- Department of Chemistry, University of Copenhagen, Copenhagen, Denmark
Colloidal syntheses of nanoparticles (NPs) are one of the preferred approaches to prepare precious metal catalysts. Unfortunately, most colloidal syntheses developed require stabilizing agents to avoid NP agglomeration and/or control NP size and morphology. While these surfactants can bring positive features, they typically block catalytically active sites on the NP surface. As a consequence, these additives often need to be removed by energy and/or time consuming steps, at the risk of complicating the synthesis, introducing irreproducibility and negatively altering the structure and properties of the prepared catalysts. Fortunately, several surfactant-free colloidal syntheses have been reported and are being developed. This Mini Review addresses the challenges in defining a surfactant-free colloidal synthesis of NPs and survey established and emerging strategies to obtain surfactant-free colloidal precious metal NPs. A focus is given to approaches that show promising features to bridge the gap between fundamental and applied research towards industrial applications.
Introduction
Nanoparticles (NPs) have found applications in a vast number of fields, ranging from medicine, imaging, sensing, water/air remediation, energy conversion or catalysis. While the benefits of NPs to address various challenges does not need to be demonstrated anymore, there is still a gap between fundamental research and the development of a nanotechnology (Stavis et al., 2018). Part of this gap comes from the production method of the NPs. Wet-chemical methods are commonly used to obtain NPs since a wide library of nanomaterials can be produced. In this bottom-up approach, a precursor molecule containing an atom of the desired element, e.g., H2PtCl6 for Pt (Quinson and Jensen 2020), is reduced in a solvent, often in presence of reducing agents and stabilizers. Some chemicals can play several roles during the synthesis, e.g., sodium citrate is both a reducing agent and stabilizer in the popular Turkevich synthesis of gold NPs (Wuithschick et al., 2015). It is very often claimed that surfactants/stabilizers/ligands or capping agents are needed to stabilize the NPs (Heuer-Jungemann et al., 2019). Examples of such stabilizers are polymers or long carbon chain molecules such as polyvinylpyrrolidone (PVP) or cetrimonium bromide (CTAB), plant extracts or DNA (Koczkur et al., 2015). The role of these additives - in the sense that these chemicals are not needed to reduce metal complexes and to form nano- or micro-materials in solution from molecules containing only one or few atoms of a transition metal element - is to avoid the undesirable agglomeration and overgrowth of the NPs in order to obtain stable colloids. The actual role of these additives during the formation of the NPs is complex to elucidate since they typically have multiple roles. For instance PVP is a dual protecting and reducing agent. This complexity prevents a detailed understanding of NP formation.
While additives allow controlling the stability but also the structure of the nanomaterials produced, they bring several challenges. 1) The additives are often toxic and their use raise safety concerns, especially at large scale (Johnson et al., 2021). 2) The additives typically derive from fossil fuels, which is not in the long term a sustainable approach to produce NPs. 3) Using additives add to the cost of a synthesis. 4) Using additives may bring impurities that reduce reproducibility, thus may impair the scalability of the NP synthesis (El Amri and Roger 2020). 5) For a range of applications like catalysis, the additives need to be removed (Cargnello et al., 2015). This is typically achieved by washing and/or heat treatments that add steps to the catalyst preparation and may impair reproducibility by potentially altering the structure of the prepared NPs, see Figure 1. The presence of residual surfactants on the NP surface might also impair further functionalization when the latter is desired, e.g., for medical applications or catalysis. 6) To advance our understanding of NP properties, the surfactant removal implies a full characterization of the as-prepared catalyst but also the cleaned catalyst, which is time and resource consuming (Losch et al., 2019).
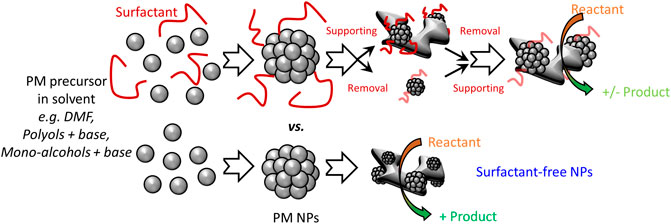
FIGURE 1. Schematic representation of some colloidal syntheses of PM NPs and illustration of the benefits of surfactant-free approaches to develop supported catalysts with controlled NP size and loading.
Colloidal syntheses have been a key tool to advance the field of catalysis (Guntern et al., 2021; Losch et al., 2019) and strategies to overcome the above drawbacks have been reported. For heterogeneous catalytic applications and electrocatalysis, the NPs are typically supported on another material. A typical approach to overcome the use of surfactants is therefore to performed so-called one-pot syntheses where the NPs are obtained directly on the support. Unfortunately, this approach is support-dependent and requires the optimization of various syntheses parameters for every new support in order to obtain NPs with the same size or composition and reach the desired loading of NPs on the support. Furthermore, the NPs might form in parts of the support like pores not accessible during catalytic reactions (Park et al., 2016). This is a severe drawback for precious metal (PM) NPs, made of non-renewable and expensive materials, which are yet among the best-known materials for a range of chemical reactions and well-established industrial catalysts. To advance further the design of NP catalysts, surfactant-free colloidal syntheses are highly desirable, 1) to simplify the synthesis of NPs and make is safer, 2) to simplify the (large scale) use and processing of the NPs, 3) to develop catalysts readily active without the need for washing and/or activation steps. This ultimately reduce production cost and makes it more straightforward to transfer the knowledge gained in fundamental research to industrial applications: With a surfactant-free synthesis, the same production method of the catalyst would be suitable for both the fundamental and applied research involved in a catalyst development (Quinson et al., 2018).
While it is a popular belief, there is actually no need to use surfactants for a number of colloidal synthetic approaches to be successful. The last comprehensive review, not technique specific, on the topic of surfactant-free synthesis is probably from 2013 by Prof Kawasaki (Kawasaki 2013). The present Mini-Review therefore focuses on work published after this date and new strategies that emerged since. The pros and cons of commonly used syntheses are highlighted. A focus is given to applications for catalysis. In particular, electro-catalytic reactions performed at room temperature benefit from surfactant-free NPs. Since in catalysis the size of the NPs strongly influence the resulting properties, the opportunities to achieve size control without using surfactants are stressed.
Overview of Surfactant-Free Colloidal Syntheses
A surfactant-free synthesis is challenging to define (Kawasaki 2013; Niu and Li 2014). From thermodynamics arguments, a NP will be stabilized in solutions by small molecules, solvent and/or electrostatic interactions. “Unprotected” NPs (Schrader et al., 2015) obtained by surfactant-free approaches here refer to syntheses where no chemicals with a molar mass greater than 100 g mol−1 is added during the synthesis. While they are commonly referred to as surfactant-free, this definition therefore excludes the majority of syntheses using benzyl-alcohol, methyl isobutyl ketone, amino-acids, plants extracts or biogenic syntheses using for instance micro-organisms.
Laser Synthesis and Processing of Colloids
The most established method to develop surfactant-free NPs is probably the laser synthesis and processing of colloids (Zhang et al., 2017). In a nutshell, in this top-down approach, a piece of metal plays the role of target for a laser beam, from which NPs form by a cavitation phenomenon. Alternatively, NPs properties can be modulated by fragmentation and melting still using a laser. The method has been extensively reviewed, especially by Prof Barcikowski and co-workers (Reichenberger et al., 2019; Zhang et al., 2017). It requires relatively simple equipment, is scalable and suitable to obtain numerous PM NPs of various compositions, e.g., by controlling the composition of the target material. Examples of NPs obtained include Au, Ag, Pt, Pd based NPs and even multi-metallic (Reichenberger et al., 2019). Since it easily leads to bare NPs mainly stabilized by electrostatic interaction, it has been used to study the effect of chemical functionalization in toxicology, medicine or catalysis.
The main drawback of this approach is the size distribution obtained for as-produced NPs, in the range of few nm up to micrometers, typically showing a bimodal distribution, that requires post-treatment like centrifugation to isolate NPs of a given size range (Kohsakowski et al., 2020). Furthermore, the synthesis require the optimization of a vast range of experimental parameters such as laser fluence, pulse duration, repetition rate or laser wavelength, and therefore a certain degree of expertise.
Plasma Synthesis
In this relatively less common approach, an electrode is used to generate a plasma and surfactant-free colloidal NPs stabilized by electrostatic interaction are obtained from inorganic complexes. The opportunities offered by this approach have been reviewed by Prof Kim and co-workers (Mun et al., 2017). The main drawback of this approach is maybe to require somewhat specific equipment. Metal NPs like Au, Ag, Pt in the size range 2–30 nm were obtained.
N,N-Dimethylformamide Synthesis
Wet chemical syntheses remain relatively easier to perform since only simple reflux setups or autoclaves are needed. The use of N,N-Dimethylformamide (DMF) as solvent, reducing and stabilizing agent has proved to lead to small NPs. In many cases, a stabilizer like PVP is added to the synthesis but it is actually not needed. In particular, Prof Obora and co-workers demonstrated the benefits of this approach for various catalytic reactions (Nagata and Obora 2020). A wide range of NPs such as Au, Ag, Pd, Pt, Ir, Rh were obtained as well as PtNi nanomaterials (Cui et al., 2012) showing high activity for the oxygen reduction reaction (ORR). A unique feature of this approach is to lead to small NPs less than 10 nm in size or even to nanoclusters made of few atoms. The main drawbacks is the use of a relatively toxic solvent, the use of relatively high temperature and the possible need to partially remove the stabilizing DMF, e.g., by heat treatment.
Polyol Synthesis
The polyols synthesis first reported and recently reviewed by Fiévet and co-workers (Fiévet et al., 2018) is an extremely popular synthesis method since it is performed in alkaline ethylene glycol, a relatively safe solvent, with a relatively high boiling point temperature, allowing to produce a very wide range of nanomaterials. In many cases, additives are added. However, since the pioneer work by Wang and co-workers with Prof Tang (Wang et al., 2000), it is well-established that no surfactants are required to obtain a range of colloidal PM NPs with a size controlled in the range 2–10 nm, including bi-metallic. The resulting “unprotected” NPs, in the sense that they are stabilized typically by CO groups and OH/OH− moieties (Schrader et al., 2015), are readily active for a range of catalytic reactions. Size control is typically achieved by adding water or controlling the Base/metal ratio. Due to its simplicity, it has also been a suitable model to study the formation mechanism of NPs. A positive feature of this approach is to lead to NPs that can be stored as unsupported powders and re-dispersed in a desired solvent (Neumann et al., 2017).
The “unprotected” NPs are ideal building blocks to study and develop a range of catalysts (Quinson et al., 2021) and Pt, Ir, Ru, Rh or Os NPs and various bi-metallic have been reported. Since several types of NPs can be obtained, the method is also suitable to investigate the effect of alloys or nanocomposites made of a mixture of NPs (Du et al., 2021). This approach is however more challenging to control to obtain small size Au, Ag and Pd NPs since the latter tend to overgrow. To date, Au NPs are best obtained using glycerol as reducing agent in mixture with water (Parveen et al., 2019). Due to the high redox potential of Au, the synthesis proceeds at room temperature. However the high viscosity of glycerol prevents the simple use of the colloidal NPs for catalysis and one-pot syntheses are preferred.
Another drawback of the polyol method is the need for washing steps or phase extraction to flocculate the NPs and/or separate them from the highly viscous polyol before the NPs can be used further, e.g., before being supported (Quinson et al., 2021). These steps are typically performed in concentrated acid and their influence on the properties of the NPs is not well established.
Mono-Alcohol Synthesis
While mono-alcohols like methanol and ethanol have long been used a solvents and reducing agents for NP synthesis, the need for surfactants was almost systematically stressed, until recently. We reported an alternative to the polyol-synthesis where alkaline methanol and ethanol and their mixtures with water were shown to be suitable solvents and reducing agents to develop surfactant-free PM NPs like Pt, Ir, Ru or Os (Quinson et al., 2018) and multi-mettalic. This so called Co4CatTM technology (Colloids for Catalysts) approach combines the benefits of the polyols synthesis but differs in that the synthesis can be performed at relatively low temperature (<100°C) and no flocculation steps is needed: Due to the low boiling point solvents used, the small NPs (<10 nm) can be readily supported by simple solvent evaporation. The solvent can be recovered and re-used to obtain NPs. Pt NPs prepared by this approach show higher activity than commercial catalyst benchmark for butanone hydrogenation since small size and well-dispersed NPs are obtained on a support. Ir NPs show up to ten times more activity compared to the state-of-the-art for the oxygen evolution reaction (OER) due to the small size (ca. 1.6 nm) of the surfactant-free NPs.
Discussion
Avoiding surfactants to develop colloidal NPs for catalysis is 1) well documented, 2) allowing size control, 3) simpler than using surfactants, 4) beneficial to develop improved catalysts, 5) more likely to result in approaches with the potential to develop profitable businesses. In lights of the benefits of surfactant-free syntheses, they are relatively less implemented that the counterpart syntheses-with-additives. This can be inferred to two main factors. (1) It is a popular belief that surfactants are absolutely needed to obtain colloids. This belief might have prevented researchers to explore strategies without additives. (2) Control over shape and crystallographic structure has been shown to possibly bring positive features for catalysis. It is true that morphology control remains relatively more complex and challenging without additives. Nevertheless, it must be noted that if shape control is achieved with surfactants, the integrity of the as-produced structure might not be maintained with attempts to remove the surfactants in order to optimize the number of active sites on the NP surface, or may not even be maintained under catalytic operation.
With regard to shape control, it must be pointed out that if surfactant-free syntheses are easier they are not necessarily simpler and might be sensitive to parameters screened in approaches using surfactants. For example, it was shown that the nature of the cation present in the base used in alkaline methanol synthesis influences the stability of the surfactant-free Pt NPs obtained (Quinson et al., 2019). This observation suggests that some degree of shape control might be possible with simple inorganic salts. In the glycerol based synthesis of Au NPs, a mixture of rods and NPs were obtained (Nalawade et al., 2013). It is anticipated that the wide range of polyols and mono-alcohols available are likely to lead under the right experimental conditions to a certain degree of shape control without adding extra shape-directing agents.
Beyond active site design, i.e., beyond the optimization of catalyst structure, a range of parametric studies can be performed using surfactant-free NPs, for instance to optimize the nature of support and loading for a given catalytic reaction. It has been shown recently using the polyol synthesis in ethylene glycol that supported small size NPs close to each other without agglomeration could lead to a significant increase in mass activity (Inaba et al., 2021). This inter-particle proximity effect, that origins in an overlap in the electric double layer structure at the catalyst-electrolyte interface, was successfully exploited to develop improved catalysts for the ORR, see Figure 2. The preparation of the catalyst to observe this effect was greatly facilitated by the use of surfactant-free NPs. It was also shown using surfactant-free NPs that the key optimization of inks for electrochemical purposes was strongly dependent on the synthesis protocol of the NPs (Inaba et al., 2017). The influence of solvents used for the synthesis in mono-alcohols and the solvent used for re-dispersion and supporting steps was also studied to develop OER catalysts. It was shown that beyond developing the right Ir NPs in the right solvent, the properties of the solvent used to disperse the support material strongly influence the final catalytic activity (Bizzotto et al., 2021). As a consequence of the high activity of surfactant-free Ir NPs, benchmarking protocols for testing Ir NP activity for the OER could be developed (Bizzotto et al., 2019). Last, “unprotected” NPs are valuable tools and control materials to evaluate the effects of ligands on NP catalytic properties, to further understand how to best functionalize NPs for catalysis for instance by ligand design (Schrader et al., 2015).
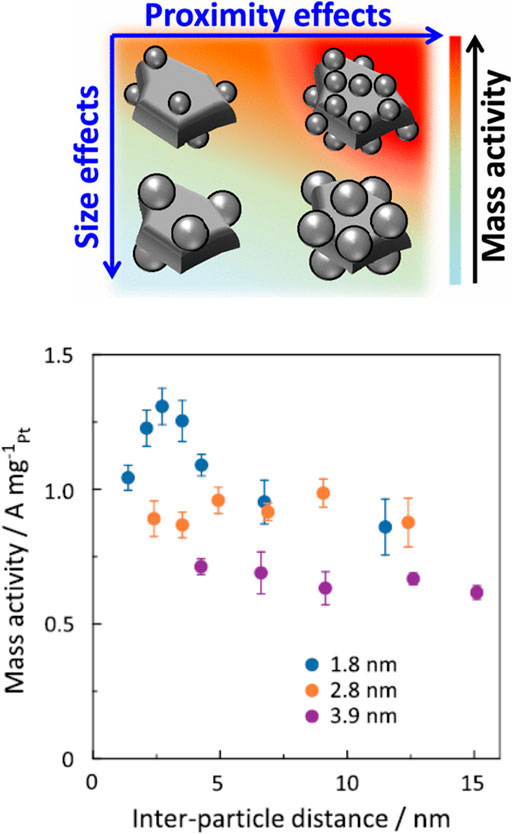
FIGURE 2. ORR mass activity at 0.9 V versus the relative hydrogen electrode for Pt NPs supported on carbon with various Pt sizes and loadings and so different inter-particle distances. Reproduced with permission from (Inaba et al., 2021) Copyright 2021 American Chemical Society.
For lab-scale experiments, relatively small quantities of materials are needed. To this extent, how the NPs are obtained is not a bottleneck. However, transferring a colloidal synthesis to larger scale, though it is possible, will gain from an approach using safe, cheap and few chemicals and compatibility with flow chemistry (Sui et al., 2020), as it is the case for the laser synthesis and processing of colloids approach. For PM NPs, the expensive price of the raw materials is not a variable easily controllable. As a consequence, any minor improvement in the synthesis of the PM NPs can have significant economic and ecological impact. Lower energy requirements, e.g., via lower temperature or even room temperature processes, would be ideal and the solvent used for the synthesis should be easily recycled to reduce even more the related costs of production. In this respect, the use of ethanol as solvent is a promising alternative (Quinson et al., 2018). Although this point has not been investigated further, the fact that a base like NaOH is needed in the mono-alcohol syntheses suggest that the dry crystal could be recovered during the supporting step of the NPs when solvent evaporation is performed. This could ultimately allow developing a synthesis with the relatively safe chemicals that are ethanol and NaOH, and the latter being recycled few times for several syntheses. Importantly, preferring surfactant-free syntheses for fundamental research makes the findings more directly transferrable to larger scale in order to bridge the gap between academia and industry.
Conclusion
Surfactant-free colloidal syntheses of PM NPs are promising and probably overlooked approaches to develop NPs directly relevant for catalytic applications. Several surfactant-free colloidal synthetic strategies have been developed over the years, showing that it is indeed possible to develop stable colloids without surfactants. With the pioneer works reviewed here in mind, it is anticipated that exploring further surfactant-free colloidal syntheses of PM NPs will bring further benefits to fundamental as well as applied research and development in general but especially in the field of catalysis.
Author Contributions
JQ: conceptualization, writing, review, editing.
Funding
JQ acknowledges the European Union’s Horizon 2020 research and innovation programme under the Marie Skłodowska-Curie grant agreement No 840523 (CoSolCat).
Conflict of Interest
The author declares that the research was conducted in the absence of any commercial or financial relationships that could be construed as a potential conflict of interest.
Publisher’s Note
All claims expressed in this article are solely those of the authors and do not necessarily represent those of their affiliated organizations, or those of the publisher, the editors and the reviewers. Any product that may be evaluated in this article, or claim that may be made by its manufacturer, is not guaranteed or endorsed by the publisher.
References
Amri, N. E., and Roger, K. (2020). Polyvinylpyrrolidone (PVP) Impurities Drastically Impact the Outcome of Nanoparticle Syntheses. J. Colloid Interf. Sci. 576, 435–443. doi:10.1016/j.jcis.2020.04.113
Bizzotto, F., Quinson, J., Schröder, J., Zana, A., and Arenz, M. (2021). Surfactant-free Colloidal Strategies for Highly Dispersed and Active Supported IrO2 Catalysts: Synthesis and Performance Evaluation for the Oxygen Evolution Reaction. J. Catal. 401, 54–62. doi:10.1016/j.jcat.2021.07.004
Bizzotto, F., Quinson, J., Zana, A., Kirkensgaard, J. J. K., Dworzak, A., Oezaslan, M., et al. (2019). Ir Nanoparticles with Ultrahigh Dispersion as Oxygen Evolution Reaction (OER) Catalysts: Synthesis and Activity Benchmarking. Catal. Sci. Technol. 9, 6345–6356. doi:10.1039/c9cy01728c
Cargnello, M., Chen, C., Diroll, B. T., Doan-Nguyen, V. V. T., Gorte, R. J., and Murray, C. B. (2015). Efficient Removal of Organic Ligands from Supported Nanocrystals by Fast thermal Annealing Enables Catalytic Studies on Well-Defined Active Phases. J. Am. Chem. Soc. 137, 6906–6911. doi:10.1021/jacs.5b03333
Cui, C., Gan, L., Li, H.-H., Yu, S.-H., Heggen, M., and Strasser, P. (2012). Octahedral PtNi Nanoparticle Catalysts: Exceptional Oxygen Reduction Activity by Tuning the alloy Particle Surface Composition. Nano Lett. 12, 5885–5889. doi:10.1021/nl3032795
Du, J., Quinson, J., Zana, A., and Arenz, M. (2021). Elucidating Pt-Based Nanocomposite Catalysts for the Oxygen Reduction Reaction in Rotating Disk Electrode and Gas Diffusion Electrode Measurements. ACS Catal. 11, 7584–7594. doi:10.1021/acscatal.1c01496
Fiévet, F., Ammar-Merah, S., Brayner, R., Chau, F., Giraud, M., Mammeri, F., et al. (2018). The Polyol Process: a Unique Method for Easy Access to Metal Nanoparticles with Tailored Sizes, Shapes and Compositions. Chem. Soc. Rev. 47, 5187–5233. doi:10.1039/c7cs00777a
Guntern, Y. T., Okatenko, V., Pankhurst, J., Varandili, S. B., Iyengar, P., Koolen, C., et al. (2021). Colloidal Nanocrystals as Electrocatalysts with Tunable Activity and Selectivity. ACS Catal. 11, 1248–1295. doi:10.1021/acscatal.0c04403
Heuer-Jungemann, A., Feliu, N., Bakaimi, I., Hamaly, M., Alkilany, A., Chakraborty, I., et al. (2019). The Role of Ligands in the Chemical Synthesis and Applications of Inorganic Nanoparticles. Chem. Rev. 119, 4819–4880. doi:10.1021/acs.chemrev.8b00733
Inaba, M., Quinson, J., and Arenz, M. (2017). pH Matters: The Influence of the Catalyst Ink on the Oxygen Reduction Activity Determined in Thin Film Rotating Disk Electrode Measurements. J. Power Sourc. 353, 19–27. doi:10.1016/j.jpowsour.2017.03.140
Inaba, M., Zana, A., Quinson, J., Bizzotto, F., Dosche, C., Dworzak, A., et al. (2021). The Oxygen Reduction Reaction on Pt: Why Particle Size and Interparticle Distance Matter. ACS Catal. 11, 7144–7153. doi:10.1021/acscatal.1c00652
Johnson, P., Trybala, A., Starov, V., and Pinfield, V. J. (2021). Effect of Synthetic Surfactants on the Environment and the Potential for Substitution by Biosurfactants. Adv. Colloid Interf. Sci. 288, 102340. doi:10.1016/j.cis.2020.102340
Kawasaki, H. (2013). Surfactant-free Solution-Based Synthesis of Metallic Nanoparticles toward Efficient Use of the Nanoparticles' Surfaces and Their Application in Catalysis and Chemo-/biosensing. Nanotechnol. Rev. 2, 5–25. doi:10.1515/ntrev-2012-0079
Koczkur, K. M., Mourdikoudis, S., Polavarapu, L., and Skrabalak, S. E. (2015). Polyvinylpyrrolidone (PVP) in Nanoparticle Synthesis. Dalton Trans. 44, 17883–17905. doi:10.1039/c5dt02964c
Kohsakowski, S., Seiser, F., Wiederrecht, J.-P., Reichenberger, S., Vinnay, T., Barcikowski, S., et al. (2020). Effective Size Separation of Laser-Generated, Surfactant-free Nanoparticles by Continuous Centrifugation. Nanotechnology 31, 095603. doi:10.1088/1361-6528/ab55bd
Losch, P., Huang, W., Goodman, E. D., Wrasman, C. J., Holm, A., Riscoe, A. R., et al. (2019). Colloidal Nanocrystals for Heterogeneous Catalysis. Nano Today 24, 15–47. doi:10.1016/j.nantod.2018.12.002
Mun, M. K., Lee, W. O., Park, J. W., Kim, D. S., Yeom, G. Y., and Kim, D. W. (2017). Nanoparticles Synthesis and Modification Using Solution Plasma Process. Appl. Sci. Converg. Technol. 26, 164–173. doi:10.5757/asct.2017.26.6.164
Nagata, T., and Obora, Y. (2020). N,N-dimethylformamide-protected Single-Sized Metal Nanoparticles and Their Use as Catalysts for Organic Transformations. ACS Omega 5, 98–103. doi:10.1021/acsomega.9b03828
Nalawade, P., Mukherjee, T., and Kapoor, S. (2013). Green Synthesis of Gold Nanoparticles Using Glycerol as a Reducing Agent. Adv. Nanoparticles 2, 76–86. doi:10.4236/anp.2013.22014
Neumann, S., Grotheer, S., Tielke, J., Schrader, I., Quinson, J., Zana, A., et al. (2017). Nanoparticles in a Box: a Concept to Isolate, Store and Re-use Colloidal Surfactant-free Precious Metal Nanoparticles. J. Mater. Chem. A. 5, 6140–6145. doi:10.1039/c7ta00628d
Niu, Z., and Li, Y. (2014). Removal and Utilization of Capping Agents in Nanocatalysis. Chem. Mater. 26, 72–83. doi:10.1021/cm4022479
Park, Y.-C., Tokiwa, H., Kakinuma, K., Watanabe, M., and Uchida, M. (2016). Effects of Carbon Supports on Pt Distribution, Ionomer Coverage and Cathode Performance for Polymer Electrolyte Fuel Cells. J. Power Sourc. 315, 179–191. doi:10.1016/j.jpowsour.2016.02.091
Parveen, R., Ullah, S., Sgarbi, R., and Tremiliosi-Filho, G. (2019). One-pot Ligand-free Synthesis of Gold Nanoparticles: The Role of Glycerol as Reducing-Cum-Stabilizing Agent. Colloids Surf. A: Physicochemical Eng. Aspects 565, 162–171. doi:10.1016/j.colsurfa.2019.01.005
Quinson, J., Bucher, J., Simonsen, S. B., Kuhn, L. T., Kunz, S., and Arenz, M. (2019). Monovalent Alkali Cations: Simple and Eco-Friendly Stabilizers for Surfactant-free Precious Metal Nanoparticle Colloids. ACS Sustainable Chem. Eng. 7, 13680–13686. doi:10.1021/acssuschemeng.9b00681
Quinson, J., and Jensen, K. M. Ø. (2020). From Platinum Atoms in Molecules to Colloidal Nanoparticles: A Review on Reduction, Nucleation and Growth Mechanisms. Adv. Colloid Interf. Sci. 286, 102300. doi:10.1016/j.cis.2020.102300
Quinson, J., Kunz, S., and Arenz, M. (2021). Beyond Active Site Design: A Surfactant‐Free Toolbox Approach for Optimized Supported Nanoparticle Catalysts. ChemCatChem 13, 1692–1705. doi:10.1002/cctc.202001858
Quinson, J., Neumann, S., Wannmacher, T., Kacenauskaite, L., Inaba, M., Bucher, J., et al. (2018). Colloids for Catalysts: A Concept for the Preparation of superior Catalysts of Industrial Relevance. Angew. Chem. Int. Ed. 57, 12338–12341. doi:10.1002/anie.201807450
Reichenberger, S., Marzun, G., Muhler, M., and Barcikowski, S. (2019). Perspective of Surfactant‐Free Colloidal Nanoparticles in Heterogeneous Catalysis. ChemCatChem 11, 4489–4518. doi:10.1002/cctc.201900666
Schrader, I., Warneke, J., Backenköhler, J., and Kunz, S. (2015). Functionalization of Platinum Nanoparticles with L-Proline: Simultaneous Enhancements of Catalytic Activity and Selectivity. J. Am. Chem. Soc. 137, 905–912. doi:10.1021/ja511349p
Schrader, I., Warneke, J., Neumann, S., Grotheer, S., Swane, A. A., Kirkensgaard, J. J. K., et al. (2015). Surface Chemistry of "unprotected" Nanoparticles: A Spectroscopic Investigation on Colloidal Particles. J. Phys. Chem. C. 119, 17655–17661. doi:10.1021/acs.jpcc.5b03863
Stavis, S. M., Fagan, J. A., Stopa, M., and Liddle, J. A. (2018). Nanoparticle Manufacturing - Heterogeneity through Processes to Products. ACS Appl. Nano Mater. 1, 4358–4385. doi:10.1021/acsanm.8b01239
Sui, J., Yan, J., Liu, D., Wang, K., and Luo, G. (2020). Continuous Synthesis of Nanocrystals via Flow Chemistry Technology. Small 16, 1902828. doi:10.1002/smll.201902828
Wang, Y., Ren, J., Deng, K., Gui, L., and Tang, Y. (2000). Preparation of Tractable Platinum, Rhodium, and Ruthenium Nanoclusters with Small Particle Size in Organic media. Chem. Mater. 12, 1622–1627. doi:10.1021/cm0000853
Wuithschick, M., Birnbaum, A., Witte, S., Sztucki, M., Vainio, U., Pinna, N., et al. (2015). Turkevich in New Robes: Key Questions Answered for the Most Common Gold Nanoparticle Synthesis. ACS Nano 9, 7052–7071. doi:10.1021/acsnano.5b01579
Keywords: nanoparticles, precious metals, colloids, surfactant-free, catalysis, polyols, mono-alcohols, synthesis
Citation: Quinson J (2021) Surfactant-Free Precious Metal Colloidal Nanoparticles for Catalysis. Front. Nanotechnol. 3:770281. doi: 10.3389/fnano.2021.770281
Received: 03 September 2021; Accepted: 04 October 2021;
Published: 14 October 2021.
Edited by:
Diego Cazorla-Amoros, University of Alicante, SpainReviewed by:
Ángel Berenguer-Murcia, University of Alicante, SpainWei Zhou, Tianjin University, China
Copyright © 2021 Quinson. This is an open-access article distributed under the terms of the Creative Commons Attribution License (CC BY). The use, distribution or reproduction in other forums is permitted, provided the original author(s) and the copyright owner(s) are credited and that the original publication in this journal is cited, in accordance with accepted academic practice. No use, distribution or reproduction is permitted which does not comply with these terms.
*Correspondence: Jonathan Quinson, am9uYXRoYW4ucXVpbnNvbkBjaGVtLmt1LmRr