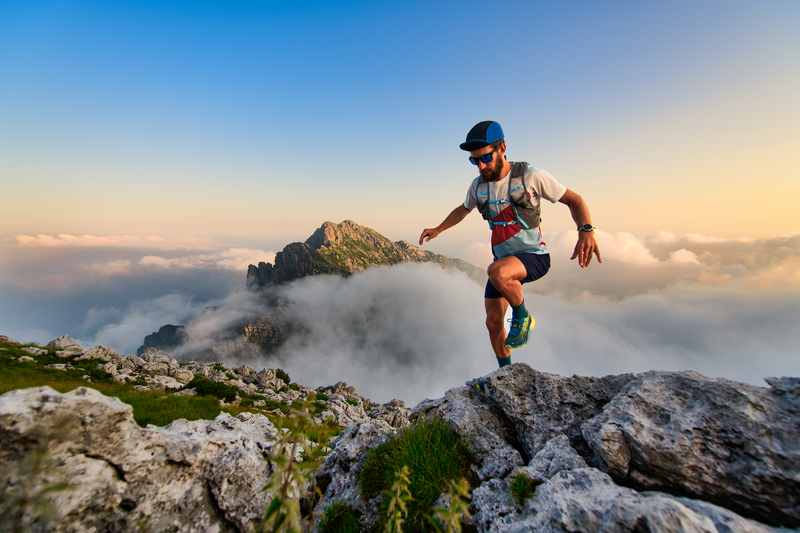
95% of researchers rate our articles as excellent or good
Learn more about the work of our research integrity team to safeguard the quality of each article we publish.
Find out more
PERSPECTIVE article
Front. Nanotechnol. , 02 December 2022
Sec. Nanomaterials
Volume 4 - 2022 | https://doi.org/10.3389/fnano.2022.1056498
For centuries, man has dominated the development of fibers and textiles to make clothing that protects them against environmental adversities, and gradually dissimilar cultural and ethnic identity traits have been created. Our garments are composed of natural elements such as animal leather, vegetable fibers, and synthetic textiles that result in ultra-resistant and durable materials. However, the textile industry has a non-sustainable character mainly because population growth will limit the use of natural resources, such as land and water, exclusively for food. At the same time, petrochemical-derived materials will gradually be replaced by more biodegradable alternatives due to their toxic accumulation in the local environment and their contribution to global climate change. The vast inventiveness of human-being is opening the possibility of replacing our clothes by mimicking, reproducing, and scaling up nature’s biosynthetic machinery through cutting-edge biotechnological approaches. Nevertheless, the new cosmovision of biotextiles must meet two requirements: 1) the appearance and performance of the clothes should be preserved to join the current textile market demand, and at the same time, 2) new functionalities should be incorporated into our clothes to embrace the impressive technological advances occurring day to day. In this regard, nanotechnological developments will be able to provide the desired properties so that the textile industry can provide bio-based materials enhanced with nanotechnology-based intelligent functionalities. This perspective article discloses nano-biotechnological approaches to address the challenge of dressing up future societies and new material consciousness.
Clothes have been part of the human being since the origins of the most primitive societies. Trying to get better weather protection, we emulate animals; their skins were our first dresses. Thousands of years later, vegetable fibers such as cotton, linen, or hemp were domesticated to obtain new types of woven fabrics. In the last 100 years, and thanks to the development of the petrochemical industry, synthetic materials such as nylon, polyester, and other polymeric fibers have been developed to generate plastic-based fabrics and threads. Nevertheless, today, we know that several factors make the textile industry non-sustainable, and some of these are:
1) The raising of animals and the cultivation of plant crops consume a lot of space and resources. Both practices are based on the excessive use of fertile soils, causing its depletion, the consumption of other natural resources such as water, and contributing to global warming by emitting greenhouse gases such as methane, carbon dioxide, nitrous oxides, etc. (Foley, 2014).
2) With the growth of the world population, the obtention of food will be more determinant for using natural resources rather than clothing. Climate change is also already affecting food security (Mbow and Rosenzweig, 2019).
3) Materials derived from the plastic industry generate great controversy due to their raw materials’ origin and low biodegradability. About 16–35% of microplastics released globally to oceans are derived from synthetic textiles. Between 200,000 and 500,000 Ton of microplastics enter the global marine environment each year (European Environment Agency, 2022).
Under this perspective ¿which options do men have to dress up in the future? Much has been said about ecological clothing, which mainly refers to fabrics that come from eco-friendly resources, like sustainably grown fibers or by upcycling wasted materials. Manufacturing processes also determine sustainability. Some of the environmental sustainability criteria in the textile industry are 1) respect for diversity and the environment, 2) reduction, recycling, reuse, and wastewater treatment, 3) energy transitions away from fossil fuels to new sources of sustainable energy, 4) practices with the potential to capture atmospheric carbon dioxides, 5) circular models such as upcycling, including the products end of life, biodegradability, and recyclability (Lee, 2017; Tilstra and Beatrice, 2021).
In this regard, biofabrication seems the most sustainable option for producing threads or textiles without using extended crops or animal cruelty to obtain raw materials for clothing (Zhong, 2020). Biotechnological and synthetic biology approaches produce self-generating fibers. They could be further transformed into threads, woven or nonwoven fabrics using novel advances such as weaving, scaffolding, electrospinning, 3D bioprinting, or other additive manufacturing strategies (Huda et al., 2007; Antinori et al., 2021; Smith and Mele, 2021; Muskan and Negi, 2022). The biofibers could be produced from several means: 1) the culture of living microorganisms such as bacteria and yeast as biofactories (e.g., recombinant proteins), 2) single bacteria or symbiotic mutualism associations between bacteria and yeast (e.g., bacterial cellulose), 3) fungal mycelium scaffolding, and 4) extraction of raw materials from agricultural wastes (e.g., vegetable biomass), among other techniques (Jones et al., 2021; Jayaprakash et al., 2022).
However, some of these novel biomaterials lack mechanical strength, performance, and durability, and often the final products need some finishing treatments to acquire the desired characteristics. Nanotechnology has emerged as a powerful tool to improve and tune materials properties that could enhance and embed new properties in self-generated biotextiles. These nano-biotechnological approaches could be envisioned by forming novel nanocomposites or adding unique nanomaterials at different stages of the biofabrication process. Therefore, this perspective article aims to show a new cosmovision of how nano-biotechnological approaches could accelerate the obtention of environmentally-friendly smart biotextiles.
A textile is a fabric generally made by weaving threads of different fibers to obtain clothing or other articles. These fibers could be of natural or synthetic origin. The natural fibers are composed either of polysaccharides (e.g., cellulose, hemicellulose, lignin, etc.) or proteins (e.g., fibroin, collagen, etc.). The main difference between proteins and polysaccharides is that proteins are macromolecules made from amino acids, while polysaccharide chains are composed of sugar molecules; both substances (amino acids and sugars) are the basic building blocks of life (Gough et al., 2020). Biofibers are biopolymers composed of polysaccharides and proteins, but these could be extracted from biomass, synthesized using bio-derived monomers, or produced by microorganisms. Another type of biotextile is composed of a β-glucan/chitin complex, which has been recently engineered from fungal mycelium to biofabricate leather-like materials. The field of biotextiles occurs at the convergence of some inter and trans-disciplines, such as biotechnology, nanotechnology, synthetic biology, polymer, and material sciences, textile technology, design, assisted manufacturing and robotics, artificial intelligence, biomedical engineering, and genetic engineering, among others (King et al., 2013).
In terms of the next generation of biotextile, the following concepts should be considered to understand the difference between these biofibers and conventional natural fibers (Biofabricate and Fashion for Good, 2020):
1) Biobased: refers to products wholly or partly derived from biomass, such as plants, trees, or animals (the biomass can have undergone physical, chemical, or biological treatment).
2) Biosynthetic: synthetic polymeric materials comprised, in whole or part, of bio-derived compounds. These compounds can be made with an input of biological origin (biomass) and/or where the process is performed by living microorganisms.
3) Biofabricated: biofabricated materials are entirely produced by living cells (e.g., mammalian) and microorganisms, such as bacteria, yeast, and fungi).
Besides the “green” origin, this term also relates to renewable, compostable, and biodegradable textiles.
The main biopolymers that could produce fibers according to their chemical composition (protein-based or polysaccharide-based) are the following:
1) Protein-based: collagen, fibroin, gelatin, casein, and actin are naturally from animal origins, while other proteins such as soy, whey, and zein could be obtained from vegetable sources.
2) Polysaccharide-based: naturally, these polymers are of vegetable origin, and the most common examples are cellulose, starch, chitin/chitosan, pectin, alginate, and carrageenan. Additionally, the β-glucan/chitin complex from fungal origin grows as fibers able to produce biotextiles.
Protein-based fibers make collagen and silk the most attractive biopolymers for developing biotextiles. Collagen’s repeating amino acid sequence allows it to form a stable secondary protein structure of triple helices. These helices can further assemble into quaternary structures, allowing collagen to assemble into fibrillar proteins found throughout the body (Shoulders and Raines, 2009). Collagen fiber could be produced from renewable natural resources, such as ocean collagen peptides, or recycled fish scales, resulting in a soft textile suitable for use on sensitive skin (Sportingtex, 2022). Also, some examples grow engineered yeast as cell factories that produce target proteins. These heterologous protein building blocks could biofabricate abrasion-resistant, water-resistant, breathable, lightweight, and durable materials with characteristics similar to leather (Modern Meadow, 2022).
Since natural silk has remarkable strength, significant efforts have been made to obtain recombinant silk fibroin, principally from the spider, dragline, or silkworm. Recent advances in synthetic biology and fermentation processes enable high-level expressions of these recombinant proteins, using bacteria, yeasts, animal cells, and plants as biofactories. The protein is later isolated and transformed into threads by techniques such as spinning (Bolt threads, 2022a). This technology could also obtain other products, such as resin and leather-like materials (Spiber, 2022). Other less common proteins, like β-sheet structured fibrous protein, have been recently explored for their exciting properties; which are isolated initially from squid ring teeth and can synthesize self-healing fibers to develop durable textiles with heat and pressure, firm and elastic as spandex and thermal responsiveness (Tandem Repeat, 2022). Likewise, more recently, self-colored wool fibers could be engineered by using recombinant proteins initially isolated from coral, jellyfish, or oysters (Wherewool).
Regarding polysaccharide-based fibers, cellulose, chitin, and alginate are the most promising biopolymers-yielding fibers. For instance, bacterial cellulose is extruded by Gram-negative species of the genera Komagataeibacter, Acetobacter, Rhizobium, Agrobacterium, Pseudomonas, Salmonella, Alcaligenes, and Sarcina (the only Gram-positive bacterial genus). Specific fermentation media and mutant strains are needed for industrial-scale production in agitated and air-lift bioreactors, membrane reactors, or horizontal bioreactors (Felgueiras et al., 2021). Bacterial cellulose could be fermented from days to several weeks with several elements to provide mechanical strength or other features (Bucha Bio, 2022; Polybion, 2022; Nanollose, 2022).
After cellulose, chitin, the second most abundant natural polysaccharide on earth, is found in several sources, including crab and shrimp shells, arthropod exoskeletons, the molluscan shell of squids, and, noticeably, the mushrooms. Chitin is the primary component in the cell wall of fungi forming a β-glucan/chitin complex which imparts structural rigidity to the mycelium. Fungal mycelium is the vast macroscopic network of biomass of microscopically interconnected tubular cells produced by filamentous fungi during their growth (Vandelook et al., 2021). By cultivating mycelium on a determined substrate, it is possible to grow materials by harnessing its ability to digest and transform cellulose into natural composites; by collecting them into proper scaffolds, it is possible to obtain sheets with many exciting characteristics (Feijóo-Vivas et al., 2021). Following physical and chemical treatment, these sheets of fungal biomass visually resemble leather and exhibit comparable material and tactile properties (Jones et al., 2021). Few companies in the world are recently harnessing the potential of mycelium to biofabricate leather-like materials (Bolt threds, 2022b; Ecovative, 2022; Mogu, 2022; Mycotech, 2022; Mycoworks, 2022; Mylium, 2022; Spora Biotech, 2022).
Another polysaccharide source is alginate, produced by bacteria and seaweed. Bacteria and seaweed alginate differ in composition, modifications, molecular mass, viscoelasticity, and polydispersity. These unique qualities lead to a wide range of applications, including alginate’s use in nanoparticles, nanotubes, microspheres, microcapsules, sponges, hydrogels, foams, elastomers, and fibers. An example is produced from the biomass of one of the most fast-growing organisms on earth-kelp (Laminaria digitata), a large brown alga also known as oarweed (AlgiKnit, 2022). One of the most popular products made from vegetable biomass are those extracted from fruit peels and mixed in different proportions of polymers such as waterborne PU; some examples are apples (Appleskin, 2022), grapes (Vegea, 2022), pineapple (Piñatex, 2022), and cactus (Desserto, 2022). Figure 1 summarizes several vanguard companies currently developing protein-based and polysaccharide-based biotextiles by making great efforts to scale up the biotechnological processes required to obtain bio-based textiles or their raw materials either by biosynthesis or biofabrication.
FIGURE 1. Scheme of the main biopolymers used for manufacturing threads and biotextiles according to their chemical composition and some worldwide producer companies. The classification scheme was adapted from reference (Biofabricate and Fashion for Good, 2020).
Comparing the advantages associated with the materials described above concerning the natural options, several elements could be highlighted: the control over the growing conditions allows the optimization of the processing times (from days to weeks) in contrast with many natural processes that take years to be completed. Moreover, the expected environmental impact of the fermentation technologies is moderate to low, mainly because of the origin of the raw materials. However, the processing costs are still high because most of these biotechnological approaches are incipient. As soon as technological development guarantees the scaling-up, the costs will become more competitive with established technologies. By themselves, bio-based textiles do not present better mechanical properties (in general), so their reinforcement is necessary. This is one of the main topics in which nanotechnology will be relevant, as described below. The comparative table (Supplementary Table S1) presented in the electronic supporting file shows some characteristics of the novel biomaterials versus those of their natural counterparts.
Recently, several nanomaterials have been used along with fibers or as coatings to enhance surface characteristics by introducing novel properties such as biocide, waterproof, antistatic, UV-protection, dirt, odor and/or stain resistance, wrinkle-resistant, higher thermal performance, among other properties. The vast range of nanostructures that could be exploitable in this field includes nanowhiskers, nanoparticles, nanoporous materials, nanotubes, and nano coatings, among others. The global clothing market using nanotechnology is expected to grow to $13.83 billion in 2026, opening the possibility of generating attractive businesses (Wood, 2022).
Nanomaterials are those materials with 1–100 nm size at least in one of its dimensions (1 nm corresponds to 10−9 m). To be able to manipulate matter on such a minimal scale, combining several disciplines such as physics, chemistry, materials science, biosciences, and others is mandatory. By making these multidisciplinary approaches, the physicochemical properties of the nanomaterials may change very much from that of the corresponding bulk material (Kolahalam et al., 2019). Nanomaterials can be classified in terms of their origin (natural or anthropogenic), their dimensionality (0D, 1D, 2D, or 3D), their crystallinity (amorphous, monocrystalline, polycrystalline), or their chemical nature (inorganic, organic, hybrid, carbon-based). These characteristics provide determined properties exploitable in several applications (Joudeh and Linke, 2022). Two main approaches could be used to obtain these nanomaterials: top-down and bottom-up, involving physical, chemical, or biological approximations. In top-down approaches, bulk materials are divided to produce nanostructured materials; these include mechanical milling, laser ablation, etching, sputtering, and electro-explosion, most of them physical approaches (Baig et al., 2021).
On the contrary, under the approaches using bottom-up techniques, individual atoms are piled up one at a time to form molecules. These molecules arrange themselves into the desired form to yield the required nanostructures; this molecular self-assembly exploits the organizational capability of matter (Khanna, 2016). Wet chemical synthesis methods (e.g., sol-gel, solvothermal and hydrothermal, micro-emulsion, and co-precipitation) are examples of bottom-up approaches (Barhoum et al., 2022). Nanoparticles could also be biofabricated to decrease the harmful effects of their production and application, the so-called “green synthesis” (Rajiv et al., 2017). Under green synthesis approaches, the use or the generation of dangerous substances such as organic solvents or harsh reagents are eliminated (Shafey, 2020), likewise the large amount of energy required in physical synthesis (Abbasi et al., 2016). Different biological sources have been used for the biofabrication of nanoparticles. For instance, several microorganisms lead to metallic nanoparticles through redox reactions occurring intra or extracellularly (Gahlawat and Choudhury, 2019). Some plants produce bio-reduction, stabilization, and protection through their secondary metabolites (such as polyphenols, terpenoids, proteins, and sugars, among others) (Sarwar et al., 2021).
The biotextiles could be improved in mechanical and/or aesthetic aspects using nano-biotechnological approaches. The mechanical or thermal characteristics for specific applications, such as bags, upholstery, shoes, soles, etc., could be achieved through mechanical reinforcement. Natural biopolymers could be strengthened by incorporating a small amount of nanofillers forming composites, some of them based on ceramics (mainly silicate-based), layered nanomaterials such as nanoclay or carbon nanofillers (e.g., carbon fibers, carbon nanotubes, graphene, and its derivatives) (Sharma et al., 2018). Recent studies have demonstrated the potential of cellulose nanofibers (bacterially produced nanofibrillated) as reinforcing elements in various bio-based polymers (Robles et al., 2015). Cellulose has high specific strength and modulus; the maximum macroscopic Young’s natural plant cellulose fibers modulus is 128 GPa, far superior to any synthetic polymer (Miao and Hamad, 2013). In addition, traditional dyeing strategies for polysaccharide-based fibers are not efficient due to the excessive concentration of dyes and harsh dyeing conditions. In this regard, nanopigments could provide a less aggressive dyeing strategy (Chavarriaga et al., 2022). In the textile industry, biofabrication has been mainly focused on antibacterial and/or antifungal, ultraviolet light protection, and durability; these properties could also be incorporated into the biotextiles (Maghimaa and Alharbi, 2020; Lotfy et al., 2021; Sadeghi-Kiakhani et al., 2022). Functionalized cotton textiles with silver nanoparticles made from black rice extract have exhibited antibacterial activity against Escherichia coli and Staphylococcus aureus, UV protection, and higher hydrophobic performance (Yu et al., 2021). Similarly, cotton textiles functionalized with copper oxide nanoparticles synthesized using Carica papaya leaf extract exhibited promising antimicrobial activity against E. coli even after 30 wash cycles, in addition to increased hydrophobicity and tensile strength (Turakhia et al., 2020).
Several techniques include nanomaterials into the fibers, including coating, spraying, transfer printing, impregnation, dipping, and padding. Of these methods, padding is the most used in the current textile industry. The nanoparticles are attached to the fabrics with a padder adjusted to suitable pressure and speed, followed by drying and curing. In the case of the coatings, the composition can be modified by adding a surfactant, solvents, and other film-forming additives, which is ideal for 3D bioprinting applications (Hosne and Hasan, 2018). However, the potential of the nano-biotechnological approaches comes from the possibility of including the nanomaterials during the biofabrication or allowing the formation of the required nanomaterial by taking advantage of green synthesis. For instance, some fungi species belonging to Aspergillus, Fusarium, Verticillium, Trichoderma, Rhizopus, Penicillium, and Pleurotus genus led to nanoparticles of titanium oxide, copper, zinc, and cadmium sulfide, gold, silver, and cadmium sulfide, allowing the incorporation in situ of the desired property (Elsakhawy et al., 2022).
Intelligent textiles are manufactured and designed to integrate technologies that offer the user greater functionality. The purpose is to provide added value for pleasure, performance, or safety. Applications range from health, safety, and security to lifestyle monitoring (Sayol, 2015). Certain materials respond to external stimuli through thermal, electrical, magnetic, or mechanical properties. These responses are reflected in physical changes such as color, shape, electric charge, and rheology, among others (Basheer, 2020). Wearable sensing devices have attracted considerable interest in monitoring human performance and health. Many devices have been invented incorporating electronics in wearable patches, wristbands, and other configurations that monitor either localized or general physiological information such as heart rate or blood glucose, as well as posture and motions (Smart fabrics with bioactive inks monitor body, 2020). Generally, the electronics involved include flexible displays, a keyboard (for instance, made from silver-plated and carbon fiber), a power supply, and microcontrollers. These approaches integrated into a clothing element can show a phone’s navigation instructions and incoming messages. With advances in decoding brain waves, the researchers also hope to use their display technology in healthcare as an assistive communication tool (KRÄMER, 2021).
Furthermore, biodegradable coatings, biofibers, and biotextiles made from biopolymers such as poly (lactic acid) (PLA), poly (glycolic acid) (PGA), lactic acid-glycolic acid copolymers, and polycaprolactone (PCL) has been widely studied for medical biodegradable polymers (Ratner, 2019). Since biodegradable polymers can be polymerized with controlled radical polymerization, the control over molecular weights and chain homogeneity is high, allowing engineered scaffolds for tissue applications. Therefore, biotextiles can also be designed for use in specific biological environments due to their biocompatibility and biostability with cells and biological fluids.
The most demanding activities in terms of performance and quality are automotive and aero spatial industries, not only from the mechanical point of view but including high strength and low weight (Abbasi et al., 2020). Automotive fabric is subject to a long list of abrasion-resistance and thermal regulations. For instance, ceramic nanocoatings can increase abrasion resistance (Gu et al., 2020). The ability to absorb energy in extreme temperature conditions inside the vehicles is possible using polymer-based phase change materials (Mondal, 2008). On the other hand, in the race to colonize new extraterrestrial spaces, innovative nanomaterials have been developed with improved self-healing, sensing, and actuating capabilities, all essential for outer space applications.
Moreover, to reduce excessive water and energy usage for washing garments, significant effort has been made to manufacture goods that are easy to clean without detergents and are durable (Combining Nanotechnology with Nature to Create Waterproof Coatings for Leather and Textiles, 2017). Many finishing products that make the fabric or the clothing fresh, hygienic, and careful have been developed using colloidal silver or copper as antimicrobial finishing (The science behind clean textile, 2014). Moreover, microencapsulation of active and volatile substances for controlled release of fragrances, drugs, phase-change materials, flame retardants, or lubricants could help in this field (Zhao et al., 2019). Figure 2 presents a scheme indicating the classification of nanomaterials, some novel properties and functionalities exploitable in the textile industry, and some examples of smart textiles.
FIGURE 2. Scheme of the types of nanomaterials that can be used in the biotextile industry according to several properties of interest and their functionalities.
Biotechnological advances to develop new biofibers or biotextile represent an attractive business. Many articles can be developed through bio-based polymers, although at present, greater emphasis has been placed on silk threads and alternative products to leather. For example, only the bio-based leather market size is estimated to be USD 647 million in 2021 and is projected to reach USD 868 million by 2026, at a CAGR of 6.1% between 2021 and 2026 (Bio-based Leather Market by Source, 2021). Emerging companies are making great efforts in their respective R&D areas to scale up their biotechnological processes. However, once the scaling limitation is overcome, these technologies will be able to globalize at great speed to enlarge the spectrum of products that can be developed.
In addition to environmental-friendly materials, the future of human society will require functional and intelligent outfits capable of integrating with technological developments related to different issues, such as early detection of human diseases and pathogens (cardiac, hypertension, viral particles, etc.), monitoring of vital functions, adaptation to digital communication media, better performance, and resistance to elements such as high temperature and radiation, etc. Because of these, nanotechnology plays a fundamental role in these developments due to the accelerated knowledge that has been generated regarding the relationship between the morphology and dimensions of a nanomaterial and its properties. Properties such as electrical, tribological, and magnetic help integrate biosensors. Adsorbent nanoparticles and/or nanocapsules can also be incorporated to manipulate chemicals and drugs of interest. Although one of the central premises of biotextile is their biodegradability, specific applications require surfaces with unquestionable durability (e.g., resistance to tearing and abrasion), and the addition of nanocoatings can improve these. In short, different properties can be increased to provide clothing with intelligence and functionality, essential to contribute to human society’s development and environmental conservation. (Wherewool, 2022).
The original contributions presented in the study are included in the article/Supplementary Material, further inquiries can be directed to the corresponding authors.
KF, MG, and LN-B contributed equally to this work in conceptualizing and writing the original draft. HR, JF, and PZ participate in writing, reviewing, and editing.
KF, MG, JF, PZ, and LN-B were employed by the company Spora Biotech, Work Center Vespucio.
All claims expressed in this article are solely those of the authors and do not necessarily represent those of their affiliated organizations, or those of the publisher, the editors and the reviewers. Any product that may be evaluated in this article, or claim that may be made by its manufacturer, is not guaranteed or endorsed by the publisher.
The Supplementary Material for this article can be found online at: https://www.frontiersin.org/articles/10.3389/fnano.2022.1056498/full#supplementary-material
Abbasi, A., Khojasteh, H., Hamadanian, M., and Salavati-Niasari, M. (2016). Synthesis of CoFe2O4 nanoparticles and investigation of the temperature, surfactant, capping agent and time effects on the size and magnetic properties. J. Mat. Sci. Mat. Electron. 27 (5), 4972–4980. http://link.springer.com/10.1007/s10854-016-4383-y. doi:10.1007/s10854-016-4383-y
Abbasi, S., Peerzada, M. H., Nizamuddin, S., and Mubarak, N. M. (2020). “Functionalized nanomaterials for the aerospace, vehicle, and sports industries,” in Handbook of functionalized nanomaterials for industrial applications (Elsevier), Amsterdam, Netherlands 795–825. https://linkinghub.elsevier.com/retrieve/pii/B9780128167878000259.
AlgiKnit (2022) Available from: https://www.algiknit.com/.
Antinori, M. E., Contardi, M., Suarato, G., Armirotti, A., Bertorelli, R., Mancini, G., et al. (2021). Advanced mycelium materials as potential self-growing biomedical scaffolds. Sci. Rep. 11 (1), 12630. http://www.nature.com/articles/s41598-021-91572-x. doi:10.1038/s41598-021-91572-x
Appleskin (2022) Available from: https://appleskin.com/Sep 23, 2022).
Baig, N., Kammakakam, I., and Falath, W. (2021). Nanomaterials: A review of synthesis methods, properties, recent progress, and challenges. Mat. Adv. 2 (6), 1821–1871. http://xlink.rsc.org/?DOI=D0MA00807A. doi:10.1039/D0MA00807A
Barhoum, A., García-Betancourt, M. L., Jeevanandam, J., Hussien, E. A., Mekkawy, S. A., Mostafa, M., et al. (2022). Review on natural, incidental, bioinspired, and engineered nanomaterials: History, definitions, classifications, synthesis, properties, market, toxicities, risks, and regulations. Nanomater 12 (2), 177. https://www.mdpi.com/2079-4991/12/2/177. doi:10.3390/nano12020177
Basheer, A. A. (2020). Advances in the smart materials applications in the aerospace industries. Aircr. Eng. Aerosp. Technol. 92 (7), 1027–1035. https://www.emerald.com/insight/content/doi/10.1108/AEAT-02-2020-0040/full/html.doi:10.1108/AEAT-02-2020-0040/full/html
Bio-based Leather Market by Source (2021). Markets and markets. Available from: https://www.marketsandmarkets.com/Market-Reports/bio-based-leather-market-206086810.html Sep 21, 2022).
Biofabricate and Fashion for Good (2020). Understanding “bio” material innovations: A primer for the fashion industry Available from: https://www.biofabricate.co/resources.
Bolt threads (2022a) Available from: https://boltthreads.com/technology/microsilk/.
Bolt threds (2022b) Available from: https://www.mylo-unleather.com/, 2022 Jul 29).
Bucha Bio (2022) Available from: https://bucha.bio/Aug 5, 2022).
Chavarriaga, E. A., Wermuth, T. B., and Lopera, A. A. (2022). Nanomaterials for inorganic pigments. Available from: https://link.springer.com/10.1007/978-3-030-86901-4_11.185–194.
Combining Nanotechnology with Nature to Create Waterproof Coatings for Leather and Textiles (2017). Azo materials. Available from: https://www.azom.com/article.aspx?ArticleID=14157 Sep 10, 2022).
Desserto (2022). Available from: https://desserto.com.mx/home.
Ecovative (2022). Ecovative unlocks the power of mycelium to grow better materials. Available from: https://www.ecovative.com/ Sep 20, 2022).
Elsakhawy, T., Omara, A. E-D., Abowaly, M., El-Ramady, H., Badgar, K., Llanaj, X., et al. (2022). Green synthesis of nanoparticles by mushrooms: A crucial dimension for sustainable soil management. Sustain. 14 (7), 4328. https://www.mdpi.com/2071-1050/14/7/4328. doi:10.3390/su14074328
European Environment Agency (2022). Microplastics from textiles: Towards a circular economy for textiles in europe, EEA’s European Topic Centre on Circular Economy.Copenhagen, Denmark.
Feijóo-Vivas, K., Bermúdez-Puga, S. A., Rebolledo, H., Figueroa, J. M., Zamora, P., and Naranjo-Briceño, L. (2021). Bioproductos desarrollados a partir de micelio de hongos: Una nueva cultura material y su impacto en la transición hacia una economía sostenible. Bionatura, 6, 1 1637–1652. https://www.revistabionatura.com/2021.06.01.29.html. doi:10.21931/RB/2021.06.01.29
Felgueiras, C., Azoia, N. G., Gonçalves, C., Gama, M., and Dourado, F. (2021). Trends on the cellulose-based textiles: Raw materials and technologies. Front. Bioeng. Biotechnol 29. https://www.frontiersin.org/articles/10.3389/fbioe.2021.608826/full. doi:10.3389/fbioe.2021.608826
Foley, J. (2014). The future of food. https://www.nationalgeographic.com/foodfeatures/feeding-9-billion/.
Gahlawat, G., and Choudhury, A. R. (2019). A review on the biosynthesis of metal and metal salt nanoparticles by microbes. RSC Adv. 9 (23), 12944–12967. http://xlink.rsc.org/?DOI=C8RA10483B. doi:10.1039/C8RA10483B
Gough, C. R., Rivera-Galletti, A., Cowan, D. A., Salas-de la Cruz, D., and Hu, X. (2020). Protein and polysaccharide-based fiber materials generated from ionic liquids: A review. Molecules 25 (15), 3362. https://www.mdpi.com/1420-3049/25/15/3362. doi:10.3390/molecules25153362
Gu, Y., Xia, K., Wu, D., Mou, J., and Zheng, S. (2020). Technical characteristics and wear-resistant mechanism of nano coatings: A review. Coatings 10 (3), 233. https://www.mdpi.com/2079-6412/10/3/233. doi:10.3390/coatings10030233
Hosne, A. K. M. A., and Hasan, M. Z. (2018). Application of nanotechnology in modern textiles: A review. Int. J. Curr. Eng. Technol. 8 (2). 227-231. http://inpressco.com/application-of-nanotechnology-in-modern-textiles-a-review/. doi:10.14741/ijcet/v.8.2.5
Huda, S., Reddy, N., Karst, D., Xu, W., Yang, W., and Yang, Y. (2007). Nontraditional biofibers for A new textile industry. J. Biobased Mat. Bioenergy 1 (2), 177–190. http://openurl.ingenta.com/content/xref?genre=article&issn=1556-6560&volume=1&issue=2&spage=177. doi:10.1166/jbmb.2007.022
Jayaprakash, K., Osama, A., Rajagopal, R., Goyette, B., and Karthikeyan, O. P. (2022). Agriculture waste biomass repurposed into natural fibers: A circular bioeconomy perspective. Bioeng. 9 (7), 296. https://www.mdpi.com/2306-5354/9/7/296. doi:10.3390/bioengineering9070296
Jones, M., Gandia, A., John, S., and Bismarck, A. (2021). Leather-like material biofabrication using fungi. Nat. Sustain. 4, 9–16. http://www.nature.com/articles/s41893-020-00606-1. doi:10.1038/s41893-020-00606-1
Joudeh, N., and Linke, D. (2022). Nanoparticle classification, physicochemical properties, characterization, and applications: A comprehensive review for biologists. J. Nanobiotechnol. 20 (1), 262. https://jnanobiotechnology.biomedcentral.com/articles/10.1186/s12951-022-01477-8. doi:10.1186/s12951-022-01477-8
Khanna, V. K. (2016). Bottom-up nanofabrication. Integrated Nanoelectronics. Springer, New Delhi, India http://link.springer.com/10.1007/978-81-322-3625-2_24. doi:10.1007/978-81-322-3625-2_24
King, M. W., Gupta, B. S., and Guidoin, R. (2013). “Introduction,” in Biotextiles as medical implants (Elsevier), Amsterdam, Netherlands xxxi–xxxvii. https://linkinghub.elsevier.com/retrieve/pii/B9781845694395500272. doi:10.1016/B978-1-84569-439-5.50027-2
Kolahalam, L. A., Kasi Viswanath, I. V., Diwakar, B. S., Govindh, B., Reddy, V., and Murthy, Y. L. N. (2019). Review on nanomaterials: Synthesis and applications. Mater. Today Proc. 18, 2182–2190. https://linkinghub.elsevier.com/retrieve/pii/S2214785319325507. doi:10.1016/j.matpr.2019.07.371
Krämer, K. (2021). Giant glowing fabric made into shirtsleeve display. Available from:article https://www.chemistryworld.com/news/giant-glowing-fabric-made-into-shirtsleeve-display/4013362.
Lee, K. E. (2017). Environmental sustainability in the textile industry, Sustainability in the Textile Industry. Springer, Singapore. 17–55. http://link.springer.com/10.1007/978-981-10-2639-3_3. doi:10.1007/978-981-10-2639-3_3
Lotfy, W. A., Alkersh, B. M., Sabry, S. A., and Ghozlan, H. A. (2021). Biosynthesis of silver nanoparticles by Aspergillus terreus: Characterization, optimization, and biological activities. Front. Bioeng. Biotechnol. 9 https://www.frontiersin.org/articles/10.3389/fbioe.2021.633468/full. doi:10.3389/fbioe.2021.633468
Maghimaa, M., and Alharbi, S. A. (2020). Green synthesis of silver nanoparticles from Curcuma longa L. and coating on the cotton fabrics for antimicrobial applications and wound healing activity. J. Photochem. Photobiol. B Biol. 204, 111806. https://linkinghub.elsevier.com/retrieve/pii/S1011134419317695. doi:10.1016/j.jphotobiol.2020.111806
Mbow, C., and Rosenzweig, C. (2019). “Food security,” in Climate change and land: An IPCC special report on climate change, desertification, land degradation, sustainable land management, food security, and greenhouse gas fluxes in terrestrial ecosystems (Geneva, Switzerland: The Intergovernmental Panel on Climate Change). https://www.ipcc.ch/srccl/chapter/chapter-5/.
Miao, C., and Hamad, W. Y. (2013). Cellulose reinforced polymer composites and nanocomposites: A critical review. Cellul. 20 (5), 2221–2262. http://link.springer.com/10.1007/s10570-013-0007-3. doi:10.1007/s10570-013-0007-3
Modern Meadow (2022) Available from: https://www.modernmeadow.com/technology.
Mogu (2022) Available from: https://mogu.bio/tag/leather/ Sep 20, 2022).
Mondal, S. (2008). Phase change materials for smart textiles – an overview. Appl. Therm. Eng. 28 (11–12), 1536–1550. https://linkinghub.elsevier.com/retrieve/pii/S1359431107002876. doi:10.1016/j.applthermaleng.2007.08.009
Muskan, Gupta D., and Negi, N. P. (2022). 3D bioprinting: Printing the future and recent advances. Bioprinting 27, e00211. https://linkinghub.elsevier.com/retrieve/pii/S2405886622000215. doi:10.1016/j.bprint.2022.e00211
Mycotech (2022) Available from: https://mycl.bio/ Sep 20, 2022).
Mycoworks (2022) Available from: https://www.mycoworks.com/ Sep 20, 2022).
Mylium (2022) Available from: https://www.mylium.nl/ Sep 20, 2022).
Nanollose (2022) Available from: https://nanollose.com/ Aug 12, 2022).
Piñatex (2022) Available from: https://www.ananas-anam.com/ Sep 23, 2022).
Polybion (2022) Available from: https://www.polybion.bio/ Aug 8, 2022).
Rajiv, P., Bavadharani, B., Kumar, M. N., and Vanathi, P. (2017). Synthesis and characterization of biogenic iron oxide nanoparticles using green chemistry approach and evaluating their biological activities. Biocatal. Agric. Biotechnol. 12, 45–49. https://linkinghub.elsevier.com/retrieve/pii/S1878818117303523. doi:10.1016/j.bcab.2017.08.015
Ratner, B. D. (2019). Biomaterials: Been there, done that, and evolving into the future. Annu. Rev. Biomed. Eng. 21 (1), 171–191. https://www.annualreviews.org/doi/10.1146/annurev-bioeng-062117-120940. doi:10.1146/annurev-bioeng-062117-120940
Robles, E., Urruzola, I., Labidi, J., and Serrano, L. (2015). Surface-modified nano-cellulose as reinforcement in poly(lactic acid) to conform new composites. Industrial Crops Prod. 71, 44–53. https://linkinghub.elsevier.com/retrieve/pii/S0926669015002654. doi:10.1016/j.indcrop.2015.03.075
Sadeghi-Kiakhani, M., Tehrani-Bagha, A. R., Miri, F. S., Hashemi, E., and Safi, M. (2022). Eco-friendly procedure for rendering the antibacterial and antioxidant of cotton fabrics via phyto-synthesized AgNPs with malva sylvestris (MS) natural colorant. Front. Bioeng. Biotechnol. 9.814374. https://www.frontiersin.org/articles/10.3389/fbioe.2021.814374/full. doi:10.3389/fbioe.2021.814374
Sarwar, N., Humayoun, U. Bin, Kumar, M., Zaidi, S. F. A., Yoo, J. H., Ali, N., et al. (2021). Citric acid mediated green synthesis of copper nanoparticles using cinnamon bark extract and its multifaceted applications. J. Clean. Prod. 292, 125974. https://linkinghub.elsevier.com/retrieve/pii/S0959652621001943. doi:10.1016/j.jclepro.2021.125974
Sayol, I. (2015). Smart Fabrics, a technology that revolutionizes experiences. Available from: https://ignasisayol.com/en/smart-textiles-can-be-programmed-to-monitor-things-like-biometrics-measurements-of-physical-attributes-or-behaviours-like-heart-rate-which-could-help-athletes-dieters-and-physicians-observing-pat/.
Shafey, A. M. E. (2020). Green synthesis of metal and metal oxide nanoparticles from plant leaf extracts and their applications: A review. Green Process. Synthesis 9 (1), 304–339. https://www.degruyter.com/document/doi/10.1515/gps-2020-0031/html. doi:10.1515/gps-2020-0031
Sharma, B., Malik, P., and Jain, P. (2018). Biopolymer reinforced nanocomposites: A comprehensive review. Mater Today Commun. 16, 353–363. https://linkinghub.elsevier.com/retrieve/pii/S235249281830117X. doi:10.1016/j.mtcomm.2018.07.004
Shoulders, M. D., and Raines, R. T. (2009). Collagen structure and stability. Annu. Rev. Biochem. 78 (1), 929–958. https://www.annualreviews.org/doi/10.1146/annurev.biochem.77.032207.120833. doi:10.1146/annurev.biochem.77.032207.120833
Smart fabrics with bioactive inks monitor body (2020) Available from: https://tectales.com/wearables-sensors/smart-fabrics-with-bioactive-inks-monitor-body.htmlhttps://news.mit.edu/2022/smart-textiles-sense-movement-0707.
Smith, J. A., and Mele, E. (2021). Electrospinning and additive manufacturing: Adding three-dimensionality to electrospun scaffolds for tissue engineering. Front. Bioeng. Biotechnol. 9, 674738. https://www.frontiersin.org/articles/10.3389/fbioe.2021.674738/full. doi:10.3389/fbioe.2021.674738
Spiber (2022). Available from: https://spiber.inc/en/.
Spora Biotech (2022) Available from: https://www.sporabiotech.com/ Sep 19, 2022).
Sportingtex (2022) Available from: https://www.sportingtex.com/collagen-fiber-fabric.
Tandem Repeat (2022). Available from: https://www.tandemrepeat.com/technology/.
The science behind clean textile (2014). Fiber2fashion. Available from: https://www.fibre2fashion.com/industry-article/7295/the-science-behind-clean-textile.
Tilstra, F., and Beatrice, G. (2021). The sustainable transition in apparel and home textiles. CBI Ministry of Foreign Affairs.Amsterdam, Netherlands Available from: https://www.cbi.eu/market-information/apparel/sustainable-transition-apparel-and-home-textiles.
Turakhia, B., Divakara, M. B., Santosh, M. S., and Shah, S. (2020). Green synthesis of copper oxide nanoparticles: A promising approach in the development of antibacterial textiles. J. Coat. Technol. Res. 17 (2), 531–540. http://link.springer.com/10.1007/s11998-019-00303-5. doi:10.1007/s11998-019-00303-5
Vandelook, S., Elsacker, E., Van Wylick, A., De Laet, L., and Peeters, E. (2021). Current state and future prospects of pure mycelium materials. Fungal Biol. Biotechnol. 8 (1), 20. https://fungalbiolbiotech.biomedcentral.com/articles/10.1186/s40694-021-00128-1. doi:10.1186/s40694-021-00128-1
Vegea (2022). Available from: https://www.vegeacompany.com/ Sep 23, 2022).
Wherewool (2022). Available from: https://www.werewool.bio/#anchor-technology.
Wood, L. (2022). Global nanotechnology clothing market (2022 to 2031) - featuring nano textile, colmar and shanghai huzheng nano technology among others. GLOBE NEWSWIRE Los Angeles, CA, USA
Yu, Z., Liu, J., He, H., Wang, Y., Zhao, Y., Lu, Q., et al. (2021). Green synthesis of silver nanoparticles with black rice (Oryza sativa L.) extract endowing carboxymethyl chitosan modified cotton with high anti-microbial and durable properties. Cellul. 28 (3), 1827–1842. http://link.springer.com/10.1007/s10570-020-03639-z. doi:10.1007/s10570-020-03639-z
Zhao, H., Fei, X., Cao, L., Zhang, B., and Liu, X. (2019). The fabrication of fragrance microcapsules and their sustained and broken release behavior. Mater. (Basel) 12 (3), 393. http://www.mdpi.com/1996-1944/12/3/393. doi:10.3390/ma12030393
Zhong, C. (2020). Industrial-scale production and applications of bacterial cellulose. Front. Bioeng. Biotechnol. 8, 605374. https://www.frontiersin.org/articles/10.3389/fbioe.2020.605374/full. doi:10.3389/fbioe.2020.605374
Keywords: bioassembling, biofabrication, biosynthesis, smart textiles, nano-biotechnology, nano-crosslinking, nano-coating
Citation: Fuentes KM, Gómez M, Rebolledo H, Figueroa JM, Zamora P and Naranjo-Briceño L (2022) Nanomaterials in the future biotextile industry: A new cosmovision to obtain smart biotextiles. Front. Nanotechnol. 4:1056498. doi: 10.3389/fnano.2022.1056498
Received: 28 September 2022; Accepted: 17 November 2022;
Published: 02 December 2022.
Edited by:
Andreas Rosenkranz, University of Chile, ChileReviewed by:
Liliana Hristian, Gheorghe Asachi Technical University of Iași, RomaniaCopyright © 2022 Fuentes, Gómez, Rebolledo, Figueroa, Zamora and Naranjo-Briceño. This is an open-access article distributed under the terms of the Creative Commons Attribution License (CC BY). The use, distribution or reproduction in other forums is permitted, provided the original author(s) and the copyright owner(s) are credited and that the original publication in this journal is cited, in accordance with accepted academic practice. No use, distribution or reproduction is permitted which does not comply with these terms.
*Correspondence: Keyla M. Fuentes, a2Z1ZW50ZXNAc3BvcmFiaW90ZWNoLmNvbQ==; Leopoldo Naranjo-Briceño, bG5hcmFuam9Ac3BvcmFiaW90ZWNoLmNvbQ==
Disclaimer: All claims expressed in this article are solely those of the authors and do not necessarily represent those of their affiliated organizations, or those of the publisher, the editors and the reviewers. Any product that may be evaluated in this article or claim that may be made by its manufacturer is not guaranteed or endorsed by the publisher.
Research integrity at Frontiers
Learn more about the work of our research integrity team to safeguard the quality of each article we publish.