Expression of Concern: The expansion of lignocellulose biomass conversion into bioenergy via nanobiotechnology
- 1Graduate School, University of Nottingham Malaysia Campus, Selangor, Malaysia
- 2Department of Chemical and Petroleum Engineering, UCSI University, Kuala Lumpur, Malaysia
- 3Department of Chemical and Environmental Engineering, Faculty of Science and Engineering, University of Nottingham Malaysia, Selangor Darul Ehsan, Malaysia
- 4Department of Chemical Engineering, College of Engineering, Khalifa University, Abu Dhabi, United Arab Emirates
- 5Faculty of Science, Center of Excellence in Catalysis for Bioenergy and Renewable Chemicals (CBRC), Chulalongkorn University, Bangkok, Thailand
- 6Department of Energy and Environmental Engineering, Saveetha School of Engineering, Saveetha Institute of Medical and Technical Sciences, Saveetha University, Chennai, India
- 7Department of Mechanical Engineering, Jyothi Engineering College, Thrissur, India
Lignocellulosic biomass has arisen as a solution to our energy and environmental challenges because it is rich in feedstock that can be converted to biofuels. Converting lignocellulosic biomass to sugar is a complicated system involved in the bioconversion process. There are indeed a variety of techniques that have been utilized in the bioconversion process consisting of physical, chemical, and biological approaches. However, most of them have drawbacks when used on a large scale, which include the high cost of processing, the development of harmful inhibitors, and the detoxification of the inhibitors that have been produced. These constraints, taken together, hinder the effectiveness of current solutions and demand for the invention of a new, productive, cost-effective, and environmentally sustainable technique for LB processing. In this context, the approach of nanotechnology utilizing various nanomaterials and nanoparticles in treating lignocellulose biomass and bioenergy conversion has achieved increased interest and has been explored greatly in recent times. This mini review delves into the application of nanotechnological techniques in the bioconversion of lignocellulose biomass into bioenergy. This review on nanotechnological application in biomass conversion provides insights and development tools for the expansion of new sectors, resulting in excellent value and productivity, contributing to the long-term economic progress.
1 Introduction
1.1 Lignocellulose
Lignocellulose is a generic term used to describe the cell wall of plants which constitutes of cellulose (38–50%), hemicellulose (17–32%), and lignin (15–30%), where cellulose and hemicellulose consist of pentose and hexose sugar monomers, whereas lignin consists of polyphenol aromatics (Jönsson and Martín, 2016; Elumalai et al., 2018; Garlapati et al., 2020). The structural functionality of lignocellulose can be elucidated as following, where cellulose forms the structure of the cell walls, while hemicellulose assists in the cross-linking between the non-cellulosic and cellulosic polymers via covalent bonding (Figure 1). On the other hand, lignin which resembles phenol formaldehyde resin encloses the polysaccharide polymers and functions as “glue” to hold the lignocellulose structure together, thus providing additional mechanical strength to the cell walls for resistance from insects or during wet conditions. Besides, lignin functions as the hydrophobic barrier that enables transport of water and many essential nutrients through the cell wall. Several studies have reported that these polymeric substances are more prominent in larger concentrations within the primary and secondary cell walls of the plant, although the fractional composition may vary significantly based on their species, genetics, or environmental factors (Sun and Cheng, 2002).
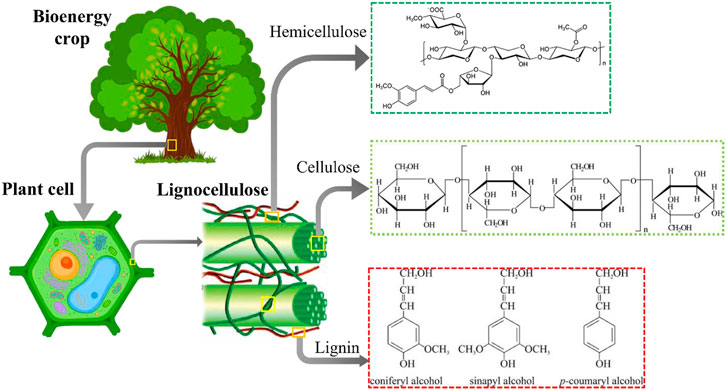
FIGURE 1. Schematic illustration showing bioenergy crop (lignocellulosic biomass), where the plant cell wall is composed of lignocellulose consisting of carbohydrate polymers such as cellulose, hemicellulose, and the aromatic polymer which is known as lignin. The cellulose and hemicellulose accommodate 6 and 5 carbon sugars which are rigidly bounded with the lignin.
In recent times, research on lignocellulosic material and its conversion into bioenergy has been growing at a relentless pace owing to the dwindling fossil fuel reserves and the environmental pollution associated with the exploitation of these resources. In an attempt to gradually transit toward a bio-based economy, various renewable energy sources have been explored to date, such as solar, wind, hydrothermal, and biomass. However, biomass-derived energy has remained the only promising renewable energy source that can potentially supersede petroleum-based fuel for transportation or to produce chemicals. The high chemical energy content of lignocellulosic biomass owing to the 75% carbohydrates from cellulose and hemicellulose has been ideal for bioenergy use (Chen, 2014; Sanusi et al., 2021). Besides, furfural, 5-hydroxymethylfurfural, and levulinic acid obtained from lignocellulose during biorefining are very favorable building blocks for the generation of energy fuels by chemical or biological synthesis processes (Elumalai et al., 2018).
Advantageously, lignocellulosic biomass is available abundantly from agriculture and forestry with an estimated annual production of 1.7-2 × 1011 tonnes per year (Elumalai et al., 2018). Besides, in 2011, 38 million tonnes of biomass were used for biofuels in the EU, out of 1.2 billion tonnes of biomass generated from various crops (Scarlat et al., 2015). In addition, it is reported that by 2030, the supply of lignocellulose biomasses in the United States alone would be 450 million dry tonnes per year, which can generate 67 million gallons of ethanol per year (Valdivia et al., 2016).
Despite these advantages, biomasses are recalcitrant in nature owing to their cellulose crystallinity and non-reactive lignin. As such, pretreatment techniques are required to allow the cellulose to be amenable to the enzymatic hydrolysis process that subsequently assists in the extraction of fermentable sugars prior to the biofuel generation processes. Besides, technological limitations such as high cost and inadequacy in the existing infrastructure have posed major challenges in attaining high quality and yield of bioenergy from lignocellulosic material. In view of these limitations, studies have reported various optimization strategies in pretreatment stages as well as enzymes and fermentation stages to enhance bioenergy production in an energy efficient and cost-effective manner. Recently, the growing interest in nanomaterials and their exceptional properties have been vastly exploited to enhance bioenergy generation. As such, in this review, first, the fundamental principles of nanomaterials and their significance in bioenergy generation are discussed thoroughly. Section 2 will detail the application of nanotechnology for biomass conversion particularly in lignocellulosic pretreatment and for enzyme immobilization. Next, the utilization of a nanobiocatalyst in bioenergy production will be reviewed. Last, the challenges and perspectives for future progress in bioenergy generation from lignocellulosic biomasses are highlighted.
1.2 Fundamentals of Nanomaterials and its Significance in Bioenergy Production
The International Standardization Organization (ISO) has defined nanomaterials as any material having external, internal, or surface structure dimensions in the nanoscale range of 1–100 nm (ISO/TS 27687:2008 Nanotechnologies, 2008). Nanoparticles (NPs) can be broadly classified into four groups: carbonic, organic, inorganic, and composites. The characteristics of NPs are dependent on their chemical structure which influences their functionality in various environments. Besides, it is interesting to note that the physical and chemical properties of NPs vary significantly from their macro counterparts which can be exploited for use in various applications such as bioenergy, electronics, medicine, ionic liquids, and consumer products (Li et al., 2005; Shuttleworth et al., 2014; He et al., 2020; Production et al., 2020). Moreover, NPs have a faster reaction rate than larger particles while being essential in the bioenergy generation process. The faster reaction rate is associated with the extremely fine particle sizes of NPs that provide a large surface area to volume ratio which in turn increases the number of active sites for various reactions or processes to occur (Misson et al., 2015).
From the perspective of bioenergy production, nanomaterials can be envisioned in many aspects to meet the rapidly growing global energy requirements owing to their exceptional properties such as large surface area, high catalytic activity, crystallinity, durability, stability, adsorption capability, and efficient storage (Zhang et al., 2011; Hamawand et al., 2020). All the aforementioned advantages of NPs can enhance the productivity, hydrolysis, and stability of cellulase enzymes for various bioenergy productions. Generally, NPs in bioenergy production can be advantageous in cellulase production, for cellulase thermal stability, in pretreatment of biomass, waste management, or sugar and biohydrogen production. For instance, in a study by Srivastava et al., it was reported that an efficient cellulase process is essential for enzymatic hydrolysis, and the use of NPs such as Fe3O4 and Fe3O4/alginate showed higher hydrolysis efficiency and enhanced cellulase production by 35 and 40% respectively (Srivastava et al., 2015). A similar group of authors also investigated the efficacy of nickel cobaltite (NiCo2O4) NPs and reported 40% more cellulase production with an enhanced thermal stability at 80°C for 7 h (Srivastava et al., 2014). The thermal and pH stability of crude cellulase have also been investigated using zinc oxide (ZnO)-based NPs, and it was shown to exhibit an enhanced thermal stability at 65°C for 10 h with a maximum pH alkaline value of 10.5; all of which are highly desirable in the field of bioethanol and biohydrogen production (Srivastava et al., 2016). In another study by Dutta et al., calcium hydroxyapatite-based NPs increased the thermal stability and enhanced the production of d-xylose by 35% (Dutta et al., 2014). The authors reported that the uses of both nanoparticle-activated xylanase and nanoparticle-activated cellulase systems are exclusive for demonstrating high activity at elevated temperatures up to 80°C. It was further added that utilization of cofactor calcium in an NP form conferred the increased thermostability and activity to the cellulase and xylanase enzymes.
Magnetic nanoparticles (MNPs) have been most commonly used in comparison with other nanoparticles in bioenergy production since the magnetic properties allow easy recoverability, and have the ability to bind to multiple target compounds, excellent biodegradability, low cytotoxicity to biomass cells, and the ease of synthesis (Pena et al., 2012). The large surface area to volume ratios of MNPs have also been utilized as carriers for the immobilization of enzymes, which leads to increased stability for use in biodiesel or bioethanol production. For instance, Xie and Ma reported that immobilization on the support with magnetic Fe3O4 NPs showed excellent pH tolerance and thermostability with conversion to biodiesel fuels up to 90%, while the immobilized lipase was reusable four more times without compromising its activity or performance (Xie and Ma, 2009). Other MNPs that were used for immobilization of enzymes include TiO2 for the hydrolysis of lignocellulosic material for bioethanol production (Ahmad and Sardar, 2014), a mix of TiO2–ZnO catalyst for biodiesel production (Madhuvilakku and Piraman, 2013), and manganese dioxide (MnO2) for bioethanol production. Moreover, the usage of nanoparticles or their microemulsion was shown to have a hydrolytic effect that is identical to that observed with chemical pretreatment in lignocellulosic biomass processing. This was seen in the study carried out by Pena and a colleague whereby when wheat straw was treated with perfluoroalkylsulfonic (PFS) and alkylsulfonic (AS) acid-functionalized magnetic nanoparticles, it produced 46% more sugar than the control (35%). The addition of nanoparticles to PFS and AS acid may have led to the stabilization and allotted hemicellulose hydrolysis which has comparable acid strength of sulfuric acid solutions (Wang et al., 2012). The authors highlighted the main advantage of using magnetic nanoparticles which lies in their notable function in pretreating lignocellulosic biomass with little quantities while still being recyclable for future usage. In another study of pretreatment, the combined effect of alkaline pretreatment with magnetite (Fe3O4) nanoparticles on rice straw for biogas production was explored (Khalid et al., 2019). The application of MNPs demonstrated a major advancement in biogas production that increases biogas and methane yield by 100 and 129%. Nanoparticles have also been used to improve sugar production at the pretreatment level. Yellow poplar sawdust was processed to 96 percent (w/w) hemicellulose at 175°C using 0.8 percent (w/v) sulfuric acid. The application of sulfuric acid with PFS and AS nanomaterials converted hemicelluloses by 66 and 61%, respectively, at 50- and 400-fold acid levels (Arora et al., 2020). Some other studies have reported that the high coercivity and excellent paramagnetic properties of MNPs are highly desirable for biogas and methane production. For instance, Abdelsalam et al. reported that Fe3O4 MNPs with a concentration up to 20 mg/L reduced the time required to achieve highest biogas and methane production (Abdelsalam et al., 2017). Besides, the Fe3O4 MNPs assisted in the effective distribution of the iron ions in the solution which ensured a sustainable supply of iron ions in the bioreactor; all of these bio-stimulated the anaerobic digestion process, thus increasing the production of biogas and methane. Table 1 summarizes some of the most recent utilization of nanoparticles to generate bioenergy such as biodiesel, bioethanol, and biogas from biomasses.
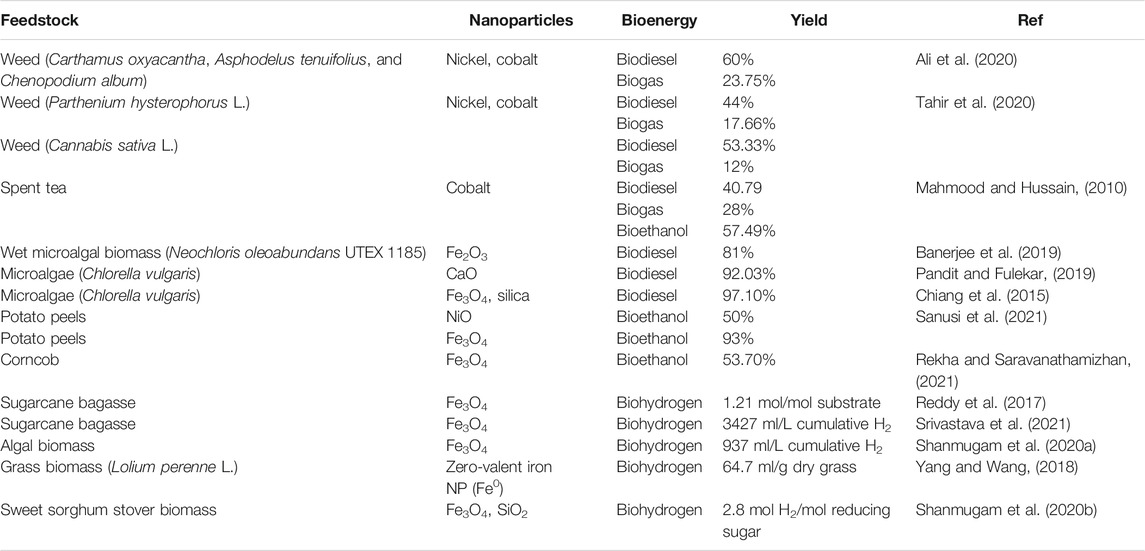
TABLE 1. Utilization of various nanoparticles for the generation of bioenergy (biodiesel, biogas, bioethanol, and bioenergy) from biomass feedstock.
2 Application of Nanotechnology for Biomass Conversion
Lignocellulosic biomass has emerged as a viable remedy to the issue concerning environmental safety. It is a substantial resource for the production of energy, with an extremely large production globally. In 2011, 38 million tonnes of biomass were used for biofuels in the EU, out of 1.2 billion tonnes of biomass generated from various crops (Scarlat et al., 2015). In addition, it is reported that by 2030, the supply of lignocellulose biomasses in the United States alone would be 450 million dry tonnes per year, which can create 67 million gallons of ethanol per year (Valdivia et al., 2016). The transformation of LB into fermentable sugars and bioenergy is a multistep process that necessitates precision technologies all the way down to the end result.
The entire conversion process of LB to significance products involves the following steps: 1) pretreatment of LB to disrupt biomass structure and make it suitable for enzymatic process, 2) enzymatic hydrolysis of components to release sugars for fermentation, and 3) fermentation to convert monomer sugars into biofuel or other biochemicals depending on the application. The Figure 2 below, shows the summary of different steps involved in the production of bioenergy via lignocellulose biomasses. The lignocellulosic biomass can be converted into bioethanol and/or biochemical products using a variety of pretreatment processes, including physical, chemical, and biological ones. However, these conventional methods are costly, inconvenient, and have some limitations. These constraints have been circumvented by introducing a unique, cost-effective, efficient, and environmentally friendly method, namely, nanotechnology. The application of nanotechnology presents a promising alternative to existing pretreatment methods. (Zanuso et al., 2021).
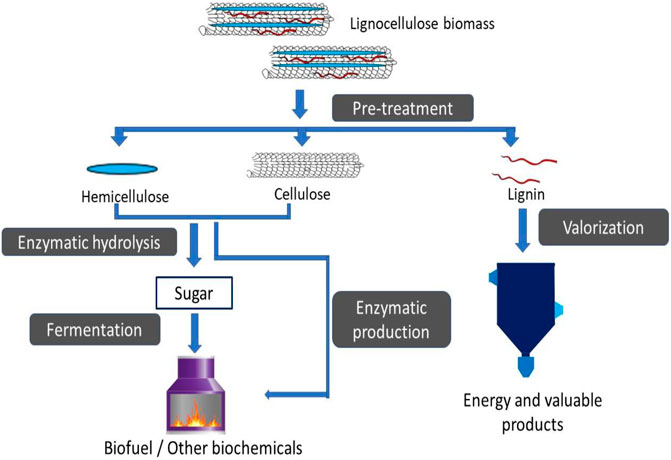
FIGURE 2. Graphic representation of steps involved in the production of bioenergy using lignocellulose biomasses. The bioconversion process of lignocellulose involves several steps that begin with the pretreatment stage where the biomass is disrupted into a simpler structure for enzymatic hydrolysis. The enzymatic process involves the use of an enzyme to convert hemicellulose and cellulose into biofuel and other biochemical production either via fermentation process or direct enzymatic conversion. The valorization process comprises the conversion of lignin into various products. Valorization of lignin through thermochemical technologies can produce aromatics, bio-oil, and carbon materials.
2.1 Lignocellulose Pretreatment
Pretreatment of LB is the first step in any bioenergy conversion which breaks down the complex structure of LB to release polymers. The pretreatment of LB is an important stage, and choosing the best pretreatment procedure is critical. However, suitable pretreatment procedures must fulfill the specific criteria, including the following: 1) improving the generation of sugars from LB or having the ability to generate sugars eventually via hydrolysis, 2) high resisting ability of the degradation or depletion of sugars, 3) they must limit the creation of by-products that obstruct succeeding hydrolysis and fermentation processes, and 4) the technique must be cost-efficient (Ingle et al., 2019).
The nanotechnology method is widely used in numerous industries, including biofuel production (Rai et al., 2019). In the nanotechnology-based approaches, nanomaterials are used, and due to their smaller size, they can easily penetrate lignocellulosic biomass cell walls and interact with lignocellulosic components (cellulose and hemicellulose) to release monomeric and oligomeric sugars (Rai et al., 2017). Nanomaterials employed for pretreatment are capable of improving the chemistry of biomass at a molecular basis. Magnetic nanoparticles are preferred because they aid in their retrieval out from reaction media for reusing, as a consequence, making the entire procedure more economical (Pena et al., 2012). The usage of nanoparticles or their microemulsion has been shown to have a hydrolytic effect that is identical to that seen with chemical pretreatment for LB processing. This was seen in the study carried out by Pena and a colleague whereby when wheat straw was treated with perfluoroalkylsulfonic (PFS) and alkylsulfonic (AS) acid-functionalized magnetic nanoparticles, it produced 46% that is more sugar than the control (35%). The addition of nanoparticles to PFS and AS acid may have caused the stabilization and allotted hemicellulose hydrolysis which has comparable acid strength of sulfuric acid solutions (Wang et al., 2012). The main advantage of using nanoparticles is their notable function in pretreating LB substances with little quantities while still being recyclable for future usage. In another study of pretreatment, the combined effect of alkaline pretreatment with magnetite (Fe3O4) nanoparticles on rice straw for biogas production was explored. The application of magnetite nanoparticles (MNPs) demonstrated a major advancement in biogas production that increases biogas and methane yield by 100 and 129%, respectively (Khalid et al., 2019). Nanoparticles have been used to improve sugar production at the pretreatment level. Yellow poplar sawdust was processed to 96 percent (w/w) hemicellulose at 175°C using 0.8 percent (w/v) sulfuric acid. The application of sulfuric acid with PFS and AS nanomaterials converted hemicelluloses by 66 and 61%, respectively, at 50- and 400-fold acid levels (Arora et al., 2020).
Nano-shear hybrid alkaline (NSHA) pretreatment is another emerging approach of nano-based pretreatment of LB. This technique involves a combination of high-speed shear and chemical reagent synergy, as well as a gentler thermal action. This reaction commonly is performed in a specific reactor known as nanomixing. The high shearing work axis is transported to the biomass nanostructure, allowing efficient lignin removal with cellulose and hemicellulose exposure in a short time. Wang developed a corn stover pretreatment method using the NSHA technique (Wang et al., 2013). In the study of using the NSHA method, findings indicated that hemicellulose and lignin were removed from the original content, leaving with up to 82% cellulose content (Arora et al., 2020). The synergetic action of cellulase enzymes degraded biomass recalcitrance and created nanoscale polysaccharide agglomeration that can be readily converted to simple sugars by NSHA pretreatment. Indeed, utilizing immobilized cellulase, the NSHA pretreatment technique exhibited a four-fold and five-fold increase in enzymatic degradation of cellulose and hemicellulose into sugars. Owing to the high shear rate of synergic effects, this mechanism indicates benefits in adding high shearing into current pretreatment procedures and many other chemical products (de Oliveira et al., 2017).
In an alternative study of the NSHA pretreatment of corn stover, a positively charged polyelectrolyte composing poly (diallyldimethylammonium chloride) (PDAC) is employed as an additive to alter the cellulosic surfaces and stabilize lignin (Duque Leidy Eugenia Peña, 2013). The morphology and chemical components of pretreated biomass were studied using scanning electron microscopy (SEM) and transmission electron microscopy (TEM). Lignin develops a globular aggregate with PDAC polyelectrolyte, altering the cell wall’s shape. The procedure using polyelectrolyte offers a benefit in the reduction of chemical consumption. Despite the potential advantages, reports on biomass pretreatment utilizing nanoparticles are scarce at the moment, as the application of nanotechnology in biomass refineries is still in its infancy.
2.2 Enzyme Immobilization Using Nanomaterials
Hydrolysis of lignocellulosic biomass utilizing nanoparticles or nanomaterials can be accomplished in two approaches: physical adsorption or covalent bonding of different enzymes on nanomaterials (Elumalai et al., 2018), and employing functionalized nanoparticles (Garlapati et al., 2020). In the biofuel production process, enzymatic hydrolysis is considered a critical stage; however, the stability of the enzymes employed in the process is a significant concern. Nanotechnology is applied in this step as it can help to stabilize these biocatalysts. Nanotechnology has recently advanced to the point that it can be utilized to immobilize enzymes. Immobilizing enzymes on nanostructures is a novel method of improving enzyme catalytic efficiency (Song et al., 2018). The term “nanobiocatalyst” refers to enzymes immobilized on NPs, and it is evidence of nanobiotechnology’s constantly developing and pioneering discoveries (Misson et al., 2015). Enzyme immobilization provides benefits in regards to simple enzyme reutilization, proving itself as a key tool for the development of second-generation biorefineries (Zanuso et al., 2021). Because of the spacer, enzyme immobilization on nanomaterials by cross-linking causes it to be more specific for substrates and more versatile (Ingle et al., 2017).
Adsorption, covalent bonding, entrapment/encapsulation, and cross-linking are the four basic types of immobilization strategies, based on how the enzymes engage with the support or with one another as shown in Figure 3. Each has its pros and cons; for example, the adsorption technique is regarded as a low-cost and straightforward approach. The weakening of the interactions and the possibility of enzyme leakage when medium conditions such as pH, temperature, or polarity are altered are the major disadvantages of this approach (Nguyen and Kim, 2017). Because covalent bonding enhances enzyme stabilization and the generated bonds are strong enough to resist enzyme outflow, it is commonly utilized for enzyme immobilization (Zanuso et al., 2021). Owing to the presence of inhibitors in lignocellulose hydrolysates, the encapsulation technique provides an advantage by its ability to generate a microenvironment that protects the enzymes from hostile environments. Cross-linking is considered a different kind of immobilization approach where it involves the creation of enzyme aggregation, also known as cross-linking enzyme aggregates (CLEAs).
The matrix utilized to immobilize enzymes differs, and it comprises nanofibers, nanoparticles (NPs), and organic metal frames, all of which serve the same purpose (Khoshnevisan et al., 2017). MNPs, nickel nanoparticles (NiNPs), iron nanoparticles (FeNPs), and other metal oxide nanoparticles have all been utilized as transporters for enzyme immobilization. The table below displays nanomaterials used for enzyme immobilization in treating LB.
Carbon nanotubes are a potential material for immobilizing enzymes. In a study by Tang et al., they used the adsorption technique to immobilize glucose oxidase (GOD) on a carbon nanotube (CNT) electrode coated with platinum nanoparticles (Tang et al., 2004). The use of nanotubes enhances the immobilization process. In another study, CD spectra investigation found that lipase bound to carbon nanotubes retained 62 percent of the native lipase’s a-helix composition (Ji et al., 2010). In general, enzyme immobilization on nanoparticles or other relevant support elements was used to improve the enzyme’s characteristics, allowing it to be used in LB pretreatment operations at a lower cost. Immobilization has been shown to counteract several inhibitory effects by preventing inhibitors from binding to the enzyme’s active site. Inhibitor tolerance may have improved as a result of this (Qi et al., 2018). The thermal stability of cellulase enzymes is essential for large-scale industrial lignocellulosic operations because high temperatures enhance biomass degradation. Immobilized cellulases have higher thermal stability, which is one of their greatest benefits for large-scale production (Ahmad and Khare, 2018). One main limitation of cellulase immobilization is that the majority of research on cellulase immobilization employs artificial cellulose as the substrate, for instance, microcrystalline cellulose or carboxymethyl cellulose. The structure of microcrystalline seems to have a consistent diameter of approximately 20–200 mm, which makes for easier mass transport as contrasted to actual LCM substrates (Zanuso et al., 2021). Mo et al. proposed the construction of scaffolds with a larger specific area and open porous structure to give more locations for enzyme immobilization to address these impacts on enzyme immobilization. In addition to offering more surface area for the enzyme to uptake, an open porosity shape makes it easier for the substrate to reach the enzyme (Mo and Qiu, 2020).
3 Nanobiocatalyst for Bioenergy Production
Despite decades of research and development work aimed at increasing the value of biomass, commercialized synthesis of bioenergy such as biodiesel and bioethanol from lignocellulose biomass thus far requires technological and economic improvement. By means of a nanotechnology application, an efficient microbial system incorporating a new metabolic pathway can be employed to produce sustainable biofuels. It is reported that with the application of nanomaterials/nanoparticles during the fermentation process, the total cost of LB to biofuel could be reduced (Singhvi and Kim, 2020). To enhance the production of bioethanol, more cost-effective and a variety of methods employing nanotechnology have been used.
Cellulases, cellobiases, and β-glucosidase were some examples of enzymes that were immobilized on various NPs and employed for the bioethanol fermentation process. This process is proven to exhibit better characteristics versus free enzymes (Datta et al., 2013). In a recent study of employing the immobilization technique, Verma et al. demonstrated that by covalently immobilizing thermostable β-glucosidase produced from Aspergillus niger on magnetic nanoparticle, they were able to preserve approximately 50% of the enzyme following numerous recycling (Alnadari et al., 2020). In performing the continuous process, one obvious benefit of immobilization is the potential to readily retrieve and recycle enzymes, as well as an enzyme-free product recovery and minimal inhibition of end product.
Hydrogenase enzyme plays a key role in the dark fermentation process for the production of biohydrogen. The use of nanoparticles and materials is considered a novel method for enhancing metabolic activity in the biohydrogen generation process. The employment of nanotechnology showed promise in increasing ferredoxin-oxidoreductase activities, resulting in increased electron transfer rates and enhanced catalytic efficiency (Bunker and Smith, 2011). In a separate study of biohydrogen production, sugarcane bagasse feedstock was hydrolyzed in a mixture of diluted sulfuric acid and titanium dioxide nanoparticles under UV light. By using this strategy, sugar and hydrogen production increased by 260 and 127%, respectively (Jafari and Zilouei, 2016). Most of the investigations addressed the interaction that occurs between enzymes and nanomaterials in enhancing the electron transfer rate, thus improving biohydrogen output and productivities. The lignocellulose biomass will be much more receptive to enzyme treatment for substrate hydrolysis into sugars and subsequent microbial fermentation to yield bioenergy and other bio-based products following interactions with nanobiocatalysts.
4 Conclusion and Future Perspective
In conclusion, the depleting fossil fuel reserves have necessitated the exploration and use of alternative resources such as lignocellulosic biomass which is an environmentally friendly approach. To address the challenges associated with biomass transformation, nanotechnology has gained importance in various applications such as in operation of catalysts and for amendments in feedstock. Besides, nanoscale instrumentation such as atomic force microscopy has assisted in measuring cellulose fibril, while other nanotechnology-based instruments have facilitated in comprehending the cell wall ultrastructure, deconstruction microscopic analysis, and enzymatic mechanisms, all of which are extremely beneficial information in the biofuel production field. On the other hand, the exceptional properties of nanomaterials such as large surface area and high catalytic activity are particularly useful to enhance the productivity of bioenergy.
Despite the advantages of nanotechnology and nanomaterials in the generation of bioenergy from lignocellulosic biomasses, there are several concerns that need to be addressed. For instance, immobilization via covalent binding is an essential technique to prevent leaching of enzymes during the reaction, especially while using solid materials as carriers. However, to date, the information relating to interaction between lipase and carrier is still scarce and needs further exploring to fully utilize the potential of nanomaterials in bioenergy generation. Besides, although the in-depth mechanism of toxicity due to nanoparticles has hitherto not been understood, the small size of nanoparticles such as some metal nanocatalyst may disrupt the normal functioning of human cells or induce toxicity among living organisms. Nanoparticles that exist in biofuel, when emitted as exhaust from vehicles or industries, can deposit in lung tissue via respiration, thus causing respiratory ailments such as asthma or bronchitis. As such, safety assessment is of utmost importance and needs to be addressed meticulously to minimize concerns around energy, safety, and environmental issues while utilizing nanomaterials for biofuel generation. A general concern exists with the current pretreatment technique which is considered non-economical for the generation of biofuel such as the hydrolysis process of biomass which consumes significant amount of energy. To overcome this limitation, microwave reactors for chemical processes can be considered to minimize length of reaction, thereby reducing energy consumption. A thorough investigation is also required pertaining to process intensification and its economics while using nanotechnology-based Fischer tropsch synthesis implemented in biomass-derived syngas. Besides, advancement in nanotechnology is also essential to develop more versatile nano-based catalytic systems which are capable of processing a wide variety of biomasses while also persisting impurities that may harm the bioenergy generation process such as acids or alkali metals. Nevertheless, if the overriding problems associated with lignocellulosic biomasses, their processes, and utilization of nanomaterials can be addressed, it is likely to expedite bioenergy generation which can represent a milestone in the green fuel sector.
Author Contributions
RS and KM wrote the first draft of the manuscript. KK, CC, and VA contributed to the conception and design of the topic. BD and PS reviewed the entire manuscript. All authors contributed to manuscript revision, read, and approved the submitted version.
Funding
This research was supported by the MyPAIR-PHC-Hibiscus Grant (MyPAIR/1/2020/STG05/UNIM/1) to Professor Ir Ts PS (Ministry of Higher Education, Malaysia) and Professor Eric Leroy (French Ministry of National Education, Higher Education and Research).
Conflict of Interest
The authors declare that the research was conducted in the absence of any commercial or financial relationships that could be construed as a potential conflict of interest.
Publisher’s Note
All claims expressed in this article are solely those of the authors and do not necessarily represent those of their affiliated organizations, or those of the publisher, the editors, and the reviewers. Any product that may be evaluated in this article, or claim that may be made by its manufacturer, is not guaranteed or endorsed by the publisher.
References
Abdelsalam, E., Samer, M., Attia, Y. A., Abdel-Hadi, M. A., Hassan, H. E., and Badr, Y. (2017). Influence of Zero Valent Iron Nanoparticles and Magnetic Iron Oxide Nanoparticles on Biogas and Methane Production from Anaerobic Digestion of Manure, Internet. Energy 120, 842–853. Available from. doi:10.1016/j.energy.2016.11.137
Abraham, R. E., Verma, M. L., Barrow, C. J., and Puri, M. (2014). Suitability of Magnetic Nanoparticle Immobilised Cellulases in Enhancing Enzymatic Saccharification of Pretreated Hemp Biomass. Biotechnol. Biofuels 7 (1), 90. Dec. doi:10.1186/1754-6834-7-90
Ahmad, R., and Sardar, M. (2014). Immobilization of Cellulase on TiO2 Nanoparticles by Physical and Covalent Methods: A Comparative Study. Indian J. Biochem. Biophys. 51, 314–320.
Ahmad, R., and Khare, S. K. (2018). Immobilization of Aspergillus niger Cellulase on Multiwall Carbon Nanotubes for Cellulose Hydrolysis. Bioresour. Tech. 252, 72–75. Mar. doi:10.1016/j.biortech.2017.12.082
Ali, S., Shafique, O., Mahmood, S., Mahmood, T., Khan, B. A., and Ahmad, I. (2020). Biofuels Production from weed Biomass Using Nanocatalyst Technology. Biomass and Bioenergy 139 (May), 105595. Available from. doi:10.1016/j.biombioe.2020.105595
Alnadari, F., Xue, Y., Zhou, L., Hamed, Y. S., Taha, M., and Foda, M. F. (2020). Immobilization of β-Glucosidase from Thermatoga Maritima on Chitin-Functionalized Magnetic Nanoparticle via a Novel Thermostable Chitin-Binding Domain. Sci. Rep. 10 (1), 1663. Dec. doi:10.1038/s41598-019-57165-5
Arora, A., Nandal, P., Singh, J., and Verma, M. L. (2020). Nanobiotechnological Advancements in Lignocellulosic Biomass Pretreatment. Mater. Sci. Energ. Tech. 3, 308–318. doi:10.1016/j.mset.2019.12.003
Banerjee, S., Rout, S., Banerjee, S., Atta, A., and Das, D. (2019). Fe2O3 Nanocatalyst Aided Transesterification for Biodiesel Production from Lipid-Intact Wet Microalgal Biomass: A Biorefinery Approach. Energ. Convers. Manage. 195, 844–853. doi:10.1016/j.enconman.2019.05.060
Bunker, C. E., and Smith, M. J. (2011). Nanoparticles for Hydrogen Generation. J. Mater. Chem. 21 (33), 12173. doi:10.1039/c1jm10856e
Chang, R. H.-Y., Jang, J., and Wu, K. C.-W. (2011). Cellulase Immobilized Mesoporous Silica Nanocatalysts for Efficient Cellulose-To-Glucose Conversion. Green. Chem. 13 (10), 2844. doi:10.1039/c1gc15563f
Chiang, Y.-D., Dutta, S., Chen, C.-T., Huang, Y.-T., Lin, K.-S., Wu, J. C. S., et al. (2015). Functionalized Fe3O4@Silica Core-Shell Nanoparticles as Microalgae Harvester and Catalyst for Biodiesel Production. ChemSusChem 8 (5), 789–794. doi:10.1002/cssc.201402996
Datta, S., Christena, L. R., and Rajaram, Y. R. S. (2013). Enzyme Immobilization: an Overview on Techniques and Support Materials. 3 Biotech 3 (1), 1–9. Feb. doi:10.1007/s13205-012-0071-7
de Oliveira, J. A. R., da Silva Martins, L. H., Komesu, A., and Neto, J. M. (2017), Nanotechnology Applications on Lignocellulosic Biomass Pretreatment. 19-37. doi:10.1007/978-3-319-45459-7_2
Duque Leidy Eugenia Peña (2013). Acid-Functionalized Nanoparticles for Biomass Hydrolysis. Manhattan, KS: Kansas State University.
Dutta, N., Mukhopadhyay, A., Dasgupta, A. K., and Chakrabarti, K. (2014). Improved Production of Reducing Sugars from rice Husk and rice Straw Using Bacterial Cellulase and Xylanase Activated with Hydroxyapatite Nanoparticles. Bioresour. Tech. 153, 269–277. doi:10.1016/j.biortech.2013.12.016
Elumalai, S., Agarwal, B., Runge, T. M., and Sangwan, R. S. (2018). Advances in Transformation of Lignocellulosic Biomass to Carbohydrate-Derived Fuel Precursors, Internet. Biorefining of Biomass to Biofuels, 87–116. Available from: http://link.springer.com/10.1007/978-3-319-67678-4_4. doi:10.1007/978-3-319-67678-4_4
Garlapati, V. K., Chandel, A. K., Kumar, S. P. J., Sharma, S., Sevda, S., Ingle, A. P., et al. (2020). Circular Economy Aspects of Lignin: Towards a Lignocellulose Biorefinery. Renew. Sustain. Energ. Rev 130, 109977. doi:10.1016/j.rser.2020.109977
Gokhale, A. A., Lu, J., and Lee, I. (2013). Immobilization of Cellulase on Magnetoresponsive Graphene Nano-Supports. J. Mol. Catal. B: Enzymatic 90, 76–86. Jun. doi:10.1016/j.molcatb.2013.01.025
Hamawand, I., Seneweera, S., Kumarasinghe, P., and Bundschuh, J. (2020). Nanoparticle Technology for Separation of Cellulose, Hemicellulose and Lignin Nanoparticles from Lignocellulose Biomass: A Short Review, Internet. Nano-Structures & Nano-Objects 24, 100601. doi:10.1016/j.nanoso.2020.100601
He, Z., Zhang, Z., and Bi, S. (2020). Nanoparticles for Organic Electronics Applications. Mater. Res. Express 7 (1). doi:10.1088/2053-1591/ab636f
Huang, P.-J., Chang, K.-L., Hsieh, J.-F., and Chen, S.-T. (2015). Catalysis of Rice Straw Hydrolysis by the Combination of Immobilized Cellulase fromAspergillus Nigeronβ-Cyclodextrin-Fe3O4Nanoparticles and Ionic Liquid. Biomed. Res. Int. 2015, 1–9. doi:10.1155/2015/409103
Ingle, A. P., Chandel, A. K., Antunes, F. A. F., Rai, M., and da Silva, S. S. (2019). New Trends in Application of Nanotechnology for the Pretreatment of Lignocellulosic Biomass. Biofuels, Bioprod. Bioref. 13 (3), 776–788. doi:10.1002/bbb.1965
Ingle, A. P., Rathod, J., Pandit, R., da Silva, S. S., and Rai, M. (2017). Comparative Evaluation of Free and Immobilized Cellulase for Enzymatic Hydrolysis of Lignocellulosic Biomass for Sustainable Bioethanol Production. Cellulose 24 (12), 5529–5540. Dec. doi:10.1007/s10570-017-1517-1
ISO/TS 27687:2008 Nanotechnologies (2008). Terminology and Definitions for Nano-Objects — Nanoparticle, Nanofibre and Nanoplate [Internet]. Available at: https://www.iso.org/standard/44278.html
Jafari, O., and Zilouei, H. (2016). Enhanced Biohydrogen and Subsequent Biomethane Production from Sugarcane Bagasse Using Nano-Titanium Dioxide Pretreatment. Bioresour. Tech. 214, 670–678. Aug. doi:10.1016/j.biortech.2016.05.007
Ji, P., Tan, H., Xu, X., and Feng, W. (2010). Lipase Covalently Attached to Multiwalled Carbon Nanotubes as an Efficient Catalyst in Organic Solvent. Aiche J. 56 (11), 3005–3011. Nov. doi:10.1002/aic.12180
Jönsson, L. J., and Martín, C. (2016). Pretreatment of Lignocellulose: Formation of Inhibitory By-Products and Strategies for Minimizing Their Effects. Bioresour. Tech. 199, 103–112.
Khalid, M. J., Zeshan, , , Waqas, A., and Nawaz, I. (2019). Synergistic Effect of Alkaline Pretreatment and Magnetite Nanoparticle Application on Biogas Production from rice Straw. Bioresour. Tech. 275, 288–296. Mar. doi:10.1016/j.biortech.2018.12.051
Khoshnevisan, K., Vakhshiteh, F., Barkhi, M., Baharifar, H., Poor-Akbar, E., Zari, N., et al. (2017). Immobilization of Cellulase Enzyme onto Magnetic Nanoparticles: Applications and Recent Advances. Mol. Catal. 442, 66–73. Dec. doi:10.1016/j.mcat.2017.09.006
Li, Y., Wu, Y., and Ong, B. S. (2005). Facile Synthesis of Silver Nanoparticles Useful for Fabrication of High-Conductivity Elements for Printed Electronics. J. Am. Chem. Soc. 127 (10), 3266–3267. doi:10.1021/ja043425k
Lima, J. S., Araújo, P. H. H., Sayer, C., Souza, A. A. U., Viegas, A. C., and de Oliveira, D. (2017). Cellulase Immobilization on Magnetic Nanoparticles Encapsulated in Polymer Nanospheres. Bioproc. Biosyst Eng 40 (4), 511–518. Apr. doi:10.1007/s00449-016-1716-4
Liu, M.-q., Dai, X.-j., Guan, R.-f., and Xu, X. (2014). Immobilization of Aspergillus niger Xylanase A on Fe3O4-Coated Chitosan Magnetic Nanoparticles for Xylooligosaccharide Preparation. Catal. Commun. 55, 6–10. Oct. doi:10.1016/j.catcom.2014.06.002
Madhuvilakku, R., and Piraman, S. (2013). Biodiesel Synthesis by TiO2-ZnO Mixed Oxide Nanocatalyst Catalyzed palm Oil Transesterification Process. Bioresour. Tech. 150, 55–59. doi:10.1016/j.biortech.2013.09.087
Mahmood, T., and Hussain, S. T. (2010). Nanobiotechnology for the Production of Biofuels from Spent tea. Afr. J Biotechnol 9 (6), 858–868.
Misson, M., Zhang, H., and Jin, B. (2015). Nanobiocatalyst Advancements and Bioprocessing Applications. J. R. Soc. Interf. 12 (102), 20140891. Jan. doi:10.1098/rsif.2014.0891
Mo, H., and Qiu, J. (2020). Preparation of Chitosan/Magnetic Porous Biochar as Support for Cellulase Immobilization by Using Glutaraldehyde. Polymers 12 (11), 2672. Nov. doi:10.3390/polym12112672
Nguyen, H. H., and Kim, M. (2017). An Overview of Techniques in Enzyme Immobilization. Appl. Sci. Converg. Technol. 26 (6), 157–163. Nov. doi:10.5757/asct.2017.26.6.157
Pandit, P. R., and Fulekar, M. H. (2019). Biodiesel Production from Microalgal Biomass Using CaO Catalyst Synthesized from Natural Waste Material. Renew. Energ. 136, 837–845. doi:10.1016/j.renene.2019.01.047
Pena, L., Angle, B., Burton, B., and Charrow, J. (2012). Follow-up of Patients with Short-Chain Acyl-CoA Dehydrogenase and Isobutyryl-CoA Dehydrogenase Deficiencies Identified through Newborn Screening: One center's Experience. Genet. Med. 14 (3), 342–347. Mar. doi:10.1038/gim.2011.9
Production, B., Khoo, K. S., Chia, W. Y., Ying, D., Tang, Y., and Show, P. L. (2020). Nanomaterials Utilization in Biomass for Biofuel and Bioenergy Production. Energies 13 (4), 892. doi:10.3390/en13040892
Qi, B., Luo, J., and Wan, Y. (2018). Immobilization of Cellulase on a Core-Shell Structured Metal-Organic Framework Composites: Better Inhibitors Tolerance and Easier Recycling. Bioresour. Tech. 268, 577–582. Nov. doi:10.1016/j.biortech.2018.07.115
Rai, M., Ingle, A. P., Gaikwad, S., Dussán, K. J., and da Silva, S. S. Role of Nanoparticles in Enzymatic Hydrolysis of Lignocellulose in Ethanol. In 2017. p. 153–171.doi:10.1007/978-3-319-45459-7_7
Rai, M., Ingle, A. P., Pandit, R., Paralikar, P., Biswas, J. K., and da Silva, S. S. (2019). Emerging Role of Nanobiocatalysts in Hydrolysis of Lignocellulosic Biomass Leading to Sustainable Bioethanol Production. Catal. Rev. 61 (1), 1–26. doi:10.1080/01614940.2018.1479503
Reddy, K., Nasr, M., Kumari, S., Kumar, S., Gupta, S. K., Enitan, A. M., et al. (2017). Biohydrogen Production from Sugarcane Bagasse Hydrolysate: Effects of pH, S/X, Fe2+, and Magnetite Nanoparticles. Environ. Sci. Pollut. Res. 24 (9), 8790–8804. doi:10.1007/s11356-017-8560-1
Rekha, B., and Saravanathamizhan, R. (2021). Catalytic Conversion of Corncob Biomass into Bioethanol. Int. J. Energ. Res 45 (3), 4508–4518. doi:10.1002/er.6119
Sanusi, I. A., Suinyuy, T. N., and Kana, G. E. B. (2021). Impact of Nanoparticle Inclusion on Bioethanol Production Process Kinetic and Inhibitor Profile. Biotechnol. Rep. 29, e00585. doi:10.1016/j.btre.2021.e00585
Scarlat, N., Dallemand, J.-F., Monforti-Ferrario, F., and Nita, V. (2015). The Role of Biomass and Bioenergy in a Future Bioeconomy: Policies and Facts. Environ. Dev. 15, 3–34. Jul. doi:10.1016/j.envdev.2015.03.006
Shanmugam, S., Hari, A., Pandey, A., Mathimani, T., Felix, L. O., and Pugazhendhi, A. (2020). Comprehensive Review on the Application of Inorganic and Organic Nanoparticles for Enhancing Biohydrogen Production. Fuel 270, 117453. doi:10.1016/j.fuel.2020.117453
Shanmugam, S., Krishnaswamy, S., Chandrababu, R., Veerabagu, U., Pugazhendhi, A., and Mathimani, T. (2020). Optimal Immobilization of Trichoderma Asperellum Laccase on Polymer Coated Fe3O4@SiO2 Nanoparticles for Enhanced Biohydrogen Production from Delignified Lignocellulosic Biomass, Internet. Fuel 273 (January), 117777. Available from. doi:10.1016/j.fuel.2020.117777
Shuttleworth, P. S., De Bruyn, M., Parker, H. L., Hunt, A. J., Budarin, V. L., Matharu, A. S., et al. (2014). Applications of Nanoparticles in Biomass Conversion to Chemicals and Fuels. Green. Chem. 16 (2), 573–584. doi:10.1039/c3gc41555d
Singhvi, M., and Kim, B. S. (2020). Current Developments in Lignocellulosic Biomass Conversion into Biofuels Using Nanobiotechology Approach. Energies 13 (20), 1–20. doi:10.3390/en13205300
Song, J., Lei, T., Yang, Y., Wu, N., Su, P., and Yang, Y. (2018). Attachment of Enzymes to Hydrophilic Magnetic Nanoparticles through DNA-Directed Immobilization with Enhanced Stability and Catalytic Activity. New J. Chem. 42 (11), 8458–8468. doi:10.1039/c8nj00426a
Song, Q., Mao, Y., Mao, Y., Wilkins, M., Segato, F., and Prade, R. (2016). Cellulase Immobilization on Superparamagnetic Nanoparticles for Reuse in Cellulosic Biomass Conversion. AIMS Bioeng. 3 (3), 264–276. doi:10.3934/bioeng.2016.3.264
Srivastava, N., Alhazmi, A., Mohammad, A., Haque, S., Srivastava, M., Pal, D. B., et al. (2021). Biohydrogen Production via Integrated Sequential Fermentation Using Magnetite Nanoparticles Treated Crude Enzyme to Hydrolyze Sugarcane Bagasse. Int. J. Hydrogen Energ.. doi:10.1016/j.ijhydene.2021.08.198
Srivastava, N., Srivastava, M., Mishra, P. K., and Ramteke, P. W. (2016). Application of ZnO Nanoparticles for Improving the thermal and pH Stability of Crude Cellulase Obtained from Aspergillus fumigatus AA001. Front. Microbiol. 7 (APR), 514–519. doi:10.3389/fmicb.2016.00514
Srivastava, N., Rawat, R., Sharma, R., Oberoi, H. S., Srivastava, M., and Singh, J. (2014). Effect of Nickel-Cobaltite Nanoparticles on Production and Thermostability of Cellulases from Newly Isolated Thermotolerant Aspergillus fumigatus NS (Class: Eurotiomycetes). Appl. Biochem. Biotechnol. 174, 1092–1103. doi:10.1007/s12010-014-0940-0
Srivastava, N., Singh, J., Ramteke, P. W., Mishra, P. K., and Srivastava, M. (2015). Improved Production of Reducing Sugars from rice Straw Using Crude Cellulase Activated with Fe3O4/Alginate Nanocomposite. Bioresour. Tech. 183, 262–266. doi:10.1016/j.biortech.2015.02.059
Sun, Y., and Cheng, J. (2002). Hydrolysis of Lignocellulosic Materials for Ethanol Production: A Review. Bioresour. Tech. 83 (1), 1–11. doi:10.1016/s0960-8524(01)00212-7
Tahir, N., Tahir, M. N., Alam, M., Yi, W., and Zhang, Q. (2020). Exploring the Prospective of Weeds (Cannabis Sativa L., Parthenium Hysterophorus L.) for Biofuel Production through Nanocatalytic (Co, Ni) Gasification. Biotechnol. Biofuels 13 (1), 1–10. Available from. doi:10.1186/s13068-020-01785-x
Tang, H., Chen, J., Yao, S., Nie, L., Deng, G., and Kuang, Y. (2004). Amperometric Glucose Biosensor Based on Adsorption of Glucose Oxidase at Platinum Nanoparticle-Modified Carbon Nanotube Electrode. Anal. Biochem. 331 (1), 89–97. Aug. doi:10.1016/s0003-2697(04)00411-7
Valdivia, M., Galan, J. L., Laffarga, J., and Ramos, J. L. (2016). Biofuels 2020: Biorefineries Based on Lignocellulosic Materials. Microb. Biotechnol. 9 (5), 585–594. Sep. doi:10.1111/1751-7915.12387
Wang, D., Ikenberry, M., Peña, L., and Hohn, K. L. (2012). Acid-Functionalized Nanoparticles for Pretreatment of Wheat Straw. Jbnb 03 (03), 342–352. doi:10.4236/jbnb.2012.33032
Wang, H., Covarrubias, J., Prock, H., Wu, X., Wang, D., and Bossmann, S. H. (2015). Acid-Functionalized Magnetic Nanoparticle as Heterogeneous Catalysts for Biodiesel Synthesis. J. Phys. Chem. C 119 (46), 26020–26028. Nov. doi:10.1021/acs.jpcc.5b08743
Wang, W., Ji, S., and Lee, I. (2013). Fast and Efficient Nanoshear Hybrid Alkaline Pretreatment of Corn stover for Biofuel and Materials Production. Biomass and Bioenergy 51, 35–42. Apr. doi:10.1016/j.biombioe.2012.12.037
Xie, W., and Ma, N. (2009). Immobilized Lipase on Fe3O4 Nanoparticles as Biocatalyst for Biodiesel Production. Energy Fuels 23 (3), 1347–1353. InternetMar 19Available from: https://pubs.acs.org/doi/10.1021/ef800648y. doi:10.1021/ef800648y
Yang, G., and Wang, J. (2018). Improving Mechanisms of Biohydrogen Production from Grass Using Zero-Valent Iron Nanoparticles. Bioresour. Tech. 266 (May), 413–420. Available from:. doi:10.1016/j.biortech.2018.07.004
Zanuso, E., Gomes, D. G., Ruiz, H. A., Teixeira, J. A., and Domingues, L. (2021). Enzyme Immobilization as a Strategy towards Efficient and Sustainable Lignocellulosic Biomass Conversion into Chemicals and Biofuels: Current Status and Perspectives. Sustain. Energ. Fuels 5 (17), 4233–4247. doi:10.1039/d1se00747e
Zhang, X., Yan, S., Tyagi, R. D., and Surampalli, R. Y. (2011). Synthesis of Nanoparticles by Microorganisms and Their Application in Enhancing Microbiological Reaction Rates. Chemosphere 82 (4), 489–494. Available from. doi:10.1016/j.chemosphere.2010.10.023
Keywords: lignocellulose, bioconversion, pretreatment, nanotechnology, bioenergy
Citation: Sankaran R, Markandan K, Khoo KS, Cheng CK, Ashokkumar V, Deepanraj B and Show PL (2021) The Expansion of Lignocellulose Biomass Conversion Into Bioenergy via Nanobiotechnology. Front. Nanotechnol. 3:793528. doi: 10.3389/fnano.2021.793528
Received: 12 October 2021; Accepted: 29 November 2021;
Published: 20 December 2021.
Edited by:
Eldon R. Rene, IHE Delft Institute for Water Education, NetherlandsReviewed by:
Jingmiao Zhang, Chongqing University, ChinaMukesh Kumar Awasthi, Northwest A and F University, China
HC Ong, National Yunlin University of Science and Technology, Taiwan
Copyright © 2021 Sankaran, Markandan, Khoo, Cheng, Ashokkumar, Deepanraj and Show. This is an open-access article distributed under the terms of the Creative Commons Attribution License (CC BY). The use, distribution or reproduction in other forums is permitted, provided the original author(s) and the copyright owner(s) are credited and that the original publication in this journal is cited, in accordance with accepted academic practice. No use, distribution or reproduction is permitted which does not comply with these terms.
*Correspondence: Kuan Shiong Khoo, a3VhbnNoaW9uZy5raG9vQGhvdG1haWwuY29t, Chin Kui Cheng, Y2hlbmcua3VpQGt1LmFjLmFl, Pau Loke Show, c2hvd3BhdWxva2VAZ21haWwuY29t