- 1 College of Environmental Science and Engineering, Fujian Key Laboratory of Pollution Control and Resource Reuse, Fujian Normal University, Fuzhou, China
- 2 State Key Laboratory of Photocatalysis on Energy and Environment, College of Chemistry, Fuzhou University, Fuzhou, China
The continuous rise in plastic waste raises serious concerns about the ensuing effects on the pollution of global environment and loss of valuable resources. Developing efficient approach to recycle the plastic has been an urgent demand for realizing a sustainable circular economy. Photocatalytic valorization directly utilizes solar energy to transform plastic pollutant into chemicals and fuels, which is hardly implemented by traditional mechanical recycling and incineration strategies, thus offering a promising approach to address the contemporary waste and energy challenges. Here, we focus on the recent advances in the high-value utilization of plastic waste through photocatalysis. The basic principle and different reaction pathways for the photocatalytic valorization of plastic waste are presented. Then, the developed representative photocatalyst systems and converted products are elaborately discussed. At last, the review closes with critical thoughts on research challenges along with some perspectives for further development of this emerging and fascinating filed.
Introduction
Plastic as one of the most influential inventions of 20th century has been widely applied in our daily life (Andrady and Neal, 2009; Bai et al., 2019; Jie et al., 2020; Gausas et al., 2021; Zhang F et al., 2021). Currently, about 360 million tonnes (Mt) of plastic are produced each year (Martín et al., 2021), and the number is expected to double over the next 2 decades (MacArthur 2017). However, only a small portion of the produced plastic is recycled after use. Around 80% of all plastic is directly incinerated or discarded as waste annually due to the durability, which exists in landfills or in the environment (Figure 1), and is hard to be degraded by ambient onslaughts such as light, water, heat, and so on (MacArthur, 2017; Jehanno and Sardon, 2019; Geyer 2020; PlasticEurope, 2020). The rapid accumulation of plastic waste has resulted in a worldwide “white pollution.” For example, it has been estimated that if the current trend continues, more than 8 Mt of plastic will enter the ocean each year, and the overall weight of the waste plastic will higher than that of fish in 2050 (Thompson et al., 2004; MacArthur, 2017; Uekert et al., 2018; Martín et al., 2021). Moreover, the huge production of plastic also exacerbates the global energy crisis, due to that almost all of the plastics are produced from petrochemicals derived from fossil fuel production, such as light (C1–C4) fractions of oil and natural gas, which accounts for ∼ 6% consumption of the fossil fuel (Vollmer et al., 2020; Martín et al., 2021). The ever-increasing demand accompanying with the continuously rised discarding of plastic causes serious environmental and energy challenges, which call for reducing, increasing reuse and recycling of the plastic to tackle the dilemma and moves toward circular economy (Lopez et al., 2017; Ru et al., 2020; Tennakoon et al., 2020).
In fact, the plastic waste is a misplaced resource. The vast majority of the plastics are polymer, which enables them to be valorized as new chemical feedstock via correct treatment (Keane, 2007). If all of the global plastic solid waste could be recycled, it would save an estimated 3.5 billion barrels of oil a year, valued at $176 billion dollars, implying a high economic benefit (Garcia and Robertson, 2017; Rahimi and García, 2017). At present, the most widely adopted strategy for the plastic waste valorization is based on mechanical recycling (Martín et al., 2021). The approach collects and reprocesses the plastic polymer as raw material for new plastic manufacturing by sorting, shredding, melting, and re-extrusion (Al-Salem et al., 2009; Kumar et al., 2011; Mark et al., 2020; Schyns and Shaver, 2021; Zhang K et al., 2021). It is favored by environmental friendliness, but commonly suffers from property deterioration of the products due to the chain scission, branching, and oxidation of the plastic polymer caused by the reprocess treatment (Mark et al., 2020; Worch and Dove, 2020). In addition, the application of the approach is limited by the type of the plastic. Only two types of polyethylene terephthalate (PET) and polyethylenes (PE) plastics are mechanically processed (Garcia and Robertson, 2017). Plastic with high temperature sensitivity or high melt viscosity not flow at elevated temperatures cannot be recycled by this approach. More importantly, the mechanical processing is incapable in handling microplastics due to their small size (≤ 5 mm) and dilution (Eerkes-Medrano et al., 2015; Tofa et al., 2019; Uekert et al., 2019; Zhang F et al., 2021).
In contrast, chemical recycling is a more promising plastic valorization strategy that now sparkle in both academic and industrial arenas. The process focuses on transformation of plastic waste into small molecules, ranging from starting monomers to constituent hydrocarbons (Worch and Dove, 2020). The products not only can be re-polymerized into virgin plastic, but also can be upcycled into alternative chemicals and fuels, thus enabling the materials reusing in a closed loop. Especially, the approach is applicable to all kinds of plastic waste (Martín et al., 2021). Nevertheless, the chemical recycling is generally an energy-intensive process, requiring high temperature and/or pressure (Worch and Dove, 2020). To endorse the circular economy framework, the development of low energy input catalytic processes to efficiently transform the plastic waste into original monomer sources and targeted products is crucial.
In this context, photocatalytic recycling directly driven by solar energy provides an advanced solution to address the key challenges associated with the traditional chemical recycling efforts. Actually, the photocatalytic treatment of plastic has been a long theme, but previous research endeavors are devoted to the degradation of plastic to CO2 (Shang et al., 2003; Zhao et al., 2007; Zhao et al., 2008; Liang et al., 2013). Until recently, the photocatalytic conversion of plastic into chemicals and fuels has been reinvigorated. It can be achieved through the selection of appropriate photocatalysts and control of the reaction conditions, thus providing an alternative method to conventional recycling process for valorization of the plastic waste. In this mini review, we focus on the up-to-date progresses of photocatalytic valorization of plastic waste to produce chemicals and fuels. The different photocatalytic conversion pathways, developed catalysts, and end products of waste plastic recycling are discussed. Also, some key issues alongside with perspectives in this field are highlighted.
Basic Principle for Photocatalytic Valorization of Plastic Waste
Photocatalysis is a mimic technology of nature photosynthesis. It typically involves three distinct steps: 1) light absorption of photocatalysts to excite charge carriers, 2) separation and transportation of the photogenerated charge carriers, and 3) redox reaction on the surface of the catalyst, as illustrated in Figure 2 (Liu et al., 2015; Yang et al., 2015; Lu et al., 2019; Shang et al., 2021). In order for photocatalysis to proceed, the energy of incident photons should be equal or higher than the band gap of photocatalysts. Meantime, the reduction potential of photoelectrons in the photocatalyst conduction band (CB) should be more negative than the value of the reactant to be reduced, while the oxidation potential of holes in the valence band (VB) should be more positive than that of the substrate to be oxidized (Habisreutinger et al., 2013; Xiao et al., 2015; Yang and Xu, 2016; Clarizia et al., 2017; Yang et al., 2018; Uekert et al., 2020a). Specifically, for photocatalytic valorization of plastic waste, the holes in the VB of photocatalyst after photoexcitation are commonly utilized for the oxidation of the plastic to generate organic products, or degrade it to CO2, while the electrons in the photocatalyst CB can be employed to reduce the protons in H2O to H2, CO2 to carbon fuels, or be captured by O2 to involve in the subsequent oxidation of the plastic.
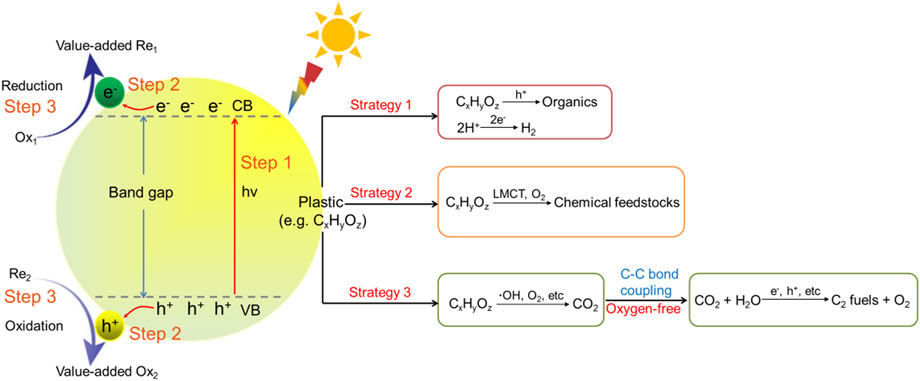
FIGURE 2. Basic principle and three typical conversion pathways for photocatalytic valorization of plastic waste (LMCT refers to ligand to metal charge transfer).
Depending on the different consumption pathways of the photogenerated charge carriers, the current reports for photocatalytic valorization of plastic waste can be classified into three conversion mechanisms (Figure 2): (i) photocatalytic plastic upgrading to fine chemicals integrated with H2 production. The process is similar to the traditional half reaction for photocatalytic H2 production. The plastic substrate acts as an electron donor and is oxidized by the holes of the excited photocatalyst to generate organic products, while the photogenerated electrons are transferred to the surface of the photocatalyst and reduce water to H2; (ii) photocatalytic valorization of waste plastic to oxygenated chemical feedstocks. In this process, the C-C bond of plastic substrate is firstly cleaved to produce primary products and radical fragment, which then be further oxidized by dissolved O2 in air or hydrolyzed under aerobic reaction conditions to give oxygenated derivatives, such as carboxylic acids; (iii) photocatalytic plastic degradation coupled with sequential CO2 reduction to produce C2 fuels. This strategy includes two processes, where the first step is the degradation of plastic into CO2 through photooxidative C-C bond cleavage over photocatalyst. Then, the produced CO2 is captured as raw material and further reduced by the same photocatalyst into C2 fuels by photoinduced C-C coupling process. To elaborately present the three different reaction pathways, some typical examples are discussed in the below section.
Photocatalytic Plastic Upgrading to Fine Chemicals Integrated With H2 Production
The initial study of photocatalytic H2 production form plastic waste can be dated back to 1981. Sakata and co-workers reported that a range of plastic [PE, polyvinyl chloride (PVC) and Teflon] could be photodecomposed to produce H2 (Kawai and Sakata, 1981). It finds that improving the alkalinity of the reaction solution results in higher H2 production rate. However, CO2 is produced as a by-product in this study and the conversion efficiency is low. After that, although there are some reports about the photocatalytic treatment of plastic, nearly all of them are focused on degradation (Feng et al., 2011; de Assis et al., 2018; Das and Mahalingam, 2019). No typical study about the photocatalytic H2 production from plastic waste has been reported. In recent years, with the urgent demand for green conversion of the continuously accumulated plastic waste and the advancement of photocatalysis, the solar-driven photocatalytic valorization re-emerges. In 2018, Reisner’s group have reported a high photocatalytic H2 evolution from a variety of plastic polymers such as polylactic acid (PLA), PET, polyurethane (PUR), and a real PET water bottle under alkaline condition (10 M NaOH), accompanying with the conversion of the polymer into organic products such as formate, acetate and pyruvate (Supplementary Figures 1A–G) (Uekert et al., 2018). Typical CdS semiconductor with suitable band gap and appropriate conduction band and valence band positions (CB-0.5V vs. NHE, VB + 1.9V vs. NHE) is employed in this work. Owing to the strong alkalinity, a thin layer of Cd oxide/hydroxide shell (CdOx) is formed on the surface of the CdS quantum dots (QDs), which can prevent the photocorrosion of the CdS. The as-prepared CdS/CdOx QDs displays high H2 evolution performance that is comparable to the activity of CdS-based composites for photoconverting of traditional organic substrates, such as furfural and glucose (Supplementary Figures 1A,B). The external quantum yields reach around 15% for PLA, 3.7 for PET, and 0.14 for PUR at λ = 430 nm.
In order to further improve the photoactivity, a simple pretreatment of the plastic substrates in 10 M NaOH solution for 24 h under dark condition of 40°C is developed. The supernatant is used for the photocatalytic reaction. As shown in Supplementary Figure 1A, it is obvious that the simple pre-treatment results in markedly enhanced H2 evolution rates, four times for both PET, and PUR. This is because that the pre-treatment initiates hydrolysis, releasing monomers into solution which can be more rapidly photocatalytic converted. The negligible pre-treatment influence on PLA is due to its easy dissolvability in NaOH. Mass spectra analysis in deuterated and nondeuterated solvent confirms that the generated H2 originates from H2O rather than the substrate (Supplementary Figure 1F). Also, no gaseous CO2 and CO3 2− is detected in the solutions after reaction (Supplementary Figure 1G). These results verify that the polymers are partially oxidized to organic molecules. Although the overall conversion remains below 40% for all polymers, the research shows a fascinating potential for energy-saving treatment of the plastic waste without greenhouse gas discharge.
Owing to that the bare semiconductor photocatalysts generally suffers from lack of surface active sites, and rapid recombination of photogenerated charge carriers, thus limiting the overall photocatalytic performance (Majeed et al., 2016; Shi et al., 2018). To resolve the problems, Liu et al. have synthesized a CdS/MoS2 nanoctahedral heterostructures by loading MoS2 as cocatalyst. The composite is synthesized using CdMoO4 nanoctahedron as template and precursor by in situ vulcanization with H2S (Supplementary Figures 2A,B) (Zhao et al., 2020). This in-situ synthesis method forms a close and sufficient S atomic contact interface between CdS and MoS2, which is conductive to the transfer of electrons from CdS to MoS2. When applied to photocatalytic conversion of plastic, the hybrid CdS/MoS2 shows significantly enhanced photocatalytic H2 evolution rates than pure CdS and pure MoS2 using PLA as reaction substrate under alkaline conditions (10 M NaOH) (Supplementary Figure 2C). Also, a high photocatalytic H2 evolution (60.8 mmol h−1 g−1) is observed for photocatalytic conversion of PET, demonstrating the universality of the CdS/MoS2 composite for integrated photocatalytic H2 production and plastic upgrading (Supplementary Figure 2D). 1H NMR analysis spectra of the PLA-NaOD solution shows a new peak after photocatalytic reforming reaction, which is ascribed to the oxidation product of alkali-induced pyruvate compounds. This was consistent with the reported results of Reisner et al. (Uekert et al., 2018). However, the addition of excess Ba(OH)2 solution into the supernate obtained after reaction forms white precipitate (Supplementary Figure 2E), indicating the generation of a certain amount of CO2 during the photoconversion of plastic. The study confirms the crucial role of loading cocatalyst to promote the photocatalytic valorization performance.
Moreover, Li et al. have constructed a 2D/2D MoS2/CdxZn1-xS (0.2 ≤ x ≤ 0.8, Mt/CxZ1−xS) heterostructure for PET photoconversion coupled with H2 production (Supplementary Figure 3A) (Li et al., 2021). As shown in Supplementary Figure 3B, in the presence of light and photocatalyst, the H2 evolution rates of MoS2/CxZ1-xS firstly increase with the increment of x until x = 0.5. 1H-NMR analysis shows that in NaOH aqueous solution, the PET is mainly hydrolyzed into ethylene glycol, terephthalic acid, and glycolate before reaction (Supplementary Figure 3C). After 5 h illumination, some small molecular organic compounds such as formate and methanol appear in the 1H-NMR spectrum, confirming the photocatalytic conversion of PET. Moreover, M4.3/C0.5Z0.5S shows high photocatalytic H2 evolution stability during 5 h continuous illumination in either PET or PET-bottle-based aqueous solution (Supplementary Figure 3D). This work extends the catalyst for the photocatalytic plastic upgrading.
Considering the toxicity of Cd in CdS/CdOx, in another work of Reisner, et al., they have further developed a new Cd-free catalyst, namely cyanamide-functionalized carbon nitride (CNX) coupled with a nickel phosphide (CNx/Ni2P) for photocatalytic valorization of plastic (Supplementary Figure 4A) (Uekert et al., 2019). Carbon nitride (CNx) has been studied widely in photocatalysis because of its non-toxic, cheap and visible light absorption. Particularly, the CNx has strong alkali resistance, thus endowing it to be a promising competitive alternative to CdS for photocatalytic conversion of plastic. As presented in Supplementary Figure 4B, under low pH conditions (1 M KOH), the CNx|Ni2P shows moderate photoactivity. The external quantum yields at λ = 430 nm is ca. 0.035 for PET and 0.101 for PLA. Increasing the alkalinity of the reaction solution can significantly improve the H2 evolution activity, as exemplified in Supplementary Figure 4C. Isotopic labeling experiments with D2O verify that H2 originates from water rather than the substrate. 1H NMR spectroscopy analysis of the reaction mixtures shows that the photoconversion of PET produces complex products, including terephthalate, formate, glyoxal, glycolate, acetate, and other intermediates yet without CO3 2− (Supplementary Figures 4D,G). On the contrary, the PLA offers a much simpler system. It is oxidized primarily to small quantities of formate (i) and acetate (iv) with a small amount of CO3 2− (Supplementary Figures 4E–G). The results are similar to those reported for photoreforming of PET with CdS/CdOx (Uekert et al., 2018). Moreover, the photoconversion of real-world plastic wastes with low concentration of plastic, including polyester microfibers, PET bottles, and oil-coated PET bottles, over the CNx|Ni2P has been evaluated, which also reveal noticeable H2 evolution activity (Supplementary Figure 4H). The result directly proves the transformation of real-world plastic waste into both H2 and valuable organics.
Furthermore, to overcome practical scaling challenges of traditional powder photocatalyst systems, such as sedimentation, difficult to recycle, and competing light absorption with waste solution, Reisner and co-workers have immobilized the CNx|Ni2P photocatalyst on textured glass (Supplementary Figure 4I) (Uekert et al., 2020b). It has been found that the 1 cm2 square CNx|Ni2P panel can photoconvert waste plastic into H2 and organic molecules with rates comparable to those of photocatalyst slurries, while enable facile photocatalyst recycling. Moreover, as shown in Supplementary Figure 4J, an up-scaled 25 cm2 panel is fabricated to photoreform municipal solid waste (MSW) in seawater under lower sunlight (0.2 Sun) for 5 days, which still keeps 50% of the activity tested under ideal conditions (0% seawater, 100 mW cm−2), thus further verifying the versatility and real-world applicability of the CNx|Ni2P panel system.
Photocatalytic Valorization of Waste Plastic to Oxygenated Chemical Feedstocks
In the above cases, the plastic is primarily hydrolyzed in alkaline solution to break the stubborn C-C chemical bonds, thereby hastening the photocatalytic process. In spite of the advantage, the alkaline pretreatment is detrimental to the natural environment. Given this aspect, it is imperative to find alternative ways to convert the plastic under mild conditions. In a study of Soo et al. (Gazi et al., 2019), they have demonstrated a vanadium (V) photocatalyst system ( Supplementary Figure 5A ) that can controllably cleave the C-C bond for organic transformations and plastic recycling in the natural environment without alkaline pretrement. The study is started by examining the performance of the V catalysts for breaking down carbon-carbon bonds in over 30 different types of chemicals. Quantitative conversions and moderate-to-high yields of aldehyde and alcohol products are obtained for all the substrates, demonstrating a broad functional group tolerance, and high catalytic applicability of the photocatalytic system.
Encouraged by the promising result, the authors further test the photocatalytic performance of the catalysts for conversion of PE ( Supplementary Figure 5B ). Notably, the reaction has to be conducted with white LEDs at 85°C in a mixture of acetonitrile and toluene to fully dissolve the substrate. The result reveals a full conversion of the PE after 6 days. Formic acid and methyl formate are identified as some of the products. However, CO2 is also detected, which is ascribed to the over-oxidation of formic acid. The reaction is suggested to proceed via LMCT mechanism. A depicted in Supplementary Figure 5C, the C-C bond of the hydroxyl-terminated polymer would cleave with the assistance of V catalyst to produce a carbonyl group as one of the primary products, while the other radical fragment would react with dissolved O2 in air to eventually give another oxygenated compound. This work highlights the practicability of the photocatalytic C-C activation for transformation of plastic wastes into chemical raw materials under alkali-free condition.
Photocatalytic Plastic Degradation Coupled With Sequential CO2 Reduction to Produce C2 Fuels
Besides one-step conversion, Xie, and co-workers (Jiao et al., 2020) recently have reported a sequential photoinduced C-C cleavage under atmosphere and C-C bond coupling in the absence of oxygen, which for the first time realizes highly selective conversion of various waste plastic into C2 fuels under simulated natural environment (room temperature, pure water, and simulated one-sun irradiation; Supplementary Figure 6A). In the research, single-unit-cell thick Nb2O5 layers (2.96 nm) is prepared from niobic acid atomic layer precursor as catalyst ( Supplementary Figure 6B ), due to its high redox potentials, valence band maximum at + 2.5 V vs. NHE and the conduction band minimum at 0.9 V vs. NHE at pH 7, which is conducive for maximal exposure of active site and thus promoting the light conversion performance. The photocatalytic conversion of real-world PE, polypropylene (PP) and PVC plastics over the ultrathin Nb2O5 shows that the catalyst can completely degrade these plastics to CO2 within 40, 60, and 90 h, respectively ( Supplementary Figure 6C ). Meanwhile, CH3COOH yields progressively increased during the photoconversion processes ( Supplementary Figure 6D ). Through a series of control experiments, isotope labeling and synchrotron radiation vacuum ultraviolet photoionization mass spectrometry, the CH3COOH products is verified to be generated by the photoreduction of the CO2 generated from degradation of plastic.
Correspondingly, a specific mechanism is proposed ( Supplementary Figure 6E ). The photoconversion of the plastic is suggested to undergo two continuous processes: initially, the plastic is oxidized by the O2 and •OH radical to cleave C-C bond and form CO2. Meantime, the O2 is reduced to O2•−, H2O2, and H2O. Subsequently, the resulting CO2 is further reduced to CH3COOH and the water was oxidized to O2 through the photoinduced C-C coupling reaction occurring between COOH intermediates. This is significantly different with the above discussed one-step strategy, providing a fascinating alternative strategy to valorize the real-word plastic without any pretrement. However, due to the poor CO2 reduction activity of the existing photocatalysts, the production of C2 fuel is relatively low at present.
Conclusion and Outlook
In summary, the latest advances in photocatalytic valorization of plastic for fuels and chemicals production are highlighted in this mini review. Three typical conversion pathways have been classified, including (i) photocatalytic plastic upgrading to fine chemicals integrated with H2 production; (ii) photocatalytic valorization of waste plastic to oxygenated chemical feedstocks and (iii) photocatalytic plastic degradation coupled with sequential CO2 reduction to produce C2 fuels. Correspondingly, the developed catalyst systems that fall into these reaction pathways, and products generated from the photocatalytic conversion of plastic are elaborately discussed. The key progresses made in this area present a fascinating alternative strategy to alleviate the white pollution crisis. Nevertheless, the investigation of photocatalytic valorization of plastic is still at an early stage of discovery, especially in comparison with the traditional photocatalytic applications, such as water splitting, CO2 reduction, and selective organic transformations. Some great challenges including the limitations of the overall reaction efficiency, selectivity, and durability lie between the laboratory study and practical application. In order to promote the deployment of the technique, more research effort is deserved.
For example, in spite of the charming future of the research field, the developed photocatalyst system for the plastic valorization is very limited at present. Over the past 40 years, a huge amount photocatalyst systems have been developed. Only for overall all water splitting-the challenging task in photocatalysis, more than 100 metal oxides-based photocatalytic systems have been reported. In this context, the exploration of new types of photocatalysts is highly desired, which would be the most direct way to advance the photocatalytic plastic valorization.
In addition, the loading of cocatalyst is essential for achieving highly efficient, selective, and steady photocatalytic plastic conversion. The cocatalyst can play multifunctional roles including (i) boosting the separation and transfer of photogenerated charge carriers, (ii) inhibiting the photocorrosion of catalysts and (iii) promoting adsorption and activation of target molecules. Till now, great progresses are achieved in the design and synthesis of noble metal-based, transition metal-based such as metal sulfide, phosphide, nitride, and oxides cocatalysts. Thus, the tailoring of active cocatalyst would greatly contribute to achieving high-efficiency photocatalytic plastic conversion system.
Furthermore, at present, alkaline pretreatment of plastic is the most commonly utilized reaction media, which would harm the environment. Moreover, the alkaline treatment generally induces non-selective cleavage of the C-C bond to mixed short-chain organic molecules, which leads to poor selectivity of the photogenerated products. Also, the alkaline reaction media may corrode some metal oxides photocatalysts and results in deactivation. As such, developing more universal and mild solvent and pretreatment method, such as mixed organic solvent, is indispensable.
Finally, to implement the photocatalytic plastic valorization from laboratory study to “practical” application, the construction of fixed photocatalyst device must be taken into account. Particularly, the fix of photocatalyst on flexibly porous substrate should be a preferred option. This would not only prevent sedimentation and promote catalyst recycling, but also alleviate the cost of reduced mass transfer between the catalyst and reactant. In the long run, only through the collective optimization of the catalyst, reaction media and reactor, the practical application of photocatlytic plastic valorization can be reliable.
Author Contributions
AC and MY conceived the idea, collected data, prepared and revised the manuscript. SW and QQ given scientific suggestions and commented on the manuscript.
Funding
This work was financially supported by the National Natural Science Foundation of China (21905049 and 21875037), the Award Program for Minjiang Scholar Professorship, and the Natural Science Foundation of Fujian Province (2020J01201).
Conflict of Interest
The handling Editor declared a past co-authorship with one of the authors (SW).
The remaining authors declare that the research was conducted in the absence of any commercial or financial relationships that could be construed as a potential conflict of interest.
Supplementary Material
The Supplementary Material for this article can be found online at: https://www.frontiersin.org/articles/10.3389/fnano.2021.723120/full#supplementary-material
Supplementary Figure 1. (A) Photocatalytic conversion of plastics over CdS/CdOx QDs under simulated solar light; (B) produced H2 amount of the photo-reforming of PET bottles under simulated solar irradiation over several days; 1H-NMR spectra of PLA (C), PET (D), and PUR (E); mass spectra of the gas evolved after photoreforming of PLA (24 h) over CdS/CdOx QDs in (F) 10 M NaOH, 10 M NaOD in D2O, or (G) 10 M NaOH. Conditions: AM 1.5G, 100 mW cm−2, 25°C. The figure is reprinted with permission from Uekert et al. (2018), copyright 2018, Royal Society of Chemistry.
Supplementary Figure 2. (A) Schematic transformation from CdMoO4 to CdS/MoS2 nanooctahedron heterostructure; (B) SEM image of CdS/MoS2; (C) photocatalytic H2 evolution activity of the different samples using pretreated PLA solution (stirring in 10 M KOH at 40°C for 24 h in the dark); (D) integrated photocatalytic H2 production and organics upgrading of furfural alcohol (FA), bacterial cellulose membrane (BC), pretreated PLA solution and PET solution over CdS/MoS2 in 10 M KOH; (E) photograph of the obtained white precipitate after adding excess Ba(OH)2 solution into the supernate taken immediately after photocatalysis. Conditions: λ ≥ 420 nm, 110.3 mW cm−2. The figure is reprinted with permission from Zhao et al. (2020), copyright 2020, Wiley.
Supplementary Figure 3. (A) The mechanism of H2 evolution coupled with the conversion of PET plastic over Mt/CxZ1-xS; (B) the control experiment and photocatalytic H2 evolution from pretreated PET solution (stirring in 10 M NaOH at 40°C for 24 h) of M4.3/CxZ1-xS (0.2 ≤ x ≤ 0.8) under simulated sunlight; (C) 1H-NMR spectra of an aqueous solution of pretreated PET plastic before and after photocatalytic H2 generation of 5 h; (D) photocatalytic H2 evolution stability of M4.3/C0.5Z0.5S under simulated sunlight and anaerobic environment. Condition: AM 1.5 G. The figure is reprinted with permission from Li et al. (2021), copyright 2021, Wiley.
Supplementary Figure 4. (A) Schematic diagram of the photocatalytic plastic valorization using a CNx|Ni2P photocatalyst; (B) long-term photoreforming of pretreated PET and PLA (stirring in 2 M KOH at 40°C for 24 h in the dark); (C) the influence of KOH concentration for photoreforming of pretreated PET (20 h irradiation); black circles in (C) mark H2 evolution per gram of substrate over CNx|Pt (2 wt%) under the same conditions; 1H NMR spectra of pretreated PET (D) and PLA (E, F) after photoreforming (insets show zoomed-out views of the spectra); (G) chemical structures and peak assignments; (H) long-term photoreforming of different plastics under simulated solar light. Conditions: AM 1.5 G, 100 mW cm−2, 25°C. The figure is reprinted with permission from Uekert et al. (2019), copyright 2019, American Chemical Society. (I) Photograph of a 25 cm2 CNx|Ni2P panel with a schematic diagram of the photoreforming process; (J) up-scaled long-term H2 evolution under ideal (100 mW cm−2, pure deionized water) and worst (20 mW cm−2, seawater) cases. Conditions: 100 mW cm−2 or 20 mW cm−2, 25°C, MSW (stirring in 0.5 M KOH at 80°C for overnight). The figure is reprinted with permission from Uekert et al. (2020a), copyright 2020, Wiley.
Supplementary Figure 5. (A) Structure of the V-based photocatalyst; (B) the conversion scheme of PE over the V catalysts; (C) proposed reaction mechanism for the cascade C-C bond cleavage in polyethylene-monoalcohol. The bonds that undergo cleavage are highlighted in bold and red. Conditions: white LEDs, 85°C. The figure is reprinted with permission from Gazi et al. (2019), copyright 2019, Wiley.
Supplementary Figure 6. (A) Schematic illustration for converting various waste plastic into C2 fuels by a designed sequential pathway under simulated natural environment conditions; (B) atomic force microscopy image of Nb2O5; (C) the yield of CO2 during the photoconversion of pure PE, PP, and PVC over the Nb2O5 atomic layers; (D) the yield of CH3COOH under the simulated solar light; (E) schematic representations for the band-edge positions of Nb2O5 atomic layers along with the potentials of CO2, H2O, H2O2, and O2 redox couples at pH 7 and the proposed two-step C-C bond cleavage and coupling mechanism from pure PE into CH3COOH. Conditions: AM 1.5G, 100 mW cm−2, 298 ± 0.2 K. The figure is reprinted with permission from Jiao et al. (2020), copyright 2020, Wiley.
References
Al-Salem, S. M., Lettieri, P., and Baeyens, J. (2009). Recycling and Recovery Routes of Plastic Solid Waste (PSW): A Review. Waste Manage. 29 (10), 2625–2643. doi:10.1016/j.wasman.2009.06.004
Andrady, A. L., and Neal, M. A. (2009). Applications and Societal Benefits of Plastics. Phil. Trans. R. Soc. B 364 (1526), 1977–1984. doi:10.1098/rstb.2008.0304
Bai, B., Jin, H., Fan, C., Cao, C., Wei, W., and Cao, W. (2019). Experimental Investigation on Liquefaction of Plastic Waste to Oil in Supercritical Water. Waste Manage. 89, 247–253. doi:10.1016/j.wasman.2019.04.017
Clarizia, L., Russo, D., Di Somma, I., Andreozzi, R., and Marotta, R. (2017). Hydrogen Generation through Solar Photocatalytic Processes: A Review of the Configuration and the Properties of Effective Metal-Based Semiconductor Nanomaterials. Energies 10 (10), 1624. doi:10.3390/en10101624
Das, S., and Mahalingam, H. (2019). Dye Degradation Studies Using Immobilized Pristine and Waste Polystyrene-TiO2/rGO/g-C3N4 Nanocomposite Photocatalytic Film in a Novel Airlift Reactor under Solar Light. J. Environ. Chem. Eng. 7 (5), 103289. doi:10.1016/j.jece.2019.103289
de Assis, G. C., Skovroinski, E., Leite, V. D., Rodrigues, M. O., Galembeck, A., Alves, M. C. F., et al. (2018). Conversion of “Waste Plastic” into Photocatalytic Nanofoams for Environmental Remediation. ACS Appl. Mater. Inter. 10 (9), 8077–8085. doi:10.1021/acsami.7b19834
Eerkes-Medrano, D., Thompson, R. C., and Aldridge, D. C. (2015). Microplastics in Freshwater Systems: A Review of the Emerging Threats, Identification of Knowledge Gaps and Prioritisation of Research Needs. Water Res. 75, 63–82. doi:10.1016/j.watres.2015.02.012
Feng, H.-M., Zheng, J.-C., Lei, N.-Y., Yu, L., Kong, K. H.-K., Yu, H.-Q., et al. (2011). Photoassisted Fenton Degradation of Polystyrene. Environ. Sci. Technol. 45 (2), 744–750. doi:10.1021/es102182g
Garcia, J. M., and Robertson, M. L. (2017). The Future of Plastics Recycling. Science 358 (6365), 870–872. doi:10.1126/science.aaq0324
Gausas, L., Kristensen, S. K., Sun, H., Ahrens, A., Donslund, B. S., Lindhardt, A. T., et al. (2021). Catalytic Hydrogenation of Polyurethanes to Base Chemicals: From Model Systems to Commercial and End-of-Life Polyurethane Materials. JACS Au 1 (4), 517–524. doi:10.1021/jacsau.1c00050
Gazi, S., Đokić, M., Chin, K. F., Ng, P. R., and Soo, H. S. (2019). Visible Light-Driven Cascade Carbon-Carbon Bond Scission for Organic Transformations and Plastics Recycling. Adv. Sci. 6 (24), 1902020. doi:10.1002/advs.201902020
Geyer, R. (2020). “Production, Use, and Fate of Synthetic Polymers,” in Plastic Waste and Recycling, 13–32. doi:10.1016/B978-0-12-817880-5.00002-5
Habisreutinger, S. N., Schmidt-Mende, L., and Stolarczyk, J. K. (2013). Photocatalytic Reduction of CO2 on TiO2 and Other Semiconductors. Angew. Chem. Int. Ed. 52 (29), 7372–7408. doi:10.1002/anie.201207199
Jehanno, C., and Sardon, H. (2019). Dynamic Polymer Network Points the Way to Truly Recyclable Plastics. Nature 568 (7753), 467–468. doi:10.1038/d41586-019-01209-3
Jiao, X., Zheng, K., Chen, Q., Li, X., Li, Y., Shao, W., et al. (2020). Photocatalytic Conversion of Waste Plastics into C2 Fuels under Simulated Natural Environment Conditions. Angew. Chem. Int. Ed. 59 (36), 15497–15501. doi:10.1002/anie.201915766
Jie, X., Li, W., Slocombe, D., Gao, Y., Banerjee, I., Gonzalez-Cortes, S., et al. (2020). Microwave-Initiated Catalytic Deconstruction of Plastic Waste into Hydrogen and High-Value Carbons. Nat. Catal. 3 (11), 902–912. doi:10.1038/s41929-020-00518-5
Kawai, T., and Sakata, T. (1981). Photocatalytic Hydrogen Production from Water by the Decomposition of Poly-Vinylchloride, Protein, Algae, Dead Insects, and Excrement. Chem. Lett. 10 (1), 81–84. doi:10.1246/cl.1981.81
Keane, M. A. (2007). Catalytic Conversion of Waste Plastics: Focus on Waste PVC. J. Chem. Technol. Biotechnol. 82 (9), 787–795. doi:10.1002/jctb.1757
Kumar, S., Panda, A. K., and Singh, R. K. (2011). A Review on Tertiary Recycling of High-Density Polyethylene to Fuel. Resour. Conservation Recycling 55 (11), 893–910. doi:10.1016/j.resconrec.2011.05.005
Li, Y., Wan, S., Lin, C., Gao, Y., Lu, Y., Wang, L., et al. (2021). Engineering of 2D/2D MoS2/CdXZn1−XS Photocatalyst for Solar H2 Evolution Coupled with Degradation of Plastic in Alkaline Solution. Sol. RRL 5 (6), 2000427. doi:10.1002/solr.202000427
Liang, W., Luo, Y., Song, S., Dong, X., and Yu, X. (2013). High Photocatalytic Degradation Activity of Polyethylene Containing Polyacrylamide Grafted TiO2. Polym. Degrad. Stab. 98 (9), 1754–1761. doi:10.1016/j.polymdegradstab.2013.05.027
Liu, S., Tang, Z.-R., Sun, Y., Colmenares, J. C., and Xu, Y.-J. (2015). One-Dimension-Based Spatially Ordered Architectures for Solar Energy Conversion. Chem. Soc. Rev. 44 (15), 5053–5075. doi:10.1039/c4cs00408f
Lopez, G., Artetxe, M., Amutio, M., Bilbao, J., and Olazar, M. (2017). Thermochemical Routes for the Valorization of Waste Polyolefinic Plastics to Produce Fuels and Chemicals. A Review. Renew. Sustain. Energ. Rev. 73, 346–368. doi:10.1016/j.rser.2017.01.142
Lu, K.-Q., Qi, M.-Y., Tang, Z.-R., and Xu, Y.-J. (2019). Earth-Abundant MoS2 and Cobalt Phosphate Dual Cocatalysts on 1D CdS Nanowires for Boosting Photocatalytic Hydrogen Production. Langmuir 35 (34), 11056–11065. doi:10.1021/acs.langmuir.9b01409
Majeed, I., Nadeem, M. A., Al-Oufi, M., Nadeem, M. A., Waterhouse, G. I. N., Badshah, A., et al. (2016). On the Role of Metal Particle Size and Surface Coverage for Photo-Catalytic Hydrogen Production: A Case Study of the Au/CdS System. Appl. Catal. B: Environ. 182, 266–276. doi:10.1016/j.apcatb.2015.09.039
Mark, L. O., Cendejas, M. C., and Hermans, I. (2020). The Use of Heterogeneous Catalysis in the Chemical Valorization of Plastic Waste. ChemSusChem 13 (22), 5808–5836. doi:10.1002/cssc.202001905
Martín, A. J., Mondelli, C., Jaydev, S. D., and Pérez-Ramírez, J. (2021). Catalytic Processing of Plastic Waste on the Rise. Chem 7, 1487–1533. doi:10.1016/j.chempr.2020.12.006
PlasticEurope (2020). Plastic-the Facts 2020. Available at: https://www.plasticseurope.org/en/ resources/publications/4312-plastics-facts-2020.
Rahimi, A., and García, J. M. (2017). Chemical Recycling of Waste Plastics for New Materials Production. Nat. Rev. Chem. 1 (6). doi:10.1038/s41570-017-0046
Ru, J., Huo, Y., and Yang, Y. (2020). Microbial Degradation and Valorization of Plastic Wastes. Front. Microbiol. 11, 442. doi:10.3389/fmicb.2020.00442
Schyns, Z. O. G., and Shaver, M. P. (2021). Mechanical Recycling of Packaging Plastics: A Review. Macromol. Rapid Commun. 42 (3), 2000415. doi:10.1002/marc.202000415
Shang, J., Chai, M., and Zhu, Y. (2003). Photocatalytic Degradation of Polystyrene Plastic under Fluorescent Light. Environ. Sci. Technol. 37 (19), 4494–4499. doi:10.1021/es0209464
Shang, W., Li, Y., Huang, H., Lai, F., Roeffaers, M. B. J., and Weng, B. (2021). Synergistic Redox Reaction for Value-Added Organic Transformation via Dual-Functional Photocatalytic Systems. ACS Catal. 11 (8), 4613–4632. doi:10.1021/acscatal.0c04815
Shi, R., Ye, H.-F., Liang, F., Wang, Z., Li, K., Weng, Y., et al. (2018). Interstitial P-Doped CdS with Long-Lived Photogenerated Electrons for Photocatalytic Water Splitting without Sacrificial Agents. Adv. Mater. 30 (6), 1705941. doi:10.1002/adma.201705941
Tennakoon, A., Wu, X., Paterson, A. L., Patnaik, S., Pei, Y., LaPointe, A. M., et al. (2020). Catalytic Upcycling of High-Density Polyethylene via a Processive Mechanism. Nat. Catal. 3 (11), 893–901. doi:10.1038/s41929-020-00519-4
Thompson, R. C., Olsen, Y., Mitchell, R. P., Davis, A., Rowland, S. J., John, A. W., et al. (2004). Lost at Sea: Where Is All the Plastic? Science 304 (5672), 838. doi:10.1126/science.1094559
Tofa, T. S., Kunjali, K. L., Paul, S., and Dutta, J. (2019). Visible Light Photocatalytic Degradation of Microplastic Residues with Zinc Oxide Nanorods. Environ. Chem. Lett. 17 (3), 1341–1346. doi:10.1007/s10311-019-00859-z
Uekert, T., Bajada, M. A., Schubert, T., Pichler, C. M., and Reisner, E. (2020b). Scalable Photocatalyst Panels for Photoreforming of Plastic, Biomass and Mixed Waste in Flow. ChemSusChem. doi:10.1002/cssc.202002580
Uekert, T., Kasap, H., and Reisner, E. (2019). Photoreforming of Nonrecyclable Plastic Waste over a Carbon Nitride/Nickel Phosphide Catalyst. J. Am. Chem. Soc. 141 (38), 15201–15210. doi:10.1021/jacs.9b06872
Uekert, T., Kuehnel, M. F., Wakerley, D. W., and Reisner, E. (2018). Plastic Waste as a Feedstock for Solar-Driven H2 Generation. Energy Environ. Sci. 11 (10), 2853–2857. doi:10.1039/c8ee01408f
Uekert, T., Pichler, C. M., Schubert, T., and Reisner, E. (2020a). Solar-Driven Reforming of Solid Waste for a Sustainable Future. Nat. Sustain. 4 (5), 383–391. doi:10.1038/s41893-020-00650-x
Vollmer, I., Jenks, M. J. F., Roelands, M. C. P., White, R. J., Harmelen, T., Wild, P., et al. (2020). Beyond Mechanical Recycling: Giving New Life to Plastic Waste. Angew. Chem. Int. Ed. 59 (36), 15402–15423. doi:10.1002/anie.201915651
Worch, J. C., and Dove, A. P. (2020). 100th Anniversary of Macromolecular Science Viewpoint: Toward Catalytic Chemical Recycling of Waste (And Future) Plastics. ACS Macro Lett. 9 (11), 1494–1506. doi:10.1021/acsmacrolett.0c00582
Xiao, F.-X., Miao, J., Tao, H. B., Hung, S.-F., Wang, H.-Y., Yang, H. B., et al. (2015). One-Dimensional Hybrid Nanostructures for Heterogeneous Photocatalysis and Photoelectrocatalysis. Small 11 (18), 2115–2131. doi:10.1002/smll.201402420
Yang, M.-Q., Gao, M., Hong, M., and Ho, G. W. (2018). Visible-to-NiR Photon Harvesting: Progressive Engineering of Catalysts for Solar-Powered Environmental Purification and Fuel Production. Adv. Mater. 30 (47), 1802894. doi:10.1002/adma.201802894
Yang, M.-Q., Han, C., Zhang, N., and Xu, Y.-J. (2015). Precursor Chemistry Matters in Boosting Photoredox Activity of Graphene/Semiconductor Composites. Nanoscale 7 (43), 18062–18070. doi:10.1039/c5nr05143f
Yang, M.-Q., and Xu, Y.-J. (2016). Photocatalytic Conversion of CO2 over Graphene-Based Composites: Current Status and Future Perspective. Nanoscale Horiz. 1 (3), 185–200. doi:10.1039/c5nh00113g
Zhang, F., Zhao, Y., Wang, D., Yan, M., Zhang, J., Zhang, P., et al. (2021). Current Technologies for Plastic Waste Treatment: A Review. J. Clean. Prod. 282, 124523. doi:10.1016/j.jclepro.2020.124523
Zhang, K., Hamidian, A. H., Tubić, A., Zhang, Y., Fang, J. K. H., Wu, C., et al. (2021). Understanding Plastic Degradation and Microplastic Formation in the Environment: A Review. Environ. Pollut. 274, 116554. doi:10.1016/j.envpol.2021.116554
Zhao, L., Dong, T., Du, J., Liu, H., Yuan, H., Wang, Y., et al. (2020). Synthesis of CdS/MoS2 Nanooctahedrons Heterostructure with a Tight Interface for Enhanced Photocatalytic H2 Evolution and Biomass Upgrading. Sol. RRL 5, 2000415. doi:10.1002/solr.202000415
Zhao, X., Li, Z., Chen, Y., Shi, L., and Zhu, Y. (2008). Enhancement of Photocatalytic Degradation of Polyethylene Plastic with CuPc Modified TiO2 Photocatalyst under Solar Light Irradiation. Appl. Surf. Sci. 254 (6), 1825–1829. doi:10.1016/j.apsusc.2007.07.154
Keywords: photocatalysis, valorization, plastic waste, solar energy, chemical fuels
Citation: Chen A, Yang M-Q, Wang S and Qian Q (2021) Recent Advancements in Photocatalytic Valorization of Plastic Waste to Chemicals and Fuels. Front. Nanotechnol. 3:723120. doi: 10.3389/fnano.2021.723120
Received: 10 June 2021; Accepted: 28 June 2021;
Published: 12 July 2021.
Edited by:
Wee-Jun Ong, Xiamen University, MalaysiaReviewed by:
Xin-Yao Yu, Zhejiang University, ChinaTing Zhu, Central South University, China
Tianpeng Ding, National University of Singapore, Singapore
Copyright © 2021 Chen, Yang, Wang and Qian. This is an open-access article distributed under the terms of the Creative Commons Attribution License (CC BY). The use, distribution or reproduction in other forums is permitted, provided the original author(s) and the copyright owner(s) are credited and that the original publication in this journal is cited, in accordance with accepted academic practice. No use, distribution or reproduction is permitted which does not comply with these terms.
*Correspondence: Min-Quan Yang, eWFuZ21xQGZqbnUuZWR1LmNu; Sibo Wang, c2lib3dhbmdAZnp1LmVkdS5jbg==; Qingrong Qian, cXJxaWFuQGZqbnUuZWR1LmNu