 2020; Ayub et al., 2021). Sametband et al. (2014) reported inhibitory properties of graphene oxide and sulfonated graphene oxide against HSV-1. Graphene oxide and sulfonated graphene oxide possess negatively charged particles like in the heparan sulfate cell receptor. As a result, both moieties compete to bind with HSV-1. The graphene blocks the binding sites, acting as an inhibitory agent (La Rosa et al., 2020). In terms of water safety, viral transmission possesses a substantial threat. Viruses, such as rotavirus, adenovirus, and norovirus, can pollute water, becoming the major cause of gastroenteritis (non-bacterial) (Bridge et al., 2010; De Graaf et al., 2016; Xue et al., 2019). These viruses are highly resistant to stressors possessed by the environment and risk of mortality in less immune individuals, young and elderly ones (de Roda Husman and Bartram, 2007; Espinosa et al., 2008). Zhou et al. (2022) developed a reduced graphene oxide composite nanoparticle functionalized with CTAB (cetyltrimethylammonium bromide) to absorb SARS-CoV-2 and rotavirus, adenovirus, and norovirus (human enteric viruses). The functionalized with CTAB, RGO-Fe3O4 have shown good absorption toward SARS-CoV-2, HAdV, HuNoV, and HRV with a maximum absorption capacity of 6.92 × 106, 2.21 × 107, 7.01 × 107, and 3.55 × 107 genome copies mg−1. The study revealed that viruses are trapped on the surface of synthesized composite by intrinsic absorption and electrostatic interaction between composite and virus. The synthesized composite can absorb the abovementioned viruses from rivers, coasts, and tap water. Unal et al., 2021 worked on graphene oxide nanosheets to inhibit the infectivity of SARS-CoV-2 cell receptors and surface protein. The study revealed that graphene oxide sheets work as a nanomaterial that can interact with SARS-CoV-2 specific receptors and surface proteins to inhibit viral infection. To understand the interaction between graphene oxide and the spike of the virus, spike-ACE2 complex, ACE2 cell receptor, and molecular docking experiments have been conducted. The results of molecular docking revealed that graphene oxide sheets interact with three types of structure present in SARS-CoV-2: closed state -6VXX is a type of spike protein of 2019-nCoV (novel coronavirus) or open state -6VYB (viral spike), open state -6VYB or closed state -6VXX (ACE2-bound spike complex), and 1R42 (ACE2). Graphene oxide showed good binding affinities toward all three surface structures 6VXX, 6M0J, and 6VYB. Graphene oxide shows more binding affinity toward ACE2 or spike compared to 6M0J. The obtained data shows that graphene oxide sheets tend to disturb the infectivity of SARS-CoV-2 even if any mutations are present on the viral spike. Deokar et al. (2017) demonstrated the synthesis of magnetic particles for the destruction of HSV-1. The author synthesized sulfonated nanoparticles with magnetic nature functionalized on reduced graphene oxide with a smaller size of approximately 5–25 nm. The synthesized graphene-based functionalized material can inactivate the HSV-1 virus up to 99.99% within 7 min. Magnetic functionalized graphene oxide captures the virus using an external magnet, which enhances the photothermal treatment. Donskyi et al. (2021) investigatedsulfate/alkyl functionalities on graphene sheets for the inactivation of SARS-CoV-2. Different lengths of alkyl chain have been synthesized with graphene oxide to determine the inhibition of feline coronavirus and SARS-CoV-2. It has been found that an alkyl chain greater than C9 can disturb coronavirus replication by disturbing its envelope. The antiviral activity against SARS-CoV-2 of the synthesized graphene oxide with dual functionalities with aliphatic chains and PGS (polyglycerol sulfate) showed IC50 (30 μg ml−1). The lower surface energy of certain materials and roughness at the nanoscale can play a great role in increasing the antiviral properties of materials, especially in the case of treated fabric or PPE. It can increase antiviral properties and superhydrophobicity. Galante et al. (2022) reported an antiviral fabric coating functionalized with coal-derived nano-graphene oxide. The graphene oxide was functionalized with octadecyl amine to increase water-repellent properties. The functionalized material is used to coat PET (polyethylene terephthalate) fabric. Furthermore, the polydimethylsiloxane (PDMS) layer was coated on the fabric to increase durability and repellency from human saliva and other fluids. The functionalized graphene oxide fabric was tested against five different types of viruses. The results revealed that the developed fabric had reduced viral titers of CoV reduction by 99.6% (2.4 logs), HAdV5 reduction by 98.6% (1.8 logs), and HSV-1 reduction by 99.4% (2.2 logs). The functionalized fabric repels droplets of human saliva and other fluids. Even after washing with bleach or mechanical abrasion, the fabric can reduce viral quantity up to 1–2 logs for a wide range of enveloped and unenveloped viruses.
3.4 Photocatalytic materials
We have discussed various surface coating antiviral materials and their antiviral activities to effectively control the viral infectious disease in hospitals or at home. Photocatalytic surfaces have gained more attention from researchers due to their constant inactivation, oxidizing, and destruction of microbes under ambient indoor environments (Reid et al., 2018; Rtimi et al., 2019; Miyauchi et al., 2020; Hamdi et al., 2021; Micochova et al., 2021; Prakash et al., 2021). Various photocatalysts have been used as antiviral and antibacterial materials, among which TiO2 is well known due to its viral and bacterial disinfection properties, as well as degradability for pollutants (Hajkova et al., 2007; Park et al., 2014; Akhtar et al., 2019; Moongraksathum et al., 2019). Exposing TiO2 to UV-A light, the ambient water and oxygen decompose on the surface of TiO2 in superoxide anions and hydroxide radicals. These substances are responsible for the decomposition of microbial and organic matter, which results in adverse effects, adducent microbial cells because of the peroxidation of lipid membrane (phospholipids present in the membrane) (Hajkova et al., 2007). Hajkova et al. (2007) also reported the photocatalytic antibacterial and antiviral effects of TiO2 films, which deactivate viruses up to 100% antiviral within 6 h of illumination. A few reports have been conducted on photoactive virucidal surfaces. For example, TiO2 photocatalysts were prepared and coated on aluminum and stainless surfaces. Ultrathin TiO2 coatings were obtained by wash-coating and screen-printing techniques. The later provides films of excellent adhesion that could tolerate washing under a water jet. The catalyst also exhibited excellent bactericidal, fungicidal, and virucidal activities against a wide variety of Gram-positive and Gram-negative bacteria, fungal spores, and T2 bacterial phage (Yao and Lun Yeung, 2011). Bactericidal and virucidal covering materials and methods for making the covering material are patented, where photocatalytic material is incorporated into thermoplastics. The sheets can be used in hospital settings to protect the furniture (Corsi et al., 2015). The self-disinfection properties of metal oxide are one of the best tools to stop the spreading of viral contamination from the infected person to an inanimate surface and from an inanimate surface to the healthy one. TiO2 is useful for photocatalysis because it has antiviral, antibacterial, and antibacterial characteristics. Excellent virus inactivation under UV light can be achieved by depositing a thin coating of TiO2 nanoparticles on a glass surface or another inert surface. TiO2 possesses good qualities regarding photocatalytic activities and antibacterial and antiviral properties. A thin layer of TiO2 nanoparticles deposited on the surface of glass or another inanimate surface can give excellent virus deactivation under UV light. In contrast, TiO2 dopped in cobalt nanoparticles was reported as a cost-effective good detection option for SARS-CoV-2 infection using electrochemical, biosensor in saliva, or nasal secretions based on coronavirus spike protein sensing. The larger surface area and catalytical and antiviral properties have made TiO2 nanoparticles an excellent candidate. Photoactive TiO2 hydrothermally grown can form reactive oxygen species, particularly hydroxyl radicals ˙OH, under the low light of 0.4 mW cm2 and wavelength of 375 nm, giving good pathogen interaction including SARS-CoV-2 (Kumar et al., 2020; Kumar et al., 2022). Thin films of TiO2 on glass for self-cleaning have been recently reported as a chapter in the book by Sirichantra (2022). The study recommends that such antiviral glasses would be useful in ambulances (Sirichantra, 2022).
4 Other virucidal nanomaterials
Carbon-based nanostructured photocatalysts have attracted attention, especially in the photocatalytic disinfection of microorganisms. With the appropriate electronic band gap structure and distinctive features of high thermal and chemical stability, a metal-free 2D polymeric stacked structure g-C3N4 (graphite carbon nitride) is a promising photocatalytic material for energy and environmental applications. It also has great potential for the inactivation and degradation of pathogens. The disinfection of microorganisms is mainly attributed to the formation of reactive oxidative species (ROS). The surface modification of g-C3N4 can significantly improve photocatalytic disinfection efficacy (Dang and Tarabara, 2019).
5 Deposition methods of nanoparticles on a fabric substrate
In the textile industry, the application of nanotechnology has good potential. The properties of fabrics are generally improved due to nanomaterials or the new abilities added to textiles (Karst and Yang, 2006; Motakef Kazemi and Sandalnia, 2020).
Cotton fabrics provide a perfect environment for bacteria and fungi growth because the moisture, temperature, and nutrition (skin dead cells, stains, sweat, and other skin emissions) on the surface of the fabric match their development and reproduction requirements. Microbes that live on the fiber surface can cause unpleasant smells, dye degradation, allergic reactions, textile deterioration, and even health issues. As a result, making antimicrobial textiles has attracted the attention of a broad array of researchers, and the market for antimicrobial textiles is quickly growing. Silver, triclosan, biguanide derivatives, N-halamines, peroxyacids, quaternary ammonium salts, and synthetic colors are examples of antibiotic compounds (Zhang et al., 2016). Nanostructured materials based on metal nanoparticles have been intensively explored for diverse applications due to their appealing physical, chemical, and catalytic properties (Wiener et al., 2013). The molecular alteration of textile fabric to produce innovative materials that are several times more effective than untreated fabric is continually progressing. These materials focus on modifying the fabric surface for enhanced antibacterial properties, soil-resistance, water-repellency, antistatic, anti-infrared, and flame-retardant qualities of traditional textiles. Fabrics possess a huge surface area and pore volume. Thereby, nanoparticles quickly adsorb and can inactivate the bacteria and other microbes (Khan et al., 2020). The nanoparticles and structures are almost identical in size to biological molecules, which makes them an interesting candidate for biological study in vivo and in vitro (Nienhaus et al., 2020). They would have a wide range of uses in synthetic textiles; biomedical, surgical, and water treatment; equipment; food processing; and packaging (Alagarasan et al., 2021).
In natural and synthetic textiles, nanoparticle-based coatings are quite common and come in different compounds. Silver nanoparticles (AgNPs) have a high level of toxicity against a wide range of bacteria but have a low level of harmfulness to human cells and durable stability. Nanomaterials made of silver (Ag) are well known for their self-cleaning and antimicrobial properties (Goharshadi et al., 2022). The antiviral activity of AgNPs (size less than 10 nm) has also been observed against COVID-19. Moreover, silver, other metals, and metal oxide nanoparticles, such as gold, zinc, tin, titanium, and copper, are used in natural and synthetic fabrics. As explained previously, three basic mechanisms can be linked to the antibacterial activity of functionalized CuO NPs on textile materials against Gram-positive and Gram-negative bacteria: copper ion release, direct interaction of CuO NPs with bacteria, and the formation of reactive oxygen species (Gulati et al., 2021). Many surfaces are frequently touched and soiled with viruses (Figure 3), which require special attention for disinfection. Coating such surfaces with antivirals can significantly reduce the transmission of viruses and other microbes. Not much work is done in this direction. However, it is a very crucial area. In the following sections, we will focus on methods reported in the literature to deposit antiviral compounds on high-touch surfaces and fabrics.
Various methods are reported in the literature to deposit antivirals onto different surfaces. Table 2 shows the different methods besides the adopted methods, dip-coating, and sonication.
5.1 Dip-coating process
The dip-coating method is a simple and efficient technique. This method is commonly used in industries to deposit any substrate such as metallic, polymer films, and ceramic and fibrous materials. The deposition process can be defined as the coating of aqueous-based liquid phase solutions onto the surface of any substrate, and then the wet coating object is dried at room temperature (Tang and Yan, 2017). Furthermore, Lu et al. focused on endowed silk to introduce the antibacterial activity and UV shielding properties by depositing CeO2 nanoparticles on the surface of silk through the dip-coating method. Thermal stability properties are evaluated using thermogravimetric analysis and its derivatives. The successfully coated CeO2 nanoparticles on silk, the ability of UV-protection, and the antibacterial property were confirmed in UV–Vis diffuse reflectance spectroscopy and colony-forming capability test, indicating that CeO2 nanoparticles are successfully coated on silk and are the best-modified material for applications of UV-protection and antibacterial applications (Bhattacharjee et al., 2020). Another material was prepared using reduced graphene oxide (RGO) coated-copper (Cu)/silver (Ag) nanoparticles on carbon cloth via an easy dip-coating method and using a coupling agent (3-glycidyloxypropyl trimethoxy silane). The RGO and Cu/Ag composites were simultaneously coated on cotton cloth samples, which showed improved hydrophobicity compared to pure cotton. The surface resistance of cotton-RGO-Cu is 6.42 KΩ/sq, with a high UV protection factor (46.45). Furthermore, after 20 wash cycles, the resistance of cotton-RGO-Cu is 16.70 KΩ/sq. The cotton-RGO-Cu fabric properties include enhanced hydrophobicity, low surface resistance, better UV protection, and thermal stability (Bhattacharjee et al., 2020). In another work, the synthesized zinc oxide nanoparticles were coated on cotton fabrics. The zinc acetate solution in various concentrations with monoethanolamine with 2-methoxy-ethanol as solvent was used to obtain nanoparticles after an aging time of 24 h. The different concentrations of nanoparticles were coated on cotton fabric via the dip-coating method. The 3 M concentrations of ZnO were well-dispersed on the fabric shown via SEM images. The UV absorbance increase with the increase in the concentration. The 2 M concentration range was found to have the maximum antibacterial and antifungal activity (Roy et al., 2020). This report discusses the low-cost method of coating nylon fabric with an antimicrobial application.
A blend of chitosan and Ag nanoparticles coating on fabric via easy dipping with different concentrations of materials is reported. The synthesized silver nanoparticles using the Lee–Meisel method showed the surface plasmon resonance band at 410 nm corresponding to a stable average nanoparticle diameter of 25 nm. The prepared fabric controlled higher bactericidal activity (Gram-positive Staphylococcus aureus) compared to (Gram-negative Pseudomonas aeruginosa) bacteria. The result showed 20% reduction in S. aureus and 60% reduction in P. aeruginosa CFU, when each coating combination is exposed. The antimicrobial effect was decreased after several washes, indicating that after washing the fabric, the coated material becomes unstable and can be easily removed. However, for the purpose of single-use face masks, new-coated material is favorable (Roy et al., 2020).
In a report, SiO2 nanoparticles are coated on cashmere fabric via the dip-coating method using acetic acid to produce a highly superhydrophobic surface. Cashmere fabric had a superhydrophobic characteristic with a contact angle greater than 150°C, according to contact angle measurements. The coated material on the fabric does not affect the cashmere fabric. The colorfastness and pilling grade of the surface-coated cashmere were improved, so the coating method had no bad impact on the cashmere fabrics’ quality (Botelho et al., 2021).
In this report, graphene oxide (GO) and cuprous oxide (Cu2O) were produced and uniformly coated on polyethylene terephthalate (PET) fabric with excellent adhesion using dopamine.
The findings show that GO acts as a Cu2O nucleation site while avoiding agglomeration, and during Cu2O reduction, it is rehabilitated to RGO. The nanoparticles coated on PET fabric show great antibacterial activity against S. aureus and E. coli, more than 99.99%. However, after 40 washes of modified PET fabric, the antibacterial rates were 90% for S. aureus and 88% for E. coli. The decrease resistivity to 7.16 × 108 Ω cm from 2.64 × 1,015 Ω cm of the modified PET fabric range. Additionally, the UV protection was improved from 45 to 460, far exceeding the excellent rating (50). Overall, in the textile field, different pathways are opened for different applications (Hu et al., 2022).
5.2 Sonochemical coating process
The sonochemical method is one of the versatile methods for coating purposes. In 1975, a textile finishing process was first reported by ultrasound exposure through deep ultrasonic irradiation and cross-linking resins such as urea-formaldehyde. Harifi and Montazer (2015) published an outstanding review about textile sonoprocessing, describing the surface finishing of fabrics using various metals, metal oxides, and combinations and achievements. Many studies have been conducted using the sonochemical method to deposit nanoparticles [copper oxide (CuO), zinc oxide (ZnO), titanium dioxide (TiO2), magnesium oxide (MgO), silver (Ag), copper (Cu), Ag/TiO2, Zn/CuO, etc.]. Inorganic particles are used to functionalize textiles (Perelshtein et al., 2015; Perkas et al., 2018; Patil et al., 2020). The large surface area is a beneficial property of metal nano-oxide, which is suitable for coating textile fabrics. The market of antimicrobial NPs of metal oxide is a better substitute for quaternary ammonium salts, triclosan, and other toxic compounds. The main advantage of sonochemically coated textiles is that after 65 washing cycles, the coated fabric has good antibacterial efficiency. Furthermore, color and biocide functions are added to textiles through the sonochemical coating method. The composite ZnO/chitosan nanoparticles coated on textile through the sonochemical method were used to increase the antibacterial activity. The ZnO and chitosan are coated on fabric by a one-step deposition process and form hybrid antimicrobial layers. The antibacterial properties of the textiles were improved, as well as their biocompatibility. The sonochemical coating technique for 30 min using a dispersion of 2 mM ZnO nanoparticle provided an antibacterial effect for two pathologically important bacterium species. The responsivity against Staphylococcus aureus and Escherichia coli was higher for chitosan and ZnO deposited with the same quantity of only ZnO. The antibacterial robustness effects were increased by 21% for S. aureus and 40% for E. coli due to the presence of biopolymers. The coated fabric was stable for many washing cycles under hospital laundering conditions. (Petkova et al., 2014). Recently, Kwiczak-Yigitbas et al. (2020) reported an ultrasonication method for the synthesized environmentally friendly cellulose fabrics comprising silver or gold nanoparticles. The authors reported cellulose mechanochemistry in terms of breaking glycosidic bonds and producing mechano-radicals. The reduced metals can be stabilized by the cellulose chains on nanoparticles, and these mechano-radicals can decrease Au3þ and Agþ ions in the solution. Silver nanoparticle–fabric composites with antibacterial properties and the catalytical composite of active gold nanoparticle–fabric with metal ion reduction properties yield up to 14% using this approach. The cloth is sonicated in aqueous solutions without the use of harmful reducing and stabilizing agents. The availability of coated fabric for medical textile applications is rapid and environmentally friendly (Kwiczak-Yiǧitbaşı et al., 2020).
5.3 Pad-dry-cure process
Different deposition processes can be used for textile fabrics utilizing modified chitosan created using metal and metal oxide nanoparticles to generate novel function materials. Using the precipitation process, some researchers have synthesized chitosan–ZnO nanocomposite. Bio-nanocomposite materials were coated on fabric via pad-dry-cure (Beninate et al., 1968) and sol-gel (Sogorkova et al., 2018) methods to increase their washing elasticity. In some instances, the washing robustness of the coatings is improved using (3-glycidyloxypropyl) trimethoxysilane. The performances of antibacterial and UV protection were tested after the bio-nanocomposite coated on fabrics. The prepared chitosan–ZnO–TiO2 nanocomposite was also used to observe the variations in UV properties. Binary chitosan–ZnO composite shows good results for antibacterial and UV protection. The treated cotton fabrics improved the impacts of multiple washing cycles compared to simple chitosan-treated materials. By using a ternary coating composite, the UV protection factor is elevated to an excellent level.
Coating multifunctional technical cotton textiles using smart biomaterials is a revolutionary approach to multifunctional cotton textile design. Two distinct (NC1, NC2) ternary nanocomposites, containing (ammonium-salicylidene) chitosan Schiff base (ASCSB), TiO2, and ZnO nanoparticles, were successfully synthesized in situ and coated to cotton fibers using the simple pad-dry-cure procedure to impart antibacterial and UV protection properties. NC1 has much TiO2, whereas NC2 has much ZnO. Spectral, microscopic, and thermal approaches were used to evaluate the physicochemical and graphic features of the novel nanocomposites. NC1 exhibited a more homogeneous distribution, higher depositing density and smaller mean nanoparticle size (48 nm) when compared to NC2 (56 nm). NC2-treated fabrics, on the contrary, had a greater nanoparticle depositing than NC1-treated fabrics. The treated cotton fibers had robust and long-lasting antimicrobial effects against S. aureus, E. coli, and Candida albicans pathogens, with NC2-treated textiles performing better than NC1-treated textiles. The NC2-remediated cotton fabrics demonstrated a higher UV protection factor (UPF) value (53) as compared to NC1-coated fabrics (35), revealing that rich-ZnO nanocomposite provided higher UV protection to cotton fabrics than the rich-TiO2 nanocomposite (Refaee et al., 2022).
Carboxymethyl chitosan and Ag/TiO2 composite nanoparticles were coated on fabrics for long-term antibacterial and UV protection (Xu et al., 2021). The Ag/TiO2 colloid solution was stabilized with carboxymethyl chitosan, and the carboxymethyl chitosan and Ag/TiO2 composite nanoparticles were subsequently coated on cotton utilizing the pad-dry-cure process. The excellent properties of modified fabric with the bacterial reduction of 99.5% and UV protection factor of 79.0, respectively. Moreover, the coated fabric properties showed no change up to 50 washing cycles.
5.4 Spray-coating
The spray-coating method can be used to create superamphiphobic polymer coatings quickly and efficiently. Several surfaces have been examined as substrates for this coating technique, including paper, glass, cotton, aluminum, and copper (Steele et al., 2009; Wang et al., 2014). To create the desired coatings, different polymer-based fluorobinders (with low surface energy) and nanoparticles, such as silica and ZnO nanoparticles (denoted as SNs and ZNs, respectively, giving microscale and nanoscale roughness), are commonly mixed as building blocks and spray-coated onto a substrate. Furthermore, fluorinated SNs (FSNs) were employed to create coatings with increased durability with clearness (Wu et al., 2016). Sasaki et al. used double-walled carbon nanotubes (DWCNTs) combined with gold nanoparticles (AuNPs) via a simple and low-cost spray-coating approach to exhibit flexible conductive fabric (Yotprayoonsak et al., 2022). The rising need for surgical masks, as well as their disposal, has resulted in considerable financial and environmental expenses since the advent of the COVID-19 pandemic. The researchers used a dual-channel spray-assisted nanocoating hybrid of shellac/copper nanoparticles (CuNPs) to increase the hydrophobicity of a non-woven surgical mask and repel aqueous droplets. The resultant surface exhibits excellent photoactivity for antimicrobial action (combined photocatalytic and photothermal capabilities), allowing the masks to be reused and self-sterilized. This photoactive antiviral mask (PAM) immediately reached a temperature of >70°C when exposed to sunlight. The masks became self-cleaning and reusable. This PAM architecture can protect against viral infection transmission (Kumar et al., 2020). Copper is a common substance that has antibacterial and antiviral properties. The antiviral activity of copper nanoparticles (CuNPs) was investigated against SARS-CoV-2 as a surface coating agent. The diameter range of 254 nm of CuNPs was formed using copper sulfate as a source and was primarily made up of CO. Combined CuNPs, and resin-based paint sprayed on the stainless-steel surface remains (CuNP/paint). After 30 min of exposure, SARS-CoV-2 lost 97.8% of its infectivity on the CuNP/paint-coated surface, and in the following 1 h, it lost more than 99.995% of its infectivity. The inactivation rate on the paint alone-coated and uncoated surfaces was roughly 36-fold faster. Although more studies are needed to explain the inactivation processes, the CuNP/paint-coated surface displayed strong inactivation of SARS-CoV-2 infectivity. This coating material is expected to be useful in public hospitals and other frequently handled places (Purniawan et al., 2022).
Using a one-step spray-coating method, we describe a straightforward and universal strategy for optimal coupling superhydrophobic and antibacterial properties on diverse textiles. This is accomplished by adhering fluorinated mesoporous silica nanoparticles (F-MSNs) and quaternary ammonium-functionalized MSNs (Q-MSNs) to various textile surfaces using PDMS as a binder. CAs of 152 and SAs of 2 indicate that the resultant double-nanoparticle arranged coatings on textiles (F/Q-MSNs coatings) have significant antibacterial activity against E. coli and S. aureus due to the “repel-and-kill” synergic effect and excellent waterproof and bacterial shielding function. In addition, F/Q-MSN coatings are resistant to sandpaper abrasion, washing, and strong acid/alkaline. Furthermore, the F/Q-MSN coating can maintain the textile’s original application characteristics, such as breathability and deformability. As a result, F/Q-MSN has developed antibacterial textile coatings that are very practicable, adaptable, and universal, with potential and diversified applications in various fields (Ye et al., 2021).
Many academic and industry researchers have attempted to produce fabrics with superhydrophobic properties inspired by various environmental life forms’ excessive wettability, such as lotus leaves’ high-water repellency. A novel wettability switching mechanism has recently sparked a surge in demand for advanced coatings, even though their production remains difficult and expensive. Cotton fabrics with irregular wettability (one face with natural superhydrophobicity, the other face with natural superhydrophilicity) were made by spraying a biocompatible commercial material mixture, hydrophobic SiO2 nanoparticles, and ethyl-alphacyanoacrylate superglue onto the fabric in one step. The method involves adjusting the distance between the sprayer and the fabric to make one side naturally superhydrophilic and the other superhydrophobic, thus managing the absorption of the fabric coatings. As a result of its great mechanical durability, the superhydrophobic side has a surface with a water contact angle of 154°C and a sliding angle of 16°C meet the standards for self-cleaning capabilities. Due to the fabric’s intrinsic superhydrophilic feature, the reverse side had a strong water absorption ability. Furthermore, the superhydrophilic side of the created cotton materials consumed blood absorption and clotting characteristics. On the contrary, the superhydrophobic side prohibited water and blood infiltration sacrificing the cotton’s intrinsic breathability. These features could be important in the development of multifunctional materials for medical purposes (Sasaki et al., 2016)*.
5.5 Magnetron sputtering
Cathodic magnetron spraying has become the process of choice for the deposition of a wide range of coatings of industrial importance. Examples include hard and wear-resistant, low friction, corrosion-resistant, decorative coatings, and coatings with specific optical or electrical properties. In the primary sputtering process, a target plate (or cathode) is bombarded by energy-sensitive ions produced in the glow-emitting plasma located in front of the target. The bombardment process causes the removal of the target atoms (i.e., sputtering), which can then condense on the substrate as a thin film (Kelly and Arnell, 2000). The deposition rate and coating thickness are influenced by the ionization and collision rates of argon (a gas used to generate plasma) on the target material in the sputtering system. A magnetic field can be produced by placing the magnets underneath the target. These secondary electrons trap through the magnetic field that the target emits into the discharge and raises the ionization of the gas, which increases the rate of collisions between the Ar+ and the target material. In changing the target material’s chemical makeup and the operational conditions, the stoichiometry of the film can be easily changed. Introducing N2, C2H2, and O2 gases together with the Ar gas, nitride, carbide, and oxide coatings may be easily created (Golosov, 2017). The most common substrates for the deposition of films using MS techniques are metals or glass. However, in recent years, the application of MS for the deposition of metallic and oxide coatings on textiles has become more widespread (Liu et al., 2017; Antunes et al., 2021; Huang et al., 2021b). Most of the coated films are tested against antibacterial properties (Kim et al., 2018; Markowska-Szczupak et al., 2021; Worananthakij et al., 2022), whereas limited literature is available on MS-coated films for antiviral assessment.
Table 3 lists various MS modes used to create coatings on various substrates to develop antiviral surfaces. A highly effective antiviral surface coating, a highly imperfect, amorphous TiOx (x 1.2), was created. The antiviral activity was noticeably stronger, comparing this amorphous shape to the more often studied crystalline anatase phase. It was discovered that the covering was remarkably clear. This film was created at room temperature without the need for any post-deposition thermal treatment using radio frequency (RF) magnetron sputtering, one of the well-known industrial-scale deposition methods. The potential for commercialization of this antiviral surface coating is enormous due to its affordability and environmental friendliness (Mittireddi et al., 2021). Applying a reliable method based on Cu magnetron sputter-deposition onto electrospun polymer nanofibers, the Cu-coated PCL (polycaprolactone) nanofibrous mats were effectively created (Manakhov et al., 2021). For the first time, molecular dynamics simulation was used to describe the large-scale irradiation of PCL films, allowing for the prediction of ion penetration depth and fine-tuning of deposition conditions. Because of the quick release of Cu2+ ions (concentration up to 3.4 μg/ml), acceptable biocompatibility, and antibacterial activity against E. coli and S. aureus, the copper-coated PCL nanofibers demonstrated that they were effective. Thus, when used for wound healing, they might show an intriguing synergistic effect. Because copper ions are required for immune system cells to act in a bacteriostatic way, the quick discharge of copper ions simultaneously kills germs and activates immune cells, stimulating regeneration.
The potential of plasma techniques was reviewed by Ma et al. (2021), concluding that “impressive recent advances in plasma functionalization of polymer surfaces suggest that plasma-assisted surface functionalization approaches are promising for the production of antiviral polymers with targeted antiviral applications ranging from in vitro prevention to in vivo therapy.”
6 Antimicrobial textiles and fabrics for protective applications
The textile industry is flourishing, and there is an urgent need to develop environmentally friendly and effective antimicrobial fabrics (Mallakpour et al., 2021). Healthcare services are crowded with patients having different diseases, where harmful microorganisms are more prevalent than in other places. Additionally, employees of healthcare facilities are at high risk for viruses. Compared to the general population, healthcare employees are more at risk; therefore, the use of antiviral fabrics is of utmost importance in healthcare centers.
During the COVID-19 pandemic, around 210 countries and all regions were affected, mostly via respiratory droplets. The doctors, patients, and the public used disposable surgical masks in high-risk areas. Masks played an important role during the COVID-19 pandemic, the spread of droplets was blocked, and the health of people was safeguarded. Recently, many researchers worked on antivirus masks (Tuñón-Molina et al., 2021). Borkow et al. reported a copper intermediate layer with an antiviral mask, containing four layers. The A and B layers consist of the outer and inner layers: (A) spunbonded polyamide fabric containing 2.2% weight (w/w) copper particles; (B) fused polypropylene fabric containing 2% (w/w) CO particles offering physical filtering performance of the mask and serving as a blockage layer; (C) inner layer made of polyester and used to mold the mask; and (A) outer layer the same as the inner layer (C). Without affecting the physical properties of the copper oxide, it acts as a potent anti-influenza biocidal agent when applied to a breathing mask (Zhong et al., 2020). Zhong et al. used laser induction to create a graphene layer over a typical surgical mask that kills viruses with electrothermal characteristics (Borkow et al., 2008). Li et al. (2019) reported a mask with two layers of non-woven fabric as biological sterilization in the intermediate layer MO Filter. The sterilization performance of the N95 mask is excellent and does not interfere with adsorption. Tang et al. sun-induced antiviral cotton fabrics made from cationic cotton fibers and anionic photosensitizers were durable and reusable and were used for face masks and protective garments. In the middle adsorption layer of the mask, antiviral materials were added. The hydrophobic and hydrophilic layers are the upper and lower layers, respectively. Moisture permeability, surface moisture, and resistance can all be improved using materials with high wettability, resulting in a disease-resistant and comfortable mask. For up to 60 min of sun exposure, virus substitutes (T7 pages) had a microbiome reduction rate of unevenly 5–6 logs (Li et al., 2017b).
Aside from the two main applications described above, other possibilities for infections are related to locations, for example, transportation and catering businesses. The possibilities of cross-infection can be considerably decreased if seat materials, interior textiles, seat belts, rugs, tablecloths, towels, and other textiles contain antiviral qualities (Zhang et al., 2021).
Henceforth, the spread of contagious diseases can largely be minimized by deploying antiviral/antimicrobial textiles/surfaces all over, specifically in hospital settings and high-touch public places.
7 Testing virucidal activity
International standards can be employed to ensure that disinfection products are of the highest quality and employed in the most effective strategies to prevent and control microbial contamination in healthcare settings. A book chapter that describes testing methods includes globally utilized documentation of testing techniques for virucidal activity (Woodall and Walker, 2020). A method for testing non-porous solid surfaces is recommended by the US EPA (EPA, 2016). However, the testing method for porous solid surfaces was unavailable before 2019.
Uniformity in testing procedures is important not only for comparison purposes of various reported materials but also for the commercialization of final products. Throughout the literature, scientists have used different approaches to test their developed antiviral products, and therefore, it is hard to compare the efficiency. However, ISO: 18184 is currently available for testing non-porous materials/textile products. Hence, it is anticipated that the upcoming literature will have data that could be employed to find reliable comparisons. Due to the lack of standardized protocols before the ISO 18184 standard, it is also hard to compare various surfaces reported in the literature (Walji and Aucoin, 2020).
Only a few methods have been documented in the literature aside from internationally accepted norms. An easy technique is presented, for instance, for vacuum-freezing viruses on an inanimate surface (coverslips). For the virucidal assay of disinfectants, coverslips are exposed to a medium mimicking the disinfectant (viral control) or disinfectant while upright in an Ultra-VU cuvette. The method also recommended the calculation of the means of the titers after each experiment. With this straightforward method, repetitions could be carried out with ease (Allen et al., 1988).
Another method reported the significance of large-volume plating (LVP) (Kampf et al., 2020). In this method, “sample volume” should be properly defined, whether it is a diluted or an actual sample. This report discussed materials and procedures for the in situ detection of living microorganisms on surfaces, specifically hard surfaces. The presence of the target microorganism is detected using the bacteriophage’s specificity for a target host organism or microbe. This patented report avoids the shortcomings of sampling and off-site detection by relying instead on in situ detection of the target microorganism. It does not require surface sampling processes or laboratory-based detection methods (Voorhees, 2011). Recently, a method has been suggested to use bacteriophage phi 6 as a substitute for SARS CoV-2 (Serrano-Aroca, 2022). The use of bacteriophage phi 6 allows carrying experiments even to the researcher who does not have access to BSL3 laboratory facilities.
8 Conclusion
Protection of high-touch surfaces (porous and non-porous) against the spread of viruses has become crucially important after the impact of COVID-19. Existing practices and policies require revision and quick implementation of revised policies and procedures. Disinfection must be taken as a holistic approach. In addition to commercially available disinfectants based on alcohol, quaternary ammonium compounds and aldehyde can effectively be combined in a dual strategy with solid copper alloy surfaces to reduce microbial contamination.
Copper, silver, zinc (their oxides), and TiO2 are the most common types of metals reported to produce nanoparticles. Iron has also shown activity, but reports on iron are limited. Compared to metals, metal oxides have shown better response. Copper-based nanoparticles in small doses show faster kinetics in the deactivation of viruses. The mode of action for metallic nanoparticles is attributed to the attachment of spike proteins, production of free radicals, cell lipid layer destruction for enveloped viruses, and the hydrophobicity of nanomaterial-treated fabric. Enhanced activity of copper oxide may be due to the inherent multiple characteristics of copper, such as redox, catalytic (OH production), charge, and stability, in addition to the properties due to the nanosize of particles.
Among organic nanostructures, fullerenes, graphene, and graphene oxide and their functionalized derivatives are reported. The results of molecular docking revealed that graphene oxide sheets interact with three types of structure present in SARS-CoV-2 (i.e., closed state -6VXX or open state -6VYB (viral spike), open state -6VYB or closed state -6VXX (ACE2-bound spike complex) and 1R42 (ACE2). Graphene oxide showed good binding affinities toward all three surface structures 6VXX, 6M0J, and 6VYB.
Modifications of graphene oxide with various carbon chain lengths were synthesized and investigated. It is found that an alkyl chain greater than C9 can disturb the replication of coronavirus by disturbing its envelope. At first viral envelope is damaged when it interacts with graphene oxide, which leads to the production of ROS and virus deactivation. The lower surface energy of certain materials, along with roughness at the nanoscale, can play a great role in increasing the antiviral properties of materials; especially in the case of treated fabric or PPE, it can increase antiviral properties and superhydrophobicity.
Furthermore, among organic nanoparticles, coating of fullerene derivatives on the surface can prevent the possible attachment of SARS-CoV-2 because it produces lipid peroxidation on the lipid layer of SARS-CoV-2 and creates a hydrophobic surface that minimizes the contact between the surface and the virus due to the air entrapped between topographies.
Another important group of antiviral nanoparticles is photocatalytic materials. TiO2 nanoparticles deposited on the surface of the glass or another inanimate surface have shown excellent virus deactivation under UV light. However, this approach would be limited to surfaces exposed to UV light and may not be effective in indoor places. Among emerging materials, g-C3N4 may be a promising candidate as a photocatalyst-based virucidal compound. Films of g-C3N4 have shown a response time of 2.4 min in killing certain microbes.
Stable and convenient methods for the deposition or incorporation of nano-antivirals on solid surfaces are necessary for the scaled production of antiviral surface coatings in the public domain. Various industrially adopted methods, for example, dip-coating, pad-dry-cure, spray coating, and magnetron sputtering methods, are tested and have shown some success. The first two methods are suitable for producing textiles, whereas the latter technique is useful for non-porous surfaces such as glass and steel. Most methods reported to incorporate nano-antivirals using dip-coating and pad-dry-cure have employed linkers (3-glycidyloxypropyl, chitosan, and monoethanolamine) for stabilizing the coating for multiple wash cycles. In a report, graphene oxide (GO) and cuprous oxide (Cu2O) were produced and uniformly coated on polyethylene terephthalate (PET) fabric with excellent adhesion using dopamine.
Sonochemical methods are becoming increasingly popular; however, they are still not found in regular textile industry setups. In the reported literature, sonochemical methods are considered faster than other methods for depositing nanoparticles on textile surfaces. In the reported sonochemical methods, linkers are also used to attach nano-antiviral on the fabric surface. However, there is a report without the use of any linker. Cellulose’s mechanochemistry is linked with the linker-free deposition of Ag/Au nanoparticles in terms of the breaking of glycosidic bonds and the production of mechano-radicals.
Copper has proven itself, whereas other virucidal materials are on the way. Copper has many faces in medical treatment and may be “New Gold” in solid self-sanitizing solid surfaces either as innate metal or surface coatings.
It may be concluded that the spread of contagious diseases can largely be minimized by deploying antiviral/antimicrobial textiles/surfaces and can be employed specifically in hospital settings and high-touch public places. Copper-based nanoparticles have great potential, and research should be pushed to develop eco-friendly, less expensive, and scalable approaches for the commercialization of nano-antiviral-based products.
Author contributions
FSH contributed to the drafting and literature survey of the first four sections, figure drawing, and harmonizing the whole draft. NQA contributed to the rest of the draft manuscript. NM conceived the idea and polished the draft. SQM contributed to the structure of the draft and adding tables. NA improvised the language and added a section.
Acknowledgments
The authors are thankful to the Sindh Higher Education Commission, Karachi, Pakistan, for funding the research through the project “Sindh Research Support Program,” SHEC/SRSP/APS-1/1/2020-21.
Conflict of interest
The authors declare that the research was conducted in the absence of any commercial or financial relationships that could be construed as a potential conflict of interest.
Publisher’s note
All claims expressed in this article are solely those of the authors and do not necessarily represent those of their affiliated organizations or those of the publisher, the editors, and the reviewers. Any product that may be evaluated in this article, or claim that may be made by its manufacturer, is not guaranteed or endorsed by the publisher.
References
Abate, C., Carnamucio, F., Giuffrè, O., and Foti, C. J. B. (2022). Metal-based compounds in antiviral therapy. Biomolecules 12, 933. doi:10.3390/biom12070933
Abo-El-Yazid, Z. H., Ahmed, O. K., El-Tholoth, M., and Ali, M. A.-S. (2022). "Green synthesized silver nanoparticles using Cyperus rotundus L. extract as a potential antiviral agent against infectious laryngotracheitis and infectious bronchitis viruses in chickens, Chem. Biol. Technol. Agric. 9, 1–11. doi:10.1186/s40538-022-00325-z
Abou Elmaaty, T., Sayed-Ahmed, K., Elsisi, H., Ramadan, S. M., Sorour, H., Magdi, M., et al. (2022). Novel antiviral and antibacterial durable polyester fabrics printed with selenium nanoparticles (SeNPs). Polym. (Basel). 14, 955. doi:10.3390/polym14050955
áde Leon, A., Santos, C. M., Felipe, M. J. L., de Leon, A. C. C., Rodrigues, D. F., and Advincula, R. C. (2015). On the antibacterial mechanism of graphene oxide (GO) Langmuir–Blodgett films. Chem. Commun. 51, 2886–2889. doi:10.1039/c4cc07836e
Ahn, E.-Y., and Park, Y. (2020). Anticancer prospects of silver nanoparticles green-synthesized by plant extracts. Mater. Sci. Eng. C 116, 111253. doi:10.1016/j.msec.2020.111253
Ahsan, T. (2020). Biofabrication of silver nanoparticles from Pseudomonas fluorescens to control tobacco mosaic virus. Egypt. J. Biol. Pest Control 30, 66–74. doi:10.1186/s41938-020-00268-3
Akhtar, S., Shahzad, K., Mushtaq, S., Ali, I., Rafe, M. H., and Fazal-ul-Karim, S. M. (2019). Antibacterial and antiviral potential of colloidal Titanium dioxide (TiO2) nanoparticles suitable for biological applications. Mat. Res. Express 6, 105409. doi:10.1088/2053-1591/ab3b27
Alagarasan, D., Harikrishnan, A., Surendiran, M., Indira, K., Khalifa, A. S., and Elesawy, B. H. (2021). Synthesis and characterization of CuO nanoparticles and evaluation of their bactericidal and fungicidal activities in cotton fabrics. Appl. Nanosci., 1–10. doi:10.1007/s13204-021-02054-5
Alavi, M., Kamarasu, P., McClements, D. J., and Moore, M. D. (2022). Metal and metal oxide-based antiviral nanoparticles: Properties, mechanisms of action, and applications. Adv. Colloid Interface Sci. 306, 102726. doi:10.1016/j.cis.2022.102726
Allen, L. B., Jo Kehoe, M., Hsu, S. C., Barfield, R., Holland, C. S., and Dimitrijevich, S. D. (1988). A simple method of drying virus on inanimate objects for virucidal testing. J. Virological Methods 19, 239–247. doi:10.1016/0166-0934(88)90018-3
Al-Shmgani, H. S., Mohammed, W. H., Sulaiman, G. M., and Saadoon, A. H. (2017). Biosynthesis of silver nanoparticles from Catharanthus roseus leaf extract and assessing their antioxidant, antimicrobial, and wound-healing activities. Artif. Cells Nanomed. Biotechnol. 45, 1234–1240. doi:10.1080/21691401.2016.1220950
Antunes, J., Matos, K., Carvalho, S., Cavaleiro, A., Cruz, S. M. A., and Ferreira, F. (2021). Carbon-based coatings in medical textiles surface functionalisation: An overview. Processes 9, 1997. doi:10.3390/pr9111997
Attia, G. H., Moemen, Y. S., Youns, M., Ibrahim, A. M., Abdou, R., and El Raey, M. A. (2021). Antiviral zinc oxide nanoparticles mediated by hesperidin and in silico comparison study between antiviral phenolics as anti-SARS-CoV-2. Colloids Surfaces B Biointerfaces 203, 111724. doi:10.1016/j.colsurfb.2021.111724
Ayub, M., Othman, M. H. D., Khan, I. U., Yusop, M. Z. M., and Kurniawan, T. A. (2021). Graphene-based nanomaterials as antimicrobial surface coatings: A parallel approach to restrain the expansion of COVID-19. Surfaces Interfaces 27, 101460. doi:10.1016/j.surfin.2021.101460
Balagna, C., Francese, R., Perero, S., Lembo, D., and Ferraris, M. (2021). Nanostructured composite coating endowed with antiviral activity against human respiratory viruses deposited on fibre-based air filters. Surf. Coatings Technol. 409, 126873. doi:10.1016/j.surfcoat.2021.126873
Banerjee, A., Qi, J., Gogoi, R., Wong, J., and Mitragotri, S. (2016). Role of nanoparticle size, shape and surface chemistry in oral drug delivery. J. Control. Release 238, 176–185. doi:10.1016/j.jconrel.2016.07.051
Baram-Pinto, D., Shukla, S., Perkas, N., Gedanken, A., and Sarid, R. (2009). Inhibition of herpes simplex virus type 1 infection by silver nanoparticles capped with mercaptoethane sulfonate. Bioconjug. Chem. 20, 1497–1502. doi:10.1021/bc900215b
Basheer, N. S., Kumar, B. R., Kurian, A., and George, S. D. (2013). Silver nanoparticle size–dependent measurement of quantum efficiency of Rhodamine 6G. Appl. Phys. B 113, 581–587. doi:10.1007/s00340-013-5513-3
Bataglioli, R. A., Rocha Neto, J. B., Calais, G. B., Lopes, L. M., Tsukamoto, J., de Moraes, A. P., et al. (2022). Hybrid alginate–copper sulfate textile coating for coronavirus inactivation. J. Am. Ceram. Soc. 105, 1748–1752. doi:10.1111/jace.17862
Behzadi, M., Vakili, B., Ebrahiminezhad, A., and Nezafat, N. (2021). Iron nanoparticles as novel vaccine adjuvants. Eur. J. Pharm. Sci. 159, 105718. doi:10.1016/j.ejps.2021.105718
Behzadinasab, S., Chin, A., Hosseini, M., Poon, L., and Ducker, W. A. (2020). A surface coating that rapidly inactivates SARS-CoV-2. ACS Appl. Mater. Interfaces 12 (31), 34723–34727.
Bekele, A. Z., Gokulan, K., Williams, K. M., and Khare, S. (2016). Dose and size-dependent antiviral effects of silver nanoparticles on feline calicivirus, a human norovirus surrogate. Foodborne Pathog. Dis. 13, 239–244. doi:10.1089/fpd.2015.2054
Bello-Lopez, J., Silva-Bermudez, P., Prado, G., Martínez, A., Ibáñez-Cervantes, G., Cureño-Díaz, M. A., et al. (2021). Biocide effect against SARS-CoV-2 and ESKAPE pathogens of a noncytotoxic silver–copper nanofilm. Biomed. Mat. 17 (1), 015002. doi:10.1088/1748-605x/ac3208
Beninate, J. V., Boylston, E. K., Drake, G. L., and Reeves, W. A. (1968). Conventional pad-dry-cure process for durable-flame and wrinkle resistance with tetrakis (hydroxymethyl) phosphonium hydroxide (THPOH). Text. Res. J. 38, 267–272. doi:10.1177/004051756803800307
Bertram, S., Glowacka, I., Müller, M. A., Lavender, H., Gnirss, K., Nehlmeier, I., et al. (2011). Cleavage and activation of the severe acute respiratory syndrome coronavirus spike protein by human airway trypsin-like protease. J. Virol. 85, 13363–13372. doi:10.1128/jvi.05300-11
Bhattacharjee, S., Macintyre, C. R., Wen, X., Bahl, P., Kumar, U., Chughtai, A. A., et al. (2020). Nanoparticles incorporated graphene-based durable cotton fabrics. Carbon 166, 148–163. doi:10.1016/j.carbon.2020.05.029
Bhosale, M., and Bhanage, B. (2015). Silver nanoparticles: Synthesis, characterization and their application as a sustainable catalyst for organic transformations. Curr. Org. Chem. 19, 708–727. doi:10.2174/1385272819666150207001154
Birkett, M., Dover, L., Cherian Lukose, C., Wasy Zia, A., Tambuwala, M. M., and Serrano-Aroca, Á. (2022). Recent advances in metal-based antimicrobial coatings for high-touch surfaces. Int. J. Mol. Sci. 23, 1162. doi:10.3390/ijms23031162
Borkow, G., and Gabbay, J. (2004). Putting copper into action: copper-impregnated products with potent biocidal activities. FASEB J. 18, 1728–1730. doi:10.1096/fj.04-2029fje
Borkow, G., Lara, H. H., Covington, C. Y., Nyamathi, A., and Gabbay, J. (2008). Deactivation of human immunodeficiency virus type 1 in medium by copper oxide-containing filters. Antimicrob. Agents Chemother. 52, 518–525. doi:10.1128/aac.00899-07
Borkow, G., Zhou, S. S., Page, T., and Gabbay, J. (2010). A novel anti-influenza copper oxide containing respiratory face mask. PloS one 5, e11295. doi:10.1371/journal.pone.0011295
Borrego, B., Lorenzo, G., Mota-Morales, J. D., Almanza-Reyes, H., Mateos, F., López-Gil, E., et al. (2016). Potential application of silver nanoparticles to control the infectivity of Rift Valley fever virus in vitro and in vivo. Nanomedicine Nanotechnol. Biol. Med. 12, 1185–1192. doi:10.1016/j.nano.2016.01.021
Botelho, C. M., Fernandes, M. M., Souza, J. M., Dias, N., Sousa, A. M., Teixeira, J. A., et al. (2021). New textile for personal protective equipment—plasma chitosan/silver nanoparticles nylon fabric. Fibers 9 (3), 3. doi:10.3390/fib9010003
Botequim, D., Maia, J., Lino, M., Lopes, L., Simões, P., Ilharco, L., et al. (2012). Nanoparticles and surfaces presenting antifungal, antibacterial and antiviral properties. Langmuir 28, 7646–7656. doi:10.1021/la300948n
Bregnocchi, A., Jafari, R., and Momen, G. (2022). Design strategies for antiviral coatings and surfaces: A review. Appl. Surf. Sci. Adv. 8, 100224. doi:10.1016/j.apsadv.2022.100224
Bridge, J. W., Oliver, D. M., Chadwick, D., Godfray, H. C. J., Heathwaite, A. L., Kay, D., et al. (2010). Engaging with the water sector for public health benefits: waterborne pathogens and diseases in developed countries. Bull. World Health Organ. 88, 873–875. doi:10.2471/blt.09.072512
Brieger, A., Rink, L., and Haase, H. (2013). Differential regulation of TLR-dependent MyD88 and TRIF signaling pathways by free zinc ions. J. I. 191, 1808–1817. doi:10.4049/jimmunol.1301261
Cai, X., Tan, S., Lin, M., Xie, A., Mai, W., Zhang, X., et al. (2011). Synergistic antibacterial brilliant blue/reduced graphene oxide/quaternary phosphonium salt composite with excellent water solubility and specific targeting capability. Langmuir 27, 7828–7835. doi:10.1021/la201499s
Castro, E., Martinez, Z. S., Seong, C.-S., Cabrera-Espinoza, A., Ruiz, M., Hernandez Garcia, A., et al. (2016). Characterization of new cationic N, N-Dimethyl [70] fulleropyrrolidinium iodide derivatives as potent HIV-1 maturation inhibitors. J. Med. Chem. 59, 10963–10973. doi:10.1021/acs.jmedchem.6b00994
Castro, E., Garcia, A. H., Zavala, G., and Echegoyen, L. (2017). Fullerenes in biology and medicine. J. Mat. Chem. B 5, 6523–6535. doi:10.1039/c7tb00855d
Castro-Mayorga, J. L., Randazzo, W., Fabra, M. J., Lagaron, J., Aznar, R., Sánchez, G., et al. (2017). Antiviral properties of silver nanoparticles against norovirus surrogates and their efficacy in coated polyhydroxyalkanoates systems. LWT - Food Sci. Technol. 79, 503–510. doi:10.1016/j.lwt.2017.01.065
Chen, N., Zheng, Y., Yin, J., Li, X., and Zheng, C. (2013). Inhibitory effects of silver nanoparticles against adenovirus type 3 in vitro. J. Virological Methods 193, 470–477. doi:10.1016/j.jviromet.2013.07.020
Chen, Y.-N., Hsueh, Y.-H., Hsieh, C.-T., Tzou, D.-Y., Chang, P.-L., and health, p. (2016). Antiviral activity of graphene–silver nanocomposites against non-enveloped and enveloped viruses. Int. J. Environ. Res. Public Health 13, 430. doi:10.3390/ijerph13040430
Cieślak, M., Kowalczyk, D., Krzyżowska, M., Janicka, M., Witczak, E., and Kamińska, I. (2022). Effect of Cu modified textile structures on antibacterial and antiviral protection. Materials 15, 6164. doi:10.3390/ma15176164
Cioffi, N., Torsi, L., Ditaranto, N., Tantillo, G., Ghibelli, L., Sabbatini, L., et al. (2005). Copper nanoparticle/polymer composites with antifungal and bacteriostatic properties. Chem. Mat. 17, 5255–5262. doi:10.1021/cm0505244
Cornelis, G., Pang, L., Doolette, C., Kirby, J. K., and McLaughlin, M. J. (2013). Transport of silver nanoparticles in saturated columns of natural soils. Sci. Total Environ. 463, 120–130. doi:10.1016/j.scitotenv.2013.05.089
Corsi, L., Lombardi, L., and Nesti, S. (2015). Bactericidal and virucidal covering material and method for making the covering material. US Patent.
Cortes, A. A., and Zuñiga, J. M. (2020). The use of copper to help prevent transmission of SARS-coronavirus and influenza viruses. A general review. Diagn. Microbiol. Infect. Dis. 98, 115176. doi:10.1016/j.diagmicrobio.2020.115176
Dakal, T. C., Kumar, A., Majumdar, R. S., and Yadav, V. (2016). Mechanistic basis of antimicrobial actions of silver nanoparticles. Front. Microbiol. 7, 1831. doi:10.3389/fmicb.2016.01831
Dang, H. T., and Tarabara, V. V. (2019). Virus deposition onto polyelectrolyte-coated surfaces: A study with bacteriophage MS2. J. colloid interface Sci. 540, 155–166. doi:10.1016/j.jcis.2018.12.107
Das, T. K., Karmakar, S., Maiti, S., Kundu, S., and Saha, A. (2020). Room temperature synthesis of NIR emitting Ag2S nanoparticles through aqueous route and its influence on structural modulation of DNA. Spectrochimica Acta Part A Mol. Biomol. Spectrosc. 227, 117536. doi:10.1016/j.saa.2019.117536
Das, G., Patra, J. K., and Shin, H.-S. (2020). Biosynthesis, and potential effect of fern mediated biocompatible silver nanoparticles by cytotoxicity, antidiabetic, antioxidant and antibacterial, studies. Mater. Sci. Eng. C 114, 111011. doi:10.1016/j.msec.2020.111011
Das Jana, I., Kumbhakar, P., Banerjee, S., Gowda, C. C., Kedia, N., Kuila, S. K., et al. (2020). Copper nanoparticle–graphene composite-based transparent surface coating with antiviral activity against influenza virus. ACS Appl. Nano Mat. 4, 352–362. doi:10.1021/acsanm.0c02713
De Graaf, M., van Beek, J., and Koopmans, M. P. (2016). Human norovirus transmission and evolution in a changing world. Nat. Rev. Microbiol. 14, 421–433. doi:10.1038/nrmicro.2016.48
De Gusseme, B., Hennebel, T., Christiaens, E., Saveyn, H., Verbeken, K., Fitts, J. P., et al. (2011). Virus disinfection in water by biogenic silver immobilized in polyvinylidene fluoride membranes. Water Res. 45, 1856–1864. doi:10.1016/j.watres.2010.11.046
de Roda Husman, A. M., and Bartram, J. (2007). Chapter 7 global supply of virus-safe drinking water. Perspect. Med. Virol. 17, 127–162. doi:10.1016/S0168-7069(07)17007-5
Demchenko, V., Rybalchenko, N., Zahorodnia, S., Naumenko, K., Riabov, S., Kobylinskyi, S., et al. (2022). Preparation, characterization, and antimicrobial and antiviral properties of silver-containing nanocomposites based on polylactic acid–chitosan. ACS Appl. Bio Mat. 5, 2576–2585. doi:10.1021/acsabm.2c00034
Deokar, A. R., Nagvenkar, A. P., Kalt, I., Shani, L., Yeshurun, Y., Gedanken, A., et al. (2017). "Graphene-based “hotplate” for the capturing and destruction of the herpes simplex virus type."Bioconjugate Chem. 28 (4), 1115-1122. doi:10.1021/acs.bioconjchem.7b00030
Deshmukh, S. P., Patil, S., Mullani, S., and Delekar, S. (2019). Silver nanoparticles as an effective disinfectant: A review. Mater. Sci. Eng. C 97, 954–965. doi:10.1016/j.msec.2018.12.102
Deyá, C., and Bellotti, N. (2017). Biosynthesized silver nanoparticles to control fungal infections in indoor environments. Adv. Nat. Sci. Nanosci. Nanotechnol. 8, 025005. doi:10.1088/2043-6254/aa6880
Díez-Pascual, A. M. (2020). Antibacterial action of nanoparticle loaded nanocomposites based on graphene and its derivatives: A mini-review. Int. J. Mol. Sci. 21, 3563. doi:10.3390/ijms21103563
Donskyi, I. S., Nie, C., Ludwig, K., Trimpert, J., Ahmed, R., Quaas, E., et al. (2021). Graphene sheets with defined dual functionalities for the strong SARS-CoV-2 interactions. Small 17, 2007091. doi:10.1002/smll.202007091
Du, T., Liang, J., Dong, N., Liu, L., Fang, L., Xiao, S., et al. (2016). Carbon dots as inhibitors of virus by activation of type I interferon response. Carbon 110, 278–285. doi:10.1016/j.carbon.2016.09.032
Du, T., Liang, J., Dong, N., Lu, J., Fu, Y., Fang, L., et al. (2018). Glutathione-capped Ag2S nanoclusters inhibit coronavirus proliferation through blockage of viral RNA synthesis and budding. ACS Appl. Mat. Interfaces 10, 4369–4378. doi:10.1021/acsami.7b13811
Du, T., Lu, J., Liu, L., Dong, N., Fang, L., Xiao, S., et al. (2020). Correction to “antiviral activity of graphene oxide–silver nanocomposites by preventing viral entry and activation of the antiviral innate immune response”. ACS Appl. Bio Mat. 3, 3440. doi:10.1021/acsabm.0c00434
Dung, T. T. N., Nam, V. N., Nhan, T. T., Ngoc, T. T. B., Minh, L. Q., Nga, B. T. T., et al. (2020). Silver nanoparticles as potential antiviral agents against African swine fever virus. Mat. Res. Express 6, 1250g9. doi:10.1088/2053-1591/ab6ad8
Dykman, L. A. (2020). Gold nanoparticles for preparation of antibodies and vaccines against infectious diseases. Expert Rev. vaccines 19, 465–477. doi:10.1080/14760584.2020.1758070
Elbeshehy, E. K., Elazzazy, A. M., and Aggelis, G. (2015). Silver nanoparticles synthesis mediated by new isolates of Bacillus spp., nanoparticle characterization and their activity against Bean Yellow Mosaic Virus and human pathogens. Front. Microbiol. 6, 453. doi:10.3389/fmicb.2015.00453
Elechiguerra, J. L., Burt, J. L., Morones, J. R., Camacho-Bragado, A., Gao, X., Lara, H. H., et al. (2005). Interaction of silver nanoparticles with HIV-1. J. Nanobiotechnology 3, 6–10. doi:10.1186/1477-3155-3-6
El-Megharbel, S. M., Alsawat, M., Al-Salmi, F. A., and Hamza, R. Z. (2021). Utilizing of (zinc oxide nano-spray) for disinfection against “SARS-CoV-2” and testing its biological effectiveness on some biochemical parameters during (COVID-19 pandemic)—” ZnO nanoparticles have antiviral activity against (SARS-CoV-2)”. Coatings 11 (4), 388. doi:10.3390/coatings11040388
El-Nahhal, I. M., Zourab, S. M., Kodeh, F. S., Selmane, M., Genois, I., and Babonneau, F. (2012). Nanostructured copper oxide-cotton fibers: synthesis, characterization, and applications. Int. Nano Lett. 2, 14–15. doi:10.1186/2228-5326-2-14
EPA. (2016). Protocol for the evaluation of bactericidal activity of hard, non-porous copper containing surface products. Available at: https://www.epa.gov/sites/production/files/2016-02/documents/copper_and_copper-alloy_surface_protocol_revised_012916.pdf.
Ermini, M. L., and Voliani, V. (2021). Antimicrobial nano-agents: The copper age. ACS Nano 15, 6008–6029. doi:10.1021/acsnano.0c10756
Espinosa, A. C., Mazari-Hiriart, M., Espinosa, R., Maruri-Avidal, L., Méndez, E., and Arias, C. F. (2008). Infectivity and genome persistence of rotavirus and astrovirus in groundwater and surface water. Water Res. 42, 2618–2628. doi:10.1016/j.watres.2008.01.018
Etemadzade, M., Ghamarypour, A., Zabihollahi, R., Shirazi, M., Sahebjamee, H., Vaziri, A. Z., et al. (2016). Synthesis and evaluation of antiviral activities of novel sonochemical silver nanorods against HIV and HSV viruses. Asian Pac. J. Trop. Dis. 6, 854–858. doi:10.1016/s2222-1808(16)61145-3
Fedorova, N. E., Klimova, R. R., Tulenev, Y. A., Chichev, E. V., Kornev, A. B., Troshin, P. A., et al. (2012). Carboxylic fullerene C60 derivatives: efficient microbicides against herpes simplex virus and cytomegalovirus infections in vitro. Mendeleev Commun. 5, 254–256. doi:10.1016/j.mencom.2012.09.009
Foffa, I., Losi, P., Quaranta, P., Cara, A., Al Kayal, T., D’Acunto, M., et al. (2022). A Copper nanoparticles-based polymeric spray coating: Nanoshield against Sars-Cov-2. J. Appl. Biomater. Funct. Mat. 20, 228080002210763. doi:10.1177/22808000221076326
Friedman, S. H., DeCamp, D. L., Sijbesma, R. P., Srdanov, G., Wudl, F., and Kenyon, G. L. (1993). Inhibition of the HIV-1 protease by fullerene derivatives: model building studies and experimental verification. J. Am. Chem. Soc. 115, 6506–6509. doi:10.1021/ja00068a005
Fujimori, Y., Sato, T., Hayata, T., Nagao, T., Nakayama, M., Nakayama, T., et al. (2012). Novel antiviral characteristics of nanosized copper (I) iodide particles showing inactivation activity against 2009 pandemic H1N1 influenza virus. Appl. Environ. Microbiol. 78, 951–955. doi:10.1128/aem.06284-11
Gaikwad, S., Ingle, A., Gade, A., Rai, M., Falanga, A., Incoronato, N., et al. (2013). Antiviral activity of mycosynthesized silver nanoparticles against herpes simplex virus and human parainfluenza virus type 3. Int. J. Nanomedicine 8, 4303–4314. doi:10.2147/ijn.s50070
Galante, A. J., Yates, K. A., Romanowski, E. G., Shanks, R. M., and Leu, P. W. (2022). Coal-derived functionalized nano-graphene oxide for bleach washable, durable antiviral fabric coatings. ACS Appl. Nano Mat. 5, 718–728. doi:10.1021/acsanm.1c03448
Galdiero, S., Falanga, A., Vitiello, M., Cantisani, M., Marra, V., and Galdiero, M. (2011). Silver nanoparticles as potential antiviral agents. Molecules 16, 8894–8918. doi:10.3390/molecules16108894
Gauri, A., Yadav, P., and Prajapati, P. K. (2020). Possible potential of Tamra Bhasma (calcined copper) in COVID-19 management. J. Res. Ayurvedic Sci. 4, 113–120. doi:10.5005/jras-10064-0111
Ghaffari, H., Tavakoli, A., Moradi, A., Tabarraei, A., Bokharaei-Salim, F., Zahmatkeshan, M., et al. (2019). Inhibition of H1N1 influenza virus infection by zinc oxide nanoparticles: another emerging application of nanomedicine. J. Biomed. Sci. 26, 70–10. doi:10.1186/s12929-019-0563-4
Ghosh, S., Mukherjee, R., Basak, D., and Haldar, J. (2020). One-step curable, covalently immobilized coating for clinically relevant surfaces that can kill bacteria, fungi, and influenza virus. ACS Appl. Mat. Interfaces 12, 27853–27865. doi:10.1021/acsami.9b22610
Ghosh, U., Ahammed, K. S., Mishra, S., and Bhaumik, A. (2022). The emerging roles of silver nanoparticles to target viral life-cycle and detect viral pathogens. Chem. Asian J. 17, e202101149. doi:10.1002/asia.202101149
Glowacka, I., Bertram, S., Müller, M. A., Allen, P., Soilleux, E., Pfefferle, S., et al. (2011). Evidence that TMPRSS2 activates the severe acute respiratory syndrome coronavirus spike protein for membrane fusion and reduces viral control by the humoral immune response. J. Virol. 85, 4122–4134. doi:10.1128/jvi.02232-10
Goharshadi, E. K., Goharshadi, K., and Moghayedi, M. (2022). The use of nanotechnology in the fight against viruses: A critical review. Coord. Chem. Rev. 464, 214559. doi:10.1016/j.ccr.2022.214559
Golosov, D. A. (2017). Balanced magnetic field in magnetron sputtering systems. Vacuum 139, 109–116. doi:10.1016/j.vacuum.2017.02.018
Gomathi, A., Rajarathinam, S. X., Sadiq, A. M., and Rajeshkumar, S. (2020). Anticancer activity of silver nanoparticles synthesized using aqueous fruit shell extract of Tamarindus indica on MCF-7 human breast cancer cell line. J. Drug Deliv. Sci. Technol. 55, 101376. doi:10.1016/j.jddst.2019.101376
Gonzalez, A., Aboubakr, H. A., Brockgreitens, J., Hao, W., Wang, Y., Goyal, S. M., et al. (2021). Durable nanocomposite face masks with high particulate filtration and rapid inactivation of coronaviruses. Sci. Rep. 11, 24318–24411. doi:10.1038/s41598-021-03771-1
Góral, D., and Góral-Kowalczyk, M. (2022). Application of metal nanoparticles for production of self-sterilizing coatings. Coatings 12, 480. doi:10.3390/coatings12040480
Grass, G., Rensing, C., and Solioz, M. (2011). Metallic copper as an antimicrobial surface. Appl. Environ. Microbiol. 77, 1541–1547. doi:10.1128/aem.02766-10
Gross, T. M., Lahiri, J., Golas, A., Luo, J., Verrier, F., Kurzejewski, J. L., et al. (2019). Copper-containing glass ceramic with high antimicrobial efficacy. Nat. Commun. 10, 1979. doi:10.1038/s41467-019-09946-9
Gulati, R., Sharma, S., and Sharma, R. K. (2021). Antimicrobial textile: recent developments and functional perspective. Polym. Bull. Berl. 79, 5747–5771. doi:10.1007/s00289-021-03826-3
Gupta, J., Irfan, M., Ramgir, N., Muthe, K., Debnath, A., Ansari, S., et al. (2022). Antiviral activity of zinc oxide nanoparticles and tetrapods against the hepatitis E and hepatitis C viruses. Front. Microbiol. 13, 881595. doi:10.3389/fmicb.2022.881595
Gurunathan, S., Han, J. W., Dayem, A. A., Eppakayala, V., and Kim, J.-H. (2012). Oxidative stress-mediated antibacterial activity of graphene oxide and reduced graphene oxide in Pseudomonas aeruginosa. Int. J. Nanomedicine 7, 5901–5914. doi:10.2147/ijn.s37397
Gurunathan, S., Qasim, M., Choi, Y., Do, J. T., Park, C., Hong, K., et al. (2020). Antiviral potential of nanoparticles—can nanoparticles fight against coronaviruses? Nanomaterials 10, 1645. doi:10.3390/nano10091645
Ha, T., Pham, T. T. M., Kim, M., Kim, Y.-H., Park, J.-H., Seo, J. H., et al. (2022). Antiviral activities of high energy E-beam induced copper nanoparticles against H1N1 influenza virus. Nanomaterials 12, 268. doi:10.3390/nano12020268
Haase, H., Ober-Blöbaum, J. L., Engelhardt, G., Hebel, S., Heit, A., Heine, H., et al. (2008). Zinc signals are essential for lipopolysaccharide-induced signal transduction in monocytes. J. Immunol. 181, 6491–6502. doi:10.4049/jimmunol.181.9.6491
Hajkova, P., Spatenka, P., Horsky, J., Horska, I., and Kolouch, A. (2007). Photocatalytic effect of TiO2 films on viruses and bacteria. Plasma process. Polym. 4, S397–S401. doi:10.1002/ppap.200731007
Haldar, J., An, D., Álvarez de Cienfuegos, L., Chen, J., and Klibanov, A. M. (2006). Polymeric coatings that inactivate both influenza virus and pathogenic bacteria. Proc. Natl. Acad. Sci. U. S. A. 103, 17667–17671. doi:10.1073/pnas.0608803103
Haldar, J., Weight, A. K., and Klibanov, A. M. (2007). Preparation, application and testing of permanent antibacterial and antiviral coatings. Nat. Protoc. 2, 2412–2417. doi:10.1038/nprot.2007.353
Halder, A., Das, S., Ojha, D., Chattopadhyay, D., and Mukherjee, A. (2018). Highly monodispersed gold nanoparticles synthesis and inhibition of herpes simplex virus infections. Mater. Sci. Eng. C 89, 413–421. doi:10.1016/j.msec.2018.04.005
Hamdi, M., Abdel-Bar, H. M., Elmowafy, E., El-Khouly, A., Mansour, M., and Awad, G. A. (2021). Investigating the internalization and COVID-19 antiviral computational analysis of optimized nanoscale zinc oxide. ACS omega 6, 6848–6860. doi:10.1021/acsomega.0c06046
Haraguchi, Y., Sakurai, H., Hussain, S., Anner, B. M., and Hoshino, H. (1999). Inhibition of HIV-1 infection by zinc group metal compounds. Antivir. Res. 43, 123–133. doi:10.1016/s0166-3542(99)00040-6
Hasan, J., Xu, Y., Yarlagadda, T., Schuetz, M., Spann, K., and Yarlagadda, P. K. (2020). Antiviral and antibacterial nanostructured surfaces with excellent mechanical properties for hospital applications. ACS Biomater. Sci. Eng. 6, 3608–3618. doi:10.1021/acsbiomaterials.0c00348
He, Q., Lu, J., Liu, N., Lu, W., Li, Y., Shang, C., et al. (2022). Antiviral properties of silver nanoparticles against SARS-CoV-2: Effects of surface coating and particle size. Nanomaterials 12, 990. doi:10.3390/nano12060990
Hewawaduge, C., Senevirathne, A., Jawalagatti, V., Kim, J. W., and Lee, J. H. (2021). Copper-impregnated three-layer mask efficiently inactivates SARS-CoV2. Environ. Res. 196, 110947. doi:10.1016/j.envres.2021.110947
Hodek, J., Zajícová, V., Lovětinská-Šlamborová, I., Stibor, I., Müllerová, J., and Weber, J. (2016). Protective hybrid coating containing silver, copper and zinc cations effective against human immunodeficiency virus and other enveloped viruses. BMC Microbiol. 16, 56–12. doi:10.1186/s12866-016-0675-x
Horie, M., Ogawa, H., Yoshida, Y., Yamada, K., Hara, A., Ozawa, K., et al. (2008). Inactivation and morphological changes of avian influenza virus by copper ions. Arch. Virol. 153, 1467–1472. doi:10.1007/s00705-008-0154-2
Hoyme, U. B. (1993). Clinical significance of credé's prophylaxis in Germany at present. Infect. Dis. Obstetrics Gynecol. 1, 32–36. doi:10.1155/s1064744993000080
Hsu, Y.-H., and Wu, W.-Y. (2019). Antibacterial AgCu coatings deposited using an asymmetric bipolar high-power impulse magnetron sputtering technique. Surf. Coatings Technol. 362, 302–310. doi:10.1016/j.surfcoat.2019.02.001
Hu, J., Zhao, Y., Meng, Y., Su, J., and Han, J. (2022). Long-lasting antimicrobial activity achieved through the synergy of graphene oxide and cuprous oxide coating on PET fabrics. Synth. Met. 286, 117033. doi:10.1016/j.synthmet.2022.117033
Huang, L., Gu, M., Wang, Z., Tang, T. W., Zhu, Z., Yuan, Y., et al. (2021). Highly efficient and rapid inactivation of coronavirus on non-metal hydrophobic laser-induced graphene in mild conditions. Adv. Funct. Mat. 31, 2101195. doi:10.1002/adfm.202101195
Huang, M.-L., Wu, Y.-Z., Fan, F., Lu, S.-G., Luo, B.-S., and Li, Y.-H. (2021). Antibacterial and ultraviolet protective neodymium-doped TiO2 film coated on polypropylene nonwoven fabric via a sputtering method. J. Eng. Fibers Fabr. 16, 155892502110252. doi:10.1177/15589250211025257
Hutasoit, N., Kennedy, B., Hamilton, S., Luttick, A., Rashid, R. A. R., and Palanisamy, S. (2020). Sars-CoV-2 (COVID-19) inactivation capability of copper-coated touch surface fabricated by cold-spray technology. Manuf. Lett. 25, 93–97. doi:10.1016/j.mfglet.2020.08.007
Huy, T. Q., Thanh, N. T. H., Thuy, N. T., Van Chung, P., Hung, P. N., Le, A.-T., et al. (2017). Cytotoxicity and antiviral activity of electrochemical–synthesized silver nanoparticles against poliovirus. J. Virological Methods 241, 52–57. doi:10.1016/j.jviromet.2016.12.015
Iarikov, D. D., Kargar, M., Sahari, A., Russel, L., Gause, K. T., Behkam, B., et al. (2014). Antimicrobial surfaces using covalently bound polyallylamine. Biomacromolecules 15, 169–176. doi:10.1021/bm401440h
Ikner, L. A., Torrey, J. R., Gundy, P. M., and Gerba, C. P., "A continuously active antimicrobial coating effective against human coronavirus 229E," medRxiv, 2020.
Innocenzi, P., and Stagi, L. (2020). Carbon-based antiviral nanomaterials: Graphene, C-dots, and fullerenes. A perspective. Chem. Sci. 11, 6606–6622. doi:10.1039/d0sc02658a
Ishida, T. (2019). Review on the role of Zn2+ ions in viral pathogenesis and the effect of Zn2+ ions for host cell-virus growth inhibition. Am. J. Biomed. Sci. Res. 2, 28–37. doi:10.34297/ajbsr.2019.02.000566
Iyigundogdu, Z. U., Demir, O., Asutay, A. B., and Sahin, F. (2017). Developing novel antimicrobial and antiviral textile products. Appl. Biochem. Biotechnol. 181, 1155–1166. doi:10.1007/s12010-016-2275-5
Jabbar, A. H., Al-Janabi, H. S. O., Hamzah, M., Mezan, S., Tumah, A., Ameruddin, A., et al. (2020). Green synthesis and characterization of silver nanoparticle (AgNPs) using pandanus atrocarpus extract. Int. J. Adv. Sci. Technol. 29, 4913–4922.
Jamunkar, R., Shrivas, K., Sinha, D., Patel, S., Patle, A., Kumar, A., et al. (2022). Application of silver nanoparticles as a new alternative antiviral agent for SARS-CoV-2: a review. Curr. Nanosci. 18, 465–477. doi:10.2174/1573413717666211118105415
Jana, I. D., Kumbhakar, P., Banerjee, S., Gowda, C. C., Kedia, N., Kuila, S. K., et al. (2021). Copper nanoparticle–graphene composite-based transparent surface coating with antiviral activity against influenza virus. ACS Appl. Nano Mat. 4, 352–362. doi:10.1021/acsanm.0c02713
Jeremiah, S. S., Miyakawa, K., Morita, T., Yamaoka, Y., and Ryo, A. (2020). Potent antiviral effect of silver nanoparticles on SARS-CoV-2. Biochem. biophysical Res. Commun. 533, 195–200. doi:10.1016/j.bbrc.2020.09.018
Jiang, Z.-J., Liu, C.-Y., and Sun, L.-W. (2005). Catalytic properties of silver nanoparticles supported on silica spheres. J. Phys. Chem. B 109, 1730–1735. doi:10.1021/jp046032g
Jung, S., Byeon, E.-Y., Kim, D.-G., Lee, D.-G., Ryoo, S., Lee, S., et al. (2021). Copper-coated polypropylene filter face mask with SARS-CoV-2 antiviral ability. Polymers 13, 1367. doi:10.3390/polym13091367
Jung, S., Yang, J.-Y., Jang, D., Kim, T., Baek, K. H., Yoon, H., et al. (2022). Sustainable antibacterial and antiviral high-performance copper-coated filter produced via ion beam treatment. Polymers 14, 1007. doi:10.3390/polym14051007
Kalishwaralal, K., Banumathi, E., Pandian, S. R. K., Deepak, V., Muniyandi, J., Eom, S. H., et al. (2009). Silver nanoparticles inhibit VEGF induced cell proliferation and migration in bovine retinal endothelial cells. Colloids Surfaces B Biointerfaces 73, 51–57. doi:10.1016/j.colsurfb.2009.04.025
Kampf, G., Todt, D., Pfaender, S., and Steinmann, E. (2020). Persistence of coronaviruses on inanimate surfaces and their inactivation with biocidal agents. J. Hosp. Infect. 104, 246–251. doi:10.1016/j.jhin.2020.01.022
Karst, D., and Yang, Y. (2006). Potential advantages and risks of nanotechnology for textiles. AATCC Rev. 6.
Kaushik, N., Subramani, C., Anang, S., Muthumohan, R., Shalimar, , Nayak, B., et al. (2017). Zinc salts block hepatitis E virus replication by inhibiting the activity of viral RNA-dependent RNA polymerase. J. Virol. 91, 007544. doi:10.1128/jvi.00754-17
Kelly, P. J., and Arnell, R. D. (2000). Magnetron sputtering: a review of recent developments and applications. Vacuum 56, 159–172. doi:10.1016/s0042-207x(99)00189-x
Khan, M., Shaik, M. R., Khan, S. T., Adil, S. F., Kuniyil, M., Khan, M., et al. (2020). Enhanced antimicrobial activity of biofunctionalized zirconia nanoparticles. ACS omega 5, 1987–1996. doi:10.1021/acsomega.9b03840
Kim, H. R., Sahu, B. B., Xiang, P. J., and Han, J. G. (2018). Direct synthesis of magnetron sputtered nanostructured Cu films with desired properties via plasma chemistry for their efficient antibacterial application. Plasma process. Polym. 15, 1800009. doi:10.1002/ppap.201800009
Kim, J., Yeom, M., Lee, T., Kim, H.-O., Na, W., Kang, A., et al. (2020). Porous gold nanoparticles for attenuating infectivity of influenza A virus. J. Nanobiotechnology 18, 54–11. doi:10.1186/s12951-020-00611-8
Kim, M., Kim, S. H., Rho, Y., Cho, E., Lee, J. H., and Lee, S.-J. (2021). Transparent, water-repellent, antiviral, antistatic, and flexible Cu–Plasma-Polymerized fluorocarbon nanocomposite thin films. ACS Appl. Mat. Interfaces 13, 10301–10312. doi:10.1021/acsami.0c21247
Konieczny, J., and Rdzawski, Z. (2012). Antibacterial properties of copper and its alloys. Archives Mater. Sci. Eng. 56, 53–60.
Korant, B., Kauer, J., and Butterworth, B. (1974). Zinc ions inhibit replication of rhinoviruses. Nature 248, 588–590. doi:10.1038/248588a0
Kraevaya, O. A., Bolshakova, V. S., Peregudov, A. S., Chernyak, A. V., Slesarenko, N. A., Markov, V. Y., et al. (2021). Water-promoted reaction of C60Ar5Cl compounds with thiophenes delivers a family of multifunctional fullerene derivatives with selective antiviral properties. Org. Lett. 23, 7226–7230. doi:10.1021/acs.orglett.1c02623
Kumar, R., Nayak, M., Sahoo, G. C., Pandey, K., Sarkar, M. C., Ansari, Y., et al. (2019). Iron oxide nanoparticles based antiviral activity of H1N1 influenza A virus. J. Infect. Chemother. 25, 325–329. doi:10.1016/j.jiac.2018.12.006
Kumar, S., Karmacharya, M., Joshi, S. R., Gulenko, O., Park, J., Kim, G.-H., et al. (2020). Photoactive antiviral face mask with self-sterilization and reusability. Nano Lett. 21, 337–343. doi:10.1021/acs.nanolett.0c03725
Kumar, A., Soni, V., Singh, P., Khan, A. A. P., Nazim, M., Mohapatra, S., et al. (2022). Green aspects of photocatalysts during corona pandemic: a promising role for the deactivation of COVID-19 virus. RSC Adv. 12, 13609–13627. doi:10.1039/d1ra08981a
Kümel, G., Schrader, S., Zentgraf, H., Daus, H., and Brendel, M. (1990). The mechanism of the antiherpetic activity of zinc sulphate. J. general virology 71, 2989–2997. doi:10.1099/0022-1317-71-12-2989
Kwiczak-Yiǧitbaşı, J., Demir, M., Ahan, R. E., Canlı, S., Şafak Şeker, U. O. z. r., and Baytekin, B. (2020). Ultrasonication for environmentally friendly preparation of antimicrobial and catalytically active nanocomposites of cellulosic textiles. ACS Sustain. Chem. Eng. 8, 18879–18888. doi:10.1021/acssuschemeng.0c05493
La Rosa, G., Bonadonna, L., Lucentini, L., Kenmoe, S., and Suffredini, E. (2020). Coronavirus in water environments: Occurrence, persistence and concentration methods-A scoping review. Water Res. 179, 115899. doi:10.1016/j.watres.2020.115899
Lara, H. H., Ayala-Nuñez, N. V., Ixtepan-Turrent, L., and Rodriguez-Padilla, C. (2010). Mode of antiviral action of silver nanoparticles against HIV-1. J. nanobiotechnology 8, 1–10. doi:10.1186/1477-3155-8-1
Lara, H. H., Ixtepan-Turrent, L., Garza-Treviño, E. N., and Rodriguez-Padilla, C. (2010). PVP-coated silver nanoparticles block the transmission of cell-free and cell-associated HIV-1 in human cervical culture. J. nanobiotechnology 8, 15–11. doi:10.1186/1477-3155-8-15
Lara, H. H., Garza-Treviño, E. N., Ixtepan-Turrent, L., and Singh, D. K. (2011). Silver nanoparticles are broad-spectrum bactericidal and virucidal compounds. J. Nanobiotechnology 9, 30–38. doi:10.1186/1477-3155-9-30
Lekutis, C., Olshevsky, U., Furman, C., Thali, M., and Sodroski, J. (1992). Contribution of disulfide bonds in the carboxyl terminus of the human immunodeficiency virus type I gp120 glycoprotein to CD4 binding. J. Acquir. Immune Defic. Syndr. 5, 78–81.
Li, Y., Lin, Z., Xu, T., Wang, C., Zhao, M., Xiao, M., et al. (2017). Delivery of VP1 siRNA to inhibit the EV71 virus using functionalized silver nanoparticles through ROS-mediated signaling pathways. RSC Adv. 7, 1453–1463. doi:10.1039/c6ra26472g
Li, S., Huang, J., Chen, Z., Chen, G., and Lai, Y. (2017). A review on special wettability textiles: theoretical models, fabrication technologies and multifunctional applications. J. Mat. Chem. A Mat. 5, 31–55. doi:10.1039/c6ta07984a
Li, P., Li, J., Feng, X., Li, J., Hao, Y., Zhang, J., et al. (2019). Metal-organic frameworks with photocatalytic bactericidal activity for integrated air cleaning. Nat. Commun. 10, 2177–2210. doi:10.1038/s41467-019-10218-9
Liu, C. Y., and Kielian, M. (2012). Identification of a specific region in the e1 fusion protein involved in zinc inhibition of semliki forest virus fusion. J. Virol. 86, 3588–3594. doi:10.1128/jvi.07115-11
Liu, H., Kim, Y., Mello, K., Lovaasen, J., Shah, A., Rice, N., et al. (2014). Aerosol-assisted plasma deposition of hydrophobic polycations makes surfaces highly antimicrobial. Appl. Biochem. Biotechnol. 172, 1254–1264. doi:10.1007/s12010-013-0593-4
Liu, Y., Leng, J., Wu, Q., Zhang, S., and Teng, X. (2017). Investigation on the properties of nano copper matrix composite via vacuum arc melting method. Mat. Res. Express 4, 106512. doi:10.1088/2053-1591/aa9096
Loo, Y. Y., Rukayadi, Y., Nor-Khaizura, M.-A.-R., Kuan, C. H., Chieng, B. W., Nishibuchi, M., et al. (2018). In vitro antimicrobial activity of green synthesized silver nanoparticles against selected gram-negative foodborne pathogens. Front. Microbiol. 9, 1555. doi:10.3389/fmicb.2018.01555
Lu, L., Sun, R. W.-Y., Chen, R., Hui, C.-K., Ho, C.-M., Luk, J. M., et al. (2008). Silver nanoparticles inhibit Hepatitis B virus replication. Antivir. Ther. 13, 253–262. doi:10.1177/135965350801300210
Lu, K.-Q., Li, Y.-H., Zhang, F., Qi, M.-Y., Chen, X., Tang, Z.-R., et al. (2020). Rationally designed transition metal hydroxide nanosheet arrays on graphene for artificial CO2 reduction. Nat. Commun. 11, 5181–5189. doi:10.1038/s41467-020-18944-1
Ma, C., Nikiforov, A., De Geyter, N., Dai, X., Morent, R., and Ostrikov, K. K. (2021). Future antiviral polymers by plasma processing. Prog. Polym. Sci. 118, 101410. doi:10.1016/j.progpolymsci.2021.101410
Maeda-Mamiya, R., Noiri, E., Isobe, H., Nakanishi, W., Okamoto, K., Doi, K., et al. (2010). In vivo gene delivery by cationic tetraamino fullerene. Proc. Natl. Acad. Sci. U. S. A. 107, 5339–5344. doi:10.1073/pnas.0909223107
Mahdi, K. N., Peters, R., van der Ploeg, M., Ritsema, C., and Geissen, V. (2018). Tracking the transport of silver nanoparticles in soil: a saturated column experiment. Water Air Soil Pollut. 229, 334–413. doi:10.1007/s11270-018-3985-9
Mallakpour, S., Azadi, E., and Hussain, C. M. (2021). Recent breakthroughs of antibacterial and antiviral protective polymeric materials during COVID-19 pandemic and after pandemic: Coating, packaging, and textile applications. Curr. Opin. Colloid & Interface Sci. 55, 101480. doi:10.1016/j.cocis.2021.101480
Manakhov, A. M., Sitnikova, N. A., Tsygankova, A. R., Alekseev, A. Y., Adamenko, L. S., Permyakova, E., et al. (2021). Electrospun biodegradable nanofibers coated homogenously by Cu magnetron sputtering exhibit fast ion release. Computational and experimental study. Membr. (Basel). 11, 965. doi:10.3390/membranes11120965
Markowska-Szczupak, A., Paszkiewicz, O., Michalkiewicz, B., Kamińska, A., and Wróbel, R. J. (2021). Fabrication of antibacterial metal surfaces using magnetron-sputtering method. Materials 14, 7301. doi:10.3390/ma14237301
Mashino, T., Shimotohno, K., Ikegami, N., Nishikawa, D., Okuda, K., Takahashi, K., et al. (2005). Human immunodeficiency virus-reverse transcriptase inhibition and hepatitis C virus RNA-dependent RNA polymerase inhibition activities of fullerene derivatives. Bioorg. Med. Chem. Lett. 15, 1107–1109. doi:10.1016/j.bmcl.2004.12.030
Matsumoto, T., Sunada, K., Nagai, T., Isobe, T., Matsushita, S., Ishiguro, H., et al. (2019). Preparation of hydrophobic La2Mo2O9 ceramics with antibacterial and antiviral properties. J. Hazard. Mater. 378, 120610. doi:10.1016/j.jhazmat.2019.05.003
Meister, T. L., Fortmann, J., Breisch, M., Sengstock, C., Steinmann, E., Köller, M., et al. (2022). Nanoscale copper and silver thin film systems display differences in antiviral and antibacterial properties. Sci. Rep. 12, 7193–7210. doi:10.1038/s41598-022-11212-w
Melk, M. M., El-Hawary, S. S., Melek, F. R., Saleh, D. O., Ali, O. M., El Raey, M. A., et al. (2021). Antiviral activity of zinc oxide nanoparticles mediated by Plumbago indica L. Extract against herpes simplex virus type 1 (HSV-1). Int. J. Nanomedicine 16, 8221–8233. doi:10.2147/ijn.s339404
Micochova, P., Chadha, A., Hesseloj, T., Fraternali, F., Ramsden, J. J., and Gupta, R. K. (2021). Rapid inactivation of SARS-CoV-2 by titanium dioxide surface coating. Wellcome Open Res. 6, 56. doi:10.12688/wellcomeopenres.16577.2
Minami, K., Okamoto, K., Harano, K., Noiri, E., and Nakamura, E. (2014). siRNA delivery targeting to the lung via agglutination-induced accumulation and clearance of cationic tetraamino fullerene. Sci. Rep. 4, 4916–4917. doi:10.1038/srep04916
Minoshima, M., Lu, Y., Kimura, T., Nakano, R., Ishiguro, H., Kubota, Y., et al. (2016). Comparison of the antiviral effect of solid-state copper and silver compounds. J. Hazard. Mater. 312, 1–7. doi:10.1016/j.jhazmat.2016.03.023
Mittireddi, R. T., Patel, N. M., Gautam, A. R. S., Soppina, V., and Panda, E. (2021). Non-stoichiometric amorphous TiOx as a highly reactive, transparent anti-viral surface coating. J. Alloys Compd. 881, 160610. doi:10.1016/j.jallcom.2021.160610
Miyauchi, M., Sunada, K., and Hashimoto, K. (2020). Antiviral effect of visible light-sensitive CuxO/TiO2 photocatalyst. Catalysts 10, 1093. doi:10.3390/catal10091093
Mizielińska, M., Nawrotek, P., Stachurska, X., Ordon, M., and Bartkowiak, A. (2021). Packaging covered with antiviral and antibacterial coatings based on ZnO nanoparticles supplemented with geraniol and carvacrol. Int. J. Mol. Sci. 22, 1717. doi:10.3390/ijms22041717
Mohammed, H., Kumar, A., Bekyarova, E., Al-Hadeethi, Y., Zhang, X., Chen, M., et al. (2020). Antimicrobial mechanisms and effectiveness of graphene and graphene-functionalized biomaterials. A scope review. Front. Bioeng. Biotechnol. 8, 465. doi:10.3389/fbioe.2020.00465
Moongraksathum, B., Chien, M.-Y., and Chen, Y.-W. (2019). Antiviral and antibacterial effects of silver-doped TiO2 prepared by the peroxo sol-gel method. J. Nanosci. Nanotechnol. 19, 7356–7362. doi:10.1166/jnn.2019.16615
Morais, D. S., Guedes, R. M., and Lopes, M. A. (2016). Antimicrobial approaches for textiles: from research to market. Materials 9, 498. doi:10.3390/ma9060498
Motakef Kazemi, N., and Sandalnia, M. (2020). In situ production and deposition of bismuth oxide nanoparticles on cotton fabric. Iran. J. Sci. Technol. Trans. Sci. 44, 1217–1223. doi:10.1007/s40995-020-00916-1
Mukherjee, K., Rivera, J. J., and Klibanov, A. M. (2008). Practical aspects of hydrophobic polycationic bactericidal “paints”. Appl. Biochem. Biotechnol. 151, 61–70. doi:10.1007/s12010-008-8151-1
Muñoz, A., Sigwalt, D., Illescas, B. M., Luczkowiak, J., Rodriguez-Perez, L., Nierengarten, I., et al. (2016). Synthesis of giant globular multivalent glycofullerenes as potent inhibitors in a model of Ebola virus infection. Nat. Chem. 8, 50–57. doi:10.1038/nchem.2387
Nacsa, J., Segesdi, J., Gyiiris, Á., Braun, T., Rausch, H., Buvári-Barcza, Á., et al. (1997). Antiretroviral effects of nonderivatizcd C6o in vitro. Fullerene Sci. Technol. 5, 969–976. doi:10.1080/15363839708013310
Nagarajan, S., Kalaivani, G., Poongothai, E., Arul, M., and Natarajan, H. (2019). Characterization of silver nanoparticles synthesized from Catharanthus roseus (Vinca rosea) plant leaf extract and their antibacterial activity. IJRAR 6, 680–685.
Nasrollahzadeh, M., Sajjadi, M., Soufi, G. J., Iravani, S., and Varma, R. S. (2020). Nanomaterials and nanotechnology-associated innovations against viral infections with a focus on coronaviruses. Nanomaterials 10, 1072. doi:10.3390/nano10061072
Nienhaus, K., Wang, H., and Nienhaus, G. (2020). Nanoparticles for biomedical applications: exploring and exploiting molecular interactions at the nano-bio interface. Mater. Today Adv. 5, 100036. doi:10.1016/j.mtadv.2019.100036
Nitta, H., Minami, K., Harano, K., and Nakamura, E. (2015). DNA binding of pentaamino [60] fullerene synthesized using click chemistry. Chem. Lett. 44, 378–380. doi:10.1246/cl.141092
Ochoa-Meza, A. R., Álvarez-Sánchez, A. R., Romo-Quiñonez, C. R., Barraza, A., Magallón-Barajas, F. J., Chávez-Sánchez, A., et al. (2019). Silver nanoparticles enhance survival of white spot syndrome virus infected Penaeus vannamei shrimps by activation of its immunological system. Fish. Shellfish Immunol. 84, 1083–1089. doi:10.1016/j.fsi.2018.10.007
Orlowski, P., Tomaszewska, E., Gniadek, M., Baska, P., Nowakowska, J., Sokolowska, J., et al. (2014). Tannic acid modified silver nanoparticles show antiviral activity in herpes simplex virus type 2 infection. PloS one 9, e104113. doi:10.1371/journal.pone.0104113
Pandiarajan, J., and Krishnan, M. (2017). Properties, synthesis and toxicity of silver nanoparticles. Environ. Chem. Lett. 15, 387–397. doi:10.1007/s10311-017-0624-4
Papp, I., Sieben, C., Ludwig, K., Roskamp, M., Böttcher, C., Schlecht, S., et al. (2010). Inhibition of influenza virus infection by multivalent sialic-acid-functionalized gold nanoparticles. Small 6, 2900–2906. doi:10.1002/smll.201001349
Park, G. W., Cho, M., Cates, E. L., Lee, D., Oh, B.-T., Vinjé, J., et al. (2014). Fluorinated TiO2 as an ambient light-activated virucidal surface coating material for the control of human norovirus. J. Photochem. Photobiol. B Biol. 140, 315–320. doi:10.1016/j.jphotobiol.2014.08.009
Park, S., Ko, Y.-S., Jung, H., Lee, C., Woo, K., and Ko, G. (2018). Disinfection of waterborne viruses using silver nanoparticle-decorated silica hybrid composites in water environments. Sci. Total Environ. 625, 477–485. doi:10.1016/j.scitotenv.2017.12.318
Parvez, M. K., Ahmed, S., and Niyazi, S. (2022). Herb-synthesized antiviral NanoparticlesHerb-synthesized antiviral nanoparticles. Adv. Biol. Res. 3, 8–10. doi:10.26855/abr.2022.03.001
Patil, A. H., Jadhav, S. A., Gurav, K. D., Waghmare, S. R., Patil, G. D., Jadhav, V. D., et al. (2020). Single step green process for the preparation of antimicrobial nanotextiles by wet chemical and sonochemical methods. J. Text. Inst. 111, 1380–1388. doi:10.1080/00405000.2019.1697160
Patoo, T. S., Khanday, F., and Qurashi, A. (2022). Prospectus of advanced nanomaterials for antiviral properties. Mat. Adv. 3, 2960–2970. doi:10.1039/d1ma00541c
Perelshtein, I., Lipovsky, A., Perkas, N., Tzanov, T., Аrguirova, M., Leseva, M., et al. (2015). Making the hospital a safer place by sonochemical coating of all its textiles with antibacterial nanoparticles. Ultrason. sonochemistry 25, 82–88. doi:10.1016/j.ultsonch.2014.12.012
Perkas, N., Perelshtein, I., and Gedanken, A. (2018). Coating textiles with antibacterial nanoparticles using the sonochemical techniqe. J. Mach. Constr. Maintenance-Problemy Eksploatacji.
Petkova, P., Francesko, A., Fernandes, M. M., Mendoza, E., Perelshtein, I., Gedanken, A., et al. (2014). Sonochemical coating of textiles with hybrid ZnO/chitosan antimicrobial nanoparticles. ACS Appl. Mat. Interfaces 6, 1164–1172. doi:10.1021/am404852d
Prakash, J., Cho, J., and Mishra, Y. K. (2021). Photocatalytic TiO2 nanomaterials as potential antimicrobial and antiviral agents: Scope against blocking the SARS-COV-2 spread. Micro Nano Eng. 14, 100100. doi:10.1016/j.mne.2021.100100
Purniawan, A., Lusida, M. I., Pujiyanto, R. W., Nastri, A. M., Permanasari, A. A., Harsono, A. A. H., et al. (2022). Synthesis and assessment of copper-based nanoparticles as a surface coating agent for antiviral properties against SARS-CoV-2. Sci. Rep. 12, 4835–4838. doi:10.1038/s41598-022-08766-0
Quan, Q., Lin, X., Zhang, N., and Xu, Y.-J. (2017). Graphene and its derivatives as versatile templates for materials synthesis and functional applications. Nanoscale 9, 2398–2416. doi:10.1039/c6nr09439b
Rahman, M. A., Saha, J., and Mondal, M. I. H. (2021). “Silver nano materials coated textiles for antiviral protection,” in 2021 International Conference on Computer, Communication, Chemical, Materials and Electronic Engineering (IC4ME2), 26-27 Dec. 2021, 1–4.
Rani, I., Goyal, A., Bhatnagar, M., Manhas, S., Goel, P., Pal, A., et al. (2021). Potential molecular mechanisms of zinc-and copper-mediated antiviral activity on COVID-19. Nutr. Res. 92, 109–128. doi:10.1016/j.nutres.2021.05.008
Rasool, K., and Lee, D. S. (2016). Inhibitory effects of silver nanoparticles on removal of organic pollutants and sulfate in an anaerobic biological wastewater treatment process. J. Nanosci. Nanotechnol. 16, 4456–4463. doi:10.1166/jnn.2016.10984
Refaee, A. A., El-Naggar, M. E., Mostafa, T. B., Elshaarawy, R. F., and Nasr, A. M. (2022). Nano-bio finishing of cotton fabric with quaternized chitosan Schiff base-TiO2-ZnO nanocomposites for antimicrobial and UV protection applications. Eur. Polym. J. 166, 111040. doi:10.1016/j.eurpolymj.2022.111040
Reid, M., Whatley, V., Spooner, E., Nevill, A. M., Cooper, M., Ramsden, J. J., et al. (2018). How does a photocatalytic antimicrobial coating affect environmental bioburden in hospitals? Infect. Control Hosp. Epidemiol. 39, 398–404. doi:10.1017/ice.2017.297
Rogers, J. V., Parkinson, C. V., Choi, Y. W., Speshock, J. L., and Hussain, S. M. (2008). A preliminary assessment of silver nanoparticle inhibition of monkeypox virus plaque formation. Nanoscale Res. Lett. 3, 129–133. doi:10.1007/s11671-008-9128-2
Roy, T. S., Shamim, S. U. D., Rahman, M. K., Ahmed, F., and Gafur, M. (2020). The development of ZnO nanoparticle coated cotton fabrics for antifungal and antibacterial applications. Mater. Sci. Appl. 11, 601–610. doi:10.4236/msa.2020.119040
Rtimi, S., Dionysiou, D. D., Pillai, S. C., and Kiwi, J. (2019). Advances in catalytic/photocatalytic bacterial inactivation by nano Ag and Cu coated surfaces and medical devices. Appl. Catal. B Environ. 240, 291–318. doi:10.1016/j.apcatb.2018.07.025
Sametband, M., Kalt, I., Gedanken, A., and Sarid, R. (2014). Herpes simplex virus type-1 attachment inhibition by functionalized graphene oxide. ACS Appl. Mater. Interfaces 6 (2), 1228–1235.
Sasaki, K., Tenjimbayashi, M., Manabe, K., and Shiratori, S. (2016). Asymmetric superhydrophobic/superhydrophilic cotton fabrics designed by spraying polymer and nanoparticles. ACS Appl. Mat. Interfaces 8, 651–659. doi:10.1021/acsami.5b09782
Seino, S., Imoto, Y., Kosaka, T., Nishida, T., Nakagawa, T., and Yamamoto, T. A. (2016). Antiviral activity of silver nanoparticles immobilized onto textile fabrics synthesized by radiochemical process. MRS Adv. 1, 705–710. doi:10.1557/adv.2016.43
Serrano-Aroca, Á. (2022). Antiviral characterization of advanced materials: Use of bacteriophage phi 6 as surrogate of enveloped viruses such as SARS-CoV-2. Int. J. Mol. Sci. 23, 5335. doi:10.3390/ijms23105335
Shady, N. H., Khattab, A. R., Ahmed, S., Liu, M., Quinn, R. J., Fouad, M. A., et al. (2020). Hepatitis C virus NS3 protease and helicase inhibitors from red sea sponge (amphimedon) species in green synthesized silver nanoparticles assisted by in silico modeling and metabolic profiling. Int. J. Nanomedicine 15, 3377–3389. doi:10.2147/ijn.s233766
Sharma, V., Kaushik, S., Pandit, P., Dhull, D., Yadav, J. P., and Kaushik, S. (2019). Green synthesis of silver nanoparticles from medicinal plants and evaluation of their antiviral potential against chikungunya virus. Appl. Microbiol. Biotechnol. 103, 881–891. doi:10.1007/s00253-018-9488-1
Shereen, M. A., Khan, S., Kazmi, A., Bashir, N., and Siddique, R. (2020). COVID-19 infection: Emergence, transmission, and characteristics of human coronaviruses. J. Adv. Res. 24, 91–98. doi:10.1016/j.jare.2020.03.005
Shi, W., Salerno, F., Ward, M. D., Santana-Bonilla, A., Wade, J., Hou, X., et al. (2021). Fullerene desymmetrization as a means to achieve single-enantiomer electron acceptors with maximized chiroptical responsiveness. Adv. Mat. 33, 2004115. doi:10.1002/adma.202004115
Shionoiri, N., Sato, T., Fujimori, Y., Nakayama, T., Nemoto, M., Matsunaga, T., et al. (2012). Investigation of the antiviral properties of copper iodide nanoparticles against feline calicivirus. J. Biosci. Bioeng. 113, 580–586. doi:10.1016/j.jbiosc.2011.12.006
Shishkov, S., Varadinova, T., Bontchev, P., Nachev, C., and Michailova, E. (1996). Complexes of zinc with picolinic and aspartic acids inactivate free varicella-zoster virions. Metal-Based Drugs 3, 11–14. doi:10.1155/mbd.1996.11
Shrivas, K., Nirmalkar, N., Deb, M. K., Dewangan, K., Nirmalkar, J., and Kumar, S. (2019). Application of functionalized silver nanoparticles as a biochemical sensor for selective detection of lysozyme protein in milk sample. Spectrochimica Acta Part A Mol. Biomol. Spectrosc. 213, 127–133. doi:10.1016/j.saa.2019.01.039
Siddiquie, R. Y., Agrawal, A., and Joshi, S. S. (2020). Surface alterations to impart antiviral properties to combat COVID-19 transmission. Trans. Indian Natl. Acad. Eng. 5, 343–347. doi:10.1007/s41403-020-00096-9
Sijbesma, R., Srdanov, G., Wudl, F., Castoro, J., Wilkins, C., Friedman, S. H., et al. (1993). Synthesis of a fullerene derivative for the inhibition of HIV enzymes. J. Am. Chem. Soc. 115, 6510–6512. doi:10.1021/ja00068a006
Sinclair, T. R., van den Hengel, S. K., Raza, B. G., Rutjes, S. A., de Roda Husman, A. M., Peijnenburg, W. J., et al. (2021). Surface chemistry-dependent antiviral activity of silver nanoparticles. Nanotechnology 32, 365101. doi:10.1088/1361-6528/ac03d6
Singh, P., Ahn, S., Kang, J.-P., Veronika, S., Huo, Y., Singh, H., et al. (2018). In vitro anti-inflammatory activity of spherical silver nanoparticles and monodisperse hexagonal gold nanoparticles by fruit extract of prunus serrulata: a green synthetic approach. Artif. Cells Nanomed. Biotechnol. 46, 2022–2032. doi:10.1080/21691401.2017.1408117
Sirichantra, J. (2022). “Antimicrobial/antiviral nano-TiO2 coatings for medical applications,” in Antimicrobial and antiviral materials (United states: CRC Press), 151–214.
Sogorkova, J., Zapotocky, V., Cepa, M., Stepankova, V., Vagnerova, H., Batova, J., et al. (2018). Optimization of cell growth on palmitoyl-hyaluronan knitted scaffolds developed for tissue engineering applications. J. Biomed. Mat. Res. A 106, 1488–1499. doi:10.1002/jbm.a.36353
Sondi, I., and Salopek-Sondi, B. (2004). Silver nanoparticles as antimicrobial agent: a case study on E. coli as a model for gram-negative bacteria. J. colloid interface Sci. 275, 177–182. doi:10.1016/j.jcis.2004.02.012
Speshock, J. L., Murdock, R. C., Braydich-Stolle, L. K., Schrand, A. M., and Hussain, S. M. (2010). Interaction of silver nanoparticles with Tacaribe virus. J. Nanobiotechnology 8, 19–9. doi:10.1186/1477-3155-8-19
Sportelli, M. C., Izzi, M., Loconsole, D., Sallustio, A., Picca, R. A., Felici, R., et al. (2022). On the efficacy of ZnO nanostructures against SARS-CoV-2. Int. J. Mol. Sci. 23, 3040. doi:10.3390/ijms23063040
Steele, A., Bayer, I., and Loth, E. (2009). Inherently superoleophobic nanocomposite coatings by spray atomization. Nano Lett. 9, 501–505. doi:10.1021/nl8037272
Steinhauer, K., Meyer, S., Pfannebecker, J., Teckemeyer, K., Ockenfeld, K., Weber, K., et al. (2018). Antimicrobial efficacy and compatibility of solid copper alloys with chemical disinfectants. PLoS One 13, e0200748. doi:10.1371/journal.pone.0200748
Suara, R. O., and Crowe, J. E. (2004). Effect of zinc salts on respiratory syncytial virus replication. Antimicrob. Agents Chemother. 48, 783–790. doi:10.1128/aac.48.3.783-790.2004
Sucipto, T. H., Churrotin, S., Setyawati, H., Kotaki, T., Martak, F., and Soegijanto, S. (2017). Antiviral activity of copper (II) chloride dihydrate against dengue virus type-2 in vero cell. Indonesian J. Trop. Infect. Dis. 6, 84–87. doi:10.20473/ijtid.v6i4.3806
Sujitha, V., Murugan, K., Paulpandi, M., Panneerselvam, C., Suresh, U., Roni, M., et al. (2015). Green-synthesized silver nanoparticles as a novel control tool against dengue virus (DEN-2) and its primary vector Aedes aegypti. Parasitol. Res. 114, 3315–3325. doi:10.1007/s00436-015-4556-2
Sun, R. W.-Y., Chen, R., Chung, N. P.-Y., Ho, C.-M., Lin, C.-L. S., and Che, C.-M. (2005). Silver nanoparticles fabricated in Hepes buffer exhibit cytoprotective activities toward HIV-1 infected cells. Chem. Commun., 5059–5061. doi:10.1039/b510984a
Sun, L., Singh, A. K., Vig, K., Pillai, S. R., and Singh, S. R. (2008). Silver nanoparticles inhibit replication of respiratory syncytial virus. J. Biomed. Nanotechnol. 4, 149–158. doi:10.1166/jbn.2008.012
Sunada, K., Minoshima, M., and Hashimoto, K. (2012). Highly efficient antiviral and antibacterial activities of solid-state cuprous compounds. J. Hazard. Mater. 235-236, 265–270. doi:10.1016/j.jhazmat.2012.07.052
Sundararaj Stanleyraj, J., Sethuraman, N., Gupta, R., Thiruvoth, S., Gupta, M., and Ryo, A. (2021). Treating COVID-19: are we missing out the window of opportunity? J. Antimicrob. Chemother. 76 (2), 283–285.
Tang, X., and Yan, X. (2017). Dip-coating for fibrous materials: mechanism, methods and applications. J. Solgel. Sci. Technol. 81, 378–404. doi:10.1007/s10971-016-4197-7
Te Velthuis, A. J., van den Worm, S. H., Sims, A. C., Baric, R. S., Snijder, E. J., and van Hemert, M. J. (2010). Zn2+ inhibits coronavirus and arterivirus RNA polymerase activity in vitro and zinc ionophores block the replication of these viruses in cell culture. PLoS Pathog. 6, e1001176. doi:10.1371/journal.ppat.1001176
Tollas, S., Bereczki, I., Borbás, A., Batta, G., Vanderlinden, E., Naesens, L., et al. (2014). Synthesis of a cluster-forming sialylthio-D-galactose fullerene conjugate and evaluation of its interaction with influenza virus hemagglutinin and neuraminidase. Bioorg. Med. Chem. Lett. 24, 2420–2423. doi:10.1016/j.bmcl.2014.04.032
Trefry, J. C., and Wooley, D. P. (2013). Silver nanoparticles inhibit vaccinia virus infection by preventing viral entry through a macropinocytosis-dependent mechanism. J. Biomed. Nanotechnol. 9, 1624–1635. doi:10.1166/jbn.2013.1659
Troshina, O. A., Troshin, P. A., Peregudov, A. S., Kozlovskiy, V. I., Balzarini, J., and Lyubovskaya, R. N. (2007). Chlorofullerene C 60 Cl 6: a precursor for straightforward preparation of highly water-soluble polycarboxylic fullerene derivatives active against HIV. Org. Biomol. Chem. 5, 2783–2791. doi:10.1039/b705331b
Tuñón-Molina, A., Takayama, K., Redwan, E. M., Uversky, V. N., Andrés, J., and Serrano-Aroca, Á. (2021). Protective face masks: current status and future trends. ACS Appl. Mat. Interfaces 13, 56725–56751. doi:10.1021/acsami.1c12227
Unal, M. A., Bayrakdar, F., Nazir, H., Besbinar, O., Gurcan, C., Lozano, N., et al. (2021). Graphene oxide nanosheets interact and interfere with SARS-CoV-2 surface proteins and cell receptors to inhibit infectivity. Small 17, 2101483. doi:10.1002/smll.202101483
Van Doremalen, N., Bushmaker, T., Morris, D. H., Holbrook, M. G., Gamble, A., Williamson, B. N., et al. (2020). Aerosol and surface stability of SARS-CoV-2 as compared with SARS-CoV-1. N. Engl. J. Med. Overseas. Ed. 382 (16), 1564–1567. doi:10.1056/nejmc2004973
Varni, J. W., Limbers, C. A., and Burwinkle, T. M. (2007). How young can children reliably and validly self-report their health-related quality of life?: An analysis of 8, 591 children across age subgroups with the PedsQL™ 4.0 Generic Core Scales. Health Qual. Life Outcomes 5, 1–13. doi:10.1186/1477-7525-5-1
Vasickova, P., Pavlik, I., Verani, M., and Carducci, A. (2010). Issues concerning survival of viruses on surfaces. Food Environ. Virol. 2, 24–34. doi:10.1007/s12560-010-9025-6
Von Borowski, R. G., and Trentin, D. S. (2021). Biofilms and coronavirus reservoirs: A perspective review. Appl. Environ. Microbiol. 87, e0085921–21. doi:10.1128/aem.00859-21
Voorhees, K. J. (2011). Methods and compositions for in situ detection of microorganisms on a surface. PCT/US2006/011958.
Walji, S.-D., and Aucoin, M. G. (2020). A critical evaluation of current protocols for self-sterilizing surfaces designed to reduce viral nosocomial infections. Am. J. Infect. Control 48, 1255–1260. doi:10.1016/j.ajic.2020.02.008
Wang, J., Wei, Y., Shi, X., and Gao, H. (2013). Cellular entry of graphene nanosheets: the role of thickness, oxidation and surface adsorption. RSC Adv. 3, 15776–15782. doi:10.1039/c3ra40392k
Wang, H., Yan, L., Gao, D., Liu, D., Wang, C., Sun, L., et al. (2014). Tribological properties of superamphiphobic PPS/PTFE composite coating in the oilfield produced water. Wear 319, 62–68. doi:10.1016/j.wear.2014.07.012
Wiener, J., Shahidi, S., and Goba, M. (2013). Laser deposition of TiO2 nanoparticles on glass fabric. Opt. Laser Technol. 45, 147–153. doi:10.1016/j.optlastec.2012.07.012
Wong, S. Y., Li, Q., Veselinovic, J., Kim, B.-S., Klibanov, A. M., and Hammond, P. T. (2010). Bactericidal and virucidal ultrathin films assembled layer by layer from polycationic N-alkylated polyethylenimines and polyanions. Biomaterials 31, 4079–4087. doi:10.1016/j.biomaterials.2010.01.119
Woodall, C., "16 - testing strategies and international standards for disinfectants," in Decontamination in hospitals and healthcare (Second Edition), J. Walker, Ed., ed: Sawston: Woodhead Publishing, 2020, pp. 371–376.
Worananthakij, W., Manklinniam, P., Limsuwan, P., Sakulkalavek, A., and Sakdanuphab, R. (2022). Highly transparent flexible CuI film for antibacterial applications. Mater. Chem. Phys. 277, 125516. doi:10.1016/j.matchemphys.2021.125516
Wu, X., Wyman, I., Zhang, G., Lin, J., Liu, Z., Wang, Y., et al. (2016). Preparation of superamphiphobic polymer-based coatings via spray-and dip-coating strategies. Prog. Org. Coatings 90, 463–471. doi:10.1016/j.porgcoat.2015.08.008
Xiang, D., Zheng, Y., Duan, W., Li, X., Yin, J., Shigdar, S., et al. (2013). Inhibition of A/Human/Hubei/3/2005 (H3N2) influenza virus infection by silver nanoparticles in vitro and in vivo. Int. J. Nanomedicine 8, 4103–4113. doi:10.2147/IJN.S53622
Xu, Q., Wang, P., Zhang, Y., and Li, C. (2021). Durable antibacterial and UV protective properties of cotton fabric coated with carboxymethyl chitosan and Ag/TiO2 composite nanoparticles. Fibers Polym. 23, 386–395. doi:10.1007/s12221-021-0352-z
Xu, P.-Y., Li, X.-Q., Chen, W.-G., Deng, L.-L., Tan, Y.-Z., Zhang, Q., et al. (2022). Progress in antiviral fullerene research. Nanomaterials 12, 2547. doi:10.3390/nano12152547
Xue, L., Cai, W., Gao, J., Zhang, L., Dong, R., Li, Y., et al. (2019). The resurgence of the norovirus GII. 4 variant associated with sporadic gastroenteritis in the post-GII. 17 period in South China, 2015 to 2017. BMC Infect. Dis. 19, 696–698. doi:10.1186/s12879-019-4331-6
Yang, M.-Q., Zhang, N., Pagliaro, M., and Xu, Y.-J. (2014). Artificial photosynthesis over graphene–semiconductor composites. Chem. Soc. Rev. 43, 8240–8254. doi:10.1039/c4cs00213j
Yao, N., and Lun Yeung, K. (2011). Investigation of the performance of TiO2 photocatalytic coatings. Chem. Eng. J. 167, 13–21. doi:10.1016/j.cej.2010.11.061
Ye, Z., Li, S., Zhao, S., Deng, L., Zhang, J., and Dong, A. (2021). Textile coatings configured by double-nanoparticles to optimally couple superhydrophobic and antibacterial properties. Chem. Eng. J. 420, 127680. doi:10.1016/j.cej.2020.127680
Yotprayoonsak, P., Anusak, N., Virtanen, J., Kangas, V., and Promarak, V. (2022). Facile fabrication of flexible and conductive AuNP/DWCNT fabric with enhanced joule heating efficiency via spray coating route. Microelectron. Eng. 255, 111718. doi:10.1016/j.mee.2022.111718
Yuan, Y.-G., Zhang, S., Hwang, J.-Y., and Kong, I.-K. (2018). Silver nanoparticles potentiates cytotoxicity and apoptotic potential of camptothecin in human cervical cancer cells. Oxidative Med. Cell. Longev. 2018, 1–21. doi:10.1155/2018/6121328
Zhang, N., Yang, M.-Q., Liu, S., Sun, Y., and Xu, Y.-J. (2015). Waltzing with the versatile platform of graphene to synthesize composite photocatalysts. Chem. Rev. 115, 10307–10377. doi:10.1021/acs.chemrev.5b00267
Zhang, Y., Xu, Q., Fu, F., and Liu, X. (2016). Durable antimicrobial cotton textiles modified with inorganic nanoparticles. Cellulose 23, 2791–2808. doi:10.1007/s10570-016-1012-0
Zhang, F., Li, Y.-H., Li, J.-Y., Tang, Z.-R., and Xu, Y.-J. (2019). 3D graphene-based gel photocatalysts for environmental pollutants degradation. Environ. Pollut. 253, 365–376. doi:10.1016/j.envpol.2019.06.089
Zhang, Y., Fan, W., Sun, Y., Chen, W., and Zhang, Y. (2021). Application of antiviral materials in textiles: areview. Nanotechnol. Rev. 10, 1092–1115. doi:10.1515/ntrev-2021-0072
Zhang, S., Dong, H., He, R., Wang, N., Zhao, Q., Yang, L., et al. (2022). Hydro electroactive Cu/Zn coated cotton fiber nonwovens for antibacterial and antiviral applications. Int. J. Biol. Macromol. 207, 100–109. doi:10.1016/j.ijbiomac.2022.02.155
Zhong, H., Zhu, Z., Lin, J., Cheung, C. F., Lu, V. L., Yan, F., et al. (2020). Reusable and recyclable graphene masks with outstanding superhydrophobic and photothermal performances. ACS Nano 14, 6213–6221. doi:10.1021/acsnano.0c02250
Zhou, S., Jin, M., Tan, R., Shen, Z., Yin, J., Qiu, Z., et al. (2022). A reduced graphene oxide-Fe3O4 composite functionalized with cetyltrimethylammonium bromide for efficient adsorption of SARS-CoV-2 spike pseudovirus and human enteric viruses. Chemosphere 291, 132995. doi:10.1016/j.chemosphere.2021.132995
Keywords: SARS-2, pandemic, contact-killing, virucidal solids, metallic nanoparticles, carbon based nanomaterials, silicon nanoparticles, self sanitizatio
Citation: Hussain FS, Abro NQ, Ahmed N, Memon SQ and Memon N (2022) Nano-antivirals: A comprehensive review. Front. Nanotechnol. 4:1064615. doi: 10.3389/fnano.2022.1064615
Received: 08 October 2022; Accepted: 24 November 2022;
Published: 13 December 2022.
Edited by:
Nicola Maria Pugno, University of Trento, ItalyReviewed by:
Hamed Barabadi, Shahid Beheshti University of Medical Sciences, IranSandra Elizabeth Rodil, National Autonomous University of Mexico, Mexico
Copyright © 2022 Hussain, Abro, Ahmed, Memon and Memon. This is an open-access article distributed under the terms of the Creative Commons Attribution License (CC BY). The use, distribution or reproduction in other forums is permitted, provided the original author(s) and the copyright owner(s) are credited and that the original publication in this journal is cited, in accordance with accepted academic practice. No use, distribution or reproduction is permitted which does not comply with these terms.
*Correspondence: Najma Memon, najma.memon@usindh.edu.pk