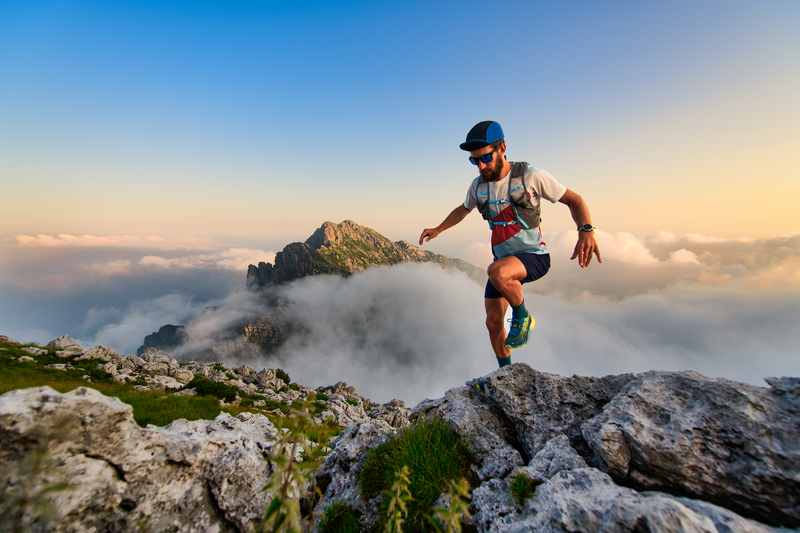
95% of researchers rate our articles as excellent or good
Learn more about the work of our research integrity team to safeguard the quality of each article we publish.
Find out more
REVIEW article
Front. Nanotechnol. , 18 November 2022
Sec. Nanomaterials
Volume 4 - 2022 | https://doi.org/10.3389/fnano.2022.1062608
This article is part of the Research Topic New Generation Nano-Biomaterials and their Potential Application in Drug Delivery and Bio-sensing View all 4 articles
Palladium (Pd) is a key component of many catalysts. Nanoparticles (NPs) offer a larger surface area than bulk materials, and with Pd cost increasing 5-fold in the last 10 years, Pd NPs are in increasing demand. Due to novel or enhanced physicochemical properties that Pd NPs exhibit at the nanoscale, Pd NPs have a wide range of applications not only in chemical catalysis, but also for example in hydrogen sensing and storage, and in medicine in photothermal, antibacterial, and anticancer therapies. Pd NPs, on the industrial scale, are currently synthesized using various chemical and physical methods. The physical methods require energy-intensive processes that include maintaining high temperatures and/or pressure. The chemical methods usually involve harmful solvents, hazardous reducing or stabilizing agents, or produce toxic pollutants and by-products. Lately, more environmentally friendly approaches for the synthesis of Pd NPs have emerged. These new approaches are based on the use of the reducing ability of phytochemicals and other biomolecules to chemically reduce Pd ions and form NPs. In this review, we describe the common physical and chemical methods used for the synthesis of Pd NPs and compare them to the plant- and bacteria-mediated biogenic synthesis methods. As size and shape determine many of the unique properties of Pd NPs on the nanoscale, special emphasis is given to the control of these parameters, clarifying how they impact current and future applications of this exciting nanomaterial.
Palladium, Pd, is a chemical element with atomic number 46. It is a silvery-white lustrous rare metal that was discovered in 1803 by the English chemist William Hyde Wollaston. Pd was named after the asteroid Pallas that had previously been discovered, in 1802. Pd, together with platinum (Pt), rhodium (Rh), ruthenium (Ru), iridium (Ir), and osmium (Os) form a group of metals that are referred to as Platinum Group Metals (PGMs). These elements have similar chemical properties overall, but Pd is the least dense and has the lowest melting temperature amongst them.
Pd is mostly extracted from ore deposits in a few countries. In 2020, the overall mine production of Pd reached 210,000 kg; major producers are illustrated in Figure 1A (Geological Survey, 2020). Recycling Pd forms an additional source of supply. In 2020, 96,000 kg of Pd was recycled, mainly from automotive catalytic converters, jewelry, and electronic scrap (Key trends in the Palladium, 2022). The production of Pd is limited and cannot keep up with the increased use. Consequently, the price for Pd has dramatically increased over the last years (Figure 1B) (Geological Survey, 2020; Palladium Prices, 2022).
FIGURE 1. Pd mine production and price. (A) the major producers of Pd, where the countries shown produced 98.5% of world’s Pd in 2020. (B) the annual mine production of Pd is not growing over time (black line), while the price of one ounce of Pd has increased 5-fold in the last 10 years (blue line).
The largest application of Pd today is in automobile catalytic converts. Nobel metals are used in catalytic converters to reduce carbon monoxide, hydrocarbons, and nitrous oxide in automotive emissions by converting them to carbon dioxide and nitrogen. 90% of these pollutants are eliminated from emissions with the use of autocatalysts (Zereini and Alt, 2006). In 1993, the catalytic converter industry began producing Pd-based catalytic converts as an alternative to the traditional Pt-Rh catalyst used. Formerly, Pd had not been considered for use in automobile catalysts by the industry for two reasons: it is not as stable as Pt, and it is more prone to contamination by impurities contained in automobile emissions. However, due to technical improvements and economic influences, Pd has been increasingly used instead of Pt (Zereini and Alt, 2006).
In 2020, 302 tons of Pd were industrially consumed, and 82% of this consumption was in the automobile industry, followed by 7% in electronics, 5% in chemical catalysis, 3% in dental alloys, 2% in jewelry, and 1% in other industries (summarized in Figure 2A) (Palladium consumption worldwide in 2020, 2022). The current Pd price remains above acceptable levels for several large-scale commercial applications; Pd-based nanomaterials, and especially NPs, thus have the potential to provide more efficient and cost-effective solutions to meet the requirements of present and evolving applications (Chen and Ostrom, 2015).
FIGURE 2. Applications of Pd in different industries (Palladium consumption worldwide in 2020, 2022). (A) the industrial consumption of bulk Pd by industry in 2020. (B) the major applications of Pd NPs in chemistry, medicine, and electronics.
Pd NPs and Pd-based alloy clusters have been extensively investigated in the last decades. Pd NPs form a versatile catalyst that has a wide range of applications in organic reactions such as in hydrogenation (Favier et al., 2019), carbon–carbon bond forming [Heck reaction (Heck and Nolley, 1972) and Suzuki coupling (Miyaura et al., 1979)], as well as in petroleum cracking (Cagran and Pottlacher, 2006). In fact, the Nobel Prize in Chemistry in 2010 was awarded to Richard F. Heck, Ei-ichi Negishi, and Akira Suzuki for the discovery of Pd-catalyzed cross-coupling reactions in organic synthesis (The Nobel Prize in Chemistry, 2010). In addition to the catalysis of organic reactions, applications of Pd NPs include fuel cells (Antolini, 2009) and hydrogen sensing and storage (Adams and Chen, 2011). Potential electronics applications also include sensors (Silva et al., 2011). Moreover, several applications in biomedical therapies including photothermal, antibacterial, and anticancer therapies have been developed or discussed (Phan et al., 2020). The applications of Pd NPs are summarized in Figure 2B and are described in more detail in Section 5. In this review, we describe and compare the existing physical, chemical, and biological methods for Pd NP production.
Pd NPs, on the industrial scale, are currently synthesized by physical or chemical methods. The commonly used physical methods are physical vapor deposition, magnetron sputtering, and laser ablation. In most cases, the physical methods of synthesis require expensive equipment and energy-intensive processes that include maintaining high temperatures and/or pressure (Qazi et al., 2016; Vishnukumar et al., 2017).
Physical vapor deposition involves different techniques that provide the conversion of the material from a condensed phase (solid or liquid) to a vapor phase and then back to a condensed phase (all carried out in vacuum) (Faraji et al., 2018; Prabakaran and Rajan, 2021). In thermal evaporation deposition technique, the material to be deposited is heated to evaporation temperature using resistive heating (the production of heat by passing an electric current through a conductor). The evaporated particles travel directly to the deposition target (substrate) where they condense and form a thin film of NPs (Rajput, 2015) (Figure 3A). Another technique is based on arc deposition, in which the surface of a cathode is struck with a high-current, low-voltage arc that vaporizes the material of the cathode producing vapor that travels to the substrate where it condenses and forms NPs (Rajput, 2015) (Figure 3B). In physical vapor deposition techniques, the particle size and film thickness can be controlled by varying the deposition parameters (Mehl et al., 2015).
FIGURE 3. Principles of the common physical methods used for Pd NP synthesis. (A) thermal evaporation deposition, (B) arc deposition, (C) magnetron sputtering, and (D) pulsed laser ablation in liquid.
Sputtering is a physical process in which atoms or molecules are ejected out of a material to a substrate by the bombardment of high-energy particles. Before the 1970s, this method used high electric fields (also known as diode sputtering). However, an enhanced variant of the method emerged later in which an additional strong magnetic field is used (known as magnetron sputtering) (Dinca and Suchea, 2019). In this process, a high electric field is applied in a vacuum chamber containing an inert gas such as argon, where the cathode is attached to the metal source and the anode is attached to the substrate. Due to the collisions of electrons (released from the cathode) with the available gas molecule, positively charged (ionized) argon atoms are formed. Once formed, they are strongly attracted to the cathode resulting in high-energy collisions with the metal leading to the ejection of one or more atoms of the material. The ejected metal atoms are accelerated toward the anode bombarding its surface. As a result, small particles of that metal are formed on the substrate. The magnetic field used in this method helps in confining and trapping the electrons released from the cathode around the source (Pal et al., 2001; Musil et al., 2006) (Figure 3C). In this method, Ar pressure influences the particle size in an inverse manner, i.e., the particle size of Pd decreases with increasing Ar pressure (Slavcheva et al., 2014). The effect of using a mix of inert gases (Ar and He) on the particle size was studied by Alexeeva et al., 2015 (Alexeeva and Fateev, 2016). The researchers found that Pd particle size decreases with increasing helium flow rate due to the more frequent occurrence of three-body collisions. In addition, the He stream is extracted out of the vacuum chamber more effectively than for Ar, and nucleation time decreases in this case.
This technique is based on the irradiation of a material with a laser beam resulting in the removal of small particles from that material. The laser flux, laser wavelength, and the duration of irradiation are some of the parameters that are used to precisely control the size of released particles of the material (Chichkov et al., 1996). This technique has different variants based on the atmosphere in the chamber. A common variant of this method, known as pulsed laser ablation in liquid, is based on the immersion of the source material in a solvent. The atomized material released from the target interacts with the solvent and nucleates to form NPs (Cristoforetti et al., 2011) (Figure 3D).
The effect of different salts on the size of Pd NPs produced by pulsed laser ablation in liquid was evaluated by Marzun et al. (2015). The ablation of Pd in deionized water results in bimodal size distribution where large NPs lead to colloidal instability. It was found that the use of salts with low ionic strength (0.005 mM phosphate, 0.01 mM carbonate, 0.03 hydroxide) significantly inhibits the formation of large NPs (>30 nm). Moreover, Mendivil et al. (2015) studied the effect of variation of ablation fluence on the size of Pd NPs formed in different liquids. It was found that in distilled water the average size of NPs increased with the decrease in ablation fluence, while the opposite effect was found in sodium dodecyl sulfate (SDS) solutions.
The chemical methods for NPs synthesis rely on the chemical reduction of metal ions to zerovalent metal atoms and their nucleation to form NPs. These methods include electrochemical deposition, sonochemical preparation, supercritical fluid nucleation, and wet chemical methods such as the sol-gel method or the reduction by alcohols or other reductants. In most cases, these chemical methods of synthesis involve hazardous solvents, reducing or stabilization agents, or produce toxic by-products (Singh et al., 2018; Manjare et al., 2021).
In this method, the metal cations are reduced and deposited on the electrode of an electrolytic cell by a direct electric current. The electrolytic cell is filled with a salt solution of the metal to be deposited, in which the positive metal cations are attracted to the cathode of the cell and reduced on its surface to zerovalent metal by gaining the required electrons (Diculescu et al., 2007; Shendage et al., 2013). In some cases, the opposite reaction may occur on the surface of the anode (oxidation of the metal to form cations), thus, the anode should be made of a material that is resistant to electrochemical oxidation, such as carbon or lead (Dufour, 2006). Figure 4A graphically illustrates this method.
FIGURE 4. Principles of the common chemical methods used for Pd NP synthesis. (A) electrochemical deposition, (B) sonochemical preparation, (C) supercritical fluid nucleation, and (D) sol-gel method.
For the synthesis of size-controlled Pd NPs using this method, a chemical stabilizing agent should be added into the solution. The stabilizing agents, such as poly (N-vinylpyrrolidone) (PVP), promote the formation process of Pd NPs, inhibit the electrodeposition process of Pd onto the cathode, and stabilize the formed NPs (Pan et al., 2008). The size of the NPs can by controlled by adjusting the applied current and the concentration of the stabilizing agent. For instance, for the synthesis of Pd NPs using tetrachloropalladate as the precursor, the concentration of PVP was found to be inversely proportional with the mean diameter of the NPs formed. Changing the concentration of PVP from 15 to 30 g dm−3 resulted in lowering the mean diameter of the NPs formed from 22.2 to 10.1 nm. For controlling the size of NPs by adjusting the applied current, the researchers found that the higher the current density, the higher the overpotential, and thus the smaller the size of the NPs formed (Pan et al., 2008).
This process is based on the application of powerful ultrasound frequencies (20 kHz–10 MHz) on a solution containing the ions of the metal in question in order to generate NPs (Gedanken, 2004). In this range of frequency, the ultrasonic waves have wavelengths ranging from 10 cm to 100 μm, much larger than the molecular size. Consequently, the effects on the chemistry of molecules do not arise from the direct interaction between sound waves and chemical species, but rather from a physical phenomenon called acoustic cavitation, which is defined as the formation, growth, and the implosive collapse of bubbles (Xu et al., 2013). When sound waves propagate through a liquid, alternating expansive and compressive waves produce dynamic tensile stress changing the density of the liquid, which creates bubbles from pre-existing impurities. At a critical size, these bubbles collapse adiabatically, producing the characteristic extreme and transient conditions of sonochemistry: temperatures above 5000 K and pressures exceeding 1000 bar (Xu et al., 2013). Under these extreme conditions in so called hot spots, high-energy chemical reactions occur such as the dissociation of chemical bonds. At the same time, nonvolatile precursors may undergo chemical reactions with radicals or other high-energy products of sonolysis inside the collapsing bubbles, providing electrons for the reduction of metal cations. When these atoms are injected into the liquid phase, they nucleate and form NPs (Xu et al., 2013). In some cases, chemical reducing and stabilizing agents such as ethylene glycol and poly (vinylpyrrolidone), respectively, are added to the liquid phase in order to control the size and the shape of Pd NPs produced (Nemamcha et al., 2006). Figure 4B graphically illustrates this method.
Similar to electrochemical deposition, a chemical stabilizing agent should be added into the solution in order to synthesize size-controlled Pd NPs sonochemically. The size and shape of the NPs formed can be controlled by varying the concentration of the stabilizing agent, the current density, the ultrasonic intensity, and the interval between two continuous ultrasonic pulses (Qiu et al., 2003). Sonochemical preparation method is also used to synthesize substrate-supported Pd NPs, such as alumina-supported Pd NPs. For that, an alcohol additive is typically added to the solution in order to accelerate the reduction of Pd(II) ions. The size of supported-NPs can be controlled by varying the alcohol additive, the concentration of alcohol additive, and the concentration of the support particles (Okitsu et al., 1999; Okitsu et al., 2000).
This method is based on the saturation of a supercritical fluid with a solid substrate, and the depressurization of the solution through a nozzle into a low-pressure chamber. This process results in the nucleation of the material of the substrate, generating NPs that are collected from the gaseous stream (Byrappa et al., 2008). There are different variants for this method, however, rapid expansion of supercritical solution (RESS) is the common variant used for Pd NPs synthesis, in which supercritical carbon dioxide is usually used as the supercritical fluid (Kameo et al., 2003). Figure 4C graphically illustrates this method.
Supercritical fluid nucleation is used to synthesize substrate-supported and functionalized Pd NPs (Cansell and Aymonier, 2009; Tang and Shim, 2015). For the synthesis of substrate-supported Pd NPs, the substate is mixed together with Pd precursor in a pressure reactor. The size of the NPs can be easily controlled by adjusting the concentration of the Pd precursor, for instance, adjusting the concentration of palladium (II) hexafluoroacetylacetonate in the process of synthesizing RGO (reduced graphene oxide)-supported Pd NPs (Tang and Shim, 2015). In addition, supercritical fluid nucleation has been used to synthesize Pd NPs functionalized with fluorinated and non-fluorinated alkyl moieties, Pd NPs capped with thiols, and Pd-impregnated Nafion membranes (Jiang et al., 2005; Moisan et al., 2008; Cansell and Aymonier, 2009). The synthesis of functionalized NPs using this approach is comparatively simple, as it limits the number of steps in the preparation of functional NPs. The size of the NPs formed can also be controlled by varying the solvent, and the operating parameters such as the temperature (Cansell and Aymonier, 2009).
This technique is based on the conversion of a colloidal solution (sol) into a gel composed of discrete metal NPs. This method involves five different stages: hydrolysis, condensation, ageing, drying, and calcination. Firstly, the NP precursor (a metal alkoxide) is dissolved in water or organic solvents to produce a homogenous solution. Then, an acid or base is added to the solution to achieve the hydrolysis/alcoholysis of the precursor, forming a colloidal solution. In a next step, the solvent is removed to achieve the condensation of adjacent molecules through metal linkages. Condensation occurs via two processes; the formation of a (-OH-) bridge between two metal centers (called olation), or the formation of (-O-) bridge between two metal centers (called oxolation). Both types of condensation increase the viscosity of the solution forming a porous structure called gel. Next, an aging step is done where the condensation of the solution continues slowly, decreasing the porosity and increasing the distance between colloidal particles. Then, the gel is thermally dried to evaporate the remaining solvent. Lastly, the dried gel is calcinated and grinded to form NP powders (Kumari et al., 2019). Figure 4D graphically illustrates this method.
The sol-gel method is used to synthesize Pd composites such as Pd@SiO2 core-shell NPs (Hu et al., 2013; Li et al., 2014). The nanosized Pd cores are formed by reducing tetrachloropalladate with sodium borohydride reducing agent and tetradecyl trimethyl ammonium bromide (TTAB) as a stabilizing agent, then the silica shells were formed by using tetraethyl orthosilicate (TEOS). The size of NPs and the thickness of the shells can be controlled by varying the concentration of the chemicals, the molar ratios, and the pH value of the solutions (Hu et al., 2013).
There are several disadvantages for the physical and chemical methods used for Pd NPs synthesis. The physical methods require energy-intensive instrumentation that include maintaining high temperature or pressure. The chemical methods may involve harmful solvents, hazardous chemical reducing or stabilization agents such as sodium borohydride and hydrazine, or produce toxic pollutants and by-products. In addition, the chemical synthesis of Pd NPs may result in adsorbing the chemical surfactants or toxic molecules on the surface of the NPs, which would compromise the use of these NPs in biomedical and pharmaceutical applications (Qazi et al., 2016; Vishnukumar et al., 2017; Fahmy et al., 2020; Manjare et al., 2021).
Recently, the biogenic synthesis of NPs has attracted attention from the scientific community as it could in principle provide simple, rapid, cost-effective, and potentially more environmentally friendly alternative processes for the synthesis of NPs. These processes are based on the use of naturally occurring biomolecules or metabolites from different organisms as reducing and stabilizing agents. In addition, these biogenic processes have been shown to offer very high levels of control over the properties of NPs, such as the size and shape (Qazi et al., 2016; Vishnukumar et al., 2017; Fahmy et al., 2020; Manjare et al., 2021).
Biogenic synthesis of NPs can be achieved through plant-mediated, bacteria-mediated, algae-mediated, or fungi-mediated processes. For the synthesis of Pd NPs, a Pd precursor (Pd salt solution) is mixed with either a plant extract or with bacterial/algal/fungal biomass, usually at room temperature. The synthesis process immediately starts and frequently lasts no more than 2 hours, which is indicated by the color of the reaction medium (the color typically changes to dark brown or black) (Fahmy et al., 2020).
The exact mechanism of the biogenic synthesis of Pd NPs is not fully understood and requires more research, however, three major steps have been suggested. Firstly, Pd2+ ions need to be reduced to zerovalent Pd by acquiring electrons from a donor biomolecule or metabolite. Then, the reduced ions nucleate and start to grow and agglomerate together forming thermodynamically stable Pd NPs. Lastly, the synthesized NPs are capped by a variety of functional groups such as aldehydes, ketones, alcohols, amines, or carboxylic acids, which help in the stabilization of the NPs. The synthesized NPs can be of different sizes or form different shapes depending on the reaction conditions (Qazi et al., 2016; Vishnukumar et al., 2017; Fahmy et al., 2020; Manjare et al., 2021). Figure 5 graphically illustrates the three major steps of the biogenic synthesis of Pd NPs.
FIGURE 5. The mechanism of biogenic synthesis of Pd NPs. The chemical compound presented in this figure (gallic acid) is an example (Jia et al., 2009).
Plants possess natural mechanisms for the detoxification of metal ions in the soil. These processes can be exploited for the purification of ground water and for coping with soil salinity (Manjare et al., 2021). The term phytoremediation has been coined for plant-based approaches for the extraction, removal, or for lowering the availability of soil pollutants (Yan et al., 2020). Phytoremediation includes several mechanisms depending on the plant in question and on the pollutant, such as phytoaccumulation, phytofiltration, phytostabilization, and phytodegradation (Ali et al., 2013). These mechanisms are the basis for the development of plant-mediated synthesis methods of NPs.
Plant-mediated synthesis of NPs is based on the bioactive compounds in plant extracts, which include polyphenols, alkaloids, terpenoids, flavonoids. These compounds are believed to be responsible for the reduction of metal ions into metal NPs (Vishnukumar et al., 2017). Extracts of various plant parts, e.g., leaves, seeds, fruits, and roots, have been successfully employed to biologically synthesize Pd NPs with different sizes and shapes (Table 1). The size and morphology of plant-mediated Pd NPs are affected by Pd precursor concentration, the concentration of the plant extract, and the duration, temperature, and pH of the reaction (Qazi et al., 2016; Vishnukumar et al., 2017; Fahmy et al., 2020; Manjare et al., 2021).
Examples: leaf extract of Anacardium occidentale was used for the synthesis of 2.5–4.5 nm spherical Pd NPs. Fourier-transform infrared spectroscopy (FTIR) analysis showed the involvement of soluble polyols in the reduction of Pd ions, and of negatively charged carboxylate ions together with protein molecules in the stabilization of Pd NPs (Sheny et al., 2012). Several other plant extracts were also successfully used to produce small spherical Pd NPs with a narrow size distribution. A fruit extract of Gardenia jasminoides Ellis yielded 3–5 nm spherical Pd NPs. In this case, FTIR analysis showed that the antioxidants present in the crude water extract of the fruit (such as geniposide, chlorogenic acid, crocins and crocetin), were involved in the production of Pd NPs, and that the properties of the Pd NPs are temperature-dependent. At different temperatures ranging from 40 to 90°C, the synthesis reaction produced Pd NPs where the size, shape, and dispersity change systematically (Jia et al., 2009). Other examples include the tuber extract of Gloriosa superba (Rokade et al., 2018), the leaf extract of Parthenium hysterophorus (Ahsan et al., 2020), and the seed extract of Piper nigrum (Kandathil et al., 2018) that also produced small spherical NPs with narrow size distributions between 5 and 8 nm, 2 and 6 nm, and 2 and 7 nm, respectively.
Other plant extracts can yield larger spherical Pd NPs or NPs populations with wide size distributions. The Pd NPs produced by the fruit peel extract of Annona squamosa L. (Roopan et al., 2012) and rind extract of Citrullus lanatus (Lakshmipathy et al., 2015) were 80 nm and 96 nm, respectively. For Annona squamosa L., the exact reducing molecule was not identified, however, aldehyde and hydroxyl functional groups were found to be involved as capping agent for the synthesized NPs. For Citrullus lanatus, the reduction and capping of Pd NPs was dependent on polyhydroxylated molecules. Regarding the large size distribution, leaf extracts of Pelargonium graveolens (Li et al., 2017) and Prunus × yedoensis (Manikandan et al., 2016) yielded 50–140 nm NPs and 50–150 nm NPs, respectively. The reason why some plant extracts produce Pd NPs with a narrow size distribution and others with a broad size distribution is unknown. However, we speculate that the large size distribution is due to the presence of several biomolecules that could act as reducing and capping agents in parallel, each resulting in a differently sized Pd NPs.
The shape of plant-mediated NPs is not limited to spherical particles. Several plant extracts were found to synthesize Pd NPs with distinct shapes. The bark extract of Bauhinia variegata produced 1–9 nm cylindrical Pd NPs (Vaghela et al., 2018), the leaf extract of Lithodora hispidula (Sm.) Griseb produced 22 nm rod-shaped Pd NPs (Turunc et al., 2017), the leaf extract of Rubus glaucus Benth. produced 55–60 nm decahedral Pd NPs (Kumar et al., 2015), and the fruit extract of Terminalia chebula produced 80–100 nm cubic Pd NPs (Kumar et al., 2013). The shape of the biogenically synthesized Pd NPs in these examples was attributed to the biomolecules acting as reducing and capping agents, or to the specific reaction conditions.
Only in some cases, the specific reducing agent/s responsible for reducing Pd2+ to Pd0 in the extract solutions were identified or at least investigated in some detail. For the leaf extract of Sapium sebiferum, the phenolic compound 6-O-galloyl-D-glucose was suggested as the reducing agent of Pd2+. This compound donates one electron per molecule from one of its hydroxyl groups (Figure 6A). As a result, the leaf extract of this plant produces 5 nm spherical Pd NPs (Tahir et al., 2016a). Another phenolic compound was found in the leaf extract of Phoenix dactylifera; catechin also donates one electron per molecule from one of it hydroxyl groups (Figure 6B), producing 2–25 nm spherical Pd NPs (Tahir et al., 2016b). For the leaf extract of Glycine max, the amino acid tyrosine was suggested to be the main reducing agent of Pd2+ (Figure 6C). The leaf extract of this plant produces 15 nm spherical Pd NPs (Kumar Petla et al., 2012). For the leaf extract of Delonix regia, different phenolic compounds, namely gallic acid, protocatechuic acid, 3-hydroxybenzoic acid, and catechin, were suggested as electron donors for the reduction of Pd2+ ions. Unlike the aforementioned compounds, gallic acid donates two electrons per molecule from two of its hydroxyl groups, thus, only one molecule is needed for the complete reduction of one Pd2+ ion (Figure 6D). The leaf extract of Delonix regia produces 2–4 nm Pd NPs (Dauthal and Mukhopadhyay, 2013).
FIGURE 6. Suggested plant compounds responsible for the reduction of Pd2+ ions. (A) 6-O-galloyl-D-glucose (Sapium sebiferum, produces 5 nm spherical Pd NPs), (B) catechin (Phoenix dactylifera, produces 2–25 nm spherical Pd NPs), (C) tyrosine (Glycine max, produces 15 nm spherical Pd NPs), and (D) gallic acid (Delonix regia, produces 2–4 nm Pd NPs).
In addition to monometallic Pd NPs, bimetallic, trimetallic, and substrate-supported Pd NPs can also be made using plant extracts (Table 2). For bimetallic Pd NPs, 5–40 nm spherical AuPd NPs were produced by Myrica gale tannin in a one-step reduction reaction. The synthesized NPs had an Au core and Pd shell structure. Interestingly, the researchers found that changing the molar ratio of Au3+/Pd2+ and the concentration of Myrica gale tannin results in changes on the morphology of the Pd shell from spherical to cubic. Adding Au3+ and Pd2+ in a 1:1 M ratio with 0.4 g/L Myrica gale tannin resulted in the synthesis of AuPd NPs with a cubic Pd shell, in which some of these bimetallic NPs had multiple Au cores per Pd shell. By decreasing the concentration of Myrica gale tannin to 0.1 g/L, spherical Pd shells were generated. Modifying the molar ratio of Au3+/Pd2+ from 1:1 to 2:1 also resulted in the synthesis of spherical Pd shells (Huang et al., 2011). Many other examples for the production of bimetallic Pd NPs using various plant extracts exist. The seed extract of Peganum harmala L. produced 24–44 nm spherical PtPd bimetallic NPs (Fahmy et al., 2021). Camellia sinensis and Vitis vinifera leaf extracts produced 20–30 nm and 2–20 nm FePd bimetallic NPs, respectively (Smuleac et al., 2011; Luo et al., 2016). The leaf extract of Cacumen platyclade produced 7–13 nm AgPd bimetallic NPs (Lu et al., 2014).
Plant extracts can also be used to produce monometallic or bimetallic Pd NPs supported on different oxide substrates. The root extract of Euphorbia condylocarpa M. Bieb. (Nasrollahzadeh et al., 2015a). and Euphorbia stracheyi Boiss. (Nasrollahzadeh and Sajadi, 2016a). and the flower extract of Fritillaria imperialis (Veisi et al., 2021) were used to produce monometallic Pd NPs supported on Fe3O4. The parameters of the biosynthesized NPs were different in each case, being 39 nm with irregular shapes, 10 nm spherical, and 20–30 nm quasi-spherical, respectively. The fruit extract of Berberis vulgaris produced 18 nm spherical Pd NPs supported on RGO (reduced graphene oxide) (Nasrollahzadeh et al., 2016), while the fruit extract of Piper longum produced 6–26 nm spherical Pd NPs supported on natrolite zeolite (Hatamifard et al., 2015). The seed extract of Theobroma cacao L. was used to produce 40 nm Pd NPs supported on CuO (Nasrollahzadeh et al., 2015b). The leaf extract of Myrtus communis L. yielded 17–25 nm spherical Pd NPs supported on TiO2 (Nasrollahzadeh and Sajadi, 2016b). Other plant extracts were successfully used to synthesize bimetallic Pd NPs supported on different oxide substrates. For instance, the leaf extract of Cacumen platycladi was used to produce 20–30 nm spherical AuPd NPs supported on TiO2 (Hong et al., 2014), and 9.7 nm spherical AuPd NPs supported on MgO (Zhan et al., 2012). Even trimetallic Pd NPs can be synthesized using plant extracts. For this, the extract of two plants was used to provide the reducing and capping agents required for the biosynthesis of trimetallic-alloy NPs. The bud extract of Syzygium aromaticum together with the leaf extract of Aegle marmelos was used to produce 8–11 nm spherical AgAuPd NPs (Rao and Paria, 2015).
Unlike in the case of plant extracts, bacteria-mediated synthesis of Pd NPs requires live bacteria for the reduction and formation of Pd NPs. The first report of bacteria able to produce Pd NPs was on the anaerobic sulfate-reducing bacterium Desulfovibrio desulfuricans (Lloyd et al., 1998). The bacterial biomass was mixed with a Pd precursor and with external, bioavailable electron donors (sodium pyruvate, formate, or H2 gas) simultaneously. The color of the reaction medium changed to black within 10 min and the color intensified within up to 24 h. The controls (heat-killed bacterial biomass and live biomass lacking the electron donors) did not undergo any color change. The examination of bacteria under Transmission electron microscopy (TEM) revealed the deposition of 50 nm Pd NPs in the periplasmic space, whereas the controls did not show any NPs. This strongly suggested that the reduction of Pd2+ ions to elemental Pd is based on the action of enzymes. The researchers suspected the involvement of hydrogenase enzymes in this process. Therefore, they inhibited those enzymes by adding 0.5 mM Cu2+. They found that Cu2+-treated bacterial solution did not change in color and did not show any Pd NPs in TEM. This supported the direct involvement of hydrogenase enzymes in the reduction of Pd2+ ions (Lloyd et al., 1998).
A second bacterium able to synthesize Pd NPs is the dissimilatory metal-reducing species Shewanella oneidensis MR-1 (De Windt et al., 2005). In that study, three main variables were tested: different electron donors, alternative electron acceptors, and the atmospheric conditions (aerobic or anaerobic). It was found that all tested electron donors resulted in the bioreduction of Pd2+ and formation of Pd NPs. However, formate and H2 resulted in larger amounts of NPs than pyruvate, lactate, and ethanol. The addition of alternative electron acceptors such as nitrate and the exposure to oxygen did not affect the formation of Pd NPs. The synthesized Pd NPs were deposited on the outer membrane and in the periplasmic space of the bacteria. Pd NPs synthesized in the presence of H2 as an electron donor were smaller than those formed in the presence of formate. In this paper, the researchers also suggested the involvement of hydrogenase enzymes in the process of Pd NPs synthesis.
Another sulfate-reducing bacterium, Desulfovibrio fructosivorans, was reported for its ability for synthesize Pd NPs (Mikheenko et al., 2008). In that study, the deposition of Pd NPs was tested for the wildtype and for mutants lacking the periplasmic NiFe hydrogenase gene, the periplasmic Fe hydrogenase gene, or both. It was found that the wildtype strain deposited small Pd NPs on both the cytoplasmic and outer membranes, while the mutant lacking both hydrogenase gene deposited large Pd NPs only on the cytoplasmic membrane. The researchers suggested that the role of periplasmic hydrogenases is to serve as a nucleation site assisting Pd NPs growth, where their gene knock-out experiments result in the absence of Pd NPs in the outer membrane.
Bacteria-mediated Pd NPs synthesis is not limited to sulfate- and metal-reducing bacteria. Two strains of cyanobacteria (Calothrix pulvinate and Anabaena flos-aquae) (Brayner et al., 2007), Clostridium pasteurianum (Chidambaram et al., 2010), and different gram-negative alpha-, beta- and Gamma-proteobacteria bacteria, including Paracoccus denitrificans, Cupriavidus necator H16, and Pseudomonas putida (Bunge et al., 2010), were reported to have the ability to synthesize Pd NPs. For the latter three species, 3–30 nm Pd NPs were synthesized in the periplasmic space when using formate as electron donor. In these cases, Pd NPs synthesis also proceeds in autoclaved cells where all proteins are inactivated, thus ruling out the direct involvement of hydrogenases in the synthesis of Pd NPs. For cyanobacteria, the nitrogenase enzyme complex was suggested to be responsible for the reduction of Pd2+ ions to metallic Pd.
Also the model bacterium E. coli can synthesize Pd NPs (Deplanche et al., 2010). In general, the biogenic synthesis process of Pd NPs is divided into two separate steps: the bacterial biosorption or uptake of Pd2+ ions from the reactions medium, and the reduction of Pd2+ ions to elemental Pd inside the bacteria (in the periplasm and/or the cytoplasm). In this study, the ability of E. coli wildtype and mutants lacking the genes encoding hydrogenase enzymes were assessed for their ability to take up and reduce Pd2+ ions. None of the mutants lost its ability to take up Pd2+ ions from the medium, suggesting that the three hydrogenases present in E. coli (Hyd-1, Hyd-2, and Hyd-3) are not involved in this step. For the reduction step, the mutants having only Hyd-1 or Hyd-2 did not show any significant difference compared to the wildtype. The mutant having only Hyd-3 was significantly slower in the reduction of Pd2+ ions. Hyd-1 and Hyd-2 hydrogenases are periplasmic hydrogenases (the catalytic subunit is exposed to the periplasmic side of the membrane), and apparently the presence of one of these hydrogenases is enough to reduce Pd2+ in the periplasm (where Pd2+ ions are expected to be). The catalytic subunit of Hyd-3 is facing the cytoplasm, and thus cannot fully reduce the Pd ions in the periplasm efficiently. The mutant lacking all the three hydrogenases was found to lose the ability to reduce Pd2+ into Pd NPs completely. Therefore, the hydrogenases are key enzymes for the reduction of Pd2+ ions in E. coli. Under TEM, the mutants having only Hyd-1, Hyd-2, or Hyd-3 hydrogenases showed somewhat different patterns of Pd NP deposition. For the mutant lacking these three hydrogenases, all the periplasmic and cytoplasmic small Pd NPs were not present. Instead, big clumps of Pd were deposited on the surface of the bacteria. These big clumps of Pd were suggested to be formed chemically rather than biologically at a slower rate compared to the biogenic process (Deplanche et al., 2010). After reports that Pd NPs can be synthesized under fermentative conditions in Clostridium pasteurianum (Chidambaram et al., 2010) and E. coli (Deplanche et al., 2010), Hennebel et al. (2011) tested five additional bacterial species (Bacteroides vulgatus, Citrobacter braakii, Clostridium butyricum, Enterococcus faecium, and Klebsiella pneumoniae) under fermentative conditions for their ability to produce biogenic Pd NPs. All species were able to reduce Pd2+ and to form crystalline Pd0 NPs under fermentative conditions. The researchers suspected that the H2 produced during the fermentation process was the reducing agent of Pd2+ ions. However, in a control experiment where the cells were centrifuged and resuspended in fresh medium to remove H2 before the addition of Pd precursor, Pd NPs were also observed at the cell surfaces. Therefore, it was concluded that Pd2+ ions are reduced enzymatically, by H2, or by a combination of the two processes. The selected bacterial species have different metabolic pathways and produce different amounts of H2 gas per 1 molecule of glucose. However, no correlation between the reduction of Pd2+ and the amount of H2 produced was found. The difference in Pd NP deposition patterns among the species was attributed to the differences in cell structure and composition, enzyme production, Pd toxicity resistance, and fermentation product type and its distribution.
New reports of bacterial strains and species able to synthesize Pd NPs (Table 3), along with methods to optimize the reaction conditions and control the yield or the properties of Pd NPs are continuously published. Great efforts have been made in order to understand the influence of different abiotic factors on the biogenic synthesis of Pd NPs, and to characterize Pd NPs produced by different microorganisms (Omajali et al., 2015; Chen et al., 2018; Chen et al., 2019; Bachar et al., 2020; Tan et al., 2020). Other studies demonstrate the successful production of bimetallic Pd NPs such as Pd/Au, Pd/Ag, Pd/Pt, and Pd/Ru NPs using various bacterial strains (Murray et al., 2018; Omajali et al., 2019; Kimber et al., 2021). But despite these efforts, we are not much closer to understanding the underlying biological mechanisms for the formation of Pd NPs. No other enzymes or proteins except for the hydrogenases have been reported to be involved until recently.
In 2020, Matsumoto et al. (2020) performed a high-throughput experiment for the identification of genes responsible for the reduction of Pd ions in E. coli. The researchers screened the Keio collection, a single-gene knockout library of E. coli BW25113 (Baba et al., 2006). The difference in Pd-ion reduction ability for approximately 4000 mutants was measured through the change in the optical density (OD600) between the mutants and the wildtype (relative reduction rate). The results showed that seven knock-out strains had a relative reduction rate increased more than 1.5-fold, suggesting that the genes missing in those knock-outs were repressing Pd reduction. At the same time, 261 knock-outs had a relative reduction rate decreased more than 0.5-fold (73 of which had a relative reduction rate lower than 0.3-fold), suggesting that the genes missing in those knock-outs are participating in Pd ion reduction. No single-gene knock-out had a relative reduction rate of zero, suggesting that the reduction process of Pd ions is complicated and is not carried out by only a single enzyme or protein.
The genes repressing Pd reduction were related to membrane transport, transcriptional regulation, and metabolism. The researchers believe that the deletion of transcriptional regulators has broad effects on metabolism and physiology, while the deletion of membrane transport or metabolic enzyme genes can directly affect Pd ion reduction. Therefore, these genes can be candidate genes for repressing Pd reduction. The list of the genes promoting Pd reduction included 3 genes from formate metabolism. The authors highlight that formate metabolism genes could be directly involved in Pd ion reduction since formate was used as an external electron donor for the synthesis of Pd NPs. Therefore, their knock-out directly affected the reduction of Pd ions (Matsumoto et al., 2020). However, these results provide just a list of candidate genes that might be involved in Pd reduction, therefore, more work is needed to find out the role of each of these genes.
In addition to plants and bacteria, other organisms such as algae, yeast, and fungi are also able to biologically synthesize Pd NPs with different sizes and shapes (Table 4). Several algal species have been successfully used to produce biogenic Pd NPs: Botryococcus braunii produces 2–7 nm Pd NPs (Anju et al., 2020), Chlorella vulgaris produces 5–20 nm spherical Pd NPs (Arsiya et al., 2017), Dictyota indica produces 19 nm spherical Pd NPs (Yazdani et al., 2018), Padina boryana produces 5–20 spherical Pd NPs (Sonbol et al., 2021), and Sargassum bovinum produces 5–10 nm octahedral Pd NPs (Momeni and Nabipour, 2015). Yeast and fungi can also biosynthesize Pd NPs. Dry granules of Saccharomyces cerevisiae yeast produced 32 nm hexagonal Pd NPs (Sriramulu and Sumathi, 2018), while the fruit extract of Agaricus bisporus mushroom produced 13–18 nm triangular Pd NPs (Mohana and Sumathi, 2020).
As an alternative to using the extract of various plant parts or the biomass of different microorganisms, specific bio-derived materials or substances for the synthesis of Pd NPs can be employed (see bottom of Table 4). For instance, a wide range of sugars and polysaccharides has been intensively utilized for this purpose. Using the products generated from acidic/alkaline treatment of glucose (Monopoli et al., 2010) and sucrose (Amornkitbamrung et al., 2014) as reducing agents for Pd2+ produced 15 nm and 4.3 nm spherical Pd NPs, respectively, while the use of a mix of glucose and starch produced 1–20 nm quasi-spherical and octahedral Pd NPs (Gioria et al., 2020). The acidic/alkaline treatment of glucose and sucrose generates chemical intermediates with aldehyde functionality, which act as reducing species for Pd ions. To produce Pd NPs with narrow size distribution and to prevent them from aggregation, a stabilizing agent should be used. For the production of Pd NPs using glucose, a chemical surfactant (tetrabutylammonium hydroxide) was used to stabilize the NPs (Monopoli et al., 2010). For the production of Pd NPs using sucrose, no additional stabilizing agent was needed as sucrose itself or its degradation products in the reaction act as a stabilizing agent preventing the NPs from aggregation (Amornkitbamrung et al., 2014). In a comparison of glucose and starch, glucose acted as a reducing agent while starch acts as a stabilizing agent (Gioria et al., 2020). The polysaccharides of different plant and bacterial gums have also been used for the biosynthesis of Pd NPs. For instance, guar gum produced 70–80 nm spherical Pd NPs (Anjum et al., 2020), gum acacia produced 9 nm spherical Pd NPs (Devi et al., 2011), and xanthan gum produced 10 nm spherical Pd NPs (Venkatesham et al., 2015). In addition, other sugars such as glucosamine (Ullah et al., 2018) and glucomannan (Chen et al., 2021) resulted in the synthesis of 3–9 nm and 6 nm spherical Pd NPs, respectively. Interestingly, gums, glucosamine, and glucomannan acted as both reducing and stabilization agents for the production of Pd NPs. These carbohydrate polymers act natural templates for the nucleation and growth of Pd NPs, acting as both reducing and stabilizing agents due to their functional and structural properties (Chen et al., 2021).
In addition to polysaccharides, other biological compounds such as vitamins and hormones were successfully used for the synthesis of Pd NPs previously. Ascorbic acid is a well-known reducing agent, and the use of ascorbic acid as a reducing agent together with sodium alginate as a stabilizing agent produced 13–33 nm spherical Pd NPs (Ameri et al., 2020). However, when chitosan was used as a stabilizing agent together with ascorbic acid, 30 nm flower-shaped Pd NPs were formed (Phan et al., 2019). The use of oxytocin hormone produced 15–20 nm polygonal Pd NPs; in this case, the amino acids present in oxytocin acted as both reducing and stabilizing agents (Bendre et al., 2020).
Many other bio-derived materials can act as both reducing and stabilizing agents and have been successfully used for the biosynthesis of Pd NPs, including compounds such as hyaluronic acid (Yin et al., 2021), lentinan (Han et al., 2019), lignin (Coccia et al., 2012), sodium alginate (Xiong et al., 2020), and tannic acid (Kumari et al., 2013). The synthesized Pd NPs were 1.5–5 nm, 2–5 nm, 16–20 nm, 2 nm, and 11.3 nm spherical Pd NPs, respectively.
Biogenic NPs, due to their unique physicochemical properties combined with properties of capping materials, display interesting catalytic reactivity, selectivity, and product yield in numerous organic reactions (Mughal et al., 2021; Palem et al., 2022). There are also extensive studies confirming their environmental advantage over physiochemically produced NPs (Palem et al., 2022). Specifically, Pd is a key catalyst in organic cross-coupling reactions such as Heck and Suzuki coupling reactions (Cui et al., 2017; Miller et al., 2017; Biffis et al., 2018). The unique electronic ground-state structure of Pd (4d105s0) and the square-planar geometry of Pd(II) complexes with additional coordination sites at the axial positions, which can accommodate various ligands, give Pd unique properties in C-C bond formation and C-O bond cleavage (Jbara et al., 2017). Therefore, a wide range of biogenically produced Pd NPs is being tested for their catalytic performance.
Examples: Pd NPs produced by Camellia sinensis leaf extract showed potent catalytic activity for the synthesis of biaryls via Suzuki cross-coupling reaction and for the reduction of 4-nitrophenol. At the same time, the NPs displayed high reusability where the catalytic activity was not affected after the reuse of catalyst for nine cycles (Lebaschi et al., 2017). Pd NPs produced by Citrullus lanatus rind extract also showed potent catalytic activity for Suzuki cross-coupling reaction, in which the catalyst displayed high product yield for 12 different coupling reactions (Lakshmipathy et al., 2015). The biogenic Pd NPs produced by Hibiscus sabdariffa L. were tested for 23 different Suzuki cross-coupling reactions. The reaction time and yield were high except for three reactions. The reusability of the catalyst was also high, the yield was not significantly affected after 6 catalytic cycles (Hekmati et al., 2017). Interestingly, Pd NPs produced by Colocasia esculenta leaf extract exhibited superior catalytic activity for Suzuki cross-coupling reactions over conventional catalysts. Here, the reaction with the biogenic catalyst required a shorter time and less catalyst. However, the reusability of the biogenic catalyst was not high, the yield reduced 20% after five catalytic cycles (KumaráBorah et al., 2015).
For Heck cross-coupling reactions, the biogenic Pd NPs produced by Rosmarinus officinalis leaf extract were tested for 22 reactions between different combinations of aryl halides and olefins. The product yield was high for all aryl halides expect for aryl chlorides. However, in all Heck olefination reactions, the selectivity for the trans-product was 100%. At the same time, the researchers optimized the conditions for Heck cross-coupling reaction between iodobenzene and methyl acrylate using the biogenic catalyst and achieved >99% product yield. This yield was higher than the yield of four conventional catalysts used for comparison in the same study (Rabiee et al., 2020). Bacterial Pd NPs also exhibit potent catalytic performance in Heck cross-coupling reactions. The biogenic Pd NPs produced by Desulfovibrio desulfuricans were tested for Heck cross-coupling reaction between iodobenzene and ethyl acrylate in different reaction conditions. The researchers found that the reaction rate can reach values up to 95% with the biogenic catalyst compared to only 82% with the conventional one (Bennett et al., 2013). Aside from cross-coupling reactions, biogenic Pd NPs exhibited also potent catalytic performance in hydrogenation reactions (Jia et al., 2009; Dauthal and Mukhopadhyay, 2013), reduction reactions (Veisi et al., 2016a; Sharmila et al., 2017a; Gopalakrishnan et al., 2017; Veisi et al., 2021), and photocatalysis (Tahir et al., 2016a; Turunc et al., 2017).
Metal NPs are widely known for their antibacterial activity. Several mechanisms of action for this activity were proposed, including the production of reactive oxygen species, DNA damage, cellular transport disruption, cellular membrane disruption, ATP depletion, and protein denaturation through the interaction with thiol groups (Rawashdeh and Haik, 2009; Rana and Kalaichelvan, 2011; Tahir et al., 2016a; Slavin et al., 2017), and probably a combination of these mechanisms makes the bacteria fail to establish a defense mechanism for adaptation and survival. Therefore, metal NPs are considered a promising agent for treating multi-drug resistant bacteria (Slavin et al., 2017). Due to the similarity of biomolecules between human and bacterial cells, metal NPs would be similarly cytotoxic to human cells. Interestingly, in a meta-analysis and statistical study of 71 published papers discussing the cytotoxicity of biogenic metal NPs on normal versus cancerous cells, it was found that biogenic metal NPs are 9 times more cytotoxic to human cancer cells than to normal cells (Barabadi et al., 2018). This huge difference was suggested to be a result of the high metabolic activity of cancer cells, which leads to the uptake of higher number of metal NPs compared to normal cells (Rai et al., 2016; Barabadi et al., 2018).
Examples: Biogenic Pd NPs display antimicrobial and anticancer activities. Some have a narrow range of activity, such as in the cases of Pd NPs produced by Solanum nigrum leaf extract (Vijilvani et al., 2020) and by Cissus quadrangularis stem extract (Anjana et al., 2019) exhibit antibacterial activity against Escherichia coli. Other biogenic Pd NPs were tested against a wider range of bacteria, and it was found that Pd NPs produced by Filicium decipiens leaf extract displayed antibacterial activity against both gram-positive (Bacillus subtilis and Staphylococcus aureus) and gram-negative (Escherichia coli and Pseudomonas aeruginosa) bacteria. The inhibition zone of gram-negative bacteria was larger than of gram-positive bacteria (twofold), which may suggest that Pd NPs can penetrate the thin outer membranes of gram-negative bacteria more easily than the thicker cell walls of gram-positive bacteria (Sharmila et al., 2017b). However, Pd NPs produced by Sapium sebiferum leaf extract demonstrated higher antibacterial activity against gram-positive than gram-negative bacteria (Tahir et al., 2016a). This suggests that different biogenic Pd NPs have different antibacterial mechanisms of action attributed to their different sizes, shapes, and their capping compounds.
The antimicrobial activities of Pd NPs are not limited to bacteria. For example, Pd NPs produced by Piper betle leaf extract exhibited antifungal activity against Aspergillus niger (Mallikarjuna et al., 2013), while Pd NPs produced by Melia azedarach leaf extract showed larvicidal activity against Aedes aegypti (Bhakyaraj et al., 2017). Moreover, Pd NPs produced by Cocos nucifera coir extract displayed anti-feedent and ovicidal activity against Callasobruchus maculates agricultural pest (Elango et al., 2017). At the same time, other biogenic Pd NPs exhibited anticancer activity, as those produced by Evolvulus alsinoides leaf extract, which were efficient against A2780 ovarian cancer cells (Gurunathan et al., 2015), and the NPs produced by Urtica, which were efficient against MDA-MB-231 human breast cancer cells, HT-29 human colon cancer cells, and MIA PaCa-2 human pancreatic cancer cells (Gulbagca et al., 2021).
Some biogenic Pd NPs displayed a wide range of activities. For instance, Pd NPs produced by Bauhinia variegate bark extract exhibited antibacterial activity against Bacillus subtilis and Stayphylococcus aureus, antifungal activity against Candida albicans, and anticancer activity against MCF-7 breast cancer cells (Vaghela et al., 2018). Pd NPs produced by Moringa oleifer flower extract demonstrated antibacterial activity against Enterococcus faecalis (and not against Bacillus cereus, Staphylococcus aureus, or E. coli) and anticancer activity against A549 lung cancer cells (Anand et al., 2016). Interestingly, Pd NPs produced by Rosmarinus officinalis leaf extract exhibited a wider range of activities. They displayed antibacterial and antifungal activities against all tested species. Pd NPs produced by Camellia sinensis leaf extract exhibited antioxidant activity, antibacterial activity against Escherichia coli and Staphylococcus epidermidis, and anticancer activity against MOLT-4 T-lymphoblastic leukemia cells (Azizi et al., 2017). Additionally to that, Pd NPs produced by Dioscorea bulbifera tuber extract showed antioxidant activity and anticancer activity against HeLa cervical cancer cells (Ghosh et al., 2015). In the same study, the researchers produced bimetallic PtPd NPs using the same plant extract. Remarkably, the bimetallic NPs exhibited higher antioxidant and anticancer activities compared to the monometallic NPs (Ghosh et al., 2015). In the majority of these studies, a general mechanism of action was suggested for the biogenic Pd NPs. However, these studies lack a detailed evaluation of the role of the surface molecules capping the biogenic NPs on both the cellular uptake of these NPs and on their mechanism of promoting toxicity.
In addition to having a wide range of antimicrobial and anticancer properties, Pd NPs have also shown promising results in several biomedical therapy approaches. Due to the diversity in their size and shape, photothermal conversion efficiency, and photostability, Pd NPs are emerging as potentially efficient photothermal therapy agents (Phan et al., 2020). NP-mediated photothermal therapy (cancer cell death by heat generated in tumor tissue) has shown great potential for the treatment of cancer. However, the development of small-size biocompatible photothermal therapy agents with high photothermal conversion efficiency is a big challenge (Xiao et al., 2014). Porous 20 nm Pd NPs have displayed photothermal conversion efficiency of 93.4% when dispersed in water and illuminated with an 808 nm laser. Using these NPs for the in vitro photothermal heating of HeLa cells led to 100% cell death when using 808 nm laser irradiation and 70% cell death when using 730 nm laser after 4 min of irradiation (Xiao et al., 2014). Theoretically, other nanomaterials exhibit higher photothermal conversion efficiencies, such as Au rods, which exhibit a 98.6% photothermal conversion efficiency. However, the large size of Au rods is a huge disadvantage and results in lowering the theoretical 98.6% photothermal conversion efficiency to 85.6% when irradiated with a 730 nm laser. Despite having a higher theoretical photothermal conversion efficiency, the large size of Au rods (60.8 nm on average) compared to 20 nm porous Pd NPs resulted in showing lower experimental photothermal conversion efficiency due to the higher fraction of scattering in the extinction (Xiao et al., 2014).
In another study, porous 22 nm Pd NPs were coated with chitosan oligosaccharide to improve their biocompatibility and further functionalized with RGD peptide (Arginylglycylaspartic acid) to increase their accumulation in MDA-MB-231 breast cancer cells. In an animal model, they have shown very promising results in the in vivo photothermal therapy of breast cancer. The researchers injected these Pd NPs intravenously in BALB/c nude mice bearing MDA-MB-231 xenograft tumors, irradiated the tumor region with 808 nm laser for 5 min, and measured the tumour progression rate for 20 days. Impressively, on the 20th day, the thermally destructed tumor was reduced and healed completely (Bharathiraja et al., 2018). Interestingly, besides their photothermal conversion efficiency, Pd NPs also produce large signals in photoacoustic imaging, which is an emerging new non-invasive biomedical technique that combines ultrasound-based signals with optical contrast to image treated cancer tissues. In (Basavegowda et al., 2016), the researchers injected the coated and functionalized Pd NPs intravenously in the animal model and diagnosed the tumor region using photoacoustic imaging. After accumulating in the tumor region, the injected NPs acted as an excellent contrast agent and generated a clear image of the tumor tissue.
Pd NPs could also play an important role in improving current cancer treatment technologies such as chemo- and radiotherapy. Song et al. (2018) have demonstrated that porous hollow Pd NPs can deliver 131I (a radioisotope that is commonly used in radiotherapy) and DOX (a chemotherapy drug that is commonly used in chemotherapy) in vivo in mice injected with MCF-7 breast cancer cells. Compared to other mesoporous nanocarriers, these NPs exhibited high drug loading and controllable drug release promoted by laser irradiation and acidic pH in tumor microenvironments. Moreover, Pd NPs have also shown promising results in other biomedical areas such as gene therapy (Kang et al., 2018), drug delivery (Gil et al., 2018), prodrug activation (Weiss et al., 2014), and biosensors applications (Yi et al., 2017).
The continuous growth of Pd NP applications have made it imperative to exploit novel methodologies for the efficient and environmentally friendly synthesis of Pd NPs. Current chemical and physical production methodologies can synthesize particles with narrow size distributions and specific shapes, which are highly related to their remarkable catalytic properties. The downside of some of those approaches is the high cost, high energy consumption, and the involvement of toxic chemicals. Biological synthesis of NPs using a broad range of species and biomolecules has emerged as a powerful alternative to the classic methodologies. These processes can potentially result in more environmentally friendly approach and might become a key part of a cyclic Pd economy when included in recycling and wastewater treatment processes.
Today, the available knowledge on the mechanisms of Pd NPs formation from different biological sources is insufficient, and more dedicated research is necessary to exert even better control over important parameters such as NP size and shape. The vast number and diversity of possible application of Pd NPs in catalysis, medicine, and biomedical therapies highlights the importance of such studies, and risks related to the toxicity of nanoparticles should not be ignored in the course of this research. As for many other new nanomaterials, we will probably see even more new applications for this exciting material in the future.
NJ wrote the first draft on the manuscript and produced all figures. All authors edited and approved the manuscript.
This work was supported by the Research Council of Norway, grant 294605 (Center for Digital Life) to DL and PM.
The authors would like to thank Ana Lucía Campaña Perilla for helpful discussions.
Author GK was employed by the company Inven2 AS.
The remaining authors declare that the research was conducted in the absence of any commercial or financial relationships that could be construed as a potential conflict of interest.
All claims expressed in this article are solely those of the authors and do not necessarily represent those of their affiliated organizations, or those of the publisher, the editors and the reviewers. Any product that may be evaluated in this article, or claim that may be made by its manufacturer, is not guaranteed or endorsed by the publisher.
Adams, B. D., and Chen, A. (2011). The role of palladium in a hydrogen economy. Mat. TodayKidlingt. 14 (6), 282–289. doi:10.1016/s1369-7021(11)70143-2
Ahsan, A., Farooq, M. A., Ahsan Bajwa, A., and Parveen, A. (2020). Green synthesis of silver nanoparticles using Parthenium hysterophorus: Optimization, characterization and in vitro therapeutic evaluation. Molecules 25 (15), 3324. doi:10.3390/molecules25153324
Al-Fakeh, M., Osman, S. O. M., Gassoumi, M., Rabhi, M., and Omer, M. (2021). Characterization, antimicrobial and anticancer properties of palladium nanoparticles biosynthesized optimally using Saudi propolis. Nanomater. (Basel). 11 (10), 2666. doi:10.3390/nano11102666
Alexeeva, O. K., and Fateev, V. N. (2016). Application of the magnetron sputtering for nanostructured electrocatalysts synthesis. Int. J. Hydrogen Energy 41 (5), 3373–3386. doi:10.1016/j.ijhydene.2015.12.147
Ali, H., Khan, E., and Sajad, M. A. (2013). Phytoremediation of heavy metals—Concepts and applications. Chemosphere 91 (7), 869–881. doi:10.1016/j.chemosphere.2013.01.075
Ameri, A., Shakibaie, M., Rahimi, H-R., Adeli-Sardou, M., Raeisi, M., Najafi, A., et al. (2020). Rapid and facile microwave-assisted synthesis of palladium nanoparticles and evaluation of their antioxidant properties and cytotoxic effects against fibroblast-like (HSkMC) and human lung carcinoma (A549) cell lines. Biol. Trace Elem. Res. 197 (1), 132–140. doi:10.1007/s12011-019-01984-0
Amornkitbamrung, L., Pienpinijtham, P., Thammacharoen, C., and Ekgasit, S. (2014). Palladium nanoparticles synthesized by reducing species generated during a successive acidic/alkaline treatment of sucrose. Spectrochimica Acta Part A Mol. Biomol. Spectrosc. 122, 186–192. doi:10.1016/j.saa.2013.10.095
Anand, K., Tiloke, C., Phulukdaree, A., Ranjan, B., Chuturgoon, A., Singh, S., et al. (2016). Biosynthesis of palladium nanoparticles by using Moringa oleifera flower extract and their catalytic and biological properties. J. Photochem. Photobiol. B Biol. 165, 87–95. doi:10.1016/j.jphotobiol.2016.09.039
Anjana, P. M., Bindhu, M. R., Umadevi, M., and Rakhi, R. B. (2019). Antibacterial and electrochemical activities of silver, gold, and palladium nanoparticles dispersed amorphous carbon composites. Appl. Surf. Sci. 479, 96–104. doi:10.1016/j.apsusc.2019.02.057
Anju, A., Gupta, K., and Chundawat, T. S. (2020). In vitro antimicrobial and antioxidant activity of biogenically synthesized palladium and platinum nanoparticles using Botryococcus braunii. Turk. J. Pharm. Sci. 17 (3), 299–306. doi:10.4274/tjps.galenos.2019.94103
Anjum, F., Gul, S., Khan, M. I., and Khan, M. A. (2020). Efficient synthesis of palladium nanoparticles using guar gum as stabilizer and their applications as catalyst in reduction reactions and degradation of azo dyes. Green Process. Synthesis 9 (1), 63–76. doi:10.1515/gps-2020-0008
Antolini, E. (2009). Palladium in fuel cell catalysis. Energy Environ. Sci. 2 (9), 915–931. doi:10.1039/b820837a
Arsiya, F., Sayadi, M. H., and Sobhani, S. (2017). Green synthesis of palladium nanoparticles using Chlorella vulgaris. Mat. Lett. 186, 113–115. doi:10.1016/j.matlet.2016.09.101
Atarod, M., Nasrollahzadeh, M., and Sajadi, S. M. (2016). Green synthesis of Pd/RGO/Fe3O4 nanocomposite using Withania coagulans leaf extract and its application as magnetically separable and reusable catalyst for the reduction of 4-nitrophenol. J. Colloid Interface Sci. 465, 249–258. doi:10.1016/j.jcis.2015.11.060
Attar, A., and Altikatoglu Yapaoz, M. (2018). Biosynthesis of palladium nanoparticles using Diospyros kaki leaf extract and determination of antibacterial efficacy. Prep. Biochem. Biotechnol. 48 (7), 629–634. doi:10.1080/10826068.2018.1479862
Azizi, S., Shahri, M. M., Rahman, H. S., Rahim, R. A., Rasedee, A., and Mohamad, R. (2017). Green synthesis palladium nanoparticles mediated by white tea (Camellia sinensis) extract with antioxidant, antibacterial, and antiproliferative activities toward the human leukemia (MOLT-4) cell line. Int. J. Nanomedicine 12, 8841–8853. doi:10.2147/IJN.S149371
Baba, T., Ara, T., Hasegawa, M., Takai, Y., Okumura, Y., Baba, M., et al. (2006). Construction of Escherichia coli K-12 in-frame, single-gene knockout mutants: The Keio collection. Mol. Syst. Biol. 2 (1), 0008. doi:10.1038/msb4100050
Bachar, O., Meirovich, M. M., Kurzion, R., and Yehezkeli, O. (2020). In vivo and in vitro protein mediated synthesis of palladium nanoparticles for hydrogenation reactions. Chem. Commun. 56 (76), 11211–11214. doi:10.1039/d0cc04812g
Bankar, A., Joshi, B., Kumar, A. R., and Zinjarde, S. (2010). Banana peel extract mediated novel route for the synthesis of palladium nanoparticles. Mat. Lett. 64 (18), 1951–1953. doi:10.1016/j.matlet.2010.06.021
Barabadi, H., Alizadeh, A., Ovais, M., Ahmadi, A., Shinwari, Z. K., and Saravanan, M. (2018). Efficacy of green nanoparticles against cancerous and normal cell lines: A systematic review and meta-analysis. IET Nanobiotechnol. 12 (4), 377–391. doi:10.1049/iet-nbt.2017.0120
Baruah, D., Das, R. N., Hazarika, S., and Konwar, D. (2015). Biogenic synthesis of cellulose supported Pd (0) nanoparticles using hearth wood extract of artocarpus lakoocha roxb—a green, efficient and versatile catalyst for suzuki and Heck coupling in water under microwave heating. Catal. Commun. 72, 73–80. doi:10.1016/j.catcom.2015.09.011
Baruwati, B., and Varma, R. S. (2009). High value products from waste: Grape pomace Extractâ”A three-in-one package for the synthesis of metal nanoparticles. ChemSusChem 2 (11), 1041–1044. doi:10.1002/cssc.200900220
Basavegowda, N., Mishra, K., Lee, Y. R., and Kim, S. H. (2016). Antioxidant and anti-tyrosinase activities of palladium nanoparticles synthesized using Saururus chinensis. J. Clust. Sci. 27 (2), 733–744. doi:10.1007/s10876-016-0984-0
Bathula, C., Subalakshmi, K., Kumar, A., Yadav, H., Ramesh, S., Shinde, S., et al. (2020). Ultrasonically driven green synthesis of palladium nanoparticles by Coleus amboinicus for catalytic reduction and Suzuki-Miyaura reaction. Colloids Surf. B Biointerfaces 192, 111026. doi:10.1016/j.colsurfb.2020.111026
Bendre, A. D., Patil, V. P., Terdale, S. S., Kodam, K. M., and Waghmode, S. B. (2020). A simple, efficient and green approach for the synthesis of palladium nanoparticles using Oxytocin: Application for ligand free Suzuki reaction and total synthesis of aspongpyrazine A. J. Organomet. Chem. 909, 121093. doi:10.1016/j.jorganchem.2019.121093
Bennett, J. A., Mikheenko, I. P., Deplanche, K., Shannon, I. J., Wood, J., and Macaskie, L. E. (2013). Nanoparticles of palladium supported on bacterial biomass: New re-usable heterogeneous catalyst with comparable activity to homogeneous colloidal Pd in the Heck reaction. Appl. Catal. B Environ. 140, 700–707. doi:10.1016/j.apcatb.2013.04.022
Bhakyaraj, K., Kumaraguru, S., Gopinath, K., Sabitha, V., Kaleeswarran, P. R., Karthika, V., et al. (2017). Eco-friendly synthesis of palladium nanoparticles using Melia azedarach leaf extract and their evaluation for antimicrobial and larvicidal activities. J. Clust. Sci. 28 (1), 463–476. doi:10.1007/s10876-016-1114-8
Bharathiraja, S., Bui, N. Q., Manivasagan, P., Moorthy, M. S., Mondal, S., Seo, H., et al. (2018). Multimodal tumor-homing chitosan oligosaccharide-coated biocompatible palladium nanoparticles for photo-based imaging and therapy. Sci. Rep. 8 (1), 500–516. doi:10.1038/s41598-017-18966-8
Biffis, A., Centomo, P., Del Zotto, A., and Zecca, M. (2018). Pd metal catalysts for cross-couplings and related reactions in the 21st century: A critical review. Chem. Rev. 118 (4), 2249–2295. doi:10.1021/acs.chemrev.7b00443
Brayner, R., Barberousse, H., Hemadi, M., Djedjat, C., Yéprémian, C., Coradin, T., et al. (2007). Cyanobacteria as bioreactors for the synthesis of Au, Ag, Pd, and Pt nanoparticles via an enzyme-mediated route. J. Nanosci. Nanotechnol. 7 (8), 2696–2708. doi:10.1166/jnn.2007.600
Bunge, M., Søbjerg, L. S., Rotaru, A., Gauthier, D., Lindhardt, A. T., Hause, G., et al. (2010). Formation of palladium (0) nanoparticles at microbial surfaces. Biotechnol. Bioeng. 107 (2), 206–215. doi:10.1002/bit.22801
Byrappa, K., Ohara, S., and Adschiri, T. (2008). Nanoparticles synthesis using supercritical fluid technology–towards biomedical applications. Adv. Drug Deliv. Rev. 60 (3), 299–327. doi:10.1016/j.addr.2007.09.001
Cagran, C., and Pottlacher, G. (2006). Thermophysical properties of palladium. platin. Met. Rev. 50 (3), 144–149. doi:10.1595/147106706x129079
Cansell, F., and Aymonier, C. (2009). Design of functional nanostructured materials using supercritical fluids. J. Supercrit. Fluids 47 (3), 508–516. doi:10.1016/j.supflu.2008.10.002
Chen, A., and Ostrom, C. (2015). Palladium-based nanomaterials: Synthesis and electrochemical applications. Chem. Rev. 115 (21), 11999–12044. doi:10.1021/acs.chemrev.5b00324
Chen, J., Wei, D., Liu, L., Nai, J., Liu, Y., Xiong, Y., et al. (2021). Green synthesis of Konjac glucomannan templated palladium nanoparticles for catalytic reduction of azo compounds and hexavalent chromium. Mat. Chem. Phys. 267, 124651. doi:10.1016/j.matchemphys.2021.124651
Chen, Y., Chen, Y., Wu, J., and Zhang, J. (2018). The effect of biotic and abiotic environmental factors on Pd (II) adsorption and reduction by Bacillus wiedmannii MSM. Ecotoxicol. Environ. Saf. 162, 546–553. doi:10.1016/j.ecoenv.2018.07.043
Chen, Y., Hu, K., and Chen, Y. (2019). The effect of biotic and abiotic environmental factors on Pd (II) adsorption and reduction by Bacillus megaterium Y-4. Chemosphere 220, 1058–1066. doi:10.1016/j.chemosphere.2019.01.011
Chichkov, B. N., Momma, C., Nolte, S., Von Alvensleben, F., and Tünnermann, A. (1996). Femtosecond, picosecond and nanosecond laser ablation of solids. Appl. Phys. A Mat. Sci. Process. 63 (2), 109–115. doi:10.1007/bf01567637
Chidambaram, D., Hennebel, T., Taghavi, S., Mast, J., Boon, N., Verstraete, W., et al. (2010). Concomitant microbial generation of palladium nanoparticles and hydrogen to immobilize chromate. Environ. Sci. Technol. 44 (19), 7635–7640. doi:10.1021/es101559r
Coccia, F., Tonucci, L., Bosco, D., Bressan, M., and d’Alessandro, N. (2012). One-pot synthesis of lignin-stabilised platinum and palladium nanoparticles and their catalytic behaviour in oxidation and reduction reactions. Green Chem. 14 (4), 1073–1078. doi:10.1039/c2gc16524d
Creamer, N. J., Mikheenko, I. P., Yong, P., Deplanche, K., Sanyahumbi, D., Wood, J., et al. (2007). Novel supported Pd hydrogenation bionanocatalyst for hybrid homogeneous/heterogeneous catalysis. Catal. Today 128 (1–2), 80–87. doi:10.1016/j.cattod.2007.04.014
Cristoforetti, G., Pitzalis, E., Spiniello, R., Ishak, R., and Muniz-Miranda, M. (2011). Production of palladium nanoparticles by pulsed laser ablation in water and their characterization. J. Phys. Chem. C 115 (12), 5073–5083. doi:10.1021/jp109281q
Cui, Z., Bai, X., Liu, Y., and Li, S. (2017). Synthesis of palladium concave Nanocubes with high-index facets and their catalytic properties. Appl. Organomet. Chem. 31 (12), e3887. doi:10.1002/aoc.3887
Dauthal, P., and Mukhopadhyay, M. (2013). Biosynthesis of palladium nanoparticles using Delonix regia leaf extract and its catalytic activity for nitro-aromatics hydrogenation. Ind. Eng. Chem. Res. 52 (51), 18131–18139. doi:10.1021/ie403410z
De Windt, W., Aelterman, P., and Verstraete, W. (2005). Bioreductive deposition of palladium (0) nanoparticles on Shewanella oneidensis with catalytic activity towards reductive dechlorination of polychlorinated biphenyls. Environ. Microbiol. 7 (3), 314–325. doi:10.1111/j.1462-2920.2005.00696.x
Deplanche, K., Caldelari, I., Mikheenko, I. P., Sargent, F., and Macaskie, L. E. (2010). Involvement of hydrogenases in the formation of highly catalytic Pd(0) nanoparticles by bioreduction of Pd(II) using Escherichia coli mutant strains. Microbiology 156 (9), 2630–2640. doi:10.1099/mic.0.036681-0
Deplanche, K., Merroun, M. L., Casadesus, M., Tran, D. T., Mikheenko, I. P., Bennett, J. A., et al. (2012). Microbial synthesis of core/shell gold/palladium nanoparticles for applications in green chemistry. J. R. Soc. Interface 9 (72), 1705–1712. doi:10.1098/rsif.2012.0003
Devi, D. K., Pratap, S. V., Haritha, R., Sivudu, K. S., Radhika, P., and Sreedhar, B. (2011). Gum acacia as a facile reducing, stabilizing, and templating agent for palladium nanoparticles. J. Appl. Polym. Sci. 121 (3), 1765–1773. doi:10.1002/app.33004
Diculescu, V. C., Chiorcea-Paquim, A-M., Corduneanu, O., and Oliveira-Brett, A. M. (2007). Palladium nanoparticles and nanowires deposited electrochemically: AFM and electrochemical characterization. J. Solid State Electrochem. 11 (7), 887–898. doi:10.1007/s10008-007-0275-7
Dinca, V., and Suchea, M. (2019). Functional nanostructured interfaces for environmental and biomedical applications. Elsevier.
Dinesh, M., Roopan, S. M., Selvaraj, C. I., and Arunachalam, P. (2017). Phyllanthus emblica seed extract mediated synthesis of PdNPs against antibacterial, heamolytic and cytotoxic studies. J. Photochem. Photobiol. B Biol. 167, 64–71. doi:10.1016/j.jphotobiol.2016.12.012
Duan, L., Li, M., and Liu, H. (2015). Biosynthesised palladium nanoparticles using Eucommia ulmoides bark aqueous extract and their catalytic activity. IET Nanobiotechnol. 9 (6), 349–354. doi:10.1049/iet-nbt.2015.0020
Edayadulla, N., Basavegowda, N., and Lee, Y. R. (2015). Green synthesis and characterization of palladium nanoparticles and their catalytic performance for the efficient synthesis of biologically interesting di (indolyl) indolin-2-ones. J. Ind. Eng. Chem. 21, 1365–1372. doi:10.1016/j.jiec.2014.06.007
Elango, G., Mohana Roopan, S., Abdullah Al-Dhabi, N., Arasu, M. V., Irukatla Damodharan, K., and Elumalai, K. (2017). Cocos nucifera coir-mediated green synthesis of Pd NPs and its investigation against larvae and agricultural pest. Artif. Cells Nanomed. Biotechnol. 45 (8), 1581–1587. doi:10.1080/21691401.2016.1262382
Fahmy, S. A., Fawzy, I. M., Saleh, B. M., Issa, M. Y., Bakowsky, U., and Azzazy, H. M. E-S. (2021). Green synthesis of platinum and palladium nanoparticles using Peganum harmala L. Seed alkaloids: Biological and computational studies. Nanomaterials 11 (4), 965. doi:10.3390/nano11040965
Fahmy, S. A., Preis, E., Bakowsky, U., and Azzazy, H. M. E-S. (2020). Palladium nanoparticles fabricated by green chemistry: Promising chemotherapeutic, antioxidant and antimicrobial agents. Mater. (Basel) 13 (17), 3661. doi:10.3390/ma13173661
Faraji, G., Kim, H. S., and Kashi, H. T. (2018). Severe plastic deformation: Methods, processing and properties. Elsevier.
Farhadi, K., Pourhossein, A., Forough, M., Molaei, R., Abdi, A., and Siyami, A. (2013). Biosynthesis of highly dispersed palladium nanoparticles using Astraglmanna aqueous extract. J. Chin. Chem. Soc. 60 (9), 1144–1149. doi:10.1002/jccs.201300006
Favier, I., Pla, D., and Gómez, M. (2019). Palladium nanoparticles in polyols: Synthesis, catalytic couplings, and hydrogenations. Chem. Rev. 120 (2), 1146–1183. doi:10.1021/acs.chemrev.9b00204
Feng, J-X., Zhang, Q-L., Wang, A-J., Wei, J., Chen, J-R., and Feng, J-J. (2014). Caffeine-assisted facile synthesis of platinum@ palladium core-shell nanoparticles supported on reduced graphene oxide with enhanced electrocatalytic activity for methanol oxidation. Electrochim. Acta 142, 343–350. doi:10.1016/j.electacta.2014.07.152
Ganaie, S. U., Abbasi, T., and Abbasi, S. A. (2016). Low-cost, environment-friendly synthesis of palladium nanoparticles by utilizing a terrestrial weed Antigonon leptopus. Part. Sci. Technol. 34 (2), 201–208. doi:10.1080/02726351.2015.1058874
Gedanken, A. (2004). Using sonochemistry for the fabrication of nanomaterials. Ultrason. Sonochem. 11 (2), 47–55. doi:10.1016/j.ultsonch.2004.01.037
Geological Survey, U. S. (2020). Mineral commodity summaries 2020. Editor D. Bernhardt, and J. F. Reilly (Reston,USA: Miner Commod Summ Sch. doi:10.3133/mcs2020.ISBN978-1-4113-4362-7
Ghosh, S., Nitnavare, R., Dewle, A., Tomar, G. B., Chippalkatti, R., More, P., et al. (2015). Novel platinum–palladium bimetallic nanoparticles synthesized by Dioscorea bulbifera: Anticancer and antioxidant activities. Int. J. Nanomedicine 10, 7477–7490. doi:10.2147/IJN.S91579
Gil, Y-G., Kang, S., Chae, A., Kim, Y-K., Min, D-H., and Jang, H. (2018). Synthesis of porous Pd nanoparticles by therapeutic chaga extract for highly efficient tri-modal cancer treatment. Nanoscale 10 (42), 19810–19817. doi:10.1039/c8nr07172a
Gioria, E., Signorini, C., Wisniewski, F., and Gutierrez, L. (2020). Green synthesis of time-stable palladium nanoparticles using microfluidic devices. J. Environ. Chem. Eng. 8 (5), 104096. doi:10.1016/j.jece.2020.104096
Gnanasekar, S., Murugaraj, J., Dhivyabharathi, B., Krishnamoorthy, V., Jha, P. K., Seetharaman, P., et al. (2018). Antibacterial and cytotoxicity effects of biogenic palladium nanoparticles synthesized using fruit extract of Couroupita guianensis Aubl. J. Appl. Biomed. 16 (1), 59–65. doi:10.1016/j.jab.2017.10.001
Gopalakrishnan, R., Loganathan, B., Dinesh, S., and Raghu, K. (2017). Strategic green synthesis, characterization and catalytic application to 4-nitrophenol reduction of palladium nanoparticles. J. Clust. Sci. 28 (4), 2123–2131. doi:10.1007/s10876-017-1207-z
Gulbagca, F., Aygün, A., Gülcan, M., Ozdemir, S., Gonca, S., and Şen, F. (2021). Green synthesis of palladium nanoparticles: Preparation, characterization, and investigation of antioxidant, antimicrobial, anticancer, and DNA cleavage activities. Appl. Organomet. Chem. 35 (8), e6272. doi:10.1002/aoc.6272
Gurunathan, S., Kim, E., Han, J. W., Park, J. H., and Kim, J-H. (2015). Green chemistry approach for synthesis of effective anticancer palladium nanoparticles. Molecules 20 (12), 22476–22498. doi:10.3390/molecules201219860
Han, Z., Dong, L., Zhang, J., Cui, T., Chen, S., Ma, G., et al. (2019). Green synthesis of palladium nanoparticles using lentinan for catalytic activity and biological applications. RSC Adv. 9 (65), 38265–38270. doi:10.1039/c9ra08051a
Hatamifard, A., Nasrollahzadeh, M., and Lipkowski, J. (2015). Green synthesis of a natrolite zeolite/palladium nanocomposite and its application as a reusable catalyst for the reduction of organic dyes in a very short time. RSC Adv. 5 (111), 91372–91381. doi:10.1039/c5ra18476b
Heck, R. F., and Nolley, J. P. (1972). Palladium-catalyzed vinylic hydrogen substitution reactions with aryl, benzyl, and styryl halides. J. Org. Chem. 37 (14), 2320–2322. doi:10.1021/jo00979a024
Hekmati, M., Bonyasi, F., Javaheri, H., and Hemmati, S. (2017). Green synthesis of palladium nanoparticles using Hibiscus sabdariffa L. flower extract: Heterogeneous and reusable nanocatalyst in Suzuki coupling reactions. Appl. Organomet. Chem. 31 (11), e3757. doi:10.1002/aoc.3757
Hennebel, T., Van Nevel, S., Verschuere, S., De Corte, S., De Gusseme, B., Cuvelier, C., et al. (2011). Palladium nanoparticles produced by fermentatively cultivated bacteria as catalyst for diatrizoate removal with biogenic hydrogen. Appl. Microbiol. Biotechnol. 91 (5), 1435–1445. doi:10.1007/s00253-011-3329-9
Hong, Y., Jing, X., Huang, J., Sun, D., Odoom-Wubah, T., Yang, F., et al. (2014). Biosynthesized bimetallic Au–Pd nanoparticles supported on TiO2 for solvent-free oxidation of benzyl alcohol. ACS Sustain. Chem. Eng. 2 (7), 1752–1759. doi:10.1021/sc500181z
Hu, Y., Tao, K., Wu, C., Zhou, C., Yin, H., and Zhou, S. (2013). Size-controlled synthesis of highly stable and active Pd@ SiO2 core–shell nanocatalysts for hydrogenation of nitrobenzene. J. Phys. Chem. C 117 (17), 8974–8982. doi:10.1021/jp3110375
Huang, X., Wu, H., Pu, S., Zhang, W., Liao, X., and Shi, B. (2011). One-step room-temperature synthesis of Au@ Pd core–shell nanoparticles with tunable structure using plant tannin as reductant and stabilizer. Green Chem. 13 (4), 950–957. doi:10.1039/c0gc00724b
Ismail, E., Khenfouch, M., Dhlamini, M., Dube, S., and Maaza, M. (2017). Green palladium and palladium oxide nanoparticles synthesized via Aspalathus linearis natural extract. J. Alloys Compd. 695, 3632–3638. doi:10.1016/j.jallcom.2016.11.390
Jbara, M., Maity, S. K., and Brik, A. (2017). Palladium in the chemical synthesis and modification of proteins. Angew. Chem. Int. Ed. 56 (36), 10644–10655. doi:10.1002/anie.201702370
Jia, L., Zhang, Q., Li, Q., and Song, H. (2009). The biosynthesis of palladium nanoparticles by antioxidants in Gardenia jasminoides Ellis: Long lifetime nanocatalysts for p-nitrotoluene hydrogenation. Nanotechnology 20 (38), 385601. doi:10.1088/0957-4484/20/38/385601
Jiang, R., Zhang, Y., Swier, S., Wei, X., Erkey, C., Kunz, H. R., et al. (2005). Preparation via supercritical fluid route of Pd-impregnated nafion membranes which exhibit reduced methanol crossover for DMFC. Electrochem. Solid-State Lett. 8 (11), A611. doi:10.1149/1.2050527
Kalaiselvi, A., Roopan, S. M., Madhumitha, G., Ramalingam, C., and Elango, G. (2015). Synthesis and characterization of palladium nanoparticles using Catharanthus roseus leaf extract and its application in the photo-catalytic degradation. Spectrochimica Acta Part A Mol. Biomol. Spectrosc. 135, 116–119. doi:10.1016/j.saa.2014.07.010
Kalpana, V. N., and Rajeswari, V. D. (2018). Synthesis of palladium nanoparticles via a green route using lagenaria siceraria: Assessment of their innate antidandruff, insecticidal and degradation activities. Mat. Res. Express 5 (11), 115406. doi:10.1088/2053-1591/aaddef
Kameo, A., Yoshimura, T., and Esumi, K. (2003). Preparation of noble metal nanoparticles in supercritical carbon dioxide. Colloids Surfaces A Physicochem. Eng. Aspects 215 (1–3), 181–189. doi:10.1016/s0927-7757(02)00439-9
Kanchana, A., Devarajan, S., and Ayyappan, S. R. (2010). Green synthesis and characterization of palladium nanoparticles and its conjugates from Solanum trilobatum leaf extract. Nano-micro Lett. 2 (3), 169–176. doi:10.1007/bf03353637
Kandathil, V., Dateer, R. B., Sasidhar, B. S., Patil, S. A., and Patil, S. A. (2018). Green synthesis of palladium nanoparticles: Applications in aryl halide cyanation and hiyama cross-coupling reaction under ligand free conditions. Catal. Lett. 148 (6), 1562–1578. doi:10.1007/s10562-018-2369-5
Kang, S., Shin, W., Kang, K., Choi, M-H., Kim, Y-J., Kim, Y-K., et al. (2018). Revisiting of Pd nanoparticles in cancer treatment: All-round excellence of porous Pd nanoplates in gene-thermo combinational therapy. ACS Appl. Mat. Interfaces 10 (16), 13819–13828. doi:10.1021/acsami.8b01000
Key trends in the Palladium market, annual report. 2020 (2022). Available at: https://ar2020.nornickel.com/commodity-market-overview/palladium#! [Accessed June 7, 2022].
Khan, M., Albalawi, G. H., Shaik, M. R., Khan, M., Adil, S. F., Kuniyil, M., et al. (2017). Miswak mediated green synthesized palladium nanoparticles as effective catalysts for the Suzuki coupling reactions in aqueous media. J. Saudi Chem. Soc. 21 (4), 450–457. doi:10.1016/j.jscs.2016.03.008
Khan, M., Khan, M., Kuniyil, M., Adil, S. F., Al-Warthan, A., Alkhathlan, H. Z., et al. (2014). Biogenic synthesis of palladium nanoparticles using Pulicaria glutinosa extract and their catalytic activity towards the Suzuki coupling reaction. Dalton Trans. 43 (24), 9026–9031. doi:10.1039/c3dt53554a
Kiani, M., Rabiee, N., Bagherzadeh, M., Ghadiri, A. M., Fatahi, Y., Dinarvand, R., et al. (2020). High-gravity-assisted green synthesis of palladium nanoparticles: The flowering of nanomedicine. Nanomedicine Nanotechnol. Biol. Med. 30, 102297. doi:10.1016/j.nano.2020.102297
Kimber, R. L., Parmeggiani, F., Neill, T. S., Merroun, M. L., Goodlet, G., Powell, N. A., et al. (2021). Biotechnological synthesis of Pd/Ag and Pd/Au nanoparticles for enhanced Suzuki–Miyaura cross-coupling activity. Microb. Biotechnol. 14 (6), 2435–2447. doi:10.1111/1751-7915.13762
Kora, A. J., and Rastogi, L. (2016). Catalytic degradation of anthropogenic dye pollutants using palladium nanoparticles synthesized by gum olibanum, a glucuronoarabinogalactan biopolymer. Ind. Crops Prod. 81, 1–10. doi:10.1016/j.indcrop.2015.11.055
Kora, A. J., and Rastogi, L. (2018). Green synthesis of palladium nanoparticles using gum ghatti (Anogeissus latifolia) and its application as an antioxidant and catalyst. Arab. J. Chem. 11 (7), 1097–1106. doi:10.1016/j.arabjc.2015.06.024
Kou, J., and Varma, R. S. (2012). Beet juice utilization: Expeditious green synthesis of noble metal nanoparticles (Ag, Au, Pt, and Pd) using microwaves. RSC Adv. 2 (27), 10283–10290. doi:10.1039/c2ra21908e
Kumar, B., Smita, K., Cumbal, L., and Debut, A. (2015). Ultrasound agitated phytofabrication of palladium nanoparticles using Andean blackberry leaf and its photocatalytic activity. J. Saudi Chem. Soc. 19 (5), 574–580. doi:10.1016/j.jscs.2015.05.008
Kumar, K. M., Mandal, B. K., Kumar, K. S., Reddy, P. S., and Sreedhar, B. (2013). Biobased green method to synthesise palladium and iron nanoparticles using Terminalia chebula aqueous extract. Spectrochimica Acta Part A Mol. Biomol. Spectrosc. 102, 128–133. doi:10.1016/j.saa.2012.10.015
Kumar Petla, R., Vivekanandhan, S., Misra, M., Kumar Mohanty, A., and Satyanarayana, N. (2012). Soybean (Glycine max) leaf extract based green synthesis of palladium nanoparticles. J. Biomater. Nanobiotechnol. 3 (01), 14–19. doi:10.4236/jbnb.2012.31003
KumaráBorah, R., JyotiáSaikia, H., KumaráDas, V., JyotiáThakur, A., and Bora, U. (2015). Biosynthesis of poly (ethylene glycol)-supported palladium nanoparticles using Colocasia esculenta leaf extract and their catalytic activity for Suzuki–Miyaura cross-coupling reactions. RSC Adv. 5 (89), 72453–72457. doi:10.1039/c5ra12657f
Kumari, M. M., Aromal, S. A., and Philip, D. (2013). Synthesis of monodispersed palladium nanoparticles using tannic acid and its optical non-linearity. Spectrochimica Acta Part A Mol. Biomol. Spectrosc. 103, 130–133. doi:10.1016/j.saa.2012.11.020
Kumari, T., Gopal, R., Goyal, A., and Joshi, J. (2019). Sol–gel synthesis of Pd@ PdO core–shell nanoparticles and effect of precursor chemistry on their structural and optical properties. J. Inorg. Organomet. Polym. Mat. 29 (2), 316–325. doi:10.1007/s10904-018-1001-x
Lakshmipathy, R., Palakshi Reddy, B., Sarada, N. C., Chidambaram, K., and Khadeer Pasha, S. K. (2015). Watermelon rind-mediated green synthesis of noble palladium nanoparticles: Catalytic application. Appl. Nanosci. 5 (2), 223–228. doi:10.1007/s13204-014-0309-2
Lebaschi, S., Hekmati, M., and Veisi, H. (2017). Green synthesis of palladium nanoparticles mediated by black tea leaves (Camellia sinensis) extract: Catalytic activity in the reduction of 4-nitrophenol and Suzuki-Miyaura coupling reaction under ligand-free conditions. J. Colloid Interface Sci. 485, 223–231. doi:10.1016/j.jcis.2016.09.027
Lengke, M. F., Fleet, M. E., and Southam, G. (2007). Synthesis of palladium nanoparticles by reaction of filamentous cyanobacterial biomass with a palladium (II) chloride complex. Langmuir 23 (17), 8982–8987. doi:10.1021/la7012446
Li, J., Yao, H., Wang, Y., and Luo, G. (2014). One-step preparation of Pd-SiO2 composite microspheres by the sol–gel process in a microchannel. Ind. Eng. Chem. Res. 53 (26), 10660–10666. doi:10.1021/ie5009458
Li, Y., Wang, H., Zhang, R., Zhang, G., Yang, Y., and Liu, Z. (2017). Biofabrication of polyphenols coated Nano palladium and its in-vitro cytotoxicity against human leukemia cell lines (K562). J. Photochem. Photobiol. B Biol. 175, 173–177. doi:10.1016/j.jphotobiol.2017.07.025
Liu, D., and Wu, F. (2017). Biosynthesis of Pd nanoparticle using onion extract for electrochemical determination of carbendazim. Int. J. Electrochem. Sci. 12, 2125–2134. doi:10.20964/2017.03.70
Liu, G., Bai, X., and Lv, H. (2017). Green synthesis of supported palladium nanoparticles employing pine needles as reducing agent and carrier: New reusable heterogeneous catalyst in the Suzuki coupling reaction. Appl. Organomet. Chem. 31 (4), e3587. doi:10.1002/aoc.3587
Lloyd, J. R., Yong, P., and Macaskie, L. E. (1998). Enzymatic recovery of elemental palladium by using sulfate-reducing bacteria. Appl. Environ. Microbiol. 64 (11), 4607–4609. doi:10.1128/aem.64.11.4607-4609.1998
Lu, F., Sun, D., Huang, J., Du, M., Yang, F., Chen, H., et al. (2014). Plant-mediated synthesis of Ag–Pd alloy nanoparticles and their application as catalyst toward selective hydrogenation. ACS Sustain. Chem. Eng. 2 (5), 1212–1218. doi:10.1021/sc500034r
Luo, F., Yang, D., Chen, Z., Megharaj, M., and Naidu, R. (2016). Characterization of bimetallic Fe/Pd nanoparticles by grape leaf aqueous extract and identification of active biomolecules involved in the synthesis. Sci. Total Environ. 562, 526–532. doi:10.1016/j.scitotenv.2016.04.060
Mallikarjuna, K., Sushma, N. J., Reddy, B. V. S., Narasimha, G., and Raju, B. D. P. (2013). Palladium nanoparticles: Single-step plant-mediated green chemical procedure using piper betle leaves broth and their anti-fungal studies. Int. J. Chem. Anal. Sci. 4 (1), 14–18. doi:10.1016/j.ijcas.2013.03.006
Mallikarjuns, K., Sushma, N. J., Narasimha, G., Rao, K. V., Manoj, L., and Raju, B. D. P. (2011). “Synthesis and spectroscopic characterization of palladium nanoparticles by using broth of edible mushroom extract,” in International conference on nanoscience, engineering and technology (ICONSET 2011) (IEEE), 612–615.
Manikandan, V., Velmurugan, P., Park, J-H., Lovanh, N., Seo, S-K., Jayanthi, P., et al. (2016). Synthesis and antimicrobial activity of palladium nanoparticles from Prunus× yedoensis leaf extract. Mat. Lett. 185, 335–338. doi:10.1016/j.matlet.2016.08.120
Manjare, S. B., Pendhari, P. D., Badade, S. M., and Thopate, S. R. (2021). Palladium nanoparticles: Plant aided biosynthesis, characterization, applications. Chem. Afr. 4 (4), 715–730. doi:10.1007/s42250-021-00284-2
Marzun, G., Nakamura, J., Zhang, X., Barcikowski, S., and Wagener, P. (2015). Size control and supporting of palladium nanoparticles made by laser ablation in saline solution as a facile route to heterogeneous catalysts. Appl. Surf. Sci. 348, 75–84. doi:10.1016/j.apsusc.2015.01.108
Matsena, M. T., and Chirwa, E. (2021). Comparative analysis of biological versus chemical synthesis of palladium nanoparticles for catalysis of chromium (VI) reduction. Sci. Rep. 11 (1), 16674–16715. doi:10.1038/s41598-021-96024-0
Matsumoto, T., Kamino, M., Yamada, R., Konishi, Y., and Ogino, H. (2020). Identification of genes responsible for reducing palladium ion in Escherichia coli. J. Biotechnol. 324, 7–10. doi:10.1016/j.jbiotec.2020.09.015
Mehl, S., Toghan, A., Bauer, T., Brummel, O., Taccardi, N., Wasserscheid, P., et al. (2015). Pd nanoparticle formation in ionic liquid thin films monitored by in situ vibrational spectroscopy. Langmuir 31 (44), 12126–12139. doi:10.1021/acs.langmuir.5b03386
Mendivil, M. I., Krishnan, B., Castillo, G. A., and Shaji, S. (2015). Synthesis and properties of palladium nanoparticles by pulsed laser ablation in liquid. Appl. Surf. Sci. 348, 45–53. doi:10.1016/j.apsusc.2015.03.075
Mikheenko, I. P., Rousset, M., Dementin, S., and Macaskie, L. E. (2008). Bioaccumulation of palladium by Desulfovibrio fructosivorans wild-type and hydrogenase-deficient strains. Appl. Environ. Microbiol. 74 (19), 6144–6146. doi:10.1128/aem.02538-07
Miller, M. A., Askevold, B., Mikula, H., Kohler, R. H., Pirovich, D., and Weissleder, R. (2017). Nano-palladium is a cellular catalyst for in vivo chemistry. Nat. Commun. 8 (1), 15906–15913. doi:10.1038/ncomms15906
Miyaura, N., Yamada, K., and Suzuki, A. (1979). A new stereospecific cross-coupling by the palladium-catalyzed reaction of 1-alkenylboranes with 1-alkenyl or 1-alkynyl halides. Tetrahedron Lett. 20 (36), 3437–3440. doi:10.1016/s0040-4039(01)95429-2
Mohana, S., and Sumathi, S. (2020). Multi-functional biological effects of palladium nanoparticles synthesized using Agaricus bisporus. J. Clust. Sci. 31 (2), 391–400. doi:10.1007/s10876-019-01652-2
Moisan, S., Marty, J-D., Cansell, F., and Aymonier, C. (2008). Preparation of functional hybrid palladium nanoparticles using supercritical fluids: A novel approach to detach the growth and functionalization steps. Chem. Commun. 2008 (12), 1428–1430. doi:10.1039/b718271f
Molaie, R., Farhadi, K., Forough, M., and Emamali, R. S. (2012). “Biological and green synthesis of palladium nanoparticles using aqueous extract of pistacia atlantica plant’s fruit: A facile biological approach,” in Proceedings of the 4th international conference on nanostructures (Kish, Iran: ICNS4), 12–14.
Momeni, S., and Nabipour, I. (2015). A simple green synthesis of palladium nanoparticles with Sargassum alga and their electrocatalytic activities towards hydrogen peroxide. Appl. Biochem. Biotechnol. 176 (7), 1937–1949. doi:10.1007/s12010-015-1690-3
Monopoli, A., Calo, V., Ciminale, F., Cotugno, P., Angelici, C., Cioffi, N., et al. (2010). Glucose as a clean and renewable reductant in the Pd-nanoparticle-catalyzed reductive homocoupling of bromo-and chloroarenes in water. J. Org. Chem. 75 (11), 3908–3911. doi:10.1021/jo1005729
Mughal, B., Zaidi, S. Z. J., Zhang, X., and Hassan, S. U. (2021). Biogenic nanoparticles: Synthesis, characterisation and applications. Appl. Sci. (Basel). 11 (6), 2598. doi:10.3390/app11062598
Murray, A. J., Zhu, J., Wood, J., and Macaskie, L. E. (2018). Biorefining of platinum group metals from model waste solutions into catalytically active bimetallic nanoparticles. Microb. Biotechnol. 11 (2), 359–368. doi:10.1111/1751-7915.13030
Musil, J., Vlcek, J., and Baroch, P. (2006). Magnetron discharges for thin films plasma processing. Mater Surf. Process by Dir. Energy Tech. 1, 67–110.
Nadagouda, M. N., and Varma, R. S. (2008). Green synthesis of silver and palladium nanoparticles at room temperature using coffee and tea extract. Green Chem. 10 (8), 859–862. doi:10.1039/b804703k
Naqvi, S., Agarwal, N. B., Singh, M. P., and Samim, M. (2021). Bio-engineered palladium nanoparticles: Model for risk assessment study of automotive particulate pollution on macrophage cell lines. RSC Adv. 11 (3), 1850–1861. doi:10.1039/d0ra09336j
Nasrollahzadeh, M. (2014). Green synthesis and catalytic properties of palladium nanoparticles for the direct reductive amination of aldehydes and hydrogenation of unsaturated ketones. New J. Chem. 38 (11), 5544–5550. doi:10.1039/c4nj01440e
Nasrollahzadeh, M., and Sajadi, S. M. (2016). Green synthesis, characterization and catalytic activity of the Pd/TiO2 nanoparticles for the ligand-free Suzuki–Miyaura coupling reaction. J. Colloid Interface Sci. 465, 121–127. doi:10.1016/j.jcis.2015.11.038
Nasrollahzadeh, M., Sajadi, S. M., Honarmand, E., and Maham, M. (2015). Preparation of palladium nanoparticles using Euphorbia thymifolia L. leaf extract and evaluation of catalytic activity in the ligand-free Stille and Hiyama cross-coupling reactions in water. New J. Chem. 39 (6), 4745–4752. doi:10.1039/c5nj00244c
Nasrollahzadeh, M., Sajadi, S. M., Maham, M., and Ehsani, A. (2015). Facile and surfactant-free synthesis of Pd nanoparticles by the extract of the fruits of Piper longum and their catalytic performance for the Sonogashira coupling reaction in water under ligand-and copper-free conditions. RSC Adv. 5 (4), 2562–2567. doi:10.1039/c4ra12875c
Nasrollahzadeh, M., Sajadi, S. M., and Maham, M. (2015). Green synthesis of palladium nanoparticles using Hippophae rhamnoides Linn leaf extract and their catalytic activity for the Suzuki–Miyaura coupling in water. J. Mol. Catal. A Chem. 396, 297–303. doi:10.1016/j.molcata.2014.10.019
Nasrollahzadeh, M., and Sajadi, S. M. (2016). Pd nanoparticles synthesized in situ with the use of Euphorbia granulate leaf extract: Catalytic properties of the resulting particles. J. Colloid Interface Sci. 462, 243–251. doi:10.1016/j.jcis.2015.09.065
Nasrollahzadeh, M., and Sajadi, S. M. (2016). Preparation of Pd/Fe3O4 nanoparticles by use of Euphorbia stracheyi Boiss root extract: A magnetically recoverable catalyst for one-pot reductive amination of aldehydes at room temperature. J. Colloid Interface Sci. 464, 147–152. doi:10.1016/j.jcis.2015.11.020
Nasrollahzadeh, M., Sajadi, S. M., Rostami-Vartooni, A., Alizadeh, M., and Bagherzadeh, M. (2016). Green synthesis of the Pd nanoparticles supported on reduced graphene oxide using barberry fruit extract and its application as a recyclable and heterogeneous catalyst for the reduction of nitroarenes. J. Colloid Interface Sci. 466, 360–368. doi:10.1016/j.jcis.2015.12.036
Nasrollahzadeh, M., Sajadi, S. M., Rostami-Vartooni, A., and Bagherzadeh, M. (2015). Green synthesis of Pd/CuO nanoparticles by Theobroma cacao L. seeds extract and their catalytic performance for the reduction of 4-nitrophenol and phosphine-free Heck coupling reaction under aerobic conditions. J. Colloid Interface Sci. 448, 106–113. doi:10.1016/j.jcis.2015.02.009
Nasrollahzadeh, M., Sajadi, S. M., Rostami-Vartooni, A., and Khalaj, M. (2015). Green synthesis of Pd/Fe3O4 nanoparticles using Euphorbia condylocarpa M. bieb root extract and their catalytic applications as magnetically recoverable and stable recyclable catalysts for the phosphine-free Sonogashira and Suzuki coupling reactions. J. Mol. Catal. A Chem. 396, 31–39. doi:10.1016/j.molcata.2014.09.029
Nasrollahzadeh, M., Sajadi, S. M., Rostami-Vartooni, A., and Khalaj, M. (2014). Journey on greener pathways: Use of Euphorbia condylocarpa M. Bieb as reductant and stabilizer for green synthesis of Au/Pd bimetallic nanoparticles as reusable catalysts in the suzuki and Heck coupling reactions in water. RSC Adv. 4 (82), 43477–43484. doi:10.1039/c4ra07173e
Naveen, B. S., and Padmesh, T. V. N. (2014). Seaweed (Sargassum ilicifolium) assisted green synthesis of palladium nanoparticles. Int. J. Sci. Eng. Res. 5, 229–231.
Nazeruddin, G. M., Prasad, S. R., Rathor, B. M., Kumbhar, D. R., Khandare, R. V., and Prasad, N. R. (2014). Extracellular one pot green synthesis of palladium nanoparticles. Int. J. Nanomater Nanostructures 1, 1.
Nemamcha, A., Rehspringer, J-L., and Khatmi, D. (2006). Synthesis of palladium nanoparticles by sonochemical reduction of palladium (II) nitrate in aqueous solution. J. Phys. Chem. B 110 (1), 383–387. doi:10.1021/jp0535801
The Nobel prize in chemistry (2010). Available at: https://www.nobelprize.org/prizes/chemistry/2010/summary/ [Accessed June 7, 2022].
Okitsu, K., Nagaoka, S., Tanabe, S., Matsumoto, H., Mizukoshi, Y., and Nagata, Y. (1999). Sonochemical preparation of size-controlled palladium nanoparticles on alumina surface. Chem. Lett. 28 (3), 271–272. doi:10.1246/cl.1999.271
Okitsu, K., Yue, A., Tanabe, S., and Matsumoto, H. (2000). Sonochemical preparation and catalytic behavior of highly dispersed palladium nanoparticles on alumina. Chem. Mat. 12 (10), 3006–3011. doi:10.1021/cm0001915
Olajire, A. A., and Mohammed, A. A. (2019). Green synthesis of palladium nanoparticles using Ananas comosus leaf extract for solid-phase photocatalytic degradation of low density polyethylene film. J. Environ. Chem. Eng. 7 (4), 103270. doi:10.1016/j.jece.2019.103270
Omajali, J. B., Gomez-Bolivar, J., Mikheenko, I. P., Sharma, S., Kayode, B., Al-Duri, B., et al. (2019). Novel catalytically active Pd/Ru bimetallic nanoparticles synthesized by Bacillus benzeovorans. Sci. Rep. 9 (1), 4715–4812. doi:10.1038/s41598-019-40312-3
Omajali, J. B., Mikheenko, I. P., Merroun, M. L., Wood, J., and Macaskie, L. E. (2015). Characterization of intracellular palladium nanoparticles synthesized by Desulfovibrio desulfuricans and Bacillus benzeovorans. J. Nanopart. Res. 17 (6), 264. doi:10.1007/s11051-015-3067-5
Osonga, F. J., Kalra, S., Miller, R. M., Isika, D., and Sadik, O. A. (2020). Synthesis, characterization and antifungal activities of eco-friendly palladium nanoparticles. RSC Adv. 10 (10), 5894–5904. doi:10.1039/c9ra07800b
Pal, M., Sasaki, T., and Koshizaki, N. (2001). Preparation of Pd/TiO2 nanocomposite by magnetron sputtering. Scr. Mater. 44 (8–9), 1817–1820. doi:10.1016/s1359-6462(01)00935-6
Palem, R. R., Shimoga, G., Kim, S. Y., Bathula, C., Ghodake, G. S., and Lee, S. H. (2022). Biogenic palladium nanoparticles: An effectual environmental benign catalyst for organic coupling reactions. J. Ind. Eng. Chem. 106, 52–68. doi:10.1016/j.jiec.2021.11.020
Palladium consumption worldwide in 2020 (2022). by industry. Available at: https://www.statista.com/statistics/693767/palladium-global-consumption-by-industry/ [Accessed June 7, 2022].
Palladium prices - Interactive Historical Chart (2022). Available at: https://www.macrotrends.net/2542/palladium-prices-historical-chart-data [Accessed June 7, 2022].
Palliyarayil, A., Jayakumar, K. K., Sil, S., and Kumar, N. S. (2018). A facile green tea assisted synthesis of palladium nanoparticles using recovered palladium from spent palladium impregnated carbon. Johns. Matthey Technol. Rev. 62 (1), 60–73. doi:10.1595/205651317x696252
Pan, W., Zhang, X., Ma, H., and Zhang, J. (2008). Electrochemical synthesis, voltammetric behavior, and electrocatalytic activity of Pd nanoparticles. J. Phys. Chem. C 112 (7), 2456–2461. doi:10.1021/jp710092z
Phan, T. T. V., Hoang, G., Nguyen, T. P., Kim, H. H., Mondal, S., Manivasagan, P., et al. (2019). Chitosan as a stabilizer and size-control agent for synthesis of porous flower-shaped palladium nanoparticles and their applications on photo-based therapies. Carbohydr. Polym. 205, 340–352. doi:10.1016/j.carbpol.2018.10.062
Phan, T. T. V., Huynh, T-C., Manivasagan, P., Mondal, S., and Oh, J. (2020). An up-to-date review on biomedical applications of palladium nanoparticles. Nanomaterials 10 (1), 66. doi:10.3390/nano10010066
Prabakaran, S., and Rajan, M. (2021). Biosynthesis of nanoparticles and their roles in numerous areas. Compr. Anal. Chem. 94, 1–47.
Qazi, F., Hussain, Z., and Tahir, M. N. (2016). Advances in biogenic synthesis of palladium nanoparticles. RSC Adv. 6 (65), 60277–60286. doi:10.1039/c6ra11695g
Qiu, X-F., Xu, J-Z., Zhu, J-M., Zhu, J-J., Xu, S., and Chen, H-Y. (2003). Controllable synthesis of palladium nanoparticles via a simple sonoelectrochemical method. J. Mater. Res. 18 (6), 1399–1404. doi:10.1557/jmr.2003.0192
Rabiee, N., Bagherzadeh, M., Kiani, M., and Ghadiri, A. M. (2020). Rosmarinus officinalis directed palladium nanoparticle synthesis: Investigation of potential anti-bacterial, anti-fungal and Mizoroki-Heck catalytic activities. Adv. Powder Technol. 31 (4), 1402–1411. doi:10.1016/j.apt.2020.01.024
Rai, M., Ingle, A. P., Birla, S., Yadav, A., and Santos, C. A. D. (2016). Strategic role of selected noble metal nanoparticles in medicine. Crit. Rev. Microbiol. 42 (5), 696–719. doi:10.3109/1040841X.2015.1018131
Rajakumar, G., Rahuman, A. A., Chung, I-M., Kirthi, A. V., Marimuthu, S., and Anbarasan, K. (2015). Antiplasmodial activity of eco-friendly synthesized palladium nanoparticles using Eclipta prostrata extract against Plasmodium berghei in Swiss albino mice. Parasitol. Res. 114 (4), 1397–1406. doi:10.1007/s00436-015-4318-1
Rajput, N. (2015). Methods of preparation of nanoparticles-a review. Int. J. Adv. Eng. Technol. 7 (6), 1806.
Rana, S., and Kalaichelvan, P. T. (2011). Antibacterial activities of metal nanoparticles. Antibact. Act. Met. Nanoparticles 11 (02), 21–23.
Rao, K. J., and Paria, S. (2015). Mixed phytochemicals mediated synthesis of multifunctional Ag–Au–Pd nanoparticles for glucose oxidation and antimicrobial applications. ACS Appl. Mater. Interfaces 7 (25), 14018–14025. doi:10.1021/acsami.5b03089
Rastogi, L., Karunasagar, D., Sashidhar, R. B., and Giri, A. (2017). Peroxidase-like activity of gum kondagogu reduced/stabilized palladium nanoparticles and its analytical application for colorimetric detection of glucose in biological samples. Sensors Actuators B Chem. 240, 1182–1188. doi:10.1016/j.snb.2016.09.066
Rawashdeh, R., and Haik, Y. (2009). Antibacterial mechanisms of metallic nanoparticles: A review. Dyn. Biochem. Process Biotechnol. Mol. Biol. 3 (2), 12–20.
Reddy, S. M., Datta, K. K. R., Sreelakshmi, C., Eswaramoorthy, M., and Reddy, B. V. (2012). Honey mediated green synthesis of Pd nanoparticles for suzuki coupling and hydrogenation of conjugated olefins. Nanosci. Nanotechnol. Lett. 4 (4), 420–425. doi:10.1166/nnl.2012.1331
Redwood, M. D., Deplanche, K., Baxter-Plant, V. S., and Macaskie, L. E. (2008). Biomass-supported palladium catalysts on Desulfovibrio desulfuricans and Rhodobacter sphaeroides. Biotechnol. Bioeng. 99 (5), 1045–1054. doi:10.1002/bit.21689
Rokade, S. S., Joshi, K. A., Mahajan, K., Patil, S., Tomar, G., Dubal, D. S., et al. (2018). Gloriosa superba mediated synthesis of platinum and palladium nanoparticles for induction of apoptosis in breast cancer. Bioinorg. Chem. Appl. 2018, 4924186. doi:10.1155/2018/4924186
Rokade, S. S., Joshi, K. A., Mahajan, K., Tomar, G., Dubal, D. S., Singh, V., et al. (2017). Novel anticancer platinum and palladium nanoparticles from Barleria prionitis. Glob. J. nanomedicine 2 (5), 555600. doi:10.19080/gjn.2017.02.555600
Roopan, S. M., Bharathi, A., Kumar, R., Khanna, V. G., and Prabhakarn, A. (2012). Acaricidal, insecticidal, and larvicidal efficacy of aqueous extract of Annona squamosa L peel as biomaterial for the reduction of palladium salts into nanoparticles. Colloids Surfaces B Biointerfaces 92, 209–212. doi:10.1016/j.colsurfb.2011.11.044
Saleh, E. A. M., Khan, A. U., Tahir, K., Almehmadi, S. J., Al-Abdulkarim, H. A., Alqarni, S., et al. (2021). Phytoassisted synthesis and characterization of palladium nanoparticles (PdNPs); with enhanced antibacterial, antioxidant and hemolytic activities. Photodiagnosis Photodyn. Ther. 36, 102542. doi:10.1016/j.pdpdt.2021.102542
Salehi, M. H., Yousefi, M., Hekmati, M., and Balali, E. (2019). In situ biosynthesis of palladium nanoparticles on Artemisia abrotanum extract-modified graphene oxide and its catalytic activity for Suzuki coupling reactions. Polyhedron 165, 132–137. doi:10.1016/j.poly.2019.02.053
Sathishkumar, M., Sneha, K., Kwak, I. S., Mao, J., Tripathy, S. J., and Yun, Y-S. (2009). Phyto-crystallization of palladium through reduction process using Cinnamom zeylanicum bark extract. J. Hazard. Mater. 171 (1–3), 400–404. doi:10.1016/j.jhazmat.2009.06.014
Sathishkumar, M., Sneha, K., and Yun, Y. S. (2009). Palladium nanocrystal synthesis using Curcuma longa tuber extract. Int. J. Mater Sci. 4 (1), 11–17.
Sayadi, M. H., Salmani, N., Heidari, A., and Rezaei, M. R. (2018). Bio-synthesis of palladium nanoparticle using Spirulina platensis alga extract and its application as adsorbent. Surfaces Interfaces 10, 136–143. doi:10.1016/j.surfin.2018.01.002
Shaik, M. R., Ali, Z. J. Q., Khan, M., Kuniyil, M., Assal, M. E., Alkhathlan, H. Z., et al. (2017). Green synthesis and characterization of palladium nanoparticles using Origanum vulgare L. extract and their catalytic activity. Molecules 22 (1), 165. doi:10.3390/molecules22010165
Shanthi, K., Sreevani, V., Vimala, K., and Kannan, S. (2017). Cytotoxic effect of Palladium nanoparticles synthesized from Syzygium aromaticum aqueous extracts and induction of apoptosis in cervical carcinoma. Proc. Natl. Acad. Sci. India Sect. B. Biol. Sci. 87 (4), 1101–1112. doi:10.1007/s40011-015-0678-7
Sharmila, G., Fathima, M. F., Haries, S., Geetha, S., Kumar, N. M., and Muthukumaran, C. (2017). Green synthesis, characterization and antibacterial efficacy of palladium nanoparticles synthesized using Filicium decipiens leaf extract. J. Mol. Struct. 1138, 35–40. doi:10.1016/j.molstruc.2017.02.097
Sharmila, G., Haries, S., Fathima, M. F., Geetha, S., Kumar, N. M., and Muthukumaran, C. (2017). Enhanced catalytic and antibacterial activities of phytosynthesized palladium nanoparticles using Santalum album leaf extract. Powder Technol. 320, 22–26. doi:10.1016/j.powtec.2017.07.026
Shendage, S. S., Patil, U. B., and Nagarkar, J. M. (2013). Electrochemical deposition of highly dispersed palladium nanoparticles on nafion-graphene film in presence of Ferrous ions for Ethanol Electrooxidation. Fuel cells 13 (3), 364–370. doi:10.1002/fuce.201300043
Sheny, D. S., Philip, D., and Mathew, J. (2012). Rapid green synthesis of palladium nanoparticles using the dried leaf of Anacardium occidentale. Spectrochimica Acta Part A Mol. Biomol. Spectrosc. 91, 35–38. doi:10.1016/j.saa.2012.01.063
Silva, S. F., Coelho, L., Frazão, O., Santos, J. L., and Malcata, F. X. (2011). A review of palladium-based fiber-optic sensors for molecular hydrogen detection. IEEE Sens. J. 12 (1), 93–102. doi:10.1109/jsen.2011.2138130
Singh, J., Dutta, T., Kim, K-H., Rawat, M., Samddar, P., and Kumar, P. (2018). ‘Green’synthesis of metals and their oxide nanoparticles: Applications for environmental remediation. J. Nanobiotechnology 16 (1), 84–24. doi:10.1186/s12951-018-0408-4
Slavcheva, E., Ganske, G., and Schnakenberg, U. (2014). Sputtered Pd as hydrogen storage for a chip-integrated microenergy system. Sci. World J. 2014. doi:10.1155/2014/146126
Slavin, Y. N., Asnis, J., Häfeli, U. O., and Bach, H. (2017). Metal nanoparticles: Understanding the mechanisms behind antibacterial activity. J. Nanobiotechnology 15 (1), 65–20. doi:10.1186/s12951-017-0308-z
Smuleac, V., Varma, R., Sikdar, S., and Bhattacharyya, D. (2011). Green synthesis of Fe and Fe/Pd bimetallic nanoparticles in membranes for reductive degradation of chlorinated organics. J. Memb. Sci. 379 (1–2), 131–137. doi:10.1016/j.memsci.2011.05.054
Søbjerg, L. S., Lindhardt, A. T., Skrydstrup, T., Finster, K., and Meyer, R. L. (2011). Size control and catalytic activity of bio-supported palladium nanoparticles. Colloids Surfaces B Biointerfaces 85 (2), 373–378. doi:10.1016/j.colsurfb.2011.03.014
Sonbol, H., Ameen, F., AlYahya, S., Almansob, A., and Alwakeel, S. (2021). Padina boryana mediated green synthesis of crystalline palladium nanoparticles as potential nanodrug against multidrug resistant bacteria and cancer cells. Sci. Rep. 11 (1), 5444–5519. doi:10.1038/s41598-021-84794-6
Song, M., Liu, N., He, L., Liu, G., Ling, D., Su, X., et al. (2018). Porous hollow palladium nanoplatform for imaging-guided trimodal chemo-photothermal-and radiotherapy. Nano Res. 11 (5), 2796–2808. doi:10.1007/s12274-017-1910-y
Sriramulu, M., and Sumathi, S. (2018). Biosynthesis of palladium nanoparticles using Saccharomyces cerevisiae extract and its photocatalytic degradation behaviour. Adv. Nat. Sci. Nanosci. Nanotechnol. 9 (2), 025018. doi:10.1088/2043-6254/aac506
Surendra, T. V., Roopan, S. M., Arasu, M. V., Al-Dhabi, N. A., and Rayalu, G. M. (2016). RSM optimized Moringa oleifera peel extract for green synthesis of M. oleifera capped palladium nanoparticles with antibacterial and hemolytic property. J. Photochem. Photobiol. B Biol. 162, 550–557. doi:10.1016/j.jphotobiol.2016.07.032
Tahir, K., Nazir, S., Ahmad, A., Li, B., Shah, S. A. A., Khan, A. U., et al. (2016). Biodirected synthesis of palladium nanoparticles using Phoenix dactylifera leaves extract and their size dependent biomedical and catalytic applications. RSC Adv. 6 (89), 85903–85916. doi:10.1039/c6ra11409a
Tahir, K., Nazir, S., Li, B., Ahmad, A., Nasir, T., Khan, A. U., et al. (2016). Sapium sebiferum leaf extract mediated synthesis of palladium nanoparticles and in vitro investigation of their bacterial and photocatalytic activities. J. Photochem. Photobiol. B Biol. 164, 164–173. doi:10.1016/j.jphotobiol.2016.09.030
Tan, L., Jones, T. R., Poitras, J., Xie, J., Liu, X., and Southam, G. (2020). Biochemical synthesis of palladium nanoparticles: The influence of chemical fixatives used in electron microscopy on nanoparticle formation and catalytic performance. J. Hazard. Mater. 398, 122945. doi:10.1016/j.jhazmat.2020.122945
Tang, L., and Shim, J-J. (2015). Supercritical CO2 mediated synthesis and catalytic activity of graphene/Pd nanocomposites. Mater. Res. Bull. 71, 53–60. doi:10.1016/j.materresbull.2015.06.043
Turunc, E., Binzet, R., Gumus, I., Binzet, G., and Arslan, H. (2017). Green synthesis of silver and palladium nanoparticles using Lithodora hispidula (Sm.) Griseb.(Boraginaceae) and application to the electrocatalytic reduction of hydrogen peroxide. Mater. Chem. Phys. 202, 310–319. doi:10.1016/j.matchemphys.2017.09.032
Ullah, S., Ahmad, A., Khan, A., Zhang, J., Raza, M., ur Rahman, A., et al. (2018). Palladium nanoparticles synthesis, characterization using glucosamine as the reductant and stabilizing agent to explore their antibacterial & catalytic applications. Microb. Pathog. 125, 150–157. doi:10.1016/j.micpath.2018.09.020
Ün, Ş., Ünlü, A., Ün, İ., and Ok, S. (2021). Green synthesis, characterization and catalytic activity evaluation of palladium nanoparticles facilitated by Punica granatum peel extract. Inorg. Nano-Metal Chem. 51 (9), 1232–1240.
Vaghela, H., Shah, R., and Pathan, A. (2018). Palladium nanoparticles mediated through bauhinia variegata: Potent in vitro anticancer activity against mcf-7 cell lines and antimicrobial assay. Curr. Nanomater 3 (3), 168–177. doi:10.2174/2405461504666190131142303
Veisi, H., Karmakar, B., Tamoradi, T., Tayebee, R., Sajjadifar, S., Lotfi, S., et al. (2021). Bio-inspired synthesis of palladium nanoparticles fabricated magnetic Fe3O4 nanocomposite over Fritillaria imperialis flower extract as an efficient recyclable catalyst for the reduction of nitroarenes. Sci. Rep. 11 (1), 4515–15. doi:10.1038/s41598-021-83854-1
Veisi, H., Nasrabadi, N. H., and Mohammadi, P. (2016). Biosynthesis of palladium nanoparticles as a heterogeneous and reusable nanocatalyst for reduction of nitroarenes and Suzuki coupling reactions. Appl. Organomet. Chem. 30 (11), 890–896. doi:10.1002/aoc.3517
Veisi, H., Rashtiani, A., and Barjasteh, V. (2016). Biosynthesis of palladium nanoparticles using Rosa canina fruit extract and their use as a heterogeneous and recyclable catalyst for Suzuki–Miyaura coupling reactions in water. Appl. Organomet. Chem. 30 (4), 231–235. doi:10.1002/aoc.3421
Venkatesham, M., Ayodhya, D., and Veerabhadram, G. (2015). Green synthesis, characterization and catalytic activity of palladium nanoparticles by xanthan gum. Appl. Nanosci. 5 (3), 315–320. doi:10.1007/s13204-014-0320-7
Vijilvani, C., Bindhu, M. R., Frincy, F. C., AlSalhi, M. S., Sabitha, S., Saravanakumar, K., et al. (2020). Antimicrobial and catalytic activities of biosynthesized gold, silver and palladium nanoparticles from Solanum nigurum leaves. J. Photochem. Photobiol. B Biol. 202, 111713. doi:10.1016/j.jphotobiol.2019.111713
Vishnukumar, P., Vivekanandhan, S., and Muthuramkumar, S. (2017). Plant-mediated biogenic synthesis of palladium nanoparticles: Recent trends and emerging opportunities. ChemBioEng Rev. 4 (1), 18–36. doi:10.1002/cben.201600017
W Raut, R., Nikam, T., Kashid, S. B., and S Malghe, Y. (2013). Rapid biosynthesis of platinum and palladium metal nanoparticles using root extract of Asparagus racemosus Linn. Adv. Mat. Lett. 4 (8), 650–654. doi:10.5185/amlett.2012.11470
Wang, W., Zhang, B., Liu, Q., Du, P., Liu, W., and He, Z. (2018). Biosynthesis of palladium nanoparticles using Shewanella loihica PV-4 for excellent catalytic reduction of chromium (VI). Environ. Sci. Nano 5 (3), 730–739. doi:10.1039/c7en01167a
Weiss, J. T., Dawson, J. C., Macleod, K. G., Rybski, W., Fraser, C., Torres-Sánchez, C., et al. (2014). Extracellular palladium-catalysed dealkylation of 5-fluoro-1-propargyl-uracil as a bioorthogonally activated prodrug approach. Nat. Commun. 5 (1), 3277–3279. doi:10.1038/ncomms4277
Xiao, J-W., Fan, S-X., Wang, F., Sun, L-D., Zheng, X-Y., and Yan, C-H. (2014). Porous Pd nanoparticles with high photothermal conversion efficiency for efficient ablation of cancer cells. Nanoscale 6 (8), 4345–4351. doi:10.1039/c3nr06843a
Xiong, Y., Huang, L., Mahmud, S., Yang, F., and Liu, H. (2020). Bio-synthesized palladium nanoparticles using alginate for catalytic degradation of azo-dyes. Chin. J. Chem. Eng. 28 (5), 1334–1343. doi:10.1016/j.cjche.2020.02.014
Xu, H., Zeiger, B. W., and Suslick, K. S. (2013). Sonochemical synthesis of nanomaterials. Chem. Soc. Rev. 42 (7), 2555–2567. doi:10.1039/c2cs35282f
Yan, A., Wang, Y., Tan, S. N., Mohd Yusof, M. L., Ghosh, S., and Chen, Z. (2020). Phytoremediation: A promising approach for revegetation of heavy metal-polluted land. Front. Plant Sci. 11, 359. doi:10.3389/fpls.2020.00359
Yang, X., Li, Q., Wang, H., Huang, J., Lin, L., Wang, W., et al. (2010). Green synthesis of palladium nanoparticles using broth of Cinnamomum camphora leaf. J. Nanopart. Res. 12 (5), 1589–1598. doi:10.1007/s11051-009-9675-1
Yates, M. D., Cusick, R. D., and Logan, B. E. (2013). Extracellular palladium nanoparticle production using Geobacter sulfurreducens. ACS Sustain. Chem. Eng. 1 (9), 1165–1171. doi:10.1021/sc4000785
Yazdani, A., Sayadi, M., and Heidari, A. (2018). Green biosynthesis of palladium oxide nanoparticles using dictyota indica seaweed and its application for adsorption. J. Water Environ. Nanotechnol. 3 (4), 337–347.
Yi, X., Wu, Y., Tan, G., Yu, P., Zhou, L., Zhou, Z., et al. (2017). Palladium nanoparticles entrapped in a self-supporting nanoporous gold wire as sensitive dopamine biosensor. Sci. Rep. 7 (1), 7941–7949. doi:10.1038/s41598-017-07909-y
Yin, D., Zhang, J., Li, W., and Fu, Y. (2021). Hyaluronic acid-guided synthesis of Pd nanocatalysts for transfer hydrogenation of 4-nitrophenol. Catal. Lett. 151 (7), 1902–1910. doi:10.1007/s10562-020-03455-x
Zereini, F., and Alt, F. (2006). Palladium emissions in the environment: Analytical methods, environmental assessment and health effects. Springer.
Zhan, G., Hong, Y., Mbah, V. T., Huang, J., Ibrahim, A-R., Du, M., et al. (2012). Bimetallic Au–Pd/MgO as efficient catalysts for aerobic oxidation of benzyl alcohol: A green bio-reducing preparation method. Appl. Catal. A General 439, 179–186. doi:10.1016/j.apcata.2012.07.005
Zhan, G., Huang, J., Du, M., Abdul-Rauf, I., Ma, Y., and Li, Q. (2011). Green synthesis of Au–Pd bimetallic nanoparticles: Single-step bioreduction method with plant extract. Mat. Lett. 65 (19–20), 2989–2991. doi:10.1016/j.matlet.2011.06.079
Zhang, W., Culley, D. E., Hogan, M., Vitiritti, L., and Brockman, F. J. (2006). Oxidative stress and heat-shock responses in Desulfovibrio vulgaris by genome-wide transcriptomic analysis. Ant. Van Leeuwenhoek 90 (1), 41–55. doi:10.1007/s10482-006-9059-9
Keywords: biogenic nanoparticles, palladium, catalyst, antimicrobial, anticancer
Citation: Joudeh N, Saragliadis A, Koster G, Mikheenko P and Linke D (2022) Synthesis methods and applications of palladium nanoparticles: A review. Front. Nanotechnol. 4:1062608. doi: 10.3389/fnano.2022.1062608
Received: 06 October 2022; Accepted: 03 November 2022;
Published: 18 November 2022.
Edited by:
Abhishek Kumar, Washington University in St. Louis, United StatesReviewed by:
Meghana Ramani, Wayne State University, United StatesCopyright © 2022 Joudeh, Saragliadis, Koster, Mikheenko and Linke. This is an open-access article distributed under the terms of the Creative Commons Attribution License (CC BY). The use, distribution or reproduction in other forums is permitted, provided the original author(s) and the copyright owner(s) are credited and that the original publication in this journal is cited, in accordance with accepted academic practice. No use, distribution or reproduction is permitted which does not comply with these terms.
*Correspondence: Dirk Linke, ZGlyay5saW5rZUBpYnYudWlvLm5v
Disclaimer: All claims expressed in this article are solely those of the authors and do not necessarily represent those of their affiliated organizations, or those of the publisher, the editors and the reviewers. Any product that may be evaluated in this article or claim that may be made by its manufacturer is not guaranteed or endorsed by the publisher.
Research integrity at Frontiers
Learn more about the work of our research integrity team to safeguard the quality of each article we publish.