- Department of Materials Science and Engineering, Department of Chemistry, University of North Texas, Denton, TX, United States
Living organisms have evolved, over billions of years, to develop specialized biostructures with switchable adhesion for various purposes including climbing, perching, preying, sensing, and protecting. According to adhesion mechanisms, switchable adhesives can be divided into four categories: mechanically-based adhesion, liquid-mediated adhesion, physically-actuated adhesion and chemically-enhanced adhesion. Mimicking these biostructures could create smart materials with switchable adhesion, appealing for many engineering applications in robotics, sensors, advanced drug-delivery, protein separation, etc. Progress has been made in developing bioinspired materials with switchable adhesion modulated by external stimuli such as electrical signal, magnetic field, light, temperature, pH value, etc. This review will be focused on new advance in biomimetic design and synthesis of the materials and devices with switchable adhesion. The underlying mechanisms, design principles, and future directions are discussed for the development of high-performance smart surfaces with switchable adhesion.
Introduction
Natural species on earth have evolved over billions of years, to develop numerous abilities to survive in different environments. One of these fascinating abilities is switchable adhesion, which is critical to many species from animals to insects for their locomotion and predation. For example, geckos can stick and walk on almost any surfaces no matter how rough or slippery, dry or wet they are (West, 1861; Dewitz, 1884; Brainerd, 1994; Federle et al., 1997). While the attachment can reliably hold as much as 100 times of their own weights (Federle et al., 2000), their fast movement proves no hassle in detachment, which shows that the adhesion is highly switchable and robust. This adhesion switching ability stems from their unique footpad structures: microscale setae with nanoscale spatulae brunches. Mimicking these natural switchable surfaces could result in novel smart surfaces for a wide variety of applications by adequately designing their structures, such as microfluidic and biological engineering (Stratakis et al., 2011; Montero de Espinosa et al., 2017). These biomimetic design principles may open new doors for bioinspired smart materials with unique switchable capabilities.
Though many species show similar behaviors as switchable adhesion, there are diverse switching mechanisms. Some predators use hooks or anchors to lock themselves on host tissues through mechanical forces (Fenner, 2000; Gorb, 2001, 2008), while some insects and frogs, however, take advantage of water to enhance the adhesion via liquid-induced adhesion (Vötsch et al., 2002; Betz and Kölsch, 2004; Dirks and Federle, 2011). Another kind of adhesion is based on special chemical bonds, such as mussel glue (Waite, 2002; Waite et al., 2005). Inspired by the unique structures and extraordinary abilities of live organisms, various artificial structures with switchable adhesion have been made for different applications (Yoon, 2019; Stratakis et al., 2020). In general, according to different mechanisms, biomimetic switchable adhesives can be divided into four categories: (1) mechanically-based adhesion, (2) liquid-mediated adhesion, (3) physically-actuated adhesion, and (4) chemically-enhanced adhesion and summarized in Table 1. Combining these mechanisms with suitable materials, the researchers have invented “smart surfaces” with tunable or reversible adhesion with single/multiple external stimuli, such as force, electrical bias, pH, temperature, light, and so on. In this review, we overview the milestone work in the biomimetic design of switchable adhesives. In Section Biomimetic Structures with Mechanically-Based Switchable Adhesion, the origin and basic biomimetic principles of mechanically-based switchable adhesion are discussed for biomimetic design. The third section is focused on biomimetic structures with liquid-assisted switchable adhesion, followed by Section Physically-Actuated Switchable Adhesion by Controlling Surface Configuration Through External Stimuli controlling surface configuration through external stimuli, and Section Biomimetic Structures With Chemically-Mediated Switchable Adhesion describing biomimetic design with chemically-based switchable adhesion. Finally, the future direction is discussed in the biomimetic design and fabrication of smart structures with switchable adhesion for technological applications.
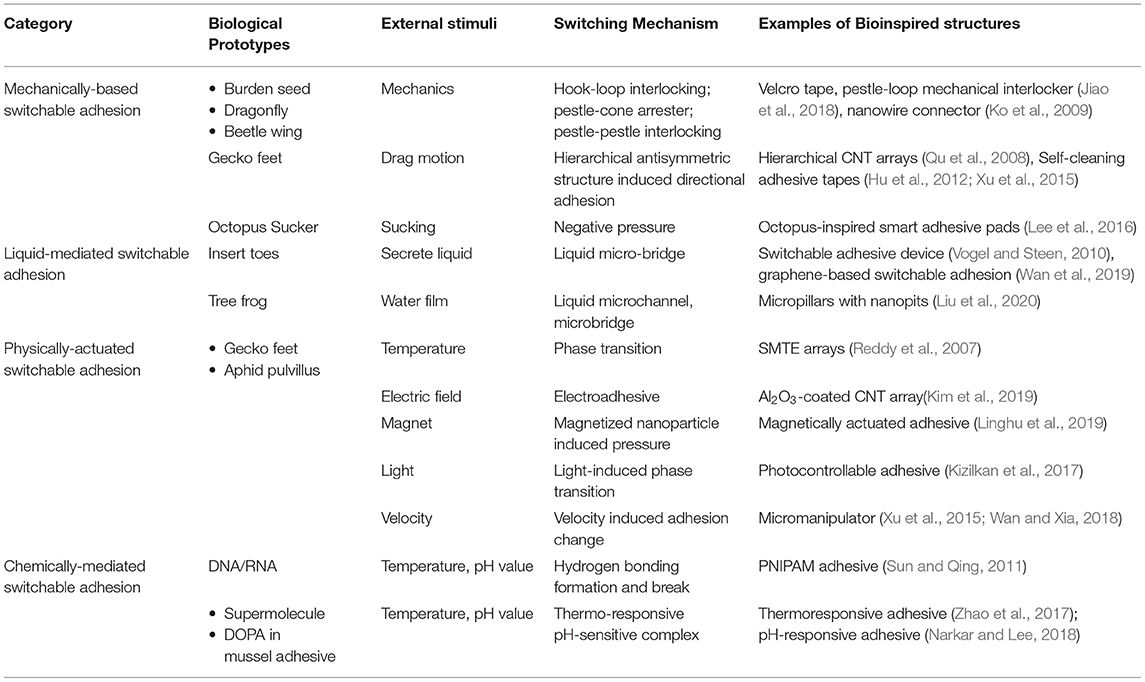
Table 1. Biological prototypes, mechanisms, structure, and examples of externally stimulated adhesives.
Biomimetic Structures With Mechanically-Based Switchable Adhesion
Burden Hooks and Insect Interlocks
Nature has created unique biological structures with specialized functions and provided the inspiration for numerous applications with well-designed structures. One fine design by nature is the “hooks-loops” interlocking in burdock's seeds. Seed dispersal is the process that plants transfer their seeds to further places. Some plants seek the help of animal vectors by attaching seeds or fruits to animal fur (Von Marilaun, 1895; Willson et al., 1990). These plants, such as Burdock (Sorensen, 1986) and Bidens (Bullock and Primack, 1977) have hook or barb structures growing on their seeds as shown in Figure 1A. The hooks and barbs can mechanically interlock with animal hairs or fibers on human clothes to form strong adhesion. Interestingly, some insects such as Hymenoptera, Heteroptera, Coleoptera (Samuelson, 1994, 1996) have similar interlock mechanism on their wings and bodies, shown in Figures 1C,E. When these insects rest their wings, the counterparts in different directions or shapes growing separately on wings and bodies can lock to each other, preventing shifting and shearing, so that the whole body could be stable (Figure 1E) (Gorb, 1999; Gorb et al., 2002).
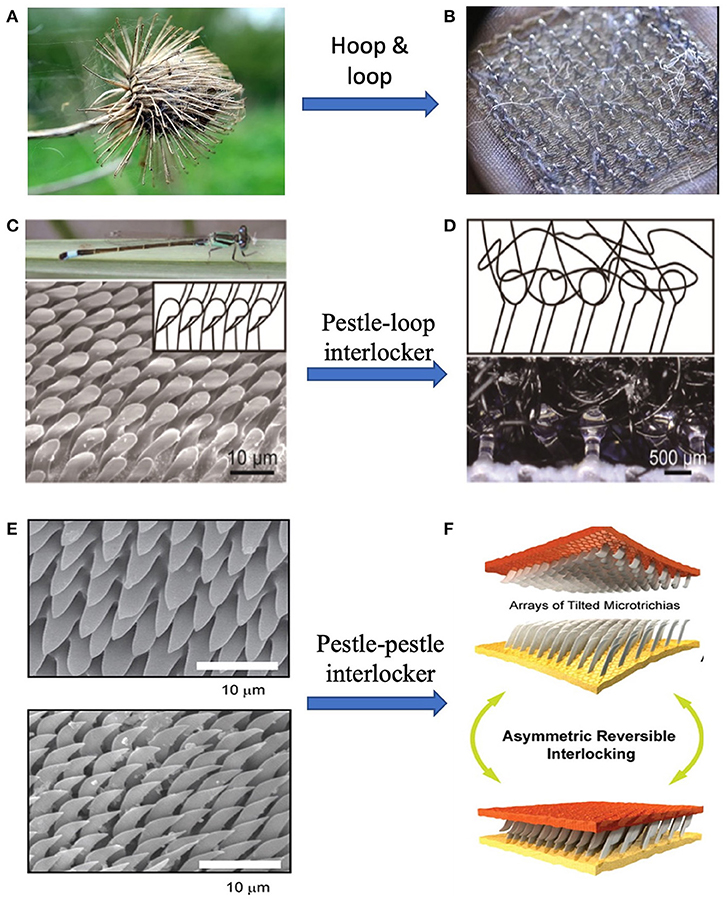
Figure 1. Velcro tapes inspired by cocklebur. (A) Cocklebur seeds, (B) Velcro tapes, and (C) Optical images of dragonfly, and pestle-shaped cilia of arrester system (Zygoptera, Ischnura elegans). (D) Schematic of mechanical interlocker (upper) and optical image of pestle-loop mechanical interlocker (lower). (E) Scanning electron microscopy (SEM) images of thoracic (upper) and elytral (lower) counterparts of the elytra-to-body locking device in the tenebrionid beetle Tenebrio molitor. (F) Illustration of unfolding (upper) and folding (lower) states of wing-locking device. [(C,D) Reproduced with permission from Jiao et al. (2018). Copyright (2021), Advanced Science. (E,F) Reproduced from Pang et al. (2012). Copyright (2021), with permission from American Chemical Society].
Inspired by these biological switchable adhesion structures, many locking devices have been developed. One of the most famous inventions from the inspiration of the mechanical interlock of Burdock is Velcro tape (Figure 1B), a fastener (De Mestral, 1955, 1961). In the 1940s a Swiss engineer, George de Mestral, noticed the tiny hocks on the seeds of the Alpine plant, which firmly attached dog fur. This unique biological structure inspired him to invent the nylon-based fastener that is now commonly used in daily life. The stiff hooks are made on one part of Velcro tape and another part is covered by soft “hairs.” When pressing two parts together, many hook-loop pairs from interlocks and the total adhesion can be very strong, while the attaching force is also reversible. Other dry adhesives based on buckling and interlocking were also reported by different groups (Chen et al., 2013; Andrews and Badyal, 2014). Even hydrogel adhesives could take advantage of reversible interlocking, where configurable microhook arrays is tuned by contacting with water (Park et al., 2017; Yi et al., 2018).
While being convenient in use, the Velcro may bother users by the noise due to its deformation, break and separating. Efforts have made to develop new types of mechanical interlocker with high quality. One example is inspired by the pestle-cone interlocker of the dragonfly (Figures 1C,D). It is discovered that the pestle-cone arrester system is able to effectively protect the “slim neck” although experiencing constantly mechanical interlock and unlock. Jiao et al. (2018) presented a pestle-loop mechanical interlocker with tunable peeling force (Figure 1F). Results suggest that the pestle is more durable than the hook and the mushroom by comparing the shape and detaching force before and after 13 attachment-detachment cycles. The pestle-loop mechanical interlocker achieves switchable peeling force from 0.4 to 6.5 N by changing the angle between vertical and loop.
With observation on the existence of mechanical interlocking in beetles, researchers have explored the mechanism of reversible adhesion system and tried to mimic the natural structures. Gorb and Popov (2002) found that the wing of a beetle is fixed by making numerous microhairs on the cuticular surface into contact to generate high lateral shear friction while minimizing the force for vertical lift-off, providing reversible attachment and detachment system. Unlike other reversible binding systems, for example, hooks or loops in Velcro, the interlocking mechanism of the wing-locking device does not require any complicated structures for physical grasping (Pang et al., 2011a).
Inspired by the wing-fixation system in the beetle, a variety of artificial arrays have been developed by different methods. Pang and co-workers (Pang et al., 2011a,b) developed a preload-dependent reversible mechanical interlocking between regularly arrayed, high-aspect-ratio polymer fiber arrays. They found that there was a linear tendency of locking force and preload, which could be explained by the increase of overlap ratio with increasing the preload. The interlocking-assisted shear adhesion force ranged from 3 to 40 N/cm2 for the nanofiber arrays. Besides the regularly ordered polymeric interlocking system, Ko et al. (2009) fabricated randomly distributed Ge/propylene (core-shell) nanowire(NW) connector adhesion structure, which has dramatic amplification due to the high aspect-ratio geometric interactions. This system exhibits high shear adhesion strength (~163 N/cm2) with anisotropic adhesion behavior. A hand-engaged NW connector (area~0.5 × 0.6 cm2) can sustain 5 kg of weight without failure. Despite having excellent shear adhesion performance, this system exhibits high shear to normal adhesion ratios of ~27 at a preload force of 2.8 N/cm2.
In short, the wing-locking-inspired device can achieve reversible and directional van der Waals (vdW) force-assisted fasteners. Usually, these systems have high-aspect-ratio hairy structures, leading to an extremely high interlocking force in the lateral direction and easy lift-off in the normal direction. Besides vdW interactions, there exists interlocking of the nanowires. However, because the normal strength is significantly lower than the shear strength, the mechanical interlocking is not the dominant binding mechanism.
Gecko Dry and Switchable Adhesion
Gecko adhesion is another famous dry adhesion that benefits from the “contact splitting” principle (Kamperman et al., 2010). It was discovered that on gecko toe pads there are millions of hierarchical fibrillar structures called setae, each of which is ~130 μm long and branches into 300–500 nm spetulae (Ruibal and Ernst, 1965; Russell, 1975), shown in Figures 2A–E. The gecko setae have been approved as being sticky due to vdW force, leading to dramatic adhesion (~10 N/cm2) on both hydrophobic and hydrophilic surfaces (Autumn et al., 2002), while the setal pads are self-cleaning (Hansen and Autumn, 2005). It was shown that the directional tilt of the fibrillar (Tian et al., 2006), and lateral friction force (Zhao et al., 2009) play critical roles in gecko adhesion and self-cleaning because the drag direction and angle decide the adhesive force when the toe pads leave the surface. Whereas, the detaching speed (Xu et al., 2015) is also an important factor that affects the detaching force. This is very interesting because the hierarchical fibrillar structure can be either adhesive or de-adhesive without water, which introduces a critical mechanism of switchable adhesive.
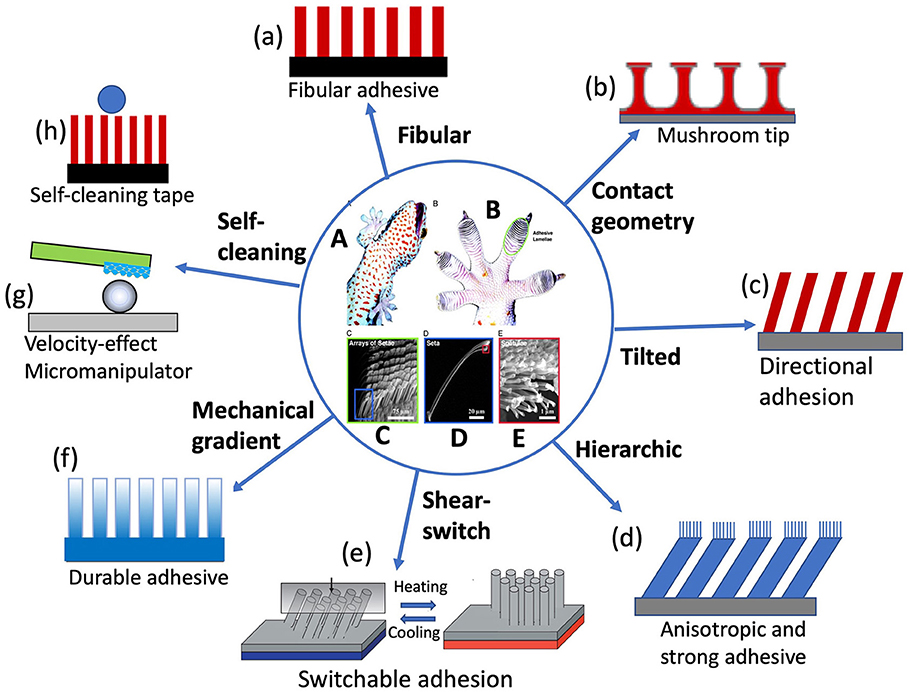
Figure 2. Gecko adhesive system and its biomimetic structures. Optical photos of (A) a tokay gecko (G. gecko) climbing vertical glass, and (B) its foot, with lamella structure (scansors) visible as overlapping pads. SEM images of (C) Array of setae, (D) single seta with branches, (E) spatular tips. Schematic illustrations of gecko-foot-mimicking adhesives with (a) monolithic fibular, (b) tip-modified (mushroom-like) structure, (c) tilted structure, (d) hierarchical structure, (e) shear-slide-modulated adhesion, (f) mechanically graded materials, (h) velocity-effect manipulation, and (i) self-cleaning structures. [Reprinted from Hansen and Autumn (2005). Copyright (2021), with the permission from National Academy of Sciences].
In fact, geckos are able to detach rapidly from walls during hunting, i.e., they exhibit highly controllable adhesion. It is found that the asymmetrical structure of the gecko setae has a significant effect on robust and simultaneous attachment and detachment. Properly orientated setae significantly reduce the forces required to peel the toe from the substrate by simply detaching above a critical angle with the substratum. Autumn (2006b) showed that Geckos peel the tips of their toes (digital hyperextension), which may put an individual seta in an orientation that aids in its detachment. Using finite element analysis of a single seta, Gao et al. (2005) found that the pulling angle determines the detachment from or sliding with respect to the surfaces. The results show that the gecko would pull its toes at a 30° to maximize the adhesion force and pull off at 90°. The effect of angle is due to the failure initiation near the inner edge of contact based on the particular design of the seta.
The gecko-inspired adhesive is so popular that there has a lot of research focusing on the topic in recent years. The early gecko-foot-mimicking adhesives are only an array of monolithic polymer rods/fibers (Figure 2a), but it approves the effectiveness of bioinspired gecko feet in enhancing the adhesion (~10 N/cm2, comparable to that of gecko feet) based on the contact splitting concept (Geim et al., 2003). Inspired by the setal/spatula tip geometry, the adhesives were fabricated into an array of composite patterns consisting of elastomeric mushroom-like microfibers, the tops of which are decorated with an extremely soft and thin layer of pressure-sensitive adhesive (Figure 2b). The adhesive gains the significantly enhanced performance (~30 N/m2) via the optimal tip shape and improved load sharing, outperforming monolithic fibers (Drotlef et al., 2019). Mimicking gecko setal structures, tilted fiber arrays were fabricated to add more bioinspired elements in the artificial gecko feet to enhance the adhesion (Figure 2c) (Lee et al., 2008). It was also found that increasing the leaning angle of nanohairs can enhance both shear adhesion and adhesion hysteresis for soft and hard materials owning to the increased contact area and reduced structural stiffness. Jeong et al. (2009) fabricated polymeric nanohair adhesive with tailored leaning angles, sizes, tip shapes, and hierarchical structures, which exhibited strong adhesion (~26 N/cm2) in the angled direction and weak adhesion (~2.2 N/cm2) in the opposite direction, with a hysteresis value of ≈10.
Analogous to gecko setal arrays (Figures 2C–E), hieratical fibrillar structures with nanofibers on the tips of microfibers (Figure 2d) were fabricated and fount to be adhesive between horizontal and vertical (Murphy et al., 2007, 2008, 2009; Tamelier et al., 2012). These adhesives showed adhesion anisotropy like gecko setae. For example, hierarchically structured vertically aligned carbon nanotube arrays render a high macroscopic force of ~ 100 N/cm2 along the lateral direction, whereas along the normal direction there is a drastic reduction of adhesion force down to ~10 N/cm2 since the normal pulling requires peeling of each entangled tip at the interface. This hierarchical adhesive could achieve the attachment with strong shear bonding and the detachment with easy normal lifting off by just changing pulling directions, promising for the application in climbing robots (Qu et al., 2008). Further researches made wall-climbing robots based on gecko-mimic adhesive tapes (Menon et al., 2004; Menon and Sitti, 2006; Kim et al., 2007). For example, Murphy et al. (2010) proposed a plastic foot structure with fibrillary adhesive and passive peeling, each foot has an adhesive area of 2.83 cm2, a total of 0.19 kg can be supported by at least two feet during locomotion. However, using a fully soft material is unable to apply sufficient pressure to the whole foot. In order to enhance payload capacity and achieve effective climbing behaviors, Shao et al. (2020) combined hard aluminum alloy, soft polydimethylsiloxane (PDMS) and biologically inspired dry adhesive materials to propose a gecko-inspired foot design with a hybrid sandwich structure. Owing to the novel structure with an approximate area of 12.56 cm2, two feet can carry a weight of 3.2 kg.
Gecko feet not only have strong reversible adhesion but also keep very clean although contacting containments every day. These two features seem contradictory when considering that they all originate from the same materials. It was proposed that the self-cleaning ability is attributed to the energy disequilibrium between the interface of the substrate and setae covering up almost the entire toe pads (Hansen and Autumn, 2005). Later experiments demonstrated that hyperextension of gecko setae arrays led to a high-speed retreat of setae from the dirt surface, consequently dislodging the dirt particles stick to the setae due to speed and geometrical effects (Hu et al., 2012; Xu et al., 2015). Self-cleaning adhesive tapes (Figure 2h) were also designed based on the gecko effect (Geim et al., 2003; Lee and Fearing, 2008; Sethi et al., 2008; Dong et al., 2019). Another application of gecko-inspired adhesives is micro-manipulator. For example, a single polymer micropillar (Mengüç et al., 2012) or a polymer microfiber with graphene serves as a “finger” (artificial gecko seta) (Xu et al., 2015; Wan and Xia, 2018), as shown in Figure 2g.
Recently, it was found that individual gecko seta was a mechanically graded material (Dong et al., 2020). The Young's modulus gradually decreases along the seta from base to tip, with up to 20 times of difference in magnitude. Finite element analysis reveals that this mechanical gradient in the seta is essential to make it more flexible and less stressed so as to generate larger frictional adhesion on rough surfaces while reducing the risk of fatigue failure of the seta. This mechanical gradient was also observed in spider attachment hairs (Flenner et al., 2020) and tarsal setae of the ladybird beetle (Peisker et al., 2013). This provides an excellent model for the development of durable adhesives. Inspired by these findings, the artificial gecko foot-hairs with gardenly distributed magnetic nanoparticles along the hairs (Figure 2f) were fabricated, achieving gradient modulus/stiffness similar to gecko setae. The bioinspired microhairs exhibit the enhanced resistance to fatigue compared with the uniform counterparts.
Octopus Sucker and Inspired Adhesives
Octopuses use suckers for collecting/holding items, locomotion, and adhesion based on suction (Packard et al., 1988; Kuba et al., 2006). The features of octopus suckers have been reported including musculature (Nachtigall, 2013), sensing properties (Graziadei and Gagne, 1976a,b), surface features (Nixon and Dilly, 1977; Packard et al., 1988), and so on. The exposed disk-like portion of the sucker is called the infundibulum, and the upper hollow portion is called the acetabulum. There is a rim covered with a loose epithelium circling the infundibulum (Girod, 1884), shown in Figure 3A. When suckers are attaching to the surface, making seal at the rim forms, the pressure in the acetabular cavity is reduced. The acetabular cavity is filled with water which can maintain the pressure (Tramacere et al., 2012). The key to the success of their suction is not the shape of their cups or the material, but a little spherical protrusion at the very center of the octopuses' suction cups (Baik et al., 2017). During the sucking, the sphere can help create a vacuum chamber in the cup and thus allowing it to stick to even rough surfaces underwater. The octopus sucker has a high suction efficiency and can be performed on almost any non-porous surfaces (Smith, 1991).
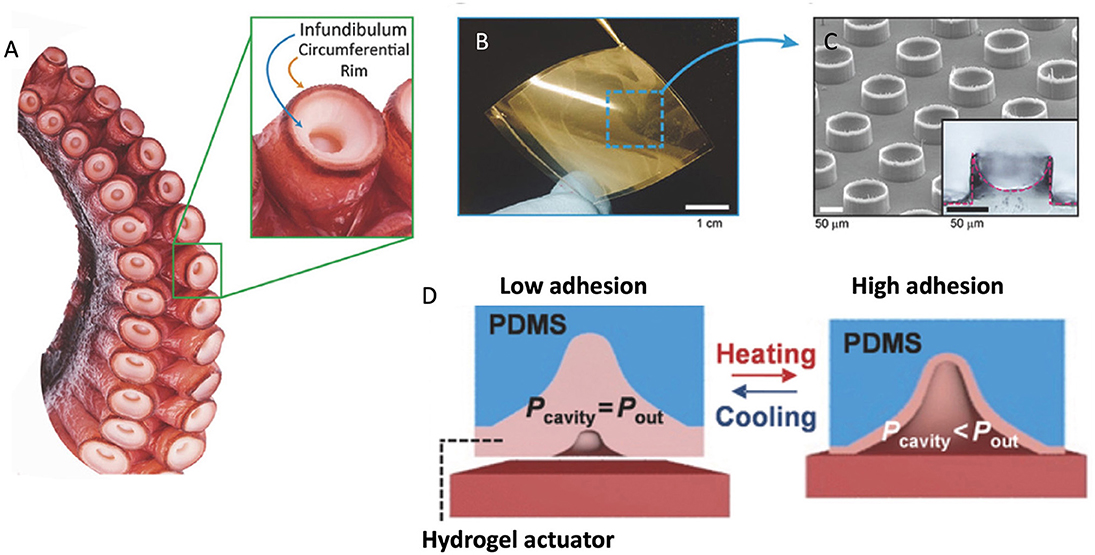
Figure 3. (A) Photograph of an octopus sucker (Octopus vulgaris) and its infundibular and circumferential rim. (B) Photographs of octopus-mimicked adhesion patches with meniscus-controlled microtips (≈3 cm × 3 cm). (C) SEM image and cross-sectional optical image (inset) of micro-suckers (100 μm diameter and 75 μm height) mimicking the infundibular and circumferential rim of an octopus sucker. (D) Schematic representation of temperature-modulated octopus-inspired switchable adhesive pad. [(A–C) Reproduced with the permission from Baik et al. (2018). Copyright (2021), Advanced Science. (D) Reproduced from Lee et al. (2016). Copyright (2021), with permission from WILEY-VCH Verlag GmbH and Co. KGaA, Weinheim].
The octopus inspired adhesives were developed based on suction mechanism, including single suckers similar to octopus ones and some reversible adhesives (Hou et al., 2012; Tramacere et al., 2012). Chen and Yang (2017) designed nanoscale sucker arrays for the use of adhesion in dry and wet environments. This adhesive maintained good performance after 80 cycles on wet smooth surfaces and was claimed to meet the demand for medical use. Baik et al. (2018) mimicked the infundibulum-acetabulum structure of octopus and made a wet-tolerant adhesive patch, which was proved better than ones with simple pitch-structures. Inspired by the rim and infundibulum of octopus suction cup, they also developed highly reversible adhesive patches with 3D microtips in micropillars (Figures 3B,C) (Baik et al., 2018). The adhesive patches can stably attach on moist, hairy, and rough skin. Mimicking muscle actuation to modulate cavity-pressure-induced adhesion of octopus suckers, Lee et al. (2016) engineered octopus-inspired smart adhesive pads (Figure 3D). They fabricated an array of microscale suckers coated with a thermally responsive polymer. At ambient condition, the walls of each pit stand properly (adhesion-off state), but at 32°C, the walls contract, inducing suction, thereby letting the entire mate to adhere to a surface (adhesion-on state). The adhesion strength also spiked from 0.32 to 94 kPa at high temperatures. Other approaches to octopus inspired switchable adhesives were reported in the literatures (Choi et al., 2015; Purtov et al., 2015).
Biomimetic Structures With Liquid-Mediated Switchable Adhesion
Insect Inspired Liquid-Mediated Adhesives
The contact between insect adhesive organs and the substrates has been studied for about 150 years (West, 1861). Though belonging to different animal groups, insects take advantage of fibrillar structures for adhesion just like what geckos and spiders do (Gorb and Beutel, 2001). Figures 4A–F shows the detailed structure of Hemisphaerota cyanea pads (Eisner and Aneshansley, 2000), which is quite similar to the aforementioned gecko's setae structure. However, many studies proved that insect adhesion is different from gecko because the liquid is involved in the insect one. When insects like beetles and flies walk on clean substrates, there are “footprint” left which is proved droplets (Eisner and Aneshansley, 2000; Betz, 2003; Langer et al., 2004). A large amount of water will lubricate while only tiny droplets were discovered left on the substrate. This indicates capillary and viscous forces were involved in the attachment of insects. The liquid could enhance the adhesion while it may also add difficulties to detachment. Thus the adhesion was believed direction-dependent (Niederegger and Gorb, 2003; Bullock et al., 2008; Labonte et al., 2016) and rate-dependent (Labonte and Federle, 2015). That is why insects can control the adhesion and fast detach and thus the shearing force and shearing direction are important factors in the mechanism of insect adhesion.
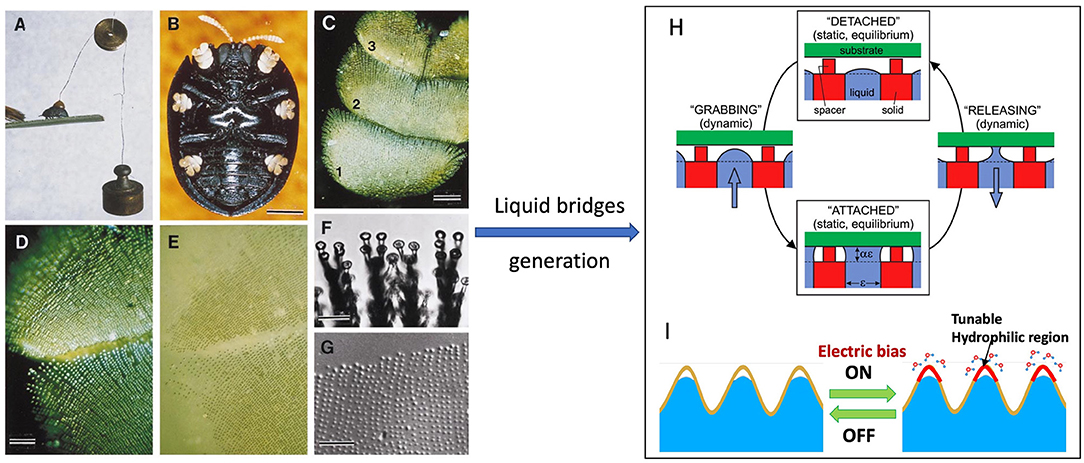
Figure 4. (A) Photograph of a beetle under a 2 g pull. (B) Ventral view of beetle with yellow tarsi. (C) Beetle tarsus (numbers refer to tarsomeres). (D) Polarized epi-illumination of tarsus adhering to glass. (E) Tarsus in contact with glass under non-polarized light; showing it is wet at contact points of the bristles. (F) Bristle pads adhering to glass. (G) Droplets remained on glass showing a tarsal “footprint.” (H) Switchable wet adhesion concept: Top and bottom states are adhesion-off and adhesion-on states, respectively. Switching from adhesion-on to adhesion-off is achieved by pumping liquid into the device; vice versa. (I) Schematic of graphene-based nano-textured surface with highly switchable adhesion triggered by electric signals. [(A–G) Reprinted from Eisner and Aneshansley (2000). Copyright (2021), with the permission from National Academy of Sciences. (H) Reprinted from Vogel and Steen (2010). Copyright (2021), with the permission from National Academy of Sciences].
Inspired by the adhesion abilities of palmetto beetles that generate adhesive forces (~33 mN) exceeding 100 times its body weight (Eisner and Aneshansley, 2000), Vogel and Steen (2010) created a switchable adhesive device, taking advantage of the surface tension force from a large number of small liquid bridges that can be significant and quickly made or broken electronically (switchable) (Figure 4H). The device consists of a plate with microscale holes from which a water droplet expands with the water/gas interface held at the orifice edge. To prevent the contact between the droplet and the substrate, spacers are set above the orifice. In the adhesion-off state, the device generates the adhesion from the dry spacers only. In grabbing, water is squeezed out of the face pad to form liquid bridges between device and substrate to generate a large adhesive force. In releasing, liquid is sucked back into the device to break the bridges. The adhesion force depends on the size of the holes. A force of 100 kPa could be generated by the device for a hole size of 0.2 mm.
Mimicking the beetle adhesion mechanism, Wan et al. recently demonstrated a graphene-based nano-textured surface with highly switchable adhesion triggered by electric signals (Wan et al., 2019). As schematically shown in Figure 4I, in a high humid environment, upon applying an electrical bias, the graphene surface can collect moisture from the environment to form a large number of water micro/nano-bridges with target surface, which drastically increases the adhesive force by a factor of 6 at an applied voltage of−15V. This graphene surface is able to pick up and place a variety of micro/nano-objects for micro-assembling.
Insect-inspired switchable adhesives usually follow the trend of humidity/capillary controlling. For example, fibrillar adhesive pads were made by Xue et al. (2013) and they found that the force can be tunable by controlling the humidity. Kwon et al. (2006) improved the adhesion of micro-patterned elastomer by adding a small amount of water.
Tree Frog Wet Adhesion
Unlike gecko and insect fibrillar structures, tree frog pads are foam-like on the surface and covered with an array of cells surrounding gaps, shown in Figures 5A–E. Each cell consists of many smaller blocks and channels (Federle et al., 2006; Persson, 2007). Wet liquid can be stored in the gaps and channels. When the pads are compressed on the substrate, liquid is released on filling the gap between pads and the substrate, forming a lot of small liquid bridge, which brings capillary adhesion (Emerson and Diehl, 1980; Barnes, 2007). During detaching, the liquid brings cracked along the pad-substrate interface by the lateral movement of frog toes (Persson, 2007). In addition, according to hydrodynamic theory, the patterns of micropillars separated by a network of interconnected microchannels effectively widens the gap between the adhesive and substrate separated by a liquid (Pilkington et al., 2016). These features reduce hydrodynamic repulsion and adhesion between adhesive and substrate (Dhong and Fréchette, 2015). Thus, the important features of tree frog in generating switchable adhesion include (1) Hexagonal surface patterning that promotes, particularly on hydrophilic surfaces, drainage, which helps remove interstitial liquids, make intimate contact, and generate vdW forces, and (2) Micropatterning that would hamper crack initiation and propagation, hence enhancing adhesion.
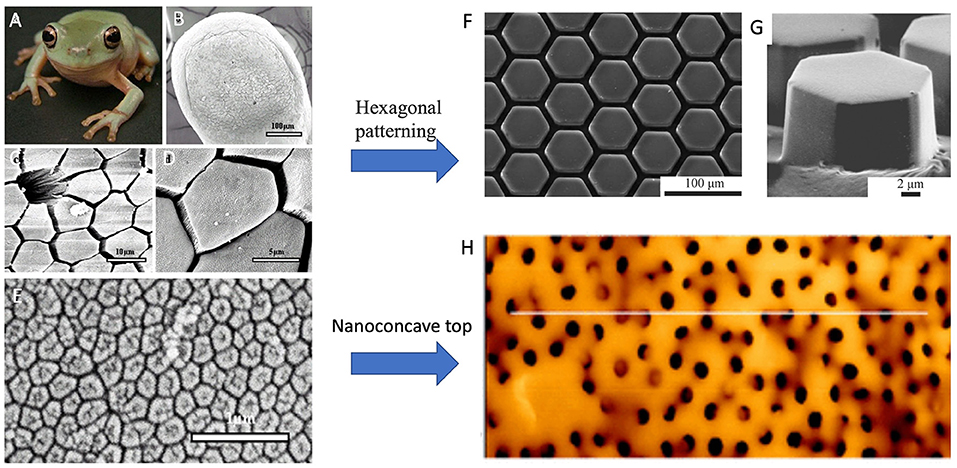
Figure 5. (A) Photograph of juvenile tree frog, Litoria caerulea (snout–vent length, ~40 mm). (B–D) SEM images of epithelium of frog pads. (B) Whole toe pad. (C) Image showing a mucous pore and hexagonal epithelial cells separated from each other at their distal ends by channels. (D) Structure of the epithelial cells. (E) SEM image of hexagonal nanostructures of a dense array on the external surface of a frog toe pad epithelial cell. Tree-frog-mimicked adhesives: (F) PDMS adhesive with hexagonal pattern for dry adhesion, and (G) Hexagonally micropatterned PDMS surface for capillary adhesion. (H) an array of composite micropillars with nanopits on the surface, mimicking the nanoconcave structure of epidermal cells on toe pads of tree frogs. [(A–E) Reproduced from Scholz et al. (2009). Copyright (2021), with permission from The Company of Biologists Ltd. (F) Reproduced from Murarash et al. (2011) Copyright (2021), with permission from Royal Society of Chemistry. (G) Reproduced from Drotlef et al. (2012). Copyright (2021), with permission from WILEY-VCH Verlag GmbH and Co. KGaA, Weinheim. (H) Reproduced from Liu et al. (2020). Copyright (2021), with permission from American Chemical Society].
Artificial frog-inspired switchable adhesive was fabricated and used to prove the adhesive mechanisms of tree frogs; the shapes of micropads would determine the adhesion as well (Drotlef et al., 2012). Typical tree-frog-foot structures: hexagonal PDMS surface patterns (Figures 5F,G) were fabricated for friction under dry conditions (Murarash et al., 2011), and capillary adhesion (Drotlef et al., 2012). Iturri et al. (2015) found significantly higher friction PDMS pillars with the presence of water, and claimed that the orientation-dependent adhesive could be reversibly used in wet conditions. An array of micropillars with nanopits on the surface (CPp) (Figure 5H) was fabricated by mimicking the nanoconcave top of epidermal cells on toe pads of tree frogs (Figure 5E) (Liu et al., 2020). The pillars were made of the composite of polystyrene (PS) nanoparticles and PDMS. Compared with the arrays of micropillars without nanopits, CPp demonstrates much larger wet adhesion since most of the liquid between CPp and the target surface is squeezed out. Due to the squeezing effect, the remaining liquid in nanopits forms multiple nanoscale liquid bridges within the contact area of a single micropillar. The multiple liquid bridges, together with the suction effect and the solid direct contact facilitate strong wet adhesion. It is shown that the adhesive force is ~36.5 times that of toe pads of tree frogs. This offers a new route for adhesives to generate strong wet adhesion.
Physically-Actuated Switchable Adhesion By Controlling Surface Configuration Through External Stimuli
Smart adhesive surfaces can be found in animals, beetles, flies, and ants, with which they can attach and detach quickly to various substrates for fast movement, prey capture, and defense against predators. Besides the unique structures and properties of the natural adhesives discussed above, the adhesion is usually modulated by altering the topography of adhesive pads through external stimuli. An aphid, for example, has a unique adhesion system when clinging to a surface (Dixon et al., 1990). When attaching a flat surface, aphid turn its pulvillus by increasing blood pressure, which increases the contact area and thus the adhesion. Upon release from the surface, the pulvillus is retracted by its tibial muscles, which reduces the contact area and hence adhesion. Another example is gecko feet that are in a non-sticky default state. During gecko locomotion, a force load and dragging steps are needed to switch its feet to an adhesive state (Autumn, 2006a; Autumn and Hansen, 2006). During the attachment, gecko performs a mechanical stimulus via gross leg movements to reorientate setal shaft and establish the contacts with the surface. An opposite process occurs during detachment. This switchable adhesion via mechanical stimulus has been implemented in synthetic systems. Beyond the mechanical stimuli, other stimuli-responsive strategies are implemented to achieve switchable adhesion, including temperature, electrical, magnetic, light, etc., as shown in Figure 6.
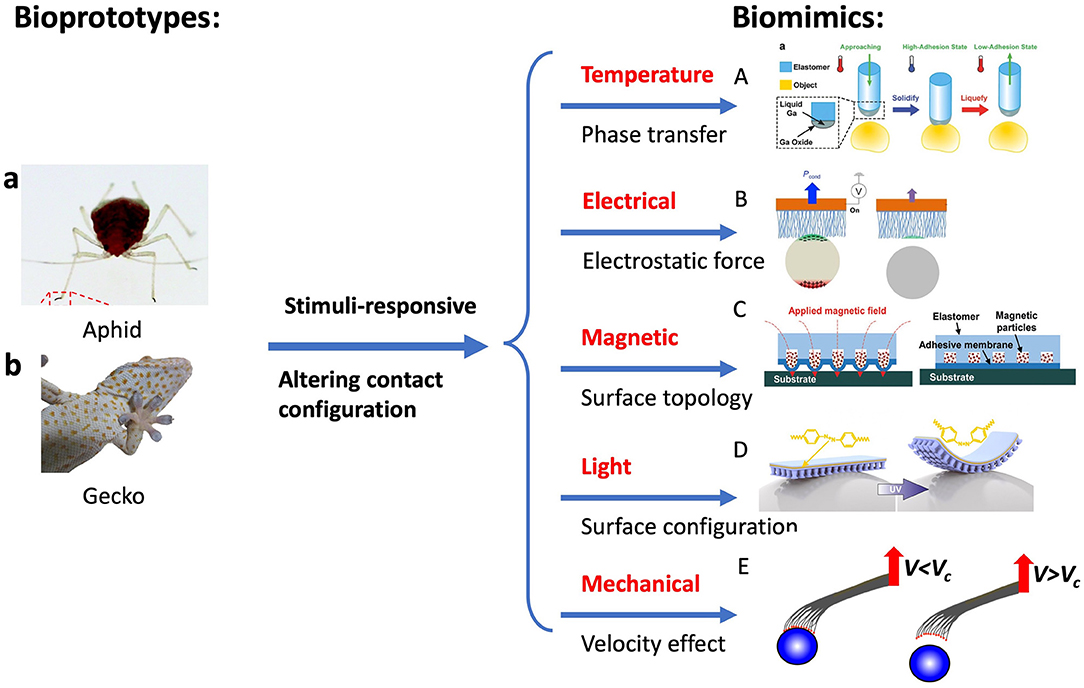
Figure 6. Bioprototypes: (a) Aphid and (b) Gecko. Switchable adhesion by controlling surface configuration through external stimuli: (A) temperature (phase change), (B) electrical (electrostatic attraction), (C) magnetic, (D) light (UV-light), and (E) velocity-effect micromanipulators. [(A) Reproduced from Adv. Mater 28(25), Ye et al. (2016). Copyright (2021) with permission from WILEY-VCH Verlag GmbH and Co. KGaA, Weinheim. (B) Reproduced with permission from Kim et al. (2019). Copyright (2021) Science Advances. (C) Reproduced from Linghu et al. (2019). Copyright (2021) with permission from Royal Society of Chemistry. (a, D) Reproduced from Kizilkan et al. (2017). Copyright (2021) with permission from The American Association for the Advancement of Science].
Temperature-Controlled Switchable Adhesives
Following the natural switchable adhesion concept, Reddy et al. (2007) used shape memory thermoplastic elastomers (SMTE) to fabricate arrays of vertical micropillars with diameter between 0.5 and 50μm and height between 10 and 100 μm by soft molding at its high transition temperature (Tht = 120–140°C). The as-fabricated topography was mechanically deformed at the low transition temperature (Tlt = 51°C), and cooled down to room temperature in the deformed position, yielding a temporary non-adhesive surface with tilted pillars (Figure 2e). When increasing the temperature above Tlt, the tilted pillars are turned into straight state, increasing the adhesion by a factor of at least 200. Similar smart soft adhesives were designed and fabricated using nematic liquid crystal elastomers (LCE) to dynamically respond to their environment (Ohzono et al., 2019). When the environmental temperature is reduced to critical value, the LCE changes from isotropic phase to nematic one, causing the adhesion more than double. Phase change of gallium (Ye et al., 2016) is also exploited to achieve switchable adhesion due to its relatively low melting point (Tm = 29.7°C) (Figure 6A). The temperature was raised to 31°C (T>Tm, liquid state) causing the low-adhesion state, and decreased to 23°C (T<Tm, solid state), leading to the high-adhesion state. Changing the temperature can switch the adhesion from the high-adhesion state to low-adhesion state with a high switching ratio of 33.4–178 under dry conditions, and 18 under wet conditions.
Electrically-Modulated Switchable Adhesives
Compared with other types of external stimuli, electrical modulation has several advantages, including versatility (i.e., no need to modify the adhesive surface), fast actuation, and compatibility with a range of environments (Wan et al., 2019). To achieve switchable adhesion, Kim et al. introduced soft nanocomposite electroadhesives, consisting of sparse forests of alumina-coated carbon nanotubes (CNTs) (Figure 6B) (Kim et al., 2019). This bioinspired adhesive can generate electrostatically-modulated switchable dry adhesion, which exhibits 40-fold lower nominal dry adhesion than typical surfaces, yet their adhesive force is enhanced 100 times by applying 30 V to the CNTs. The adhesive can perform digital transfer printing of various objects including Ag nanowire films, metal and polymer microparticles, and unpackaged light-emitting diodes. Graule et al. (2016) developed a switchable electroadhesive device that can perch and detach on nearly any surfaces while consumed power is approximately three orders of magnitude less than required to sustain flight. A flying robotic insect with these electroadhesives can firmly perch on various materials, such as glass, wood, and a natural leaf.
Magnetically-Stimulated Aphid-Inspired Adhesives
Linghu et al. (2019) developed a magnetically actuated adhesive. This stamp-like adhesive has open reservoirs, in which magnetic particles are filled and encapsulated by a thin surface membrane (Figure 6C). In the initial stage, the flat surface of membrane is maintained to generate sufficient adhesion (the adhesion-ON state) for attachment to a substrate. To switch the adhesion, a gradient magnetic field is applied to magnetize the magnetic particles. These magnetized particles push the thin surface membrane to bulge around the interface, making the membrane peel at the outer perimeter and propagate to the center, thereby reducing the contact with the substrate, decreasing the interfacial adhesion. This state is referred to as the adhesion-OFF state. Therefore, through magnetic actuation, the adhesion of aphid-inspired adhesives can be switched between a strong (i.e., adhesion ON) state and a weak (i.e., adhesion OFF) state from a high value of 20.5 KPa to a low value of 7.3 kPa. Drotlef et al. (2013) fabricated magnetically actuated arrays of micropillars that can reversibly change their geometry upon applying a magnetic field. A gecko-inspired magnetically-tuned elastomeric micropatterns containing magnetic microparticles were also fabricated for switchable adhesion, which work under dry and wet conditions. More recently, magnetically-switchable adhesion to non-magnetic objects was fabricated with a two-phase composite consisting of silicone elastomer matrix and dispersed magneto-rheological fluid (Testa et al., 2020). The composite can achieve up to a 9-fold increase of the adhesion of non-magnetic objects in the application of a 250 mT field.
Light-Modulated Switchable Adhesives
An artificial, photocontrollable adhesive was designed and fabricated, which can be switched on and off simply by shining a UV light on it (Kizilkan et al., 2017). This adhesive is a tape that consists of three layers. The top layer is mushroom-shaped pillars with sticky flat tops that actually touch the surface of the target material (e.g., glass) (Figure 6D) (Kizilkan et al., 2017). These pillars are embedded in a layer of polydimethylsiloxane, which is bonded to a layer of azobenzene liquid crystals (ALC) that are sensitive to UV light. At the bottom, there is another layer of polydimethylsiloxane. When UV light is aimed at them, they change position relative to one another. In the absence of UV light, the top layer of the adhesive can generate enough adhesion to stick to a glass surface. When the adhesive is exposed to a UV light the ALC curls slightly pulling the sticky parts from the glass surface to release the adhesive. Varying strength of UV light tunes the degree of adherence required. Under ultraviolet light illumination, the adhesive force of the material decreases by a factor of 2.7 but is quickly recovered after the light is turned off. This bioinspired light-modulated device can pick up and drop down planar and three-dimensional solid objects. Zhou et al. (2019) fabricated a photo-responsive azobenzene-contained polymer (azopolymer) for light-controlled switchable adhesion. The azopolymer P1 experiences reversible solid-to-liquid transitions under light illumination due to trans–cis photoisomerization. Like conventional adhesives, trans P1 can glue two substrates. Upon being exposed to UV light the trans-to-cis isomerization P1liquefies, weakening the adhesion. The underwater adhesion of trans-P1-glued quartz (~0.93 MPa) is reduced to ~0.08 MPa after UV irradiation. This represents a strategy for generating reversible bonding and debonding.
Mechanically-Stimulated Adhesives
Inspired by the aphid adhesion and release strategy, an artificial aphid adhesion device is developed, consisting of an inflatable membrane clamped to the metallic cylinder filled with air (mechanical simulation) (Carlson et al., 2012; Dening et al., 2014). A cylindrical elastomer pillar with a mushroom-like cap and annular chamber was also designed to generate switchable dry adhesion dynamically modulated by subsurface pneumatic pressure (Mohammadi Nasab et al., 2020). These adhesives achieve adhesion-ON state and switch to adhesion-OFF with an adhesion switching ratio of 5 upon filling with pressurized air into the chamber.
Xu et al. (2015) demonstrated that the same contact-shape-dependent dynamic adhesion (observed in gecko spatulae/setae) can be utilized to design micromanipulators for precise assembly. To prove this concept, a synthetic glass microfiber was made having a spatula-shaped platelet on top (Xu et al., 2015; Wan and Xia, 2018). It was shown that the same weakly dependency of adhesion on pull-off velocity on surfaces of different chemical compositions. Adopting the principle of contact shape-dependent dynamic adhesion responses, micromanipulators were fabricated, which demonstrates highly controllable microparticle manipulation capabilities by simply tuning the retreating velocity (Figure 6E).
Biomimetic Structures With Chemically-Mediated Switchable Adhesion
Hydrogen Bonds in DNA/RNA for Switchable Adhesion
The DNA double helix consists of base pairs that connecting each other with hydrogen bonds. The base pairs also contribute to the folded structure of both DNA and RNA (Cockburn et al., 1976; Fischer and Lerman, 1983; Guéron et al., 1987). The hydrogen bonds were observed directly (Dingley and Grzesiek, 1998) and play key roles in the stabilization of protein and nucleic acid secondary structure (Saenger, 1984; Jeffrey and Saenger, 2012). With the specific base pairing, such as guanine-cytosine and adenine-thymine shown in Figures 7A,B, the DNA can backup genetic information due to weak reversible hydrogen bonding, which can be used to design high-performance smart adhesives (Sun and Qing, 2011; Berg et al., 2015).
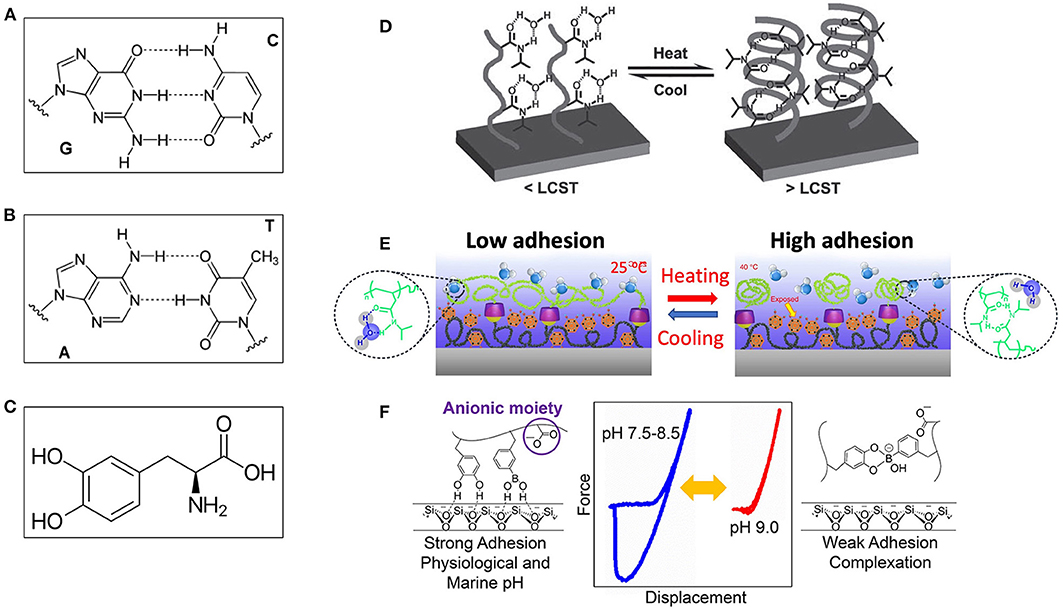
Figure 7. (A) A GC based DNA pair with three hydrogen bonds, and (B) An AT-based DNA pair with two hydrogen bonds, where dashed lines represent non-covalent hydrogen bonds between the pairs. (C) A 3,4-dihydroxy-L-phenylalanine (DOPA) group. (D) Temperature-regulated hydrogen bonding adhesive mechanism via the coil–globule transition of PNIPAAm film: (Left) Adhesion-on state: structure of thermoresponsive glycopolymers P1 and P2 at the temperature below lower critical solution temperature (LCST); (Right) Adhesion-off state: E. coli MG1655pGFP bacteria aggregation controlled by thermal oscillation across the LCST in the presence of polymer P2. (E) Working mechanism of underwater switchable adhesion: (Left) Schematic drawing showing low interfacial adhesion at temperature below LCST, and (Right) The collapsed pNIPAM-CD chains due to the formation of numerous agglomerates during the phase transition at the temperature higher than LCST, leading to the explosion of the adhesive group, and consequently enhanced interfacial adhesion. (F) A pH-responsive switchable adhesive comprising dopamine methacrylamide and 3-acrylamido phenylboronic acid: (Left) Adhesion-on state at low pH value, (Right) adhesion-off state at high pH value, and (Middle) the force curves at different pH values. [(D) Reproduced from Sun and Qing (2011). Copyright (2021) with permission from WILEY-VCH Verlag GmbH and Co. KGaA, Weinheim. (E) Reproduced with permission from Zhao et al. (2017). Copyright (2021) Nature Communications. (F) Reproduced from Narkar and Lee (2018). Copyright (2021) with permission from American Chemical Society].
Extensive efforts have been put into hydrogen bond based switchable adhesives. Viswanathan et al. (2006) used a novel adenine-derivatized triethoxysilane to modify silicon surface, and applied a thymine-functionalized to adenine-modified PS surfaces. These two surfaces achieved reversible attachment due to thymine-adenine hydrogen bonds. Another series of artificial polymers including poly(N-isopropylacrylamide) (PNIPAM) and its copolymers are famous smart materials with switchable adhesion. The switchable transition is due to the complex hydrogen bond network formed among the PNIPAM chains by lower the temperature to the critical temperature (Figure 7D) (Sun and Qing, 2011). Not only the surface hydrogen bond, but also the surface morphology can be tuned (Hou et al., 2009; Gao et al., 2010), and thus aforementioned adhesive microstructures are potentially combined with PNIPAM. pH-response and photosensitive PNIPAM based polymers were also reported (Wang et al., 2006; Xia et al., 2006; Matsubara et al., 2007; Russew and Hecht, 2010).
Supermolecule-Mediated Switchable Adhesives
Many marine organisms, including mussels, sandcastle worms, and barnacles, possess a strong wet adhesion ability via catechol chemistry, polyelectrolyte complex, and supramolecular architectures (Shao and Stewart, 2010; Ahn et al., 2013; Heinzmann et al., 2016). These proteins contain a high content of 3,4-dihydroxyphenylalanine (DOPA) residues (Figure 7C), and can achieve robust binding to underwater rocks via chelation of the catechol functionality of DOPA with the inorganic oxides. In addition, metals could strengthen the adhesive structure through a self-healing mechanism of metal coordination complexes (Shafiq et al., 2012). Because of its versatility, catechol-containing natural molecules have been used for different applications (Sparks et al., 2012; Panchireddy et al., 2018; Fan et al., 2020). However, to make the adhesion switchable, the interfacial materials have to be modified (Nishida et al., 2013; Narkar et al., 2016).
Mimicking the natural wet adhesion through controlling catechol chemistry, polyelectrolyte complex, and supramolecular architectures, Zhao et al. (2017) synthesized bioinspired adhesive. This adhesive comprises two copolymers: a mussel-inspired guest-adhesive, and a thermoresponsive host. The guest copolymer is made by conjugating the DOPA polymer with the guest motif adamantine (AD) and methoxyethyl acrylate (MEA) monomer. In the polymer, DOPA serves as the adhesive moiety, AD as the guest moiety and MEA as the hydrophobic matrix to improve the adhesion property of DOPA. A host copolymer pNIPAM-CD is further created to reversibly regulate the wet adhesion property. Finally, the as-prepared guest copolymer was deposited on a silicon substrate by the self-assembly of the host copolymer via the host–guest chemistry. This copolymer shows the reversible, tunable, and fast regulation of the wet adhesion on diverse surfaces modulated by temperature (Figure 7E).
Narkar and Lee (2018) developed a pH-responsive switchable adhesive comprising dopamine methacrylamide and 3-acrylamide phenylboronic acid (Figure 7F). This adhesive can strongly adhere to quartz substrate at a neutral to mildly basic pH (7.5–8.5), but its adhesion drastically reduces due to the formation of catechol–boronate complex when pH is further increased to 9.0 (18- and 7-fold reduction compared with the values measured at pHs 7.5 and 8.5, respectively). The adhesive is reversible, and its interfacial adhesion property of the adhesive can be tuned by changing pH in consecutive contact cycles. Nevertheless, an acidic pH (3.0) has to be used to break the catechol–boronate complex to recover the elevated adhesive property.
Summary and Future Direction
In this review we discussed and summarized four types of switchable adhesion: mechanically-based, liquid-mediated, physically-stimulated and chemically-enhanced adhesions. Based on the fundamental theories of the switchable adhesion, biomimetic smart surfaces with switchable adhesion modulated have been developed for various applications. The unique microstructures possessed by gecko, insects, octopuses or frogs determine the performance of these adhesives while water and supramolecules could be a media to enhance the attaching force and switchability. Modifications to these structures can result in additional properties such as self-cleaning and or dry/wet dual-application. Apart from the structure uniqueness, external stimuli including shearing forces, velocity, electric and magnetic fields, temperature, lights, pH value, etc. were used to control the adhesion switchability, which expands the approaches of biomimetic design. In addition, external stimuli can also help to control surface morphology, which brings the possibility of combining two or more adhesion mechanisms for better performance.
The applications of biomimetic switchable adhesive are not limited to traditional attachment/detachment, but also sensors, micromanipulation, wearable devices and medical services. Though a lot of great achievements have been made, there are still many challenges that need to be conquered. The materials used for previous bio-inspired adhesives are mostly polymers. Compared with animal bodies which are self-healing, the most critical weakness of these bioinspired smart adhesion materials and devices is low fatigue. Durability is an essential property in a reliable mechanical system and in commercial applications. Hence, reliable materials and designs are acquired in future work.
Another challenge is the multifunctionality. Multifunctional materials increase the function of the adhesion systems. However, most of the current biomimetic switchable adhesives possess single function: attachment-detachment, while the biological adhesive systems are multifunctional. For example, the elytra surface of Namib Desert Darkling beetles, with the combination of hydrophobic-hydrophilic bump shell structures, can not only protect their bodies and prevent the evaporation of body water, but also collect water vapor from the air (Nørgaard and Dacke, 2010). Therefore, future biomimetic design should consider the integration of multifunctions in the next-generation smart adhesion systems.
Future biomimics could also be directed to combining two or more bioprototypes from Nature into one material or device to achieve multifunctionality. Presently, most biomimicking materials are made by mimicking one biological materials structure. Taking the advantage of more than one biological structure could create new materials with unique multifunctionality. For example, a hybrid bioinspired adhesive consisting of gecko-foot-mimicked pillars coated with a thin layer of a synthetic polymer that mimics the wet adhesive proteins found in mussel holdfasts can achieve reversible attachment to various surfaces in both dry and wet environments (Lee et al., 2007).
Author Contributions
All authors listed have made a substantial, direct and intellectual contribution to the work, and approved it for publication.
Conflict of Interest
The authors declare that the research was conducted in the absence of any commercial or financial relationships that could be construed as a potential conflict of interest.
Publisher's Note
All claims expressed in this article are solely those of the authors and do not necessarily represent those of their affiliated organizations, or those of the publisher, the editors and the reviewers. Any product that may be evaluated in this article, or claim that may be made by its manufacturer, is not guaranteed or endorsed by the publisher.
Acknowledgments
We thank the support from the National Science Foundation under the contract (1662288).
References
Ahn, Y., Jang, Y., Selvapalam, N., Yun, G., and Kim, K. (2013). Supramolecular velcro for reversible underwater adhesion. Angew. Chem. 125, 3222–3226. doi: 10.1002/ange.201209382
Andrews, H. G., and Badyal, J. P. S. (2014). Bioinspired hook surfaces based upon a ubiquitous weed (Galium aparine) for dry adhesion. J. Adhes. Sci. Technol. 28, 1243–1255. doi: 10.1080/01694243.2014.891435
Autumn, K. (2006a). Frictional adhesion: a new angle on gecko attachment. J. Exp. Biol. 209, 3569–3579. doi: 10.1242/jeb.02486
Autumn, K. (2006b). How gecko toes stick: the powerful fantastic adhesive used by geckos is made of nanoscale hairs that engage tiny forces, inspiring envy among human imitators. Am. Sci. 94, 124–133. doi: 10.1511/2006.58.124
Autumn, K., and Hansen, W. (2006). Ultrahydrophobicity indicates a non-adhesive default state in gecko setae. J. Comp. Physiol. A 192, 1205–1212. doi: 10.1007/s00359-006-0149-y
Autumn, K., Sitti, M., Liang, Y. A., Peattie, A. M., Hansen, W. R., Sponberg, S., et al. (2002). Evidence for van der Waals adhesion in gecko setae. Proc. Natl. Acad. Sci. U.S.A. 99, 12252–12256. doi: 10.1073/pnas.192252799
Baik, S., Kim, D. W., Park, Y., Lee, T.-J., Ho Bhang, S., and Pang, C. (2017). A wet-tolerant adhesive patch inspired by protuberances in suction cups of octopi. Nature 546, 396–400. doi: 10.1038/nature22382
Baik, S., Kim, J., Lee, H. J., Lee, T. H., and Pang, C. (2018). highly adaptable and biocompatible octopus-like adhesive patches with meniscus-controlled unfoldable 3D microtips for underwater surface and hairy skin. Adv. Sci. 5:1870045. doi: 10.1002/advs.201870045
Barnes, W. J. P. (2007). Functional morphology and design constraints of smooth adhesive pads. MRS Bull. 32, 479–485. doi: 10.1557/mrs2007.81
Berg, J. M., Stryer, L., and Tymoczko, J. L. (2015). Stryer Biochemie. Berlin; Heidelberg: Springer-Verlag.
Betz, O. (2003). Structure of the tarsi in somestenus species (coleoptera, staphylinidae): external morphology, ultrastructure, and tarsal secretion. J. Morphol. 255, 24–43. doi: 10.1002/jmor.10044
Betz, O., and Kölsch, G. (2004). The role of adhesion in prey capture and predator defence in arthropods. Arthropod Struct. Dev. 33, 3–30. doi: 10.1016/j.asd.2003.10.002
Bullock, J. M. R., Drechsler, P., and Federle, W. (2008). Comparison of smooth and hairy attachment pads in insects: friction, adhesion and mechanisms for direction-dependence. J. Exp. Biol. 211, 3333–3343. doi: 10.1242/jeb.020941
Bullock, S. H., and Primack, R. B. (1977). Comparative experimental study of seed dispersal on animals. Ecology 58, 681–686. doi: 10.2307/1939019
Carlson, A., Wang, S., Elvikis, P., Ferreira, P. M., Huang, Y., and Rogers, J. A. (2012). Active, programmable elastomeric surfaces with tunable adhesion for deterministic assembly by transfer printing. Adv. Funct. Mater. 22, 4476–4484. doi: 10.1002/adfm.201201023
Chen, C. M., Chiang, C. L., Lai, C. L., Xie, T., and Yang, S. (2013). Buckling-based strong dry adhesives via interlocking. Adv. Funct. Mater. 23, 3813–3823. doi: 10.1002/adfm.201300052
Chen, Y. C., and Yang, H. (2017). Octopus-inspired assembly of nanosucker arrays for dry/wet adhesion. ACS Nano 11, 5332–5338. doi: 10.1021/acsnano.7b00809
Choi, M. K., Park, O. K., Choi, C., Qiao, S., Ghaffari, R., Kim, J., et al. (2015). Cephalopod-inspired miniaturized suction cups for smart medical skin. Adv. Healthc. Mater. 5, 80–87. doi: 10.1002/adhm.201500285
Cockburn, A. F., Newkirk, M. J., and Firtel, R. A. (1976). Organization of the ribosomal RNA genes of dictyostelium discoideum: mapping of the nontrascribed spacer regions. Cell 9, 605–613. doi: 10.1016/0092-8674(76)90043-X
De Mestral, G. (1955). Velvet Type Fabric and Method of Producing Same. U.S. Patent No. 2,717,437. Washington, DC: U.S. Patent and Trademark Office.
De Mestral, G. (1961). Separable Fasting Device. U.S. Patent No. 3,009,235. Washington, DC: U.S. Patent and Trademark Office.
Dening, K., Heepe, L., Afferrante, L., Carbone, G., and Gorb, S. N. (2014). Adhesion control by inflation: implications from biology to artificial attachment device. Appl. Phys. A 116, 567–573. doi: 10.1007/s00339-014-8504-2
Dewitz, H. (1884). Ueber die Fortbewegung der Thiere an senkrechten, glatten Flächen vermittelst eines Secretes. Pflüger Arch Gesammte Physiol Menschen Thiere 33, 440–481. doi: 10.1007/BF01628473
Dhong, C., and Fréchette, J. (2015). Coupled effects of applied load and surface structure on the viscous forces during peeling. Soft Matter 11, 1901–1910. doi: 10.1039/C4SM02616K
Dingley, A. J., and Grzesiek, S. (1998). Direct observation of hydrogen bonds in nucleic acid base pairs by internucleotide2JNNcouplings. J. Am. Chem. Soc. 120, 8293–8297. doi: 10.1021/ja981513x
Dirks, J. H., and Federle, W. (2011). Fluid-based adhesion in insects – principles and challenges. Soft Matter. 7:11047. doi: 10.1039/c1sm06269g
Dixon, A. F. G., Croghan, P. C., and Gowing, R. P. (1990). The mechanism by which aphids adhere to smooth surfaces. J. Exp. Biol. 152, 243–253. doi: 10.1136/bmj.300.6719.253
Dong, X., Zhang, R., Tian, Y., Ramos, M. A., Hu, T. S., Wang, Z., et al. (2020). Functionally graded gecko setae and the biomimics with robust adhesion and durability. ACS Appl. Polymer Mater. 2, 2658–2666. doi: 10.1021/acsapm.0c00282
Dong, X., Zhao, H., Wang, Z., Ouzounian, M., Hu, T. S., Guo, Y., et al. (2019). Gecko-inspired composite micro-pillars with both robust adhesion and enhanced dry self-cleaning property. Chin. Chem. Lett. 30, 2333–2337. doi: 10.1016/j.cclet.2019.07.007
Drotlef, D.-M., Blümler, P., and del Campo, A. (2013). Magnetically actuated patterns for bioinspired reversible adhesion (Dry and Wet). Adv. Mater. 26, 775–779. doi: 10.1002/adma.201303087
Drotlef, D.-M., Dayan, C. B., and Sitti, M. (2019). Bio-inspired composite microfibers for strong and reversible adhesion on smooth surfaces. Integr. Comp. Biol. 59, 227–235. doi: 10.1093/icb/icz009
Drotlef, D. M., Stepien, L., Kappl, M., Barnes, W. J. P., Butt, H. J., and del Campo, A. (2012). Insights into the adhesive mechanisms of tree frogs using artificial mimics. Adv. Funct. Mater. 23, 1137–1146. doi: 10.1002/adfm.201202024
Eisner, T., and Aneshansley, D. J. (2000). Defense by foot adhesion in a beetle (Hemisphaerota cyanea). Proc. Natl. Acad. Sci. U.S.A. 97, 6568–6573. doi: 10.1073/pnas.97.12.6568
Emerson, S. B., and Diehl, D. (1980). Toe pad morphology and mechanisms of sticking in frogs. Biol. J. Linnean Soc. 13, 199–216. doi: 10.1111/j.1095-8312.1980.tb00082.x
Fan, X., Zhou, W., Chen, Y., Yan, L., Fang, Y., and Liu, H. (2020). An antifreezing/antiheating hydrogel containing catechol derivative urushiol for strong wet adhesion to various substrates. ACS Appl. Mater. Interfaces 12, 32031–32040. doi: 10.1021/acsami.0c09917
Federle, W., Barnes, W. J., Baumgartner, W., Drechsler, P., and Smith, J. (2006). Wet but not slippery: boundary friction in tree frog adhesive toe pads. J. R. Soc. Interface 3, 689–697. doi: 10.1098/rsif.2006.0135
Federle, W., Maschwitz, U., Fiala, B., Riederer, M., and Hölldobler, B. (1997). Slippery ant-plants and skilful climbers: selection and protection of specific ant partners by epicuticular wax blooms in Macaranga (Euphorbiaceae). Oecologia 112, 217–224. doi: 10.1007/s004420050303
Federle, W., Rohrseitz, K., and Holldobler, B. (2000). Attachment forces of ants measured with a centrifuge: better ‘wax-runners' have a poorer attachment to a smooth surface. J. Exp. Biol. 203, 505–512. doi: 10.1079/9780851994321.0000
Fenner, M. (Ed.). (2000). “Animals as seed dispersers,” in The Seeds: The Ecology of Regeneration in Plant Communities (Wallingford: Cabi Publishing), 111–124.
Fischer, S. G., and Lerman, L. S. (1983). DNA fragments differing by single base-pair substitutions are separated in denaturing gradient gels: correspondence with melting theory. Proc. Natl. Acad. Sci. U.S.A. 80, 1579–1583. doi: 10.1073/pnas.80.6.1579
Flenner, S., Schaber, C. F., Krasnov, I., Stieglitz, H., Rosenthal, M., Burghammer, M., et al. (2020). Multiple mechanical gradients are responsible for the strong adhesion of spider attachment hair. Adv. Mater. 32:2002758. doi: 10.1002/adma.202002758
Gao, H., Wang, X., Yao, H., Gorb, S., and Arzt, E. (2005). Mechanics of hierarchical adhesion structures of geckos. Mech. Mater. 37, 275–285. doi: 10.1016/j.mechmat.2004.03.008
Gao, J., Liu, Y., Xu, H., Wang, Z., and Zhang, X. (2010). Biostructure-like surfaces with thermally responsive wettability prepared by temperature-induced phase separation micromolding. Langmuir 26, 9673–9676. doi: 10.1021/la100256b
Geim, A. K., Dubonos, S. V., Grigorieva, I. V., Novoselov, K. S., Zhukov, A. A., and Shapoval, S. Y. (2003). Microfabricated adhesive mimicking gecko foot-hair. Nat. Mater. 2, 461–463. doi: 10.1038/nmat917
Girod, P. (1884). Recherches sur la peau des céphalopodes. La ventouse. Arch. Zool. Exp. Gén. 2, 379–401.
Gorb, S., and Beutel, R. (2001). Evolution of locomotory attachment pads of hexapods. Naturwissenschaften 88, 530–534. doi: 10.1007/s00114-001-0274-y
Gorb, S. N. (1999). Ultrastructure of the thoracic dorso-medial field (TDM) in the elytra-to-body arresting mechanism in Tenebrionid Beetles (Coleoptera: Tenebrionidae). J. Morphol. 240, 101–113.
Gorb, S. N. (2001). Attachment Devices of Insect Cuticle. Berlin; Heidelberg: Springer Science and Business Media.
Gorb, S. N. (2008). Biological attachment devices: exploring nature's diversity for biomimetics. Philos. Trans. R. Soc. A Math. Phys. Eng. Sci. 366, 1557–1574. doi: 10.1098/rsta.2007.2172
Gorb, S. N., Beutel, R. G., Gorb, E. V., Jiao, Y., Kastner, V., Niederegger, S., et al. (2002). Structural design and biomechanics of friction-based releasable attachment devices in insects. Integr. Comp. Biol. 42, 1127–1139. doi: 10.1093/icb/42.6.1127
Gorb, S. N., and Popov, V. L. (2002). Probabilistic fasteners with parabolic elements: biological system, artificial model and theoretical considerations. Philos. Trans. R. Soc. Lond. A Math. Phys. Eng. Sci. 360, 211–225. doi: 10.1098/rsta.2001.0926
Graule, M. A., Chirarattananon, P., Fuller, S. B., Jafferis, N. T., Ma, K. Y., Spenko, M., et al. (2016). Perching and takeoff of a robotic insect on overhangs using switchable electrostatic adhesion. Science 352, 978–982. doi: 10.1126/science.aaf1092
Graziadei, P. P. C., and Gagne, H. T. (1976a). An unusual receptor in the octopus. Tissue Cell 8, 229–240. doi: 10.1016/0040-8166(76)90049-5
Graziadei, P. P. C., and Gagne, H. T. (1976b). Sensory innervation in the rim of the octopus sucker. J. Morphol. 150, 639–679. doi: 10.1002/jmor.1051500304
Guéron, M., Kochoyan, M., and Leroy, J. L. (1987). A single mode of DNA base-pair opening drives imino proton exchange. Nature 328, 89–92. doi: 10.1038/328089a0
Hansen, W. R., and Autumn, K. (2005). Evidence for self-cleaning in gecko setae. Proc. Natl. Acad. Sci. U.S.A. 102, 385–389. doi: 10.1073/pnas.0408304102
Heinzmann, C., Weder, C., and de Espinosa, L. M. (2016). Supramolecular polymer adhesives: advanced materials inspired by nature. Chem. Soc. Rev. 45, 342–358. doi: 10.1039/C5CS00477B
Hou, H., Kim, W., Grunlan, M., and Han, A. (2009). A thermoresponsive hydrogel poly(N-isopropylacrylamide) micropatterning method using microfluidic techniques. J. Micromech. Microeng. 19:127001. doi: 10.1088/0960-1317/19/12/127001
Hou, J., Wright, E., Bonser, R. H. C., and Jeronimidis, G. (2012). Development of biomimetic squid-inspired suckers. J. Bionic Eng. 9, 484–493. doi: 10.1016/S1672-6529(11)60144-3
Hu, S., Lopez, S., Niewiarowski, P. H., and Xia, Z. (2012). Dynamic self-cleaning in gecko setae via digital hyperextension. J. R. Soc. Interface 9, 2781–2790. doi: 10.1098/rsif.2012.0108
Iturri, J., Xue, L., Kappl, M., García-Fernández, L., Barnes, W. J. P., Butt, H. J., et al. (2015). Torrent frog-inspired adhesives: attachment to flooded surfaces. Adv. Funct. Mater. 25, 1499–1505. doi: 10.1002/adfm.201403751
Jeffrey, G. A., and Saenger, W. (2012). Hydrogen Bonding in Biological Structures. Berlin; Heidelberg: Springer Science and Business Media.
Jeong, H. E., Lee, J.-K., Kim, H. N., Moon, S. H., and Suh, K. Y. (2009). A nontransferring dry adhesive with hierarchical polymer nanohairs. Proc. Natl. Acad. Sci. U.S.A. 106, 5639–5644. doi: 10.1073/pnas.0900323106
Jiao, J., Zhang, F., Jiao, T., Gu, Z., and Wang, S. (2018). Bioinspired superdurable pestle-loop mechanical interlocker with tunable peeling force, strong shear adhesion, and low noise. Adv. Sci. 5:1700787. doi: 10.1002/advs.201700787
Kamperman, M., Kroner, E., del Campo, A., McMeeking, R. M., and Arzt, E. (2010). Functional adhesive surfaces with “gecko” effect: the concept of contact splitting. Adv. Eng. Mater. 12, 335–348. doi: 10.1002/adem.201000104
Kim, S., Jiang, Y., Thompson Towell, K. L., Boutilier, M. S. H., Nayakanti, N., Cao, C., et al. (2019). Soft nanocomposite electroadhesives for digital micro- and nanotransfer printing. Sci. Adv. 5:eaax4790. doi: 10.1126/sciadv.aax4790
Kim, S., Spenko, M., Trujillo, S., Heyneman, B., Mattoli, V., and Cutkosky, M. R. (2007). “Whole body adhesion: hierarchical, directional and distributed control of adhesive forces for a climbing robot,” in Proceedings 2007 IEEE International Conference on Robotics and Automation (New York, NY), 1268–1273.
Kizilkan, E., Strueben, J., Staubitz, A., and Gorb, S. N. (2017). Bioinspired photocontrollable microstructured transport device. Sci. Robot. 2:eaak9454. doi: 10.1126/scirobotics.aak9454
Ko, H., Lee, J., Schubert, B. E., Chueh, Y. L., Leu, P. W., Fearing, R. S., et al. (2009). Hybrid core–shell nanowire forests as self-selective chemical connectors. Nano Lett. 9, 2054–2058. doi: 10.1021/nl900343b
Kuba, M. J., Byrne, R. A., Meisel, D. V., and Mather, J. A. (2006). Exploration and habituation in intact free moving Octopus vulgaris. Int. J. Comp. Psychol. 19, 426–438.
Kwon, J., Cheung, E., Park, S., and Sitti, M. (2006). Friction enhancement via micro-patterned wet elastomer adhesives on small intestinal surfaces. Biomed. Mater. 1, 216–220. doi: 10.1088/1748-6041/1/4/007
Labonte, D., Clemente, C. J., Dittrich, A., Kuo, C. Y., Crosby, A. J., Irschick, D. J., et al. (2016). Extreme positive allometry of animal adhesive pads and the size limits of adhesion-based climbing. Proc. Natl. Acad. Sci. U.S.A. 113, 1297–1302. doi: 10.1073/pnas.1519459113
Labonte, D., and Federle, W. (2015). Rate-dependence of “wet” biological adhesives and the function of the pad secretion in insects. Soft Matter 11, 8661–8673. doi: 10.1039/C5SM01496D
Langer, M. G., Ruppersberg, J. P., and Gorb, S. (2004). Adhesion forces measured at the level of a terminal plate of the fly's seta. Proc. R. Soc. Lon. B Biol. Sci. 271, 2209–2215. doi: 10.1098/rspb.2004.2850
Lee, H., Lee, B. P., and Messersmith, P. B. (2007). A reversible wet/dry adhesive inspired by mussels and geckos. Nature 448, 338–341. doi: 10.1038/nature05968
Lee, H., Um, D. S., Lee, Y., Lim, S., Kim, H., and Ko, H. (2016). Octopus-inspired smart adhesive pads for transfer printing of semiconducting nanomembranes. Adv. Mater. 28, 7457–7465. doi: 10.1002/adma.201601407
Lee, J., and Fearing, R. S. (2008). Contact self-cleaning of synthetic gecko adhesive from polymer microfibers. Langmuir 24, 10587–10591. doi: 10.1021/la8021485
Lee, J., Fearing, R. S., and Komvopoulos, K. (2008). Directional adhesion of gecko-inspired angled microfiber arrays. Appl. Phys. Lett. 93:191910. doi: 10.1063/1.3006334
Linghu, C., Wang, C., Cen, N., Wu, J., Lai, Z., and Song, J. (2019). Rapidly tunable and highly reversible bio-inspired dry adhesion for transfer printing in air and a vacuum. Soft Matter 15, 30–37. doi: 10.1039/C8SM01996G
Liu, Q., Meng, F., Wang, X., Yang, B., Tan, D., Li, Q., et al. (2020). Tree frog-inspired micropillar arrays with nanopits on the surface for enhanced adhesion under wet conditions. ACS Appl. Mater. Interfaces 12, 19116–19122. doi: 10.1021/acsami.9b22532
Matsubara, K., Watanabe, M., and Takeoka, Y. (2007). A thermally adjustable multicolor photochromic hydrogel. Angew. Chem. 119, 1718–1722. doi: 10.1002/ange.200603554
Mengüç, Y., Yang, S. Y., Kim, S., Rogers, J. A., and Sitti, M. (2012). Gecko-inspired controllable adhesive structures applied to micromanipulation. Adv. Funct. Mater. 22, 1246–1254. doi: 10.1002/adfm.201101783
Menon, C., Murphy, M., and Sitti, M. (2004). “Gecko inspired surface climbing robots,” In 2004 IEEE International Conference on Robotics and Biomimetics (New York, NY), 431–436.
Menon, C., and Sitti, M. (2006). A biomimetic climbing robot based on the gecko. J. Bionic Eng. 3, 115–125. doi: 10.1016/S1672-6529(06)60015-2
Mohammadi Nasab, A., Luo, A., Sharifi, S., Turner, K. T., and Shan, W. (2020). Switchable adhesion via subsurface pressure modulation. ACS Appl. Mater. Interfaces 12, 27717–27725. doi: 10.1021/acsami.0c05367
Montero de Espinosa, L., Meesorn, W., Moatsou, D., and Weder, C. (2017). Bioinspired polymer systems with stimuli-responsive mechanical properties. Chem. Rev. 117, 12851–12892. doi: 10.1021/acs.chemrev.7b00168
Murarash, B., Itovich, Y., and Varenberg, M. (2011). Tuning elastomer friction by hexagonal surface patterning. Soft Matter 7:5553. doi: 10.1039/c1sm00015b
Murphy, M. P., Aksak, B., and Sitti, M. (2007). Adhesion and anisotropic friction enhancements of angled heterogeneous micro-fiber arrays with spherical and spatula tips. J. Adhes. Sci. Technol. 21, 1281–1296. doi: 10.1163/156856107782328380
Murphy, M. P., Aksak, B., and Sitti, M. (2008). Gecko-inspired directional and controllable adhesion. Small 5, 170–175. doi: 10.1002/smll.200801161
Murphy, M. P., Kim, S., and Sitti, M. (2009). Enhanced adhesion by gecko-inspired hierarchical fibrillar adhesives. ACS Appl. Mater. Interfaces 1, 849–855. doi: 10.1021/am8002439
Murphy, M. P., Kute, C., Meng,üç, Y., and Sitti, M. (2010). Waalbot II: adhesion recovery and improved performance of a climbing robot using fibrillar adhesives. Int. J. Rob. Res. 30, 118–133. doi: 10.1177/0278364910382862
Nachtigall, W. (2013). Biological Mechanisms of Attachment: The Comparative Morphology and Bioengineering of Organs for Linkage, Suction, and Adhesion. Berlin; Heidelberg: Springer Science and Business Media.
Narkar, A. R., Barker, B., Clisch, M., Jiang, J., and Lee, B. P. (2016). pH responsive and oxidation resistant wet adhesive based on reversible catechol–boronate complexation. Chem. Mater. 28, 5432–5439. doi: 10.1021/acs.chemmater.6b01851
Narkar, A. R., and Lee, B. P. (2018). Incorporation of anionic monomer to tune the reversible catechol–boronate complex for ph-responsive, reversible adhesion. Langmuir 34, 9410–9417. doi: 10.1021/acs.langmuir.8b00373
Niederegger, S., and Gorb, S. (2003). Tarsal movements in flies during leg attachment and detachment on a smooth substrate. J. Insect Physiol. 49, 611–620. doi: 10.1016/S0022-1910(03)00048-9
Nishida, J., Kobayashi, M., and Takahara, A. (2013). Light-triggered adhesion of water-soluble polymers with a caged catechol group. ACS Macro Lett. 2, 112–115. doi: 10.1021/mz300524q
Nixon, M., and Dilly, P. N. (1977). Sucker surfaces and prey capture. Symp. Zool. Soc. Lond. 38, 447–511.
Nørgaard, T., and Dacke, M. (2010). Fog-basking behaviour and water collection efficiency in Namib Desert Darkling beetles. Front. Zool. 7:23. doi: 10.1186/1742-9994-7-23
Ohzono, T., Saed, M. O., and Terentjev, E. M. (2019). Enhanced dynamic adhesion in nematic liquid crystal elastomers. Adv. Mater. 31:1902642. doi: 10.1002/adma.201902642
Packard, A., Trueman, E. R., and Clarke, M. R. (1988). The skin of cephalopods (Coleoids): general and special adaptations. Form Funct. 3, 37–67.
Panchireddy, S., Grignard, B., Thomassin, J.-M., Jerome, C., and Detrembleur, C. (2018). Catechol containing polyhydroxyurethanes as high-performance coatings and adhesives. ACS Sustain. Chem. Eng. 6, 14936–14944. doi: 10.1021/acssuschemeng.8b03429
Pang, C., Kang, D., Kim, T., and Suh, K. Y. (2011a). Analysis of preload-dependent reversible mechanical interlocking using beetle-inspired wing locking device. Langmuir 28, 2181–2186. doi: 10.1021/la203853r
Pang, C., Kim, S. M., Rahmawan, Y., and Suh, K. Y. (2012). Beetle-inspired bidirectional, asymmetric interlocking using geometry-tunable nanohairs. ACS Appl. Mater. Interfaces 4, 4225–4230. doi: 10.1021/am3009289
Pang, C., Kim, T., Bae, W. G., Kang, D., Kim, S. M., and Suh, K. Y. (2011b). Bioinspired reversible interlocker using regularly arrayed high aspect-ratio polymer fibers. Adv. Mater. 24, 475–479. doi: 10.1002/adma.201103022
Park, H.-H., Seong, M., Sun, K., Ko, H., Kim, S. M., and Jeong, H. E. (2017). Flexible and shape-reconfigurable hydrogel interlocking adhesives for high adhesion in wet environments based on anisotropic swelling of hydrogel microstructures. ACS Macro Lett. 6, 1325–1330. doi: 10.1021/acsmacrolett.7b00829
Peisker, H., Michels, J., and Gorb, S. N. (2013). Evidence for a material gradient in the adhesive tarsal setae of the ladybird beetle Coccinella septempunctata. Nat. Commun. 4, 1–7. doi: 10.1038/ncomms2576
Persson, B. N. J. (2007). Wet adhesion with application to tree frog adhesive toe pads and tires. J. Phys. Condensed Matter 19:376110. doi: 10.1088/0953-8984/19/37/376110
Pilkington, G. A., Gupta, R., and Fréchette, J. (2016). Scaling hydrodynamic boundary conditions of microstructured surfaces in the thin channel limit. Langmuir 32, 2360–2368. doi: 10.1021/acs.langmuir.5b04134
Purtov, J., Frensemeier, M., and Kroner, E. (2015). Switchable adhesion in vacuum using bio-inspired dry adhesives. ACS Appl. Mater. Interfaces 7, 24127–24135. doi: 10.1021/acsami.5b07287
Qu, L., Dai, L., Stone, M., Xia, Z., and Wang, Z. L. (2008). Carbon nanotube arrays with strong shear binding-on and easy normal lifting-off. Science 322, 238–242. doi: 10.1126/science.1159503
Reddy, S., Arzt, E., and del Campo, A. (2007). Bioinspired surfaces with switchable adhesion. Adv. Mater. 19, 3833–3837. doi: 10.1002/adma.200700733
Ruibal, R., and Ernst, V. (1965). The structure of the digital setae of lizards. J. Morphol. 117, 271–293. doi: 10.1002/jmor.1051170302
Russell, A. P. (1975). A contribution to the functional analysis of the foot of the Tokay, Gekko gecko (Reptilia: Gekkonidae). J. Zool. 176, 437–476. doi: 10.1111/j.1469-7998.1975.tb03215.x
Russew, M. M., and Hecht, S. (2010). Photoswitches: from molecules to materials. Adv. Mater. 22, 3348–3360. doi: 10.1002/adma.200904102
Saenger, W. (1984). Forces stabilizing associations between bases: hydrogen bonding and base stacking. Springer Adv. Texts Chem. 116–158.
Samuelson, G. A. (1994). “An elytron to body meshing mechanism of possible significance in the higher classification of Chrysomelidae (Coleoptera),” in Anonymous, Proceedings of the Third International Symposium on the Chrysomelidae, Leiden: Backhuys Publishers, 136–147.
Samuelson, G. A. (1996). “Binding sites: elytron-to-body meshing structures of possible significance in the higher classification of Chrysomeloidea,” in Biology, The Classification, Phylogeny and Genetics, eds P. H. A. Jolivet and M. L. Cox (Amsterdam: SPB Academic Publishing), 267–290.
Scholz, I., Barnes, W. J. P., Smith, J. M., and Baumgartner, W. (2009). Ultrastructure and physical properties of an adhesive surface, the toe pad epithelium of the tree frog, Litoria caerulea White. J. Exp. Biol. 212, 155–162. doi: 10.1242/jeb.019232
Sethi, S., Ge, L., Ci, L., Ajayan, P. M., and Dhinojwala, A. (2008). Gecko-inspired carbon nanotube-based self-cleaning adhesives. Nano Lett. 8, 822–825. doi: 10.1021/nl0727765
Shafiq, Z., Cui, J., Pastor-Pérez, L., San Miguel, V., Gropeanu, R. A., Serrano, C., et al. (2012). Bioinspired underwater bonding and debonding on demand. Angew. Chem. 124, 4408–4411. doi: 10.1002/ange.201108629
Shao, D., Chen, J., Ji, A., Dai, Z., and Manoonpong, P. (2020). “Hybrid soft-rigid foot with dry adhesive material designed for a gecko-inspired climbing robot,” in 2020 3rd IEEE International Conference on Soft Robotics (New York, NY: RoboSoft).
Shao, H., and Stewart, R. J. (2010). Biomimetic underwater adhesives with environmentally triggered setting mechanisms. Adv. Mater. 22, 729–733. doi: 10.1002/adma.200902380
Smith, A. M. (1991). Negative pressure generated by octopus suckers: a study of the tensile strength of water in nature. J. Exp. Biol. 157, 257–271.
Sorensen, A. E. (1986). Seed dispersal by adhesion. Annu. Rev. Ecol. Syst. 17, 443–463. doi: 10.1146/annurev.es.17.110186.002303
Sparks, B. J., Hoff, E. F. T., Hayes, L. P., and Patton, D. L. (2012). Mussel-inspired thiol–ene polymer networks: influencing network properties and adhesion with catechol functionality. Chem. Mater. 24, 3633–3642. doi: 10.1021/cm302301e
Stratakis, E. (2020). Laser engineering of biomimetic surfaces. Mater. Sci. Eng. R Rep. 141:100562. doi: 10.1016/j.mser.2020.100562
Stratakis, E., Ranella, A., and Fotakis, C. (2011). Biomimetic micro/nanostructured functional surfaces for microfluidic and tissue engineering applications. Biomicrofluidics 5:013411. doi: 10.1063/1.3553235
Sun, T., and Qing, G. (2011). Biomimetic smart interface materials for biological applications. Adv. Mater. 23, H57–H77. doi: 10.1002/adma.201004326
Tamelier, J., Chary, S., and Turner, K. L. (2012). Vertical anisotropic microfibers for a gecko-inspired adhesive. Langmuir 28, 8746–8752. doi: 10.1021/la3004855
Testa, P., Chappuis, B., Kistler, S., Style, R. W., Heyderman, L. J., and Dufresne, E. R. (2020). Switchable adhesion of soft composites induced by a magnetic field. Soft Matter 16, 5806–5811. doi: 10.1039/D0SM00626B
Tian, Y., Pesika, N., Zeng, H., Rosenberg, K., Zhao, B., McGuiggan, P., et al. (2006). Adhesion and friction in gecko toe attachment and detachment. Proc. Natl. Acad. Sci. U.S.A. 103, 19320–19325. doi: 10.1073/pnas.0608841103
Tramacere, F., Beccai, L., Mattioli, F., Sinibaldi, E., and Mazzolai, B. (2012). “Artificial adhesion mechanisms inspired by octopus suckers,” in 2012 IEEE International Conference on Robotics and Automation (New York, NY), 3846–3851.
Viswanathan, K., Ozhalici, H., Elkins, C. L., Heisey, C., Ward, T. C., and Long, T. E. (2006). Multiple hydrogen bonding for reversible polymer surface adhesion. Langmuir 22, 1099–1105. doi: 10.1021/la052253h
Vogel, M. J., and Steen, P. H. (2010). Capillarity-based switchable adhesion. Proc. Natl. Acad. Sci. U.S.A. 107, 3377–3381. doi: 10.1073/pnas.0914720107
Von Marilaun, A. K. (1895). The Natural History of Plants, Their Forms, Growth, Reproduction, and Distribution: From the German of Anton Kerner Von Marilaun, Vol. 4. London: Blackie and Son.
Vötsch, W., Nicholson, G., Müller, R., Stierhof, Y. D., Gorb, S., and Schwarz, U. (2002). Chemical composition of the attachment pad secretion of the locust Locusta migratoria. Insect Biochem. Mol. Biol. 32, 1605–1613. doi: 10.1016/S0965-1748(02)00098-X
Waite, J. H. (2002). Adhesion a la Moule. Integr. Comp. Biol. 42, 1172–1180. doi: 10.1093/icb/42.6.1172
Waite, J. H., Andersen, N. H., Jewhurst, S., and Sun, C. (2005). Mussel adhesion: finding the tricks worth mimicking. J. Adhes. 81, 297–317. doi: 10.1080/00218460590944602
Wan, Y., Gao, Y., and Xia, Z. (2019). Highly switchable adhesion of n-doped graphene interfaces for robust micromanipulation. ACS Appl. Mater. Interfaces 11, 5544–5553. doi: 10.1021/acsami.8b18793
Wan, Y., and Xia, Z. (2018). Self-cleaning and controlled adhesion of gecko feet and their bioinspired micromanipulators. MRS Adv. 3, 1641–1646. doi: 10.1557/adv.2018.51
Wang, J., Hu, J., Wen, Y., Song, Y., and Jiang, L. (2006). Hydrogen-bonding-driven wettability change of colloidal crystal films: from superhydrophobicity to superhydrophilicity. Chem. Mater. 18, 4984–4986. doi: 10.1021/cm061417s
West, T. (1861). XXV. The foot of the fly; its structure and action: elucidated by comparison with the feet of other insects, andc.-part I. Trans. Linnean Soc. Lond. 23, 393–421. doi: 10.1111/j.1096-3642.1860.tb00139.x
Willson, M. F., Rice, B. L., and Westoby, M. (1990). Seed dispersal spectra: a comparison of temperate plant communities. J. Veget. Sci. 1, 547–562. doi: 10.2307/3235789
Xia, F., Feng, L., Wang, S., Sun, T., Song, W., Jiang, W., et al. (2006). Dual-responsive surfaces that switch between superhydrophilicity and superhydrophobicity. Adv. Mater. 18, 432–436. doi: 10.1002/adma.200501772
Xu, Q., Wan, Y., Hu, T. S., Liu, T. X., Tao, D., Niewiarowski, P. H., et al. (2015). Robust self-cleaning and micromanipulation capabilities of gecko spatulae and their bio-mimics. Nat. Commun. 6:8949. doi: 10.1038/ncomms9949
Xue, L., Kovalev, A., Dening, K., Eichler-Volf, A., Eickmeier, H., Haase, M., et al. (2013). Reversible adhesion switching of porous fibrillar adhesive pads by humidity. Nano Lett. 13, 5541–5548. doi: 10.1021/nl403144w
Ye, Z., Lum, G. Z., Song, S., Rich, S., and Sitti, M. (2016). Phase change of gallium enables highly reversible and switchable adhesion. Adv. Mater. 28, 5088–5092. doi: 10.1002/adma.201505754
Yi, H., Seong, M., Sun, K., Hwang, I., Lee, K., Cha, C., et al. (2018). Wet-responsive, reconfigurable, and biocompatible hydrogel adhesive films for transfer printing of nanomembranes. Adv. Funct. Mater. 28:1706498. doi: 10.1002/adfm.201706498
Yoon, C. (2019). Advances in biomimetic stimuli responsive soft grippers. Nano Convergence 6:20. doi: 10.1186/s40580-019-0191-4
Zhao, B., Pesika, N., Zeng, H., Wei, Z., Chen, Y., Autumn, K., et al. (2009). Role of tilted adhesion fibrils (Setae) in the adhesion and locomotion of Gecko-like systems†. J. Phys. Chem. B 113, 3615–3621. doi: 10.1021/jp806079d
Zhao, Y., Wu, Y., Wang, L., Zhang, M., Chen, X., Liu, M., et al. (2017). Bio-inspired reversible underwater adhesive. Nat. Commun. 8:2218. doi: 10.1038/s41467-017-02387-2
Keywords: bioinspired materials, switchable adhesion, bioprototype, gecko, stimuli-responsive
Citation: Wang J, Wan Y, Wang X and Xia Z (2021) Bioinspired Smart Materials With Externally-Stimulated Switchable Adhesion. Front. Nanotechnol. 3:667287. doi: 10.3389/fnano.2021.667287
Received: 12 February 2021; Accepted: 15 March 2021;
Published: 08 September 2021.
Edited by:
Ruchi Agrawal, TERI Gram, Gurugram, Haryana 122003, IndiaReviewed by:
Emmanuel Stratakis, Foundation for Research and Technology Hellas (FORTH), GreeceHoon Eui Jeong, Ulsan National Institute of Science and Technology, South Korea
Copyright © 2021 Wang, Wan, Wang and Xia. This is an open-access article distributed under the terms of the Creative Commons Attribution License (CC BY). The use, distribution or reproduction in other forums is permitted, provided the original author(s) and the copyright owner(s) are credited and that the original publication in this journal is cited, in accordance with accepted academic practice. No use, distribution or reproduction is permitted which does not comply with these terms.
*Correspondence: Zhenhai Xia, emhlbmhhaS54aWFAdW50LmVkdQ==
†These authors have contributed equally to this work