- 1Laboratorio Especializado en Análisis de Alimentos, Facultad de Química, Universidad Autónoma de Querétaro, Querétaro, Mexico
- 2Bio-Foods Research Lab, Tecnologico de Monterrey, Escuela de Ingeniería y Ciencias, Querétaro, Mexico
- 3Laboratorio de Biología Molecular, Departamento de Ingeniería Bioquímica, Tecnológico Nacional de México en Celaya, Celaya, Mexico
- 4Cátedras CONACYT-TecNM en Celaya, Departamento de Ingeniería Bioquímica, Tecnológico Nacional de México en Celaya, Celaya, Mexico
Most of the active pharmaceutical compounds are often prone to display low bioavailability and biological degradation represents an important drawback. Due to the above, the development of a drug delivery system (DDS) that enables the introduction of a pharmaceutical compound through the body to achieve a therapeutic effect in a controlled manner is an expanding application. Henceforth, new strategies have been developed to control several parameters considered essential for enhancing delivery of drugs. Nanostructure synthesis by microemulsions (ME) consist of enclosing a substance within a wall material at the nanoscale level, allowing to control the size and surface area of the resulting particle. This nanotechnology has shown the importance on targeted drug delivery to improve their stability by protecting a bioactive compound from an adverse environment, enhanced bioavailability as well as controlled release. Thus, a lower dose administration could be achieved by minimizing systemic side effects and decreasing toxicity. This review will focus on describing the different biocompatible nanostructures synthesized by ME as controlled DDS for therapeutic purposes.
Introduction
A drug delivery system (DDS) is the process that enables the introduction of a pharmaceutical compound though the body to achieve a therapeutic effect in a controlled manner (Tiwari et al., 2012). This method includes the administration, the release, and subsequent transport of its bioactive ingredients across the biological membranes to the site of action (Bassyouni et al., 2015).
However, intrinsic factors related to the nature of the pharmaceutical compound such as solubility, molecular weight, pKa, and affinity for the receptor, as well as extrinsic factors such as the physiological stage of the organism or enzymatic interaction with the surrounding environment, are being considered the major disadvantages of these DDS (Vega-Vásquez et al., 2020).These limitations make the bioactive compound susceptible to degradation or inactivation, decreasing its bioavailability (Chen F. et al., 2018). As a result, a higher frequency of drug administration must be needed, having a direct impact on the cost in addition to increasing the risk of side effects, which can be avoided by applying these processes in the pharmaceutical field (Kumar, 2013).
Nanostructures have been successfully applied in DDS due to their nanoscale particle size that leads to an exponential increase in surface area relative to volume which allows controlling the rate, time, and place of the drug release in the body, improving in that way their effectiveness (Lu et al., 2016). Also, a variety of biodegradable polymeric materials such as proteins, polysaccharides, synthetic polymers, and inorganic metallic salts have been used for the nanostructure’s synthesis, due to their biocompatibility as well as their mechanical properties that must provide prolonged protection to the pharmaceutical compound allowing chemical stability over time (Patra J. K. et al., 2018). Therefore, several methods such as phase separation (Reichheld et al., 2017), spray drying (Salama, 2020), and ME processes (Egito et al., 2020) are methods that have proven to be successful for nanocarriers synthesis.
ME-based methods are found to be a versatile route to synthesize a variety of nanostructures such as liposomes, nanocapsules, and dendrimers, to name a few (Agrawal and Agrawal, 2012). The manipulation of various components like surfactant or a mixture of surfactants, frequently in combination with a co-surfactant, involved in the formation of an ME enables the synthesis of nanostructures that can improve the target specificity and therapeutic activity of active compounds, and reduce toxicity of drugs, showing an enormous potential to be used as DDS (Fanun, 2012).
In this review article, we have attempted to describe the different nanostructures synthesized by ME techniques as a medium for generating new DDS for therapeutic purposes.
Nanostructures as Drug Carriers
We can understand DDS as the modification of the environment around the drug, by creating an interface between the pharmaceutical compound and the microenvironment allowing to control drug interactions and that facilitates its delivery (Langer, 1998; Allen and Cullis, 2004). However, DDS have faced some challenges inherent to the size of the material used to modify the microenvironment of the drug, like poor bioavailability, in vivo stability, and poor solubility, absorption route in the body or issues with target-specific delivery. Over time, DDS had to adapt to new classes of therapeutics in order to enhance the aforementioned disadvantages, particularly improve drug targeting to specific tissues and diminish adverse effects of drugs (Martinho et al., 2011). Therefore, the application of nanostructures could be an excellent option to achieve new horizons to this field.
Nanostructures employed as DDS are complex nanoscale systems, composed of an external layer (shell), which may be functionalized with a variety of polymers and/or metal ions, and the internal layer (core), which is the central section of the nanostructure and chemically composed of different compounds (Figure 1). Therefore, the nanostructure-mediated strategy considered to entrap a pharmaceutical compound is possible in the core and shell, and the respective distribution depends on their physicochemical characteristics and also on the type of nanostructure (Mendes et al., 2018).
Nanostructures in the size range from 1 to 100 nm -even 300 nm-can be uptaken by cells in more effective ways compared with large particles ranging from 1 to 10 μm, henceforth, they can directly interact with desired cells with better efficiency and, therefore, reduce side effects (Kabanov et al., 2002; Mirza and Siddiqui, 2014). Also, these nanostructures are designed to respond to internal or external stimuli such as pH, temperature, light, and redox (Rudramurthy et al., 2016; Khan et al., 2019).
In this sense, nanostructures used as DDS must be safe, easily soluble, biocompatible, and bioavailable at the site of treatment. Also, they stay in the blood circulatory system for a prolonged period and enable the controlled release of the drug at specific doses and also ensure action of the drug in the target location (Kumar et al., 2020).
ME is a promising technique employed to synthesize nanostructures in which certain properties can be controlled, such as size, geometry, morphology, homogeneity, and surface area (Orive et al., 2004; Razzacki et al., 2004). Therefore, the optimal selection of an ideal DDS depends on the drug properties, the synthesis of the nanostructure, and the method to obtain it, which determine the different properties and the release characteristics of the incorporated pharmaceutical compound (Kabanov et al., 2002). There is extensive research about the development of ME-based proposed as DDS such as liposomes, nanocapsules, dendrimers, and polymeric nanoparticles.
To this point, most of the ME based nanostructures approved for therapeutic use are polymer-based or lipid-based components, however, some non-polymeric nanoparticles, like quantum dots and metallic nanoparticles, are used in some clinical applications. In the further sections, we will summarize these different types of polymer- and non-polymeric based nanoparticles that are currently used as DDS (Figure 2).
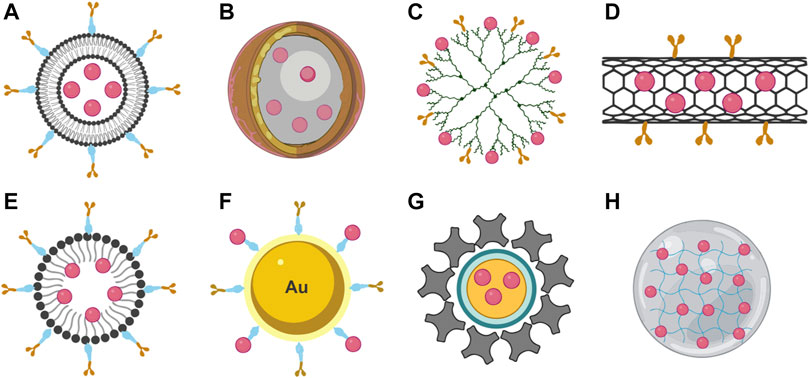
FIGURE 2. Types of nanostructures used as DDS. (A) Liposome, (B) nanocapsule, (C) dendrimer, (D) nanotube, (E) polymeric micelle, (F) metallic nanostructure, (G) quantum dots, and (H) nanogel.
Types of Nanostructures
Nanostructures can be categorized into polymer-, non-polymeric-, and lipid-based nanoparticles. To this point, most of the nanoparticles approved for therapeutic use are polymer-based or lipid-based components. However, some non-polymeric nanoparticles, like quantum dots and metallic nanoparticles, are being used in some clinical applications. In the further sections, we will summarize these different types of polymer- and non-polymeric-based nanoparticles that are currently used as DDS.
Lipid-Based Nanoparticles
Many bioactive molecules used for therapeutic purposes are non-soluble in aqueous systems. Lipid-based nanoparticles (LBNPs) such as liposomes, solid lipid nanoparticles (SLN), and nanostructured lipid carriers (NLC) constitute a broad and diverse group of nanostructures that can transport hydrophobic and hydrophilic pharmaceutical compounds, display very low or no toxicity, and increase the time of drug action by means of a prolonged half-life and a controlled release of the drug (García-Pinel et al., 2019).
Liposomes are one of the first lipid-based nanostructures investigated as a drug carrier that possess a spherical-vesicle structure composed of bilayers of phospholipids and steroids or other surfactants (Figure 2A). They can be synthesized through the hydration of dry phospholipids and form spontaneously when certain lipids are dispersed in the media where liposomes are prepared. The United States Food and Drug Administration (FDA) approved lipids used for the preparation of liposomal vesicles are 1,2-distearoyl-sn-glycero-3-pho sphoethanolamine, hydrogenated phosphatidylcholine from soybean lecithin, egg yolk phosphatidylglycerol, and 1,2-distearoyl-glycero-3-phosphocholine. Among all other techniques, the thin film hydration technique is the most suitable and feasible for the preparation of liposomes. Polyethylene glycol (PEG), chitosan, silk-fibroin, and polyvinyl alcohol (PVA) are generally used for surface functionalization and to avoid opsonization of the lipid vesicles.
The liposome can be prepared in different structures, size, and composition (Kumar, 2019). This nanostructure has been in sign the design of delivery systems because of their ability to fuse with cell membranes -inherent to their lipidic bilayer- and release their content into the cytoplasm. The outer shell diameter can range in size from 60 to 300 nm with a hollow core with a diameter of 50–100 nm where the molecule of interest can be loaded to further delivery (Daraee et al., 2016). Different types of liposomes can be synthesized, and they are classified according to the number of lipidic bilayers that compose the liposome. Unilamellar vesicles (can be small or large) possess an aqueous hollow surrounded by a single lipidic bilayer. It can be loaded with both hydrophilic and hydrophobic molecules due to their structural properties; hydrophilic molecules can be loaded on the aqueous phase and hydrophilic molecules can be dissolved within the lipidic membrane (Oberholzer and Luisi, 2002). This feature is of particular interest since it allows us to load more than one drug within the lipidic or aqueous phases, which in turn allows that the different molecules can be released in sequence with the dissociation of layers from the outer shell to the inner core (Patil and Jadhav, 2014). Liposome formulations are used for delivering anticancer drugs, neurotransmitters, antibiotics, anti-inflammatory, antirheumatic, vaccines, gene therapy, and others.
In the cancer therapy field, recent studies have reported that liposomes integrated with different therapies may be beneficial in a broad range of tumors, not only facilitating antitumor effect but also modulating the tumor immune environment efficacy compared with monotherapy. In addition, adjusting the ratio mix of the pH responder and the lipid can optimize sensibility and specificity of the liposome. Recently, liposomes with matrix metalloproteinase-responsive behavior loaded with a programmed death ligand 1 (PD-L1) inhibitor and doxorubicin (DOX), a low dose achieved better anti-tumor efficacy than the components administered separately (Liu et al., 2019) or even changing composition of the outer shell, like tyrosine hydroxylase peptide-modified liposome, that protects antigen α-galactosylceramide (αGC) from the uptake in β-cells and trigger antitumor responses (Yang et al., 2016b). Interestingly, a combination of cancer therapies can be mixed and enhanced with designed liposomes. One approach is the combination of phototherapy and immunotherapy to deliver a photosensitizer molecule, when activated by light, produces reactive oxygen species to destroy cancer cells (Yang et al., 2016a; Kleinovink et al., 2017; Huis et al., 2020) and the later immunotherapy can improve the therapeutic index of these modalities by enhancing the stability and biocompatibility of cargos as well as reducing sides effects (Calixto et al., 2016; Zhu et al., 2017). In this sense, Li et al. (2018) reported the effectiveness of fluorophore-loaded liposomes (IR-7), a high near infrared region (NIR) absorbance, linked to a multivalent immunoadjuvant, hyaluronic acid-cytosine-phosphate-guanine (HA-CpG). This nanostructure can effectively regulate tumor microenvironment for synergistic photothermal therapy (PTT) and immunotherapy, by inducing the necrosis of cancer cells and promote the maturation of dendritic cells, realizing effective antigen presentation and activation of T cells to recognize and kill cancer cells. Recently, a liposome-based nanoplatform that encapsulated catalases, NIR photosensitizers, and DOX, improved the level of O2 in tumors via catalyzing intratumoral H2O2 has been developed. The generated O2 could further modulate immune cytokines to activate the immune system, enhancing tumor inhibition in vivo (Shi et al., 2020).
Recently, liposomal vaccine formulation showed a selective delivery of tumor antigen to the antigen-presenting cells through incorporation of gangliosides to the outer shell, a natural ligand of macrophages, resulting as an effective platform for cross-presentation and antigen-specific T cell activation (Affandi et al., 2020).
Outer shell modification or functionalization not only enhance targeting, they also can be used to produce liposomes that avoid the mononuclear phagocyte system uptake in order to increase circulation time (Mufamadi et al., 2011). In this sense, Oxaliplatin, a first-line chemotherapy for metastatic colorectal cancer, has been conjugated with a long-circulating liposome PEGylated thermosensitive based, that not only intensify the anti-tumor effect but also increase the retention time up to 24 h, achieving selective release at tumor sites, according to the report by Li et al. (2020).
As a vaccine delivery carrier, they protect antigens from degradation, carry simple or multiple hydrophilic and lipophilic antigens, control the release of the antigens, enhance cellular uptake, and improve antigen-specific immune response (Machhi et al., 2021). It suits different immunization routes including injection, topical administration, oral uptake, and inhalation (Trentini et al., 2014; Wang et al., 2014). Several lipids possess strong adjuvant activity, specially dimethyldioctadecylammonium bromide, a cationic lipid that showed the deposition of the antigen at the injection site, cellular antigen internalization, an antigen association (Anderluzzi et al., 2021). Another report shows that liposomes fabricated with tri-palmitoyl-S-glyceryl cysteine linked to a penta-peptide that carries mRNA for cancer immunotherapy triggers Toll-like receptors preventing tumor growth by increasing cytotoxic T lymphocytes (CTLs) population (Lee et al., 2020). In this sense, liposomes have gained much attention as a platform to deliver nucleic acid-based vaccines since they possess high loading capacity, sustainable release, no leakage, and easy manufacturing.
In response to the COVID-19 pandemic, SARS-CoV-2 mRNA vaccines were designed and produced by ModernaTX (Jackson et al., 2020) and Pfizer-BioNTech SE (Mulligan et al., 2020),which contain a synthetic SARS-CoV-2 mRNA inside a lipid-based nanocarrier to achieve a high-efficient delivery into cells after intramuscular injection by the lipidic nature of the liposome allowing endosomal release of the mRNA into cytoplasm, increase the half-life of mRNA and the latter, lead to a prolonged protein expression at the site of injection (Geall et al., 2012; Pardi et al., 2015) and the latter, development a synergistic effect for immune response against SARS-CoV-2 virus.
Nanocapsules
Nanocapsules are nanomaterials that consist of a liquid/solid core coated with a polymeric shell (Frank et al., 2019) that can be used as a DDS (Figure 2B). They provide a unique nanostructure that increases drug loading efficiency due to the reduced polymeric matrix content of nanoparticles (Raffin Pohlmann et al., 2002). Furthermore, encapsulated drug payloads can be protected from degradation or burst release by the polymeric shell that, also, can be functionalized to interact with certain biomolecules to target drug delivery (Zoabi et al., 2021).
Shell materials are critical in the development of nanocapsules, since properties of selected polymers to synthesize them exerts significant influence on stability, encapsulation efficiency, release profile, and biodistribution (Deng et al., 2020). PEG and PVA are the most used biocompatible polymers to synthesize nanocapsules due to their ability to assist drug release by diffusion and, also, they can be removed from blood via the reticuloendothelial system (Shastri, 2005; Almeida et al., 2011). However, various polymers can be used to formulate the nanocapsule shell to comply with application requirements, and they can be classified as natural or synthetic polymers.
Polysaccharides have been broadly used to synthesize nanocapsules, since they possess highly desirable properties like high biocompatibility. One of the common natural polysaccharides used is the chitosan due to their gelation conditions, mucoadhesive properties, and cationic charge that allows the shell to have electrostatic attractive interactions. Also, it can be endogenously metabolizable into degradable products (Li et al., 2008; De Matteis et al., 2018). Chitosan-based nanocapsules have been used as a delivery system to infectious diseases since cationic charge on the surface improves interactions with bacteria membranes (Jeon et al., 2014; Anversa Dimer et al., 2020). However, the cationic charge of the chitosan shell can induce aggregation of the nanoparticle, protein adsorption, and fast removal from blood circulation. Therefore, anionic polymers can be used to counteract this undesirable feature (Cafaggi et al., 2007; Chen et al., 2010; Shagholani et al., 2015; Belbekhouche et al., 2018). Some research groups explore the possibility of formulating new types of bactericides using this kind of nanostructure enhancing antibacterial activity by increasing the number of chitosan layers. By this approach, Belbekhouche et al. (2019a) developed a chitosan (cationic)-alginate (anionic) nanocapsule that prevents the development of Escherichia coli and Staphylococcus aureus through membrane dysfunction and denaturation of cellular components due to flexible conformation of the nanomaterial.
Additionally, other polysaccharides have been employed to prepare nanocapsules as drug carriers for different applications, such as poly(cyclodextrin), that have been used to elaborate nanocapsules to efficient encapsulation and deliver 4-hydroxy tamoxifen to podocytes for the treatment in hyperplasia of the breast (Belbekhouche et al., 2019b). Also, heparin-based nanocapsules have been formulated for intracellular release of artesunate in malaria therapy, achieving a high release of the load and an extended half-life, facilitating the intracellular release (Ismail et al., 2019). Hyaluronan nanocapsules have been developed for the intracellular delivery of hydrophobic anticancer drug (docetaxel), enhancing their cytotoxicity -due to its encapsulation- and show to be more stable during storage (Oyarzun-Ampuero et al., 2013).
It has been proposed that protein-based polymers can be used to produce polymeric shells for nanocapasules due to their biocompatibility (by its nature) and tunable properties (De Frates et al., 2018). One example is the use of human serum albumin, which reduces the immunogenicity of the nanoparticle, also serves as a targeting ligand for albondin receptors, which in turn are overexpressed on endothelial cells of tumor blood vessels, providing an excellent drug targeted system. Gaber and collaborators exploited this approach when reporting the development of an albumin-shell oily-core nanocapsule loaded with a combination of exemestane and hesperidin for targeted breast cancer therapy, demonstrating an enhanced internalization onto breast cancer cells leading to a significant reduction of tumor. Overall, this approach offers a promising platform for cancer treatment (Gaber et al., 2019), Also, synthesized materials present certain advantages over natural material since they can be tuned with different chemicals to gain different properties, like ionic, mechanical, solubility, or degradability, according to the application needs (da Silva Júnior et al., 2017). In this sense, poly(ε-caprolactone)-based nanocapsules are an excellent option since they have a low cost and are more suitable for long-term delivery systems compared to other synthetic materials (Gomes et al., 2019). Recently, a poly(ε-caprolactone)-based nanocapsule was developed to load pilocarpine, a glaucoma therapy, increasing the payload and achieving a long-term release for almost 42 days after administration (Lee C.-H. et al., 2017).
Dendrimers
Dendrimers are synthetic polymeric nanostructures characterized by a large number of branching points, a spherical-shaped structure, and monodispersity (Figure 2C) (Tomalia et al., 1985). These polymer systems have three well-defined chemical structures: the core, a central nucleus of a single atom or group of atoms; the branches, repeating building blocks, known as “generations,” attached to the core that grow and give size to the dendrimer; and functional groups on the surface, which have the capacity of being tunable, according to the application given to the dendrimer (Zhu et al., 2019).
The dendrimers synthesis contemplates divergent and convergent methods. The divergent approaches are from the core to the shell, generation by generation, first with the activation of functional surface groups, and second, with the addition of branching monomer units (Fana et al., 2020). This method can modify the surface of dendrimer molecules by changing the end groups at the outermost layer. However, the formation of defective dendrimers that arise from incomplete growth and side reactions is the main disadvantage of this method (Rai et al., 2016).
In the convergent approach, compounds are constructed from the periphery to the core, involving two stages, first branching units are grown and connected to other groups; second, when the growing branches, called dendrons, are large enough, they attach to a core for the formation of a complete dendrimer (Hawker and Fréchet, 1990). This method allows better structure control of dendrimers because of the low possibility of side reactions. However, the production of high generation dendrimers can be a problem because steric barrier occurs in the reactions of the dendrons and the core molecule (Caminade et al., 2006).
Hence, the most important difficulty in a dendrimer synthesis process is to ensure the full substitution of all reactive groups to avoid structure defects. To overcome those problems, other methods like “hypercores” and “branched monomers,” involving the pre-assembly of oligomeric species to hasten up the rate of dendrimer synthesis (Chavda, 2007); “double exponential,” allowing the preparation of monomers in either the divergent and convergent growth from a single starting material (Kawaguchi et al., 1995); and “lego” and “click chemistry,” leading a higher growth in the number of terminal surface groups in few reactions (Arseneault et al., 2015), are being developed with the intention to minimize the number of reaction steps, the starting materials, the reaction time, and the cost associated with the dendrimers production (Kesharwani et al., 2014).
In addition to the dendrimers physicochemical properties mentioned before, polyvalency, precise molecular weight, increased solubilization, control over macromolecular growth, internal cavities available to host small molecules, biocompatibility, and absence of immunogenicity provide them the ability to be used as a novel DDS (Sandoval-Yañez and Rodriguez, 2020).
Poly-(amidoamine) (PAMAM) and polypropylenimines (PPI) are the most dendrimers polymers evaluated for their efficient drug delivery due to the availability of a great density of amine groups on their surface Also, the dendrimers structure determines the number and type of bioactive molecule in a DDS, which can be covalently conjugated to the dendrimer terminal groups or enclosed within the core by hydrogen bonding, electrostatic interaction, or hydrophobic linkages (Nikzamir et al., 2021).
In this sense, dendrimers, as carriers of different drugs, should be nontoxic, non-immunogenic, permeable, able to target specific structures, have the ability to extend the circulation time of the drugs, and protect them from the environment (Chauhan, 2018). In oral drug delivery, the dendrimers have been shown to improve the drug solubility due to their capability to decrease non-specific interactions with protein of food, leading to increase the drug absorption via intestinal epithelium (Sadekar and Ghandehari, 2012). Fourth generation PAMAM dendrimer covalently linked to ibuprofen (PAMAM-G4-OH) were synthesized by Kolhe et al. (2006). These authors suggested that the dendrimer improved the drug efficacy by enhancing cellular delivery compared to pure ibuprofen. In ocular drug delivery, dendrimers are an ideal carrier system due to non-irritating, biocompatible, sterile, isotonic, and biodegradable properties (Raj et al., 2020). Viewed in this way, Vandamme and Brobeck (2005) improved the bioavailability of the pilocarpine increasing the resident time in the eye of the drug by using PAMAM dendrimers. These nanostructures have been shown to be efficient as a transdermal delivery system of non-steroidal anti-inflammatory drugs (NSAIDs). Several researchers have indicated that the PAMAM dendrimer complex with NSAIDs can increase the permeation of drugs through penetration enhancers (Manikkath et al., 2017). In pulmonary delivery drugs Conti et al. (2014) developed inhalable generation four amine-terminated PAMAM dendrimer (G4-NH2) siRNA complexes (dendriplexes) by spray drying technique using mannitol and an emulsification diffusion method using chitosan-g-lactic acid. The results showed that the approach of encapsulating siRNAG4-NH2 by emulsification diffusion has superior potential as a pulmonary delivery system. Finally, dendrimers have emerged as versatile carriers as targeted delivery drug systems by passive as well as active routes, which is achieved by the branching units and surface groups of dendrimers (Agrawal and Agrawal, 2012). This approach is particularly effective in treating fatal disorders like cancer as reported by Quintana et al. (2002), who synthesized folate conjugated dendrimers for targeting anticancer bioactive tumors. Since the folate receptors are overexpressed on the surface of different types of cancer cells such as ovarian and breast cancer, hence folate conjugated dendrimers can efficiently target anticancer bioactive cancer cells.
Nanotubes
Carbon nanotubes (CNTs) are cylindrical molecules made of carbon atoms. They are graphene sheets rolled into a seamless cylinder that can be open ended or capped, having nanometric diameters and a length of several micrometers (Elhissi et al., 2012) (Figure 2D). CNTs have physicochemical properties, such as electrical and thermal conductivity, strength, stiffness, toughness, and low density. Also, their small size, high surface area to volume ratio, and their ability to be functionalized give them exceptional properties to be used in the medical field (Kushwaha et al., 2013).
CNTs are divided into two categories: single-walled carbon nanotubes (SWCNT), which consist of single layer of cylinder graphene; and multi-walled carbon nanotubes (MWCNTs), which contain multiple layers of graphene sheets (Figure 3). Both, SWCNTs and MWCNTs, are capped at ends of the tubes with a hemispherical arrangement of carbon networks called “fullerenes” wrapped up by the graphene sheet (Iijima, 1991).
CNTs have hydrophobic surfaces in which a functionalization process of these nanostructures has been proposed as a solution to this problem. Functionalization is a chemical synthesis process in which desired functional groups can be introduced onto the CNTs walls (Kaur et al., 2018). Due to the above, the process of functionalization imparts high solubility with enhanced biocompatibility to the CNTs, being highly suitable for encapsulation of therapeutic molecules for multimodal targeted delivery. There are two methods through which the functionalization process can be carried out: covalent (chemical bond formation) and noncovalent bonding (physioadsorption) (Beg et al., 2018).
Covalent bonding between nanotubes and the attached molecule involves the polymerization of monomers from surface-derived initiators on CNTs or the addition of preformed polymer chains, respectively (Madani et al., 2011). In order to achieve this type of functionalization, CNTs are subjected to treatment with chemical and high temperature reflux conditions. The oxidation of CNTs is the most common technique used for generating covalent functionalization, in which a concentrated acid such as nitric or sulfuric are sonicated and heated, allowing the side-wall covalent functionalization; that results in the attachment of carboxylic acid groups, rendering CNTs water-soluble (Jahangirian et al., 2017). In DDS, the covalent functionalization of drug molecules to CNTs surface has been less reported. However, for therapeutic biomacromolecules, such as proteins, peptides, DNA, and siRNA, covalent functionalization has been widely practiced (Chen et al., 2011).
The noncovalent bonding involves the attachment of therapeutic drug molecules onto the surface of CNTs by adsorption mechanism. The hydrophobic and π-π stacking interaction force between the chains of adsorbed molecules and the surface of CNTs helps in the functionalization on the surface (Tang et al., 2012). The hydrophobic interaction is the more widely used method in drug delivery and it can be carried out by coating CNTs with amphiphilic surfactant molecules or polymers creating a micelle-type structure. On the other hand, the π-π stacking interaction force between the chains of adsorbed pyrene molecules and the surface of CNTs can help in the functionalization on the surface. However, this process was shown to be unstable, as it is cleaved by nucleases, limiting the biologic applications (Liu et al., 2010).
Functionalized CNTs have some potential advantages over other delivery systems, such as an exceptionally high drug loading capacity due to their high surface area and the possibility for incorporating additional therapeutic and diagnostic moieties, either on the surface or their inner cavity (Yadav and Mohite, 2020).
The applications of CNTs in drug delivery through the oral route have been used in tablet coating with hydrogels such as ethyl cellulose, cellulose acetate, and hydroxypropyl methylcellulose for achieving the desired sustained release profile of drug delivery. In 2012, Madaeni and collaborators (Madaeni et al., 2012) reported a carboxyl functionalized MWCNT for sustained therapeutic action of indomethacin. In targeted drug delivery, the cylindrical CNTs shape enable a transmembrane penetration into the target cell; due to the above, choriocarcinoma, carcinoma of cervix, breast cancer, prostate cancer, brain gliomas, and testicular tumors, are some of the disorders in where the CNTs have the potential for delivery of anticancer agents (Dineshkumar et al., 2015). In this sense, Bottini et al. (2011) have reported that the generation of poly(ethylene glycol) (PEG)-modified CNTs have favorable pharmacokinetic and toxicology profiles, i.e., SWCNTs functionalized with PEG and conjugated with the monoclonal antibodies are able to selectively target the CD20 cell surface receptor present on β-cells, with little nonspecific binding to negative T-cells. This approach has proved to be an alternative way in targeting drug molecules to the cancer cells. The potential of CNTs has also been used for ocular drug delivery, due to the advantage of enhanced retention ability in the retinal site to release the drug molecules (Chaurasia et al., 2015). However, the ocular route has not been explored much in this regard.
Polymeric Micelles
Polymeric micelles (PMs) are other nanostructures, which have gained attention for encapsulating and delivering hydrophobic drugs (Figure 2E). PMs, with diameters ranging from 10 to 100 nm, are self-assembled nanostructures formed in aqueous solutions characterized by a core–shell structure; the inner core is composed of the hydrophobic regions which encapsulates the poorly water-soluble drug, whereas the outer shell or corona of the hydrophilic block of the copolymer protects the drug from the aqueous environment (Xu et al., 2013).
Hence, PMs always contain a nonionic water-soluble compound and an ionic compound that can be neutralized by an oppositely charged surfactant to form a hydrophobic core. The electrostatic interaction between the ionic segment of the block polymer and the surfactant group changes these segments from water-soluble to water-insoluble, leading to a hydrophobic core in the micelles (Ahmad et al., 2014).
Self-assembled PMs have special characteristics as delivery systems, such as high loading capacity and improved solubility of drugs, decreased systemic unfavorable effects, enhanced permeability and retention effect, and their possible modification of physicochemical characteristics (Vonarbourg et al., 2006).
The PMs carriers for the purpose of drug delivery are synthesized under three distinct designs: the micelle-forming polymer–drug conjugates, in which the drug incorporation within the micellar carrier is achieved through the formation of hydrolysable chemical bonds between the functional groups of the polymeric backbone and the drug (Girase et al., 2020); the polymeric micellar nano-containers design, which can be achieved only if the block copolymer and the drug are water soluble. In this system, the formation of hydrophobic interactions or hydrogen bonds between the micelle-forming block copolymer and drug provides the basis for the solubilization of drugs in the polymeric micelles (Shahin and Lavasanifar, 2010); and, finally, the polyion complex micelles, in which the incorporation and delivery of different therapeutic moieties that carry charge are needed to promote the electrostatic interactions between oppositely charged polymer and drug combination (Chen W. et al., 2018).
The drugs get released from PMs by two major pathways: dissociation of the micelle followed by the separation of the drug from monomers and drug–polymer bond breakage within the micelle followed by diffusional escape from the delivery system (Aliabadi and Lavasanifar, 2006). Also, it is important to know that the incorporation efficiency of PMs depends on the initial amount of drug added. After maximum loading capacity, the drug is precipitated. In this sense, the drug loading efficiency also depends on the aggregation number of the PMs. Micelles with high aggregation number cause more solubility of the given drugs in the inner core (Lee et al., 2012).
Polymeric segments composed of poly(ethylene oxide) (PEO) (Iurciuc-Tincu et al., 2020) and biodegradable polymers like polyesters like PEG (Xu et al., 2019) are employed to form the hydrophilic outer shell of PMs. Other authors have reported the use of poly(acryloylmorpholine), poly(trimethylene carbonate), and poly(vinylpyrrolidone) (Zhang et al., 2006; Nagarwal et al., 2011) (Gjuroski et al., 2019), respectively. For the hydrophobic core of PMs, poly(glycolic acid), poly(D-lactic acid), poly(D,L-lactic acid), copolymers of lactide/glycolide, and poly(e-caprolactone) are some of the most commonly used polyesters (Bassyouni et al., 2015).
One of the most important applications of PMs is in oral chemotherapy, because most anti-tumor drugs are not orally bioavailable (Feng et al., 2011). As a result, the absorption improving mechanisms by PMs may involve different membrane permeating behavior, inhibition of efflux transports as well as bioadhesive properties (Gong et al., 2012). Paclitaxel is a significant anti-tumor agent and has been shown to exhibit high activity against various solid tumors, including ovarian, breast, non-small cell lung cancers, and head and neck carcinomas. Jing and co-workers developed a PM from paclitaxel/mPEG–PLA block copolymer prodrug. The antitumor activity of this PM was evaluated by MTT assay against human liver cancer H7402 cells and the results indicated that paclitaxel can be released from the conjugate without losing cytotoxicity (Zhang et al., 2005). In targeted drug delivery, (Kabanov et al., 1989) showed that the neuroleptic activity of intraperitoneally administered haloperidol is increased by more than two orders of magnitude after its incorporation into poly(ethylene oxide) –poly(pro-pylene oxide) –poly(ethylene oxide) (PEO–PPO–PEO) micelles coupled with brain specific antibodies. The enhancement of drug efficacy was attributed to specific targeting and increasing the drug permeability through biological membranes given by the polymeric amphiphiles.
Metallic Nanostructures
Metallic nanostructures have a highly potential use in targeted DDS. These metal-based nanoparticles are generally made from gold, silver, gadolinium, and iron oxide, which are delivered in a colloidal form and hold the potential to carry large drug doses increasing their therapeutic index (Ahmad et al., 2010). Drug loading on this type of nanostructure can be achieved through non-covalent interactions, where no specific bond cleavage is required for drug release and only alteration in native physical forces is required. Several functionalization methods for this type of nanomaterial are currently used, like oligo or PEG, BSA, amino acids or tailored peptides, to name a few, which each of them possesses unique features to make them suitable for their biological application (Lee et al., 2008; Lipka et al., 2010; Rink et al., 2010).
The gold nanoparticles have been widely used as drug and gene delivery vehicles (Figure 2F). They can be synthesized in a variety of forms such as rod or dot, with sizes ranging from 0.8 to 200 nm, that make them easily detectable within micromolar concentrations, warranting their favorable optical applications (Wang L. et al., 2017). Recently, there is a new trend in metallic nanostructure synthesis where microorganisms like bacteria, fungi, algae, yeast, or even plants can form the nanoparticle intra- or extracellularly by mixing an adequate growth media with metal precursor solution (Metz et al., 2015; Moghaddam et al., 2015). Using this synthesis approach, there are several reports of gold, silver, cadmium sulfide, and titanium dioxide-based nanostructures synthesized using different bacteria, like Escherichia coli or Bacillus subtilis, and surprisingly, they still possess all the aforementioned characteristics (Iravani, 2014).
With regard to biocompatibility, cells have been shown to intake gold nanoparticles without cytotoxic effects (Fadeel and Garcia-Bennett, 2010). Hirsch and collaborators reported that intra-tumoral injection of gold-nanoparticles resulted in irreversible tissue damage upon exposure to low doses of near infrared light (Hirsch et al., 2003).
Also, metallic nanoparticles have been used as new carriers in cancer treatment due to the significant advantages they possess, such as increased stability and half-life as drug carriers in circulation, required biodistribution, and passive or active targeting into the required tumor site (Mody et al., 2010). The development of tumor-targeted gold nanoparticles encoded with a Raman and further encapsulated with a thiol-modified PEG coat was reported by Qian et al. (2008). Additionally, to specific targeted tumor cells, the pegylated gold nanoparticles were then conjugated with an antibody against epidermal growth factor receptor, which is sometimes overexpressed in certain types of cancer cells. The results obtained suggested a high specific recognition and detection of human cancer cells, as well as active targeting of epidermal growth factor receptor-positive tumor xenografts in animal models.
Recently, a ferric-ferrous mixture of nanoparticles (5–20 nm) were conjugated with erythropoietin (EPO) to deliver it to injured sites in the central nervous system with EPO receptors, increasing the amounts of EPO available for neuro-protective action. More interesting was the fact that the nanostructure can be guided using magnetic control without displaying neurotoxicity in the form of a particle or solution (Nguyen et al., 2020).
Quantum Dots
Quantum dots (QDs) are a particular category of nanoparticle characterized by a crystalline structure. There are core-QDs, which have uniform internal composition, and core-shell QDs, which had different molecules as core and shell that cover it (Figure 2G). In this sense, the basic structure of QDs is made up of a semiconductor core, a shell, and a cap. The shell acts as a protective block for the core —which controls their overall properties— and is responsive for capping ligands coat the core and the caps provide solubility in aqueous media. Cadmium sulfide and cadmium selenide QDs are among the most popular core materials for these nanostructures (Juzenas et al., 2008), since they provide noble optical characteristics, nevertheless, cadmium is probably toxic to human cells (Mo et al., 2017).
Their diameter typically ranges from 1 to 50 nm, which is a desirable property that had made them a tool in biomedical research, especially for multiplexed, quantitative, and long-term fluorescence imaging and detection that are not available for individual molecules or bulk semiconductor solids. One of the most important emerging applications of QDs is like traceable drug delivery, because of the potential to elucidate the pharmacokinetics and pharmacodynamics of drugs (Probst et al., 2013). The combination of using DD and diagnose in a single application is known as theranostics, a field in which QDs have been widely exploited.
As pointed out by Zhao and Zhu (2016), a quintessential QD nanocarrier material for DD should possess the following properties: should not react with payload drug, high drug capacity and encapsulation efficiency, appropriate preparation and purification method, good biocompatibility and low cytotoxicity, mechanical strength and stability, appropriate particle size and shape, and longer residence time in vivo. Recently, they gained research interest as DD since their surface can be modify ad hoc (attached through COOH groups) with tailored peptides, folate, or any other biological molecules to targeted delivery to specific organs (Heyn, 2016; Khodadadei et al., 2017) therefore reducing systemic toxicity.
In this sense, Wang et al. (2017) engineered a QD NIR fluorescence indium phosphate core and a zinc acetate shell, whose cytotoxicity are less compared with cadmium-based cores, with EGFR nanobody (low weight protein derived from heavy-chain antibody) conjugated onto the surface in order to target EGFR-overexpressing tumor cells and deliver aminoflavone. This study shows a successful strategy in which a nanobody surface modification can target specific cells and improve the cellular uptake and cytotoxicity.
Recently, graphene QDs have positioned in the sight of researchers because they possess good solubility, large surface area, easily functionalized edges, and present excellent biocompatibility. Interestingly, graphene QDs alone, without functionalization, are transported through a biological membrane by a lipid raft-mediated mechanism (Wang et al., 2015), where the smaller the size of QD, the higher the apparent membrane permeability is. More recently, Du et al. (2020) used graphene QDs as an oral DD for vanadium compounds, which are anti-diabetic agents. They tested this nanocarrier in a type 2-diabetic mice model (db/db) and they observed a delayed glucose lowering profile but more profound effects on insulin enhancement and β-cell protection after 3 weeks of treatment. One interested observation was that they report that QD alone could effectively lower blood lipid levels on the aforementioned mice model.
There are two main techniques used to synthesize QDs: top-down processing methods and bottom-up approach. Top-down processing method consists of making a bulk semiconductor thinned by electron beam lithography, reactive-ion etching, and/or wet chemical etching. On the other hand, bottom-up or self-assembly techniques implies precipitation methods (wet-chemical method) which are generally microemulsion methods, sol-gel, competitive reactions, to name a few (Valizadeh et al., 2012). Other techniques are those based on the vapor-phase method to produce QDs that begin with processes in which layers are grown atom by atom; these methods are mainly used to produce QDs from III-V semiconductors and II-IV semiconductors (Bera et al., 2010).
Nanogels
Nanogels are three-dimensional networks formed of hydrophilic polymers cross-linked by either physical or chemical bonds (Figure 2H). The natural polymeric macromolecules used to form these nanostructures include chitosan, dextran, and sodium alginate in which the presence of numerous polar groups such as –OH, –COOH, –CONH2, and –SO3H distributed along the polymer chain gives the hydrophilic nature to nanogels. Also, synthetic polymers include poly(N-isopropylacrylamide) (PNIPAm), poly(ethylene oxide) (PEO), poly(ethyleneimine) (PEI), and cholesterol-bearing pullulans are considered to form nanogels despite the risk of the presence of toxic compounds within their structure (Cuggino et al., 2016).
Nanogels have been promoted to use as carriers of bioactive molecules due to their advantageous properties such as nanoscale particle size, great stability, large inner surface area, biocompatibility, and good permeation capability. Also, these nanostructures are designed to respond to internal or external stimuli such as pH, temperature, light, and redox (Soni and Yadav, 2016).
The synthesis of nanogels by ME is especially used to control their particle size. In this method a colloidal suspension of the polymer is made by the homogeneous nucleation of water-soluble monomers that are used to synthesize stabilized nanogels. Nanogels of smaller particle size can be synthesized by adding an ionic surfactant, which also increases the colloidal stability of the formulations. As the surfactant concentration decreases, particle size increases in the nanogel formulation (Sharma et al., 2016).
According to their properties as DDS, nanogels are classified in two different types. Nanohydrogels, which can carry a variety of low and high molecular weight drugs, can absorb a large quantity of water or biological fluids within its structure acting as a filter for the diffusion of solutes, while preserving its arrangement. The second type of nanogels is nano-organogels, which have the capability to hold oily bioactive compounds, forming aggregates in contact with water while enfolding their hydrophilic regions at the center (Suhail et al., 2019).
Nanogels thus have the unique capability to encapsulate more than one bioactive compound in the same carrier which is not feasible in other nanostructures such as dendrimers, PMs, or liposomes (Hajebi et al., 2019). Considering these properties, a nanogel loaded with nanovesicles as an efficient topical delivery of drug can be a feasible approach. In this sense, Abdel-Rashid (2019) developed an ocular surfactant-based nanovesicle carried in mucoadhesive chitosan-based nanogel for topical delivery of acetazolamide (ACZ) to lowering intraocular pressure, which results are significantly prolonged in comparation to ACZ tablets alone.
Great potential has been shown by nanogels in many fields including delivery of genes, chemotherapy drugs, targeting of specific organs, and several others. Nanogels are used in the treatment of cancer as carriers of low-molecular weight drugs such as doxorubicin, cisplatin, 5-fluorouracil, and heparin, to name a few (Jayakumar et al., 2012). Synthetized doxorubicin loaded chitin nanogels showed a relatively higher toxicity on prostate, breast, lung, and liver cancer cells. As an example, Shi and collaborators (2017) developed a selectively intracellular delivery pH and reduction dual-responsive polypeptide nanogel as a hepatoma chemotherapy; this nanogel maintains structural integrity and minimal doxorubicin release in the circulatory system and shows on-demand cell uptake via endocytosis, accumulation in tumor tissue, cell proliferation inhibition, tumor suppression activity, and low cytotoxicity in off target cells. In the same way, Sahu and collaborators (2019) synthesized a chitosan-based pH responsive biodegradable nanogel loaded with 5-fluorouracil as a topical chemotherapy of aggressive melanoma in mice. This nanogel shows a sustained drug release kinetics and induce apoptosis on target cells at low drug doses by an endocytosis mechanism. They report that payload releases at slightly acidic microenvironment resulting in selective accumulation on tumor site, which they state to be a promising platform for efficient and sustained delivery of chemotherapy agent.
Nanogels composed of a polysaccharide pullulan backbone with cholesterol hydrophobic moieties (cholesterol-bearing pullulan) as an artificial absorber of amyloid proteins can be used to inhibit the aggregation of amyloid β-protein, represents a promising approach for therapy of Alzheimer’s disease (Kousalová and Etrych, 2018). Also, nanogels have proven to be an efficient DDS to pulmonary tissue since these particles avoid fast renal clearance and the phagocytosis by macrophages. In this sense, previous studies have shown that an engineered trypsin-responsive and neutrophil elastase-responsive polymeric nanogel can be an easily exchanging enzyme-responsive crosslinkers, releasing the payload after enzymatic degradation and, therefore, therapeutic nanoparticle can reach the smallest capillary vessels and penetrate pulmonary tissues to treat acute and chronic pulmonary diseases (Mejías and Roy, 2019).
Also, a xanthan gum-based nanogel was designed and formulated as DDS for diflunisal (DIF) for topical anti-inflammatory activity to treat rheumatoid arthritis. This nanogel showed an increased drug bioavailability and high drug release profile, ensured a modulate and localized drug effect by maintaining a higher degree of retention of payload, therefore providing a prolonged effect (Bashir et al., 2021). Hence, this approach can be an attractive alternative compared with conventional topical formulation or even a promising substitute to oral DDS.
Microemulsions as a Synthesis Method for Drug Delivery Systems
The design and development of new DDS that enhance the efficacy of existing drugs is an ongoing process in pharmaceutical research. Since there are many types of DDS that have been developed, ME have provided a great potential for achieving the goal in drug targeting. In this sense, they can be used to formulate and synthesize new DDS such as liposomes, nanocapsules, dendrimers, polymeric micelles, metallic nanostructures, quantum dots, and nanogels (Fanun, 2012). Selected examples of nanostructures synthesized by ME are represented in Table 1. Nanostructures as colloidal systems with a nano-scale organization as well as high surface area to volume ratio enable penetration of entrapped therapeutic agents across tissues allowing us to increase the residence time of bioactive molecules while the frequency of drug administration is reduced (Albanese et al., 2012).
In this sense, the implementation of efficient synthesize methods are needed in order to preserve the properties of nanostructures used as DDS. Therefore, ME has been proposed as a versatile technique that allows us to control the particle size, morphology, homogeneity, and surface area of the nanostructures used as DDS (Malik et al., 2012).
ME are defined as thermodynamically stable and isotropic dispersions of two immiscible liquids, where a surfactant stabilizes micro-domains in combination with a cosurfactant (Lawrence and Rees, 2012). These self-aggregated colloidal systems, with an average droplet diameter of 10–140 nm, could be classified as water-in-oil (W/O) ME, where the water is dispersed homogeneously in an organic media; oil-in-water (O/W) ME, where oil is dispersed in water, and bicontinuous ME, consisting of water and oil nanochannels separated by flexible surfactant monolayer (Fanun, 2009).
The synthesis method by ME offers unique properties such as ultralow interfacial tension, large interfacial area, thermodynamic stability, and the ability to solubilize otherwise immiscible liquids, making it feasible to be used to produce nanostructures as delivery platforms (Muzaffar et al., 2013).
Different routes of delivery that include transdermal, oral, ocular, parenteral, and others have been the focus of the medical research.
Transdermal
Transdermal drug delivery is viable for administering low molecular weight drugs that are susceptible to the first-pass metabolism. Furthermore, this route provides a non-invasive route of administration, prolonged drug release, and reduced side effects.
Transdermal drug delivery directly depends on the structure, physiology, and barrier properties of skin. Therefore, the carrier attributes the increased absorption of drugs in transdermal applications by the enhancement of penetration through the skin. In this sense, the use of ME has been widely implemented as a strategy to improve the transport into and across the skin barrier (Kogan and Garti, 2006).
Taking this into account, liposomes are an excellent option for transdermal drug delivery, since phospholipids (as the main component) are amphiphilic molecules that can be used to encapsulate hydrophilic and hydrophobic drugs. The latter, liposomes can be absorbed onto the skin and fuse with the lipidic bilayer of the most superficial layer of the epidermis (stratum corneum), where the lipids facilitate drug permeation into skin (Yu Y.-Q. et al., 2021). Recently, a wide variety of anti-inflammatory drugs have been loaded onto liposomes for localized transdermal delivery, like diclofenac (Sacha et al., 2019) or baicalein (Kim et al., 2019), to name a few.
In 2020, Pandey and collaborators (Pandey et al., 2020) formulated a repaglinide loaded nanostructured lipid carrier-gel (RG-NLCs-gel) system to improve the bioavailability of this anti-diabetic drug by transdermal route. The RG-NLCs ME was prepared by high-speed homogenizer technique. The in vivo pharmacokinetic study showed two times improvement in the bioavailability in comparison to marketed oral tablets in a rat model.
Taking aside the advantage of transdermal nanocarriers, the main disadvantage is that to date it is still difficult to overcome the skin barrier, uncontrolled drug release, limited penetration, and lack of specific targeting, focusing on localized delivery. However, functionalization and surface modification strategies can help to overcome these main issues (Yu Z. et al., 2021).
Oral
The most common route of drug administration for systemic or local drug delivery, for the gastrointestinal (GI) tract, is the oral route. This route of drug administration presents desirable advantages for patients since it is non-invasive and has ease of use, however, it may not be favorable to use during an emergency since it possesses a slower absorption compared to other routes (Homayun et al., 2019). Despite the aforementioned advantages to patients, oral drug delivery possesses a number of challenges like poor drug stability, solubility, and low permeability across mucosal barriers, since these parameters are affected by the physiological environment of the GI tract (Hua, 2020).
More than % of new chemical entities exhibit poor aqueous solubility, resulting in unsatisfactory oral drug delivery. Due to the above, ME can significantly improve the oral bioavailability of hydrophobic drugs (Fanun, 2012).
However, the shortcomings related to the formulation of ME used as oral delivery systems include inadequate solubility in lipidic constituents, lower drug loading ability, and higher risks of GI irritation due to the presence of high quantities of surfactants (30–60% w/w) (Ohadi et al., 2020).
As a solution to the above, it has been proposed the use of biosurfactants such as lipopeptides and glycolipids in ME formulation instead of surfactants to increase the safety and minimizes toxicity and gastric irritation typically associated with the surfactant use. Hence, ME techniques using an O/W biosurfactant mixture are useful in the production of the stable and uniform nanoparticles (Ohadi et al., 2018). The development of a novel ME that contained oleth-5 as a surfactant to enhance the oral absorption of all-trans retinoic acid (ATRA) was reported by Subongkot and Ngawhirunpat (2017). This ME significantly improved the intestinal absorption of ATRA. Also, the intestinal membrane cytotoxicity of the ATRA-loaded ME did not differ from fish oil.
Ocular
The ocular drug delivery can be achieved by multiple routes of administrations, where the topical via, like eye drops, guarantee high compliance by patients. Topical ocular drop delivery is an efficient administration route for the treatment of anterior segment illness but inefficient to treat posterior segment of the eye since multiple ocular barriers make it inefficient to drug delivering (Rodrigues et al., 2018; Kim and Woo, 2021).
Thus, nanoparticles offer a particular benefit to overcome the limitations mentioned above due to the large surface area and their capability to support many surfaces functional modifications to improve drug delivery (Jacob et al., 2018). As an example, there are reports of formulation and characterization of ME composed of oleic acid, non-ionic surfactants between 20/60, 1-propanol and phosphate buffer, where the authors uncovered that the drug is entrapped between the oxyethylene groups of hydrophilic shells of ME and its stability was enhanced for ocular application (Peng et al., 2011; Rashid et al., 2019).
Furthermore, ME are highly stable, possess low toxicity and irritation, and improved drug bioavailability, making them a great option as a DDS in ocular drug delivery. It is important to mention that, after topical application of ME to the eye, this is absorbed through the cornea, where the ME prolonged the contact time between the drug and the corneal epithelial cells (Damiani et al., 2019).
Parenteral
The parenteral route of administration is highly recommended to deliver poorly water-soluble molecules that can exhibit erratic absorption characteristics and low systemic bioavailability (Kolluru et al., 2021). In this sense, parenteral drug delivery route allows us to overcome the limitations mentioned above by bypassing the GI tract.
ME have evolved as a novel vehicle for parenteral delivery of the hydrophobic drugs. Kale and Patravale (2008) evaluated a lorazepam (LZM) ME for compatibility with parenteral fluids, globule size, in vitro hemolysis, and stability of the drug using a Capmul MCM as an oily phase. LZM ME were compatible with parenteral dilution fluids and the in vitro hemolysis studies indicated that ME were well tolerated by erythrocytes. Nanoparticles used to DDS had the advantage of reducing side effects and helping to protect the drug from being rapidly cleared from the body (Kolluru et al., 2020).
Challenges of Microemulsion-Based Nanostructures
Since there are many types of nanostructures that have been developed as DDS, the synthesis method by ME has provided a great potential to achieve drug targeting through these nanoscale structures (Fanun, 2012).
ME are composed of polar and non-polar phases stabilized with an amphiphilic agent decreasing the interfacial tension between these two components. This chemical structure provides to ME an excellent thermodynamic stability being one of its major advantages (McClements, 2012). Due to the above, ME has shown great potential in terms of producing DDS with increased bioavailability of pharmaceutical compounds, particularly those as poorly water-soluble (Ohadi et al., 2020).
Nevertheless, the shortcomings related to ME-based nanostructures include inadequate solubility in lipidic compounds, lower drug loading ability, and higher risks of GI irritation by the presence of high levels of surfactants (30–60% w/w) as well as poor storage stability (He et al., 2010).
Hence, the use of biosurfactants, a group of diverse amphipathic metabolites produced by several microorganisms, has been proposed as an alternative instead of surfactants for ME synthesis. Generally, lower molecular weight biosurfactants including glycolipids such as rhamnolipids, and lipopeptides such as surfactin, have excellent surface-active properties due to their relatively simpler chemical structures provide them thermodynamically stability. Therefore, their isotropic systems are considered to be very promising in the development of nanostructures for DDS increasing the safety and minimizing toxicity and gastric irritation specifically for oral or parenteral routes (Rodrigues, 2015).
Due to the above, more studies focused on complex interactions between the pharmaceutical compound and the physiological environment need to be performed to facilitate the use of ME for the formulation, stability, and packaging of drug delivery nanostructures.
Conclusion
In this review, we discussed the different types of nanomaterials synthesized by ME methods and their applications as a DDS to target specific areas or even the use for delivery of vaccines since it has been in the spotlight recently. Nanomaterials have been widely used to enhance the penetration of large and/or hydrophilic fractions of therapy molecules and can be composed by different polymers or lipids. Nowadays, nucleic acid-based therapies use nanomaterials as delivery systems since they facilitate targeting and penetration. Additionally, cancer therapies have shown certain predilection for nanocarriers, however, chronic and large-scale toxicity tests in humans must be done, only in vitro studies have been reported. It should be noted that the selection of a type of nanomaterial mainly depends on the pharmacological properties and application purpose. Regardless of the type of the nanomaterial selected, the general idea of using nanomaterials as a DDS is to enhance bioavailability of the drug, reduce side effects, and improve target-tissue delivery of payload reducing harmful effects to tissue environment. We give an overview of the different routes of administration and drug delivery medicinal areas like oral, pulmonary, and cancer cells above others as targeted drug delivery based on biocompatible nanomaterials. Despite the potential progression achieved in the nanotechnology area, highlighting the need to test all the new development biocompatible nanomaterials in suitable in vivo models and compare with products that are already in the market to demonstrate the suitability for clinical trials or even improve production scale-up process to develop an industrial-scale model to be cost-effective is feasible.
Author Contributions
All authors listed have made a substantial, direct, and intellectual contribution to the work and approved it for publication.
Funding
GS-M is a Catedras CONACYT fellow supported by the Consejo Nacional de Ciencia y Tecnología (CONACyT) from Mexico.
Conflict of Interest
The authors declare that the research was conducted in the absence of any commercial or financial relationships that could be construed as a potential conflict of interest.
Publisher’s Note
All claims expressed in this article are solely those of the authors and do not necessarily represent those of their affiliated organizations, or those of the publisher, the editors, and the reviewers. Any product that may be evaluated in this article, or claim that may be made by its manufacturer, is not guaranteed or endorsed by the publisher.
Acknowledgments
The authors would like to thank Rafael Olivares-Moreno for making the figures and feedback to manuscript writing; to Fabiola E. Tristán-Flores for feedback to manuscript writing; Sandra I. Silva-Martínez and Laura Silva-Martínez for reviewing manuscript writing.
References
Abdel-Rashid, R. S., Helal, D. A., Omar, M. M., and El Sisi, A. M. (2019). Nanogel Loaded with Surfactant Based Nanovesicles for Enhanced Ocular Delivery of Acetazolamide. Ijn 14, 2973–2983. doi:10.2147/IJN.S201891
Affandi, A. J., Grabowska, J., Olesek, K., Lopez Venegas, M., Barbaria, A., Rodríguez, E., et al. (2020). Selective Tumor Antigen Vaccine Delivery to Human CD169+antigen-Presenting Cells Using Ganglioside-Liposomes. Proc. Natl. Acad. Sci. USA 117, 27528–27539. doi:10.1073/pnas.2006186117
Agrawal, O. P., and Agrawal, S. (2012). An Overview of New Drug Delivery System: Microemulsion. Asian J. Pharm. Sci. Technol. 1, 5–12. doi:10.5455/jbh.20120706124153
Ahmad, M. Z., Akhter, S., Jain, G. K., Rahman, M., Pathan, S. A., Ahmad, F. J., et al. (2010). Metallic Nanoparticles: Technology Overview & Drug Delivery Applications in Oncology. Expert Opin. Drug DeliveryDrug Deliv 7, 927–942. doi:10.1517/17425247.2010.498473
Ahmad, Z., Shah, A., Siddiq, M., and Kraatz, H.-B. (2014). Polymeric Micelles as Drug Delivery Vehicles. RSC Adv. 4, 17028–17038. doi:10.1039/c3ra47370h
Albanese, A., Tang, P. S., and Chan, W. C. W. (2012). The Effect of Nanoparticle Size, Shape, and Surface Chemistry on Biological Systems. Annu. Rev. Biomed. Eng. 14, 1–16. doi:10.1146/annurev-bioeng-071811-150124
Aliabadi, H. M., and Lavasanifar, A. (2006). Polymeric Micelles for Drug Delivery. Expert Opin. Drug Deliv. 3, 139–162. doi:10.1517/17425247.3.1.139
Allen, T. M., and Cullis, P. R. (2004). Drug Delivery Systems: Entering the Mainstream. Science 303, 1818–1822. doi:10.1126/science.1095833
Almeida, J. P. M., Chen, A. L., Foster, A., and Drezek, R. (2011). In Vivo Biodistribution of Nanoparticles. Nanomedicine 6, 815–835. doi:10.2217/nnm.11.79
Anderluzzi, G., Schmidt, S. T., Cunliffe, R., Woods, S., Roberts, C. W., Veggi, D., et al. (2021). Rational Design of Adjuvants for Subunit Vaccines: The Format of Cationic Adjuvants Affects the Induction of Antigen-specific Antibody Responses. J. Controlled Release 330, 933–944. doi:10.1016/j.jconrel.2020.10.066
Anversa Dimer, F., de Souza Carvalho-Wodarz, C., Goes, A., Cirnski, K., Herrmann, J., Schmitt, V., et al. (2020). PLGA Nanocapsules Improve the Delivery of Clarithromycin to Kill Intracellular Staphylococcus aureus and Mycobacterium Abscessus. Nanomedicine: Nanotechnology, Biol. Med. 24, 102125. doi:10.1016/j.nano.2019.102125
Arseneault, M., Wafer, C., and Morin, J.-F. (2015). Recent Advances in Click Chemistry Applied to Dendrimer Synthesis. Molecules 20, 9263–9294. doi:10.3390/molecules20059263
Bae, Y., Song, S. J., Mun, J. Y., Ko, K. S., Han, J., and Choi, J. S. (2017). Apoptin Gene Delivery by the Functionalized Polyamidoamine (PAMAM) Dendrimer Modified with Ornithine Induces Cell Death of HepG2 Cells. Polymers 9, 197. doi:10.3390/polym9060197
Barve, A., Jain, A., Liu, H., Zhao, Z., and Cheng, K. (2020). Enzyme-responsive Polymeric Micelles of Cabazitaxel for Prostate Cancer Targeted Therapy. Acta Biomater. 113, 501–511. doi:10.1016/j.actbio.2020.06.019
Bashir, M., Ahmad, J., Asif, M., Khan, S.-U. -D., Irfan, M., Y Ibrahim, A., et al. (2021). Nanoemulgel, an Innovative Carrier for Diflunisal Topical Delivery with Profound Anti-inflammatory Effect: In Vitro and In Vivo Evaluation. Ijn Vol. 16, 1457–1472. doi:10.2147/IJN.S294653
Bassyouni, F., Elhalwany, N., Abdel Rehim, M., and Neyfeh, M. (2015). Advances and New Technologies Applied in Controlled Drug Delivery System. Res. Chem. Intermed. 41, 2165–2200. doi:10.1007/s11164-013-1338-2
Beg, S., Rahman, M., Jain, A., Saini, S., Hasnain, M. S., Swain, S., et al. (2018). Emergence in the Functionalized Carbon Nanotubes as Smart Nanocarriers for Drug Delivery Applications. Elsevier, 105–133. doi:10.1016/B978-0-12-813691-1.00004-X
Belbekhouche, S., Bousserrhine, N., Alphonse, V., Le Floch, F., Charif Mechiche, Y., Menidjel, I., et al. (2019a). Chitosan Based Self-Assembled Nanocapsules as Antibacterial Agent. Colloids Surf. B: Biointerfaces 181, 158–165. doi:10.1016/j.colsurfb.2019.05.028
Belbekhouche, S., Mansour, O., and Carbonnier, B. (2018). Promising Sub-100 Nm Tailor Made Hollow Chitosan/poly(acrylic Acid) Nanocapsules for Antibiotic Therapy. J. Colloid Interf. Sci. 522, 183–190. doi:10.1016/j.jcis.2018.03.061
Belbekhouche, S., Oniszczuk, J., Pawlak, A., El Joukhar, I., Goffin, A., Varrault, G., et al. (2019b). Cationic Poly(cyclodextrin)/alginate Nanocapsules: From Design to Application as Efficient Delivery Vehicle of 4-hydroxy Tamoxifen to Podocyte In Vitro. Colloids Surf. B: Biointerfaces 179, 128–135. doi:10.1016/j.colsurfb.2019.03.060
Bera, D., Qian, L., Tseng, T.-K., and Holloway, P. H. (2010). Quantum Dots and Their Multimodal Applications: A Review. Materials 3, 2260–2345. doi:10.3390/ma3042260
Boroumand Moghaddam, A., Namvar, F., Moniri, M., Md. Tahir, P., Azizi, S., and Mohamad, R. (2015). Nanoparticles Biosynthesized by Fungi and Yeast: A Review of Their Preparation, Properties, and Medical Applications. Molecules 20, 16540–16565. doi:10.3390/molecules200916540
Bottini, M., Rosato, N., and Bottini, N. (2011). PEG-modified Carbon Nanotubes in Biomedicine: Current Status and Challenges Ahead. Biomacromolecules 12, 3381–3393. doi:10.1021/bm201020h
Cafaggi, S., Russo, E., Stefani, R., Leardi, R., Caviglioli, G., Parodi, B., et al. (2007). Preparation and Evaluation of Nanoparticles Made of Chitosan or N-Trimethyl Chitosan and a Cisplatin-Alginate Complex. J. Controlled Release 121, 110–123. doi:10.1016/j.jconrel.2007.05.037
Calixto, G., Bernegossi, J., De Freitas, L., Fontana, C., Chorilli, M., and Grumezescu, A. M. (2016). Nanotechnology-based Drug Delivery Systems for Photodynamic Therapy of Cancer: A Review. Molecules 21, 342. doi:10.3390/molecules21030342
Caminade, A.-M., Turrin, C.-O., Laurent, R., Maraval, A., and Majoral, J.-P. (2006). Synthetic Pathways Towards Phosphorus Dendrimers and Dendritic Architectures. Coc 10, 2333–2355. doi:10.2174/138527206778992680
Chaurasia, S., Lim, R., Lakshminarayanan, R., and Mohan, R. (2015). Nanomedicine Approaches for Corneal Diseases. Jfb 6, 277–298. doi:10.3390/jfb6020277
Chen, F., Li, K., Hart-Smith, G., Xu, Y. D., Jiang, Y., Lu, H., et al. (2018a). Light-sheet Microscopy as a Tool to Understanding the Behaviour of Polyion Complex Micelles for Drug Delivery. Chem. Commun. 54, 12618–12621. doi:10.1039/C8CC04986F
Chen, H., Wang, L., Yeh, J., Wu, X., Cao, Z., Wang, Y. A., et al. (2010). Reducing Non-specific Binding and Uptake of Nanoparticles and Improving Cell Targeting with an Antifouling PEO-B-Pγmps Copolymer Coating. Biomaterials 31, 5397–5407. doi:10.1016/j.biomaterials.2010.03.036
Chen, L., Xie, H., and Yu, W. (2011). Functionalization Methods of Carbon Nanotubes and its Applications. Carbon Nanotub. Appl. Electron Devices 41 (2), 215–222. doi:10.5772/18547
Chen, W., Zhou, S., Ge, L., Wu, W., and Jiang, X. (2018b). Translatable High Drug Loading Drug Delivery Systems Based on Biocompatible Polymer Nanocarriers. Biomacromolecules 19, 1732–1745. doi:10.1021/acs.biomac.8b00218
C. Nagarwal, R., Dhanawat, M., Das, N., K. Pandit, J., Pandit, K., et al. (2011). Nanocrystal Technology in the Delivery of Poorly Soluble Drugs: An Overview. Cdd 8, 398–406. doi:10.2174/156720111795767988
Conti, D. S., Brewer, D., Grashik, J., Avasarala, S., and Da Rocha, S. R. P. (2014). Poly(amidoamine) Dendrimer Nanocarriers and Their Aerosol Formulations for siRNA Delivery to the Lung Epithelium. Mol. Pharmaceutics 11, 1808–1822. doi:10.1021/mp4006358
Cuggino, J. C., Molina, M., Wedepohl, S., Igarzabal, C. I. A., Calderón, M., and Gugliotta, L. M. (2016). Responsive Nanogels for Application as Smart Carriers in Endocytic pH-Triggered Drug Delivery Systems. Eur. Polym. J. 78, 14–24. doi:10.1016/j.eurpolymj.2016.02.022
da Silva Júnior, W. F., de Oliveira Pinheiro, J. G., Moreira, C. D. L. F. A., de Souza, F. J. J., and de Lima, Á. A. N. (2017). Alternative Technologies to Improve Solubility and Stability of Poorly Water-Soluble Drugs. Multifunctional Syst. Combined Deliv. Biosensing Diagn., 281–305. doi:10.1016/b978-0-323-52725-5.00015-0
Damiani, G., Eggenhöffner, R., Pigatto, P. D. M., and Bragazzi, N. L. (2019). Nanotechnology Meets Atopic Dermatitis: Current Solutions, Challenges and Future Prospects. Insights and Implications from a Systematic Review of the Literature. Bioactive Mater. 4, 380–386. doi:10.1016/j.bioactmat.2019.11.003
Daraee, H., Etemadi, A., Kouhi, M., Alimirzalu, S., and Akbarzadeh, A. (2016). Application of Liposomes in Medicine and Drug Delivery. Artif. Cell Nanomedicine, Biotechnol. 44, 381–391. doi:10.3109/21691401.2014.953633
De Matteis, L., Jary, D., Lucía, A., García-Embid, S., Serrano-Sevilla, I., Pérez, D., et al. (2018). New Active Formulations Against M. tuberculosis: Bedaquiline Encapsulation in Lipid Nanoparticles and Chitosan Nanocapsules. Chem. Eng. J. 340, 181–191. doi:10.1016/j.cej.2017.12.110
DeFrates, K., Markiewicz, T., Gallo, P., Rack, A., Weyhmiller, A., Jarmusik, B., et al. (2018). Protein Polymer-Based Nanoparticles: Fabrication and Medical Applications. Ijms 19, 1717. doi:10.3390/ijms19061717
Deng, S., Gigliobianco, M. R., Censi, R., and Di Martino, P. (2020). Polymeric Nanocapsules as Nanotechnological Alternative for Drug Delivery System: Current Status, Challenges and Opportunities. Nanomaterials 10, 847. doi:10.3390/nano10050847
Dineshkumar, B., Krishnakumar, K., Bhatt, A., Paul, D., Cherian, J., John, A., et al. (2015). Single-walled and Multi-Walled Carbon Nanotubes Based Drug Delivery System: Cancer Therapy: A Review. Indian J. Cancer 52, 262–264. doi:10.4103/0019-509X.176720
Du, J., Feng, B., Dong, Y., Zhao, M., and Yang, X. (2020). Vanadium Coordination Compounds Loaded on Graphene Quantum Dots (GQDs) Exhibit Improved Pharmaceutical Properties and Enhanced Anti-diabetic Effects. Nanoscale 12, 9219–9230. doi:10.1039/d0nr00810a
Duong, T. T., Isomäki, A., Paaver, U., Laidmäe, I., Tõnisoo, A., Yen, T. T. H., et al. (2021). Nanoformulation and Evaluation of Oral Berberine-Loaded Liposomes. Molecules 26, 2591. doi:10.3390/molecules26092591
Egito, E. S. T., Amaral-Machado, L., Alencar, E. N., and Oliveira, A. G. (2020). Microemulsion Systems: From the Design and Architecture to the Building of a New Delivery System for Multiple-Route Drug Delivery. Drug Deliv. Transl. Res. 11, 2108–2133. doi:10.1007/s13346-020-00872-8
Elhissi, A. M. A., Ahmed, W., Hassan, I. U., Dhanak, V. R., and D'Emanuele, A. (2012). Carbon Nanotubes in Cancer Therapy and Drug Delivery. J. Drug Deliv. 2012, 1–10. doi:10.1155/2012/837327
Fadeel, B., and Garcia-Bennett, A. E. (2010). Better Safe Than Sorry: Understanding the Toxicological Properties of Inorganic Nanoparticles Manufactured for Biomedical Applications. Adv. Drug Deliv. Rev. 62, 362–374. doi:10.1016/j.addr.2009.11.008
Fana, M., Gallien, J., Srinageshwar, B., Dunbar, G. L., and Rossignol, J. (2020). Pamam Dendrimer Nanomolecules Utilized as Drug Delivery Systems for Potential Treatment of Glioblastoma: A Systematic Review. Ijn Vol. 15, 2789–2808. doi:10.2147/IJN.S243155
Fanun, M. (2012). Microemulsions as Delivery Systems. Curr. Opin. Colloid Interf. Sci. 17, 306–313. doi:10.1016/j.cocis.2012.06.001
Fanun, M. (2009). Oil Type Effect on Diclofenac Solubilization in Mixed Nonionic Surfactants Microemulsions. Colloids Surf. A: Physicochemical Eng. Aspects 343, 75–82. doi:10.1016/j.colsurfa.2009.01.032
Feng, S.-S., Zhao, L., and Tang, J. (2011). Nanomedicine for Oral Chemotherapy. Nanomedicine 6, 407–410. doi:10.2217/nnm.11.7
Field, L. D., Walper, S. A., Susumu, K., Lasarte-Aragones, G., Oh, E., Medintz, I. L., et al. (2018). A Quantum Dot-Protein Bioconjugate that Provides for Extracellular Control of Intracellular Drug Release. Bioconjug. Chem. 29, 2455–2467. doi:10.1021/acs.bioconjchem.8b00357
Frank, L. A., Gazzi, R. P., de Andrade Mello, P., Buffon, A., Pohlmann, A. R., and Guterres, S. S. (2019). Imiquimod-loaded Nanocapsules Improve Cytotoxicity in Cervical Cancer Cell Line. Eur. J. Pharmaceutics Biopharmaceutics 136, 9–17. doi:10.1016/j.ejpb.2019.01.001
Gaber, M., Hany, M., Mokhtar, S., Helmy, M. W., Elkodairy, K. A., and Elzoghby, A. O. (2019). Boronic-targeted Albumin-Shell Oily-Core Nanocapsules for Synergistic Aromatase Inhibitor/herbal Breast Cancer Therapy. Mater. Sci. Eng. C 105, 110099. doi:10.1016/j.msec.2019.110099
García-Pinel, B., Porras-Alcalá, C., Ortega-Rodríguez, A., Sarabia, F., Prados, J., Melguizo, C., et al. (2019). Lipid-based Nanoparticles: Application and Recent Advances in Cancer Treatment. Nanomaterials 9, 638. doi:10.3390/nano9040638
Geall, A. J., Verma, A., Otten, G. R., Shaw, C. A., Hekele, A., Banerjee, K., et al. (2012). Nonviral Delivery of Self-Amplifying RNA Vaccines. Proc. Natl. Acad. Sci. 109, 14604–14609. doi:10.1073/pnas.1209367109
Gedda, M. R., Madhukar, P., Vishwakarma, A. K., Verma, V., Kushwaha, A. K., Yadagiri, G., et al. (2020). Evaluation of Safety and Antileishmanial Efficacy of Amine Functionalized Carbon-Based Composite Nanoparticle Appended with Amphotericin B: An In Vitro and Preclinical Study. Front. Chem. 8, 510. doi:10.3389/fchem.2020.00510
Girase, M. L., Patil, P. G., and Ige, P. P. (2020). Polymer-drug Conjugates as Nanomedicine: A Review. Int. J. Polymeric Mater. Polymeric Biomater. 69, 990–1014. doi:10.1080/00914037.2019.1655745
Gjuroski, I., Girousi, E., Meyer, C., Hertig, D., Stojkov, D., Fux, M., et al. (2019). Evaluation of Polyvinylpyrrolidone and Block Copolymer Micelle Encapsulation of Serine Chlorin e6 and Chlorin e4 on Their Reactivity Towards Albumin and Transferrin and Their Cell Uptake. J. Controlled Release 316, 150–167. doi:10.1016/j.jconrel.2019.10.010
Gomes, M. L. S., da Silva Nascimento, N., Borsato, D. M., Pretes, A. P., Nadal, J. M., Novatski, A., et al. (2019). Long-lasting Anti-platelet Activity of Cilostazol from Poly(ε-Caprolactone)-Poly(ethylene Glycol) Blend Nanocapsules. Mater. Sci. Eng. C 94, 694–702. doi:10.1016/j.msec.2018.10.029
Gong, J., Chen, M., Zheng, Y., Wang, S., and Wang, Y. (2012). Polymeric Micelles Drug Delivery System in Oncology. J. Controlled Release 159, 312–323. doi:10.1016/j.jconrel.2011.12.012
Gotov, O., Battogtokh, G., and Ko, Y. T. (2018). Docetaxel-Loaded Hyaluronic Acid-Cathepsin B-Cleavable-Peptide-Gold Nanoparticles for the Treatment of Cancer. Mol. Pharmaceutics 15, 4668–4676. doi:10.1021/acs.molpharmaceut.8b00640
Hajebi, S., Rabiee, N., Bagherzadeh, M., Ahmadi, S., Rabiee, M., Roghani-Mamaqani, H., et al. (2019). Stimulus-responsive Polymeric Nanogels as Smart Drug Delivery Systems. Acta Biomater. 92, 1–18. doi:10.1016/j.actbio.2019.05.018
Han, B., Yang, Y., Chen, J., Tang, H., Sun, Y., Zhang, Z., et al. (2020). Preparation, Characterization, and Pharmacokinetic Study of a Novel Long-Acting Targeted Paclitaxel Liposome with Antitumor Activity. Ijn 15, 553–571. doi:10.2147/IJN.S228715
Hawker, C. J., and Frechet, J. M. J. (1990). Preparation of Polymers with Controlled Molecular Architecture. A New Convergent Approach to Dendritic Macromolecules. J. Am. Chem. Soc. 112, 7638–7647. doi:10.1021/ja00177a027
He, C.-X., He, Z.-G., and Gao, J.-Q. (2010). Microemulsions as Drug Delivery Systems to Improve the Solubility and the Bioavailability of Poorly Water-Soluble Drugs. Expert Opin. Drug Deliv. 7, 445–460. doi:10.1517/17425241003596337
Heyn, H. (2016). The Role of the Genetic Code in the DNA Methylation Landscape Formation. Elsevier, 1–18. doi:10.1016/B978-0-12-800140-0.00001-7
Hirsch, L. R., Stafford, R. J., Bankson, J. A., Sershen, S. R., Rivera, B., Price, R. E., et al. (2003). Nanoshell-mediated Near-Infrared Thermal Therapy of Tumors Under Magnetic Resonance Guidance. Proc. Natl. Acad. Sci. 100, 13549–13554. doi:10.1073/pnas.2232479100
Homayun, B., Lin, X., and Choi, H.-J. (2019). Challenges and Recent Progress in Oral Drug Delivery Systems for Biopharmaceuticals. Pharmaceutics 11, 129. doi:10.3390/pharmaceutics11030129
Hua, S. (2020). Advances in Oral Drug Delivery for Regional Targeting in the Gastrointestinal Tract - Influence of Physiological, Pathophysiological and Pharmaceutical Factors. Front. Pharmacol. 11, 00524. doi:10.3389/fphar.2020.00524
Huis, R. V., Ritsma, L., Kleinovink, J. W., Que, I., Ossendorp, F., and Cruz, L. J. (2020). Photodynamic Cancer Therapy Enhances Accumulation of Nanoparticles in Tumor-Associated Myeloid Cells. J. Controlled Release 320, 19–31. doi:10.1016/j.jconrel.2019.12.052
Iijima, S. (1991). Helical Microtubules of Graphitic Carbon. Nature 354, 56–58. doi:10.1038/354056a0
Iravani, S. (2014). Bacteria in Nanoparticle Synthesis: Current Status and Future Prospects. Int. Scholarly Res. Notices 2014, 1–18. doi:10.1155/2014/359316
Ismail, M., Du, Y., Ling, L., and Li, X. (2019). Artesunate-heparin Conjugate Based Nanocapsules with Improved Pharmacokinetics to Combat Malaria. Int. J. Pharmaceutics 562, 162–171. doi:10.1016/j.ijpharm.2019.03.031
Jackson, L. A., Anderson, E. J., Rouphael, N. G., Roberts, P. C., Makhene, M., Coler, R. N., et al. (2020). An mRNA Vaccine Against SARS-CoV-2 - Preliminary Report. N. Engl. J. Med. 383, 1920–1931. doi:10.1056/nejmoa2022483
Jacob, J., Haponiuk, J. T., Thomas, S., and Gopi, S. (2018). Biopolymer Based Nanomaterials in Drug Delivery Systems: A Review. Mater. Today Chem. 9, 43–55. doi:10.1016/j.mtchem.2018.05.002
Jahangirian, H., Ghasemian lemraski, E., Webster, T. J., Rafiee-Moghaddam, R., and Abdollahi, Y. (2017). A Review of Drug Delivery Systems Based on Nanotechnology and Green Chemistry: Green Nanomedicine. Ijn 12, 2957–2978. doi:10.2147/IJN.S127683
Jayakumar, R., Nair, A., Rejinold, N. S., Maya, S., and Nair, S. V. (2012). Doxorubicin-loaded pH-Responsive Chitin Nanogels for Drug Delivery to Cancer Cells. Carbohydr. Polym. 87, 2352–2356. doi:10.1016/j.carbpol.2011.10.040
Jeon, S. J., Oh, M., Yeo, W.-S., Galvão, K. N., and Jeong, K. C. (2014). Underlying Mechanism of Antimicrobial Activity of Chitosan Microparticles and Implications for the Treatment of Infectious Diseases. PLoS One 9, e92723. doi:10.1371/journal.pone.0092723
Jin, H., Gao, S., Song, D., Liu, Y., and Chen, X. (2021). Intratumorally CpG Immunotherapy with Carbon Nanotubes Inhibits Local Tumor Growth and Liver Metastasis by Suppressing the Epithelial-Mesenchymal Transition of Colon Cancer Cells. Anticancer 32, 278–285. doi:10.1097/CAD.0000000000001000
Juzenas, P., Chen, W., Sun, Y.-P., Coelho, M. A. N., Generalov, R., Generalova, N., et al. (2008). Quantum Dots and Nanoparticles for Photodynamic and Radiation Therapies of Cancer. Adv. Drug Deliv. Rev. 60, 1600–1614. doi:10.1016/j.addr.2008.08.004
Kabanov, A. V., Chekhonin, V. P., Alakhov, V. Y., Batrakova, E. V., Lebedev, A. S., Melik-Nubarov, N. S., et al. (1989). The Neuroleptic Activity of Haloperidol Increases after its Solubilization in Surfactant Micelles. FEBS Lett. 258, 343–345. doi:10.1016/0014-5793(89)81689-8
Kabanov, A. V., Lemieux, P., Vinogradov, S., and Alakhov, V. (2002). Pluronic Block Copolymers: Novel Functional Molecules for Gene Therapy. Adv. Drug Deliv. Rev. 54, 223–233. doi:10.1016/S0169-409X(02)00018-2
Kale, A. A., and Patravale, V. B. (2008). Development and Evaluation of Lorazepam Microemulsions for Parenteral Delivery. AAPS PharmSciTech 9, 966–971. doi:10.1208/s12249-008-9131-z
Kaur, J., Gill, G. S., and Jeet, K. (2019). Applications of Carbon Nanotubes in Drug Delivery A Comprehensive Review. Elsevier, 113–135. doi:10.1016/B978-0-12-814031-4.00005-2
Kawaguchi, T., Walker, K. L., Wilkins, C. L., and Moore, J. S. (1995). Double Exponential Dendrimer Growth. J. Am. Chem. Soc. 117, 2159–2165. doi:10.1021/ja00113a005
Kesharwani, P., Jain, K., and Jain, N. K. (2014). Dendrimer as Nanocarrier for Drug Delivery. Prog. Polym. Sci. 39, 268–307. doi:10.1016/j.progpolymsci.2013.07.005
Khan, I., Saeed, K., and Khan, I. (2019). Nanoparticles: Properties, Applications and Toxicities. Arabian J. ChemistryJ. Chem 12, 908–931. doi:10.1016/j.arabjc.2017.05.011
Khodadadei, F., Safarian, S., and Ghanbari, N. (2017). Methotrexate-loaded Nitrogen-Doped Graphene Quantum Dots Nanocarriers as an Efficient Anticancer Drug Delivery System. Mater. Sci. Eng. C 79, 280–285. doi:10.1016/j.msec.2017.05.049
Kim, A. R., Lee, N. H., Park, Y. M., and Park, S. N. (2019). Preparation and Characterization of Novel Pseudo Ceramide Liposomes for the Transdermal Delivery of Baicalein. J. Drug Deliv. Sci. Techn. 52, 150–156. doi:10.1016/j.jddst.2019.04.009
Kim, H. M., and Woo, S. J. (2021). Ocular Drug Delivery to the Retina: Current Innovations and Future Perspectives. Pharmaceutics 13, 108. doi:10.3390/pharmaceutics13010108
Kleinovink, J. W., Fransen, M. F., Löwik, C. W., and Ossendorp, F. (2017). Photodynamic-immune Checkpoint Therapy Eradicates Local and Distant Tumors by CD8+ T Cells. Cancer Immunol. Res. 5, 832–838. doi:10.1158/2326-6066.CIR-17-0055
Kogan, A., and Garti, N. (2006). Microemulsions as Transdermal Drug Delivery Vehicles. Adv. Colloid Interf. Sci. 123-126, 369–385. doi:10.1016/j.cis.2006.05.014
Kolhe, P., Khandare, J., Pillai, O., Kannan, S., Lieh-Lai, M., and Kannan, R. M. (2006). Preparation, Cellular Transport, and Activity of Polyamidoamine-Based Dendritic Nanodevices with a High Drug Payload. Biomaterials 27, 660–669. doi:10.1016/j.biomaterials.2005.06.007
Kolluru, L., Atre, P., and Rizvi, S. (2021). Characterization and Applications of Colloidal Systems as Versatile Drug Delivery Carriers for Parenteral Formulations. Pharmaceuticals 14, 108. doi:10.3390/ph14020108
Kolluru, L. P., Chandran, T., Shastri, P. N., Rizvi, S. A. A., and D’Souza, M. J. (2020). Development and Evaluation of Polycaprolactone Based Docetaxel Nanoparticle Formulation for Targeted Breast Cancer Therapy. J. Nanopart Res. 22, 05096. doi:10.1007/s11051-020-05096-y
Kousalová, J., and Etrych, T. (2018). Polymeric Nanogels as Drug Delivery Systems. Physiol. Res. 67, s305–s317. doi:10.33549/physiolres.933979
Kumar, M. R. (2013). A Comprehensive Review on Gastroretentive Drug Delivery System. Acta Chim. Pharm. Indica 3, 149–164.
Kumar, R., Dalvi, S. V., and Siril, P. F. (2020). Nanoparticle-Based Drugs and Formulations: Current Status and Emerging Applications. ACS Appl. Nano Mater. 3, 4944–4961. doi:10.1021/acsanm.0c00606
Kumar, R. (2019). Lipid-Based Nanoparticles for Drug-Delivery Systems. Nanocarriers Drug Deliv., 249–284. doi:10.1016/b978-0-12-814033-8.00008-4
Kushwaha, S. K. S., Ghoshal, S., Rai, A. K., and Singh, S. (2013). Carbon Nanotubes as a Novel Drug Delivery System for Anticancer Therapy: A Review. Braz. J. Pharm. Sci. 49, 629–643. doi:10.1590/S1984-82502013000400002
Lawrence, M. J., and Rees, G. D. (2012). Microemulsion-based media as Novel Drug Delivery Systems. Adv. Drug Deliv. Rev. 64, 175–193. doi:10.1016/j.addr.2012.09.018
Lee, C.-H., Li, Y.-J., Huang, C.-C., and Lai, J.-Y. (2017a). Poly(ε-caprolactone) Nanocapsule Carriers with Sustained Drug Release: Single Dose for Long-Term Glaucoma Treatment. Nanoscale 9, 11754–11764. doi:10.1039/c7nr03221h
Lee, K., Kim, S. Y., Seo, Y., Kim, M. H., Chang, J., and Lee, H. (2020). Adjuvant Incorporated Lipid Nanoparticles for Enhanced mRNA-Mediated Cancer Immunotherapy. Biomater. Sci. 8, 1101–1105. doi:10.1039/c9bm01564g
Lee, S. H., Bae, K. H., Kim, S. H., Lee, K. R., and Park, T. G. (2008). Amine-functionalized Gold Nanoparticles as Non-cytotoxic and Efficient Intracellular siRNA Delivery Carriers. Int. J. Pharmaceutics 364, 94–101. doi:10.1016/j.ijpharm.2008.07.027
Lee, S., Saito, K., Lee, H.-R., Lee, M. J., Shibasaki, Y., Oishi, Y., et al. (2012). Hyperbranched Double Hydrophilic Block Copolymer Micelles of Poly(ethylene Oxide) and Polyglycerol for pH-Responsive Drug Delivery. Biomacromolecules 13, 1190–1196. doi:10.1021/bm300151m
Lee, Y. K., Kim, S.-W., Park, J.-Y., Kang, W. C., Kang, Y.-J., and Khang, D. (2017b). Suppression of Human Arthritis Synovial Fibroblasts Inflammation Using Dexamethasone-Carbon Nanotubes via Increasing Caveolin-dependent Endocytosis and Recovering Mitochondrial Membrane Potential. Ijn 12, 5761–5779. doi:10.2147/IJN.S142122
Li, L., Yang, S., Song, L., Zeng, Y., He, T., Wang, N., et al. (2018a). An Endogenous Vaccine Based on Fluorophores and Multivalent Immunoadjuvants Regulates Tumor Micro-environment for Synergistic Photothermal and Immunotherapy. Theranostics 8, 860–873. doi:10.7150/THNO.19826
Li, P., Dai, Y. N., Zhang, J. P., Wang, A. Q., and Wei, Q. (2008). Chitosan-alginate Nanoparticles as a Novel Drug Delivery System for Nifedipine. Int. J. Biomed. Sci. 4, 221–228.
Li, S., Zhang, T., Xu, W., Ding, J., Yin, F., Xu, J., et al. (2018b). Sarcoma-targeting Peptide-Decorated Polypeptide Nanogel Intracellularly Delivers Shikonin for Upregulated Osteosarcoma Necroptosis and Diminished Pulmonary Metastasis. Theranostics 8, 1361–1375. doi:10.7150/thno.18299
Li, Y., Xu, P., He, D., Xu, B., Tu, J., and Shen, Y. (2020). Long-circulating Thermosensitive Liposomes for the Targeted Drug Delivery of Oxaliplatin. Ijn 15, 6721–6734. doi:10.2147/IJN.S250773
Lipka, J., Semmler-Behnke, M., Sperling, R. A., Wenk, A., Takenaka, S., Schleh, C., et al. (2010). Biodistribution of PEG-Modified Gold Nanoparticles Following Intratracheal Instillation and Intravenous Injection. Biomaterials 31, 6574–6581. doi:10.1016/j.biomaterials.2010.05.009
Liu, J., Gu, C., Cabigas, E. B., Pendergrass, K. D., Brown, M. E., Luo, Y., et al. (2013). Functionalized Dendrimer-Based Delivery of Angiotensin Type 1 Receptor siRNA for Preserving Cardiac Function Following Infarction. Biomaterials 34, 3729–3736. doi:10.1016/j.biomaterials.2013.02.008
Liu, T., Li, G., Wu, X., Chen, S., Zhang, S., Han, H., et al. (2021). Β-Cyclodextrin-graft-poly(amidoamine) Dendrons as the Nitric Oxide Deliver System for the Chronic Rhinosinusitis Therapy. Drug Deliv. 28, 306–318. doi:10.1080/10717544.2021.1876183
Liu, Y., Chen, X.-G., Yang, P.-P., Qiao, Z.-Y., and Wang, H. (2019). Tumor Microenvironmental pH and Enzyme Dual Responsive Polymer-Liposomes for Synergistic Treatment of Cancer Immuno-Chemotherapy. Biomacromolecules 20, 882–892. doi:10.1021/acs.biomac.8b01510
Liu, Z., Sun, X., Nakayama-Ratchford, N., and Dai, H. (2010). Supramolecular Chemistry on Water-Soluble Carbon Nanotubes for Drug Loading and Delivery. ACS Nano 4, 7726. doi:10.1021/nn103081g
Lu, H., Wang, J., Wang, T., Zhong, J., Bao, Y., and Hao, H. (20162016). Recent Progress on Nanostructures for Drug Delivery Applications. J. Nanomater. 2016, 1–12. doi:10.1155/2016/5762431
Machhi, J., Shahjin, F., Das, S., Patel, M., Abdelmoaty, M. M., Cohen, J. D., et al. (2021). Nanocarrier Vaccines for SARS-CoV-2. Adv. Drug Deliv. Rev. 171, 215–239. doi:10.1016/j.addr.2021.01.002
Madaeni, S. S., Derakhshandeh, K., Ahmadi, S., Vatanpour, V., and Zinadini, S. (2012). Effect of Modified Multi-Walled Carbon Nanotubes on Release Characteristics of Indomethacin from Symmetric Membrane Coated Tablets. J. Membr. Sci. 389, 110–116. doi:10.1016/j.memsci.2011.10.021
Malik, M. A., Wani, M. Y., and Hashim, M. A. (2012). Microemulsion Method: A Novel Route to Synthesize Organic and Inorganic Nanomaterials. Arabian J. Chem. 5, 397–417. doi:10.1016/j.arabjc.2010.09.027
Manikkath, J., Hegde, A. R., Kalthur, G., Parekh, H. S., and Mutalik, S. (2017). Influence of Peptide Dendrimers and Sonophoresis on the Transdermal Delivery of Ketoprofen. Int. J. Pharmaceutics 521, 110–119. doi:10.1016/j.ijpharm.2017.02.002
Martinho, N., Damgé, C., and Reis, C. P. (2011). Recent Advances in Drug Delivery Systems. Jbnb 02, 510–526. doi:10.4236/jbnb.2011.225062
McCallion, C., Peters, A. D., Booth, A., Rees-Unwin, K., Adams, J., Rahi, R., et al. (2019). Dual-action CXCR4-Targeting Liposomes in Leukemia: Function Blocking and Drug Delivery. Blood Adv. 3, 2069–2081. doi:10.1182/bloodadvances.2019000098
McClements, D. J. (2012). Nanoemulsions Versus Microemulsions: Terminology, Differences, and Similarities. Soft Matter 8, 1719–1729. doi:10.1039/c2sm06903b
Mejías, J. C., and Roy, K. (2019). In-vitro and Iin-Vvivo Characterization of a Multi-Stage Enzyme-Responsive Nanoparticle-In-Microgel Pulmonary Drug Delivery System. J. Controlled Release 316, 393–403. doi:10.1016/j.jconrel.2019.09.012
Mendes, M., Sousa, J., Pais, A., and Vitorino, C. (2018). Clinical Applications of Nanostructured Drug Delivery Systems, 43, 116. doi:10.1016/b978-0-08-102198-9.00004-1
Metz, K. M., Sanders, S. E., Pender, J. P., Dix, M. R., Hinds, D. T., Quinn, S. J., et al. (2015). Green Synthesis of Metal Nanoparticles via Natural Extracts: The Biogenic Nanoparticle Corona and its Effects on Reactivity. ACS Sustain. Chem. Eng. 3, 1610–1617. doi:10.1021/acssuschemeng.5b00304
Mirza, A. Z., and Siddiqui, F. A. (2014). Nanomedicine and Drug Delivery: A Mini Review. Int. Nano Lett. 4, 7. doi:10.1007/s40089-014-0094-7
Mo, D., Hu, L., Zeng, G., Chen, G., Wan, J., Yu, Z., et al. (2017). Cadmium-containing Quantum Dots: Properties, Applications, and Toxicity. Appl. Microbiol. Biotechnol. 101, 2713–2733. doi:10.1007/s00253-017-8140-9
Mody, V., Siwale, R., Singh, A., and Mody, H. (2010). Introduction to Metallic Nanoparticles. J. Pharm. Bioall Sci. 2, 282. doi:10.4103/0975-7406.72127
Mufamadi, M. S., Pillay, V., Choonara, Y. E., Du Toit, L. C., Modi, G., Naidoo, D., et al. (2011). A Review on Composite Liposomal Technologies for Specialized Drug Delivery. J. Drug Deliv. 2011, 1–19. doi:10.1155/2011/939851
Mulligan, M. J., Lyke, K. E., Kitchin, N., Absalon, J., Gurtman, A., Lockhart, S., et al. (2020). Phase I/II Study of COVID-19 RNA Vaccine BNT162b1 in Adults. Nature 586, 589–593. doi:10.1038/s41586-020-2639-4
Muzaffar, F., Singh, U. K., and Chauhan, L. (2013). Review on Microemulsion as Futuristic Drug Delivery. Int. J. Pharm. Pharm. Sci. 5, 39–53.
Nguyen, C. T., Kim, C. R., Le, T. H., Koo, K.-i., and Hwang, C. H. (2020). Magnetically Guided Targeted Delivery of Erythropoietin Using Magnetic Nanoparticles. Medicine 99, e19972. doi:10.1097/MD.0000000000019972
Nikzamir, M., Hanifehpour, Y., Akbarzadeh, A., and Panahi, Y. (2021). Applications of Dendrimers in Nanomedicine and Drug Delivery: A Review. J. Inorg. Organomet. Polym. 31, 2246–2261. doi:10.1007/s10904-021-01925-2
Niu, J., Yuan, M., Chen, C., Wang, L., Tang, Z., Fan, Y., et al. (2020). Berberine-loaded Thiolated Pluronic F127 Polymeric Micelles for Improving Skin Permeation and Retention. Ijn 15, 9987–10005. doi:10.2147/IJN.S270336
Oberholzer, T., and Luisi, P. L. (2002). The Use of Liposomes for Constructing Cell Models. J. Biol. Phys. 28, 733–744. doi:10.1023/A:1021267512805
Ohadi, M., Dehghannoudeh, G., Forootanfar, H., Shakibaie, M., and Rajaee, M. (2018). Investigation of the Structural, Physicochemical Properties, and Aggregation Behavior of Lipopeptide Biosurfactant Produced by Acinetobacter Junii B6. Int. J. Biol. Macromolecules 112, 712–719. doi:10.1016/j.ijbiomac.2018.01.209
Ohadi, M., Shahravan, A., Dehghan, N., Eslaminejad, T., Banat, I. M., and Dehghannoudeh, G. (2020). Potential Use of Microbial Surfactant in Microemulsion Drug Delivery System: A Systematic Review. Dddt 14, 541–550. doi:10.2147/DDDT.S232325
Orive, G., Gascón, A. R., Hernández, R. M., Domı́nguez-Gil, A., and Pedraz, J. L. (2004). Techniques: New Approaches to the Delivery of Biopharmaceuticals. Trends Pharmacol. Sci. 25, 382–387. doi:10.1016/j.tips.2004.05.006
Oyarzun-Ampuero, F. A., Rivera-Rodríguez, G. R., Alonso, M. J., and Torres, D. (2013). Hyaluronan Nanocapsules as a New Vehicle for Intracellular Drug Delivery. Eur. J. Pharm. Sci. 49, 483–490. doi:10.1016/j.ejps.2013.05.008
Pandey, S. S., Patel, M. A., Desai, D. T., Patel, H. P., Gupta, A. R., Joshi, S. V., et al. (2020). Bioavailability Enhancement of Repaglinide from Transdermally Applied Nanostructured Lipid Carrier Gel: Optimization, In Vitro and In Vivo Studies. J. Drug Deliv. Sci. Techn. 57, 101731. doi:10.1016/j.jddst.2020.101731
Pardi, N., Tuyishime, S., Muramatsu, H., Kariko, K., Mui, B. L., Tam, Y. K., et al. (2015). Expression Kinetics of Nucleoside-Modified mRNA Delivered in Lipid Nanoparticles to Mice by Various Routes. J. Controlled Release 217, 345–351. doi:10.1016/j.jconrel.2015.08.007
Patil, Y. P., and Jadhav, S. (2014). Novel Methods for Liposome Preparation. Chem. Phys. Lipids 177, 8–18. doi:10.1016/j.chemphyslip.2013.10.011
Patra, A., Satpathy, S., Shenoy, A., Bush, J., Kazi, M., and Hussain, M. D. (2018a). Formulation and Evaluation of Mixed Polymeric Micelles of Quercetin for Treatment of Breast, Ovarian, and Multidrug Resistant Cancers. Ijn 13, 2869–2881. doi:10.2147/IJN.S153094
Patra, J. K., Das, G., Fraceto, L. F., Campos, E. V. R., Rodriguez-Torres, M. d. P., Acosta-Torres, L. S., et al. (2018b). Nano Based Drug Delivery Systems: Recent Developments and Future Prospects. J. Nanobiotechnol 16, 8. doi:10.1186/s12951-018-0392-8
Peng, C. C., Bengani, L. C., Jung, H. J., Leclerc, J., Gupta, C., and Chauhan, A. (2011). Emulsions and Microemulsions for Ocular Drug Delivery. J. Drug Deliv. Sci. Techn. 21, 111–121. doi:10.1016/S1773-2247(11)50010-3
Probst, C. E., Zrazhevskiy, P., Bagalkot, V., and Gao, X. (2013). Quantum Dots as a Platform for Nanoparticle Drug Delivery Vehicle Design. Adv. Drug Deliv. Rev. 65, 703–718. doi:10.1016/j.addr.2012.09.036
Qian, C., Yan, P., Wan, G., Liang, S., Dong, Y., and Wang, J. (2018). Facile Synthetic Photoluminescent Graphene Quantum Dots Encapsulated β-cyclodextrin Drug Carrier System for the Management of Macular Degeneration: Detailed Analytical and Biological Investigations. J. Photochem. Photobiol. B: Biol. 189, 244–249. doi:10.1016/j.jphotobiol.2018.10.019
Qian, X., Peng, X.-H., Ansari, D. O., Yin-Goen, Q., Chen, G. Z., Shin, D. M., et al. (2008). In Vivo Tumor Targeting and Spectroscopic Detection with Surface-Enhanced Raman Nanoparticle Tags. Nat. Biotechnol. 26, 83–90. doi:10.1038/nbt1377
Qin, M., Wang, L., Wu, D., Williams, C. K., Xu, D., Kranz, E., et al. (2020). Enhanced Delivery of Rituximab into Brain and Lymph Nodes Using Timed-Release Nanocapsules in Non-human Primates. Front. Immunol. 10, 03132. doi:10.3389/fimmu.2019.03132
Quintana, A., Raczka, E., Piehler, L., Lee, I., Myc, A., Majoros, I., et al. (2002). Design and Function of a Dendrimer-Based Therapeutic Nanodevice Targeted to Tumor Cells through the Folate Receptor. Pharm. Res. 19, 1310–1316. doi:10.1023/A:1020398624602
Raffin Pohlmann, A., Weiss, V., Mertins, O., Pesce da Silveira, N., and Stanisçuaski Guterres, S. (2002). Spray-dried Indomethacin-Loaded Polyester Nanocapsules and Nanospheres: Development, Stability Evaluation and Nanostructure Models. Eur. J. Pharm. Sci. 16, 305–312. doi:10.1016/S0928-0987(02)00127-6
Rai, A. K., Tiwari, R., Maurya, P., and Yadav, P. (2016). Dendrimers: A Potential Carrier for Targeted Drug Delivery System. Pharm. Biol. Eval. 3, 275–287. doi:10.5281/zenodo.56068
Raj, V. K., Mazumder, R., and Madhra, M. (2020). Ocular Drug Delivery System: Challenges and Approaches. Int. J. App Pharm., 49–57. doi:10.22159/ijap.2020v12i5.38762
Rashid, M. A., Naz, T., Abbas, M., Nazir, S., Younas, N., Majeed, S., et al. (2019). Chloramphenicol Loaded Microemulsions: Development, Characterization and Stability. Colloid Interf. Sci. Commun. 28, 41–48. doi:10.1016/j.colcom.2018.11.006
Razzazan, A., Atyabi, F., Kazemi, B., and Dinarvand, R. (2016). In Vivo Drug Delivery of Gemcitabine with PEGylated Single-Walled Carbon Nanotubes. Mater. Sci. Eng. C 62, 614–625. doi:10.1016/j.msec.2016.01.076
Reichheld, S. E., Muiznieks, L. D., Keeley, F. W., and Sharpe, S. (2017). Direct Observation of Structure and Dynamics During Phase Separation of an Elastomeric Protein. Proc. Natl. Acad. Sci. USA 114, E4408–E4415. doi:10.1073/pnas.1701877114
Riaz, M. K., Zhang, X., Wong, K. H., Chen, H., Liu, Q., Chen, X., et al. (2019). Pulmonary Delivery of Transferrin Receptors Targeting Peptide Surface-Functionalized Liposomes Augments the Chemotherapeutic Effect of Quercetin in Lung Cancer Therapy. Ijn 14, 2879–2902. doi:10.2147/IJN.S192219
Rink, J. S., McMahon, K. M., Chen, X., Mirkin, C. A., Thaxton, C. S., and Kaufman, D. B. (2010). Transfection of Pancreatic Islets Using Polyvalent DNA-Functionalized Gold Nanoparticles. Surgery 148, 335–345. doi:10.1016/j.surg.2010.05.013
Rodrigues, G. A., Lutz, D., Shen, J., Yuan, X., Shen, H., Cunningham, J., et al. (2018). Topical Drug Delivery to the Posterior Segment of the Eye: Addressing the Challenge of Preclinical to Clinical Translation. Pharm. Res. 35, 2519. doi:10.1007/s11095-018-2519-x
Rodrigues, L. R. (2015). Microbial Surfactants: Fundamentals and Applicability in the Formulation of Nano-Sized Drug Delivery Vectors. J. Colloid Interf. Sci. 449, 304–316. doi:10.1016/j.jcis.2015.01.022
Sacha, M., Faucon, L., Hamon, E., Ly, I., and Haltner-Ukomadu, E. (2019). Ex Vivo Transdermal Absorption of a Liposome Formulation of Diclofenac. Biomed. Pharmacother. 111, 785–790. doi:10.1016/j.biopha.2018.12.079
Sadekar, S., and Ghandehari, H. (2012). Transepithelial Transport and Toxicity of PAMAM Dendrimers: Implications for Oral Drug Delivery. Adv. Drug Deliv. Rev. 64, 571–588. doi:10.1016/j.addr.2011.09.010
Sahu, P., Kashaw, S. K., Sau, S., Kushwah, V., Jain, S., Agrawal, R. K., et al. (2019). pH Responsive 5-Fluorouracil Loaded Biocompatible Nanogels for Topical Chemotherapy of Aggressive Melanoma. Colloids Surf. B: Biointerfaces 174, 232–245. doi:10.1016/j.colsurfb.2018.11.018
Salama, A. H. (2020). Spray Drying as an Advantageous Strategy for Enhancing Pharmaceuticals Bioavailability. Drug Deliv. Transl. Res. 10, 1–12. doi:10.1007/s13346-019-00648-9
Sandoval-Yañez, C., and Castro Rodriguez, C. (2020). Dendrimers: Amazing Platforms for Bioactive Molecule Delivery Systems. Materials 13, 570–620. doi:10.3390/ma13030570
Seifalian, A., Naderi, N., Dissanayake, O., Tan, A., and Seifalian, A. M. (2011). A New Era of Cancer Treatment: Carbon Nanotubes as Drug Delivery Tools. Ijn 6, 2963–2979. doi:10.2147/ijn.s16923
Shagholani, H., Ghoreishi, S. M., and Mousazadeh, M. (2015). Improvement of Interaction Between PVA and Chitosan via Magnetite Nanoparticles for Drug Delivery Application. Int. J. Biol. Macromolecules 78, 130–136. doi:10.1016/j.ijbiomac.2015.02.042
Shahin, M., and Lavasanifar, A. (2010). Novel Self-Associating Poly(ethylene Oxide)-B-Poly(ɛ-Caprolactone) Based Drug Conjugates and Nano-Containers for Paclitaxel Delivery. Int. J. Pharmaceutics 389, 213–222. doi:10.1016/j.ijpharm.2010.01.015
Sharma, A., Garg, T., Aman, A., Panchal, K., Sharma, R., Kumar, S., et al. (2016). Nanogel-an Advanced Drug Delivery Tool: Current and Future. Artif. Cell Nanomedicine, Biotechnol. 44, 165–177. doi:10.3109/21691401.2014.930745
Shastri, V. (2003). Non-Degradable Biocompatible Polymers in Medicine: Past, Present and Future. Cpb 4, 331–337. doi:10.2174/1389201033489694
Shi, B., Huang, K., Ding, J., Xu, W., Yang, Y., Liu, H., et al. (2017). Intracellularly Swollen Polypeptide Nanogel Assists Hepatoma Chemotherapy. Theranostics 7, 703–716. doi:10.7150/thno.16794
Shi, C., Li, M., Zhang, Z., Yao, Q., Shao, K., Xu, F., et al. (2020). Catalase-based Liposomal for Reversing Immunosuppressive Tumor Microenvironment and Enhanced Cancer Chemo-Photodynamic Therapy. Biomaterials 233, 119755. doi:10.1016/j.biomaterials.2020.119755
Soni, G., and Yadav, K. S. (2016). Nanogels as Potential Nanomedicine Carrier for Treatment of Cancer: A Mini Review of the State of the Art. Saudi Pharm. J. 24, 133–139. doi:10.1016/j.jsps.2014.04.001
Subongkot, T., and Ngawhirunpat, T. (2017). Development of a Novel Microemulsion for Oral Absorption Enhancement of All-Trans Retinoic Acid. Ijn 12, 5585–5599. doi:10.2147/IJN.S142503
Suhail, M., Rosenholm, J. M., Minhas, M. U., Badshah, S. F., Naeem, A., Khan, K. U., et al. (2019). Nanogels as Drug-Delivery Systems: A Comprehensive Overview. Ther. Deliv. 10, 697–717. doi:10.4155/tde-2019-0010
Sun, F., Zheng, Z., Lan, J., Li, X., Li, M., Song, K., et al. (2019). New Micelle Myricetin Formulation for Ocular Delivery: Improved Stability, Solubility, and Ocular Anti-inflammatory Treatment. Drug Deliv. 26, 575–585. doi:10.1080/10717544.2019.1622608
Tang, A. C. L., Hwang, G.-L., Tsai, S.-J., Chang, M.-Y., Tang, Z. C. W., Tsai, M.-D., et al. (2012). Biosafety of Non-surface Modified Carbon Nanocapsules as a Potential Alternative to Carbon Nanotubes for Drug Delivery Purposes. PLoS One 7, e32893. doi:10.1371/journal.pone.0032893
Taresco, V., Francolini, I., Padella, F., Bellusci, M., Boni, A., Innocenti, C., et al. (2015). Design and Characterization of Antimicrobial Usnic Acid Loaded-Core/shell Magnetic Nanoparticles. Mater. Sci. Eng. C 52, 72–81. doi:10.1016/j.msec.2015.03.044
Tiwari, G., Tiwari, R., Bannerjee, S., Bhati, L., Pandey, S., Pandey, P., et al. (2012). Drug Delivery Systems: An Updated Review. Int. J. Pharma Investig. 2, 2–11. doi:10.4103/2230-973x.96920
Tomalia, D. A., Baker, H., Dewald, J., Hall, M., Kallos, G., Martin, S., et al. (1985). A New Class of Polymers: Starburst-Dendritic Macromolecules. Polym. J. 17, 117–132. doi:10.1295/polymj.17.117
Trentini, M. M., de Oliveira, F. M., Nogueira Gaeti, M. P., Batista, A. C., Lima, E. M., Kipnis, A., et al. (2014). Microstructured Liposome Subunit Vaccines Reduce Lung Inflammation and Bacterial Load after Mycobacterium tuberculosis Infection. Vaccine 32, 4324–4332. doi:10.1016/j.vaccine.2014.06.037
Valizadeh, A., Mikaeili, H., Samiei, M., Farkhani, S. M., Zarghami, N., Kouhi, M., et al. (2012). Quantum Dots: Synthesis, Bioapplications, and Toxicity. Nanoscale Res. Lett. 7, 480. doi:10.1186/1556-276X-7-480
Vandamme, T. F., and Brobeck, L. (2005). Poly(amidoamine) Dendrimers as Ophthalmic Vehicles for Ocular Delivery of Pilocarpine Nitrate and Tropicamide. J. Controlled Release 102, 23–38. doi:10.1016/j.jconrel.2004.09.015
Vega-Vásquez, P., Mosier, N. S., and Irudayaraj, J. (2020). Nanoscale Drug Delivery Systems: From Medicine to Agriculture. Front. Bioeng. Biotechnol. 8, 79. doi:10.3389/fbioe.2020.00079
Vonarbourg, A., Passirani, C., Saulnier, P., and Benoit, J.-P. (2006). Parameters Influencing the Stealthiness of Colloidal Drug Delivery Systems. Biomaterials 27, 4356–4373. doi:10.1016/j.biomaterials.2006.03.039
Wang, L., Hu, C., and Shao, L. (2017a). The Antimicrobial Activity of Nanoparticles: Present Situation and Prospects for the Future. Ijn 12, 1227–1249. doi:10.2147/IJN.S121956
Wang, N., Wang, T., Zhang, M., Chen, R., and Deng, Y. (2014). Using Procedure of Emulsification-Lyophilization to Form Lipid A-Incorporating Cochleates as an Effective Oral Mucosal Vaccine Adjuvant-Delivery System (VADS). Int. J. Pharmaceutics 468, 39–49. doi:10.1016/j.ijpharm.2014.04.002
Wang, X.-y., Lei, R., Huang, H.-d., Wang, N., Yuan, L., Xiao, R.-y., et al. (2015). The Permeability and Transport Mechanism of Graphene Quantum Dots (GQDs) Across the Biological Barrier. Nanoscale 7, 2034–2041. doi:10.1039/c4nr04136d
Wang, Y., Wang, Y., Chen, G., Li, Y., Xu, W., and Gong, S. (2017b). Quantum-Dot-Based Theranostic Micelles Conjugated with an Anti-EGFR Nanobody for Triple-Negative Breast Cancer Therapy. ACS Appl. Mater. Inter. 9, 30297–30305. doi:10.1021/acsami.7b05654
Xu, M., Zhang, C. Y., Wu, J., Zhou, H., Bai, R., Shen, Z., et al. (2019). PEG-detachable Polymeric Micelles Self-Assembled from Amphiphilic Copolymers for Tumor-Acidity-Triggered Drug Delivery and Controlled Release. ACS Appl. Mater. Inter. 11, 5701–5713. doi:10.1021/acsami.8b13059
Xu, W., Ling, P., and Zhang, T. (2013). Polymeric Micelles, a Promising Drug Delivery System to Enhance Bioavailability of Poorly Water-Soluble Drugs. J. Drug Deliv. 2013, 1–15. doi:10.1155/2013/340315
Yadav, A. R., and Mohite, S. K. (2020). Carbon Nanotubes as an Effective Solution for Cancer Therapy. Res. J. Pharm. Dos. Forms Technol. 12, 301–307. doi:10.5958/0975-4377.2020.00050.6
Yan, Y., Li, X.-Q., Duan, J.-L., Bao, C.-J., Cui, Y.-N., Su, Z.-B., et al. (2019). Nanosized Functional miRNA Liposomes and Application in the Treatment of TNBC by Silencing Slug Gene. Ijn 14, 3645–3667. doi:10.2147/IJN.S207837
Yan, Y., Wang, R., Hu, Y., Sun, R., Song, T., Shi, X., et al. (2018). Stacking of Doxorubicin on Folic Acid-Targeted Multiwalled Carbon Nanotubes for In Vivo Chemotherapy of Tumors. Drug Deliv. 25, 1607–1616. doi:10.1080/10717544.2018.1501120
Yang, Y., Hu, Y., and Wang, H. (2016a). Targeting Antitumor Immune Response for Enhancing the Efficacy of Photodynamic Therapy of Cancer: Recent Advances and Future Perspectives. Oxidative Med. Cell Longevity 2016, 1–11. doi:10.1155/2016/5274084
Yang, Y., Tai, X., Shi, K., Ruan, S., Qiu, Y., Zhang, Z., et al. (2016b). A New Concept of Enhancing Immuno-Chemotherapeutic Effects Against B16F10 Tumor via Systemic Administration by Taking Advantages of the Limitation of EPR Effect. Theranostics 6, 2141–2160. doi:10.7150/thno.16184
Yu, Y.-Q., Yang, X., Wu, X.-F., and Fan, Y.-B. (2021a). Enhancing Permeation of Drug Molecules across the Skin via Delivery in Nanocarriers: Novel Strategies for Effective Transdermal Applications. Front. Bioeng. Biotechnol. 9, 646554. doi:10.3389/fbioe.2021.646554
Yu, Z., Meng, X., Zhang, S., Chen, Y., Zhang, Z., and Zhang, Y. (2021b). Recent Progress in Transdermal Nanocarriers and Their Surface Modifications. Molecules 26, 3093. doi:10.3390/molecules26113093
Zafar Razzacki, S., Thwar, P. K., Yang, M., Ugaz, V. M., and Burns, M. A. (2004). Integrated Microsystems for Controlled Drug Delivery. Adv. Drug Deliv. Rev. 56, 185–198. doi:10.1016/j.addr.2003.08.012
Zhang, D., Zhang, J., Zeng, J., Li, Z., Zuo, H., Huang, C., et al. (2019). Nano-gold Loaded with Resveratrol Enhance the Anti-hepatoma Effect of Resveratrol In Vitro and In Vivo. J. Biomed. Nanotechnol. 15, 288–300. doi:10.1166/jbn.2019.2682
Zhang, X., Li, Y., Chen, X., Wang, X., Xu, X., Liang, Q., et al. (2005). Synthesis and Characterization of the Paclitaxel/MPEG-PLA Block Copolymer Conjugate. Biomaterials 26, 2121–2128. doi:10.1016/j.biomaterials.2004.06.024
Zhang, Z., Grijpma, D. W., and Feijen, J. (2006). Thermo-sensitive Transition of Monomethoxy Poly(ethylene Glycol)-Block-Poly(trimethylene Carbonate) Films to Micellar-like Nanoparticles. J. Controlled Release 112, 57–63. doi:10.1016/j.jconrel.2006.01.010
Zhao, M.-X., and Zhu, B.-J. (2016). The Research and Applications of Quantum Dots as Nano-Carriers for Targeted Drug Delivery and Cancer Therapy. Nanoscale Res. Lett. 11, 9. doi:10.1186/s11671-016-1394-9
Zhong, Q., and Da Rocha, S. R. P. (2016). Poly(amidoamine) Dendrimer-Doxorubicin Conjugates: In Vitro Characteristics and Pseudosolution Formulation in Pressurized Metered-Dose Inhalers. Mol. Pharmaceutics 13, 1058–1072. doi:10.1021/acs.molpharmaceut.5b00876
Zhu, G., Lynn, G. M., Jacobson, O., Chen, K., Liu, Y., Zhang, H., et al. (2017). Albumin/vaccine Nanocomplexes that Assemble In Vivo for Combination Cancer Immunotherapy. Nat. Commun. 8, 02191. doi:10.1038/s41467-017-02191-y
Zhu, Y., Liu, C., and Pang, Z. (2019). Dendrimer-based Drug Delivery Systems for Brain Targeting. Biomolecules 9, 790–829. doi:10.3390/biom9120790
Keywords: nanostructures, microemulsion (ME), drug delivery systems (DDS), emulsion synthesis, biocompatible materials
Citation: Arredondo-Ochoa T and Silva-Martínez GA (2022) Microemulsion Based Nanostructures for Drug Delivery. Front. Nanotechnol. 3:753947. doi: 10.3389/fnano.2021.753947
Received: 05 August 2021; Accepted: 02 December 2021;
Published: 06 January 2022.
Edited by:
Esmeralda Mendoza-Mendoza, Autonomous University of San Luis Potosí, MexicoReviewed by:
Raj Kumar, University of Nebraska Medical Center, United StatesArti Vashist, Florida International University, United States
Copyright © 2022 Arredondo-Ochoa and Silva-Martínez. This is an open-access article distributed under the terms of the Creative Commons Attribution License (CC BY). The use, distribution or reproduction in other forums is permitted, provided the original author(s) and the copyright owner(s) are credited and that the original publication in this journal is cited, in accordance with accepted academic practice. No use, distribution or reproduction is permitted which does not comply with these terms.
*Correspondence: Teresita Arredondo-Ochoa, dGVyZXNpdGEuYXJyZWRvbmRvQHVhcS5teA==; Guillermo A. Silva-Martínez, Z3VpbGxlcm1vLnNpbHZhQGNvbmFjeXQubXg=