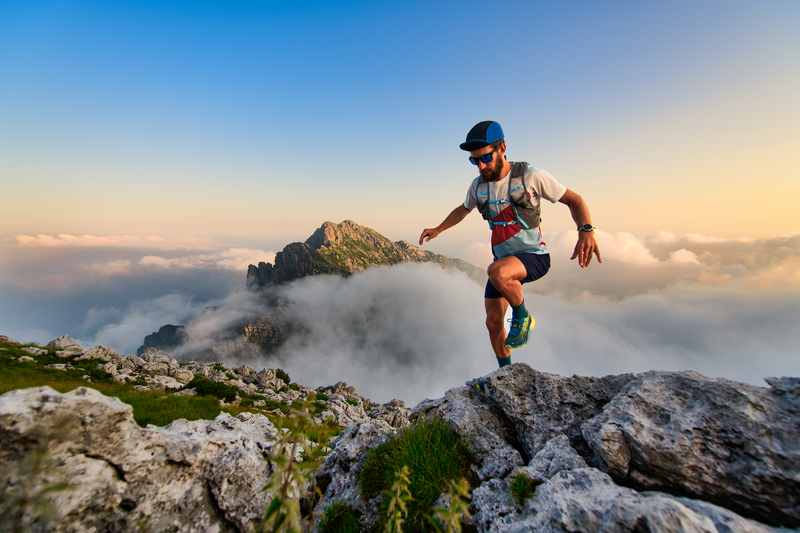
94% of researchers rate our articles as excellent or good
Learn more about the work of our research integrity team to safeguard the quality of each article we publish.
Find out more
REVIEW article
Front. Nanotechnol. , 09 July 2021
Sec. Biomedical Nanotechnology
Volume 3 - 2021 | https://doi.org/10.3389/fnano.2021.681305
This article is part of the Research Topic Nanotechnology for Precision Cancer Therapy: Advances in gene therapy, immunotherapy, and 3D bioprinting View all 11 articles
Nanoparticles have tremendous therapeutic potential in the treatment of cancer as they increase drug delivery, attenuate drug toxicity, and protect drugs from rapid clearance. Since Doxil®, the first FDA-approved nanomedicine, several other cancer nanomedicines have been approved and have successfully increased the efficacy over their free drug counterparts. Although their mechanisms of action are well established, their effects towards our immune system, particularly in the tumor microenvironment (TME), still warrant further investigation. Herein, we review the interactions between an approved cancer nanomedicine with TME immunology. We also discuss the challenges that need to be addressed for the full clinical potential of ongoing cancer nanomedicines despite the encouraging preclinical data.
Tumor immunology is the interaction between cells of the immune system with tumor cells which lead to our understanding in the mechanisms of both tumor rejection and tumor progression (Copier and Dalgleish, 2013). In cancer, tumors may undergo “spontaneous regression” in which a tumor disappears on its own. This phenomenon can be attributed to the active immune system that is triggered by a secondary immune stimulation such as an active infection, which can then initiate an antitumor cell immune response (Tadmor, 2019).
In principle, our immune system protects us against cancer through three primary roles which are 1) elimination of the potentially virus-induced tumor infection, 2) prompt resolution of inflammation that is conducive for tumorigenesis, and 3) identification and elimination of tumor cells based on their expression of tumor-specific antigens (Swann and Smyth, 2007). The third process is called immune surveillance that ideally eliminates all tumors promptly upon identification of their antigen. However, some malignancies appear to escape immune surveillance by either inducing tolerance rather than an active immune response or the immune system eventually is too overwhelmed and hence the tumor progresses (Ostrand-Rosenberg, 2008; Mak et al., 2014).
In immune surveillance, tumor antigens (TAs) play important parts in the development of the tumor microenvironment (TME). They generally fall into two classes, tumor-associated antigens (TAAs) and tumor-specific antigens (TSAs), TAs are presented by major histocompatibility complex (MHC) I and II on the surface of tumor cells and trigger immune response in the host (Mak et al., 2014). TAAs are normal proteins or carbohydrates expressed in a way that is abnormal relative to its status in the healthy, fully differentiated cells in the surrounding tissue of origin. For example, they may be expressed in abnormal concentrations and at wrong locations and times. Meanwhile, TSAs are new macromolecules that are unique to the tumor and are not produced by any type of normal cells. Due to their non-self nature, TSAs constitute true immunogens capable of eliciting an immune response. Overall, TAs can be categorized into several types including oncofetal, oncoviral, overexpressed or accumulated, cancer-testis, lineage-restricted, mutated, post-translationally altered, and idiotypic (Zarour et al., 2003). Hence, identification of TAAs and TSAs serve as a reliable biomarker for tumor diagnosis as well as a target for the development of cancer vaccines (Aly, 2012).
In the TME, there are two possible interactions that might happen. First is the antitumor immunity that works to prevent tumorigenesis in the first place (Munhoz and Postow, 2016). In antitumor immunity, both innate and adaptive immune responses are activated by TAs leading to tumor control. In this immunity, leukocytes such as tumor-infiltrating lymphocytes (TILs) which are mature CD4+ or CD8+ or B cells directly respond to the presence of a tumor cell (Mak et al., 2014). The second interaction is the evasion of antitumor immunity or immune escape as the immune system does not always succeed in controlling tumorigenesis. It is widely accepted that tumor immunoediting is a dynamic process that not only involves antitumor immunity, but shapes the immunogenicity of developing tumors as well. There are three distinct phases of tumor immunoediting which are elimination, equilibrium, and escape (Muenst et al., 2016). All three phases of tumor immunoediting are manifested through metabolic and cellular changes, in which the differences influence different types of cancer (Teng et al., 2008; Wenbo and Wang, 2017).
Elimination is a phase where evolving tumors are successfully rejected by the innate and adaptive immune response through various mechanisms (IFNγ, Perforin, TRAIL, IFNα/β, NKG2D) (Swann and Smyth, 2007). Then, some of the tumor cells that are not completely eliminated may enter the equilibrium phase when the immune system controls tumor outgrowth and tumor cells enter a dormant state or continue to evolve over a period of time (Dunn et al., 2004). In this phase, the constant interaction of tumor cells with the immune system over a period of time may edit the phenotype of the developing tumor into a less immunogenic state (Teng et al., 2008). Being in this state, the tumor cells are no longer susceptible to immune attack and this is where the tumor cells may escape from immune control and proliferate in an unrestricted manner, leading to clinically apparent tumors (Muenst et al., 2016). According to Mak et al., 2014, there are two forms of escape from immune control that are thought to be associated with all TMEs, regardless of which leukocytes respond to the malignancy. First is the abnormal property of the tumor vasculature comprised of capillaries that wind in and out of a tumor mass that hinder leukocyte extravasation into the tumor site. The second form of escape is from the elevated levels of plasma TGFβ that is established to promote malignant transformation of fibroblasts and stimulate angiogenesis within the tumor, termed as immunosuppression.
Over the years, the Food and Drug Administration (FDA) in the US and its equivalent in the EU, the European Medicines Agency (EMA), have certified a number of nanomedicine-based drugs for cancer diagnostic and therapeutic purposes, and many other formulations are currently being evaluated (Martinelli et al., 2019). Worldwide, nearly 250 formulations based on the nanotechnology platform have been approved for the market or are in various clinical stages for evaluation (Bremer-Hoffmann et al., 2018) (Table 1). The approval process for nanomedicine in humans regulated by the FDA is essentially the same as for any other regulated drug, device, or biologic (Eifler and Thaxton, 2011). According to the FDA, development of a drug and its approval is categorized into three major phases as outlined in Figure 1. Following discovery of the material, the pre-clinical phase of testing usually involves animal studies to demonstrate the efficacy, safety, and toxicity profile and to identify appropriate dose ranges (Tinkle et al., 2014). The FDA approval process is time consuming, labor intensive, and rigorous, hence it is estimated that it takes approximately 10–15 years to develop a new medicine (DiMasi et al., 2003). For nanomedicine, the important aspect regarding its R&D, highlighted by the FDA, is the comprehensive characterization of the nanomaterial considering its efficacy, toxicity, and physiochemical properties (Bobo et al., 2016). These findings are compiled into an Investigational New Drug (IND) application for FDA consideration. Upon approval of an IND, clinical trials, which are divided into three phases, are conducted to determine the safety and efficacy of the new nanomedicine. Since 2005, more than 30 new and abbreviated drug applications involving nanomaterials have been approved by the FDA (D’Mello et al., 2017). This is remarkable for a newly developing field. By comparison, for recombinant proteins and for antibody-based therapeutics, it took almost 2 decades of developments before the first drugs started to make it to the market (Reichert, 2003). More than 50 drug products containing nanomaterials are FDA approved for clinical use and more than a dozen of them have been approved in the last decade (Bobo et al., 2016; D’Mello et al., 2017).
FIGURE 1. Process of new drug development according to the FDA, adopted from (Lipsky and Sharp, 2001).
Previously, most cancer therapies were designed to directly killed/removed tumor cells either by pharmacological agents, surgery, or radiotherapy. Then it moved to targeted therapy when specific drugs with some molecular targets such as selective kinase inhibitors and monoclonal antibodies were developed (Falzone et al., 2018). While these therapies significantly improved quality of life as well as survival of cancer patients, variable efficacy and safety issues persistently limited the full capacity of cancer therapies. Nanomedicine offers these therapies a better targeting approach that would increase drug accumulation into a tumor without affecting other healthy cells, thus reducing systemic toxicities (Gao et al., 2019). Furthermore, nanomedicine is established to address several issues with current cancer therapies including the low response rate of free drugs as well as the emergence of drug resistance. Like the drug itself, introduction of NPs would induce a different interaction in the body, particularly with the immune system, either targeted or spontaneous.
Cancer chemotherapy is often immunosuppressive and drug resistance usually occurs after a short period of tumor shrinkage. Certain chemotherapeutic drugs such as doxorubicin have the potential to increase tumor immunogenicity through activation of immunogenic cell death (ICD). ICD is defined as the chronic exposure of damage-associated molecular patterns (DAMPs) in the TME, which provide long-lasting antitumor immunity (Zhou et al., 2019). Doxil is shown to increase the expression of CD80 on mature dendritic cells which activate an anti-tumor T cell response (Rios-Doria et al., 2015), improve macrophage immunostimulatory (M1) content in tumor tissue and efficacy of immune checkpoint blocking antibodies anti-CTLA-4/anti-PD-1 (Panagi et al., 2020), upregulate MHC-1 and Fas, and sensitize CTL killing and Fas-mediated death in vitro (Alagkiozidis et al., 2009). Meanwhile, Abraxane is taken up by macrophages via macropinocytosis which induces M1 cytokine expression and promotes nitric oxide synthase expression, thus increasing cytotoxicity towards tumor cells (Cullis et al., 2017). Furthermore, Abraxane is shown to enhance drug uptake and penetration into tumors in vitro, hence the superior efficacy in numerous cancer types compared to Taxol alone (Yuan et al., 2020).
Mepact is a liposome conjugated to a synthetic analog of a bacterial cell wall component and is used as an adjuvant in standard chemotherapy. This potent, non-specific immunomodulator mediates the activation of monocytes and macrophages, thus modulating the balance of immune responses such as increased circulating TNF and IL-6 (Punzo et al., 2020). Not only that, Mepact is demonstrated to be a possible anti-resorption agent by reducing pro-osteoporotic markers, thus explaining the improved overall survival from osteosarcoma (Ando et al., 2011; Bellini et al., 2017). Oncaspar, a PEGylated form of native Escheria coli-asparaginase is indicated for treatment of acute lymphoblastic leukemia. The PEGylation showed diminished asparaginase immunogenicity without affecting its enzymatic properties (Heo et al., 2019). Reduction of Oncaspar’s immunogenicity is portrayed by the decrease of neutralizing antibodies that may induce hypersensitivity and/or loss of enzyme activity. Ontak is an engineered fusion protein of IL-2 and diphtheria toxin that targets the IL-2 receptor, such as CD25 on tumor-infiltrating cells regulatory T cells (Tregs), the internalization releases diphtheria toxin, causing apoptosis (Foss, 2006). The effect of Ontak on immunosuppressive Tregs further enhances anticancer immune responses. Furthermore, CD25 that can be targeted by the IL-2 fusion protein on Ontak is also present on lymphoid tumor cells and dendritic cells effector T cells, making this recombinant protein a great pharmacological intervention strategy (Lutz et al., 2014). However, due to production issues related to bacterial immunotoxin, Ontak was discontinued in 2014 although currently there are several Ontak-like formulations under development that use other bacterial expression systems (Shafiee et al., 2019).
The tumor microenvironment (TME) consists of a complex ecosystem with blood vessels, immune cells, fibroblast, extracellular matrix, cytokines, and hormones that promote the growth of cancer. So, the normalization of the TME to a normal tissue environment may inhibit the growth of cancer and improve cancer therapeutics including checkpoint blockers and TNFR agonists. In in vitro studies, nanoparticles such as gold have been demonstrated to facilitate TME normalization, increase blood perfusion, and reduce hypoxia (Li et al., 2016; Li et al., 2017; Xiao et al., 2017). Instead of playing a role in TME normalization directly, the efficacy of nanomedicine is enhanced when adjuvanted with several approaches of TME normalization including anti-inflammatory agents, immune checkpoint blockade, and stromal and tumor vessel normalization (Zheng and Gao, 2019). Furthermore, studies showed that TME normalization improves the delivery of nanomedicine in a size-dependent manner (Chauhan et al., 2012). Delivery of Doxil, with a diameter of ∼100 nm is hindered upon normalization of blood vessels by the VEGFR-2 blocker while enhanced delivery of the smaller diameter Abraxane was demonstrated, hence greater accumulation within the TME. Meanwhile, Onivyde, liposomal irinotecan, is shown to enhance accumulation of active metabolites within the TME, thus improving its antitumor activity with minimal systemic toxicity (Zhang, 2016). Another issue in pharmacological intervention in cancer that needs to be addressed is their defective vasculature. Due to this, macromolecules such as drugs could not be retained in tumor cells and leak out into interstitial space, limiting its efficacy. Nanoparticles, due to their physiochemical properties, could be utilized to address this issue using the principle of enhanced permeability and retention (EPR) (Maeda, 2017). SMANCS, a conjugate of a hydrophobic polymer with antitumor Neocarzinostin is the first nanomedicine using this EPR principle, was developed to selectively deliver drugs to solid tumors and prolong intratumoral concentration of the drug (Maeda, 2012).
Chemotherapy is known to induce several side effects such as myelosuppression, cardiotoxicity, and even skin toxicity which is a dose-limiting factor that often limits drug efficacy. Since chemotherapy suppresses the hematopoietic system and impairs its protective mechanism, neutropenia is one of the serious adverse events associated with the risk of life-threatening infections. Doxil is reported to be much less toxic to the immune system than free doxorubicin with comparable efficacy (O’Brien et al., 2004). In a systematic review, Abraxane is demonstrated to induce a higher number of hematological toxic effects (neutropenia, leucopenia, increased alanine aminotransferase and aspartate aminotransferase) and frequent non-hematological toxic effects (peripheral sensory neuropathy) compared to the free drug-paclitaxel group (Zong et al., 2017). Abraxane is also reported to cause drug-induced immune hemolytic anemia, a rare but fatal adverse event that affects only one patient in a one million population (Thomas and Shillingburg, 2015). Marqibo is designed to overcome the dosing and pharmacokinetic limitation of Vincristine. Marqibo is demonstrated to increase the circulation time with targeted and intense delivery of Vincristine without augmented toxicities including hematologic toxicity (Deitcher et al., 2014). Most common adverse events for Onivyde and Vyxeos are neutropenia, abdominal pain, and diarrhea that are considered manageable, except for prolonged severe neutropenia in patients receiving Vyxeos (Zhang, 2016; Tzogani et al., 2020). Cardiotoxicity is another toxicity induced by chemotherapeutic drugs including nanomedicine. Doxil and Daunoxome are both demonstrated to reduce the rate of cardiotoxicity compared to their free drugs, Doxorubicin and Daunorubicin, which are significantly limited by dose-dependent cardiotoxicity (O’Brien et al., 2004; Fassas and Anagnostopoulos, 2009). Palmar-plantar erythrodysesthesia or hand-foot syndrome is a type of skin toxicity that could develop from some cancer treatments. This type of skin toxicity is demonstrated to often occur in patients receiving PEGylated liposomal doxorubicin such as Doxil (Huang et al., 2018; Ni et al., 2020). Polymer-based nanomedicine including Genexol and Eligard are demonstrated to show good safety profiles in terms of the absence of increased toxicities and occurrence of adverse events (Sartor, 2003; Kim et al., 2004).
Hypersensitivity upon administration of a variety of drugs is common, including nanomedicine formulation. Doxil is reported to cause hypersensitivity, which is a non IgE-mediated allergy caused by activation of a complement referred to as complement activation-related pseudo allergy (CARPA) (Chanan-Khan et al., 2003). The mechanism of CARPA upon administration of Doxil is partly associated with some pre-existing anti-PEG antibodies (Neun et al., 2018). Since solvent-based taxane administration such as paclitaxel induces a high rate of hypersensitivity, albumin-bound paclitaxel, Abraxane, represents a valid treatment option as fewer hypersensitivity reactions towards Abraxane compared to free paclitaxel have been reported (Zong et al., 2017; Parisi et al., 2019). Unlike PEG, the absence of cross-reactivity between a previous history of hypersensitivity towards taxanes and Abraxane indicate the advantageous safety profile of this nanomedicine (Pellegrino et al., 2017). However, another approach of PEGylation of E. coli asparaginase in Oncaspar successfully reduced immunogenicity of the enzyme, which subsequently reduced the occurrence of hypersensitivity (Heo et al., 2019). As an immunomodulator, Mepact could activate immune responses with a standby effect, thus causing a hypersensitivity reaction such as pericardial effusion (Şimşek et al., 2020). The mechanism of this reaction might be due to both the active and inactive ingredients of Mepact that target immune cells in the lungs (Anderson et al., 2010). The most common related effects for Mepact are chills, fever, and headache in the initial dose and delayed fatigue in the subsequent doses (Jimmy et al., 2017).
In conclusion, incorporation of NPs with cancer drugs induce a different effect towards host immune responses compared to free drugs, either intended or spontaneous. Its immunogenicity, normalization of the TME, tolerability, and other infusion-related reactions could be due to NPs’ own physiochemical characteristics or interaction between the drugs (Figure 2).
FIGURE 2. Immune responses of cancer nanomedicine. Combination of nanoparticles with cancer drugs induce a different effect towards immune responses compared to free drugs in terms of (A) Normalization of the tumor microenvironment, (B) Immunogenicity, (C) Hypersensitivity, and (D) Tolerability. (A) Due to permeable vasculature in the tumor microenvironment, nanomedicine is designed with an enhanced permeability and retention (EPR) effect to enhance the effect of the drug. (B) Upon introduction of nanomedicine, immune cells such as antigen-presenting cells (APC) and T cells promote the release of mediators and induce immunogenic cell death (ICD) pathways. (D) Nanomedicine is demonstrated to increase the tolerability of drugs due to targeted release into tumor cells. (C) However, nanomedicine could provoke the immune response to release complements responsible for hypersensitivity reactions. Created with BioRender.com.
Although involvement of NPs in human clinical settings increased a decade ago, extensive research to improve biocompatibility and efficacy of NPs is still needed. Despite several challenges that need to be addressed in the application of NPs as a nanomedicine, its advantages outweigh those challenges, making NPs a highly potential tool (Table 2).
Despite the abundance of encouraging experimental data on NPs for medical purposes, only a few reach clinical use. This statement is supported by Greish et al., who explored more than 20,000 scientific papers published on nanomedicine, and found of these, only 15 nanoparticle-based anti-cancer drugs had reached the market as of 2017. It is clear that the number of publications claiming to have found new, effective, and safe anticancer formulations, compared to the number of compounds that actually reached the clinic, is remarkably small (Greish et al., 2018). In their review, Greish et al. discuss different biological aspects that hinder the clinical progression of cancer nanomedicine, which include misconception of EPR phenomenon, overlooking the acquired pharmacokinetics and clearance of nanomedicine through reticuloendothelial system, and accelerated blood clearance. The limitations of animal models and heterogeneity of human tumors further restricted the clinical application of formulated nanomedicines.
Safety is the most important aspect in the development of new drugs. Although the size of nanoparticles represents their strength, for some nanomedicines it has also brought some shortcomings. The small size of NPs cause some of these particles to accumulate in the spleen and liver, which is a major safety concern in patients (Resnik and Tinkle, 2007). In some cases, the injected doses of nanosized molecules are cleared by reticuloendothelial system cells with a minimal percentage of the drug dose reaching the tumors which lowers the efficacy of the treatment.
Even when some studies reach clinical validation, logistics issues including mass production, consistency, and reproducibility of complex nanomedicine systems are the main hurdles. Furthermore, the controlled and scale-up manufacture of each component, batch-to-batch reproducibility, and stability of designed nanomedicines are essential for approval by the regulatory authorities (Greish et al., 2018). Not only that, regulation and standards for nanomedicine by regulatory bodies are severely lacking and could be geographically differed as one nanomedicine is approved in one country but not in others (Zhang et al., 2020). Due to these challenges (Figure 3), hundreds of nanomedicine formulations have failed in different phases of clinical trials, or even worse, some are withdrawn from the market even after its approval.
To overcome these issues, several solutions can be proposed. In order to address the biological challenges of nanomedicine in cancer that is a heterogenous disease, thorough designation of nanomedicine and identification of the right animal models and patients in preclinical investigations should be in mind when designing a new drug entity. Good laboratory practice (GLP) is a standard to ensure the safety and quality of new therapeutics during clinical transition by many countries. However, GLP for nanomedicine has not been made available yet, hence it is imperative to formulate GLP for nanomedicine to enhance its success rate in the market (Zhang et al., 2020). In addressing logistic issues, careful examination of the cost-benefit analysis should be done during the early stage of nanomedicine development.
Despite challenges, the latest technologies and advantages of nanoparticles continue to encourage research communities to develop new, better nanomedicines. It is recognized as a proven strategy to alleviate the side effects of cancer therapies and enhance their efficacies. Nevertheless, development of nanomedicine should always accentuate their interactions with host immune responses, as in cancer, it’s tangibly interlinked between one another. Although there are several aversions to nanomedicine due to the induction of unwanted hypersensitivity, available findings suggested that targeted approach of nanomedicine provides a favorable effect in the immune system, from its immunogenicity and interaction in the TME to its tolerability. With this understanding of the interaction of nanomedicine with the immune system, the future of nanomedicine is promising as long as the shift to improve the clinical impact of nanomedicine moves alongside it.
SA, RI, WW, and KP wrote this paper. RM, JB, MP, JJ, and JL supervised this work and revised the manuscript. RM acquired funding for this work. All authors contributed to the paper and approved the submitted version.
This work was supported by Universiti Sains Malaysia Research University Grant (Grant Number: 1001/PPSP/8012294).
The authors declare that the research was conducted in the absence of any commercial or financial relationships that could be construed as a potential conflict of interest.
Alagkiozidis, I., Facciabene, A., Carpenito, C., Benencia, F., Jonak, Z., Adams, S., et al. (2009). Increased Immunogenicity of Surviving Tumor Cells Enables Cooperation between Liposomal Doxorubicin and IL-18. J. Transl. Med. 7 (1), 104. doi:10.1186/1479-5876-7-104
Aly, H. A. A. (2012). Cancer Therapy and Vaccination. J. Immunol. Methods 382 (1-2), 1–23. doi:10.1016/j.jim.2012.05.014
Anderson, P. M., Tomaras, M., and McConnell, K. (2010). Mifamurtide in Osteosarcoma - A Practical Review. Drugs Today 46 (5), 327. doi:10.1358/dot.2010.46.5.1500076
Ando, K., Mori, K., Corradini, N., Redini, F., and Heymann, D. (2011). Mifamurtide for the Treatment of Nonmetastatic Osteosarcoma. Expert Opin. Pharmacother. 12 (2), 285–292. doi:10.1517/14656566.2011.543129
Barenholz, Y. (2012). Doxil - the First FDA-Approved Nano-Drug: Lessons Learned. J. Control. Release 160 (2), 117–134. doi:10.1016/j.jconrel.2012.03.020
Batist, G., Barton, J., Chaikin, P., Swenson, C., and Welles, L. (2005). Myocet (Liposome-encapsulated Doxorubicin Citrate): a New Approach in Breast Cancer Therapy. Expert Opin. Pharmacother. 3 (12), 1739–1751. doi:10.1517/14656566.3.12.1739
Bawa, R. (2011). Regulating Nanomedicine - Can the FDA Handle it? Cdd 8 (3), 227–234. doi:10.2174/156720111795256156
Bellini, G., Pinto, D. D., Tortora, C., Manzo, I., Punzo, F., Casale, F., et al. (2017). The Role of Mifamurtide in Chemotherapy-Induced Osteoporosis of Children with Osteosarcoma. Ccdt 17 (7), 650–656. doi:10.2174/1568009616666161215163426
Bhaskar, S., Tian, F., Stoeger, T., Kreyling, W., de la Fuente, J. M., Grazú, V., et al. (2010). Multifunctional Nanocarriers for Diagnostics, Drug Delivery and Targeted Treatment across Blood-Brain Barrier: Perspectives on Tracking and Neuroimaging. Part. Fibre Toxicol. 7, 3. doi:10.1186/1743-8977-7-3
Bobo, D., Robinson, K. J., Islam, J., Thurecht, K. J., and Corrie, S. R. (2016). Nanoparticle-Based Medicines: A Review of FDA-Approved Materials and Clinical Trials to Date. Pharm. Res. 33 (10), 2373–2387. doi:10.1007/s11095-016-1958-5
Brandenburg, G., Poppenborg, S., Radcke, C., Wittmann, J., Krähmer, R., Pasut, G., et al. (2020). “Challenges in the Analytical Characterization of PEGylated Asparaginase,” in Polymer-Protein Conjugates: From Pegylation and Beyonds. Editors G. Pasut, and S. Zalipsky, 205–231. doi:10.1016/b978-0-444-64081-9.00010-3
Bremer-Hoffmann, S., Halamoda-Kenzaoui, B., and Borgos, S. E. (2018). Identification of Regulatory Needs for Nanomedicines. J. Interdiscip. Nanomedicine 3 (1), 4–15. doi:10.1002/jin2.34
Chanan-Khan, A., Szebeni, J., Savay, S., Liebes, L., Rafique, N. M., Alving, C. R., et al. (2003). Complement Activation Following First Exposure to Pegylated Liposomal Doxorubicin (Doxil): Possible Role in Hypersensitivity Reactions. Ann. Oncol. 14 (9), 1430–1437. doi:10.1093/annonc/mdg374
Chauhan, V. P., Stylianopoulos, T., Martin, J. D., Popović, Z., Chen, O., Kamoun, W. S., et al. (2012). Normalization of Tumour Blood Vessels Improves the Delivery of Nanomedicines in a Size-dependent Manner. Nat. Nanotech. 7 (6), 383–388. doi:10.1038/nnano.2012.45
Copier, J., and Dalgleish, A. (2013). Tumour Immunology. Chichester: John Wiley & Sons, Ltd. doi:10.1002/9780470015902.a0001429.pub2
Cullis, J., Siolas, D., Avanzi, A., Barui, S., Maitra, A., and Bar-Sagi, D. (2017). Macropinocytosis of Nab-Paclitaxel Drives Macrophage Activation in Pancreatic Cancer. Cancer Immunol. Res. 5 (3), 182–190. doi:10.1158/2326-6066.cir-16-0125
Deitcher, O. R., Glaspy, J., Gonzalez, R., Sato, T., Bedikian, A. Y., Segarini, K., et al. (2014). High-dose Vincristine Sulfate Liposome Injection (Marqibo) Is Not Associated with Clinically Meaningful Hematologic Toxicity. Clin. Lymphoma Myeloma Leuk. 14 (3), 197–202. doi:10.1016/j.clml.2013.10.012
DiMasi, J. A., Hansen, R. W., and Grabowski, H. G. (2003). The price of Innovation: New Estimates of Drug Development Costs. J. Health Econ. 22 (2), 151–185. doi:10.1016/s0167-6296(02)00126-1
D'Mello, S. R., Cruz, C. N., Chen, M.-L., Kapoor, M., Lee, S. L., and Tyner, K. M. (2017). The Evolving Landscape of Drug Products Containing Nanomaterials in the United States. Nat. Nanotech. 12 (6), 523–529. doi:10.1038/nnano.2017.67
Dunn, G. P., Old, L. J., and Schreiber, R. D. (2004). The Three Es of Cancer Immunoediting. Annu. Rev. Immunol. 22 (1), 329–360. doi:10.1146/annurev.immunol.22.012703.104803
Eifler, A. C., and Thaxton, C. S. (2011). Nanoparticle Therapeutics: FDA Approval, Clinical Trials, Regulatory Pathways, and Case Study. Clin. Trials, Regul. Pathways, Case Study 726, 325–338. doi:10.1007/978-1-61779-052-2_21
Falzone, L., Salomone, S., and Libra, M. (2018). Evolution of Cancer Pharmacological Treatments at the Turn of the Third Millennium. Front. Pharmacol. 9, 1300. doi:10.3389/fphar.2018.01300
Fassas, A., and Anagnostopoulos, A. (2009). The Use of Liposomal Daunorubicin (DaunoXome) in Acute Myeloid Leukemia. Leuk. Lymphoma 46 (6), 795–802. doi:10.1080/10428190500052438
Foss, F. (2006). Clinical Experience with Denileukin Diftitox (ONTAK). Semin. Oncol. 33, 11–16. doi:10.1053/j.seminoncol.2005.12.017
Fu, Q., Sun, J., Zhang, W., Sui, X., Yan, Z., and He, Z. (2009). Nanoparticle Albumin - Bound (NAB) Technology Is a Promising Method for Anti-cancer Drug Delivery. Pra 4 (3), 262–272. doi:10.2174/157489209789206869
Galvin, P., Thompson, D., Ryan, K. B., McCarthy, A., Moore, A. C., Burke, C. S., et al. (2012). Nanoparticle-based Drug Delivery: Case Studies for Cancer and Cardiovascular Applications. Cell. Mol. Life Sci. 69, 389–404. doi:10.1007/s00018-011-0856-6
Gao, S., Yang, D., Fang, Y., Lin, X., Jin, X., Wang, Q., et al. (2019). Engineering Nanoparticles for Targeted Remodeling of the Tumor Microenvironment to Improve Cancer Immunotherapy. Theranostics 9 (1), 126–151. doi:10.7150/thno.29431
Gradishar, W. J., Tjulandin, S., Davidson, N., Shaw, H., Desai, N., Bhar, P., et al. (2005). Phase III Trial of Nanoparticle Albumin-Bound Paclitaxel Compared with Polyethylated Castor Oil-Based Paclitaxel in Women with Breast Cancer. Jco 23 (31), 7794–7803. doi:10.1200/jco.2005.04.937
Greish, K., Mathur, A., Bakhiet, M., and Taurin, S. (2018). Nanomedicine: Is it Lost in Translation? Ther. Deliv. 9 (4), 269–285. doi:10.4155/tde-2017-0118
Guo, X., Wang, L., Wei, X., and Zhou, S. (2016). Polymer-based Drug Delivery Systems for Cancer Treatment. J. Polym. Sci. Part. A: Polym. Chem. 54 (22), 3525–3550. doi:10.1002/pola.28252
Hartmann, M., Mayer-Nicolai, C., and Pfaff, O. (2013). Approval Probabilities and Regulatory Review Patterns for Anticancer Drugs in the European Union. Crit. Rev. Oncol. Hematol. 87 (2), 112–121. doi:10.1016/j.critrevonc.2013.01.004
Havel, H., Finch, G., Strode, P., Wolfgang, M., Zale, S., Bobe, I., et al. (2016). Nanomedicines: From Bench to Bedside and beyond. Aaps J. 18 (6), 1373–1378. doi:10.1208/s12248-016-9961-7
Heo, Y.-A., Syed, Y. Y., and Keam, S. J. (2019). Pegaspargase: A Review in Acute Lymphoblastic Leukaemia. Drugs 79 (7), 767–777. doi:10.1007/s40265-019-01120-1
Huang, K.-G., Moreira-Barros, J., and Tsai, T.-H. (2018). Pegylated Liposomal Doxorubicin-Induced Palmar-Plantar Erythrodysesthesia. Gynecol. Minim. Invasive Ther. 7 (1), 44. doi:10.4103/gmit.gmit_8_17
Jimmy, R., Stern, C., Lisy, K., and White, S. (2017). Effectiveness of Mifamurtide in Addition to Standard Chemotherapy for High-Grade Osteosarcoma: a Systematic Review. JBI Database Syst. Rev. Implementation Rep. 15 (8), 2113–2152. doi:10.11124/jbisrir-2016-003105
Kim, T.-Y., Kim, D.-W., Chung, J.-Y., Shin, S. G., Kim, S.-C., Heo, D. S., et al. (2004). Phase I and Pharmacokinetic Study of Genexol-PM, a Cremophor-free, Polymeric Micelle-Formulated Paclitaxel, in Patients with Advanced Malignancies. Clin. Cancer Res. 10 (11), 3708–3716. doi:10.1158/1078-0432.ccr-03-0655
Krauss, A. C., Gao, X., Li, L., Manning, M. L., Patel, P., Fu, W., et al. (2019). FDA Approval Summary: (Daunorubicin and Cytarabine) Liposome for Injection for the Treatment of Adults with High-Risk Acute Myeloid Leukemia. Clin. Cancer Res. 25 (9), 2685–2690. doi:10.1158/1078-0432.ccr-18-2990
Li, W., Zhao, X., Du, B., Li, X., Liu, S., Yang, X.-Y., et al. (2016). Gold Nanoparticle-Mediated Targeted Delivery of Recombinant Human Endostatin Normalizes Tumour Vasculature and Improves Cancer Therapy. Sci. Rep. 6 (1), 30619. doi:10.1038/srep30619
Li, W., Li, X., Liu, S., Yang, W., Pan, F., Yang, X.-Y., et al. (2017). Gold Nanoparticles Attenuate Metastasis by Tumor Vasculature Normalization and Epithelial–Mesenchymal Transition Inhibition. Int. J. Nanomedicine 12, 3509–3520. doi:10.2147/ijn.s128802
Lipsky, M. S., and Sharp, L. K. (2001). From Idea to Market: The Drug Approval Process. J. Am. Board Fam. Pract. 14 (5), 362–367.
Lutz, M. B., Baur, A. S., Schuler-Thurner, B., and Schuler, G. (2014). Immunogenic and Tolerogenic Effects of the Chimeric IL-2-diphtheria Toxin Cytocidal Agent Ontakon CD25+cells. OncoImmunology 3 (3), e28223. doi:10.4161/onci.28223
Maeda, H. (2001). SMANCS and Polymer-Conjugated Macromolecular Drugs: Advantages in Cancer Chemotherapy. Adv. Drug Deliv. Rev. 46 (1-3), 169–185. doi:10.1016/s0169-409x(00)00134-4
Maeda, H. (2012). Macromolecular Therapeutics in Cancer Treatment: The EPR Effect and beyond. J. Controlled Release 164 (2), 138–144. doi:10.1016/j.jconrel.2012.04.038
Maeda, H. (2017). Polymer Therapeutics and the EPR Effect. J. Drug Target. 25 (9-10), 781–785. doi:10.1080/1061186x.2017.1365878
Mak, T. W., M. E. Saunders, and B. D. Jett (2014). “Tumor Immunology,” in Primer to the Immune Response. Second ed (Academic Cell), 423–455.
Martinelli, C., Pucci, C., and Ciofani, G. (2019). Nanostructured Carriers as Innovative Tools for Cancer Diagnosis and Therapy. APL Bioeng. 3 (1), 011502. doi:10.1063/1.5079943
Massadeh, S., and Al Aamery, M. (2016). Nano-materials for Gene Therapy: An Efficient Way in Overcoming Challenges of Gene Delivery. J. Biosens. Bioelectron. 07 (01). doi:10.4172/2155-6210.1000195
Muenst, S., Läubli, H., Soysal, S. D., Zippelius, A., Tzankov, A., and Hoeller, S. (2016). The Immune System and Cancer Evasion Strategies: Therapeutic Concepts. J. Intern. Med. 279 (6), 541–562. doi:10.1111/joim.12470
Munhoz, R. R., and Postow, M. A. (2016). Recent Advances in Understanding Antitumor Immunity. F1000Res 5, 2545. doi:10.12688/f1000research.9356.1
Neun, B., Barenholz, Y., Szebeni, J., and Dobrovolskaia, M. (2018). Understanding the Role of Anti-PEG Antibodies in the Complement Activation by Doxil In Vitro. Molecules 23 (7), 1700. doi:10.3390/molecules23071700
Ni, C., Fang, J., Qian, H., Xu, Q., and Shen, F. (2020). Liposomal Doxorubicin-Related Palmar-Plantar Erythrodysesthesia (Hand-foot Syndrome): a Case Report. J. Int. Med. Res. 48 (12), 030006052097485. doi:10.1177/0300060520974854
O’Brien, M. E. R., Wigler, N., Inbar, M., Rosso, R., Grischke, E., Santoro, A., et al. (2004). Reduced Cardiotoxicity and Comparable Efficacy in a Phase III trial of Pegylated Liposomal Doxorubicin HCl(CAELYX™/Doxil®) versus Conventional Doxorubicin Forfirst-Line Treatment of Metastatic Breast Cancer. Ann. Oncol. 15 (3), 440–449. doi:10.1093/annonc/mdh097
Ostrand-Rosenberg, S. (2008). Immune Surveillance: a Balance between Protumor and Antitumor Immunity. Curr. Opin. Genet. Dev. 18 (1), 11–18. doi:10.1016/j.gde.2007.12.007
Panagi, M., Voutouri, C., Mpekris, F., Papageorgis, P., Martin, M. R., Martin, J. D., et al. (2020). TGF-β Inhibition Combined with Cytotoxic Nanomedicine Normalizes Triple Negative Breast Cancer Microenvironment towards Anti-tumor Immunity. Theranostics 10 (4), 1910–1922. doi:10.7150/thno.36936
Parisi, A., Palluzzi, E., Cortellini, A., Sidoni, T., Cocciolone, V., Lanfiuti Baldi, P., et al. (2019). First-line Carboplatin/nab-Paclitaxel in Advanced Ovarian Cancer Patients, after Hypersensitivity Reaction to Solvent-Based Taxanes: a Single-Institution Experience. Clin. Transl. Oncol. 22 (1), 158–162. doi:10.1007/s12094-019-02122-x
Patra, J. K., Das, G., Fraceto, L. F., Campos, E. V. R., Rodriguez-Torres, M. d. P., Acosta-Torres, L. S., et al. (2018). Nano Based Drug Delivery Systems: Recent Developments and Future Prospects. J. Nanobiotechnol. 16 (1), 71. doi:10.1186/s12951-018-0392-8
Pellegrino, B., Boggiani, D., Tommasi, C., Palli, D., and Musolino, A. (2017). Nab-paclitaxel after Docetaxel Hypersensitivity Reaction: Case Report and Literature Review. Acta Biomed. 88 (3), 329–333. doi:10.23750/abm.v88i3.6138
Pillai, G., and Ceballos-Coronel, M. L. (2013). Science and Technology of the Emerging Nanomedicines in Cancer Therapy: A Primer for Physicians and Pharmacists. SAGE Open Med. 1, 205031211351375. doi:10.1177/2050312113513759
Pillai, G. (2014). Nanomedicines for Cancer Therapy: An Update of FDA Approved and Those under Various Stages of Development. SOJ Pharmacy & Pharmaceutical Sciences. doi:10.15226/2374-6866/1/2/00109
Poirot-Mazères, I. (2011). Chapitre 6. Legal Aspects of the Risks Raised by Nanotechnologies in the Field of Medicine. J. Int. Biotethique 22 (1), 99–118. doi:10.3917/jib.221.0099
Punzo, F., Bellini, G., Tortora, C., Pinto, D. D., Argenziano, M., Pota, E., et al. (2020). Mifamurtide and TAM-like Macrophages: Effect on Proliferation, Migration and Differentiation of Osteosarcoma Cells. Oncotarget 11 (7), 687–698. doi:10.18632/oncotarget.27479
Reichert, J. M. (2003). Trends in Development and Approval Times for New Therapeutics in the United States. Nat. Rev. Drug Discov. 2 (9), 695–702. doi:10.1038/nrd1178
Resnik, D. B., and Tinkle, S. S. (2007). Ethical Issues in Clinical Trials Involving Nanomedicine. Contemp. Clin. Trials 28 (4), 433–441. doi:10.1016/j.cct.2006.11.001
Rios-Doria, J., Durham, N., Wetzel, L., Rothstein, R., Chesebrough, J., Holoweckyj, N., et al. (2015). Doxil Synergizes with Cancer Immunotherapies to Enhance Antitumor Responses in Syngeneic Mouse Models. Neoplasia 17 (8), 661–670. doi:10.1016/j.neo.2015.08.004
Sajja, H., East, M., Mao, H., Wang, Y., Nie, S., and Yang, L. (2009). Development of Multifunctional Nanoparticles for Targeted Drug Delivery and Noninvasive Imaging of Therapeutic Effect. Cddt 6 (1), 43–51. doi:10.2174/157016309787581066
Sartor, O. (2003). Eligard: Leuprolide Acetate in a Novel Sustained-Release Delivery System. Urology 61 (2), 25–31. doi:10.1016/s0090-4295(02)02396-8
Seigneuric, R., Markey, L., S.A. Nuyten, D., Dubernet, C., T.A. Evelo, C., Finot, E., et al. (2010). From Nanotechnology to Nanomedicine: Applications to Cancer Research. Cmm 10 (7), 640–652. doi:10.2174/156652410792630634
Shafiee, F., Aucoin, M. G., and Jahanian-Najafabadi, A. (2019). Targeted Diphtheria Toxin-Based Therapy: A Review Article. Front. Microbiol. 10. doi:10.3389/fmicb.2019.02340
Şimşek, M., Ataş, E., Bağrıaçık, E. Ü., Günal, A., and Ünay, B. (2020). Type 4 Hypersensitivity Development in a Case Due to Mifamurtide. Turk J. Pediatr. 62 (4), 694–699. doi:10.24953/turkjped.2020.04.025
Swann, J. B., and Smyth, M. J. (2007). Immune Surveillance of Tumors. J. Clin. Invest. 117 (5), 1137–1146. doi:10.1172/jci31405
Tadmor, T. (2019). Time to Understand More about Spontaneous Regression of Cancer. Acta Haematol. 141 (3), 156–157. doi:10.1159/000496680
Tejada-Berges, T., Granai, C., Gordinier, M., and Gajewski, W. (2014). Caelyx/Doxil for the Treatment of Metastatic Ovarian and Breast Cancer. Expert Rev. Anticancer Ther. 2 (2), 143–150. doi:10.1586/14737140.2.2.143
Teng, M. W. L., Swann, J. B., Koebel, C. M., Schreiber, R. D., and Smyth, M. J. (2008). Immune-mediated Dormancy: an Equilibrium with Cancer. J. Leukoc. Biol. 84 (4), 988–993. doi:10.1189/jlb.1107774
Thomas, R., and Shillingburg, A. (2015). Drug-induced Immune Hemolytic Anemia Associated with Albumin-Bound Paclitaxel. J. Community Support. Oncol. 13 (8), 298–299. doi:10.12788/jcso.0160
Tinkle, S., McNeil, S. E., Mühlebach, S., Bawa, R., Borchard, G., Barenholz, Y. C., et al. (2014). Nanomedicines: Addressing the Scientific and Regulatory gap. Ann. N.Y. Acad. Sci. 1313 (1), 35–56. doi:10.1111/nyas.12403
Tzogani, K., Penttilä, K., Lapveteläinen, T., Hemmings, R., Koenig, J., Freire, J., et al. (2020). EMA Review of Daunorubicin and Cytarabine Encapsulated in Liposomes (Vyxeos, CPX ‐351) for the Treatment of Adults with Newly Diagnosed, Therapy‐Related Acute Myeloid Leukemia or Acute Myeloid Leukemia with Myelodysplasia‐Related Changes. Oncol. 25 (9). doi:10.1634/theoncologist.2019-0785
Ventola, C. L. (2017). Progress in Nanomedicine: Approved and Investigational Nanodrugs. P & T 42 (12), 742–755.
Wenbo, L., and Wang, J. (2017). Uncovering the Underlying Mechanism of Cancer Tumorigenesis and Development under an Immune Microenvironment from Global Quantification of the Landscape. J. R. Soc. Interf. 14 (131), 20170105. doi:10.1098/rsif.2017.0105
Xiao, W., Ruan, S., Yu, W., Wang, R., Hu, C., Liu, R., et al. (2017). Normalizing Tumor Vessels to Increase the Enzyme-Induced Retention and Targeting of Gold Nanoparticle for Breast Cancer Imaging and Treatment. Mol. Pharmaceutics 14 (10), 3489–3498. doi:10.1021/acs.molpharmaceut.7b00475
Yuan, H., Guo, H., Luan, X., He, M., Li, F., Burnett, J., et al. (2020). Albumin Nanoparticle of Paclitaxel (Abraxane) Decreases while Taxol Increases Breast Cancer Stem Cells in Treatment of Triple Negative Breast Cancer. Mol. Pharmaceutics 17 (7), 2275–2286. doi:10.1021/acs.molpharmaceut.9b01221
Zarour, H. M., DeLeo, A., Finn, O. J., and Storkus, W. J. (2003). “Categories of Tumor Antigens,” in Holland-frei Cancer Medicine. Editors K. DW, P. RE, W. RR, and et. al. Sixth ed. (Memphis: BC Decker).
Zhang, C., Yan, L., Wang, X., Zhu, S., Chen, C., Gu, Z., et al. (2020). Progress, Challenges, and Future of Nanomedicine. Nano Today 35, 101008. doi:10.1016/j.nantod.2020.101008
Zhang, H. (2016). Onivyde for the Therapy of Multiple Solid Tumors. Ott 9, 3001. doi:10.2147/ott.s105587
Zheng, J., and Gao, P. (2019). Toward Normalization of the Tumor Microenvironment for Cancer Therapy. Integr. Cancer Ther. 18, 153473541986235. doi:10.1177/1534735419862352
Zhou, J., Wang, G., Chen, Y., Wang, H., Hua, Y., and Cai, Z. (2019). Immunogenic Cell Death in Cancer Therapy: Present and Emerging Inducers. J. Cel. Mol. Med. 23 (8), 4854–4865. doi:10.1111/jcmm.14356
Keywords: tumor micoenvironment, immunogenicity, hypersensitivity, cytotoxicity, drug development
Citation: Ahmad S, Idris RAM, Wan Hanaffi WN, Perumal K, Boer JC, Plebanski M, Jaafar J, Lim JK and Mohamud R (2021) Cancer Nanomedicine and Immune System—Interactions and Challenges. Front. Nanotechnol. 3:681305. doi: 10.3389/fnano.2021.681305
Received: 16 March 2021; Accepted: 25 May 2021;
Published: 09 July 2021.
Edited by:
Zhi Ping (Gordon) Xu, The University of Queensland, AustraliaReviewed by:
Bapu Surnar, University of Miami Hospital, United StatesCopyright © 2021 Ahmad, Idris, Wan Hanaffi, Perumal, Boer, Plebanski, Jaafar, Lim and Mohamud. This is an open-access article distributed under the terms of the Creative Commons Attribution License (CC BY). The use, distribution or reproduction in other forums is permitted, provided the original author(s) and the copyright owner(s) are credited and that the original publication in this journal is cited, in accordance with accepted academic practice. No use, distribution or reproduction is permitted which does not comply with these terms.
*Correspondence: Rohimah Mohamud, cm9oaW1haG1AdXNtLm15
Disclaimer: All claims expressed in this article are solely those of the authors and do not necessarily represent those of their affiliated organizations, or those of the publisher, the editors and the reviewers. Any product that may be evaluated in this article or claim that may be made by its manufacturer is not guaranteed or endorsed by the publisher.
Research integrity at Frontiers
Learn more about the work of our research integrity team to safeguard the quality of each article we publish.