- Department of Microbiology, Immunology and Genetics, University of North Texas Health Science Center, Fort Worth, TX, United States
Lung cancer is the leading cause of cancer related deaths globally, making it a major health concern. The lung’s permissive rich microenvironment is ideal for supporting outgrowth of disseminated tumors from pre-existing extra-pulmonary malignancies usually resulting in high mortality. Tumors occurring in the lungs are difficult to treat, necessitating the need for the development of advanced treatment modalities against primary tumors and secondary lung metastasis. In this review, we explore the pulmonary route as an attractive drug delivery approach to treat lung tumors. We also discuss the potential of pulmonary delivery of cancer vaccine vectors to induce mucosal immunity capable of preventing the seeding of tumors in the lung.
Introduction
The World Health Organization (WHO) reported lung cancer to be the most common cause of cancer deaths worldwide, contributing to 1.76 million deaths in 2018 alone (Ferlay et al., 2019). In 2020, the United States (US) estimates 228,820 new cases of lung cancer and 135,720 projected deaths; the highest among all cancer related deaths (Siegel, Miller et al., 2020). Despite advances in treatment, the 5-year survival rate for primary lung cancer remains at 19% compared to prostate cancer, melanoma of skin and female breast cancer having 5-year survival rates of 98, 92, and 90%, respectively (Lu et al., 2019, Siegel et al., 2020). The persistent low survivorship among lung cancer patients is in part due to the large proportion of patients (57%) diagnosed with metastatic disease, for which the 5-year relative survival is a meager 5% (Howlader et al., 2020). This suggests a further unmet need to improve treatment strategies that can reduce lung cancer occurrence and prevent metastasis thereby increasing survival rates. Lung cancer is broadly categorized into small cell lung cancer (SCLC) and non-small cell lung cancer (NSCLC). NSCLC is the most prevalent form, accounting for about 85% of all lung cancer cases and has a 5-year survival rate of 24% (Howlader et al., 2020). NSCLC is further classified into adenocarcinoma, squamous cell carcinoma and large cell carcinoma. SCLC represents the most aggressive type of lung cancer with the poorest 5-year survival rate of 6% (Howlader et al., 2020).
In addition to primary lung cancer, extra-pulmonary tumors are capable of remodeling the lung microenvironment to support the establishment and outgrowth of disseminated tumor cells to generate secondary metastatic tumors in the lung (Peinado et al., 2017) (Figure 1). Circulating tumor cells (CTCs) that reach the lungs directly through blood circulation via intravasation or through the lymphatics are termed disseminated tumor cells (DTCs). Successful colonization of the lungs by DTCs require the initial formation of a permissive microenvironment; often referred to as a pre metastatic niche through an interplay between tumor derived components, the rich lung stromal tumor microenvironment and mobilized bone marrow derived cells (BMDCs) (Liu and Cao, 2016). The tumor derived molecular components that govern pre-metastatic niche formation include extracellular vesicles (exosomes, microvesicles and large oncosomes) and tumor derived secreted factors (growth factors, cytokines and chemokines). These induce certain changes in the lung microenvironment leading to the recruitment of BMDCs into the lung, where they act together to support metastasis. Such cross talk among various tumor derived factors, the lung stromal microenvironment and the mobilized BMDCs promotes metastasis by mechanisms including immunosuppression, inflammation, angiogenesis and lymphangiogenesis (Liu and Cao, 2016). For example, lung tropic 4,175 exosomes were identified to co-localized with S100A4-positive fibroblasts and lung epithelial cells expressing the surfactant protein C where they induced the expression of pro-inflammatory and metastatic inducible S100 genes (Hoshino et al., 2015). Small nuclear RNA enriched exosomes were reported to induce chemokine secretion in the lung and promote recruitment of neutrophils to the pre metastatic niche by activating alveolar epithelial TLR3 (Liu et al., 2016). Extracellular vesicles secreted by cancer associated fibroblasts carrying protein cargos have been implicated in the formation of pre metastatic niche in the lung by targeting lung fibroblasts through TGF-B signaling enhancing remodeling of the extracellular matrix (Kong et al., 2019). Furthermore, primary tumor derived chemokine, CCL2 induces the expression of TLR4 ligands, S100A8 and SAA3 by lung club cells to support vascular permeability in the lung and stimulate the migration of myeloid cells (Maru, 2015).
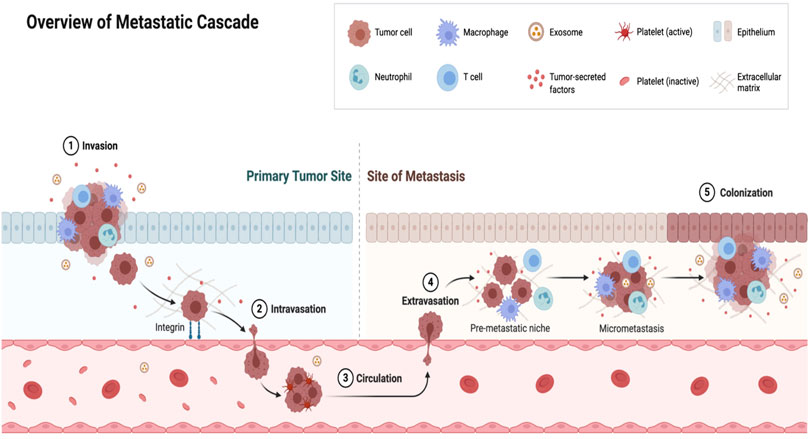
FIGURE 1. Events of lung metastasis. The primary tumor regulates the development of metastasis in a distal organ such as the lung by secreting factors such as (cytokines and exosomes) that primes pre-metastatic niche formation. Following local invasion by primary tumor cells, the broken away tumor cells intravasate into circulation as circulating tumor cells (CTCs). Upon surviving shear stress as a result of blood and immune surveillance, CTCs that reach a distal organ with a pre metastatic niche extravasate into the organ with the help of the pre metastatic niche where they exist as disseminated tumor cells (DTCs). The pre-metastatic niche promotes the survival of DTCs and remodel the microenvironment by inducing immunosuppression and other events to drive micro-metastasis and eventual colonization of the distal organ by the primary tumor cells.
Lung metastases are identified in 20–54% of all cancer patients and spread to the lungs is usually a marker of advanced malignant disease which is associated with poor survival (Mohammed et al., 2011). Table 1 below lists the leading primary cancers with high risk of lung metastasis and low survival rates. Consequently, prevention or successful treatment of secondary lung metastasis due to an existing primary extrapulmonary tumor is considered the gold standard to improve 5-year survival rates in cancer patients. However, tumors occurring in the lung either as a primary tumor or metastasis from an existing extra pulmonary tumor remain difficult to treat despite advancements in current treatment approaches involving surgery, chemotherapy, radiation and immunotherapy. In this review, we provide a summary of current treatments and prospective future directions to improve the management of tumors that occur in the lungs either as a primary tumor or metastasis from extra-pulmonary tumors. We focus primarily on immunotherapy as an option to prevent the seeding of tumor cells in the lungs following the occurrence of extra pulmonary tumors by highlighting the pulmonary route as an attractive strategy to deliver “prophylactic” cancer vaccines.
Conventional Treatments of Primary and Secondary Metastatic Lung Cancer
Surgical removal is the primary course of treatment for non-metastatic lung cancers where tumors are confined in the lung as seen in stage I and II NSCLC (Lackey and Donington, 2013). The 5-year survival rate in resected stage 1 NSCLC patient is 70%, but this drops to 25% in stage III patients (Imperatori et al., 2010). Thus, surgery becomes ineffective as the tumor progresses, requiring alternative treatment modalities. In cases of lung metastases from distal primary tumors, surgery may be effective in prolonging survival in patients with metastases that is limited to pulmonary parenchyma without the involvement of other organs (Warwick and Page, 2007). By comparison, surgery is rarely an option for SCLC, the most aggressive type of lung cancer (Barnes et al., 2017; Xu et al., 2019).
Chemotherapy is the primary treatment for SCLC due its propensity to spread quickly to distal organs (Ganti et al., 2007; Karim and Zekri, 2012; Yang S. et al., 2019). Initial chemotherapy for SCLC patients usually consists of a combination of etoposide and a platinum agent (either carboplatin or cisplatin) (Ganti et al., 2007; Yang S. et al., 2019). In terms of NSCLC involving the lymph nodes, neoadjuvant chemotherapy given months before surgical resection of tumors in the lung is the standard course of action (McElnay and Lim, 2014). Chemotherapy given months before surgical procedure increases the cure rate in patients with stage III NSCLC (McElnay and Lim, 2014). Chemotherapy can also be given after surgical resection (adjuvant chemotherapy) in patients with NSCLC. This is beneficial because at the time of surgery there may be micrometastasis which may not be detected and removed as part of surgical procedure and may lead to recurrence of the tumor. Pulmonary metastasis is a sign of advanced systemic disease and systemic chemotherapy is usually part of the model of therapy (Chojniak et al., 2006). Depending on the type and nature of primary tumor and extent of lung involvement, it may be combined with surgery either as neoadjuvant or adjuvant chemotherapy to treat lung lesions and the primary tumor. For example, patients with primary osteosarcoma and pulmonary metastases (e.g., Less than eight metastatic lesions) who were treated with neoadjuvant ifosfamide, surgical resection of both primary and metastatic tumors and high dose methotrexate, ifosfamide, doxorubicin and cisplatin had a five-year disease free survival rate of 66.7% (Harris et al., 1998).
In contrast to chemotherapy, radiation therapy is a suitable option for early stage lung cancer especially in cases where the patient is inoperable due to compromised pulmonary function or co-morbid conditions such as hypertension and diabetes (Timmerman et al., 2010). Although conventional radiation therapy in management of early stage lung cancer is disappointing, advancement in the field following the adoption of stereotactic technology has improved treatment outcomes. Stereotactic body radiation therapy (SBRT) enables the delivery of high dose radiation to tumors while sparing healthy tissues. Although radiation is highly targeted, it is not effective in eliminating tumors when it has spread from the lung. SBRT may be effective in treating patients with limited metastatic disease due to high rate of local control and minimal toxicity (Carvajal et al., 2015; Filippi et al., 2016; Rieber et al., 2016; Agolli et al., 2017).
Emerging Immune-Based Cancer Treatment for Primary and Secondary Lung Metastasis
The Concept of Immunotherapy
The hallmark of the immune system is the ability to recognize, eradicate and establish memory defenses against pathogenic encounters and foreign substances (Schreiber et al., 2011). The immune defense system is also considered the first line against the genesis and progression of tumors (Davis et al., 2015). During tumor development, mutations occur leading to potentially immunogenic cancer cells that can be recognized and killed by the host immune system (Pandya et al., 2016). For example, non-self-antigens expressed by tumor cells can be presented on MHC-1 molecules and recognized by cytotoxic T-cells leading to T-cell activation and killing of tumors (Speiser and Ohashi, 1998). Hence, infiltration of solid tumors by immune cells (T-cells and B-cells) has been identified as a good prognostic biomarker in most types of cancers as it correlates to favorable clinical outcomes. Therefore, immune surveillance is able to prevent the occurrence of primary tumors as well as the rise of metastasis. Yet, tumors can evade the immune system by suppressing immune cell function through various mechanisms that mimic immune tolerance to escape immune surveillance (Cacan, 2017; Claisse et al., 2017; Noguchi et al., 2017). Tumor cells achieve this through deliberate loss of antigenicity by downregulating the expression of tumor antigens required for initiation of an immune response and through mechanisms that disrupt antigen presentation, such as loss of major histocompatibility complex-1 (Schreiber et al., 2011). Tumor cells again evade immune surveillance by exploiting immune cell intrinsic mechanisms of immune tolerance by expressing PD-L1, where PD-1/PD-L1 interaction between tumors and cytotoxic T-cells have been shown to play a vital role in limiting antitumor immunity (Pardoll, 2012; Sharma and Allison, 2015). Other mechanisms include release of immunosuppressive cytokines by tumors and tumor cell intrinsic aberrations/unique metabolic pathways that lead to impaired T-cell activation and accumulation in the tumor microenvironment aiding in tumor evasion (Spranger et al., 2015; Casey et al., 2016; Koyama et al., 2016; Peng et al., 2016). For example, the activation WNT/B- catenin signaling, PTEN loss, Myc signaling and HIF-1 alpha signaling are known for poor T-cell priming and reduced intratumoral accumulation of cytotoxic T-lymphocytes (Spranger et al., 2015; Zelenay et al., 2015; Casey et al., 2016; Koyama et al., 2016; Peng et al., 2016).
Immunotherapy is aimed at augmenting immune responses and/or inhibiting the suppressive activity by tumors from immune system’s antitumor attack. Earlier immunotherapy drugs, such as recombinant cytokine administration, were used to activate and expand the immune system to fight against tumors. Most notable, was the administration of high dose interleukin-2 (IL-2), which is approved for management of metastatic renal cell carcinoma and melanoma (Schreiber et al., 2011). IFN-alpha is also one of the earliest approved immune-based adjuvant therapies used for patients with resected high-risk melanoma (Hancock et al., 2004). These are less commonly used today because of their toxic effects which warranted the development of newer advanced agents.
Current Advances in Immunotherapy
Recently more advanced approaches have arisen as the immune suppressive mechanisms exploited by tumor cells became clearer. Agents targeting the programmed death -1 receptor and its ligand (PD-1 and PD-L1) axis, termed as immune checkpoint inhibitors (ICIs) have emerged as effective therapeutic approaches in lung tumors because it restores T-cell mediated immunity (Pardoll, 2012). In 2015, nivolumab, an antibody disrupting the PD-1 and PD-L1 pathway was approved as the first immune checkpoint inhibitor for NSCLC (Altorki et al., 2019). Since then, other ICIs have been approved to be used in different stages of lung cancer. Pembrolizumab is the standard treatment for patients having PD-L1 expression greater than 50% whereas nivolumab and atezolizumab are all available options for patients who do not respond to treatment after platinum doublet chemotherapy (Sapalidis et al., 2018; Lim et al., 2020). By extension, the immune check inhibitors may be beneficial in pulmonary metastases if the extra thoracic primary tumor expresses high levels of PD-L1. Other check point inhibitors target the CTLA-4 axis which limits the proliferation and survival of T cells. CTLA-4 antibodies turn off this inhibitory mechanism leading to the activation of cytotoxic T cells involve in killing tumors (Zhao et al., 2018). However, emerging research has shown that, the mechanism behind therapeutic CTLA-4 antibodies is their ability to cause selective depletion of Tregs in the tumor microenvironment (Liu and Zheng, 2018; Hoy et al., 2019). Some examples are ipilimumab and tremelimumab, with ipilimumab being the first drug in this category to be approved for clinical use as part of first line or second line regimen for treating advanced melanoma (Lipson and Drake, 2011; Robert et al., 2011). In May 2020, the FDA approved the combination of nivolumab, an ICI, plus Ipilimumab (anti-CTLA4 antibody) as first line regimen for patients with metastatic NSCLC cancer with PD-L1 tumor expression ≥1%. Thus, CTLA-4 responsive tumors may be efficacious in preventing pulmonary metastasis. An additional immunotherapy recently developed is the chimeric antigen receptor (CAR) T-cells therapy. It has been very successful in treating hematological malignancies but has been disappointing thus far in solid tumors due to lack of antigenic targets (Chen et al., 2019). The identification of some antigenic targets on lung cancers such as mesothelin, disialoganglioside (GD2), MUC 1, NY-ESO-1, human epidermal growth factor and epidermal growth factor receptor has begun research into testing the efficacy of CAR T-cells therapy in lung cancer (Hu et al., 2020). Currently, CAR T-cell therapy targeting the above mentioned antigens are in different stages of clinical trials as potential immunotherapy against thoracic malignancies (Kiesgen et al., 2018; Oliveres et al., 2018).
Other immunotherapy strategies include the use of monoclonal antibodies that target receptors that play crucial roles in the lung microenvironment signaling pathways to support tumor growth in the lung (Hanahan and Coussens, 2012; Pitt et al., 2016). For example, bevacizumab, a VEGF antibody inhibiting tumor angiogenesis has been approved for use in combination with chemotherapy for management of advance, non-squamous NSCLC (Pilotto et al., 2014).
Table 2 provides a list of current immunotherapies used in primary and secondary lung cancers.
Pulmonary Delivery Approaches to Manage Lung Cancer and Secondary Lung Metastasis
The Historical Use and Benefit Nasal-Pulmonary Drug Delivery Systems
The pulmonary route, either intranasally or via inhalation is a common form of drug delivery. It has been used in the treatment of respiratory diseases such as asthma, chronic obstructive pulmonary disease (COPD) and viral infections (e.g., influenza) that affect the airways where corticosteroids, beta-2 adrenergic agonist and antiviral agents are administered directly into the lung (Levin et al., 1996; Mossad, 2003; Lee et al., 2010). The advantages are well known including accessibility, efficient high drug concentrations in the lung and hence a minimal amount of the drug is needed to achieve higher efficacy with minimal side effects in the above-mentioned conditions (Rau, 2005). Currently, there is considerable attention in formulation of drugs for pulmonary delivery for substantial reasons. Pulmonary delivery is not affected by first pass metabolism as the lungs exhibit very low metabolic activity (Pilcer and Amighi, 2010). Importantly, the lung's anatomy aids its use as an entryway of drugs. It has a large surface area (100 m2) and thin absorption membrane (0.1–0.2 μm) that supports rapid absorption of substances including drugs and high blood flow (5 L/min) which quickly distributes molecules throughout the body. This supports the reason for the use of pulmonary route to treat systemic diseases such as diabetes (inhaled insulin) (Chan and Cheng-Lai, 2017), making it ideal to be considered as a viable option in the treatment of primary lung cancer and secondary lung metastasis.
Inhaled Chemotherapy
In recent years, pulmonary delivery has been considered an improved drug delivery approach in delivering chemotherapeutic drugs locally to the tumor site in the lungs as it offers several benefits such as increase in the amount of drug deposited in tumors, less drug concentration required to achieve efficacy and minimal toxicity due to limited systemic distribution of drugs (Zarogoulidis et al., 2012b; Zarogoulidis et al., 2012c; Goel et al., 2013; Sardeli et al., 2020). Evaluation of this delivery approach in human subjects has shown promising results. For example, inhaled 5-fluorouracil (5-FU) in human subjects led to higher drug concentrations in tumors than in surrounding tissues (Tatsumura et al., 1993). Zarogoulidis et al. also showed that inhaled carboplatin provided improved anticancer efficacy and prolonged survival of patients with NSCLC compared with patients who received intravenous carboplatin (Zarogoulidis et al., 2012b). However, the physicochemical properties of chemotherapeutics such as molecular weight and solubility, may limit their penetration and accumulation into pulmonary tumors despite improved localization of these drugs in the lung following pulmonary delivery (Minchinton and Tannock, 2006; Zarogoulidis et al., 2012a). Again, the nature of the tumor including size, cellularity and density of interstitial tissues may limit drug penetration following pulmonary administration (Mangal et al., 2017). These characteristics ultimately decrease the anti-tumor efficacy of chemotherapeutics following pulmonary delivery and may lead to unwanted side effects due to the potential exposure of healthy cells in the lung to the toxic effects of chemotherapeutics due to their inability to penetrate and accumulate into tumors.
Nanotechnology has been used to offset similar barriers in the administration of systemic chemotherapy (Senapati et al., 2018). The success of this approach has fomented an interest in exploring the prospects of incorporating chemotherapeutics into nano-based systems such as liposomes, polymeric nanoparticles and micelles capable of pulmonary delivery. Nanoparticles are able to penetrate and accumulate in solid tumors by a phenomenon known as the enhance permeability and retention effect (Mohanty et al., 2012) due to the uniqueness of tumor tissue vasculature (poor fenestrations) and inefficient lymphatic drainage. Also, unlike free drug, nanoparticle encapsulated drug can be taken up directly by tumor cells via endocytosis leading to enhanced accumulation of the drug in tumors (Guo et al., 2018). Importantly, nanoparticle encapsulated chemotherapeutics are more efficacious and safer because they are able to accumulate more in pulmonary tumor tissues, thereby sparing healthy cells due to less systemic drug circulation. Nanoparticle encapsulating chemotherapeutic agents delivered via the pulmonary route have been shown to have increased efficacy in treatment of primary lung tumors and inhibiting metastasis from an existing extra-pulmonary primary tumor. For example, Inhaled lipid coated formulation of 5-FU achieved sustained drug release and enhanced anticancer properties (Hitzman, et al., 2006a; Hitzman, et al., 2006b). Lemarie et al. also showed that pulmonary delivery of nanoparticle encapsulating gemcitabine leads to minimal systemic cytotoxicity due to lower plasma drug concentration (Lemarie et al., 2011). In addition, nanoparticles can be surface functionalized with a targeting moiety such as molecules that are only expressed on the surface of tumors to allow for targeted delivery of drugs (Mout et al., 2012). This receptor ligand conjugation approach further enhances the concentration of drug locally with reduced doses and less cytotoxicity to healthy tissues. However, the adverse effects of pulmonary administration of chemotherapeutic drugs including its effect on the lung parenchyma needs to be properly assessed. Neurotoxicity was noted to be associated with pulmonary delivery of paclitaxel liposome aerosol as treatment to inhibit pulmonary metastasis from an extra-pulmonary tumor (Koshkina et al., 2001). Inhaled doxorubicin has been associated with severe cardiotoxicity (Hershey et al., 1999). Two phase I and phase I/II trials indicated metallic taste, mild bronchospasm and moderate reduction of pulmonary function test as side-effects associated with aerosol treatments (Zarogoulidis et al., 2012a).
Inhaled Immunotherapy
The efficacy of chemotherapeutics administered into the lungs via pulmonary delivery supports the potential for a strategy to be employed for the purpose of immunotherapy delivery approaches via the nasal-pulmonary tract. The potential advantages are a safe and non-invasive alternative to current approaches that require direct injection of immunomodulators into tumors (Hamid et al., 2020) which are typically hard to reach by direct injection such as those located in the lungs. Notwithstanding, in situations that require repeated injection to maintain immune responses, the pulmonary administration offers a noninvasive approach allowing for non-invasive repeated treatment modalities that directly target the lung without the potential for systemic adverse effects. In efforts to reduce toxicities from systemic administration of IL-2, the pulmonary route has been investigated as a viable alternative where low dose IL-2 has shown some efficacy for the treatment of lung metastasis in patients with melanoma and renal carcinoma (Enk et al., 2000; Huland et al., 2003; Posch et al., 2014). Recently in efforts to make ICIs more efficacious while reducing side effects, methods of sustained and tumor targeted delivery of ICIs are being explored. Some of the delivery strategies currently under investigation include: the use of viral vectors, delivery using bacteria and delivery of ICIs as DNA encoded monoclonal antibodies (Engeland et al., 2014; Lin et al., 2017; Perales-Puchalt et al., 2019; Reul et al., 2019; Gurbatri et al., 2020). However, most of the above delivery strategies require intratumoral or intravenous injections requiring hospitalization. This makes pulmonary delivery of ICIs an attractive alternative that requires much attention and research in treating both primary lung cancer and secondary lung metastasis as pulmonary delivery such as inhalation can easily be performed in an outpatient setting.
Pulmonary Delivery of Therapeutic Lung Cancer Vaccine as Part of Inhaled Immunotherapy
Dendritic Cell-Based Cancer Vaccines
The immune system does not only recognize and eliminate substances that are non-self but also generates memory after eliminating these substances (Schreiber et al., 2011). This forms the basis of vaccination; the ability of the immune system to mount a robust and specific immune response upon exposure to a previously eliminated antigen. This approach of inducing immunity is being used to mobilize antitumor immunity to help patients that do not benefit enough from current immunotherapies such as checkpoint inhibitors. The direct induction of antitumor immunity by vaccination relies on dendritic cells (DC) because of the unique role they play as part of the immune system where they serve as a bridge between the innate and adaptive immunity by initiation and directing the adaptive immune response (Harari et al., 2020). There are two broad strategies in which DCs are being used to promote anticancer immunity. The first approach involves the release of tumor antigens into the body either through in situ vaccination approaches or direct introduction of tumor antigen carried by a vaccine vector and the ex-vivo approach, which relies on loading patients DCs with tumor antigen in vitro followed by administration of those DCs back to the patient (Disis, 2014). Although studies have shown less clinical benefits, DC-based vaccines could still be an essential part of an integrated anticancer strategy in managing tumors occurring in the lungs. This will require the identification of a suitable tumor antigen capable of invoking robust anticancer immunity, an appropriate vaccine vector for delivery of the antigen and an appropriate delivery strategy into the body.
Cancer Vaccine Antigens
Therapeutic cancer vaccines either employs whole tumor cells or lysates which contains a broad range of antigenic repertoire or a single/few selected and validated cancer antigens in the form of peptide or recombinant proteins. Most cancer vaccines target tumor associated antigens (TAAs), which are abnormally or overexpressed proteins by cancer cells due to mutations in proteins that drive their transcription. TAAs include cancer germline antigens which are normally expressed only in trophoblast tissue and germline cells (e.g., MAGE- A1, MAGE A3, and NY-ESO-1), cell lineage differentiation antigen, which are normally expressed by cells early in life but usually absent during adulthood (e.g., prostate specific antigen (PSA) and prostate acid phosphatase (PAP) and antigens that are present at relatively low levels on normal cells but overexpressed by cancer cells (e.g., HER2 and MUC-1). Because they are not exclusively expressed by cancer cell but are expressed by certain normal cells early on in life or at relatively low levels, immune activation to these antigens is low because T cells and B cells that strongly recognize these antigens are clonally deleted from the repertoire during negative selection. Vaccination with TAAs therefore require strong vaccine adjuvants and repeated vaccination to generate robust immune response capable of immunological memory. However, this may lead to the expansion of self-reactive T cells causing autoimmune disease. For instance, Palmer et al., showed that adoptive transfer of CD8+ T cells specific for melanoma associated antigen triggered severe ocular autoimmunity in mice as this antigen is also expressed in eye melanoma cells (Palmer et al., 2008). The potency and off target effects of vaccines must be critically evaluated when it involves TAAs. The etiology of approximately 10% of human cancers is viral infection. This means that vaccination to prevent or treat viral infection that induces the cancer can lead to cancer prevention. For example, vaccination against Hepatitis B Virus, a known cause of hepatocellular carcinoma is known to decrease both the rate of infection and incidence of hepatocellular carcinoma.
Neoantigens are non-self-proteins that arise due to cancer specific mutations. They could specifically be recognized by T-cell receptors specific for the antigen in the groove of MHC molecules. In this regard, neoantigens are potentially ideal targets for the construction of therapeutic cancer vaccines. Unlike TAAs, neoantigens are unlikely to mediate immune tolerance and autoimmunity when used with strong vaccine adjuvants. The expression of neoantigens is driven by mutations hence they differ in patients having same type of tumor. The benefit of neoantigen as a cancer vaccine is fully realized when personalized for each patient type where the patient tumor genome is sequenced to identify mutations from which neoantigens are expressed. The identified neoantigen can then be delivered to the patient to induce immune response (Castle et al., 2019). The advantage of using a selected few of validate cancer antigens is the generation of a more focused immune response. However, the ability of tumors to downregulate the expression of antigenic targets may render such vaccines ineffective (Odunsi et al., 2007). This can be corrected with the use of whole tumor cell lysate that generates a strong and broad polyclonal T cell responses from its polyvalent antigens hence preventing the emergence of tumors that are immune-resistant because of their ability to downregulate the expression of some antigenic targets (Chiang et al., 2015). In addition, the use of whole tumor cell lysate does not require prior knowledge of the patient’s HLA haplotype and allows for the induction of both CD4+ and CD8+ T cells (González et al., 2014). However, such mechanisms cannot be guaranteed with the use of peptides and recombinant proteins. The disadvantage of using whole tumor cell lysate is that they contain self-antigen that can mediate tolerance and immunoregulatory cytokines and factors that dampens immune response. Whole tumor cell lysate may contain IL-10 and transforming growth factor which inhibits the expression of MHC molecules and co stimulatory molecules by APCs and inhibits T-cell proliferation (Loercher et al., 1999), major histocompatibility complex class 1 related proteins A and B (MICA and MICB) that inhibits killing of tumors mediated by immune cells expression of NKG2D (Groh et al., 2002), Fas ligand which may cause lymphocyte death (Rabinowich et al., 1998) and VEGF which suppresses DCs function (Dikov et al., 2005). Inducing immunogenic cell death (ICD) in tumor cells through irradiation, HOCl oxidation and hyperthermia treatments prior to extraction of whole tumor cell lysate have been found to increase the immunogenicity of the lysate (Vandenberk et al., 2015). ICD leads to the expression of NKG2D ligands, heat shock proteins and calreticulin on the surface of cells and release of immunostimulatory factors that can stimulate immune effectors (Zhou et al., 2019). The priming and boost approach can also help derive the full benefit from whole tumor cell lysate (Harari et al., 2020). In this approach, cancer specific T-cell responses are first induced by administration of whole tumor cell lysate in the priming phase. The patient’s immunological response is then analyzed to identify and characterize effective cancer epitopes. The validated cancer antigens are then used to prepare a personalized synthetic vaccine which is administered to the patient to sustain T cell responses in the boosting phase. Therapeutic cancer vaccine against lung cancer in the form of pulsing patients’ dendritic cells in vitro with whole tumor cell lysate followed by administration of the dendritic cells to the patients showed mixed responses in patients (Um et al., 2010; Kamigaki et al., 2013).
Utility of the Pulmonary Route for Delivery of Cancer Vaccine Vectors—A Case for the use of Nanoparticles
The selection of an appropriate vaccine vector capable of pulmonary delivery of antigens to dendritic cells in the lung and regional lymph nodes may be helpful in generating a robust mucosal immunity in the lung that can eliminate a primary lung tumor or prevent the seeding of an existing extra-primary tumor to the lung. Although some works have been done with regards to respiratory infections where the pulmonary administration of vaccines has been shown to induce robust mucosal immunity against infections when studied using influenza, tuberculosis and more recently Covid-19 models (Bhide et al., 2018; Hassan et al., 2020; Jiang et al., 2020), the pulmonary route has so far not been considered in delivering vaccines against lung tumors although it offers a promising alternative for generating robust respiratory antitumor immunity capable of restricting the growth of primary lung tumors and preventing the seeding of extra pulmonary tumors in the lung. So far, DC—based vaccines have been delivered to individuals through intravenous, subcutaneous, intradermal, intralymphaitc and intratumoral route (Fong et al., 2001; Dohnal et al., 2007; West et al., 2009). For lung occurring tumors, the intravenous and subcutaneous route have been exploited as a way of vaccine delivery into the body to combat lung lesions (Um et al., 2010; Wurz et al., 2014). The pulmonary delivery of DCs that have been primed in vitro by a tumor antigen or administration of antigens to prime DCs in the lungs can lead to the sensitization of a large percentage of T-cells in the lungs. Easy accessibility, non-invasiveness and the potential of administration in the out-patient setting are advantages of vaccine delivery by the pulmonary route.
Currently, cancer vaccine vectors that have been studied include cellular vaccines, virus vector vaccine and molecular vaccine (Hollingsworth and Jansen, 2019). Cellular vaccines include the use of killed tumor cells or adoptive transfer of antigen presenting cells (APCs) from the cancer patient loaded with tumor antigens. Bacteria and yeast can be used as vectors in the formulation of cellular vaccine to deliver tumor antigens (Nascimento and Leite, 2012). Viral vaccine vectors involve the use of viruses that encode a tumor antigen to stimulate immune response against the tumor antigen (Choi and Chang, 2013). Molecular vaccine has to do with the administration of a peptide derived from the tumor antigen or DNA/RNA that encodes a tumor antigen or peptide to drive immune response. Pulmonary delivery of these vaccine vectors to induce lung specific immunity have so far been tested against respiratory infections in mice. For example, Jiang et al. showed that there was generation of specific lung and localized immune response against mycobacterium tuberculosis following intranasal administration of mycobacterium antigens carried by the vector Listeria ivanovii (Jiang et al., 2020). Intranasal delivery of peptides and DNA vaccine have also been proven to induce lung specific immune response in mice (Tesoro Cruz et al., 2008; Yang J. et al., 2019). Immune response against vectors (viral or bacterial) carrying a tumor antigen can neutralize the vector, limiting repeated vaccination which is required to generate immune response capable of immunological memory (Saxena et al., 2013). This can be a drawback with the use of vectors (viral or bacterial) for pulmonary vaccine delivery. Again, the administration of naked proteins, peptides and DNA are easily cleared by mucosal ciliary movement before they are able to induce immune response.
In view of this, nanotechnology is emerging as an approach to target anticancer therapies to the lung including their potential use in activation of immune responses in the lung (Marasini et al., 2017; Al-Halifa et al., 2019; Alshweiat et al., 2019). Nanotechnology have been used to target antigens to dendritic cells either in vivo or ex vivo as a way of improving the clinical outcomes of therapeutic cancer vaccines as summarized in Table 3. Nanoparticles protect antigens susceptible to degradation by proteases and enhance their cellular uptake by antigen presenting cells (APCs), they allow for co-loading and co-delivery of antigens and adjuvants in a sustained released manner enhancing the priming of cytosolic T-lymphocytes (CTL) (Goldberg, 2015). During active targeting, the surface of nanoparticles can be decorated with DC receptor ligands such as ligands for Fc receptors and the C-type lectin receptor family leading to the induction of strong immune response compared to non-targeted nanoparticles conjugates encapsulating antigens (Tacken et al., 2007). These therapeutic cancer vaccines based on nano formulations can be targeted to dendritic cells in the lung via pulmonary delivery. Besides the potential to generate potent mucosal immunity due to the direct delivery of antigens and adjuvants into the lungs where proteolytic activity is low, it offers a noninvasive alternative route to deliver vaccines (Al-Halifa et al., 2019). These advantages of pulmonary deliver of nano-based vaccines make it ideal to considered as a viable model in the management of lung occurring tumors where it can serve as adjunct treatment for primary lung cancer to mobilize the body’s immune system to fight the tumor following chemotherapy and/or radiation treatment especially in cases where the tumor has not spread beyond the lung draining lymph nodes. Again, protection against secondary lung metastasis from an existing primary tumor can be achieved following pulmonary administration of a nano-based vaccine against the primary tumor.
The deposition of particles in the respiratory tract following inhalation is size dependent such that particles smaller than the average mesh spacing of the airway mucus are able to penetrate it, escaping physiological mucus clearance to be deposited in the alveolar spaces of the lung parenchyma where their clearance is delayed (Schneider et al., 2017). Once particles get in the gas exchange region, the main mechanism of clearance is by alveolar macrophages. Particles less than 200 nm are less efficiently cleared increasing their retention time in the lung parenchyma compared to larger particles (Blank, et al., 2013). These make nanoparticles ideal for targeting antigens to dendritic cells in the lung parenchyma. Following the deposition of antigens, these antigens are required to be picked up by resident dendritic cells and migrate to a regional lymph node where they can activate naïve T-cells to induce anti-tumor immunity. Smaller particles, however, can easily traffic to the lymph nodes from the site of delivery where they will encounter a high proportion of antigen presenting and naïve T-lymphocytes that can be activated to generate anti-tumor immunity (Choi et al., 2010). For nanoparticles targeting dendritic cells in the lungs, size is important to ensure effective antitumor immunity. Nanoparticle size ranging from 20 to 50 nm are detected in higher quantities in the lung draining lymph node compared to larger nanoparticles (Blank et al., 2013). Again, higher frequencies of nanoparticles ranging from 20 to 50 nm are trafficked by lung parenchyma dendritic cells to regional lymph nodes where they induce stronger antigen specific CD4 T -cell proliferation compared to larger nanoparticle size (Nochi et al., 2010; Blank et al., 2013). However, intranasal administration of cationic liposome-hyaluronic acid hybrid with average size of 250 nm was able to induce antigen specific immunity (Fan et al., 2015).
The behavior of inhaled particles (aerodynamic behavior) is guided by their aerodynamic diameter which is the diameter of a sphere of unit density and affects how they are deposited in the lungs. Particles with aerodynamic diameter >5 μm are deposited predominately in the upper airways by inertial impaction due their inability to change their trajectory with tidal air (Sturm and Hofmann, 2009). Particles with aerodynamic diameter between 1 and 5 μm are predominately deposited in the lower airways involving the bronchioles and alveoli by gravitational sedimentation (Rissler et al., 2017). Particles with aerodynamic diameter to be less than 1 μm do not get deposited but remain suspended in the airstream leading to their likelihood to be exhaled following inhalation (Mangal et al., 2017; Rissler et al., 2017). Because nanoparticles have the tendency to aggregate to form uncontrolled sizes leading to inconsistent and unpredictable aerosolization, nanoparticles are often administered as particles/droplets with an aerodynamic diameter of 1–5 μm (Desai, 2012) to enhance their delivery in the lung parenchyma. This is facilitated with the use of nebulizers and metered dose inhalers (MDIs) that are able to convert nanoparticle suspensions into inhalable droplets.
Although the surface charge of nanoparticles does not impact their distribution in the lung, it influences their interaction with lung dendritic cells. Positively charged nanoparticles are preferentially taken up by lung dendritic cells whiles their negatively charged counterparts are engulfed by alveolar macrophages leading to their clearance (Fromen et al., 2016). This means that cationic nanoparticles are more successful at inducing stronger adaptive immune response following pulmonary delivery.
The use of nanoparticle-based vaccines for pulmonary delivery continues to show promise. For example, Nochi et al. created an adjuvant free intranasal vaccine from nanogels made from self-assembly pullulan polymers modified with cholesteryl and clostridium botulinum type A neurotoxin that was able to induce both IgG and IgA antibodies response (Nochi et al., 2010). Fan et al. induced potent humoral immune responses through the intranasal delivery of cationic liposome-hyaluronic acid hybrid nanoparticles made to deliver antigens against Yersinia pestis (Fan et al., 2015). Li et al. have developed carbon dots nanoparticles capable of intranasal delivery that enhanced immunization efficacy by significantly increasing humoral immunity and memory T-cell formation (Li S. et al., 2018). These examples together with the recent study by Zhao and his coworkers where they synthesized an inhalable phosphatidylserine coated liposome loaded with immunostimulants (STING agonist cyclic guanosine monophosphate-adenosine monophosphate—cGAMP) that stimulated APCs activation and cross presentation and synergizes with radiation treatment to protect against secondary lung metastasis following the occurrence of a primary breast tumor after intranasal administration (Liu et al., 2019) show that nanoparticles can be used to deliver tumor antigens to induce antitumor respiratory immunity following pulmonary delivery.
Host derived extracellular vehicles (EVs) such as exosomes are also emerging as attractive vehicles to target antigens to dendritic cells. Exosomes are nano-sized EVs (size ranging from 30 to 100 nm) that play important roles in cell-to-cell communication (Meng et al., 2019). Endogenous drug delivery systems such as exosomes have advantage over synthetic nano formulations because of their native biocompatibility in vivo which helps them enhance drug delivery and therapeutic efficiency (Batrakova and Kim, 2015; Peng and Mu, 2016). Tumor derived exosomes are rich sources of tumor rejection antigen capable of inducing tumor specific immunity (Luan et al., 2017). However, tumor cell derived exosomes can also contain immunosuppressive proteins that dampens immune response enabling tumor cells to escape immune surveillance (Xu et al., 2020). Antigen presenting cells such as dendritic cells derived exosomes have shown great promise in delivery of tumor antigens to induce antitumor immunity (Whiteside, 2016). They have the capacity to transfer peptide -MHC complexes that have been exposed to an antigen to other DCs that have not encountered similar antigens (Wahlund et al., 2017). The pulmonary delivery of exosomes loaded with a tumor antigen can serve as an appropriate drug delivery platform to treat lung tumors.
The Conceptual Framework
Therapeutic cancer vaccines have been used to treat tumors and prevent metastasis by targeting the primary tumor. If the seed soil hypothesis by Stephen Paget that explains metastasis is anything to go by, then targeting the soil (future metastatic sites) may serve as a means to prevent metastasis. The seed soil hypothesis proposes that, the primary tumor secreted factors prepares the soil (future metastatic sites) for colonization by detached cells form the primary tumor (seed) that reach the distal organ either through circulation or the lymphatics. As stated in the introduction one of the events that occurs in the future metastatic sites as a result of molecular and cellular changes fashioned by the tumor derived factors is immune suppression. For the seed to successfully colonize future metastatic sites they have to evade immune surveillance by CD8+ T-cells and NK cells that directly kill tumor cells. Tumor cells derived chemokines and cytokines recruits suppressive and regulatory immune cells such myeloid derived suppressor cells (MDSCs), tumor associated macrophages (TAMs), Tumor associated neutrophils and Tregs into distal organs to support metastasis by inducing immunosuppression. Pulmonary Tregs, MDSCs and alveolar macrophages have been shown to restrain antitumor T-cells response. Targeted induction of respiratory immunity can reverse the immunosuppression induced in the lung prior to lung metastasis from an extra pulmonary malignancy by creating an immune protective microenvironment to prevent and/or minimize the seeding of tumors in the lungs. This has great potential to serve as a prophylactic approach to preclude immune evasion by CTCs that reach the lungs. Although many studies have demonstrated the promise of mobilizing antitumor immunity using vaccination strategies, its efficacy in the protection against lung metastases, particularly in the context of existing extra pulmonary primary tumor development has not been tested. The design of an appropriate vaccine capable of intranasal administration may serve as a prophylactic cancer vaccine to prevent lung metastasis from extra pulmonary malignancies by generating robust mucosal anti-tumor immunity in the lungs. Its success will have a significant impact on the number of deaths caused by extra-pulmonary malignancies. The ease of administration, as such therapy can be self-administered will be an added advantage (Figure 2).
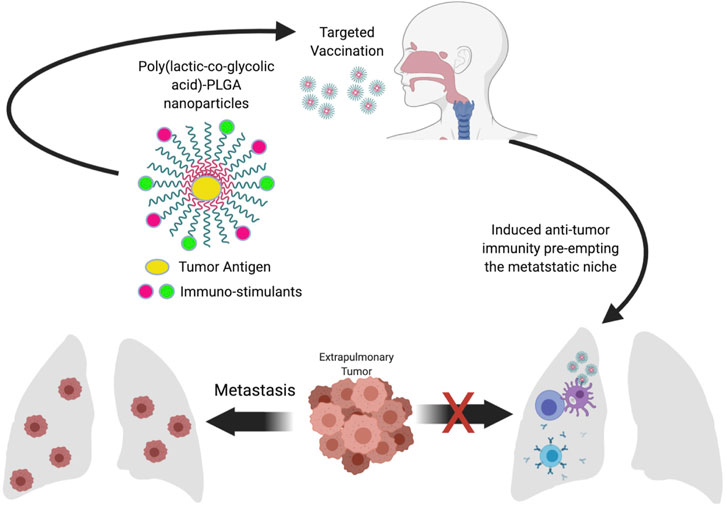
FIGURE 2. Conceptual framework. Pulmonary administration of immunostimulant coated nanoparticles encapsulating tumor antigens induced lung specific anti-tumor immunity capable of forestalling immunosuppression by the premetastatic niche leading to the ability of immunosurveillance mediated by cytotoxic T-cells and antibodies to clear disseminated tumor cells and prevent the colonization of the lung by the existing extra-pulmonary tumor.
Author Contributions
There remains current unmet need in reducing mortality associated with primary and metastatic lung cancer. This review sheds light on the past, present and future prospects of nasal-pulmonary route as an advanced approach of delivering immune-based therapeutics for the treatment of primary and metastatic lung cancer. Insights drawn from this review is expected to lead to further knowledge prompting anti-cancer research.
Funding
Research reported in this publication was supported by the National Institute of Cancer Research of the Health under Award 1 P20 CA233355-01 (Vishwanatha).
Conflict of Interest
The authors declare that the research was conducted in the absence of any commercial or financial relationships that could be construed as a potential conflict of interest.
Acknowledgments
The authors declare that the research was conducted in the absence of any commercial or financial relationships that could be construed as a potential conflict of interest.The authors would like to thank JC, C N, and KL for their contribution in review of this manuscript. Research reported in this publication was supported by the National Institute of Cancer Research of the Health under Award P20CA233355-03 (Vishwanatha); Project Lead: Jones (5173).
Glossary
NSCLC Non-small cell lung cancer
SCLC Small cell lung cancer
CTCs Circulating tumor cells
DTCs Disseminated tumor cells
BMDCs Bone marrow derived cells
MHC Major histocompatibility complex
PD-L1 Programmed death-ligand 1
PD-1 Programmed cell death protein 1
WNT Wingless-related integration site
B-catenin Beta-catenin
PTEN Phosphate and tensin homolog
HIF-1 alpha Hypoxia-inducible factor 1-alpha
IFN-alpha Interferon-alpha
ICIs Immune check point inhibitors
CTLA4 Cytotoxic T-lymphocyte-associated protein 4
Tregs Regulatory T cells
VEGF Vascular endothelial growth factor
CAR T-Cells Chimeric antigen receptor T cells
HER-2 Human epidermal growth factor receptor-2
EGFR Epidermal growth factor receptor
ALK Anaplastic lymphoma kinase
IL-2 Interleukin-2
TAA Tumor associated antigen
MAGE-A1 Melanoma-associated antigen 1
MAGE-A3 Melanoma-associated antigen 3
NY-ESO-1 New York esophageal squamous cell carcinoma 1
PSA Prostate-specific antigen
PAP Papanicolaou test
ICD Immunogenic cell death
References
Agolli, L., Bracci, S., Nicosia, L., Valeriani, M., De Sanctis, V., and Osti, M. F. (2017). Lung metastases treated with stereotactic ablative radiation therapy in oligometastatic colorectal cancer patients: outcomes and prognostic factors after long-term follow-up. Clin. Colorectal Cancer 16 (1), 58–64. doi:10.1016/j.clcc.2016.07.004
Akita, H., Marubashi, S., Wada, H., Hama, N., Kawamoto, K., Kobayashi, S., et al. (2015). Combination therapy with S-1 and interferon-α in hepatocellular carcinoma patients with lung metastasis. Mol. Clin. Oncol. 3 (2), 322–328. doi:10.3892/mco.2014.463
Al-Halifa, S., Gauthier, L., Arpin, D., Bourgault, S., and Archambault, D. (2019). Nanoparticle-based vaccines against respiratory viruses. Front. Immunol. 10, 22. doi:10.3389/fimmu.2019.00022
Alshweiat, A., Ambrus, R., and Csóka, I. (2019). Intranasal nanoparticulate systems as alternative route of drug delivery. Cmc 26 (35), 6459–6492. doi:10.2174/0929867326666190827151741
Altorki, N. K., Markowitz, G. J., Gao, D., Port, J. L., Saxena, A., Stiles, B., et al. (2019). The lung microenvironment: an important regulator of tumour growth and metastasis. Nat. Rev. Cancer 19 (1), 9–31. doi:10.1038/s41568-018-0081-9
Barnes, H., See, K., Barnett, S., and Manser, R. (2017). Surgery for limited-stage small-cell lung cancer. Cochrane Database Syst. Rev. 4 (4), CD011917. doi:10.1002/14651858.CD011917.pub2
Batrakova, E. V., and Kim, M. S. (2015). Using exosomes, naturally-equipped nanocarriers, for drug delivery. J. Controlled Release 219, 396–405. doi:10.1016/j.jconrel.2015.07.030
Bhide, Y., Tomar, J., Dong, W., de Vries-Idema, J., Frijlink, H. W., Huckriede, A., et al. (2018). Pulmonary delivery of influenza vaccine formulations in cotton rats: site of deposition plays a minor role in the protective efficacy against clinical isolate of H1N1pdm virus. Drug Deliv. 25 (1), 533–545. doi:10.1080/10717544.2018.1435748
Bianchi, M., Sun, M., Jeldres, C., Shariat, S. F., Trinh, Q.-D., Briganti, A., et al. (2012). Distribution of metastatic sites in renal cell carcinoma: a population-based analysis. Ann. Oncol. 23 (4), 973–980. doi:10.1093/annonc/mdr362
Blank, F., Stumbles, P. A., Seydoux, E., Holt, P. G., Fink, A., Rothen-Rutishauser, B., et al. (2013). Size-dependent uptake of particles by pulmonary antigen-presenting cell populations and trafficking to regional lymph nodes. Am. J. Respir. Cel Mol. Biol. 49 (1), 67–77. doi:10.1165/rcmb.2012-0387oc
Bray, F., Ferlay, J., Soerjomataram, I., Siegel, R. L., Torre, L. A., and Jemal, A. (2018). Global cancer statistics 2018: GLOBOCAN estimates of incidence and mortality worldwide for 36 cancers in 185 countries. CA: A Cancer J. Clinicians 68 (6), 394–424. doi:10.3322/caac.21492
Cacan, E. (2017). Epigenetic-mediated immune suppression of positive co-stimulatory molecules in chemoresistant ovarian cancer cells. Cell Biol. Int. 41 (3), 328–339. doi:10.1002/cbin.10729
Carvajal, C., Navarro-Martin, A., Cacicedo, J., Ramos, R., and Guedea, F. (2015). Stereotactic body radiotherapy for colorectal lung oligometastases: preliminary single-institution results. J. Buon. 20 (1), 158–165.
Casey, S. C., Tong, L., Li, Y., Do, R., Walz, S., Fitzgerald, K. N., et al. (2016). MYC regulates the antitumor immune response through CD47 and PD-L1. Science 352 (6282), 227–231. doi:10.1126/science.aac9935
Castle, J. C., Uduman, M., Pabla, S., Stein, R. B., and Buell, J. S. (2019). Mutation-derived neoantigens for cancer immunotherapy. Front. Immunol. 10, 1856. doi:10.3389/fimmu.2019.01856
Cha, B. G., Jeong, J. H., and Kim, J. (2018). Extra-large pore mesoporous silica nanoparticles enabling Co-delivery of high amounts of protein antigen and toll-like receptor 9 agonist for enhanced cancer vaccine efficacy. ACS Cent. Sci. 4 (4), 484–492. doi:10.1021/acscentsci.8b00035
Chan, J., and Cheng-Lai, A. (2017). Inhaled insulin. Cardiol. Rev. 25 (3), 140–146. doi:10.1097/crd.0000000000000143
Chen, J., López-Moyado, I. F., Seo, H., Lio, C.-W. J., Hempleman, L. J., Sekiya, T., et al. (2019). NR4A transcription factors limit CAR T cell function in solid tumours. Nature 567 (7749), 530–534. doi:10.1038/s41586-019-0985-x
Chen, R., Tao, Y., Xu, X., Shan, L., Jiang, H., Yin, Q., et al. (2018). The efficacy and safety of nivolumab, pembrolizumab, and atezolizumab in treatment of advanced non-small cell lung cancer. Discov. Med. 26 (143), 155–166.
Chiang, C., Coukos, G., and Kandalaft, L. (2015). Whole tumor antigen vaccines: where are we? Vaccines 3 (2), 344–372. doi:10.3390/vaccines3020344
Cho, N.-H., Cheong, T.-C., Min, J. H., Wu, J. H., Lee, S. J., Kim, D., et al. (2011). A multifunctional core-shell nanoparticle for dendritic cell-based cancer immunotherapy. Nat. Nanotech. 6 (10), 675–682. doi:10.1038/nnano.2011.149
Choi, H. S., Ashitate, Y., Lee, J. H., Kim, S. H., Matsui, A., Insin, N., et al. (2010). Rapid translocation of nanoparticles from the lung airspaces to the body. Nat. Biotechnol. 28 (12), 1300–1303. doi:10.1038/nbt.1696
Choi, Y., and Chang, J. (2013). Viral vectors for vaccine applications. Clin. Exp. Vaccin. Res. 2 (2), 97–105. doi:10.7774/cevr.2013.2.2.97
Chojniak, R., Yu, L. S., and Younes, R. N. (2006). Response to chemotherapy in patients with lung metastases: how many nodules should be measured? Cancer Imaging 6 (1), 107–112. doi:10.1102/1470-7330.2006.0017
Claisse, G., Thaunat, O., Mousson, C., Wood, K. J., Rifle, G., and Mariat, C. (2017). How to escape the immune response. Transplantation 101 (12), 2825–2829. doi:10.1097/tp.0000000000001639
Cruz, L. J., Rueda, F., Cordobilla, B., Simón, L., Hosta, L., Albericio, F., et al. (2011). Targeting nanosystems to human DCs via Fc receptor as an effective strategy to deliver antigen for immunotherapy. Mol. Pharmaceutics 8 (1), 104–116. doi:10.1021/mp100178k
D’Ambrosio, N., Lyo, J. K., Young, R. J., Haque, S. S., and Karimi, S. (2010). Imaging of metastatic CNS neuroblastoma. Am. J. Roentgenology 194 (5), 1223–1229. doi:10.2214/ajr.09.3203
Davis, Z. B., Felices, M., Verneris, M. R., and Miller, J. S. (2015). Natural killer cell adoptive transfer therapy. Cancer J. 21 (6), 486–491. doi:10.1097/ppo.0000000000000156
Desai, N. (2012). Challenges in development of nanoparticle-based therapeutics. Aaps J. 14 (2), 282–295. doi:10.1208/s12248-012-9339-4
Dikov, M. M., Ohm, J. E., Ray, N., Tchekneva, E. E., Burlison, J., Moghanaki, D., et al. (2005). Differential roles of vascular endothelial growth factor receptors 1 and 2 in dendritic cell differentiation. J. Immunol. 174 (1), 215–222. doi:10.4049/jimmunol.174.1.215
Disis, M. L. (2014). Mechanism of action of Immunotherapy, Semin. oncol. 41, S3–S13. doi:10.1053/j.seminoncol.2014.09.004
Dohnal, A. M., Witt, V., Hügel, H., Holter, W., Gadner, H., and Felzmann, T. (2007). Phase I study of tumor Ag-loaded IL-12 secreting semi-mature DC for the treatment of pediatric cancer. Cytotherapy 9 (8), 755–770. doi:10.1080/14653240701589221
Elayadi, M., Magdy, S., Khalil, E., and Zekri, W. (2020). Management and outcome of pediatric metastatic wilms’ tumor at the national cancer Institute, Egypt. J. Egypt. Natl. Canc. Inst. 32 (1), 19. doi:10.1186/s43046-020-00031-7
Engeland, C. E., Grossardt, C., Veinalde, R., Bossow, S., Lutz, D., Kaufmann, J. K., et al. (2014). CTLA-4 and PD-L1 checkpoint blockade enhances oncolytic measles virus therapy. Mol. Ther. 22 (11), 1949–1959. doi:10.1038/mt.2014.160
Enk, A. H., Nashan, D., Robben, A., and Knop, J. r. (2000). High dose inhalation interleukin-2 therapy for lung metastases in patients with malignant melanoma. Cancer 88 (9), 2042–2046. doi:10.1002/(sici)1097-0142(20000501)88:9<2042::aid-cncr9>3.0.co;2-j
Fan, Y., Sahdev, P., Ochyl, L. J., Akerberg, J., and Moon, J. J. (2015). Cationic liposome-hyaluronic acid hybrid nanoparticles for intranasal vaccination with subunit antigens. J. Controlled Release 208, 121–129. doi:10.1016/j.jconrel.2015.04.010
Fang, R. H., Hu, C.-M. J., Luk, B. T., Gao, W., Copp, J. A., Tai, Y., et al. (2014). Cancer cell membrane-coated nanoparticles for anticancer vaccination and drug delivery. Nano Lett. 14 (4), 2181–2188. doi:10.1021/nl500618u
Ferlay, J., Colombet, M., Soerjomataram, I., Mathers, C., Parkin, D. M., Piñeros, M., et al. (2019). Estimating the global cancer incidence and mortality in 2018: GLOBOCAN sources and methods. Int. J. Cancer 144 (8), 1941–1953. doi:10.1002/ijc.31937
Filippi, A. R., Guerrera, F., Badellino, S., Ceccarelli, M., Castiglione, A., Guarneri, A., et al. (2016). Exploratory analysis on overall survival after either surgery or stereotactic radiotherapy for lung oligometastases from colorectal cancer. Clin. Oncol. 28 (8), 505–512. doi:10.1016/j.clon.2016.02.001
Fong, L., Brockstedt, D., Benike, C., Wu, L., and Engleman, E. G. (2001). Dendritic cells injected via different routes induce immunity in cancer patients. J. Immunol. 166 (6), 4254–4259. doi:10.4049/jimmunol.166.6.4254
Fromen, C. A., Rahhal, T. B., Robbins, G. R., Kai, M. P., Shen, T. W., Luft, J. C., et al. (2016). Nanoparticle surface charge impacts distribution, uptake and lymph node trafficking by pulmonary antigen-presenting cells. Nanomedicine: Nanotechnology, Biol. Med. 12 (3), 677–687. doi:10.1016/j.nano.2015.11.002
Gago, J. P., Câmara, G., Dionísio, J., and Opinião, A. (2016). Pulmonary metastasis as sole manifestation of relapse in previously treated localised prostate cancer: three exceptional case reports. Ecancermedicalscience 10, 645. doi:10.3332/ecancer.2016.645
Gandhi, L., Rodríguez-Abreu, D., Gadgeel, S., Esteban, E., Felip, E., De Angelis, F., et al. (2018). Pembrolizumab plus chemotherapy in metastatic non-small-cell lung cancer. N. Engl. J. Med. 378 (22), 2078–2092. doi:10.1056/nejmoa1801005
Ganti, A. K., Zhen, W., and Kessinger, A. (2007). Limited-stage small-cell lung cancer: therapeutic options. Oncol. 21 (3), 303–323.
Goel, A., Sahni, J., Ali, J., and Baboota, S. (2013). Exploring targeted pulmonary delivery for treatment of lung cancer. Int. J. Pharma Investig. 3 (1), 8–14. doi:10.4103/2230-973x.108959
Goldberg, M. S. (2015). Immunoengineering: how nanotechnology can enhance cancer immunotherapy. Cell 161 (2), 201–204. doi:10.1016/j.cell.2015.03.037
González, F. E., Gleisner, A., Falcón-Beas, F., Osorio, F., López, M. N., and Salazar-Onfray, F. (2014). Tumor cell lysates as immunogenic sources for cancer vaccine design. Hum. Vaccin. Immunother. 10 (11), 3261–3269. doi:10.4161/21645515.2014.982996
Groh, V., Wu, J., Yee, C., and Spies, T. (2002). Tumour-derived soluble MIC ligands impair expression of NKG2D and T-cell activation. Nature 419 (6908), 734–738. doi:10.1038/nature01112
Guo, P., Liu, D., Subramanyam, K., Wang, B., Yang, J., Huang, J., et al. (2018). Nanoparticle elasticity directs tumor uptake. Nat. Commun. 9 (1), 130. doi:10.1038/s41467-017-02588-9
Gurbatri, C. R., Lia, I., Vincent, R., Coker, C., Castro, S., Treuting, P. M., et al. (2020). Engineered probiotics for local tumor delivery of checkpoint blockade nanobodies. Sci. Translational Med. 12 (530). doi:10.1126/scitranslmed.aax0876
Hamid, O., Ismail, R., and Puzanov, I. (2020). Intratumoral immunotherapy-update 2019. Oncol. 25 (3), e423–e438. doi:10.1634/theoncologist.2019-0438
Hanahan, D., and Coussens, L. M. (2012). Accessories to the crime: functions of cells recruited to the tumor microenvironment. Cancer Cell 21 (3), 309–322. doi:10.1016/j.ccr.2012.02.022
Hancock, B. W., Wheatley, K., Harris, S., Ives, N., Harrison, G., Horsman, J. M., et al. (2004). Adjuvant interferon in high-risk melanoma: the AIM HIGH study-United Kingdom coordinating committee on cancer research randomized study of adjuvant low-dose extended-duration interferon alfa-2a in high-risk resected malignant melanoma. Jco 22 (1), 53–61. doi:10.1200/jco.2004.03.185
Harari, A., Graciotti, M., Bassani-Sternberg, M., and Kandalaft, L. E. (2020). Antitumour dendritic cell vaccination in a priming and boosting approach. Nat. Rev. Drug Discov. 19 (9), 635–652. doi:10.1038/s41573-020-0074-8
Harris, M. B., Gieser, P., Goorin, A. M., Ayala, A., Shochat, S. J., Ferguson, W. S., et al. (1998). Treatment of metastatic osteosarcoma at diagnosis: a pediatric oncology group study. Jco 16 (11), 3641–3648. doi:10.1200/jco.1998.16.11.3641
Hassan, A. O., Kafai, N. M., Dmitriev, I. P., Fox, J. M., Smith, B. K., Harvey, I. B., et al. (2020). A single-dose intranasal Chad vaccine protects upper and lower respiratory tracts against SARS-CoV-2. Cell 183 (1), 169–184. doi:10.1016/j.cell.2020.08.026
Hassan, H. A. F. M., Smyth, L., Wang, J. T.-W., Costa, P. M., Ratnasothy, K., Diebold, S. S., et al. (2016). Dual stimulation of antigen presenting cells using carbon nanotube-based vaccine delivery system for cancer immunotherapy. Biomaterials 104, 310–322. doi:10.1016/j.biomaterials.2016.07.005
Hershey, A. E., Kurzman, I. D., Forrest, L. J., Bohling, C. A., Stonerook, M., Placke, M. E., et al. (1999). Inhalation chemotherapy for macroscopic primary or metastatic lung tumors: proof of principle using dogs with spontaneously occurring tumors as a model. Clin. Cancer Res. 5 (9), 2653–2659.
Hitzman, C. J., Elmquist, W. F., Wattenberg, L. W., and Wiedmann, T. S. (2006a). Development of a respirable, sustained release microcarrier for 5-fluorouracil I: in vitro assessment of liposomes, microspheres, and lipid coated nanoparticles. J. Pharm. Sci. 95 (5), 1114–1126. doi:10.1002/jps.20591
Hitzman, C. J., Elmquist, W. F., and Wiedmann, T. S. (2006b). Development of a respirable, sustained release microcarrier for 5-fluorouracil II: in vitro and in vivo optimization of lipid coated nanoparticles. J. Pharm. Sci. 95 (5), 1127–1143. doi:10.1002/jps.20590
Hollingsworth, R. E., and Jansen, K. (2019). Turning the corner on therapeutic cancer vaccines. NPJ Vaccin. 4, 7. doi:10.1038/s41541-019-0103-y
Hoshino, A., Costa-Silva, B., Shen, T.-L., Rodrigues, G., Hashimoto, A., Tesic Mark, M., et al. (2015). Tumour exosome integrins determine organotropic metastasis. Nature 527 (7578), 329–335. doi:10.1038/nature15756
Hotta, K., Aoe, K., Kozuki, T., Ohashi, K., Ninomiya, K., Ichihara, E., et al. (2018). A phase II study of trastuzumab emtansine in HER2-positive non-small cell lung cancer. J. Thorac. Oncol. 13 (2), 273–279. doi:10.1016/j.jtho.2017.10.032
Howlader, N., Noone, A. M., Krapcho, M., Miller, D., Brest, A., and Yu, M. (2020). SEER cancer statistics review, 1975–2017. Bethesda, MD: National Cancer Institute.
Hoy, H., Lynch, T., and Beck, M. (2019). Surgical treatment of lung cancer. Crit. Care Nurs. Clin. North America 31 (3), 303–313. doi:10.1016/j.cnc.2019.05.002
Hu, Z., Zheng, X., Jiao, D., Zhou, Y., Sun, R., Wang, B., et al. (2020). LunX-CAR T cells as targeted therapy for non-small-cell lung cancer. Mole. Ther. Oncolytics 17, 361–370. doi:10.1016/j.omto.2020.04.008
Huang, J. L., Chen, H. Z., and Gao, X. L. (2018). Lipid-coated calcium phosphate nanoparticle and beyond: a versatile platform for drug delivery. J. Drug Target. 26 (5-6), 398–406. doi:10.1080/1061186x.2017.1419360
Huland, E., Burger, A., Fleischer, J., Fornara, P., Hatzmann, E., Heidenreich, A., et al. (2003). Efficacy and safety of inhaled recombinant interleukin-2 in high-risk renal cell cancer patients compared with systemic interleukin-2: an outcome study. Folia Biol. (Praha) 49 (5), 183–190.
Imperatori, A., La Salvia, D., Rotolo, N., Nardecchia, E., Bandera, M., Toungoussova, O., et al. (2010). Five-year survival of stage IIIA-IIIB (non-N3) non-small cell lung cancer patients after platinum/gemcitabine induction chemotherapy and surgery. J. Chemother. 22 (3), 191–196. doi:10.1179/joc.2010.22.3.191
Iwama, T., Uchida, T., Sawada, Y., Tsuchiya, N., Sugai, S., Fujinami, N., et al. (2016). Vaccination with liposome-coupled glypican-3-derived epitope peptide stimulates cytotoxic T lymphocytes and inhibits GPC3-expressing tumor growth in mice. Biochem. Biophysical Res. Commun. 469 (1), 138–143. doi:10.1016/j.bbrc.2015.11.084
Jiang, M.-J., Liu, S.-J., Su, L., Zhang, X., Li, Y.-Y., Tang, T., et al. (2020). Intranasal vaccination with Listeria ivanovii as vector of Mycobacterium tuberculosis antigens promotes specific lung-localized cellular and humoral immune responses. Scientific Rep. 10 (1), 302. doi:10.1038/s41598-019-57245-6
Kamigaki, T., Kaneko, T., Naitoh, K., Takahara, M., Kondo, T., Ibe, H., et al. (2013). Immunotherapy of autologous tumor lysate-loaded dendritic cell vaccines by a closed-flow electroporation system for solid tumors. Anticancer Res. 33 (7), 2971–2976.
Karim, S. M., and Zekri, J. (2012). Chemotherapy for small cell lung cancer: a comprehensive review. Oncol. Rev. 6 (1), e4. doi:10.4081/oncol.2012.e4
Kiesgen, S., Chicaybam, L., Chintala, N. K., and Adusumilli, P. S. (2018). Chimeric antigen receptor (CAR) T-cell therapy for thoracic malignancies. J. Thorac. Oncol. 13 (1), 16–26. doi:10.1016/j.jtho.2017.10.001
Kokate, R. A., Chaudhary, P., Sun, X., Thamake, S. I., Maji, S., Chib, R., et al. (2016). Rationalizing the use of functionalized poly-lactic-co-glycolic acid nanoparticles for dendritic cell-based targeted anticancer therapy. Nanomedicine 11 (5), 479–494. doi:10.2217/nnm.15.213
Kong, J., Tian, H., Zhang, F., Zhang, Z., Li, J., Liu, X., et al. (2019). Extracellular vesicles of carcinoma-associated fibroblasts creates a pre-metastatic niche in the lung through activating fibroblasts. Mol. Cancer 18 (1), 175. doi:10.1186/s12943-019-1101-4
Koshkina, N. V., Waldrep, J. C., Roberts, L. E., Golunski, E., Melton, S., and Knight, V. (2001). Paclitaxel liposome aerosol treatment induces inhibition of pulmonary metastases in murine renal carcinoma model. Clin. Cancer Res. 7 (10), 3258–3262.
Koyama, S., Akbay, E. A., Li, Y. Y., Aref, A. R., Skoulidis, F., Herter-Sprie, G. S., et al. (2016). STK11/LKB1 deficiency promotes neutrophil recruitment and proinflammatory cytokine production to suppress T-cell activity in the lung tumor microenvironment. Cancer Res. 76 (5), 999–1008. doi:10.1158/0008-5472.can-15-1439
Kranz, L. M., Diken, M., Haas, H., Kreiter, S., Loquai, C., Reuter, K. C., et al. (2016). Systemic RNA delivery to dendritic cells exploits antiviral defence for cancer immunotherapy. Nature 534 (7607), 396–401. doi:10.1038/nature18300
Lackey, A., and Donington, J. (2013). Surgical management of lung cancer. Semin. Intervent Radiol. 30 (2), 133–140. doi:10.1055/s-0033-1342954
Lee, I.-H., Kwon, H.-K., An, S., Kim, D., Kim, S., Yu, M. K., et al. (2012). Imageable antigen-presenting gold nanoparticle vaccines for effective cancer immunotherapy in vivo. Angew. Chem. Int. Ed. 51 (35), 8800–8805. doi:10.1002/anie.201203193
Lee, J. K., Suh, D. I., and Koh, Y. Y. (2010). The role of inhaled and/or nasal corticosteroids on the bronchodilator response. Korean J. Pediatr. 53 (11), 951–956. doi:10.3345/kjp.2010.53.11.951
Lee, S. H., Gupta, M. K., Bang, J. B., Bae, H., and Sung, H.-J. (2013). Current progress in reactive oxygen species (ROS)-responsive materials for biomedical applications. Adv. Healthc. Mater. 2 (6), 908–915. doi:10.1002/adhm.201200423
Lemarie, E., Vecellio, L., Hureaux, J., Prunier, C., Valat, C., Grimbert, D., et al. (2011). Aerosolized gemcitabine in patients with carcinoma of the lung: feasibility and safety study. J. Aerosol Med. Pulm. Drug Deliv. 24 (6), 261–270. doi:10.1089/jamp.2010.0872
Levin, D. C., Little, K. S., Laughlin, K. R., Galbraith, J. M., Gustman, P. M., Murphy, D., et al. (1996). Addition of anticholinergic solution prolongs bronchodilator effect of beta 2 agonists in patients with chronic obstructive pulmonary disease. Am. J. Med. 100 (1), 40s–48s. doi:10.1016/s0002-9343(96)80073-8
Li, S., Guo, Z., Zeng, G., Zhang, Y., Xue, W., and Liu, Z. (2018). Polyethylenimine-modified fluorescent carbon dots as vaccine delivery system for intranasal immunization. ACS Biomater. Sci. Eng. 4 (1), 142–150. doi:10.1021/acsbiomaterials.7b00370
Li, B. T., Shen, R., Buonocore, D., Olah, Z. T., Ni, A., Ginsberg, M. S., et al. (2018). Ado-trastuzumab emtansine for patients with HER2-mutant lung cancers: results from a phase II basket trial. Jco 36 (24), 2532–2537. doi:10.1200/jco.2018.77.9777
Lim, S. M., Hong, M. H., and Kim, H. R. (2020). Immunotherapy for non-small cell lung cancer: current landscape and future perspectives. Immune Netw. 20 (1), e10. doi:10.4110/in.2020.20.e10
Lin, A. H., Twitty, C. G., Burnett, R., Hofacre, A., Mitchell, L. A., Espinoza, F. L., et al. (2017). Retroviral replicating vector delivery of miR-PDL1 inhibits immune checkpoint PDL1 and enhances immune responses in vitro. Mol. Ther.—Nucleic Acids 6, 221–232. doi:10.1016/j.omtn.2016.11.007
Lipson, E. J., and Drake, C. G. (2011). Ipilimumab: an anti-CTLA-4 antibody for metastatic melanoma. Clin. Cancer Res. 17 (22), 6958–6962. doi:10.1158/1078-0432.ccr-11-1595
Liu, Y., and Cao, X. (2016). Characteristics and significance of the pre-metastatic niche. Cancer Cell 30 (5), 668–681. doi:10.1016/j.ccell.2016.09.011
Liu, Y., Crowe, W. N., Wang, L., Lu, Y., Petty, W. J., Habib, A. A., et al. (2019). An inhalable nanoparticulate STING agonist synergizes with radiotherapy to confer long-term control of lung metastases. Nat. Commun. 10 (1), 5108. doi:10.1038/s41467-019-13094-5
Liu, Y., Gu, Y., Han, Y., Zhang, Q., Jiang, Z., Zhang, X., et al. (2016). Tumor exosomal RNAs promote lung pre-metastatic niche formation by activating alveolar epithelial TLR3 to recruit neutrophils. Cancer Cell 30 (2), 243–256. doi:10.1016/j.ccell.2016.06.021
Liu, Y., and Zheng, P. (2018). How does an anti-CTLA-4 antibody promote cancer immunity? Trends Immunol. 39 (12), 953–956. doi:10.1016/j.it.2018.10.009
Loercher, A. E., Nash, M. A., Kavanagh, J. J., Platsoucas, C. D., and Freedman, R. S. (1999). Identification of an IL-10-producing HLA-DR-negative monocyte subset in the malignant ascites of patients with ovarian carcinoma that inhibits cytokine protein expression and proliferation of autologous T cells. J. Immunol. 163 (11), 6251–6260.
Lu, T., Yang, X., Huang, Y., Zhao, M., Li, M., Ma, K., et al. (2019). Trends in the incidence, treatment, and survival of patients with lung cancer in the last four decades. Cmar 11, 943–953. doi:10.2147/cmar.s187317
Luan, X., Sansanaphongpricha, K., Myers, I., Chen, H., Yuan, H., and Sun, D. (2017). Engineering exosomes as refined biological nanoplatforms for drug delivery. Acta Pharmacol. Sin. 38 (6), 754–763. doi:10.1038/aps.2017.12
Mangal, S., Gao, W., Li, T., and Zhou, Q. (2017). Pulmonary delivery of nanoparticle chemotherapy for the treatment of lung cancers: challenges and opportunities. Acta Pharmacol. Sin. 38 (6), 782–797. doi:10.1038/aps.2017.34
Mankor, J. M., Disselhorst, M. J., Poncin, M., Baas, P., Aerts, J. G. J. V., and Vroman, H. (2020). Efficacy of nivolumab and ipilimumab in patients with malignant pleural mesothelioma is related to a subtype of effector memory cytotoxic T cells: translational evidence from two clinical trials. EBioMedicine 62, 103040. doi:10.1016/j.ebiom.2020.103040
Marasini, N., Skwarczynski, M., and Toth, I. (2017). Intranasal delivery of nanoparticle-based vaccines. Ther. Deliv. 8 (3), 151–167. doi:10.4155/tde-2016-0068
Maru, Y. (2015). The lung metastatic niche. J. Mol. Med. 93 (11), 1185–1192. doi:10.1007/s00109-015-1355-2
Matikas, A., Kentepozidis, Ν., Ardavanis, A., Vaslamatzis, M., Polyzos, A., Emmanouilides, C., et al. (2016). Efficacy and tolerance of frontline bevacizumab-based chemotherapy for advanced non-small cell lung cancer patients: a multicenter, phase IV study of the Hellenic Oncology Research Group (HORG). Cancer Chemother. Pharmacol. 78 (2), 369–376. doi:10.1007/s00280-016-3094-7
McElnay, P., and Lim, E. (2014). Adjuvant or neoadjuvant chemotherapy for NSCLC. J. Thorac. Dis. 6 (2), S224–S227. doi:10.3978/j.issn.2072-1439.2014.04.26
Meng, W., Hao, Y., He, C., Li, L., and Zhu, G. (2019). Exosome-orchestrated hypoxic tumor microenvironment. Mol. Cancer 18 (1), 57. doi:10.1186/s12943-019-0982-6
Mieszawska, A. J., Mulder, W. J. M., Fayad, Z. A., and Cormode, D. P. (2013). Multifunctional gold nanoparticles for diagnosis and therapy of disease. Mol. Pharmaceutics 10 (3), 831–847. doi:10.1021/mp3005885
Min, Y., Roche, K. C., Tian, S., Eblan, M. J., McKinnon, K. P., Caster, J. M., et al. (2017). Antigen-capturing nanoparticles improve the abscopal effect and cancer immunotherapy. Nat. Nanotech. 12 (9), 877–882. doi:10.1038/nnano.2017.113
Minchinton, A. I., and Tannock, I. F. (2006). Drug penetration in solid tumours. Nat. Rev. Cancer 6 (8), 583–592. doi:10.1038/nrc1893
Mitry, E., Guiu, B., Cosconea, S., Jooste, V., Faivre, J., and Bouvier, A.-M. (2010). Epidemiology, management and prognosis of colorectal cancer with lung metastases: a 30-year population-based study. Gut 59 (10), 1383–1388. doi:10.1136/gut.2010.211557
Mohammed, T.-L. H., Chowdhry, A., Reddy, G. P., Amorosa, J. K., Brown, K., Dyer, D. S., et al. (2011). ACR appropriateness criteria screening for pulmonary metastases. J. Thorac. Imaging 26 (1), W1–W3. doi:10.1097/rti.0b013e3182010bf9
Mohanty, N., Moore, D., Xu, Z., Sreeprasad, T. S., Nagaraja, A., Rodriguez, A. A., et al. (2012). Nanotomy-based production of transferable and dispersible graphene nanostructures of controlled shape and size. Nat. Commun. 3, 844. doi:10.1038/ncomms1834
Mossad, S. B. (2003). Demystifying FluMist, a new intranasal, live influenza vaccine. Cleveland Clinic J. Med. 70 (9), 801–806. doi:10.3949/ccjm.70.9.801
Mout, R., Moyano, D. F., Rana, S., and Rotello, V. M. (2012). Surface functionalization of nanoparticles for nanomedicine. Chem. Soc. Rev. 41 (7), 2539–2544. doi:10.1039/c2cs15294k
Nascimento, I. P., and Leite, L. C. C. (2012). Recombinant vaccines and the development of new vaccine strategies. Braz. J. Med. Biol. Res. 45 (12), 1102–1111. doi:10.1590/s0100-879x2012007500142
Neuman, H. B., Patel, A., Hanlon, C., Wolchok, J. D., Houghton, A. N., and Coit, D. G. (2007). Stage-IV melanoma and pulmonary metastases: factors predictive of survival. Ann. Surg. Oncol. 14 (10), 2847–2853. doi:10.1245/s10434-007-9448-y
Nochi, T., Yuki, Y., Takahashi, H., Sawada, S.-i., Mejima, M., Kohda, T., et al. (2010). Nanogel antigenic protein-delivery system for adjuvant-free intranasal vaccines. Nat. Mater. 9 (7), 572–578. doi:10.1038/nmat2784
Noguchi, T., Ward, J. P., Gubin, M. M., Arthur, C. D., Lee, S. H., Hundal, J., et al. (2017). Temporally distinct PD-L1 expression by tumor and host cells contributes to immune escape. Cancer Immunol. Res. 5 (2), 106–117. doi:10.1158/2326-6066.cir-16-0391
Odunsi, K., Qian, F., Matsuzaki, J., Mhawech-Fauceglia, P., Andrews, C., Hoffman, E. W., et al. (2007). Vaccination with an NY-ESO-1 peptide of HLA class I/II specificities induces integrated humoral and T cell responses in ovarian cancer. Proc. Natl. Acad. Sci. 104 (31), 12837–12842. doi:10.1073/pnas.0703342104
Oliveres, H., Caglevic, C., Passiglia, F., Taverna, S., Smits, E., and Rolfo, C. (2018). Vaccine and immune cell therapy in non-small cell lung cancer. J. Thorac. Dis. 10 (13), S1602–S1614. doi:10.21037/jtd.2018.05.134
Palmer, D. C., Chan, C.-C., Gattinoni, L., Wrzesinski, C., Paulos, C. M., Hinrichs, C. S., et al. (2008). Effective tumor treatment targeting a melanoma/melanocyte-associated antigen triggers severe ocular autoimmunity. Proc. Natl. Acad. Sci. 105 (23), 8061–8066. doi:10.1073/pnas.0710929105
Pandya, P. H., Murray, M. E., Pollok, K. E., and Renbarger, J. L. (2016). The immune system in cancer pathogenesis: potential therapeutic approaches. J. Immunol. Res. 2016, 4273943. doi:10.1155/2016/4273943
Pardoll, D. M. (2012). The blockade of immune checkpoints in cancer immunotherapy. Nat. Rev. Cancer 12 (4), 252–264. doi:10.1038/nrc3239
Peinado, H., Zhang, H., Matei, I. R., Costa-Silva, B., Hoshino, A., Rodrigues, G., et al. (2017). Pre-metastatic niches: organ-specific homes for metastases. Nat. Rev. Cancer 17 (5), 302–317. doi:10.1038/nrc.2017.6
Peng, Q., and Mu, H. (2016). The potential of protein-nanomaterial interaction for advanced drug delivery. J. Controlled Release 225, 121–132. doi:10.1016/j.jconrel.2016.01.041
Peng, W., Chen, J. Q., Liu, C., Malu, S., Creasy, C., Tetzlaff, M. T., et al. (2016). Loss of PTEN promotes resistance to T cell-mediated immunotherapy. Cancer Discov. 6 (2), 202–216. doi:10.1158/2159-8290.cd-15-0283
Perales-Puchalt, A., Duperret, E. K., Muthumani, K., and Weiner, D. B. (2019). Simplifying checkpoint inhibitor delivery through in vivo generation of synthetic DNA-encoded monoclonal antibodies (DMAbs). Oncotarget 10 (1), 13–16. doi:10.18632/oncotarget.26535
Perica, K., Tu, A., Richter, A., Bieler, J. G., Edidin, M., and Schneck, J. P. (2014). Magnetic field-induced T cell receptor clustering by nanoparticles enhances T cell activation and stimulates antitumor activity. ACS Nano 8 (3), 2252–2260. doi:10.1021/nn405520d
Pilcer, G., and Amighi, K. (2010). Formulation strategy and use of excipients in pulmonary drug delivery. Int. J. Pharm. 392 (1-2), 1–19. doi:10.1016/j.ijpharm.2010.03.017
Pilotto, S., Bonomi, M., Massari, F., Milella, M., Ciuffreda, L., Brunelli, M., et al. (2014). Anti-angiogenic drugs and biomarkers in non-small-cell lung cancer: a “hard days night”. Cpd 20 (24), 3958–3972. doi:10.2174/13816128113196660757
Pirker, R., and Filipits, M. (2012). Translational lung cancer research Cetuximab in non-small-cell lung cancer. Transl Lung Cancer Res. 1, 54–60. doi:10.3978/j.issn.2218-6751.11.01
Pitt, J. M., Marabelle, A., Eggermont, A., Soria, J.-C., Kroemer, G., and Zitvogel, L. (2016). Targeting the tumor microenvironment: removing obstruction to anticancer immune responses and immunotherapy. Ann. Oncol. 27 (8), 1482–1492. doi:10.1093/annonc/mdw168
Posch, C., Weihsengruber, F., Bartsch, K., Feichtenschlager, V., Sanlorenzo, M., Vujic, I., et al. (2014). Low-dose inhalation of interleukin-2 bio-chemotherapy for the treatment of pulmonary metastases in melanoma patients. Br. J. Cancer 110 (6), 1427–1432. doi:10.1038/bjc.2014.62
Rabinowich, H., Reichert, T. E., Kashii, Y., Gastman, B. R., Bell, M. C., and Whiteside, T. L. (1998). Lymphocyte apoptosis induced by Fas ligand- expressing ovarian carcinoma cells. Implications for altered expression of T cell receptor in tumor-associated lymphocytes. J. Clin. Invest. 101 (11), 2579–2588. doi:10.1172/jci1518
Reul, J., Frisch, J., Engeland, C. E., Thalheimer, F. B., Hartmann, J., Ungerechts, G., et al. (2019). Tumor-specific delivery of immune checkpoint inhibitors by engineered AAV vectors. Front. Oncol. 9, 52. doi:10.3389/fonc.2019.00052
Rieber, J., Streblow, J., Uhlmann, L., Flentje, M., Duma, M., Ernst, I., et al. (2016). Stereotactic body radiotherapy (SBRT) for medically inoperable lung metastases-A pooled analysis of the German working group “stereotactic radiotherapy”. Lung Cancer 97, 51–58. doi:10.1016/j.lungcan.2016.04.012
Rissler, J., Gudmundsson, A., Nicklasson, H., Swietlicki, E., Wollmer, P., and Löndahl, J. (2017). Deposition efficiency of inhaled particles (15-5000 nm) related to breathing pattern and lung function: an experimental study in healthy children and adults. Part. fibre Toxicol. 14 (1), 10. doi:10.1186/s12989-017-0190-8
Robert, C., Thomas, L., Bondarenko, I., O'Day, S., Weber, J., Garbe, C., et al. (2011). Ipilimumab plus dacarbazine for previously untreated metastatic melanoma. N. Engl. J. Med. 364 (26), 2517–2526. doi:10.1056/nejmoa1104621
Saginala, K., Barsouk, A., Aluru, J. S., Rawla, P., Padala, S. A., and Barsouk, A. (2020). Epidemiology of bladder cancer. Med. Sci. (Basel) 8 (1), 15. doi:10.3390/medsci8010015
Sandler, A., Gray, R., Perry, M. C., Brahmer, J., Schiller, J. H., Dowlati, A., et al. (2006). Paclitaxel-carboplatin alone or with bevacizumab for non-small-cell lung cancer. N. Engl. J. Med. 355 (24), 2542–2550. doi:10.1056/nejmoa061884
Sapalidis, K., Zarogoulidis, P., Huang, H., Bai, C., Wen, Y., Wang, L., et al. (2018). Inhaled immunotherapy administration for lung cancer; efficient? Certainly possible. J. Cancer 9 (6), 1121–1126. doi:10.7150/jca.24397
Sardeli, C., Zarogoulidis, P., Kosmidis, C., Amaniti, A., Katsaounis, A., Giannakidis, D., et al. (2020). Inhaled chemotherapy adverse effects: mechanisms and protection methods. Lung Cancer Manag. 8 (4), 19. doi:10.2217/lmt-2019-0007
Saxena, M., Van, T. T. H., Baird, F. J., Coloe, P. J., and Smooker, P. M. (2013). Pre-existing immunity against vaccine vectors - friend or foe? Microbiology (Reading, England) 159 (1), 1–11. doi:10.1099/mic.0.049601-0
Schneider, C. S., Xu, Q., Boylan, N. J., Chisholm, J., Tang, B. C., Schuster, B. S., et al. (2017). Nanoparticles that do not adhere to mucus provide uniform and long-lasting drug delivery to airways following inhalation. Sci. Adv. 3 (4), e1601556. doi:10.1126/sciadv.1601556
Schreiber, R. D., Old, L. J., and Smyth, M. J. (2011). Cancer immunoediting: integrating immunity’s roles in cancer suppression and promotion. Science 331 (6024), 1565–1570. doi:10.1126/science.1203486
Senapati, S., Mahanta, A. K., Kumar, S., and Maiti, P. (2018). Controlled drug delivery vehicles for cancer treatment and their performance. Signal. Transduct Target. Ther. 3, 7. doi:10.1038/s41392-017-0004-3
Sharma, P., and Allison, J. P. (2015). The future of immune checkpoint therapy. Science 348 (6230), 56–61. doi:10.1126/science.aaa8172
Shields, C. W. t., Wang, L. L., Evans, M. A., and Mitragotri, S. (2020). Materials for immunotherapy. Adv. Mater. 32 (13), e1901633. doi:10.1002/adma.201901633
Siegel, R. L., Miller, K. D., and Jemal, A. (2020). Cancer statistics. CA A. Cancer J. Clin. 70 (1), 7–30. doi:10.3322/caac.21590
Somasundaram, A., Socinski, M. A., and Villaruz, L. C. (2016). Immune checkpoint blockade in lung cancer. Discov. Med. 22 (119), 55–65.
Song, H., Du, Y., Sgouros, G., Prideaux, A., Frey, E., and Wahl, R. L. (2007). Therapeutic potential of 90Y- and 131I-labeled anti-CD20 monoclonal antibody in treating non-Hodgkin’s lymphoma with pulmonary involvement: a Monte Carlo-based dosimetric analysis. J. Nucl. Med. 48 (1), 150–157.
Speiser, D. E., and Ohashi, P. S. (1998). Activation of cytotoxic T cells by solid tumours? CMLS, Cell. Mol. Life Sci. 54 (3), 263–271. doi:10.1007/s000180050148
Spranger, S., Bao, R., and Gajewski, T. F. (2015). Melanoma-intrinsic β-catenin signalling prevents anti-tumour immunity. Nature 523 (7559), 231–235. doi:10.1038/nature14404
Sturm, R., and Hofmann, W. (2009). A theoretical approach to the deposition and clearance of fibers with variable size in the human respiratory tract. J. Hazard. Mater. 170 (1), 210–218. doi:10.1016/j.jhazmat.2009.04.107
Tacken, P. J., de Vries, I. J. M., Torensma, R., and Figdor, C. G. (2007). Dendritic-cell immunotherapy: from ex vivo loading to in vivo targeting. Nat. Rev. Immunol. 7 (10), 790–802. doi:10.1038/nri2173
Tatsumura, T., Koyama, S., Tsujimoto, M., Kitagawa, M., and Kagamimori, S. (1993). Further study of nebulisation chemotherapy, a new chemotherapeutic method in the treatment of lung carcinomas: fundamental and clinical. Br. J. Cancer 68 (6), 1146–1149. doi:10.1038/bjc.1993.495
Tesoro Cruz, E., Feria Romero, I. A., López Mendoza, J. G., Orozco Suárez, S., Hernández González, R., Favela, F. B., et al. (2008). Efficient post-exposure prophylaxis against rabies by applying a four-dose DNA vaccine intranasally. Vaccine 26 (52), 6936–6944. doi:10.1016/j.vaccine.2008.09.083
Thomas, S. N., Vokali, E., Lund, A. W., Hubbell, J. A., and Swartz, M. A. (2014). Targeting the tumor-draining lymph node with adjuvanted nanoparticles reshapes the anti-tumor immune response. Biomaterials 35 (2), 814–824. doi:10.1016/j.biomaterials.2013.10.003
Timmerman, R., Paulus, R., Galvin, J., Michalski, J., Straube, W., Bradley, J., et al. (2010). Stereotactic body radiation therapy for inoperable early stage lung cancer. JAMA 303 (11), 1070–1076. doi:10.1001/jama.2010.261
Um, S.-J., Choi, Y. J., Shin, H.-J., Son, C. H., Park, Y.-S., Roh, M. S., et al. (2010). Phase I study of autologous dendritic cell tumor vaccine in patients with non-small cell lung cancer. Lung Cancer 70 (2), 188–194. doi:10.1016/j.lungcan.2010.02.006
Vandenberk, L., Belmans, J., Van Woensel, M., Riva, M., and Van Gool, S. W. (2015). Exploiting the immunogenic potential of cancer cells for improved dendritic cell vaccines. Front. Immunol. 6, 663. doi:10.3389/fimmu.2015.00663
Wahlund, C. J. E., Güclüler, G., Hiltbrunner, S., Veerman, R. E., Näslund, T. I., and Gabrielsson, S. (2017). Exosomes from antigen-pulsed dendritic cells induce stronger antigen-specific immune responses than microvesicles in vivo. Sci. Rep. 7 (1), 17095. doi:10.1038/s41598-017-16609-6
Wang, X., Feng, Y., Dong, P., and Huang, J. (2019). A mini review on carbon quantum dots: preparation, properties, and electrocatalytic application. Front. Chem. 7, 671. doi:10.3389/fchem.2019.00671
Wang, X., Li, X., Yoshiyuki, K., Watanabe, Y., Sogo, Y., Ohno, T., et al. (2016). Comprehensive mechanism analysis of mesoporous-silica-nanoparticle-induced cancer immunotherapy. Adv. Healthc. Mater. 5 (10), 1169–1176. doi:10.1002/adhm.201501013
Warwick, R., and Page, R. (2007). Resection of pulmonary metastases from colorectal carcinoma. Eur. J. Surg. Oncol. (EJSO) 33 (2), S59–S63. doi:10.1016/j.ejso.2007.09.018
West, E., Morgan, R., Scott, K., Merrick, A., Lubenko, A., Pawson, D., et al. (2009). Clinical grade OK432-activated dendritic cells. J. Immunother. 32 (1), 66–78. doi:10.1097/cji.0b013e31818be071
Whiteside, T. L. (2016). Tumor-derived exosomes and their role in cancer progression. Adv. Clin. Chem. 74, 103–141. doi:10.1016/bs.acc.2015.12.005
Wu, Q., Li, J., Zhu, S., Wu, J., Chen, C., Liu, Q., et al. (2017). Breast cancer subtypes predict the preferential site of distant metastases: a SEER based study. Oncotarget 8 (17), 27990–27996. doi:10.18632/oncotarget.15856
Wurz, G. T., Kao, C.-J., Wolf, M., and DeGregorio, M. W. (2014). Tecemotide: an antigen-specific cancer immunotherapy. Hum. Vaccin. Immunother. 10 (11), 3383–3393. doi:10.4161/hv.29836
Xia, X., Mai, J., Xu, R., Perez, J. E. T., Guevara, M. L., Shen, Q., et al. (2015). Porous silicon microparticle potentiates anti-tumor immunity by enhancing cross-presentation and inducing type I interferon response. Cell Rep. 11 (6), 957–966. doi:10.1016/j.celrep.2015.04.009
Xiao, W., Zheng, S., Liu, P., Zou, Y., Xie, X., Yu, P., et al. (2018). Risk factors and survival outcomes in patients with breast cancer and lung metastasis: a population-based study. Cancer Med. 7 (3), 922–930. doi:10.1002/cam4.1370
Xu, L., Zhang, G., Song, S., and Zheng, Z. (2019). Surgery for small cell lung cancer: a surveillance, epidemiology, and end results (seer) survey from 2010 to 2015. Medicine 98 (40), e17214. doi:10.1097/md.0000000000017214
Xu, Z., Ramishetti, S., Tseng, Y.-C., Guo, S., Wang, Y., and Huang, L. (2013). Multifunctional nanoparticles co-delivering Trp2 peptide and CpG adjuvant induce potent cytotoxic T-lymphocyte response against melanoma and its lung metastasis. J. Controlled Release 172 (1), 259–265. doi:10.1016/j.jconrel.2013.08.021
Xu, Z., Zeng, S., Gong, Z., and Yan, Y. (2020). Exosome-based immunotherapy: a promising approach for cancer treatment. Mol. Cancer 19 (1), 160. doi:10.1186/s12943-020-01278-3
Yang, J., Luo, Y., Shibu, M. A., Toth, I., and Skwarczynskia, M. (2019). Cell-penetrating peptides: efficient vectors for vaccine delivery. Cdd 16 (5), 430–443. doi:10.2174/1567201816666190123120915
Yang, S., Zhang, Z., and Wang, Q. (2019). Emerging therapies for small cell lung cancer. J. Hematol. Oncol. 12 (1), 47. doi:10.1186/s13045-019-0736-3
Zarogoulidis, P., Chatzaki, K., Porpodis, K., Domvri, K., Hohenforst-Schmidt, W., Schmidt, E. P., et al. (2012a). Inhaled chemotherapy in lung cancer: future concept of nanomedicine. IJN 7, 1551–1572. doi:10.2147/ijn.s29997
Zarogoulidis, P., Eleftheriadou, E., Sapardanis, I., Zarogoulidou, V., Lithoxopoulou, H., Kontakiotis, T., et al. (2012b). Feasibility and effectiveness of inhaled carboplatin in NSCLC patients. Invest. New Drugs 30 (4), 1628–1640. doi:10.1007/s10637-011-9714-5
Zarogoulidis, P., Gialeli, C., and Karamanos, N. K. (2012c). Inhaled chemotherapy in lung cancer: safety concerns of nanocomplexes delivered. Ther. Deliv. 3 (9), 1021–1023. doi:10.4155/tde.12.77
Zelenay, S., van der Veen, A. G., Böttcher, J. P., Snelgrove, K. J., Rogers, N., Acton, S. E., et al. (2015). Cyclooxygenase-dependent tumor growth through evasion of immunity. Cell 162 (6), 1257–1270. doi:10.1016/j.cell.2015.08.015
Zhang, Z., Tongchusak, S., Mizukami, Y., Kang, Y. J., Ioji, T., Touma, M., et al. (2011). Induction of anti-tumor cytotoxic T cell responses through PLGA-nanoparticle mediated antigen delivery. Biomaterials 32 (14), 3666–3678. doi:10.1016/j.biomaterials.2011.01.067
Zhao, Y., Yang, W., Huang, Y., Cui, R., Li, X., and Li, B. (2018). Evolving roles for targeting CTLA-4 in cancer immunotherapy. Cell Physiol. Biochem. 47 (2), 721–734. doi:10.1159/000490025
Keywords: lung, cancer, immune, therapy, nasal, delivery, pulmonary, nanotechnology
Citation: Donkor M and Jones HP (2021) The Proposition of the Pulmonary Route as an Attractive Drug Delivery Approach of Nano-Based Immune Therapies and Cancer Vaccines to Treat Lung Tumors. Front. Nanotechnol. 3:635194. doi: 10.3389/fnano.2021.635194
Received: 30 November 2020; Accepted: 19 January 2021;
Published: 04 March 2021.
Edited by:
Ângela Sousa, University of Beira Interior, PortugalReviewed by:
Rafael Rosell, Catalan Institute of Oncology, SpainNitesh K. Kunda, St. John’s University, United States
Copyright © 2021 Donkor and Jones. This is an open-access article distributed under the terms of the Creative Commons Attribution License (CC BY). The use, distribution or reproduction in other forums is permitted, provided the original author(s) and the copyright owner(s) are credited and that the original publication in this journal is cited, in accordance with accepted academic practice. No use, distribution or reproduction is permitted which does not comply with these terms.
*Correspondence: Harlan P. Jones, aGFybGFuLmpvbmVzQHVudGhzYy5lZHU=