- 1Center for Biomaterials and Tissue Engineering, Universitat Politècnica de València, Valencia, Spain
- 2Department of Medicine, Universitat Jaume I, Castellón de la Plana, Spain
- 3Networking Research Center on Bioengineering, Biomaterials and Nanomedicine, Valencia, Spain
The therapy of neural nerve injuries that involve the disruption of axonal pathways or axonal tracts has taken a new dimension with the development of tissue engineering techniques. When peripheral nerve injury (PNI), spinal cord injury (SCI), traumatic brain injury (TBI), or neurodegenerative disease occur, the intricate architecture undergoes alterations leading to growth inhibition and loss of guidance through large distance. To improve the limitations of purely cell-based therapies, the neural tissue engineering philosophy has emerged. Efforts are being made to produce an ideal scaffold based on synthetic and natural polymers that match the exact biological and mechanical properties of the tissue. Furthermore, through combining several components (biomaterials, cells, molecules), axonal regrowth is facilitated to obtain a functional recovery of the neural nerve diseases. The main objective of this review is to investigate the recent approaches and applications of neural tissue engineering approaches.
Introduction
Injuries to the nervous system that involve the disruption of axonal bundles have a major impact on the population, on medical and healthcare, and social and economic field. Autonomous regeneration of damaged or degenerated axonal tracts is infrequent since a large number of factors are involved limiting this recovery.
Axon regeneration in the central nervous system (CNS) is severely restricted after traumatic brain injury (TBI), stroke, spinal cord injury (SCI), and related conditions that involve axonal disruption. In contrast, peripheral nervous system (PNS) axons can regenerate in some cases allowing functional recovery when the damage involves a relatively short distance (Manoli et al., 2009; Aijie et al., 2018). After damage, CNS axons have an intrinsically limited capability to regenerate and they are surrounded by a local inhibitory environment (Curcio and Bradke, 2018). Moreover, the loss of neuronal populations and synaptic connections is most irreversible due to the limited outgrowth capacity of mature neurons (y Cajal, 1930). The perineuronal network, including reactive and migrating cells to the injured area, and the chemical composition of the ECM at the formed scar, constitute part of the inhibitory factors (Busch and Silver, 2007). In axonal pathways, the axons of neurons form parallel bundles (nerves in the PNS, tracts in the CNS).
Nowadays, conventional medicine does not have effective and successful treatments for these injuries, and the treatment of symptoms is often the best solution. In order to reverse it and achieve the functional reconnection of neurons, tissue engineering currently opts for the use of biocompatible three-dimensional biomaterials, cells, and bioactive molecules in order to restore the integrity of damaged tissue, respecting the original anatomy as much as possible, while recovering its functionality. Biomaterials, both natural and synthetic, have shown consistently positive neural tissue engineering results, including neurite outgrowth, differentiation of human neural stem cells, and nerve gap bridging (Gonzalez-Perez et al., 2017; Yang et al., 2017; Zhou et al., 2017; Xue et al., 2018).
Specifically, one of the proposed neural tissue engineering strategies has been nerve guidance conduits, not only for peripheral nerve injuries but also for tracts of the CNS. A nerve conduit should provide a suitable environment for neuron survival and axonal extension, guide axonal projections, and mimic the biomechanics with adequate mechanical properties (Vijayavenkataraman, 2020). The use of biocompatible scaffolds represents a benefit when used as a vehicle with minimal tissue invasiveness in comparison with intraparenchymal transplantation approaches, conferring protection of the transplanted cells against the hostile environment generated at the injury site (Thompson et al., 2018). Different fabrication techniques are available to produce biomaterials that guide axonal outgrowth such as aligned scaffolds (Wu et al., 2018), fibers or filaments (McMurtrey, 2014), and tubular structures (Cullen et al., 2012; Kim et al., 2018; Sarker et al., 2018).
Cell Biology of the Nervous System
The nervous system is a complex assemblage of cells in charge of directing, supervising, and controlling all the functions and activities of our internal organs and body.
The nervous system is subdivided anatomically into the CNS and the PNS and functionally into the somatic nervous system and the autonomic nervous system (visceral). The CNS is formed of the brain and spinal cord. The PNS comprises the nerves emerging from the brain (named cranial nerves) and from the spinal cord (named spinal nerves).
The nervous systems integrate a lot of function like sensation and body movements, which can be divided into sensory or motor signals. The sensory signals are transmitted through the sensory or ascending pathway to processing centers in the spinal cord and brain, resulting in an appropriate response for motor activity. This response is carried through the nervous system to the muscles and glands along motor or descending pathways (Mai and Paxinos, 2012).
The primary type of cell in the nervous system is the neuron, which is the excitable cell type in charge of sending and receiving signals through action potentials. The neuron comprises four structurally defined regions: a cell body or soma (1) that extend a single nerve process named axon (2), which ends at presynaptic terminals (3) containing boutons, and a variable number of branching processes named dendrites (4) (Figure 1A). Neurons can be structurally classified in multipolar (one axon and multiple highly branched dendrites), bipolar (one axon and one dendrite), and pseudounipolar neurons (two axons: peripheral and central) (Noback et al., 1968) (Figure 1B) and functionally classified in sensory or afferent neurons (carry information toward the CNS), interneurons (relay messages within the CNS), and motor or efferent neurons (carry information away from the CNS to muscles and glands).
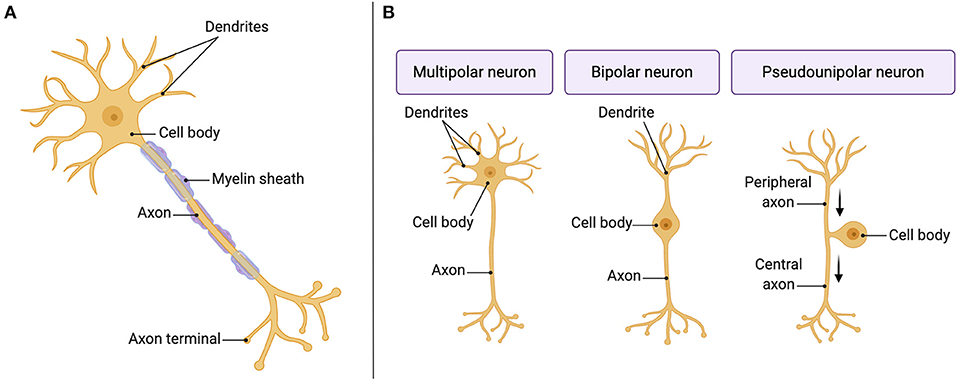
Figure 1. The neuron: the primary cell in the nervous system. (A) Structural parts of a neuron. (B) Different structural classes of neurons.
The other cell type in nervous tissue is the smaller and more prevalent neuroglia (glia), the supportive cells that surround the somas, axons, and dendrites of neurons in both the CNS and PNS (Mai and Paxinos, 2012).
The PNS glia consist of Schwann cells (SC) that surround neuron axon and perineuronal satellite cells surrounding the neuron cell body (Figure 2A) (Stierli et al., 2019). SC wrap themselves around a short segment of an axon many times as a sort of insulating blanket, creating a shiny white protective layer around the axon named myelin sheath and forming the myelinating axons. The unmyelinated axons are also encased in SC. Satellite cells are flat cells that enclose and support the cell bodies of neurons in the PNS. Both SC and satellite cells envelop and separate unmyelinated axons from each other and are located in the interneuronal space between neurons (Butt and Verkhratsky, 2018).
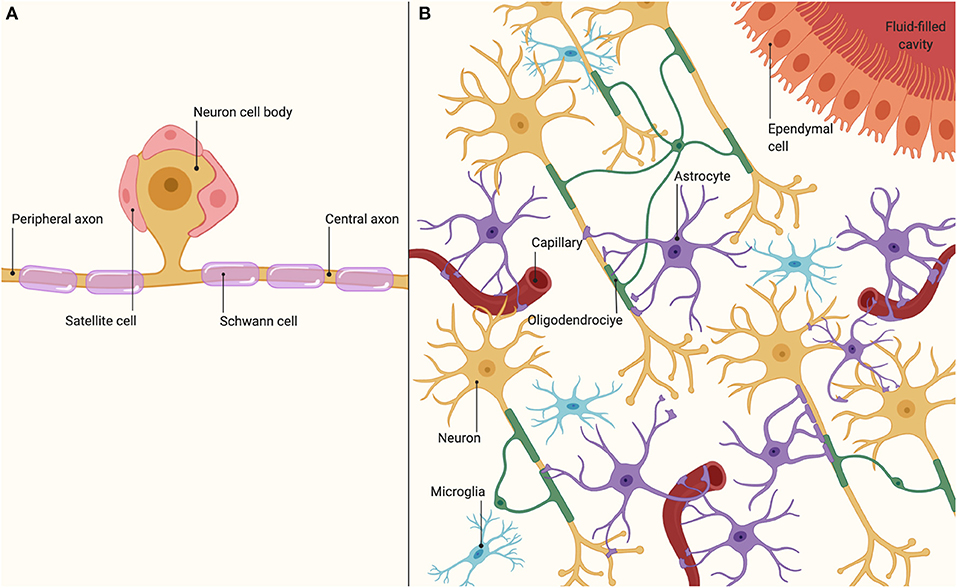
Figure 2. Nervous system cell organization. (A) Cells and organization in the peripheral nervous system. (B) Cells and organization in the central nervous system.
The CNS glia includes astrocytes (astroglia), oligodendrocytes (oligodendroglia), microglia, and ependymal cells (Figure 2B). The astrocytes have several processes and are in charge of anchoring capillaries and neurons cells bodies and dendrites in place, make contact with ependymal cells of the ventricular system, regulating the extracellular environment of the brain, aiding in the formation of the blood-brain barrier, and repairing damaged brain tissue (Jäkel and Dimou, 2017). The oligodendrocytes are the equivalents of SC of the PNS fabricating and maintaining CNS myelin, with the difference that a single oligodendrocyte can extend its processes wrapping around multiple axons (Kastriti and Adameyko, 2017). Microglial are cells with functions related to both immune response and maintaining homeostasis. The ependymal cells are cuboidal glial cells that line the central canal of the spinal cord and the ventricles of the brain, and they are involved in the production and circulation of cerebrospinal fluid (Butt and Verkhratsky, 2018).
In brief, there are many roles in which the glia is involved. Glial cells give the CNS structural support and protection for neuron networks and guide the developing migrating neurons from their sites of origin to their correct destination and the outgrowth of their axons. They also produce trophic and growth factors to the nervous system regeneration and plasticity and myelin sheaths to insulate the axon and increase the velocity of action potential propagation (oligodendrocytes in CNS and SC in PNS) (Jäkel and Dimou, 2017; Kastriti and Adameyko, 2017; Avraham et al., 2020; Bolívar et al., 2020). Microglia remove debris produced following injury or neuronal death and monitors the CNS, and astrocytes act as bridges that transport nutrients from the capillaries to the neurons and also proliferate to develop astrocytic scars to repair nervous tissue following an injury (reactive gliosis) (Mai and Paxinos, 2012; Butt and Verkhratsky, 2018).
Bundles of Axons in the Nervous System: Peripheral Nerves and Central Nervous System Tracts
Bundles of axons receive such specific names as nerve (in the PNS) and tract or fasciculus, among others (in the CNS).
Nerves contain only the axon part of the neuron. The cell bodies of sensory neurons are located in adjacent structures to the spinal cord [dorsal root ganglion (DRG)] or in cranial ganglia, while the cell bodies of motor neurons are within the CNS (spinal cord or brainstem) (Noback et al., 1968). Each nerve of the PNS consists of three essential tissue elements: axons, SC (and myelin sheaths), and connective tissue (endoneurium, perineurium, and epineurium) (Figure 3A). In the PNS, there are also ganglia, formed by cell bodies and satellite cells associated with the peripheral nerves. A peripheral nerve consists of numerous nerve fibers formed by axons and associated SC. Each axon–SC unit is surrounded by a basal lamina sheath composed by collagen, laminin, and fibronectin, and the endoneurium wraps all this structure. Groups of insulated nerve fibers are bound together into fascicles by the perineurium. In turn, groups of fascicles and blood vessels, which serve to nourish cells, are bound together by the epineurium. The myelin sheath, which surrounds an axon inside a nerve fiber, is a structure composed of many continuous spiral laminated layers of the plasma membrane and allows transmission of faster and more efficient electrochemical impulses. Nearly all nerve fibers over 3 μm in diameter are myelinated, and those under <3 μm are unmyelinated. Only one SC encapsulates a myelinated nerve fiber, but a group of unmyelinated fibers might share the same SC (Figure 3B) (Grinsell and Keating, 2014).
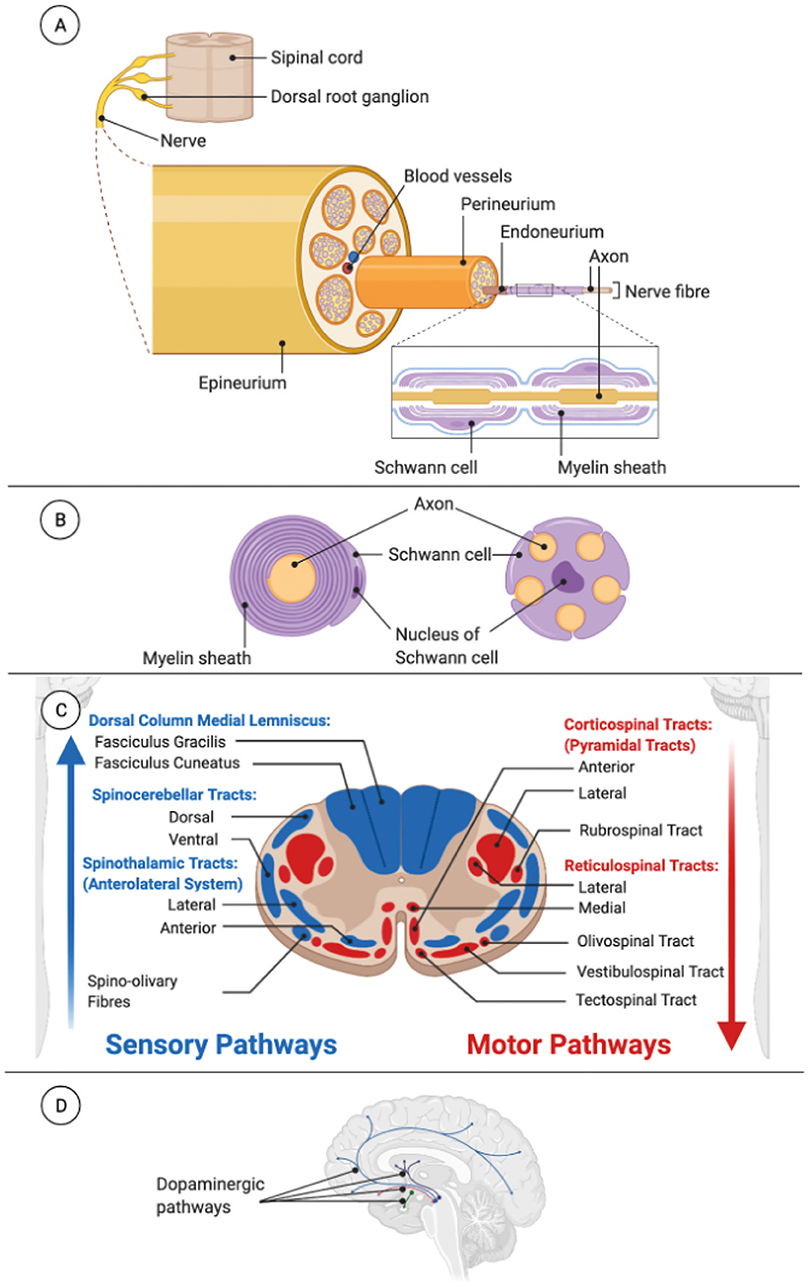
Figure 3. Bundles of axons in the nervous system. (A) Peripheral nerve anatomy. (B) Myelinated (left) and unmyelinated (right) nerve fibers in the peripheral nervous system. (C) Spinal cord tracts divided in sensory or ascending tracts (blue) and motor or descending tract (red) to illustrate the complexity of the tract organization in the spinal cord. (D) Dopaminergic pathways as an example of the large number of pathways inside the brain. Dopaminergic pathways are the bundles of neuron projections in the brain that synthesize and release the neurotransmitter dopamine. Alterations in these pathways may be involved in multiple diseases and disorders such as Parkinson's disease, addiction, or attention deficit hyperactivity disorder.
Bundle axons of the CNS are located in the white matter. Within the white matter of the spinal cord, the sensory fibers of the pathways form groups called ascending tracts or fasciculi, carrying information up to the brain. Fibers of the motor pathways form groups referred to as descending tracts, carrying information from the brain to the peripheral effectors (muscles and glands). So, the spinal cord consists of a large number of ascending and descending tracts, each located in particular parts of the dorsal, lateral, or ventral column of the white matter (Figure 3C) (Mai and Paxinos, 2012). In the brain, there are many axonal pathways. One example is the axonal pathways between the substantia nigra pars compacta (SNpc) and the striatum, which is altered in Parkinson's disease. SNpc neurons send long-projecting axons to the striatum, and in Parkinson's disease, there is a selective loss of the dopaminergic neurons, so this neurodegeneration deprives the striatum of crucial dopaminergic inputs and causes ineffective feedback of motor pathway (Davie, 2008) (Figure 3D).
In summary, axonal bundles represent an aligned and unidirectional architecture that makes axonal nervous tissue development possible. When peripheral nerve injury (PNI), SCI, TBI, or neurodegenerative disease occur, the intricate architecture undergoes alterations leading to growth inhibition and loss of guidance (Moon et al., 2000).
Regeneration in the Peripheral and Central Nervous System
Precisely because of all the marked differences between CNS and PNS discussed above, the mechanisms involved after a damage occurs and their potential for regeneration are very different (Kastriti and Adameyko, 2017). Moreover, the evolution and outcome of the regenerative situation may depend on very different factors.
Traumatic injuries, interruption of blood supply, or neurodegenerative diseases can damage axons, cell bodies, or somas in the nervous system and produce a disconnection of axonal bundles (Griffin et al., 2013; Hill et al., 2016; Anjum et al., 2020).
In injuries of the PNS, functional restoration following significant nerve lesions in length (>10 mm) is generally poor due to insufficient axonal reinnervation of distal targets (Lundborg et al., 1982; Pfister et al., 1987). The situation is less desperate when only the reconnection of the distal and proximal ends of the injured axonal bundle in relatively short distances is needed (Manoli et al., 2009; Aijie et al., 2018). In this situation, the distal part of the axon, which is disconnected from the cell body, undergoes Wallerian degeneration, resulting in fragmentation and disintegration of the axon in the first 18–48 h. SC surrounding the axon survive and produce proinflammatory cytokines such as tumor necrosis factor-alpha (TNF-α) and interleukin-1-alpha (IL-1α), playing a critical role in macrophage recruitment to the injury site (Shamash et al., 2002). After 1–2 weeks, reasonably fast as compared with the CNS, macrophages and SC remove axonal and myelin debris. Besides, macrophages secrete cytokines such as interleukin-1 (IL-1) and platelet-derived growth factor (PDGF) that stimulate SC to divide, de-differentiate, and proliferate distal to the injury. These stimulated SC are induced to secrete growth factors such as nerve growth factor (NGF) or insulin-like growth factor-1 (IGF-1), in order to improve neuron survival after axotomy and stimulate axons regeneration. It is in this moment when the Band Büngner is formed (Jessen and Mirsky, 2016). The Band Büngner consists in SC and their basal lamina conforming a cylinder that serves as a pathway to guide axon and their growth cones, from proximal end to distal end, across the gap. In optimal conditions, the growth cones can extend at a rate of 1–3 mm/day (Griffin et al., 2013).
Moreover, SC and regenerating axons express cell surface adhesion molecules like neural cell adhesion molecule (N-CAM) and N-cadherin, which promote regeneration (Wanner and Wood, 2002). Also, SC secrete neurotrophic factors that promote axonal elongation, the survival of injured neurons, and vascularization of the distal nerve. These factors include brain-derived neurotrophic factor (BDNF), NGF, neurotrophin-3 (NT-3), glial cell line-derived neurotrophic factor (GDNF), and vascular endothelial growth factor (VEGF) (Zhang et al., 2000; Gupta et al., 2005; Jessen and Mirsky, 2016). Finally, SC myelinate the regrowing axon forming new myelin sheaths (Dubový et al., 2014). Meanwhile, at the site of axonal damage, a motile growth cone-like structure is developed. Changes in gene expression and protein production occur within the cell body, and new proteins are transported to the growing axon tip, such as tubulin alpha 1 and the growth-associated protein GAP43 (GAP43), which is upregulating in both sensory and motor neurons, until axons reconnect with their targets (Figure 4A).
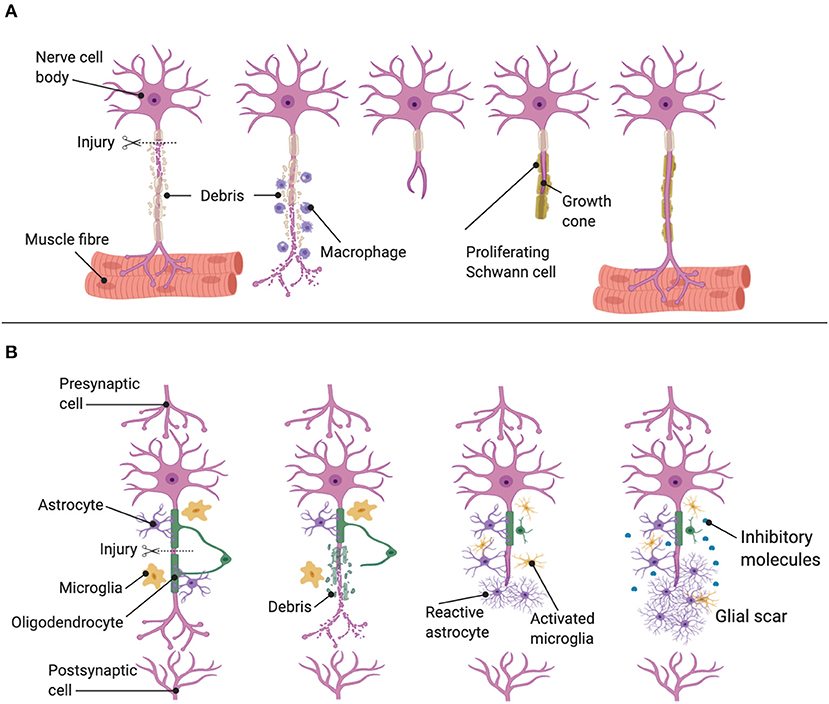
Figure 4. Regeneration mechanisms after injuries. (A) Regenerative situation in PNS. Proliferating SC and macrophages work together to remove myelin debris, release neurotrophins, and lead axons toward their synaptic targets, resulting in restored neuronal function. (B) Regenerative situation in CNS. The few neurons that survive axotomy attempt regeneration and subsequently meet an impenetrable glial scar composed myelin and cellular debris, as well as astrocytes, oligodendrocytes, and microglia.
The damage and regeneration produced in axonal tracts of the CNS are more complicated than in the PNS. David and Aguayo (Richardson et al., 1980; David and Aguayo, 1981) confirmed that CNS mature neurons could regenerate in the presence of permissive peripheral nerve grafts, suggesting that the peripheral nerve glial environment was permissive to central axonal regrowth. After these findings, the proregenerative environment in PNS and inhibitor factors in CNS for the axonal growth were identified. The inhibitor factors in the CNS include the limited intrinsic ability of CNS axons to regenerate (y Cajal, 1930; Goldberg et al., 2002), the long-distance required for regrowth, a local inhibitory environment (Curcio and Bradke, 2018), migrating cells to the injured area forming a glial scar, and the chemical composition of the extracellular matrix (ECM) at the formed scar (Busch and Silver, 2007; Paixão and Klein, 2010). After a CNS axon is injured, Wallerian degeneration in the damage tract occurs. However, the degenerating myelin and axonal debris after the apoptosis of oligodendrocytes persist much longer due to the insufficient recruitment of macrophages. These molecules that inhibit neurite growth are contained in myelin, for example, Nogo-A, myelin-associated glycoprotein (MAG), and oligodendrocyte myelin glycoprotein (OMgp). Nogo-A is a potent inhibitor of neurite growth and blocks axonal regeneration in the CNS. Moreover, a few hours after injury, microglia are the first glial cells to react. Astrocytes become reactive and collaborate in forming the glial scar, with inhibitory molecules, like proteoglycans, remaining for several weeks to months and complicating the axonal regeneration (Fitch and Silver, 2008) (Figure 4B).
Therefore, due to the limitations of the body to regenerate axonal bundles discussed above, it is necessary to develop a biomimetic approach that can establish a bridge across the lesion while providing optimal morphological, chemical, and biological signals for the restoration of nervous tissue.
Neural Tissue Engineering: Therapies for Tissue Engineering
With the development of the most precise cell analysis tools and the discovery that the cells of an adult organism maintained a certain capacity to generate new tissue, even in the CNS, a new approach emerged to try to technically deal with any damage or pathology: the tissue engineering. According to Langer and Vacanti (1993), tissue engineering consists of applying the fundamentals of biology and engineering to develop functional substitutes for damaged tissue, with three general components: scaffolds for cell transplantation and support, cells that can create a functional matrix, and bioactive factors that support and regulate the activity of cells (Figure 5). A functional substitute refers to an ideal solution that fully restores affected function, integrates as well as possible with the host's surrounding tissue, and lasts for the rest of the affected person's life.
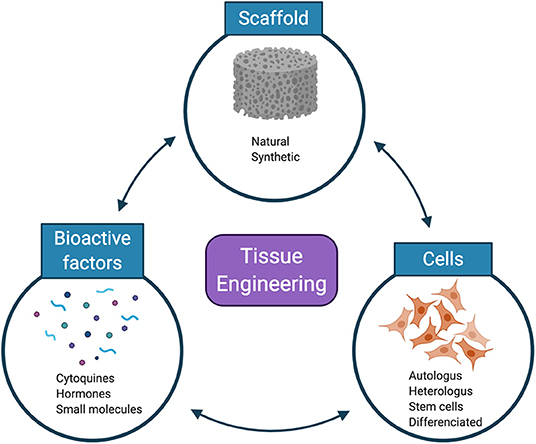
Figure 5. The tissue-engineering triad. Scaffolds, cells, and bioactive factors are used in isolation or in combination to recapitulate the desired tissue.
Tissue engineering therapies has obtained relevant advances in the reconstruction of useful and healthy tissue in practically all tissues and organs of the human body (Ramiya et al., 2000; L'Heureux et al., 2007; Gómez-Barrena et al., 2015) during the last 20 or 30 years. However, recovering lost connections in the nervous system has traditionally been a very complex challenge and in which researchers have had to be especially cautious and methodical.
Diseases and injuries of the human nervous system are devastating to the individual and have substantial societal implications and costs due to they are highly prevalent, affecting more than one billion people around the world (WHO, 2007).
As mentioned above, after an injury in the PNS, the situation for the regeneration is more straightforward than in the CNS. When the gap resulting from the injury is very small, nerve endings can rejoin with sutures (Triolo and Srivastava, 2019), but when this is not possible because of the high tension in the nerve endings the gold-standard traditional technique is the autograph, employing a nerve segment taken from a less important site from the same individual (Moore, 2014). Autograft offers encouraging results, and it is not easy to find a device that gives an equally satisfactory result (Schmidt and Leach, 2003), being currently used as the standard reference or positive control of other experimental solutions (Daly et al., 2013; Niu et al., 2014). However, autografts have limitations like the low availability of donor nerve (Ijkema-Paassen et al., 2004), the dimension mismatches (Nichols et al., 2004), host immune response, and the comorbidity associated with additional surgery (Deumens et al., 2010). Another traditional option is to use donor cadaveric nerve allografts or xenografts (from an animal), but, as with allograft, have limitations like requirement of immunosuppression or decellularization to prevent immune rejection (Platt et al., 1990; Evans et al., 1994; Ray and Mackinnon, 2010).
Others trials have been performed with other more abundant but similar tissues such as tendon collagen (Alberti et al., 2014), muscle fiber epimysium (Yang et al., 2013), or even CNS ECM (Crapo et al., 2012), to avoid healthy sectioning nerves. However, although the results are promising, the trend is to reach therapies that do not require the use of host tissues.
Besides, the regeneration in the CNS is minimal, and there is no clinical treatment with a proven improvement of the CNS repair. In SCI, TBI, and degenerative disease, initial damages result in fast acute primary injury events. Current treatments, instead of trying to recover damaged tissue, try to prevent the secondary injury with high doses of steroids in SCI (Lyons et al., 1990), and maintaining adequate oxygen supply, blood flow, and pressure in TBI. For more severe brain injuries, surgery is required to remove hematomas and repair contusions (Pettikiriarachchi et al., 2010).
Due to the limited ability of the body to regenerate axonal bundles, it is necessary to develop a biomimetic strategy that can provide a bridge across the lesion while giving optimal morphological, chemical, and biological signals for the recovery of nervous tissue. So, tissue engineering in the nervous system have provided new alternative therapeutic avenues. Neural tissue engineering defends the use of external biomaterial supports, with cells and bioactive molecules (Langer and Vacanti, 1993) to overcome the inhibitory environment and achieve a successful repair of the nervous system. The first idea to overcome the drawbacks of the previous techniques was to develop materials with dimensions, morphology and characteristics determined as an implantable devices or biomaterials. The objective is to obtain biocompatible structures that are integrated into the surrounding tissue and that can be invaded or replaced by native cells and recover lost functionality.
Natural Biomaterials
The first biomaterials used, and that continues to be used today, had a natural origin and an identical or very similar nature to the tissue to be restored. The motivation of the use of natural biomaterials is that it would generate less rejection by the host cells as they are substances that they can recognize. In general, natural polymers promote excellent cell adhesion and growth. Not only matrices based on generic mixtures of ECM substances have been used (Bellamkonda et al., 1995) but also scaffolds of purified ECM molecules like collagen (Bozkurt et al., 2007) or other proteins (Shadforth et al., 2012), hyaluronic acid (Austin et al., 2012), and some polysaccharides (Mammadov et al., 2012). Scaffolds are defined as three-dimensional porous stable biomaterials designed to have some or all of these characteristics: promote cell-biomaterial interactions, cell adhesion and ECM deposition, allow the exchange of oxygen, nutrients, and factors, be biodegradable, and non-cytotoxic. However, although biomaterials of natural origin can be manufactured in a multitude formats such as in situ gellable solutions, scaffolds, fibers or tubular conduits, the truth is that their mechanical properties are poor and many times they biodegrade too quickly to allow adequate support (Tarun et al., 2011).
Collagen
The most common, type I, a fibrillary type of collagen, the main component of connective tissues which provide structure and support (Sherman et al., 2015). Collagen-based nerve guide conduits have been used to repair small nerve injuries (5 mm gap) (Archibald et al., 1995). However, they have also given good results in the regeneration of larger distances, as in 15 mm gap in rat sciatic nerve (Gonzalez-Perez et al., 2017) or in combination with NGF, partially reconstructing a 35-mm gap in dog sciatic nerve model (Yao et al., 2018b). Collagen is also used in combination with other polymers and proteins (Kiyotani et al., 1996; Cao et al., 2011) and is the only biopolymer approved for the clinical use in neural tissue engineering (Kehoe et al., 2012).
Gelatin
Gelatin is a denatured protein obtained by hydrolysis of animal collagen. Gelatin electrospun, in combination with other polymers, is usual. For example, gelatin/PCL's combination supports the neurite outgrowth and SC proliferation in vitro (Gupta et al., 2009; Alvarez-Perez et al., 2010) and in vivo (Kriebel et al., 2017). Also, gelatin nanoparticles have been developed for neural tissue engineering (Naseri-Nosar et al., 2017).
Hyaluronic Acid
Hyaluronic acid (HA) is an unbranched glycosaminoglycan. HA is present naturally in all vertebrates and is a significant constituent of the ECM in many parts of the human body. This material has gained significant interest in the area of neural tissue engineering, supporting neurite outgrowth. HA hydrogels enhance the survival rates and proliferation of neural precursors, been very promising for therapeutic approaches in the PNS (Suri and Schmidt, 2010; Thomas et al., 2017) and the CNS (Horn et al., 2007; Liang et al., 2013). HA can be used in combination with other natural polymers to promote regeneration, like collagen (Zhang et al., 2008) and chitosan (Li et al., 2018), and also with synthetic polymers (Wang et al., 2011).
Alginate
Alginate is a naturally derived, linear polysaccharide obtained from brown algae and bacteria. Alginate gels promote peripheral nerve regeneration across a 50-mm-long gap in cat sciatic nerve (Suzuki et al., 1999) and a 10-mm nerve gap in rats, increasing the regenerating axons' diameter (Hashimoto et al., 2002). Alginate can be used in combination with other polymers to develop scaffolds, showing great potential for peripheral nerve regeneration (Ansari et al., 2017; Wang et al., 2017b) and SCI treatment (Liu et al., 2017; Sitoci-Ficici et al., 2018).
Chitosan
Chitosan is a linear polysaccharide derived by the chemical deacetylation of chitin, structural polysaccharide naturally found in crustaceans and shellfish. Chitosan hydrogels promote cell adhesion, cell interaction, cell survival, and neurite outgrowth (Valmikinathan et al., 2012; Wang et al., 2017c). Chitosan scaffolds with NGF show regeneration in PNS (Li et al., 2017a,b) and CNS (Li et al., 2006).
Advantages and disadvantages of natural biomaterials are described in Table 1.
Synthetic Biomaterials
Another common approach is the use of biomaterials of synthetic origin. For example, poly-ε-caprolactone (PCL) (Nisbet et al., 2009; Gelain et al., 2011), poly-L-lactic acid (PLLA) (Hurtado et al., 2011; Binan et al., 2014), poly-D,L-lactic-co-glycolic acid (PLGA) (Olson et al., 2009; Kim et al., 2018), among others (Sinis et al., 2005; Srinivasan et al., 2015). These materials seemed to make up for the shortcomings of natural biomaterials since they can be manufactured with a multitude of techniques and architectures adapted to the type of tissue to be regenerated (Tamunonengiofori Banigo et al., 2019). Furthermore, by choosing the proper polymer or its composition, it is possible to obtain greater control over biodegradability (Lu et al., 2000). A wide variety of formats can be obtained, such as aligned scaffolds (Ngo et al., 2003; Wu et al., 2018), fibers or filaments (Kim et al., 2008; Hurtado et al., 2011), and tubular structures (Dai et al., 2013; Ni et al., 2013; Sarker et al., 2018). However, they also have certain limitations, such as reduced bioactivity that increases the risk of rejection after implantation. Therefore, the choice of the origin of the biomaterials to be used is especially important, being widespread systems composed of combination of various types of biomaterials (McMurtrey, 2014).
Polycaprolactone
Polycaprolactone (PCL) fibers have most commonly been generated by the method of electrospinning (Nectow et al., 2012). PCL can be combined with natural polymers or coated with cells to improve its biocompatibility, cell adhesion properties, and promote regeneration of nerve fibers (Aurand et al., 2012) or assist the directional growth of regenerating axons in peripheral nerves (Flynn et al., 2003).
Poly-L-Lactic Acid
Poly-L-lactic acid (PLLA) has been employed in the development of single or multilayer guidance conduits (Lu et al., 2009), nanofibrous conduit scaffolds with single or multiple microchannels (Sun et al., 2012; Zhao et al., 2013), or like fibers allocated in the lumen of tubular conduits (Ngo et al., 2003; Li et al., 2015) providing a support for cell adhesion, migration and elongation in a guided way.
Poly-D,L-Lactic-co-Glycolic Acid
Poly-D,L-lactic-co-glycolic acid (PLGA) is a copolymer of polylactic acid (PLA) and polyglycolic acid (PGA). PLGA has been successful in drug delivery microparticles and conduits for nerve regeneration (Xue et al., 2012). PLGA seems to be extremely useful in transporting therapeutic agents across the BBB (Malinovskaya et al., 2017; Mir et al., 2017).
Conductive Polymers
Conductive polymers are those with electrons present in their backbone, which enables them to conduct electricity. Nerve cells respond to electrical stimulation. Some common conductive polymers used are polypyrrole (PPY) and carbon nanotubes. PPy is an organic polymer formed by the polymerization of pyrrole monomer. PPy has been used in combination with other biodegradable non-natural polymers such as PCL, PLA, and PLGA to increased neurite growth (Lee et al., 2009). Carbon nanotubes (CNTs) are allotropes of carbon with a cylindrical structure. CNTs in combinations with other polymers exhibit increased neurite growth and elongation in all directions (Malarkey et al., 2009).
Advantages and disadvantages of synthetic biomaterials are described in Table 2.
Neurotrophic Factors and Cells for Neural Tissue Engineering
The role of neurotrophic factors in neural tissue regeneration has been widely studied (Terenghi, 1999; Blesch et al., 2002). Neurotrophic factors can be incorporated into the biomaterial to modulate cells' behavior and improve their survival and outgrowth. One family of neurotrophic factors, the neurotrophins, has been heavily investigated. The neurotrophins include NGF, BDNF, and NT-3. Other neurotrophic important factors are GDNF and acidic and basic fibroblast growth factor (aFGF, bFGF). They offer an improved regenerations results both in PNS (Bloch et al., 2001; Zurn et al., 2002) and SCI (Of et al., 1996; Oudega and Hagg, 1999; Ramer et al., 2000).
Apart from the aforementioned, and as part of the search for therapies with regenerative potential, cell transplantation appeared. In the first place, the transplantation of mature cells from the patient, obtained through biopsies was tested (Huang et al., 2008). However, the number of cells obtained by biopsies was not always sufficient, and the cell proliferation rate was relatively low. Adult stem cell transplantation, which offered great potential thanks to its multipotentiality, was also tested (Koch et al., 2009; Rooney et al., 2009). Nevertheless, their availability is limited and their survival rate is low after transplantation. In 2006, Takahashi and Yamanaka (2006) artificially converted differentiated cells into a pluripotent state in vitro, naming them induced pluripotent stem cells (iPSCs). This discovery represented a promising alternative approach for regenerative medicine thanks to the possibility of a cell autotransplantation that can be better long term integrated by being compatible with the host's immune system. However, there is a reluctance to use these cells due to the lack of regulation and the tumorigenic and immunogenic properties of iPSCs (Ohm et al., 2010).
Unfortunately, to date, cell transplantation alone in the nervous system is not an option for tissue recovery, due to the associated problems. Therefore, tissue engineering advocates combining a cell source with the use of the biomaterials. Biomaterials would provide, on the one hand, a three-dimensional environment which could accommodate the cells, and on the other hand, they could be devices for controlled release of bioactive molecules in a localized way in the desired tissue (Ren et al., 2014; Wang et al., 2017a). The cells would play a vital role by producing growth factors and ECM molecules, promoting tissue recovery. The cells used specifically in neural tissue regeneration include SC, bone marrow-derived mesenchymal stem cells, and other stem cell sources like embryonic stem cells, nerve stem cells, mesenchymal stem cells, and induced pluripotent stem cells (Bhangra et al., 2016).
Tissue Engineering Approaches in the Nervous System
Despite its complexity and the limitations that have already been described to achieve regeneration of the nervous system, various hopeful strategies have been developed for the regeneration of this tissue, such as directly injected gels (Austin et al., 2012), porous three-dimensional scaffolds similar to a sponge (Zhang et al., 2011), meshes with regular pore geometry (Suri et al., 2011), elongated and oriented microfilaments (Hurtado et al., 2011), and other configurations that are useful for other tissues. However, the best approximations are the so-called nerve guidance conduits (NGCs) due to their tubular structure that serves as a physical guide to direct axons sprouting, contain the growth factors secreted by the injured tissue, and reduce the infiltration of scar tissue (Raza et al., 2020). These guided nerve conduits have been widely used not only in nerve regeneration with promising results (Heuser and Maier, 2005) but also to regenerate spinal cord (Gelain et al., 2011) or even brain (Nisbet et al., 2009; Elias and Spector, 2012; Harris et al., 2016).
Tissue Engineering Approaches in the Peripheral Nervous System: Nerve Guidance Conduits
NGC is understood to be any device with a stable and elongated structure whose purpose is to support the growth of axons that have lost the ability to establish synaptic connections due to injury or degeneration. Initially, simple hollow silicone conduits were used for PNI regeneration (Lundborg et al., 1982; Francel et al., 1997). However, these were quickly replaced and optimized because they were not degradable, and they formed an excessive fibrous capsule due to problems with compression of the nerves. With the silicone conduit approach, the different phases of regeneration into a hollow NGC were described in a 10-mm nerve gap from the rat sciatic nerve, corresponding to the sequenced phases of Wallerian degeneration and resulting in regeneration mechanism. First, the fluid phase, where the conduit is filled with plasma exudate containing neurotrophic factors and ECM molecules (around 12 h after the injury). Second, the matrix phase, where fibrin cables are formed along the gap (around 1 week after injury). Third, the cellular phase, where SC invade the gap, migrate, and proliferate, aligned along the fibrin cable, forming the Bands of Büngner. Fourth, the axonal phase, where the first axons are visible migrating to their distal targets (around 2 weeks after injury). Fifth, the myelin phase, where SC produce myelin and wrapped around each axon (around 4 weeks after injury) (Lundborg et al., 1982). Hence, several FDA approved devices are consisting of hollow NGCs that can be classified in these categories: non-resorbable devices, natural resorbable devices, and synthetic resorbable devices (Kehoe et al., 2012). However, in longer gaps, the formation of the fibrin cable is compromised. SC, cannot align through the injury site, reducing the formation of the Bands of Büngner, necessary structures for the guidance of re-growing axons (Hoffman-Kim et al., 2010). Moreover, it is known that hollow NGC can derive in inadequate reinnervation. So, to control the axon growth, many strategies focus on filling the lumen with a diversity of configurations to provide a supporting structure, either mechanical or biological, that promotes cells growth, guidance, and correct targeting (Meyer et al., 2016; López-Cebral et al., 2017).
In conclusion, FDA-approved devices are recommended only for small gaps (up to 3 cm) and their biological performance is inferior compared with autografts, the need for new biomaterials and for the development of alternative conduits for peripheral nerve regeneration, and also CNS regeneration, remains imperative.
Different studies have clarified the parameters that would be a significant advantage in achieving glial cell survival and axon extension along the different NGCs. Therefore, there are many design variables that an ideal NGC should consider and incorporate (Figure 6).
Biodegradability and Porosity
The biomaterial employed must be biodegradable and biocompatible and produce no inflammatory response (Anderson et al., 2008), and prevent fibrous tissue ingrowth into the injury site. For example, HA inhibits the glial scar formation after brain damage (Lin et al., 2009). The biodegradable conduits are preferred over non-degradable conduits in clinical trials. For example, a collagen conduit was implanted in a 12-mm nerve gap of patients with an improved nerve functions (Taras et al., 2011). The biomaterial must be porous to allow an exchange of oxygen, nutrients, and factors between the lumen of the NGC and the interstitial fluid (Kokai et al., 2009; Her et al., 2013; Oh et al., 2013).
Intraluminal Channels
The NGC could incorporate intraluminal channels. Neural cells tend to grow and develop best on spherical or cylindrical structures, presumably by analogy with similar structures such as nerves, spinal cord, or brain tracts. PLLA nerve conduits with channels of 200 μm diameters showed positive physicochemical characteristics and promising neuron oriented differentiation (Liu et al., 2018).
Oriented Nerve Guidance
The NGC should present a guidance cue for the extending growth cone to reduce misdirection. The use of films or fibers as lumen filler has been proposed as an excellent way to obtain a structure as similar as possible to nervous tissue (Heuser and Maier, 2005; Clements et al., 2009) so that the filler serves as a guide structure and intermediate support. For example, nerve conduits with PLGA fibers in the lumen was useful for guiding the growing axons (Quigley et al., 2013). Moreover, surface topography has been shown to influence cellular behavior, including cell morphology, guidance, proliferation, and differentiation (Reimer et al., 2016).
Release of Bioactive Factors
The NGC could release bioactive factors incorporated into the biomaterial, to modulate the behavior of cells and improve their survival and growth. The biomaterial could also be functionalized with microparticles of a different material loaded with the bioactive molecule of interest, for example, salicylic acid in order to reduce inflammation and scar tissue formation because of SA in PNI (Lee et al., 2016). Neurotrophic factors such as NGF are of particular interest in promoting the peripheral nerve regeneration (Kuihua et al., 2014).
Incorporation of Support Cells
The NGC could incorporate supporting cells. Almost all the studies published in this field show a perceptible improvement in the result obtained with the NGC in vivo when they contain precultured neuroglia in the lumen in comparison with the empty NGC. The benefits of cell therapies for tissue engineering have already been discussed previously, but thanks to the fact that the cells are confined in the scaffolds, the cells multiply their effect by not dying or migrating to other areas, which was one of the main drawbacks of cells transplantation technique. For example, SC are of great interest for neural tissue engineering and help in PNI after introduced in a NGC (Dai et al., 2013; Meyer et al., 2016; Cerqueira et al., 2018). SC can be isolated from nerve tissue, cultured and expanded in vitro, and incorporated in the NGC (Allmeling et al., 2008) promoting the regeneration. It should be noted that although SC are neuroglia originating in the PNS (Sinis et al., 2005), there are precedents for the reconnection of tracts in the CNS (David and Aguayo, 1981; Olson et al., 2009; Yao et al., 2018a).
Electrical Conductivity
The NGC could present conductive biomaterials. Studies on conductive polymers and electrical stimulation appear as a relatively novel approach to increase neurite extension and axonal regeneration (Alrashdan et al., 2010). Electroconductive conduits supported sciatic nerve regeneration in rats after 8 weeks postsurgery (George et al., 2009).
A multitude of strategies has been developed for the regeneration of PNI taking all these factors into account (Sarker et al., 2018). A vast diversity of NGCs formats has been employed, including porous cylindrical structures of collagen (Bozkurt et al., 2012), structures based on PLGA and PCL microfilaments (Kim et al., 2018; Quan et al., 2019), tubular conduits with one or more channels (Oh et al., 2018), HA with fibers inside the lumen (Vilariño-Feltrer et al., 2016), and conduits with PLGA aligned conductive fibers (Jing et al., 2018), always with results superior compared with the one achieved by a hollow silicone tube.
Tissue Engineering Approaches in the Central Nervous System: Spinal Cord Injury, Traumatic Brain Injury, and Neurodegenerative Disease
As mentioned above, axonal bundles represent a unidirectional and aligned architecture allowing systematic axonal development within the nerve tissue. However, when an injury or disease in the PNS or CNS occurs, this complex architecture suffers disruption leading to the inhibition of growth and loss of guidance, whose recovery is especially tricky in the CNS (Tian et al., 2015).
Commonly, human SCI occurs after a contusion injury that compresses the spinal cord. However, many of SCI consists of complete transection of the spinal cord, with a complete disruption of the entire spinal cord. Moreover, there are other circumstances like large lesions or chronic situations where an injury has occurred some time previously, where it may be necessary to use some form of an oriented bridging substrate that bypasses the injury site and restores continuity across a traumatized area (Sakiyama-Elbert et al., 2012).
The spinal cord consists of a considerable amount of ascending and descending tracts, each located in particular parts of the dorsal, lateral, or ventral column. When SCI occurs, ideally, this spatial organization should be maintained by implanted structures, allowing that regenerating axons can find their proper targets in the gray matter behind the injury site. For this reason, biomaterials with channels or guides that are oriented parallel to the spinal cord tracts, like NGCs, among others, seem to constitute the optimal configuration. Under this premise, many strategies have been developed for SCI such as HA conduits with PLLA fibers in the lumen (Martínez-Ramos et al., 2019), scaffolds with highly aligned conduits made of fibrin (Scott et al., 2011), conduits made of aligned PLLA nanofiber (Hurtado et al., 2011), multichannel (Gros et al., 2010) or individual cannel agarose scaffold (Stokols and Tuszynski, 2006), PLGA multiple channel bridges (Tuinstra et al., 2012), among others (Tsai et al., 2006; Horn et al., 2007; Wong et al., 2008).
Another group of disorders of the CNS affect the brain, such as TBI or neurodegenerative diseases such as Parkinson's disease. In the same way that occurs with SCI or PNI, they all have in common the loss of long-distance axonal connections.
The brain is a tremendously complex organ, and a multitude of tissue engineering approaches have been carried out to address the brain injuries. Hydrogels have been attractive for application to the brain due to their consistency similar to soft brain tissues and high permeability that allows the exchange of oxygen and nutrients (Li et al., 2012; Gwon et al., 2017). Even micro- and nanoparticles also offer advantages in brain tissue engineering. As systems are small and can be customized according to the polymer or molecules used, they are capable of crossing the blood-brain barrier, being carriers of molecules or drugs of interest (Dhar et al., 2011; Rassu et al., 2015).
However, taking into account the idea of regenerative bridges to facilitate long-distance axonal regeneration, several recent studies of great interest have been developed. For example, microcolumns containing tubular agarose hydrogels with collagenous matrix inside have been produced, making use of the confining capacity of astroglia cells to create a neural guide pathway (Winter et al., 2016; Katiyar et al., 2018). A more complex system of microtissue engineered neural networks, containing mature primary cortical neurons and long axonal tracts in the lumen of hydrogel microcolumns, have also been developed to mimic gray and white matter (Harris et al., 2016), proposed as a possible treatment for Parkinson's disease (Struzyna et al., 2018).
Conclusively, from a neurological perspective, the challenge will be to design scaffolds that sustain multiple functions, a structure that can modulate a variety of cellular and signaling functions, and that can effectively support and guide the regenerative growth of axonal bundles or axonal descending and ascending tracts from diverse sources.
Conclusion
Due to its complex anatomical architecture, the repair of the nervous system, among other causes, is one of the great challenges of modern medicine. Significant advances have been made in cell-based therapies, nanotechnologies, and novel biomaterials, as well as a better understanding of the nervous system's knowledge. Results to date have shown that cell-based therapies are not sufficient for the repair of neural tissue after damage. However, tissue engineering sheds light on this problem. Tissue engineering advocates the combination of biomaterials together with cells and bioactive molecules to mimic the environment to regenerate while supporting the repair of new tissue. More multidisciplinary approaches will be needed in the future to apply these therapeutic strategies in clinics.
Author Contributions
LD wrote this paper. CM-R and MP supervised this work and revised the manuscript. All authors contributed to the article and approved the submitted version.
Funding
The authors acknowledge financial support from the Spanish Ministry of Economy and Competitiveness through MINECO grants, funded by AEI RTI2018-095872-B-C21 and C22/ERDF. LD acknowledges scholarship FPU15/04975 from the Spanish Ministry of Education, Culture and Sports.
Conflict of Interest
The authors declare that the research was conducted in the absence of any commercial or financial relationships that could be construed as a potential conflict of interest.
References
Aijie, C., Xuan, L., Huimin, L., Yanli, Z., Yiyuan, K., Yuqing, L., et al. (2018). Nanoscaffolds in promoting regeneration of the peripheral nervous system. Nanomedicine 13, 1067–1085. doi: 10.2217/nnm-2017-0389
Alberti, K. A., Hopkins, A. M., Tang-Schomer, M. D., Kaplan, D. L., and Xu, Q. (2014). The behavior of neuronal cells on tendon-derived collagen sheets as potential substrates for nerve regeneration. Biomaterials 35, 3551–3557. doi: 10.1016/j.biomaterials.2013.12.082
Allmeling, C., Jokuszies, A., Reimers, K., Kall, S., Choi, C. Y., Brandes, G., et al. (2008). Spider silk fibres in artificial nerve constructs promote peripheral nerve regeneration. Cell Prolif. 41, 408–420. doi: 10.1111/j.1365-2184.2008.00534.x
Alrashdan, M. S., Park, J. C., Sung, M. A., Yoo, S. B., Jahng, J. W., Lee, T. H., et al. (2010). Thirty minutes of low intensity electrical stimulation promotes nerve regeneration after sciatic nerve crush injury in a rat model. Acta Neurol. Belg. 110, 168–179.
Alvarez-Perez, M. A., Guarino, V., Cirillo, V., and Ambrosio, L. (2010). Influence of gelatin cues in PCL electrospun membranes on nerve outgrowth. Biomacromolecules 11, 2238–2246. doi: 10.1021/bm100221h
Anderson, J. M., Rodriguez, A., and Chang, D. T. (2008). Foreign body reaction to biomaterials. Semin. Immunol. 20, 86–100. doi: 10.1016/j.smim.2007.11.004
Anjum, A., Yazid, M. D., Fauzi Daud, M., Idris, J., Ng, A. M. H., Selvi Naicker, A., et al. (2020). Spinal cord injury: pathophysiology, multimolecular interactions, and underlying recovery mechanisms. Int. J. Mol. Sci. 21:7533. doi: 10.3390/ijms21207533
Ansari, S., Diniz, I. M., Chen, C., Sarrion, P., Tamayol, A., Wu, B. M., et al. (2017). Human periodontal ligament- and gingiva-derived mesenchymal stem cells promote nerve regeneration when encapsulated in alginate/hyaluronic acid 3D scaffold. Adv. Healthc. Mater. 6, 1–10. doi: 10.1002/adhm.201700670
Archibald, S. J., Shefner, J., Krarup, C., and Madison, R. D. (1995). Monkey median nerve repaired by nerve graft or collagen nerve guide tube. J. Neurosci. 15, 4109–4123. doi: 10.1523/JNEUROSCI.15-05-04109.1995
Aurand, E. R., Lampe, K. J., and Bjugstad, K. B. (2012). Defining and designing polymers and hydrogels for neural tissue engineering. Neurosci. Res. 72, 199–213. doi: 10.1016/j.neures.2011.12.005
Austin, J. W., Kang, C. E., Baumann, M. D., DiDiodato, L., Satkunendrarajah, K., Wilson, J. R., et al. (2012). The effects of intrathecal injection of a hyaluronan-based hydrogel on inflammation, scarring and neurobehavioural outcomes in a rat model of severe spinal cord injury associated with arachnoiditis. Biomaterials 33, 4555–4564. doi: 10.1016/j.biomaterials.2012.03.022
Avraham, O., Deng, P. Y., Jones, S., Kuruvilla, R., Semenkovich, C. F., Klyachko, V. A., et al. (2020). Satellite glial cells promote regenerative growth in sensory neurons. Nat. Commun. 11, 1–17. doi: 10.1038/s41467-020-18642-y
Bellamkonda, R., Ranieri, J. P., Bouche, N., and Aebischer, P. (1995). Hydrogel-based three-dimensional matrix for neural cells. J. Biomed. Mater. Res. 29, 663–671. doi: 10.1002/jbm.820290514
Bhangra, K. S., Busuttil, F., Phillips, J. B., and Rahim, A. A. (2016). Using stem cells to grow artificial tissue for peripheral nerve repair. Stem Cells Int. 2016:18. doi: 10.1155/2016/7502178
Binan, L., Tendey, C., De Crescenzo, G., El Ayoubi, R., Ajji, A., and Jolicoeur, M. (2014). Differentiation of neuronal stem cells into motor neurons using electrospun poly-l-lactic acid/gelatin scaffold. Biomaterials 35, 664–674. doi: 10.1016/j.biomaterials.2013.09.097
Blesch, A., Lu, P., and Tuszynski, M. H. (2002). Neurotrophic factors, gene therapy, and neural stem cells for spinal cord repair. Brain Res. Bull. 57, 833–838. doi: 10.1016/S0361-9230(01)00774-2
Bloch, J., Fine, E. G., Bouche, N., Zurn, A. D., and Aebischer, P. (2001). Nerve growth factor- and neurotrophin-3-releasing guidance channels promote regeneration of the transected rat dorsal root. Exp. Neurol. 172, 425–432. doi: 10.1006/exnr.2001.7778
Bolívar, S., Navarro, X., and Udina, E. (2020). Schwann cell role in selectivity of nerve regeneration. Cells 9:2131. doi: 10.3390/cells9092131
Bozkurt, A., Brook, G. A., Moellers, S., Lassner, F., Sellhaus, B., Weis, J., et al. (2007). In vitro assessment of axonal growth using dorsal root ganglia explants in a novel three-dimensional collagen matrix. Tissue Eng. 13, 2971–2979. doi: 10.1089/ten.2007.0116
Bozkurt, A., Lassner, F., O'Dey, D., Deumens, R., Böcker, A., Schwendt, T., et al. (2012). The role of microstructured and interconnected pore channels in a collagen-based nerve guide on axonal regeneration in peripheral nerves. Biomaterials 33, 1363–1375. doi: 10.1016/j.biomaterials.2011.10.069
Busch, S. A., and Silver, J. (2007). The role of extracellular matrix in CNS regeneration. Curr. Opin. Neurobiol. 17, 120–127. doi: 10.1016/j.conb.2006.09.004
Butt, A., and Verkhratsky, A. (2018). Neuroglia: realising their true potential. Brain Neurosci. Adv. 2:239821281881749. doi: 10.1177/2398212818817495
Cao, J., Sun, C., Zhao, H., Xiao, Z., Chen, B., Gao, J., et al. (2011). The use of laminin modified linear ordered collagen scaffolds loaded with laminin-binding ciliary neurotrophic factor for sciatic nerve regeneration in rats. Biomaterials 32, 3939–3948. doi: 10.1016/j.biomaterials.2011.02.020
Cerqueira, S. R., Lee, Y. S., Cornelison, R. C., Mertz, M. W., Wachs, R. A., Schmidt, C. E., et al. (2018). Decellularized peripheral nerve supports Schwann cell transplants and axon growth following spinal cord injury. Biomaterials 177, 176–185. doi: 10.1016/j.biomaterials.2018.05.049
Clements, I. P., Kim, Y., English, A. W., Lu, X., Chung, A., and Bellamkonda, R. V. (2009). Thin-film enhanced nerve guidance channels for peripheral nerve repair. Biomaterials 30, 3834–3846. doi: 10.1016/j.biomaterials.2009.04.022
Crapo, P. M., Medberry, C. J., Reing, J. E., Tottey, S., van der Merwe, Y., Jones, K. E., et al. (2012). Biologic scaffolds composed of central nervous system extracellular matrix. Biomaterials 33, 3539–3547. doi: 10.1016/j.biomaterials.2012.01.044
Cullen, D. K., Tang-Schomer, M. D., Struzyna, L., Patel, A. R., Johnson, V. E., et al. (2012). Microtissue engineered constructs with living axons for targeted nervous system reconstruction. Tissue Eng. Part A 18:120817094501006. doi: 10.1089/ten.tea.2011.0534
Curcio, M., and Bradke, F. (2018). Axon regeneration in the central nervous system: facing the challenges from the inside. Annu. Rev. Cell Dev. Biol. 34, 495–521. doi: 10.1146/annurev-cellbio-100617-062508
Dai, L. G., Huang, G. S., and Hsu, S. H. (2013). Sciatic nerve regeneration by cocultured schwann cells and stem cells on microporous nerve conduits. Cell Transplant. 22, 2029–2039. doi: 10.3727/096368912X658953
Daly, W. T., Knight, A. M., Wang, H., de Boer, R., Giusti, G., Dadsetan, M., et al. (2013). Comparison and characterization of multiple biomaterial conduits forperipheral nerve repair. Biomaterials 34, 8630–8639. doi: 10.1016/j.biomaterials.2013.07.086
David, S., and Aguayo, A. (1981). Axonal elongation into peripheral nervous system “bridges” after central nervous system injury in adult rats. Science (80-.). 214, 931–933. doi: 10.1126/science.6171034
Davie, C. A. (2008). A review of Parkinson's disease. Br. Med. Bull. 86, 109–127. doi: 10.1093/bmb/ldn013
Deumens, R., Bozkurt, A., Meek, M. F., Marcus, M. A. E., Joosten, E. A. J., Weis, J., et al. (2010). Repairing injured peripheral nerves: bridging the gap. Prog. Neurobiol. 92, 245–276. doi: 10.1016/j.pneurobio.2010.10.002
Dhar, S., Reddy, E. M., Prabhune, A., Pokharkar, V., Shiras, A., and Prasad, B. L. V. (2011). Cytotoxicity of sophorolipid-gellan gum-gold nanoparticle conjugates and their doxorubicin loaded derivatives towards human glioma and human glioma stem cell lines. Nanoscale 3, 575–580. doi: 10.1039/C0NR00598C
Dubový, P., Klusáková, I., and Hradilová Svíženská, I. (2014). Inflammatory profiling of Schwann cells in contact with growing axons distal to nerve injury. Biomed Res. Int. 2014:691041. doi: 10.1155/2014/691041
Elias, P. Z., and Spector, M. (2012). Implantation of a collagen scaffold seeded with adult rat hippocampal progenitors in a rat model of penetrating brain injury. J. Neurosci. Methods 209, 199–211. doi: 10.1016/j.jneumeth.2012.06.003
Evans, P. J., Midha, R., and Mackinnon, S. E. (1994). The peripheral nerve allograft: a comprehensive review of regeneration and neuroimmunology. Prog. Neurobiol. 43, 187–233. doi: 10.1016/0301-0082(94)90001-9
Fitch, M. T., and Silver, J. (2008). CNS injury, glial scars, and inflammation: inhibitory extracellular matrices and regeneration failure. Exp Neurol. 209, 294–301. doi: 10.1016/j.expneurol.2007.05.014
Flynn, L., Dalton, P. D., and Shoichet, M. S. (2003). Fiber templating of poly(2-hydroxyethyl methacrylate) for neural tissue engineering. Biomaterials 24, 4265–4272. doi: 10.1016/S0142-9612(03)00334-X
Francel, P. C., Francel, T. J., Mackinnon, S. E., and Hertl, C. (1997). Enhancing nerve regeneration across a silicone tube conduit by using interposed short-segment nerve grafts. J. Neurosurg. 87, 887–892. doi: 10.3171/jns.1997.87.6.0887
Gelain, F., Panseri, S., Antonini, S., Cunha, C., Donega, M., Lowery, J., et al. (2011). Transplantation of nanostructured composite scaffolds results in the regeneration of chronically injured spinal cords. ACS Nano 5, 227–236. doi: 10.1021/nn102461w
George, P. M., Saigal, R., Lawlor, M. W., Moore, M. J., LaVan, D. A., Marini, R. P., et al. (2009). Three-dimensional conductive constructs for nerve regeneration. J. Biomed. Mater. Res. - Part A 91, 519–527. doi: 10.1002/jbm.a.32226
Goldberg, J. L., Klassen, M. P., Hua, Y., and Barres, B. A. (2002). Amacrine-signaled loss of intrinsic axon growth ability by retinal ganglion cells. Science (80-.). 296, 1860–1864. doi: 10.1126/science.1068428
Gómez-Barrena, E., Rosset, P., Lozano, D., Stanovici, J., Ermthaller, C., and Gerbhard, F. (2015). Bone fracture healing: cell therapy in delayed unions and nonunions. Bone 70, 93–101. doi: 10.1016/j.bone.2014.07.033
Gonzalez-Perez, F., Cobianchi, S., Heimann, C., Phillips, J. B., Udina, E., and Navarro, X. (2017). Stabilization, rolling, and addition of other extracellular matrix proteins to collagen hydrogels improve regeneration in chitosan guides for long peripheral nerve gaps in rats. Neurosurgery 80, 465–474. doi: 10.1093/neuros/nyw068
Griffin, J. W., Hogan, M. C. V., Chhabra, A. B., and Deal, D. N. (2013). Peripheral nerve repair and reconstruction. J. Bone Jt. Surg. Ser. A 95, 2144–2151. doi: 10.2106/JBJS.L.00704
Grinsell, D., and Keating, C. P. (2014). Peripheral nerve reconstruction after injury: a review of clinical and experimental therapies. Biomed Res. Int. 2014:698256. doi: 10.1155/2014/698256
Gros, T., Sakamoto, J. S., Blesch, A., Havton, L. A., and Tuszynski, M. H. (2010). Regeneration of long-tract axons through sites of spinal cord injury using templated agarose scaffolds. Biomaterials 31, 6719–6729. doi: 10.1016/j.biomaterials.2010.04.035
Gupta, D., Venugopal, J., Prabhakaran, M. P., Dev, V. R. G., Low, S., Choon, A. T., et al. (2009). Aligned and random nanofibrous substrate for the in vitro culture of Schwann cells for neural tissue engineering. Acta Biomater. 5, 2560–2569. doi: 10.1016/j.actbio.2009.01.039
Gupta, R., Gray, M., Chao, T., Bear, D., Modafferi, E., and Mozaffar, T. (2005). Schwann cells upregulate vascular endothelial growth factor secondary to chronic nerve compression injury. Muscle Nerve 31, 452–460. doi: 10.1002/mus.20272
Gwon, K., Kim, E., and Tae, G. (2017). Heparin-hyaluronic acid hydrogel in support of cellular activities of 3D encapsulated adipose derived stem cells. Acta Biomater. 49, 284–295. doi: 10.1016/j.actbio.2016.12.001
Harris, J. P., Struzyna, L. A., Murphy, P. L., Adewole, D. O., Kuo, E., and Cullen, D. K. (2016). Advanced biomaterial strategies to transplant preformed micro-tissue engineered neural networks into the brain. J. Neural Eng. 13:16019. doi: 10.1088/1741-2560/13/1/016019
Hashimoto, T., Suzuki, Y., Kitada, M., Kataoka, K., Wu, S., Suzuki, K., et al. (2002). Peripheral nerve regeneration through alginate gel: analysis of early outgrowth and late increase in diameter of regenerating axons. Exp. Brain Res. 146, 356–368. doi: 10.1007/s00221-002-1173-y
Her, G. J., Wu, H. C., Chen, M. H., Chen, M. Y., Chang, S. C., and Wang, T. W. (2013). Control of three-dimensional substrate stiffness to manipulate mesenchymal stem cell fate toward neuronal or glial lineages. Acta Biomater. 9, 5170–5180. doi: 10.1016/j.actbio.2012.10.012
Heuser, V. I., and Maier, W. (2005). Peripheral nerve regeneration across an 80-mm gap bridged by a polyglycolic acid (PGA)–collagen tube filled with laminin-coated collagen fibers: a histological and electrophysiological evaluation of regenerated nerves. Brain Res. 868, 315–28. doi: 10.1016/s0006-8993(00)02207-1
Hill, C. S., Coleman, M. P., and Menon, D. K. (2016). Traumatic axonal injury: mechanisms and translational opportunities. Trends Neurosci. 39, 311–324. doi: 10.1016/j.tins.2016.03.002
Hoffman-Kim, D., Mitchel, J. A., and Bellamkonda, R. V. (2010). Topography, cell response, and nerve regeneration. Annu. Rev. Biomed. Eng. 12, 203–231. doi: 10.1146/annurev-bioeng-070909-105351
Horn, E. M., Beaumont, M., Shu, X. Z., Harvey, A., Prestwich, G. D., Horn, K. M., et al. (2007). Influence of cross-linked hyaluronic acid hydrogels on neurite outgrowth and recovery from spinal cord injury. J Neurosurg Spine 6, 133–140. doi: 10.3171/spi.2007.6.2.133
Huang, J. H., Zager, E. L., Zhang, J., Groff, I. V. R. F, Pfister, B. J., et al. (2008). Harvested human neurons engineered as live nervous tissue constructs: implications for transplantation - Laboratory investigation. J. Neurosurg. 108, 343–347. doi: 10.3171/JNS/2008/108/2/0343
Hurtado, A., Cregg, J. M., Wang, H. B., Wendell, D. F., Oudega, M., Gilbert, R. J., et al. (2011). Robust CNS regeneration after complete spinal cord transection using aligned poly-l-lactic acid microfibers. Biomaterials 32, 6068–6079. doi: 10.1016/j.biomaterials.2011.05.006
Ijkema-Paassen, J., Jansen, K., Gramsbergen, A., and Meek, M. F. (2004). Transection of peripheral nerves, bridging strategies and effect evaluation. Biomaterials 25, 1583–1592. doi: 10.1016/S0142-9612(03)00504-0
Jäkel, S., and Dimou, L. (2017). Glial cells and their function in the adult brain: a journey through the history of their ablation. Front. Cell. Neurosci. 11, 1–17. doi: 10.3389/fncel.2017.00024
Jessen, K. R., and Mirsky, R. (2016). The repair Schwann cell and its function in regenerating nerves. J. Physiol. 594, 3521–3531. doi: 10.1113/JP270874
Jing, W., Ao, Q., Wang, L., Huang, Z., Cai, Q., Chen, G., et al. (2018). Constructing conductive conduit with conductive fibrous infilling for peripheral nerve regeneration. Chem. Eng. J. 345, 566–577. doi: 10.1016/j.cej.2018.04.044
Kastriti, M. E., and Adameyko, I. (2017). Specification, plasticity and evolutionary origin of peripheral glial cells. Curr. Opin. Neurobiol. 47, 196–202. doi: 10.1016/j.conb.2017.11.004
Katiyar, K. S., Winter, C. C., Gordián-Vélez, W. J., O'donnell, J. C., Song, Y. J., Hernandez, N. S., et al. (2018). Three-dimensional tissue engineered aligned astrocyte networks to recapitulate developmental mechanisms and facilitate nervous system regeneration. J. Vis. Exp. 2018, 1–17. doi: 10.3791/55848
Kehoe, S., Zhang, X. F., and Boyd, D. (2012). FDA approved guidance conduits and wraps for peripheral nerve injury: a review of materials and efficacy. Injury 43, 553–572. doi: 10.1016/j.injury.2010.12.030
Kim, S. M., Lee, M. S., Jeon, J., Lee, D. H., Yang, K., Cho, S. W., et al. (2018). Biodegradable nerve guidance conduit with microporous and micropatterned poly(lactic-co-glycolic acid)-accelerated sciatic nerve regeneration. Macromol. Biosci. 18, 1–14. doi: 10.1002/mabi.201800290
Kim, Y., tae Haftel, V. K., Kumar, S., and Bellamkonda, R. V. (2008). The role of aligned polymer fiber-based constructs in the bridging of long peripheral nerve gaps. Biomaterials 29, 3117–3127. doi: 10.1016/j.biomaterials.2008.03.042
Kiyotani, T., Teramachi, M., Takimoto, Y., Nakamura, T., Shimizu, Y., and Endo, K. (1996). Nerve regeneration across a 25-mm gap bridged by a polyglycolic acid-collagen tube: a histological and electrophysiological evaluation of regenerated nerves. Brain Res. 740, 66–74. doi: 10.1016/S0006-8993(96)00848-7
Koch, P., Kokaia, Z., Lindvall, O., and Brüstle, O. (2009). Emerging concepts in neural stem cell research: autologous repair and cell-based disease modelling. Lancet Neurol. 8, 819–829. doi: 10.1016/S1474-4422(09)70202-9
Kokai, L. E., Lin, Y. C., Oyster, N. M., and Marra, K. G. (2009). Diffusion of soluble factors through degradable polymer nerve guides: Controlling manufacturing parameters. Acta Biomater. 5, 2540–2550. doi: 10.1016/j.actbio.2009.03.009
Kriebel, A., Hodde, D., Kuenzel, T., Engels, J., Brook, G., and Mey, J. (2017). Cell-free artificial implants of electrospun fibres in a three-dimensional gelatin matrix support sciatic nerve regeneration in vivo. J. Tissue Eng. Regen. Med. 11, 3289–3304. doi: 10.1002/term.2237
Kuihua, Z., Chunyang, W., Cunyi, F., and Xiumei, M. (2014). Aligned SF/P(LLA-CL)-blended nanofibers encapsulating nerve growth factor for peripheral nerve regeneration. J. Biomed. Mater. Res. Part A 102, 2680–2691. doi: 10.1002/jbm.a.34922
Langer, R., and Vacanti, J. P. (1993). Tissue engineering. Educ. Forum 260, 920–926. doi: 10.1126/science.8493529
Lee, J. Y., Bashur, C. A., Goldstein, A. S., and Schmidt, C. E. (2009). Polypyrrole-coated electrospun PLGA nanofibers for neural tissue applications. Biomaterials 30, 4325–4335. doi: 10.1016/j.biomaterials.2009.04.042
Lee, Y. S., Griffin, J., Masand, S. N., Shreiber, D. I., and Uhrich, K. E. (2016). Salicylic acid-based poly(anhydride-ester) nerve guidance conduits: impact of localized drug release on nerve regeneration. J. Biomed. Mater. Res. - Part A 104, 975–982. doi: 10.1002/jbm.a.35630
L'Heureux, N., McAllister, T. N., and De La Fuente, L. M. (2007). Tissue-engineered blood vessel for adult arterial revascularization. N. Engl. J. Med. 357, 1451–1453. doi: 10.1056/NEJMc071536
Li, D., Pan, X., Sun, B., Wu, T., Chen, W., Huang, C., et al. (2015). Nerve conduits constructed by electrospun P(LLA-CL) nanofibers and PLLA nanofiber yarns. J. Mater. Chem. B 3, 8823–8831. doi: 10.1039/C5TB01402F
Li, G., Xiao, Q., McNaughton, R., Han, L., Zhang, L., Wang, Y., et al. (2017a). Nanoengineered porous chitosan/CaTiO3 hybrid scaffolds for accelerating Schwann cells growth in peripheral nerve regeneration. Colloids Surf. B Biointerfaces 158, 57–67. doi: 10.1016/j.colsurfb.2017.06.026
Li, G., Xiao, Q., Zhang, L., Zhao, Y., and Yang, Y. (2017b). Nerve growth factor loaded heparin/chitosan scaffolds for accelerating peripheral nerve regeneration. Carbohydr. Polym. 171, 39–49. doi: 10.1016/j.carbpol.2017.05.006
Li, M., Mondrinos, M. J., Chen, X., Gandhi, M. R., Ko, F. K., and Lelkes, P. I. (2006). Elastin blends for tissue engineering scaffolds. J. Biomed. Mater. Res. Part A 79, 963–973. doi: 10.1002/jbm.a.30833
Li, R., Liu, H., Huang, H., Bi, W., Yan, R., Tan, X., et al. (2018). Chitosan conduit combined with hyaluronic acid prevent sciatic nerve scar in a rat model of peripheral nerve crush injury. Mol. Med. Rep. 17, 4360–4368. doi: 10.3892/mmr.2018.8388
Li, X., Katsanevakis, E., Liu, X., Zhang, N., and Wen, X. (2012). Engineering neural stem cell fates with hydrogel design for central nervous system regeneration. Prog. Polym. Sci. 37, 1105–1129. doi: 10.1016/j.progpolymsci.2012.02.004
Liang, Y., Walczak, P., and Bulte, J. W. M. (2013). The survival of engrafted neural stem cells within hyaluronic acid hydrogels. Biomaterials 34, 5521–5529. doi: 10.1016/j.biomaterials.2013.03.095
Lin, C. M., Lin, J. W., Chen, Y. C., Shen, H. H., Wei, L., Yeh, Y. S., et al. (2009). Hyaluronic acid inhibits the glial scar formation after brain damage with tissue loss in rats. Surg. Neurol. 72, S50–4. doi: 10.1016/j.wneu.2009.09.004
Liu, S., Sandner, B., Schackel, T., Nicholson, L. S., Chtarto, A., Tenenbaum, L., et al. (2017). Regulated viral BDNF delivery in combination with Schwann cells promotes axonal regeneration through capillary alginate hydrogels after spinal cord injury. Acta Biomater. 60, 167–180. doi: 10.1016/j.actbio.2017.07.024
Liu, S., Sun, X., Wang, T., Chen, S., Zeng, C., guang Xie, G., et al. (2018). Nano-fibrous and ladder-like multi-channel nerve conduits: degradation and modification by gelatin. Mater. Sci. Eng. C 83, 130–142. doi: 10.1016/j.msec.2017.11.020
López-Cebral, R., Silva-Correia, J., Reis, R. L., Silva, T. H., and Oliveira, J. M. (2017). Peripheral nerve injury: current challenges, conventional treatment approaches, and new trends in biomaterials-based regenerative strategies. ACS Biomater. Sci. Eng. 3, 3098–3122. doi: 10.1021/acsbiomaterials.7b00655
Lu, L., Peter, S. J. D, Lyman, M., Lai, H. L., Leite, S. M., et al. (2000). In vitro and in vivo degradation of porous poly(DL-lactic-co-glycolic acid) foams. Biomaterials 21, 1837–1845. doi: 10.1016/S0142-9612(00)00047-8
Lu, M. C., Huang, Y. T., Lin, J. H., Yao, C. H., Lou, C. W., Tsai, C. C., et al. (2009). Evaluation of a multi-layer microbraided polylactic acid fiber-reinforced conduit for peripheral nerve regeneration. J. Mater. Sci. Mater. Med. 20, 1175–1180. doi: 10.1007/s10856-008-3646-4
Lundborg, G., Dahlin, L. B., Danielsen, N., Gelberman, R. H., Longo, F. M., Powell, H. C., et al. (1982). Nerve regeneration in silicone chambers: influence of gap lenght and of distal stump component. Exp. Neurol. 76, 361–375. doi: 10.1016/0014-4886(82)90215-1
Lyons, M. K., Partington, M. D., Meyer, F. B., Yarkony, G. M., Roth, E. J., Senegor, M., et al. (1990). A randomized, controlled trial of methylprednisolone or naloxolone in the treatment of acute spinal-cord injury. New English J. Med. 323, 1120–1123. doi: 10.1056/NEJM199010253231712
Mai, J. K., and Paxinos, G. (2012). The Human Nervous System. San Diego, CA: Elsevier. doi: 10.1016/B978-0-12-374236-0.10001-X
Malarkey, E. B., Fisher, K. A., Bekyarova, E., Liu, W., Haddon, R. C., and Parpura, V. (2009). Conductive single-walled carbon nanotube substrates modulate neuronal growth. Nano Lett. 9, 264–268. doi: 10.1021/nl802855c
Malinovskaya, Y., Melnikov, P., Baklaushev, V., Gabashvili, A., Osipova, N., Mantrov, S., et al. (2017). Delivery of doxorubicin-loaded PLGA nanoparticles into U87 human glioblastoma cells. Int. J. Pharm. 524, 77–90. doi: 10.1016/j.ijpharm.2017.03.049
Mammadov, B., Mammadov, R., Guler, M. O., and Tekinay, A. B. (2012). Cooperative effect of heparan sulfate and laminin mimetic peptide nanofibers on the promotion of neurite outgrowth. Acta Biomater. 8, 2077–2086. doi: 10.1016/j.actbio.2012.02.006
Manoli, T., Schulz, L., Stahl, S., Jaminet, P., and Schaller, H.-E. (2009). Evaluation of sensory recovery after reconstruction of digital nerves of the hand using muscle-in-vein conduits in comparison to nerve suture or nerve autografting. Microsurgery. 34, 608–615. doi: 10.1002/micr.22302
Martínez-Ramos, C., Doblado, L. R., Mocholi, E. L., Alastrue-Agudo, A., Petidier, M. S., Giraldo, E., et al. (2019). Biohybrids for spinal cord injury repair. J. Tissue Eng. Regen. Med. 13, 509–521. doi: 10.1002/term.2816
McMurtrey, R. J. (2014). Patterned and functionalized nanofiber scaffolds in three-dimensional hydrogel constructs enhance neurite outgrowth and directional control. J. Neural Eng. 11:066009. doi: 10.1088/1741-2560/11/6/066009
Meyer, C., Wrobel, S., Raimondo, S., Rochkind, S., Heimann, C., Shahar, A., et al. (2016). Peripheral nerve regeneration through hydrogel-enriched Chitosan conduits containing engineered Schwann cells for drug delivery. Cell Transplant. 25, 159–182. doi: 10.3727/096368915X688010
Mir, M., Ahmed, N., and Rehman, A. (2017). Recent applications of PLGA based nanostructures in drug delivery. Colloids Surf. B Biointerfaces 159, 217–231. doi: 10.1016/j.colsurfb.2017.07.038
Moon, L. D. F., Brecknell, J. E., Franklin, R. J. M., Dunnett, S. B., and Fawcett, J. W. (2000). Robust regeneration of CNS axons through a track depleted of CNS glia. Exp. Neurol. 161, 49–66. doi: 10.1006/exnr.1999.7230
Moore, A. M. (2014). Nerve transfers to restore upper extremity function: a paradigm shift. Front. Neurol. 5, 1–2. doi: 10.3389/fneur.2014.00040
Naseri-Nosar, M., Salehi, M., and Hojjati-Emami, S. (2017). Cellulose acetate/poly lactic acid coaxial wet-electrospun scaffold containing citalopram-loaded gelatin nanocarriers for neural tissue engineering applications. Int. J. Biol. Macromol. 103, 701–708. doi: 10.1016/j.ijbiomac.2017.05.054
Nectow, A. R., Marra, K. G., and Kaplan, D. L. (2012). Biomaterials for the development of peripheral nerve guidance conduits. Tissue Eng. Part B Rev. 18, 40–50. doi: 10.1089/ten.teb.2011.0240
Ngo, T. T. B., Waggoner, P. J., Romero, A. A., Nelson, K. D., Eberhart, R. C., and Smith, G. M. (2003). Poly(L-lactide) microfilaments enhance peripheral nerve regeneration across extended nerve lesions. J. Neurosci. Res. 72, 227–238. doi: 10.1002/jnr.10570
Ni, H. C., Tseng, T. C., Chen, J. R., Hsu, S. H., and Chiu, I. M. (2013). Fabrication of bioactive conduits containing the fibroblast growth factor 1 and neural stem cells for peripheral nerve regeneration across a 15 mm critical gap. Biofabrication 5:035010. doi: 10.1088/1758-5082/5/3/035010
Nichols, C. M., Brenner, M. J., Fox, I. K., Tung, T. H., Hunter, D. A., Rickman, S. R., et al. (2004). Effects of motor versus sensory nerve grafts on peripheral nerve regeneration. Exp. Neurol. 190, 347–355. doi: 10.1016/j.expneurol.2004.08.003
Nisbet, D. R., Rodda, A. E., Horne, M. K., Forsythe, J. S., and Finkelstein, D. I. (2009). Neurite infiltration and cellular response to electrospun polycaprolactone scaffolds implanted into the brain. Biomaterials 30, 4573–4580. doi: 10.1016/j.biomaterials.2009.05.011
Niu, Y., Chen, K. C., He, T., Yu, W., Huang, S., and Xu, K. (2014). Scaffolds from block polyurethanes based on poly(ε-caprolactone) (PCL) and poly(ethylene glycol) (PEG) for peripheral nerve regeneration. Biomaterials 35, 4266–4277. doi: 10.1016/j.biomaterials.2014.02.013
Noback, C. R., Strominger, N. L., Demarest, R. J., and Ruggiero, D. A. (1968). The Human Nervous System. Totowa: Humana Press. doi: 10.1176/appi.psychotherapy.1968.22.1.119
Of G. Genetically F. To M. Ngf S. Basic O. R. Elicit F. G. F. . (1996). Grafts of fibroblasts genetically modified to secret NGF, BDNF, NT-3, or basic FGF elicit differential responses in the adult spinal cord. Science (80-.). 5, 191–204. doi: 10.1016/0963-6897(95)02028-4
Oh, S. H., Kang, J. G., Kim, T. H., Namgung, U., Song, K. S., Jeon, B. H., et al. (2018). Enhanced peripheral nerve regeneration through asymmetrically porous nerve guide conduit with nerve growth factor gradient. J. Biomed. Mater. Res. Part A 106, 52–64. doi: 10.1002/jbm.a.36216
Oh, S. H., Kim, J. R., Kwon, G. B., Namgung, U., Song, K. S., and Lee, J. H. (2013). Effect of surface pore structure of nerve guide conduit on peripheral nerve regeneration. Tissue Eng. Part C Methods 19, 233–243. doi: 10.1089/ten.tec.2012.0221
Ohm, J. E., Mali, P., Van Neste, L., Berman, D. M., Liang, L., Pandiyan, K., et al. (2010). Cancer-related epigenome changes associated with reprogramming to induced pluripotent stem cells. Cancer Res. 70, 7662–7673. doi: 10.1158/0008-5472.CAN-10-1361
Olson, H. E., Rooney, G. E., Gross, L., Nesbitt, J. J., Galvin, K. E., Knight, A., et al. (2009). Neural stem cell- and Schwann cell-loaded biodegradable polymer scaffolds support axonal regeneration in the transected spinal cord. Tissue Eng. Part A 15, 1797–1805. doi: 10.1089/ten.tea.2008.0364
Oudega, M., and Hagg, T. (1999). Neurotrophins promote regeneration of sensory axons in the adult rat spinal cord. Brain Res. 818, 431–438. doi: 10.1016/S0006-8993(98)01314-6
Paixão, S., and Klein, R. (2010). Neuron-astrocyte communication and synaptic plasticity. Curr. Opin. Neurobiol. 20, 466–473. doi: 10.1016/j.conb.2010.04.008
Pettikiriarachchi, J. T. S., Parish, C. L., Shoichet, M. S., Forsythe, J. S., and Nisbet, D. R. (2010). Biomaterials for brain tissue engineering. Aust. J. Chem. 63, 1143–1154. doi: 10.1071/CH10159
Pfister, B. J., Gordon, T., Loverde, J. R., Kochar, A. S., Mackinnon, S. E., and Kacy Cullen, D. (1987). Biomedical engineering strategies for peripheral nerve repair: surgical applications, state of the art, and future challenges. Pract. 387, 65–84.
Platt, J. L., Vercellotti, G. M., Dalmasso, A. P., Matas, A. J., Bolman, R. M., Najarian, J. S., et al. (1990). Transplantation of discordant xenografts: a review of progress. Immunol. Today 11, 450–456. doi: 10.1016/0167-5699(90)90174-8
Quan, Q., Meng, H., Chang, B., Hong, L., Li, R., Liu, G. B., et al. (2019). Novel 3-D helix-flexible nerve guide conduits repair nerve defects. Biomaterials 207, 49–60. doi: 10.1016/j.biomaterials.2019.03.040
Quigley, A. F., Bulluss, K. J., Kyratzis, I. L. B., Gilmore, K., Mysore, T., Schirmer, K. S. U., et al. (2013). Engineering a multimodal nerve conduit for repair of injured peripheral nerve. J. Neural Eng. 10:016008. doi: 10.1088/1741-2560/10/1/016008
Ramer, M. S., Priestley, J. V., and McMahon, S. B. (2000). Functional regeneration of sensory axons into the adult spinal cord. Nature 403, 312–316. doi: 10.1038/35002084
Ramiya, V. K., Maraist, M., Arfors, K. E., Schatz, D. A., Peck, A. B., and Cornelius, J. G. (2000). Reversal of insulin-dependent diabetes using islets generated in vitro from pancreatic stem cells. Nat. Med. 6, 278–282. doi: 10.1038/73128
Rassu, G., Soddu, E., Cossu, M., Brundu, A., Cerri, G., Marchetti, N., et al. (2015). Solid microparticles based on chitosan or methyl-β-cyclodextrin: a first formulative approach to increase the nose-to-brain transport of deferoxamine mesylate. J. Control. Release 201, 68–77. doi: 10.1016/j.jconrel.2015.01.025
Ray, W. Z., and Mackinnon, S. E. (2010). Management of nerve gaps: autografts, allografts, nerve transfers, and end-to-side neurorrhaphy. Exp. Neurol. 223, 77–85. doi: 10.1016/j.expneurol.2009.03.031
Raza, C., Riaz, H. A., Anjum, R., Shakeel, N., and ul, A. (2020). Repair strategies for injured peripheral nerve: review. Life Sci. 243:117308. doi: 10.1016/j.lfs.2020.117308
Reimer, A., Vasilevich, A., Hulshof, F., Viswanathan, P., Van Blitterswijk, C. A., De Boer, J., et al. (2016). Scalable topographies to support proliferation and Oct4 expression by human induced pluripotent stem cells. Sci. Rep. 6, 1–8. doi: 10.1038/srep18948
Ren, H., Han, M., Zhou, J., Zheng, Z. F., Lu, P., Wang, J. J., et al. (2014). Repair of spinal cord injury by inhibition of astrocyte growth and inflammatory factor synthesis through local delivery of flavopiridol in PLGA nanoparticles. Biomaterials 35, 6585–6594. doi: 10.1016/j.biomaterials.2014.04.042
Richardson, P. M., McGuinness, U. M., and Aguayo, A. J. (1980). Axons from CNS neurones regenerate into PNS grafts. Nature 284, 264–265. doi: 10.1038/284264a0
Rooney, G. E., McMahon, S. S., Ritter, T., Garcia, Y., Moran, C., Madigan, N. N., et al. (2009). Neurotrophic factor-expressing mesenchymal stem cells survive transplantation into the contused spinal cord without differentiating into neural cells. Tissue Eng. Part A 15, 3049–3059. doi: 10.1089/ten.tea.2009.0045
Sakiyama-Elbert, S., Johnson, P. J., Hodgetts, S. I., Plant, G. W., and Harvey, A. R. (2012). Scaffolds to Promote Spinal Cord Regeneration. 1st Edn. Amsterdam: Elsevier B.V. doi: 10.1016/B978-0-444-52137-8.00036-X
Sarker, M. D., Naghieh, S., McInnes, A. D., Schreyer, D. J., and Chen, X. (2018). Regeneration of peripheral nerves by nerve guidance conduits: Influence of design, biopolymers, cells, growth factors, and physical stimuli. Prog. Neurobiol. 171, 125–150. doi: 10.1016/j.pneurobio.2018.07.002
Schmidt, C. E., and Leach, J. B. (2003). Neural tissue engineering: strategies for repair and regeneration. Annu. Rev. Biomed. Eng. 5, 293–347. doi: 10.1146/annurev.bioeng.5.011303.120731
Scott, J. B., Afshari, M., Kotek, R., and Saul, J. M. (2011). The promotion of axon extension in vitro using polymer-templated fibrin scaffolds. Biomaterials 32, 4830–4839. doi: 10.1016/j.biomaterials.2011.03.037
Shadforth, A. M. A., George, K. A., Kwan, A. S., Chirila, T. V., and Harkin, D. G. (2012). The cultivation of human retinal pigment epithelial cells on Bombyx mori silk fibroin. Biomaterials 33, 4110–4117. doi: 10.1016/j.biomaterials.2012.02.040
Shamash, S., Reichert, F., and Rotshenker, S. (2002). The cytokine network of wallerian degeneration: Tumor necrosis factor-α, interleukin-1α, and interleukin-1β. J. Neurosci. 22, 3052–3060. doi: 10.1523/JNEUROSCI.22-08-03052.2002
Sherman, V. R., Yang, W., and Meyers, M. A. (2015). The materials science of collagen. J. Mech. Behav. Biomed. Mater. 52, 22–50. doi: 10.1016/j.jmbbm.2015.05.023
Sinis, N., Schaller, H. E., Schulte-Eversum, C., Schlosshauer, B., Doser, M., Dietz, K., et al. (2005). Nerve regeneration across a 2-cm gap in the rat median nerve using a resorbable nerve conduit filled with Schwann cells. J. Neurosurg. 103, 1067–1076. doi: 10.3171/jns.2005.103.6.1067
Sitoci-Ficici, K. H., Matyash, M., Uckermann, O., Galli, R., Leipnitz, E., Later, R., et al. (2018). Non-functionalized soft alginate hydrogel promotes locomotor recovery after spinal cord injury in a rat hemimyelonectomy model. Acta Neurochir. 160, 449–457. doi: 10.1007/s00701-017-3389-4
Srinivasan, A., Tahilramani, M., Bentley, J. T., Gore, R. K., Millard, D. C., Mukhatyar, V. J., et al. (2015). Microchannel-based regenerative scaffold for chronic peripheral nerve interfacing in amputees. Biomaterials 41, 151–165. doi: 10.1016/j.biomaterials.2014.11.035
Stierli, S., Imperatore, V., and Lloyd, A. C. (2019). Schwann cell plasticity-roles in tissue homeostasis, regeneration, and disease. Glia 67, 2203–2215. doi: 10.1002/glia.23643
Stokols, S., and Tuszynski, M. H. (2006). Freeze-dried agarose scaffolds with uniaxial channels stimulate and guide linear axonal growth following spinal cord injury. Biomaterials 27, 443–451. doi: 10.1016/j.biomaterials.2005.06.039
Struzyna, L. A., Browne, K. D., Brodnik, Z. D., Burrell, J. C., Harris, J. P., Chen, H. I., et al. (2018). Tissue engineered nigrostriatal pathway for treatment of Parkinson's disease. J. Tissue Eng. Regen. Med. 12, 1702–1716. doi: 10.1002/term.2698
Sun, C., Jin, X., Holzwarth, J. M., Liu, X., Hu, J., Gupte, M. J., et al. (2012). Development of channeled nanofibrous scaffolds for oriented tissue engineering. Macromol. Biosci. 12, 761–769. doi: 10.1002/mabi.201200004
Suri, S., Han, L. H., Zhang, W., Singh, A., Chen, S., and Schmidt, C. E. (2011). Solid freeform fabrication of designer scaffolds of hyaluronic acid for nerve tissue engineering. Biomed. Microdevices 13, 983–993. doi: 10.1007/s10544-011-9568-9
Suri, S., and Schmidt, C. E. (2010). Cell-laden hydrogel constructs of hyaluronic acid, collagen, and laminin for neural tissue engineering. Tissue Eng. Part A 16, 1703–1716. doi: 10.1089/ten.tea.2009.0381
Suzuki, Y., Tanihara, M., Ohnishi, K., Suzuki, K., Endo, K., and Nishimura, Y. (1999). Cat peripheral nerve regeneration across 50 mm gap repaired with a novel nerve guide composed of freeze-dried alginate gel. Neurosci. Lett. 259, 75–78. doi: 10.1016/S0304-3940(98)00924-0
Takahashi, K., and Yamanaka, S. (2006). Induction of pluripotent stem cells from mouse embryonic and adult fibroblast cultures by defined factors. Cell 126, 663–676. doi: 10.1016/j.cell.2006.07.024
Tamunonengiofori Banigo, A., Chidi Iwuji, S., and Chibuike Iheaturu, N. (2019). Application of biomaterials in tissue engineering: a review. J. Chem. Pharm. Res. 11, 1–16.
Taras, J. S., Jacoby, S. M., and Lincoski, C. J. (2011). Reconstruction of digital nerves with collagen conduits. J. Hand Surg. Am. 36, 1441–1446. doi: 10.1016/j.jhsa.2011.06.009
Tarun, G., Ajay, B., Bhawna, K., Sunil, K., and Ravi, J. (2011). Scaffold: tissue engineering and regenerative medicine. Int. Res. J. Pharm. 2, 37–42.
Terenghi, G. (1999). Peripheral nerve regeneration and neurotrophic factors. J. Anat. 194, 1–14. doi: 10.1046/j.1469-7580.1999.19410001.x
Thomas, R. C., Vu, P., Modi, S. P., Chung, P. E., Landis, R. C., Khaing, Z. Z., et al. (2017). Sacrificial crystal templated hyaluronic acid hydrogels as biomimetic 3D tissue scaffolds for nerve tissue regeneration. ACS Biomater. Sci. Eng. 3, 1451–1459. doi: 10.1021/acsbiomaterials.7b00002
Thompson, R. E., Pardieck, J., Smith, L., Kenny, P., Crawford, L., Shoichet, M., et al. (2018). Effect of hyaluronic acid hydrogels containing astrocyte-derived extracellular matrix and/or V2a interneurons on histologic outcomes following spinal cord injury. Biomaterials 162, 208–223. doi: 10.1016/j.biomaterials.2018.02.013
Tian, L., Prabhakaran, M. P., and Ramakrishna, S. (2015). Strategies for regeneration of components of nervous system: scaffolds, cells and biomolecules. Regen. Biomater. 2, 31–45. doi: 10.1093/rb/rbu017
Triolo, F., and Srivastava, A. K. (2019). Current Approaches to Tissue Engineering of the Nervous System. London: Elsevier Inc. doi: 10.1016/B978-0-12-801238-3.65854-3
Tsai, E. C., Dalton, P. D., Shoichet, M. S., and Tator, C. H. (2006). Matrix inclusion within synthetic hydrogel guidance channels improves specific supraspinal and local axonal regeneration after complete spinal cord transection. Biomaterials 27, 519–533. doi: 10.1016/j.biomaterials.2005.07.025
Tuinstra, H. M., Aviles, M. O., Shin, S., Holland, S. J., Zelivyanskaya, M. L., Fast, A. G., et al. (2012). Multifunctional, multichannel bridges that deliver neurotrophin encoding lentivirus for regeneration following spinal cord injury. Biomaterials 33, 1618–1626. doi: 10.1016/j.biomaterials.2011.11.002
Valmikinathan, C. M., Mukhatyar, V. J., Jain, A., Karumbaiah, L., Dasari, M., and Bellamkonda, R. V. (2012). Photocrosslinkable chitosan based hydrogels for neural tissue engineering. Soft Matter 8, 1964–1976. doi: 10.1039/C1SM06629C
Vijayavenkataraman, S. (2020). Nerve guide conduits for peripheral nerve injury repair: a review on design, materials and fabrication methods. Acta Biomater. 106, 54–69. doi: 10.1016/j.actbio.2020.02.003
Vilariño-Feltrer, G., Martínez-Ramos, C., Monleón-De-La-Fuente, A., Vallés-Lluch, A., Moratal, D., Barcia Albacar, J. A., et al. (2016). Schwann-cell cylinders grown inside hyaluronic-acid tubular scaffolds with gradient porosity. Acta Biomater. 30, 199–211. doi: 10.1016/j.actbio.2015.10.040
Wang, B., Yuan, J., Xu, J., Chen, X., Ying, X., and Dong, P. (2017a). Brain-derived and glial cell line-derived neurotrophic factor fusion protein immobilization to laminin. Exp. Ther. Med. 13, 178–186. doi: 10.3892/etm.2016.3925
Wang, G., Wang, X., and Huang, L. (2017b). Feasibility of chitosan-alginate (Chi-Alg) hydrogel used as scaffold for neural tissue engineering: a pilot study in vitro. Biotechnol. Biotechnol. Equip. 31, 766–773. doi: 10.1080/13102818.2017.1332493
Wang, S., Sun, C., Guan, S., Li, W., Xu, J., Ge, D., et al. (2017c). Chitosan/gelatin porous scaffolds assembled with conductive poly(3,4-ethylenedioxythiophene) nanoparticles for neural tissue engineering. J. Mater. Chem. B 5, 4774–4788. doi: 10.1039/C7TB00608J
Wang, Y., Wei, Y. T., Zu, Z. H., Ju, R. K., Guo, M. Y., Wang, X. M., et al. (2011). Combination of hyaluronic acid hydrogel scaffold and PLGA microspheres for supporting survival of neural stem cells. Pharm. Res. 28, 1406–1414. doi: 10.1007/s11095-011-0452-3
Wanner, I. B., and Wood, P. M. (2002). N-cadherin mediates axon-aligned process growth and cell-cell interaction in Rat Schwann Cells. J. Neurosci. 22, 4066–4079. doi: 10.1523/JNEUROSCI.22-10-04066.2002
Winter, C. C., Katiyar, K. S., Hernandez, N. S., Song, Y. J., Struzyna, L. A., Harris, J. P., et al. (2016). Transplantable living scaffolds comprised of micro-tissue engineered aligned astrocyte networks to facilitate central nervous system regeneration. Acta Biomater. 38, 44–58. doi: 10.1016/j.actbio.2016.04.021
Wong, D. Y., Leveque, J. C., Brumblay, H., Krebsbach, P. H., Hollister, S. J., and LaMarca, F. (2008). Macro-architectures in spinal cord scaffold implants influence regeneration. J. Neurotrauma 25, 1027–1037. doi: 10.1089/neu.2007.0473
Wu, S., Chen, M. S., Maurel, P., Lee, Y. S., Bunge, M. B., and Arinzeh, T. L. (2018). Aligned fibrous PVDF-TrFE scaffolds with Schwann cells support neurite extension and myelination in vitro. J. Neural Eng. 15:056010. doi: 10.1088/1741-2552/aac77f
Xue, C., Hu, N., Gu, Y., Yang, Y., Liu, Y., Liu, J., et al. (2012). Joint use of a chitosan/PLGA scaffold and MSCs to bridge an extra large gap in dog sciatic nerve. Neurorehabil. Neural Repair 26, 96–106. doi: 10.1177/1545968311420444
Xue, C., Zhu, H., Tan, D., Ren, H., Gu, X., Zhao, Y., et al. (2018). Electrospun silk fibroin-based neural scaffold for bridging a long sciatic nerve gap in dogs. J. Tissue Eng. Regen. Med. 12, e1143–53. doi: 10.1002/term.2449
y Cajal, S. R. (1930). Degeneration and regeneration of the nervous system. Nature 125, 230–231. doi: 10.1038/125230a0
Yang, R., Xu, C., Wang, T., Wang, Y., Wang, J., Quan, D., et al. (2017). PTMAc-PEG-PTMAc hydrogel modified by RGDC and hyaluronic acid promotes neural stem cells' survival and differentiation: in vitro. RSC Adv. 7, 41098–41104. doi: 10.1039/C7RA06614G
Yang, X. N., Jin, Y. Q., Bi, H., Wei, W., Cheng, J., Liu, Z. Y., et al. (2013). Peripheral nerve repair with epimysium conduit. Biomaterials 34, 5606–5616. doi: 10.1016/j.biomaterials.2013.04.018
Yao, S., Yu, S., Cao, Z., Yang, Y., Yu, X., Mao, H. Q., et al. (2018a). Hierarchically aligned fibrin nanofiber hydrogel accelerated axonal regrowth and locomotor function recovery in rat spinal cord injury. Int. J. Nanomedicine 13, 2883–2895. doi: 10.2147/IJN.S159356
Yao, Y., Cui, Y., Zhao, Y., Xiao, Z., Li, X., Han, S., et al. (2018b). Efect of longitudinally oriented collagen conduit combined with nerve growth factor on nerve regeneration after dog sciatic nerve injury. J. Biomed. Mater. Res. Part B Appl. Biomater. 106, 2131–2139. doi: 10.1002/jbm.b.34020
Zhang, F., He, C., Cao, L., Feng, W., Wang, H., Mo, X., et al. (2011). Fabrication of gelatin-hyaluronic acid hybrid scaffolds with tunable porous structures for soft tissue engineering. Int. J. Biol. Macromol. 48, 474–481. doi: 10.1016/j.ijbiomac.2011.01.012
Zhang, H., Wei, Y. T., Tsang, K. S., Sun, C. R., Li, J., Huang, H., et al. (2008). Implantation of neural stem cells embedded in hyaluronic acid and collagen composite conduit promotes regeneration in a rabbit facial nerve injury model. J. Transl. Med. 6, 1–11. doi: 10.1186/1479-5876-6-67
Zhang, J. Y., Luo, X. G., Xian, C. J., Liu, Z. H., and Zhou, X. F. (2000). Endogenous BDNF is required for myelination and regeneration of injured sciatic nerve in rodents. Eur. J. Neurosci. 12, 4171–4180. doi: 10.1046/j.1460-9568.2000.01312.x
Zhao, C., Tan, A., Pastorin, G., and Ho, H. K. (2013). Nanomaterial scaffolds for stem cell proliferation and differentiation in tissue engineering. Biotechnol. Adv. 31, 654–668. doi: 10.1016/j.biotechadv.2012.08.001
Zhou, X., Yang, A., Huang, Z., Yin, G., Pu, X., and Jin, J. (2017). Enhancement of neurite adhesion, alignment and elongation on conductive polypyrrole-poly(lactide acid) fibers with cell-derived extracellular matrix. Colloids Surf. B Biointerfaces 149, 217–225. doi: 10.1016/j.colsurfb.2016.10.014
Keywords: biomaterial, polymers, nervous system, regenerative medicine, neural tissue engineering
Citation: Doblado LR, Martínez-Ramos C and Pradas MM (2021) Biomaterials for Neural Tissue Engineering. Front. Nanotechnol. 3:643507. doi: 10.3389/fnano.2021.643507
Received: 18 December 2020; Accepted: 03 March 2021;
Published: 26 April 2021.
Edited by:
Sandeep Kumar, Guru Jambheshwar University of Science and Technology, IndiaReviewed by:
Chetna Dhand, Advanced Materials and Processes Research Institute (CSIR), IndiaRuixiang Li, Shanghai University of Traditional Chinese Medicine, China
Copyright © 2021 Doblado, Martínez-Ramos and Pradas. This is an open-access article distributed under the terms of the Creative Commons Attribution License (CC BY). The use, distribution or reproduction in other forums is permitted, provided the original author(s) and the copyright owner(s) are credited and that the original publication in this journal is cited, in accordance with accepted academic practice. No use, distribution or reproduction is permitted which does not comply with these terms.
*Correspondence: Cristina Martínez-Ramos, cris_mr_1980@hotmail.com