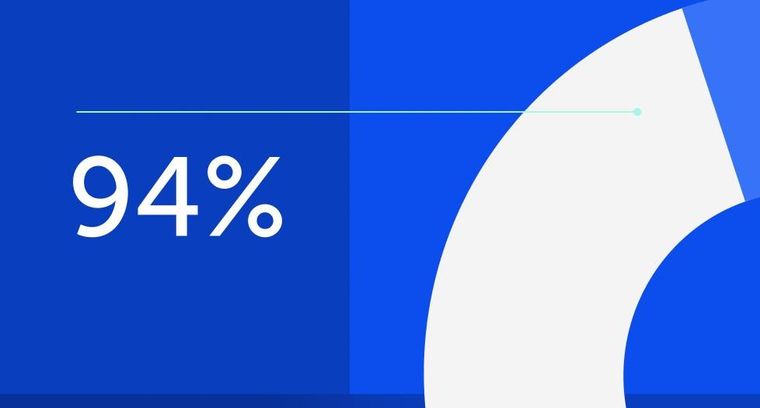
94% of researchers rate our articles as excellent or good
Learn more about the work of our research integrity team to safeguard the quality of each article we publish.
Find out more
REVIEW article
Front. Nanotechnol., 09 December 2022
Sec. Nanomaterials
Volume 4 - 2022 | https://doi.org/10.3389/fnano.2022.1060756
This article is part of the Research TopicNanotechnology and Nanoscience to manage SARS-CoV-2 Variants of ConcernView all 14 articles
Researchers are now looking to nanomaterials to fight serious infectious diseases that cause outbreaks and even pandemics. SARS-CoV-2 brought chaos to almost every walk of life in the past 2 years and has challenged every available treatment method. Although vaccines were developed in no time against it, the most pressing issue was the emergence of variants of concern arising because of the rapidly evolving viral strains. The higher pathogenicity and, in turn, the higher mortality rate of infections caused by these variants renders the existing vaccines less effective and the effort to produce further vaccines a costly endeavor. While several techniques, such as immunotherapy and repurposed pharmaceutical research, are being studied to minimize viral infection, the fundamentals of nanotechnology must also be considered to enhance the anti-SARS-CoV-2 efforts. For instance, silver nanoparticles (AgNPs) have been applied against SARS-CoV-2 effectively. Similarly, nanomaterials have been tested in masks, gloves, and disinfectants to aid in controlling SARS-CoV-2. Nanotechnology has also contributed to diagnoses such as rapid and accurate detection and treatment such as the delivery of mRNA vaccines and other antiviral agents into the body. The development of polymeric nanoparticles has been dubbed a strategy of choice over traditional drugs because of their tunable release kinetics, specificity, and multimodal drug composition. Our article explores the potential of nanomaterials in managing the variants of concern. This will be achieved by highlighting the inherent ability of nanomaterials to act against the virus on fronts such as inhibition of SARS-CoV-2 entry, inhibition of RNA replication in SARS-CoV-2, and finally, inhibition of their release. In this review, a detailed discussion on the potential of nanomaterials in these areas will be tallied with their potential against the current and emerging future variants of concern.
The history of humankind has witnessed more than 15 pandemics and numerous outbreaks since the earliest recorded pandemic during the Peloponnesian war in 430 B.C. Some of the most recent epidemics were severe acute respiratory syndrome (SARS), Middle East Respiratory Syndrome (MERS), and Ebola (Lombardi et al., 2021). In late 2019, a high number of patients with pneumonia of unknown cause were reported in Wuhan city of China. They soon confirmed a novel coronavirus, “SARS-CoV-2 caused it.” SARS-CoV-2 was revealed to be phylogenetically associated with SARS viruses according to its genomic analysis (Rume and Islam, 2020). It was declared a “public health emergency of international concern” by WHO on 30 January 2020, and only 39 days later, on 11 March 2020, they declared the COVID-19 pandemic (Tarkar and Technology, 2020). The general symptoms of COVID-19 infection are fever, nausea, cough, loss of smell and taste, fatigue, diarrhea, sore throat, and vomiting. Although, the severity of COVID-19 leads to respiratory failure, cardiac injury, and even death (Struyf et al., 2022). As of 1 November 2022, there have been 635,472,882 confirmed cases of the coronavirus disease (COVID-19), including 6,593,723 deaths, according to the world health organization (WHO) (worldometer, 2022). Vaccination has always been the most effective strategy for controlling epidemics and outbreaks (Cleve, 2021). To tackle the disease, companies and organizations worldwide have attempted to develop COVID-19 vaccines to achieve herd immunity since the start of the pandemic (Haynes et al., 2020). Different techniques have been employed to create vaccines against SARS-CoV-2 (Jeyanathan et al., 2020). The approaches to COVID-19 vaccine development include live-attenuated vaccines, adenovirus vectors, inactivated viruses, recombinant protein, and DNA and RNA vaccines. RNA vaccines emerged as a revolution to cope with the pandemic. However, the consistently changing characteristic of the virus is a challenge. SARS-CoV-2 is undergoing rapid changes in its genome and structure, and new variants are constantly resulting. Some of the variants of SAR-CoV-2 are Alpha (emerged in September 2020, United Kingdom), Beta (May 2020, South Africa), Gamma (November 2020), Delta (October 2020), and Omicron (November 2020, many counties). Because more novel SARS-CoV-2 variants are expected, the currently available vaccines, preventive measures, and antiviral drugs are, therefore, only temporarily effective.
Consequently, it is time to investigate rapid and accurate diagnostic tools and therapeutics with higher efficacy. Considering these challenges, experts suggest that nanotechnology can play a pivotal role in facing these challenges and managing COVID-19. Nanotechnology has the potential to overcome the limitations in the existing therapies and strategies used against SARS-CoV-2. Nanotechnology offers different possibilities and approaches to combat the virus. We can develop novel and rapid diagnostic kits and therapeutics through nanotechnology and design more effective drugs and vaccines (Ruiz-Hitzky et al., 2020). The size, shape, surface-to-volume ratio, and other unique physiochemical properties of nanomaterials enrich them with potential for purposes like drug delivery, antibody development, vaccine formulation, and personalized treatment (Paliwal et al., 2020). Nanoparticles, quantum dots, nano-based biosensors, lipid-based nanoparticles, and nano-assemblies of polymers or proteins are the types of nano-systems that have already been employed for the detection, treatment, delivery, and development of vaccines against the virus (Jindal et al., 2017; Alphandéry, 2020). Various metallic nanoparticles can potentially be used for diagnosing and identifying SARS-CoV-2 and other variants of concern (VOCs) (Rashidzadeh et al., 2021). Organic and polymeric nanoparticles play a pivotal role in the drug delivery systems of coronaviruses. Specific ligands can be used to functionalize the antigen-loaded nanoparticles. For instance, Raghuwanshi et al. (2012) developed plasmid DNA-loaded chitosan nanoparticles and used them as a vaccine for different variants of SARS. Literature reviews have already shown the immense potential of nanotechnology in coping with pandemics like COVID-19 (Yasamineh et al., 2022). Detailed studies are available that report the potential of nanotechnology against SARS-CoV-2 (Sahu et al., 2021). There are, however, limited studies on how nanotechnology can contribute towards the fight against variants of SARS-CoV-2 to reduce the burden and stop the pandemic quickly. For instance, Huang X. et al. (2022) have reported the use of nanotechnology-based strategies against VOCs. However, most of this study is focused on nanoparticle-based antibody eliciting vaccines. An account of the use of nanomaterials especially metallic nanoparticles at the front of prevention, treatment and its future implications was needed. The main aim was to emphasize the role of nanomaterials synthesized through different means and possessing potential of preventing VOCs. Therefore, in this review, we will explore the efforts of nanomaterials, including metallic nanoparticles, prevent and tackle SARS-CoV-2. We will mainly focus on the potential of nanotechnology and nanomaterials to cope with variants of SARS-CoV-2 and its future concerns.
During any outbreak, vaccination has always been the best weapon. While analyzing the previous outbreaks, vaccination has proved a convenient tool that has always been practiced in achieving herd immunity. Through vaccination, the susceptibility of an individual to viral infection is reduced. In addition, it also minimizes the transmissibility of infections among a population (Gellin et al., 2001). Until the availability of vaccines, therapeutic drugs were used as a temporary solution. In therapeutic strategy, antimicrobial drugs, i.e., antivirals, are used to eliminate the virus from the body. These drugs kill the virus or inhibit its growth or other aspects of its life cycle. Both these approaches play a crucial role in combating any viral strain. However, they have certain challenges to face. For example, in the case of vaccination, new viral strains are always imminent (Le Page, 2021). To cope with the emergence of new strains, it is always necessary to predict their emergence, and be ready to develop new vaccines every few years, and sometimes every year. At the same time, strict patient compliance is an essential condition for the effectiveness of therapeutics. Therapeutic drugs against viral infections may have harsh side effects that sometimes outweigh the benefits. Both strategies demand a lot of resources and time, which are very expensive in emergencies such as pandemics.
Nanotechnology has the potential to overcome these limitations. Nanotechnology has attracted the attention of the medical science community and offers advances in the diagnosis, prevention, and treatment of diseases such as COVID-19. It provides opportunities in the shape of nanomedicines and nanomaterials to tackle the disease. Nanomaterials have been studied widely over the past few decades for their size, shape, and surface-to-volume ratio. These properties make them efficient for the rapid identification and detection of SARS-CoV-2, the design of proper personal protective equipment (PPE), the creation of vaccines, and the treatment of the infection. The food and drug administration (FDA) of the United States has approved 49 antigen diagnostic devices for COVID-19, most of which are based on lateral flow assays (LFA) using either gold nanoparticles (AuNPs) or quantum dots (Xu et al., 2022). AuNPs based-biosensors were recently designed to detect SARS-CoV-2 that can bind to lab-designed DNA receptors rapidly. This technique is very sensitive as SARS-CoV-2 has single-stranded RNA, which readily binds to stabilized complementary DNA receptors (Hasanzadeh et al., 2021). Nanoparticle-based biosensors coupled with highly sensitive loop-mediated isothermal technique (LAMP) are also used for virus detection, and one such biosensor was recently fabricated for SARS-CoV-2 screening. This assay is reliable, less expensive, and highly efficient (Hasanzadeh et al., 2021).
Literature suggests that using nanoparticles to treat COVID-19 is safe and effective for drug encapsulation and toxicity reduction (Lombardo et al., 2019). Chitosan nanoparticles, named Novochizol, possess mucoadhesive properties (Tharayil et al., 2021). Therefore, they can treat the intestinal tract reactions caused by SARS-CoV-2 infection. Chitosan can also encapsulate and carry drugs to the lung to treat severe COVID-19 patients (Cavalcanti and Cajuba de Britto Lira Nogueira, 2020). SARS-CoV-2 can be captured, neutralized, and prevented from infecting host cells using nano-sponges developed by Zhang et al. (2020). These nano-sponges have surface-binding receptors capable of attracting SAR-CoV-2. Although the administration of nanoparticle-based drugs was a complicated issue, that is also addressed. For instance, Itani et al. (2020) suggest the intranasal administration of theranostic nanoparticles to treat COVID-19 as they enable the carrying of therapeutic portions like antibodies.
Nanotechnology also has a pivotal role in the drug delivery systems. Nanoparticles have already been used for this purpose and are still in service. Different therapeutic molecules have been used to deliver antiviral drugs in an inhalable form. However, their poor stability has always been an issue that can be solved by coupling them with different nano-delivery systems. For example, nano-carriers are used to overcome the problem of poorly soluble drugs, which otherwise can cause lung toxicity (Beck-Broichsitter et al., 2012). This means that there is an immense potential for nanotechnology-based strategies in therapeutic applications in case of COVID-19.
Nanotechnology also finds its application in the development of vaccines. Nanovesicles obtained from spike, envelope, and membrane proteins of SARS-CoV-2 have been used as nano vaccines against the virus (Kato et al., 2019). The primary purpose of developing nanoparticle-based vaccines is to provoke an adaptive immune response to generate immunological memory. These memory B cells induced by nanoparticle-based vaccines are expected to live longer than normal vaccine-generated memory cells. This results in antibodies that neutralize the interaction between SARS-CoV-2 and host cell, hence viral clearance (Guerrini et al., 2022). Similarly, lipid-based nanoparticles and peptide-based vaccines for SARS-CoV-2 and some other enveloped viruses have also been employed (Lim et al., 2021). This shows that nanotechnology has the potential to overcome the limitations of traditional strategies for vaccine development against coronavirus infections.
Like other RNA viruses, SARS-CoV-2 also constantly changes through mutation. Generally, due to some selective advantages of the virus, new variants become more common. Whenever a virus replicates itself, structural changes occur to its genome. These characteristic changes of the virus are led by mutations, including characteristics that affect its ability to spread and/or to cause more severe illness and death (Otto et al., 2021). Combining the genetic material from two different variants creates a new recombinant variant. This leads to the creation of variants. More transmissible variants cause severe illness, and those that can easily evade immune response are more public health concerns among many SARS-CoV-2 variants detected (Walensky et al., 2021).
Since the beginning of the COVID-19 pandemic, the genetic lineages of SARS-CoV-2 have been emerging and circulating the world. In the early stage of pandemics, mutations have been found in the evolved sequence of 103 strains (Awadasseid et al., 2021). The evolutionary rate of SARS-CoV-2 per year has been estimated between 0.0004 and 0.002 mutations per nucleotide. The phylogenetic classification of new lineage emerging SARS-CoV-2 has been difficult because they often differ by just a few nucleotides (Tao et al., 2021). These variants can increase virus transmission rates and reinfection risk and reduce the protection afforded by neutralizing monoclonal antibodies and vaccination.
Different variants of SARS-CoV-2 are grouped into three categories. The first, known as Variants of Interest (VOI), have mutations in binding receptors. These genetic mutations suggest that they are conferred by natural infection or vaccination. They may partially escape immunity and become more contagious. This category includes variants described in Brazil and United Kingdom, known as P.2 and B.1.525 and a variant associated with a rapid spread in New York, B.1.526. The second category is Variants of Concern or variants of public health importance (VOC), which may cause more severe infections with increased hospitalization and high mortality rate as they are more contagious and virulent than VOIs. It includes B.1.1.7 (also known as Alpha) lineage first detected in the United Kingdom, identified as the first major variant of concern which has now been detected in at least 80 countries. Other VOCs include Beta, Gamma, and Delta (Figure 1). The third category that could cause more severe clinical manifestation is known as variants of high consequence, providing anti-viral-resistant variants and variants with real loss of preventive efficacy of vaccines, antibodies, and monoclonal therapy. Till now, none of the SARS-CoV-2 variants has been classified in this category (Henry and Walke, 2021; Lauring and Malani, 2021).
FIGURE 1. A general description of SARS-CoV-2 variants of concern and the potential of nanotechnology in dealing with them.
As with all viruses, SARS-COV-2 will continue to evolve if it continues to spread. The more the virus spreads, the more pressure there is for the virus to change. So, the best way to prevent more variants from emerging is to stop the spread of the virus (Halim, 2021). As many people live with innate and acquired immunosuppression, viral evolution in immunocompromised patients can be essential in emerging new variants. Thus, with persistent SARS-CoV-2 infection, rapid viral evolution has been described in immunosuppressed patients, which can be a vital factor in the emergence of such variants (Corey et al., 2021). The emergence of new variants highlights the importance of early identification and genomic surveillance for predicting future variants (Abdool Karim and de Oliveira, 2021).
Mutations in the spike glycoprotein are an area of high interest that potentially compromise vaccine effectiveness by escaping from host antibodies (Lauring and Hodcroft, 2021). The emerging SARS-CoV-2 variants demand effective vaccinations. For more effective vaccines and to control the spread of SARS-CoV-2, nanotechnology plays a vital role in fighting against SARS-CoV-2 and controlling the transmission rate that will, in return, control new variations. The hope for a quick ending to the pandemic was dampened despite the remarkable efficacy of mRNA vaccines against the original SARS-CoV-2 strain due to the high mutation rate. Many studies indicate that the transmission rate and virulence, along with reduced neutralization, have been increased by new variants, making them even more dangerous and troublesome. The most profound contribution of nanotechnology is the development of successful and highly effective nanotechnology-based messenger RNA vaccines. Nanotechnology offers other solutions, including ACE2-based nano-decoys, engineered neutralizing antibodies, and nanoparticle vaccine-elicited neutralizing antibodies. Mutation in the S protein of SARS-CoV-2 decreases antibody neutralization and increases its transmission. Thus, targeting the inhibiting of ACE2 receptors’ interaction with the S protein of the variants through nanotechnology could be the most straightforward and promising strategy. The key advantage of nanoparticle-based vaccines, particularly mRNA-Lipid nanoparticles-based vaccines, includes modularity, rapid manufacturing, and high efficacy (Huang X. et al., 2022).
Prevention and mitigation against SARS-CoV-2 are as crucial as the other two pillars of diagnosis and therapeutics to combat the virus (Kamat et al., 2021). The first case of SARS-CoV-2 was reported in 2019, which quickly spread like wildfire, with cases in almost every country in no time because of its high transmissibility rate (Petersen et al., 2020). It is transmitted to humans directly or indirectly by air passage and touching contaminated surfaces (Cirrincione et al., 2020). To block its transmission route, it was initially recommended to use personal protective equipment such as masks, gloves, and clothes, use hand sanitizers, and avoid public gatherings that work up to some extent (Cirrincione et al., 2020). Otherwise, it causes a burden on healthcare centers and pharmaceutical industries (Mallhi et al., 2020). To solve these rising problems, researchers are searching for the most suitable solutions; ultimately, nanotechnology is one of the most promising solutions. Nanomaterials can be essential in preventing the spread of SARS-CoV-2 and its variants (Table 1) (Ramakrishnan et al., 2021) because of their distinctive properties, as mentioned before. Nanotechnology aid as a helping hand against SARS-CoV-2 prevention in the following ways.
The rate of infection of SARS-CoV-2 is much higher than the previous epidemic SARS-CoV-1. Despite several preventive measures, many people have been infected with SARS-CoV-2. One of the factors, due to which, healthcare workers as well as public became vulnerable was the use of contaminated Personal Protective Equipment (PPE) in hospitals (Sportelli et al., 2020). Nanotechnology based strategies could provide better protection when applied during the manufacturing of PPE. These include the use of angiotensin-converting enzyme-2 (ACE-2) coated nanoparticles; iron oxide coated nanomaterials, and coating by silver nanoparticles, etc. Using these nanomaterials in face masks, eye-protecting glasses, hand sanitizers, and clothes can exhibit antiviral effects, and thus a higher prevention capacity to avoid spread and hence the emergence of SARS-CoV-2 variants (Dhama et al., 2021; Mohapatra et al., 2021).
Protective masks based on the use of nanomaterials promise a remarkably effective way of preventing spread of SARS-CoV-2. For instance, a type of protective mask based on graphene nanomaterials has been developed by directly transferring graphene layers onto disposable surgical masks via a dual-mode laser-induced transferring technique (Pal et al., 2021). It was reported that graphene-coated masks possess self-cleaning abilities. Additionally, due to the hydrophobic nature of laser-modified graphene, it repels the incoming respiratory droplets. Similarly, these laser-modified mask temperatures can attain up to 80°C due to sunlight-induced photothermal activity and thus kill any microorganisms. This means that these masks could be used for a long time because of its self-disinfection and reusability against bacteria and viruses.
Similarly, the laser-induced transfer method has directly fabricated plasmonic silver nanoparticles on N95 masks. It also showed self-cleaning abilities with better protection, releasing silver ions with respiratory droplets against bacteria and the SARS-CoV-2 virus (Kiremitler et al., 2022). Furthermore, Aydemir and Ulusu (2020) reported coating masks and nasal filters with ACE2 enzyme for protection against SARS-CoV-2. The PPE fabrics woven by polyamide 6.6 fibers (PA66) embedded with zinc ions were reported to reduce the Influenza A H1N1 virus and SARS-CoV-2 titer and give protection against the viral spread (Gopal et al., 2021). The advantage of this embedded fabric is that it provides protection even after 50 washes. Similarly, Hewawaduge et al. (2021) reported the inactivation of SARS-CoV-2 by incorporating Copper Sulfide in the three-layer mask to be a lifesaver during previous and future pandemics.
In addition to masks, nanoparticles are used in gloves against SARS-CoV-2. It is known that SARS-CoV-2 enters the body through the conversion of ACE2 receptors; blocking the receptors or lowering the quantities of this enzyme in the body may help to control the infection. For this purpose, the ACE2 proteins coated on nanoparticles have shown remarkable potential and chemical reliability, which can be used to make gloves (Mallakpour et al., 2022). These gloves, similar to protective masks, can protect against infection by killing it in coated films.
The problem of contamination in PPEs was coupled with the fact there was a shortage of PPE due to high demand. This led to a shortage of PPE and, as a result, a high infection rate among healthcare workers. The public faced a high infection rate supplies with PPE during the pandemic. On the other side, it also caused a burden on the environment. Daily, more than 450 tons of medical waste have been created since COVID-19 started. Scientists are exploring ways to extend PPE usage time while ensuring sterility. Different methods have been explored, like moist heat, hydrogen peroxide, and ultraviolet germicidal irradiation. In this area, the use of nanoparticles is found to be more efficacious (Xu et al., 2021). However, more effective, and safe strategies were needed that could decontaminate the existing PPE to reduce spread.
The mask has a central layer of zeolitic imidazolate framework (ZIF-67) trapped in a polystyrene hierarchical porous nanofiber membrane (ZIF-67/PS HPNFM), which provides effective filtration of airborne hazardous substances and particulate matter. Because of their high porosity, surface area, and stability in an acidic environment, ZIFs make an effective adsorbent. Using electrospinning technology, ZIFs could be added to the nanofibers to improve their capacity to filter hazardous gas compounds and particulate matter. This new class of reusable filter fibers has improved air permeability. The membrane-based masks could filter out pollutants. As a result, these masks could be cleaned with ethanol and water without losing their ability to filter (Liao et al., 2021). SARS-CoV-2 can be neutralized using a novel antiviral technique called photocatalysis. After light irradiation, photocatalytic materials produce reactive oxygen species (ROS) when oxygen is present. These ROS then destroys the virus, causing damage to its proteins, nucleic acids, and lipid membrane. Titanium oxide (TiO2)-based photocatalytic materials have prepared nanowire-based filters for facemasks. The photocatalytic capabilities in these masks produce ROS upon UV illumination. To effectively trap pathogens of various sizes, the size of the facemask filter can be adjusted during the manufacturing of TiO2 nanowires on the filter paper. It has been shown as easily sterilizable, reusable, and antiviral, thus acting as a powerful prophylactic weapon against rapid transmission of SARS-CoV-2 (Karmacharya et al., 2021).
To prevent the transmission of SARS-CoV-2, one approach is to slow down the dissemination of the virus by disinfecting air, skin, or surrounding surfaces. For this purpose, chemicals are used (such as alcohol, peroxides, quaternary amines, and chlorine). Still, they have certain limitations, such as 100% viral inhibition being required in high amounts, ineffective after a short time, and hazardous to public health and the environment. It has been reported that nanostructure coated surfaces reduce SARS-CoV-2 viability up to more than 90% in 10 min to 2 h (Hasan et al., 2020). Consequently, metallic nanoparticles (silver, copper, and TiO2 nanoparticles) are used as an alternative because of their broad range of antiviral activity, persistence, and effectiveness for a long time at a low dosage (Talebian et al., 2020). These metal nanoparticles are toxic to the pathogen. As discussed earlier, they inhibit microbes directly or through the generation of ROS by disrupting proteins, RNA, intracellular organelles or cell walls, and cell membranes. Among metals, silver is the most used antimicrobial; its bactericidal mechanism is based on the binding of silver atoms with thiol (SH) and disulfide (S-S) groups present in bacterial cell membrane proteins, resulting in membrane disruption and eventual necrobiosis. However, many reports have proved its antiviral effectiveness against several human pathogens such as influenza virus, hepatitis B virus (HBV), norovirus, and SARS-CoV. In a study, AgNPs used in polycotton fabrics inhibited SARS-CoV-2. Several Ag-based sanitizers and disinfectants have also been introduced to sanitize hands and inanimate surfaces (Ghedini et al., 2021). Copper (Cu) has also been considered the best antimicrobial metal. Poggio et al. (2020) studied an antimicrobial potential of a range of copper alloys to inhibit the transmission of Human Coronavirus 229E. The virus inactivation occurs on brass and copper-nickel surfaces at room temperature due to the release of copper ions and the generation of ROS.
Similarly, ZnO-NPs cause cleavage of oligosaccharides from the glycan shield by hydroxyl radicals and ROS-mediated degradation of viral entry. However, due to the high cytotoxicity of ZnO-NPs, it would be best suited to use them as a surface disinfectant to minimize the interaction of the virus with human cells (Lin et al., 2021). Moreover, research has shown to inactivate the SARS-CoV-2 alpha variant (as a VOC) only after 1 min of contact with surfaces by copper-silver (Cu-Ag) nanocomposites. Testing these nanomaterials on three different surfaces showed that Cu (∼56, ∼59, and ∼48 wt%, respectively) is most effective, followed by Ag content (∼28, ∼13, and ∼11 wt%, respectively). These findings suggest that the administration of this nanocomposite as surface disinfectant in highly crowded places (e.g., schools, public transportation, public toilets, hospital, and live-stock reservoirs) potentially halt the spread of the SARS-CoV-2 virus (Mosselhy et al., 2022).
The pathogenicity and virulence factor of viruses can be determined by their survival rate in the environment and their ability to transmit to humans and animals without a host cell. In healthcare centers, virus containing waste is discarded in the environment after using PPE and other equipment, which can again transmit to humans and animals through air and water. Many treatment strategies are used to inactivate the virus. However, studies have shown that the pathogen fragments remained in effluents. The applications of nanotechnology could prove worthwhile in these cases. The potential of nanotechnology in combination with solar-based disinfection is a novel approach to inactivate human pathogens. ZnO nanoparticles have been used for wastewater disinfection, and they are reported to be more efficacious (Al-Gheethi et al., 2020). TiO2 nanoparticles possess the potential to decontaminate air filtration systems, aerosols, surfaces, and water by inactivation viral propagation. Due to their photocatalysis mechanism, the electrons are excited from the valence band to the conduction band that initiates the reaction and releases ROS, such as hydroxyl radical and superoxide anion, which gives TiO2 its disinfectant properties against viruses (Magalhaes et al., 2017). Activated carbon nanomaterials could control the variants of SARS-CoV-2 because of their superior ability to capture viruses. Commercially available powdered activated carbons capture viral particles in their nanopores and eliminate them through hydrophobic interactions with the virus surface to remove them from the environment. These findings indicate a good strategy for the controlled elimination of SARS-CoV-2, which can spread through aqueous media (Ruiz-Hitzky et al., 2020).
Similarly, an efficient nano-enabled photoelectrochemical oxidation is considered an excellent choice to provide virus-free air. This air purifier can trap indoor microorganisms (bacteria and viruses, including coronaviruses), molds, allergens, and other air pollutants. It is observed that after testing this technology, it is found to be more efficient as an air purifier (Kaushik and Dhau, 2022).
In achieving an effective response to sudden pandemics of such as COVID-19, significant challenges remain. Although, the considerable success of delivering conventional viral vaccines could generate solid antiviral responses. Due to concerns about traditional vaccines, like weak immunogenicity, toxicity, intrinsic instability in vivo, and the need for multiple administrations, improvements and further progress are required (Kim et al., 2014; Huang et al., 2021). The use of nanotechnology in the production of vaccines gives another solution to these problems (Abd Elkodous et al., 2021a). Nanoparticle-based antigen delivery has many advantages over traditional vaccine delivery systems, such as safe delivery vehicles, vaccine adjuvant, improved antigen stability, targeted delivery, long-time controlled release, and evasion of immune responses. Nanotechnology-based vaccines are easier to design, synthesize, and scale up in a larger volume than traditional vaccine approaches (Malabadi et al., 2021).
To enhance the humoral and cellular immune responses, nanocarrier-based delivery systems provide opportunities to overcome such problems. Numerous vaccine nanocarriers have been designed and investigated to promote a protective immune response for the utility of antigens and adjuvants to immune cells. For proper vaccine delivery via oral or mucosal routes, solid nanocarriers can facilitate entry into the gut-associated lymphoid tissue and mucosa-associated lymphoid tissues and protect protein-based antigen vaccines from degradation (Kim et al., 2014). Nanoparticles have been developed with diverse functions by taking advantage of pathogen-adopted specific delivery and translocation mechanisms known as bioinspired or biomimetic nanoparticles with good biocompatibility, extended circulation time, and enhanced accumulation at the infection sites. To design more effective vaccine formulations, biomimetic nanoparticles have unique immunostimulatory properties and antigenic characteristics. MERS-CoV-specific IgA cannot persist for an extended period while protecting patients with the infection. The delivery of biomimetic nanoparticles protects the adjuvant from degradation. Also, it protects the body from systemic toxicity caused by free adjuvants, which could cause side effects such as nausea, fever, drowsiness, and diarrhea (Huang et al., 2021). Stable, effective, and target-specific delivery of an antigen can be generated through multifunctional nano vaccines that can significantly increase the immune response. However, production costs and manufacturing processes can be improved for many-component nano-vaccines with complex structures. To enhance the immunogenicity of a vaccine antigen, nanotechnology-based vaccine delivery systems have been developed by modulating antigen delivery to the immune cells (Borges et al., 2005).
Working mechanisms of nanotechnology-based vaccine formulations have supported the utility of nanocarriers in vaccination. Phagocytic cells, such as macrophages and dendritic cells (DC), readily take up particles smaller than 10 μm. The efficiency of antigen recognition and presentation has improved the cellular uptake of antigens through this property (Oyewumi et al., 2010). To eradicate SARS-CoV-2 completely and thus reduce the risk of emergence of VOCs, immediate control measures in terms of vaccination are essential. This crisis also demands an urgent analysis of all the available nanotechnology tools. For designing the vaccine carrier, nanomedicine strategies are used. Since nanomaterials are ideal for antigen delivery as adjuvants, nanotechnology benefits the modern vaccine design and mimics the structural features of viruses. In the smooth delivery of the antigen into the host cell, nanoparticles help trigger antigen-specific immune responses. Antigens can either be encapsulated inside the nanocarriers or bound (conjugated) to the surface of the nanoparticles and administered together with the adjuvant to the target (Malabadi et al., 2021).
The key target for vaccine development, therapeutic antibody generation, and the clinical diagnosis of COVID-19 is spike S protein (glycoprotein). S protein helps the virus to enter the target cells. Still, this endocytosis simultaneously depends on the initiative activation of S protein by cellular proteases and the binding of S protein to membrane ACE2 receptors. Therefore, a vaccine against the S protein prevents the proliferation and spread of SARS-CoV-2 (Huang X. et al., 2022). In current clinical trials for SARS-CoV-2, nanotechnology-based vaccines are the leading alternative to viral vector vaccines. The recent support of two lipid nanoparticle-based vaccines has demonstrated that nanomedicine can be an important asset in controlling VOCs. The delivery of drugs and molecules is facilitated by nanomedicine that would otherwise be useful or viable therapeutics (Milane and Amiji, 2021).
The prefusion spike protein (Pre) of SARS-CoV-2 used in vaccines neutralizes SARS-CoV-2 variants but elicits less antibody-dependent cellular cytotoxicity activity than S2 proteins. S2 proteins induce IgG antibodies with potent and broad antibody-dependent cellular cytotoxicity (ADCC) activity but weaker neutralization. The immunogenicity of S2 and Pre has been shown to improve by incorporating them into double-layered protein nanoparticles. The resulting protein nanoparticles Pre/S2 elicit higher neutralizing antibodies than Pre alone and stronger ADCC than S2 alone (Ma et al., 2022).
Viruses primarily enter our bodies through respiratory droplets. To stop its transmission, it is essential to stimulate mucosal immunity. Inhalable bionic virus nano-vaccines have been used to raise respiratory mucosal immunity to prevent the virus. This nano-vaccine structure is like SARS-CoV-2, consisting of nucleic acid, capsid, and spike protein. When administered through the nasal route, it activates cytokines and helps the receptor binding domain to mature helper T cells and B cells (Zheng et al., 2021). Nasally administered hACE2 mice had higher mucosal immunity response capacity and produced high titer sIgA against SARS-CoV-2 in the respiratory mucosa compared to mice that received the vaccine intramuscularly and intraperitoneally. The outcomes of the virus challenge experiment showed that the inhalable nano-vaccine approach could effectively protect the body (Huang J. et al., 2022).
Similarly, the efficacy of oral vaccines based on self-replicating RNA lipid nanoparticles has been tested against SARS-CoV-2 variants (alpha and delta, i.e., B.1.1.7 and B.1.617, respectively). The study reported that a high quantity of SARS-CoV-2 specific IgG and IgA antibodies were observed after administering RNA LNPs-based vaccines (Figure 2). It is reported to simultaneously neutralize the alpha and delta variants of SARS-CoV-2 (Mohammadi et al., 2021).
FIGURE 2. Illustration of different types of nanomaterials that could help in eliciting a strong immune response against SARS-CoV-2.
Although vaccines against SARS-CoV-2 have been successfully established, the emerging VOCs are still a challenge in their complete prevention. Nanotechnology applications for developing vaccines are a way forward with efficient vaccines. As discussed, the application of nanomaterials to the development of vaccines could cause the direct and indirect spread of the disease and, thus, the emergence of VOCs (Kamat et al., 2021; Mohamed et al., 2022).
Diagnostics proved critical in containing COVID-19 and allowed rapid deployment of control measures to prevent virus transmission by tracing and isolating people (Chau et al., 2020). Researchers have always aimed to develop highly sensitive and effective techniques for identifying pathogens (Table 2). Nano-sized materials can initiate highly effective surface contacts between the analyte and the sensor used for detection resulting in rapid, reliable, and accurate virus detection. Nanomaterials are also beneficial for the bioconjugation of molecules, plus high surface energy. Nanotechnology is expected to improve cellular and subcellular diagnostics using high-throughput sensors and imaging techniques (Kamat et al., 2021).
TABLE 2. Different types of nanomaterials and their diagnostic applications in detecting SARS-CoV-2.
Biosensors have been created to detect human immunodeficiency virus, influenza, and other viral infections (Misra et al., 2022). Initially constrained by poor sensitivity and specificity, nanotechnology significantly influences biosensors because the search to produce a portable, quick, low-cost, and multiplex device is crucial. Nanotechnology makes detecting many analytes easier, mass transferring information across short distances and tailoring sensing of a wide range of analytes for fast action (Pradhan et al., 2021). Moutaouakil et al. (2020) hypothesized that the integration of nanomaterials into biosensors would improve rapid detection, specificity, sensitivity, integration of nanoscale measurements, and new label-free detection techniques due to their unique optical, physical, mechanical, and electrical properties. Nano-based biosensors have the advantage of biocompatibility and compactness. The fascinating optical properties of nanoparticles, such as surface plasmon resonance, are used in diagnostics to enhance electromagnetic radiation phenomena (absorption and scattering). Similarly, quenching, and fluorescence features of graphene, silver, gold, and other metal oxides-based nanomaterials are features commonly used in biosensors (Yildiz et al., 2021).
For instance, graphene-based biosensors are ideal for various applications due to their high electrical conductivity and surface area. They can be used to detect the coronavirus S antibody (Özmen et al., 2021). Similarly, when AuNPs and AgNPs linked to antibodies attach to RNA or viral antigens, a detectable signal can identify SARS-CoV-2 (Maddali et al., 2021). Furthermore, toroidal plasmonic meta-sensors that detect viral protein S concentrations in the femtomolar range have been produced. Monoclonal antibodies conjugated to functionalized AuNPs have been seen at dosages of up to 4.2 fmol (Ahmadivand et al., 2021).
Researchers have recently developed a one-step Protein-S-specific nano-plasmonic resonance optical sensor that detects viruses quickly and directly with little sample preparation. Antibodies highly specific against SARS-CoV-2 have been restrained on surfaces of nano-sensor chips to which whole coronavirus particles adhere via protein S, resulting in plasmon resonance or intensity changes that can be monitored optically with a detection device (Rhouati et al., 2021). Nano-plasmonic sensor chips offer the advantage of being inexpensive and scalable while maintaining consistency and repeatability. The flexible and non-contactable nature of the nanostructures allowed the researchers to integrate the sensors into a standard 96 microplate or a microfluidic cuvette, which enables them to be used for measurements (Iravani, 2020).
Similarly, a wearable device using AuNP-based sensors combined with artificial intelligence that can be controlled using a smartphone app can detect SARS-CoV-2 in just 15 min in exhaled breaths. This detection identifies viruses based on variations in the resistivity of the nanomaterial biosensor layer (Torrente-Rodríguez et al., 2020). Plasmonic detection technology can significantly reduce the frequency of false positives. A clinical diagnostic test was created by the researchers having a dual function like a surface plasmon resonance sensing transduction and plasmonic photothermal. The experiments take place on two-dimensional gold nano-islands. SARS-CoV-2 nucleic acids hybridize to complementary DNA receptors on gold Nano islands. This device can be stimulated with different wavelengths because of two separate angles of incidence: one from localized surface Plasmon resonance and the other from a plasmonic photothermal biosensor. It can recognize the envelope genes (E) of RdRp-COVID, F1ab-COVID, and SARS-CoV-2. The dual-purpose localized surface Plasmon resonance biosensor can identify specific SARS-CoV-2 sequences in a multigene mix and has a detection limit of 0.22 p.m. (Qiu et al., 2020).
Researchers have also created a plasmonic nano-hole array that transmits light for label-free pathogen detection in biological conditions without sample preparation. Capturing intact virions on immobilized group-specific antiviral immunoglobulins on the sensor surface allows you to quantify them. The virus attaches to a suspended Nano hole network that connects incident light to surface Plasmon’s, causing the surface Plasmon resonance frequency to shift red (Kevadiya et al., 2021). Silicon nanowires are being developed as ultra-sensitive mark-free sensor technology. After being modified with antibodies, silicon nanowires transistors can detect a single virus (Preetam et al., 2022).
Layqah and Eissa (2019) developed an immune sensor using AuNPs coated with carbon electrodes. In this method, the spike protein of COVID-19 acts as an interactive bridge between the virus and the small portion of antibodies present in the sample. At the same time, the actual differences are used for virus identification. If the presence of the virus is negative in the sample, the binding of the antibody to the SI protein induces the generation of a very low current spike. If the presence of the virus is positive, a high current spike is generated. However, the detection limit ranges between 0.4 and 1.0 pg/ml. Ishikawa et al. (2009) employed nanowires instead of nanoparticles to detect SARS-CoV-2.
Chiral biosensors have been suggested as a vital tool for diagnosing SARS COVID-19 virus. A study reveals that the COVID-19 virus was revealed by opening chiral zirconium quantum dots. When the virus is present, magnetic nanoparticles and zirconium quantum dots interact, resulting in magneto plasmonic light. The virus was identified by measuring the fluorescence intensity of fluorescent magneto Plasmon monohybrids separated by external magnets (Asdaq et al., 2021). Another study employed chiral immune sensors made of self-assembled layers of quantum dots and chiroplasmic AuNPs. COVID-19 can be identified in blood samples by mixing quantum dots and AuNPs with the viral sample; modifications of the chiral optical response are disclosed using the circular dichroism technique, which has a low detection limit (47.91 EID/50 L) (Ahmed et al., 2017). Overall, biosensors and other materials science-based detection techniques have the potential to provide rapid and portable SARS-CoV-2 diagnostic tests.
Diagnostic tests for coronaviruses have used metallic, polymeric, silica, iron oxide, and quantum dot nanoparticles. Among these, AuNPs are by far the most commonly used metal systems. These nanoparticles are broadly used because their surface plasmons have the characteristic wavelength-selective absorption with relatively high molar extinction coefficients. An AuNP-based colorimetric assay was developed and complemented with thiol-modified antisense oligonucleotides with sufficient specificity for the SARS-CoV-2 N-gene. As a result, it demonstrated the possibility of selective and rapid (10 min) diagnosis of COVID-19 with the naked eye (Kevadiya et al., 2021). When the SARS-CoV-2 target RNA sequence was present, thiol-modified ASOs covered the AuNPs aggregate, and a change in surface plasmon resonance was observed. However, the amount of virus injected may affect the test (Moitra et al., 2020). As in a colorimetric assay, SARS-CoV protein E was immobilized on AuNPs using an Au-binding polypeptide that plays a role of a mediator. These AuNPs were also functionalized using a GFP, and when they interacted with a complementary protein antibody, they changed their color and absorbance, allowing measurable detection of SARS-CoV (Rasmi et al., 2021). AuNPs with a diameter of 17 nm have also been used in a biosensor to detect SARS-CoV based on fluctuations in fluorescence (Gao et al., 2021). The colloidal gold immunochromatography test is a simple and rapid screening method widely used in various disciplines to diagnose diseases. It has several advantages, including speed, affordability, and simplicity. Serologic point-of-care (POC) diagnostics are based on lateral flow immunoassay (LFIA) methods (Figure 3). The FDA has approved many LFIA-based POC diagnostics (Wang et al., 2021a). One of these tests is Cellex Inc.’s COVID-19 IgM/IgG rapid test, which allows for the identification and qualitative differentiation of IgM and IgG antibodies against SARS-CoV-2 in 15–20 min (Mahmoudinobar et al., 2021).
The nucleic acid of SARS-CoV-2 is detected using real-time RT-PCR. However, the RT-PCR diagnostic kit provides a wide range of false positive or negative results. Nanomaterial-based technology offers viable alternatives to RT-PCR for rapid and accurate virus detection (Engelmann et al., 2021). For instance, 3 aminopropyl triethoxysilane and coprecipitation of magnetic nanoparticles can be employed to simplify the extraction of viral RNA and the functionalization of polyamine ester, which could then be used in as many as 50,000 diagnostic tests (Chacón-Torres et al., 2020).
To identify the genetic sequences of SARS-CoV, silica-coated superparamagnetic nanoparticles were used at different stages of polymerase chain reaction (PCR) experiments (Gong et al., 2008). A recent work uses polystyrene nanoparticles doped with lanthanides that produce fluorescence capable of detecting the presence of viruses, the results obtained by RT-PCR (Maddali et al., 2021).
Chacón-Torres et al. (2020) examined magnetic nanoparticles by isolating SARS-CoV-2 virus RNA using RT-PCR techniques. These nanoparticles employ polymers to capture RNA on their surfaces through carboxyl groups (amino ester). The carboxyl group is employed in a combination of RNA magnetic nanoparticles for RT-PCR diagnostics. This process takes lesser time and is relatively safe. In another study, magnetic nanoparticles were used to isolate virus particles rather than extraction. The findings show that virus particles bind tightly to nanoparticles through functionalized targeting receptors. Superparamagnetic iron oxide nanoparticles were used because their external magnet helps separate virus particles (Vahedifard and Chakravarthy, 2021). Zhu et al. (2020a) used one-step reverse transcription loop-mediated isothermal amplification conjugated with nanoparticle-based biosensors in a single-tube reaction to diagnose COVID-19 accurately and quickly. The diagnostic system produced has a sensitivity of 12 copies per reaction and no cross-reactivity with templates other than COVID-19. In oropharyngeal swab specimens from COVID-19 patients, the developed RT-LAMP-NBS has a sensitivity and specificity of 100%. Conclusively, nanomaterials used in PCR experiments reduce reaction time, increase signal amplification selectivity, and improve detection sensitivity.
When a new viral disease emerges, no vaccine is available for it immediately, and people have to rely on available antiviral therapeutics, which are effective initially but can also cause adverse effects that outweigh the benefits (Zhou et al., 2021). Since the epidemics of coronaviruses such as SARS-CoV and MERS-CoV, two decades have passed, but still, no efficient drug against coronavirus has been approved so far. For this purpose, nanotechnology opens new avenues that have converged enormous attention and have already been studied for its potential applications in treating viral infections, including COVID-19 (Table 3) (Campos et al., 2020; Vahedifard and Chakravarthy, 2021). This section focuses on the potential of nano-based treatment strategies to fight several viral infections, emphasizing SARS-CoV-2-associated COVID-19 and its emerging variants.
Many antiviral drugs have been developed to treat various viral infections. Though they possess good antiviral activity, several challenges limit their efficacy, including the emergence of drug-resistant viruses, poor solubility, instability during storage and application, low bioavailability, and potential adverse side effects (Delshadi et al., 2021). Nanomaterials can play an essential role in nano-medicine due to their size, shape, and surface charge, which enable them to efficiently deliver therapeutic agents such as drugs (precisely at the proper target and time) and vaccines. Their surface functionalization and size allow them to have antigens and adjuvants for immunization (Gurunathan et al., 2020). The nano-delivery system solves the problems associated with traditional drugs because it enhances the bioavailability, reduces toxicity, protects the drug from in vivo degradation, extends circulation time, increases the drug’s half-life, and helps it cross biological barriers. The known nano-carriers for antiviral drug delivery are polymeric nanoparticles, metallic nanoparticles, liposomes, dendrimers, micelles, and nanocrystals (Govender et al., 2008; Jindal and Gopinath, 2020).
Polymeric nanoparticles are considered efficient drug carriers because of their high surface area and versatile conjugation properties (Charelli et al., 2022). The biodegradability and small size of polymeric nanoparticles allow them to be used as colloidal drug carriers because they can easily penetrate and absorb the cells and enable the continuous release of the drug to the target site after days or even weeks of administration (Khalil et al., 2011). Chitosan is a natural biocompatible polysaccharide often utilized as a nano-carrier of antiviral medications. Ramana et al. (2014) employed chitosan nanoparticles to load the antiretroviral saquinavir (the most effective medicine for AIDS). They found a drug loading efficiency of 75% and target specificity of more than 92%. It can release antiviral drugs through swelling, diffusion, and erosion mechanisms. The chitosan nanoparticles take advantage of a large surface area in pulmonary drug delivery and directly deliver drugs to the infected lungs of the patient (Chowdhury et al., 2021). In a recent study for the treatment of COVID-19, Tan et al. (2021) developed a nano-carrier drug delivery system by loading lopinavir in PLGA (poly lactic-co-glycolic acid). They then coated the nanoparticles with macrophage membrane to form drug-loaded macrophage biomimetic nanocarriers (PLGA-LPV@M). This nano-carrier relieved inflammation by specifically targeting inflammation sites and neutralizing pro-inflammatory cytokines, macrophages, and neutrophils.
It also reduced viral load (Tan et al., 2021). To treat COVID-19, administering antiviral drugs directly to the lungs is required as it is the primary site of infection, and most deaths are associated with malfunctioning lungs. Li et al. formed liposomal aerosol for the pulmonary delivery of remdesivir to enhance its in vivo activity. The encapsulation of remdesivir in liposomes showed high solubility and improved biocompatibility, thus indicating it to be a better option to enhance in vivo therapeutic effects for COVID-19 and similar respiratory infections (Peraman et al., 2021). Various in vitro and in vivo studies revealed the increased bioavailability of drugs in the form of liposomal nano-formulations across the barrier of the mucosal membrane. FDA-approved drugs like doxorubicin and amphotericin B are available in this form (Higgins et al., 2020).
Metallic nanoparticles like silver (Ag), gold (Au), titanium (Ti), zinc (Zn), palladium (Pd), and copper (Cu) have been widely used as nanocarriers for therapeutic agents such as antibodies, drugs, genes, peptides, etc. Due to the attractive intermolecular forces between nanoparticles and target drug molecules, the surface of metallic nanoparticles can be easily functionalized to be utilized as an effective carrier of therapeutic drugs (Chandrakala et al., 2022). Metal nanoparticles synthesized from plant extract are desirable for their application in drug delivery because of their reduced toxicity, scalability, and cost-effectiveness (Mehta et al., 2020). Quantum dots (QDs) are nanocrystals with a high binding affinity with therapeutic molecules and target specificity. Some studies showed the target-specific delivery of saquinavir when conjugated with QDs and target DNA, making it an efficient antiretroviral therapeutic agent (Mukherjee et al., 2020). Single-walled carbon nanotubes (CNTs) have been tested for their drug-carrying capability in fish models. CNTs functionalized with ribavirin showed increased drug uptake and reduced significant therapeutic dosages. Hence CNTs can be employed as nanocarriers for targeted drug delivery (Neghab et al., 2020). Small interfering RNAs (siRNAs) are broad-spectrum antiviral agents that can be designed to degrade the target viral genome. Encapsulation of siRNA in FDA-approved nanomaterials like lipid nanoparticles (LNPs), nano polymers, or lipid-polymer hybrid can be used for the stable and target delivery of siRNA for the degradation of the SARS-CoV-2 viral genome (Ullah et al., 2020).
The development of a novel drug delivery system has envisioned researchers repurposing the drugs (during the unavailability of specific therapeutic agents) effectively for the current and future outbreaks. The developed nano designs for drug delivery can be active against various viral diseases crossing the barriers of patients’ heterogeneity. Polymeric, lipid-based, and inorganic nanoparticles are designed in specific ways to carry antiviral drugs in a personalized manner, which can potentially be applied to the population to treat existing and possibly emerging viral diseases (Mitchell et al., 2021).
After reaching the target site, the first step of SARS-CoV-2 is the attachment and entry into the host cell. This attachment and access are facilitated by viral spike glycoproteins targeting the ACE2 receptor in the lungs, heart, kidneys, testis, and intestine cells. Blocking the entry of the virus in the first place is the ideal treatment strategy that can be obtained by applying nanomaterials. The size and surface charge of nanomaterials enable them to attach to the spike protein preventing its binding to the target receptor, thus blocking the initiation of infection (Carvalho and Conte-Junior, 2021). Nanoparticles can directly control the entry of viruses just like AgNPs can inhibit the entrance of respiratory viruses; graphene can hinder the attachment of HIV to the host cell; AgNPs conjugated with graphene oxide (GO) have shown effective activity against feline coronavirus and other enveloped viruses (Chintagunta et al., 2021). Nanomaterials like AuNPs and carbon quantum dots, due to their large surface area to volume ratio, can interact with many biomolecules, including the spike protein of SARS-CoV-2 preventing its entry into the cells (Figure 4). AuNPs mimic a binding receptor of viruses like herpes simplex virus, lentivirus, and human papillomavirus resulting in viral degradation. A study revealed that GO could inactivate viruses before entering the cell. The spike glycoproteins of the viral envelope were destroyed when incubated with GO for 1 h (Vahedifard and Chakravarthy, 2021). AgNPs are also evaluated for their antiviral activity to inhibit SARS-CoV-2. Luciferase-based pseudovirus entry assay showed that 10 nm sized AgNPs in the concentration of 1–10 ppm blocked the entry of this virus by disrupting its integrity (Jeremiah et al., 2020). A docking study revealed the binding of iron oxide nanoparticles with the S1 receptor binding domain, preventing the adsorption of SARS-CoV-2 to the host cell (Chue-Gonçalves et al., 2021).
In addition to the interaction with spike protein, the entry of SARS-CoV-2 into the host cell can be blocked by inhibiting the ACE2 receptor. The studies of human and mouse cell lines showed that DX600 is a specific and effective human ACE2 inhibitor. DX600, in conjugation with nano-copolymer like polylactic-co-glycolic acid (PLGA) and polyethylene glycol (PEG), would be able to reach and inhibit targeted ACE2 receptors (Neghab et al., 2020). The interaction of GO with spike protein, ACE2, and their complex has been studied in a molecular docking analysis which showed the high affinity of GO for both receptor and viral protein. This study revealed the capacity of GO sheets to block infection by disrupting viral spike proteins even with any mutations in them (Unal et al., 2021).
Once SARS-CoV-2 is attached and entered into the host cell, it releases the RNA genome into the cytoplasm, translating it into proteins in viral replication (Harrison et al., 2020). To stop the virus’s progress from spreading infection, inhibiting viral genome replication is one of the most successful steps. For this purpose, nanoparticles are desirable antiviral agents that can be designed to help them attach and penetrate the target cell and either directly or indirectly inactivate the virus by blocking its replication (Pilaquinga et al., 2021). The metal and mineral nanoparticles can block various structural targets of SARS-CoV-2 to prevent its replication (Sarkar and Das Mukhopadhyay, 2021). AgNPs have been studied as antiviral agents against many life-threatening viruses and have been revealed to be efficient therapeutic agents having the potential to treat other diseases. AgNPs have shown the inhibition of HIV1 by binding to gp120, preventing the virus’s entry and replication by blocking the formation of a complex between virion and CD4. Also, AgNPs have been discovered by Lu and coworkers to stop the production of intracellular HBV RNA. Due to the successful results, AgNPs are suggested by many studies to be employed for the treatment of COVID-19 (Siadati et al., 2020). The antiviral mechanism of AgNPs involves the production of reactive oxygen species (ROS), leading to oxidative stress that damage the viral genome and proteins, initiate an immunological response, and prevent the viral RNA synthesis by blocking the attachment of the virus to its receptor (Das et al., 2020). In a recent study, AgNPs (10 nm) coated with polyvinylpyrrolidone applied in vitro to Vero cells infected with SARS-CoV-2 showed almost complete inhibition of viral replication with low toxicity (Jeremiah et al., 2020). Mesoporous silica nanoparticles (SiNPs) can interact with several ligands to stop the virus’s entry and inhibit its replication (Asdaq et al., 2021).
Zinc ionophores can block the replication of the viral genome by inhibiting RNA-dependent RNA polymerase (RdRNP) by releasing higher concentrations of Zn2+ ions. Metal nanoparticles can produce an increased level of ROS that can penetrate the cell membrane and interfere with RNA and protein synthesis, thus hindering post-attachment viral replication (Sarkar and Das Mukhopadhyay, 2021). A recent molecular docking study has been performed to determine the interaction of CNTs and fullerenes with the significant structural targets of SARS-CoV-2 like RdRNP, main protease, papain-like protease, and nucleocapsid protein containing RNA binding domain. All these proteins play an essential role in the transcription and replication of the viral genome. This study showed the strong binding affinity of CNTs and fullerenes with all the tested targets presenting it as a potential antiviral agent against SARS-CoV-2 and similar viruses (Skariyachan et al., 2021). Ag-TiO2 single-atom nanozyme (SAN) has been studied as an antiviral agent in transgenic mice against SARS-CoV-2. The study revealed the adsorption capacity of Ag-TiO2 SAN to the spike protein leading to phagocytosis of the virus-AgTiO2 complex, thus blocking the replication of the virus (Wang et al., 2021b). A study presented nano-formulations against coronavirus in four types: the encapsulation of antiviral dyphylline in PEG-PLGA, graphene oxide sheets functionalized with AgNPs, Ag2S nanoclusters, and Ag nanowires. All these types have shown an effective role against coronavirus by preventing its replication, blocking cellular endosomal acidification, and synthesis of viral RNA. Dyphylline is an ATPase blocker that showed replication inhibition of coronavirus in fcwf-4 cells in nano-formulation. For the treatment of COVID-19, the nanomaterials mimicking ACE2 receptors like AuNPs would be efficient as this receptor is responsible for the internalization and replication of SARS-CoV-2. The binding of AuNPs to the virus would potentially block its propagation in the cells (Alphandéry, 2020).
Nanotechnology can be phenomenal in developing new strategies to tackle the emerging variants of SARS-CoV-2. The SARS-CoV-2 variants that have emerged because of the virus’s S protein’s ongoing changes have aggravated and prolonged the current pandemic, which the original SARS-CoV-2 first caused. Compared to earlier COVID-19 waves, the Omicron form has decreased hospitalization and mortality rates, despite its improved viral transmission and immune evasion (Bhattacharyya and Hanage, 2022). For this purpose, Johnson & Johnson, the two existing mRNA vaccine producers (Moderna and Pfizer-BioNTech), and the development of novel Omicron variant-specific vaccinations have all been disclosed (Thakur and Ratho, 2022). However, the effectiveness of these vaccinations has declined, considering the new SARS-CoV-2 variants. The Food and Drug Administration has consequently declared that these establish guidelines for expediting the assessment of updated vaccinations against specific variants. These variants can potentially be defeated by using innovative nanotechnology-based techniques that target the altered S protein of the virus. Due to their higher initial efficacy than traditional vaccinations, nanotechnology-based vaccines can still protect people from these variants. In addition, they can be quickly and easily updated to increase their effectiveness against the SARS-CoV-2 variants. This means that developing next-generation vaccines against SARS-CoV-2 variants will significantly aid nanotechnology-based solutions’ extraordinarily high efficacy and rapid production cycle times. To create innovative nanotechnology-based therapies to battle SARS-CoV-2 variations, a range of nanostructures (for instance, DNA origami) that directly capture variants or accurately display neutralizing antibodies or ACE2 receptors on their surfaces might be modified. We anticipate that, despite the COVID-19 pandemic’s continuous nature and the SARS-CoV-2 variants’ quick worldwide spread, nanotechnology’s innovations and advancements will enable various strategies to speed up the pandemic’s termination.
Some gaps need to be filled to make nanotechnology-based solutions to SARS-CoV-2 variants more effective. To this end, the pharmaceutical industry must work on research nano therapies to treat COVID-19, considering the potential presented by nanotechnology, nanomedicine, and biotechnology. From a pharmaceutical standpoint, developing stimuli-responsive, immune-supportive, and immunomodulating agents using nano-pharmacology principles, designing stimuli-responsive, immunomodulating, and immune-supportive antimicrobial/antiviral therapeutic agents with better efficacy and fewer side effects, optimizing dosages and delivery systems for carriers and targets and investigating biocompatible, bio-functionalized, nano drug loading systems are some of the undeveloped areas that need to be considered.
Similarly, there is an inherent risk associated with using nanostructures. The safety-to-risk ratio of nanostructures should be increased so that the present pandemic issue might be seen as an excellent chance to revolutionize nanomedicine. In-depth research, experience sharing, and information exchange across many businesses, regulatory bodies, and, ultimately, different nations are necessary for the ultimate result. Developing early-stage regulatory guidelines and conducting studies on opportunities and barriers to realizing nanotechnology-based solutions to SARS-CoV-2 variants is essential. This way, nanotechnology can help the world’s medical community to fight current and subsequent pandemics effectively (Singh et al., 2021).
Finally, advanced machine learning and Artificial intelligence are other ways forward for proper and effective modeling and interpretation of cell-nanomaterial interactions, which are required to formulate quantitative nanostructure activity effectively. The effective use of nanomedicine in the pandemic can be further complemented by other related technologies like robotics, telemedicine, and 3D printing (Miyazawa et al., 2021).
All authors listed have made a substantial, direct, and intellectual contribution to the work and approved it for publication.
The authors acknowledge the role of the Department of Biotechnology, University of Malakand for providing conducive environment for collaboration on this project.
The authors declare that the research was conducted in the absence of any commercial or financial relationships that could be construed as a potential conflict of interest.
All claims expressed in this article are solely those of the authors and do not necessarily represent those of their affiliated organizations, or those of the publisher, the editors and the reviewers. Any product that may be evaluated in this article, or claim that may be made by its manufacturer, is not guaranteed or endorsed by the publisher.
Abd Elkodous, M., El-Sayyad, G. S., Abdel-Daim, M. M. J. E. S., and Research, P. (2021a). Engineered nanomaterials as fighters against SARS-CoV-2: The way to control and treat pandemics. Environ. Sci. Pollut. Res. 28 (30), 40409–40415. doi:10.1007/s11356-020-11032-3
Abd Elkodous, M., Olojede, S., Morsi, M., and El-Sayyad, G. S. J. R. a. (2021b). Nanomaterial-based drug delivery systems as promising carriers for patients with COVID-19. RSC Adv. 11 (43), 26463–26480. doi:10.1039/d1ra04835j
Abdool Karim, S. S., and de Oliveira, T. (2021). New SARS-CoV-2 variants—Clinical, public health, and vaccine implications. N. Engl. J. Med. Overseas. Ed. 384 (19), 1866–1868. doi:10.1056/nejmc2100362
Ahmadivand, A., Gerislioglu, B., Ramezani, Z., Kaushik, A., Manickam, P., Ghoreishi, S. A. J. B., et al. (2021). Functionalized terahertz plasmonic metasensors: Femtomolar-level detection of SARS-CoV-2 spike proteins. Biosens. Bioelectron. X. 177, 112971. doi:10.1016/j.bios.2021.112971
Ahmed, S. R., Nagy, É., and Neethirajan, S. J. R. a. (2017). Self-assembled star-shaped chiroplasmonic gold nanoparticles for an ultrasensitive chiro-immunosensor for viruses. RSC Adv. 7 (65), 40849–40857. doi:10.1039/c7ra07175b
Al-Gheethi, A., Al-Sahari, M., Abdul Malek, M., Noman, E., Al-Maqtari, Q., Mohamed, R., et al. (2020). Disinfection methods and survival of SARS-CoV-2 in the environment and contaminated materials. a Bibliometr. Anal. 12 (18), 7378.
Almanza-Reyes, H., Moreno, S., Plascencia-López, I., Alvarado-Vera, M., Patrón-Romero, L., Borrego, B., et al. (2021). Evaluation of silver nanoparticles for the prevention of SARS-CoV-2 infection in health workers: In vitro and in vivo. PLoS ONE 16 (8), e0256401. doi:10.1371/journal.pone.0256401
Alphandéry, E. J. B. C. (2020). The potential of various nanotechnologies for coronavirus diagnosis/treatment highlighted through a literature analysis. Bioconjug. Chem. 31 (8), 1873–1882. doi:10.1021/acs.bioconjchem.0c00287
Asdaq, S. M. B., Ikbal, A. M. A., Sahu, R. K., Bhattacharjee, B., Paul, T., Deka, B., et al. (2021). Nanotechnology integration for SARS-CoV-2 diagnosis and treatment: An approach to preventing pandemic. Nanomater. (Basel). 11 (7), 1841. doi:10.3390/nano11071841
Awadasseid, A., Wu, Y., Tanaka, Y., and Zhang, W. (2021). SARS-CoV-2 variants evolved during the early stage of the pandemic and effects of mutations on adaptation in Wuhan populations. Int. J. Biol. Sci. 17 (1), 97–106. doi:10.7150/ijbs.47827
Aydemir, D., and Ulusu, N. N. (2020). Correspondence: Angiotensin-converting enzyme 2 coated nanoparticles containing respiratory masks, chewing gums and nasal filters may be used for protection against COVID-19 infection. Travel Med. Infect. Dis. 37, 101697. doi:10.1016/j.tmaid.2020.101697
Bayin, Q., Huang, L., Ren, C., Fu, Y., Ma, X., and Guo, J. J. T. (2021). Anti-SARS-CoV-2 IgG and IgM detection with a GMR based LFIA system. Talanta 227, 122207. doi:10.1016/j.talanta.2021.122207
Beck-Broichsitter, M., Merkel, O. M., and Kissel, T. J. J. o. c. r. (2012). Controlled pulmonary drug and gene delivery using polymeric. nano-carriers 161 (2), 214–224.
Bhattacharyya, R. P., and Hanage, W. P. (2022). Challenges in inferring intrinsic severity of the SARS-CoV-2 Omicron variant. N. Engl. J. Med. Overseas. Ed. 386 (7), e14. doi:10.1056/nejmp2119682
Borges, O., Borchard, G., Verhoef, J. C., de Sousa, A., and Junginger, H. E. (2005). Preparation of coated nanoparticles for a new mucosal vaccine delivery system. Int. J. Pharm. 299 (1-2), 155–166. doi:10.1016/j.ijpharm.2005.04.037
Campos, E. V., Pereira, A. E., De Oliveira, J. L., Carvalho, L. B., Guilger-Casagrande, M., De Lima, R., et al. (2020). How can nanotechnology help to combat COVID-19? J. Nanobiotechnology 18 (1), 125–223. doi:10.1186/s12951-020-00685-4
Carvalho, A. P. A., and Conte-Junior, C. A. J. G. c. (2021). Recent advances on nanomaterials to COVID-19 management: A systematic review on antiviral/virucidal agents and mechanisms of SARS-CoV-2 inhibition/inactivation. Glob. Chall. 5 (5), 2000115. doi:10.1002/gch2.202000115
Cavalcanti, I. D. L., and Cajuba de Britto Lira Nogueira, M. J. J. o. N. R. (2020). Pharm. Nanotechnol. which Prod. are been Des. against COVID-19? 22 (9), 1–11.
Chacón-Torres, J. C., Reinoso, C., Navas-León, D. G., Briceño, S., and González, G. J. S. r. (2020). Optimized and scalable synthesis of magnetic nanoparticles for RNA extraction in response to developing countries' needs in the detection and control of SARS-CoV-2. Sci. Rep. 10 (1), 19004–19010. doi:10.1038/s41598-020-75798-9
Chandrakala, V., Aruna, V., and Angajala, G. J. E. M. (2022). Review on metal nanoparticles as nanocarriers: Current challenges and perspectives in drug delivery systems, 1–23.
Charelli, L. E., de Mattos, G. C., de Jesus Sousa-Batista, A., Pinto, J. C., and Balbino, T. A. J. J. o. N. R. (2022). Polym. nanoparticles as Ther. agents against coronavirus Dis. 24 (1), 1–15.
Chau, C. H., Strope, J. D., Figg, W. D. J. P. T. J. o. H. P., and Therapy, D. (2020). COVID-19 clinical diagnostics and testing technology. Pharmacotherapy. 40 (8), 857–868. doi:10.1002/phar.2439
Chintagunta, A. D., Nalluru, S., and Ns, S. K. J. E. m. (2021). Nanotechnol. An Emerg. approach combat COVID-19 4 (1), 119–130.
Chowdhury, N. K., Choudhury, R., Sonawane, G. A., Mavinamar, S., Lyu, X., Pandey, R. P., et al. (2021). Nanoparticles as an effective drug delivery system in COVID-19. 143, 112162.
Chue-Gonçalves, M., Pereira, G. N., Faccin-Galhardi, L. C., Kobayashi, R. K., and Nakazato, G. J. N. (2021). Metal nanoparticles against viruses: Possibilities to fight. SARS-CoV-2 11 (11), 3118.
Cirrincione, L., Plescia, F., Ledda, C., Rapisarda, V., Martorana, D., Moldovan, R. E., et al. (2020). COVID-19 pandemic: Prevention and protection measures to be adopted at the workplace. Sustainability 12 (9), 3603. doi:10.3390/su12093603
Cleve, M. J. N. (2021). What the lightning-fast quest for Covid vaccines means for other diseases. 589, 16–18.
Cohen, A. A., Gnanapragasam, P. N., Lee, Y. E., Hoffman, P. R., Ou, S., Kakutani, L. M., et al. (2021). Mosaic nanoparticles elicit cross-reactive immune responses to zoonotic coronaviruses in mice. Science 371 (6530), 735–741. doi:10.1126/science.abf6840
Corey, L., Beyrer, C., Cohen, M. S., Michael, N. L., Bedford, T., and Rolland, M. (2021). SARS-CoV-2 variants in patients with immunosuppression. New England J. Medicine, 385 (6), 562–566.
Das, C., Paul, S. S., Saha, A., Singh, T., Saha, A., Im, J., et al. (2020). <p>Silver-Based nanomaterials as therapeutic agents against coronaviruses: A review</p>. Int. J. Nanomedicine 15, 9301–9315. doi:10.2147/ijn.s280976
Delshadi, R., Bahrami, A., McClements, D. J., Moore, M. D., and Williams, L. J. J. o. C. R. (2021). Development of nanoparticle-delivery systems for antiviral agents: A review. J. Control. Release 331, 30–44. doi:10.1016/j.jconrel.2021.01.017
Derakhshan, M. A., Amani, A., and Faridi-Majidi, R. J. A. a. m. (2021). State-of-the-Art Nanodiagnostics Nanotherapeutics against SARS-CoV-2 13 (13), 14816–14843.
Dhama, K., Patel, S. K., Kumar, R., Masand, R., Rana, J., Yatoo, M., et al. (2021). The role of disinfectants and sanitizers during COVID-19 pandemic: Advantages and deleterious effects on humans and the environment. Environ. Sci. Pollut. Res. 28 (26), 34211–34228. doi:10.1007/s11356-021-14429-w
Engelmann, I., Alidjinou, E. K., Ogiez, J., Pagneux, Q., Miloudi, S., Benhalima, I., et al. (2021). Preanalytical issues and cycle threshold values in SARS-CoV-2 real-time RT-PCR testing: Should test results include these? ACS Omega 6 (10), 6528–6536. doi:10.1021/acsomega.1c00166
Foffa, I., Losi, P., Quaranta, P., Cara, A., Al Kayal, T., D’Acunto, M., et al. (2022). A Copper nanoparticles-based polymeric spray coating: Nanoshield against Sars-Cov-2. J. Appl. Biomater. Funct. Mat. 20, 228080002210763. doi:10.1177/22808000221076326
Gao, Y., Han, Y., Wang, C., Qiang, L., Gao, J., Wang, Y., et al. (2021). Rapid and sensitive triple-mode detection of causative SARS-CoV-2 virus specific genes through interaction between genes and nanoparticles. Anal. Chim. Acta X. 1154, 338330. doi:10.1016/j.aca.2021.338330
Gellin, B., Modlin, J. F., and Breiman, R. F. (2001). Vaccines as tools for advancing more than public health: Perspectives of a former director of the national vaccine program office. Clin. Infect. Dis. 32 (2), 283–288. doi:10.1086/318461
Ghaemi, F., Amiri, A., Bajuri, M. Y., Yuhana, N. Y., and Ferrara, M. (2021). Role of different types of nanomaterials against diagnosis, prevention and therapy of COVID-19. Sustain. Cities Soc. 72, 103046. doi:10.1016/j.scs.2021.103046
Ghedini, E., Pizzolato, M., Longo, L., Menegazzo, F., Zanardo, D., and Signoretto, M. J. F. i. C. E. (2021). Which are the main surface disinfection approaches at the time of SARS-CoV-2? Front. Chem. Eng. 2, 589202. doi:10.3389/fceng.2020.589202
Gong, P., He, X., Wang, K., Tan, W., Xie, W., Wu, P., et al. (2008). Combination of functionalized nanoparticles and polymerase chain reaction-based method for SARS-CoV gene detection. J. Nanosci. Nanotechnol. 8 (1), 293–300. doi:10.1166/jnn.2008.18130
Gopal, V., Nilsson-Payant, B. E., French, H., Siegers, J. Y., Yung, W.-s., Hardwick, M., et al. (2021). Zinc-embedded fabrics inactivate SARS-CoV-2 and influenza A virus. bioRxiv. 2011. doi:10.1101/2020.11.02.365833
Govender, T., Ojewole, E., Naidoo, P., and Mackraj, I. J. D. d. (2008). Polymeric nanoparticles for enhancing antiretroviral drug therapy. Drug Deliv. (Lond). 15 (8), 493–501. doi:10.1080/10717540802321776
Guerrini, G., Magrì, D., Gioria, S., Medaglini, D., and Calzolai, L. J. N. N. (2022). Characterization of nanoparticles-based vaccines for COVID-19. Nat. Nanotechnol. 17 (6), 570–576. doi:10.1038/s41565-022-01129-w
Guo, J., Wang, Y., Niu, S., Li, H., Tian, Y., Yu, S., et al. (2020). Highly sensitive fluorescence-linked immunosorbent assay for the determination of human IgG in serum using quantum dot nanobeads and magnetic Fe3O4 nanospheres. ACS Omega 5 (36), 23229–23236. doi:10.1021/acsomega.0c02987
Gurunathan, S., Qasim, M., Choi, Y., Do, J. T., Park, C., Hong, K., et al. (2020). Nanomater. (Basel). 10 (9), 1645. doi:10.3390/nano10091645
Halim, M. J. J. C. M. R. (2021). A report on COVID-19 variants. COVID-19 Vaccines Impact Var. Efficacy Vaccines. 3 (3), 1–19.
Harrison, A. G., Lin, T., and Wang, P. J. T. i. i. (2020). Mechanisms of SARS-CoV-2 transmission and pathogenesis. Trends Immunol. 41 (12), 1100–1115. doi:10.1016/j.it.2020.10.004
Hasan, J., Pyke, A., Nair, N., Yarlagadda, T., Will, G., Spann, K., et al. (2020). Antiviral nanostructured surfaces reduce the viability of SARS-CoV-2. ACS Biomater. Sci. Eng. 6 (9), 4858–4861. doi:10.1021/acsbiomaterials.0c01091
Hasanzadeh, A., Alamdaran, M., Ahmadi, S., Nourizadeh, H., Bagherzadeh, M. A., Jahromi, M. A. M., et al. (2021). Nanotechnology against COVID-19: Immunization, diagnostic and therapeutic studies, 336, 354–374.
Hassanpour, M., Rezaie, J., Nouri, M., and Panahi, Y. J. I. (2020). Genetics, and EvolutionThe role of extracellular vesicles in COVID-19 virus infection. Infect. Genet. Evol. 85, 104422. doi:10.1016/j.meegid.2020.104422
Haynes, B. F., Corey, L., Fernandes, P., Gilbert, P. B., Hotez, P. J., Rao, S., et al. (2020). Prospects for a safe COVID-19 vaccine. Sci. Transl. Med. 12 (568), eabe0948. doi:10.1126/scitranslmed.abe0948
Henry, T., and Walke, M. (2021). SARS-CoV-2 variants of concern in the United States—challenges and opportunities.
Hewawaduge, C., Senevirathne, A., Jawalagatti, V., Kim, J. W., and Lee, J. H. (2021). Copper-impregnated three-layer mask efficiently inactivates SARS-CoV2. Environ. Res. 196, 110947. doi:10.1016/j.envres.2021.110947
Higgins, T. S., Wu, A. W., Illing, E. A., Sokoloski, K. J., Weaver, B. A., Anthony, B. P., et al. (2020). Intranasal antiviral drug delivery and coronavirus disease 2019 (COVID-19): A state of the art review. Otolaryngol. Head. Neck Surg. 163 (4), 682–694. doi:10.1177/0194599820933170
Huang, J. C., Chang, Y.-F., Chen, K.-H., Su, L.-C., Lee, C.-W., Chen, C.-C., et al. (2009). Detection of severe acute respiratory syndrome (SARS) coronavirus nucleocapsid protein in human serum using a localized surface plasmon coupled fluorescence fiber-optic biosensor. Biosens. Bioelectron. X. 25 (2), 320–325. doi:10.1016/j.bios.2009.07.012
Huang, J., Ding, Y., Yao, J., Zhang, M., Zhang, Y., Xie, Z., et al. (2022a). Nasal nanovaccines for SARS-CoV-2 to address COVID-19. Vaccines (Basel). 10 (3), 405. doi:10.3390/vaccines10030405
Huang, L., Rong, Y., Pan, Q., Yi, K., Tang, X., Zhang, Q., et al. (2021). SARS-CoV-2 vaccine research and development: Conventional vaccines and biomimetic nanotechnology strategies. Asian J. Pharm. Sci. 16 (2), 136–146. doi:10.1016/j.ajps.2020.08.001
Huang, X., Kon, E., Han, X., Zhang, X., Kong, N., Mitchell, M. J., et al. (2022b). Nanotechnology-based strategies against SARS-CoV-2 variants. Nat. Nanotechnol. 17, 1027–1037. doi:10.1038/s41565-022-01174-5
Idris, A., Davis, A., Supramaniam, A., Acharya, D., Kelly, G., Tayyar, Y., et al. (2021). A SARS-CoV-2 targeted siRNA-nanoparticle therapy for COVID-19. Mol. Ther. 29 (7), 2219–2226. doi:10.1016/j.ymthe.2021.05.004
Iravani, S. J. M. A. (2020). Nano-and biosensors for the detection of SARS-CoV-2: Challenges and opportunities. Mat. Adv. 1 (9), 3092–3103. doi:10.1039/d0ma00702a
Ishikawa, F. N., Chang, H.-K., Curreli, M., Liao, H.-I., Olson, C. A., Chen, P.-C., et al. (2009). Label-free, electrical detection of the SARS virus N-protein with nanowire biosensors utilizing antibody mimics as capture probes. ACS Nano 3 (5), 1219–1224. doi:10.1021/nn900086c
Itani, R., Tobaiqy, M., and Al Faraj, A. J. T. (2020). Optimizing use of theranostic nanoparticles as a life-saving strategy for treating COVID-19 patients. Theranostics 10 (13), 5932–5942. doi:10.7150/thno.46691
Jeremiah, S. S., Miyakawa, K., Morita, T., Yamaoka, Y., and Ryo, A. J. B. (2020). Potent antiviral effect of silver nanoparticles on SARS-CoV-2. Biochem. Biophys. Res. Commun. 533 (1), 195–200. doi:10.1016/j.bbrc.2020.09.018
Jeyanathan, M., Afkhami, S., Smaill, F., Miller, M. S., Lichty, B. D., and Xing, Z. J. N. R. I. (2020). Immunological considerations for COVID-19 vaccine strategies. Nat. Rev. Immunol. 20 (10), 615–632. doi:10.1038/s41577-020-00434-6
Jindal, A. B., Bachhav, S. S., and Devarajan, P. V. J. I. J. o. P. (2017). In situ hybrid nano drug delivery system (IHN-DDS) of antiretroviral drug for simultaneous targeting to multiple viral reservoirs: An in vivo proof of concept. Int. J. Pharm. X. 521 (1-2), 196–203. doi:10.1016/j.ijpharm.2017.02.024
Jindal, S., and Gopinath, P. J. N. E. (2020). Nanotechnology based approaches for combatting COVID-19 viral infection. Nano Ex. 1 (2), 022003. doi:10.1088/2632-959x/abb714
Kamat, S., Kumari, M., and Jayabaskaran, C. J. J. o. C. R. (2021). Nano-engineered tools in the diagnosis, therapeutics, prevention, and mitigation of SARS-CoV-2. J. Control. Release 338, 813–836. doi:10.1016/j.jconrel.2021.08.046
Karmacharya, M., Kumar, S., Gulenko, O., and Cho, Y.-K. J. A. A. B. M. (2021). Advances in facemasks during the COVID-19 pandemic era. ACS Appl. Bio Mat. 4 (5), 3891–3908. doi:10.1021/acsabm.0c01329
Kashyap, U., and Saha, S. K. J. T. o. t. I. N. A. o. E. (2020). Enhanced design of PPE based on electrostatic principle to eliminate viruses (SARS-CoV-2). Trans. Indian Natl. Acad. Eng. 5 (2), 337–341. doi:10.1007/s41403-020-00101-1
Kato, T., Takami, Y., Deo, V. K., and Park, E. Y. J. J. o. b. (2019). Preparation of virus-like particle mimetic nanovesicles displaying the S protein of Middle East respiratory syndrome coronavirus using insect cells. J. Biotechnol. 306, 177–184. doi:10.1016/j.jbiotec.2019.10.007
Kaushik, A. K., and Dhau, J. S. J. A. S. S. A. (2022). Photoelectrochemical oxidation assisted air purifiers; perspective as potential tools to control indoor SARS-CoV-2 Exposure. Appl. Surf. Sci. Adv. 9, 100236. doi:10.1016/j.apsadv.2022.100236
Kevadiya, B. D., Machhi, J., Herskovitz, J., Oleynikov, M. D., Blomberg, W. R., Bajwa, N., et al. (2021). Diagnostics for SARS-CoV-2 infections. Nat. Mat. 20 (5), 593–605. doi:10.1038/s41563-020-00906-z
Khalil, N. M., Carraro, E., Cótica, L. F., and Mainardes, R. M. J. E. o. o. d. d. (2011). Potential of polymeric nanoparticles in AIDS treatment and prevention. Expert Opin. Drug Deliv. 8 (1), 95–112. doi:10.1517/17425247.2011.543673
Kim, M.-G., Park, J. Y., Shon, Y., Kim, G., Shim, G., and Oh, Y.-K. (2014). Nanotechnology and vaccine development. asian J. Pharm. Sci. 9 (5), 227–235. doi:10.1016/j.ajps.2014.06.002
Kiremitler, N. B., Kemerli, M. Z., Kayaci, N., Karagoz, S., Pekdemir, S., Sarp, G., et al. (2022). Nanostructures for the prevention, diagnosis, and treatment of SARS-CoV-2: A review. ACS Appl. Nano Mat. 5 (5), 6029–6054. doi:10.1021/acsanm.2c00181
Kumar, V., Mishra, S., Sharma, R., Agarwal, J., Ghoshal, U., Khanna, T., et al. (2020). Development of RNA-based assay for rapid detection of SARS-CoV-2 in clinical samples, 1–7.
Lauring, A. S., and Hodcroft, E. B. (2021). Genetic variants of SARS-CoV-2—what do they mean? JAMA 325 (6), 529–531. doi:10.1001/jama.2020.27124
Lauring, A. S., and Malani, P. N. (2021). Variants of SARS-CoV-2. JAMA 326 (9), 880. doi:10.1001/jama.2021.14181
Layqah, L. A., and Eissa, S. J. M. A. (2019). An electrochemical immunosensor for the corona virus associated with the Middle East respiratory syndrome using an array of gold nanoparticle-modified carbon electrodes. Microchim. Acta 186 (4), 224–310. doi:10.1007/s00604-019-3345-5
Le Page, M. (2021). Threats from new variants. New Sci. 249 (3316), 8–9. doi:10.1016/s0262-4079(21)00003-8
Li, Z., Yi, Y., Luo, X., Xiong, N., Liu, Y., Li, S., et al. (2020). Development and clinical application of a rapid IgM-IgG combined antibody test for SARS-CoV-2 infection diagnosis. J. Med. Virol. 92 (9), 1518–1524. doi:10.1002/jmv.25727
Liao, M., Liu, H., Wang, X., Hu, X., Huang, Y., Liu, X., et al. (2021). A technical review of face mask wearing in preventing respiratory COVID-19 transmission. Curr. Opin. Colloid Interface Sci. 52, 101417. doi:10.1016/j.cocis.2021.101417
Lim, H. X., Lim, J., Jazayeri, S. D., Poppema, S., and Poh, C. L. J. B. J. (2021). Development of multi-epitope peptide-based vaccines against SARS-CoV-2. Biomed. J. 44 (1), 18–30. Development of multi-epitope peptide-based vaccines against SARS-CoV-2. 44. doi:10.1016/j.bj.2020.09.005
Lin, N., Verma, D., Saini, N., Arbi, R., Munir, M., Jovic, M., et al. (2021). Antiviral nanoparticles for sanitizing surfaces: A roadmap to self-sterilizing against COVID-19, 40, 101267.
Lombardi, A. F., Afsahi, A. M., Gupta, A., and Gholamrezanezhad, A. J. L. r. m. (2021). Severe acute respiratory syndrome (SARS), Middle East respiratory syndrome (MERS), influenza, and COVID-19, beyond the lungs: A review article. Radiol. Med. 126 (4), 561–569. doi:10.1007/s11547-020-01311-x
Lombardo, D., Kiselev, M. A., and Caccamo, M. T. J. J. o. N. (2019). Smart nanoparticles for drug delivery application: Development of versatile nanocarrier platforms in biotechnology and nanomedicine.
Ma, Y., Wang, Y., Dong, C., Gonzalez, G. X., Zhu, W., Kim, J., et al. (2022). SARS-CoV-2 spike stem protein nanoparticles elicited broad ADCC and robust neutralization against variants in mice.
Maddali, H., Miles, C. E., Kohn, J., and O'Carroll, D. M. J. C. (2021). Optical biosensors for virus detection: Prospects for SARS-CoV-2/COVID-19. ChemBioChem 22 (7), 1176–1189. doi:10.1002/cbic.202000744
Magalhaes, P., Andrade, L., Nunes, O. C., and Mendes, A. J. R. o. A. M. S. (2017). Titanium dioxide photocatalysis: Fundamentals and application on photoinactivation. 51(2).
Mahari, S., Roberts, A., Shahdeo, D., and Gandhi, S. J. B. (2020). eCovSens-ultrasensitive novel in-house built printed circuit board based electrochemical device for rapid detection of nCovid-19 antigen, a spike protein domain 1 of SARS-CoV-2.
Mahmoudinobar, F., Britton, D., and Montclare, J. K. J. P. E. (2021). Protein-based lateral flow assays for COVID-19 detection, 34. Design, and selection
Malabadi, R. B., Meti, N. T., and Chalannavar, R. K. (2021). Applications of nanotechnology in vaccine development for coronavirus (SARS-CoV-2) disease (Covid-19). Int. J. Res. Sci. Innovations 8 (2), 191–198.
Mallakpour, S., Behranvand, V., and Hussain, C. M. (2022). Worldwide fight against COVID-19 using nanotechnology, polymer science, and 3D printing technology. Polym. Bull., 1–19. doi:10.1007/s00289-021-04006-z
Mallhi, T. H., Liaqat, A., Abid, A., Khan, Y. H., Alotaibi, N. H., Alzarea, A. I., et al. (2020). Multilevel engagements of pharmacists during the COVID-19 pandemic: The way forward. Front. Public Health 8, 561924. doi:10.3389/fpubh.2020.561924
Mehta, M., Prasher, P., Sharma, M., Shastri, M. D., Khurana, N., Vyas, M., et al. (2020). Advanced drug delivery systems can assist in targeting coronavirus disease (COVID-19): A hypothesis. Med. Hypotheses 144, 110254. doi:10.1016/j.mehy.2020.110254
Milane, L., and Amiji, M. (2021). Clinical approval of nanotechnology-based SARS-CoV-2 mRNA vaccines: Impact on translational nanomedicine. Drug Deliv. Transl. Res. 11 (4), 1309–1315. doi:10.1007/s13346-021-00911-y
Misra, R., Acharya, S., and Sushmitha, N. J. R. i. m. v. (2022). Nanobiosensor-based diagnostic tools in viral infections: Special emphasis on Covid-19. Rev. Med. Virol. 32 (2), e2267. doi:10.1002/rmv.2267
Mitchell, M. J., Billingsley, M. M., Haley, R. M., Wechsler, M. E., Peppas, N. A., and Langer, R. J. N. R. D. D. (2021). Engineering precision nanoparticles for drug delivery. Nat. Rev. Drug Discov. 20 (2), 101–124. doi:10.1038/s41573-020-0090-8
Miyazawa, T., Itaya, M., Burdeos, G. C., Nakagawa, K., and Miyazawa, T. (2021). A critical review of the use of surfactant-coated nanoparticles in nanomedicine and food nanotechnology. Int. J. Nanomedicine 16, 3937–3999. doi:10.2147/ijn.s298606
Mohamed, N. A., Abou-Saleh, H., Mohamed, H. A., Al-Ghouti, M. A., Crovella, S., and Zupin, L. J. V. (2022). Think like a virus: Toward improving nanovaccine development against. SARS-CoV-2 14 (7), 1553.
Mohammadi, G., Koranni, Z. S., and Jebali, A. J. I. I. (2021). The oral vaccine based on self-replicating RNA lipid nanoparticles can simultaneously neutralize both SARS-CoV-2 variants alpha and delta, 101, 108231.
Mohapatra, R. K., Das, P. K., Pintilie, L., Dhama, K. J. E. J. o. B., and Sciences, A. (2021). Infection capability of SARS-CoV-2 on different surfaces. Egypt. J. Basic Appl. Sci. 8 (1), 75–80. doi:10.1080/2314808x.2021.1907915
Moitra, P., Alafeef, M., Dighe, K., Frieman, M. B., and Pan, D. J. A. n. (2020). Selective naked-eye detection of SARS-CoV-2 mediated by N gene targeted antisense oligonucleotide capped plasmonic nanoparticles. ACS Nano 14 (6), 7617–7627. doi:10.1021/acsnano.0c03822
Mosselhy, D. A., Kareinen, L., Kivistö, I., Virtanen, J., Loikkanen, E., Ge, Y., et al. (2022). Inhibition of SARS-CoV-2 alpha variant and murine noroviruses on copper-silver nanocomposite surfaces. Nanomater. (Basel). 12 (7), 1037. doi:10.3390/nano12071037
Moutaouakil, A. E., Poovathy, S., Belmoubarik, M., and Peng, W. K. J. a. p. a. (2020). Graphene-based biosensor for viral detection.
Mufamadi, M. S. (2020). Nanotechnology shows promise for next-generation vaccines in the fight against COVID-19. Springer.
Mukherjee, S., Mazumder, P., Joshi, M., Joshi, C., Dalvi, S. V., and Kumar, M. J. E. r. (2020). Biomedical application, drug delivery and metabolic pathway of antiviral nanotherapeutics for combating viral pandemic: A review. Environ. Res. 191, 110119. doi:10.1016/j.envres.2020.110119
Neghab, H. K., Azadeh, S. S., Soheilifar, M. H., and Dashtestani, F. J. A. J. o. M. B. (2020). Nanoformulation-based antiviral combination therapy for treatment of COVID-19. Avicenna J. Med. Biotechnol. 12 (4), 255–256.
Otto, S. P., Day, T., Arino, J., Colijn, C., Dushoff, J., Li, M., et al. (2021). The origins and potential future of SARS-CoV-2 variants of concern in the evolving COVID-19 pandemic. Curr. Biol. 31 (14), R918–R929. doi:10.1016/j.cub.2021.06.049
Owida, H. A., Al-Nabulsi, J. I., Turab, N. M., and Louzi, N. J. J. o. N. (2022). Nanotechnology role development for COVID-19 pandemic management, 2022.
Oyewumi, M. O., Kumar, A., and Cui, Z. (2010). Nano-microparticles as immune adjuvants: Correlating particle sizes and the resultant immune responses. Expert Rev. vaccines 9 (9), 1095–1107. doi:10.1586/erv.10.89
Özmen, E. N., Kartal, E., Turan, M. B., Yazıcıoğlu, A., Niazi, J. H., Qureshi, A. J. M. S., et al. (2021). Graphene and carbon nanotubes interfaced electrochemical nanobiosensors for the detection of SARS-CoV-2 (COVID-19) and other respiratory viral infections: A review. Mater. Sci. Eng. C 129, 112356. doi:10.1016/j.msec.2021.112356
Pal, K., Kyzas, G. Z., Kralj, S., and Gomes de Souza, F. (2021). Sunlight sterilized, recyclable and super hydrophobic anti-COVID laser-induced graphene mask formulation for indelible usability. J. Mol. Struct. 1233, 130100. doi:10.1016/j.molstruc.2021.130100
Paliwal, P., Sargolzaei, S., Bhardwaj, S. K., Bhardwaj, V., Dixit, C., and Kaushik, A. J. F. i. N. (2020). Grand challenges in bio-nanotechnology to manage the COVID-19 pandemic. Front. Nanotechnol. 2, 571284. doi:10.3389/fnano.2020.571284
Pandey, A., Nikam, A. N., Mutalik, S. P., Fernandes, G., Shreya, A. B., Padya, B. S., et al. (2020). Architectured therapeutic and diagnostic nanoplatforms for combating SARS-CoV-2: Role of inorganic, organic, and radioactive materials. ACS Biomater. Sci. Eng. 7 (1), 31–54. doi:10.1021/acsbiomaterials.0c01243
Peraman, R., Sure, S. K., Dusthackeer, V., Chilamakuru, N. B., Yiragamreddy, P. R., Pokuri, C., et al. (2021). Insights on recent approaches in drug discovery strategies and untapped drug targets against drug resistance. Futur. J. Pharm. Sci. 7 (1), 56–25. doi:10.1186/s43094-021-00196-5
Petersen, E., Koopmans, M., Go, U., Hamer, D. H., Petrosillo, N., Castelli, F., et al. (2020). Comparing SARS-CoV-2 with SARS-CoV and influenza pandemics. Lancet Infect. Dis. 20 (9), e238–e244. doi:10.1016/s1473-3099(20)30484-9
Pilaquinga, F., Morey, J., Torres, M., Seqqat, R., and Pina, M. D. L. N. (2021). Silver nanoparticles as a potential treatment against SARS-CoV -2: A review. Wiley Interdiscip. Rev. Nanomed. Nanobiotechnol. 13 (5), e1707. doi:10.1002/wnan.1707
Poggio, C., Colombo, M., Arciola, C. R., Greggi, T., Scribante, A., and Dagna, A. J. M. (2020). Copper-alloy surfaces and cleaning regimens against the spread of SARS-CoV-2 in dentistry and orthopedics. From fomites to anti-infective nanocoatings. Materials 13 (15), 3244. doi:10.3390/ma13153244
Pradhan, A., Lahare, P., Sinha, P., Singh, N., Gupta, B., Kuca, K., et al. (2021). Biosensors as nano-analytical tools for COVID-19 detection. Sensors (Basel). 21 (23), 7823. doi:10.3390/s21237823
Preetam, S., Dash, L., Sarangi, S. S., Sahoo, M. M., and Pradhan, A. K. J. B.-N. I. (2022). Application of nanobiosensor in health care sector, 251–270.
Qiu, G., Gai, Z., Tao, Y., Schmitt, J., Kullak-Ublick, G. A., and Wang, J. J. A. n. (2020). Dual-functional plasmonic photothermal biosensors for highly accurate severe acute respiratory syndrome coronavirus 2 detection. ACS Nano 14 (5), 5268–5277. doi:10.1021/acsnano.0c02439
Rabiee, N., Ahmadi, S., Soufi, G. J., Hekmatnia, A., Khatami, M., Fatahi, Y., et al. (2022). Quantum dots against sars-cov-2: Diagnostic and therapeutic potentials.
Raghuwanshi, D., Mishra, V., Das, D., Kaur, K., and Suresh, M. R. J. M. p. (2012). Dendritic cell targeted chitosan nanoparticles for nasal DNA immunization against SARS CoV nucleocapsid protein. Mol. Pharm. 9 (4), 946–956. doi:10.1021/mp200553x
Raja, R. K., Nguyen-Tri, P., Balasubramani, G., Alagarsamy, A., Hazir, S., Ladhari, S., et al. (2021). SARS-CoV-2 and its new variants: A comprehensive review on nanotechnological application insights into potential approaches, 1–29.
Ramakrishnan, S. G., Robert, B., Salim, A., Ananthan, P., Sivaramakrishnan, M., Subramaniam, S., et al. (2021). Nanotechnology based solutions to combat zoonotic viruses with special attention to SARS, MERS, and COVID 19: Detection, protection and medication, 159, 105133.
Ramana, L. N., Sharma, S., Sethuraman, S., Ranga, U., and Krishnan, U. M. (2014). Evaluation of chitosan nanoformulations as potent anti-HIV therapeutic systems. Biochimica Biophysica Acta - General Subj. 1840 (1), 476–484. doi:10.1016/j.bbagen.2013.10.002
Rashidzadeh, H., Danafar, H., Rahimi, H., Mozafari, F., Salehiabar, M., Rahmati, M. A., et al. (2021). Nanotechnology against the novel coronavirus (severe acute respiratory syndrome coronavirus 2): Diagnosis, treatment, therapy and future perspectives. Nanomedicine 16 (6), 497–516. doi:10.2217/nnm-2020-0441
Rasmi, Y., Saloua, K. S., Nemati, M., and Choi, J. R. J. N. (2021). Recent progress in nanotechnology for COVID-19 prevention, diagnostics and treatment. Nanomater. (Basel). 11 (7), 1788. doi:10.3390/nano11071788
Rhouati, A., Teniou, A., Badea, M., and Marty, J. L. J. S. (2021). Analysis of recent bio-/nanotechnologies for coronavirus diagnosis and therapy. Sensors (Basel). 21 (4), 1485. doi:10.3390/s21041485
Roh, C., and Jo, S. K. (2011). Quantitative and sensitive detection of SARS coronavirus nucleocapsid protein using quantum dots-conjugated RNA aptamer on chip. J. Chem. Technol. Biotechnol. 86 (12), 1475–1479.
Ruiz-Hitzky, E., Darder, M., Wicklein, B., Ruiz-Garcia, C., Martín-Sampedro, R., Del Real, G., et al. (2020). Nanotechnol. responses COVID-19. 9 (19), 2000979.
Rume, T., and Islam, S. D.-U. J. H. (2020). Environmental effects of COVID-19 pandemic and potential strategies of sustainability. Heliyon 6 (9), e04965. doi:10.1016/j.heliyon.2020.e04965
Sahu, A. K., Sreepadmanabh, M., Rai, M., and Chande, A. J. V. (2021). SARS-CoV-2: Phylogenetic origins, pathogenesis, modes of transmission, and the potential role of nanotechnology. Virusdisease 32 (1), 1–12. doi:10.1007/s13337-021-00653-y
Sarkar, P. K., and Das Mukhopadhyay, C. J. I. N. L. (2021). Ayurvedic metal nanoparticles could be novel antiviral agents against SARS-CoV-2. Int. Nano Lett. 11 (3), 197–203. doi:10.1007/s40089-020-00323-9
Seo, G., Lee, G., Kim, M. J., Baek, S.-H., Choi, M., Ku, K. B., et al. (2020). Rapid detection of COVID-19 causative virus (SARS-CoV-2) in human nasopharyngeal swab specimens using field-effect transistor-based biosensor. ACS Nano 14 (4), 5135–5142. doi:10.1021/acsnano.0c02823
Shukla, B. K., Tyagi, H., Bhandari, H., and Garg, S. (2021). “Nanotechnology-based approach to combat pandemic covid 19: A review,” in Macromolecular symposia (Wiley Online Library).
Siadati, S. A., Afzali, M., Sayyadi, M. J. C. R., and Letters, (2020). Could silver nano-particles control the 2019-nCoV virus? An urgent glance past 3 (1), 9–11.
Singh, P., Singh, D., Sa, P., Mohapatra, P., Khuntia, A. K., Sahoo, S., et al. (2021). Insights from nanotechnology in COVID-19: Prevention, detection, therapy and immunomodulation. Nanomedicine 16 (14), 1219–1235. doi:10.2217/nnm-2021-0004
Skariyachan, S., Gopal, D., Deshpande, D., Joshi, A., Uttarkar, A., and Niranjan, V. J. I. (2021). Genetics, et alCarbon fullerene and nanotube are probable binders to multiple targets of SARS-CoV-2: Insights from computational modeling and molecular dynamic simulation studies. Infect. Genet. Evol. 96, 105155. doi:10.1016/j.meegid.2021.105155
Sportelli, M. C., Izzi, M., Kukushkina, E. A., Hossain, S. I., Picca, R. A., Ditaranto, N., et al. (2020). Nanomater. (Basel). 10 (4), 802. doi:10.3390/nano10040802
Struyf, T., Deeks, J. J., Dinnes, J., Takwoingi, Y., Davenport, C., Leeflang, M. M., et al. (2022). Signs and symptoms to determine if a patient presenting in primary care or hospital outpatient settings has COVID-19.5
Talebian, S., Wallace, G. G., Schroeder, A., Stellacci, F., and Conde, J. J. N. n. (2020). Nanotechnology-based disinfectants and sensors for SARS-CoV-2. Nat. Nanotechnol. 15 (8), 618–621. doi:10.1038/s41565-020-0751-0
Tan, Q., He, L., Meng, X., Wang, W., Pan, H., Yin, W., et al. (2021). Macrophage biomimetic nanocarriers for anti-inflammation and targeted antiviral treatment in COVID-19. J. Nanobiotechnology 19 (1), 173–216. doi:10.1186/s12951-021-00926-0
Tao, K., Tzou, P. L., Nouhin, J., Gupta, R. K., de Oliveira, T., Kosakovsky Pond, S. L., et al. (2021). The biological and clinical significance of emerging SARS-CoV-2 variants. Nat. Rev. Genet. 22 (12), 757–773. doi:10.1038/s41576-021-00408-x
Tarkar, P. (2020). Impact of COVID-19 pandemic on education system. Int. J. Adv. Sci. Technol. 29 (9), 3812–3814.
Thakur, V., and Ratho, R. K. J. J. o. m. v. (2022). Omicron (B. 1.1. 529): A new SARS-CoV-2 variant of concern mounting worldwide fear. J. Med. Virol. 94 (5), 1821–1824. doi:10.1002/jmv.27541
Tharayil, A., Rajakumari, R., Kumar, A., Choudhary, M. D., Palit, P., and Thomas, S. J. E. M. (2021). New insights into application of nanoparticles in the diagnosis and screening of novel coronavirus (SARS-CoV-2). emergent Mat. 4 (1), 101–117. doi:10.1007/s42247-021-00182-w
Tian, B., Gao, F., Fock, J., Dufva, M., and Hansen, M. F. (2020). Homogeneous circle-to-circle amplification for real-time optomagnetic detection of SARS-CoV-2 RdRp coding sequence. Biosens. Bioelectron. X. 165, 112356. doi:10.1016/j.bios.2020.112356
Tian, E. K., Wang, Y., Ren, R., Zheng, W., and Liao, W. (2021). Gold nanoparticle: Recent progress on its antibacterial applications and mechanisms. J. Nanomater.
Torrente-Rodríguez, R. M., Lukas, H., Tu, J., Min, J., Yang, Y., Xu, C., et al. (2020). SARS-CoV-2 RapidPlex: A graphene-based multiplexed telemedicine platform for rapid and low-cost. COVID-19 diagnosis Monit. 3 (6), 1981–1998.
Ullah, A., Qazi, J., Rahman, L., Kanaras, A. G., Khan, W. S., Hussain, I., et al. (2020). Nanoparticles-assisted delivery of antiviral-siRNA as inhalable treatment for human respiratory viruses: A candidate approach against SARS-COV-2. Nano Sel. 1 (6), 612–621. doi:10.1002/nano.202000125
Unal, M. A., Bayrakdar, F., Nazir, H., Besbinar, O., Gurcan, C., Lozano, N., et al. (2021). Graphene oxide nanosheets interact and interfere with SARS-CoV-2 surface proteins and cell receptors to inhibit infectivity. Small 17 (25), 2101483. doi:10.1002/smll.202101483
Vahedifard, F., and Chakravarthy, K. J. E. m. (2021). Nanomedicine for COVID-19: The role of nanotechnology in the treatment and diagnosis of COVID-19. emergent Mat. 4 (1), 75–99. doi:10.1007/s42247-021-00168-8
Walensky, R. P., Walke, H. T., and Fauci, A. S. (2021). SARS-CoV-2 variants of concern in the United States—Challenges and opportunities. Jama 325 (11), 1037–1038. doi:10.1001/jama.2021.2294
Wang, C., Liu, M., Wang, Z., Li, S., Deng, Y., and He, N. J. N. T. (2021a). Point-of-care diagnostics for infectious diseases: From methods to devices. Nano Today 37, 101092. doi:10.1016/j.nantod.2021.101092
Wang, D., Zhang, B., Ding, H., Liu, D., Xiang, J., Gao, X. J., et al. (2021b2022). TiO2 supported single Ag atoms nanozyme for elimination of SARS-CoV2, 40. worldometer, 101243. Coronavirus deaths [Online]. [Accessed Nov 2022].
Xu, C., Lei, C., Hosseinpour, S., Ivanovski, S., Walsh, L. J., and Khademhosseini, A. J. N. S. R. (2022). Nanotechnology for the management of COVID-19 during the pandemic and in the post-pandemic era.
Xu, E. Z., Lee, C., Pritzl, S. D., Chen, A. S., Lohmueller, T., Cohen, B. E., et al. (2021). (INVITED) Infrared-to-ultraviolet upconverting nanoparticles for COVID-19-related disinfection applications. Opt. Mater. X 12, 100099. doi:10.1016/j.omx.2021.100099
Yasamineh, S., Kalajahi, H. G., Yasamineh, P., Yazdani, Y., Gholizadeh, O., Tabatabaie, R., et al. (2022). An overview on nanoparticle-based strategies to fight viral infections with a focus on COVID-19. J. Nanobiotechnology 20 (1), 440–526. doi:10.1186/s12951-022-01625-0
Yildiz, G., Bolton-Warberg, M., and Awaja, F. J. A. B. (2021). Graphene and graphene oxide for bio-sensing: General properties and the effects of graphene ripples. Acta Biomater. 131, 62–79. doi:10.1016/j.actbio.2021.06.047
Zhang, Q., Honko, A., Zhou, J., Gong, H., Downs, S. N., Vasquez, J. H., et al. (2020). Cellular nanosponges inhibit SARS-CoV-2 infectivity. Nano Lett. 20 (7), 5570–5574. doi:10.1021/acs.nanolett.0c02278
Zhao, Z., Xiao, Y., Xu, L., Liu, Y., Jiang, G., Wang, W., et al. (2021). Glycyrrhizic acid nanoparticles as antiviral and anti-inflammatory agents for COVID-19 treatment. ACS Appl. Mat. Interfaces 13 (18), 20995–21006. doi:10.1021/acsami.1c02755
Zheng, B., Peng, W., Guo, M., Huang, M., Gu, Y., Wang, T., et al. (2021). Inhalable nanovaccine with biomimetic coronavirus structure to trigger mucosal immunity of respiratory tract against COVID-19. Chem. Eng. J. 19418, 129392. doi:10.1016/j.cej.2021.129392
Zhou, J., Krishnan, N., Jiang, Y., Fang, R. H., and Zhang, L. J. N. T. (2021). Nanotechnology for virus treatment. Nano Today 36, 101031. doi:10.1016/j.nantod.2020.101031
Zhu, X., Wang, X., Han, L., Chen, T., Wang, L., Li, H., et al. (2020a). Multiplex reverse transcription loop-mediated isothermal amplification combined with nanoparticle-based lateral flow biosensor for the diagnosis of COVID-19. Biosens. Bioelectron. X. 166, 112437. doi:10.1016/j.bios.2020.112437
Zhu, X., Wang, X., Han, L., Chen, T., Wang, L., Li, H., et al. (2020b). Reverse transcription loop-mediated isothermal amplification combined with nanoparticles-based biosensor for diagnosis of COVID-19.
Keywords: SARS-CoV-2, variants of concern (VOCs), COVID-19, nanomaterial, nanotechnology, mutations
Citation: Iqbal R, Khan S, Ali HM, Khan M, Wahab S and Khan T (2022) Application of nanomaterials against SARS-CoV-2: An emphasis on their usefulness against emerging variants of concern. Front. Nanotechnol. 4:1060756. doi: 10.3389/fnano.2022.1060756
Received: 03 October 2022; Accepted: 30 November 2022;
Published: 09 December 2022.
Edited by:
Valtencir Zucolotto, University of São Paulo, BrazilReviewed by:
Bwalya Angel Witika, Sefako Makgatho Health Sciences University, South AfricaCopyright © 2022 Iqbal, Khan, Ali, Khan, Wahab and Khan. This is an open-access article distributed under the terms of the Creative Commons Attribution License (CC BY). The use, distribution or reproduction in other forums is permitted, provided the original author(s) and the copyright owner(s) are credited and that the original publication in this journal is cited, in accordance with accepted academic practice. No use, distribution or reproduction is permitted which does not comply with these terms.
*Correspondence: Tariq Khan, dGFyaXFraGFuQHVvbS5lZHUucGs=
†These authors have contributed equally to this work and share first authorship
Disclaimer: All claims expressed in this article are solely those of the authors and do not necessarily represent those of their affiliated organizations, or those of the publisher, the editors and the reviewers. Any product that may be evaluated in this article or claim that may be made by its manufacturer is not guaranteed or endorsed by the publisher.
Research integrity at Frontiers
Learn more about the work of our research integrity team to safeguard the quality of each article we publish.