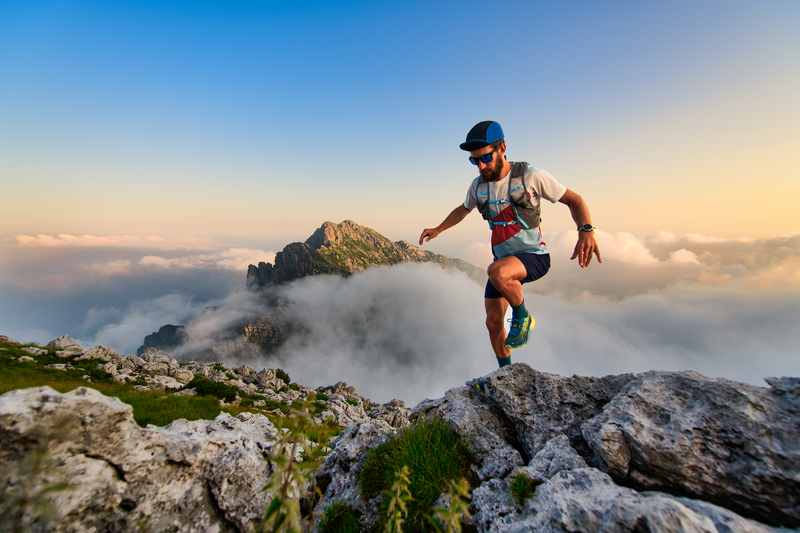
95% of researchers rate our articles as excellent or good
Learn more about the work of our research integrity team to safeguard the quality of each article we publish.
Find out more
MINI REVIEW article
Front. Nanotechnol. , 12 March 2021
Sec. Nanodevices
Volume 3 - 2021 | https://doi.org/10.3389/fnano.2021.633931
Untethered, wirelessly interconnected devices are becoming pervasive in today’s society forming the Internet of Things. These autonomous devices and systems continue to scale to reduced dimensions at the millimeter scale and below, presenting major challenges to how we provide power to these devices. This article surveys existing approaches to harvest energy from the ambient or externally supplied sources including radio-frequency, optical, mechanical, thermal, nuclear, chemical, and biological modalities to provide electrical power for micro- and nano-systems. The outlook for scaling these energy conversion approaches to small dimensions is discussed in the context of both existing technologies and possible future nanoscience developments.
Self-powered systems at the microscale and nanoscale that incorporate sensors, computation, and wireless data communications enable transformative networks for health, safety, and to enrich our lives. The Internet of Things (IoT) has already transformed society in areas including smart homes, medical devices, manufacturing, infrastructure, and transportation. There are currently billions of connected IoT devices, and an estimate of approximately 127 new devices connected to the web every second in 2020 (Maayan, 2020). Scaling down the dimensions of cyber-physical systems to the millimeter scale and below is a continued progression of IoT, termed the Internet of Tiny Things (IoT2) or Internet of Nano Things (IoNT) with completely new functionalities and application spaces due to the large number, density, and integration capabilities at these reduced dimensions. In this work, we will use the term IoT2 to be inclusive of all sub-millimeter systems at both the nanoscale and microscale. IoT2 systems are enabled by a combination of advances including low-power circuits and heterogeneous integration (Oh et al., 2019). These small autonomous devices are often called motes as a part of a vision of “smart dust” (Warneke et al., 2001).
Delivery of sufficient power and energy to autonomous systems is a key requirement for their success. There are a variety of solutions available for power and energy delivery for systems at approximately the centimeter scale and larger, including battery powered systems, direct wired connections, and wireless power delivery. However, scaling dimensions below a centimeter presents new challenges such as rapidly decreasing efficiency of wireless power transfer at millimeter and smaller dimensions (Rabaey et al., 2011), while the system constraints for IoT2 devices cannot utilize conventional integration technologies involving printed circuit boards, current battery technologies, or physically accessible ports for wired connections (e.g., universal serial bus). The approximate power density required for these systems is on the order of 100 nW/mm2 based on recent mm-scale systems. This article will summarize power and energy sources and considerations as systems are reduced to the micro- and nano-scale, with attention devoted specifically to the outlook for IoT2 systems.
A range of energy harvesting approaches are needed for IoT2 devices since there will be differing access to energy sources according to the application of interest. The energy source may be either available through the background ambient, or intentionally provided through external excitation. Various modes are illustrated in Figure 1 and compared in Table 1.
Energy from radio-frequency (RF) sources can be harvested from the ambient or from an intentional supply of radiation. RF technology is easily the most widely used approach for IoT technology, where devices such as RFID tags and readers have been around for decades with wide proliferation from applications ranging from credit cards and card readers to tracking devices for health and safety. These RFID applications use inductive coupling for small chips, but can typically still couple to coil antennas with form factors that are on the centimeter scale or larger. The link efficiency is determined by the coil geometry, coil quality factor, frequency, and distance between transmitter and receiver, where efficiency decreases dramatically when scaling down to dimensions near 1 mm (Rabaey et al., 2011). Reducing dimensions further into the micrometer and nanometer scale, while also maintaining suitable efficiency, will require corresponding increases in frequency and/or novel approaches for deep subwavelength antennas. Scaling to higher frequencies may be practical for some systems in the near field, though propagation losses and efficiency of associated high-frequency electronics must be considered for the nanosystem design. The use of metamaterials may be used to achieve high efficiency at subwavelength dimensions and to achieve directionality (Arslanagić and Ziolkowski, 2018).
Light provides a form of energy that is well suited to scaling to the micrometer and nanometer scale. Energy harvesting can be readily achieved using photovoltaic (PV) cells designed for ambient outdoor or indoor lighting, or additional illumination sources. PV cells can directly provide an operating voltage on the order of 0.5 V, depending on the illumination source and PV cell technology, which may be increased through series connections (Moon et al., 2019) or tandem cell designs (Moon et al., 2020). Scaling down device dimensions below 1 mm can reduce conversion efficiency due to perimeter non-radiative recombination effects in semiconductors (Moon et al., 2017), where passivation of semiconductor surfaces remains a key to achieving high energy conversion efficiency. At higher power density, untethered self-powered sensors have been demonstrated at dimensions of 100 µm (Cortese et al., 2020).
As an alternative to the PV effect, optical energy harvesting may also be achieved using optical antennas. The dimensions of these optical antennas are on the nanometer scale (“nantennas”), and theoretically offer efficiencies great than 60% (Vandenbosch and Ma, 2012). The concept of optical coupling using antennas may also be combined with photovoltaics through the use of plasmons (Jang et al., 2016). Plasmon coupling to PV cells can provide enhanced optical absorption and a means of optical coupling at near and subwavelength dimensions.
Challenges for optical energy harvesting are in requirements for line of sight system configuration and the need for an optical source (for example, sensors embedded in concrete would not be realistic). Variability in ambient light sources, and angular incidence (or line of sight) are extremely important in considering photovoltaic energy harvesting for applications. Instability in light levels will generally require energy storage in the system. Variable light levels can be accommodated by light level detection circuitry to aid power management (Lee et al., 2018).
Mechanical energy from stray vibrations or objects in motion may be harvested using piezoelectric devices (Yang et al., 2018). Piezoelectric energy harvesters are widely used for applications including infrastructure monitoring sensors, tire pressure sensors, and pacemakers. These devices typically use micro-electro-mechanical systems technology utilizing cantilevers or membranes with dimensional scales that are larger than millimeter. Continued dimensional reductions may use nanowire technologies, where piezoelectric nanogenerators have been used to power microelectronics (Xu et al., 2010). While such nanogenerators often utilize arrays of nanowires in an energy harvesting device, power generation has been demonstrated in a single nanowire (Moorthy et al., 2017), showing great promise for dimensional scaling to power nanosystems. Piezoelectric nanogenerators typically provide efficient operation at frequencies on the order of 100 Hz.
Mechanical systems may also generate electricity via the triboelectric effect and electrostatic induction (Wang, 2013). There are typically three different classes of coupling mechanical energy to electricity in these devices: vertical contact separation, in-plane sliding, and single electrodes. These devices have thus far been employed at relatively large dimensions (centimeter scale) where the term “nanogenerator” is a reference to the basis of generating electricity through displacement current rather than the physical dimensions of the device (Wang, 2020). The dimensions can in principle be scaled to sub-mm dimensions, requiring appropriate design of dielectric layers and gap spacing between electrodes. Triboelectric nanogenerators show great promise based on recent advances in improving charge density generation (Liu et al., 2019). Further considerations will require compatibility with the relatively large voltages associated with static electricity discharge.
A special case of mechanical energy harvesting is in the ultrasonic range (approximately 1–20 kHz). Ultrasound provides a low loss pathway for wireless power transfer in biological tissue and has received much attention for biosensor applications. Scaled systems at the sub-mm scale have been demonstrated, and show great promise for realizing “neural dust” for brain-machine interfaces that requiring highly scaled dimensions with relatively small working distances (Seo et al., 2016).
Waste heat can be harvested over a range of modalities including thermoelectric, thermophotovoltaic, and thermoradiative approaches. The thermoelectric approach is based on the Seebeck effect, requiring a temperature gradient across the device. Thermoelectric thin films, superlattices, and quantum dots can effectively be used for power generation at scaled dimensions. Miniaturized systems with an active volume of 1 mm3 have been demonstrated, capable of harvesting 775 µW/mm3 for an external temperature difference of 9 K (Venkatasubramanian et al., 2007). Thermophotovoltaic and thermoradiative approaches rely on radiative transfer, with net received radiation and emitted radiation, respectively. The device efficiency depends strongly on the temperature of the heat source and choice of material to match the bandgap energy to the blackbody emission of the heat radiator. Chip-scale thermophotovoltaic harvesters using microscale heat reactors have been demonstrated over a 1 cm2 area, providing 344 mW of power (Chan et al., 2013). Further scaling of dimensions to the sub-mm scale will be extremely challenging, requiring either extreme scaling of such microreactors, or system operation in close proximity to a heat source at very high temperature. The thermoradiative approach is the inverse of the thermophotovoltaic approach, where heat flux into the device is radiatively emitted to a colder ambient to generate electricity (Byrnes et al., 2014; Strandberg, 2015). Thermoradiative harvesting is still in the exploratory stage with limited experimental demonstrations. In principle, the thermoradiative approach is scalable to the sub-mm scale, where utility for energy harvesting will likely apply to niche applications where systems are in direct contact to a substantial heat flux and/or in an environment with low ambient radiation (e.g., deep space).
Nuclear energy at small scales can generate electrical current in a semiconductor junction through absorption of beta particles from radioactive sources. These devices are commonly referred to as betavoltaic cells or batteries, and require an internal radioactive sources such as tritium or nickel-63. Semiconductor junctions have used a variety of semiconductors including silicon, silicon carbide, and gallium nitride. The betavoltaic technology is continuing to advance within the field of nanoscience, including nanowire based devices (Wagner et al., 2020). Betavoltaics can offer a stable source of power over a long period of time (approximately 10 years or more), and have been used in applications such a implantable medical devices and defense applications requiring tamper-proof source of power. The power density available for betavoltaics is on the order of nW/mm2 (Hang and Lal, 2003), suitable for many low-power systems. Primary concerns with betavoltaics are in health and safety of working with radioactive materials, and in proper shielding and containment in devices. Scaling betavoltaic devices down to sub-mm dimensions will likely be limited to these concerns with packaging to ensure health and safety.
Scaled fuel cells offer a means to use chemical and biological sources to generate electricity in micro and nanosystems. Methanol and ethanol are often used as fuels for proton exchange membrane fuel cells, where devices on the centimeter scale have been commercialized (Kundu et al., 2007). The microreactors in these systems are conventionally fabricated using MEMS technology, where scaling to small dimensions will be limited by the ability to manage gas flow and a fuel cartridge. Microbial fuel cells utilize decomposing organic matter and micro-organisms that can transfer electrons extracellularly (exoelectrogens) to generate electricity. This provides an attractive approach for remote operation of environmental nanosystems that can provide energy from naturally occurring biological material in soil, sediment, or decomposing plant matter (Yamashita et al., 2019). One area where microbial fuel cells have shown success is in biosensors for online monitoring of wastewater (Do et al., 2020). Similar to chemical fuel cells, the scaling of microbial fuel cells will be determined by requirements for controlling flow of fuel. An additional consideration for microbial fuel cells is the start up time associated with microbial colonization, which is typically on the order of several days.
A variety of energy harvesting modalities are available for future development of nanosystems, through either ambient sources or intentionally providing an external source. The cost, environment, and system complexity of the application will have the primary bearing on the optimal selection of the energy harvesting approach. The majority of energy harvesting approaches for miniaturized systems have targeted centimeter scale devices that enable a broad range of IoT applications and wirelessly interconnected circuits. While literature and the media often refer to micro- and nano-technology when referring to these approaches, they are typically referring to chip-scale and larger systems, and/or nanoscience that has enabled the overall larger system size. There remains a large research gap in translating energy harvesting device technologies to power IoT2 systems at the sub-mm scale.
The physical interactions governing energy conversion pose unique challenges to scaling down system dimensions. While radio-frequency provides one of the most efficient means of wireless power transfer in today’s IoT systems, powering nanosystems at the sub-mm scale will require breakthroughs in achieving high efficiency at subwavelength dimensions. Similarly, mechanical systems based on MEMS technologies and piezoelectric or triboelectric effects will require new structural design approaches in order to provide high energy conversion efficiency, and will likely push the boundaries of nanofabrication processes for mechanical structures. Systems requiring complex platforms for packaging, such as microreactors used in thermophotovoltaic and fuel cells, will require major engineering breakthroughs in order to become practical at scaled dimensions. Approaches that directly show promise for dimensional scaling are optical (photovoltaic), thermal (thermoelectric), and nuclear (betavoltaic), where the energy conversion process can be achieved through thin layers of solid state materials for which power density can scale with device area.
The modalities for energy conversion have wide variation regarding the stability of the source and the electrical signals generated, while there are also a vast array of tolerances and specifications for differing IoT2 systems. Advanced low-power circuit design has demonstrated efficiencies >80% to handle power regulation and voltage upconversion to support IoT2 devices, where a non-exhaustive list of demonstrations includes photovoltaics (Lo et al., 2018), piezoelectrics (Wu et al., 2017), and hybrid systems combining multiple modes such as solar/vibration/RF (Chowdary et al., 2016). The power budget for an IoT2 device will depend on the rate of sensing, sampling, and external communications (typically among the most power-hungry operations). Analogous to off-grid residential power, self-powering may be achieved in cases where the energy source has a temporal profile that matches use. Top-down system design can provide the most efficient energy/power use through considering primary aspects of the sensing interface, the sensing rate, and the sampling scheme (Yahya et al., 2018), including an overall energy-neutral scheme for the network (Hassan et al., 2019).
In cases where higher instantaneous power is required, energy storage is required. Energy storage and power generation can be achieved over short timescales using capacitor networks. Longer term storage can utilize chip-scale storage via supercapacitors or solid state batteries, where a review of these technologies is worthy of a stand alone review. Micro-scale supercapacitors with a volume of less than 0.1 cm3 have demonstrated a range of energy capacities and power densities (Shen et al., 2017) to support IoT2 systems, where further development in scaling to smaller sizes is needed. Microscale lithium-ion batteries (Liu et al., 2017) can provide an energy storage solution, where there are current surface mount packages currently available and substantial effort devoted to continued miniaturization.
Applications for IoT2 devices, and requirements for power budget and energy harvesting modality, can generally be divided into a few different classes: health/biological monitoring, asset monitoring, and environmental monitoring. For health/biological monitoring, sensors external to the body can use a wide range of approaches, and are typically not limited to the microscale. Bio-implantable devices have much higher constraints on accessible energy sources, dimensions, and toxicity requirements. An example application with extreme requirements are floating autonomous neural sensors, where ultrasound, infrared, and RF have all been pursued (Yang et al., 2020). Micro and nanoscale sensors have tremendous opportunity to realize “body dust” for biological monitoring that extends beyond wearable and implantable devices (Carrara, 2020). Asset monitoring and surveillance provides the ability to operate and maintain machines and infrastructure, track items, assess possible failure modes before they occur, and to ensure safety. These applications often have a predictable environment and mode that is event driven, allowing for a more straightforward path towards design of the power system. For example, the power system for an industrial machine monitoring device could harvest energy from stray vibrations or waste heat during operation with regular sensing and reporting intervals that fit within the power budget. Environmental monitoring devices generally serve to detect surroundings such as temperature, humidity, and chemical environment, which often have relatively slow variation with time. Environmental monitoring systems can be optimized to harvest the most abundant ambient source (e.g., stray light or vibrations), where the greatest power demand is typically related to wireless transmission of data. For all applications, a life-cycle assessment is needed to ensure an appropriate choice of energy harvesting mode and power management supports the intended product lifetime and reliability specifications.
As nanoscience and nanotechnology continues to evolve, new device architectures may emerge that can directly convert energy via nanostructures such as 2D material layers, nanowires, or quantum dots, providing new avenues for energy conversion that overcome conventional boundaries placed by existing microelectronics and MEMS technology. Multiscale metamaterials provide a pathway to engineer materials and structures with tailored physical properties to optimize energy harvesting (Tan et al., 2019). Nanoscience breakthroughs have the opportunity to achieve high efficiency at dimensions that approach fundamental limits such as the wavelength of the source, or in some cases enable subwavelength energy conversion phenomena. The implementation of nanoscale energy harvesting technologies in IoT2 devices will depend critically on the design of electrical interfaces and circuitry. Traditional IoT systems often place energy harvesting components and power management circuitry as modular components in the system. The progression towards continued scaling may further open opportunities for more distributed energy harvesting and power conversion, for example, singular nanowires or quantum dots directly self-powering a sensor within the IoT2 system.
The author confirms being the sole contributor of this work and has approved it for publication.
The author declares that the research was conducted in the absence of any commercial or financial relationships that could be construed as a potential conflict of interest.
Arslanagić, S., and Ziolkowski, R. W. (2018). Highly subwavelength, superdirective cylindrical nanoantenna. Phys. Rev. Lett. 120, 237401. doi:10.1103/PhysRevLett.120.237401
Byrnes, S. J., Blanchard, R., and Capasso, F. (2014). Harvesting renewable energy from Earth’s mid-infrared emissions. Proc. Natl. Acad. Sci. U.S.A. 111, 3927–3932. doi:10.1073/pnas.1402036111
Carrara, S. (2020). Body dust: well beyond wearable and implantable sensors. IEEE Sensors J., 1. doi:10.1109/JSEN.2020.3029432
Chan, W. R., Bermel, P., Pilawa-Podgurski, R. C. N., Marton, C. H., Jensen, K. F., Senkevich, J. J., et al. (2013). Toward high-energy-density, high-efficiency, and moderate-temperature chip-scale thermophotovoltaics. Proc. Natl. Acad. Sci. U.S.A. 110, 5309–5314. doi:10.1073/pnas.1301004110
Chowdary, G., Singh, A., and Chatterjee, S. (2016). An 18 nA, 87% efficient solar, vibration and RF energy-harvesting power management system with a single shared inductor. IEEE J. Solid-State Circuits 51, 2501–2513. doi:10.1109/JSSC.2016.2585304
Cortese, A. J., Smart, C. L., Wang, T., Reynolds, M. F., Norris, S. L., Ji, Y., et al. (2020). Microscopic sensors using optical wireless integrated circuits. Proc. Natl. Acad. Sci. U.S.A. 117, 9173–9179. doi:10.1073/pnas.1919677117
Do, M. H., Ngo, H. H., Guo, W., Chang, S. W., Nguyen, D. D., Liu, Y., et al. (2020). Microbial fuel cell-based biosensor for online monitoring wastewater quality: a critical review. Sci. Total Environ. 712, 135612. doi:10.1016/j.scitotenv.2019.135612
Hang, G., and Lal, A. (2003). “Nanopower betavoltaic microbatteries,” in TRANSDUCERS ’03. 12th international conference on solid-state sensors, actuators and microsystems. digest of technical papers (Cat. No.03TH8664), Boston, MA, June 8–12, 2003, Vol. 1 (Boston, MA: IEEE), 36–39. doi:10.1109/SENSOR.2003.1215247
Hassan, N., Chou, C. T., and Hassan, M. (2019). eNEUTRAL IoNT: energy-neutral event monitoring for internet of nano things. IEEE Internet Things J. 6, 2379–2389. doi:10.1109/JIOT.2019.2907046
Jang, Y. H., Jang, Y. J., Kim, S., Quan, L. N., Chung, K., and Kim, D. H. (2016). Plasmonic solar cells: from rational design to mechanism overview. Chem. Rev. 116, 14982–15034. doi:10.1021/acs.chemrev.6b00302
Kundu, A., Jang, J. H., Gil, J. H., Jung, C. R., Lee, H. R., Kim, S.-H., et al. (2007). Micro-fuel cells-current development and applications. J. Power Sources 170, 67–78. doi:10.1016/j.jpowsour.2007.03.066
Lee, I., Moon, E., Kim, Y., Phillips, J., and Blaauw, D. (2019). “A 10mm3 light-dose sensing IoT2 system with 35-to-339 nW 10-to-300klx light-dose-to-digital converter,” in 2019 symposium on VLSI technology, Kyoto, Japan, June 9–14, 2019 (Cleveland, OH: IEEE), C180–C181. doi:10.23919/VLSIT.2019.8776556
Liu, L., Weng, Q., Lu, X., Sun, X., Zhang, L., and Schmidt, O. G. (2017). Advances on microsized on-chip lithium-ion batteries. Small 13, 1701847. doi:10.1002/smll.201701847
Liu, W., Wang, Z., Wang, G., Liu, G., Chen, J., Pu, X., et al. (2019). Integrated charge excitation triboelectric nanogenerator. Nat. Commun. 10, 1426. doi:10.1038/s41467-019-09464-8
Lo, C.-L., Cheng, H.-C., Liao, P.-C., Chen, Y.-L., and Chen, P.-H. (2018). An 82.1%-power-efficiency single-inductor triple-source quad-mode energy harvesting interface with automatic source selection and reversely polarized energy recycling”, in 2018 IEEE Asian solid-state circuits conference (A-SSCC), Tainan, Taiwan, November 5–7, 2018 (Tainan, Taiwan: IEEE), 165–168. doi:10.1109/ASSCC.2018.8579313
Maayan, G. D. (2020). The IoT rundown for 2020: stats, risks, and solutions. Security Today. Available at: https://securitytoday.com/articles/2020/01/13/the-iot-rundown-for-2020.aspx#:∼:text=During%202020%E2%80%94global%20spending%20on,market%20will%20reach%20%241.1%20trillion.
Moon, E., Barrow, M., Lim, J., Blaauw, D., and Phillips, J. D. (2020). Dual-junction GaAs photovoltaics for low irradiance wireless power transfer in submillimeter-scale sensor nodes. IEEE J. Photovolt. 10, 1721–1726. doi:10.1109/JPHOTOV.2020.3025450
Moon, E., Blaauw, D., and Phillips, J. D. (2017). Infrared energy harvesting in millimeter-scale gaas photovoltaics. IEEE Trans. Electron. Devices 64, 4554–4560. doi:10.1109/ted.2017.2746094
Moon, E., Lee, I., Blaauw, D., and Phillips, J. D. (2019). High‐efficiency photovoltaic modules on a chip for millimeter‐scale energy harvesting. Prog. Photovoltaics Res. Appl. 27, 540–546. doi:10.1002/pip.3132
Moorthy, B., Baek, C., Wang, J. E., Jeong, C. K., Moon, S., and Park, K.-I. (2017). Piezoelectric energy harvesting from a PMN-PT single nanowire. RSC Adv. 7, 260–265. doi:10.1039/C6RA24688E
Oh, S., Cho, M., Wu, X., Kim, Y., Chuo, L., Lim, W., et al. (2019). IoT2—the internet of tiny things: realizing mm-scale sensors through 3d die stacking,” in 2019 Design, automation test in Europe conference exhibition, Florence, Italy, March 25–29, 2019, (IEEE) 686–691.
Rabaey, J. M., Mark, M., Chen, D., Sutardja, C., Chongxuan Tang, C., Gowda, S., et al. (2011). Powering and communicating with mm-size implants,” in 2011 design, automation and test in Europe, Grenoble, France, March 14–18, 2019 (Grenoble, France: IEEE), 1–6. doi:10.1109/DATE.2011.5763123
Seo, D., Neely, R. M., Shen, K., Singhal, U., Alon, E., Rabaey, J. M., et al. (2016). Wireless recording in the peripheral nervous system with ultrasonic neural dust. Neuron 91, 529–539. doi:10.1016/j.neuron.2016.06.034
Shen, C., Xu, S., Xie, Y., Sanghadasa, M., Wang, X., and Lin, L. (2017). A review of on-chip micro supercapacitors for integrated self-powering systems. J. Microelectromech. Syst. 26, 949–965. doi:10.1109/JMEMS.2017.2723018
Strandberg, R. (2015). Theoretical efficiency limits for thermoradiative energy conversion. J. Appl. Phys. 117, 055105. doi:10.1063/1.4907392
Tan, T., Yan, Z., Zou, H., Ma, K., Liu, F., Zhao, L., et al. (2019). Renewable energy harvesting and absorbing via multi-scale metamaterial systems for internet of things. Appl. Energy 254, 113717. doi:10.1016/j.apenergy.2019.113717
Vandenbosch, G. A. E., and Ma, Z. (2012). Upper bounds for the solar energy harvesting efficiency of nano-antennas. Nano Energy 1, 494–502. doi:10.1016/j.nanoen.2012.03.002
Venkatasubramanian, R., Watkins, C., Stokes, D., Posthill, J., and Caylor, C. (2007). Energy harvesting for electronics with thermoelectric devices using nanoscale materials,” in 2007 IEEE international electron devices meeting, Washington, DC, December 10–12, 2007 (Washington, DC USA: IEEE), 367–370. doi:10.1109/IEDM.2007.4418948
Wagner, D. L., Novog, D. R., and LaPierre, R. R. (2020). Design and optimization of nanowire betavoltaic generators. J. Appl. Phys. 127, 244303. doi:10.1063/1.5138119
Wang, Z. L. (2020). On the first principle theory of nanogenerators from Maxwell’s equations. Nano Energy 68, 104272. doi:10.1016/j.nanoen.2019.104272
Wang, Z. L. (2013). Triboelectric nanogenerators as new energy technology for self-powered systems and as active mechanical and chemical sensors. ACS Nano 7, 9533–9557. doi:10.1021/nn404614z
Warneke, B., Last, M., Liebowitz, B., and Pister, K. S. J. (2001). Smart dust: communicating with a cubic-millimeter computer. Computer 34, 44–51. doi:10.1109/2.895117
Wu, L., Do, X.-D., Lee, S.-G., and Ha, D. S. (2017). A self-powered and optimal sshi circuit integrated with an active rectifier for piezoelectric energy harvesting. IEEE Trans. Circuits Syst. I Regul. Pap. 64, 537–549. doi:10.1109/TCSI.2016.2608999
Xu, S., Hansen, B. J., and Wang, Z. L. (2010). Piezoelectric-nanowire-enabled power source for driving wireless microelectronics. Nat. Commun. 1, 93. doi:10.1038/ncomms1098
Yahya, F., Lukas, C., and Calhoun, B. (2018). A top-down approach to building battery-less self-powered systems for the internet-of-things. Int. J. Eng. Res. Appl. 8, 21. doi:10.3390/jlpea8020021
Yamashita, T., Hayashi, T., Iwasaki, H., Awatsu, M., and Yokoyama, H. (2019). Ultra-low-power energy harvester for microbial fuel cells and its application to environmental sensing and long-range wireless data transmission. J. Power Sources 430, 1–11. doi:10.1016/j.jpowsour.2019.04.120
Yang, K.-W., Oh, K., and Ha, S. (2020). Challenges in scaling down of free-floating implantable neural interfaces to millimeter scale. IEEE Access 8, 133295–133320. doi:10.1109/ACCESS.2020.3007517
Keywords: energy conversion devices, Internet of things, radio-frequency, photovoltaic, piezoelectric, thermoelectric, betavoltaic, fuel cells
Citation: Phillips JD (2021) Energy Harvesting in Nanosystems: Powering the Next Generation of the Internet of Things. Front. Nanotechnol. 3:633931. doi: 10.3389/fnano.2021.633931
Received: 27 November 2020; Accepted: 25 January 2021;
Published: 12 March 2021.
Edited by:
Candido Pirri, Polytechnic University of Turin, ItalyReviewed by:
Kenjiro Fukuda, RIKEN, JapanCopyright © 2021 Phillips. This is an open-access article distributed under the terms of the Creative Commons Attribution License (CC BY). The use, distribution or reproduction in other forums is permitted, provided the original author(s) and the copyright owner(s) are credited and that the original publication in this journal is cited, in accordance with accepted academic practice. No use, distribution or reproduction is permitted which does not comply with these terms.
*Correspondence: Jamie D. Phillips, anBoaWxsaUB1ZGVsLmVkdQ==
Disclaimer: All claims expressed in this article are solely those of the authors and do not necessarily represent those of their affiliated organizations, or those of the publisher, the editors and the reviewers. Any product that may be evaluated in this article or claim that may be made by its manufacturer is not guaranteed or endorsed by the publisher.
Research integrity at Frontiers
Learn more about the work of our research integrity team to safeguard the quality of each article we publish.